- 1National Engineering Laboratory of Crop Stress Resistance Breeding, School of Life Sciences, Anhui Agricultural University, Hefei, China
- 2Laboratory of Modern Biotechnology, School of Forestry and Landscape Architecture, Anhui Agricultural University, Hefei, China
TEOSINTE BRANCHED 1, CYCLOIDEA, and PROLIFERATING CELL FACTORS (T), members of a plant-specific gene family, play significant roles during plant growth and development, as well as in response to environmental stress. However, knowledge about this family in moso bamboo (Phyllostachys edulis) is limited. Therefore, in this study, the first genome-wide identification, classification, characterization, and expression pattern analysis of the TCP transcription factor family in moso bamboo was performed. Sixteen TCP members were identified from the moso bamboo genome using a BLASTP algorithm-based method and verified using the Pfam database. Based on a multiple-sequence alignment, the members were divided into two subfamilies, and members of the same family shared highly conserved motif structures. Subcellular localization and transactivation activity analyses of four selected genes revealed that they were nuclear localized and had self-activation activities. Additionally, the expression levels of several PeTCP members were significantly upregulated under abscisic acid, methyl jasmonate, and salicylic acid treatments, indicating that they play crucial plant hormone transduction roles in the processes of plant growth and development, as well as in responses to environmental stresses. Thus, the current study provides previously lacking information on the TCP family in moso bamboo and reveals the potential functions of this gene family in growth and development.
Introduction
Plants encounter a variety of environmental stresses during their growth and development; therefore, they possess various protective systems at the whole-plant, tissue, cellular, subcellular, genetic, and molecular levels (Sheshadri et al., 2016). Stress-inducible genes are the major molecular factors involved in environmental stress responses and increased tolerance (Yamaguchishinozaki and Shinozaki, 2005). These genes have generally been divided into two categories, one involved directly in stress tolerance and the other in signal transduction and transcriptional regulation. Transcription factors (TFs) are important groups of regulatory genes (Wu et al., 2015). A series of TFs that are involved in governing developmental responses to the environment have been identified and reported in plants, such as MYB (salt and drought tolerance) (Zhang et al., 2012; Cui et al., 2013), ERF (ethylene responsive factors) (Lata et al., 2014), WRKY (regulation of stress response) (He et al., 2012; Yan et al., 2014; Cai et al., 2017), HD-Zip (drought and salt tolerance) (Zhao et al., 2011, 2014), and bZIP (pathogen defense regulation) (Sheshadri et al., 2016). In addition, a previous study reported that TCP TFs directly translate environmental signals to cope with environmental stresses during plant growth (Danisman, 2016).
TCPs, a family of plant-specific TFs (Martíntrillo and Cubas, 2010), were named based on the first three identified members, maize teosinte branched1 (TB1), Antirrhinum cycloidea (CYC), and rice PCF proteins (PCFs) (Doebley et al., 1997; Kosugi and Ohashi, 1997; Cubas et al., 1999; Luo et al., 1999). The TCPs contain a distinguished domain with a non-canonical basic helix–loop–helix structure based on a sequence alignment analysis of TCP proteins, namely TCP domain (Doebley et al., 1997; Kosugi and Ohashi, 1997; Cubas et al., 1999; Luo et al., 1999). Interestingly, most of the members of the ECE clade contain a conserved R domain that might be involved in protein–protein interactions (Cubas et al., 1999), while some members of the CIN clade independently acquired an R domain (Cubas, 2002).
At present, many functional analyses of TCPs have been reported in various plant developmental processes. For example, TCPs play essential roles during the morphogenetic period in plants. TB1 and TB1-like proteins from maize, rice, sorghum, and Arabidopsis repress the development of axillary branches (Hubbard et al., 2002; Takeda et al., 2003; Kebrom et al., 2006; Aguilar-Martínez et al., 2007; Finlayson, 2007). In Arabidopsis, some members are involved in leaf development. For example, TCP14 and TCP15 interact with the ubiquitin receptors DA1, DAR1, and DAR2, which regulate internode length and leaf shape by repressing endoreduplication (Peng et al., 2015). Five members (TCP2, TCP3, TCP4, TCP10, and TCP24) have been implicated in the regulation of leaf morphogenesis (Schommer et al., 2008). Additionally, AtTCP20, acting upstream of AtTCP9, controls leaf development through the jasmonate signaling pathway. GhCYC2, a TCP member in chrysanthemum, regulates the development of petals (Broholm et al., 2008). In Pisum sativum, two CYC-like TCP proteins control floral zygomorphy (Wang et al., 2008). In cotton, TCPs play positive roles in fiber elongation (Hao et al., 2012). In addition, TCP proteins might play important roles during the generative growth phase, such as in the development and maturation of fruits (Parapunova et al., 2014). TCPs not only contribute to plant morphogenetics, they also mediate the regulation of rhythms. For instance, TCP proteins can regulate the circadian clock in Arabidopsis thaliana (Giraud et al., 2010) through binding to the TGGGC(C/T) elements. Furthermore, TCP TFs contribute to plant resistance to diverse abiotic stresses. For example, OsPCF5 is involved in drought- and salinity-stress tolerance, and OsPCF6 takes part in cold-stress tolerance in rice (Wang et al., 2014). OsTCP15 plays a part in the mesocotyl elongation response to darkness in rice (Hu et al., 2014). TCP20 can interact with NLP6&7 and support root meristem growth under N starvation conditions in Arabidopsis thaliana (Guan et al., 2017). OsPCF2 activates OsNHX1 expression and enhances its salt tolerance (Almeida et al., 2016). Furthermore, OsPCF6 and OsTCP21, identified as target genes of Osa-miR319b, are involved in cold-stress tolerance (Wang et al., 2014).
Moso bamboo with high ecological, cultural, and economic values is faced with various environmental stresses in the course of its growth and development. This requires moso bamboo to respond and resist adverse conditions in a timely manner. Genomic studies in bamboo, including genome-wide full-length cDNA sequencing (Peng et al., 2010), chloroplast genome sequencing (Zhang et al., 2011), identification of syntenic genes between bamboo and other grasses (Gui et al., 2010), phylogenetic analysis of Bambusoideae subspecies (Sungkaew et al., 2009), and the construction of the draft genome sequence of moso bamboo, laid the foundation for researching and improving stress resistance at the genetic level. So far, various gene families having potential resistance functions in moso bamboo have been analyzed, such as the WRKY TF family (Li et al., 2017; Wu M. et al., 2017), homeodomain leucine zipper subfamily (Chen et al., 2017), and AP2/ERF TF family (Wu et al., 2015). The study of TCP TFs remains enigmatic, even though they play significant roles in plant development and growth as well as in stress resistance. In the present study, we searched the moso bamboo genome to identify the genes encoding TCP TFs (PeTCPs). In total, 16 PeTCPs were identified from the moso bamboo genome. The characteristics of these genes, including structure, phylogenetic relationship, promoter elements, evolution divergence, subcellular localization, transactivation activity, and expression patterns, were subsequently identified.
Materials and Methods
Identification of TCP Genes
To obtain comprehensive and non-redundant moso bamboo proteins containing the TCP domain, a BLASTP algorithm-based search provided by the BambooGDB database1 was first performed using the seed sequences of reported rice TCP proteins (Yao et al., 2007; Mondragónpalomino and Trontin, 2011; Dhaka et al., 2017). The default parameters were adopted, and the cutoff value was set to 0.01. The obtained putative TCP proteins were subsequently removed manually and further verified using the Pfam2 database (Finn et al., 2006, 2010). Detailed information on each putative TCP protein, coding sequences (CDSs), and amino acid lengths, as well as physicochemical parameters, were obtained from the BambooGDB database. Subcellular localizations of PeTCPs were predicted using ProtComp 9.0. Gene IDs of rice, Brachypodium distachyon, Arabidopsis thaliana, poplar, and Sorghum were obtained from earlier reports (Yao et al., 2007; Francis et al., 2016; Ma et al., 2016) and their sequences were downloaded from TIGR3, BioMart4, TAIR5, and Phytozome6 databases, respectively.
Phylogenetic and Multiple Sequence Alignment Analysis
To study the phylogenetic relationships of TCP genes among different species, Clustal X 2.0 (Larkin et al., 2007) was used to perform multiple sequence alignments, and MEGA 6.0 (Tamura et al., 2013) was subsequently used to construct a phylogenetic tree based on the multiple alignment results by using the neighbor-joining method (parameters: 1,000 bootstraps).
Gene Structure and Conserved Motif Analyses
To investigate the exon/intron structures of the TCP genes, the Gene Structure Display Server was used with the corresponding CDSs and genomic DNA sequences on default parameter settings. The online tool MEME7 was used to identify and analyze the conserved motifs of TCP proteins (parameter setting: maximum number of motifs, 10; maximum width, 100).
Synonymous (Ka) to Non-synonymous (Ks) Mutation Ratio Analyses Among Moso Bamboo, Rice, Maize, and Brachypodium distachyon
Ka and Ks were computed using DnaSP 5 software (Librado and Rozas, 2009) based on the pairwise alignment of homologous pairs between moso bamboo and other grass species using MEGA 6.0. The divergence time (T) was calculated using the formula T = Ks/2λ (λ = 6.5 × 10-9) (Peng et al., 2013).
Putative Promoter cis-Acting Element Analysis
The 2,000-bp upstream sequences of the PeTCP gene sequences were submitted to PlantCARE (Lescot et al., 2002)8 to predict putative promoter cis-acting elements.
Plant Material Growth Conditions and Stress Treatments
Three-month-old seedlings were germinated and grown in an artificial growth chamber (planted in a flowerpot with a diameter of approximately 20 cm) with a 16-h light/8-h dark cycle at 22°C. These seeds had been collected in the Tianmu Mountain National Nature Reserve in Zhejiang Province, China. To analyze the tissue expression patterns of TCP genes, samples of six tissues, including mature leaves, young leaves, stems, shoots, rhizomes, and roots, were collected. To investigate the expression patterns of TCP genes under stress-related plant hormone treatments, seedlings were sprayed with 0.1 mM abscisic acid (ABA), 0.1 mM methyl jasmonate (Me-JA), or 1 mM salicylic acid (SA) treatments. Additionally, control samples were similarly sprayed with distilled water. For each stress treatment, all of the samples were collected at six time points (0, 1, 3, 6, 12, and 24 h). All plant samples were immediately stored in a -80°C freezer after collection for RNA extraction. Three repeated trials and three biological replicates were performed for each sample.
Transactivation Activity Analysis in Yeast
The pGBKT7 vector (Clontech, Palo Alto, CA, United States) was used to study the transcriptional activity levels of four PeTCP proteins in yeast. The full-length PeTCPs open reading frames were PCR amplified and independently cloned into the pGBKT7 vector (Clontech; containing the GAL4 DNA-binding domain) using the gene-specific primers listed in Supplementary Table S5. Subsequently, pGBKT7-PeTCP recombinant vectors, the positive control pGBKT7-53+pGADT7-T, and the negative control pGBKT7 empty plasmids were used to transform the yeast strain using the lithium acetate method. The transformed strains were further serially cultured on various SD selective media, including SD/-Trp and SD/-Trp/-His/-Ade/X-α-Gal, and incubated at 30°C for 3–5 days.
qRT-PCR Analysis
The qRT-PCR experiment was used to explore the expression levels of TCP members in different tissues or developmental stages, and under abiotic stress and plant hormone treatments. Total RNA was extracted from the plant samples using TRIzol and cetrimonium bromide methods and was then reverse transcribed into cDNA using a PrimeScriptTM RT Reagent Kit (TaKaRa, Dalian, China). Gene-specific primers were designed using Primer Express 3.0, and tonoplast intrinsic protein 41 (TIP41) was used as an internal control (Fan et al., 2013). TransStart® Tip Green qPCR Super Mix (TransGen Biotech, Beijing, China) was used in the qRT-PCR master mix and the program was run as specified in the instructions.
