- 1Centro de Estudios Fotosintéticos y Bioquímicos (CEFOBI-CONICET), Universidad Nacional de Rosario, Rosario, Argentina
- 2Institute of Developmental and Molecular Biology of Plants, Plant Molecular Physiology and Biotechnology Group, Heinrich-Heine-Universität, Cluster of Excellence on Plant Sciences, Düsseldorf, Germany
Arabidopsis thaliana possesses three cytosolic (NADP-ME1-3) and one plastidic (NADP-ME4) NADP-dependent malic enzymes. NADP-ME2 and -ME4 show constitutive expression, in contrast to NADP-ME1 and -ME3, which are restricted to particular tissues. Here, we show that NADP-ME1 transcript and protein were almost undetectable during normal vegetative growth, but gradually increased and reached levels higher than those of the other isoforms in the latest stages of seed development. Accordingly, in knockout nadp-me1 mature seeds the total NADP-ME activity was significantly lower than in wild type mature seeds. The phenotypic analysis of nadp-me1 plants indicated alterations of seed viability and germination. Besides, the treatment with abscisic acid (ABA), NaCl and mannitol specifically induced the accumulation of NADP-ME1 in seedlings. In line with this, nadp-me1 plants show a weaker response of primary and lateral root length and stomatal opening to the presence of ABA. The results suggest that NADP-ME1 plays a specialized role, linked to ABA signaling during the seed development as well as in the response to water deficit stress.
Introduction
NADP-dependent malic enzyme (NADP-ME; EC 1.1.1.40) catalyzes the oxidative decarboxylation of malate to generate pyruvate, CO2 and NADPH. In plants, the NADP-ME family is represented by several members, localized to cytosol and plastids. One of the better-established roles of this enzyme is the participation as malate decarboxylase in C4 and CAM photosynthesis (Drincovich et al., 2011; Saigo et al., 2013). Other functions are suggested based on the importance of malate balance for pH regulation, stomatal opening or lipogenesis (Laporte et al., 2002; Hurth et al., 2005; Gerrard Wheeler et al., 2016). Arabidopsis possesses three cytosolic (NADP-ME1-3) and one plastidic NADP-ME isoforms (NADP-ME4; AT1G79750) (Gerrard Wheeler et al., 2005). NADP-ME2 (AT5G11670) is responsible for most of the NADP-ME activity measured in mature organs and has been involved in sugar metabolism in veins (Brown et al., 2010) and in the oxidative burst triggered by hemibiotrophic fungal pathogen infection (Voll et al., 2012). NADP-ME3 (AT5G25880) is only found in trichomes and pollen (Gerrard Wheeler et al., 2005).
Regarding NADP-ME1 (AT2G19900), its expression is very low in seedlings and adult plants, but significantly higher levels were found in maturing seeds and roots (Gerrard Wheeler et al., 2005). This enzyme belongs to a particular phylogenetic group composed by NADP-MEs from different plant species formerly named as group IV in Gerrard Wheeler et al. (2005). In Zea mays, a similar NADP-ME that is also specific of embryo roots, has shown similar expression pattern and kinetic characteristics such as lower catalytic efficiency and activation by succinate (Detarsio et al., 2008; Gerrard Wheeler et al., 2008; Alvarez et al., 2013). Thus, the delimited localization and particular kinetic properties of NADP-ME1 probably reflect a particular role yet unknown. In a recent work based on transcriptome analysis and reverse genetics aimed at identifying differentially expressed genes during the imbibition and after-ripened seeds, NADP-ME1 was found up-regulated in dormant Arabidopsis genotypes (Yazdanpanah et al., 2017). The knockout mutant nadp-me1 showed disturbed seed traits compared to Col-0 plants (Yazdanpanah et al., 2017).
In this work, to disclose the biological role of A. thaliana NADP-ME1, we performed a deeper analysis of its expression pattern than the one performed in Gerrard Wheeler et al. (2005). In order to quantify and define the temporal and spatial NADP-ME1 expression, we employed reporter genes, measured the transcript accumulation and enzymatic activity and studied the promoter region. Besides, in order to identify the physiological processes in which NADP-ME1 may be involved, we analyzed phenotypic parameters throughout Arabidopsis plant life using nadp-me1 knockout plants, identifying those processes that are affected by the absence of NADP-ME1.
Here, we found that NADP-ME1 is the isoform that contributes the most to NADP-ME activity at mature seed stage. Abscisic acid (ABA), NaCl and mannitol treatments specifically induced the accumulation of NADP-ME1 in seedlings. nadp-me1 knockout mutant seeds are less sensitive than wild type (WT) seeds to ABA-mediated repression of the germination and they loss viability earlier than WT seeds. Besides, other targets of ABA signaling as stomata opening and roots architecture resulted altered in the nadp-me1. Overall, these findings indicate that there is a tight link of NADP-ME1 with processes related to ABA responses in seeds, roots and leaves.
Materials and Methods
Plant Lines, Growing Conditions and Sampling
Arabidopsis thaliana Columbia-0 lines analyzed in this work include homozygous knockout mutants with T-DNA inserted into the genes encoding NADP-ME1 (nadp-me1; SALK_036898) and NADP-ME2 (nadp-me2; SALK_020607) and a triple mutant nadp-me2x3x4 obtained by crosses (Gerrard Wheeler et al., 2005). All these alleles have been previously characterized and the position of the single T-DNA insertion into each NADP-ME gene was verified by amplifying and sequencing the T-DNA flanking genomic regions (Gerrard Wheeler et al., 2005). No expression of the corresponding NADP-ME gene was detected in the knockout lines (Gerrard Wheeler et al., 2005).
Arabidopsis transgenic lines were obtained by transforming wild type plants (Columbia-0, WT) with a construct carrying the complete coding sequence of NADP-ME1 fused to YFP (yellow fluorescent protein) gene, under the control of the NADP-ME1 (referred as NADP-ME1::YFP) or the double 35SCaMV promoter. The construction that holds NADP-ME1 promoter contains the 2,000 bp long sequence upstream transcription + 1 site and the first intron of the gene. The binary vector ER-yb (Nelson et al., 2007) was used. Inflorescences were incubated with cultures of Agrobacterium tumefaciens strain GV3101 using the protocol described in Clough and Bent (1998). The transformed plants were selected with the herbicide BASTA. Four homozygous T3 lines for each construction were analyzed.
Seeds were sterilized with 0.5% (v/v) Triton X-100 and 50% (v/v) ethanol for 3 min, washed with 95% (v/v) ethanol and dried on filter paper. Seeds were stratified for 72 h at 4°C in the dark to synchronize germination, unless otherwise is stated. Plants were grown in 1× MS plates (Murashige and Skoog, 1962) or in soil in a culture room at days of 16 h of light with a flux density of 100 μE m-2 s-1 at 23–25°C. Seeds were collected at different stages, including 7, 12, and 18 days after pollination (DAP), mature (28 DAP), and 1 and 2 days after imbibition (DAI). Seedlings of 8 days grown in MS plates were transferred to plates supplemented with 100 mM NaCl, 225 mM mannitol or 0.5–10 μM ABA and collected at different times. All samples were frozen in liquid N2 and stored at -80°C.
Real Time Polymerase Chain Reaction (qPCR) Assays
Total RNA was extracted using a method developed for seed samples of Arabidopsis (Oñate-Sánchez and Vicente-Carbajosa, 2008) or a phenol-based one (Chomczynski and Sacchi, 1987) and plant RNA purification columns (PureLink, Amicon) for the rest of plant tissues, and then treated with RQ1DNase (Promega). The quantity and quality were evaluated by spectrophotometric measurements and electrophoresis in agarose gels. cDNAs were synthesized using MMLV (Promega) and random primers (Biodynamics). Relative expression was determined using specific primers (NADP-ME1 left: 5′-CAAGGCAATAAAACCGACTG-3′, NADP-ME1 right: 5′-CATTTTTGCTAGTGGAAGCC-3′, NA DP-ME2 left: 5′-ACGATGGCAAAACCTACTTG-3′, NADP-ME2 right: 5′-ATTGGCGTAATGCTCTTCTG-3′, NADP-ME3 left: 5′-GGCACCAATCAGACTCAGATCT-3′, NADP-ME3 rig-ht: 5′-AGCAAGTCCTTTATTGTAACGT-3′, NADP-ME4 left:5′-CTTTCGAACCCAACTTCTCA-3′, NADP-ME4 right: 5′-CATTATTAGCCCGAGTCCAA-3′, YFP left: 5′-ACGTAAACGGCCACAAGTTC-3′, YFP right: 5′-AAGTCGTGCTGCTTCATGTG-3′) and polyubiquitin 10 gene (AT4G05320; Czechowski et al., 2005) as normalizer. The amplifications were performed on a Stratagene Mx3000P cycler, using the SYBR Green I dye (Invitrogen) as a fluorescent reporter. PCR controls were made to ensure that the RNA samples were free of DNA contamination. The PCR specificity was verified by melting curve and gel electrophoresis analysis of the products. The relative expression was calculated using a modified version of the 2-ΔΔCt method (Pfaffl, 2001), the efficiencies and the propagation of errors determined according to Liu and Saint (2002) and Hellemans et al. (2007). Each sample was run in triplicate and determined in three biological replicas.
