- 1Plant Physiology, Institute of Biology, Humboldt University of Berlin, Berlin, Germany
- 2Leibniz-Institute of Vegetable and Ornamental Crops, Großbeeren, Germany
- 3Erfurt Research Centre for Horticultural Crops, University of Applied Sciences Erfurt, Erfurt, Germany
Together with several proteins involved in brassinosteroid (BR) signaling and synthesis, the membrane steroid binding protein 1 (MSBP1) was identified within the interactome of the sucrose transporter of tomato (SlSUT2). We asked whether MSBP1 is also involved in BR signaling as assumed for the AtMSBP1 protein from Arabidopsis and whether it impacts root colonization with arbuscular mycorrhizal (AM) fungi in a similar way as shown previously for SlSUT2. In addition, we asked whether brassinosteroids per se affect efficiency of root colonization by AM fungi. We carried out a set of experiments with transgenic tobacco plants with increased and decreased MSBP1 expression levels. We investigated the plant and the mycorrhizal phenotype of these transgenic plants and tested the involvement of MSBP1 in BR metabolism by application of epi-brassinolide and brassinazole, an inhibitor of BR biosynthesis. We show that the phenotype of the transgenic tobacco plants with increased or reduced MSBP1 expression is consistent with an inhibitory role of MSBP1 in BR signaling. MSBP1 overexpression could be mimicked by brassinazole treatment. Interestingly, manipulation of MSBP1 expression in transgenic tobacco plants not only affected plant growth and development, but also the host plant responses toward colonization with AM fungi, as well as arbuscular architecture. Moreover, we observed that brassinosteroids indeed have a direct impact on the nutrient exchange in AM symbiosis and on the biomass production of colonized host plants. Furthermore, arbuscular morphology is affected by changes in MSBP1 expression and brassinolide or brassinazole treatments. We conclude that host plant growth responses and nutrient exchange within the symbiosis with AM fungi is controlled by brassinosteroids and might be impeded by the MSBP1 protein.
Highlights
- Behavior of transgenic tobacco plants with up- or down-regulated MSBP1 expression is consistent with an inhibitory role of MSBP1 in BR signaling.
- We show for the first time that brassinosteroids per se positively affect the symbiosis of tobacco and tomato plants with an arbuscular mycorrhizal fungus.
- MSBP1 expression, and brassinolide treatment affects arbuscular morphology as well as host plant growth responses.
Introduction
Brassinosteroids are phytohormones involved in cell elongation and expansion, in pollen and stamen development (Clouse and Sasse, 1998; Zhu et al., 2015), as well as in fruit ripening (Symons et al., 2006). The physiological role of brassinosteroids (BRs) in plant development, formation of the vascular system and pollen maturation has been investigated intensively during the past few years. In addition, a role in plant defense against pathogens could be shown (Zhang et al., 2015). However, it is still unclear whether the inhibitory role of BRs in defense responses against plant pathogens is also effective in other biotic interactions such as mycorrhizal symbioses. Epi-brassinolide treatment in wheat and rice has slight synergistic effects on mycorrhization under salt stress conditions (Tofighi et al., 2017). Tomato dx mutants defective in BR biosynthesis showed decreased mycorrhization (Bitterlich et al., 2014a,b). BR-deficient lkb pea mutants, however, did not show changes in root colonization (Foo et al., 2013) suggesting species-dependent differences.
We recently showed that the tomato sucrose transporter SlSUT2 directly interacts with membrane proteins involved in BR synthesis and signaling. These interaction partners were the sterol reductase DWARF1/DIMINUTO (DIM1) involved in BR synthesis, the BR co-receptor kinase BAK1, and the less well characterized membrane-steroid binding protein 1 (MSBP1) (Bitterlich et al., 2014b). Interaction between BAK1 and MSBP1 has been already described in Arabidopsis (Song et al., 2009) and bimolecular fluorescence complementation (BiFC) as well as the yeast two-hybrid split ubiquitin system (SUS) were able to confirm this interaction also for the two tomato paralogs interacting with the sucrose transporter SlSUT2 (Bitterlich et al., 2014b). Whether all three proteins are able to form a trimeric complex or whether they compete with each other for binding, is unknown. It has been suggested that the interaction between BAK1 and MSBP1 inhibits activation of the BR receptor BRI1 by BAK1 (Song et al., 2009).
MSBP1 and the BAK1 protein were identified as the two strongest interaction partners of SlSUT2. Inhibition of SlSUT2 expression in transgenic tomato plants leads to delayed fruit development, reduced pollen germination rates and inhibited pollen tube growth (Hackel et al., 2006), whereas mycorrhizal colonization of SlSUT2-antisense plants is significantly increased (Bitterlich et al., 2014b). Apart from mycorrhization intensity, the phenotype of SlSUT2-inhibited plants resembles BR deficient mutant plants.
BAK1 is well characterized and the promiscuous leucine rich repeat (LRR) receptor kinase interacts with BRI1 in BR signaling and with other receptor kinases in plant immune responses and cell death pathway (Belkhadir et al., 2012; Segonzac et al., 2014).
The role of MSBP1 is, however, only partially understood. In Arabidopsis, MSBP1 binds to progesterone, stigmasterol and brassinosteroid with low affinity (Yang et al., 2005) and is thought to inhibit BR signaling by removing BAK1 from the plasma membrane by internalization as heteromeric complexes (Song et al., 2009). In Arabidopsis, MSBP1 is light induced (Shi et al., 2011) and involved in vesicle trafficking and auxin redistribution (Yang et al., 2008). MSBP1 inhibits cell elongation (Yang et al., 2005), potentially via its inhibitory effect on BR signaling. A recent publication describes MSBP1 to be important for salinity tolerance in yeast cells, and in Arabidopsis and barley (Witzel et al., 2018). In barley, MSBP1 abundance correlates with adaptation of root architecture in response to salinity, potentially via stimulation of auxin response through PIN2 homologs and enhancement of BR receptor endocytosis, thereby reducing BR signaling (Witzel et al., 2018). A critical role of MSBP1 is postulated for mycorrhiza development in Medicago roots after its induction by a diffusible signal emitted by the fungus (Kuhn et al., 2010). Thus, the MSBP1 protein has multiple functions and is still not fully understood in detail.
To further elucidate the impact of MSBP1 on plant development, its relation to brassinosteroid signaling and its role in the mycorrhizal symbiosis, we analyzed the development of tobacco plants with increased or decreased MSBP1-expression. For overexpression, we used the homologous gene of tomato in order to avoid any down-regulation of RNA accumulation due to co-suppression. Secondly, we conducted a detailed study of the arbuscular mycorrhizal (AM) phenotype in these transgenic plants including an analysis of arbuscule morphology. In order to test the hypothesis that MSBP1 is involved in BR signaling, we studied the effects of treatments with the synthetic BR epi-brassinolide and the BR inhibitor brassinazole on the plant and AM phenotype.
Materials and Methods
Generation of Constructs
The open reading frame of the MSBP1-like protein from tomato (XP_004240738) comprises 723 bp and encodes a 240 amino acids protein (Supplementary Figure S1). A 127 bp XbaI-BglII fragment was used for cloning into the pUC-RNAi vector (Chen et al., 2003) in sense and antisense orientation. The whole 454 bp fragment including the intron sequence was excised with PstI and transferred into the binary vector pBinAR Kan linearized with SbfI. The construct was checked by sequencing. Sequence similarity between the tomato (XM_004240690) and the tobacco ortholog (XM_016639423) within the XbaI-BglII fragment is 91% identity.
The full length cDNA of MSBP1 (XP_004240738) was amplified via PCR from reversely transcribed RNA isolated from tomato leaves or flowers using primers carrying KpnI- and BamHI restriction sites (Supplementary Table S1). Ligation into the binary vector was carried out via KpnI-BamHI in sense orientation followed by sequence analysis.
Transformation of Nicotiana tabacum via Agrobacterium tumefaciens
Gene transfer into plants (Nicotiana tabacum var. Samsun SNN) was performed with Agrobacterium tumefaciens (strain C58C1, pGV2260; Deblaere et al., 1985). Regenerated plants were screened by PCR for integration of the construct using NPTII primers (Supplementary Table S1). Seeds of plants containing integrated DNA were selected in tissue culture on selective media with the appropriate antibiotics and placed in the greenhouse for further analysis.
Plant Growth
Tobacco and tomato (Solanum lycopersicum cv. Moneymaker) plants were grown in the greenhouse with a cycle of 16 h light (22°C) and 8 h darkness (15°C). The mean photosynthetic photon flux density was about 150 μmol photons m-2 s-1 and additional illumination was provided by high-pressure sodium lamps SON-T Green Power and metal halide lamps MASTER LPI-T Plus (Philips Belgium, Brussels).