Statistical Analysis
Statistical significance was determined using a paired Student’s t-test9. The mean ± standard deviations from the mean (SD) of at least three replicates are presented, and significant differences relative to controls are indicated at ∗p < 0.05 and ∗∗p < 0.01.
Results
Identification and Characterization of TCP TFs
Prospective TCP members from the moso bamboo genome were obtained from BambooGDB using a BLASTP algorithm-based search with seed sequences from reported rice TCP proteins. A total of 16 TCPs, named PeTCP1–16 based on their physical locations on the scaffold (Table 1), were subsequently identified after confirming the presence of the conserved TCP DNA-binding domain (PF03634) using the Pfam database and deleting redundant sequences manually based on the results of a sequence alignment performed using Clustal X. The detailed characteristics of the PeTCPs, consisting of gene name, accession number, locational information, and physicochemical parameters, are provided in Table 1. The CDS lengths ranged from 303 to 1,221 bp, resulting in amino acid sequences ranging from 100 to 406 aa. The molecular weight ranged from 10.40 to 43.37 kDa, and the theoretical isoelectric point varied from 4.78 to 10.76. In addition, the locational information of PeTCP genes revealed that the 16 TCP genes were distributed in different scaffolds, with none of the genes being located on the same scaffold (Table 1).
To explore the phylogenetic relationship of TCP TFs among different species and investigate the potential function of PeTCP TFs compared with other well-studied TCP members, a phylogenetic tree was constructed using the neighbor-joining method. The tree was based on the sequence alignment and analysis of 137 full-length amino acid sequences from moso bamboo, rice (Yao et al., 2007), Brachypodium distachyon, sorghum (Francis et al., 2016), Arabidopsis thaliana (Yao et al., 2007), and poplar (Ma et al., 2016; Figure 1A). The information on TCPs was obtained from previous studies (Supplementary Table S1). TCPs can be divided into two distinct classes: Class I and Class II. Class I was named the PCF group (Cubas, 2002). Class II was further divided into two groups: CIN and CYC/TB1. In moso bamboo, the number of TCPs identified as PCF, CIN, and CYC/TB1 protein members were 10, 5, and 1, respectively. The counting of TCP TFs among the six species showed that the maximum and minimum numbers of TCPs were found in poplar and moso bamboo, respectively (Figure 1B). The CYC/TB1 group is the smallest.
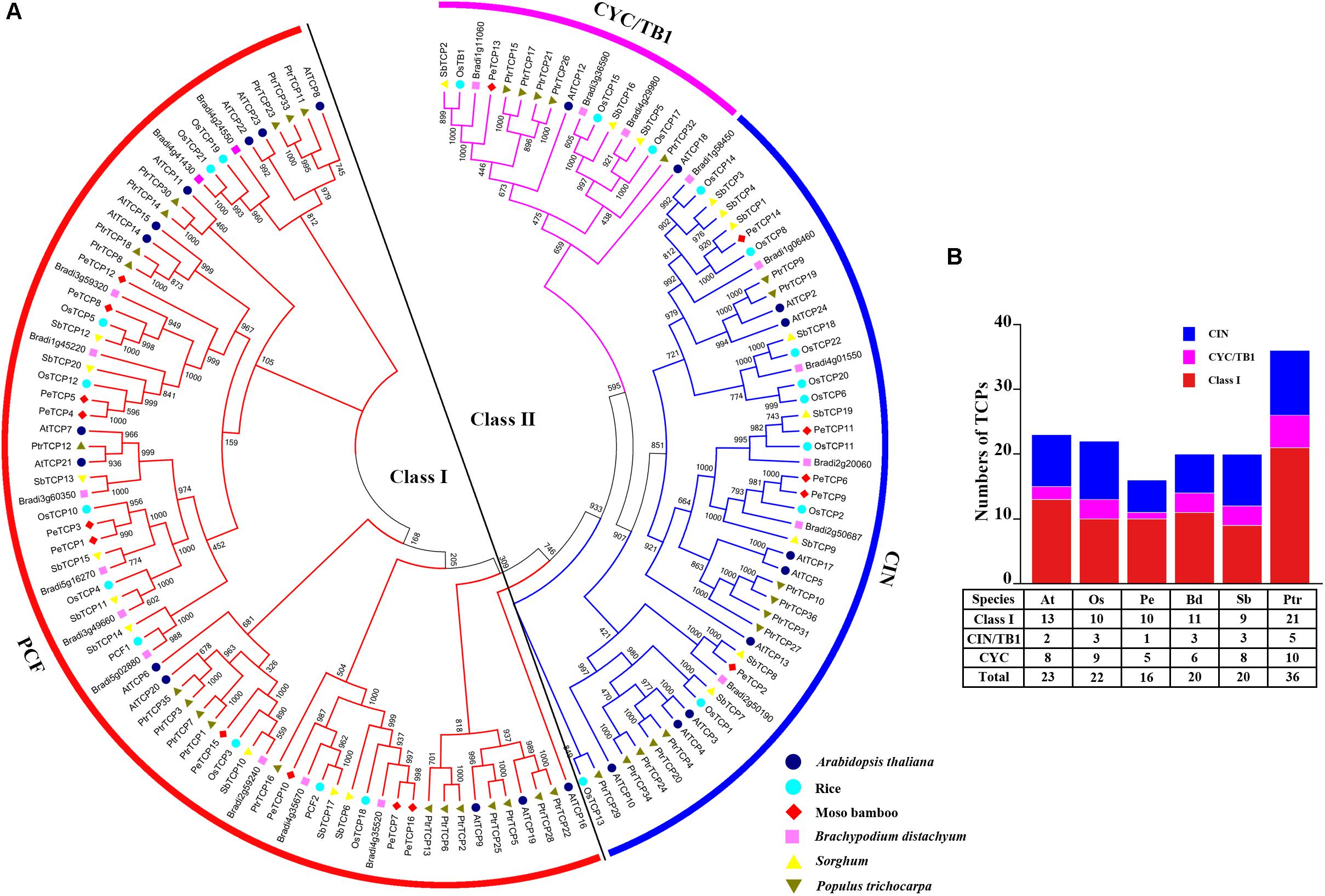
FIGURE 1. Phylogenetic analysis and statistical analysis of TCP members in different species. (A) Phylogenetic analysis of TCP members from moso bamboo, rice, Brachypodium distachyon, Sorghum, Arabidopsis thaliana, and poplar. Geometric figures of different colors and shapes are used to mark the TCP members from different species. (B) Statistical analysis of TCP members from moso bamboo, rice, Brachypodium distachyon, Sorghum, Arabidopsis thaliana, and poplar.
Multiple Sequences Alignment
To investigate the conservation and diversity of the TCP domain regions in TCP proteins, a multiple sequences alignment was performed using Clustal X software based on the amino acid sequences of each TCP domain (Figure 2). Alignment results showed that differences and similarities coexisted among these members. For example, complete basic helix I–loop–helix II regions could be found in all TCP members of Class II, resulting in the conservation of this group. In contrast, TCP members of Class I displayed a higher diversity level. For example, four genes, PeTCP4, PeTCP7, PeTCP8, and PeTCP10, contained only partial TCP domains. In addition, TCP members of Class II had 4-amino acid insertions compared with Class I in the basic regions. In addition, the arginine-rich R domain was found in the C-terminus of PeTCP13.
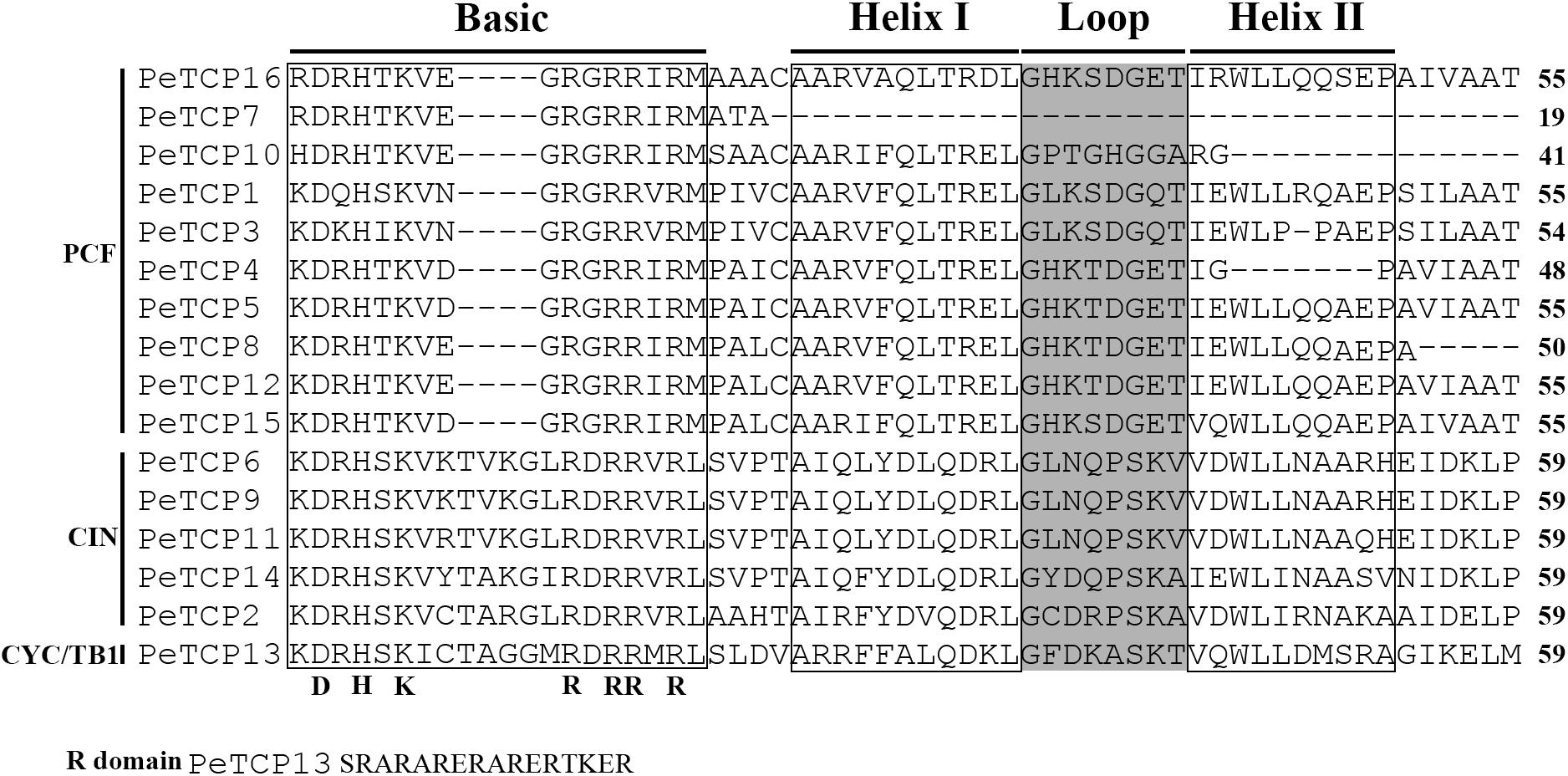
FIGURE 2. Multiple sequence analysis of TCP domain. Multiple sequence alignment was carried out with Clustal X 2.0.
Gene Structures and Conserved Motifs Analysis
The Gene Structures Display Server online tool was used to explore the exon/intron structures of PeTCP genes (Figure 3) based on the CDSs and the corresponding genomic DNA sequences of the PeTCP genes. Of these, 10 PeTCP genes lacked an intron, and the remaining members of the PeTCP genes only had one or two introns. This result was consistent with previous reports. As shown in Figure 2, the online MEME tool (parameter setting: maximum number of motifs, 10; maximum width, 100) was used to predict the conserved PeTCP protein motifs by submitting full-length amino acid sequences and identifying 10 specific motifs (Figure 4 and Supplementary Table S2). The sequence of each motif was verified using the Pfam database and only one motif (motif 1) encoded the TCP domains. The remaining motifs did not encode any domains. Motif 1 was present in every moso bamboo TCP protein we verified, providing further support for the reliability of our identification. Among the PeTCPs, the number of motifs varied from one (PeTCP10) to nine (PeTCP5). Some motifs were specific to particular members. For example, motif 2 existed only in PeTCP4, 5, 8, and 12; motif 10 was present only in PeTCP4, 5, 8, 12, and 15; and motif 8 only appeared in PeTCP4, 5, 7, and 16. These three motifs existed in Class I with a high specificity. Furthermore, all the members of the Class II PeTCPs were characterized by motif 7 in the N-terminal TCP domain. By comparison, the C-terminal TCP domain of motif 3 was detected in various PeTCP members that were widely distributed in the TCP family, except four PeTCP genes (PeTCP3, 4, 7, and 10).