Microscopy Analysis
Detection of YFP was achieved using a YFP filter (excitation, 488 nm; emission, 505–550 nm) and a Karl Zeiss Lsm880 or a Nikon Eclipse TE-2000 Model-E2 confocal microscope. Roots were mounted in propidium iodide dye (Invitrogen) and the imaging settings were 488 nm excitation and >585 nm emission.
NADP-ME Activity Measurements in Extracts and Western Blot Analysis
Samples were homogenized in mortars according to Badia et al. (2015) and the extracts were desalted through Sephadex G-50 spin columns. Protein concentration was determined by the Bio-Rad protein assay using total serum protein as standard. NADP-ME activity was assayed at 30°C in a Jasco spectrophotometer following the appearance of NADPH at 340 nm (𝜀340 nm =6.22 mM-1 cm-1) using 50 mM MOPS-KOH pH 6.8, 10 mM MgCl2, 0.5 mM NADP and 10 mM malate. One unit (U) is defined as the amount of enzyme that catalyzes the formation of 1 μmol of NADPH min-1 under the specified conditions. Each sample was measured in triplicate and determined in three biological replicas.
SDS–PAGE was performed in 10% (w/v) polyacrylamide gels according to Laemmli, 1970. Proteins were then electroblotted onto a nitrocellulose membrane. Antibodies against green fluorescent protein (Abcam), which also immunodetect YFP fusion proteins, were used. Bound antibodies were visualized by linking to alkaline phosphatase conjugated goat anti-rabbit IgG according to the instructions of the manufacturer (Sigma). Alkaline phosphatase activity was detected colorimetrically.
Determination of Phenotypic Parameters
The analyzed lines were grown simultaneously with a randomized physical arrangement and frequently rotated. Germination was evaluated by counting the number of seeds with visible radicles, in plate growth assays without previous stratification. Seeds freshly collected or stored at not controlled conditions for 1–11 years were used. For primary and lateral root length measurement, 5-day-old seedlings were transplanted to MS plates without or with 10 μM ABA and monitored for 6 days.
Controlled Deterioration Test
The controlled deterioration test was performed as described previously (Mao and Sun, 2015) with minor modifications. Briefly, freshly harvested seeds were dried in a desiccator containing silica gel and then equilibrated for 3 days at 15°C and 85–90% relative humidity (RH) in a hygrostat of KCl. Then, the hygrostat was transferred to 40°C which resulted in 80–85% RH. After 1–7 days at high temperature, the seeds were stored at 20°C and 33% RH for 3 days in a hygrostat of MgCl2 and dried again in a desiccator with silica gel (6% RH). The RH and temperature were monitored in all steps with a datalogger. The germination was assayed in replicates of 100 seeds in agar plates and recorded after 7 days.
Stomatal Opening Assays
WT and nadp-me1 plants were grown in soil pots for 2–3 weeks. The stomatal response was evaluated in detached leaves measuring the stomatal aperture after treatment with 30 μM ABA according to Wohlbach et al. (2008).
In silico Phylogenetic Analysis
Protein sequences were retrieved from Phytozome 9.1 database1, using A. thaliana NADP-ME2 as query. The evolutionary history was inferred using the Neighbor-Joining method (Saitou and Nei, 1987). The evolutionary distances were computed using the Poisson correction method (Zuckerkandl and Pauling, 1965). The analysis involved 40 amino acid sequences. All positions containing gaps and missing data were eliminated. There was a total of 488 positions in the final dataset. Evolutionary analyses were conducted in MEGA7 (Kumar et al., 2016). The detection of ABA related elements was performed with a phylogenetic footprinting tool with a set of characterized motifs (Supplementary Table S1, cis-analyzer, Gismondi unpublished).
Statistical Analysis
In general, for comparisons between WT and nadp-me1 Student’s t test was used whenever the general assumptions of normality and equal variance were achieved. Mann-Whitney rank sum test was used for the other cases. For comparison for more than two lines, one-way ANOVA or its non-parametric equivalent Kruskal-Wallis test was used. For categorical variables such as germination analysis, the data was analyzed using comparisons of proportions through the Z statistic. The sample size and the significance are indicated in the figure legends.
Results
NADP-ME1 Transcript and Protein Increase During Seed Maturation in Arabidopsis
The expression profile of NADP-ME1 was analyzed along Arabidopsis seed maturation and germination. The transcript level of NADP-ME1 sharply increases during seed maturation from 7 DAP until complete maturation, reaching a value 136-fold higher at 18 DAP (Figure 1A). By contrast, the transcript levels of NADP-ME2, NADP-ME3, and NADP-ME4 remain fairly constant along seed maturation (Figure 1A). In germinating seeds the transcript level of NADP-ME1 drastically decreases to the low levels detected at 7 DAP (Figure 1A). A similar pattern of NADP-ME1 expression was observed using the YFP reporter gene under the control of NADP-ME1 promoter. In embryos, YFP fluorescence is undetectable up to 9 DAP; increase from 11 to 18 DAP; and then decrease in germinating embryos (Figure 1D).
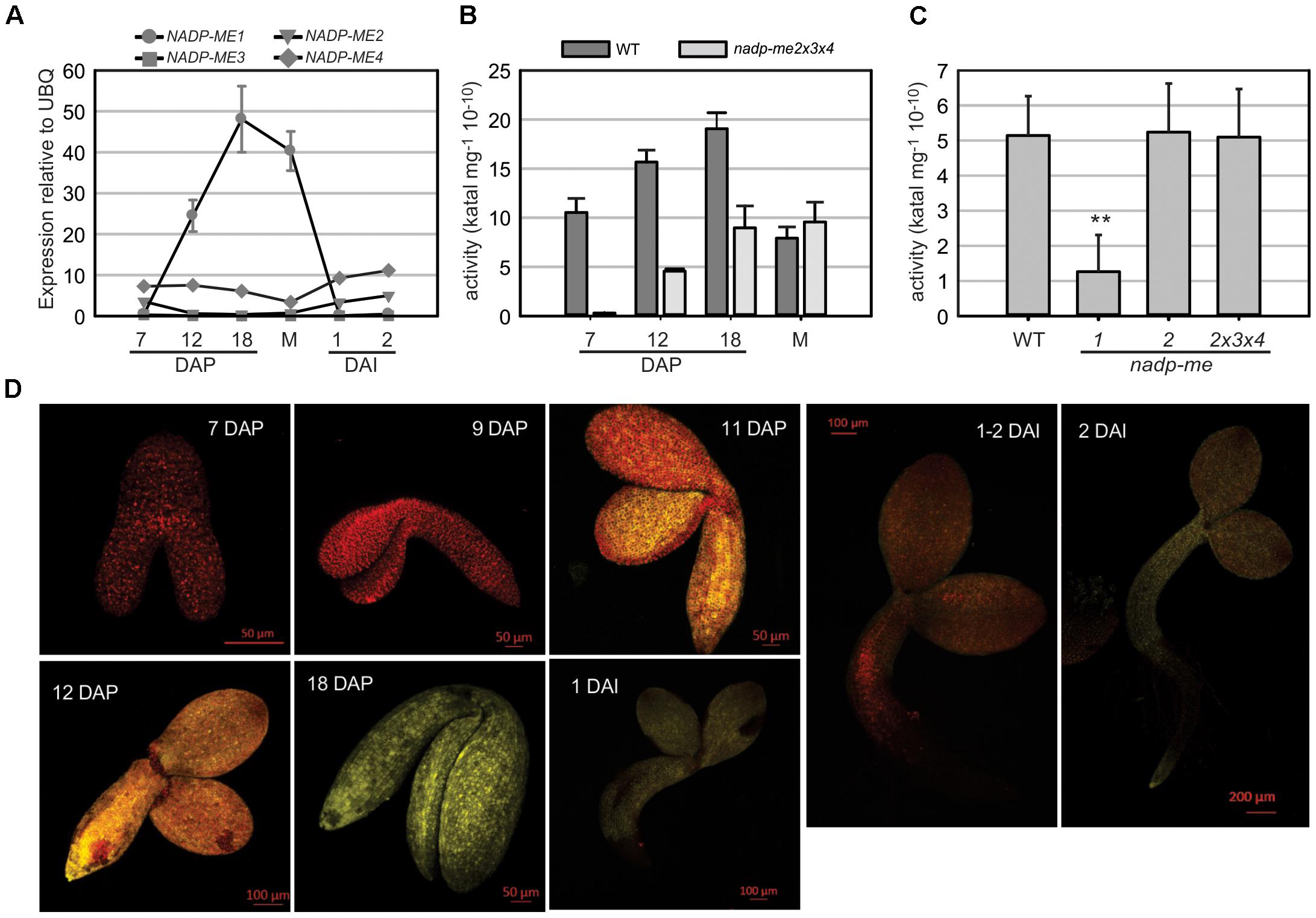
FIGURE 1. NADP-ME1 expression in Arabidopsis seeds. (A) Relative level of the transcripts of NADP-ME genes in seeds collected at 7, 12, and 18 days after pollination (DAP), mature stage (M), and at 1 and 2 days after imbibition (DAI). The polyubiquitin 10 (UBQ) gene was used as reference. NADP-ME activity was assayed in siliques throughout maturation (B) and in mature seeds (C) for WT and simple or triple mutant lines. The values are the average of three independent experiments ± SD. In figure (C) the two asterisks (∗∗) indicates that there is a significant difference between nadp-me1 and the rest of the lines (p < 0.001) according to the ANOVA test followed by a Bonferroni t-test of multiple comparisons versus control group (WT). (D) Expression of the fusion protein NADP-ME1::YFP during seed development and germination. The reporter contains the complete coding sequence of NADP-ME1 fused to YFP gene, under the control of the NADP-ME1 promoter. YFP fluorescence in representative embryos at 7; 9; 11; 12; and 18 DAP and at 1, 1–2, and 2 DAI are shown. Scale bars are indicated in each panel. These results were confirmed in two independent lines.