Epi-Brassinolide and Brassinazole Treatments
Epibrassinolide (epi-BL; ≤85%. Sigma-Aldrich) and brassinazole (BRZ; ≤98% (HPLC), Sigma-Aldrich) were dissolved in DMSO and freshly diluted in water to a final concentration of 1 μM (Asami et al., 2000). Both chemicals were applied to the root system of tobacco plants starting 1 week after inoculation. Fifty milliliter of 1 μM BRZ was applied every other day, whereas control (supplemented with the same amount of DMSO) and epi-BL was applied weekly over a total period of 4 weeks.
Mycorrhization Experiments
For mycorrhization experiments, seedlings of tobacco and tomato wild type plants and the corresponding transgenic lines were planted in 3 L pots with a sand-vermiculite mixture (1:1; agra-Vermiculite, RHP, TX Rhenen, the Netherlands; quarry sand grain size: 0.2–1.0 mm, Euroquarz, Ottendorf-Okrilla, Germany). Half of the pots were inoculated with Rhizoglomus irregularis QS 81 (INOQ, Schnega, Germany) in a ratio of 1:10 inoculusubstrate. The control plants were grown in the same substrate without inoculum. The plants were grown in the greenhouse in a randomized design and watered every day with 200–400 ml of nutrient solution (De Kreji et al., 1997), containing 10% of the standard phosphate concentration (0.1 mM). Plants were harvested after 6 weeks if not indicated otherwise. Samples of roots and leaves were taken in liquid nitrogen and stored at –80°C. For determination of dry mass, plants were dried for 4 days at 65°C. Approximately 1 g of each fresh root system was stored in 20% EtOH for later analysis of fungal colonization.
The root samples were stained with trypan blue after (Koske and Gemma, 1989). Scoring of colonization patterns was determined after the method of Trouvelot et al. (1986) and calculations were carried out with the MYCOCALC software1. Quantification of fungal vesicles was carried out according to the classification for arbuscule abundance described in Trouvelot et al. (1986) Alternatively, roots were stained with wheat germ agglutinin (WGA). WGA-FITC (Sigma-Aldrich, St. Louis, MO, United States) staining was performed over night at a concentration of 10 μg ml-1 in PBS after clearing to the roots for 2 h at 50°C in 10% KOH as described by Banhara et al. (2015). Root samples were imaged with TCS-SP2 (Leica, Bensheim, Germany) or LSM800 (Zeiss, Jena, Germany) confocal microscopes with excitation at 488 nm for WGA-FITC and detection at 500–540 nm. Tubule diameter was quantified from 20 to 80 tubules per arbuscule and 10–15 arbuscules per genotype or treatment using the Zeiss confocal software blue edition ZEN2.0.
RNA Analysis
RNA extraction was performed with Trisure (Bioline, Luckenwalde, Germany) or peqGold Trifast (Peqlab, Erlangen, Germany) according to the manufacturer’s protocol. Reverse transcription was performed with the Qiagen Omniscript RT Kit according to the manual (Qiagen, Hilden, Germany). Oligo(dT) primers served for the initial reverse transcription reaction on approximately 1 μg of total RNA after digestion with RNase-free DNase (Qiagen).
Aliquots of 0.2 μl of the 10 μl RT-reaction were used for the subsequent PCR reaction in the presence of SYBR Green with HotGoldStar DNA-Polymerase (Eurogentec, Seraing, Belgium) in a BioRad Cycler using the CFX System software based on the following progra denaturation at 95°C for 30 s, annealing for 30 s at 61°C and elongation for 30 s at 72°C, in a program of 45 cycles in 10 μl reaction volume. Primers (sequences in Supplementary Table S1) were designed to obtain a 50–150 bp amplicon with the help of the Primer3 software2. Melting curve analysis revealed amplification of one single amplicon, respectively. RNA accumulation analyses were performed with 2–3 technical replicates and 3–5 biological replicates for each measurement. Normalized expression levels were calculated with ubiquitin, tubulin and EF1α as reference genes (Supplementary Table S1) based on the following formula:
With RQ = relative quantity of a sample; Ref = reference target; GOI = gene of interest; n = number of reference targets. The average expression stability (M-value) of tubulin and ubiquitin references was 0.3955.
Vascular Staining
Thin hand-cut sections were first incubated in safranin solution (1 g safranin diluted in 100 ml de-ionized water), de-stained with ethanol-acetic acid (100 ml 70% ethanol + 0.5 ml HCl) and subsequently stained with astrablue (0.5 g astrablue (Omikron, Reitberg) in 100 ml de-ionized water).
Element Analysis via ICP-OE Spectrometry
For the analysis via ICP-OES (inductively coupled plasma optical emission spectrometry), the samples were completely dried at 80°C and pestled. 0.5 g sample were mixed with 5 ml 65% HNO3 and 3 ml 30% H2O2. The digestion in the microvave MarsXpress (CEM Corporation) and subsequent steps were carried out according to the instructions of the Gemeinschaftslabor Analytik (GLA) des Albrecht-Daniel-Thaer-Instituts für Agrar – und Gartenbauwissenschaften der HU Berlin.
The ICP-OES was performed with the iCAP 6300 Duo MFC (Thermo Fisher Scientific) by Dr. Kirsten Weiss (GLA). The analyzed elements were Ca (317.9 nm), Fe (259.9 nm), K (766.4 nm), Mn (257.6 nm), Zn (213.8 nm), and P (213.6 nm).
Sterol Analysis of Microsomal Membranes
The isolation of the microsomal fraction was performed according to Larsson (1985). Fifty gram leaves of 6 weeks old tobacco plants were used for the experiment. Isolated microsomal fractions were stored at -20°C. The chloroform-methanol-extraction was performed according to the following protocol for phase separation. One volume of the sample was mixed with two volumes of chloroform/methanol (2:1), mixed properly and centrifuged 5 min at 2000 rpm at room temperature. The lower phase (containing the lipids) was collected and the rest was re-extracted as before. Both lower phases were pooled, stored at room temperature and shipped to the University Bonn. The subsequent steps were undertaken by the lipidomics platform3 headed by Peter Dörmann as described in Wewer et al. (2011).
Statistics
To test whether the individuals of every single transgenic line differ significantly in their expression levels from the WT, the t-test with a cutoff level of α = 0.05 was used. To investigate whether phenotypical traits differ between plant genotypes and treatments factorial ANOVA was carried in accordance with the experimental design and means were compared by Tukey test as the post hoc procedure (α = 0.05).
Results
MSBP1 Affects Plant Development
The gene SlMSBP1 codes for a 240 amino acid protein with one putative membrane spanning domain at its N-terminal domain and a conserved heme/steroid binding domain between position 71 and 167 (Supplementary Figure S1A). SlMSBP1 contains two conserved tyrosine-based ITAM activation motifs (YXX-aliphatic amino acid) at its C-terminal end, typically found twice at the cytoplasmic tail of cell surface proteins of the immune system (Abbas et al., 2015). In addition, two tyrosine-based sorting signals (YXXφ, φ = bulky, hydrophobic amino acid) are present, known for involvement in vesicle trafficking, endocytosis and cell sorting (Supplementary Figure S1B; Yang et al., 2008). Its topology predicts the N-terminus at the extracellular side of the membrane.
In order to avoid co-suppression during overexpression of the gene SlMSBP1, tobacco was chosen as a close relative of tomato. Transgenic tobacco plants with reduced or increased MSBP1 expression were generated and analyzed regarding size, leaf morphology, xylem proliferation, gene expression, flowering, and sugar accumulation. Quantitative qPCR revealed successful down-regulation of NtMSBP1 expression in RNAi plants and overexpression of SlMSBP1 in overexpressing (OE) lines (Figures 1A,B).