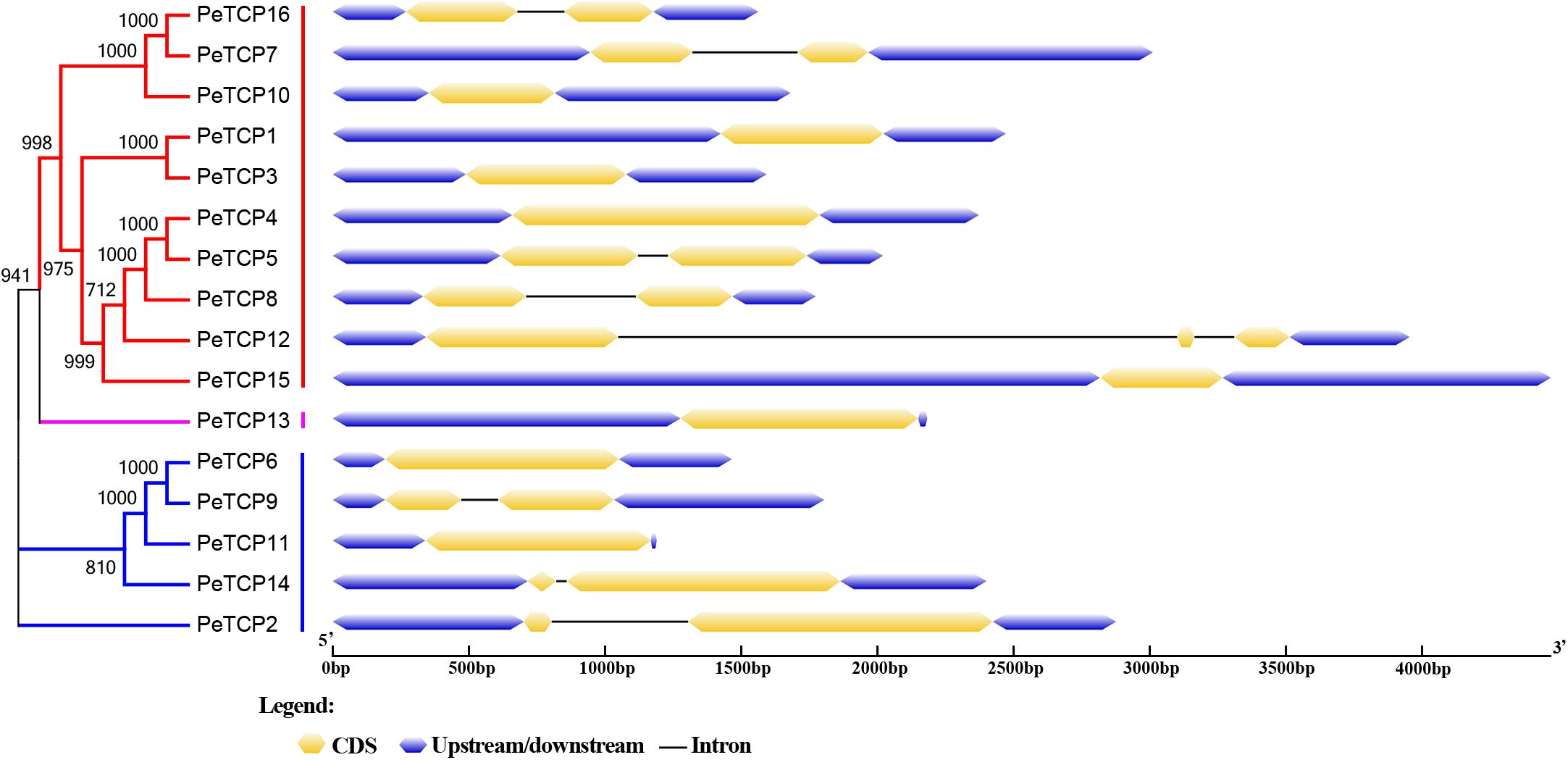
FIGURE 3. Gene structures of TCPs in moso bamboo. Gene structures were performed using the GSDS online tool. Exons, introns, and untranslated regions (UTRs) are indicated by yellow rectangles, gray lines, and blue rectangles, respectively.
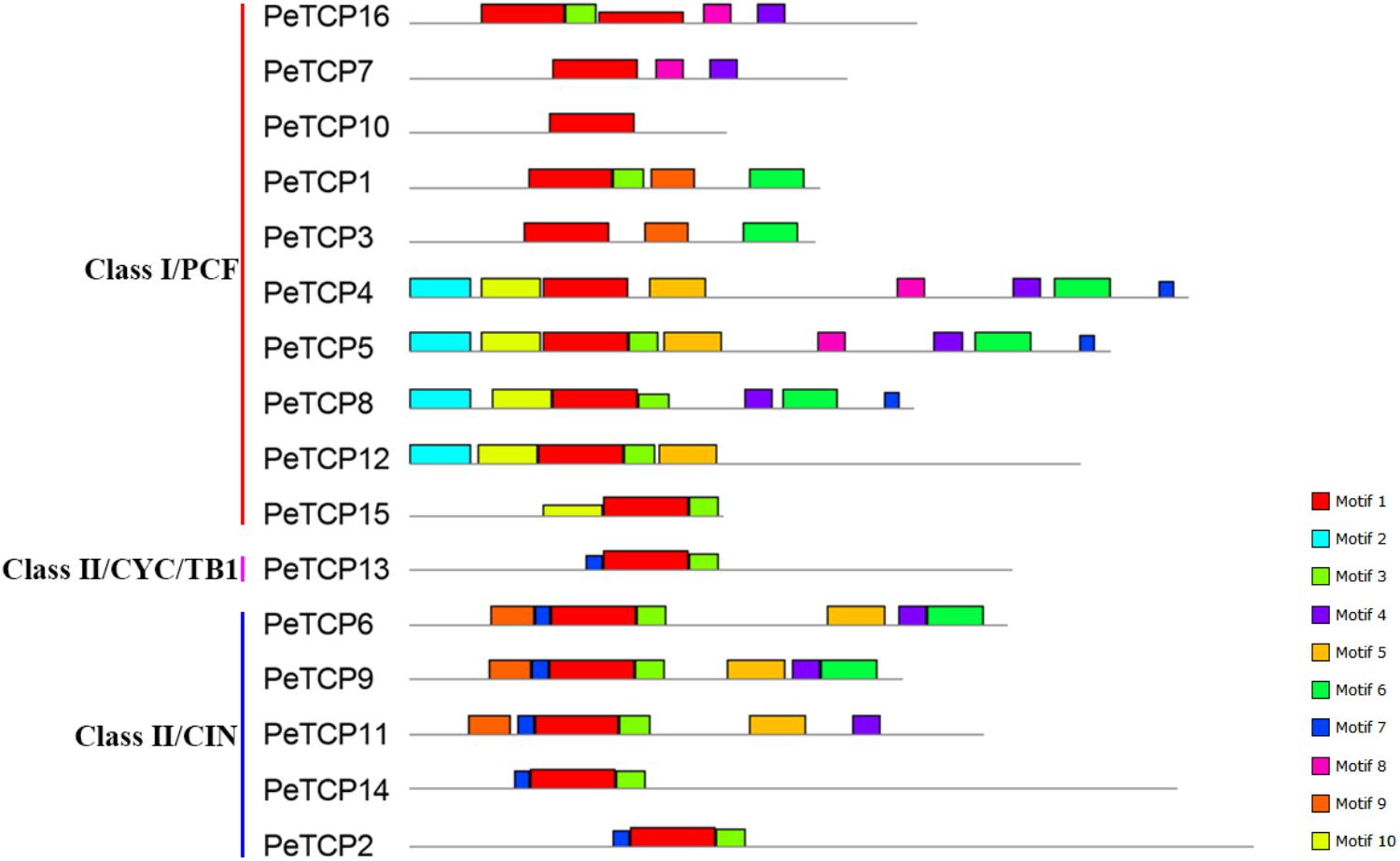
FIGURE 4. Schematic representation of the 10 conserved motifs in PeTCPs. Conserved motifs of the PeTCPs were identified using the online MEME program based on 16 full-length amino acid sequences with the following parameters: maximum number of motifs, 10; maximum width, 100. The lengths and positions of different motifs in the protein sequences are identified by the lengths and positions of the different color blocks.
Putative Promoter cis-Acting Element Analysis of Stress-Related Plant Hormone
cis-Elements are linear nucleotide fragments of non-coding DNA (Biłas et al., 2016). They are present in promoter regions and other transcribed DNA strands (Vaughn et al., 2012). The promoter regions of stress-inducible genes contain cis-elements that directly influence the gene regulation involved in stress-responsive gene expression (Yamaguchishinozaki and Shinozaki, 2005). Many stress-responsive genes that encode TFs, with functions of signal transduction and transcriptional regulation, play significant roles in environmental stress tolerance (Yamaguchishinozaki and Shinozaki, 2005; Sheshadri et al., 2016). Various TFs interact with cis-acting elements in promoter regions and form transcriptional initiation complexes on the TATA box (core promoter) upstream of the transcriptional initiation site. The transcriptional initiation complex then activates RNA polymerase to start the transcription of stress-responsive genes.
When plants are under stressed conditions, TF binding to cis-elements is the major genetic level change occurring in the plants. Hence, identifying the cis-acting elements in the stress-responsive promoters is important to understand the molecular switches affected by stress-inducible genes. In this study, to explore the cis-element patterns and types of PeTCP genes, the cis-elements in the 2,000 bp upstream of the promoter regions of 16 PeTCP genes were predicted using the PlantCARE database. Many types of cis-elements involved in environmental stress responses were identified, including ABA-, Me-JA-, and SA-responsive elements (Figure 5). The TCA-element (Goldsbrough et al., 1993) and SARE motif associated with SA responsiveness were present in nine PeTCP genes (PeTCP1–3, 5, 9–12, and 15). Interestingly, the Me-JA-responsive elements, CGTCA and TGACG, existed in pairs in six PeTCPs (PeTCP2, 7, 11, 13, 15, and 16). The ABA-responsive elements (ABRE cis-acting element) (Shen and Ho, 1995) were found in the promoter regions of PeTCP6–8, 10, 12, 14, and 15. Among these TCP genes and environmental stress-responsive elements, we found that PeTCP9 and PeTCP14 had single types of responsive elements, SA-responsive elements and ABAREs, respectively. The other members presented more than one type of stress-responsive elements, such as four types in PeTCP12 and three types in PeTCP15.