NADP-ME activity in Arabidopsis siliques increases from 7 DAP to 18 DAP (Figure 1B), following a similar profile as that observed for NADP-ME1 transcript level (Figure 1A). The increase of NADP-ME activity in nadp-me2x3x4 triple mutant, in which NADP-ME1 is the only NADP-ME found, matches the increase of activity found in WT. Thus, the NADP-ME activity profile in Arabidopsis WT may be endorsed to NADP-ME1 increase (Figure 1B). When comparing NADP-ME activity in mature seeds of WT and nadp-me mutant lines, the lack of NADP-ME2 alone or in combination with NADP-ME3 and NADP-ME4 does not affect the total NADP-ME activity. In contrast, a drastic decrease of NADP-ME activity is observed in nadp-me1 knockout mutant (Figure 1C). Overall, it is clear that NADP-ME1 is the isoform that contributes the most to NADP-ME activity at mature seed stage (Figure 1C).
NADP-ME1 Is Up-Regulated by NaCl, Mannitol and ABA in Arabidopsis Seedlings and Roots
NADP-ME1-4 transcript levels were assayed in Arabidopsis rosettes of 8 days (Figure 2A and Supplementary Figure S1A). As previously shown (Gerrard Wheeler et al., 2005), the transcripts of NADP-ME1 and -3 display very low levels in relation to NADP-ME2 and -4 in control conditions (MS, Supplementary Figure S1A). However, when 8-day-old seedlings are treated with 100 mM NaCl; 225 mM mannitol or 10 μM ABA for 6 h, a strong induction NADP-ME1 transcript is observed (53, 79, and 55 times, respectively). Neither NADP-ME2 nor NADP-ME3 or NADP-ME4 shows such a significant response as NADP-ME1 (Figure 2A and Supplementary Figure S1A). We further analyzed the response of 8-day-old seedlings to different ABA concentrations (0.5, 1, 5, and 10 μM of ABA) and exposure times. The level of NADP-ME1 transcript increases 5–6 folds in 0.5, 1, and 5 μM ABA and 10 folds in 10 μM ABA, reaching a level, which is almost twice as high as that of NADP-ME2 (Supplementary Figure S1B). When the length of the ABA treatment was tested, we found that NADP-ME1 increases 6 and 12 folds at 6 and 12 h, respectively and NADP-ME2 levels did not varied significantly (Supplementary Figure S1C).
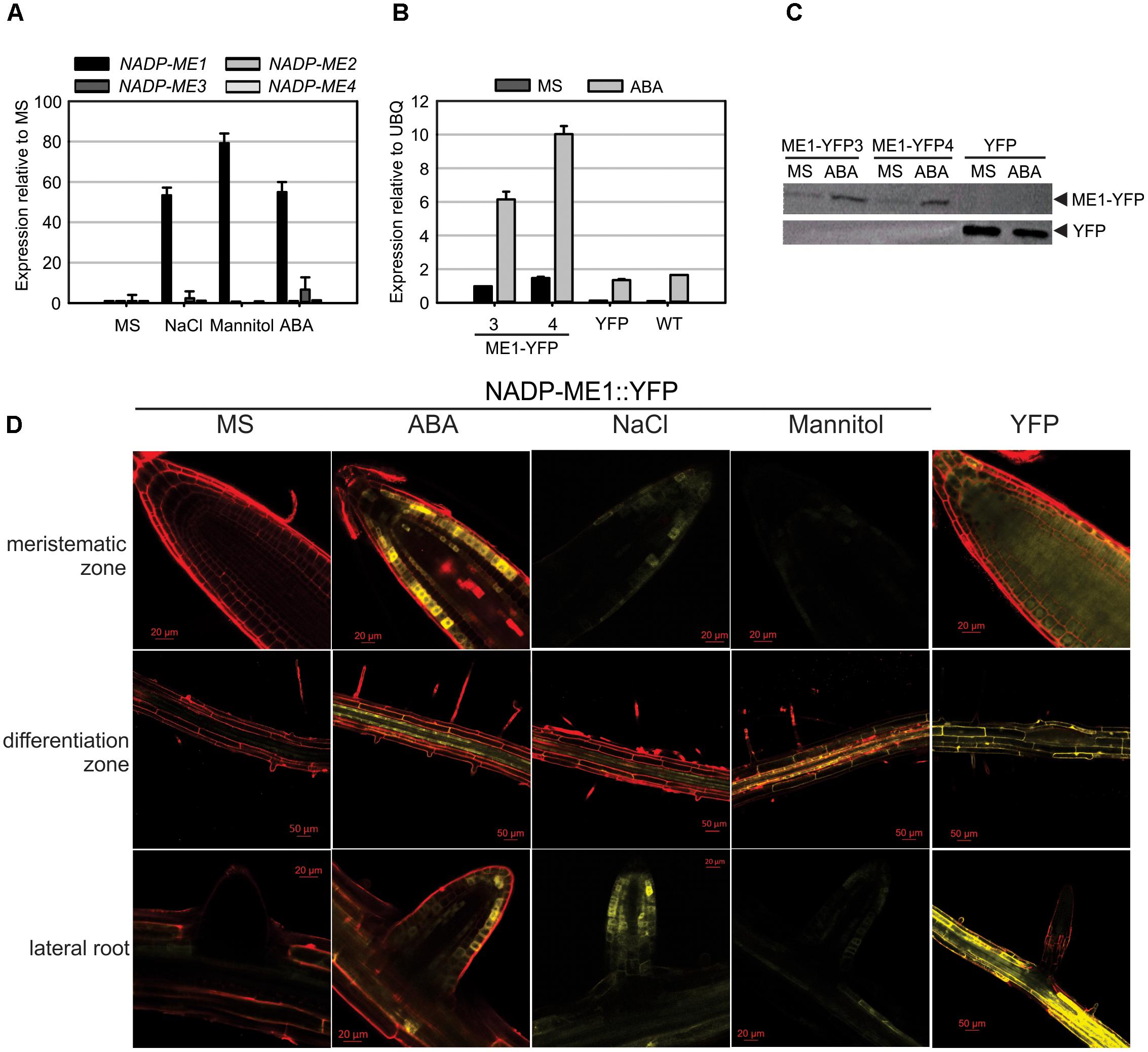
FIGURE 2. NADP-ME1 expression in response to NaCl, mannitol, and ABA. (A) Relative levels of the transcripts of NADP-ME genes in rosettes in control conditions (MS) or after 6 h treatments of seedling with 100 mM NaCl, 225 mM mannitol, or 10 μM ABA. The polyubiquitin 10 gene (UBQ) was used as reference. (B) Response of NADP-ME1 promoter in transgenic lines. ME1-YFP3 and 4 are two independent transgenic lines expressing NADP-ME1::YFP under the control of NADP-ME1 promoter. YFP denotes a line expressing the YFP coding sequence under the control of the double 35SCaMV promoter. The level of NADP-ME1 was compared in control conditions (MS) and after treatment with 10 μM ABA during 6 h was applied. The values are the average of three independent experiments ± SD. (C) Western blot of the seedling protein extract (30 μg) from the transgenic lines. Molecular mass markers were run in parallel and stained with Coomassie Blue to localize the position of fusion and YFP proteins. (D) Expression of the fusion protein NADP-ME1::YFP in roots in response to ABA, NaCl, and mannitol. YFP fluorescence in different parts of roots of 8-day-old seedlings incubated with 10 μM ABA, 100 mM NaCl, or 225 mM mannitol for 6 h. Scale bars are indicated in each panel. Right panels show the fluorescence distribution of the control YFP line. These results were confirmed in two independent lines.
