- 1Key Laboratory of South China Agricultural Plant Molecular Analysis and Genetic Improvement, South China Botanical Garden, Chinese Academy of Sciences, Guangzhou, China
- 2College of Life Sciences, University of Chinese Academy of Sciences, Beijing, China
- 3Key Laboratory of Applied Botany, South China Botanical Garden, Chinese Academy of Sciences, Guangzhou, China
Dehydrin (DHN) genes can be rapidly induced to offset water deficit stresses in plants. Here, we reported on a dehydrin gene (IpDHN) related to salt tolerance isolated from Ipomoea pes-caprae L. (Convolvulaceae). The IpDHN protein shares a relatively high homology with Arabidopsis dehydrin ERD14 (At1g76180). IpDHN was shown to have a cytoplasmic localization pattern. Quantitative RT-PCR analyses indicated that IpDHN was differentially expressed in most organs of I. pes-caprae plants, and its expression level increased after salt, osmotic stress, oxidative stress, cold stress and ABA treatments. Analysis of the 974-bp promoter of IpDHN identified distinct cis-acting regulatory elements, including an MYB binding site (MBS), ABRE (ABA responding)-elements, Skn-1 motif, and TC-rich repeats. The induced expression of IpDHN in Escherichia coli indicated that IpDHN might be involved in salt, drought, osmotic, and oxidative stresses. We also generated transgenic Arabidopsis lines that over-expressed IpDHN. The transgenic Arabidopsis plants showed a significant enhancement in tolerance to salt/drought stresses, as well as less accumulation of hydrogen peroxide (H2O2) and the superoxide radical (O2−), accompanied by increasing activity of the antioxidant enzyme system in vivo. Under osmotic stresses, the overexpression of IpDHN in Arabidopsis can elevate the expression of ROS-related and stress-responsive genes and can improve the ROS-scavenging ability. Our results indicated that IpDHN is involved in cellular responses to salt and drought through a series of pleiotropic effects that are likely involved in ROS scavenging and therefore influence the physiological processes of microorganisms and plants exposed to many abiotic stresses.
Introduction
The halophyte Ipomoea pes-caprae L., belonging to the Convolvulaceae family, is mainly distributed in the littoral region of tropical and subtropical areas worldwide (Miryeganeh et al., 2014) and has attracted attention due to its sand fixation, wind resistance, landscape greening, and ecological restoration abilities in coral islands and coastal zones (Ouyang et al., 2011). Throughout their whole life cycle, halophytes are subjected to abiotic stresses, including extreme salt damage, drought or dehydration, osmotic stress, and nutritional imbalance, depending on their habitats (Kumari et al., 2015). Understanding how halophytes respond to salinity or other abiotic stresses will provide us with genetic resources and tactics to develop salt-resistant crop varieties and therefore lay the basis for further land improvements and solonchak agriculture (Xu et al., 2016). High soil salinity and water deficiency are the major constraints on the growth and yield of many plant species (Pierik and Testerink, 2014). Halophytes, as the best potential resource for salt stress-tolerance genes, have provided a favorable platform for the characterization of salt-responding genes that can be adopted for improving salinity tolerance in crop plants through genetic engineering (Mishra and Tanna, 2017).
Dehydrins (DHNs) belong to LEA proteins, a large family of highly hydrophilic proteins, and are classified into Group II LEA proteins (Graether and Boddington, 2014). In addition to accumulating during the seed maturation process, LEA proteins also increase responding to abiotic stresses that could cause cellular dehydration or water-deficit, such as freezing, high salinity, and drought (Hand et al., 2011). Typically, most DHNs have three types of conserved sequence motifs, i.e., K- (EKKGIMDKIKEKLPG), Y- ([T/V]D[E/Q]YGNP), and S- (serine-track) motifs, among which K- is the core segment and sets one or more repeats, forming amphiphilic α-helixes located at the C-terminal end of the proteins (Cuevas-Velazquez et al., 2014). Accordingly, DHNs fall into five subclasses, including Kn, KnS, YnKn, SKn, and YnSKn, mainly based on the replication of conserved K-, S-, and Y-segment motifs (Close, 1997). Further studies indicate that the K-segments are the functional core parts of DHNs that mediate cellular stress tolerance (Drira et al., 2013), bind to anionic phospholipid vesicles (Koag et al., 2009), or maintain enzyme activity (Yang et al., 2015).
Generally, DHNs are considered as stress proteins involved in multifunctional roles for plant protective reactions against dehydration by holding water molecules, binding transition metals to scavenge ROS, or binding to DNAs, proteins, sugars, or phospholipids to maintain biological activity (Liu et al., 2017). In addition, DHNs (or other LEA proteins) are known as IDPs and may serve as hub proteins and coordinate crosstalk with cellular signals and pathways involved in appropriate responses to stress conditions, or may be involved in the formation and stability of the cytoplasmic glassy state subjected to dehydration (Sun et al., 2013).
In recent years, numerous physiological studies have focused on the positive relationship between cellular accumulation of DHNs and increased plant tolerance to water-deficit stresses, including mainly cold, drought, and high salinity (Graether and Boddington, 2014). For example, an acidic SK3 DHN isolated from Opuntia streptacantha (Cactaceae) could lead to enhanced tolerance to freezing when overexpressed in Arabidopsis (Ochoa-Alfaro et al., 2012); wheat DHN’s (DHN-5) overexpression enhanced the salinity tolerance of transgenic Arabidopsis plants (Saibi et al., 2015); and Saussurea involucrata, a hardy dicotyledonous plant growing in alpine region, is capable of tolerating severe cold and arid conditions. Two DHN members, SiDHN1 and SiDHN2, can elevate the cold tolerance of the transgenic overexpression of tobacco and showed an induced expression pattern when challenging cold stress in S. involucrata (Qiu et al., 2014; Guo et al., 2017). Oleaster is a typical drought-enduring plant in the Mediterranean area, and an oleaster DHN gene, named OesDHN, can improve drought tolerance when overexpressed in Arabidopsis (Chiappetta et al., 2015). Prunus mume ‘Beijingyudie’ is also a hardy plant and can withstand extremely cold temperatures even as low as −19°C, and the overexpression of four P. mume dehydrins in Escherichia coli and tobacco resulted in increased freezing resistance (Bao et al., 2017). Two Hevea brasiliensis DHN genes, HbDHN1 and HbDHN2, could lead to a significant increase in the tolerance to salt, drought, and osmotic stresses when overexpressed in Arabidopsis (Cao et al., 2017). A DHN gene, CdDHN4, from bermudagrass (Cynodon dactylon L.), also showed an induced expression pattern when challenged by high/low temperatures, drought, salt and ABA (Lv et al., 2017). And also, SbDhn1 from Sorghum bicolor, encoding an YSK2-type DHN, showed obvious transcript accumulation when subjected to high temperature and osmotic stress, and overexpression in tobacco plants led to a protective effect under high temperature and osmotic stress treatments (Halder et al., 2017). The above series of reports indicate that plant DHNs might be extensively involved in abiotic stresses, mainly water deficit.
Although there are numerous studies on the biological functions of DHNs in various plant species, the functional characterization of salt-responsive genes of I. pes-caprae, a unique halophyte, requires further investigation: DHNs from this species involved in salt and drought tolerance have not been reported until now. In our previous research, we constructed a cDNA library of I. pes-caprae and obtained a series of possible candidate salt-tolerance genes using the FOX gene hunting system, including a DHN gene (IpSR38, IpDHN, GenBank accession no.: KX426069) (data not published). In the current report, we focused mainly on the further functional characterization of IpDHN involved in salt and drought tolerance, as well as in ROS scavenging.
Materials and Methods
Plant Materials, Growth Conditions, and Stress Treatments
Ipomoea pes-caprae seeds were collected from the seaside area of Zhuhai City (22°16′25.37″N, 113°34′18.00″E), Guangdong Province, China. Different parts of I. pes-caprae were frozen with liquid nitrogen and stored in ultra-cold storage freezers (−80°C) until used for RNA isolation. For culturing the seedlings of I. pes-caprae, the seeds were sterilized with 70% ethanol followed by breaking of the seed coat with emery paper prior to placement on MS basal salts distributed on plates with sand and soil and placed outdoors from April to November in Guangzhou City. The seedlings were used for stress treatment assays to check the expression patterns of IpDHN. Subsequently, salt (300 mM NaCl), simulated drought or dehydration (300 mM mannitol), oxidative (0.1 mM methylviologen), cold (0°C) stresses, and ABA treatment (0.1 mM) were applied to the I. pes-caprae seedlings to detect the expression pattern of IpDHN. Experiments were performed following a completely randomized design with three replications and were repeated three times.
Arabidopsis thaliana (ecotype: Col-0) plants used for transgenic over-expression assay were grown on solid MS medium for approximately 10 days, and then transferred into soil. All plants were incubated in a growth green house with constant temperature (22°C) and relative humidity (70%) under a photoperiod of 16 h light/8 h darkness.
Isolation of Full-Length IpDHN CDNA
A full-length cDNA library of I. pes-caprae was constructed and screened with a yeast salt-sensitive mutant (AXT3) (Zhou et al., 2015) complementary approach (data not published). Then, a full-length cDNA encoding DHN (IpDHN) that could rescue the phenotype of AXT3 was selected, and further investigations were performed.
Sequence Analysis of the IpDHN
The CDS of IpDHN cDNA sequence was translated with online ORFfinder translator tool1. The S- and K-segments of IpDHN were characterized using the ExPASy prosite server2. The 3D prediction of IpDHN was also conducted with the online program3 PHYRE2 . MEGA6 was used to perform protein homology comparison and phylogenetic reconstruction by the neighbor-joining (NJ) method (Tamura et al., 2013). Bootstrap values were set with 1000 replicates to assess the relative support for each branch.
IpDHN protein (NCBI accession no.: AQZ36519.1) was aligned with known DHNs using ClustalW software4. The amino acid sequences of different DHNs were as follows: Daucus carota (DcDHN; NCBI accession no.: BAA82445.1), Oryza sativa (Os02g44870.1; NCBI accession no.: XP015627209.1), Vitis vinifera (VvDHN; NCBI accession no.: XP_002285919.1), Solanum tuberosum (StDHN; NCBI accession no.: AAB53203.1), Capsicum annuum (CaDHN; NCBI accession no.: NP_001311855.1), Musa ABB Group (MusaDHN-1; NCBI accession no.: AEI54683.1), Coffea canephora (CcDHN; NCBI accession no.: ABC68275.1), Ipomoea nil (InDHN; NCBI accession no.: XP_019187872.1), and 10 Arabidopsis dehydrins, including At1g76180.1, At1g20440.1, At1g20450.1, At4g38410.1, At3g50980.1, At2g21490.1 At4g39130.1, At3g50790.1, At1g54410.1, and At5g66400.1.
Isolation of the Genomic Region and the Promoter of IpDHN
Two primers (IpDHNF and IpDHNR; Supplementary Table S1) were designed according to the full-length cDNA sequencing data of IpDHN to obtain the genomic region sequences of IpDHN with standard PCR amplification. The genomic DNA was extracted from seedlings of I. pes-caprae using the EasyPure Plant Genomic DNA Kit (TransGen Biotech, Beijing). PCR was performed for 5 min at 94°C, followed by 35 cycles of amplification (94°C for 30 s, 55°C for 40 s, and 68°C for 2 min) and 68°C for 10 min. Then, the purified PCR products were ligated into a pGEM T-vector (Promega, Shanghai) and were sequenced.
The 5′ flanking region upstream of the translation start codon (promoter sequence) of IpDHN was isolated from I. pes-caprae genomic DNA through genome walking using a Genome Walking Kit (Takara, Dalian) according to the manufacturer’s instructions. For nested PCR, the IpDHN gene-specific primers, IpDHNSP1, IpDHNSP2, and IpDHNSP3 (Supplementary Table S1), and the adaptor primer AP1 were used. The primers IpDHNSP1, IpDHNSP2, and IpDHNSP3 were designed according to the genomic sequence of IpDHN (including the intron). The IpDHN promoter was amplified via PCR and cloned into the pGEM-T Easy vector (Promega, Shanghai) for sequencing. The putative cis-acting elements of the IpDHN promoter were analyzed using the online tool PlantCARE5 (Lescot et al., 2002).
Bacterial Overexpression and Salt, Osmotic, Dehydration, and H2O2 Tolerance Assays in E. coli
To further confirm the biological and biochemical functions of IpDHN, the exact ORF (open reading frame) of IpDHN cDNA was inserted into expression vector pGEX 6p-1 (GE Healthcare, Sweden) to generate the GST-tag recombinant plasmid IpDHN-pGEX 6p-1. The GST-IpDHN sequence was PCR amplified using the primers (IpDHNEPF and IpDHNEPR) listed in Supplementary Table S1. The PCR fragments were subsequently inserted into the BamHI site of pGEX 6p-1, following the GST-tag with the in-fusion technique (BD In-Fusion PCR cloning Kit, Takara Bio USA), yielding the recombinant plasmid IpDHN-pGEX 6p-1. The recombinant plasmid and pGEX 6p-1 (as a negative control) were then transformed into E. coli BL21 (DE3). Then, two single colonies (containing IpDHN-pGEX 6p-1 or pGEX 6p-1 vectors) were inoculated in liquid LB medium and allowed to grow overnight at 37°C under constant shaking at 200 rpm. Inoculum (1%) from the culture grown overnight was added to fresh liquid LB medium (100 mL) containing 100 μg/mL of ampicillin and allowed to grow at 37°C and 180 rpm. Expressions were induced at an OD600 of 0.5 by 0.2 mM isopropyl β-D-thiogalactopyranoside (IPTG), and the E. coli cells were allowed to grow at 30°C for 2–6 h with constant shaking at 180 rpm. The induced bacteria were harvested and protein profiles were examined by 12% SDS PAGE.
A spot assay was carried out to confirm the tolerance of recombinant E. coli, with at least three replicates per sample. To assess salt, H2O2, and osmotic stresses, cell cultures of E. coli containing pGEX 6p-1/IpDHN-pGEX 6p-1 were adjusted to an OD600 value to 1.0 and then diluted gradiently (to 1:10, 1:100, and 1:1000). Two microliters of each sample was spotted onto the LB plates containing 0.2 mM IPTG and the stress treatment (5% NaCl, 6% NaCl, 2 M sorbitol, 5 mM H2O2, or 8 mM H2O2). For the drought-endurance test, 1 mL of OD-adjusted cell cultures was centrifuged, and the supernatant was discarded; the bacterial precipitate in tubes was immediately placed in a 40°C drying oven for 4 h. Then, the samples were added to 100 μL liquid LB medium and thawed at 37°C for 1 h to recover. Subsequently, the samples were diluted and spread out on LB plates containing 0.2 mM IPTG. The plates were placed into incubator at 37°C for 14 h. The bacterial colonies were counted with colony-forming units (cfu).
To determine the growth curve in liquid culture medium, 1 mL inoculum (OD600 = 1.0) was added to 10 mL LB medium (plus 0.2 mM IPTG) containing salt (3% or 4% NaCl), sorbitol (0.8 M or 1 M) or H2O2 (0.7 mM or 0.9 mM), and incubated at 37°C with shaking at 180 rpm. The aliquots were collected from each treatment sample every 2 h for a period of 12 h, and optical density (OD600) was recorded. Abiotic stress (salt, osmotic, and oxidative stress) tolerances were evaluated according to the E. coli growth status with respect to control cultures (bacterial cells and vector controls).
Subcellular Localization Analysis
The CDS sequence (without the termination codon) of the IpDHN cDNA was amplified by PCR using the primer pair IpDHNGF and IpDHNGR (Supplementary Table S1). The PCR fragment was subcloned into the BamHI site of the pUC/GFP vector to generate an IpDHN-GFP in-frame fusion protein under the CaMV 35S promoter, following the in-fusion technique (BD In-Fusion PCR Cloning Kit, Takara Bio USA), generating the recombinant plasmid IpDHN-pUC/GFP. After sequencing confirmation, the fusion construct or empty vector (as a control) and the NLS-mCherry vector were individually co-transfected into protoplasts (3 × 104 protoplasts) using a PEG-calcium solution (0.4 g mL−1 PEG 4000, 0.2 M mannitol, 0.1 M CaCl2). After washing and resuspension in a W5 solution (154 mM NaCl, 125 mM CaCl2, 5 mM KCl, 5 mM glucose, 2 mM MES), mesophyll protoplasts were incubated under white light for 12–18 h. Fluorescence was visualized using a confocal laser scanning microscope (LSM 510 META, Zeiss, Germany).
