- 1Department of Biochemistry, Max-Planck Institute for Chemical Ecology, Jena, Germany
- 2Research Group Mass Spectrometry/Proteomics, Max-Planck Institute for Chemical Ecology, Jena, Germany
The pea aphid (Acyrthosiphon pisum), a phloem-sucking insect, has undergone a rapid radiation together with the domestication and anthropogenic range expansion of several of its legume host plants. This insect species is a complex of at least 15 genetically different host races that can all develop on the universal host plant Vicia faba. However, each host race is specialized on a particular plant species, such as Medicago sativa, Trifolium pratense, or Pisum sativum, which makes it an attractive model insect to study ecological speciation. Previous work revealed that pea aphid host plants produce a specific phytohormone profile depending on the host plant – host race combination. Native aphid races induce lower defense hormone levels in their host plant than non-native pea aphid races. Whether these changes in hormone levels also lead to changes in other metabolites is still unknown. We used a mass spectrometry-based untargeted metabolomic approach to identify plant chemical compounds that vary among different host plant-host race combinations and might therefore, be involved in pea aphid host race specialization. We found significant differences among the metabolic fingerprints of the four legume species studied prior to aphid infestation, which correlated with aphid performance. After infestation, the metabolic profiles of M. sativa and T. pratense plants infested with their respective native aphid host race were consistently different from profiles after infestation with non-native host races and from uninfested control plants. The metabolic profiles of P. sativum plants infested with their native aphid host race were also different from plants infested with non-native host races, but not different from uninfested control plants. The compounds responsible for these differences were putatively identified as flavonoids, saponins, non-proteinogenic amino acids and peptides among others. As members of these compound classes are known for their activity against insects and aphids in particular, they may be responsible for the differential performance of host races on native vs. non-native host plants. We conclude that the untargeted metabolomic approach is suitable to identify candidate compounds involved in the specificity of pea aphid – host plant interactions.
Introduction
Insects are the most diverse group of eukaryotic species on earth (Stork, 1993), and herbivorous species constitute a major group of insects (Strong et al., 1984; Mitter et al., 1988). Many herbivorous insect species are specialized on certain plant species (Bernays and Graham, 1988). Even within a single insect species, specialization can occur with different populations feeding preferentially on different plant taxa (Drès and Mallet, 2002). Adaptation to multiple plant species can lead to the formation of host races or biotypes within a species of an insect herbivore and might therefore play an important role in insect speciation. Nevertheless, the mechanisms behind these adaptations are hardly understood so far. Why is a certain host race able to feed on one plant but not another one? To some extent, differences in the constitutive chemical profiles of the plant species might be crucial (Hegnauer, 2001). However, various plants can also react differently to a specific insect herbivore (Arimura et al., 2005; Wu and Baldwin, 2010; Hogenhout and Bos, 2011; Sanchez-Arcos et al., 2016). Such differential plant responses can become apparent in changes in i.e., the plant metabolome (Jansen et al., 2009; Tzin et al., 2015).
Secondary metabolites used by plants as defenses against herbivores can act directly as feeding deterrents or toxins that decrease food intake or food-utilization efficiency (Gabrys and Tjallingii, 2002; Kim et al., 2008), decrease survival (Behmer et al., 2011), or indirectly as attractants for natural enemies of herbivores (reviewed in Unsicker et al., 2009). Chemical compounds involved in direct and indirect plant defense include terpenoids, phenolics, cyanogenic glycosides, glucosinolates, and alkaloids (Dreyer et al., 1985; Avé et al., 1987; Zagrobelny et al., 2004; Unsicker et al., 2009; Mithöfer and Boland, 2012; Maag et al., 2015; Züst and Agrawal, 2016). Specialized insect herbivores have to somehow cope with the presence of defensive secondary metabolites in their host plants, and have evolved specific adaptations to enable feeding (Ehrlich and Raven, 1964; Berenbaum and Zangerl, 1998).
To evaluate the potential defensive effects of plant metabolites on insect herbivores, the identity and concentration of these substances must be measured. Targeted analyses can be used for such studies if previous knowledge suggests that a specific metabolite or metabolite class like flavonoids or glucosinolates might be important. However, in some cases previous knowledge about the chemical composition of the plants involved or compounds relevant to herbivores is not available. In these cases the untargeted investigation of the whole metabolome by the use of metabolomics, might be a good tool to reveal candidate chemicals that are the cause of different plant-insect interactions (Fiehn, 2002; Krastanov, 2010; Nakabayashi and Saito, 2013).
One especially interesting plant-insect herbivore system to study the role of plant metabolites for speciation is the interaction between the pea aphid complex (Acyrthosiphon pisum Harris, Homoptera, Aphididae) and its legume (Fabaceae) host plants. The pea aphid underwent a rapid diversification about 6500–9500 years ago (Peccoud et al., 2009b) that led to the development of at least 15 different sympatric host races or biotypes each specialized on one or a few legume host plants (called native host plants) on which they perform well and prefer for feeding (Peccoud et al., 2009a, 2015). Such host plant preferences lead to assortative mating and reproductive isolation among populations (Caillaud and Via, 2000; Peccoud et al., 2009a). However, all pea aphid host races can perform well on Vicia faba, which is considered to be the universal host plant for all pea aphid host races characterized to date. The existence of the different host races and the fact that the pea aphid genome is entirely sequenced (The International Aphid Genomics Consortium, 2010) has made the pea aphid a model for studying ecological speciation (Brisson and Stern, 2006; Peccoud and Simon, 2010) and provides the opportunity to investigate the mechanisms underlying host plant adaptation.
Pea aphids like all aphids feed on phloem sap. With their sucking mouthparts called stylets they navigate through the epidermis and mesophyll to reach the phloem. During this penetration process they pierce many plant cells, salivate into the cells and also suck tiny amounts of cell contents (Tjallingii and Esch, 1993; Martin et al., 1997). It is assumed that aphid salivary proteins are involved in the adaptation of pea aphid host races to host plants (Jaquiery et al., 2012). Recent studies with other aphid species have revealed a close relation between proteins secreted with the saliva into the plant and host plant reactions (Rodriguez and Bos, 2013; Kaloshian and Walling, 2016). While several aphid proteins were found to facilitate aphid feeding (Will et al., 2007; Bos et al., 2010; Atamian et al., 2013; Elzinga et al., 2014; Naessens et al., 2015); other aphid proteins induce defense reactions in the plant and could lead to an incompatible aphid – plant interaction (Chaudhary et al., 2014; Elzinga et al., 2014). Concerning the pea aphid host races and their adaptation to their native hosts, despite the efforts made to investigate the role of candidate saliva proteins on host plant adaptation (Jaquiery et al., 2012; Guy et al., 2016; Boulain et al., 2018; Nouhaud et al., 2018) saliva proteins important for host plant specialization are not yet known. However, it is known that legumes differ in their production of defense hormones depending on whether native or non-native pea aphid host races are feeding on plants (Sanchez-Arcos et al., 2016). These hormone differences might lead to changes in plant metabolomes, especially for compounds having a deterrent or toxic impact on aphids.
Several studies show that plant secondary compounds have detrimental effects on pea aphids (Züst and Agrawal, 2016). For example, higher levels of saponins and phenolic compounds led to a reduction of aphid population growth (Golawska et al., 2006; Golawska and Lukasik, 2009) and increased mortality (De Geyter et al., 2012). Diverse flavonoid glycosides reduced aphid fecundity (Golawska and Lukasik, 2012; Golawska et al., 2012a), while nitrogen-containing compounds caused a rejection of a potential host plant by pea aphids (Kordan et al., 2012). Although these targeted approaches revealed that some secondary metabolites affect pea aphid performance, in most of these studies just one plant species was used and often the pea aphid host race was unknown. Thus, the contribution of plant compounds to the maintenance and performance of pea aphid host races on legume plants is still largely unknown. Only one study (Hopkins et al., 2017) combined a metabolomic profiling approach with behavioral tests to understand the chemical signatures that underlie host preferences by A. pisum. This study investigated the metabolome of uninfested plants of different plant species belonging to the genera Medicago and Trifolium, but was limited to constitutive defense compounds or other compounds important for initial acceptance.
To pave the way for later targeted analyses in which the contribution of plant metabolites to the maintenance and performance of pea aphid host races on different legume plants could be analyzed, we applied an untargeted mass spectrometry-based metabolomic approach. Polar and semi-polar fractions of three native host plants of the pea aphid, Medicago sativa, Pisum sativum, Trifolium pratense, and the universal host Vicia faba, each infested with their native or one of two non-native aphid host races were analyzed and compared to fractions of uninfested control plants. These data made it possible to evaluate the use of metabolomics in identifying plant metabolites potentially involved in determining host race-host plant interactions in pea aphids.
Materials and Methods
Plant Material
Four legume plant species, Medicago sativa (alfalfa) cultivar (cv.) “Giulia,” Trifolium pratense (red clover) cv. “Dajana,” Pisum sativum (pea) cv. “Baccara,” and Vicia faba (broad bean) cv. “The Sutton,” were grown in 10 cm diameter plastic pots with a standardized soil mixture (7:20 mixture of Klasmann Tonsubstrat and Klasmann Kultursubstrat TS1, Klasmann-Deilmann GmbH, Geeste, Germany), in climate chambers at 20°C, 70 ± 10% relative humidity, and under a 16 h light/8 h dark photoperiod. The plants were watered twice a week. To have a sufficient amount of plant material for the extraction of metabolites, M. sativa and T. pratense plants were used 4 weeks after sowing, while P. sativum and V. faba were used 3 weeks after sowing.
Aphids
Three pea aphid (Acyrthosiphon pisum Harris) clones, each representing one pea aphid host race, were used in the experiments: the clone L84 representing the Medicago race (here called MR), the clone T3-8V1 representing the Trifolium race (TR), and the clone Colmar representing the Pisum race (PR). Aphids were initially collected from their native host plants T. pratense, M. sativa, and P. sativum, respectively, and genotypically assigned to their respective host race (for detailed information see Table S1 in Peccoud et al., 2009b). All aphids were reared on 4 week old broad bean plants. To synchronize the age of the aphids for the experiments, five apterous female adults were placed on a broad bean plant and were allowed to reproduce for 48 h and were then removed from the plants. Nymphs were kept on the plants for 9 days until they reached adulthood. Then they were transferred to new plants were they reproduced. This procedure was repeated until enough synchronized young adult aphids were available for the experiment. To avoid escape of aphids, all aphid-containing plants were covered with air permeable cellophane bags (18.8 × 39 cm, Armin Zeller, Nachf. Schütz & Co, Langenthal, Switzerland), and placed in a climate chamber under the same conditions described for the plant material.
Experimental Design
Five adult apterous female aphids of each host race were placed in magnetic clip-cages (Ø 3.5 cm), on leaves of each plant species (two leaves for M. sativa and T. pratense, one leaf for P. sativum and V. faba plants). Leaves from all four plant species enclosed in magnetic clip cages but without aphids served as controls (Supplementary Figure 1). Ten replicates of each combination were employed. All the infested and control plants were placed in climate chambers at 20°C, 70 ± 10% relative humidity, and under a 16 h light/8 h dark photoperiod. Plant material was sampled after 48 h, a period which allowed the aphids to settle and the plant to react to the aphid infestation (Sanchez-Arcos et al., 2016).