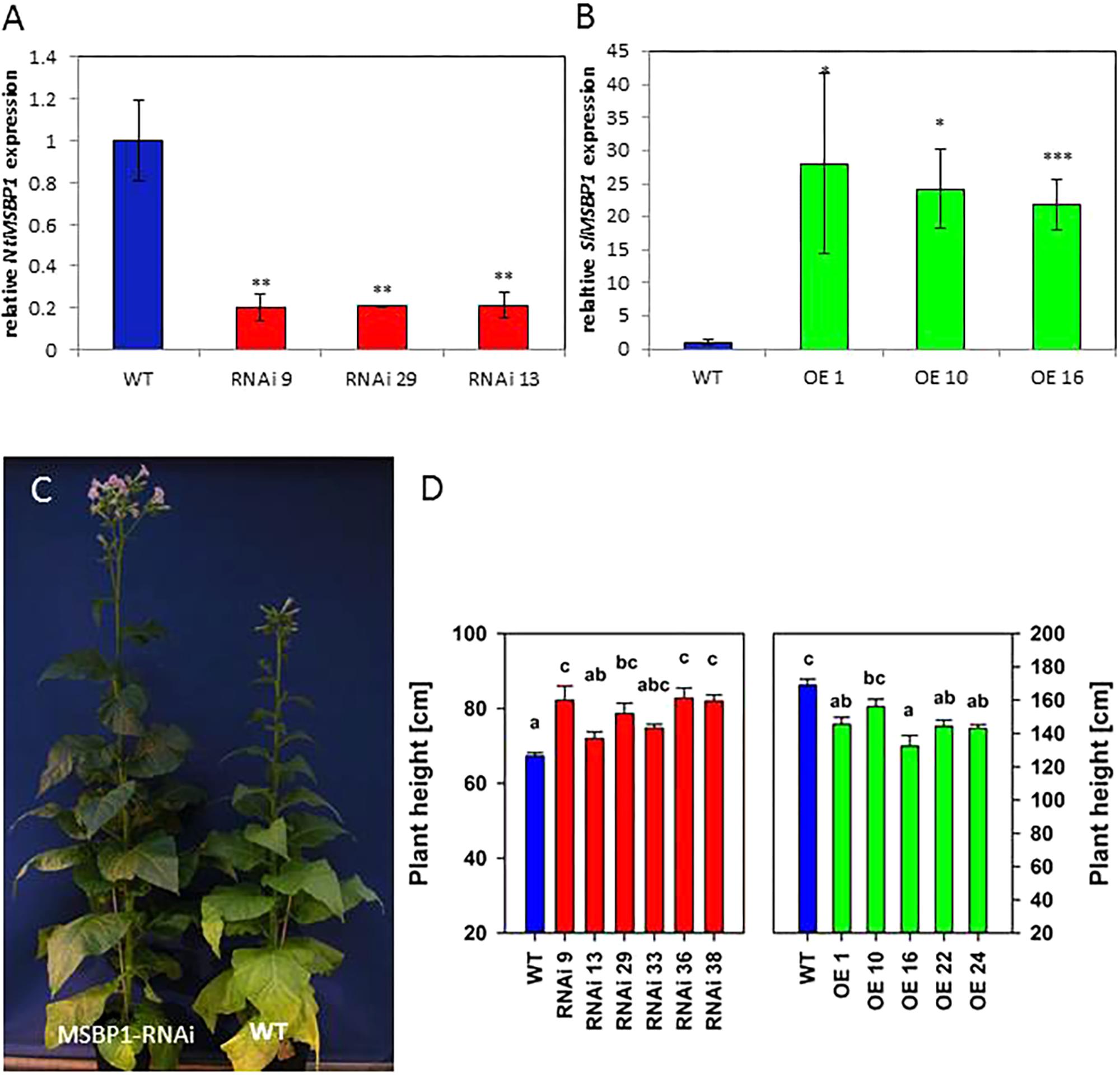
Figure 1. Silencing of NtMSBP1 and overexpression of MSBP1 in transgenic tobacco plants. (A) RNA accumulation analysis in source leaves of NtMSBP1-RNAi lines and wild type plants. NtMSBP1 relative transcript levels were quantified by qPCR using tobacco genes encoding the translation factor EF1-α and ubiquitin for calibration. The relative expression values of RNAi-plants were normalized to the value obtained in WT plants (= 1) with ∗∗P < 0.01. (B) SlMSBP1 expression in MSBP1 overexpressing lines and WT plants. In case of MSBP1-overexpressing plants SlMSBP1-specific primers were used. Mean values and SEM are shown for 3–5 biological replicates (with ∗p < 0.05, ∗∗∗p < 0.001). (C) Tobacco NtMBSP1-RNAi line #9 (left) and wild type plant (right). (D) Heights of NtMBSP1-RNAi lines (left), MSBP1 overexpressing lines (right) and wild type plants. Mean values and standard deviations are shown for 5–8 replicates (transgenic lines) or 16–28 replicates (wild type). Significant differences between values of the RNAi lines and wild type plants are indicated by different letters according to one way ANOVA with post hoc Tukey test and the SE is given. Differences in total plant height in the two experiments are due to differences in pot size and growth conditions.
Transgenic MSBP1-RNAi tobacco were significantly higher than tobacco WT plants (Figures 1C,D), whereas their leaf area (length and width of source leaves) was smaller (Figure 2A). The leaf length/width ratio in NtMSBP1-RNAi plants shifted significantly toward leaf length (Figure 3A) and, the specific leaf area (SLA = m2 kg-1 DW) was decreased (Supplementary Figure S2A). This was accompanied by thicker leaves resulting from a thicker layer of the spongy parenchyma (Figure 2B). Inverse phenotypical traits were observed for the MSBP1-OE lines. Total plant size decreased compared to wild type plants (Figure 1D) and the specific leaf area (SLA) increased (Supplementary Figure S2B) implying the development of thinner leaves.
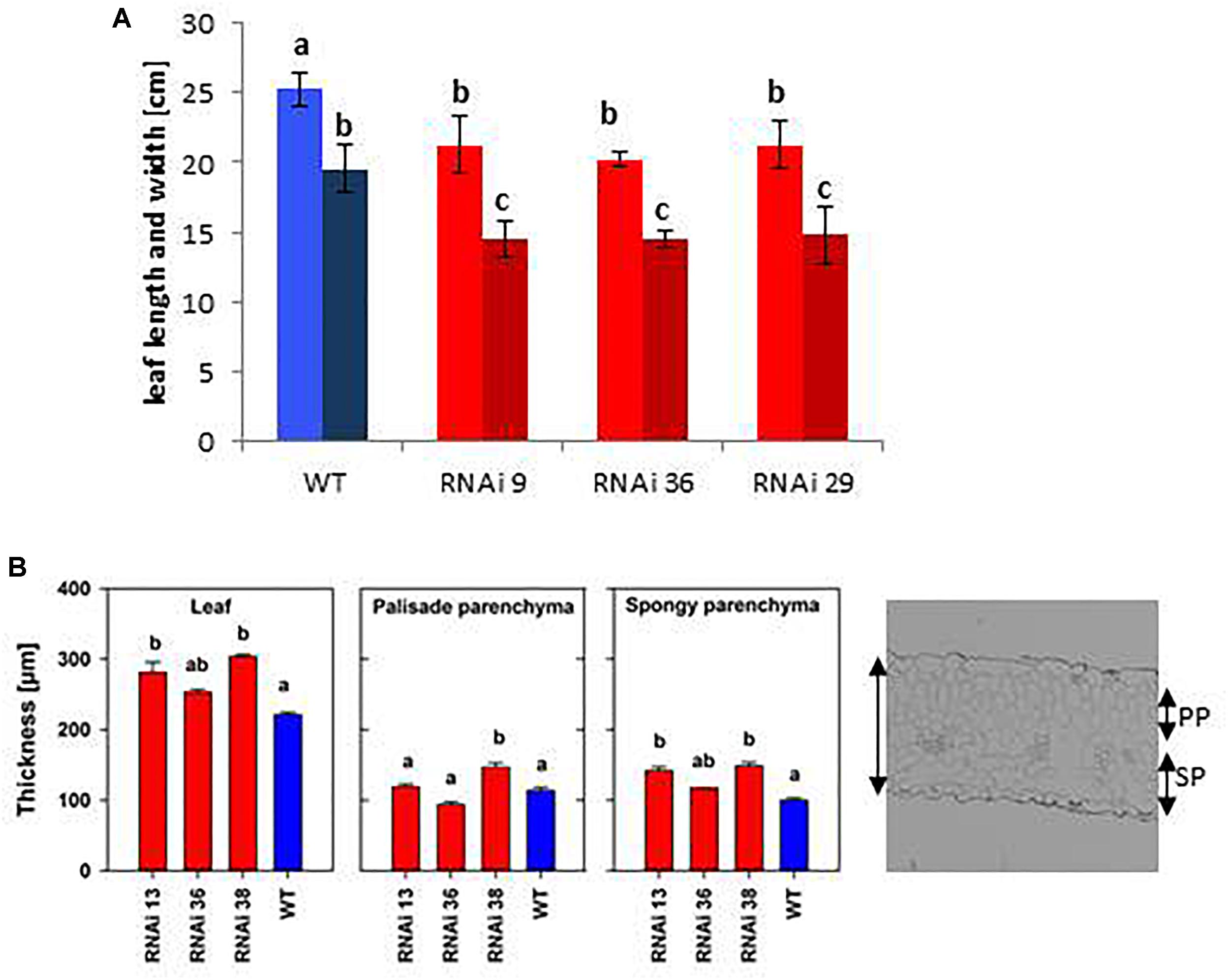
Figure 2. Leaf morphological parameters of NtMSBP1-RNAi tobacco plants. (A) Leaf length (light colored) and width (dark colored) and (B) total thickness of leaves, of palisade (PP) and spongy parenchyma (SP) are shown. Mean values and standard deviations of wild type plants and MSBP1-RNAi lines are presented for 4–5 replicates. Significant differences between values of the RNAi lines and wild type plants are indicated by different letters according to one way ANOVA.
