- 1Department of Plant and Environmental Sciences, Copenhagen Plant Science Centre, University of Copenhagen, Frederiksberg, Denmark
- 2CIBIO/InBIO – Research Centre in Biodiversity and Genetic Resources, University of Porto, Vairão, Portugal
- 3Department of Biology, University of Porto, Porto, Portugal
All living organisms require zinc as an essential micronutrient. Maintaining appropriate intracellular zinc supply, and avoiding deficiency or toxic excess, requires a tight regulation of zinc homeostasis. In Arabidopsis, bZIP19 and bZIP23 (basic-leucine zipper) transcription factors are the central regulators of the zinc deficiency response. Their targets include members of the ZIP (Zrt/Irt-like Protein) transporter family, involved in cellular zinc uptake, which are up-regulated at zinc deficiency. However, the mechanisms by which these transcription factors are regulated by cellular zinc status are not yet known. Here, to further our insight, we took advantage of the zinc deficiency hypersensitive phenotype of the bzip19 bzip23 double mutant, and used it as background to produce complementation lines of each Arabidopsis F-bZIP transcription factor, including bZIP24. On these lines, we performed complementation and localization studies, analyzed the transcript level of a subset of putative target genes, and performed elemental tissue profiling. We find evidence supporting that the zinc-dependent activity of bZIP19 and bZIP23 is modulated by zinc at protein level, in the nucleus, where cellular zinc sufficiency represses their activity and zinc deficiency is required. In addition, we show that these two transcription factors are functionally redundant to a large extent, and that differential tissue-specific expression patterns might, at least partly, explain distinct regulatory activities. Finally, we show that bZIP24 does not play a central role in the Zn deficiency response. Overall, we provide novel information that advances our understanding of the regulatory activity of bZIP19 and bZIP23.
Introduction
Zinc (Zn) is an essential micronutrient for all living organisms. It is a key structural and catalytic component of a large number of proteins, as cofactor in many enzymes, transcription factors and protein interaction domains. It is estimated that Zn-binding proteins make up to nearly 10% of the proteomes in eukaryotic cells (Coleman, 1992; Andreini et al., 2006). Maintaining appropriate intracellular Zn availability, and avoiding deficiency or toxic excess, requires a tight regulation of Zn uptake, transport, distribution and storage activities (Clemens, 2001). Such regulation of the Zn homeostasis network is finely tuned according to Zn intracellular levels, fluxes, and external fluctuations. One of the primary means by which cells can regulate their Zn levels is through Zn-dependent changes in the expression of genes required for Zn transport and storage (Choi and Bird, 2014). Members of the ZIP (Zrt/Irt-like Protein) and CDF (Cation Diffusion Facilitator) transporter families are ubiquitously used in eukaryotes for Zn uptake and efflux, into and out of the cytosol, respectively (Eide, 2006; Blindauer, 2015). Understanding how changes in cellular Zn status are sensed and affect the expression of Zn homeostasis genes is important, in particular to know how organisms respond to Zn deficiency.
Most knowledge on how eukaryotic cells sense Zn deficiency comes from yeast model systems. In Saccharomyces cerevisiae the Zn-responsive transcriptional activator Zap1 plays a central role in maintaining Zn homeostasis (Zhao et al., 1998). In Zn-limited cells Zap1 binds to Zinc Responsive Elements (ZRE) in target gene promoters activating gene expression. These include plasma membrane and tonoplast localized ZIP family members, among others, involved in increased Zn uptake transport to the cytosol. This regulatory activity involves independent mechanisms in which Zn binds activation domains (AD1, AD2) and the DNA binding domain of Zap1, resulting in either sensing of cellular Zn deficiency or limiting Zap1 activity in the presence of Zn (Zhao et al., 1998; Lyons et al., 2000; Bird et al., 2003; Frey et al., 2011).
In Arabidopsis thaliana (Arabidopsis), bZIP19 and bZIP23, two members of the basic-leucine zipper (bZIP) dimerizing transcription factor family, are involved in the regulation of Zn homeostasis, being the central regulators of the Zn deficiency response (Assunção et al., 2010; Inaba et al., 2015). They belong to the three-member F subfamily of Arabidopsis bZIP proteins (F-bZIP), which also includes bZIP24, known to be involved in salt stress tolerance regulation (Jakoby et al., 2002; Popova et al., 2008; Yang et al., 2009). The double mutant bzip19 bzip23 (bzip19/23) is hypersensitive to Zn deficiency and has no apparent phenotype on control growth conditions. The bZIP19 and bZIP23 bind to a 10 bp Zinc Deficiency Response Element (ZDRE, RTGTCGACAY) present in the promoter region of their putative target genes (Assunção et al., 2010) which include members of the Arabidopsis ZIP transporter family (i.e., ZIP1/3/4/5/9/10/12 and IRT3). Expression of these ZIP genes is up-regulated in response to Zn deficiency and is unresponsive in the bzip19/23 mutant background (Assunção et al., 2010). However, the specific physiological roles of ZIP transporters in plant Zn homeostasis is still incompletely understood (Sinclair and Krämer, 2012).
Among the ZIP putative target genes of bZIP19 and bZIP23, yeast complementation studies showed that ZIP1/3/4/12 and IRT3 mediate Zn uptake (Grotz et al., 1998; Lin et al., 2009; Assunção et al., 2010; Milner et al., 2013), and IRT3 and ZIP1 localize to the plasma membrane and vacuolar membrane, respectively (Lin et al., 2009; Milner et al., 2013). It is however ZIP4 that offered most insight on its role in the bZIP19/23 regulatory network. Both transcription factors bind to promoter fragments of ZIP4, containing two ZDRE motifs (Assunção et al., 2010), where the most upstream one is necessary for gene expression (Lin et al., 2016). Moreover, in vivo ZIP4 promoter GUS reporter assay, in wild-type and bzip19/23 mutant backgrounds, indicated that the bZIP19 and bZIP23 transcription factors act as the main regulators of ZIP4 (Castro et al., 2017). Together with a strong root and shoot GUS reporter expression of ZIP4 in response to Zn deficiency (Lin et al., 2016), the evidence points at an important role of ZIP4 transporter in the Zn deficiency response. The small set of bZIP19/23 putative target genes also include nicotianamine synthase (NAS) genes, NAS2 and NAS4, whose transcription is induced by Zn deficiency (Weber et al., 2004; van de Mortel et al., 2006; Assunção et al., 2010). Nicotianamine (NA) is produced by NAS enzyme and it is a low-molecular-weight metal chelator suggested to play an important role in iron (Fe) and Zn homeostasis, involved in their distribution and movement within the plant (Curie et al., 2009; Deinlein et al., 2012; Schuler et al., 2012; Clemens et al., 2013).
While gaining more knowledge on the target genes of bZIP19 and bZIP23, the mechanisms by which these transcription factors are regulated by cellular Zn status are still unknown (Assunção et al., 2013). Although there is a modest increase of bZIP19 and bZIP23 transcript levels at Zn deficiency (Assunção et al., 2010; Inaba et al., 2015), Zn might directly or indirectly affect protein function and regulate the transcription factors activity. This could involve, for example, protein subcellular localization, dimerization properties, DNA binding activity or protein-protein interactions (Choi and Bird, 2014). Here, to further our insight on the effect of cellular Zn status on the bZIP19 and bZIP23 activity, we took advantage of the Zn deficiency hypersensitive phenotype of the bzip19/23 double mutant, and used it as background to obtain stably transformed lines constitutively overexpressing each of the F-group bZIP genes. On these lines, we performed complementation and localization studies, analyzed the transcript level of a subset of putative target genes (ZIP4, and also ZIP1, ZIP5, NAS2 and NAS4), and performed elemental profiling of plant tissue. Our analyses also included complementation with bZIP24, the third member of the F-bZIPs, to examine any functional relation to the Zn deficiency response. The results provided novel information that advances our understanding of the Zn sensing and regulatory activity of bZIP19 and bZIP23.
Materials and Methods
Plasmid Construction and Plant Transformation
The pCaMV35S::bZIP-CFP-HA constructs for stable transformation of the Arabidopsis bzip19/23 mutant were generated as follows: the full-length CDS of bZIP19 (At4g35040), bZIP23 (At2g16770), and bZIP24 (At3g51960) were amplified from an Arabidopsis cDNA library using forward and reverse primers containing NotI and AscI restriction sites, respectively (Supplementary Table S1). The PCR products were purified by kit (PureLink, Invitrogen) and cloned into pGEM-T Easy vector using T4 DNA Ligase (Promega), then cloned into the NotI/AscI restriction enzyme sites of the pENTRTM/D-TOPO vector (Invitrogen), followed by in vitro site-directed recombination into pEarleyGate 102 Gateway vector (Earley et al., 2006), carrying a CaMV35S promoter, a C-terminal CFP fluorophore and HA-tag, using LR ClonaseTM II Enzyme Mix (Invitrogen). All constructs were verified by restriction enzyme digestion analysis and sequencing. The constructs were transformed into Agrobacterium tumefaciens strain GV3101 (pMP90) by electroporation and used for Arabidopsis bzip19/23 mutant transformation by the Floral dip method (Clough and Bent, 1998). Transgenic plants were selected by Basta (phosphinothricin) resistance and homozygous transgenic seeds (T3 generation) of three to four independent lines per construct, were selected. The overexpression of each F-bZIP gene was confirmed in the respective lines by real-time quantitative RT-PCR (RT-qPCR). The lines were referred to as bzip19/23-OE19, bzip19/23-OE23 and bzip19/23-OE24. The pCaMV35S::GFP-bZIP constructs for transient transformation of Nicotiana benthamiana leaves were generated as described above by in vitro site-directed recombination from the pENTRTM/D-TOPO constructs into pMCD43 Gateway vector (Curtis, 2003), carrying a CaMV35S promoter and a N-terminal GFP fluorophore, using LR ClonaseTM II Enzyme Mix (Invitrogen). Transformed A. tumefaciens clones, obtained as previously described and containing each construct confirmed by PCR, were grown overnight at 28°C in LB media supplemented with selective antibiotics. Cell cultures were centrifuged 15 min at 3000 g and the pellet suspended in MgCl2 10 mM solution to reach an OD600 of 0.5. Bacteria solutions were incubated with gentle shaking at room temperature for 2–3 h prior to syringe-inoculation into the leaves of 4–5 week old N. benthamiana plants at the abaxial side. In order to generate promoter-GUS fusion lines, the promoter regions (the whole intergenic region upstream the start codon) of bZIP19 and bZIP23 were amplified from Arabidopsis genomic DNA and cloned into the NotI/AscI restriction enzyme sites of the pENTRTM/D-TOPO vector as described above (Supplementary Table S1), followed by in vitro site-directed recombination into pBGWFS7 Gateway vector (Karimi et al., 2002) carrying a C-terminal GFP fluorophore and GUS reporter gene, using LR ClonaseTM II Enzyme Mix (Invitrogen). Transformation of A. tumefaciens and Arabidopsis were performed as previously described. Transgenic plants were selected by Basta resistance and seeds (T2 generation) of 10 independent lines per construct, were analyzed. They were referred to as pbZIP19::GUS and pbZIP23::GUS lines.