Plant Material Sampling and Metabolite Extraction
For plant material sampling, the clip cages were carefully opened, and aphids were removed using a paintbrush. Control plants without aphids were brushed in the same way as aphid-infested plants to control for possible induction of metabolic changes due to contact with the paintbrush. Leaves enclosed in the clip cages were harvested and rapidly frozen in liquid nitrogen. Frozen samples were stored overnight in Eppendorf tubes (2 ml) at −80°C and then freeze-dried for 48 h. Dried plant material was homogenized into a fine powder by adding three stainless steel beads (3 mm Ø) in each tube and vigorous shaking for 4 min on a paint shaker (Skandex shaker SO-10m, Fast & Fluid Management, Sassenheim, The Netherlands). Portions of 10 mg dried plant material were extracted with 1 ml ice-cold extraction solution containing 80% methanol acidified with 0.1% formic acid and 0.1 μg/ml of L-(+)-α-phenylglycine (as a lock mass internal standard). Samples were immediately vortexed for 10 s and continuously sonicated in a water bath at room temperature (20°C) for 15 min at a maximum frequency of 35 kHz. After centrifugation (10 min at 4,500 g and −10°C), supernatants were filtered using 0.45 μm PTFE AcroPrepTM 96-well filtration plates (Pall Corporation, Port Washington, NY, United States) and a vacuum filtration unit. All filtered plant extracts were stored at −80°C until LC-Orbitrap-MS analysis.
Plant Extract Analysis
From each plant extract 10 μl were analyzed using a UHPLC system of the Ultimate 3000 series RSLC (Dionex, Sunnyvale, CA, United States) connected to an LTQ-Orbitrap XL mass spectrometer (Thermo Fisher Scientific, Bremen, Germany). UHPLC was performed on an AcclaimTM C18 column (150 × 2.1 mm, 2.2 μm, Dionex) pre-fitted with a C-18, 3.5 μm guard column (2.1 × 10 mm, Waters, Dublin, Ireland). Separation was accomplished using a gradient of 0.1% (v/v) formic acid in water (solvent A) and 0.1% formic acid in acetonitrile (solvent B) as follows: 0–5 min isocratic 100% (v/v) A, 5–32 min gradient phase to 100% B, 32–42 min isocratic 100% B, 42–42.1 min gradient phase to 100% (v/v) A, 42.1–47 min isocratic 100% A. The flow rate was set to 300 μl min−1. The electrospray ionization (ESI) source parameters were set to 4.5 kV spray voltage, and 35 V capillary transfer voltage at a capillary temperature of 275°C. The samples were measured in the negative (NI) and positive (PI) ionization modes in separate runs using 30,000 m/Δm resolving power (mass range of m/z 150–2000) in the Orbitrap mass analyzer. XcaliburTM software (Thermo Fisher ScientificTM, Waltham, MA, United States) was used for data acquisition and visualization.
UHPLC-MS2 analysis of selected compounds was carried out by injecting l μl of each extract into a UHPLC system (Dionex UltiMate 3000, Thermo Fisher Scientific, Dreieich, Germany) coupled to a Q-Exactive Plus mass spectrometer (Thermo Fisher Scientific). Separation was performed on a AccucoreTM C18 column (2.1 × 100 mm, 2.6 μm, Thermo Fisher Scientific), using a gradient of 0.1% (v/v) formic acid in water (solvent A) and 0.1% formic acid in acetonitrile (solvent B) as follows: 0–0.2 min isocratic 100% (v/v) A, 0.2–8 min gradient phase to 100% B, 8–11 min isocratic 100% B, 11.1–12 min isocratic 100% (v/v) A. A constant flow rate of 400 μl min−1 was set. For the parallel reaction monitoring, selective ion scanning in the negative ionization mode was used with the following parameters: target ions [M-H]− (m/z 1085.55 and m/z 605.19); resolution: 17,500; AGC target: 2 × 105; maximum IT: 100 ms; sheath gas flow rate: 60; aux gas flow rate: 20; sweep gas flow rate: 5; spray voltage: 3.3 kV; capillary temperature: 360°C; S-lens RF level: 50; aux gas heater temperature: 400°C; acquisition time frame: 3.75–5.88 min.
Data Preprocessing
Raw data files were converted to mzXML format files using the MSconvert tool (ProteoWizard 3.0x software) and uploaded to the interactive XCMS online platform (Tautenhahn et al., 2012). Parameter settings for XCMS data processing were as follows: A multigroup analysis was run in the centWave mode for feature detection (Δ m/z = 2.5 ppm, minimum peak width = 10 s, and maximum peak width = 60 s); correction of the retention time was performed with an obiwarp method (profStep = 1); and for chromatogram alignment: minfrac = 0.5, bw = 5, mzwid = 0.015, max = 100, minsamp = 1. Tables with the intensities of the detected features were obtained as output. Features that were missing in 3 or more out of the 10 samples for each treatment combination were classified as sporadic features and were discarded from the data set. This resulted in 5735 features in the negative ionization mode and 9057 features in the positive ionization mode.
Data Analysis
For the initial colonization of plants by aphids, constitutive compounds that are uniquely characteristic of a plant species might play a crucial role. A feature was classified as unique to a certain plant species when it fulfilled the following criteria: It appeared in at least eight of ten samples of the plant species of interest, and was absent in the other plant species or appeared in not more than two out of ten samples per plant species. In the same way features were selected that appeared in two or more plant species. Thus 3132 features (NI) and 5323 features (PI) were analyzed. Unique features (1081 NI; 1013 PI) were filtered again to remove adducts or isotopes which resulted in 280 (NI) and 289 (PI) possible metabolites.
To determine how the metabolic profiles differed between uninfested control plants, principal component analyses (PCAs) were performed with the web-based tool MetaboAnalyst (Xia et al., 2009; Xia and Wishart, 2011). Due to technical reasons (limited number of features which can be processed in the program) 40% of the features that were near constant throughout the plant species based on the interquartile range were filtered out prior to analyses. Thus 3441 features out of 5735 features (NI) and 5434 features out of 9057 features (PI) were analyzed in the PCA. Features were then mean-centered and divided by the standard deviation of each feature (equivalent to auto scaling) to make them comparable. In order to support the results of the PCA and to check which compound classes contribute most to the separation of the different host plants, partial least squares discriminant analyses (PLS-DA) with unique features only were performed (Supplementary Figure 3).
To determine how metabolic profiles changed within a given host plant species after pea aphid infestation, several PCAs were performed. Since it was assumed that most features would not differ among plants of the same species infested with different aphid host races and uninfested control plants, PCA analyses were carried out on the 5% of metabolomic features that changed most in each species. To identify the 5% most differently regulated host race features, all features were compared by a non-parametric one-way ANOVA on ranks and sorted by their false discovery rate (FDR). Before PCA these 5% most differently regulated features were normalized by log-transformation and scaled by mean centering and division by the standard deviation of each feature (auto-scaling) to make them comparable.
To detect chemical compounds that might be involved in plant defense, we looked for features that were down regulated in plants when a native aphid race was feeding but up regulated when non-native aphid races were applied using the pattern hunter tool in the MetabolAnalyst tool. A Spearman rank correlation analysis was performed against given patterns. A pattern was specified as a series of numbers, where each number corresponded to the concentration levels of the features in the corresponding group. For instance, the pattern “2-1-2-2” corresponding to the groups “uninfested control plants – plants infested with the native aphid race – plants infested with non-native aphid race A – plants infested with non-adapted aphid race B” searched for features down regulated (positive correlation) or up regulated (negative correlation) exclusively by the native aphid race. To test whether intensities of selected features differed among the four treatments, one-way analyses of variance (one-way ANOVA) were performed. In case of significant differences, Tukey HSD tests were executed to reveal which groups were different from each other. These univariate analyses were conducted using R software version 3.2.0 (R Development Core Team, 2015).
Selected features were assigned to chemical groups through putative identifications by performing library mass searches allowing a mass deviation in all databases of 5 ppm, and checked for spectrum matches in METLIN, Human Metabolome Database (HMDB), MetFrag, MassBank, KEGG, and LipidMaps. Features not found in these libraries or databases were considered as “unknown.”
Results
Pea Aphid Host Plant Species Differ in Their Constitutive Metabolic Profiles
To evaluate how the metabolic profiles of the four uninfested plant species, M. sativa, T. pratense, P. sativum and V. faba, differed, PCA were conducted. PCA plots showed a clear separation of all four plant species in both ionization modes (Figure 1). Biological replicates of each plant species always grouped together with small confidence intervals. The first principal components (PC1) explained 26.5 and 24.8% of the total variability for negative ionization mode (NI) and positive ionization mode (PI) datasets, respectively, whereas the second principal components (PC2) accounted for 20.8 and 20.9% (for NI and PI modes, respectively) of the total variability of the data set. For both ionization modes, the metabolic profiles of M. sativa, T. pratense, and V. faba were separated mainly along PC1. P. sativum metabolic profiles were separated from those of the other plant species along PC2.