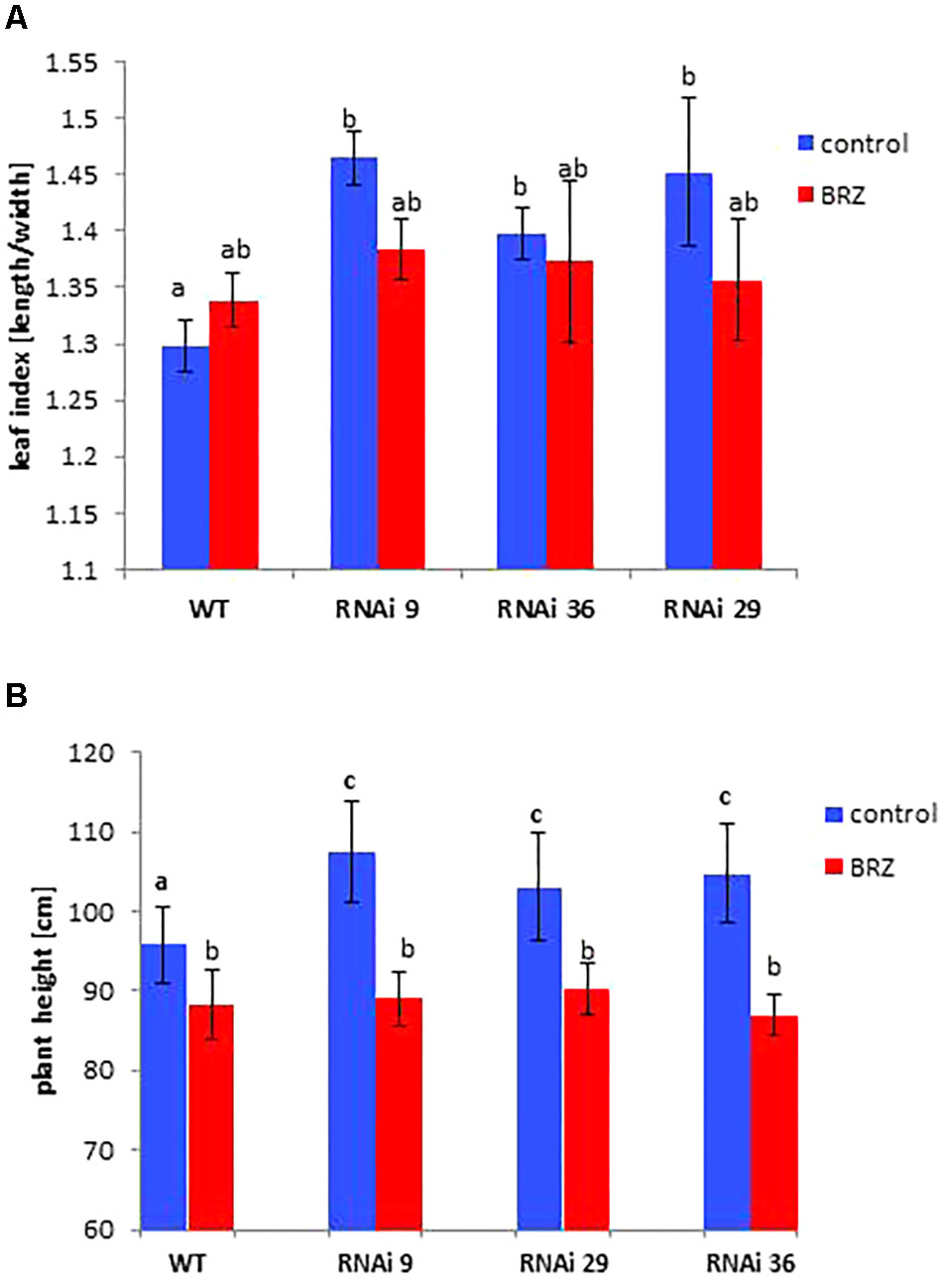
Figure 3. Rescue of the phenotypic changes of NtMSBP1-RNAi tobacco plants. (A) Shown are mean values and standard deviations of the ratios between leaf length and width (leaf index) and of (B) total plant of WT plants and MSBP1-RNAi tobacco lines 4–6 replicates). Leaves were locally treated with 1 μM BRZ or not. Significant differences according to a one-way ANOVA are indicated by different letters (α= 0.05).
MSBP1 as Inhibitor of BR Signaling
In order to test the hypothesis that MSBP1 acts as an inhibitor of brassinosteroid (BR) signaling, tobacco wild type and MSBP1-RNAi plants were sprayed every other day with brassinazole (BRZ), an inhibitor of BR biosynthesis. BRZ treatment diminished the differences in plant height, and leaf index between tobacco WT and NtMSBP1-RNAi plants (Figure 3).
Since brassinosteroids are described to promote Arabidopsis flowering (Li et al., 2010), flowering behavior was investigated in MSBP1-RNAi and OE lines and phenotypes were tried to be rescued by treatments with the synthetic BR epi-brassinolide (epi-BL). BRZ treatment was also included in the experiment. BRZ treatments delayed flowering of wild type plants by several days (Supplementary Figure S3). However, the onset of flowering of MSBP1-OE lines was not responding to the BRZ treatment. In addition, they were rather insensitive to epi-BL (Supplementary Figure S4). In contrast, flowering of MSBP1-RNAi plants was sensitive to epi-BL treatment (Supplementary Figure S5), i.e., they blossomed earlier than wild type plants under similar conditions.
BR deficient Arabidopsis mutants are characterized by xylem under-proliferation (Szekeres et al., 1996), whereas a BRZ treatment inhibits secondary xylem development (Nagata et al., 2001). Consistently, a higher number of xylem vessels in vascular bundles of MSBP1-RNAi plants was visually detected (Supplementary Figure S6), but quantification of the total xylem area in cross-sections revealed no significant differences between tobacco WT and transgenic lines.
MSBP1 Affects Mycorrhiza Functioning
MSBP1 is known for its regulatory function of AM colonization in M. truncatula (Kuhn et al., 2010). In addition, a BR biosynthesis mutant showed reduced colonization by AM fungi (Bitterlich et al., 2014a). Therefore, the role of MSBP1 in the mycorrhizal symbiosis was investigated. Inoculation of MSBP1-RNAi and –OE tobacco lines with the AM fungus Rhizoglomus irregularis was performed. Mycorrhizal parameters were estimated after staining, and the activity of the fungus was quantified by qPCR of fungus-specific marker genes.
Root infection frequency (F), hyphal spread inside the roots (M) and arbuscule abundance (A) were not different in NtMSBP1-RNAi lines compared to the wild type (data not shown). In agreement with these data, the mRNA quantification of the AM fungus-specific marker gene RiGAPDH in colonized roots of NtMSBP1-RNAi plants revealed no significant changes in the transcript amount in most of the transgenic lines (Supplementary Figure S7). Mycorrhizal parameters and fungal activity were also quantified in MSBP1-OE lines. Statistical evaluation of the degree of colonization by staining and of fungal activity as determined by the level of RiGAPDH transcript quantification did not show a significant effects taking all MSBP1-overexpressing lines into account (Supplementary Figure S7).
While a positive effect of mycorrhization on biomass production of wild type plants was observed, this effect disappeared in RNAi lines, and MSBP1-OE lines rather showed a decrease in total biomass production upon mycorrhization (Figure 4 and Supplementary Figure S10A). Regarding internode elongation (Supplementary Figure S10B), the MSBP1-overexpressing plants also do not show a positive response upon mycorrhization and were insensitive toward epi-BL treatment. Differences in the total biomass between inoculated and non-inoculated plants (shown in Figure 4 and Supplementary Figure S10A) depend on the level of MSBP1-transcripts analyzed in the root tissue of the plants from the same experiment (shown in Supplementary Figure S8). MSBP1 overexpression resulted in a decrease in biomass development after mycorrhization of the corresponding lines (Figure 4 and Supplementary Figure S10A).