Quantitative RT-PCR Analysis
For detection of the expression of IpDHN in I. pes-caprae plants, total RNA was isolated from different tissues of I. pes-caprae with HiPure Plant RNA Kits (Magen, Guangzhou), and cDNA was synthesized from total RNA using TransScript One-Step gDNA Removal and cDNA Synthesis SuperMix (TransGen Biotech, Beijing) with Oligo (dT)15 primers according to the manufacturer’s instructions. QRT-PCR was conducted using a LightCycler® 480 Gene Scanning system (Roche, Switzerland) and TransStart Tip Green qPCR SuperMix (TransGen Biotech, Beijing, China). The expression levels of IpDHN in various organs of seedlings and adult I. pes-caprae plants were detected, including the seedling root, seedling leaf, bud, mature root, vine, mature leaf, flower bud, petal, and young seeds 7 days after pollination (DAP). The I. pes-caprae seedling samples (roots, vines, and leaves), treated with salt, simulated drought or dehydration, oxidative and frost stresses and ABA, were also checked to examine the expression changes of IpDHN. Each tissue sample was collected from three dependent adjacent plants in the same location. All gene expression data obtained via quantitative RT-PCR were normalized to the expression of IpUBQ (GenBank Accession No.: MF502417). The primers used for quantitative RT-PCR are listed in Supplementary Table S1.
For detection of the expressions of antioxidant system-related genes (CAT1, FSD1, CSD1, and APX2) and abiotic stress-related genes (NCED3, HAI2, RD29A, RD29B, HVA22D, ANAC19, RD22, and RD26) in Arabidopsis (WT or transgenic plants), total RNA was isolated from rosette leaves at different time points (with or without treatments), and cDNA synthesis was performed following the above procedure. The reference gene for quantitative RT-PCR in Arabidopsis was ACT2 (At3g18780). The primers used for quantitative RT-PCR are listed in Supplementary Table S1.
DNA Constructs and Plant Transformation
To generate the recombinant vector for the overexpression assay in transgenic Arabidopsis, the full-length cDNA of IpDHN was PCR amplified using the primer pair IpDHNOXF and IpDHNOXR (Supplementary Table S1) to obtain the CDS region of IpDHN. The PCR product was cloned into the BamHI site of the pBIm plasmid (Yang et al., 2005) to generate IpDHN-pBIm, with an expression cassette under the control of the CaMV 35S promoter.
After sequencing confirmation, the construct was transferred into Agrobacterium tumefaciens strain GV3101, the positive clone was picked out and cultured. The T-DNA region containing IpDHN and NPTII expression cassette was transformed into Arabidopsis with the floral dip method. Seeds of the T1 and T2 generations were germinated on MS agar medium containing 50 mg/L kanamycin to finally obtain homozygous lines. Positive transgenic plants were selected according to the segregation ratio (resistant:sensitive = 3:1) and were confirmed by genomic PCR with the primer pair IpDHNOXF/IpDHNOXR.
Abiotic Stress Tolerance Assays in Transgenic Arabidopsis
The seed germination rate of IpDHN transgenic Arabidopsis was detected under NaCl (100, 125, 150, 175, and 400 mM) and mannitol (200, 300, and 400 mM) challenges to detect the positive effect of IpDHN overexpression on the improvement in the salt and osmotic tolerance of transgenic Arabidopsis seed germination. In addition, the root length was also measured to evaluate the influence of IpDHN overexpression on the seedlings of transgenic Arabidopsis under abiotic stresses. WT Arabidopsis was used as a control.
Salt and drought tolerance assays were also assesses with transgenic Arabidopsis adult plants. Both WT and transgenic seeds were synchronously germinated on MS medium plates. Ten-day-old seedlings were transplanted into sieve-like square pots filled with soil mixture and were well watered. Thirty plants of each genotype were planted in a growth pots and trays placed in greenhouse as described above without watering for 10 days. Subsequently, the plants were subjected to the following assays. For drought-tolerance assays, WT and transgenic plants (IpDHN OX1, and IpDHN OX2) were maintained under a continuous drought for 9 days and were re-watered for 7 days. For salt-tolerance assays, plants of each genotype were planted in sieve-like pots and were well watered as described for the drought-tolerance treatment. Water was withheld for 10 days before irrigation with NaCl solution (150 mM and 200 mM) from the bottom of the trays. When the soil was completely saturated with salt water, the NaCl solution was removed and the plants were cultured normally. The plants grew in salt-dosed soils for 10 days and were re-watered with fresh water for 7 days.
Measurement of Physiological Parameters
Rosette leaves were gathered from 3-week-old seedlings growing in soil in a greenhouse at room temperature, with or without treatments, including 200 mM NaCl for 24 h or 300 mM mannitol for 24 h. Subsequently, several plant physiological parameters were detected and calculated, including RWC (relative water content), IL (ion leakage), the proline (Pro) content, the MDA content, CAT activity, and SOD activity. Experiments were performed following a completely randomized design with three replications and were repeated three times.
The RWC was calculated as described previously (Hu et al., 2013). Fresh weight (FW) of Arabidopsis rosette leaves were recorded followed by soaking the leaves for 4 h in distilled water at room temperature with constant light. After this treatment the turgid weight (TW) was recorded. The leaves were then dried for 24 h at 80°C to obtain the total dry weight (DW). RWC was calculated from the equation: RWC (%) = [(FW–DW)/(TW–DW)] ∗ 100.
Ion leakage was measured with a DDS-307A conductivity meter (Shanghai Jingke, China), according to Hu et al. (2013) with slight modifications. Arabidopsis rosette leaves were cut into strips (1 mm) and incubated in 10 mL double distilled water at room temperature, shaking at 150 rpm for 2 h. Initial conductivity (C1) was measured with a conductivity meter followed by boiling the samples for 30 min to measure complete IL. The leaves were then cooled to room temperature to measure the electrolyte conductivity (C2). The electrolyte conductivity of double distilled water was also measured (C0). IL was calculated according to the equation: IL (%) = (C1–C0)/(C2–C0) ∗ 100.
The free proline (Pro) content, malondialdehyde (MDA) content, and SOD and CAT activities were determined using Proline, MDA, SOD, and Catalase Assay Kits, respectively, according to the manufacturer’s instructions (Nanjing Jiancheng Bioengineering Institute, China).
Rosette leaves were collected from 2-week-old seedlings growing in soil; the leaf stalks were immersed in 150/200 mM NaCl or 300 mM mannitol solutions for 3 h. In situ detection of H2O2 and O2− was determined using vacuum-infiltration with 0.2% nitro-blue tetrazolium (NBT) or 1 mg/mL 3.3′-diaminobenzidine (DAB) solution, respectively, for 12 h followed by clearing in 75% ethanol, as previously described (Cao et al., 2017).
Statistical Analysis
All of the experiments in this study were repeated three times in independent experiments, and the data shown are the mean ± SD. In this research, statistical analyses were performed using the statistical tools (Student’s t-test) of Microsoft Excel software.
Results
IpDHN Encodes an SK3 Type Dehydrin
IpDHN and other candidate salt stress-related genes were identified from a cDNA full-length library, generated from total RNA of I. pes-caprae seedlings, based on the FOX gene hunting system (unpublished data), screened with a salt-sensitive yeast mutant strain, AXT3 (Zhou et al., 2015). The full-length IpDHN cDNA was inserted into the pYES-DEST-52 expression vector under the control of a galactose-induced promoter, PGAL. Our previous research indicated that the induced expression of IpDHN in yeast could partly rescue the salt-sensitive phenotype of the AXT3 yeast mutant and also improved the salt tolerance of the WT yeast W303. We also found that IpDHN could elevate the tolerance of yeast strains (yap1Δ and skn7Δ) to oxidative stress (Supplementary Figure S1).
The full-length IpDHN cDNA is 983 bp, including an 85-bp 5′ -untranslated region (UTR), a 244-bp 3′ -UTR region and a 654-bp ORF (Supplementary Figure S2A). Analysis of the protein sequence using the ExPASy ProtParam calculated the isoelectric point to be 5.34, with a molecular mass of 24.162 kD and an overall hydropathicity average (GRAVY) of −1.364, indicating that IpDHN is strongly hydrophilic. The amino acid composition of the IpDHN protein is shown in Supplementary Table S2.
SmartBLAST analysis with the amino acid sequence of IpDHN showed that it is highly homologous to DHN InDHN from Ipomoea nil, CcDHN from Coffea canephora, CaDHN1 from Capsicum annuum, and StDHN from Solanum tuberosum (Supplementary Figure S2B). Further analysis showed that the IpDHN protein contained an S segment and three K segments (Supplementary Figure S2B). The IpDHN protein was rich in hydrophilic amino acids such as Glu (22.58%), Lys (18.89%), Ser (6.45%), and His (4.61%), but lacked the hydrophobic amino acid Trp (0) (Supplementary Table S2). In addition, in silico prediction with the Protein Fold Recognition Server tool (PHYRE2) suggested that more than 80% of the amino acid residues of IpDHN were disordered, indicating that IpDHN is an IDP. The interactive 3D view in JSmol (PHYRE2) showed that IpDHN was highly disordered (Supplementary Figure S3). A phylogenetic tree was constructed to evaluate the molecular evolutionary relationships between IpDHN and other plant DHN proteins. The results showed that the IpDHN protein can be grouped as the SKn type (Figure 1).
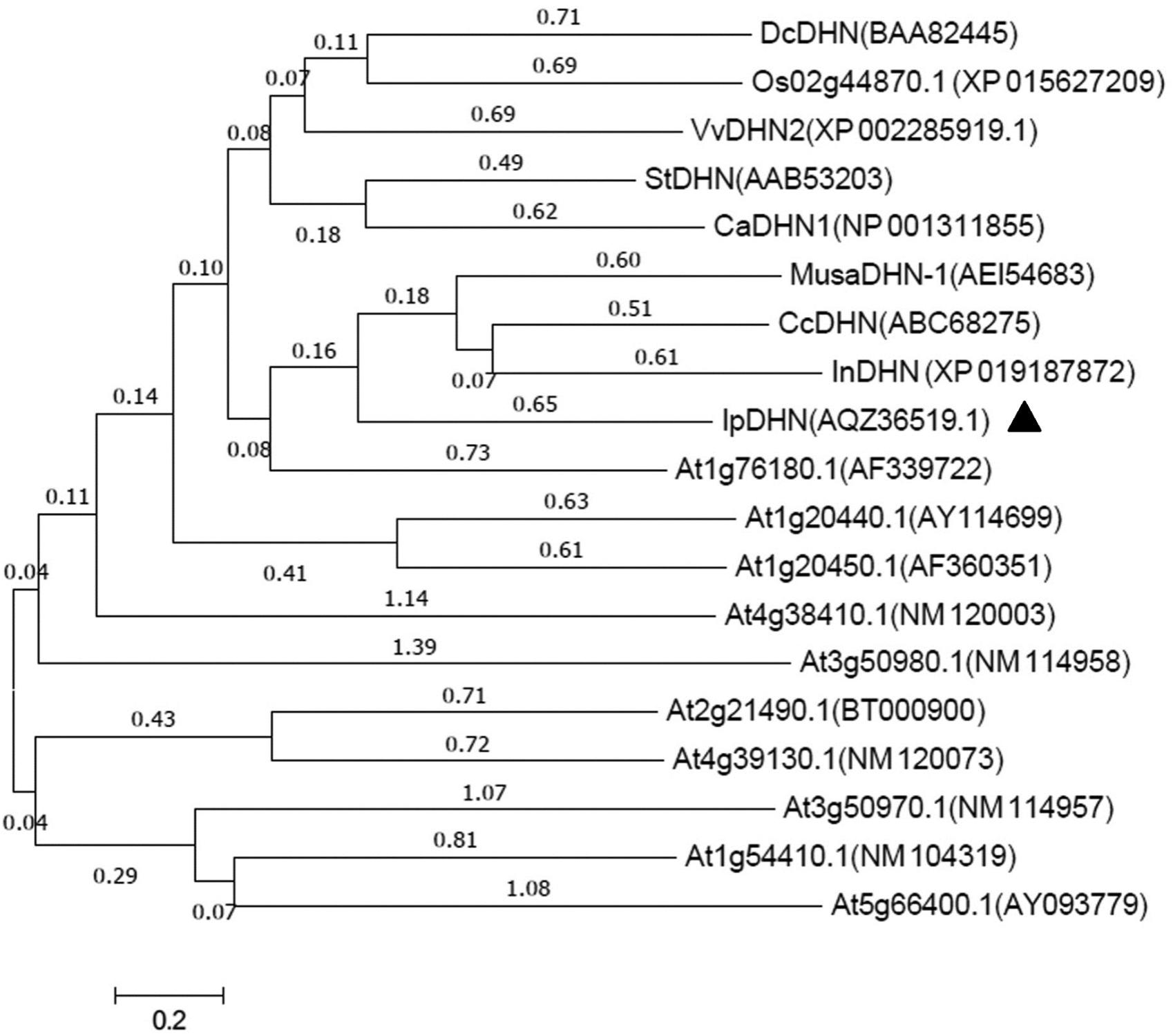
FIGURE 1. Phylogenetic relationships between the IpDHN protein and dehydrins from other plant species. The molecular phylogeny was constructed from a complete protein sequence alignment of DHNs by the neighbor-joining method with ClustalW. The numbers beside the branches indicate bootstrap values. The symbol “” shows the position of IpDHN in the phylogenetic tree.
The Structure of the IpDHN Genomic Sequence of the Coding and Promoter Regions
A comparison of genomic (Supplementary Figure S2C) and cDNA (Supplementary Figure S2B) sequences showed that the IpDHN contained a single intron of 264 bp. We also isolated a 974 bp IpDHN promoter region. Analysis of the IpDHN promoter using PlantCARE6 indicated that numerous potential cis-acting elements that respond to environmental signals were present (Figure 2), and these elements are classified in Supplementary Table S3. A core promoter element, i.e., a TATA-box sequence, was located 178 bp upstream of the translational start site (TSS). We also identified an ABA-responsive element (ABRE, TACGTG sequence, 48 bp upstream of TSS) and three Myb binding sites (MBS1 and MBS2, CGGTCA/CAACTG sequences, 52, 718, and 925 bp upstream of TSS), which might be involved in drought induction and other abiotic stresses. A typical cis-acting element Skn-1-motif (GTCAT), proven to be capable of activating gene expression in the endosperm, was found 246 bp upstream of TSS, which further implicated IpDHN in belonging to seed storage proteins, matching the characteristics of LEA proteins. Furthermore, a TC-rich repeat regulatory element (ATTTTCTCCA), with homology to that identified in defense and stress-responsive genes, was detected 767 bp upstream of TSS (Figure 2). The other cis-elements in the IpDHN promoter region are listed in Supplementary Table S3.