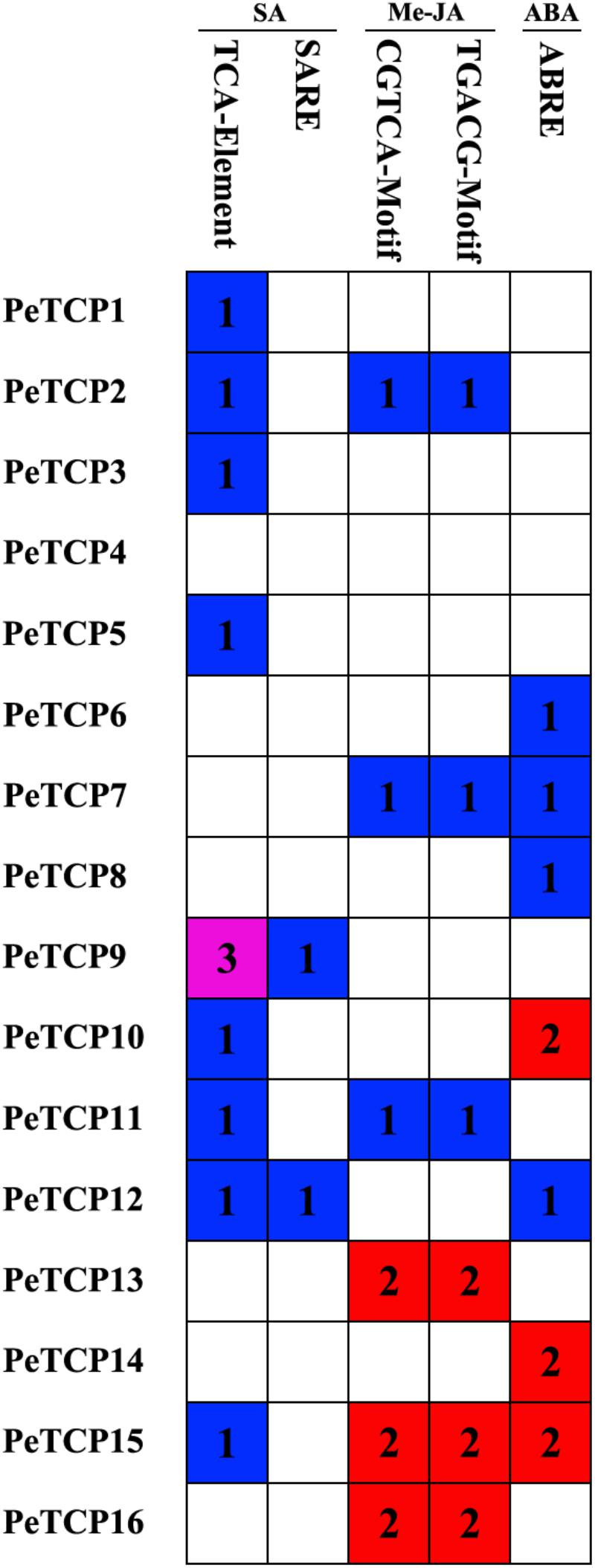
FIGURE 5. cis-Acting elements related to ABA, Me-JA, and SA in the promoter regions of PeTCPs. A colored block with a number represents the cis-element number of PeTCPs.
Selection Pressure and Divergence of TCPs Between Moso Bamboo and Three Other Grass Species
To explore the evolutionary patterns, divergence and selection pressure of the TCPs, the homologous pairs between moso bamboo and other three grass species (Supplementary Table S3), including eight paralogous pairs and 30 orthologous pairs, were identified using phylogeny-based and bidirectional best-hit methods. The Ka/Ks ratio is widely applied to measure genetic evolution and selection pressure. According to the natural selection theory, if the ratio is greater than 1, then positive selection is indicated; equal to 1 indicates neutral selection and less than 1 indicates negative or stabilizing selection. Scatter plot statistics showed that PeTCPs have undergone significant negative or stabilizing selection over the course of evolution (Figure 6). By contrast, the ratios of two homologous pairs (PeTCP2/OsTCP1 and PeTCP10/SbTCP15) were greater than 1, indicating positive selection during evolution (Figure 6). Meanwhile, the divergence times of the TCPs were also calculated. The eight paralogous pairs indicated that PeTCPs underwent duplication events from ∼5.39 to 58.90 million years ago (MYA). Additionally, the orthologous pairs demonstrated that PeTCPs and OsTCPs were separated around 19.35 to 55.73 MYA, BdTCPs around 22.48 to 59.87 MYA, and SbTCPs around 25.78 to 66.55 MYA.
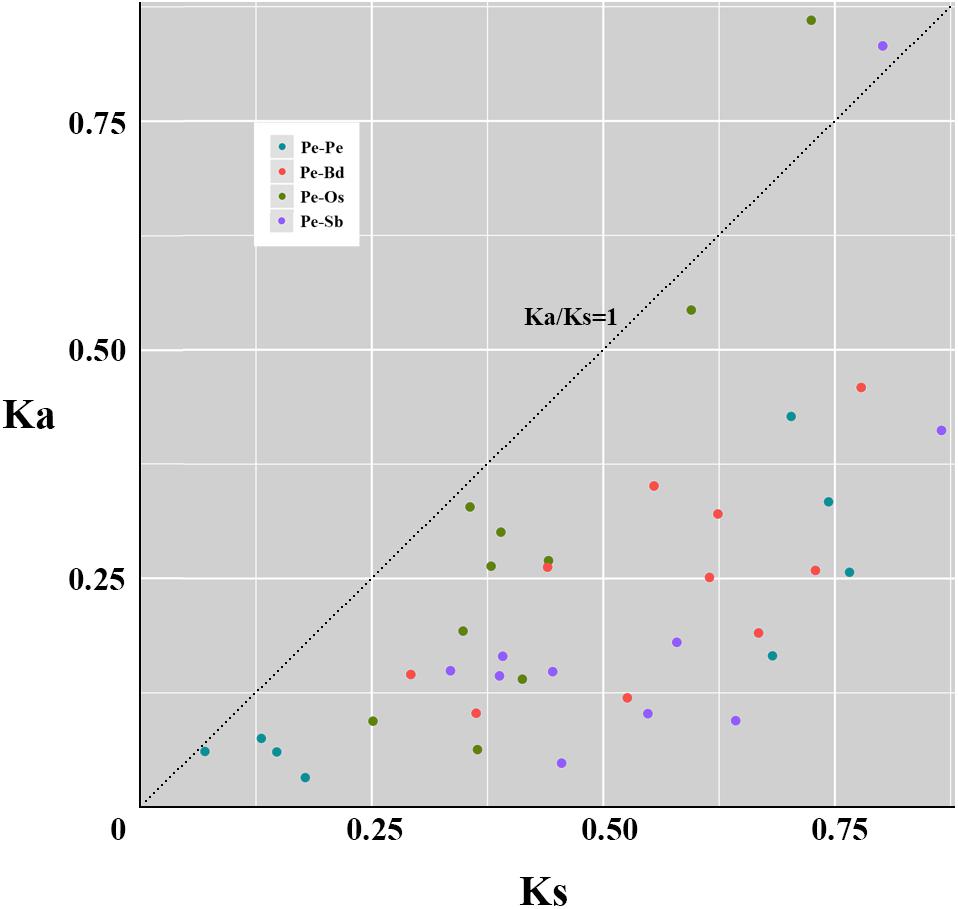
FIGURE 6. Scatter plot statistics of Ka and Ks values among grass species. The black dotted line with slope one is used to show Ka/Ks = 1. A circle of different colors exhibits homologous pairs of different types. Pe-Pe: paralogous pairs of PeTCPs; Pe-Os: orthlogous pairs of TCPs between moso bamboo and rice; Pe-Bd: orthlogous pairs of TCPs between moso bamboo and Brachypodium distachyon; Pe-Sb: orthlogous pairs of TCPs between moso bamboo and Sorghum.
Tissue-Specific TCP Expression Levels of Different Tissues and Development Stages in Moso Bamboo
Gene expression pattern analyses in various tissues and developmental stages can contribute to understanding the roles of genes. To research the expression patterns of PeTCP genes in different tissues or developmental stages, including mature leaf, young leaf, stem, shoot, rhizome, and root tissues from the same strain of moso bamboo, the related transcription levels of the PeTCP genes were analyzed using qRT-PCR. As exhibited in Figure 7, all 16 PeTCP genes in the young and mature leaf, 13 genes (except PeTCP1, 3, and 15) in the stem, 12 genes (except PeTCP1, 7, 8, and 13) in root, four genes (PeTCP5, 6, 9, and 11) in rhizome, and four genes (PeTCP5, 6, 11, and 14) in shoot showed high expression levels. In particular, three genes (PeTCP5, 6, and 11) showed high expression levels in all six tissues. In addition, all of the PeTCP genes displayed similar expression patterns between young and mature leaves, as well as between shoot and rhizome.
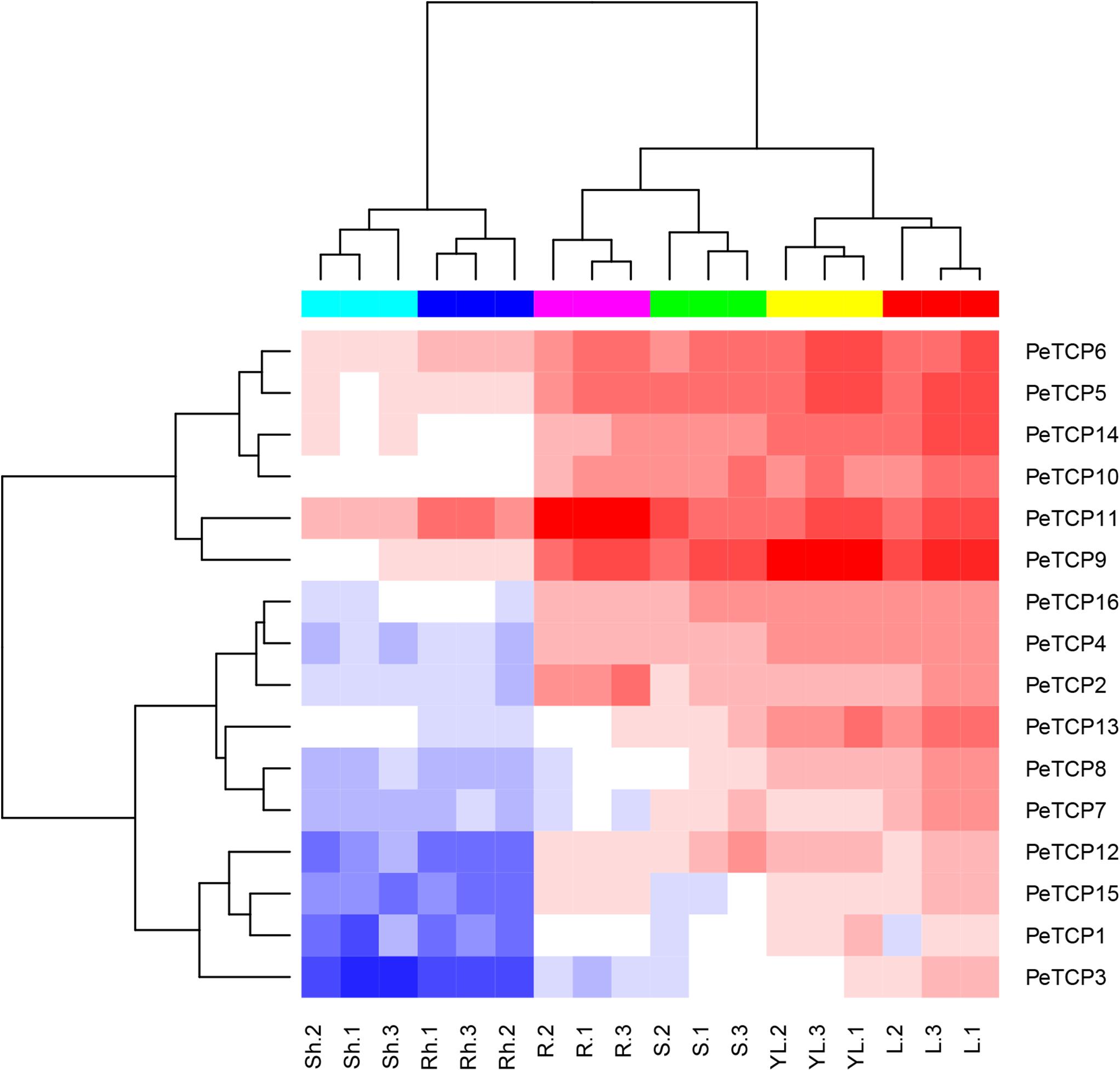
FIGURE 7. Expression patterns of TCP genes in different tissues and developmental stages of moso bamboo. Lanes: YL, young leaf; L, mature leaf; S, stem; Sh, shoot; Rh, rhizome; R, root. The mean values and SDs were obtained from three biological and three technical replicates.
