- School of Agriculture, Meiji University, Tokyo, Japan
Cyanobacteria possess an atypical tricarboxylic acid (TCA) cycle with various bypasses. Previous studies have suggested that a cyclic flow through the TCA cycle is not essential for cyanobacteria under normal growth conditions. The cyanobacterial TCA cycle is, thus, different from that in other bacteria, and the biochemical properties of enzymes in this TCA cycle are less understood. In this study, we reveal the biochemical characteristics of malate dehydrogenase (MDH) from Synechocystis sp. PCC 6803 MDH (SyMDH). The optimal temperature of SyMDH activity was 45–50°C and SyMDH was more thermostable than MDHs from other mesophilic microorganisms. The optimal pH of SyMDH varied with the direction of the reaction: pH 8.0 for the oxidative reaction and pH 6.5 for the reductive reaction. The reductive reaction catalysed by SyMDH was activated by magnesium ions and fumarate, indicating that SyMDH is regulated by a positive feedback mechanism. The Km-value of SyMDH for malate was approximately 210-fold higher than that for oxaloacetate and the Km-value for NAD+ was approximately 19-fold higher than that for NADH. The catalytic efficiency of SyMDH for the reductive reaction, deduced from kcat-values, was also higher than that for the oxidative reaction. These results indicate that SyMDH is more efficient in the reductive reaction in the TCA cycle, and it plays key roles in determining the direction of the TCA cycle in this cyanobacterium.
Introduction
Cyanobacteria performing oxygenic photosynthesis synthesise various compounds from carbon dioxide using light energy. Cyanobacteria are widely used as hosts in metabolic engineering to produce renewable resources. Synechocystis sp. PCC 6803 (hereafter Synechocystis 6803) is one of the most highly studied cyanobacteria because it has many advantageous features, such as rapid proliferation and ease of transformation. Besides genetics, biochemical analyses of enzymes related to oxaloacetate metabolism proceed using Synechocystis 6803 enzymes (Ito et al., 2017; Takeya et al., 2017), and thus this cyanobacterium is widely used for basic studies of primary carbon metabolism.
The tricarboxylic acid (TCA) cycle is one of the most important biochemical reactions in aerobic energy production, and is common among most respiring organisms. Reductants are generated by oxidation of metabolites through the TCA cycle, leading to ATP production through the process of respiration, which uses these reductants. Metabolites in the TCA cycle, such as oxaloacetate and 2-oxoglutarate, are precursors of various metabolites, including amino acids, sugars, and lipids (Owen et al., 2002). The cyanobacterial TCA cycle is also involved in various metabolic systems, which can lead to the production of useful materials, such as succinate (Osanai et al., 2015), amino acids (Matsunaga et al., 1991), ethylene (Xiong et al., 2015) via acetyl-CoA, and TCA cycle derivatives from fixing carbon dioxide by oxygenic photosynthesis using light energy.
Compared to studies on enzymes in the Calvin cycle, biochemical analysis of enzymes of the TCA cycle in cyanobacteria is limited. The cyanobacterial TCA cycle was once thought to be an incomplete cycle owing to the lack of 2-oxoglutarate dehydrogenase (OGDH); however, it has been demonstrated that 2-oxoglutarate decarboxylase and succinate semialdehyde dehydrogenase produce succinate from 2-oxoglutarate (Zhang and Bryant, 2011; Steinhauser et al., 2012). In addition, the γ-aminobutyric acid (GABA) shunt produces succinate from glutamate in Synechocystis 6803 (Xiong et al., 2014), and the glyoxylic acid shunt is found in the cyanobacterium Chlorogloeopsis fritschii strain PCC 9212 (Zhang and Bryant, 2015). Thus, the cyanobacterial TCA cycles are potentially closed with these alternative shunts. However, these studies only analysed the first half of the TCA cycle, from citrate to succinate. The latter half of the TCA cycle has been studied by in silico analysis (Knoop et al., 2013; Rubin et al., 2015). Kinetic values, such as kcat and Km, of cyanobacterial TCA cycle enzymes have not been determined, except for isocitrate dehydrogenase (Muro-Pastor and Florencio, 1992, 1994). Biochemical analysis of phosphoenolpyruvate carboxylase (PEPC), which produces oxaloacetate from phosphoenolpyruvate, reveals that Synechocystis 6803 PEPC is uniquely tolerant to feedback inhibition by malate and aspartate (Takeya et al., 2017). In addition to the oxidative cycle, the cyanobacterial TCA cycle reverses to a reductive reaction (called the reductive branch of the TCA cycle) under dark, anaerobic conditions (Hasunuma et al., 2016).
Malate dehydrogenase (MDH) is an enzyme that catalyses the interconversion between malate and oxaloacetate using NAD(P)H. MDHs are largely conserved in most species, irrespective of variation in the TCA cycle (Huynen et al., 1999; Minárik et al., 2002). MDH catalyses the oxidative reaction in the TCA cycle (malate to oxaloacetate) in vivo, although MDH thermodynamically prefers the reductive reaction (oxaloacetate to malate) in vitro (Molenaar et al., 1998). Thus, MDH is a unique enzyme that prefers the reductive reaction in the TCA cycle; however, the biochemical parameters of Synechocystis 6803 MDH (SyMDH) have not been determined. MDH functions to protect against oxidative stress in Escherichia coli (Wu et al., 2007; Singh et al., 2008), also suggesting the physiological importance of MDHs in bacteria. In this study, SyMDH was purified, and its biochemical functions were demonstrated for the first time, revealing unique regulatory mechanisms of SyMDH.
Materials and Methods
Construction of Cloning Vectors for Recombinant Protein Expression
A BamHI-XhoI DNA fragment of the citH (sll0891) ORF from the Synechocystis 6803 genome was amplified by PCR using KOD Plus Neo polymerase (Toyobo, Osaka, Japan) with the primers: forward, GAAGGTCGTGGGATCATGAATATTTTGGAGTATGCTC and reverse, GATGCGGCCGCTCGAGTTAACCGTCGCTAACCAT. The resultant fragments were excised with BamHI-XhoI (Takara Bio, Shiga Japan) and cloned into the BamHI-XhoI site of pGEX5X-1 (GE Healthcare Japan, Tokyo, Japan) using the In-Fusion HD Cloning Kit (Takara Bio, Shiga, Japan). Sequence integrity was confirmed by sequencing.
Affinity Purification of Recombinant Proteins
Expression vectors were transformed into E. coli BL21 (DH5α, Takara Bio). Two litres of E. coli containing the vectors were cultivated at 30°C with shaking (150 rpm), and protein expression was induced overnight by adding 0.01 mM isopropyl β-D-1-thiogalactopyranoside (Wako Chemicals, Osaka, Japan).
Affinity chromatography was performed for protein purification as described in a previous study (Osanai et al., 2009). Two litres of E. coli cell culture were disrupted by sonication VC-750 (EYELA, Tokyo, Japan) for 5 min with 30% intensity and centrifuged at 5,800 ×g for 2 min at 4°C. The supernatant was transferred to a new 50-mL plastic tube on ice and 640 μL of glutathione-Sepharose 4B resin (GE Healthcare Japan) was mixed into the supernatant. After gentle rotating for 30 min, 1 mM ATP and 1 mM MgSO4⋅7H2O were added and samples were incubated with gentle shaking for 30 min to remove intracellular chaperons. After centrifugation (5,800 ×g for 2 min at 4°C), the supernatant was removed and resins were re-suspended in 700 μL of PBS-T (1.37 M NaCl, 27 mM KCl, 81 mM Na2HPO4⋅12H2O, 14.7 mM KH2PO4, 0.05% Tween-20) with 1 mM ATP/1 mM MgSO4⋅7H2O. The resin was washed with 500 μL of PBS-T (1.37 M NaCl, 27 mM KCl, 81 mM Na2HPO4⋅12H2O, 14.7 mM KH2PO4, 0.05% Tween-20) and eluted three times with 500 μL of GST elution buffer (50 mM Tris–HCl, pH 8.0, 10 mM reduced glutathione). Proteins were concentrated with VivaSpin 500 MWCO 50,000 spin columns (Sartorius, Göttingen, Germany) and protein concentration was measured with a PIERCE BCA Protein Assay Kit (Thermo Fisher Scientific, Rockford, IL, United States). Protein purification was validated by SDS-PAGE, including staining using InstantBlue (Expedion Protein Solutions, San Diego, CA, United States).