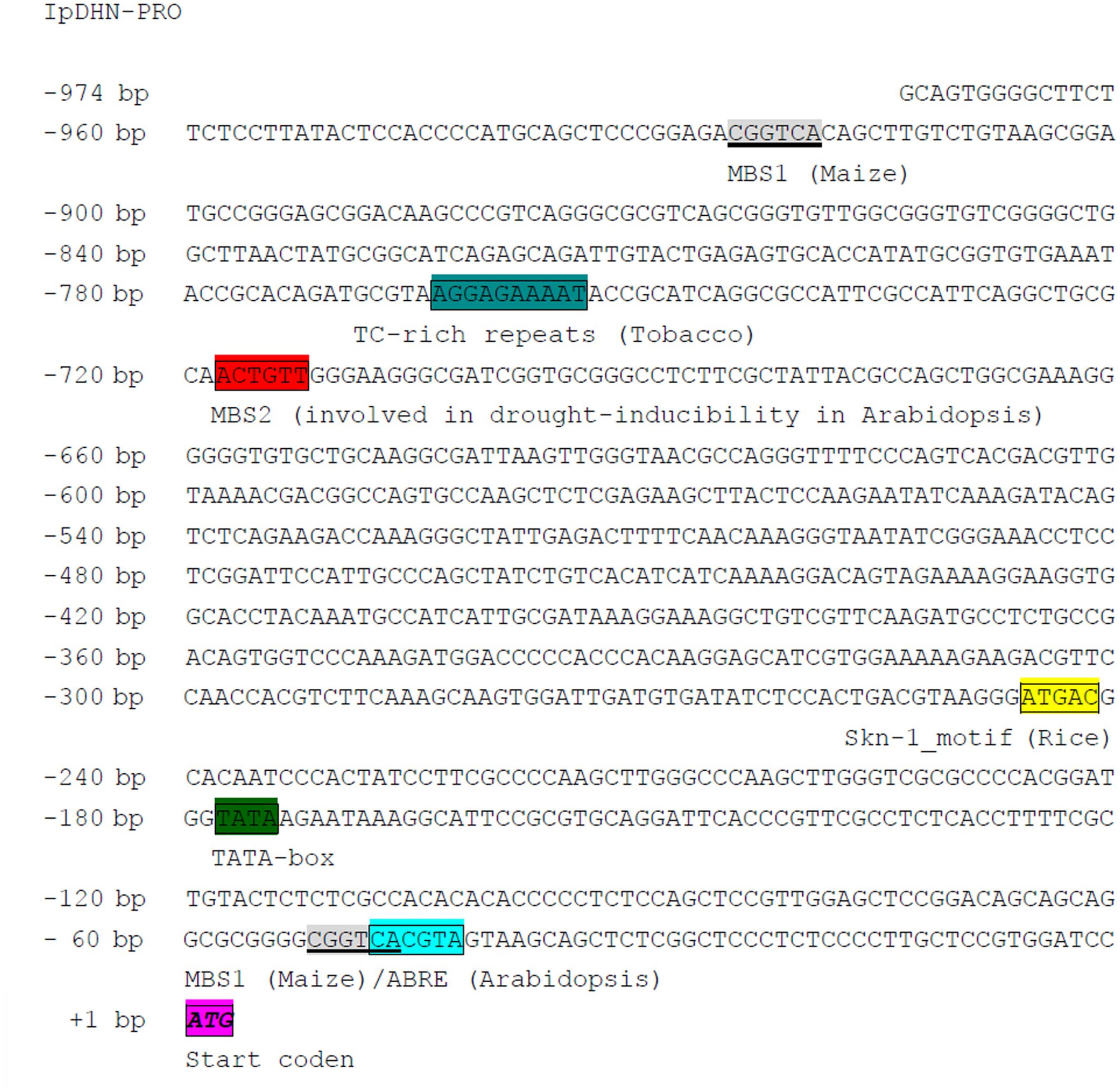
FIGURE 2. Nucleotide sequence of the IpDHN promoter (974 bp). Nucleotides are numbered on the left. The putative translation start sites, TATA box, and other important cis-regulatory elements are boxed and labeled.
Salt, Dehydration and H2O2 Tolerance Assays of E. coli
The coding region of IpDHN cDNA was amplified and cloned into the pGEX 6p-1 vector (BamHI site). After sequence confirmation, the recombinant plasmid IpDHN-pGEX 6p-1 and empty vector pGEX 6p-1 (as a negative control) were expressed in E. coli BL21 (DE3) cells. The GST-IpDHN and GST proteins were analyzed by SDS-PAGE (12%). As shown in Figure 3A, obvious expression of recombinant IpDHN (fused with GST) and the GST protein was observed after 2 h, compared with non-induced cells. The fusion protein (GST-IpDHN) presented a induced band of approximately 50 kDa, which is accordant with the expected size of GST-IpDHN, which was undetectable in non-induced E. coli cells (Figure 3A), indicating that the GST-IpDHN fusion protein was correctly expressed in recombinant E. coli. The difference in the molecular weight between the fusion and GST proteins confirmed the predicted 24-kDa molecular weight of the IpDHN protein.
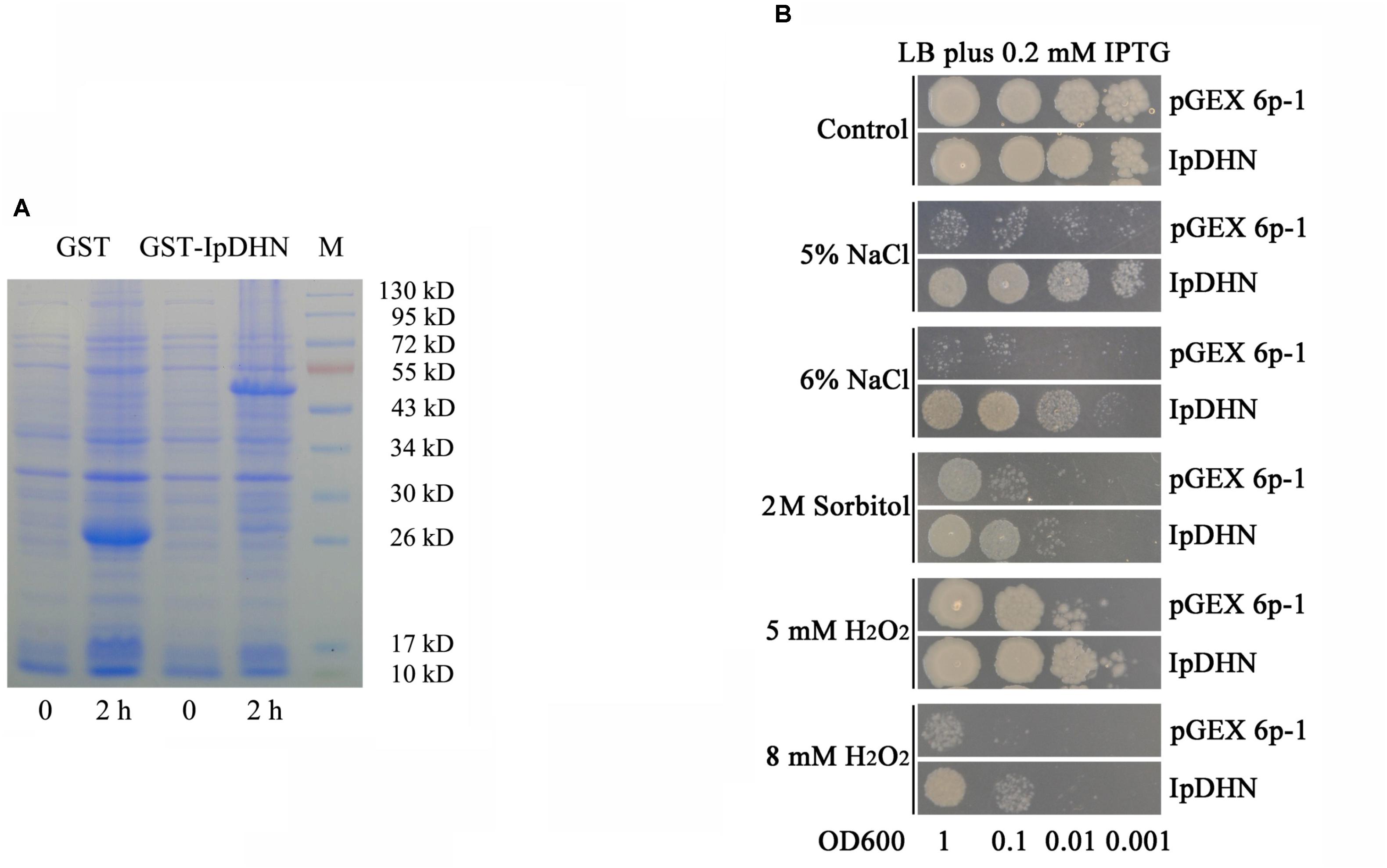
FIGURE 3. Functional analysis of IpDHN induced-expression for salt, drought, and H2O2 tolerance in Escherichia coli. (A) Induced expression of the GST-IpDHN (IpDHN- pGEX 6p-1) fusion protein and single GST (pGEX 6p-1 empty vector) protein in E. coli. 0 and 2 h: the IPTG induction time. (B) The growth performance of E. coli BL21 (pGEX 6p-1, upper)/(IpDHN-pGEX 6p-1) on LB plates containing stress factors. Control (top): LB medium; 5% NaCl: LB medium containing 5% NaCl; 6% NaCl: LB medium containing 6% NaCl; 2 M Sorbitol: LB medium containing 2 M sorbitol; 5 mM H2O2: LB medium containing 5 mM H2O2; 8 mM H2O2: LB medium containing 8 mM H2O2. The cell cultures were adjusted to OD600 to 1 and were then diluted serially (1:10, 1:100, and 1:1000, respectively). Two microliters of each sample was spotted onto the LB plates containing 0.2 mM IPTG.
The bacteria spot and liquid culture assays were performed with IPTG-induced E. coli cells to confirm the positive effect of IpDHN’s over-accumulation on salt, osmotic, and oxidative stresses tolerance. As can be observed in Figure 3B, obvious differences in E. coli growth status were observed for the spot assay, where the numbers of colonies were much higher in several stress treatments for recombinant E. coli cells with induced GST-IpDHN compared to E. coli cells with the empty vector (GST) control. The E. coli liquid culture growth curve assay under stress conditions indicated that the growth of E. coli cells accumulating the GST-IpDHN fusion protein increased significantly and rapidly compared to E. coli cells expressing the GST protein only (Supplementary Figure S4A).
The tolerance of E. coli with the empty vector (GST) control and GST-IpDHN subjected to drought stress was also determined by spreading dilutions of cultures on LB plates containing 0.2 mM IPTG, after drying the bacterial precipitate at 40°C for 4 h and recovering the precipitates in 100 μL liquid LB medium for 1 h. The Colony-Forming Units (cfu) counting assay shows a significant difference in the number of living E. coli cells before and after desiccation stress (Supplementary Figure S4B). Before the drying process, the control (GST) and GST-IpDHN expressing E. coli cells showed very similar colony numbers (CFU, expressed in ×106 units), but after desiccation, although only a very small fraction of cells survived, the number of colonies expressing GST-IpDHN was six times higher than control cells. Our result here indicates that IpDHN’s induction in E. coli cells improved their survival capacity after desiccation.
Subcellular Localization of IpDHN
The subcellular localization of IpDHN was determined by a GFP-fusion protein transient expression assay in Arabidopsis mesophyll protoplasts. The results showed that IpDHN-GFP primarily distributed in the entire cytoplasm of Arabidopsis mesophyll protoplasts (Figure 4, upper row), with a similar localization pattern for the control GFP (Figure 4, lower row). However, the IpDHN-GFP was clearly missing in the nucleus, but the control GFP exhibited strong signals in the nucleus, which were detected throughout the cell (Figure 4). Based on these in vivo targeting results, we concluded that IpDHN is predominantly localized to the cytoplasm but might not undergo nuclear distribution.
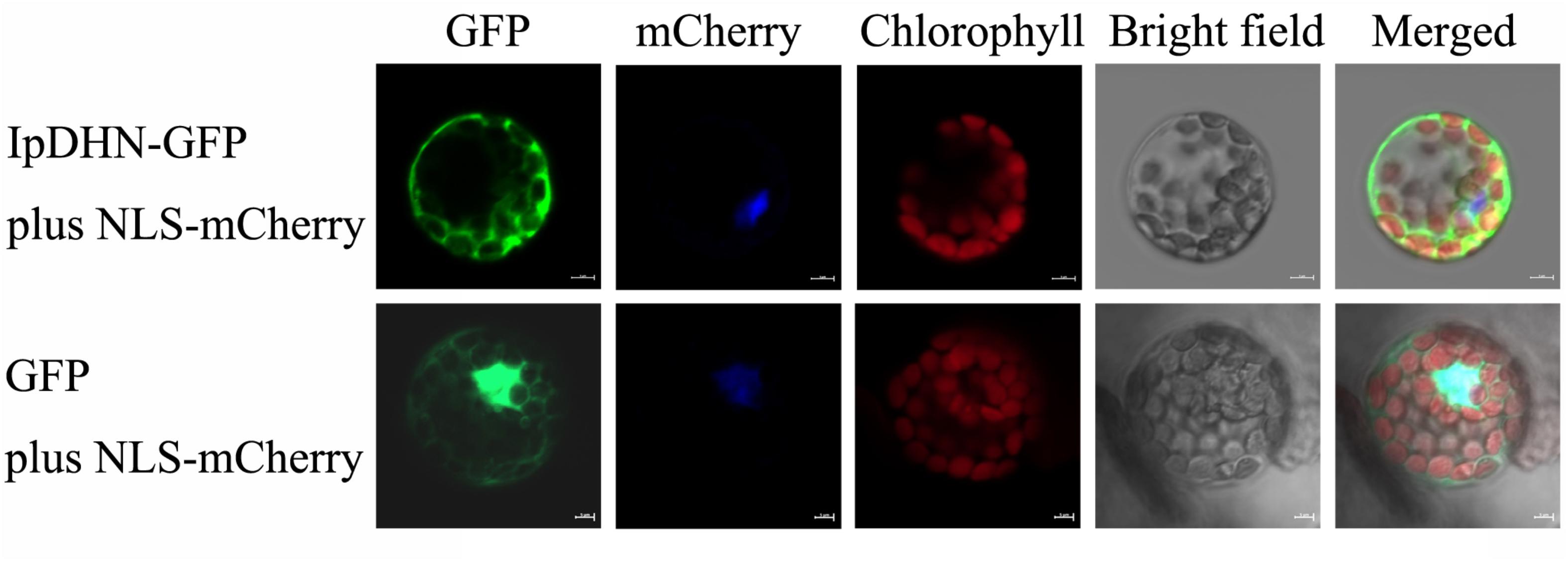
FIGURE 4. Subcellular localization of IpDHN. Arabidopsis protoplasts expressing the 35S:IpDHN-GFP fusion protein (upper) and 35S:GFP (lower) observed under a laser scanning confocal microscope. The blue color indicates the nucleus using mCherry as the nuclear marker. The red color indicated autofluorescence emitted by chloroplasts. Bar represents 5 μm.
Quantitative RT-PCR Analysis of IpDHN Under Abiotic Stresses and ABA Treatment
To investigate the expression pattern of IpDHN in I. pes-caprae, quantitative RT-PCR was performed with total RNAs extracted from various tissues. Our results demonstrated that IpDHN was expressed constitutively in most I. pes-caprae tissues (Figure 5A). The highest level of the IpDHN transcript was detected in the mature root, vine and leaf; the young root and flower petal also showed a high expression of IpDHN, while IpDHN was weakly expressed in the tissues/cells that were rapidly growing, dividing and metabolizing, such as the young leaf, shoot bud, flower bud, and young seed. The expression pattern of IpDHN indicated that the biological role of IpDHN was more involved in abiotic stress response as opposed to cellular growth and development.