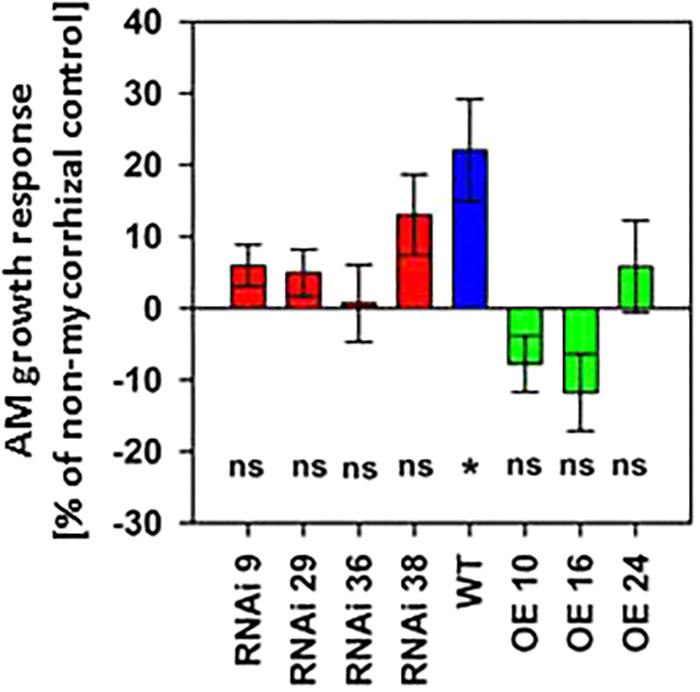
Figure 4. Total biomass production of NtMSBP1-RNAi and MSBP1-overexpressing tobacco lines compared to non-mycorrhizal control plants. Mean values and standard deviations are shown for six replicates of mycorrhizal and non-mycorrhizal wild type plants and of NtMSBP1-RNAi and MSBP1-overexpressing lines. Whether the growth AM response is different from zero was tested by ANOVA (n = 6, P < 0.05).
Further analysis of mycorrhization was performed by staining fungal structures using WGA-FITC conjugate (Figure 5). This analysis revealed differences in arbuscular morphology between roots of wild type plants, MSBP1-RNAi and MSBP1-OE overexpression lines (Figure 5). The diameter of the finest tubular branches of stained arbuscules (for details see schematic drawing in Supplementary Figure S11) was determined by help of the confocal software ZEN 2.0. Compared to the wild type, the tubule diameter was significantly decreased in two MSBP1-overexpressing lines and consistently increased only in RNAi lines (Figures 5C–E). In order to test, if tubular diameter had an impact on the nutrient transfer in mycorrhizal plants, a multi-element analysis was carried out. This revealed that the concentration of phosphorus, iron and zinc is increased only in MSBP1- transgenic plants in response to mycorrhization, whereas WT showed lower amounts (Supplementary Figure S12). Regarding the accumulation of mineral elements such as manganese, and calcium in the above-ground parts of mycorrhizal plants, only MSBP1-RNAi plants show higher contents than non-mycorrhizal control plants and significantly higher content of phosphate, iron, manganese and calcium than mycorrhizal WT plants (Supplementary Figure S12). Thus, MBSP1-silencing seems to improve plant nutrition via the mycorrhizal pathway.
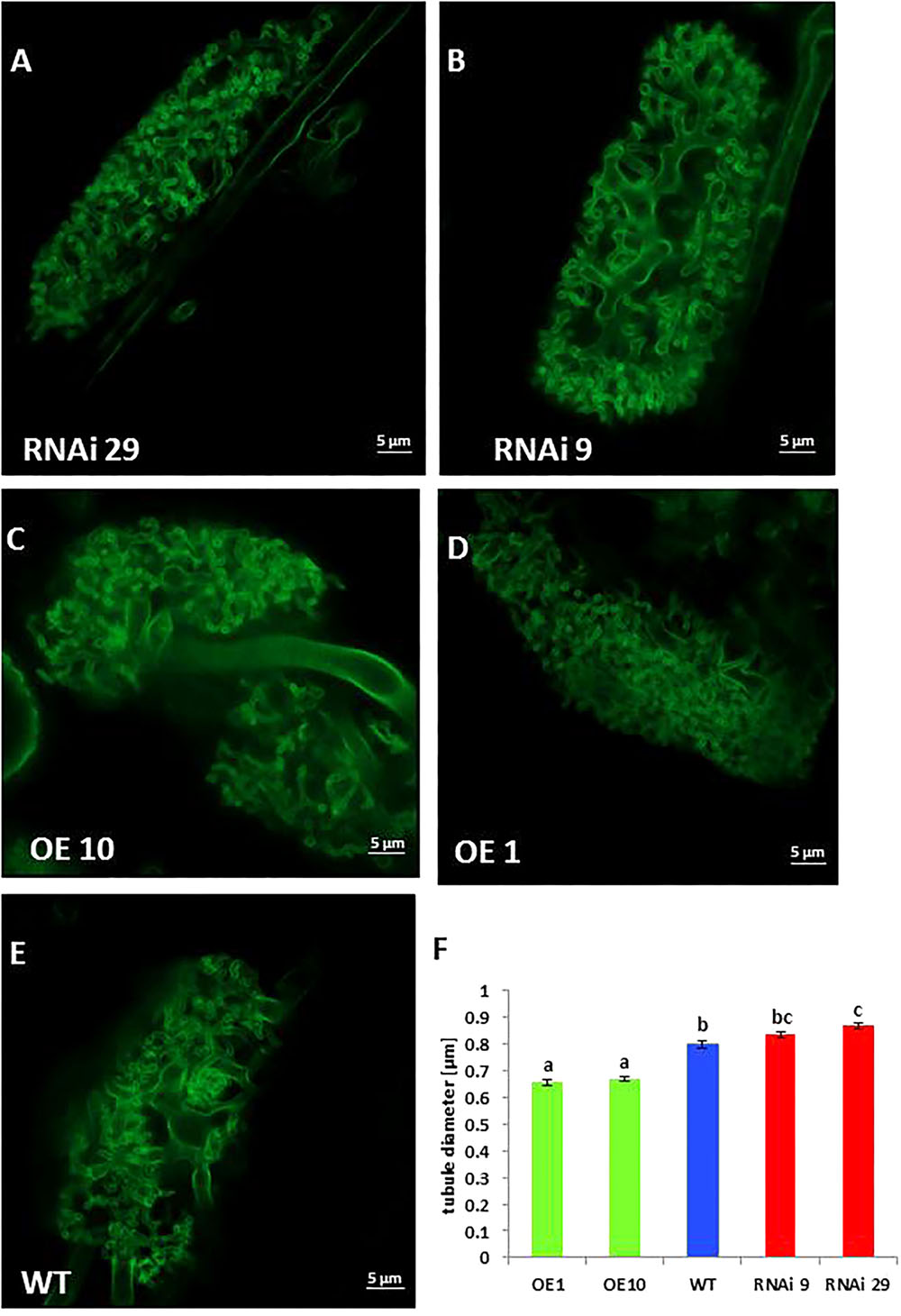
Figure 5. Arbuscule morphology of NtMSBP1-RNAi (A,B), MSBP1-overexpressing (C,D) or wild type (E) tobacco lines. Roots were stained with WGA-FITC and analyzed by confocal microscopy. Measurement of tubule diameters was performed using the ZEN2.0 confocal software (F). Mean values and standard deviations of at least 20 different tubules from 10 different arbuscules for each genotype are shown and the SEM is given. Significant differences according to a one-way ANOVA are indicated by different letters with P < 0.001. Scale bars are 5 μm in length.
Regarding the membrane sterol content of roots, the amount of cholesterol incorporated into membranes is slightly decreased in both, MSBP1-silenced as well as MSBP1-overexpressing plants (Supplementary Figure S13). Thus it is unlikely that the sterol mass fraction is responsible for altered arbuscular architecture.
Effects of BRs on Mycorrhizal Tobacco and Tomato WT Plants
Since expression levels of MSBP1 affect the mycorrhizal symbiosis and MSBP1 is assumed to inhibit BR signaling, it was tested whether similar effects could be achieved using BR or BR inhibitors. Tomato and tobacco WT plants inoculated or not with the AM fungus R. irregularis were treated with epi-BL or BRZ and analyzed. Arbuscules showed the same morphological effect, when BRZ was applied as previously seen in MSBP1-OE lines: arbuscules appear highly branched with a bushy appearance (Figure 6). Quantification of tubule diameter of arbuscular branches revealed significantly smaller values after BRZ treatment than for the untreated control (Figure 6D). Interestingly, epi-BL-treatment resulted in even larger values for arbuscular tubule diameters (Figure 6D).
