- Department of Agronomy, Iowa State University, Ames, IA, United States
A direct role for cholesterol signaling in mammals is clearly established; yet, the direct role in signaling for a plant sterol or sterol precursor is unclear. Fluctuations in sitosterol and stigmasterol levels during development and stress conditions suggest their involvement in signaling activities essential for plant development and stress compensation. Stigmasterol may be involved in gravitropism and tolerance to abiotic stress. The isolation of stigmasterol biosynthesis mutants offers a promising tool to test the function of sterol end products in signaling responses to developmental and environmental cues.
Introduction
Unlike mammals and fungi, plants produce mixtures of sterols, including sitosterol, stigmasterol, campesterol, and cholesterol (Figure 1). The interaction of sterols with phospholipids helps plant cells to maintain plasma membrane fluidity and permeability during stress conditions (Grunwald, 1971; Hartmann, 1998). In addition, sterols are precursors in the synthesis of steroid hormones, e.g., testosterone, estrogen, glucocorticoids, and mineral corticoids in mammals, ecdysteroids in insects and crustaceans, antheridiol and oogoniol (mating hormones of fungi), and brassinosteroids (BR) in plants (Fujioka et al., 1997; Noguchi et al., 1999; Nomura et al., 1999; Clouse, 2002).
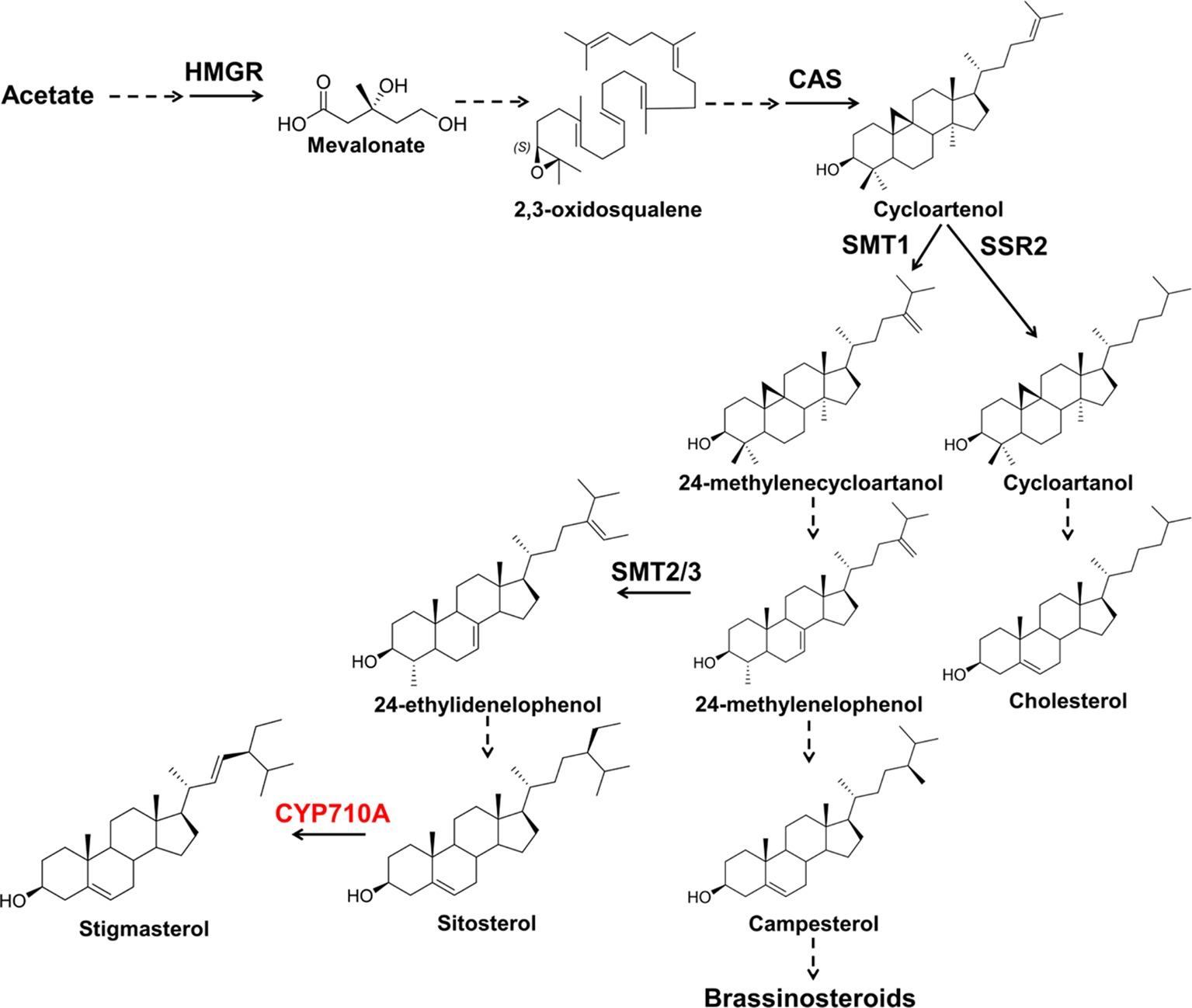
Figure 1. The plant sterol pathway leading to stigmasterol. Plants produce a mixture of sterols, campesterol (24-methyl) and sitosterol and stigmasterol (24-ethyl sterols). Stigmasterol is derived from sitosterol by the action of sterol C-22 desaturases. Campesterol is the preferred precursor of brassinosteroids (BR). Dashed arrows indicate multiple steps and solid arrows denote single step in the pathway. HMGR, 3-hydroxy-3-methylglutaryl-CoA reductase; CAS, cycloartenol synthase; SMT1, sterol methyltransferase 1; SMT2/3, sterol methyltransferase2/3; SSR2, sterol sidechain reductase2; CYP710A, sterol C-22 desaturase.
Campesterol is the precursor of BR (Fujioka and Yokota, 2003), and the crucial role of BR in plant growth and development is well established (Fujioka et al., 1997; Choe et al., 1998, 1999; Noguchi et al., 1999; Nomura et al., 1999; Clouse, 2002), while sitosterol is implicated in cellulose synthesis (Peng et al., 2002; Schrick et al., 2012). It is, however, unclear whether fluctuations in stigmasterol concentration observed during development and conditions of stress are responsible for cellular signaling. Nonetheless, evidence pointing to stigmasterol as a potential signal for cellular defense and gravitropic responses is emerging (Griebel and Zeier, 2010; Dalal et al., 2016). Functional characterization of genes controlling stigmasterol biosynthesis might help increase our understanding of the direct role of this sterol in plant development and stress responses. Therefore, our purpose is to examine genetic, developmental, and environmental conditions affecting stigmasterol production and suggest experimental approaches to investigate the role of stigmasterol in cell signaling.
The Biosynthesis of Stigmasterol
Stigmasterol is produced in the mevalonate pathway following a series of enzyme-catalyzed reactions leading to the generation of 2,3-oxidosqualene (Schaller, 2003; Bach, 2016). Subsequently, 2,3-oxidosqualene is converted to cycloartenol by cycloartenol synthase (Schaller, 2003; Gas-Pascual et al., 2014; Sonawane et al., 2016). Cycloartenol is the target of branch-point enzymes including sterol side chain reductase 2 (SSR2) and sterol methyl transferase 1 (SMT1). SSR2 channels cycloartenol to the cholesterol branch, while SMT1 catalyzes the alkylation of cycloartenol to produce precursors for plant sterols (Benveniste, 1986; Nes and Venkatramesh, 1999; Diener et al., 2000; Schaeffer et al., 2001; Sonawane et al., 2016). Downstream of SMT1, other branching enzymes SMT2/3, directs carbon toward sitosterol and stigmasterol (Carland et al., 2010). Besides biosynthesis, free stigmasterol content can also be modulated by converting it to sterol conjugates such as steryl esters, steryl glucosides, and acyl steryl glucosides. Steryl esters are conjugated by acyl transferases (Chen et al., 2007; Bouvier-Navé et al., 2010) and the steryl glucosides by UDP-glucose: sterol glucosyltransferase (DeBolt et al., 2009).