Subcellular Localization and Transactivation Activity
In general, TFs can regulate the transcription of target genes by binding to specific cis-elements in their promoters and this binding occurs in the nucleus. Based on their characteristics as TFs, PeTCPs should localize in the nuclei. To examine this feature, the full-length CDSs without stop codons were cloned from moso bamboo cDNAs using specific primers (Supplementary Table S4). Later, they were independently transformed into the pCAMBIA1305 vector containing GFP under the control of the CaMV 35S promoter. The resulting 35S::GFP::PeTCPs and 35S::GFP fusion proteins were subsequently transiently expressed in Nicotiana tabacum leaves by Agrobacterium-mediated transformation. After 36 h of expression, the leaves of Nicotiana tabacum harboring the fusion proteins were observed using confocal laser scanning microscopy (Carl Zeiss LSM710, Germany). GFP fluorescence and light-field observations were recorded in separate channels and then merged into an overlay image. Fluorescence microscopy showed that PeTCP4, 5, 10, and 11 were clearly localized in the nucleus according to the GFP signals (Figure 8).
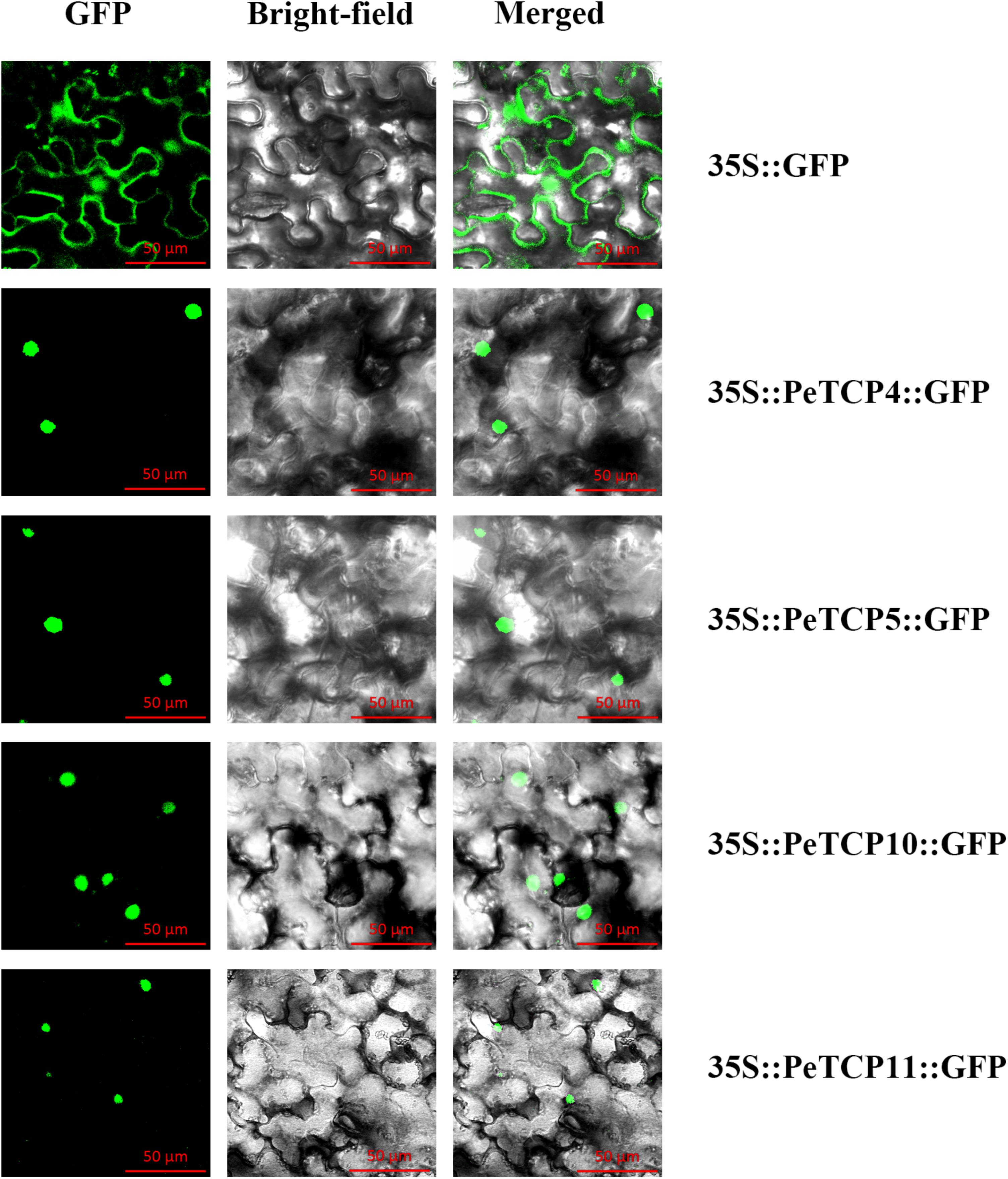
FIGURE 8. Subcellular localization of four PeTCPs. The four PeTCP-GFP fusion proteins (PeTCP4-GFP, PeTCP5-GFP, PeTCP10-GFP, and PeTCP11-GFP) and GFP as a control were transiently expressed in N. tabacum leaves and observed under a fluorescence microscope.
To explore the transactivation activity of PeTCPs, the pGBKT7::PeTCPs (specific primers listed in Supplementary Table S5), the positive control plasmids pGBKT7-53 and pGADT7-T, and the negative control plasmid were independently transformed into the Y2HGold yeast strain. All of these transformants could readily grow and exhibited visible white colonies on the SD/-Trp medium (Figure 9). In the SD/-Ade/-His/-Trp/X-α-GAL medium, only the yeast cells containing PeTCPs and the positive controls grew well and turned blue. In contrast, the negative control group did not grow on this medium (Figure 9). Thus, the four selected PeTCP fusion constructs activated the transcription of the His3 and LacZ reporter genes, indicating that they had transcriptional activity in yeast strains.
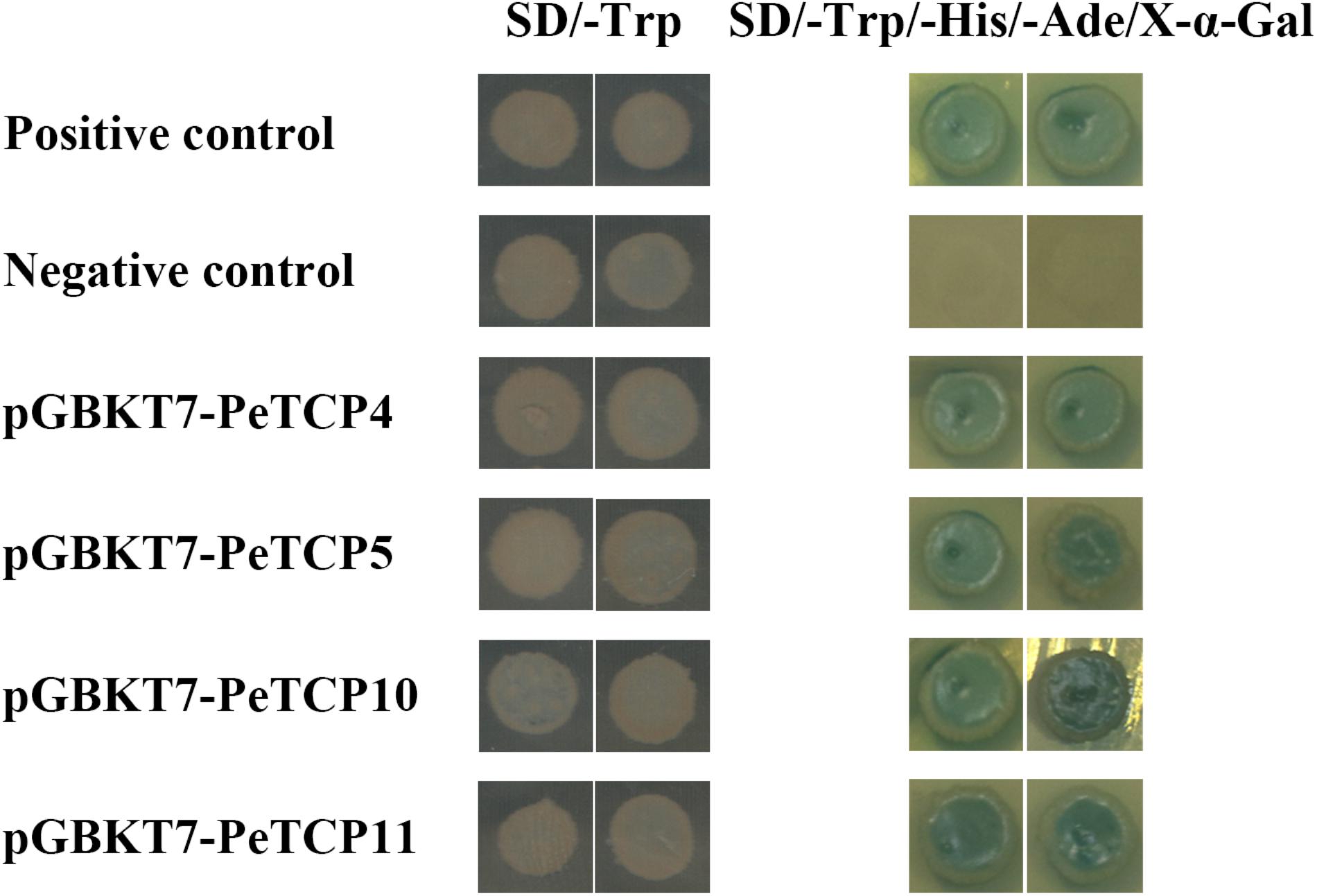
FIGURE 9. Transactivational analyses of PeTCP proteins in yeast Y2HGold strain. The positive constructs, negative constructs, and fusion constructs were transformed into yeast Y2HGold strain and successively incubated in SD/-Trp media and SD-His/-Ade/-Trp plate supplemented with X-α-GAL.
Expression Patterns After ABA, Me-JA, and SA Treatment
Plant hormones, especially ABA, Me-JA, and SA, have well-established roles in plant stress-signaling networks and developmental processes. Many corresponding responsive cis-elements have been found in the promoter regions of the PeTCPs. Hence, the qRT-PCR test was used to investigate the potential functions of PeTCPs in response to ABA, Me-JA, and SA (Figure 10). After the ABA treatment, the transcriptional levels of nine PeTCPs increased to significantly high levels, three (PeTCP8, 10, and 15) of which were at their highest levels at 12 h and then showed downward trends at 24 h. The remaining members having high transcriptional levels (PeTCP2, 7, 12–14, and 16) showed a downward trend during the early treatment and increased at 24 h. Seven PeTCPs exhibited low expression levels during treatment, especially at the early post-treatment stage. After the SA treatment, seven TCPs (PeTCP1–3, 5, 7–8, and 12) were upregulated and compared with control groups, especially PeTCP1 at 3 h, with expression levels more than 150-fold higher than those of the control group. The remaining PeTCP members showed varying degrees of downregulation after the SA treatment. In addition, for the Me-JA treatment, PeTCP members also had different expression levels. PeTCP1–2, 7–11, 15, and 16 showed an upregulation trend or maintained high expression levels at different time points. In contrast, the expression levels of PeTCP3–6 and PeTCP12–14 were lower than in the control groups.