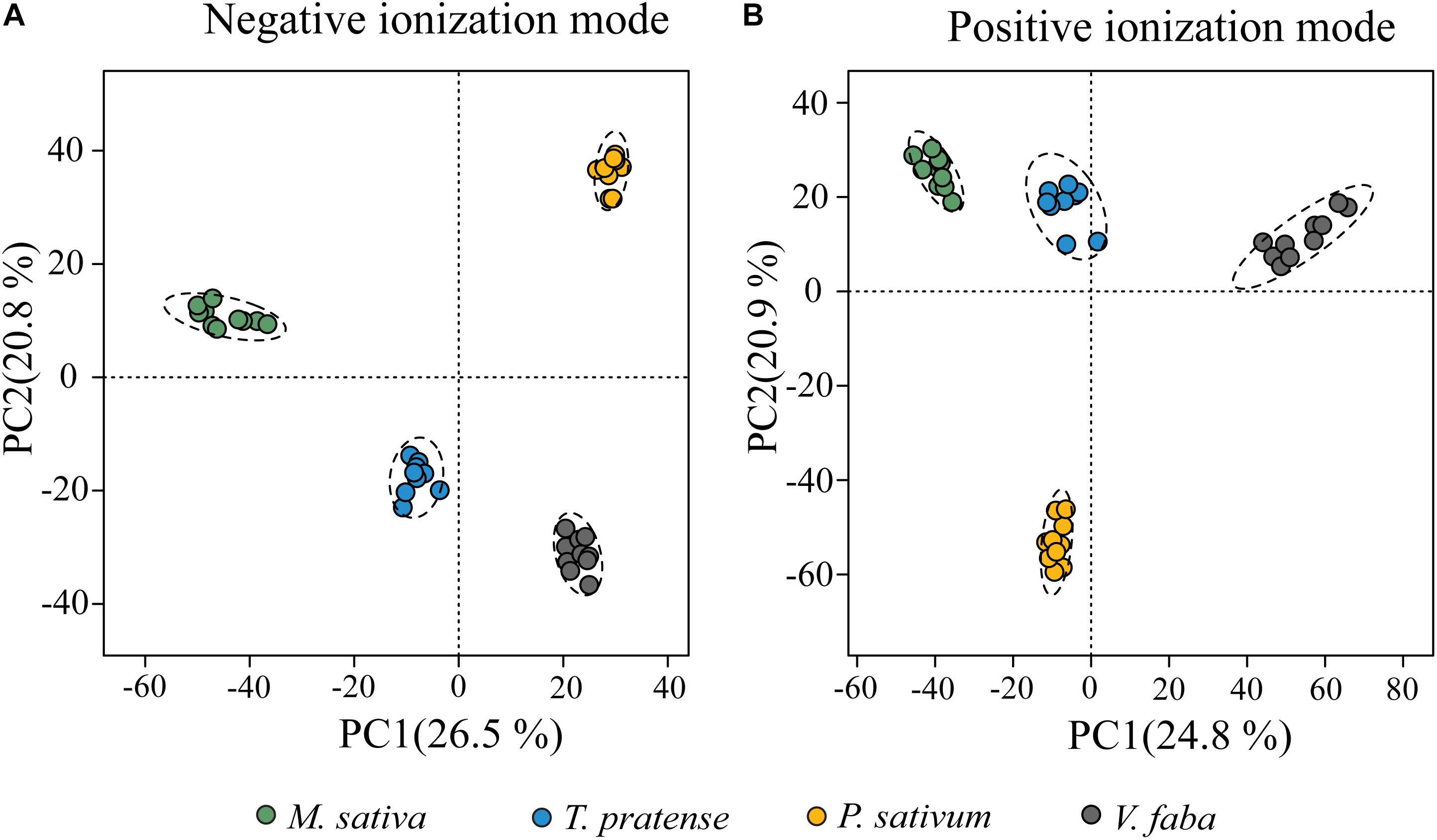
Figure 1. PCA plots of metabolic features of leaves of pea aphid host plant species harvested prior to infestation by aphids. Features derived from UHPLC-Orbitrap mass spectrometry of aqueous methanol were analyzed in (A) negative and (B) positive ionization modes. Colored circles represent the metabolic profiles of individual plants. Dotted ellipses represent the 95% confidence regions for each group.
To visualize the characteristic features shared among plant species, Venn diagrams were used (Figure 2). In both ionization modes, most features were shared between all four plants species (1676 out of 3132 features or 53.5% and 3865 out of 5323 features or 72.6% of all features for NI and PI, respectively), while features unique to a certain plant species were much less common. Only 34.5% (1081 features; NI) and 19% (1013 features; PI) of all features were assigned to only one plant species. M. sativa and T. pratense plants possessed a higher number of unique features in comparison to P. sativum and V. faba plants, while V. faba displayed the lowest number of unique features. Additionally, M. sativa and T. pratense plants shared more common features, with 149 and 109 features for NI and PI modes, respectively, than any other pair of plant species (Figure 2).
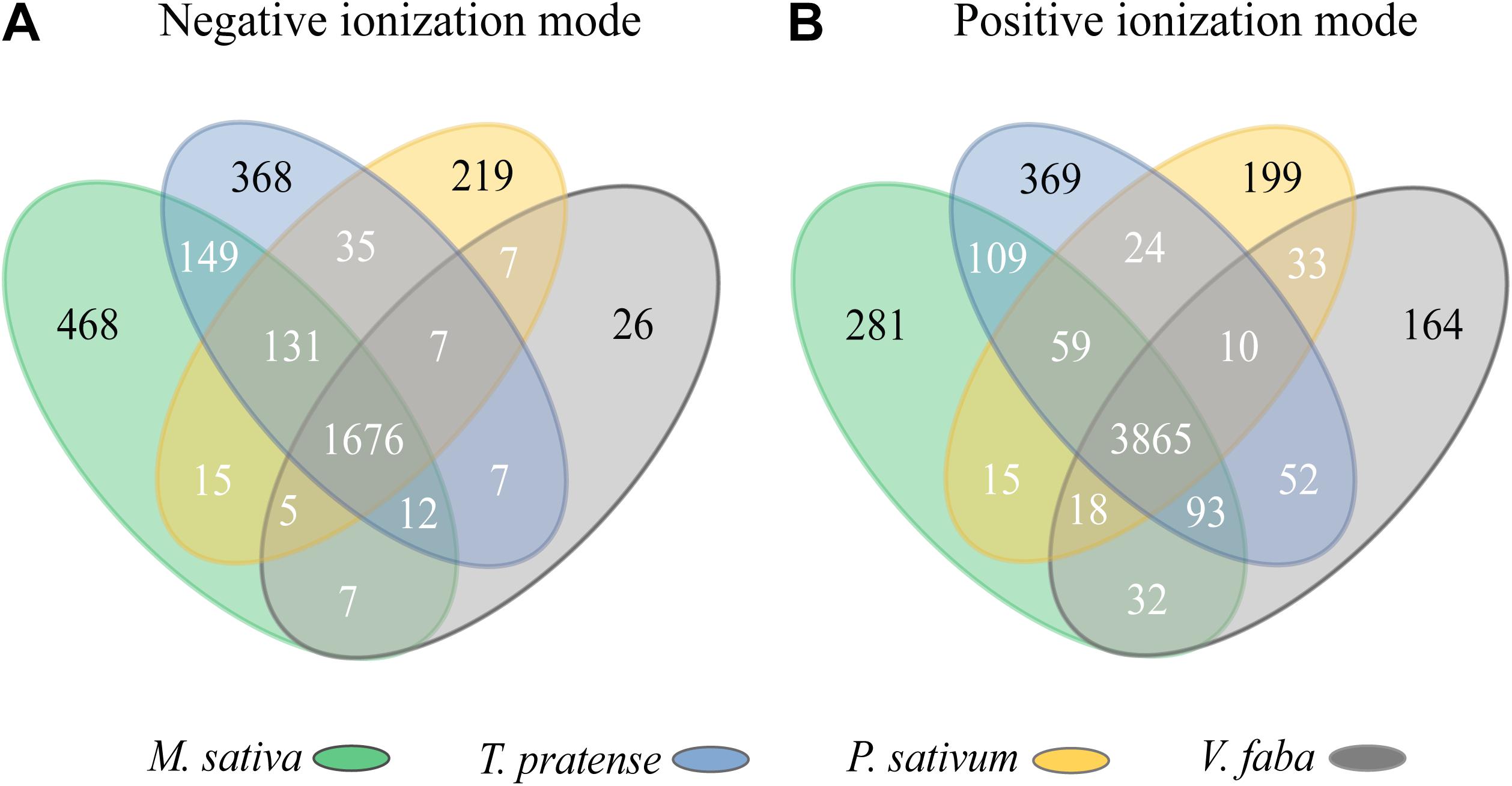
Figure 2. Venn diagrams depicting the metabolic features of uninfested pea aphid host plant species in (A) negative and (B) positive ionization modes. Highlighted are the features unique to each species (black numbers) and shared with others (white numbers). Colored areas enclose the values for each plant species.
Metabolites unique to a pea aphid host plant could serve as host identification cues responsible for the acceptance or rejection of a potential host by the different host races, and might even function as defenses against non-native races. To obtain an overview of the unique compounds of each plant species, we assigned putative identifications to the unique features and organized them by chemical classes (Table 1 and Supplementary Table 1). M. sativa displayed the highest number of unique metabolites with 107 and 86 in NI and PI mode, respectively, followed by T. pratense with 103 and 83 metabolites, P. sativum with 57 (NI) and 53 (PI) metabolites, and V. faba with 13 (NI) and 67 (PI) metabolites. From all these compounds 55% (156 out of 280 compounds; NI) and 40% (115 out of 289 compounds; PI) were putatively identified. The most common class of putatively identified unique compounds among all plant species was the flavonoids. Steroidal and triterpene saponins were not only specific but also the most abundant classes in M. sativa (Table 1, Supplementary Table 1 and Supplementary Figure 3).
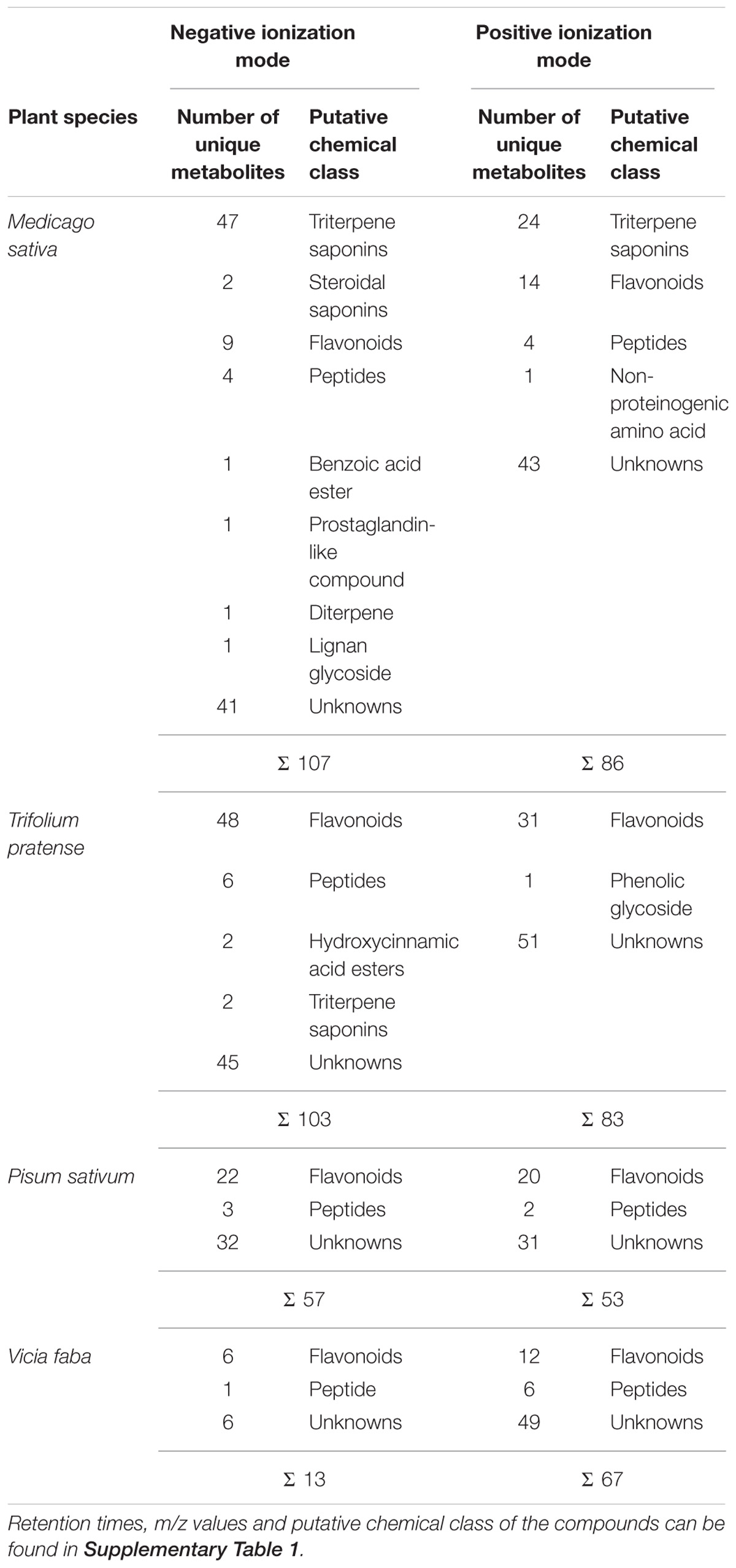
Table 1. Numbers of unique metabolites in uninfested M. sativa, T. pratense, P. sativum and V. faba plants and their putative chemical classification.