Plant Material and Growth Conditions
Arabidopsis wild-type (accession Columbia, Col-0) and the bzip19/23 double mutant (Col-0 background, descibed in Assunção et al., 2010) were used in all experiments. Synchronized seeds were stratified in water at 4°C for 3 days and surfaced sterilized by soaking for 5 min in 1 mL 70% (v/v) ethanol, followed by 10 min incubation in 1 mL of bleach solution [20% (v/v) commercial bleach with 3.5% (w/v) effective chloride; 0.1% (v/v) Tween-20]. Seeds were rinsed 5 times with sterile ultrapure water (Milli-Q Element, Merck) and resuspended in a sterile 0.25% (w/v) agarose solution. For the analysis with MS grown seedlings, sterilized seeds were sown on half-strength murashige and skoog (MS) medium with 1.5% (w/v) sucrose, 0.5 g L-1 MES and 1.2% (w/v) phyto-agar (Duchefa) at pH 5.7 in 120 mm square plastic Petri dishes, with 50 mL medium per plate, placed vertically. The glassware used for the preparation of the MS medium was previously rinsed with 0.1N HCl solution followed by 5 times rinsing with ultrapure water. The medium was prepared with ultrapure water and contained 15 μM ZnSO4 (control; Zn sufficient media) or no added Zn (-Zn; Zn-deficient media). The wild-type, bzip19/23 mutant, bzip19/23-OE19, bzip19/23-OE23, and bzip19/23-OE24 lines were grown together in plates (ca. 5 seedlings per genotype) at control or –Zn MS media, for 10 or 14 days. For each transformed line, three independent lines were tested, with at least four plates (replicates) per independent line and per Zn condition. For the analysis with hydroponically grown plants, sterilized seeds of the above mentioned lines were germinated on 1.5 mL plastic microtubes, cut open ca. 2 cm from the bottom and filled with 0.8% (w/v) phyto-agar solution. The microtubes were placed inside a hole at the lead of 50 mL plastic tubes cut open at the bottom, and these were placed in the lead of a 10 L container, which held 8 × 6 holes. A modified half-strength Hoagland nutrient solution was used, containing: 2 mM Ca(NO3)2, 1 mM MgSO4.7H2O, 3 mM KNO3, 1 mM KH2PO4, 25 μM Fe-Na-EDTA, 25 μM H3BO3, 3 μM MnSO4.H2O, 0.1 μM CuSO4.5H2O, 0.5 μM (NH4)6Mo7O24, 50 μM KCl, with 1 mM MES at pH 5.7, with either 2 μM ZnSO4.7H2O (control; Zn sufficient media) or with 0.002 μM ZnSO4.7H2O (-Zn; Zn-deficient media). The plastic containers, for the control and –Zn treatments, were rinsed with 0.1 N HCl solution followed by 5 times rinsing with ultrapure water prior to use, and the nutrient solutions were prepared with ultrapure water. Seeds were germinated and grown for 8 weeks in either control or -Zn nutrient solution, with 6 plants per genotype, in a 10 L container, which was aerated throughout the experiment. The nutrient solutions were replaced once a week, during the first 4 weeks, and twice in the weeks thereafter. The hydroponics setup and the MS plates were in a growth chamber with 8/16 h and 16/8 h light/dark cycle, respectively, 125 μmol m-2 s-1 white light, 22/20°C light/dark temperature, and 70% relative humidity.
Subcellular Localization Analysis
Ten-day-old seedlings of wild-type, bzip19/23 mutant, bzip19/23-OE19, bzip19/23-OE23 and bzip19/23-OE24 lines were grown in control or –Zn MS medium. For laser scanning confocal microscopy (LSCM) analysis, roots were transferred to microscope slides containing propidium iodide (PI) to stain root cell walls and were visualized using a laser scanning confocal inverted microscope Leica TCS SP5 II (Leica Microsystems) with a HC PL APO CS 63x /1.30 Glycerine objective. Ar 458 nm and 514 nm laser lines were used for CFP and PI excitation, respectively. The emission settings were between 470–500 nm for CFP and 590–630 nm for PI. For each transformed line, three independent lines were tested, with observations of ca. 2–3 seedlings per independent line and Zn condition. For the analysis with N. benthamiana, three plants were infiltrated per construct and were visualized 4–5 days after infiltration using a laser scanning confocal microscope Leica TCS SP5-X (Leica Microsystems) with a HCX PL APO 20x /0.70 water objective. White light laser at 480 nm was used for GFP excitation. The emission settings were between 500–580 nm for GFP, 631–721 nm for far-red with transmission (bright filed) also recorded.
Real-Time Quantitative RT-PCR Analysis
Fourteen-day-old seedlings of wild-type, bzip19/23 mutant, bzip19/23-OE19, bzip19/23-OE23 and bzip19/23-OE24 lines, grown in control or –Zn MS medium were harvested and immediately frozen in liquid nitrogen in pools of 5 seedlings per line and per Zn treatment × 3 different plates grown simultaneously and considered as biological replica. Seedlings were grinded in a microtube in liquid nitrogen, with the help of polypropylene pestles. Total RNA was extracted using the RNeasy Plant Mini Kit (QIAGEN), and RNA quantity and integrity were assessed using both a Nanodrop spectrophotometer (Thermo Scientific), and standard agarose-gel electrophoretic analysis. The RNA samples were treated with Recombinant DNase I (Sigma-Aldrich) and first strand cDNA synthesis was generated from 0.5 μg total RNA using a SuperScriptTM III First-Strand Synthesis SuperMix (Invitrogen). Primers for RT-qPCR (Supplementary Table S2) were designed using NCBI Primer-BLAST1 and the primer amplification efficiency for each primer pair was between 1.9 and 2.1. RT-qPCR was performed with a LightCycler 96 Real-Time PCR Systtem (Roche Diagnostics) in 96-well plates, using HOT FIREPol EvaGreen qPCR Mix (Solis BioDyne) in the PCR reaction mixture, according to manufacturer’s instructions (i.e., final volume of 20 μL containing 4 μL of the EvaGreen qPCR mix, 1 μL of 10 μM primers, and 1 μL of a 1/10 dilution of cDNA as template). The PCR conditions were as follows: 95oC for 15 min, followed by 40 cycles of 95oC for 15 s, 60oC for 20 s, and 72oC for 20 s, with a final step at 98oC. ACT2 (At3g18780) was used as the reference gene. Reactions were performed in 2–3 technical replicas per biological replica and in 3 biological replica per line and per Zn treatment. The calculated cycle threshold (Ct) value for each gene was normalized to the control ACT2 gene calculated Ct value. The relative transcript levels were expressed against the wild-type seedlings grown at control conditions, and calculated according to the Livak 2-ΔΔCT method (Livak and Schmittgen, 2001).
Tissue Elemental Analysis by ICP-MS
Shoot and root fresh tissue was harvested from 8-week-old hydroponically grown plants from wild-type, bzip19/23 mutant, bzip19/23-OE19, bzip19/23-OE23 and bzip19/23-OE24 lines, grown at control or –Zn nutrient solution. Roots were desorbed with ice-cold 1 mM CaCl2 solution for 15 min followed by 3 times washing with ultrapure water for 1 min. Shoot and root tissue from 4 plants per line were harvested separately, blotted dry, dried for 3 days at 60°C and the shoot and root total dry weight measured. For bzip19/23 mutant and bzip19/23-OE24 lines grown at –Zn, 6 plants were harvested, following the same procedure, and were analyzed in pools of 2. Tissue digestion was performed with ultra-pure acids (70% HNO3 and 15% H2O2) at 240°C and 80 bars for 15 min in a pressurized microwave oven (Ultrawave, Milestone Inc.) enabling the amount of acid and final dilution to be adjusted according to the amount of plant material (Olsen et al., 2016). All samples were diluted to 3.5% HNO3 prior to multi-elemental analysis using Inductively-Coupled Plasma Mass Spectrometry (ICP-MS) (7900 ICP-MS, Agilent Technologies) run in collision mode (Helium). The ICP-MS was equipped with a SeaSpray nebuliser and a double pass scott type spray chamber, moreover, automatic sample introduction was performed from an 89 position Integrated autosampler (Agilent Technologies). Certified reference material (NIST1515, apple leaf, National Institute of Standards and Technology, United States) was included to evaluate data quality and drift correction was performed based on drift samples for every 10 samples. Data were processed using Agilent ICP Masshunter Software version 4.3.
Histochemical Staining for β-Glucuronidase (GUS) Assay
Histochemical GUS staining was performed in 14-day-old seedlings of pbZIP19::GUS and pbZIP23::GUS lines grown in control or –Zn MS medium. Seedlings were immersed in GUS staining solution containing 50 mM phosphate buffer, 10 mM Na2-EDTA, 20% (v/v) methanol, 0.1% (v/v) Triton X-100, 1.4 mM K3[Fe(CN)6], 1.4 mM K4[Fe(CN)6].3H2O with 1.9 mM X-Gluc and incubated overnight at 37oC in the dark (Jefferson et al., 1987). After incubation, the pigments were removed by repeated incubations in 50, 70, and 96% (v/v) ethanol successively, and seedlings were stored in 70% (v/v) glycerol.