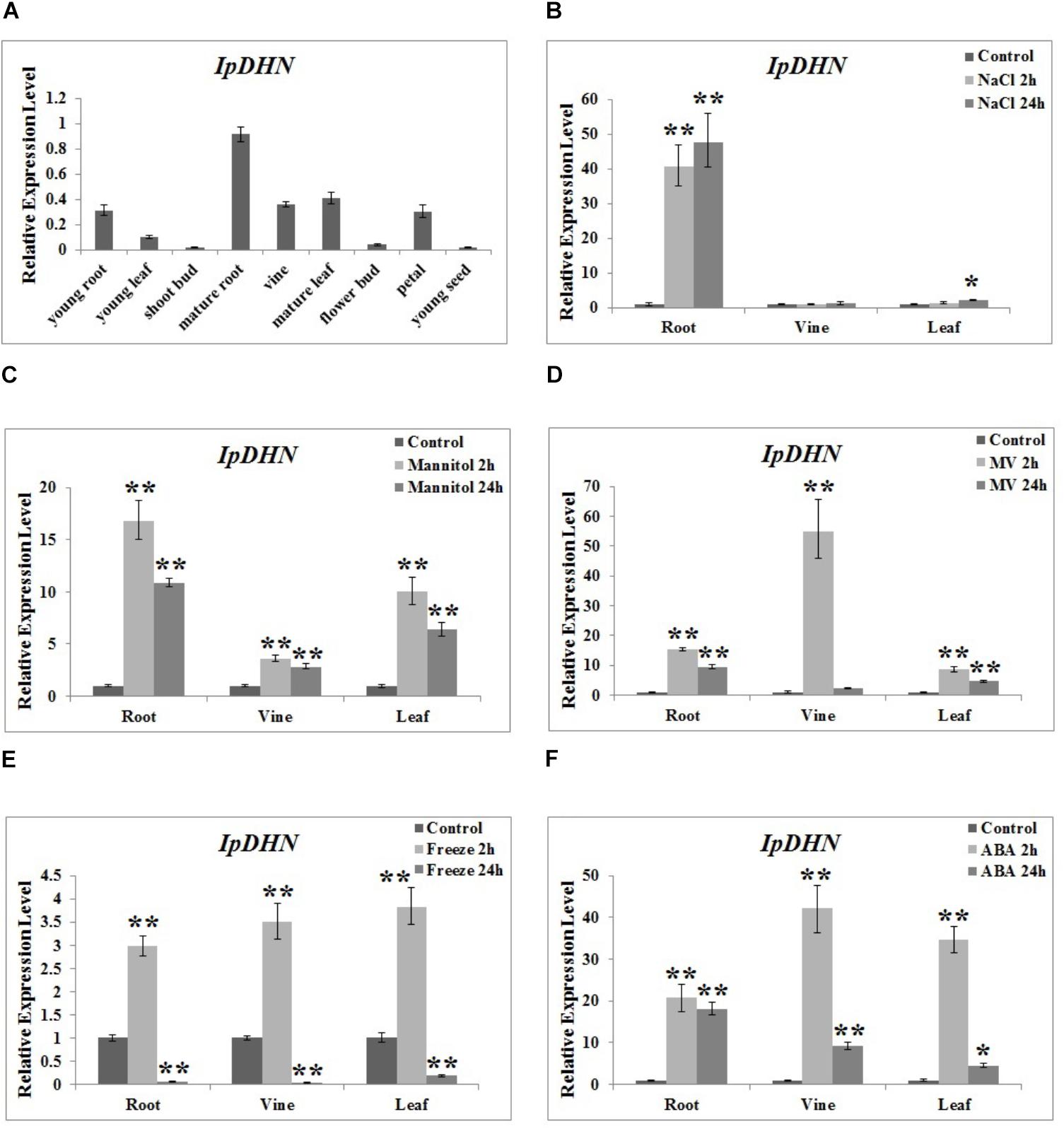
FIGURE 5. Expression profiles of the IpDHN gene among Ipomoea pes-caprae tissues. (A) Differential expression of IpDHN in various tissues (young root, young leaf, shoot bud, mature root, vine, mature leaf, flower bud, petal, young seed). (B–F) Time-course expression patterns of IpDHN in response to different abiotic stresses: NaCl (B), Mannitol (C), MV (D), freeze (E), and ABA treatment (F). Error bars indicate the SD based on three replicates. Asterisks indicate significant differences from the control (Student’s t-test P-values, ∗p < 0.05, ∗∗p < 0.01).
To determine the expression of IpDHN under stress, the transcript of IpDHN in the root, vine, and leaf of I. pes-caprae seedlings was analyzed by quantitative RT-PCR. Under 300 mM NaCl salt stress, the transcript level of IpDHN showed the greatest increase to more than 40-fold in the root and slight increases in the vine and leaf tissues (Figure 5B). Under dehydration stress (300 mM mannitol, simulating drought stress), the whole I. pes-caprae seedling showed an increased expression pattern of IpDHN, and in the root, the induced level reached almost 20-fold (Figure 5C). Low temperature (0°C) could also rapidly and slightly induce the expression of IpDHN, with a peak at 2 h (Figure 5E). We also checked the expression change of IpDHN under methyl viologen (oxidative stress) and ABA treatment (Figures 5D,F). Our results showed that both methyl viologen and ABA could greatly and rapidly increase the transcript level of IpDHN (Figure 5).
Overexpression of IpDHN1 in Arabidopsis Enhances Tolerance to Salt and Drought
To further determine its biological function, IpDHN was over-expressed in Arabidopsis. Two homozygous T3 lines (IpDHN OX1 and IpDHN OX2) were selected for IpDHN, and quantitative RT-PCR was performed. The results showed that both IpDHN overexpression lines were highly expressed in the transgenic Arabidopsis (Figure 6A). In addition, the RT-PCR results also showed that the expression of IpDHN (IpDHN OX1 and IpDHN OX2) was higher than that of the reference gene AtActin2 (At3g18780) in two transgenic lines (Figure 6).
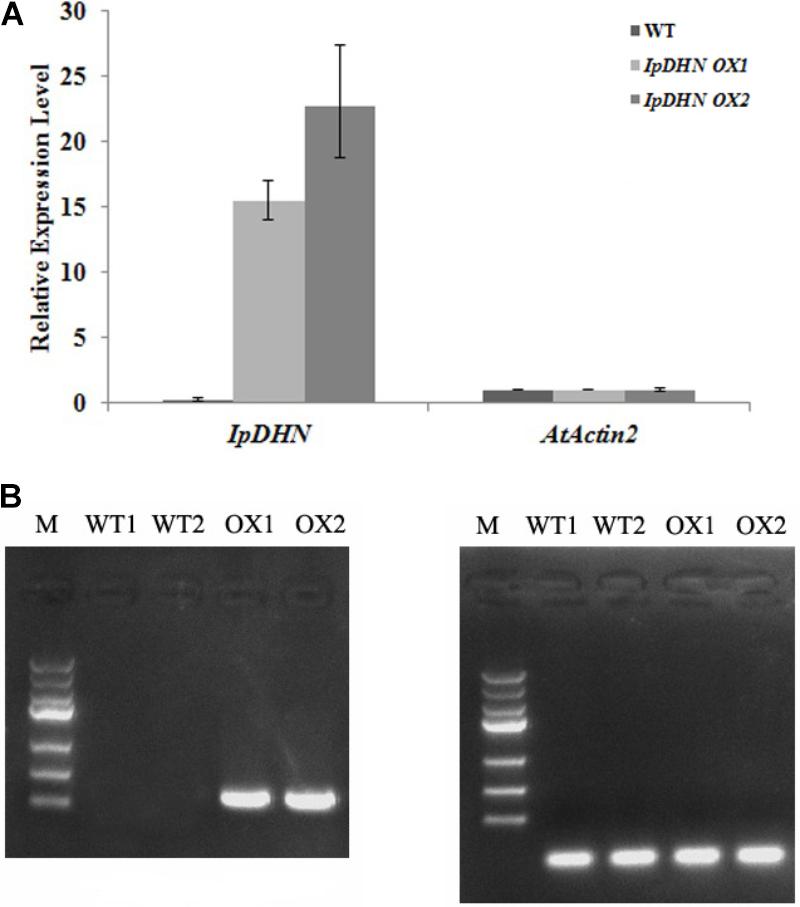
FIGURE 6. The overexpression analyses of IpDHN in transgenic Arabidopsis lines (IpDHN OX1 and IpDHN OX2). (A) Quantitative RT-PCR analysis of IpDHN in transgenic Arabidopsis lines and WT Arabidopsis (WT). AtActin2 was used as an internal control. Error bars indicate the SD based on three replicates. (B) RT-PCR analysis of IpDHN in transgenic Arabidopsis lines and two WT lines. (C) RT-PCR analysis of AtActin2 in transgenic Arabidopsis lines and two WT lines as a control.
To detect whether the over-accumulation of IpDHN in transgenic Arabidopsis could affect osmotic stress tolerance at seedling emergence stage, the seed germination rates of IpDHN OX1, IpDHN OX2 and the WT were monitored under different concentrations of NaCl or mannitol challenges. As shown in Figure 7, under non-stress conditions or a low level of osmotic stress (100 mM NaCl, 100 or 125 mM mannitol), no significant differences in the seed germination rate were observed between the WT and transgenic plants; however, when grown on MS medium supplemented with a higher concentration of NaCl (150, 175, and 200 mM) or mannitol (300 and 400 mM), the transgenic seeds (IpDHN OX1 and IpDHN OX2) showed significantly higher germination rates than those of the WT. At a low level of osmotic stress (100 mM NaCl, 100 or 125 mM mannitol), the transgenic seedlings showed significantly larger cotyledons than those of the WT, even with similar seed germination rates (Figure 7). The obvious effect of IpDHN on improving osmotic stress during seed germination was also observed when the seeds were challenged continuously with 150 mM NaCl or 200 mM or 300 mM mannitol for 3 weeks (Supplementary Figure S5).
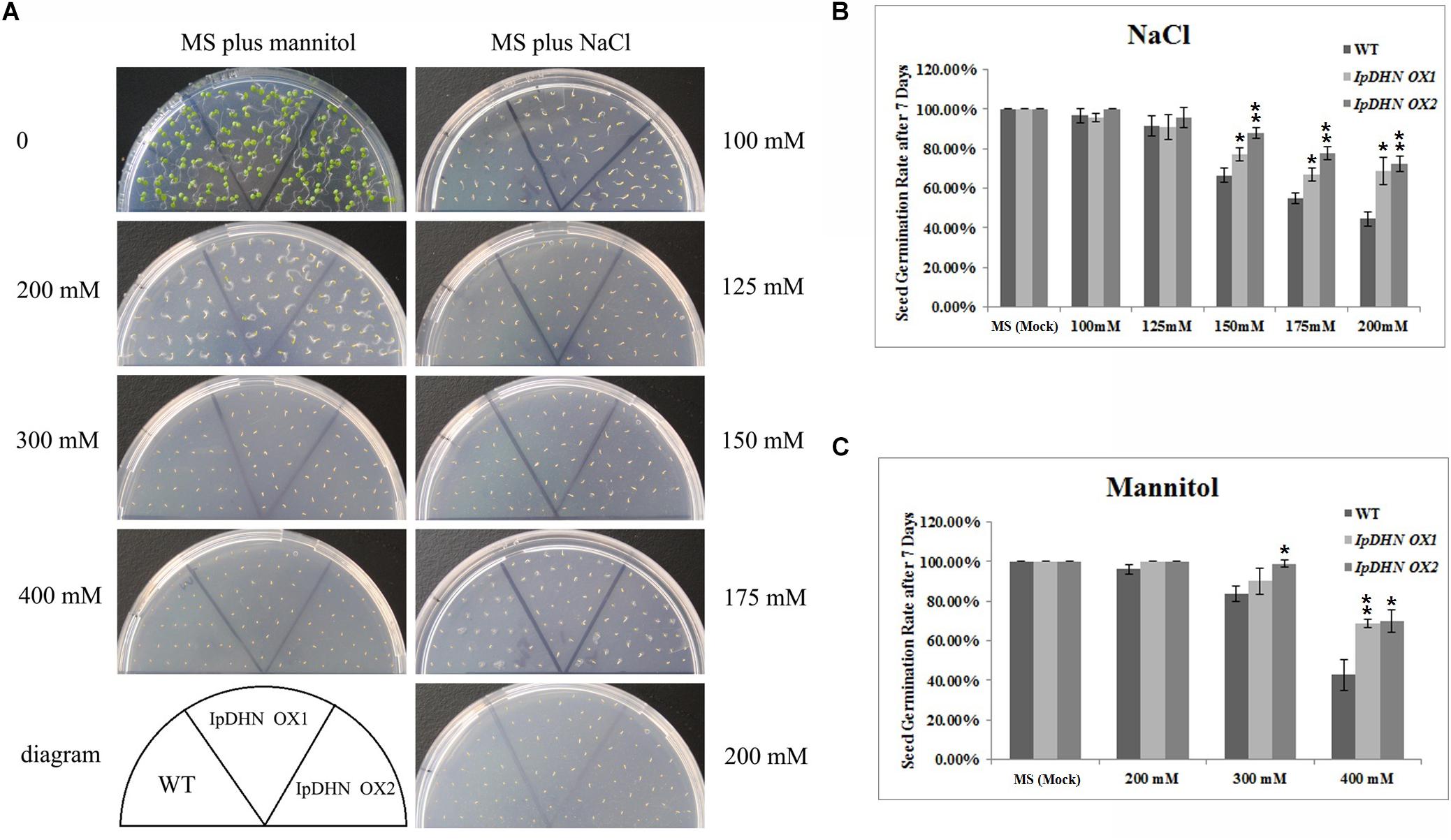
FIGURE 7. Osmotic and salt stress analyses of transgenic plants with IpDHN with respect to the seed germination rate. (A) Photographs of transgenic lines (IpDHN OX1 and IpDHN OX2) and WT seeds germinated on MS medium or MS medium with mannitol (left, 200, 300, and 400 mM) or NaCl (right, 100, 125, 150, 175, and 200 mM) for 7 days. Seed germination rates were calculated for the WT and transgenic lines under NaCl (B) and mannitol (C) stress after 7 days. Error bars indicate the SD based on three replicates. Asterisks indicate significant differences from the WT (control, Student’s t-test P-values, ∗p < 0.05, ∗∗p < 0.01).
To further test osmotic tolerance, the root length of Arabidopsis seedlings was also measured to assess the tolerance of plant growth. Four-day-old seedlings were transferred onto MS/agar plates containing NaCl or mannitol and were further cultivated vertically for 7 days after which the root lengths were measured. As we can see from Figure 8, there are no significant differences in root length were observed between the WT and transgenic lines when grown on medium without NaCl or mannitol. Correspondingly, for the Correspondingly, for the challenges of medium containing 125 mM NaCl or 200 mM mannitol, the root lengths of both sets of transgenic plants were significantly longer than those of the WT plants, suggesting that the overexpression of IpDHN enhances salt and drought/dehydration tolerance at the seedling stage (Figure 8).