Metabolic Profiles of Host Plant Species Are Modified Differently by the Various Pea Aphid Host Races
To find out how plant metabolic profiles were modified after infestation by the various pea aphid host races, PCAs were performed based on the 5% of metabolomic features that changed most among plants infested with different pea aphid host races and uninfested control plants. In general, the metabolic profiles of uninfested control plants were separated from the profiles of aphid-infested plants, and thus plant metabolomes changed significantly upon aphid infestation. The degree of separation among profiles of plants infested with different host races depended on the plant species. Whereas the metabolic profiles of V. faba and M. sativa changed substantially depending on the attacking host race, profiles of T. pratense and P. sativum changed to a smaller extent (Figure 3).
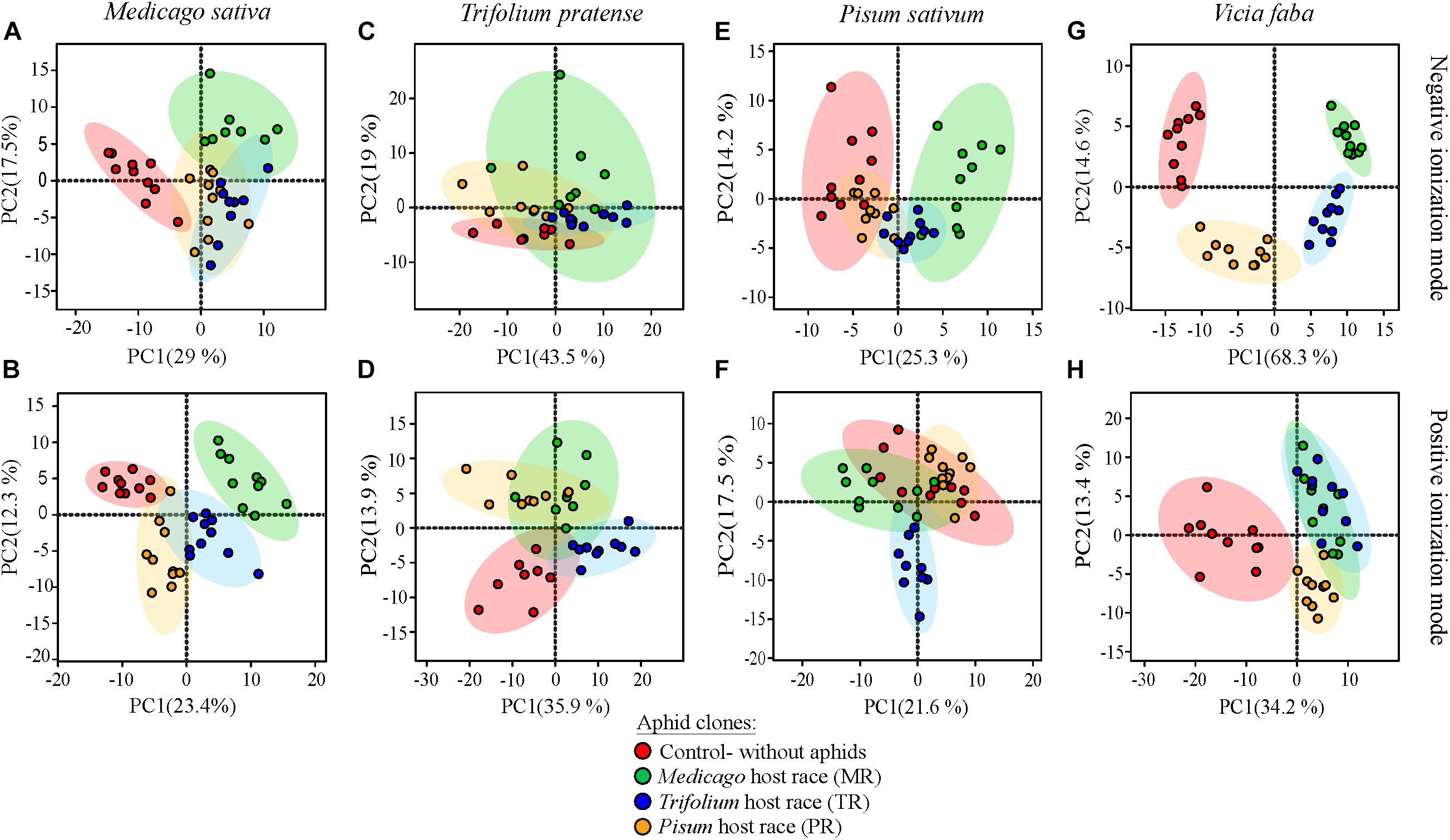
Figure 3. PCA plots of metabolic features of leaves of pea aphid host plant species before and after infestation by three different aphid host races. Features are derived from UHPLC-Orbitrap mass spectrometry of aqueous methanol extracts conducted in negative (top row: A,C,E,G) and positive (bottom row: B,D,F,H) ionization modes for M. sativa (A,B), T. pratense (C,D), P. sativum (E,F), and V. faba (G,H). Only the 5% of metabolomic features that changed most are plotted. Small colored circles represent individual plant metabolic profiles after infestation with the MR (green), TR (blue), or PR (yellow) host race or of uninfested control plants (red). Colored ellipses represent the 95% confidence regions for each group.
The metabolic profiles of aphid-infested M. sativa plants were separated from the profiles of uninfested control plants along the first principal component (Figures 3A,B). The first PC explained 29% (NI) and 23.4% (PI) of the total variability. Among the infested plants, the metabolic profiles of those infested with the native MR host race were separated from those infested with the non-native TR and PR host races mainly along the second principal component. This explained 17.5% (NI) and 12.3% (PI) of the total variability.
The metabolic profiles of aphid-infested T. pratense plants also separated from those of uninfested control plants, but only in the positive ionization mode (Figure 3D) and not the negative ionization mode (Figure 3C). Furthermore, those plants infested with the native TR host race were grouped apart from those of the non-native MR and PR host races, especially in the positive ionization mode (Figure 3D). Of the total variability in the metabolic profiles of T. pratense, 62.5% (NI) and 49.8% (PI), was explained by the first two principal components.
The proportion of variability in the metabolic profiles of P. sativum that could be explained by the first two principal components was slightly lower than in the other plant species, 39.5% (NI) and 39.1% (PI). In contrast to the other three plant species where metabolic profiles of uninfested plants separated well from profiles of aphid-infested plants, in P. sativum the metabolic profiles of uninfested plants overlapped to some extent with those of plants infested with the native PR host race (Figures 3E,F). However, in the negative ionization mode, the metabolic profiles of plants infested with the non-native MR and TR host races were separated from those of uninfested control plants along the first principal component (Figure 3E). In the positive ionization mode, the metabolic profiles of plants infested with non-native TR race separated from the metabolic profiles of the other treatments along the second principal component (Figure 3F).
A large proportion of the variability in metabolic profiles of the universal host plant V. faba could be explained by the first two principal components (82.9% for NI and 47.6% for PI). Metabolic profiles changed drastically between the differently treated plants, especially in the negative ionization mode. There was a clear separation between infested and uninfested plants in both ionization modes along the first principal component (Figures 3G,H). The second principal component separated MR host race-infested and uninfested plants from PR and TR host race-infested plants in the negative ionization mode (Figure 3G).
Some Metabolites Are Reduced by Native Pea Aphid Host Races, but Induced by Non-native Races
Pea aphid host races perform much better on their native host plants than on other species (Ferrari et al., 2006, 2008; Peccoud et al., 2009a, 2015; Schwarzkopf et al., 2013). This difference may be a consequence of the ability of each race to suppress defense signaling processes on its native plant and reduce the levels of defenses (Sanchez-Arcos et al., 2016). When feeding on a non-native host plant, on the other hand, aphid feeding may trigger signaling that leads to the induction of defenses like toxic or deterrent compounds. To test these ideas, we searched for metabolites that showed (1) significantly reduced levels only after infestation with native aphid host races, or (2) significantly increased levels only after infestation with non-native aphid host races (Table 2 and Supplementary Table 2).
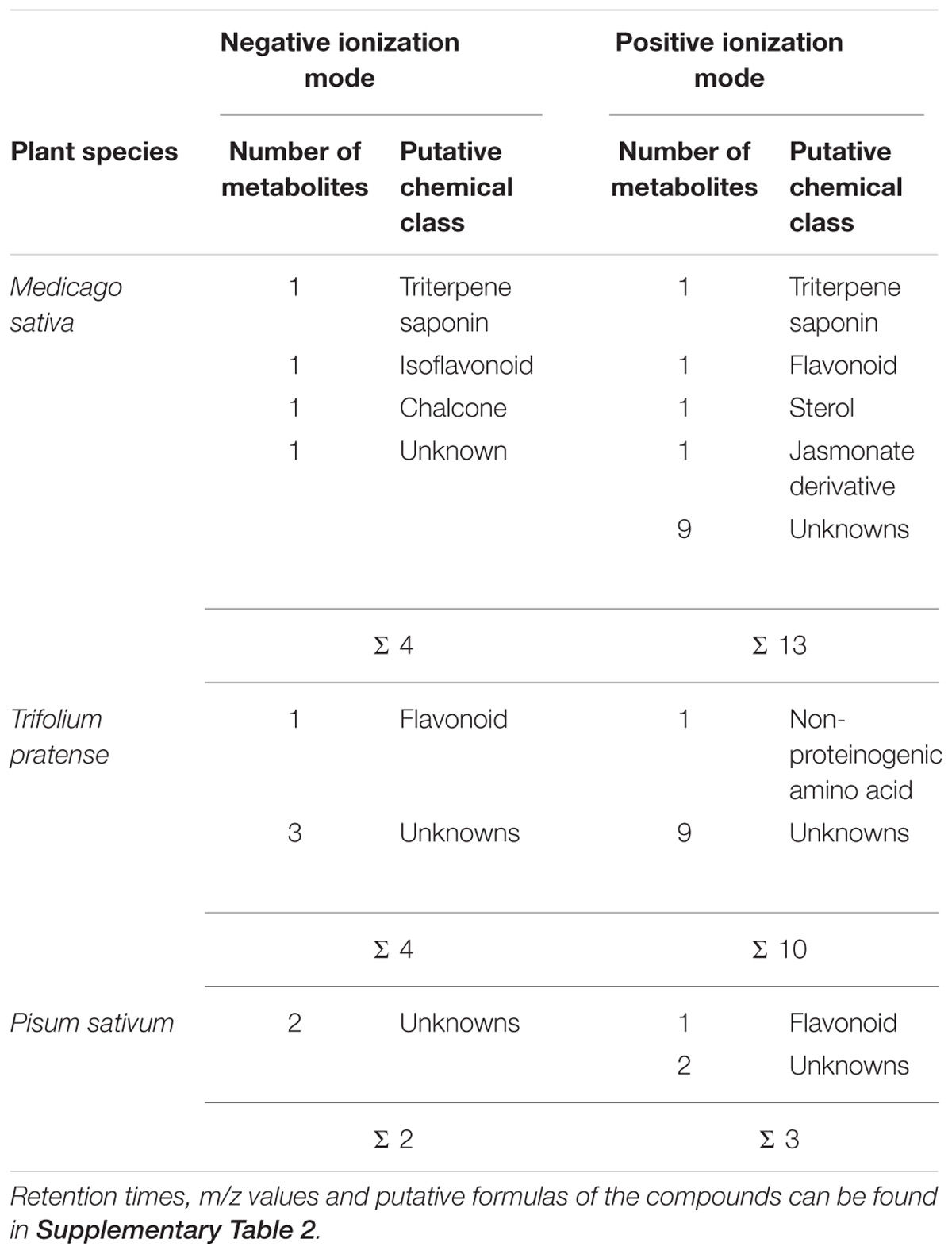
Table 2. Numbers of compounds down-regulated only after infestation with native, but not non-native aphid host races or up-regulated only after infestation with non-native, but not native host races, and their putative chemical classification.