Enzyme Assays
7.8 μg or 10 μg of SyMDHs were used to measure oxidative or reductive reactions, respectively. The purified protein was mixed with 1 mL of assay solution (100 mM potassium phosphate buffer [pH 8.0 or pH 6.5], 0.1–32 mM nicotinamide adenine dinucleotide (NAD+), 0.01–0.64 mM nicotinamide adenine dinucleotide hydride (NADH), 0.2–32 mM malate, 0.02–0.4 mM oxaloacetate). The optimal temperature and the optimal pH were measured at the concentration exhibiting maximum activity (NAD+: 8.0 mM, NADH: 0.1 mM, malate: 4.0 mM, oxaloacetate: 0.1 mM). For the cell extract assay, cells from 1 L culture were collected by centrifugation and resuspend in 100 mM potassium phosphate buffer (pH7.0). The cells were disrupted by sonication and centrifuged at 5,800 × g for 30 min at 4°C. The protein concentration was quantified with BCA Protein Assay Kit (Thermo) and 420 μg of total proteins was added to 1 mL assay solution. Absorbance was measured at 340 nm using a UV-1850 spectrophotometer (Shimadzu, Tokyo, Japan). Vmax and Km-values were determined using a Lineweaver–Burk double reciprocal plot. Results were plotted as a graph of the rate of reaction against the concentration of substrate and coenzyme using Kaleida Graph ver. 4.5 software. When the data did not show substrate inhibition, we performed curve fitting used the Michaelis–Menten equation (Eq. 1). When the data exhibited substrate inhibition, we performed curve fitting using the modified Michaelis–Menten equation (Eq. 2) (Eszes et al., 1996).
v and Vmax indicate reaction velocity and maximum reaction velocity, respectively. [S], Km, and Ki indicate substrate concentration, the half-maximum concentration giving rise to 50% Vmax and an inhibition constant, respectively.
Results
Measurement of Kinetic Parameters
To determine the kinetic parameters of SyMDH, glutathione S-transferase (GST)-tagged SyMDH (GST-SyMDH) proteins were expressed in E. coli and purified by affinity chromatography (Figure 1A). SyMDH activity in the oxidative reaction (malate to oxaloacetate) was the highest at pH 8.0 and at a temperature of 50°C (Figures 1B,C). SyMDH activity in the reductive reaction (oxaloacetate to malate) was the highest at pH 6.5 and at 45°C (Figures 1B,C). Kinetic parameters of SyMDH were determined by a Lineweaver–Burk double reciprocal plot using the specific activity values in Figures 2, 3. These results are summarised in Tables 1, 2. SyMDH displayed approximately 1.7-fold (kcat) and 361-fold (kcat/Km) preferences for oxaloacetate reduction over malate oxidation and approximately 4.7-fold (kcat) and 90.5-fold (kcat/Km) preferences for NADH oxidation over NAD+ reduction (Table 1). The catalytic efficiency of the reductive reaction was higher than that of the oxidation reaction for both the substrate and the coenzyme. The Km-value for malate was approximately 210-fold higher than that for oxaloacetate, and the Km-value for NAD+ was approximately 19-fold higher than that for NADH (Table 2). SyMDH appeared to prefer oxaloacetate and NADH as substrate and coenzyme, respectively, in vitro. SyMDH had enzymatic activity toward NAD+ and NADH, but no activity toward NADP+ and NADPH both in vitro and in vivo (Supplementary Figures S1, S2). We also determined kinetic parameters of SyMDH using the Michaelis–Menten equation. These results are summarised in Supplementary Tables S1, S2. These calculations showed that SyMDH prefers oxaloacetate and NADH as substrate and coenzyme, respectively; the Km-value for malate was approximately 84.4-fold higher than that for oxaloacetate, and the Km-value for NAD+ was approximately 71.4-fold higher than that for NADH (Supplementary Table S2). SyMDH exhibited substrate inhibition by NAD+ (Figure 2B), and the value of Ki was 14.5 mM (Supplementary Table S1).
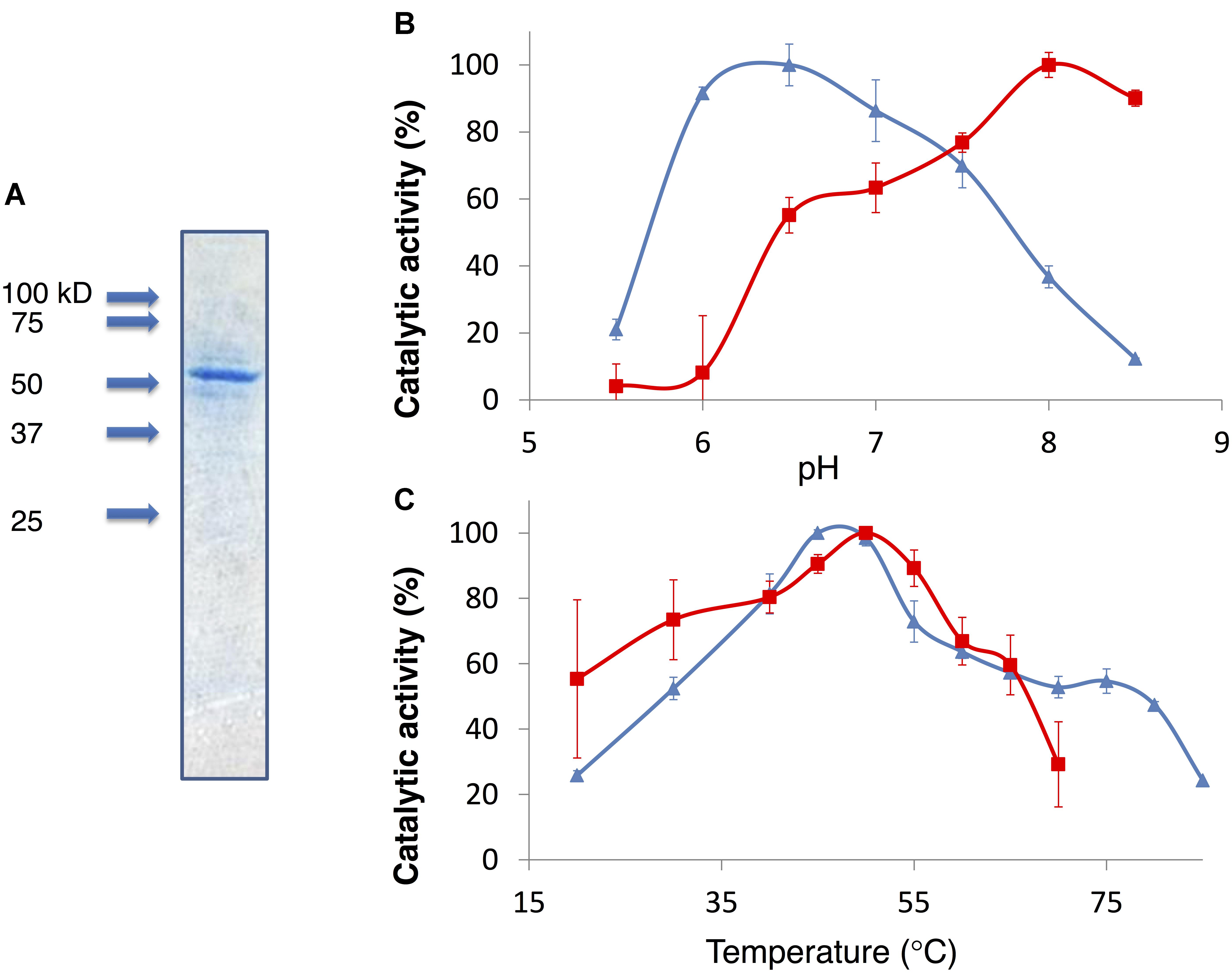
FIGURE 1. (A) Purification of GST-tagged SyMDH. Proteins were electrophoresed on a 12% SDS-PAGE gel. The gel was stained with InstantBlue. Arrowheads indicate the molecular weight. (B) The effect of pH on SyMDH activity. Red square represents the specific activity in the oxidative reaction (malate to oxaloacetate). Blue triangle represents the specific activity in the reductive reaction (oxaloacetate to malate). Data represent the relative values of the mean from three independent experiments. (C) The effect of temperature on SyMDH activity. Red square represents the specific activity in the oxidative reaction (malate to oxaloacetate). Blue triangle represents the specific activity in the reductive reaction (oxaloacetate to malate). Data represent the relative values of the mean from three independent experiments.