To test if NADP-ME1 protein was affected by the presence of ABA, we used the transgenic NADP-ME1::YFP lines to immunodetect the fusion protein. Consistent with the previous observations, a strong NADP-ME1 induction by ABA at the level of transcript and protein was observed in the lines expressing NADP-ME1::YFP under the control of NADP-ME1 promoter (Figures 2B,C).
To further analyze the expression pattern of NADP-ME1 under water stress and ABA treatments we observed the YFP fluorescence in roots of 8-day-old seedlings of the transgenic lines expressing NADP-ME1::YFP under the control of NADP-ME1 promoter. ABA, NaCl and mannitol produced similar induction of the NADP-ME1, especially in the root apical meristem, the differentiation zone and in lateral roots (Figure 2D).
Seeds of nadp-me1 Mutant Are Less Sensitive to the ABA Repression of Germination and Loss Viability More Rapidly Than WT
The number of nadp-me1 and WT germinated seeds with visible radicles was counted at different times after seeding. Almost 100% of nadp-me1 seeds germinate approximately 50 h after seeding in MS medium but WT seeds reach that value almost 48 h later (Figure 3A). In the presence of exogenous ABA, the percentages of germinated seeds are lower for both lines but nadp-me1 seeds reach 2.5–3 folds higher germination percentages than WT at 62 and 86 h (Figure 3B). Then, these results show that under both conditions the germination rate of nadp-me1 is faster than WT and a lower sensitivity of nadp-me1 seeds to ABA repression of germination.
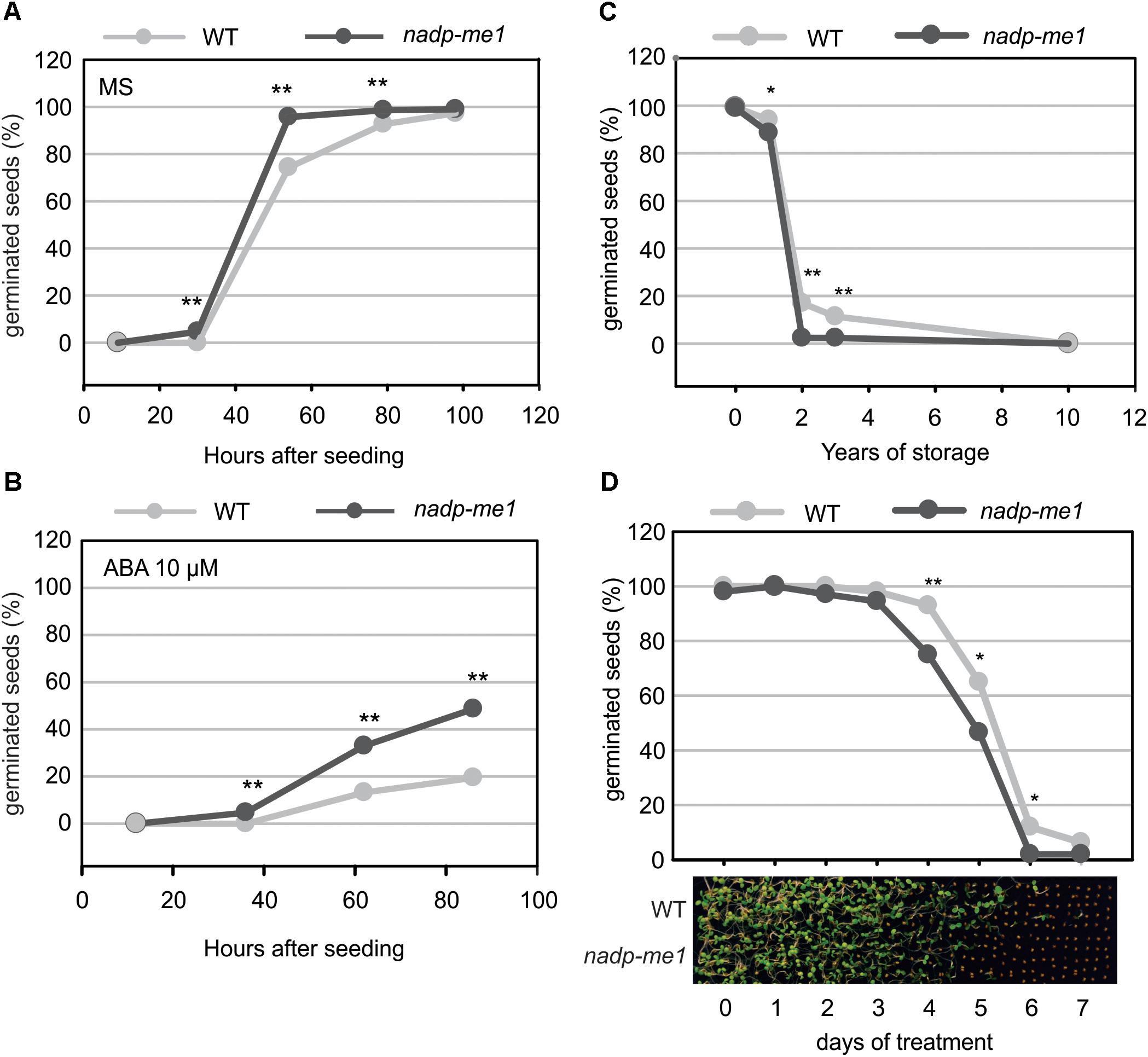
FIGURE 3. Germination and seed viability assay in nadp-me1 mutant. Germination was evaluated by counting the number of seeds with visible radicles in MS plates (A) or MS supplemented with 10 μM ABA (B). Germination was also evaluated using long time stored seeds (C) or after exposing them to high temperature (40°C) for 1–7 days (D). The proportion of germinated seeds after evaluating 300 seeds per line in each case is shown. One or two asterisks denote significant differences of p < 0.05 or p < 0.001, respectively, between WT and nadp-me1 according to the analysis of proportions of germinated seed (z-test) in each line at each time point.
Seed viability was also analyzed for the nadp-me1 mutant. The viability of recently harvested nadp-me1 and WT seeds is 100%. However, when we tested seeds stored for long times, we found that nadp-me1 seeds loss viability earlier than WT (Figure 3C).
To examine whether the longevity is affected in seeds lacking NADP-ME1, we performed a controlled deterioration test based on the exposure of seeds to high temperature (40°C) and relative humidity (80–85%) for several days. Although the viability is the same as WT without treatment and after complete treatment (7 days), nadp-me1 seed decay is faster than WT (Figure 3D).
Stomata and Roots in Knockout nadp-me1 Are Less Sensitive to ABA
Accordingly to the very low levels of NADP-ME1 expression observed in leaves, nadp-me1 mutant plants do not show differences in stomatal aperture compared to WT under normal conditions. However, in the presence of 30 μM ABA the stomata pore size is larger in plants lacking NADP-ME1 than in WT (Figure 4A).