M. sativa plants contained the highest number of metabolites that fit with these patterns, with 4 and 13 metabolites detected in the NI and PI modes, respectively, followed by T. pratense with 4 (NI) and 10 (PI) metabolites and P. sativum with 2 (NI) and 3 (PI) metabolites. A number of compounds showing these patterns could be assigned to chemical classes including several flavonoids, a triterpene saponin, a sterol and a jasmonate derivate in M. sativa, a flavonoid and a non-proteinogenic amino acid in T. pratense and a flavonoid in P. sativum. The abundance of two of the M. sativa compounds in different pea aphid treatments is illustrated in Figure 4. The levels of a putatively identified triterpene saponin (MS: with [M-H]− ion at m/z 1085.55, [M+FA (formic acid)-H]− adduct ion at m/z 1131.56, and [M-shikimic acid-H]− ion at m/z 911.46; Supplementary Figures 2A,B) were decreased by aphid infestation only with the native MR host race. On the other hand, the amount of a compound, putatively classified as a glycosylated flavonoid (MS: with [M-H]− ion at m/z 605.19, MS2: fragment ions at m/z: 577.16 and 561.20, Supplementary Figures 2C,D), was significantly increased upon infestation with the non-native TR and PR host races, but remained at similar levels as in uninfested control plants when the native MR host race infested the plant (Figure 4B).
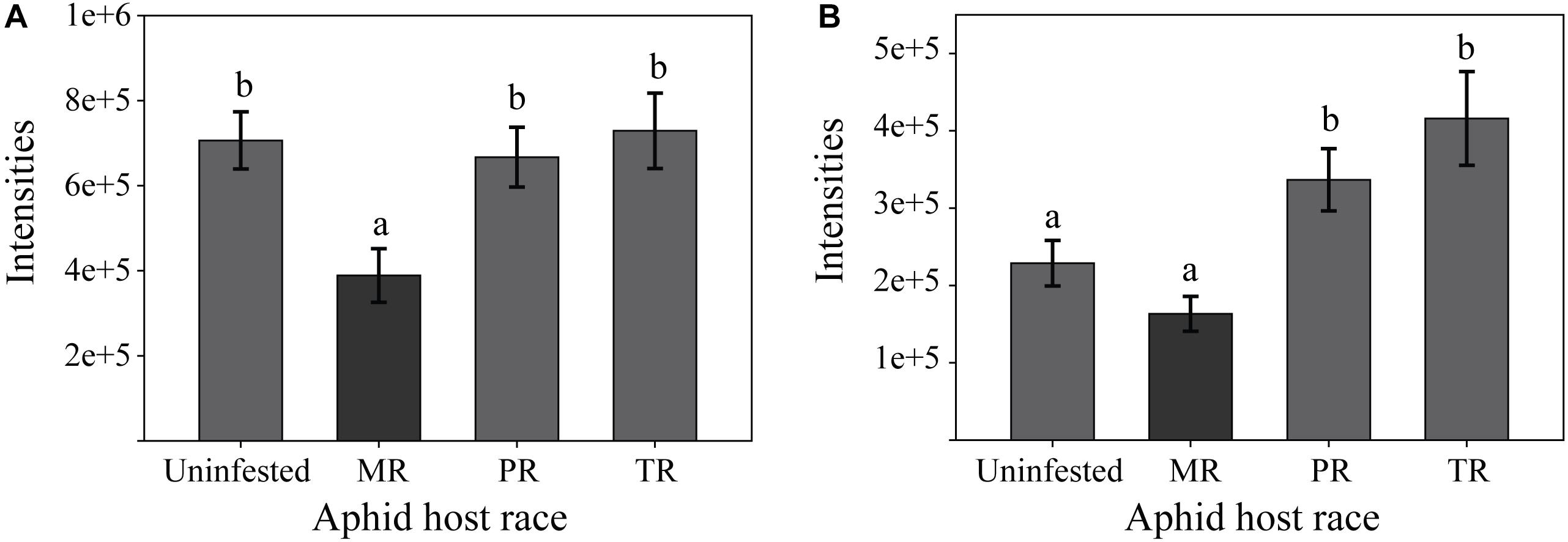
Figure 4. Examples of pea aphid host plant metabolites from M. sativa that are decreased only after infestation with the native aphid host race (A) or that are significantly increased only after infestation with non-native aphid host races (B). (A) Metabolite with [M-H] − m/z 1085.55, was decreased only by the native MR host race. (B) Metabolite with [M-H]− m/z 605.19 was induced only by the non-native PR and TR host races. Both metabolites were detected in negative ionization mode. Bars represent means ± SE. MR, Medicago race; TR, Trifolium race; PR, Pisum race; Uninfested, uninfested control plants. Different letters indicate significant differences (P ≤ 0.05) based on One-way ANOVA followed by Tukey HSD test. MS and MS2 spectra of these two compounds can be seen in Supplementary Figure 2.
Discussion
Chemical Differences Among Pea Aphid Host Plants Prior to Infestation Are Correlated With Subsequent Aphid Performance
Untargeted mass spectrometry-based analysis of the metabolites of four legume host plants of the pea aphid showed significant differences among these plants prior to aphid feeding (Figure 1). These differences might help explain the differential performance of the various pea aphid host races on specific host plants since individual metabolites could stimulate or deter feeding in disparate ways. Interestingly, the chemical complexity of the four plant species (the number of unique features found in our mass spectrometry analysis) is correlated with the performance of pea aphid host races on the various plants. Medicago sativa, which contained the highest number of unique chemical features (Figure 2), was previously found to be completely unsuitable for feeding by all other host races except the Medicago host race (Peccoud et al., 2009a; Schwarzkopf et al., 2013; Sanchez-Arcos et al., 2016). Trifolium pratense, which contained the second highest number of unique chemical features, was found to be unsuitable for other host races (Peccoud et al., 2009a), but did support some growth and reproduction of the Medicago host race (Peccoud et al., 2009a; Sanchez-Arcos et al., 2016).
The exceptional behavior of the Medicago race on T. pratense might be ascribed to the many chemical features shared between M. sativa and T. pratense (Figure 2), which also parallels their close phylogenetic relationship as members of the Tribe Trifolieae of the Fabaceae (Wojciechowski et al., 2004; The Legume Phylogeny Working Group et al., 2013). On the other hand, V. faba and P. sativum, both belonging to the Tribe Vicieae (Wojciechowski et al., 2004), had a much lower number of unique features, and can therefore be considered less chemically complex than M. sativa and T. pratense. This might be the reason for the good performance of all pea aphid host races on V. faba and their intermediate performance on P. sativum (Peccoud et al., 2009a; Schwarzkopf et al., 2013; Sanchez-Arcos et al., 2016).
The negative correlations between host plant chemical complexity and pea aphid performance suggest that the unique metabolites of each host might play a role in limiting aphid feeding. The most abundant class of unique chemical compounds found in M. sativa, and also responsible for the separation of M. sativa from other plant species were putatively identified as steroidal and triterpene saponins (Supplementary Figure 3). Saponins originate from the mevalonate pathway (Supplementary Figure 4), and although saponins are distributed in many plant families, they are frequently found in legumes (Vincken et al., 2007), especially the genus Medicago, which is well known for containing a complex mixture of triterpene saponins with a broad spectrum of biological properties (Carelli et al., 2015). Saponins are reported to be defenses against aphids (De Geyter et al., 2012; Golawska et al., 2012b, 2014) as well as other insect herbivores (De Geyter et al., 2007; Chaieb, 2010), pathogens (Oleszek et al., 1990; Abbruscato et al., 2014), and nematodes (D’Addabbo et al., 2011). Saponins exhibit strong detergent properties, and can interact with membranes disturbing permeability and leading to cell death and necrosis (Wink, 2008).
The most frequent class of unique chemical compounds putatively identified in the other pea aphid host plants studied was the flavonoids. They are synthesized by the phenylpropanoid pathway (Supplementary Figure 4) and are widely distributed in all plants (Iwashina, 2000), but flavonoids, particularly isoflavonoids, are especially abundant in legumes (Velázques et al., 2010; Wink, 2013). Flavonoids can be deterrent or toxic for insects (Brignolas et al., 1998; Widstrom and Snook, 2001; Haribal and Feeny, 2003) including phloem feeders such as aphids (Montgomery and Arn, 1974; Lattanzio et al., 2000), and also have activity against microbes (Grayer and Harborne, 1994; Rauha et al., 2000). Interestingly, in a previous metabolomics investigation of host plant choice in pea aphids, L-phenylalanine and L-tyrosine were found to be associated with differential acceptability of species of Medicago and Trifolium by the Medicago and Trifolium host races (Hopkins et al., 2017). Since L-phenylalanine is one of the major precursors of flavonoids, its correlation with pea aphid host choice may be due to the flavonoids formed from this amino acid. Thus untargeted chemical analysis of pea aphid host plants has revealed several candidate groups of compounds that may play a role in host race specificity. Future studies on these compounds are warranted to determine how they are involved in defining pea aphid-host plant interactions.