Structurally, stigmasterol is similar to sitosterol but differs from sitosterol due to a double bond at position C-22 introduced by the sterol C-22 desaturase (Benveniste, 2002; Morikawa et al., 2006). Arabidopsis contains four genes encoding sterol C-22 desaturases belonging to the cytochrome P450, CYP710A superfamily (Benveniste, 2004; Morikawa et al., 2006) and two sterol C-22 desaturases are responsible for stigmasterol biosynthesis in Physcomitrella patens, a moss in which the major sterol is stigmasterol (Morikawa et al., 2009). The number of genes encoding/predicted to encode sterol C-22 desaturase, however, varies across species (Supplementary Table S1). It is noteworthy that SMT2/3 and CYP710A1 are the only two unique enzymes leading to the biosynthesis of stigmasterol in the stigmasterol branch, while both campesterol and stigmasterol branches (Figure 1) share intermediate enzymes. This is a case similar to cholesterol synthesis in plants, where the pathway involves both unique and shared enzymes with the campesterol branch (Sonawane et al., 2016).
Developmental Regulation of Stigmasterol
In mammalian systems, cholesterol biosynthesis is regulated via negative feedback suppression of several key genes (Goldstein and Brown, 1990). Thus, analysis of gene transcripts may help increase our understanding of the regulation of sterol biosynthesis during plant development (Schrick et al., 2011; Sonawane et al., 2016; Suza and Chappell, 2016). For instance, in the developing seeds of tobacco (N. tabacum), pea (Pisum sativum), rape (Brassica napus), and seedlings of N. benthamiana and B. napus, increased gene expression and enzyme activities coincides with sterol accumulation (Harker et al., 2003; Schrick et al., 2011; Suza and Chappell, 2016). In addition, apical tissues of B. campestris contain high levels of cholesterol but exhibit a decline in cholesterol and a rise in sitosterol at later stages of development (Hobbs et al., 1996). Moreover, varying concentrations of stigmasterol and its precursor are noticeable at both the seed and whole plant developmental stages. For instance, during germination of tobacco seed, stigmasterol increases two-fold (Bush and Grunwald, 1972), and in mung bean (Vigna radiata) seedlings, younger sections of hypocotyls contain higher levels of stigmasterol compared to sitosterol (Stalleart and Geuns, 1994).
Stigmasterol content also increases in tomato (Solanum lycopersicon) during fruit ripening and is associated with an increase in CYP710A11 gene expression (Whitaker and Gapper, 2008). In addition, in maize (Zea mays) seedlings, the concentration of stigmasterol is higher in roots than in shoots (Kemp et al., 1967). Similar to the findings of Kemp et al. (1967), N. benthamiana seedlings display striking differences in sterol composition between organs, with higher stigmasterol content in roots than in leaves (Suza and Chappell, 2016). In contrast, stigmasterol concentration is elevated in P. sativum leaves but lower in seeds (Schrick et al., 2011). Taken together, the developmental profile of sterols and gene expression data from Arabidopsis (Supplementary Figure S1) suggests highly coordinated regulation of stigmasterol metabolism in plants.
Impact of Biotic and Abiotic Stress on Stigmasterol
In Solanaceous plants, e.g., potato (Solanum tuberosum), cholesterol production rises to match the demand for the synthesis of steroid glycoalkaloids in response to wounding or pathogen infection (Choi et al., 1992; Hartmann, 1998; Arnqvist et al., 2003). Similarly, pathogenic bacteria and reactive oxygen species stimulate the biosynthesis of stigmasterol in Arabidopsis (Griebel and Zeier, 2010; Sewelam et al., 2014). Furthermore, genes encoding sterol C-22 desaturase are responsive to phytohormones, suggesting a role for stigmasterol in various stress responses (Supplementary Figure S1). Indeed, the overexpression of one of the Arabidopsis stigmasterol biosynthesis genes resulted in enhanced resistance to bacterial pathogens (Wang et al., 2012). Recently, Gamir et al. (2017) reported that PATHOGENESIS-RELATED PROTEIN 1 (PR-1) can bind sterols including stigmasterol in vitro. The authors conclude that PR-1 inhibits pathogen growth by sequestering sterols from pathogens (Gamir et al., 2017). However, it remains to be demonstrated whether the predicted ability of PR-1 to bind stigmasterol has a real biological significance.
Stigmasterol concentration increases in roots of wheat (Triticum aestivum) exposed to salt (Magdy et al., 1994). In addition, salt-induced increase in stigmasterol is associated with salt exclusion capacity of citrus (Citrus medica) rootstocks (Douglas and Walker, 1983), possibly due to the activation of the plasma membrane H+-ATPase by stigmasterol (Grandmougin-Ferjani et al., 1997). The plasma membrane H+-ATPase is the primary transporter of protons out of the cell (Muramatsu et al., 2002), and its activity is essential for maintaining ion homeostasis (Niu et al., 1995). Indeed, stigmasterol treatment of germinating seeds improved salt tolerances of faba beans (Vicia faba L.) and flax (Linum usitatissimum) (Hashem et al., 2011; Hassanein et al., 2012).
Plants grown in saline conditions experience retardation of root growth, but Ca2+ supply ameliorates these deleterious effects of salinity stress (Shabala et al., 2003). The beneficial effect of Ca2+ in the context of salinity stress is associated with the stabilization of plasma membrane and enhanced exchange of cations such as Na+ (Hirschi, 2004). It appears that Ca2+ may stimulate stigmasterol production in roots (Pilar et al., 1993; Magdy et al., 1994), and possibly, the stigmasterol induced by Ca2+ affects the plasma membrane H+-ATPase (Grandmougin-Ferjani et al., 1997), leading to enhanced extrusion of Na+ from the cell (Qiu et al., 2003).
Stigmasterol is elevated at the expense of sitosterol in tomato (Lycopersicon esculentum) when stored at 15°C (Whitaker, 1991). Indeed, analysis of Arabidopsis over-expressing AtCYP710A1 and Atcyp710a1 mutant lines suggests a role for stigmasterol in tolerance to unfavorable temperatures (Senthil-Kumar et al., 2013). Higher levels of sitosterol are detected in etiolated barley (Hordeum vulgare) tissues compared to stigmasterol, but the two sterols are detected in equal amounts in green tissues (Bush et al., 1971). Similar to etiolated barley, soybean plants grown under filtered sunlight conditions accumulate sitosterol, while stigmasterol levels decrease (Izzo and Navari-Izzo, 1981). The fluctuations in stigmasterol content in response to various environmental cues suggest that the conversion of sitosterol to stigmasterol may modulate plant response to environmental stimuli.