Statistical Analysis
One-way ANOVA followed by Tukey’s post hoc test, and Student’s t-test were calculated with IBM SPSS Statistics V22.0 software.
Results
Complementation Study in bzip19/23 Double Mutant Background
In order to obtain complementation lines of the Arabidopsis F-bZIPs (bZIP19, bZIP23 and bZIP24) in the bzip19/23 double mutant background, we produced stably transformed lines expressing each of the F-bZIPs cDNA under control of the constitutive CaMV 35S promoter and containing a C-terminal CFP fluorophore (i.e., bzip19/23-OE19, bzip19/23-OE23 and bzip19/23-OE24). We found an increased transcript level of the overexpressed genes in these lines (Supplementary Figure S1), and a western blot (WB) anti-HA, albeit only tested in the bzip19/23-OE19 line, confirmed the expected protein molecular weight for the bZIP19-CFP-HA expressed protein (Supplementary Figure S2 and Supplementary Methods). The latter also indicated a similar protein amount at control and Zn-deficient growth conditions.
To test the complementation of the bzip19/23 mutant Zn deficiency hypersensitive phenotype, the lines were grown in hydroponics under control or Zn-deficient conditions. The bzip19/23-OE19 and bzip19/23-OE23 lines complemented the bzip19/23 phenotype at Zn deficiency, displaying normal growth, comparable to the wild-type. The bzip19/23-OE24 line, on the other hand, showed severe growth impairment under Zn deficiency, comparable with the bzip19/23 mutant (Figure 1A). The plants were allowed to grow 8 weeks before harvesting plant material for tissue elemental analyses. Throughout the experiment, the same phenotype pattern was maintained, with bzip19/23 and bzip19/23-OE24 plants displaying an even more severe Zn deficiency hypersensitive phenotype. The rosette of bzip19/23-OE24 plants appeared however slightly bigger than the ones from the bzip19/23 mutant (Figure 1B). The tissue dry weight data were in agreement with these observations (Figures 1C,D). We also analyzed the lines at control or Zn-deficient MS medium, with bzip19/23-OE19 and bzip19/23-OE23 seedlings complementing the bzip19/23 mutant, whereas the 5-day-old and 14-day-old bzip19/23-OE24 seedlings, at Zn deficiency, displayed shorter root length than the wild-type, but did not show the Zn deficiency phenotype of bzip19/23 mutant (Supplementary Figures S3A,B).
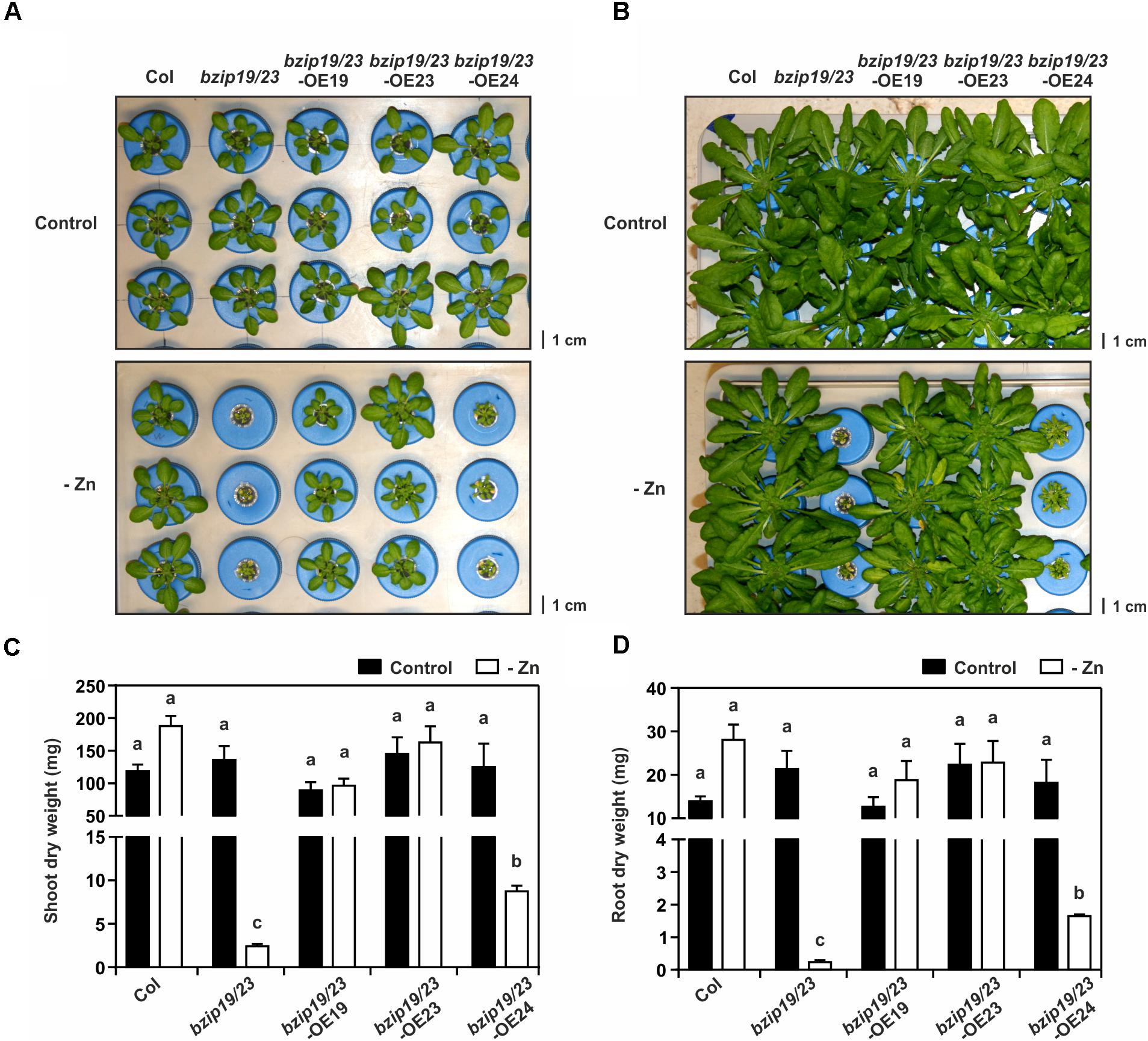
Figure 1. Complementation study with Arabidopsis bzip19/23-OE19, bzip19/23-OE23 and bzip19/23-OE24 lines, and wild-type (Col) and bzip19/23 double mutant lines, grown in hydroponics with control (control) or Zn-deficient (-Zn) nutrient solution. Phenotype of 4-week-old plants (A) and 8-week-old plants (B), Shoot (C), and root (D) dry weight (mg) of 8-week-old plants grown at control (black bar) or –Zn (open bar). Data are represented as means ± SE (n = 4 for all treatments, except bzip19/23 and bzip19/23-OE24 at –Zn with n = 3). Different letters indicate significant differences (p < 0.05) after one-way ANOVA followed by Tukey’s post hoc test.
Expression and Subcellular Localization Analysis Under Different Zn Supply
We analyzed the expression of bZIP19, bZIP23, and bZIP24 in wild-type seedlings grown at control or Zn deficiency. Their transcript levels were in line with previous data (Assunção et al., 2010), and indicated a small but not significant increase in bZIP19 and bZIP23 transcript abundance at Zn deficiency, not visible in bZIP24 (Figure 2).
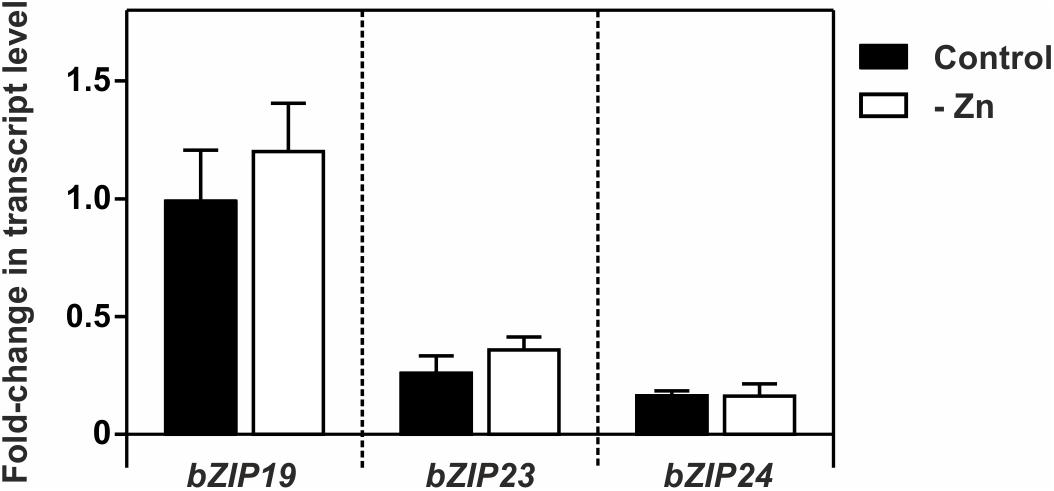
Figure 2. Transcript level profiles of Arabidopsis bZIP19, bZIP23 and bZIP24 using real-time quantitative RT-PCR, in 14-day-old wild-type seedlings grown in control (control, dark bars) or Zn-deficient (-Zn, open bars) MS medium. Bars represent mean fold-change in transcript level of three biological replica ± SE. There were no statistically significant differences between control and –Zn, determined by Student t-test.
We further investigated the subcellular localization of the Arabidopsis F-bZIP proteins, and whether it is affected by cellular Zn status, in seedlings of bzip19/23-OE19, bzip19/23-OE23 and bzip19/23-OE24 lines, grown at control or Zn-deficiency (Supplementary Figure S3). The fluorescence of the C-terminal CFP fluorophore was visualized with confocal laser scanning microscopy (CLSM). The fluorescent signal for the three F-bZIP protein fusions (bZIP19-CFP, bZIP23-CFP, and bZIP24-CFP) localized at the cell nucleus. Our analysis also revealed that the subcellular localization pattern observed for all F-bZIPs was identical between seedlings grown at control or Zn-deficiency (Figure 3). In addition, we performed a localization analysis using transient expression in N. benthamiana leaves with each Arabidopsis F-bZIP cDNA under control of the constitutive CaMV 35S promoter and containing an N-terminal GFP fluorophore. We again found a nuclear localization for the three F-bZIP proteins, thus showing agreement between the results with a C- and N-terminal fluorophore (Supplementary Figure S4).