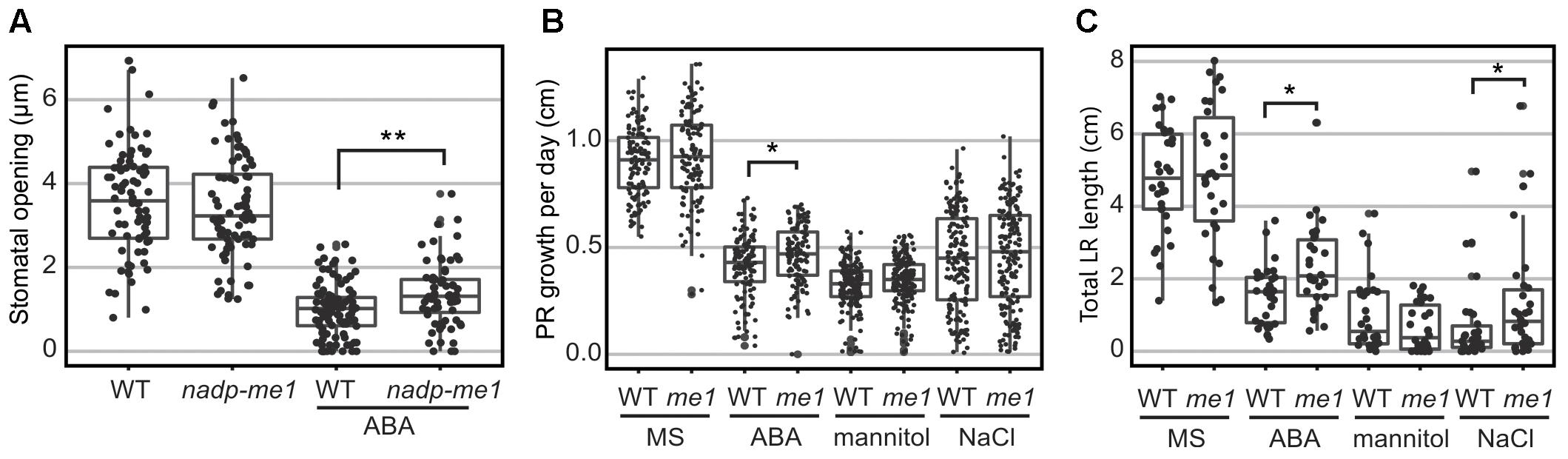
FIGURE 4. Stomatal opening and root length assays in nadp-me1 mutant. (A) Stomata pore size was determined in the light and in the presence or absence of 30 μM ABA. Primary (PR, B) and lateral (LR, C) root length were assayed in seedling transferred to MS plates supplemented with 10 μM ABA, 100 mM NaCl, or 225 mM mannitol for 6 days. Between 70 and 100 stomata, 30 primary roots, and 120–160 secondary roots per line and condition were measured. All values are presented and the statistical descriptions of each set of data are shown as box plots. One (p < 0.05) or two (p < 0.001) asterisks denote significant differences between WT and nadp-me1 according to t-test or Mann-Whitney rank sum test for data that passed or fail normality test and equal variance test, respectively.
Root growth responses to ABA and NaCl are also different in nadp-me1 and WT. When 5-day-old seedlings are transferred to MS medium supplemented with 10 μM ABA, increased primary root (PR) elongation rate and total length of lateral roots (LR) are found in nadp-me1 mutants with respect to WT (Figures 3B,C). When the seedlings are transferred to MS supplemented with 100 mM NaCl, the rate of growth of the PR is not significantly different for nadp-me1 mutants compared to WT, but the average total length of LR is higher for the mutant plants (Figures 3B,C). Although NADP-ME1 expression was increased in roots under mannitol treatment (Figure 2D), no significant difference was found regarding to root growth between WT and nadp-me1 mutant (Figures 3B,C).
ABA-Response Elements Are Conserved in NADP-ME1 Related Sequences
The sequence analysis of the complete set of NADP-ME isoforms from the dicot species A. thaliana, Glycine max, Medicago truncatula, Phaseolus vulgaris, Ricinus communis, and the monocot species Oryza sativa, Setaria italica, and Zea mays shows that monocot cyt2, cyt3 and plastidic isoforms belong to a monophyletic cluster independent from dicot cyt2 and plastidic isoforms that also cluster together (Figure 5A). On the other hand, monocot cyt1 and dicot cyt1 groups show origins different from the rest of the family members and are more similar to the ancestral NADP-ME present in the origin of angiosperms (Figure 5A).
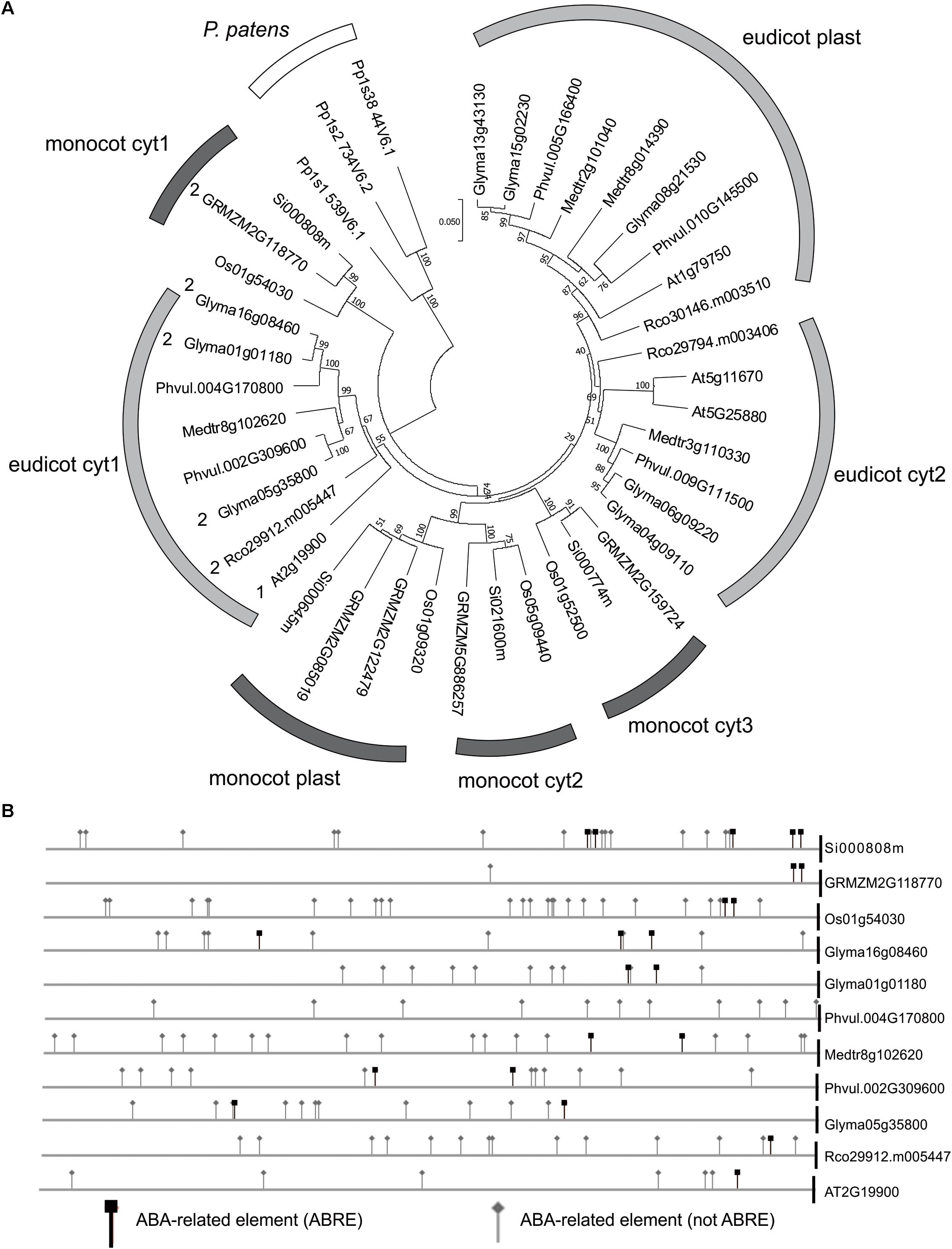
FIGURE 5. ABA and drought response elements in the promoters of NADP-ME1 and related genes. (A) The evolutionary history of the complete set of NADP-ME isoforms from the dicot species Arabidopsis (At), soybean (Glyma), Medicago truncatula (Medtr), Phaseolus vulgaris (Phvul), Ricinus communis (Rco) and the monocot species rice (Os), Setaria italica (Si), and maize (GRMZM) was inferred using the Neighbor-Joining method. The optimal tree with the sum of branch length = 2.44632768 is shown. The tree is drawn to scale, with branch lengths in the same units as those of the evolutionary distances used to infer the phylogenetic tree. The evolutionary distances are in the units of the number of amino acid substitutions per site. (B) The occurrence of ABA responsive elements was analyzed in the promoters of monocot cyt1 and dicot cyt1 groups.