Potential Role for Stigmasterol in Cell Signaling
Cholesterol modulates its own biosynthesis in mammalian cells via negative feedback (Marigo and Tabin, 1996; Edwards and Ericsson, 1999). Research in Solanum species suggested the existence of analogous cholesterol feedback mechanisms in plants (Bhatt and Bhatt, 1984); however, the idea that cholesterol modulates sterol biosynthesis in plants did not escape skepticism, since unlike mammals, plants synthesize an array of sterol end products (Hartmann, 1998). Production of several sterol end products presents a challenge in elucidating role of sterol end products in cell signaling in plants.
Analysis of Arabidopsis sterol biosynthesis mutants suggests that sterols play critical roles in plant development independent of BR (Lindsey et al., 2003) by influencing position-dependent cell fate during embryogenesis (Jang et al., 2000; Schrick et al., 2000; Clouse, 2002). For example, the fackel mutants lacking a functional sterol C-14 reductase display embryonic defects and dwarfism at the seedling stage and produce less BR, but exogenous BR fails to complement the mutant (Mayer et al., 1991; Jang et al., 2000; Schrick et al., 2000), whereas the loss of SMT1 function in smt1/cph plants results in the accumulation of cholesterol, defective embryo development, and increased sensitivity to Ca2+. Similar to fackel, the defective phenotype of smt1/cph plants cannot be rescued by exogenous BR (Diener et al., 2000).
The SMT2/3 (COTYLEDON VASCULAR PATTERNING1—CVP1) locus converts 24-methylene lophenol to 24-ethylidene lophenol (Carland et al., 2002). Consequently, Arabidopsis plants overexpressing SMT2 accumulate sitosterol at the expense of campesterol and display reduced stature and growth (Schaller et al., 1998; Schaeffer et al., 2001). The smt2/cvp1 plants exhibit aberrant alignment of vascular strands and misshapen vascular cells, reduced levels of sitosterol, and higher concentration of campesterol (Schaeffer et al., 2001; Carland et al., 2002); however, the aberrant phenotype of AtSMT2 and smt2/cvp1 plants is not associated with defective BR signaling (Schaller et al., 1998; Schaeffer et al., 2001; Carland et al., 2002).
Another classic Arabidopsis sterol mutant is hydra, with defective embryonic morphogenesis, seedling cell patterning, and root growth (Lindsey et al., 2003). HYDRA1 and HYDRA2/FACKEL encode sterol isomerase and C-14 reductase, respectively (Souter et al., 2002). Similar to fackel, hydra mutants produce less campesterol, but BR application does not rescue their phenotypic defects. Interestingly, both hydra1 and hydra2/fackel mutants produce high levels of stigmasterol compared to the wild type (Souter et al., 2002). Whether dysregulation of stigmasterol is the cause for the pleiotropic defects in the hydra mutants is unclear.
The compactness in the packing of plasma membrane (PM) lipid bilayer acyl chains—referred as membrane order (or liquid-ordered)—is influenced by sterol composition (Roche et al., 2008). The separation of liquid-ordered and liquid-disordered phases in the PM is observed in vivo in tobacco cells (Gerbeau-Pissot et al., 2014). In “raft hypothesis,” stress induction can lead to the formation of larger structures (proposed lipid rafts) from liquid-ordered nanodomains enriched in sterols and sphingolipids (Lingwood and Simons, 2010). The interaction of sterols with phospholipids to form lipid rafts in mammalian membrane systems is crucial for correct signaling and activity of intrinsic membrane proteins. Lipid rafts are associated with many plant proteins involved in redox regulation, hormone transport and signaling, and ion homeostasis (Willemsen et al., 2003; Borner et al., 2005; Lefebvre et al., 2007; Zauber et al., 2014). Examples of proteins associated with lipid rafts and sterols include GLABRA2 (GL2), SCRAMBLED (SCM), and PIN-FORMED (PIN). PIN proteins are involved in the transport of auxin to mediate polar cell growth and root gravitropism (Moore, 2002). GL2 is a phospholipid/sterol-binding transcription factor involved in the regulation of root hair development (Masucci et al., 1996), whereas SCM is a receptor for positional cues to modulate expression of GL2 and other cell fate regulators during root hair development (Grierson et al., 2014). Indeed, proteome analysis of smt1/cph, with an altered plasma membrane composition, revealed a compromised cell signaling (Zauber et al., 2014). Sterol depletion in the plasma membrane by cyclodextrin and filipin suggests the sensing of modifications of cell environment at the PM is sterol dependent in plants, which can lead to adaptive cell responses through regulated signaling processes (Roche et al., 2008; Bonneau et al., 2010). In tobacco cells, the proportion of ordered phases transiently increased during the early steps of the signaling triggered by cryptogein and flagellin, two elicitors of plant defense reactions (Gerbeau-Pissot et al., 2014).
The composition of free sterols and sterol conjugates influences the liquid-ordered phase formation (Grosjean et al., 2015). Stigmasterol by itself lacks the ability to increase membrane order, whereas sitosterol and campesterol increase the order. However, by interacting together with glycosylinositolphosphoceramide, the major sphingolipid in plant, stigmasterol can increase the membrane order, while the interaction with glucosylceramide decreased the order. Sitosterol by itself induces the production of many small domains, which increases in size together with the addition of free sterol-sphingolipid and free sterol-sterylglycoside/acylsterylglycoside combination (Grosjean et al., 2015). These findings suggest a role for specific sterol species to fine tune the membrane sterol composition, thereby regulating signaling events.
The orc mutation is allelic to SMT1, and analysis of the smt1orc plants revealed trace amounts of stigmasterol and aberrant localization of PIN2 and PIN3 (Willemsen et al., 2003). Therefore, regulated membrane sterol composition is important for correct positioning of proteins, such as PIN, and physiological responses such as root gravitropism (Men et al., 2008). The hydra2/fackel plants show an ectopic expression of GL2 in trichoblasts, resulting in a glabrous root phenotype possibly due to a compromised function of GL2 (Souter et al., 2002). There is a possibility that GL2 activity in hydra/fackel plants is diminished by a sterol molecule which causes a conformational change blocking DNA interaction with certain trans-factors (Schrick et al., 2004). Conversely, a sterol or its derivative may bind GL2 and tether it to the membrane in a manner similar to the way cholesterol tethers Hedgehog in vertebrate systems (Jeong and McMahon, 2002). Since stigmasterol plays a role in cell proliferation (Hartmann, 1998) and hydra/fackel plants accumulate high levels of stigmasterol (Lindsey et al., 2003), a dysregulated metabolism of stigmasterol may interfere with various cellular processes during development. Indeed, GL2 expression is dysregulated in the developing siliques of the Arabidopsis acbp1 mutant with high stigmasterol (Lung et al., 2017). Perhaps, the aberrant SCM distribution in roots of the ugt80B1 is due to deficiency in a stigmasterol conjugate (Pook et al., 2017), offering additional support for a role for stigmasterol in cell signaling.
Stigmasterol Role in Modulating Cell Biology
Stigmasterol is one of the major sterols in plasma membranes of plant cells and plays a role in cell proliferation (Hartmann, 1998) and activation of plasma membrane H+-ATPase (Grandmougin-Ferjani et al., 1997). In plants, the plasma membrane H+-ATPase is the primary transporter of protons out of the cell, thus creating a pH and electrochemical gradient across the plasma membrane (Muramatsu et al., 2002). The activity of the plasma membrane H+-ATPase is essential for maintaining ion homeostasis, since carrier-mediated ion transport is coupled to a downhill pH gradient (Niu et al., 1995). In addition, the activity of the plasma membrane H+-ATPase promotes the adaptation of maize roots to low pH (Yan et al., 1998) and low phosphorous availability in soybeans (Shen et al., 2006).