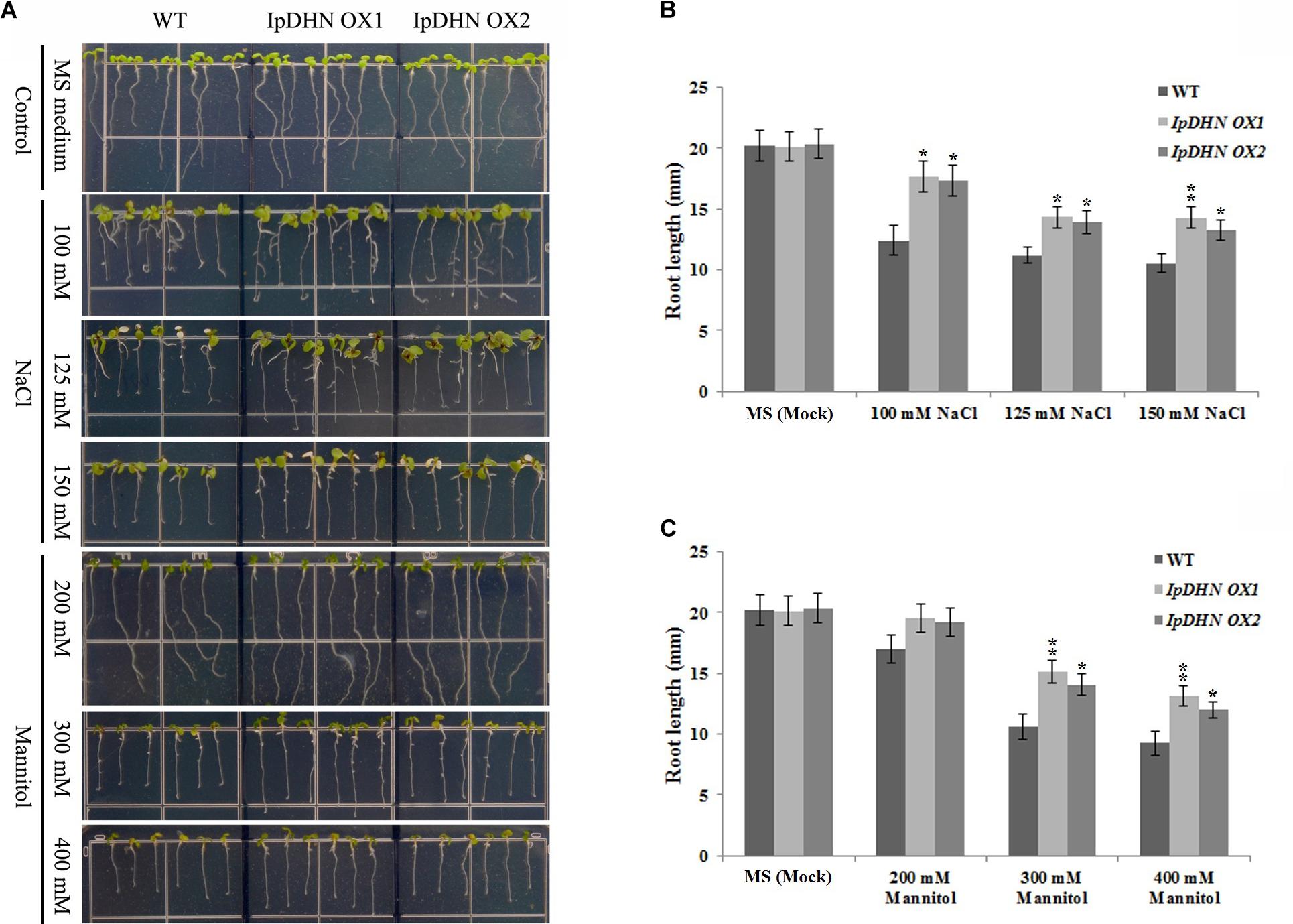
FIGURE 8. Osmotic and salt stresses analyses of transgenic plants with IpDHN with respect to seedling root length. Four-day-old seedlings were transplanted to MS medium containing NaCl or mannitol and were then grown for 7 days before measuring the root length. (A) Photographs of transgenic lines (IpDHN OX1 and IpDHN OX2) and WT seedlings on MS medium or MS medium with NaCl (right, 100, 125, and 150 mM) or mannitol (200, 300, and 400 mM). (B,C) Seedling root length of WT and transgenic lines under NaCl (B) and mannitol (C) stress after 7 days. Error bars indicate the SD based on three replicates. Asterisks indicate significant differences from the WT (control, Student’s t-test P-values, ∗p < 0.05, ∗∗p < 0.01).
We also conducted salt and drought stress assays on adult Arabidopsis plants to further characterize the phenotypes of IpDHN overexpression in Arabidopsis. In general, we characterized the phenotypes of the IpDHN overexpression lines through adult-plant stress assay in pots (Figure 9). The seedlings of these lines were transferred to well-watered soil in pots, and watering was withheld for approximately 10 days to gradually reduce the water content of soil. Two levels of high salinity, 150 and 200 mM NaCl infiltrated soil, were taken in this assay. Under normal conditions, no differences in the growth of the transgenic lines and the WT controls were observed. While after challenges of salinity stress, almost all of the WT plants showed a severe reduction of growth (Figures 9A,B). We observed severe dehydration of the leaves, and the whole plant was wilted. By contrast, although some of the leaves of most of the DHN transgenic plants showed chlorosis, as a whole, they showed resistance to salinity stress and were still able to grow.
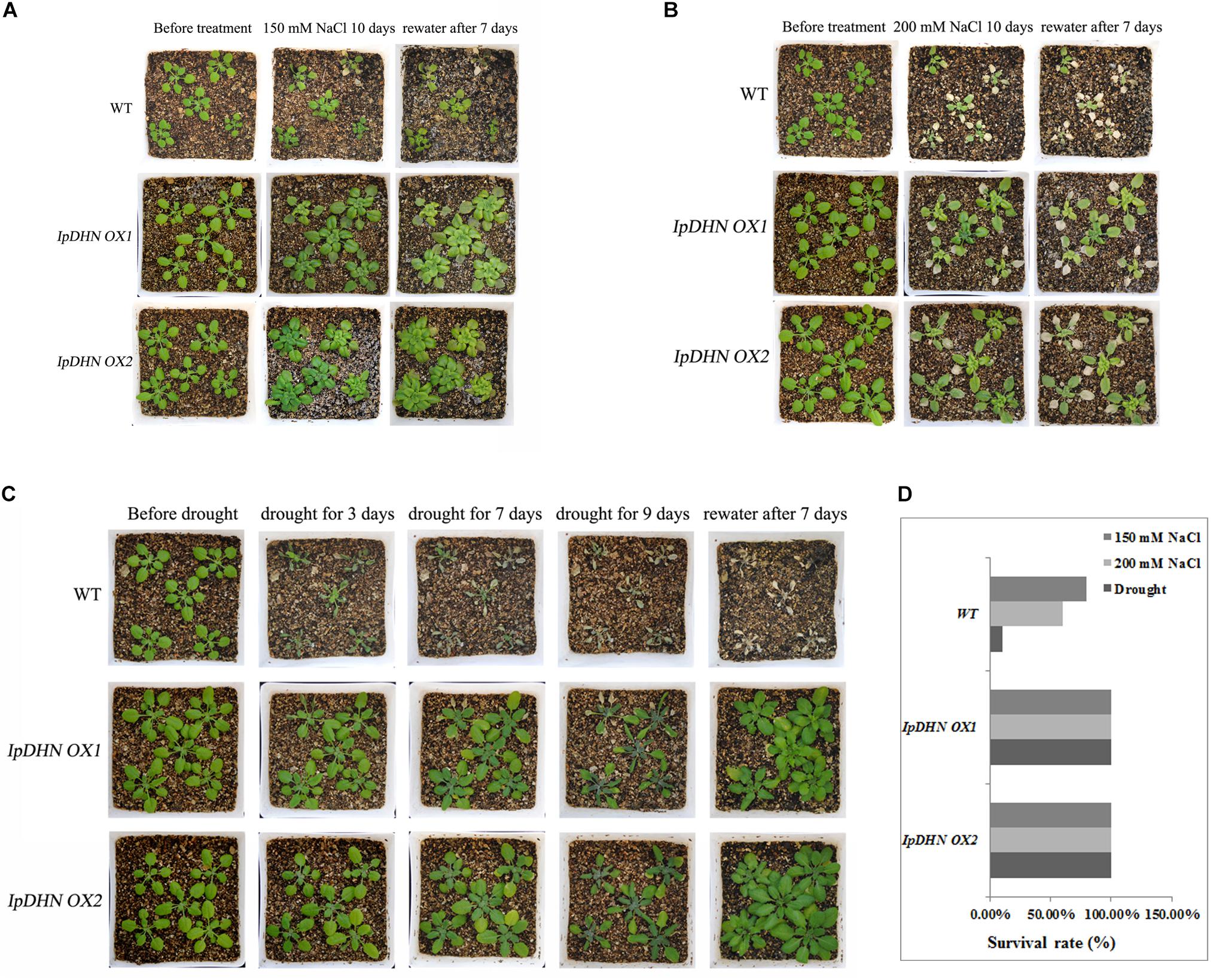
FIGURE 9. Photographs and survival rate of the transgenic lines (IpDHN OX1 and IpDHN OX2) and WT plants grown in pots under normal and salt/drought conditions. (A) The effects of 150 mM NaCl on transgenic lines and WT. (B) The effects of 200 mM NaCl on transgenic lines and WT. (C) The effects of water withholding on transgenic lines and WT. (D) The statistics for the survival rate of transgenic lines and WT Arabidopsis after salt/drought stresses. Thirty plants from the WT and from the two transgenic lines (IpDHN OX1 and IpDHN OX2) were treated with various concentrations of NaCl or drought.
The IpDHN transgenic plants also showed improvement of drought tolerance. The adult Arabidopsis plants (including IpDHN OX1, IpDHN OX2 and WT), from which watering was withheld for 10 days, were adapted for the drought-tolerance assay. Along with the gradual water loss, the majority of the WT plants exhibited obvious wilting due to severe stress while the survival rate of the transgenic lines (IpDHN OX1 and IpDHN OX2) was apparently higher than that of the WT controls (Figure 9C). After the rewatering treatment, the IpDHN OX lines were largely restored, while the WT controls showed a lethal phenotype (Figure 9C).
The above results indicated that IpDHN increased plant tolerance to salinity and drought stress and significantly improved the survival rates of transgenic Arabidopsis under osmotic stress (Figure 9D). These results also indicated that the accumulation of IpDHN in transgenic Arabidopsis provides better protection for basic cellular activities in vivo under osmotic stress.
Overexpression of IpDHN Increases RWC, Proline Content and Decreases IL and the MDA Content Under Osmotic Stresses
To further clarify the possible physiological mechanisms involved in cellular protection mediated by IpDHN, several physiological indices, including RWC, IL, the proline (Pro) content, and MDA content, which are mainly related to cellular osmotic stress tolerance, were tested in WT and IpDHN transgenic Arabidopsis plants under salt and osmotic stress treatments. Compared to the WT Arabidopsis plants, the RWC and proline content was a little higher than those in transgenic lines when they were subjected to salt stress (200 mM NaCl, 1 day) or osmotic stress (300 mM mannitol, 1 day Figures 10A,B), which indicated that in transgenic lines, the cells had better water status and stress resistance than in the WT plants. IL is an important indicator of membrane injury and represents the integrity of cell biomembrane system. The IL value was higher in WT than in the transgenic lines, suggesting that the transgenic plants suffered less membrane damage than WT (Figure 10C). Accordingly, the MDA levels also displayed a pattern similar to IL, being lower in the transgenic lines relative to WT (Figure 10D), probably mediated by the cellular IpDHN’s accumulation. These physiological indices indicated that the transgenic lines were more resistant to salt and drought stresses, with an indication of the IpDHN protection roles for the cellular membrane system, even for cell vitality under osmotic stresses.
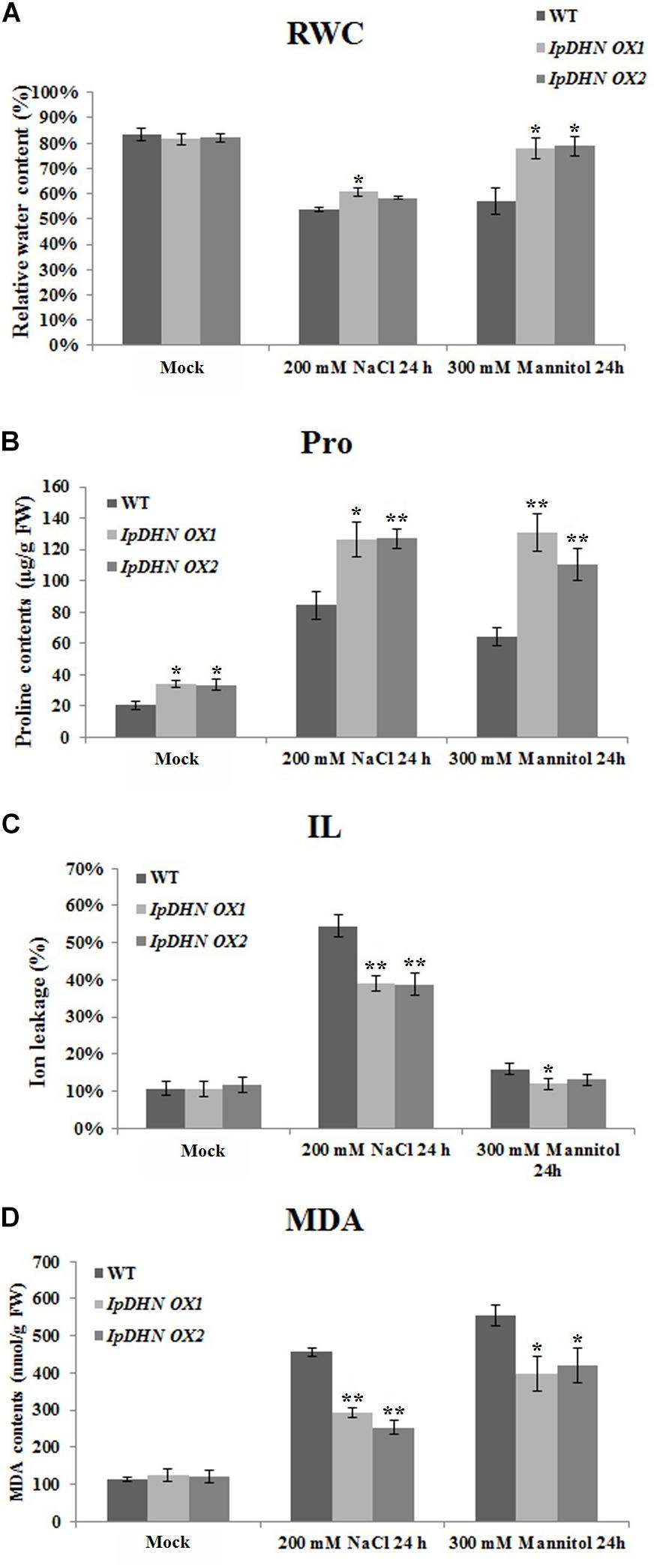
FIGURE 10. Changes in physiological parameters of IpDHN overexpressing Arabidopsis and WT seedlings (4 weeks) under 200 mM NaCl and 200 mM mannitol treatments for 24 h. (A) RWC; (B) free proline content; (C) IL; (D) MDA. All determinations were carried out on three biological replicates. Error bars indicate the SD based on three replicates. Asterisks indicate significant differences from the WT (control, Student’s t-test P-values, ∗p < 0.05, ∗∗p < 0.01).
IpDHN Transgenic Overexpressing Arabidopsis Resist Oxidative Damage by Increasing ROS Scavenger Accumulation
From Figure 10, we can see that the proline content (Figure 10A) and MDA content (Figure 10C) showed obvious differences between the WT and IpDHN overexpression plants, which means that the cells of the IpDHN transgenic plants showed stronger activity and better resistance to osmotic stresses. The proline and MDA contents are also important indices of cellular oxidative stress, as well as cell injury in response to stresses (Ben Rejeb et al., 2014; Yuan et al., 2017). Abiotic stresses, such as drought, cold, salt and heat, could cause increases in the levels of ROS in plant in vivo, which subsequently result in a reduction of plant growth and even loss of crop yield worldwide (You and Chan, 2015). When plant cells are subjected to stresses, proline can act as an osmolyte as well as a ROS scavenger (Ben Rejeb et al., 2014), whereas the accumulation of MDA is often the final product of membrane lipid peroxidation caused by ROS accumulation. In other words, the differences in the physiological indices between the WT and IpDHN transgenic plants are eventually reflected in the cellular ROS accumulation and scavenging caused by osmotic stresses. Hence, it was necessary to explore ROS accumulation in the IpDHN transgenic lines and WT. From Figure 11, we can see that the H2O2 and O2− contents increased in both the WT and IpDHN transgenic lines after salt and mannitol (osmotic) stresses. The DAB (Figure 11A) and NBT (Figure 11B) staining assays indicated that the two transgenic line plants contained less H2O2 and O2− than WT. Some enzymatic antioxidants, such as CAT and SOD, might play significant roles in ROS scavenging and may influence the cellular ROS balance. In our research, the activities of two significant antioxidant enzymes (SOD and CAT) were measured in the leaves of potted plants. Under normal growth conditions, the IpDHN transgenic lines and WT plants showed no apparent difference in SOD activity, while the CAT activity was slightly higher in the IpDHN transgenic lines than in the WT. However, the SOD and CAT activities were significantly higher in the IpDHN transgenic lines than in the WT plants (Figures 11C,D). These results suggested that overexpression of IpDHN reduced ROS accumulation, probably by the protection roles to basal metabolisms of IpDHN’s accumulation, and then by some means enhancing the cellular SOD and CAT activities under salt/dehydration stresses, which eventually can help to lessen the poisonous effect of ROS.