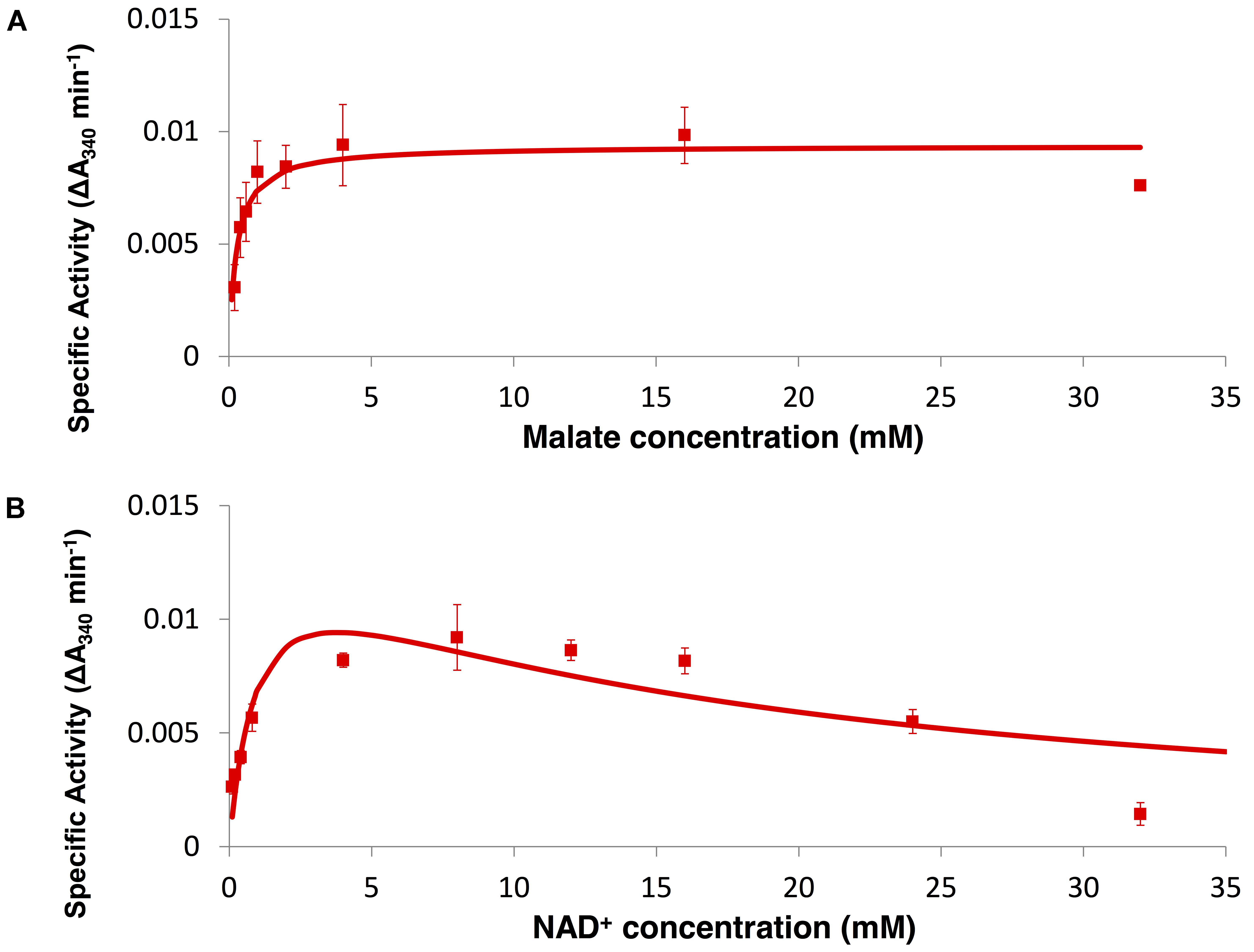
FIGURE 2. Enzyme assay of SyMDH in the oxidative reaction in vitro. (A) Activity was measured by varying the malate concentration at a fixed NAD+ concentration (8.0 mM). The graphs show the mean ± SD obtained from three independent experiments. (B) Activity was measured by varying the NAD+ concentration at a fixed malate concentration (4.0 mM). The graphs show the mean ± SD obtained from three independent experiments.
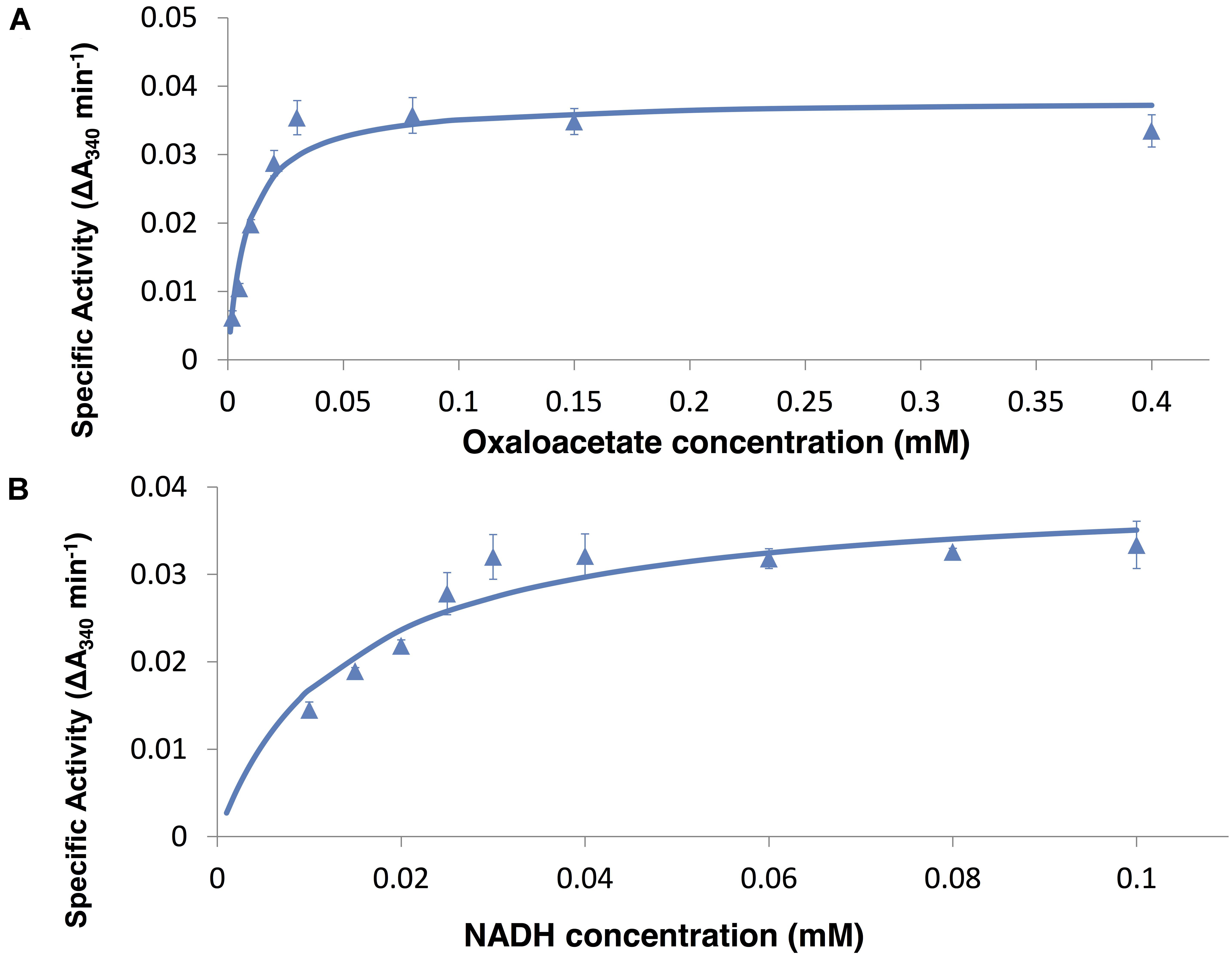
FIGURE 3. Enzyme assay of SyMDH in the reductive reaction in vitro. (A) Activity was measured by varying the oxaloacetate concentration at a fixed NADH concentration (0.1 mM). The graphs show the mean ± SD obtained from three independent experiments. (B) Activity was measured by varying the NADH concentration at a fixed oxaloacetate concentration (0.1 mM). The graphs show the mean ± SD obtained from three independent experiments.