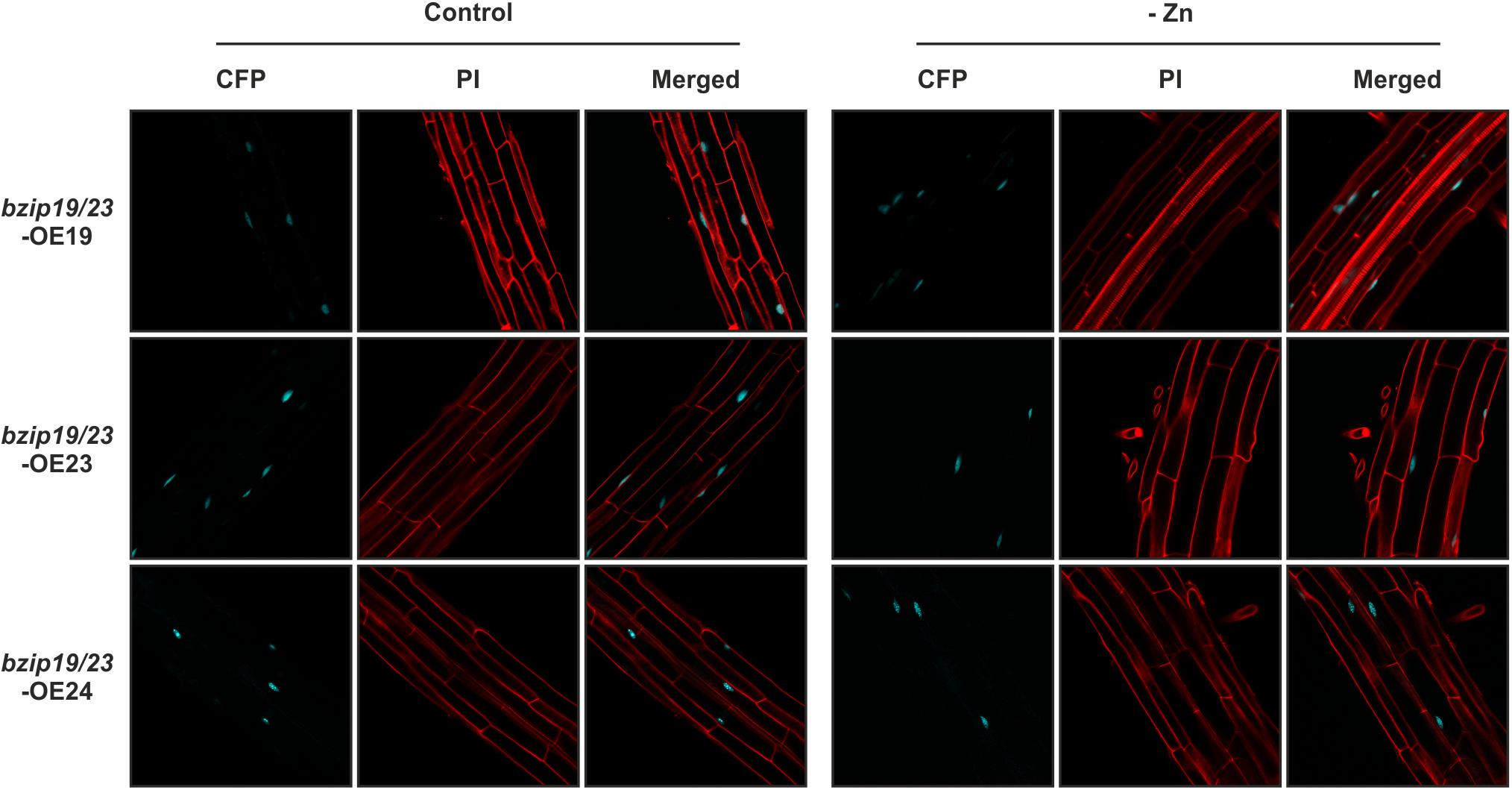
Figure 3. Subcellular localization analysis of bZIP19-CFP, bZIP23-CFP, and bZIP24-CFP fusion proteins in roots of 10-day-old seedlings of Arabidopsis bzip19/23-OE19, bzip19/23-OE23, and bzip19/23-OE24 lines, respectively, grown in control (control) or Zn-deficient (-Zn) MS medium. Emissions of CFP and propidium iodide (PI) were visualized with confocal laser scanning microscopy (CLSM).
Expression Analysis of bZIP19/23 Putative Target Genes
Next, we investigated the expression of a subset of the bZIP19 and bZIP23 putative target genes (ZIP1, ZIP4, ZIP5, NAS2, and NAS4), in seedlings of bzip19/23-OE19, bzip19/23-OE23 and bzip19/23-OE24 lines, grown at control or Zn-deficiency. The analysis of ZIP1, ZIP4, and ZIP5 showed induction of gene expression at Zn deficiency in the wild-type, in particular for ZIP4 and ZIP5, whereas their transcript levels in the bzip19/23 mutant, in both Zn conditions, were comparable or lower to the wild-type at control. In bzip19/23-OE19 and bzip19/23-OE23 lines their expression profiles were overall comparable with the wild-type, though with higher transcript level for ZIP1 and ZIP4 in bzip19/23-OE19. The bzip19/23-OE24 line, on the other hand, exhibited transcript levels comparable with the bzip19/23 mutant, but showing a small induction of ZIP4 and ZIP5 at Zn deficiency (Figures 4A–C). The analysis of NAS2 and NAS4 showed expression profiles in the different lines comparable to that of the ZIP genes. The wild-type showed Zn deficiency induction, though not significant for NAS4, whereas bzip19/23 had transcript levels comparable or lower to the wild-type at control. The expression of NAS2 and NAS4 in bzip19/23-OE19 and bzip19/23-OE23 lines were comparable with the wild-type, but with a pronounced Zn deficiency induction of NAS4 and with higher transcript levels in bzip19/23-OE19. In the bzip19/23-OE24 line the transcript levels of NAS2 were comparable with the bzip19/23 mutant, whereas NAS4 exhibited some increased expression at both Zn conditions (Figures 4D,E).
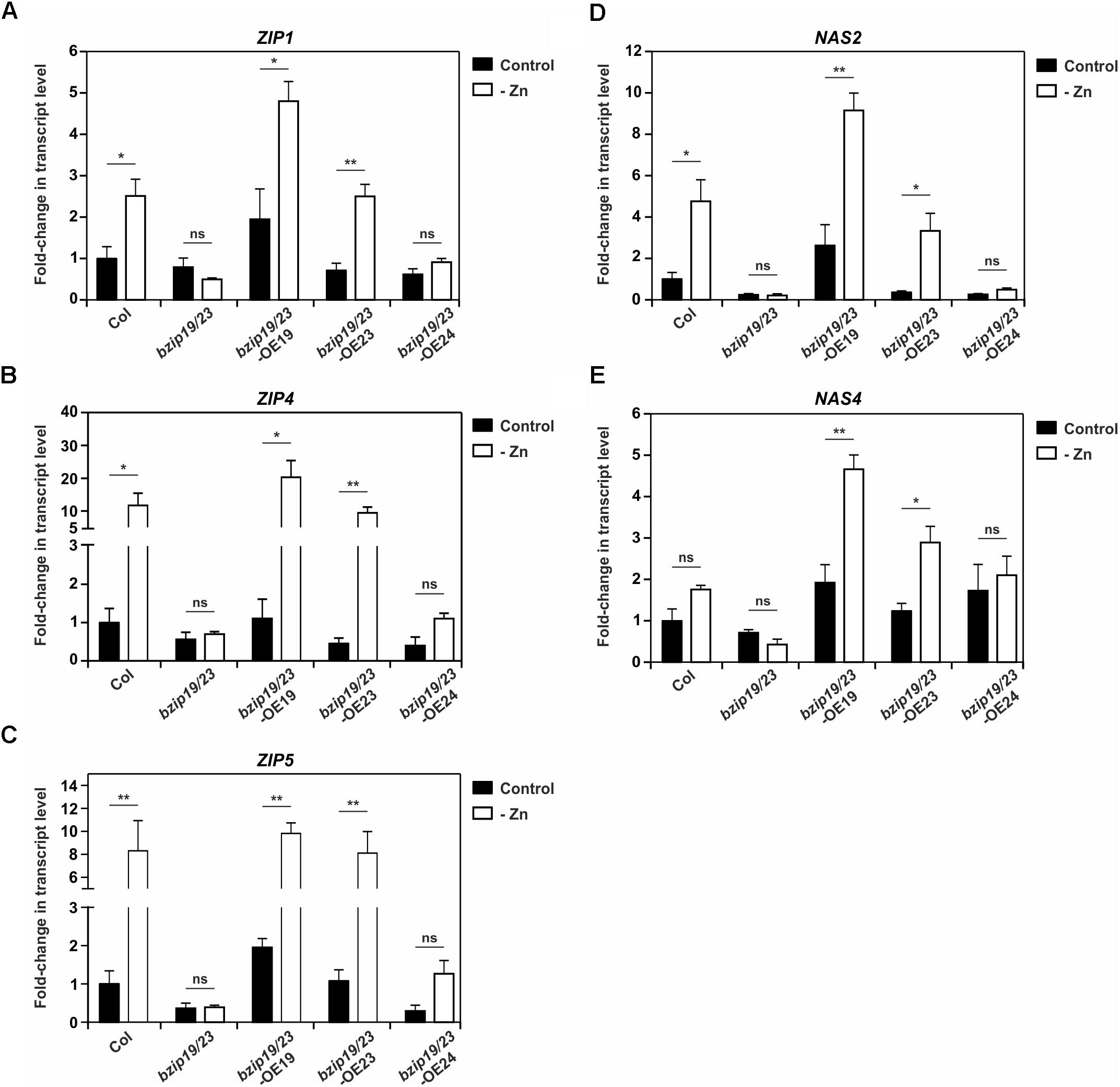
Figure 4. Transcript level profiles of Arabidopsis bZIP19 and bZIP23 putative target genes in 14-day-old seedlings of wild-type (Col), bzip19/23 double mutant, bzip19/23-OE19, bzip19/23-OE23 and bzip19/23-OE24 lines, grown in control (control, dark bars) or Zn-deficient (-Zn, open bars) MS medium. Real-time quantitative RT-PCR was used to determine the transcript levels of ZIP1 (A), ZIP4 (B), ZIP5 (C), NAS2 (D), and NAS4 (E). Bars represent mean fold-change in transcript level of three biological replica ± SE. Statistically significant differences between control and –Zn were determined by Student t-test (∗p < 0.05; ∗∗p < 0.01; ns, not significant).