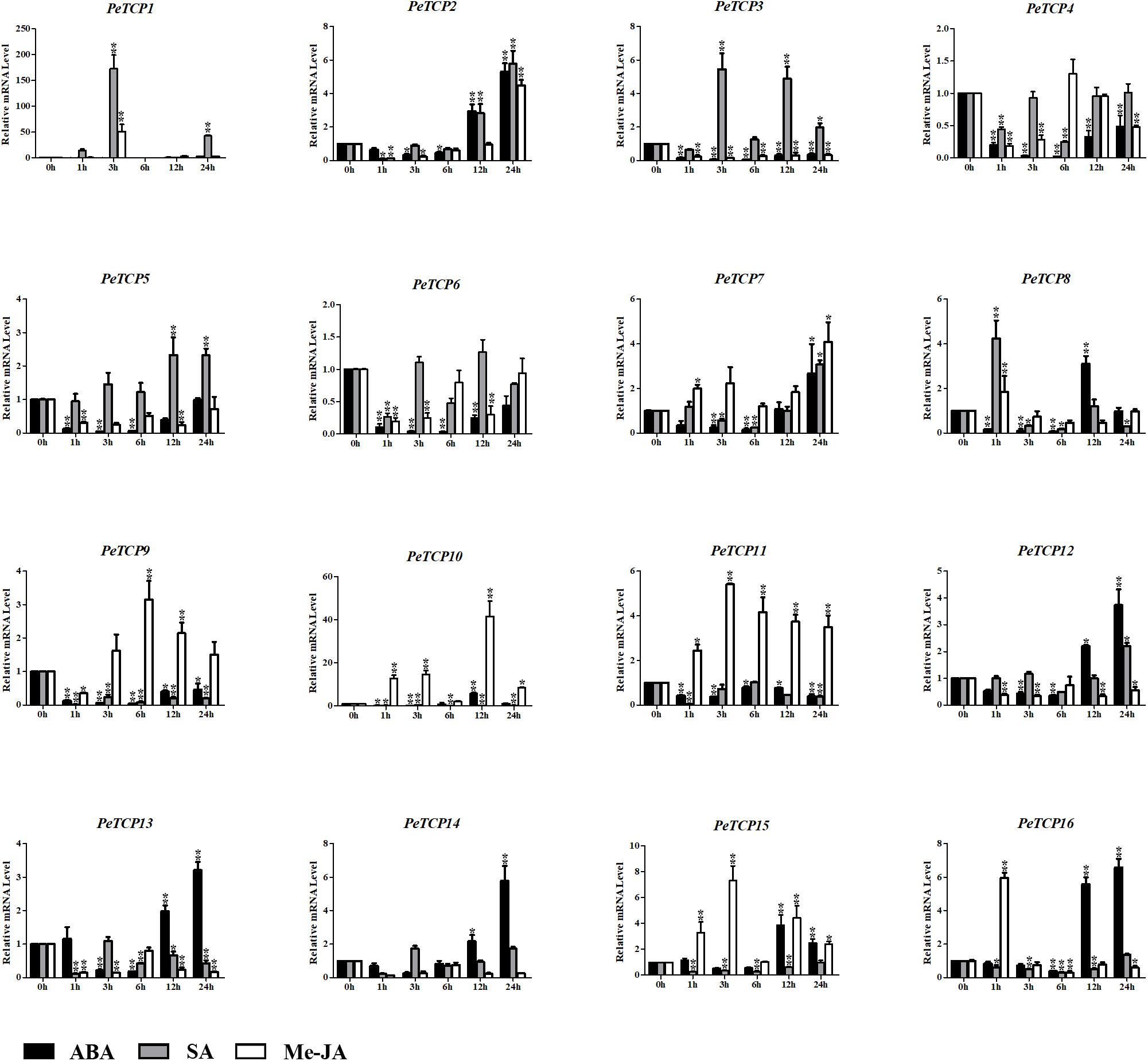
FIGURE 10. Expression levels of PeTCPs under ABA, Me-JA, and SA treatment by qRT-PCR. The Y-axis and X-axis indicate the relative expression levels and the time courses of plant hormone treatments, respectively. Error bars, 6 ± SE. Asterisks indicate significant difference compared to the transcription level of control groups, as determined by Student’s t-test (∗p < 0.05, ∗∗p < 0.01).
Discussion
TCP TFs in Moso Bamboo
The plant-specific TCP TFs with versatile functions in various plant growth and development processes have been functionally analyzed in the model plant species Arabidopsis thaliana and rice. Additionally, they have been genome-wide identified in other higher plants, including in dicotyledons such as tea plant (Wu Z.J. et al., 2017), apple (Xu et al., 2014), Gossypium raimondii (Ma et al., 2013), watermelon (Shi et al., 2016), Prunus mume (Zhou et al., 2016), strawberry (Wei et al., 2016), Chrysanthemum morifolium (Wang et al., 2017), and poplar (Ma et al., 2016), and in monocotyledons such as maize (Chai et al., 2017) and Sorghum (Francis et al., 2016). Here, 16 TCPTFs were identified in the moso bamboo genome (Table 1), and a series of bioinformatics analyses were performed to explore their potential structural and functional characteristics. Compared with other species, moso bamboo contains less TCP members, even though it has the largest genome size (2.075 Gb) (Peng et al., 2013), which may signify that gene loss events occurred over the course of evolution.
The sequence alignment of 16 TCP proteins showed that a non-canonical basic helix–loop–helix motif in the N-terminus is present in the TCP members (Figure 2). These members can be divided into two classes based on the presence of four amino acids in the basic motif (Cubas et al., 1999). Class I contains 16 residues, while Class II contains 20 residues. Additionally, two extremely uncharged hydrophilic amino acids, threonine, and glycine, exist in all members of Class II. An arginine-rich R domain outside the conserved TCP domain, with the predicted function of facilitating protein–protein interactions, exists in several class II TCP members (Cubas et al., 1999). For instance, four genes (OsTCP6, 7, 13, and 14) in rice, four (AtTCP2, 12, 18, and 24) in Arabidopsis thaliana, maize TB1, and four (FvTCP3, 6, 9, and 14) in Fragaria vesca (Wei et al., 2016) contain an arginine-rich R domain according to previous reports. Similarly, PeTCP13, the TB1-like gene in moso bamboo, was identified as having an R domain. Thus, the genes containing an R domain may play similar roles in plant growth and development.
However, an intrinsically disordered region exists in the C-terminus of AtTCP8 and has been identified as a transactivation domain (Valsecchi et al., 2013). Furthermore, similar to reports in Arabidopsis, TCP proteins of moso bamboo are also rich in disorder-promoting residues. The transactivation activity experiments with PeTCP4, 5, 10, and 11 revealed that these four PeTCPs are transcriptional genes in yeast (Figure 9).
The TCP domain is a plant-specific DNA-binding domain (Kosugi and Ohashi, 2002), and the members containing this domain regulate the expression levels of other proteins by binding to their promoters. For instance, PCF2, which encodes a rice TCP protein, was identified as a DNA-binding protein that recognizes the PCNA promoter (Kosugi and Ohashi, 1997). It also acts as a transcriptional activator of OsNHX1 in salt tolerance by binding to the OsNHX1 promoter (Almeida et al., 2016). PeTCP10, a PCF2-like gene, may have a similar binding site and regulatory mechanism as that found in rice.
As DNA-binding proteins, the TCP factors are expected to be targeted to the nuclei. Nuclear localization signals are present in many of them, and nuclear localization has been confirmed for several TCP members by the immunoprecipitation of nuclear extracts or GFP-protein fusions. The four members examined in this study were located in the nucleus (Figure 8), consistent with earlier reports (Wei et al., 2016). In contrast, some TCP members were not localized in the nucleus. For example, FvTCP17 is confirmed to localize in the nucleus and cytoplasm as assessed by the transformation of GFP-protein fusions into Arabidopsis thaliana mesophyll protoplasts (Wei et al., 2016).
In addition, the exon/intron and conserved motif distribution patterns of PeTCP paralogous genes, such as PeTCP1/3, 6/11, and 7/16, were very similar. These similarities between paralogous pair members might signify their similar functions during moso bamboo growth and development.
The Potential Functions of TCP TFs in Stress-Related Plant Hormone Transduction in Moso Bamboo
Abscisic acid is produced in both dehydrated vegetative tissues under water deficit conditions and maturing seeds, and it regulates the expression levels of many genes that may function in dehydration tolerance (Yamaguchishinozaki and Shinozaki, 2005). Relationships between the TCPs and ABA have been reported. An earlier report (Mukhopadhyay and Tyagi, 2015) showed that OsTCP19 which is induced by salt, drought, and cold stresses can improve ABA signal transduction by promoting the expression of ABA INSENSITIVE4 and interacting directly with the encoded protein. In Arabidopsis thaliana, TCP14 interacts with the DNA BINDING WITH ONE FINGER 6 TF to inhibit the activation of the ABA biosynthetic gene ABA DEFICIENT1 and other ABA-related stress genes to promote the germination of Arabidopsis seeds (Tatematsu et al., 2008; Ruedaromero et al., 2012). ABA-inducible genes contain a conserved ABRE, which functions in the ABA-dependent gene expression induced by osmotic and cold stresses (Yamaguchishinozaki and Shinozaki, 2005). ABRE is a major cis-acting element in ABA-responsive gene expression. It exists in the promoter regions of several PeTCPs, according to cis-element prediction results (Figure 5). Additionally, the fluctuations in PeTCP gene expression levels were induced after the ABA treatment (Figure 10), indicating that these genes may be involved in the ABA signal transduction pathway in moso bamboo through a mechanism similar to that of rice or Arabidopsis thaliana.
Meanwhile, TCPs interact with the genes that are involved in the biosynthesis of JA and other oxylipins, which affect development, abiotic stress responses, and plant–microbe interactions. In this pathway, LOX2, the best-characterized TCP-controlled gene, encodes a chloroplast enzyme involved in JA synthesis from α-linolenic acid (Vick and Zimmerman, 1983). Its expression is controlled by Class I and Class II TCPs, especially TCP4, through an antagonistic mode of action (Schommer et al., 2008). The inactivation of TCP4 results in LOX2 downregulation, which reduces JA synthesis and increases plant susceptibility to stress (Sugio et al., 2014). Similarly, the expression patterns of TCPs in moso bamboo were diverse after the Me-JA treatment. Additionally, PeTCP2, the TCP4-like gene, maintains a higher expression level after the Me-JA treatment (Figure 10). Thus, the JA regulatory mechanism may be conserved in the TCPs in moso bamboo.
In addition to their functions involving ABA and JA, TCPs also play crucial roles in stimulating the synthesis of, and response to, SA. In Arabidopsis thaliana, several TCPs interact with the SA biosynthetic enzyme ISOCHORISMATE SYNTHASE 1 gene, and the gene’s expression is enhanced by the TCPs, such as TCP8, 9, and 20, binding to the TCP-binding motif in its promoter region (Xiaoyan et al., 2015). There are many SA-related cis-elements in the promoter regions of PeTCPs (Figure 5), and the expression levels of several PeTCP genes significantly increased after the SA treatment (Figure 10), indicating that PeTCPs may have roles in the signal transduction of SA, as reported.
Interestingly, the expression of some genes by SA, Me-JA, and ABA are different from that predicted (Figure 10). For example, expression induction of PeTCP2 has been induced by ABA treatment although ABA-related cis-elements are not predicted in the promoter region of PeTCP2; expression induction of TCP 10 has been induced by Me-JA treatment although Me-JA-related cis-elements are not predicted in the promoter region of PeTCP10. On the other hand, expression alteration of PeTCP10 was not observed by ABA treatment although ABA-related cis-elements were detected. Meanwhile, this is observed in TCPs of strawberry (Wei et al., 2016). For example, expression induction of PvTCP3, -5, -12, -13, -16, and -19 have been induced by ABA treatment although ABA-related cis-elements are not predicted in their promoter region. These results may show that expression induction of genes are complex biological processes.
Conclusion
In this study, we investigated the phylogenetics, multiple sequence alignment, gene structures, conserved motifs, cis-acting elements, and divergence time analysis of the 16 predicted TCP TFs in the moso bamboo genome. We used qRT-PCR to explore the expression patterns of the 16 TCP genes in different tissues and developmental stages, as well as after three – ABA, Me-JA, and SA – plant hormone treatments. Additionally, the subcellular localization and transcription activity analysis of four selected TCP members were investigated in moso bamboo. The results of this study increase the understanding of TCP functions in diverse aspects of plant growth and development.