Effect of Various Effectors on SyMDH Activity
The reductive reaction catalysed by bacterial MDHs is inhibited by TCA cycle metabolites, such as excess oxaloacetate and divalent metal ions (Takahashi-Íñiguez et al., 2016). Therefore, we measured the activity of SyMDH in the reductive reaction in the presence of various effectors. SyMDH was inhibited by excess NAD+ in the reductive reaction (Figure 2B). With the exception of cobalt, magnesium, and copper ions, all other metal ions showed little effect on SyMDH (Figure 4). SyMDH activity increased approximately 140 and 160% with the addition of 1 mM Co(NO3)2⋅6H2O and 1 mM MgCl2, respectively (Figure 4). In the presence of 10 mM MgCl2, the activity of SyMDH increased to approximately 190% (Figure 4). Among the metal ions tested, only copper ions reduced the activity of SyMDH. In the presence of 1 mM CuSO4⋅5H2O, SyMDH activity decreased to approximately 40% of normal activity (Figure 4). SyMDH activity could not be measured in the presence of 10 mM calcium, manganese, cobalt, zinc, or copper ions due to the formation of a precipitate (Figure 4). SyMDH activity rose approximately 170 and 190% with the addition of 1 and 10 mM fumarate, respectively (Figure 4). SyMDH activity with oxaloacetate at a concentration of 0.01–0.6 mM was measured in the presence of 10 mM magnesium and fumarate, and the kinetic parameters were calculated by Lineweaver–Burk plots (Figure 5A). Both the Km and Vmax-values of this substrate and reaction, respectively, increased with the addition of 10 mM MgCl2 and fumarate (Figures 5B,C). To strengthen the validity of our results, we also performed biochemical assays using cell extracts (Supplementary Figure S3a). Unlike in vitro, the Km-value did not change in vivo in the presence of 10 mM MgCl2 and fumarate (Supplementary Figure S3b). The Vmax-value increased in vivo similar to in vitro in the presence of 10 mM MgCl2 and fumarate (Supplementary Figure S3c).
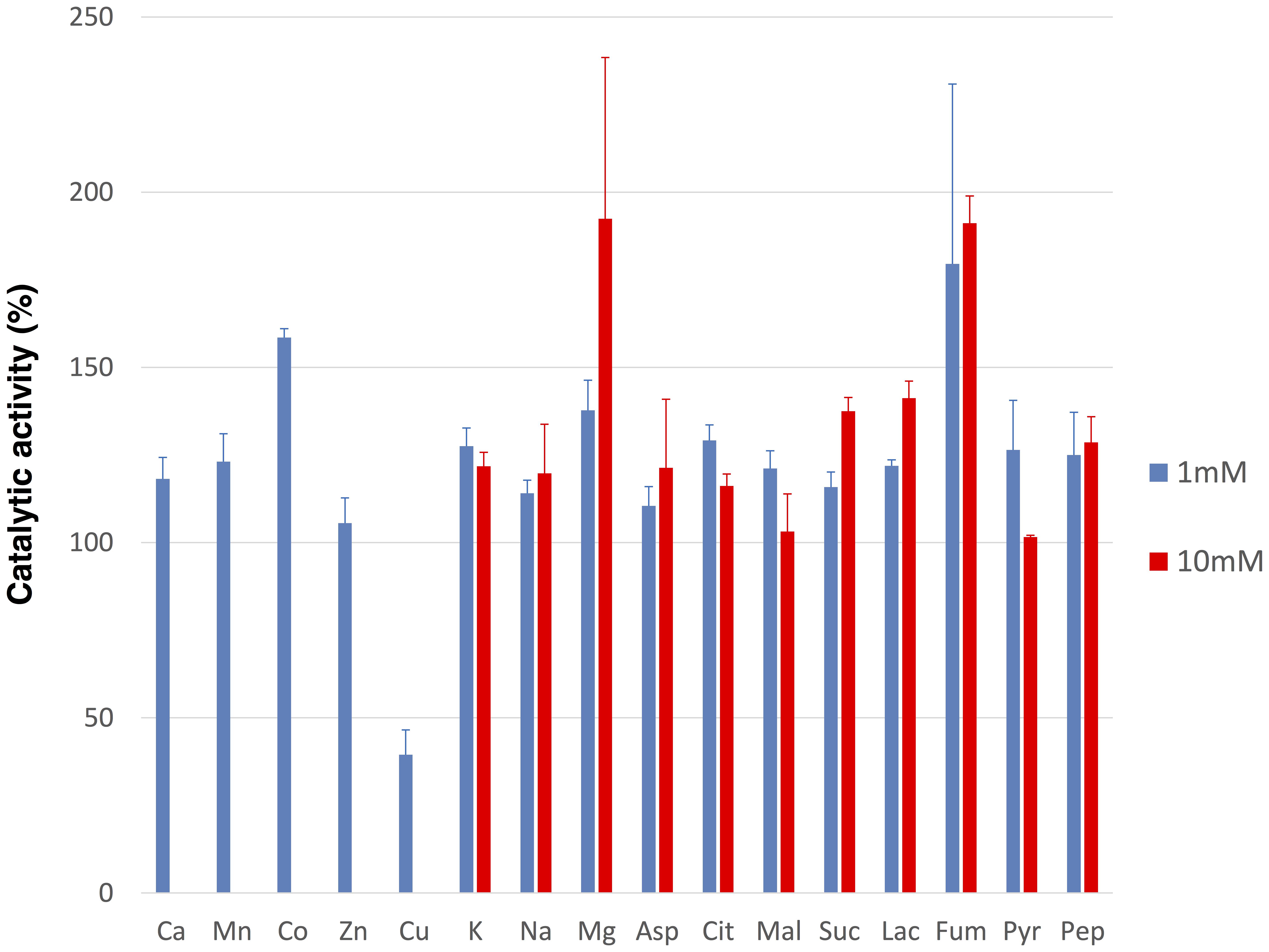
FIGURE 4. Effects of various metal ions and compounds on the SyMDH in the reductive reaction in vitro. 10 μg of SyMDH was pre-incubated with 100 mM potassium phosphate (pH 6.5), 0.1 mM NADH, 0.1 mM oxaloacetate and effectors, at 45°C. The graphs show the mean ± SD obtained from three independent experiments. Activity of SyMDH in the absence of effectors was set at 100%. Ca, CaCl2; Mn, MnCl2⋅4H2O; Co, Co(NO3)2⋅6H2O; Zn, ZnSO4⋅7H2O; Cu, CuSO4⋅5H2O; K, KCl; Na, NaCl; Mg, MgCl2; Asp, L-Aspartate; Cit, Citrate; Mal, L-Malate; Suc, Succinate; Lac, L-lactate; Fum, Fumarate; Pyr, Pyruvate; Pep, Phosphoenolpyruvate.
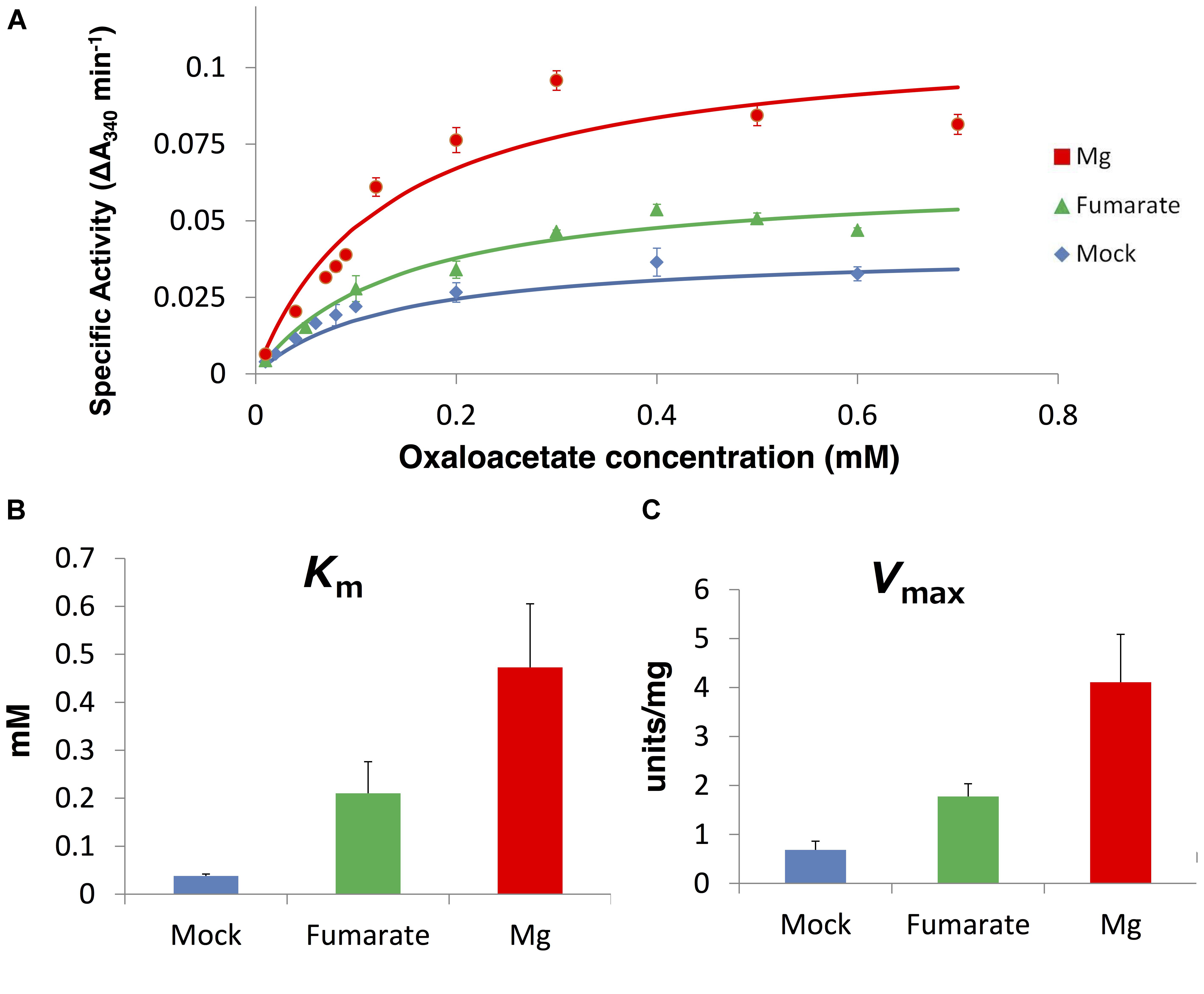
FIGURE 5. The Km and Vmax-values for oxaloacetate in the presence of 10 mM fumarate and 10 mM magnesium ion in vitro. (A) Saturation curves of the activity of SyMDH. Blue line indicates mock, green line indicates presence of fumarate, and red line indicates the presence of magnesium. The graph shows the mean of three independent experiments. (B) Km (mean ± SD) (units/mg protein) values in the presence of 10 mM fumarate and 10 mM magnesium ion, obtained from three independent experiments. (C) Vmax (mean ± SD) values for oxaloacetate, obtained from three independent experiments. Mock indicates the enzymatic activity in the absence of additional compounds.