Tissue Elemental Analysis of Hydroponically Grown Plants
To further investigate the complementation of the bzip19/23 mutant with the Arabidopsis F-bZIPs, we analyzed the elemental profiles of shoot and root tissue from the bzip19/23-OE19, bzip19/23-OE23, and bzip19/23-OE24 lines grown in hydroponics at control or Zn-deficiency. The root tissue harvested from the bzip19/23 mutant plants was extremely small, in line with the severity of the Zn deficiency phenotype (Figure 1), not being adequate to include in the analysis. The results from the elemental profiling revealed that, at control conditions, the element concentrations in shoots and roots did not differ strongly between wild-type, mutant and complementation lines (Figure 5 and Supplementary Table S3).
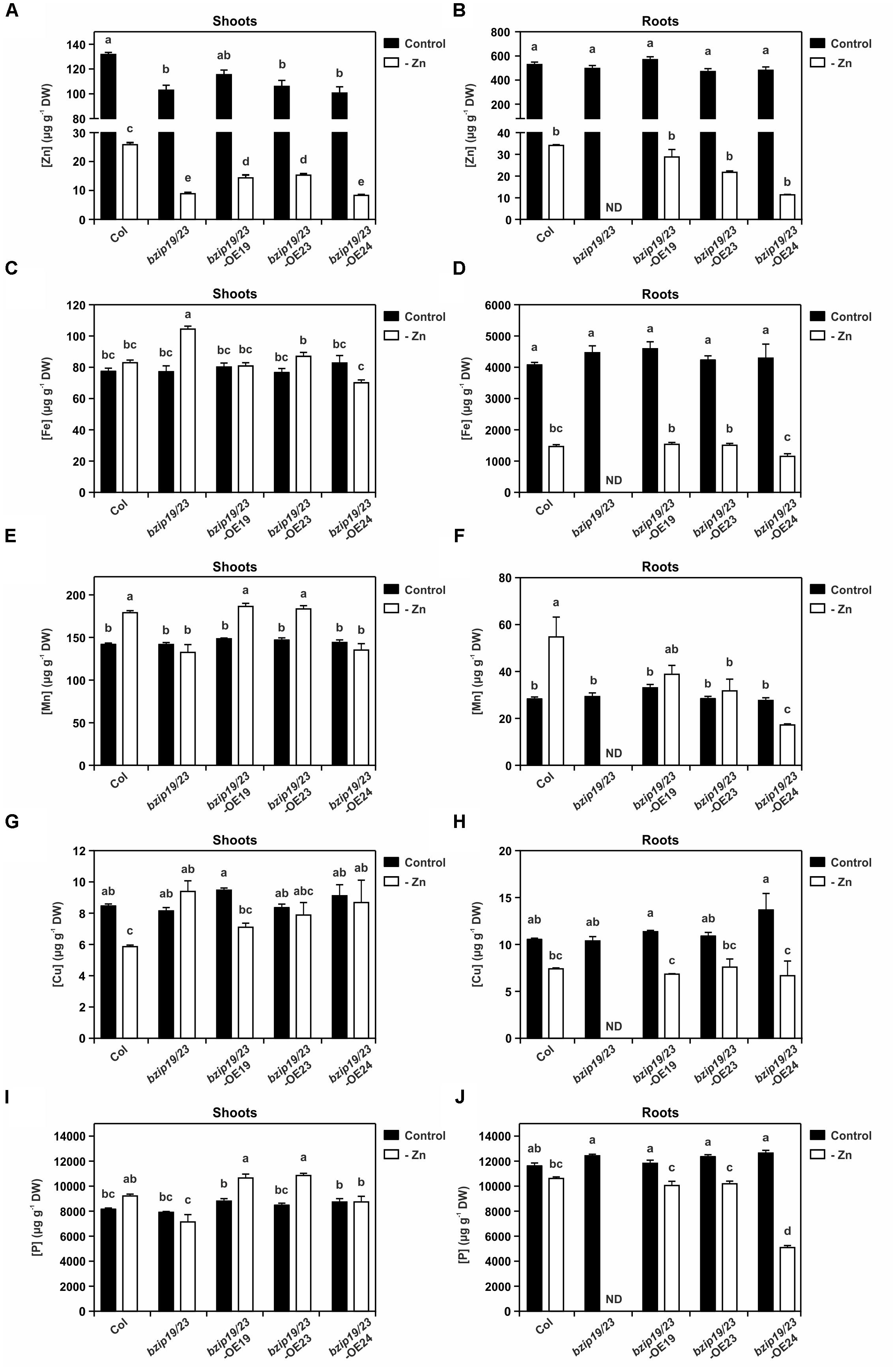
Figure 5. Tissue elemental profiling determined with inductively coupled plasma-mass spectrometry (ICP-MS) in 8-week-old plants of Arabidopsis wild-type (Col), bzip19/23 double mutant, bzip19/23-OE19, bzip19/23-OE23 and bzip19/23-OE24 lines, grown in hydroponics with control (control, dark bars) or Zn-deficient (-Zn, open bars) nutrient solution. Zn concentration in shoots (A), and in roots (B), Fe concentration in shoots (C), and in roots (D), Mn concentration in shoots (E), and in roots (F), Cu concentration in shoots (G), and in roots (H), P concentration in shoots (I), and in roots (J), in μg g-1 dry weight (DW). Data are represented as means ±SE (sample sizes as in Figure 1, except for bzip19/23 roots at –Zn, with no data, ND). Different letters indicate significant differences (p < 0.05) after one-way ANOVA followed by Tukey’s post hoc test.
At Zn deficiency however, there were significant differences in tissue element concentrations between the lines. The Zn content in shoots of bzip19/23 mutant and bzip19/23-OE24 lines was significantly lower than in the wild-type and the bzip19/23-OE19 and bzip19/23-OE23 lines, with these having lower content than the wild-type (Figure 5A). Overall, shoot and root Zn content in all lines at Zn deficiency decreased to ca. 0.1 that of the control (Figures 5A,B). With respect to other analyzed elements, the Fe content in shoots at Zn-deficient conditions was similar to control conditions, except for the bzip19/23 mutant and bzip19/23-OE24, which were significantly higher and lower, respectively (Figure 5C). The Fe content in roots of wild-type and complementation lines, on the other hand, decreased at Zn deficiency to ca. 0.3 that of the control (Figure 5D). The manganese (Mn) content in shoots of wild-type, bzip19/23-OE19 and bzip19/23-OE23 lines at Zn deficiency was significantly higher than bzip19/23 mutant and bzip19/23-OE24 lines. These exhibited similar content in both Zn conditions, whereas wild-type, bzip19/23-OE19 and bzip19/23-OE23 lines increased to ca. 1.3 that of the control (Figure 5E). Also in roots, the Mn content at Zn deficiency increased in wild-type, in bzip19/23-OE19 and, to a lesser extent, in bzip19/23-OE23, and decreased in bzip19/23-OE24 in relation to control (Figure 5F). The content of copper (Cu) in roots of wild-type and complementation lines at Zn deficiency decreased to ca. 0.6 that of the control, while in shoots it decreased in wild-type and bzip19/23-OE19 lines (Figures 5G,H). The phosphorus (P) content in shoots of wild-type, bzip19/23-OE19 and bzip19/23-OE23 lines at Zn deficiency increased to ca. 1.2 that of the control, whereas bzip19/23 mutant and bzip19/23-OE24 remained comparable at both Zn conditions (Figure 5I). In roots, the wild-type and complementation lines showed a decrease in P content from control to Zn deficiency, which was more pronounced in bzip19/23-OE24 than in the wild-type, bzip19/23-OE19 and bzip19/23-OE23 lines (Figure 5J).
Tissue Expression Analysis of bZIP19 and bZIP23
Lastly, we studied tissue-specific expression of bZIP19 and bZIP23 by producing stably transformed lines in wild-type Arabidopsis background with the bZIP19 or bZIP23 promoters fused to β-glucuronidase (GUS), pbZIP19::GUS and pbZIP23::GUS lines (Figure 6A). The histochemical GUS staining assay of seedlings grown at control or Zn-deficiency revealed differences between the two lines. The pbZIP19::GUS seedlings showed a stronger reporter GUS expression in roots than in shoots, whereas the pbZIP23::GUS seedlings showed the opposite pattern, with a stronger expression in shoots than in roots (Figure 6B). In addition, there were no differences in the reporter GUS expression between seedlings grown at control or Zn-deficiency for both lines.