In Arabidopsis, exogenous stigmasterol activates the expression of genes involved in cell expansion and division (He et al., 2003). Furthermore, exogenous stigmasterol increases flower numbers of chamomile (Chamomilla recutita L. Rausch) (Abd El-Wahed and Krima, 2004) and in vitro multiplication of shoots of Marubakaido apple rootstock (Malus prunifolia (Wild.) Borkh) (Pereira-Netto, 2012). In tobacco seeds, depletion of cycloartenol by increased activity of SMT1 was associated with elevated activity of HMGR in tobacco seeds (Holmberg et al., 2002). By contrast, studies in P. sativum showed that stigmasterol inhibits HMGR activity (Stermer et al., 1994), suggesting a role for stigmasterol in regulation of sterol biosynthesis.
In Arabidopsis, the key gene controlling stigmasterol production is CYP710A1, but Arabidopsis also produces low levels of brassicasterol from the C-22 desaturation of epi-campesterol by CYP710A2 (Benveniste, 2002; Morikawa et al., 2006). The expression of CYP710A2 mRNA responds rapidly to gravity stimulation (Kimbrough et al., 2004), suggesting a role for sterol C-22 desaturation in plants response to gravity. The recent discovery that InteractoR Of SYnaptotagmin1 (ROSY1), a regulator of cellular trafficking and gravitropic response binds stigmasterol (Dalal et al., 2016), supports the idea that CYP710A genes and stigmasterol play a role in root response to gravity. In addition, the rosy1-1 mutant is impaired in auxin transport but is more tolerant to salt stress (Dalal et al., 2016), suggesting a connection between ROSY1 and stigmasterol in regulating auxin transport and abiotic stress responses.
Stigmasterol is induced by Ca2+ (Pilar et al., 1993), and Arabidopsis mutants defective in Ca2+ uptake have a compromised cell expansion, short root hairs, and stunted roots (Foreman et al., 2003). Since gravistimulation induces Ca2+ (Monshausen et al., 2011), Ca2+ may stimulate CYP710A2 expression and stigmasterol production in roots. Therefore, CYP710A proteins might participate in a similar signaling pathway with ROSY1 to modulate plant cell response to gravity and salt stress (Dalal et al., 2016).
Concluding Remarks and Future Directions
There is need to validate gene expression data from microarray experiments (Supplementary Figure S1) and correlate hormone/stress induced gene expression with stigmasterol concentration. In addition, it is intriguing that blocking BR biosynthesis affects CYP710A gene expression (Supplementary Figure S1), suggesting a role for BR in regulating stigmasterol metabolism. Based on the mechanism for cholesterol and lipid sensing in mammals and insects, the notion of plant (stigma)sterol sensor(s) is not far-fetched. Indeed, the discovery of plant proteins with sterol/lipid sensing/binding domains offers a promising avenue for testing the signaling role of stigmasterol (Figure 2).
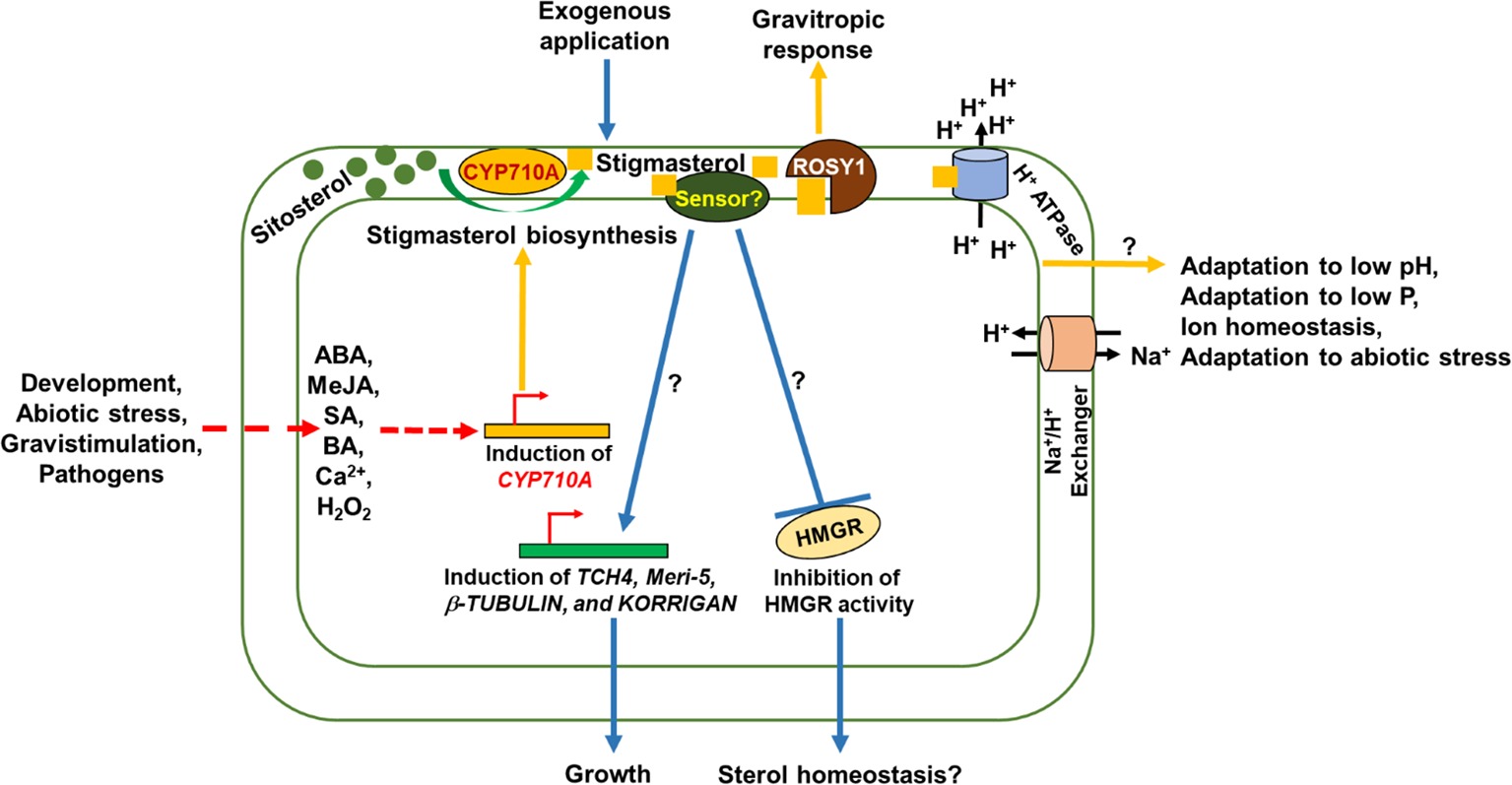
Figure 2. Schematic representation of functions of stigmasterol and its signaling roles in plant cells. Stigmasterol biosynthesis occurs during development, abiotic stress, gravistimulation, pathogen attack, and in response to signaling molecules such as abscisic acid (ABA), methyl jasmonate (MeJA), salicylic acid (SA), calcium (Ca2+), and hydrogen peroxide (H2O2). Stigmasterol binds to ROSY1 leading to gravitropic response. Stigmasterol activates H+-ATPase to create pH and electrochemical gradient across the plasma membrane. The pH gradient leads to activation of the Na+/H+ exchanger to exclude Na+ to adapt to salinity stress. Furthermore, the activity of H+-ATPase is necessary for ion homeostasis, adaptation to low pH, low phosphorus, and abiotic stress. Exogenous application of stigmasterol impacts growth and sterol homeostasis via an unidentified (stigma)sterol sensor.