Thermal Properties of SyMDH Activity
Synechocystis 6803 MDH activity was measured by varying temperature (20–50°C). The Km and the Vmax were calculated by both a Lineweaver–Burk double reciprocal plot (Figures 5, 6) and curve fitting used the Michaelis–Menten equation (Supplementary Figures S4, S5). The Km and the Vmax-values for malate tend to decrease as the temperature rise, although the Vmax-values less dependent on the temperature (Figure 6 and Supplementary Figure S4). On the contrary, the Km and the Vmax-values for oxaloacetate increased as the temperature rise (Figure 7 and Supplementary Figure S5). The Km and the Vmax for malate at 20°C were approximately 2.7-fold and 1.9-fold higher than that at 50°C, respectively (Figure 6). The Km and Vmax for oxaloacetate at 20°C were approximately 0.19- and 0.13-fold higher than that at 50°C, respectively (Figure 7). The Km and Vmax of SyMDH demonstrated its temperature dependency.
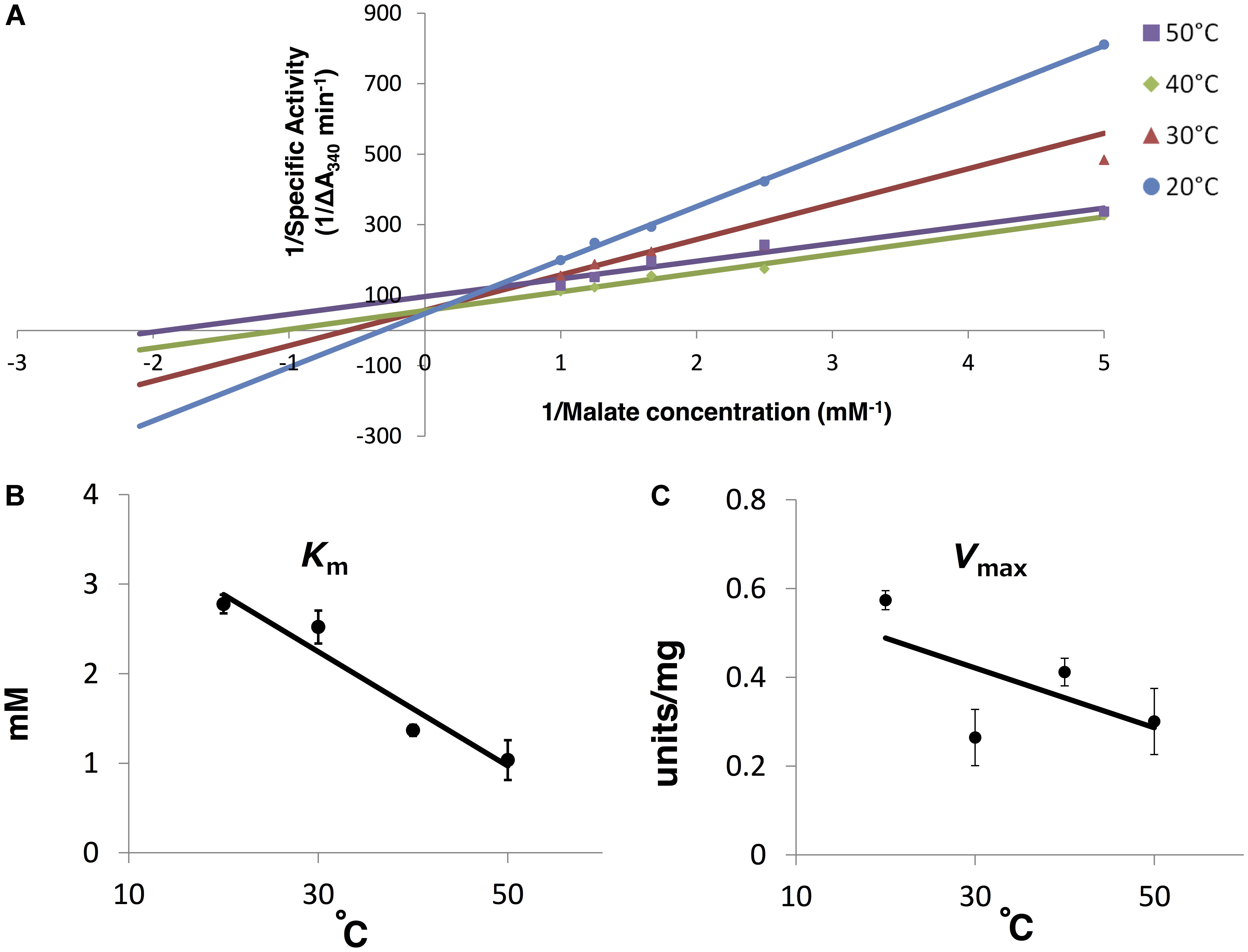
FIGURE 6. Thermal profiles of SyMDH in the oxidative reaction in vitro. (A) Lineweaver–Burk plot of the SyMDH activity in the oxidative reaction at 20–50°C. Blue, red, green, and purple lines indicate condition at 20, 30, 40, and 50°C, respectively. The graph shows the mean of three independent experiments. (B) Km (mean ± SD) values for malate were obtained from three independent experiments by varying the temperature (20–50°C). (C) Vmax (mean ± SD) (units/mg protein) values for malate were obtained from three independent experiments by varying the temperature (20–50°C).