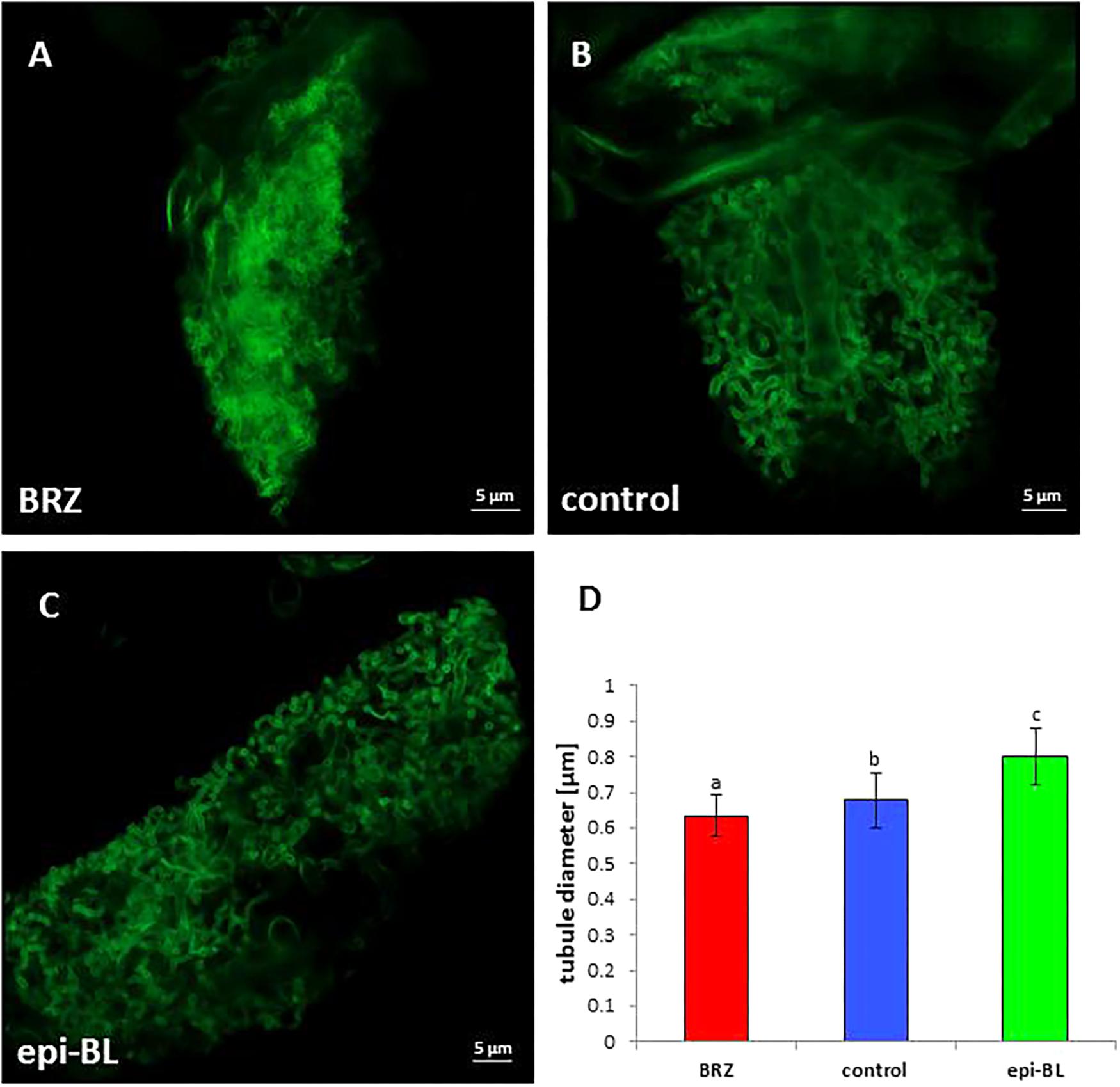
Figure 6. Arbuscule morphology in tobacco wild type roots. Tobacco mycorrhiza treated with brassinazole (A), with DMSO as control (B), or with epi-brassinolide (C). Roots were stained with WGA-FITC and analyzed by CLSM. (D) Tubule diameter estimation determined with the ZEN 2.0 software. Mean values and SE are shown for 20–80 tubular branches from 15 different arbuscule replicates for each treatment. Significant differences between DMSO control and BRZ or epi-BL treatment were calculated by a one-way ANOVA and indicated by different letters (with P < 0.05). Scale bars are 5 μm in length.
Tobacco WT plants treated with epi-BL showed the largest mycorrhiza-induced increases in biomass production in AM plants compared to non-treated or BRZ-treated plants (Figures 7A–C). These results were reproducible in different experiments where plants were harvested either 9 weeks (Figure 7A) or 5 weeks after inoculation with R. irregularis with more prominent differences after 5 weeks (Figures 7B,C). The amount of fungal-specific transcripts for RiGAPDH was also reduced in BRZ-irrigated roots (Figure 7D). Up-regulation of the plant phosphate transporter NtPT4 expression argues for functionality of arbuscular structures in tobacco WT plants (Figure 7D).
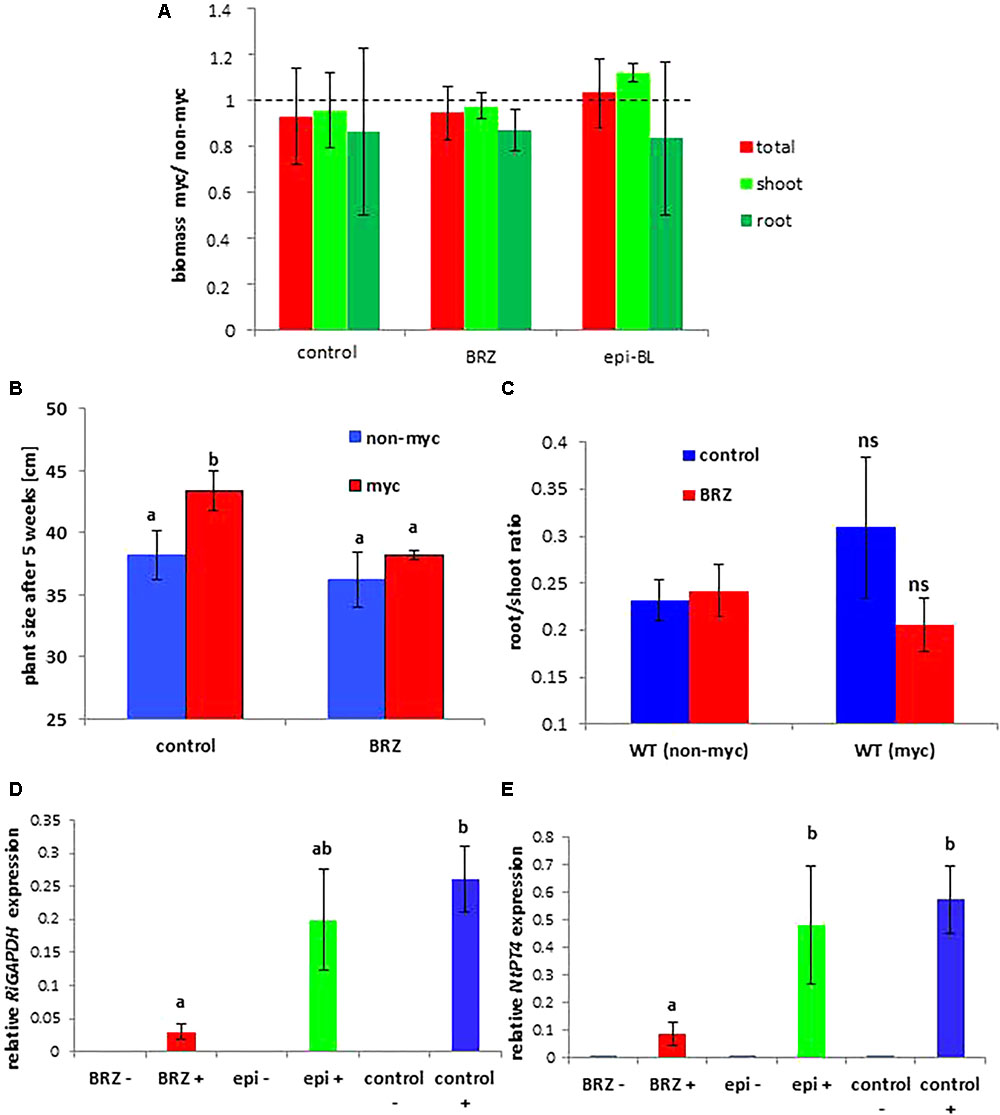
Figure 7. Mycorrhization parameters as influenced by brassinazole (BRZ) and epi-brassinolide (epi-BL) treatment in tobacco WT plants. (A) Shown are mean values and standard deviations of mycorrhiza-induced increase in (A) plant biomass of five replicates of wild type tobacco plants treated or not with brassinazole (BRZ) or with epi-brassinolide (epi-BL). Although biomass parameters do not reveal significant changes, a biomass increase by mycorrhization is only observed in epi-BL treated plants. Tobacco WT plants were harvested after 9 weeks of colonization. (B) Mycorrhiza-induced increase in plant size is only observed in control plants, whereas the gain in biomass by mycorrhization is abolished by BRZ treatment. (C) root/shoot ratios of three replicates WT tobacco plants treated with or without BRZ. Tobacco WT plants in (B,C) were harvested 5 weeks after inoculation with R. irregularis. Results were highly reproducible in different experiments. (D, E) Quantification of fungal RiGAPDH (left) and tobacco NtPT4 (left) transcripts using tubulin as reference genes. Mean values and SEM are shown for three biological and two technical replicates. RNA accumulation values of RiGAPDH in non-mycorrhizal plants were below the detection level and are therefore not shown. One-way ANOVA with α= 0.05 reveals significant differences indicated by different letters.