The potential candidate for a stigmasterol sensing system would be ROSY1, which shows binding specificity for stigmasterol to regulate root response to gravity (Dalal et al., 2016). Other candidates include Arabidopsis Niemann-Pick disease type C like proteins (AtNPC1-1 and AtNPC1-2) (Feldman et al., 2015), possessing putative sterol sensing domains reminiscent of SCAP and related regulators of sterol metabolism in animals and yeast (Goldstein and Brown, 1990; Nohturfft and Losick, 2002). Evaluating the sterol binding specificity of plant NPC proteins might provide clues as to whether AtNPC1-1 and AtNPC1-2 act as sterol sensors to modulate lipid metabolism; however, testing the implication of stigmasterol interaction with plant sterol sensing proteins requires circumventing gene redundancy (Supplementary Table S1). The creation of double/triple/quadruple mutants for Arabidopsis CYP710A genes may help in overcoming the challenge. Alternatively, crop or model grass species predicted to encode single copies of CYP710A (Supplementary Table S1), and rich genetic resources, such as maize or Brachypodium, may provide an opportunity to attempt to eliminate the production of stigmasterol via insertional mutagenesis or gene editing approaches.
Sterol glucosides are synthesized at the PM (Zauber et al., 2014), while the CYP710A is predicted to localize to the apoplast (Supplementary Table S1). This begs the question of what would be the cellular site of stigmasterol synthesis, since plant sterols are believed to originate primarily within the ER (Hartmann, 1998). Experiments to test the impact of ectopic expression of CYP710A via retention to ER or vacuole may help identify the preferred site of stigmasterol synthesis. This information will be helpful in designing gene constructs to manipulate stigmasterol content in a more targeted fashion.
Data Availability
Publicly available datasets were analyzed in this study. These data can be found here: https://www.arabidopsis.org/servlets/Search?type=general&search_action=detail&method=1&show_obsolete=F&name=cyp710a&sub_type=gene&SEARCH_EXACT=4&SEARCH_CONTAINS=1.
Author Contributions
WS and SA designed the research and wrote the paper.
Funding
This work was financially supported by USAID project iAGRI (grant no. 621-A-00-11-000090-00).
Conflict of Interest Statement
The authors declare that the research was conducted in the absence of any commercial or financial relationships that could be construed as a potential conflict of interest.
Acknowledgments
We apologize to those colleagues whose important contributions may have been omitted owing to space constraints.
Supplementary Material
The Supplementary Material for this article can be found online at: https://www.frontiersin.org/articles/10.3389/fpls.2019.00354/full#supplementary-material
Abbreviations
BR, Brassinosteroid; CPH, Cephalopod; CVP, Cotyledon vascular patterning; CYP710A, Sterol C-22 desaturase; GL2, GLABRA2; NPC, Niemann-Pick disease type C; PIN, PIN-FORMED; PR-1, PATHOGENESIS-RELATED PROTEIN 1; PM, Plasma membrane; ROSY1, InteractoR of SYnaptotagmin; SCM, SCRAMBLED; SMT, Sterol methyltransferase; SSR, Sterol sidechain reductase.
References
Abd El-Wahed, M. S. A., and Krima, M. G. E. D. (2004). Stimulation of growth, flowering, biochemical constituents and essential oil of chamomile plant (Chamomilla recutita L., Rausch) with spermidine and stigmasterol application. Bulg. J. Plant Physiol. 30, 89–102.
Arnqvist, L., Dutta, P. C., Jonsson, L., and Sitbon, F. (2003). Reduction of cholesterol and glycoalkaloid levels in transgenic potato plants by overexpression of a type 1 sterol methyltransferase cDNA. Plant Physiol. 131, 1792–1799. doi: 10.1104/pp.102.018788
Bach, T. J. (2016). Secondary metabolism: high cholesterol in tomato. Nat. Plants 3:16213. doi: 10.1038/nplants.2016.213
Benveniste, P. (1986). Sterol biosynthesis. Annu. Rev. Plant Physiol. 37, 275–308. doi: 10.1146/annurev.pp.37.060186.001423
Benveniste, P. (2004). Biosynthesis and accumulation of sterols. Annu. Rev. Plant Biol. 55, 429–457. doi: 10.1146/annurev.arplant.55.031903.141616
Bhatt, P. N., and Bhatt, D. P. (1984). Regulation of sterol biosynthesis in Solanum species. J. Exp. Bot. 35, 890–896. doi: 10.1093/jxb/35.6.890
Bonneau, L., Gerbeau-Pissot, P., Thomas, D., Der, C., Lherminier, J., Bourque, S., et al. (2010). Plasma membrane sterol complexation, generated by filipin, triggers signaling responses in tobacco cells. Biochim. Biophys. Acta 1798, 2150–2159. doi: 10.1016/j.bbamem.2010.07.026
Borner, G. H. H., Sherrier, D. J., Weimar, T., Michaelson, L. V., Hawkins, N. D., MacAskill, A., et al. (2005). Analysis of detergent-resistant membranes in Arabidopsis. Evidence for plasma membrane lipid rafts. Plant Physiol. 137, 104–116. doi: 10.1104/pp.104.053041
Bouvier-Navé, P., Berna, A., Noiriel, A., Compagnon, V., Carlsson, A. S., Banas, A., et al. (2010). Involvement of the phospholipid sterol acyltransferase1 in plant sterol homeostasis and leaf senescence. Plant Physiol. 152, 107–119. doi: 10.1104/pp.109.145672
Bush, P. B., and Grunwald, C. (1972). Sterol changes during germination of Nicotiana tabacum seeds. Plant Physiol. 50, 69–72. doi: 10.1104/pp.50.1.69
Bush, P. B., Grunwald, C., and Davis, D. L. (1971). Changes in sterol composition during greening of etiolated barley shoots. Plant Physiol. 47, 745–749. doi: 10.1104/pp.47.6.745
Carland, F., Fujioka, S., and Nelson, T. (2010). The sterol methyltransferases SMT1, SMT2, and SMT3 influence Arabidopsis development through nonbrassinosteroid products. Plant Physiol. 153, 741–756. doi: 10.1104/pp.109.152587
Carland, F. M., Fujioka, S., Takatsuto, S., Yoshida, S., and Nelson, T. (2002). The identification of CVP1 reveals a role for sterols in vascular patterning. Plant Cell 14, 2045–2058. doi: 10.1105/tpc.003939