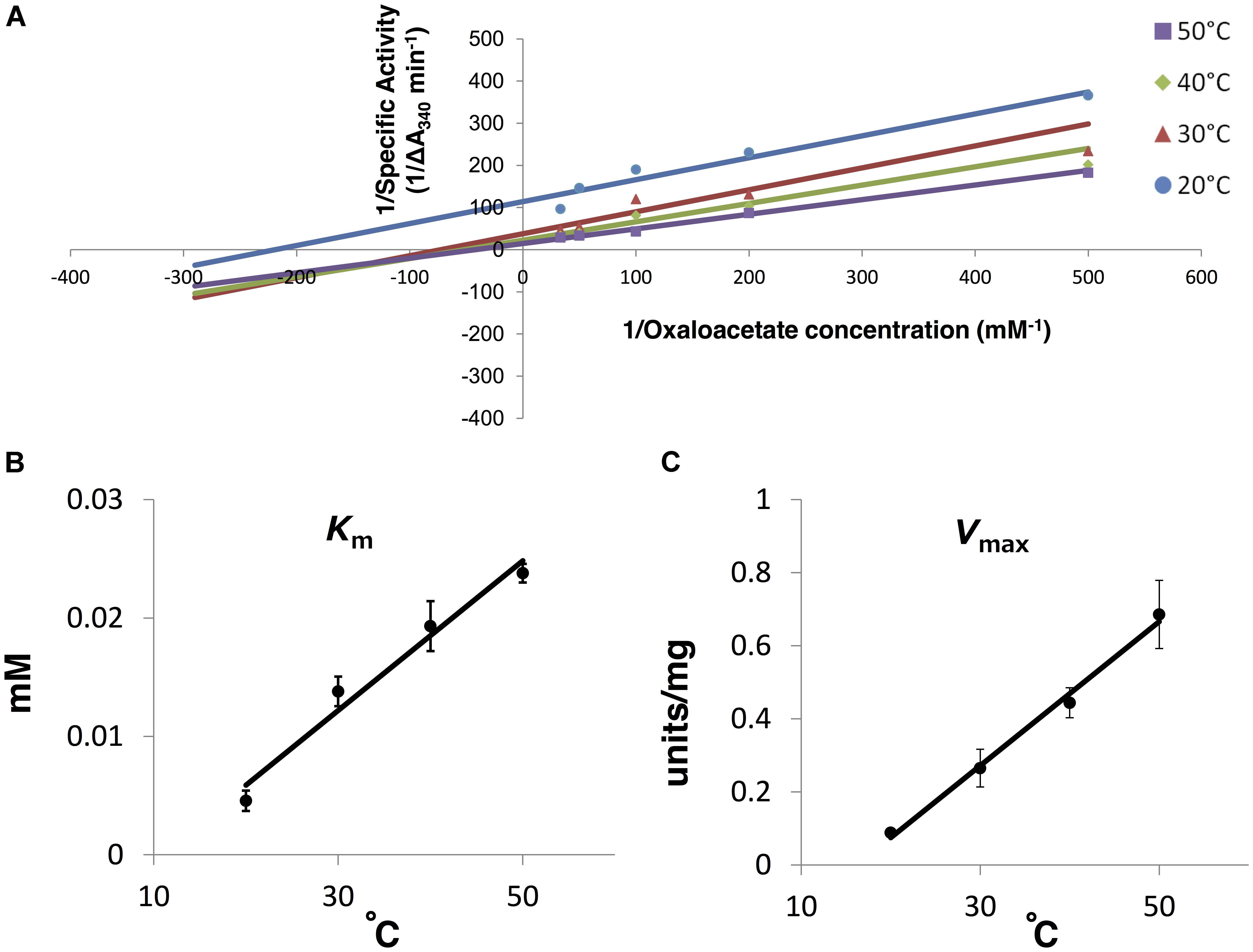
FIGURE 7. Thermal profiles of SyMDH in the reductive reaction in vitro. (A) Lineweaver–Burk plot of the SyMDH activity in the reductive reaction at 20–50°C. Blue, red, green, and purple lines indicate condition at 20, 30, 40, and 50°C, respectively. The graph shows the mean of three independent experiments. (B) Km (mean ± SD) values for oxaloacetate were obtained from three independent experiments by varying the temperature (20–50°C). (C) Vmax (mean ± SD) (units/mg protein) values for oxaloacetate were obtained from three independent experiments by varying the temperature (20–50°C).
Discussion
We purified recombinant SyMDH protein and revealed the biochemical properties of cyanobacterial MDH for the first time. The optimal pH of SyMDH was different for the oxidative reaction and the reductive reaction (Figure 1B). Cyanobacteria utilise a reductive branch of TCA cycle and excrete succinate under dark anaerobic conditions (Hasunuma et al., 2016). The intracellular pH of cyanobacteria decreases during the transition from light to dark conditions (Coleman and Coleman, 1981; Mangan et al., 2016). Therefore, to adapt to the drastic changes in primary metabolism during the light and dark cycle, SyMDH is thought to shift its substrate affinity according to the intracellular pH. SyMDH was stable at a wide range of temperature, being particularly tolerant to high temperatures (Figure 1C). Among the mesophilic microorganisms, MDHs from Streptomyces avermitilis, Streptomyces coelicolor, and Nitrosomonas europaea maintain their activity at 50°C (Mikulášová et al., 1998; Ge et al., 2010; Deutch, 2013), but these MDHs are completely inactivated at 60–70°C (Mikulášová et al., 1998; Ge et al., 2010; Deutch, 2013). SyMDH maintains its activity in both oxidative and reductive reactions at 60–70°C (Figure 1C). Therefore, SyMDH is the most thermostable enzyme among MDHs from the mesophilic microorganisms investigated thus far. The optimal temperatures of SyMDH were 50 and 45°C, for the oxidative and reductive reaction, respectively (Figure 1C). Thus, optimal temperature of SyMDH (45–50°C) and optimal growth temperature of Synechocystis 6803 (30–35°C) were different. Generally, an enzymatic reaction is promoted by increasing temperature, because the kinetic energy of the reactants increases. However, an enzyme denature at high temperatures. Since SyMDH is a heat-stable enzyme (Figure 1B), the enzyme activity became the highest at around 50°C, which is higher than the optimal growth temperature in Synechocystis 6803. Besides Synechocystis 6803, microorganisms having the MDHs with the optimal temperature much higher than the optimal growth temperature are S. avermitilis and S. coelicolor, N. europaea (Mikulášová et al., 1998; Ge et al., 2010; Deutch, 2013). SyMDH activity was suppressed by copper (Figure 4), as was observed for the MDH from Pseudomonas stutzeri (Labrou and Clonis, 1997). P. stutzeri MDH is also inhibited by citrate (Labrou and Clonis, 1997), but SyMDH was slightly activated by citrate (Figure 4). The only reported activators of bacterial MDHs are >0.18 mM malate and 3 M NaCl (Cendrin et al., 1997; Labrou and Clonis, 1997), but SyMDH was significantly activated by magnesium ions and fumarate (Figure 5 and Supplementary Figure S3), suggesting that SyMDH is regulated by a positive feedback mechanism. These results are indicative of the diversity of regulation among MDHs. Intracellular concentrations of malate and fumarate in E. coli cells are 1.7 and 0.11 mM, respectively (Bennett et al., 2009). Since SyMDH showed maximum activity at 5 mM malate (Figure 2) and was activated with 1 mM fumarate (Figure 4), it is plausible that SyMDH activity was regulated by the TCA cycle metabolites. Excess NAD+ (>4 mM) caused substrate inhibition in SyMDH (Figure 2B). MDHs from Methanobacterium thermoautotrophicum and P. stutzeri are also inhibited by excess NAD+ (>0.5 mM) and NAD+ (>250 mM), respectively (Labrou and Clonis, 1997; Thompson et al., 1997). Intracellular concentrations of NAD+ in E. coli cells is 2.6 mM (Bennett et al., 2009), thus, SyMDH activity is thought to inhibited by NAD+ present in Synechocystis 6803.
The affinity of oxaloacetate and NADH for SyMDH was higher than the affinity of malate and NAD+, respectively (Table 1). Generally, bacterial MDHs show higher affinity for oxaloacetate than malate (Takahashi-Íñiguez et al., 2016), and SyMDH was consistent with this. When comparing the substrate affinity among bacterial MDHs, the Km (malate)/Km (oxaloacetate) ratio in descending order is as follows: N. europaea (250), Synechocystis 6803 (210), Syntrophic propionate-oxidising bacterium strain MPOB (80.0), and Methanobacterium thermoautotrophicum (13.3) (Table 2). The previous study demonstrated that the NAD+ concentration is approximately 500 times higher than NADH concentration in Synechocystis 6803 (Osanai et al., 2014). Therefore, although our biochemical analysis showed that SyMDH has higher coenzyme specificity toward NADH than NAD+, SyMDH can catalyse both reductive and oxidative reactions in vivo. The Km (NAD+)/Km (NADH) ratio in descending order is as follows: Syntrophic propionate-oxidising bacterium strain MPOB (36.7), Methanothermus fervidus (28.0), and Synechocystis 6803 (19.0). These aforementioned microorganisms are thought to have low MDH activity in the oxidation reaction. This is because N. europaea is deficient in 2-oxoglutarate dehydrogenase (Beyer et al., 2009) and succinyl-CoA is formed via phosphoenolpyruvate and oxaloacetate using a reductive branch of TCA cycle (Deutch, 2013). In addition, syntrophic propionate-oxidising bacterium strain MPOB, Methanobacterium thermoautotrophicum, and Methanothermus fervidus are anaerobic microorganisms (Harmsen et al., 1996; Thompson et al., 1997; Stetter et al., 1981), and therefore, their oxidative TCA cycles are barely functioning. As with microorganisms in which the oxidative TCA cycle does not appear to function, the Km (for malate)/Km (for oxaloacetate) ratio and the Km (NAD+)/Km (NADH) ratio of SyMDH were very high. Therefore, SyMDH is likely to have low activity in the oxidative reaction. This conclusion is supported by flux analyses. Previous studies measured metabolic flow by estimating the flux rates of metabolites per dry cell weight (DCW) per unit hour in Synechocystis 6803 under mixotrophic conditions and found that all fluxes in TCA cycle reactions were clockwise (0.02–0.11 mmol gDCW-1 h-1), except for the interconversion between malate and oxaloacetate, which was anticlockwise (0.13 mmol gDCW-1 h-1; Nakajima et al., 2014). Similar results were observed under photoheterotrophic, nitrogen-limited, and dark conditions (Nakajima et al., 2014, 2017; Wan et al., 2017). In vivo studies have shown that many genes of the cyanobacterial TCA cycle are unnecessary for normal growth (Broddrick et al., 2016). Even if expression of fumarase, which catalyses the reversible hydration/dehydration of fumarate to malate, is blocked, growth of cyanobacteria under continuous light is not affected (Rubin et al., 2015). Therefore, the oxidative reaction of SyMDH is also thought to be unnecessary in cyanobacteria, because fumarase-deficient cyanobacteria grow normally. These studies support our biochemical studies suggesting that the oxidative reaction of SyMDH is very weak and almost non-functional. The kinetic parameters of SyMDH were affected by temperature (Figures 6, 7 and Supplementary Figures S4, S5). Km-value for oxaloacetate was always lower than that for malate in range of 20–50°C, thus, it is considered that SyMDH always show higher affinity for oxaloacetate than malate within 20–50°C and the reaction direction of SyMDH tends to flow from oxaloacetate to malate within the growth temperature of Synechocystis 6803.