Author Contributions
H-LL designed the experiments, carried out the main bioinformatics analyses, and drafted the manuscript. MW and Y-MG participated in the design of the study. FL helped to draft the manuscript. FC implemented the software and helped to draft the manuscript. YX participated in its design and coordination and helped to draft the manuscript. All authors read and approved the final manuscript.
Funding
The present study was financially supported by grants from the National Natural Science Foundation of China (Grant No. 31670672), the National Science and Technology Support Planning Subjects (2015BAD04B03), and the Science and Technology Major Project of Anhui Province (15czz03119).
Conflict of Interest Statement
The authors declare that the research was conducted in the absence of any commercial or financial relationships that could be construed as a potential conflict of interest.
Acknowledgments
We thank Lesley Benyon, Ph.D., from Liwen Bianji, Edanz Group China (www.liwenbianji.cn/ac), for editing the English text of a draft of this manuscript.
Supplementary Material
The Supplementary Material for this article can be found online at: https://www.frontiersin.org/articles/10.3389/fpls.2018.01263/full#supplementary-material
Footnotes
- ^https://trace.ncbi.nlm.nih.gov/Traces/sra/?study=ERP001341
- ^http://pfam.sanger.ac.uk/search
- ^http://rice.plantbiology.msu.edu/
- ^http://www.biomart.org/
- ^http://www.arabidopsis.org/
- ^https://phytozome.jgi.doe.gov/pz/portal.html
- ^http://meme.sdsc.edu/meme/intro.html
- ^http://www.physics.csbsju.edu/stats/
- ^http://bioinformatics.psb.ugent.be/webtools/plantcare/html/
References
Aguilar-Martínez, J. A., Poza-Carrión, C., and Cubas, P. (2007). Arabidopsis BRANCHED1 acts as an integrator of branching signals within axillary buds. Plant Cell 19, 458–472. doi: 10.1105/tpc.106.048934
Almeida, D. M., Gregorio, G. B., Oliveira, M. M., and Saibo, N. J. M. (2016). Five novel transcription factors as potential regulators of OsNHX1 gene expression in a salt tolerant rice genotype. Plant Mol. Biol. 93, 61–77. doi: 10.1007/s11103-016-0547-7
Biłas, R., Szafran, K., Hnatuszko-Konka, K., and Kononowicz, A. K. (2016). Cis-regulatory elements used to control gene expression in plants. Plant Cell Tissue Organ Cult. 127, 1–19. doi: 10.1007/s11240-016-1057-7
Broholm, S. K., Tähtiharju, S., Laitinen, R. A. E., Albert, V. A., Teeri, T. H., and Elomaa, P. (2008). A TCP domain transcription factor controls flower type specification along the radial axis of the Gerbera (Asteraceae) inflorescence. Proc. Natl. Acad. Sci. U.S.A. 105, 9117–9122. doi: 10.1073/pnas.0801359105
Cai, R., Dai, W., Zhang, C., Wang, Y., Wu, M., Zhao, Y., et al. (2017). The maize WRKY transcription factor ZmWRKY17 negatively regulates salt stress tolerance in transgenic Arabidopsis plants. Planta 246, 1215–1231. doi: 10.1007/s00425-017-2766-9
Chai, W., Jiang, P., Huang, G., Jiang, H., and Li, X. (2017). Identification and expression profiling analysis of TCP family genes involved in growth and development in maize. Physiol. Mol. Biol. Plants 23, 779–791. doi: 10.1007/s12298-017-0476-1
Chen, D., Chen, Z., Wu, M., Wang, Y., Wang, Y., Yan, H., et al. (2017). Genome-wide identification and expression analysis of the HD-zip gene family in moso bamboo (Phyllostachys edulis). J. Plant Growth Regul. 36, 323–337. doi: 10.1038/srep24520
Cubas, P. (2002). “Role of TCP genes in the evolution of key morphological characters in Angiosperms,” in Developmental Genetics and Plant Evolution, eds Q. C. B. Cronk, R. M. Bateman, and J. A. Hawkins (London: Taylor and Francis), 247–266.
Cubas, P., Lauter, N., Doebley, J., and Coen, E. (1999). The TCP domain: a motif found in proteins regulating plant growth and development. Plant J. 18, 215–222. doi: 10.1046/j.1365-313X.1999.00444.x
Cui, M. H., Yoo, K. S., Hyoung, S., Nguyen, H. T., Kim, Y. Y., Kim, H. J., et al. (2013). An Arabidopsis R2R3-MYB transcription factor, AtMYB20, negatively regulates type 2C serine/threonine protein phosphatases to enhance salt tolerance. FEBS Lett. 587, 1773–1778. doi: 10.1016/j.febslet.2013.04.028
Danisman, S. (2016). TCP transcription factors at the interface between environmental challenges and the plant’s growth responses. Front. Plant Sci. 7:1930. doi: 10.3389/fpls.2016.01930
Dhaka, N., Bhardwaj, V., Sharma, M. K., and Sharma, R. (2017). Evolving tale of TCPs: new paradigms and old lacunae. Front. Plant Sci. 8:479. doi: 10.3389/fpls.2017.00479
Doebley, J., Stec, A., and Hubbard, L. (1997). The evolution of apical dominance in maize. Nature 386, 485–488. doi: 10.1038/386485a0
Fan, C., Ma, J., Guo, Q., Li, X., Hui, W., and Lu, M. (2013). Selection of reference genes for quantitative real-time pcr in bamboo (Phyllostachys edulis). PLoS One 8:e56573. doi: 10.1371/journal.pone.0056573
Finlayson, S. A. (2007). Arabidopsis teosinte branched1-like 1 regulates axillary bud outgrowth and is homologous to monocot teosinte branched1. Plant Cell Physiol. 48, 667–677. doi: 10.1093/pcp/pcm044
Finn, R. D., Mistry, J., Schusterböckler, B., Griffithsjones, S., Hollich, V., Lassmann, T., et al. (2006). Pfam: clans, web tools and services. Nucleic Acids Res. 34, D247–D251. doi: 10.1093/nar/gkj149
Finn, R. D., Mistry, J., Tate, J., Coggill, P., Heger, A., Pollington, J. E., et al. (2010). The Pfam protein families database. Nucleic Acids Res. 38, D211–D222. doi: 10.1093/nar/gkp985
Francis, A., Dhaka, N., Bakshi, M., Jung, K. H., Sharma, M. K., and Sharma, R. (2016). Comparative phylogenomic analysis provides insights into TCP gene functions in sorghum. Sci. Rep. 6:38488. doi: 10.1038/srep45801
Giraud, E., Ng, S., Carrie, C., Duncan, O., Low, J., Lee, C. P., et al. (2010). TCP transcription factors link the regulation of genes encoding mitochondrial proteins with the circadian clock in Arabidopsis thaliana. Plant Cell 22, 3921–3934. doi: 10.1105/tpc.110.074518
Goldsbrough, A. P., Albrecht, H., and Stratford, R. (1993). Salicylic acid-inducible binding of a tobacco nuclear protein to a 10 bp sequence which is highly conserved amongst stress-inducible genes. Plant J. Cell Mol. Biol. 3, 563–571. doi: 10.1046/j.1365-313X.1993.03040563.x
Guan, P., Ripoll, J. J., Wang, R., Vuong, L., Bailey-Steinitz, L. J., Ye, D., et al. (2017). Interacting TCP and NLP transcription factors control plant responses to nitrate availability. Proc. Natl. Acad. Sci. U.S.A. 114, 2419–2424. doi: 10.1073/pnas.1615676114
Gui, Y. J., Zhou, Y., Wang, Y., Wang, S., Wang, S. Y., Hu, Y., et al. (2010). Insights into the bamboo genome: syntenic relationships to rice and sorghum. Chin. Bull. Bot. 52, 1008–1015. doi: 10.1111/j.1744-7909.2010.00965.x
Hao, J., Tu, L., Hu, H., Tan, J., Deng, F., Tang, W., et al. (2012). GbTCP, a cotton TCP transcription factor, confers fibre elongation and root hair development by a complex regulating system. J. Exp. Bot. 63, 6267–6281. doi: 10.1093/jxb/ers278
He, H., Dong, Q., Shao, Y., Jiang, H., Zhu, S., Cheng, B., et al. (2012). Genome-wide survey and characterization of the WRKY gene family in Populus trichocarpa. Plant Cell Rep. 31, 1199–1217. doi: 10.1007/s00299-012-1241-0
Hu, Z., Yamauchi, T., Yang, J., Jikumaru, Y., Tsuchida-Mayama, T., Ichikawa, H., et al. (2014). Strigolactone and cytokinin act antagonistically in regulating rice mesocotyl elongation in darkness. Plant Cell Physiol. 55, 30–41. doi: 10.1093/pcp/pct150
Hubbard, L., Mcsteen, P., Doebley, J., and Hake, S. (2002). Expression patterns and mutant phenotype of teosinte branched1 correlate with growth suppression in maize and teosinte. Genetics 162, 1927–1935.