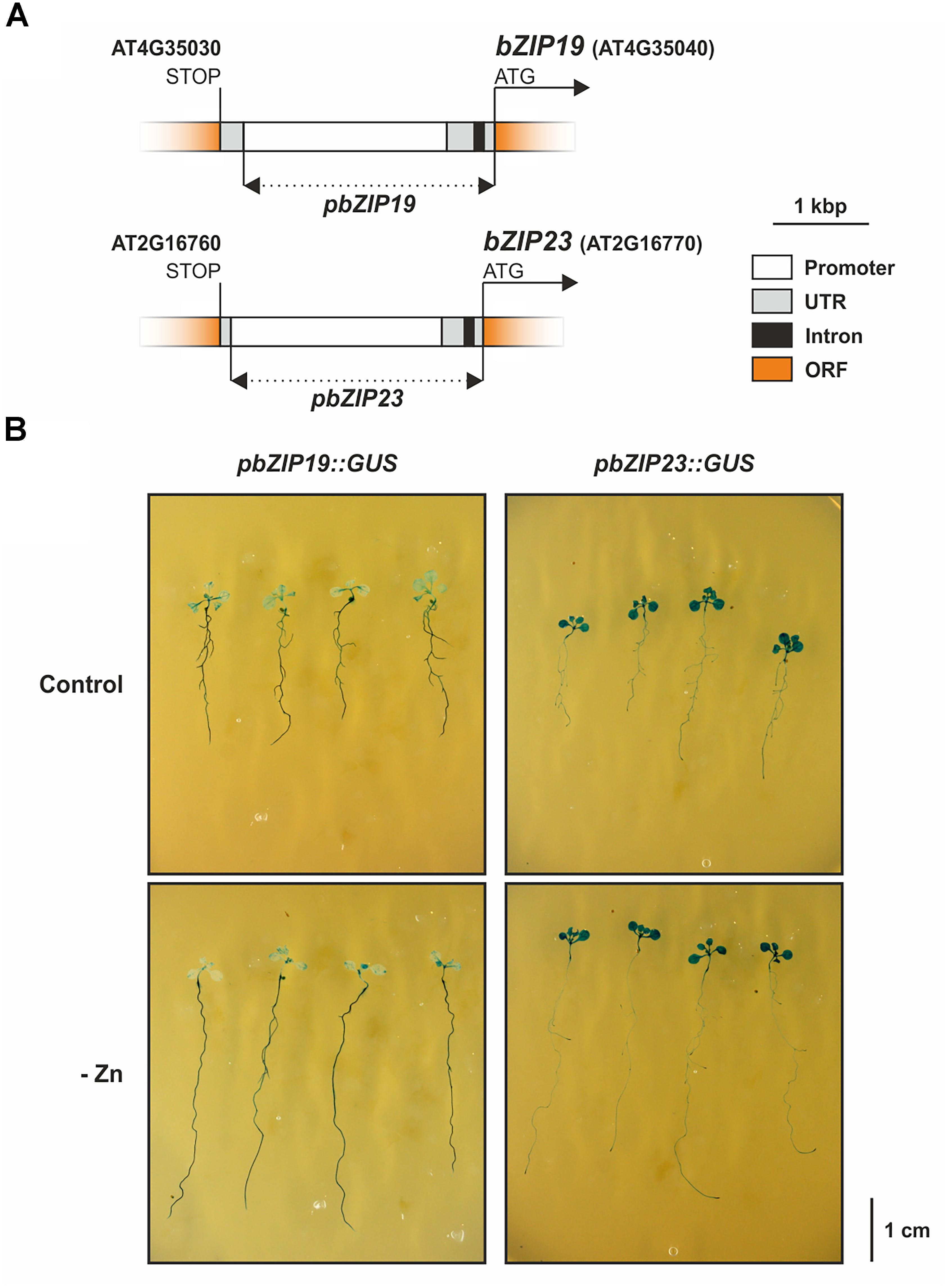
Figure 6. Arabidopsis bZIP19 and bZIP23 tissue expression analysis with promoter-reporter gene fusions. Schematic representation of the bZIP19 and bZIP23 promoter regions, represented by a dashed line, cloned for promoter::GUS fusion constructs (A), histochemical staining for β-glucuronidase (GUS) assay in 14-day-old seedlings of pbZIP19::GUS and pbZIP23::GUS lines, grown in control (control) or Zn-deficient (-Zn) MS medium (B).
Discussion
The Activity of bZIP19 and bZIP23 Transcription Factors Requires Zn Deficiency Sensing
We found a modest effect of Zn supply on the bZIP19 and bZIP23 transcript levels, and no noticeable effect for bZIP24, in line with previous report (Assunção et al., 2010). In addition, our promoter-reporter fusion analysis of bZIP19 and bZIP23 showed no visible differences between seedlings grown at control or Zn-deficient conditions. This suggests that the Zn-dependent activity of bZIP19 and bZIP23 is not modulated by Zn at the transcriptional level. At the protein level, our results showed a nuclear localization of bZIP19 and bZIP23 in roots of the bzip19/23-OE19 and bzip19/23-OE23 lines. This is in line with previously reported nuclear localization of bZIP19 and bZIP23 in roots of Arabidopsis bZIP19-GFP and bZIP23-GFP overexpressor lines (Inaba et al., 2015). We further showed that the protein nuclear localization is unaffected when seedlings are grown at control or Zn-deficiency. This strongly indicates that changes in cellular Zn level do not interfere with protein subcellular localization, and likely do not interfere with protein stability. Thus a Zn-dependent change in protein subcellular localization does not seem to be a mechanism by which Zn regulates the activity of bZIP19 and bZIP23 transcription factors.
The expression profiles of a subset of putative target genes of bZIP19/23 provided novel insight on how cellular Zn status impacts on bZIP19 and bZIP23 activity. As previously known, the bzip19/23 mutant fails to induce the expression of ZIP1, ZIP4, ZIP5, NAS2, and NAS4 at Zn deficiency (Assunção et al., 2010; Castro et al., 2017). Our transcript level analyses showed that the wild-type expression patterns were generally restored in the bzip19/23-OE19 and bzip19/23-OE23 lines, and also, their tissue elemental analysis showed a largely similar pattern to the wild-type. This is consistent with the complementation of the bzip19/23 mutant in these lines, supporting the role of bZIP19 and bZIP23 as central players in the Zn deficiency response. Interestingly, our results showed target gene expression induction at Zn-deficiency in bzip19/23-OE19 and bzip19/23-OE23 lines, likewise in the wild-type, suggesting that the activity of bZIP19 and bZIP23 transcription factors requires Zn deficiency and is repressed by cellular Zn sufficiency. Both transcription factors contain a region that is rich in cysteine and histidine residues located at their N-terminus which was hypothesized to be a Zn-binding motif (Assunção et al., 2013). This motif would act as a Zn-sensor, modulating bZIP19 and bZIP23 activity (e.g., affecting dimerization properties, DNA binding activity or protein-protein interactions) according to cellular Zn status (Assunção et al., 2013). Our results are in line with this hypothesis, and overall they indicate that the Zn-dependent activity of bZIP19 and bZIP23 are not controlled at the transcriptional level, but instead cellular Zn status modulates their activity at the protein level. This modulation does not seem to involve subcellular targeting, but cellular Zn sufficiency limits or represses their activity and, conversely, Zn deficiency is required for target gene expression.
Further Insight on bZIP19 and bZIP23 Regulatory Activity
The expression of ZIP1, ZIP4, ZIP5, NAS2, and NAS4 in the bzip19/23-OE19 and bzip19/23-OE23 lines reinforces their role as target genes of bZIP19 and bZIP23. They are likely playing a role in Zn transport and Zn NA-mediated distribution (Guerinot, 2000; Clemens et al., 2013) in response to Zn deficiency, and they all contain ZDRE-motifs, to which bZIP19 and bZIP23 bind to, in their promoter regions (Assunção et al., 2010). The regulation of NAS genes seems to be complex and controlled by multiple mechanisms, comprising direct or indirect regulation by FIT (FER-Like Iron Deficiency-Induced Transcription Factor), the key regulator in the Arabidopsis Fe deficiency response (Bauer et al., 2007; Palmer et al., 2013; Chen et al., 2018), being suggested to balance the interrelationship of Fe and Zn homeostasis (Chen et al., 2018). We observed that the position of NAS2 and NAS4 promoter ZDRE-motif is at the 5′UTR, comprising the ATG start codon, which might be difficult to integrate with a transcriptional activation function of bZIP19 and bZIP23, adding complexity to their regulation mechanism.
The complementation of the bzip19/23 mutant shows that bZIP19 and bZIP23 can form functional homodimers and suggests redundancy between them, as previously reported (Assunção et al., 2010). However, analyses of single mutants, i.e., bzip19 and bzip23, showed a phenotype in bzip19 suggesting partial redundancy instead (Assunção et al., 2010; Inaba et al., 2015). Detailed analysis on these single mutants suggested that, in addition to a common set of ZIP target genes, bZIP19 and bZIP23 could also regulate distinct genes, being ZIP9, ZIP4, ZIP5 predominantly regulated by bZIP19, and ZIP12 mainly regulated by bZIP23 (Inaba et al., 2015). Our results indicated nonetheless a similar performance between bzip19/23-OE19 and bzip19/23-OE23 lines, where their target genes expression analysis and tissue elemental profiling revealed an overall comparable pattern between the two lines. This supports that bZIP19 and bZIP23 transcription factors are, to a large extent, functionally redundant. Possibly, an overlapping or a distinct regulatory activity between bZIP19 and bZIP23 might instead rely, at least partially, on their tissue- and cellular-specific expression patterns. In line with this, our results showed that there is differential tissue-specific expression, with bZIP19 more expressed in roots and bZIP23 more expressed in shoots. Perhaps a more relevant role for bZIP19 in the Zn deficiency response in roots could explain the reported bzip19 Zn deficiency phenotype (Assunção et al., 2010; Inaba et al., 2015). Further studies are necessary to verify the bZIP19 and bZIP23 specific expression patterns under different developmental stages and growth conditions.
The tissue elemental analysis of the different lines showed, as expected, a drastic decrease in Zn tissue content at Zn deficiency. Interestingly, the difference in shoot Zn concentration between the bzip19/23 mutant and bzip19/23-OE24 lines, exhibiting a severe Zn deficiency phenotype, and the wild-type, bzip19/23-OE19 and bzip19/23-OE23 lines, not exhibiting a visible Zn deficiency phenotype, is well in line with the reported critical deficiency level below 15–20 μg g-1 DW in leaves (Marschner, 1995). The element concentration profiling in shoots and roots did not differ strongly between all the lines at control conditions, but at Zn deficiency it provided interesting additional insight, pointing at possible interactions between the Zn deficiency regulatory network and other micronutrients homeostasis. In particular, results showed that the concentration of Mn in shoots significantly increased under Zn deficiency in wild-type, as previously observed (Campos et al., 2017), and also in the bzip19/23-OE19 and bzip19/23-OE23 lines, while there was no variation in the bzip19/23 mutant between control and Zn deficiency. The ZIP1 gene, target of bZIP19 and bZIP23, encodes the ZIP1 Zn transporter which is implicated in the transport of Mn, possibly in the remobilization from the vacuole (Grotz et al., 1998; Milner et al., 2013). Considering that ZIP family members are able to mediate the transport of a range of micronutrient cations, including Zn, Fe, Mn, Cu (Guerinot, 2000; Wintz et al., 2003; Eide, 2006), our results strongly suggest that bZIP19/23 targets could be involved in Mn uptake and transport, at least under Zn deficiency. Moreover, results showed a decrease in the concentration of Cu in wild-type shoots at Zn deficiency, as previously reported (Campos et al., 2017), also observed in bzip19/23-OE19, and to a lesser extent, bzip19/23-OE23 lines, but not in the bzip19/23 mutant, suggesting crosstalk between the bZIP19/23 regulatory network and Cu homeostasis, which deserves further dissection.