In silico analysis of the promoter and 5′UTR region of monocot cyt1 and dicot cyt1 genes shows that they conserve elements that are linked to the ABA response (Figure 5B and Supplementary Tables S1, S2). In this sense, we observed an increment of the NADP-ME1 transcript level in response to dose and time of ABA treatment (Supplementary Figures S1B,C). It is also important to mention that these regulatory elements are also present in the bryophyte Physcomitrella patens, suggesting an ancestral and strongly conserved regulation of NADP-ME genes (Figure 5 and Supplementary Table S3).
Discussion
NADP-ME1 Expression Is Up-Regulated by ABA and Its Absence Weakens the ABA Response in Different Arabidopsis Organs
Despite A. thaliana having three cytosolic NADP-ME isoforms, NADP-ME1 displays a distinctive and specific expression pattern. Our results indicate that under normal conditions, NADP-ME1 transcript is detected almost exclusively in maturing seeds (Figure 1), while it accumulates in the rosettes and roots under saline or osmotic stresses (Figure 2). Besides, we found that ABA increases NADP-ME1 transcript, which is correlated with an increase in NADP-ME1 protein (Figures 2A–C). This up-regulation is exclusively exerted over NADP-ME1, since NADP-ME2, NADP-ME 3 and NADP-ME 4 do not show this response in the conditions assayed here.
Considering that ABA mediates part of the response to saline and osmotic stress and the regulation of NADP-ME1 expression by ABA treatment, the sharp increase in the expression of NADP-ME1 in maturing seeds could be caused by the accumulation of ABA (Figure 1A). Thus, it seems that this phytohormone acts as one of the major signals controlling NADP-ME1 expression.
Besides the control of NADP-ME1 expression exerted by ABA, we show that the absence of NADP-ME1 affects ABA response in different Arabidopsis organs. Particularly, we found that the lack of NADP-ME1 affects not only the longevity of the seeds but also the control of the germination (Figure 3). nadp-me1 mutants exhibit less tolerance to prolonged storage and are less sensitive to the inhibition of the germination exerted by ABA (Figure 3). Plants lacking NADP-ME1 also exhibit less sensitivity than the WT to stomatal closure and root growth inhibition induced by ABA and NaCl (Figure 4). The mannitol treatment did not show a significant difference in WT and nadp-me1 root response. This could be explained by the existence of ABA-independent pathways that mediate the osmotic response, which would not be affected in the nadp-me1 mutant.
It is well-known that ABA regulates vital processes associated to normal late seed development such as synthesis of reserve compounds, tolerance to desiccation, dormancy, longevity, and germination (North et al., 2010). In addition, this phytohormone accumulates in large quantities during saline, osmotic or drought stress conditions and controls root growth and transpiration through stomatal closure (Priest et al., 2006; Christmann et al., 2007). ABA target genes belong to functional categories such as seed maturation (oleosins, dehydrins, or late embryogenesis abundant proteins), protein stability (proteases), cellular structure (expansins and wall synthesis enzymes), signaling (kinases and phosphatases), response to stress (heat shock proteins) and metabolism (Reeves et al., 2011). Here, we found that there is a link between NADP-ME1 expression and ABA in Arabidopsis thaliana. Moreover, our results suggest that NADP-ME1 would be important to cope with conditions of water deficit, where the plant responses are mainly mediated by ABA.
NADP-ME1: An Old and Conserved NADP-ME With Particular Physiological Roles Linked to ABA Response
In different studies it has been shown that that the expression pattern of the evolutionary related NADP-ME cyt1 genesare similar to NADP-ME1. In G. max and R. communis the transcripts encoding the isoforms homologous to NADP-ME1 also showed an induction along the seed maturation (Gerrard Wheeler et al., 2016). The study of the Z. mays NADP-ME family showed that one cytosolic isoform also accumulated along the grain maturation and increased after ABA treatment in roots (Detarsio et al., 2008; Alvarez et al., 2013). Furthermore, Chi et al. (2004) observed that a cytosolic isoform of O. sativa is specifically induced by mannitol and NaCl and confers salt tolerance in transgenic over-expressing Arabidopsis (Chen and Long, 2007). Furthermore, the presence of ABA related elements in NADP-ME cyt1 genes from monocotyledonous, dicotyledonous and P. patens (Figure 5B), suggests an ancestrally conserved biological role. In spite of the evolutionary distance of this bryophyte with respect to angiosperms, ABA is also involved in tolerance to stress by balancing the water level in its tissues (Takezawa et al., 2011). Notably, it is proposed that ABA biosynthetic pathway and its sensing machinery originally evolved for cellular protection from water deficits of ancestral land plants but independently have been used in angiosperms to provide drought tolerance in vegetative tissues and desiccation tolerance in seeds. This could indicate that the participation of NADP-ME in the response to ABA existed in plants even before the appearance of stomata, vascular system and seeds and co-evolved with ABA responses in land plants.
Based on the phylogenetic and promoter analysis and in the phenotypic analysis carried out here, we propose that NADP-ME1 is an isoform that plays a very specialized role compared to the rest of the NADP-ME family members, possibly linked to the signaling initiated by ABA, as an intermediary or final effector (Figure 6). This enzyme could fulfill its role by consuming malate and/or generating pyruvate and NADPH in the cytosol. Malate is a metabolic intermediate that is being recognized for its regulatory functions (Finkemeier et al., 2013). For example, the decrease of ion entrance through a vacuolar channel that promotes the stomata closure is regulated by the decrease of the cytosolic malate concentration (De Angeli et al., 2013). Besides, the growth and the response of the roots to the availability of water also depend on the hydric potential generated by the movement of osmolytes through cellular compartments (Robbins and Dinneny, 2015). On the other hand, the NADPH generated could be used for the biosynthesis of compounds that accompany the stress response or to control reactive oxygen species generation. Although future experiments are necessary to elucidate the mechanism by which NADP-ME1 participates in the ABA signaling pathway responses, the results presented here clearly show the participation of this particular isoenzyme from the NADP-ME family in the response to a hormone, which is a key in different physiological responses in plants. NADP-ME1 could work as an intermediate or end effector, possibly regulating the concentration of the reaction substrates and/or products to feed metabolic pathways and regulatory functions triggered by the water stress (Figure 6). Most work on ABA focuses on the discovery of molecular aspects about multiple phosphorylation cascades and activation of transcription factors that are triggered by its signal (Raghavendra et al., 2010; Umezawa et al., 2010; Guo et al., 2011) but less is known about the interaction of ABA signaling with carbon metabolism. Here we found that a NADP-ME is also involved in ABA responses. The observation of a mild phenotype of nadp-me1 (Figures 3, 4) is in accordance with the multiple and coordinated signaling pathways involved in ABA responses. The findings shown here contribute to the understanding of the maturation and germination seed processes and the response of plants to water stress, and may help us to establish innovative strategies to generate crops with a better use of natural resources.