Host Plant Compounds Induced or Suppressed by Pea Aphid Host Races May Influence Their Performance
Pea aphids may be affected not only by the constitutive chemistry of their host plants, but also by the changes induced upon aphid feeding. Thus in this study we compared the metabolic composition of host plants after feeding by the different host races with the composition of uninfested plants. Our results showed that host plant metabolomes were indeed modified by aphid feeding with modification occurring in a host race-dependent manner. The metabolic profiles of M. sativa and T. pratense plants after aphid infestation were consistently different from those of their respective uninfested control plants, and the metabolic profiles measured after feeding by the Medicago and Trifolium native host races were different from those after feeding by non-native host races (Figures 3A–D). In contrast, the metabolic profile of P. sativum after infestation by the native Pisum host race was not different from that of the control plants, but infestation with the non-native host races generated distinct changes in metabolic profiles. These patterns of metabolic change correspond well with changes in defense hormone levels found in a previous study after pea aphid feeding (Sanchez-Arcos et al., 2016). The salicylic and jasmonate levels of M. sativa and T. pratense were substantially altered by aphid infestation. Native host races caused slight induction or reduction of hormone levels, and non-native host races caused significant increases. In P. sativum, the native host race did not modify defense hormone levels compared to those found in uninfested control plants.
The metabolic changes measured after aphid feeding may result from a series of aphid behaviors that occur during the establishment of phloem feeding. En route to the phloem, aphids pierce many plant cells and secrete watery saliva into these cells (Martin et al., 1997; Powell, 2005). They also secrete gelling saliva into the apoplast to form a sheath around the stylet. Interestingly, even in incompatible interactions (such as those resulting from non-native host races) when aphids are not able to feed on the sieve elements, they insert their stylets and salivate into the plant (Schwarzkopf et al., 2013). The saliva contains proteins some of which may function as herbivore associated molecular patterns that bind to plant recognition receptors leading to the activation of plant defense responses (Hogenhout and Bos, 2011). For instance, a proteinaceous elicitor from M. persicae saliva induced resistance against this aphid in Arabidopsis, and pre-treatment of the host plant with aphid saliva decreased aphid fecundity (De Vos and Jander, 2009). Some of the metabolic changes observed after pea aphid infestation in this study might be a direct consequence of the defense responses induced by salivary proteins.
Aphids that are able to feed on a plant, such as native host races, may prevent the induction of defense responses by secreting effector proteins into the plant (Hogenhout and Bos, 2011; Elzinga and Jander, 2013; Van Bel and Will, 2016). For example, the effector molecule C002 from the pea aphid was shown to be essential for feeding on its universal host plant V. faba, and silencing of the C002 gene reduced aphid fecundity (Mutti et al., 2008). However, plants have developed mechanisms to recognize such effector molecules and consequently activate their defense. Thus the chemical changes occurring after pea aphid infestation may reflect the outcome of the plant-aphid interaction.
The specificity of host race performance on different host plants may well be due to chemical differences among plants induced by infestation. Plants commonly synthesize new chemical compounds after herbivore attack, but this phenomenon has been studied for phloem feeding insects in only a few cases (Züst and Agrawal, 2016). Thus, in this study we sought compounds that were either induced by non-native host races but not native races, or suppressed by native host races but not non-native races. Such substances might be critical in facilitating the good performance of native host races and poor performance on non-native races. Interestingly, the number of compounds that were up or down regulated in this manner was again higher in M. sativa and T. pratense than in P. sativum (Table 2).
One of the compounds with these characteristics, putatively classed as a triterpene saponin, was unique to M. sativa and down regulated only by the native MR host race (Figure 4A). Saponins in plants have been reported to play a widespread role in resistance against insect herbivores (Applebaum et al., 1969; Fields et al., 2010; Nielsen et al., 2010), including in M. sativa (Nozzolillo et al., 1997; Adel et al., 2000), and in resistance against aphids in particular (Soulé et al., 2000; Golawska et al., 2012b). The adverse effects of saponins against the pea aphid have been observed in several studies (Golawska, 2007; De Geyter et al., 2012). These results suggest that the down regulation of the M. sativa saponin by the native Medicago host race, as described above, could be responsible for the increased performance on its native host plant. At the same time, the inability of non-native host races to down regulate this saponin may contribute to their poor performance due to the potential deterrent or toxic effects of this compound.
Another compound whose levels varied in a host race-specific manner was putatively classed as a sterol. Plant sterols are essential dietary requirements for insects, which cannot produce their own de novo, but need sterols as structural components of cell membranes and precursors in the formation of ecdysteroids (molting and sex hormones) (Clark and Bloch, 1959; Behmer and Nes, 2003; Behmer, 2017). However, sterols can also have negative effects on insects (Behmer, 2017). For instance the sterol stigmasterol suppressed the lifetime reproductive output of pea aphids when added to an artificial diet (Bouvaine et al., 2012). The aphid M. persicae had a reduced reproduction and a higher mortality when feeding on tobacco plants with high levels of atypical steroids (Behmer et al., 2011). Thus the sterol identified in this study may also represent a good candidate to be tested further with different pea aphid host races to deduce its role in determining patterns of host plant specificity.
Another compound that was induced in M. sativa after infestation with non-native, but not native host races was putatively classified as a flavonoid (Figure 4B). Increased levels of flavonoids in legumes after pea aphid infestation were previously reported to cause detrimental effects on the pea aphid (Golawska and Lukasik, 2012). Host race-specific regulation of flavonoids also occurred in T. pratense. Thus flavonoids are also good candidates to explain the differential performance of pea aphid host races.
Conclusion
An untargeted metabolomics approach allowed the detection of a number of candidate compounds that could be responsible for the disparate performance of various pea aphid host races on leguminous plant species. Some of these candidates are constitutive substances that are unique to one of the host plants. Other candidates were differentially induced or suppressed following infestation by the various host races. The most abundant chemical classes represented were saponins and flavonoids. Based on these results, untargeted metabolomics should be more widely considered as a valuable technique to discover plant metabolites of potential importance in plant interactions with herbivores and pathogens. When related plant species differ in their susceptibility or resistance, metabolomic comparison before and after enemy attack may reveal the chemical compounds responsible.
Author Contributions
CS-A and GK conceived and designed the experiments. CS-A performed the experiments. MK measured the metabolic profiles. CS-A and GK analyzed the data. CS-A, GK, and JG interpreted the results and wrote the manuscript. All authors critically revised and consented to the final version of the manuscript.
Funding
This project was supported by the Max Planck Society, Germany, and the German Academic Exchange Service (DAAD) within the framework of the PPP – Procope German-French collaboration program (Project Code 57049694).
Conflict of Interest Statement
The authors declare that the research was conducted in the absence of any commercial or financial relationships that could be construed as a potential conflict of interest.
Acknowledgments
We thank Jean-Christophe Simon (INRA, Le Rheu, France) for providing the aphid clones, Kerstin Zipfel, Christoph Scheck, Lindsey Roark, and Kristina Schädel for experimental help, Andreas Weber and Elke Goschala for plant cultivation, Tim Baumeister for his help with the visualization of PLS-DA results, and Ilka Vosteen, Elizabeth Pringle, and Maria Paulmann for helpful comments on previous versions of the manuscript.
Supplementary Material
The Supplementary Material for this article can be found online at: https://www.frontiersin.org/articles/10.3389/fpls.2019.00188/full#supplementary-material
References
Abbruscato, P., Tosi, S., Crispino, L., Biazzi, E., Menin, B., Picco, A. M., et al. (2014). Triterpenoid glycosides from ‘as antifungal agents against Pyricularia oryzae. J. Agric. Food Chem. 62, 11030–11036. doi: 10.1021/jf5049063
Adel, M. M., Sehnal, F., and Jurzysta, M. (2000). Effects of alfalfa saponins on the moth Spodoptera littoralis. J. Chem. Ecol. 26, 1065–1078. doi: 10.1023/A:1005445217004
Applebaum, S. W., Marco, S., and Birk, Y. (1969). Saponins as possible factors of resistance of legume seeds to attack of insects. J. Agric. Food Chem. 17:618. doi: 10.1021/jf60163a020
Arimura, G., Kost, C., and Boland, W. (2005). Herbivore-induced, indirect plant defences. Biochim. Biophys. Acta 1734, 91–111. doi: 10.1016/j.bbalip.2005.03.001
Atamian, H. S., Chaudhary, R., Dal Cin, V., Bao, E., Girke, T., and Kaloshian, I. (2013). In planta expression or delivery of potato aphid Macrosiphum euphorbiae effectors Me10 and Me23 enhances aphid fecundity. Mol. Plant Microbe Interact. 26, 67–74. doi: 10.1094/MPMI-06-12-0144-FI
Avé, D. A., Gregory, P., and Tingey, W. M. (1987). Aphid repellent sesquiterpenes in glandular trichomes of Solanum berthaultii and Solanum tuberosum. Entomol. Exp. Appl. 44, 131–138. doi: 10.1111/j.1570-7458.1987.tb01057.x
Behmer, S. T. (2017). Overturning dogma: tolerance of insects to mixed-sterol diets is not universal. Curr. Opin. Insect Sci. 23, 89–95. doi: 10.1016/j.cois.2017.08.001
Behmer, S. T., Grebenok, R. J., and Douglas, A. E. (2011). Plant sterols and host plant suitability for a phloem-feeding insect. Funct. Ecol. 25, 484–491. doi: 10.1111/j.1365-2435.2010.01810.x
Behmer, S. T., and Nes, W. D. (2003). Insect sterol nutrition and physiology: a global overview. Adv. Insect Physiol. 31, 1–72. doi: 10.1016/S0065-2806(03)31001-X
Berenbaum, M. R., and Zangerl, A. R. (1998). “Population-level adaptation to host-plant chemicals: the role of cytochrome P450 monooxygenases,” in Genetic Structure and Local Adaptation in Natural Insect Populations, eds S. Mopper and S. Y. Strauss (Boston, MA: Springer-Science+Business Media, B.V), 91–112. doi: 10.1007/978-1-4757-0902-5_5
Bernays, E., and Graham, M. (1988). On the evolution of host speificity in phytophagous arthropods. Ecology 69, 886–892. doi: 10.2307/1941237
Bos, J. I. B., Prince, D., Pitino, M., Maffei, M. E., Win, J., and Hogenhout, S. A. (2010). A functional genomics approach identifies candidate effectors from the aphid species Myzus persicae (green peach aphid). PLoS Genetics 6:e1001216. doi: 10.1371/journal.pgen.1001216
Boulain, H., Legeai, F., Guy, E., Morliere, S., Douglas, N. E., Oh, J., et al. (2018). Fast evolution and lineage-specific gene family expansions of aphid salivary effectors driven by interactions with host-plants. Genome Biol. Evol. 10, 1554–1572. doi: 10.1093/gbe/evy097
Bouvaine, S., Behmer, S. T., Lin, G. G., Faure, M.-L., Grebenok, R. J., and Douglas, A. E. (2012). The physiology of sterol nutrition in the pea aphid Acyrthosiphon pisum. J. Insect Physiol. 58, 1383–1389. doi: 10.1016/j.jinsphys.2012.07.014
Brignolas, F., Lieutier, F., Sauvard, D., Christiansen, E., and Berryman, A. A. (1998). Phenolic predictors for Norway spruce resistance to the bark beetle Ips typographus (Coleoptera: Scolytidae) and an associated fungus, Ceratocystis polonica. Can. J. Forest Res. 28, 720–728. doi: 10.1139/x98-037
Brisson, J. A., and Stern, D. L. (2006). The pea aphid, Acyrthosiphon pisum: an emerging genomic model system for ecological, developmental and evolutionary studies. Bioessays 28, 747–755. doi: 10.1002/bies.20436
Caillaud, M. C., and Via, S. (2000). Specialized feeding behavior influences both ecological specialization and assortative mating in sympatric host races of pea aphids. Am. Nat. 156, 606–621. doi: 10.1086/316991
Carelli, M., Biazzi, E., Tava, A., Losini, I., Abbruscato, P., Depedro, C., et al. (2015). Sapogenin content variation in Medicago inter-specific hybrid derivatives highlights some aspects of saponin synthesis and control. New Phytol. 206, 303–314. doi: 10.1111/nph.13162
Chaudhary, R., Atamian, H. S., Shen, Z., Briggs, S. P., and Kaloshian, I. (2014). GroEL from the endosymbiont Buchnera aphidicola betrays the aphid by triggering plant defense. Proc. Natl. Acad. Sci. 111, 8919–8924. doi: 10.1073/pnas.1407687111
Clark, A. J., and Bloch, K. (1959). Conversion of ergosterol to 22-dehydrocholesterol in Blattella germancia. J. Biol. Chem. 234, 2589–2594.