In the well-documented interaction between Zn and inorganic phosphorus (Pi) homeostasis, Zn-deficient plants overaccumulate Pi in the shoot due to increased Pi uptake and/or distribution (Bouain et al., 2014). In line with this, our tissue P analysis showed, at Zn deficiency, an increase in shoot P concentration in the wild-type, bzip19/23-OE19 and bzip19/23-OE3 lines, accompanied by a decrease in root P concentration. Recently, bZIP23 was shown to be involved as a repressor in a novel pathway regulating Pi acquisition under Zn deficiency, through binding to a ZDRE-motif variant to which bZIP19 cannot bind (Kisko et al., 2018). This new ZDRE-motif is located at the 5′UTR of LPCAT1 gene, which plays a key role in coordinating Pi homeostasis, suggesting that the binding of bZIP23 might physically block gene transcription under Zn deficiency (Kisko et al., 2018). Our results revealed no variation in shoot P content in the bzip19/23 mutant between control and Zn deficiency, supporting the involvement of bZIP23 in this regulatory pathway. However, we did not find significant differences between the bzip19/23-OE19 and bzip19/23–OE23 lines in shoot and root P content suggesting that bZIP19 can perform the same regulatory activity as bZIP23.
bZIP24 Does Not Play a Central Role in the Zn Deficiency Response
In addition to bZIP19 and bZIP23, the Arabidopsis bZIP F subfamily contains bZIP24, with the three members sharing a high degree of amino acid sequence similarity (Jakoby et al., 2002). Phylogenetic analysis of F-bZIP members across land plants suggests a monophyletic origin prior to seed plant evolution, and branching out of two clades: Group 1 and Group 2 (Castro et al., 2017). The bZIP19 and bZIP23, likely two paralogs resulting from a duplication event, are included in Group 1, whereas bZIP24 is in Group 2 and appears to have undergone diversifying selection (Castro et al., 2017). bZIP24 is suggested to be a negative regulator in salt stress induced acclimation responses, involved in regulating the maintenance of osmotic and ionic balance (Yang et al., 2009). We included bZIP24 in our experiments to examine any functional relation to the bZIP19 and bZIP23 Zn deficiency response. Our results showed that bZIP24 was unable to fully complement the bzip19/23 mutant, which is consistent with the Zn deficiency hypersensitive phenotype of bzip19/23 despite the endogenous bZIP24. Previously, bZIP24 was shown to form homodimers, and to localize in the nucleus and cytosol in roots of Arabidopsis bZIP24-GFP overexpressor line, with the signal in the nucleus increasing upon salt treatment (Yang et al., 2009). Our results revealed that, in addition to no effect of Zn status at the transcription level, the subcellular localization analysis in roots of the bzip19/23-OE24 line showed bZIP24 in the nucleus with no alteration upon Zn treatment.
Both bzip19/23 mutant and bzip19/23-OE24 lines suffered from severe Zn deficiency in the hydroponically grown plants. The expression profiles of ZIP and NAS family members clearly showed that the bzip19/23-OE24 line had overall a pattern of expression comparable with the bzip19/23 mutant. The tissue elemental profiling was also generally comparable between the two lines. A slightly less severe Zn deficiency phenotype was observed in the rosette of the bzip19/23-OE24 plants, whose seedlings showed a better performance than the bzip19/23 mutant. It can be hypothesized that these observations rely on a modest improvement of Zn uptake and an improved distribution within the plant by NA (Clemens et al., 2013), since bzip19/23-OE24 in relation to bzip19/23 mutant showed a small expression increase of ZIP4, ZIP5 and NAS4 genes, while the Zn concentration, at least in the shoot, of these two lines remained comparable at Zn deficiency. Nonetheless, with respect to the Zn deficiency response, our results showed that the Group 2 bZIP24 does not play a central role in the Zn deficiency response, contrary to the Group 1 bZIP19 and bZIP23, although a contribution to Zn homeostasis cannot be ruled out.
Conclusion
We used complementation lines as a tool to further our insight on the Zn sensing and regulatory mechanism of bZIP19 and bZIP23 transcription factors. Our findings indicate that the Zn-dependent activities of bZIP19 and bZIP23 are not controlled at the transcriptional level, but at the protein level where cellular Zn status modulates their activity. This does not seem to involve interference with their nuclear localization, where cellular Zn deficiency is required for target gene expression and Zn sufficiency limits or represses their activity. We provided evidence that the two transcription factors are, to a large extent, functionally redundant, and that distinct regulatory activities may rely on their differential tissue-specific expression patterns. Our results with complementation lines also unveiled interesting indications of interactions with other micronutrient homeostasis networks, which deserve further research. Finally, we showed that the bZIP24 is not a key player in the Zn deficiency response, as bZIP19 and bZIP23 are. These Group 1 F-bZIP orthologs are possibly functionally conserved across land plants (Assunção et al., 2010; Castro et al., 2017). Considering that Zn deficiency in agricultural soils, crops and human diet represents a global challenge2 furthering knowledge on these regulatory mechanisms is of fundamental and practical importance.
Author Contributions
GHL, PHC, and AGLA designed the research, performed experimental work, and analyzed the data. AC performed confocal microscopy and data analysis. AGLA drafted the manuscript revised by all authors.
Funding
This research was funded by the Danish Council for Independent Research, DFF-YDUN program (4093–00245B) and by the Portuguese Foundation for Science and Technology, FCT-IF program (IF/01641/2014) (GHL, PHC, AC, and AGLA). Analysis by ICP-MS was performed at CHEMI Center at PLEN, University of Copenhagen. Imaging data was collected at the Center for Advanced Bioimaging at PLEN, and Advanced Light Microscopy Platform at I3S, University of Porto.
Conflict of Interest Statement
The authors declare that the research was conducted in the absence of any commercial or financial relationships that could be construed as a potential conflict of interest.
Acknowledgments
We thank Jan K. Schjørring and Herlander Azevedo for critical reading of the manuscript.
Supplementary Material
The Supplementary Material for this article can be found online at: https://www.frontiersin.org/articles/10.3389/fpls.2018.01955/full#supplementary-material
Footnotes
References
Andreini, C., Banci, L., Bertini, I., and Rosato, A. (2006). Zinc through the three domains of life. J. Proteome Res. 5, 3173–3178. doi: 10.1021/pr0603699
Assunção, A. G. L., Herrero, E., Lin, Y.-F., Huettel, B., Talukdar, S., Smaczniak, C., et al. (2010). Arabidopsis thaliana transcription factors bZIP19 and bZIP23 regulate the adaptation to zinc deficiency. Proc. Natl. Acad. Sci. U.S.A. 107, 10296–10301. doi: 10.1073/pnas.1004788107
Assunção, A. G. L., Persson, D. P., Husted, S., Schjørring, J. K., Alexander, R. D., and Aarts, M. G. M. (2013). Model of how plants sense zinc deficiency. Metallomics 5, 1110–1116. doi: 10.1039/c3mt00070b
Bauer, P., Ling, H.-Q., and Guerinot, M. L. (2007). FIT, the FER-LIKE iron deficiency induced transcription factor in Arabidopsis. Plant Physiol. Biochem. 45, 260–261. doi: 10.1016/j.plaphy.2007.03.006
Bird, A. J., McCall, K., Kramer, M., Blankman, E., Winge, D. R., and Eide, D. J. (2003). Zinc fingers can act as Zn2+ sensors to regulate transcriptional activation domain function. EMBO J. 22, 5137–5146. doi: 10.1093/emboj/cdg484
Blindauer, C. A. (2015). Advances in the molecular understanding of biological zinc transport. Chem. Commun. 51, 4544–4563. doi: 10.1039/c4cc10174j
Bouain, N., Shahzad, Z., Rouached, A., Khan, G. A., Berthomieu, P., Abdelly, C., et al. (2014). Phosphate and zinc transport and signalling in plants: toward a better understanding of their homeostasis interaction. J. Exp. Bot. 65, 5725–5741. doi: 10.1093/jxb/eru314
Campos, A. C. A. L., Kruijer, W., Alexander, R., Akkers, R. C., Danku, J., Salt, D. E., et al. (2017). Natural variation in Arabidopsis thaliana reveals shoot ionome, biomass, and gene expression changes as biomarkers for zinc deficiency tolerance. J. Exp. Bot. 68, 3643–3656. doi: 10.1093/jxb/erx191
Castro, P. H., Lilay, G. H., Munoz-Merida, A., Schjoerring, J. K., Azevedo, H., and Assunção, A. G. L. (2017). Phylogenetic analysis of F-bZIP transcription factors indicates conservation of the zinc deficiency response across land plants. Sci. Rep. 7:3806. doi: 10.1038/s41598-017-03903-6