Tomato WT plants showed a mycorrhiza-induced increase in fresh weights when treated with DMSO (control) or with BRZ (Supplementary Figure S14). In the presence of exogenously applied epi-BL this increase in fresh biomass after mycorrhization was even higher (Supplementary Figure S14A). Quantification of fungal transcripts (RiGAPDH) and of the transcript of the arbuscule specific phosphate transporter gene PT4 confirmed successful root colonization in all cases. Gain in biomass is highest in epi-BL treated plants although the expression of RiGAPDH and SlPT4 is higher in BRZ treated samples (Supplementary Figure S14). Plant growth responses of epi-BL treated mycorrhizal tomato and tobacco WT plants are therefore comparable.
Thus, MSBP1-inhibition in transgenic tobacco plants causes similar effects like epi-BL treatment of tobacco and tomato WT plants in terms of mycorrhization indicating that the effect of MSBP1 on arbuscular morphology might be via its inhibitory function on BR signaling.
Discussion
MSBP1 as Inhibitor of BR Signaling
The first hypothesis of the current study stated that MSBP1 is an inhibitor of brassinosteroid (BR) signaling also in tobacco as previously postulated for AtMSBP1 in Arabidopsis thaliana (Song et al., 2009). This hypothesis was tested with the help of transgenic tobacco plants where it was investigated, if up- or down-regulation of MSBP1 expression affects leaf morphology, plant height and flowering behavior (Figures 1, 2 and Supplementary Figures S3–S5). As previously observed in dim1 mutants defective in BR biosynthesis, leaf thickness is affected also in MSBP1-silenced plants: the leaves of MSBP1-RNAi plants are thicker, leading to a reduced SLA, whereas the opposite can be observed in MSBP1-overexpressors. This is in agreement with a potential role of MSBP1 as an inhibitor of BR signaling in tobacco. Rescue experiments in previous studies were successfully used to restore the dwarfed phenotype of BR-defective mutants (Azpiroz et al., 1998). Therefore, we aimed at restoration of the MSBP1 phenotype by application of the BR inhibitor brassinazole (BRZ). The fact that BRZ treatment restored the WT phenotype of plant and leaf morphology in MSBP1-RNAi plants (Figure 3) additionally argues for an inhibitory role of MSBP1 in BR signaling.
The hypersensitivity of MSBP1-inhibited plants and the rather insensitive behavior of MSBP1-overexpressing plants of flower induction in response to the BR epi-brassinolide (epi-BL; Supplementary Figures S3–S5) treatment further points to a negative role of MSBP1 in BR signal perception and transduction. Plant size, flowering behavior, xylem proliferation, BR responses of MSBP1-silenced and –overexpressing plants further supports the assumption that MSBP1 acts as a negative BR signaling component.
BRs Affect Mycorrhization and Arbuscular Morphology
Our previous investigations revealed reduced arbuscular abundance and mycorrhization intensity of mutants defective in BR biosynthesis. The tomato dx mutant as well as the rice brd2-1 mutant which both are deficient in the DWF1 sterol reductase show reduced mycorrhization intensity if inoculated with Rhizoglomus irregularis (Bitterlich et al., 2014a,b). Therefore, an impact of brassinosteroids on mycorrhization can be expected if supplied directly to the root system of mycorrhizal plants.
Although experiments with tobacco wild type plants externally treated with epi-BL do not show significant effects on the amount of fungus-specific RiGAPDH and of the mycorrhiza-induced plant phosphate transporter PT4 transcripts (Figure 7D),a positive effect of epi-BL on the biomass production of mycorrhizal tobacco and tomato WT plants was observed (Figure 7 and Supplementary Figure S14A). In parallel, arbuscular architecture in WT roots changed when plants were treated externally treated with BRZ or epi-BL (Figure 6). Epi-BL treated roots showed arbuscules with higher tubular diameters, whereas BRZ treated roots harbored arbuscules with smaller tubular diameters than the corresponding mock control (Figure 6).
If MSBP1 is indeed active as an inhibitor of brassinosteroid signaling, then inhibition of BR signaling in MSBP1 overexpressing plants could have similar effects on mycorrhization as observed in case of inhibition of BR biosynthesis by BRZ application. In agreement with this hypothesis, a very similar phenomenon was observed in transgenic MSBP1 tobacco plants, that is, inhibition of MSBP1 expression resulting in higher tubular diameter of arbuscules, whereas overexpression of the putative inhibitor of BR signaling led to smaller tubules (Figures 5, 6).
A reduction of the diameter of tubular branches and highly branched arbuscules with “bushy” appearance would lead to an increase of the interface surface between the host plant and the fungus. Consequently, this could increase exchange activities between both symbiotic partners where the fungus receives carbon containing compounds such as sugars and lipids (Siebers et al., 2016; Bravo et al., 2017), whereas the plant obtains mineral nutrients (Garcia et al., 2016). Against our expectation, the increase in the surface area between plant and fungus is not leading to increased uptake of mineral elements. In contrast, the P, Mn, Fe, Zn, and Ca contents in colonized plants are significantly higher in MSBP1-RNAi plants with arbuscules showing a higher diameter of tubules than in MSBP1-overexpressing or WT plants (Supplementary Figure S12). This did, however, not lead to an increased mycorrhizal effect as this effect disappeared also in lines with down-regulated MSBP1 expression (Figure 4). The reasons for that merit further investigation and may relate to a counter-acting increased transfer of carbohydrates to the fungus, or else. The activity of R. irregularis, however, was not affected by manipulation of MSBP1 expression as revealed by qPCR and ink staining of colonized roots (Supplementary Figures S9, S11B).
From recent investigations it became clear that within AM symbiosis the plant partner defines the tubule architecture where the fungus adapts to Lanfranco et al. (2016). Arbuscule morphology is known to be affected when H+-ATPases or ABC-transporter expression is disturbed (Zhang et al., 2010; Krajinski et al., 2014) leading in most cases to truncated or aborted arbuscules. Nothing is described so far regarding effects on the ramification morphology of arbuscular branches. It should be investigated in future experiments whether the branching of fungal arbuscules and the curvature of the periarbuscular membrane is related to the biophysical properties of the membrane and how this is crucial for mycorrhizal functioning.
MSBP1 Has Multiple Functions
As mentioned above MSBP1 is assumed to act as inhibitor of BR signaling and analysis of MSBP1-silenced and – overexpressing plants is consistent with this assumption. In this case it is expected that down-regulation of MSBP1 positively impacts mycorrhization since BRs have a positive effect on mycorrhization in tobacco and tomato WT plants.
MSBP1 might fulfill a dual function by inhibiting BR signaling on the one side, and enhancing vesicle trafficking and endocytosis of membrane proteins on the other (Yang et al., 2008; Song et al., 2009). Also its negative effect on BR signaling was previously explained by enhancement of endocytosis of the BR co-receptor BAK1 (Song et al., 2009).
For SlSUT2, a sucrose retrieval carrier at the periarbuscular membrane, enhanced endocytosis is assumed to impact root colonization by AM fungi (Bitterlich et al., 2014a,b). Sucrose supply to the fungus and BR-dependent effects might have two opposite effects of MSBP1 on root colonization. And the factors determining which of both effects predominates are still unknown. Interestingly, the expression of sucrose transporter SlSUT2 is upregulated in response to biotic interactions, whereas MSBP1 expression is rather down-regulated (Supplementary Figures S8A, S15) according to the TomExpress database, supporting this hypothesis.
Differences between Medicago truncatula and the solanaceous host plants might be due to a shift of this equilibrium to one or the other side. Alternatively, these differences can be due to species-dependent differences or the fact that silencing of MSBP1 in Medicago was limited locally to the root system only (Kuhn et al., 2010).
MSBP1 Affects Sterol Content and Membrane Composition
MSBP1 seems also to impact the sterol composition of the membrane since both, the overexpressing as well as the RNAi plants showed a slightly decreased cholesterol content (Supplementary Figure S13). In previous studies, it was discussed whether MSPB1 affects AM colonization via regulation of the sterol homeostasis and interferes with microdomain assembly (Bapaume and Reinhardt, 2012). In Arabidopsis, AtMSBP1 is able to bind to progesterone, testosterone and epi-brassinolide in vitro, but also to stigmasterol (Yang et al., 2008), which is part of the plasma membrane and is often distributed asymmetrically in the two leaflets of the plasma membrane. Phytosterols are known to be enriched in membrane microdomains and affect the fluidity and dynamics of the plasma membrane. Membrane microdomains are considered as signaling platforms, which are not only involved in symbiotic but also in pathogenic plant interactions (Bhat et al., 2005; Keinath et al., 2010).
Further investigations are necessary to answer the question whether the multiple MSBP1 effects on vesicle trafficking, endocytosis, arbuscular morphology, and membrane composition are based on the ability of MSBP1 to bind to sterol components.