Chen, Q., Steinhauer, L., Hamerlindl, J., Keller, W., and Zou, J. (2007). Biosynthesis of phytosterol esters: identification of a sterol O-acyltransferase in Arabidopsis. Plant Physiol. 145, 974–984. doi: 10.1104/pp.107.106278
Choe, S., Dilkes, B. P., Fujioka, S., Takatsuto, S., Sakurai, A., and Feldmann, K. A. (1998). The DWF4 gene of Arabidopsis encodes a cytochrome P450 that mediates multiple 22alpha-hydroxylation steps in brassinosteroid biosynthesis. Plant Cell 10, 231–243. doi: 10.1105/tpc.10.2.231
Choe, S., Noguchi, T., Fujioka, S., Takatsuto, S., Tissier, C. P., Gregory, B. D., et al. (1999). The Arabidopsis dwf7/ste1 mutant is defective in the delta7 sterol C-5 desaturation step leading to brassinosteroid biosynthesis. Plant Cell 11, 207–221. doi: 10.1105/tpc.11.2.207
Choi, D., Ward, B. L., and Bostock, R. M. (1992). Differential induction and suppression of potato 3-hydroxy-3-methylglutaryl coenzyme A reductase genes in response to Phytophthora infestans and to its elicitor arachidonic acid. Plant Cell 4, 1333–1344. doi: 10.1105/tpc.4.10.1333
Clouse, S. D. (2002). Arabidopsis mutants reveal multiple roles for sterols in plant development. Plant Cell 14, 1995–2000. doi: 10.1105/tpc.140930
Dalal, J., Lewis, D. R., Tietz, O., Brown, E. M., Brown, C. S., Palme, K., et al. (2016). ROSY1, a novel regulator of gravitropic response is a stigmasterol binding protein. J. Plant Physiol. 196–197, 28–40. doi: 10.1016/j.jplph.2016.03.011
DeBolt, S., Scheible, W. -R., Schrick, K., Auer, M., Beisson, F., Bischoff, V., et al. (2009). Mutations in UDP-Glucose:sterol glucosyltransferase in Arabidopsis cause transparent testa phenotype and suberization defect in seeds. Plant Physiol. 151, 78–87. doi: 10.1104/pp.109.140582
Diener, A. C., Li, H., Zhou, W., Whoriskey, W. J., Nes, W. D., and Fink, G. R. (2000). Sterol methyltransferase 1 controls the level of cholesterol in plants. Plant Cell 12, 853–870. doi: 10.1105/tpc.12.6.853
Douglas, T. J., and Walker, R. R. (1983). 4-Desmethylsterol composition of citrus rootstocks of different salt exclusion capacity. Physiol. Plant. 58, 69–74. doi: 10.1111/j.1399-3054.1983.tb04145.x
Edwards, P. A., and Ericsson, J. (1999). Sterols and isoprenoids: signaling molecules derived from the cholesterol biosynthetic pathway. Annu. Rev. Biochem. 68:157–185. doi: 10.1146/annurev.biochem.68.1.157
Feldman, M. J., Poirier, B. C., and Lange, B. M. (2015). Misexpression of the Niemann-Pick disease type C1 (NPC1)-like protein in Arabidopsis causes sphingolipid accumulation and reproductive defects. Planta 242, 921–933. doi: 10.1007/s00425-015-2322-4
Foreman, J., Demidchik, V., Bothwell, J. H. F., Mylona, P., Miedema, H., Torres, M. A., et al. (2003). Reactive oxygen species produced by NADPH oxidase regulate plant cell growth. Nature 422, 442–446. doi: 10.1038/nature01485
Fujioka, S., Li, J., Choi, Y. H., Seto, H., Takatsuto, S., Noguchi, T., et al. (1997). The Arabidopsis deetiolated2 mutant is blocked early in brassinosteroid biosynthesis. Plant Cell 9, 1951–1962. doi: 10.1105/tpc.9.11.1951
Fujioka, S., and Yokota, T. (2003). Biosynthesis and metabolism of brassinosteroids. Annu. Rev. Plant Biol. 54, 137–164. doi: 10.1146/annurev.arplant.54.031902.134921
Gamir, J., Darwiche, R., Van’t Hof, P., Choudhary, V., Stumpe, M., Schneiter, R., et al. (2017). The sterol-binding activity of PATHOGENESIS-RELATED PROTEIN 1 reveals the mode of action of an antimicrobial protein. Plant J. 89, 502–509. doi: 10.1111/tpj.13398
Gas-Pascual, E., Berna, A., Bach, T. J., and Schaller, H. (2014). Plant oxidosqualene metabolism: cycloartenol synthase-dependent sterol biosynthesis in Nicotiana benthamiana. PLoS One 9:e109156. doi: 10.1371/journal.pone.0109156
Gerbeau-Pissot, P., Der, C., Thomas, D., Anca, I. -A., Grosjean, K., Roche, Y., et al. (2014). Modification of plasma membrane organization in tobacco cells elicited by cryptogein. Plant Physiol. 164, 273–286. doi: 10.1104/pp.113.225755
Goldstein, J. L., and Brown, M. S. (1990). Regulation of the mevalonate pathway. Nature 343:425. doi: 10.1038/343425a0
Grandmougin-Ferjani, A., Schuler-Muller, I., and Hartmann, M. A. (1997). Sterol modulation of the plasma membrane H+-ATPase activity from corn roots reconstituted into soybean lipids. Plant Physiol. 113, 163–174. doi: 10.1104/pp.113.1.163
Griebel, T., and Zeier, J. (2010). A role for beta-sitosterol to stigmasterol conversion in plant-pathogen interactions. Plant J. 63, 254–268. doi: 10.1111/j.1365-313X.2010.04235.x
Grierson, C., Nielsen, E., Ketelaarc, T., and Schiefelbein, J. (2014). Root hairs. Arabidopsis Book 12:e0172. doi: 10.1199/tab.0172
Grosjean, K., Mongrand, S., Beney, L., Simon-Plas, F., and Gerbeau-Pissot, P. (2015). Differential effect of plant lipids on membrane organization: specificities of phytosphingolipids and phytosterols. J. Biol. Chem. 290, 5810–5825. doi: 10.1074/jbc.M114.598805
Grunwald, C. (1971). Effects of free sterols, steryl ester, and steryl glycoside on membrane permeability. Plant Physiol. 48, 653–655. doi: 10.1104/pp.48.5.653
Harker, M., Hellyer, A., Clayton, J., Duvoix, A., Lanot, A., and Safford, R. (2003). Co-ordinate regulation of sterol biosynthesis enzyme activity during accumulation of sterols in developing rape and tobacco seed. Planta 216, 707–715. doi: 10.1007/s00425-002-0913-3
Hartmann, M. -A. (1998). Plant sterols and the membrane environment. Trends Plant Sci. 3, 170–175. doi: 10.1016/S1360-1385(98)01233-3
Hashem, H. A., Bassuony, F. M., Hassanein, R. A., Baraka, D. M., and Khalil, R. R. (2011). Stigmasterol seed treatment alleviates the drastic effect of NaCl and improves quality and yield in flax plants. Aust. J. Crop. Sci. 5, 1858–1867.
Hassanein, R. A., Hashem, H. A., and Khalil, R. R. (2012). Stigmasterol treatment increases salt stress tolerance of faba bean plants by enhancing antioxidant systems. Plant Omics 5, 476–485.