D’Addabbo, T., Carbonara, T., Leonetti, P., Radicci, V., Tava, A., and Avato, P. (2011). Control of plant parasitic nematodes with active saponins and biomass from Medicago sativa. Phytochem. Rev. 10, 503–519. doi: 10.1007/s11101-010-9180-2
De Geyter, E., Lambert, E., Geelen, D., and Smagghe, G. (2007). Novel advances with plant saponins as natural insecticides to control pest insects. Pest Technol. 1, 96–105.
De Geyter, E., Smagghe, G., Rahbe, Y., and Geelen, D. (2012). Triterpene saponins of Quillaja saponaria show strong aphicidal and deterrent activity against the pea aphid Acyrthosiphon pisum. Pest Manag. Sci. 68, 164–169. doi: 10.1002/ps.2235
De Vos, M., and Jander, G. (2009). Myzus persicae (green peach aphid) salivary components induce defence responses in Arabidopsis thaliana. Plant Cell Environ. 32, 1548–1560. doi: 10.1111/j.1365-3040.2009.02019.x
Drès, M., and Mallet, J. (2002). Host races in plant-feeding insects and their importance in sympatric speciation. Philos. Trans. R. Soc. London Ser. B Biol. Sci. 357, 471–492. doi: 10.1098/rstb.2002.1059
Dreyer, D. L., Jones, K. C., and Molyneux, R. J. (1985). Feeding deterrency of some pyrrolizidine, indolizidine, and quinolizidine alkaloids towards pea aphid (Acyrthosipon pisum) and evidence for phloem transport of indolizidine alkaloid swainsonine. J. Chem. Ecol. 11, 1045–1051. doi: 10.1007/BF01020674
Ehrlich, P. R., and Raven, P. H. (1964). Butterflies and plants: a study in coevoltion. J. Ecol. 18, 586–608.
Elzinga, D. A., De Vos, M., and Jander, G. (2014). Suppression of plant defenses by a Myzus persicae (Green peach aphid) salivary effector protein. Mol. Plant Microbe Interact. 27, 747–756. doi: 10.1094/MPMI-01-14-0018-R
Elzinga, D. A., and Jander, G. (2013). The role of protein effectors in plant-aphid interactions. Curr. Opin. Plant Biol. 16, 451–456. doi: 10.1016/j.pbi.2013.06.018
Ferrari, J., Godfray, H. C. J., Faulconbridge, A. S., Prior, K., and Via, S. (2006). Population differentiation and genetic variation in host choice among pea aphids from eight host plant genera. Evolution 60, 1574–1584. doi: 10.1111/j.0014-3820.2006.tb00502.x
Ferrari, J., Via, S., and Godfray, H. C. J. (2008). Population differentiation and genetic variation in performance on eight hosts in the pea aphid complex. Evolution 62, 2508–2524. doi: 10.1111/j.1558-5646.2008.00468.x
Fiehn, O. (2002). Metabolomics - the link between genotypes and phenotypes. Plant Mol. Biol. 48, 155–171. doi: 10.1023/A:1013713905833
Fields, P. G., Woods, S., and Taylor, W. G. (2010). Triterpenoid saponins synergize insecticidal pea peptides: effect on feeding and survival of Sitophilus oryzae (Coleoptera: Curculionidae). Can. Entomol. 142, 501–512. doi: 10.4039/n10-024
Gabrys, B., and Tjallingii, W. F. (2002). The role of sinigrin in host plant recognition by aphids during initial plant penetration. Entomol. Exp. Appl. 104, 89–93. doi: 10.1046/j.1570-7458.2002.00994.x
Golawska, S. (2007). Deterrence and toxicity of plant saponins for the pea aphid Acyrthosiphon pisum Harris. J. Chem. Ecol. 33, 1598–1606. doi: 10.1007/s10886-007-9333-y
Golawska, S., Bogumil, L., and Wieslaw, O. (2006). Effect of low and high-saponin lines of alfalfa on pea aphid. J. Insect Physiol. 52, 737–743. doi: 10.1016/j.jinsphys.2006.04.001
Golawska, S., and Lukasik, I. (2009). Acceptance of low-saponin lines of alfalfa with varied phenolic concentrations by pea aphid (Homoptera: Aphididae). Biologia 64, 377–382. doi: 10.2478/s11756-009-0051-5
Golawska, S., and Lukasik, I. (2012). Antifeedant activity of luteolin and genistein against the pea aphid, Acyrthosiphon pisum. J. Pest Sci. 85, 443–450. doi: 10.1007/s10340-012-0452-z
Golawska, S., Lukasik, I., Kapusta, I., and Janda, B. (2012a). Do the contents of luteolin, tricin, and chrysoeriol glycosides in alfalfa (Medicago sativa L.) affect the behavior of pea aphid (Acyrthosiphon pisum)? Pol. J. Environ. Stud. 21, 1613–1619.
Golawska, S., Lukasik, I., Wojcicka, A., and Sytykiewicz, H. (2012b). Relationship between saponin content in alfalfa and aphid development. Acta Biol. Cracov. Ser. Bot. 54, 39–46. doi: 10.2478/v10182-012-0022-y
Golawska, S., Sprawka, I., and Lukasik, I. (2014). Effect of saponins and apigenin mixtures on feeding behavior of the pea aphid, Acyrthosiphon pisum Harris. Biochem. Syst. Ecol. 55, 137–144. doi: 10.1016/j.bse.2014.03.010
Grayer, R. J., and Harborne, J. B. (1994). A survey of antifungal compounds from higher plants, 1982-1993. Phytochemistry 37, 19–42. doi: 10.1016/0031-9422(94)85005-4
Guy, E., Boulain, H., Aigu, Y., Le Pennec, C., Chawki, K., Morliere, S., et al. (2016). Optimization of agroinfiltration in Pisum sativum provides a new tool for studying the salivary protein functions in the pea aphid complex. Front. Plant Sci. 7:1171. doi: 10.3389/fpls.2016.01171
Haribal, M., and Feeny, P. (2003). Combined roles of contact stimulant and deterrents in assessment of host-plant quality by ovipositing zebra swallowtail butterflies. J. Chem. Ecol. 29, 653–670. doi: 10.1023/A:1022820719946
Hegnauer, R. (2001). Chemotaxonomie der Pflanzen. Basel: Birkhäuser Verlag. doi: 10.1007/978-3-0348-7986-6
Hogenhout, S. A., and Bos, J. I. B. (2011). Effector proteins that modulate plant-insect interactions. Curr. Opin. Plant Biol. 14, 422–428. doi: 10.1016/j.pbi.2011.05.003
Hopkins, D. P., Cameron, D. D., and Butlin, R. K. (2017). The chemical signatures underlying host plant discrimination by aphids. Sci. Rep. 7:8498. doi: 10.1038/s41598-017-07729-0
Iwashina, T. (2000). The structure and distribution of the flavonoids in plants. J. Plant Res. 113, 287–299. doi: 10.1007/PL00013940
Jansen, J. J., Allwood, J. W., Marsden-Edwards, E., Van Der Putten, W. H., Goodacre, R., and Van Dam, N. M. (2009). Metabolomic analysis of the interaction between plants and herbivores. Metabolomics 5, 150–161. doi: 10.1007/s11306-008-0124-4
Jaquiery, J., Stoeckel, S., Nouhaud, P., Mieuzet, L., Maheo, F., Legeai, F., et al. (2012). Genome scans reveal candidate regions involved in the adaptation to host plant in the pea aphid complex. Mol. Ecol. 21, 5251–5264. doi: 10.1111/mec.12048
Kaloshian, I., and Walling, L. L. (2016). Hemipteran and dipteran pests: effectors and plant host immune regulators. J. Integr. Plant Biol. 58, 350–361. doi: 10.1111/jipb.12438
Kim, J. H., Lee, B. W., Schroeder, F. C., and Jander, G. (2008). Identification of indole glucosinolate breakdown products with antifeedant effects on Myzus persicae (green peach aphid). Plant J. 54, 1015–1026. doi: 10.1111/j.1365-313X.2008.03476.x
Kordan, B., Dancewicz, K., Wroblewska, A., and Gabrys, B. (2012). Intraspecific variation in alkaloid profile of four lupine species with implications for the pea aphid probing behaviour. Phytochem. Lett. 5, 71–77. doi: 10.1016/j.phytol.2011.10.003
Krastanov, A. (2010). Metabolomics - the state of art. Biotechnol. Biotechnol. Equip. 24, 1537–1543. doi: 10.2478/V10133-010-0001-Y
Lattanzio, V., Arpaia, S., Cardinali, A., Di Venere, D., and Linsalata, V. (2000). Role of endogenous flavonoids in resistance mechanism of Vigna to aphids. J. Agric. Food Chem. 48, 5316–5320. doi: 10.1021/jf000229y
Maag, D., Erb, M., Köllner, T. G., and Gershenzon, J. (2015). Defensive weapons and defense signals in plants: some metabolites serve both roles. Bioessays 37, 167–174. doi: 10.1002/bies.201400124
Martin, B., Collar, J. L., Tjallingii, W. F., and Fereres, A. (1997). Intracellular ingestion and salivation by aphids may cause the acquisition and inoculation of non-persistently transmitted plant viruses. J. Gen. Virol. 78, 2701–2705. doi: 10.1099/0022-1317-78-10-2701
Mithöfer, A., and Boland, W. (2012). Plant defense against herbivores: chemical aspects. Ann. Rev. Plant Biol. 63, 431–450. doi: 10.1146/annurev-arplant-042110-103854
Mitter, C., Farrell, B., and Wiegmann, B. (1988). The phylogenetic study of adaptive zones: has phytophagy promoted insect diversification? Am. Nat. 132, 107–128. doi: 10.1086/284840
Montgomery, M. E., and Arn, H. (1974). Feeding response of Aphis pomi, Myzus persicae, and Amphorophora agathonica to phlorizin. J. Insect Physiol. 20, 413–421. doi: 10.1016/0022-1910(74)90071-7
Mutti, N. S., Louis, J., Pappan, L. K., Pappan, K., Begum, K., Chen, M. S., et al. (2008). A protein from the salivary glands of the pea aphid, Acyrthosiphon pisum, is essential in feeding on a host plant. Proc. Natl. Acad. Sci. U.S.A. 105, 9965–9969. doi: 10.1073/pnas.0708958105
Naessens, E., Dubreuil, G., Giordanengo, P., Baron, O. L., Minet-Kebdani, N., Keller, H., et al. (2015). A secreted MIF cytokine enables aphid feeding and represses plant immune responses. Curr. Biol. 25, 1898–1903. doi: 10.1016/j.cub.2015.05.047
Nakabayashi, R., and Saito, K. (2013). Metabolomics for unknown plant metabolites. Anal. Bioanal. Chem. 405, 5005–5011. doi: 10.1007/s00216-013-6869-2