Our study revealed that SyMDH shows a higher affinity for substances produced through the reductive reaction than those produced through the oxidative reaction, similar to MDHs derived from anaerobic microorganisms in which the oxidative TCA cycle seems to be barely functioning. Cyanobacteria have been found to close the TCA cycle using various bypasses (Zhang and Bryant, 2011; Steinhauser et al., 2012; Xiong et al., 2014). However, the results in this study indicate that the oxidative TCA cycle of Synechocystis 6803 may be functionally linear, and not cyclic in nature, because SyMDH preferentially undergoes a reductive reaction rather than an oxidative reaction and turns off the cyclic process of the oxidative TCA cycle.
Author Contributions
MT designed the research, performed the experiments, analysed the data, and wrote the manuscript. SI analysed the data. HS performed the experiments. TO analysed the data and wrote the manuscript.
Funding
This work was supported by the Ministry of Education, Culture, Sports, Science and Technology, Japan, by a grant to TO, from ALCA (Project Name “Production of cyanobacterial succinate by the genetic engineering of transcriptional regulators and circadian clocks”) (Grant No. JPMJAL1306), from the Japan Science and Technology Agency, and by JSPS KAKENHI Grant-in-Aid for Scientific Research on Innovative Areas (Grant No. 16H06559).
Conflict of Interest Statement
The authors declare that the research was conducted in the absence of any commercial or financial relationships that could be construed as a potential conflict of interest.
Supplementary Material
The Supplementary Material for this article can be found online at: https://www.frontiersin.org/articles/10.3389/fpls.2018.00947/full#supplementary-material
References
Bennett, B. D., Kimball, E. H., Gao, M., Osterhout, R., Van Dien, S. J., and Rabinowitz, J. D. (2009). Absolute metabolite concentrations and implied enzyme active site occupancy in Escherichia coli. Nat. Chem. Biol. 8, 593–599. doi: 10.1038/nchembio.186
Beyer, S., Gilch, S., Meyer, O., and Schmidt, I. (2009). Transcription of genes coding for metabolic key functions in Nitrosomonas europaea during aerobic and anaerobic growth. J. Mol. Microbiol. Biotechnol. 16, 187–197. doi: 10.1159/000142531
Broddrick, J. T., Rubin, B. E., Welkie, D. G., Du, N., Mih, N., Diamond, S., et al. (2016). Unique attributes of cyanobacterial metabolism revealed by improved genome-scale metabolic modeling and essential gene analysis. Proc. Natl. Acad. Sci. U.S.A. 113, E8344–E8353. doi: 10.1073/pnas.1613446113
Cendrin, F., Chroboczek, J., Zaccai, G., Eisenberg, H., and Mevarech, M. (1997). Cloning, sequencing, and expression in Escherichia coli of the gene coding for malate dehydrogenase of the extremely halophilic archaebacterium Haloarcula marismortui. Biochemistry 32, 4308–4313. doi: 10.1021/bi00067a020
Coleman, J. R., and Coleman, B. (1981). Inorganic carbon accumulation and photosynthesis in a blue-green alga as a function of external pH. Plant Physiol. 67, 917–921. doi: 10.1104/pp.67.5.917
Deutch, C. E. (2013). L-Malate dehydrogenase activity in the reductive arm of the incomplete citric acid cycle of Nitrosomonas europaea. Antonie Van Leeuwenhoek 104, 645–655. doi: 10.1007/s10482-013-9973-6
Eszes, C. M., Sessions, R. B., Clarke, A. R., Moreton, K. M., and Holbrook, J. J. (1996). Removal of substrate inhibition in a lactate dehydrogenase from human muscle by a single residue change. FEBS Lett. 399, 193–197. doi: 10.1016/S0014-5793(96)01317-8
Ge, Y. D., Cao, Z. Y., Wang, Z. D., Chen, L. L., Zhu, Y. M., and Zhu, G. P. (2010). Identification and biochemical characterization of a thermostable malate dehydrogenase from the mesophile Streptomyces coelicolor A3(2). Biosci. Biotechnol. Biochem. 74, 2194–2201. doi: 10.1271/bbb.100357
Harmsen, H. J., Kengen, H. M., Akkermans, A. D., Stams, A. J., and Vos, W. M. (1996). Detection and localization of syntrophic propionate-oxidizing bacteria in granular sludge by in situ hybridization using 16S rRNA-based oligonucleotide probes. Appl. Environ. Microbiol. 62, 1656–1663.
Hasunuma, T., Matsuda, M., and Kondo, A. (2016). Improved sugar-free succinate production by Synechocystis sp. PCC 6803 following identification of the limiting steps in glycogen catabolism. Metab. Eng. Commun. 3, 130–141. doi: 10.1016/j.meteno.2016.04.003
Honka, E., Fabry, S., Niermann, T., Palm, P., and Hensel, R. (1990). Properties and primary structure of the L-malate dehydrogenase from the extremely thermophilic archaebacterium Methanothermus fervidus. Eur. J. Biochem. 188, 623–632. doi: 10.1111/j.1432-1033.1990.tb15443.x
Huynen, M. A., Dandekar, T., and Bork, P. (1999). Variation and evolution of the citric-acid cycle: a genomic perspective. Trends Microbiol. 7, 281–291. doi: 10.1016/S0966-842X(99)01539-5
Ito, S., Takeya, M., and Osanai, T. (2017). Substrate specificity and allosteric regulation of a d-lactate dehydrogenase from a unicellular cyanobacterium are altered by an amino acid substitution. Sci. Rep. 7:15052. doi: 10.1038/s41598-017-15341-5
Knoop, H., Gründel, M., Zilliges, Y., Lehmann, R., Hoffmann, S., Lockau, W., et al. (2013). Flux balance analysis of cyanobacterial metabolism: the metabolic network of Synechocystis sp. PCC 6803. PLoS Comput. Biol. 9:e1003081. doi: 10.1371/journal.pcbi.1003081
Labrou, N. E., and Clonis, Y. D. (1997). L-Malate dehydrogenase from Pseudomonas stutzeri: purification and characterization. Arch. Biochem. Biophys. 337, 103–114. doi: 10.3923/ajbkr.2011.478.485
Mangan, N. M., Flamholz, A., Hood, R. D., Milo, R., and Savage, D. F. (2016). pH determines the energetic efficiency of the cyanobacterial CO2 concentrating mechanism. Proc. Natl. Acad. Sci. U.S.A. 113, E5354–E5362. doi: 10.1073/pnas.1525145113
Matsunaga, T., Takeyama, H., Sudo, H., Oyama, N., Ariura, S., Takano, H., et al. (1991). Glutamate production from CO2 by marine cyanobacterium Synechococcus sp. using a novel biosolar reactor employing light-diffusing optical fibers. Appl. Biochem. Biotechnol. 28:157. doi: 10.1007/BF02922597
Mikulášová, D., Kollárová, M., Miginiac-Maslow, M., Decottignies, P., Jacquot, J. P., Kutejová, E., et al. (1998). Purification and characterization of the malate dehydrogenase from Streptomyces aureofaciens. FEMS Microbiol. Lett. 159, 299–305. doi: 10.1016/S0378-1097(97)00567-3
Minárik, P., Tomásková, N., Kollárová, M., and Antalík, M. (2002). Malate dehydrogenases structure and function. Gen. Physiol. Biophys. 21, 257–265.