Chen, C.-L., Cui, Y., Cui, M., Zhou, W.-J., Wu, H.-L., and Ling, H.-Q. (2018). A FIT-binding protein is involved in modulating iron and zinc homeostasis in Arabidopsis. Plant. Cell Environ. 41, 1698–1714. doi: 10.1111/pce.13321
Choi, S., and Bird, A. J. (2014). Zinc’ing sensibly: controlling zinc homeostasis at the transcriptional level. Metallomics 6, 1198–1215. doi: 10.1039/C4MT00064A
Clemens, S. (2001). Molecular mechanisms of plant metal tolerance and homeostasis. Planta 212, 475–486. doi: 10.1007/s004250000458
Clemens, S., Deinlein, U., Ahmadi, H., Höreth, S., and Uraguchi, S. (2013). Nicotianamine is a major player in plant Zn homeostasis. BioMetals 26, 623–632. doi: 10.1007/s10534-013-9643-1
Clough, S. J., and Bent, A. F. (1998). Floral dip: a simplified method for Agrobacterium-mediated transformation of Arabidopsis thaliana. Plant J. 16, 735–743. doi: 10.1046/j.1365-313x.1998.00343.x
Coleman, J. E. (1992). Zinc proteins: enzymes, storage proteins, transcription factors, and replication proteins. Annu. Rev. Biochem. 61, 897–946. doi: 10.1146/annurev.bi.61.070192.004341
Curie, C., Cassin, G., Couch, D., Divol, F., Higuchi, K., Le Jean, M., et al. (2009). Metal movement within the plant: contribution of nicotianamine and yellow stripe 1-like transporters. Ann. Bot. 103, 1–11. doi: 10.1093/aob/mcn207
Curtis, M. D. (2003). A gateway cloning vector set for high-throughput functional analysis of genes in planta. Plant Physiol. 133, 462–469. doi: 10.1104/pp.103.027979
Deinlein, U., Weber, M., Schmidt, H., Rensch, S., Trampczynska, A., Hansen, T. H., et al. (2012). Elevated nicotianamine levels in Arabidopsis halleri roots play a key role in zinc hyperaccumulation. Plant Cell 24, 708–723. doi: 10.1105/tpc.111.095000
Earley, K. W., Haag, J. R., Pontes, O., Opper, K., Juehne, T., Song, K., et al. (2006). Gateway-compatible vectors for plant functional genomics and proteomics. Plant J. 45, 616–629. doi: 10.1111/j.1365-313X.2005.02617.x
Eide, D. J. (2006). Zinc transporters and the cellular trafficking of zinc. Biochim. Biophys. Acta 1763, 711–722. doi: 10.1016/j.bbamcr.2006.03.005
Frey, A. G., Bird, A. J., Evans-Galea, M. V., Blankman, E., Winge, D. R., and Eide, D. J. (2011). Zinc-regulated DNA binding of the yeast Zap1 zinc-responsive activator. PLoS One 6:e22535. doi: 10.1371/journal.pone.0022535
Grotz, N., Fox, T., Connolly, E., Park, W., Guerinot, M. L., and Eide, D. (1998). Identification of a family of zinc transporter genes from Arabidopsis that respond to zinc deficiency. Proc. Natl. Acad. Sci. U.S.A. 95, 7220–7224. doi: 10.1073/pnas.95.12.7220
Guerinot, M. L. (2000). The ZIP family of metal transporters. Biochim. Biophys. Acta 1465, 190–198. doi: 10.1016/S0005-2736(00)00138-3
Inaba, S., Kurata, R., Kobayashi, M., Yamagishi, Y., Mori, I., Ogata, Y., et al. (2015). Identification of putative target genes of bZIP19, a transcription factor essential for Arabidopsis adaptation to Zn deficiency in roots. Plant J. 84, 323–334. doi: 10.1111/tpj.12996
Jakoby, M., Weisshaar, B., Dröge-Laser, W., Vicente-Carbajosa, J., Tiedemann, J., Kroj, T., et al. (2002). bZIP transcription factors in Arabidopsis. Trends Plant Sci. 7, 106–111. doi: 10.1016/S1360-1385(01)02223-3
Jefferson, R. A., Kavanagh, T. A., and Bevan, M. W. (1987). GUS fusions: beta-glucuronidase as a sensitive and versatile gene fusion marker in higher plants. EMBO J. 6, 3901–3907. doi: 10.1002/j.1460-2075.1987.tb02730.x
Karimi, M., Inzé, D., and Depicker, A. (2002). GATEWAY vectors for Agrobacterium-mediated plant transformation. Trends Plant Sci. 7, 193–195. doi: 10.1016/S1360-1385(02)02251-3
Kisko, M., Bouain, N., Safi, A., Medici, A., Akkers, R. C., Secco, D., et al. (2018). LPCAT1 controls phosphate homeostasis in a zinc-dependent manner. eLife 7:e32077. doi: 10.7554/eLife.32077
Lin, Y.-F., Hassan, Z., Talukdar, S., Schat, H., and Aarts, M. G. M. (2016). Expression of the ZNT1 zinc transporter from the metal hyperaccumulator Noccaea caerulescens confers enhanced zinc and cadmium tolerance and accumulation to Arabidopsis thaliana. PLoS One 11:e0149750. doi: 10.1371/journal.pone.0149750
Lin, Y.-F., Liang, H.-M., Yang, S.-Y., Boch, A., Clemens, S., Chen, C.-C., et al. (2009). Arabidopsis IRT3 is a zinc-regulated and plasma membrane localized zinc/iron transporter. New Phytol. 182, 392–404. doi: 10.1111/j.1469-8137.2009.02766.x
Livak, K. J., and Schmittgen, T. D. (2001). Analysis of relative gene expression data using real-time quantitative PCR and the 2-ΔΔCT method. Methods 25, 402–408. doi: 10.1006/meth.2001.1262
Lyons, T. J., Gasch, A. P., Gaither, L. A., Botstein, D., Brown, P. O., and Eide, D. J. (2000). Genome-wide characterization of the Zap1p zinc-responsive regulon in yeast. Proc. Natl. Acad. Sci. U.S.A. 97, 7957–7962. doi: 10.1073/pnas.97.14.7957
Milner, M. J., Seamon, J., Craft, E., and Kochian, L. V. (2013). Transport properties of members of the ZIP family in plants and their role in Zn and Mn homeostasis. J. Exp. Bot. 64, 369–381. doi: 10.1093/jxb/ers315
Olsen, L. I., Hansen, T. H., Larue, C., Østerberg, J. T., Hoffmann, R. D., Liesche, J., et al. (2016). Mother-plant-mediated pumping of zinc into the developing seed. Nat. Plants 2:16036. doi: 10.1038/nplants.2016.36
Palmer, C. M., Hindt, M. N., Schmidt, H., Clemens, S., and Guerinot, M. L. (2013). MYB10 and MYB72 are required for growth under iron-limiting conditions. PLoS Genet. 9:e1003953. doi: 10.1371/journal.pgen.1003953
Popova, O. V., Yang, O., Dietz, K.-J., and Golldack, D. (2008). Differential transcript regulation in Arabidopsis thaliana and the halotolerant Lobularia maritima indicates genes with potential function in plant salt adaptation. Gene 423, 142–148. doi: 10.1016/j.gene.2008.07.017
Schuler, M., Rellán-Álvarez, R., Fink-Straube, C., Abadía, J., and Bauer, P. (2012). Nicotianamine functions in the phloem-based transport of iron to sink organs, in pollen development and pollen tube growth in Arabidopsis. Plant Cell 24, 2380–2400. doi: 10.1105/tpc.112.099077
Sinclair, S. A., and Krämer, U. (2012). The zinc homeostasis network of land plants. Biochim. Biophys. Acta 1823, 1553–1567. doi: 10.1016/j.bbamcr.2012.05.016
van de Mortel, J. E., Almar Villanueva, L., Schat, H., Kwekkeboom, J., Coughlan, S., Moerland, P. D., et al. (2006). Large expression differences in genes for iron and zinc homeostasis, stress response, and lignin biosynthesis distinguish roots of Arabidopsis thaliana and the related metal hyperaccumulator Thlaspi caerulescens. Plant Physiol. 142, 1127–1147. doi: 10.1104/pp.106.082073
Weber, M., Harada, E., Vess, C., Roepenack-Lahaye, E. V., and Clemens, S. (2004). Comparative microarray analysis of Arabidopsis thaliana and Arabidopsis halleri roots identifies nicotianamine synthase, a ZIP transporter and other genes as potential metal hyperaccumulation factors. Plant J. 37, 269–281. doi: 10.1046/j.1365-313X.2003.01960.x
Wintz, H., Fox, T., Wu, Y.-Y., Feng, V., Chen, W., Chang, H.-S., et al. (2003). Expression profiles of Arabidopsis thaliana in mineral deficiencies reveal novel transporters involved in metal homeostasis. J. Biol. Chem. 278, 47644–47653. doi: 10.1074/jbc.M309338200
Yang, O., Popova, O. V., Süthoff, U., Lüking, I., Dietz, K.-J., and Golldack, D. (2009). The Arabidopsis basic leucine zipper transcription factor AtbZIP24 regulates complex transcriptional networks involved in abiotic stress resistance. Gene 436, 45–55. doi: 10.1016/j.gene.2009.02.010
Keywords: zinc deficiency, Arabidopsis thaliana, F-bZIP, regulation, ZIP transporters, plant nutrition
Citation: Lilay GH, Castro PH, Campilho A and Assunção AGL (2019) The Arabidopsis bZIP19 and bZIP23 Activity Requires Zinc Deficiency – Insight on Regulation From Complementation Lines. Front. Plant Sci. 9:1955. doi: 10.3389/fpls.2018.01955
Received: 17 August 2018; Accepted: 17 December 2018;
Published: 22 January 2019.
Edited by:
Felipe Klein Ricachenevsky, Universidade Federal de Santa Maria, BrazilReviewed by:
Ping Lan, Institute of Soil Science (CAS), ChinaAnn Cuypers, University of Hasselt, Belgium
Ana Carolina Atala Lombelo Campos, Dümmen Orange, Netherlands
Copyright © 2019 Lilay, Castro, Campilho and Assunção. This is an open-access article distributed under the terms of the Creative Commons Attribution License (CC BY). The use, distribution or reproduction in other forums is permitted, provided the original author(s) and the copyright owner(s) are credited and that the original publication in this journal is cited, in accordance with accepted academic practice. No use, distribution or reproduction is permitted which does not comply with these terms.
*Correspondence: Ana G. L. Assunção, agla@plen.ku.dk
†Present address: Pedro Humberto Castro, CIBIO/InBIO – Research Centre in Biodiversity and Genetic Resources, University of Porto, Vairão, Portugal