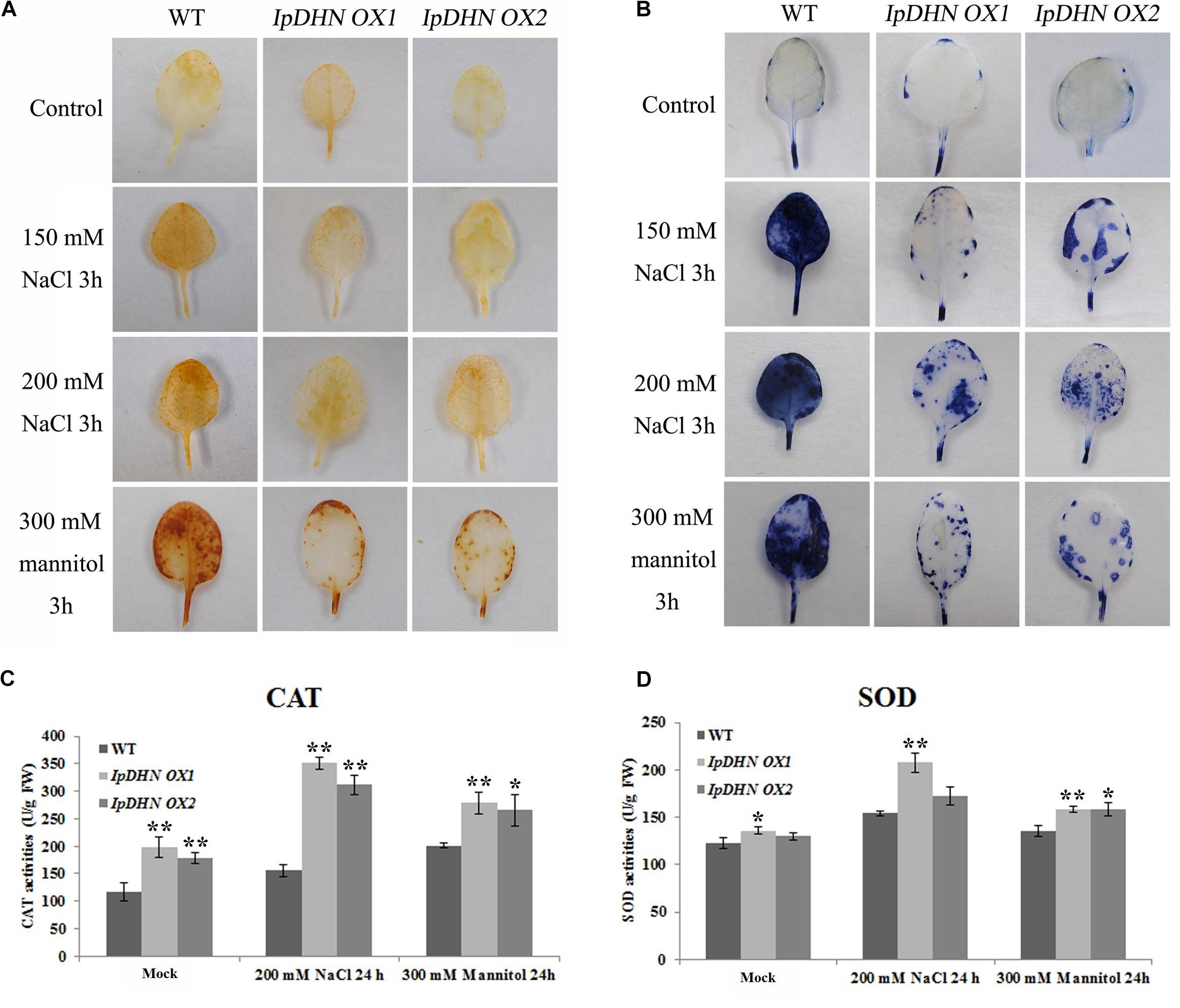
FIGURE 11. Oxidative stress analyses of transgenic lines and WT plants. Histochemical staining assays were used to detect H2O2 and O2− by DAB (A) or NBT (B) staining, respectively. (C) Analysis of CAT activities in the WT and transgenic lines under normal conditions and osmotic stresses. (D) Analysis of SOD activities in the WT and transgenic lines under normal conditions and osmotic stresses. Error bars indicate the SD based on three replicates. Asterisks indicate significant differences from the WT (control, Student’s t-test P-values, ∗p < 0.05, ∗∗p < 0.01).
Overexpression of IpDHN Induces Expression of Stress-Responsive Genes
To explore the molecular mechanisms underlying the biological function of IpDHN in salt and drought resistance in plants, the expression levels of a number of antioxidative and water deficit-responsive genes in the IpDHN transgenic lines and WT plants subjected to 200 mM mannitol and 200 mM NaCl treatments were analyzed by quantitative RT-PCR (Figure 12). The four oxidative stress-responsive marker genes (CAT1, FSD1, CSD1, and APX2) examined in Arabidopsis showed significantly upregulated transcription in the WT and IpDHN transgenic plants under stress conditions (Figure 12A). Moreover, the other eight drought/dehydration or ABA responsive genes (NCED3, HAI2, RD29A, RD29B, HVA22D, ANAC19, RD22, and RD26) showed an upregulated expression pattern in IpDHN transgenic Arabidopsis compared with the WT plants. In addition, under osmotic stresses, these genes showed a greater increase of the induced expression pattern in IpDHN transgenic Arabidopsis compared with the WT plants (Figure 12B). This result suggested that IpDHN accumulation in plants might up-regulate the expression of these abiotic stress-related genes, and then these upstream regulatory genes can improve plant resistance to salt and drought stresses in some way.
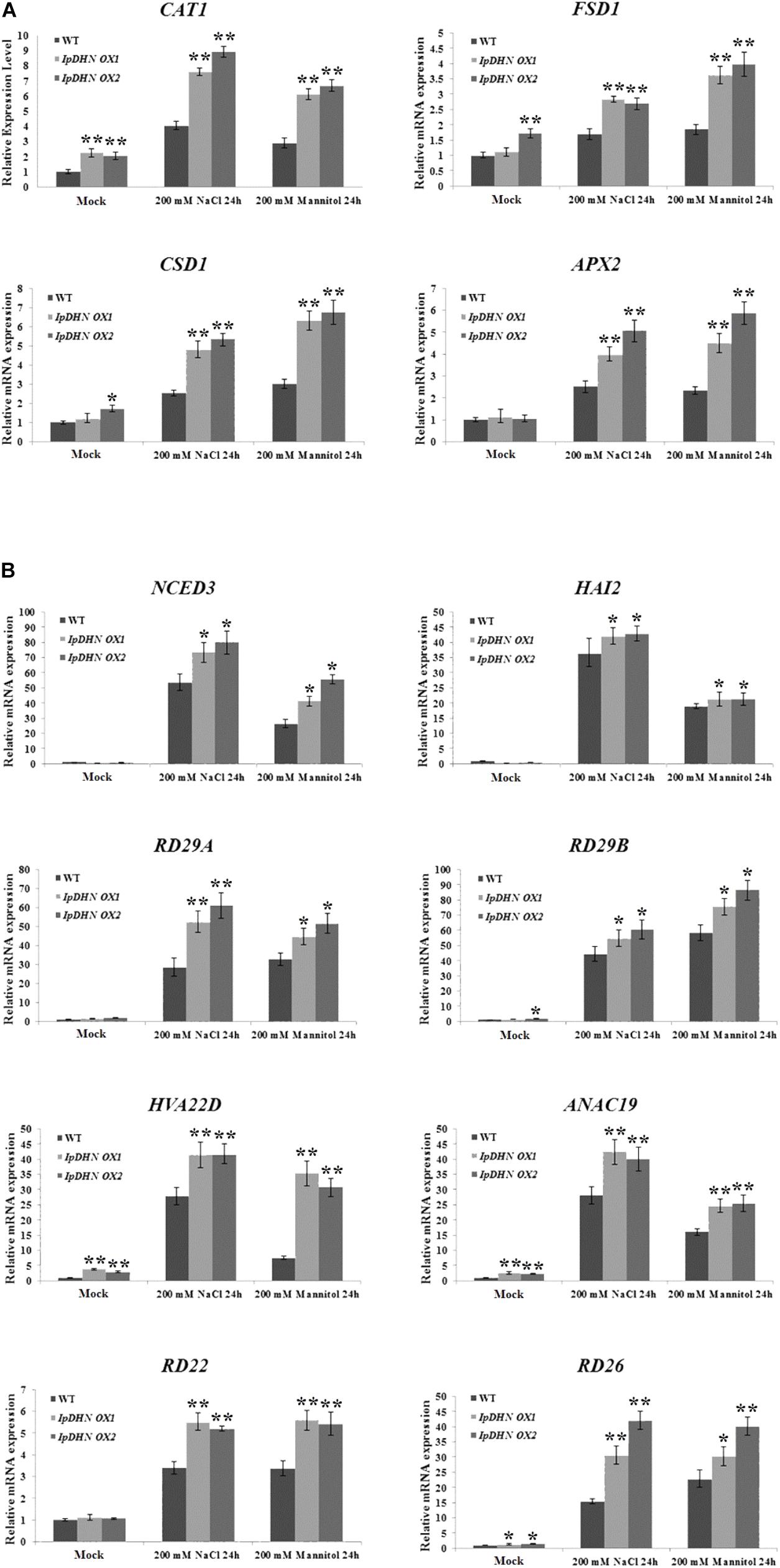
FIGURE 12. Analysis of expression levels of ROS-related and stress-responsive genes in the WT and the transgenic line by qRT-PCR under normal and osmotic conditions. (A) CAT1, FSD1, CSD1, and APX2; (B) NCED3, HAI2, RD29A, RD29B, HVA22D, ANAC19, RD22, and RD26. Error bars indicate the SD based on three replicates. Asterisks indicate significant differences from the WT (control, Student’s t-test P-values, ∗p < 0.05, ∗∗p < 0.01).
Discussion
In plants, DHNs function in various pathways, conferring water-deficit stress tolerance. Plant DHN cDNAs were first identified in barley and corn seedlings undergoing dehydration treatment, with induced expression pattern under ABA and salt treatment (Close et al., 1989). Subsequently, a series of plant DHNs have been reported. Plant DHNs that have been functionally characterized and identified are involved in responses to abiotic stresses, not only in the model plant Arabidopsis and crops (Hara, 2010; Eriksson et al., 2011; Hanin et al., 2011; Kosová et al., 2014), but also in some wild plants, especially those with tolerance to extremely adverse conditions, such as halophytes (Ayarpadikannan et al., 2012; Yamamoto et al., 2015), drought-enduring plants (Yang et al., 2014; Chiappetta et al., 2015), resurrection plants (Giarola et al., 2015), and hardy plants (Ochoa-Alfaro et al., 2012; Bao et al., 2017; Guo et al., 2017). In this study, a salt-stress related dehydrin from the halophyte I. pes-caprae, named IpDHN, was identified and characterized to explore the possible mechanisms of I. pes-caprae response to extreme salt and drought stresses and to further explore the potential implication of IpDHN in areas of genetic breeding in improving the performance of plants under stress.
Environmental stress, especially high salt stress, is an important cause of poor plant growth and crop reduction. At present, numerous studies have focused on the functional genes that play pivotal roles in salt tolerance and have committed to elucidating the genetic and molecular bases of improving plant resistance; consequently, some truly useful or positive genetic resources for salt stress have been discovered (Ventura et al., 2015). In this study, the multifunctionality of IpDHN was explored based on sequence analyses, gene expression patterns, promoter isolation, transgenic overexpression assay, and other physiological and biochemical tests. This is the first report of the characterization of an abiotic stress tolerance dehydrin from the extreme halophyte I. pes-caprae.
Dehydrins are a class of high hydrophilic proteins that often responding to water-deficit stresses, such as freezing, drought, and high salinity. Sequence analysis indicated that IpDHN shares typical DHN motifs, including an S-segment and three K-segments, similar to the SKn-type DHNs of other plant species (Hara, 2010) (Supplementary Figure S2B). IpDHN showed high amino acid identity with the banana DHN MusaDHN-1 (Supplementary Figure S2B and Figure 1), which was reported as an osmotic stress-tolerance protein, and when overexpressing MusaDHN-1 in transgenic banana plants, the plants showed superior performance under drought and salt stress conditions (Shekhawat et al., 2011). In Arabidopsis, there is a small subfamily of DHNs that belong to the LEA family (Alsheikh et al., 2005; Hara, 2010; Candat et al., 2014). Phylogenetic analysis indicated that IpDHN has close evolutionary relationships with ERD14 (At1g76180.1), COR47 (At1g20440.1), and ERD10 (At1g20450.1) (Figure 1). Previous studies showed that in Arabidopsis, both ERD14 and ERD10 accumulated under dehydration and salt treatments, while COR47 accumulated primarily in response to low temperature (Nylander et al., 2001; Kovacs et al., 2008). Several potential phosphorylation sites, including mainly the S-fragment and other serine/threonine sites, have been found in all three acidic DHNs (Alsheikh et al., 2005), and the phosphorylation status of DHNs in vivo would affect the ion-binding feature and even the biological role in plants (Riera et al., 2004; Hara, 2010). Here, in IpDHN, a typical S-segment containing 9 tandem serines was also found and implied that IpDHN has similar phosphorylation characteristics and biological functions involved in dehydration responses and ion-binding features (Supplementary Figure S2B). DHNs are highly hydrophilic, and in our research, analysis of amino acid constituents showed that IpDHN contained significant amounts of hydrophilic amino acids (Supplementary Table S2), further indicating the high hydrophily of IpDHN. The subcellular localization of IpDHN also seemed to have similar patterns to ERD14, ERD10, and COR47 based on the GFP fusion protein detection assay (Figure 4) (Shekhawat et al., 2011), which further implied that IpDHN had an approximate organelle distribution.
Expression profiles revealed that, similar to ERD14, ERD10, and COR47, IpDHN is expressed constitutively in all tissues (Nylander et al., 2001). In addition, IpDHN was significantly induced by multiple abiotic stresses, such as high salinity, dehydration, oxidative stress, and cold (Figure 5). Furthermore, IpDHN was also induced by ABA treatment, consistent with ERD14, ERD10, and COR47, suggesting that IpDHN participated in the response to abiotic stress possibly mediated by the ABA signaling pathway. The isolated promoter region of IpDHN contains several stress-related cis-elements, further revealing that the expression of IpDHN is responsive to multiple stresses.