Molenaar, D., van der Rest, M. E., and Petrović, S. (1998). Biochemical and genetic characterization of the membrane associated malate dehydrogenase (acceptor) from Corynebacterium glutamicum. Eur. J. Biochem. 25, 395–403. doi: 10.1046/j.1432-1327.1998.2540395.x
Muro-Pastor, M. I., and Florencio, F. J. (1992). Purification and properties of NADP-isocitrate dehydrogenase from the unicellular cyanobacterium Synechocystis sp. PCC 6803. Eur. J. Biochem. 203, 99–105. doi: 10.1111/j.1432-1033.1992.tb19833.x
Muro-Pastor, M. I., and Florencio, F. J. (1994). NADP(+)-isocitrate dehydrogenase from the cyanobacterium Anabaena sp. strain PCC 7120: purification and characterization of the enzyme and cloning, sequencing, and disruption of the icd gene. J. Bacteriol. 176, 2718–2726. doi: 10.1128/jb.176.9.2718-2726.1994
Nakajima, T., Kajihata, S., Yoshikawa, K., Matsuda, F., Furusawa, C., Hirasawa, T., et al. (2014). Integrated metabolic flux and omics analysis of Synechocystis sp. PCC 6803 under mixotrophic and photoheterotrophic conditions. Plant Cell Physiol. 55, 1605–1612. doi: 10.1093/pcp/pcu091
Nakajima, T., Yoshikawa, K., Toya, Y., Matsuda, F., and Shimizu, H. (2017). Metabolic flux analysis of the Synechocystis sp. PCC 6803 ΔnrtABCD mutant reveals a mechanism for metabolic adaptation to nitrogen-limited conditions. Plant Cell Physiol. 58, 537–545. doi: 10.1093/pcp/pcw233
Osanai, T., Imashimizu, M., Seki, A., Sato, S., Tabata, S., Imamura, S., et al. (2009). ChlH, the H subunit of the Mg-chelatase, is an anti-sigma factor for SigE in Synechocystis sp. PCC 6803. Proc. Natl. Acad. Sci. U.S.A. 106, 6860–6865. doi: 10.1073/pnas.0810040106
Osanai, T., Oikawa, A., Shirai, T., Kuwahara, A., Iijima, H., Tanaka, K., et al. (2014). Capillary electrophoresis-mass spectrometry reveals the distribution of carbon metabolites during nitrogen starvation in Synechocystis sp. PCC 6803. Environ. Microbiol. 16, 512–524. doi: 10.1111/1462-2920.12170
Osanai, T., Shirai, T., Iijima, H., Nakaya, Y., Okamoto, M., Kondo, A., et al. (2015). Genetic manipulation of a metabolic enzyme and a transcriptional regulator increasing succinate excretion from unicellular cyanobacterium. Front. Microbiol. 6:1064. doi: 10.3389/fmicb.2015.01064
Owen, O. E., Kalhan, S. C., and Hanson, R. W. (2002). The key role of anaplerosis and cataplerosis for citric acid cycle function. J. Biol. Chem. 277, 30409–30412. doi: 10.1074/jbc.R200006200
Pitson, S. M., Mendz, G. L., Srinivasan, S., and Hazell, S. L. (1999). The tricarboxylic acid cycle of Helicobacter pylori. Eur. J. Biochem. 260, 258–267. doi: 10.1046/j.1432-1327.1999.00153.x
Rubin, B. E., Wetmore, K. M., Price, M. N., Diamond, S., Shultzaberger, R. K., Lowe, L. C., et al. (2015). The essential gene set of a photosynthetic organism. Proc. Natl. Acad. Sci. U.S.A. 112, 6634–6643. doi: 10.1073/pnas.1519220112
Singh, R., Lemire, J., Mailloux, R. J., and Appanna, V. D. (2008). A novel strategy involved in [corrected] anti-oxidative defense: the conversion of NADH into NADPH by a metabolic network. PLoS One 3:e2682. doi: 10.1371/journal.pone.0002682
Steinhauser, D., Fernie, A. R., and Araújo, W. L. (2012). Unusual cyanobacterial TCA cycles: not broken just different. Trends Plant Sci. 17, 503–509. doi: 10.1016/j.tplants.2012.05.005
Stetter, K. O., Thomm, M., Winter, J. G. W., Juber, H., Zillig, W., Janecovic, D., et al. (1981). Methanothermus fervidus sp., a novel extremely thermophilic methanogen isolated from an icelandic hot spring. Zentralbl. Bakterial. Hyg. I Abt. Orig. C 2, 166–178. doi: 10.1016/S0721-9571(81)80038-5
Takahashi-Íñiguez, T., Aburto-Rodríguez, N., Vilchis-González, A. L., and Flores, M. E. (2016). Function, kinetic properties, crystallization, and regulation of microbial malate dehydrogenase. J. Zhejiang Univ. Sci. B 17, 247–261. doi: 10.1631/jzus.B1500219
Takeya, M., Hirai, M. Y., and Osanai, T. (2017). Allosteric inhibition of phosphoenolpyruvate carboxylases is determined by a single amino acid residue in cyanobacteria. Sci. Rep. 24:41080. doi: 10.1038/srep41080
Thompson, H., Tersteegen, A., Thauer, R. K., and Hedderich, R. (1997). Two malate dehydrogenases in Methanobacterium thermoautotrophicum. Arch. Microbiol. 170, 38–42. doi: 10.1007/s002030050612
van Kuijk, B. L., and Stams, A. J. (1996). Purification and characterization of malate dehydrogenase from the syntrophic propionate-oxidizing bacterium strain MPOB. FEMS Microbiol. Lett. 144, 141–144. doi: 10.1111/j.1574-6968.1996.tb08520.x
Wan, N., DeLorenzo, D. M., He, L., You, L., Immethun, C. M., Wang, G., et al. (2017). Cyanobacterial carbon metabolism: fluxome plasticity and oxygen dependence. Biotechnol. Bioeng. 114, 1593–1602. doi: 10.1002/bit.26287
Wise, D. J., Anderson, C. D., and Anderson, B. M. (1997). Purification and kinetic characterization of Haemophilus parasuis malate dehydrogenase. Arch. Biochem. Biophys. 344, 176–183. doi: 10.1006/abbi.1997.0186
Wu, H., Li, Z. M., Zhou, L., and Ye, Q. (2007). Improved succinic acid production in the anaerobic culture of an Escherichia coli pflB ldhA double mutant as a result of enhanced anaplerotic activities in the preceding aerobic culture. Appl. Environ. Microbiol. 73, 7837–7843. doi: 10.1128/AEM.01546-07
Wynne, S. A., Nicholls, D. J., Scawen, M. D., and Sundaram, T. K. (1996). Tetrameric malate dehydrogenase from a thermophilic Bacillus: cloning, sequence and overexpression of the gene encoding the enzyme and isolation and characterization of the recombinant enzyme. Biochem. J. 317(Pt 1), 235–245. doi: 10.1042/bj3170235
Xiong, W., Brune, D., and Vermaas, W. F. (2014). The γ-aminobutyric acid shunt contributes to closing the tricarboxylic acid cycle in Synechocystis sp. PCC 6803. Mol. Microbiol. 93, 786–796. doi: 10.1111/mmi.12699
Xiong, W., Morgan, J. A., Ungerer, J., Wang, B., Maness, P. C., and Yu, J. (2015). The plasticity of cyanobacterial metabolism supports direct CO2 conversion to ethylene. Nat. Plants 1:15053. doi: 10.1038/nplants.2015.53
Zhang, S., and Bryant, D. A. (2011). The tricarboxylic acid cycle in cyanobacteria. Science 334, 1551–1553. doi: 10.1126/science.1210858
Keywords: biochemistry, cyanobacteria, malate dehydrogenase, metabolic enzyme, TCA cycle
Citation: Takeya M, Ito S, Sukigara H and Osanai T (2018) Purification and Characterisation of Malate Dehydrogenase From Synechocystis sp. PCC 6803: Biochemical Barrier of the Oxidative Tricarboxylic Acid Cycle. Front. Plant Sci. 9:947. doi: 10.3389/fpls.2018.00947
Received: 28 November 2017; Accepted: 12 June 2018;
Published: 13 July 2018.
Edited by:
Peer Schenk, The University of Queensland, AustraliaReviewed by:
Martin Hagemann, University of Rostock, GermanyShuyi Zhang, Massachusetts Institute of Technology, United States
Copyright © 2018 Takeya, Ito, Sukigara and Osanai. This is an open-access article distributed under the terms of the Creative Commons Attribution License (CC BY). The use, distribution or reproduction in other forums is permitted, provided the original author(s) and the copyright owner(s) are credited and that the original publication in this journal is cited, in accordance with accepted academic practice. No use, distribution or reproduction is permitted which does not comply with these terms.
*Correspondence: Takashi Osanai, dG9zYW5haUBtZWlqaS5hYy5qcA==