He, J. -X., Fujioka, S., Li, T. -C., Kang, S. G., Seto, H., Takatsuto, S., et al. (2003). Sterols regulate development and gene expression in Arabidopsis. Plant Physiol. 131, 1258–1269. doi: 10.1104/pp.014605
Hirschi, K. D. (2004). The calcium conundrum. Both versatile nutrient and specific signal. Plant Physiol. 136, 2438–2442. doi: 10.1104/pp.104.046490
Hobbs, D. H., Hume, J. H., Rolph, C. E., and Cooke, D. T. (1996). Changes in lipid composition during floral development of Brassica campestris. Phytochemistry 42, 335–339. doi: 10.1016/0031-9422(95)00964-7
Holmberg, N., Harker, M., Gibbard, C. L., Wallace, A. D., Clayton, J. C., Rawlins, S., et al. (2002). Sterol C-24 methyltransferase type 1 controls the flux of carbon into sterol biosynthesis in tobacco seed. Plant Physiol. 130, 303–311. doi: 10.1104/pp.004226
Izzo, R., and Navari-Izzo, F. (1981). Sterol composition and accumulation in Glycine max (L.) Merr leaves under different filtered sunlight conditions. Plant Physiol. 67, 1073–1077. doi: 10.1104/pp.67.6.1073
Jang, J. C., Fujioka, S., Tasaka, M., Seto, H., Takatsuto, S., Ishii, A., et al. (2000). A critical role of sterols in embryonic patterning and meristem programming revealed by the fackel mutants of Arabidopsis thaliana. Genes Dev. 14, 1485–1497. doi: 10.1101/gad.14.12.1485
Jeong, J., and McMahon, A. P. (2002). Cholesterol modification of Hedgehog family proteins. J. Clin. Invest. 110, 591–596. doi: 10.1172/JCI200216506
Kemp, R. J., Goad, L. J., and Mercer, E. I. (1967). Changes in the levels and composition of the esterified and unesterified sterols of maize seedlings during germination. Phytochemistry 6, 1609–1615. doi: 10.1016/S0031-9422(00)82892-7
Kimbrough, J. M., Salinas-Mondragon, R., Boss, W. F., Brown, C. S., and Sederoff, H. W. (2004). The fast and transient transcriptional network of gravity and mechanical stimulation in the Arabidopsis root apex. Plant Physiol. 136, 2790–2805. doi: 10.1104/pp.104.044594
Lefebvre, B., Furt, F., Hartmann, M. -A., Michaelson, L. V., Carde, J. -P., Sargueil-Boiron, F., et al. (2007). Characterization of lipid rafts from Medicago truncatula root plasma membranes: a proteomic study reveals the presence of a raft-associated redox system. Plant Physiol. 144, 402–418. doi: 10.1104/pp.106.094102
Lindsey, K., Pullen, M. L., and Topping, J. F. (2003). Importance of plant sterols in pattern formation and hormone signalling. Trends Plant Sci. 8, 521–525. doi: 10.1016/j.tplants.2003.09.012
Lingwood, D., and Simons, K. (2010). Lipid rafts as a membrane-organizing principle. Science 327, 46–50. doi: 10.1126/science.1174621
Lung, S. -C., Liao, P., Yeung, E. C., Hsiao, A. -S., Xue, Y., and Chye, M. -L. (2017). Acyl-CoA-binding protein ACBP1 modulates sterol synthesis during embryogenesis. Plant Physiol. 174, 1420–1435. doi: 10.1104/pp.17.00412
Magdy, M., Mansour, F., Hasselt, P. R., and Kuiper, P. J. C. (1994). Plasma membrane lipid alterations induced by NaCl in winter wheat roots. Physiol. Plant. 92, 473–478. doi: 10.1111/j.1399-3054.1994.tb08838.x
Marigo, V., and Tabin, C. J. (1996). Regulation of patched by sonic hedgehog in the developing neural tube. Proc. Natl. Acad. Sci. USA 93, 9346–9351. doi: 10.1073/pnas.93.18.9346
Masucci, J. D., Rerie, W. G., Foreman, D. R., Zhang, M., Galway, M. E., Marks, M. D., et al. (1996). The homeobox gene GLABRA2 is required for position-dependent cell differentiation in the root epidermis of Arabidopsis thaliana. Development 122, 1253–1260.
Mayer, U., Torres Ruiz, R. A., Berleth, T., Misera, S., and Jurgens, G. (1991). Mutations affecting body organization in the Arabidopsis embryo. Nature 353, 402–407. doi: 10.1038/353402a0
Men, S., Boutte, Y., Ikeda, Y., Li, X., Palme, K., Stierhof, Y. -D., et al. (2008). Sterol-dependent endocytosis mediates post-cytokinetic acquisition of PIN2 auxin efflux carrier polarity. Nat. Cell Biol. 10, 237–244. doi: 10.1038/ncb1686
Monshausen, G. B., Miller, N. D., Murphy, A. S., and Gilroy, S. (2011). Dynamics of auxin-dependent Ca2+ and pH signaling in root growth revealed by integrating high-resolution imaging with automated computer vision-based analysis. Plant J. 65, 309–318. doi: 10.1111/j.1365-313X.2010.04423.x
Moore, I. (2002). Gravitropism: lateral thinking in auxin transport. Curr. Biol. 12, R452–R454. doi: 10.1016/S0960-9822(02)00943-0
Morikawa, T., Mizutani, M., Aoki, N., Watanabe, B., Saga, H., Saito, S., et al. (2006). Cytochrome P450 CYP710A encodes the sterol C-22 desaturase in Arabidopsis and tomato. Plant Cell 18, 1008–1022. doi: 10.1105/tpc.105.037012
Morikawa, T., Saga, H., Hashizume, H., and Ohta, D. (2009). CYP710A genes encoding sterol C22-desaturase in Physcomitrella patens as molecular evidence for the evolutionary conservation of a sterol biosynthetic pathway in plants. Planta 229, 1311–1322. doi: 10.1007/s00425-009-0916-4
Muramatsu, Y., Harada, A., Ohwaki, Y., Kasahara, Y., Takagi, S., and Fukuhara, T. (2002). Salt-tolerant ATPase activity in the plasma membrane of the marine angiosperm Zostera marina L. Plant Cell Physiol. 43, 1137–1145. doi: 10.1093/pcp/pcf139
Nes, W., and Venkatramesh, M. (1999). Enzymology of phytosterol transformations. Crit. Rev. Biochem. Mol. Biol. 34, 81–93. doi: 10.1080/10409239991209219
Niu, X., Bressan, R. A., Hasegawa, P. M., and Pardo, J. M. (1995). Ion homeostasis in NaCl stress environments. Plant Physiol. 109, 735–742. doi: 10.1104/pp.109.3.735
Noguchi, T., Fujioka, S., Takatsuto, S., Sakurai, A., Yoshida, S., Li, J., et al. (1999). Arabidopsis det2 is defective in the conversion of (24R)-24-methylcholest-4-En-3-one to (24R)-24-Methyl-5α-cholestan-3-one in brassinosteroid biosynthesis. Plant Physiol. 120, 833–840. doi: 10.1104/pp.120.3.833
Nohturfft, A., and Losick, R. (2002). Cell biology: fats, flies, and palmitate. Science 296, 857–858. doi: 10.1126/science.1072154
Nomura, T., Takatsuto, S., Reid, J. B., and Yokota, T. (1999). Brassinosteroid/sterol synthesis and plant growth as affected by Ika and Ikb mutations of pea. Plant Physiol. 119, 1517–1526. doi: 10.1104/pp.119.4.1517
Peng, L., Kawagoe, Y., Hogan, P., and Delmer, D. (2002). Sitosterol-β-glucoside as primer for cellulose synthesis in plants. Science 295, 147–150. doi: 10.1126/science.1064281
Pereira-Netto, A. B. (2012). Stigmasterol-driven enhancement of the in vitro multiplication rate for the marubakaido apple rootstock. Trees 26, 581–586. doi: 10.1007/s00468-011-0621-3