Nielsen, N. J., Nielsen, J., and Staerk, D. (2010). New resistance-correlated saponins from the insect-resistant crucifer Barbarea vulgaris. J. Agric. Food Chem. 58, 5509–5514. doi: 10.1021/jf903988f
Nouhaud, P., Gautier, M., Gouin, A., Jaquiery, J., Peccoud, J., Legeai, F., et al. (2018). Identifying genomic hotspots of differentiation and candidate genes involved in the adaptive divergence of pea aphid host races. Mol. Ecol. 27, 3287–3300. doi: 10.1111/mec.14799
Nozzolillo, C., Arnason, J. T., Campos, F., Donskov, N., and Jurzysta, M. (1997). Alfalfa leaf saponins and insect resistance. J. Chem. Ecol. 23, 995–1002. doi: 10.1023/B:JOEC.0000006384.60488.94
Oleszek, W., Price, K. R., Colquhoun, I. J., Jurzysta, M., Ploszynski, M., and Fenwick, G. R. (1990). Isolation and identification of alfalfa (Medicago sativa L) root saponins - their activity in relation to a fungal bioassay. J. Agric. Food Chem. 38, 1810–1817. doi: 10.1021/jf00099a006
Peccoud, J., Maheo, F., De La Huerta, M., Laurence, C., and Simon, J.-C. (2015). Genetic characterisation of new host-specialised biotypes and novel associations with bacterial symbionts in the pea aphid complex. Insect Conserv. Div. 8, 484–492. doi: 10.1111/icad.12131
Peccoud, J., Ollivier, A., Plantegenest, M., and Simon, J. C. (2009a). A continuum of genetic divergence from sympatric host races to species in the pea aphid complex. Proc. Natl. Acad. Sci. U.S.A. 106, 7495–7500. doi: 10.1073/pnas.0811117106
Peccoud, J., Simon, J. C., Mclaughlin, H. J., and Moran, N. A. (2009b). Post-Pleistocene radiation of the pea aphid complex revealed by rapidly evolving endosymbionts. Proc. Natl. Acad. Sci. U.S.A. 106, 16315–16320. doi: 10.1073/pnas.0905129106
Peccoud, J., and Simon, J. C. (2010). The pea aphid complex as a model of ecological speciation. Ecol. Entomol. 35, 119–130. doi: 10.1111/j.1365-2311.2009.01147.x
Powell, G. (2005). Intracellular salivation is the aphid activity associated with inoculation of non-persistently transmitted viruses. J. Gen. Virol. 86, 469–472. doi: 10.1099/vir.0.80632-0
R Development Core Team (2015). R: A Language and Environment for Statistical Computing. Vienna: R Foundation for Statistical Computing.
Rauha, J. P., Remes, S., Heinonen, M., Hopia, A., Kahkonen, M., Kujala, T., et al. (2000). Antimicrobial effects of Finnish plant extracts containing flavonoids and other phenolic compounds. Int. J. Food Microbiol. 56, 3–12. doi: 10.1016/S0168-1605(00)00218-X
Rodriguez, P. A., and Bos, J. I. B. (2013). Toward understanding the role of aphid effectors in plant infestation. Mol. Plant Microbe Interact. 26, 25–30. doi: 10.1094/MPMI-05-12-0119-FI
Sanchez-Arcos, C., Reichelt, M., Gershenzon, J., and Kunert, G. (2016). Modulation of legume defense signaling pathways by native and non-native pea aphid clones. Front. Plant Sci. 7:1872. doi: 10.3389/fpls.2016.01872
Schwarzkopf, A., Rosenberger, D., Niebergall, M., Gershenzon, J., and Kunert, G. (2013). To feed or not to feed: plant factors located in the epidermis, mesophyll, and sieve elements influence pea aphid’s ability to feed on legume species. PLoS One 8:e75298. doi: 10.1371/journal.pone.0075298
Soulé, S., Guntner, C., Vazquez, A., Argandona, V., Moyna, P., and Ferreira, F. (2000). An aphid repellent glycoside from Solanum laxum. Phytochemistry 55, 217–222. doi: 10.1016/S0031-9422(00)00273-9
Stork, N. E. (1993). How many species are there? Biodivers. Conserv. 2, 215–232. doi: 10.1007/BF00056669
Strong, D. R., Lawton, J. H., Southwood, T. R. E., Strong, D. R., Lawton, J. H., and Southwood, T. R. E. (1984). Insects on Plants; Community Patterns and Mechanisms. London: Blackwell Scientific Publications.
Tautenhahn, R., Patti, G. J., Rinehart, D., and Siuzdak, G. (2012). XCMS Online: a web-based platform to process untargeted metabolomic data. Anal. Chem. 84, 5035–5039. doi: 10.1021/ac300698c
The International Aphid Genomics Consortium (2010). Genome sequence of the pea aphid Acyrthosiphon pisum. PLoS Biol. 8:e1000313. doi: 10.1371/journal.pbio.1000313
The Legume Phylogeny Working Group, Bruneau, A., Doyle, J. J., Herendeen, P., Hughes, C., Kenicer, G., et al. (2013). Legume phylogeny and classification in the 21st century: progress, prospects and lessons for other species-rich clades. Taxon 62, 217–248. doi: 10.12705/622.8
Tjallingii, W. F., and Esch, T. H. (1993). Fine-structure of aphid stylet routes in plant-tissues in correlation with EPG signals. Physiol. Entomol. 18, 317–328. doi: 10.1111/j.1365-3032.1993.tb00604.x
Tzin, V., Fernandez-Pozo, N., Richter, A., Schmelz, E. A., Schoettner, M., Schafer, M., et al. (2015). Dynamic maize responses to aphid feeding are revealed by a time series of transcriptomic and metabolomic assays. Plant Physiol. 169, 1727–1743. doi: 10.1104/pp.15.01039
Unsicker, S. B., Kunert, G., and Gershenzon, J. (2009). Protective perfumes: the role of vegetative volatiles in plant defense against herbivores. Curr. Opin. Plant Biol. 12, 479–485. doi: 10.1016/j.pbi.2009.04.001
Van Bel, A. J. E., and Will, T. (2016). Functional evaluation of proteins in watery and gel saliva of aphids. Front. Plant Sci. 7:1840. doi: 10.3389/fpls.2016.01840
Velázques, E., Silva, L. R., and Peix, Á (2010). Legumes: a healthy and ecological source of flavonoids. Curr. Nutr. Food Sci. 6, 109–144. doi: 10.2174/157340110791233247
Vincken, J. P., Heng, L., De Groot, A., and Gruppen, H. (2007). Saponins, classification and occurrence in the plant kingdom. Phytochemistry 68, 275–297. doi: 10.1016/j.phytochem.2006.10.008
Widstrom, N. W., and Snook, M. E. (2001). Recurrent selection for maysin, a compound in maize silks, antibiotic to earworm. Plant Breed. 120, 357–359. doi: 10.1046/j.1439-0523.2001.00610.x
Will, T., Tjallingii, W. F., Thonnessen, A., and Van Bel, A. J. E. (2007). Molecular sabotage of plant defense by aphid saliva. Proc. Natl. Acad. Sci. U.S.A. 104, 10536–10541. doi: 10.1073/pnas.0703535104
Wink, M. (2008). Evolutionary advantage and molecular modes of action of multi-component mixtures used in phytomedicine. Curr. Drug Metabol. 9, 996–1009. doi: 10.2174/138920008786927794
Wink, M. (2013). Evolution of secondary metabolites in legumes (Fabaceae). S. Afr. J. Bot. 89, 164–175. doi: 10.1016/j.sajb.2013.06.006
Wojciechowski, M. F., Lavin, M., and Sanderson, M. J. (2004). A phylogeny of legumes (Leguminosae) based on analyses of the plastid matK gene resolves many well-supported subclades within the family. Am. J. Bot. 91, 1846–1862. doi: 10.3732/ajb.91.11.1846
Wu, J., and Baldwin, I. T. (2010). New insights into plant responses to the attack from insect herbivores. Ann. Rev. Genet. 44, 1–24. doi: 10.1146/annurev-genet-102209-163500
Xia, J., and Wishart, D. S. (2011). Web-based inference of biological patterns, functions and pathways from metabolomic data using MetaboAnalyst. Nat. Protoc. 6, 743–760. doi: 10.1038/nprot.2011.319
Xia, J. G., Psychogios, N., Young, N., and Wishart, D. S. (2009). MetaboAnalyst: a web server for metabolomic data analysis and interpretation. Nucleic Acids Res. 37, W652–W660. doi: 10.1093/nar/gkp356
Zagrobelny, M., Bak, S., Rasmussen, A. V., Jorgensen, B., Naumann, C. M., and Moller, B. L. (2004). Cyanogenic glucosides and plant-insect interactions. Phytochemistry 65, 293–306. doi: 10.1016/j.phytochem.2003.10.016
Keywords: Acyrthosiphon pisum, pea aphid host races, legume metabolome, saponins, flavonoids, Medicago sativa, Trifolium pratense, Pisum sativum
Citation: Sanchez-Arcos C, Kai M, Svatoš A, Gershenzon J and Kunert G (2019) Untargeted Metabolomics Approach Reveals Differences in Host Plant Chemistry Before and After Infestation With Different Pea Aphid Host Races. Front. Plant Sci. 10:188. doi: 10.3389/fpls.2019.00188
Received: 15 March 2018; Accepted: 05 February 2019;
Published: 28 February 2019.
Edited by:
Andreia Figueiredo, Universidade de Lisboa, PortugalReviewed by:
Anna-Maria Botha-Oberholster, Stellenbosch University, South AfricaJavier Sanchez, University of Zurich, Switzerland
Copyright © 2019 Sanchez-Arcos, Kai, Svatoš, Gershenzon and Kunert. This is an open-access article distributed under the terms of the Creative Commons Attribution License (CC BY). The use, distribution or reproduction in other forums is permitted, provided the original author(s) and the copyright owner(s) are credited and that the original publication in this journal is cited, in accordance with accepted academic practice. No use, distribution or reproduction is permitted which does not comply with these terms.
*Correspondence: Grit Kunert, Z2t1bmVydEBpY2UubXBnLmRl
†Present address: Carlos Sanchez-Arcos, Institute for Inorganic and Analytical Chemistry, Friedrich Schiller University Jena, Jena, Germany Marco Kai, Department of Biochemistry, University Rostock, Rostock, Germany