Kebrom, T. H., Burson, B. L., and Finlayson, S. A. (2006). Phytochrome B represses teosinte branched1 expression and induces sorghum axillary bud outgrowth in response to light signals. Plant Physiol. 140, 1109–1117. doi: 10.1104/pp.105.074856
Kosugi, S., and Ohashi, Y. (1997). PCF1 and PCF2 specifically bind to cis-elements in the rice proliferating cell nuclear antigen gene. Plant Cell 9, 1607–1619. doi: 10.1105/tpc.9.9.1607
Kosugi, S., and Ohashi, Y. (2002). DNA binding and dimerization specificity and potential targets for the TCP protein family. Plant J. 30, 337–348. doi: 10.1046/j.1365-313X.2002.01294.x
Larkin, M. A., Blackshields, G., Brown, N. P., Chenna, R., Mcgettigan, P. A., Mcwilliam, H., et al. (2007). Clustal W and Clustal X version 2.0. Bioinformatics 23, 2947–2948. doi: 10.1093/bioinformatics/btm404
Lata, C., Mishra, A. K., Muthamilarasan, M., Bonthala, V. S., Khan, Y., and Prasad, M. (2014). Genome-wide investigation and expression profiling of AP2/ERF transcription factor superfamily in foxtail millet (Setaria italica L.). PLoS One 9:e113092. doi: 10.1371/journal.pone.0113092
Lescot, M., Déhais, P., Thijs, G., Marchal, K., Moreau, Y., Van, D. P. Y., et al. (2002). PlantCARE, a database of plant cis-acting regulatory elements and a portal to tools for in silico analysis of promoter sequences. Nucleic Acids Res. 30, 325–327. doi: 10.1093/nar/30.1.325
Li, L., Mu, S., Cheng, Z., Cheng, Y., Zhang, Y., Miao, Y., et al. (2017). Characterization and expression analysis of the WRKY gene family in moso bamboo. Sci. Rep. 7:6675. doi: 10.1038/s41598-017-06701-2
Librado, P., and Rozas, J. (2009). DnaSP v5: a software for comprehensive analysis of DNA polymorphism data. Bioinformatics 25, 1451–1452. doi: 10.1093/bioinformatics/btp187
Luo, D., Carpenter, R., Copsey, L., Vincent, C., Clark, J., and Coen, E. (1999). Control of organ asymmetry in flowers of antirrhinum. Cell 99, 367–376. doi: 10.1016/S0092-8674(00)81523-8
Ma, J., Wang, Q., Sun, R., Xie, F., Jones, D. C., and Zhang, B. (2013). Genome-wide identification and expression analysis of TCP transcription factors in Gossypium raimondii. Sci. Rep. 4:6645. doi: 10.1038/srep06645
Ma, X., Ma, J., Di, F., Li, C., Jiang, Y., and Luo, K. (2016). Genome-wide identification of TCP family transcription factors from Populus euphratica and their involvement in leaf shape regulation. Sci. Rep. 6:32795. doi: 10.1038/srep32795
Martíntrillo, M., and Cubas, P. (2010). TCP genes: a family snapshot ten years later. Trends Plant Sci. 15, 31–39. doi: 10.1016/j.tplants.2009.11.003
Mondragónpalomino, M., and Trontin, C. (2011). High time for a roll call: gene duplication and phylogenetic relationships of TCP-like genes in monocots. Ann. Bot. 107, 1533–1544. doi: 10.1093/aob/mcr059
Mukhopadhyay, P., and Tyagi, A. K. (2015). OsTCP19 influences developmental and abiotic stress signaling by modulating ABI4-mediated pathways. Sci. Rep. 5:9998. doi: 10.1038/srep09998
Parapunova, V., Busscher, M., Busscherlange, J., Lammers, M., Karlova, R., Bovy, A. G., et al. (2014). Identification, cloning and characterization of the tomato TCP transcription factor family. BMC Plant Biol. 14:157. doi: 10.1186/1471-2229-14-157
Peng, Y., Chen, L., Lu, Y., Wu, Y., Dumenil, J., Zhu, Z., et al. (2015). The ubiquitin receptors DA1, DAR1, and DAR2 redundantly regulate endoreduplication by modulating the stability of TCP14/15 in Arabidopsis. Plant Cell 27, 649–662. doi: 10.1105/tpc.114.132274
Peng, Z., Lu, T., Li, L., Liu, X., Gao, Z., Hu, T., et al. (2010). Genome-wide characterization of the biggest grass, bamboo, based on 10,608 putative full-length cDNA sequences. BMC Plant Biol. 10:116. doi: 10.1186/1471-2229-10-116
Peng, Z., Lu, Y., Li, L., Zhao, Q., Feng, Q., Gao, Z., et al. (2013). The draft genome of the fast-growing non-timber forest species moso bamboo (Phyllostachys heterocycla). Nat. Genet. 45, 456–461. doi: 10.1038/ng.2569
Ruedaromero, P., Barrerosicilia, C., Gómezcadenas, A., Carbonero, P., and Oñatesánchez, L. (2012). Arabidopsis thaliana DOF6 negatively affects germination in non-after-ripened seeds and interacts with TCP14. J. Exp. Bot. 63, 1937–1949. doi: 10.1093/jxb/err388
Schommer, C., Palatnik, J. F., Aggarwal, P., Chételat, A., Cubas, P., Farmer, E. E., et al. (2008). Control of jasmonate biosynthesis and senescence by mir319 targets. PLoS Biol. 6:e230. doi: 10.1371/journal.pbio.0060230
Shen, Q., and Ho, T. H. (1995). Functional dissection of an abscisic acid (ABA)-inducible gene reveals two independent ABA-responsive complexes each containing a G-box and a novel cis-acting element. Plant Cell 7, 295–307. doi: 10.1105/tpc.7.3.295
Sheshadri, S. A., Nishanth, M. J., and Simon, B. (2016). Stress-mediated cis-element transcription factor interactions interconnecting primary and specialized metabolism in planta. Front. Plant Sci. 7:1725. doi: 10.3389/fpls.2016.01725
Shi, P., Guy, K. M., Wu, W., Fang, B., Yang, J., Zhang, M., et al. (2016). Genome-wide identification and expression analysis of the ClTCP transcription factors in Citrullus lanatus. BMC Plant Biol. 16:85. doi: 10.1186/s12870-016-0765-9
Sugio, A., Maclean, A. M., and Hogenhout, S. A. (2014). The small phytoplasma virulence effector SAP11 contains distinct domains required for nuclear targeting and CIN-TCP binding and destabilization. New Phytol. 202, 838–848. doi: 10.1111/nph.12721
Sungkaew, S., Stapleton, C. M. A., Salamin, N., and Hodkinson, T. R. (2009). Non-monophyly of the woody bamboos (Bambuseae; Poaceae): a multi-gene region phylogenetic analysis of Bambusoideae s.s. J. Plant Res. 122, 95–108. doi: 10.1007/s10265-008-0192-6
Takeda, T., Suwa, Y., Suzuki, M., Kitano, H., Ueguchi-Tanaka, M., Ashikari, M., et al. (2003). The OsTB1 gene negatively regulates lateral branching in rice. Plant J. Cell Mol. Biol. 33, 513–520. doi: 10.1046/j.1365-313X.2003.01648.x
Tamura, K., Stecher, G., Peterson, D., Filipski, A., and Kumar, S. (2013). MEGA6: molecular evolutionary genetics analysis version 6.0. Mol. Biol. Evol. 30, 2725–2729. doi: 10.1093/molbev/mst197
Tatematsu, K., Nakabayashi, K., Kamiya, Y., and Nambara, E. (2008). Transcription factor AtTCP14 regulates embryonic growth potential during seed germination in Arabidopsis thaliana. Plant J. 53, 42–52. doi: 10.1111/j.1365-313X.2007.03308.x
Valsecchi, I., Guittard-Crilat, E., Maldiney, R., Habricot, Y., Lignon, S., Lebrun, R., et al. (2013). The intrinsically disordered C-terminal region of Arabidopsis thaliana TCP8 transcription factor acts both as a transactivation and self-assembly domain. Mol. Biosyst. 9, 2282–2295. doi: 10.1039/c3mb70128j
Vaughn, J. N., Ellingson, S. R., Mignone, F., and Arnim, A. V. (2012). Known and novel post-transcriptional regulatory sequences are conserved across plant families. RNA 18, 368–384. doi: 10.1261/rna.031179.111
Vick, B. A., and Zimmerman, D. C. (1983). The biosynthesis of jasmonic acid: a physiological role for plant lipoxygenase. Biochem. Biophys. Res. Commun. 111, 470–477. doi: 10.1016/0006-291X(83)90330-3
Wang, J., Wang, H., Ding, L., Song, A., Shen, F., Jiang, J., et al. (2017). Transcriptomic and hormone analyses reveal mechanisms underlying petal elongation in Chrysanthemum morifolium ‘Jinba’. Plant Mol. Biol. 93, 593–606. doi: 10.1007/s11103-017-0584-x
Wang, S. T., Sun, X. L., Hoshino, Y., Yu, Y., Jia, B., Sun, Z. W., et al. (2014). MicroRNA319 positively regulates cold tolerance by targeting OsPCF6 and OsTCP21 in rice (Oryza sativa L.). PLoS One 9:e91357. doi: 10.1371/journal.pone.0091357
Wang, Z., Luo, Y., Li, X., Wang, L., Xu, S., Yang, J., et al. (2008). Genetic control of floral zygomorphy in pea (Pisum sativum L.). Proc. Natl. Acad. Sci. U.S.A. 105, 10414–10419. doi: 10.1073/pnas.0803291105
Wei, W., Hu, Y., Cui, M. Y., Han, Y. T., Gao, K., and Feng, J. Y. (2016). Identification and transcript analysis of the TCP transcription factors in the diploid woodland strawberry Fragaria vesca. Front. Plant Sci. 7:1937. doi: 10.3389/fpls.2016.01937
Wu, H., Lv, H., Li, L., Liu, J., Mu, S., Li, X., et al. (2015). Genome-wide analysis of the AP2/ERF transcription factors family and the expression patterns of DREB genes in moso bamboo (Phyllostachys edulis). PLoS One 10:e0126657. doi: 10.1371/journal.pone.0126657
Wu, M., Liu, H., Han, G., Cai, R., Pan, F., and Xiang, Y. (2017). A moso bamboo WRKY gene PeWRKY83 confers salinity tolerance in transgenic Arabidopsis plants. Sci. Rep. 7:11721. doi: 10.1038/s41598-017-10795-z
Wu, Z. J., Wang, W. L., and Zhuang, J. (2017). TCP family genes control leaf development and its responses to hormonal stimuli in tea plant [Camellia sinensis (L.) O. Kuntze]. Plant Growth Regul. 83, 43–53. doi: 10.1007/s10725-017-0282-3
Xiaoyan, W., Jiong, G., Zheng, Z., Xianxin, D., Xiaolei, W., Guodong, R., et al. (2015). TCP transcription factors are critical for the coordinated regulation of isochorismate synthase 1 expression in Arabidopsis thaliana. Plant J. Cell Mol. Biol. 82, 151–162. doi: 10.1111/tpj.12803
Xu, R., Sun, P., Jia, F., Lu, L., Li, Y., Zhang, S., et al. (2014). Genomewide analysis of TCP transcription factor gene family in Malus domestica. J. Genet. 93, 733–746. doi: 10.1007/s12041-014-0446-0
Yamaguchishinozaki, K., and Shinozaki, K. (2005). Organization of cis-acting regulatory elements in osmotic- and cold-stress-responsive promoters. Trends Plant Sci. 10, 88–94. doi: 10.1016/j.tplants.2004.12.012
Yan, H., Jia, H., Chen, X., Hao, L., An, H., and Guo, X. (2014). The cotton WRKY transcription factor GhWRKY17 functions in drought and salt stress in transgenic Nicotiana benthamiana through ABA signaling and the modulation of reactive oxygen species production. Plant Cell Physiol. 55, 2060–2076. doi: 10.1093/pcp/pcu133
Yao, X., Ma, H., Wang, J., and Zhang, D. (2007). Genome-wide comparative analysis and expression pattern of TCP gene families in Arabidopsis thaliana and Oryza sativa. J. Integr. Plant Biol. 49, 885–897. doi: 10.1111/j.1672-9072.2007.00509.x
Zhang, Y. J., Ma, P. F., and Li, D. Z. (2011). High-throughput sequencing of six bamboo chloroplast genomes: phylogenetic implications for temperate woody bamboos (Poaceae: Bambusoideae). PLoS One 6:e20596. doi: 10.1371/journal.pone.0020596
Zhang, Z., Liu, X., Wang, X., Zhou, M., Zhou, X., Ye, X., et al. (2012). An R2R3 MYB transcription factor in wheat, TaPIMP1, mediates host resistance to Bipolaris sorokiniana and drought stresses through regulation of defense- and stress-related genes. New Phytol. 196, 1155–1170. doi: 10.1111/j.1469-8137.2012.04353.x
Zhao, Y., Ma, Q., Jin, X., Peng, X., Liu, J., Deng, L., et al. (2014). A novel maize homeodomain-leucine zipper (HD-Zip) I gene, Zmhdz10, positively regulates drought and salt tolerance in both rice and Arabidopsis. Plant Cell Physiol. 55, 1142–1156. doi: 10.1093/pcp/pcu054
Zhao, Y., Zhou, Y., Jiang, H., Li, X., Gan, D., Peng, X., et al. (2011). Systematic analysis of sequences and expression patterns of drought-responsive members of the HD-Zip gene family in maize. PLoS One 6:e28488. doi: 10.1371/journal.pone.0028488
Keywords: moso bamboo (Phyllostachys edulis), TCP transcription factors, expression patterns, subcellular localization, transcription activity
Citation: Liu H-L, Wu M, Li F, Gao Y-M, Chen F and Xiang Y (2018) TCP Transcription Factors in Moso Bamboo (Phyllostachys edulis): Genome-Wide Identification and Expression Analysis. Front. Plant Sci. 9:1263. doi: 10.3389/fpls.2018.01263
Received: 06 January 2018; Accepted: 10 August 2018;
Published: 05 October 2018.
Edited by:
Juan Caballero, Universidad Autónoma de Querétaro, MexicoReviewed by:
Jin Chen, University of Kentucky, United StatesKeisuke Nagai, Nagoya University, Japan
Copyright © 2018 Liu, Wu, Li, Gao, Chen and Xiang. This is an open-access article distributed under the terms of the Creative Commons Attribution License (CC BY). The use, distribution or reproduction in other forums is permitted, provided the original author(s) and the copyright owner(s) are credited and that the original publication in this journal is cited, in accordance with accepted academic practice. No use, distribution or reproduction is permitted which does not comply with these terms.
*Correspondence: Yan Xiang, eGlhbmd5YW5haGF1QHNpbmEuY29t