Pilar, C., Antonio, O., and Antonio, C. (1993). Effects of saline stress and calcium on lipid composition in bean roots. Phytochemistry 32, 1131–1136. doi: 10.1016/S0031-9422(00)95077-5
Pook, V. G., Nair, M., Ryu, K., Arpin, J. C., Schiefelbein, J., Schrick, K., et al. (2017). Positioning of the SCRAMBLED receptor requires UDP-Glc:sterol glucosyltransferase 80B1 in Arabidopsis roots. Sci. Rep. 7:5714. doi: 10.1038/s41598-017-05925-6
Qiu, Q. S., Barkla, B. J., Vera-Estrella, R., Zhu, J. K., and Schumaker, K. S. (2003). Na+/H+ exchange activity in the plasma membrane of Arabidopsis. Plant Physiol. 132, 1041–1052. doi: 10.1104/pp.102.010421
Roche, Y., Gerbeau-Pissot, P., Buhot, B., Thomas, D., Bonneau, L., Gresti, J., et al. (2008). Depletion of phytosterols from the plant plasma membrane provides evidence for disruption of lipid rafts. FASEB J. 22, 3980–3991. doi: 10.1096/fj.08-111070
Schaeffer, A., Bronner, R., Benveniste, P., and Schaller, H. (2001). The ratio of campesterol to sitosterol that modulates growth in Arabidopsis is controlled by STEROL METHYLTRANSFERASE 2;1. Plant J. 25, 605–615. doi: 10.1046/j.1365-313x.2001.00994.x
Schaller, H. (2003). The role of sterols in plant growth and development. Prog. Lipid Res. 42, 163–175. doi: 10.1016/S0163-7827(02)00047-4
Schaller, H., Bouvier-Nave, P., and Benveniste, P. (1998). Overexpression of an Arabidopsis cDNA encoding a sterol-C24(1)-methyltransferase in tobacco modifies the ratio of 24-methyl cholesterol to sitosterol and is associated with growth reduction. Plant Physiol. 118, 461–469. doi: 10.1104/pp.118.2.461
Schrick, K., Cordova, C., Li, G., Murray, L., and Fujioka, S. (2011). A dynamic role for sterols in embryogenesis of Pisum sativum. Phytochemistry 72, 465–475. doi: 10.1016/j.phytochem.2011.01.009
Schrick, K., Debolt, S., and Bulone, V. (2012). Deciphering the molecular functions of sterols in cellulose biosynthesis. Front. Plant Sci. 3:84. doi: 10.3389/fpls.2012.00084
Schrick, K., Mayer, U., Horrichs, A., Kuhnt, C., Bellini, C., Dangl, J., et al. (2000). FACKEL is a sterol C-14 reductase required for organized cell division and expansion in Arabidopsis embryogenesis. Genes Dev. 14, 1471–1484. doi: 10.1101/gad.14.12.1471
Schrick, K., Nguyen, D., Karlowski, W. M., and Mayer, K. F. (2004). START lipid/sterol-binding domains are amplified in plants and are predominantly associated with homeodomain transcription factors. Genome Biol. 5:R41. doi: 10.1186/gb-2004-5-6-r41
Senthil-Kumar, M., Wang, K., and Mysore, K. S. (2013). AtCYP710A1 gene-mediated stigmasterol production plays a role in imparting temperature stress tolerance in Arabidopsis thaliana. Plant Signal. Behav. 8:e23142. doi: 10.4161/psb.23142
Sewelam, N., Jaspert, N., Van Der Kelen, K., Tognetti, V. B., Schmitz, J., Frerigmann, H., et al. (2014). Spatial H2O2 signaling specificity: H2O2 from chloroplasts and peroxisomes modulates the plant transcriptome differentially. Mol. Plant 7, 1191–1120. doi: 10.1093/mp/ssu070
Shabala, S., Shabala, L., and Volkenburgh, E. V. (2003). Effect of calcium on root development and root ion fluxes in salinised barley seedlings. Funct. Plant Biol. 30, 507–514. doi: 10.1071/FP03016
Shen, H., Chen, J., Wang, Z., Yang, C., Sasaki, T., Yamamoto, Y., et al. (2006). Root plasma membrane H+-ATPase is involved in the adaptation of soybean to phosphorus starvation. J. Exp. Bot. 57, 1353–1362. doi: 10.1093/jxb/erj111
Sonawane, P. D., Pollier, J., Panda, S., Szymanski, J., Massalha, H., Yona, M., et al. (2016). Plant cholesterol biosynthetic pathway overlaps with phytosterol metabolism. Nat. Plants 3:16205. doi: 10.1038/nplants.2016.205
Souter, M., Topping, J., Pullen, M., Friml, J., Palme, K., Hackett, R., et al. (2002). hydra Mutants of Arabidopsis are defective in sterol profiles and auxin and ethylene signaling. Plant Cell 14, 1017–1031. doi: 10.1105/tpc.001248
Stalleart, V. M., and Geuns, J. M. C. (1994). Phospholipid and free sterol composition of hypocotyl plasma membranes of ageing mung bean seedlings. Phytochemistry 36, 1177–1180. doi: 10.1016/S0031-9422(00)89633-8
Stermer, B. A., Bianchini, G. M., and Korth, K. L. (1994). Regulation of HMG-CoA reductase activity in plants. J. Lipid Res. 35, 1133–1140.
Suza, W. P., and Chappell, J. (2016). Spatial and temporal regulation of sterol biosynthesis in Nicotiana benthamiana. Physiol. Plant. 157, 120–134. doi: 10.1111/ppl.12413
Wang, K., Senthil-Kumar, M., Ryu, C. -M., Kang, L., and Mysore, K. S. (2012). Phytosterols play a key role in plant innate immunity against bacterial pathogens by regulating nutrient efflux into the apoplast. Plant Physiol. 158, 1789–1802. doi: 10.1104/pp.111.189217
Whitaker, B. D. (1991). Changes in lipids of tomato fruit stored at chilling and non-chilling temperatures. Phytochemistry 30, 757–761. doi: 10.1016/0031-9422(91)85247-W
Whitaker, B. D., and Gapper, N. (2008). Ripening-specific stigmasterol increase in tomato fruit is associated with increased sterol C-22 desaturase (CYP710A11) gene expression. J. Agric. Food Chem. 56, 3828–3835. doi: 10.1021/jf7037983
Willemsen, V., Friml, J., Grebe, M., van den Toorn, A., Palme, K., and Scheres, B. (2003). Cell polarity and PIN protein positioning in Arabidopsis require STEROL METHYLTRANSFERASE1 function. Plant Cell 15, 612–625. doi: 10.1105/tpc.008433
Yan, F., Feuerle, R., Schaffer, S., Fortmeier, H., and Schubert, S. (1998). Adaptation of active proton pumping and plasmalemma ATPase activity of corn roots to low root medium pH. Plant Physiol. 117, 311–319. doi: 10.1104/pp.117.1.311
Keywords: stigmasterol, sterol end products, cell signaling, physiology, abiotic, sterol pathway regulation
Citation: Aboobucker SI and Suza WP (2019) Why Do Plants Convert Sitosterol to Stigmasterol? Front. Plant Sci. 10:354. doi: 10.3389/fpls.2019.00354
Edited by:
John Hancock, University of the West of England, United KingdomReviewed by:
Albert Ferrer, University of Barcelona, SpainSébastien Mongrand, Centre National de la Recherche Scientifique (CNRS), France
Copyright © 2019 Aboobucker and Suza. This is an open-access article distributed under the terms of the Creative Commons Attribution License (CC BY). The use, distribution or reproduction in other forums is permitted, provided the original author(s) and the copyright owner(s) are credited and that the original publication in this journal is cited, in accordance with accepted academic practice. No use, distribution or reproduction is permitted which does not comply with these terms.
*Correspondence: Walter P. Suza, d3BzdXphQGlhc3RhdGUuZWR1