It was previously reported that dehydrins exist widely in plants not only in halophytes or drought-endurance plants, which means that besides the biochemical features of DHN proteins per se, the gene expression regulation pattern might be an issue as crucial as the proteins. Halophytes have been considered to be more tolerant of abiotic stress due to their high and differential regulation patterns of the similar basic set of stress-responsive genes present among all plants (Glenn et al., 1999; Mishra and Tanna, 2017). The promoter region, especially the transcriptional regulatory elements detected by some transcription factors, should be critical entry points to understand the transcriptional regulatory mechanism and gene expression pattern. Since halophytes have highly developed biochemical tolerance mechanisms to high salinity (Glenn et al., 1999), the halophytic promoters have emerged as a promising and powerful candidate for plant genetic engineering about abiotic stress tolerance in crops for the regulable or high-level expression of transgenes (Mishra and Tanna, 2017). Recently, some studies on cis-elements of stress-responsive genes’ promotors from halophytes have been reported. A putative promoter region (1,023 bp) of the SbGSTU gene from Salicornia brachiata showed salinity and dehydration-induced activity in transgenic tobacco (Tiwari et al., 2016). The promoter of the AlSAP gene from the halophyte grass Aeluropus littoralis also showed a stress-inducible expression pattern in transgenic rice (Ben-Saad et al., 2015). A promoter region of the SNARE gene (SbSLSP) from S. brachiata was cloned, and sequence analyses indicated it had several cis-regulatory motifs related to abiotic stress; the expression of SbSLSP seemed to be up-regulated upon salinity and dehydration stresses (Singh et al., 2016). In the current study, the cloned IpDHN promoter region (974 bp) contained several potential cis-acting elements that respond to environmental stress, such as ABRE, MBS, and TC-rich repeats (Figure 2). In addition, the expression of IpDHN in I. pes-caprae was also regulated by abiotic stresses and ABA (Figure 5). The ABA responsive element (ABRE) is a key cis element in ABA signaling (Hobo et al., 1999), and has been reported broadly involving in ABA signaling and osmotic stress (Mishra et al., 2014; Watanabe et al., 2017). Furthermore, a designed synthetic promoter containing tandem ABREs has been proved having inducible response of exogenous ABA and stresses with tissue-specific patterns, and has the potential application and contribute to developmental and environmental control of specific gene expression in specific organs (Wu et al., 2018). The myb-binding site (MBS) cis element has been known to be important for plant ABA signaling and stress response, especially responding to drought inducibility (Urao et al., 1993; Abe et al., 2003). Here our qRT-PCR analysis in I. pes-caprae seedlings indicated that IpDHN was dramatically induced by salt, mannitol and ABA, and we speculate that this putative IpDHN promoter may be a stress-responsive promoter. Considering the less obvious difference of amino acid sequence between the IpDHN (DHN from halophyte I. pes-caprae) and InDHN (DHN from glycophyte Ipomoea nil) (Supplementary Figure S2B), here we can conclude that the promoter region of IpDHN might play a bigger part in I. pes-caprae adapting salt/drought environment. Accordingly, this promoter’s biological features can be verified by promoter-driven GUS expression transgenic assay in Arabidopsis.
As single-celled organisms, yeast and E. coli have been used as tools to study gene functions in multicellular organisms, especially with respect to plant osmotic stress tolerance (Zhu et al., 1997; Dang et al., 2014; Saucedo et al., 2017). IpDHN cDNA was isolated by a cDNA library screening assay with a yeast salt sensitive mutant, AXT3 (data not published), with the purpose of characterizing the salt-tolerance molecular mechanism of I. pes-caprae. In our study, because of the advantages of protein induction in E. coli, we also checked whether the accumulation of IpDHN in E. coli cells could enhance the abiotic tolerance of transgenic E. coli strains. To date, many previous reports have focused primarily on the major groups of LEA protein (including DHNs) involved in osmotic stresses that have been examined in E. coli (Ling et al., 2016; Saucedo et al., 2017). Arctic Cerastium arcticum is a hardy plant, and Kim et al. (2013) reported that a C. arcticum DHN CaDHN gene enhanced the tolerance of yeast to oxidants, osmotic stresses, and metal toxicity. Another study conducted on the hardy plant Prunus mume showed that five dehydrin genes from P. mume (PmLEA8, PmLEA10, PmLEA19, PmLEA20, and PmLEA29) could enhance E. coli tolerance to salt and sorbitol stresses (Bao et al., 2017). A Y3SK2-type dehydrin gene from cucumber (Cucumis sativus), CsLEA11, could also enhance tolerance to high or low temperature when overexpressed in E. coli (Zhou et al., 2017). Our research demonstrated that GST-IpDHN can be easily and obviously induced in E. coli BL21 (Figure 3A), and the spot assay, single clone counting assay, and cell growth curves of E. coli showed that IpDHN could significantly enhance salt/drought and sorbitol tolerance of E. coli cells (Figure 3B and Supplementary Figures S4A,B).
Some reports indicated that DHNs can directly scavenge free ROS due to their high percentages of amino acid residues, such as glycine, histidine, and lysine (Dean et al., 1997; Hanin et al., 2011), or can bind to metals to prevent excessive ROS formation in intracellular compounds (Hanin et al., 2011). In a previous study, we found that IpDHN could partly rescue the H2O2 sensitivity of the yeast mutant strains yap1Δ and skn7Δ to H2O2, as well as elevate the salt tolerance of AXT3 and W303 (Supplementary Figure S1). A similar assay for improved H2O2 tolerance was also performed using the E. coli expression system. Herein, the accumulation of GST-IpDHN proteins obviously increased the tolerance of E. coli to H2O2 (Figure 3B), which further demonstrates that IpDHN has antioxidative ability.
Cellular ROS accumulation as a result of stress is a widespread phenomenon in plants (You and Chan, 2015), and this research provided evidence that IpDHN could be involved in ROS scavenging (Figures 3B, 11 and Supplementary Figures S1, S4A) and could thereby improve organism’s tolerance to environmental stresses, such as dehydration, high salinity, and oxidative stresses. The excessive accumulation of IpDHN in cells maintains not only the water status under stress conditions (Figures 7–9), but also directly scavenges ROS for cellular detoxification (Figures 11A,B). Furthermore, it can be hypothesized that IpDHN acts as a molecular chaperone to increase the activity of protective enzymes (Figures 11C,D) or to activate some transcriptional processes to up-regulate the expression of stress-responsive genes, including some antioxidant enzyme system genes and other stress-responsive genes (Figures 12A,B).
There is increasing evidence that plant DHNs can scavenge ROS generated from cellular metabolism, thereby increasing the ability to cope with abiotic stresses based on a plant transgenic heterologous overexpression assay (Liu et al., 2015; Saibi et al., 2015; Cao et al., 2017; Li et al., 2017; Halder et al., 2018). Generally, the cellular accumulation of DHNs has pleiotropic effects on increasing plant tolerance to abiotic stresses, not only by binding directly to water molecules, ROS, transition metals, membrane lipids, or cellular skeletons to maintain the basic cellular metabolism, but also by binding to proteins, such as enzymes and transcription factors, and serving as molecular chaperones to maintain the basic activities of these functional proteins, thereby producing a positive influence on cell livability under abiotic stresses. In this study, a series of transgenic assays (including yeast, E. coli, and plant) were performed with overexpressed IpDHN, and the results indicated that the cellular accumulation of IpDHN indeed improved the tolerance to salt and drought stresses, possibly by activating the oxygen scavenging system.
A physiological assessment showed that IpDHN-overexpressed Arabidopsis plants showed better indicators than the wild-type plants, which is related to better salt/drought tolerance in transgenic Arabidopsis than in the WT plants. RWC is a relevant tool for the measurement of plant osmotic stress tolerance. The value of RWC in transgenic Arabidopsis was slightly higher than in WT when they were subjected NaCl or mannitol stresses (Figure 10A). Cellular accumulation of free proline is frequently observed during the challenge of plants to adverse environmental and stresses (Ben Rejeb et al., 2014). Proline acts as both an osmoprotectant and a potent non-enzymatic antioxidant, thereby maintaining cell viability and preventing oxidative damage caused by ROS. A higher accumulation of proline was measured in the IpDHN overexpression lines (Figure 10B), indicating that under osmotic stress challenges, IpDHN overexpression provided better protection by regulating proline metabolism to maintain the growth of plants. As a significant indicator of cellular membrane injury (Dexter et al., 1932), the IL value was significantly higher in the WT than in transgenic lines, suggesting that the transgenic plants suffered less membrane damage than the WT (Figure 10C). MDA is a final product of lipid peroxidation caused by ROS and is therefore a key indicator of osmotic stress injury in plants (Moore and Roberts, 1998). Thus, our research implied that lipid peroxidation caused by accumulation of ROS was somewhat alleviated in transgenic plants under osmotic stresses (Figure 10D).
As we can see from Figure 11, transgenic overexpression lines (IpDHN OX1 and IpDHN OX2) accumulated a lower level of H2O2 (Figure 11A) and O2− (Figure 11B) under drought/osmotic stress compared to the WT, which demonstrates that the ROS scavenging systems might be more effective in IpDHN transgenic plants than in WT. The activities of two antioxidant-related enzymes were measured in this research. In general, SOD and CAT activities were significantly higher in the transgenic than in the WT plants, and by combining the quantitative RT-PCR analyses results of CAT1 and CSD1 in Arabidopsis (Figure 12A), we can draw a preliminary conclusion that, by means of some pleiotropic effects, the overexpression of IpDHN in plants can significantly improve tolerance to salt/drought stresses, probably by mediating ROS scavenging, together with the regulation of gene expression and proteins or enzymes activities.
Conclusion
In summary, here we reported the isolation and characterization of a DHN gene, IpDHN, from the halophyte I. pes-caprae. Our research showed that IpDHN belongs to an SK3 type DHN. Expression of IpDHN in I. pes-caprae is induced by mannitol, salt, MV, cold, and ABA. Analysis of the promoter of IpDHN identified cis-elements involved in growth, developmental processes, and a variety of stress responses. Overexpression of IpDHN in microorganisms and Arabidopsis displayed complex patterns involved in abiotic stresses, including mainly salt and drought tolerance. In Arabidopsis, the overexpression of IpDHN increased the resistance of transgenic plants to high salinity and drought probably by changing the accumulation of several physiological parameters and by promoting the expression of some key genes in the osmotic stress pathway, ROS scavenging system, and drought-responsive pathway. These findings indicated that IpDHN acts as a downstream effect factor in plant response to high salinity and drought stresses and may be a promising candidate gene for genetic breeding of salt-tolerant plants. In summary, our research confirmed the significant value of the continued investigation into the function and mechanisms of the IpDHN gene in I. pes-caprae for the further development of stress-tolerant crops in genetic breeding.
Author Contributions
MZ, KX, and SJ conceived the study and designed the experiments. MZ, HZ, JZ, and HS performed the experiments. HZ and JZ analyzed the data with suggestions by MZ. MZ drafted and revised the manuscript. All authors read and approved the final manuscript.
Funding
This research was supported by grants from the ‘Strategic Priority Research Program’ of the Chinese Academy of Sciences (XDA13020500), the National Key Research and Development Program of China (2016YFC1403002), and “the Twelfth Five-Year” National S&T Projects for Rural Areas (2015BAL04B04). The funders had no roles in the study design, data collection and analysis, decision to publish, or preparation of the manuscript.
Conflict of Interest Statement
The authors declare that the research was conducted in the absence of any commercial or financial relationships that could be construed as a potential conflict of interest.
Supplementary Material
The Supplementary Material for this article can be found online at: https://www.frontiersin.org/articles/10.3389/fpls.2018.01454/full#supplementary-material
Abbreviations
ABRE, ABA responding elements; CAT, catalase; cDNA, complementary DNA; CDS, coding cDNA sequence; DHN, dehydrin; ERD, early responsive to dehydration; GFP, green fluorescent protein; IDP, intrinsically disordered proteins; IL, ion leakage; LEA, late embryogenesis abundant; MBS, MYB binding site; MDA, malondialdehyde; MS, Murashige and Skoog; ORF, open reading frame; OX, overexpression; PCR, polymerase chain reaction; qRT-PCR, quantitative reverse transcription polymerase chain reaction; ROS, reactive oxygen species; RWC, relative water content; SOD, superoxide dismutase; WT, wild type.
Footnotes
- ^ https://www.ncbi.nlm.nih.gov/orffinder/
- ^ http://prosite.expasy.org/
- ^ http://www.sbg.bio.ic.ac.uk/phyre2/html/page.cgi?id=index
- ^ http://clustalw.ddbj.nig.ac.jp/
- ^ http://bioinformatics.psb.ugent.be/webtools/plantcare/html/
- ^ http://bioinformatics.psb.ugent.be/webtools/plantcare/html/
References
Abe, H., Urao, T., Ito, T., Seki, M., Shinozaki, K., and Yamaguchi-Shinozaki, K. (2003). Arabidopsis AtMYC2 (bHLH) and AtMYB2 (MYB) function as transcriptional activators in abscisic acid signaling. Plant Cell 15, 63–78. doi: 10.1105/tpc.006130
Alsheikh, M. K., Svensson, J. T., and Randall, S. K. (2005). Phosphorylation regulated ion-binding is a property shared by the acidic subclass dehydrins. Plant Cell Environ. 28, 1114–1122. doi: 10.1111/j.1365-3040.2005.01348.x
Ayarpadikannan, S., Chung, E., Cho, C. W., So, H. A., Kim, S. O., Jeon, J. M., et al. (2012). Exploration for the salt stress tolerance genes from a salt-treated halophyte, Suaeda asparagoides. Plant Cell Rep. 31, 35–48. doi: 10.1007/s00299-011-1137-4
Bao, F., Du, D., An, Y., Yang, W., Wang, J., Cheng, T., et al. (2017). Overexpression of Prunus mume dehydrin genes in tobacco enhances tolerance to cold and drought. Front. Plant Sci. 8:151. doi: 10.3389/fpls.2017.00151
Ben Rejeb, K., Abdelly, C., and Savouré, A. (2014). How reactive oxygen species and proline face stress together. Plant Physiol. Biochem. 80, 278–284. doi: 10.1016/j.plaphy.2014.04.007
Ben-Saad, R., Meynard, D., Ben-Romdhane, W., Mieulet, D., Verdeil, J. L., Al-Doss, A., et al. (2015). The promoter of the AlSAP gene from the halophyte grass Aeluropus littoralis directs a stress-inducible expression pattern in transgenic rice plants. Plant Cell Rep. 34, 1791–1806. doi: 10.1007/s00299-015-1825-6
Candat, A., Paszkiewicz, G., Neveu, M., Gautier, R., Logan, D. C., Avelange-Macherel, M. H., et al. (2014). The ubiquitous distribution of late embryogenesis abundant proteins across cell compartments in Arabidopsis offers tailored protection against abiotic stress. Plant Cell 26, 3148–3166. doi: 10.1105/tpc.114.127316
Cao, Y., Xiang, X., Geng, M., You, Q., and Huang, X. (2017). Effect of HbDHN1 and HbDHN2 genes on abiotic stress responses in Arabidopsis. Front. Plant Sci. 8:470. doi: 10.3389/fpls.2017.00470
Chiappetta, A., Muto, A., Bruno, L., Woloszynska, M., Van Lijsebettens, M., and Bitonti, M. B. (2015). A dehydrin gene isolated from feral olive enhances drought tolerance in Arabidopsis transgenic plants. Front. Plant Sci. 6:392. doi: 10.3389/fpls.2015.00392
Close, T. J. (1997). Dehydrins: a commonality in the response of plants to dehydration and low temperature. Physiol. Plant. 100, 291–296. doi: 10.1111/j.1399-3054.1997.tb04785.x
Close, T. J., Kortt, A. A., and Chandler, P. M. (1989). A cDNA-based comparison of dehydration-induced proteins (dehydrins) in barley and corn. Plant Mol. Biol. 13, 95–108.
Cuevas-Velazquez, C. L., Rendón-Luna, D. F., and Covarrubias, A. A. (2014). Dissecting the cryoprotection mechanisms for dehydrins. Front. Plant Sci. 5:583. doi: 10.3389/fpls.2014.00583
Dang, N. X., Popova, A. V., Hundertmark, M., and Hincha, D. K. (2014). Functional characterization of selected LEA proteins from Arabidopsis thaliana in yeast and in vitro. Planta 240, 325–336. doi: 10.1007/s00425-014-2089-z
Dean, R. T., Fu, S., Stocker, R., and Davies, M. J. (1997). Biochemistry and pathology of radical-mediated protein oxidation. Biochem. J. 324(Pt 1), 1–18.