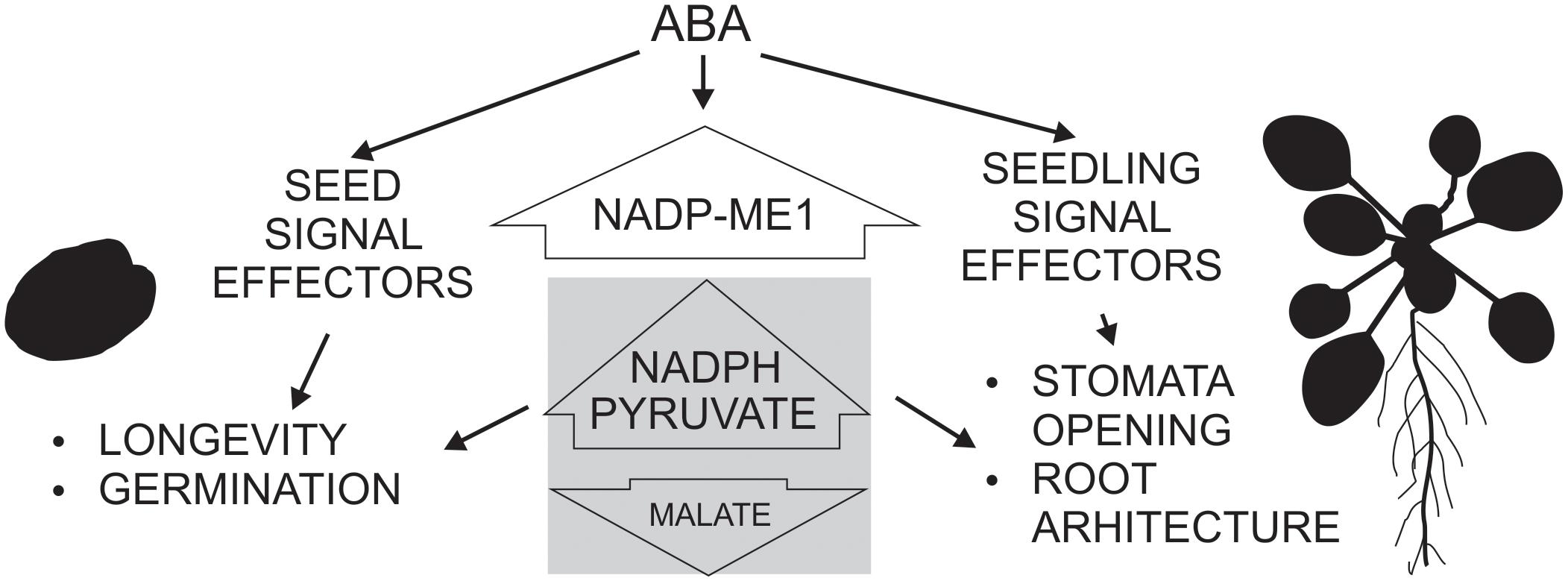
FIGURE 6. Schematic scheme showing the participation of NADP-ME1 in ABA response in Arabidopsis. ABA induces NADP-ME1 expression in particular organs and cell types producing a modification of malate, pyruvate, and NADPH levels, which impact on seed longevity and germination, stomata opening and root architecture.
Author Contributions
CLA, TP, GT, MB, MG, MGW, and MS performed the experiments. VM contributed to the plant lines. CLA, CSA, MD, MGW, and MS planned the experiments, analyzed the data, and wrote the paper.
Funding
CSA, MD, MGW, and MS belong to the Researcher Career of National Council of Scientific and Technical Research (CONICET). CLA, TP, MB, and MG are fellows of the same institution. This work has been financially supported by National Agency for Promotion of Science and Technology, CONICET, and Deutsche Forschungsgemeinschaft.
Conflict of Interest Statement
The authors declare that the research was conducted in the absence of any commercial or financial relationships that could be construed as a potential conflict of interest.
Acknowledgments
The authors thank Rodrigo Vena, María J. Maymó, Marcos A. Tronconi, María C. Craia, and Luisina Vitor Horen for the technical assistance to carry out this work. This work has been released as a pre-print at https://www.biorxiv.org/content/early/2018/06/16/348771 (Arias et al., 2018).
Supplementary Material
The Supplementary Material for this article can be found online at: https://www.frontiersin.org/articles/10.3389/fpls.2018.01637/full#supplementary-material
FIGURE S1 | NADP-ME1 expression in response to NaCl, mannitol and ABA. (A) Relative levels of the transcripts of NADP-ME genes in rosettes in control conditions (MS) or after 6 h treatments of seedling with 100 mM NaCl, 225 mM mannitol or 10 μM ABA. The expression levels of NADP-ME genes are normalized to expression of the reference gene polyubiquitin 10 (UBQ). In (B,C) the levels of NADP-ME1 and NADP-ME2 transcripts after 12 h of ABA treatment or 10 μM ABA, respectively, are shown. The values are the average of three independent experiments ± SD.
TABLE S1 | Motifs selected for the analysis of occurrence in promoters and 5′UTR.
TABLE S2 | Location of motifs in the promoters and 5′UTR of the genes selected.
TABLE S3 | Location of motifs in the promoters and 5′UTR of NADP-ME genes from P. patens.
Abbreviations
ABA, abscisic acid; DAI, days after imbibition; DAP, days after pollination; LR, lateral roots; ME, malic enzyme; MS, Murashige and Skoog medium; PR, primary root; qPCR, real time polymerase chain reaction assay; RH, relative humidity; U, unit; WT, wild type; YFP, yellow fluorescent protein.
Footnotes
References
Alvarez, C. E., Saigo, M., Margarit, E., Andreo, C. S., and Drincovich, M. F. (2013). Kinetics and functional diversity among the five members of the NADP-malic enzyme family from Zea mays, a C4 species. Photosynth. Res. 115, 65–80. doi: 10.1007/s11120-013-9839-9
Arias, C., Pavlovic, T., Torcolese, G., Badia, M., Gismondi, M., Maurino, V., et al. (2018). NADP-Dependent malic enzyme 1 participates in the abscisic acid response in Arabidopsis thaliana. bioRxiv. Available at: https://doi.org/10.1101/348771
Badia, M. B., Arias, C. L., Tronconi, M. A., Maurino, V. G., Andreo, C. S., Drincovich, M. F., et al. (2015). Enhanced cytosolic NADP-ME2 activity in A. thaliana affects plant development, stress tolerance and specific diurnal and nocturnal cellular processes. Plant Sci. 240, 193–203. doi: 10.1016/j.plantsci.2015.09.015
Brown, N. J., Palmer, B. G., Stanley, S., Hajaji, H., Janacek, S. H., Astley, H. M., et al. (2010). C4 acid decarboxylases required for C4 photosynthesis are active in the mid-vein of the C3 species Arabidopsis thaliana, and are important in sugar and amino acid metabolism. Plant J. 61, 122–133. doi: 10.1111/j.1365-313X.2009.04040.x
Chen, Y., and Long, M. (2007). A cytosolic NADP-malic enzyme gene from rice (Oryza sativa L.) confers salt tolerance in transgenic Arabidopsis. Biotechnol. Lett. 29, 1129–1134. doi: 10.1007/s10529-007-9347-0
Chi, W., Yang, J., Wu, N., and Zhang, F. (2004). Four rice genes encoding NADP malic enzyme exhibit distinct expression profiles. Biosci. Biotechnol. Biochem. 68, 1865–1874. doi: 10.1271/bbb.68.1865
Chomczynski, P., and Sacchi, N. (1987). Single-step method of RNA isolation by acid guanidinium thiocyanate-phenol-chloroform extraction. Anal. Biochem. 162, 156–159. doi: 10.1016/0003-2697(87)90021-2
Christmann, A., Weiler, E. W., Steudle, E., and Grill, E. (2007). A hydraulic signal in root-to-shoot signalling of water shortage. Plant J. 52, 167–174. doi: 10.1111/j.1365-313X.2007.03234.x
Clough, S. J., and Bent, A. F. (1998). Floral dip: a simplified method for Agrobacterium-mediated transformation of Arabidopsis thaliana. Plant J. 16, 735–743. doi: 10.1046/j.1365-313x.1998.00343.x
Czechowski, T., Stitt, M., Altmann, T., Udvardi, M. K., and Scheible, W. R. (2005). Genome-wide identification and testing of superior reference genes for transcript normalization in Arabidopsis. Plant Physiol. 139, 5–17. doi: 10.1104/pp.105.063743
De Angeli, A., Zhang, J., Meyer, S., and Martinoia, E. (2013). AtALMT9 is a malate-activated vacuolar chloride channel required for stomatal opening in Arabidopsis. Nat. Commun. 4:1804. doi: 10.1038/ncomms2815
Detarsio, E., Maurino, V. G., Alvarez, C. E., Müller, G. L., Andreo, C. S., and Drincovich, M. F. (2008). Maize cytosolic NADP-malic enzyme (ZmCytNADP-ME): a phylogenetically distant isoform specifically expressed in embryo and emerging roots. Plant Mol. Biol. 68, 355–367. doi: 10.1007/s11103-008-9375-8
Drincovich, M. F., Lara, M. V., Maurino, V. G., and Andreo, C. S. (2011). “C4 decarboxylases: different solutions for the same biochemical problem, the provision of CO2 to rubisco in the bundle sheath cells,” in C4 Photosynthesis and Related CO2 Concentrating Mechanisms, eds A. S. Raghavendra and R. F. Sage (Heidelberg: Springer), 277–300.