Dexter, S. T., Tottingham, W., and Graber, L. (1932). Investigations of the hardiness of plants by measurement of electrical conductivity. Plant Physiol. 7, 63–78. doi: 10.1104/pp.7.1.63
Drira, M., Saibi, W., Brini, F., Gargouri, A., Masmoudi, K., and Hanin, M. (2013). The K-segments of the wheat dehydrin DHN-5 are essential for the protection of lactate dehydrogenase and β-glucosidase activities in vitro. Mol. Biotechnol. 54, 643–650. doi: 10.1007/s12033-012-9606-8
Eriksson, S. K., Kutzer, M., Procek, J., Gröbner, G., and Harryson, P. (2011). Tunable membrane binding of the intrinsically disordered dehydrin Lti30, a cold-induced plant stress protein. Plant Cell 23, 2391–2404. doi: 10.1105/tpc.111.085183
Giarola, V., Challabathula, D., and Bartels, D. (2015). Quantification of expression of dehydrin isoforms in the desiccation tolerant plant Craterostigma plantagineum using specifically designed reference genes. Plant Sci. 236,103–115. doi: 10.1016/j.plantsci.2015.03.014
Glenn, E. P., Brown, J. J., and Blumwald, E. (1999). Salt tolerance and crop potential of halophytes. Crit. Rev. Plant Sci. 18, 227–255. doi: 10.1080/07352689991309207
Graether, S. P., and Boddington, K. F. (2014). Disorder and function: a review of the dehydrin protein family. Front. Plant Sci. 5:576. doi: 10.3389/fpls.2014.00576
Guo, X., Zhang, L., Zhu, J., Liu, H., and Wang, A. (2017). Cloning and characterization of SiDHN, a novel dehydrin gene from Saussurea involucrata Kar. et Kir. that enhances cold and drought tolerance in tobacco. Plant Sci. 256, 160–169. doi: 10.1016/j.plantsci.2016.12.007
Halder, T., Upadhyaya, G., Basak, C., Das, A., Chakraborty, C., and Ray, S. (2018). Dehydrins impart protection against oxidative stress in transgenic tobacco plants. Front. Plant Sci. 9:136. doi: 10.3389/fpls.2018.00136
Halder, T., Upadhyaya, G., and Ray, S. (2017). YSK2 type dehydrin (SbDhn1) from sorghum bicolor showed improved protection under high temperature and osmotic stress condition. Front. Plant Sci. 8:918. doi: 10.3389/fpls.2017.00918
Hand, S. C., Menze, M. A., Toner, M., Boswell, L., and Moore, D. (2011). LEA proteins during water stress: not just for plants anymore. Annu. Rev. Physiol. 73, 115–134. doi: 10.1146/annurev-physiol-012110-142203
Hanin, M., Brini, F., Ebel, C., Toda, Y., Takeda, S., and Masmoudi, K. (2011). Plant dehydrins and stress tolerance: versatile proteins for complex mechanisms. Plant Signal. Behav. 6, 1503–1509. doi: 10.4161/psb.6.10.17088
Hara, M. (2010). The multifunctionality of dehydrins: an overview. Plant Signal. Behav. 5, 503–508. doi: 10.4161/psb.11085
Hobo, T., Asada, M., Kowyama, Y., and Hattori, T. (1999). ACGT-containing abscisic acid response element (ABRE) and coupling element 3 (CE3) are functionally equivalent. Plant J. 19, 679–689.
Hu, W., Huang, C., Deng, X., Zhou, S., Chen, L., Li, Y., et al. (2013). TaASR1, a transcription factor gene in wheat, confers drought stress tolerance in transgenic tobacco. Plant Cell Environ. 36, 1449–1464. doi: 10.1111/pce.12074
Kim, I. S., Kim, H. Y., Kim, Y. S., Choi, H. G., Kang, S. H., and Yoon, H. S. (2013). Expression of dehydrin gene from arctic Cerastium arcticum increases abiotic stress tolerance and enhances the fermentation capacity of a genetically engineered Saccharomyces cerevisiae laboratory strain. Appl. Microbiol. Biotechnol. 97, 8997–9009. doi: 10.1007/s00253-013-4729-9
Koag, M. C., Wilkens, S., Fenton, R. D., Resnik, J., Vo, E., and Close, T. J. (2009). The K-segment of maize DHN1 mediates binding to anionic phospholipid vesicles and concomitant structural changes. Plant Physiol. 150, 1503–1514. doi: 10.1104/pp.109.136697
Kosová, K., Vítámvás, P., and Prášil, I. T. (2014). Wheat and barley dehydrins under cold, drought, and salinity-what can LEA-II proteins tell us about plant stress response? Front. Plant Sci. 5:343. doi: 10.3389/fpls.2014.00343
Kovacs, D., Kalmar, E., Torok, Z., and Tompa, P. (2008). Chaperone activity of ERD10 and ERD14, two disordered stress-related plant proteins. Plant Physiol. 147, 381–390. doi: 10.1104/pp.108.118208
Kumari, A., Das, P., Parida, A. K., and Agarwal, P. K. (2015). Proteomics, metabolomics, and ionomics perspectives of salinity tolerance in halophytes. Front. Plant Sci. 6:537. doi: 10.3389/fpls.2015.00537
Lescot, M., Déhais, P., Thijs, G., Marchal, K., Moreau, Y., Van de Peer, Y., et al. (2002). PlantCARE, a database of plant cis-acting regulatory elements and a portal to tools for in silico analysis of promoter sequences. Nucleic Acids Res. 30, 325–327.
Li, Q., Zhang, X., Lv, Q., Zhu, D., Qiu, T., Xu, Y., et al. (2017). Physcomitrella Patens dehydrins (PpDHNA and PpDHNC) confer salinity and drought tolerance to transgenic Arabidopsis plants. Front. Plant Sci. 8:1316. doi: 10.3389/fpls.2017.01316
Ling, H., Zeng, X., and Guo, S. (2016). Functional insights into the late embryogenesis abundant (LEA) protein family from Dendrobium officinale (Orchidaceae) using an Escherichia coli system. Sci. Rep. 6:39693. doi: 10.1038/srep39693
Liu, H., Yu, C., Li, H., Ouyang, B., Wang, T., Zhang, J., et al. (2015). Overexpression of ShDHN, a dehydrin gene from Solanum habrochaites enhances tolerance to multiple abiotic stresses in tomato. Plant Sci. 231, 198–211. doi: 10.1016/j.plantsci.2014.12.006
Liu, Y., Song, Q., Li, D., Yang, X., and Li, D. (2017). Multifunctional roles of plant dehydrins in response to environmental stresses. Front. Plant Sci. 8:1018. doi: 10.3389/fpls.2017.01018
Lv, A., Fan, N., Xie, J., Yuan, S., An, Y., and Zhou, P. (2017). Expression of CdDHN4, a novel YSK2-type dehydrin gene from bermudagrass, responses to drought stress through the ABA-dependent signal pathway. Front. Plant Sci. 8:748. doi: 10.3389/fpls.2017.00748
Miryeganeh, M., Takayama, K., Tateishi, Y., and Kajita, T. (2014). Long-distance dispersal by sea-drifted seeds has maintained the global distribution of Ipomoea pes-caprae subsp. brasiliensis (Convolvulaceae). PLoS One 9:e91836. doi: 10.1371/journal.pone.0091836
Mishra, A., and Tanna, B. (2017). Halophytes: potential resources for salt stress tolerance genes and promoters. Front. Plant Sci. 8:829. doi: 10.3389/fpls.2017.00829
Mishra, S., Shukla, A., Upadhyay, S., Sanchita, Sharma, P., Singh, S., et al. (2014). Identification, occurrence, and validation of DRE and ABRE Cis-regulatory motifs in the promoter regions of genes of Arabidopsis thaliana. J. Integr. Plant Biol. 56, 388–399. doi: 10.1111/jipb.12149
Moore, K., and Roberts, L. J. (1998). Measurement of lipid peroxidation. Free Radic. Res. 28, 659–671.
Nylander, M., Svensson, J., Palva, E. T., and Welin, B. V. (2001). Stress-induced accumulation and tissue-specific localization of dehydrins in Arabidopsis thaliana. Plant Mol. Biol. 45, 263–279.
Ochoa-Alfaro, A. E., Rodríguez-Kessler, M., Pérez-Morales, M. B., Delgado-Sánchez, P., Cuevas-Velazquez, C. L., Gómez-Anduro, G., et al. (2012). Functional characterization of an acidic SK3 dehydrin isolated from an Opuntia streptacantha cDNA library. Planta 235, 565–578. doi: 10.1007/s00425-011-1531-8
Ouyang, P. Y., Liu, N., Zhang, W. W., Wang, J., and Jian, S. G. (2011). Biological and ecophysiological characteristics of a beach plant Ipomoea pes-caprae. J. Hunan Univ. Sci. Technol. 26, 117–121.
Pierik, R., and Testerink, C. (2014). The art of being flexible: how to escape from shade, salt, and drought. Plant Physiol. 166, 5–22. doi: 10.1104/pp.114.239160
Qiu, H., Zhang, L., Liu, C., He, L., Wang, A., Liu, H. L., et al. (2014). Cloning and characterization of a novel dehydrin gene, SiDhn2, from Saussurea involucrata Kar. et Kir. Plant Mol. Biol. 84, 707–718. doi: 10.1007/s11103-013-0164-7
Riera, M., Figueras, M., López, C., Goday, A., and Pagès, M. (2004). Protein kinase CK2 modulates developmental functions of the abscisic acid responsive protein Rab17 from maize. Proc. Natl. Acad. Sci. U.S.A. 101, 9879–9884. doi: 10.1073/pnas.0306154101
Saibi, W., Feki, K., Ben Mahmoud, R., and Brini, F. (2015). Durum wheat dehydrin (DHN-5) confers salinity tolerance to transgenic Arabidopsis plants through the regulation of proline metabolism and ROS scavenging system. Planta 242, 1187–1194. doi: 10.1007/s00425-015-2351-z
Saucedo, A. L., Hernández-Domínguez, E. E., de Luna-Valdez, L. A., Guevara-García, A. A., Escobedo-Moratilla, A., Bojorquéz-Velázquez, E., et al. (2017). Insights on structure and function of a late embryogenesis abundant protein from Amaranthus curettes: an intrinsically disordered protein involved in protection against desiccation, oxidant conditions, and osmotic stress. Front. Plant Sci. 8:497. doi: 10.3389/fpls.2017.00497
Shekhawat, U. K., Srinivas, L., and Ganapathi, T. R. (2011). MusaDHN-1, a novel multiple stress-inducible SK3-type dehydrin gene, contributes affirmatively to drought- and salt-stress tolerance in banana. Planta 234, 915–932. doi: 10.1007/s00425-011-1455-3
Singh, D., Yadav, N. S., Tiwari, V., Agarwal, P. K., and Jha, B. (2016). A SNARE-like superfamily protein SbSLSP from the halophyte Salicornia brachiata confers salt and drought tolerance by maintaining membrane stability, K+/Na+ ratio, and antioxidant machinery. Front. Plant Sci. 7:737. doi: 10.3389/fpls.2016.00737
Sun, X., Rikkerink, E. H., Jones, W. T., and Uversky, V. N. (2013). Multifarious roles of intrinsic disorder in proteins illustrate its broad impact on plant biology. Plant Cell 25, 38–55. doi: 10.1105/tpc.112.106062
Tamura, K., Stecher, G., Peterson, D., Filipski, A., and Kumar, S. (2013). MEGA6: molecular evolutionary genetics analysis version 6.0. Mol. Biol. Evol. 30,2725–2729. doi: 10.1093/molbev/mst197
Tiwari, V., Patel, M. K., Chaturvedi, A. K., Mishra, A., and Jha, B. (2016). Functional characterization of the tau class glutathione-s-transferases gene (SbGSTU) Promoter of Salicornia brachiata under salinity and osmotic stress. PLoS One 11:e0148494. doi: 10.1371/journal.pone.0148494
Urao, T., Yamaguchi-Shinozaki, K., Urao, S., and Shinozaki, K. (1993). An Arabidopsis myb homolog is induced by dehydration stress and its gene product binds to the conserved MYB recognition sequence. Plant Cell 5, 1529–1539. doi: 10.1105/tpc.5.11.1529
Ventura, Y., Eshel, A., Pasternak, D., and Sagi, M. (2015). The development of halophyte-based agriculture: past and present. Ann. Bot. 115, 529–540. doi: 10.1093/aob/mcu173
Watanabe, K. A., Homayouni, A., Gu, L., Huang, K. Y., Ho, T. D., and Shen, Q. J. (2017). Transcriptomic analysis of rice aleurone cells identified a novel abscisic acid response element. Plant Cell Environ. 40, 2004–2016. doi: 10.1111/pce.13006
Wu, R., Duan, L., Pruneda-Paz, J. L., Oh, D. H., Pound, M., Kay, S., et al. (2018). The 6xABRE synthetic promoter enables the spatiotemporal analysis of ABA-mediated transcriptional regulation. Plant Physiol. 177, 1650–1665. doi: 10.1104/pp.18.00401
Xu, C., Tang, X., Shao, H., and Wang, H. (2016). Salinity tolerance mechanism of economic halophytes from physiological to molecular hierarchy for improving food quality. Curr. Genomics 17, 207–214. doi: 10.2174/1389202917666160202215548
Yamamoto, N., Takano, T., Tanaka, K., Ishige, T., Terashima, S., Endo, C., et al. (2015). Comprehensive analysis of transcriptome response to salinity stress in the halophytic turf grass Sporobolus virginicus. Front. Plant Sci. 6:241. doi: 10.3389/fpls.2015.00241
Yang, L. X., Wang, R. Y., Ren, F., Liu, J., Cheng, J., and Lu, Y. T. (2005). AtGLB1 enhances the tolerance of Arabidopsis to hydrogen peroxide stress. Plant Cell Physiol. 46, 1309–1316. doi: 10.1093/pcp/pci140
Yang, W., Zhang, L., Lv, H., Li, H., Zhang, Y., Xu, Y., et al. (2015). The K-segments of wheat dehydrin WZY2 are essential for its protective functions under temperature stress. Front. Plant Sci. 6:406. doi: 10.3389/fpls.2015.00406
Yang, Y., Sun, X., Yang, S., Li, X., and Yang, Y. (2014). Molecular cloning and characterization of a novel SK3-type dehydrin gene from Stipa purpurea. Biochem. Biophys. Res. Commun. 448, 145–150. doi: 10.1016/j.bbrc.2014.04.075
You, J., and Chan, Z. (2015). ROS regulation during abiotic stress responses in crop plants. Front. Plant Sci. 6:1092. doi: 10.3389/fpls.2015.01092
Yuan, H. M., Sheng, Y., Chen, W. J., Lu, Y. Q., Tang, X., Ou-Yang, M., et al. (2017). Overexpression of Hevea brasiliensis HbICE1 enhances cold tolerance in Arabidopsis. Front. Plant Sci. 8:1462. doi: 10.3389/fpls.2017.01462
Zhou, Y., He, P., Xu, Y., Liu, Q., Yang, Y., and Liu, S. (2017). Overexpression of CsLEA11, a Y3SK2-type dehydrin gene from cucumber (Cucumis sativus), enhances tolerance to heat and cold in Escherichia coli. AMB Express 7:182. doi: 10.1186/s13568-017-0483-1
Zhou, Y., Yin, X., Duan, R., Hao, G., Guo, J., and Jiang, X. (2015). SpAHA1 and SpSOS1 coordinate in transgenic yeast to improve salt tolerance. PLoS One 10:e0137447. doi: 10.1371/journal.pone.0137447
Keywords: Ipomoea pes-caprae L., dehydrin, salt, drought, promoter
Citation: Zhang H, Zheng J, Su H, Xia K, Jian S and Zhang M (2018) Molecular Cloning and Functional Characterization of the Dehydrin (IpDHN) Gene From Ipomoea pes-caprae. Front. Plant Sci. 9:1454. doi: 10.3389/fpls.2018.01454
Received: 06 June 2018; Accepted: 12 September 2018;
Published: 11 October 2018.
Edited by:
Melvin John Oliver, Agricultural Research Service (USDA), United StatesReviewed by:
Manoj Prasad, National Institute of Plant Genome Research (NIPGR), IndiaBrett Williams, Queensland University of Technology, Australia
Copyright © 2018 Zhang, Zheng, Su, Xia, Jian and Zhang. This is an open-access article distributed under the terms of the Creative Commons Attribution License (CC BY). The use, distribution or reproduction in other forums is permitted, provided the original author(s) and the copyright owner(s) are credited and that the original publication in this journal is cited, in accordance with accepted academic practice. No use, distribution or reproduction is permitted which does not comply with these terms.
*Correspondence: Mei Zhang, emhhbmdtZWlAc2NiZy5hYy5jbg==
†These authors have contributed equally to this work