Finkemeier, I., König, A. C., Heard, W., Nunes-Nesi, A., Pham, P. A., Leister, D., et al. (2013). Transcriptomic analysis of the role of carboxylic acids in metabolite signalling in Arabidopsis leaves. Plant Physiol. 162, 239–253. doi: 10.1104/pp.113.214114
Gerrard Wheeler, M. C., Arias, C. L., Righini, S., Badia, M. B., Andreo, C. S., Drincovich, M. F., et al. (2016). Differential contribution of malic enzymes during soybean and castor seeds maturation. PLoS One 11:e0158040. doi: 10.1371/journal.pone.0158040
Gerrard Wheeler, M. C., Arias, C. L., Tronconi, M. A., Maurino, V. G., Andreo, C. S., and Drincovich, M. F. (2008). Arabidopsis thaliana NADP-malic enzyme isoforms: high degree of identity but clearly distinct properties. Plant Mol. Biol. 67, 231–242. doi: 10.1007/s11103-008-9313-9
Gerrard Wheeler, M. C., Tronconi, M. A., Drincovich, M. F., Andreo, C. S., Flügge, U. I., and Maurino, V. G. (2005). A comprehensive analysis of the NADP-malic enzyme gene family of Arabidopsis thaliana. Plant Physiol. 139, 39–51. doi: 10.1104/pp.105.065953
Guo, J., Yang, X., Weston, D. J., and Chen, J. G. (2011). Abscisic acid receptors: past, present and future. J. Integr. Plant Biol. 53, 469–479. doi: 10.1111/j.1744-7909.2011.01044.x
Hellemans, J., Mortier, G., De Paepe, A., Speleman, F., and Vandesompele, J. (2007). qBase relative quantification framework and software for management and automated analysis of real-time quantitative PCR data. Genome Biol. 8:R19. doi: 10.1186/gb-2007-8-2-r19
Hurth, M. A., Suh, S. J., Kretzschmar, T., Geis, T., Bregante, M., Gambale, F., et al. (2005). Impaired pH homeostasis in Arabidopsis lacking the vacuolar dicarboxylate transporter and analysis of carboxylic acid transport across the tonoplast. Plant Physiol. 137, 901–910. doi: 10.1104/pp.104.058453
Kumar, S., Stecher, G., and Tamura, K. (2016). MEGA7: molecular evolutionary genetics analysis version 7.0 for bigger datasets. Mol. Biol. Evol. 33, 1870–1874. doi: 10.1093/molbev/msw054
Laporte, M. M., Shen, B., and Tarczynski, M. C. (2002). Engineering for drought avoidance: expression of maize NADP-malic enzyme in tobacco results in altered stomatal function. J. Exp. Bot. 53, 699–705. doi: 10.1093/jexbot/53.369.699
Liu, W., and Saint, D. A. (2002). A new quantitative method of real time reverse transcription polymerase chain reaction assay based on simulation of polymerase chain reaction kinetics. Anal. Biochem. 302, 52–59. doi: 10.1006/abio.2001.5530
Mao, Z., and Sun, W. (2015). Arabidopsis seed-specific vacuolar aquaporins are involved in maintaining seed longevity under the control of ABSCISIC ACID INSENSITIVE 3. J. Exp. Bot. 66, 4781–4794. doi: 10.1093/jxb/erv244
Murashige, T., and Skoog, F. (1962). A revised medium for rapid growth and bioassays with tobacco tissue cultures. Physiol. Plant. 15, 473–497. doi: 10.1111/j.1399-3054.1962.tb08052.x
Nelson, B. K., Cai, X., and Nebenführ, A. (2007). A multicolored set of in vivo organelle markers for co-localization studies in Arabidopsis and other plants. Plant J. 51, 1126–1136. doi: 10.1111/j.1365-313X.2007.03212.x
North, H., Baud, S., Debeaujon, I., Dubos, C., Dubreucq, B., Grappin, P., et al. (2010). Arabidopsis seed secrets unravelled after a decade of genetic and omics-driven research. Plant J. 61, 971–981. doi: 10.1111/j.1365-313X.2009.04095.x
Oñate-Sánchez, L., and Vicente-Carbajosa, J. (2008). DNA-free RNA isolation protocols for Arabidopsis thaliana, including seeds and siliques. BMC Res. Notes 1:93. doi: 10.1186/1756-0500-1-93
Pfaffl, M. W. (2001). A new mathematical model for relative quantification in real-time RT-PCR. Nucleic Acids Res. 29:e45. doi: 10.1093/nar/29.9.e45
Priest, D. M., Ambrose, S. J., Vaistij, F. E., Elias, L., Higgins, G. S., Ross, A. R., et al. (2006). Use of the glucosyltransferase UGT71B6 to disturb abscisic acid homeostasis in Arabidopsis thaliana. Plant J. 46, 492–502. doi: 10.1111/j.1365-313X.2006.02701.x
Raghavendra, A. S., Gonugunta, V. K., Christmann, A., and Grill, E. (2010). ABA perception and signalling. Trends Plant Sci. 15, 395–401. doi: 10.1016/j.tplants.2010.04.006
Reeves, W. M., Lynch, T. J., Mobin, R., and Finkelstein, R. R. (2011). Direct targets of the transcription factors ABA-Insensitive(ABI)4 and ABI5 reveal synergistic action by ABI4 and several bZIP ABA response factors. Plant Mol. Biol. 75, 347–363. doi: 10.1007/s11103-011-9733-9
Robbins, N. E. II, and Dinneny, J. R. (2015). The divining root: moisture-driven responses of roots at the micro- and macro-scale. J. Exp. Bot. 66, 2145–2154. doi: 10.1093/jxb/eru496
Saigo, M., Tronconi, M. A., Gerrard Wheeler, M. C., Alvarez, C. E., Drincovich, M. F., and Andreo, C. S. (2013). Biochemical approaches to C4 photosynthesis evolution studies: the case of malic enzymes decarboxylases. Photosynth. Res. 117, 177–187. doi: 10.1007/s11120-013-9879-1
Saitou, N., and Nei, M. (1987). The neighbor-joining method: a new method for reconstructing phylogenetic trees. Mol. Biol. Evol. 4, 406–425.
Takezawa, D., Komatsu, K., and Sakata, Y. (2011). ABA in bryophytes: how a universal growth regulator in life became a plant hormone? J. Plant Res. 124, 437–453. doi: 10.1007/s10265-011-0410-5
Umezawa, T., Nakashima, K., Miyakawa, T., Kuromori, T., Tanokura, M., Shinozaki, K., et al. (2010). Molecular basis of the core regulatory network in ABA responses: sensing, signaling and transport. Plant Cell Physiol. 51, 1821–1839. doi: 10.1093/pcp/pcq156
Voll, L. M., Zell, M. B., Engelsdorf, T., Saur, A., Gerrard Wheeler, M. C., Drincovich, M. F., et al. (2012). Loss of cytosolic NADP-malic enzyme 2 in Arabidopsis thaliana is associated with enhanced susceptibility towards Colletotrichum higginsianum. New Phytol. 195, 189–202. doi: 10.1111/j.1469-8137.2012.04129.x
Wohlbach, D. J., Quirino, B. F., and Sussman, M. R. (2008). Analysis of the Arabidopsis histidine kinase ATHK1 reveals a connection between vegetative osmotic stress sensing and seed maturation. Plant Cell 20, 1101–1117. doi: 10.1105/tpc.107.055871
Yazdanpanah, F., Hanson, J., Hilhorst, H. W. M., and Bentsink, L. (2017). Differentially expressed genes during the imbibition of dormant and after-ripened seeds - a reverse genetics approach. BMC Plant Biol. 17:151. doi: 10.1186/s12870-017-1098-z
Keywords: phytohormone, plant metabolism, root, seed development, water deficit
Citation: Arias CL, Pavlovic T, Torcolese G, Badia MB, Gismondi M, Maurino VG, Andreo CS, Drincovich MF, Gerrard Wheeler MC and Saigo M (2018) NADP-Dependent Malic Enzyme 1 Participates in the Abscisic Acid Response in Arabidopsis thaliana. Front. Plant Sci. 9:1637. doi: 10.3389/fpls.2018.01637
Received: 27 June 2018; Accepted: 22 October 2018;
Published: 06 November 2018.
Edited by:
Adriano Nunes-Nesi, Universidade Federal de Viçosa, BrazilReviewed by:
Jos Schippers, RWTH Aachen Universität, GermanyMarkus Teige, Universität Wien, Austria
Copyright © 2018 Arias, Pavlovic, Torcolese, Badia, Gismondi, Maurino, Andreo, Drincovich, Gerrard Wheeler and Saigo. This is an open-access article distributed under the terms of the Creative Commons Attribution License (CC BY). The use, distribution or reproduction in other forums is permitted, provided the original author(s) and the copyright owner(s) are credited and that the original publication in this journal is cited, in accordance with accepted academic practice. No use, distribution or reproduction is permitted which does not comply with these terms.
*Correspondence: Mariana Saigo, c2FpZ29AY2Vmb2JpLWNvbmljZXQuZ292LmFy