Conclusion
• Brassinosteroids affect the morphology of arbuscular branches, the nutrient content of colonized host plants and the biomass production in response to mycorrhization. Treatment with the inhibitor BRZ had a negative effect on fungus-specific gene expression. Therefore, a fine-tuning role of BRs in the AM symbiosis is suggested.
• Effects of up- or down-regulation of MSBP1 in transgenic tobacco plants are consistent with an inhibitory role of MSBP1 in BR signaling. The MSBP1-overexpression phenotype can be mimicked by inhibition of BR synthesis via BRZ.
• MSBP1 potentially affects mycorrhization via its inhibitory role in BR signaling. A balanced expression of the protein is therefore important for a positive outcome of the symbiosis.
Data Availability
All datasets generated for this study are included in the manuscript and/or the Supplementary Files.
Author Contributions
CK planned and designed the research. BJ, HJ, LvS, and MR performed the experiments. LvS, MB, PF, and CK analyzed and interpreted the data. PF and CK wrote the manuscript.
Funding
Financial support came from the Leibniz-Gemeinschaft and the DFG open access fond to the Humboldt-University (DFG).
Conflict of Interest Statement
The authors declare that the research was conducted in the absence of any commercial or financial relationships that could be construed as a potential conflict of interest.
Acknowledgments
We would like to acknowledge Bettina Hause for a helpful discussion and Boris Hedtke as well as Kersten Träder for plant transformation, Angelika Pötter for the care of greenhouse plants, and Aleksandra Hackel for excellent technical assistance. We thank Luis Hildebrandt, Lena Roling, Karoline Diesing, and Mustapha Ennajeh for valuable help during harvest of mycorrhizal plants and Benjamin Herzog for preliminary work. Inoculum was kindly provided by the INOQ GmbH, Schnega, Germanya.
Supplementary Material
The Supplementary Material for this article can be found online at: https://www.frontiersin.org/articles/10.3389/fpls.2019.00571/full#supplementary-material
Footnotes
- ^www2.dijon.inra.fr/mychintec/Mycocalc-prg/download.html
- ^http://bioinfo.ut.ee/primer3-0.4.0/
- ^https://www.imbio.uni-bonn.de/molekulare-biotechnologie/lipidomics-platform
References
Abbas, A. K., Lichtman, A. H., and Pillai, S. (2015). Basic Immunology: Functions and Disorders of the Immune System. Philadelphia, PA: Elsevier.
Asami, T., Min, Y. K., Nagata, N., Yamagishi, K., Takatsuto, S., Fujioka, S., et al. (2000). Characterization of brassinazole, a triazole-type brassinosteroid biosynthesis inhibitor. Plant Physiol. 123, 93–100. doi: 10.1104/pp.123.1.93
Azpiroz, R., Wu, Y., Locascio, J. C., and Feldmann, K. A. (1998). An Arabidopsis brassinosteroid-dependent mutant is blocked in cell elongation. Plant Cell 10, 219–230. doi: 10.1105/tpc.10.2.219
Banhara, A., Ding, Y., Kuhner, R., Zuccaro, A., and Parniske, M. (2015). Colonization of root cells and plant growth promotion by Piriformospora indica occurs independently of plant common symbiosis genes. Front. Plant Sci. 6:667. doi: 10.3389/fpls.2015.00667
Bapaume, L., and Reinhardt, D. (2012). How membranes shape plant symbioses: signaling and transport in nodulation and arbuscular mycorrhiza. Front. Plant Sci. 3:223. doi: 10.3389/fpls.2012.00223
Belkhadir, Y., Jaillais, Y., Epple, P., Balsemao-Pires, E., Dangl, J. L., and Chory, J. (2012). Brassinosteroids modulate the efficiency of plant immune responses to microbe-associated molecular patterns. Proc. Natl. Acad. Sci. U.S.A. 109, 297–302. doi: 10.1073/pnas.1112840108
Bhat, R. A., Miklis, M., Schmelzer, E., Schulze-Lefert, P., and Panstruga, R. (2005). Recruitment and interaction dynamics of plant penetration resistance components in a plasma membrane microdomain. Proc. Natl. Acad. Sci. U.S.A. 102, 3135–3140. doi: 10.1073/pnas.0500012102
Bitterlich, M., Krügel, U., Boldt-Burisch, K., Franken, P., and Kühn, C. (2014a). Interaction of brassinosteroid functions and sucrose transporter SlSUT2 regulate the formation of arbuscular mycorrhiza. Plant Signal. Behav. 9:e970426. doi: 10.4161/15592316.2014.970426
Bitterlich, M., Krügel, U., Boldt-Burisch, K., Franken, P., and Kühn, C. (2014b). The sucrose transporter SlSUT2 from tomato interacts with brassinosteroid functioning and affects arbuscular mycorrhiza formation. Plant J. 78, 877–889. doi: 10.1111/tpj.12515
Bravo, A., Brands, M., Wewer, V., Dormann, P., and Harrison, M. J. (2017). Arbuscular mycorrhiza-specific enzymes FatM and RAM2 fine-tune lipid biosynthesis to promote development of arbuscular mycorrhiza. New Phytol. 214, 1631–1645. doi: 10.1111/nph.14533
Chen, S., Hofius, D., Sonnewald, U., and Börnke, F. (2003). Temporal and spatial control of gene silencing in transgenic plants by inducible expression of double-stranded RNA. Plant J. 36, 731–740. doi: 10.1046/j.1365-313x.2003.01914.x
Clouse, S. D., and Sasse, J. M. (1998). BRASSINOSTEROIDS: essential regulators of plant growth and development. Annu. Rev. Plant Physiol. Plant Mol. Biol. 49, 427–451. doi: 10.1146/annurev.arplant.49.1.427
Deblaere, R., Bytebier, B., De Greve, H., Deboeck, F., Schell, J., Van Montagu, M., et al. (1985). Efficient octopine Ti plasmid-derived vectors for Agrobacterium mediated gene transfer to plants. Nucleic Acids Res. 13, 4777–4788. doi: 10.1093/nar/13.13.4777
De Kreji, C., Voogt, W., Van den Bos, A. L., and Baas, R. (1997). Voedingsoplossingen Gesloten Teeltsystemen, Tomaat. Proefstation voor Bloemisterij en Glasgroente, Naaldwijk/Aalsmeer. Netherlands: Brochure VG 2, 21.
Foo, E., Ross, J. J., Jones, W. T., and Reid, J. B. (2013). Plant hormones in arbuscular mycorrhizal symbioses: an emerging role for gibberellins. Ann. Bot. 111, 769–779. doi: 10.1093/aob/mct041
Garcia, K., Doidy, J., Zimmermann, S. D., Wipf, D., and Courty, P. E. (2016). Take a trip through the plant and fungal transportome of mycorrhiza. Trends Plant Sci. 21, 937–950. doi: 10.1016/j.tplants.2016.07.010
Hackel, A., Schauer, N., Carrari, F., Fernie, A. R., Grimm, B., and Kühn, C. (2006). Sucrose transporter LeSUT1 and LeSUT2 inhibition affects tomato fruit development in different ways. Plant J. 45, 180–192. doi: 10.1111/j.1365-313x.2005.02572.x
Keinath, N. F., Kierszniowska, S., Lorek, J., Bourdais, G., Kessler, S. A., Shimosato-Asano, H., et al. (2010). PAMP (pathogen-associated molecular pattern)-induced changes in plasma membrane compartmentalization reveal novel components of plant immunity. J. Biol. Chem. 285, 39140–39149. doi: 10.1074/jbc.M110.160531
Koske, R. E., and Gemma, J. N. (1989). A modified procedure for staining roots to detect VA mycorrhizas. Mycol. Res. 92, 486–488. doi: 10.1016/s0953-7562(09)80692-8
Krajinski, F., Courty, P. E., Sieh, D., Franken, P., Zhang, H., Bucher, M., et al. (2014). The H+-ATPase HA1 of medicago truncatula is essential for phosphate transport and plant growth during arbuscular mycorrhizal symbiosis. Plant Cell 26, 1808–1817. doi: 10.1105/tpc.113.120436
Kuhn, H., Kuster, H., and Requena, N. (2010). Membrane steroid-binding protein 1 induced by a diffusible fungal signal is critical for mycorrhization in Medicago truncatula. New Phytol. 185, 716–733. doi: 10.1111/j.1469-8137.2009.03116.x
Lanfranco, L., Bonfante, P., and Genre, A. (2016). The mutualistic interaction between plants and arbuscular mycorrhizal fungi. Microbiol. Spectr. 4, 1–20.
Larsson, C. (1985). “Plasma membranes,” in Cell Components. Modern Methods of Plant Analysis, eds H. F. Linskins and J. F. Jackson (Berlin: Springer Verlag).
Li, J., Li, Y., Chen, S., and An, L. (2010). Involvement of brassinosteroid signals in the floral-induction network of Arabidopsis. J. Exp. Bot. 61, 4221–4230. doi: 10.1093/jxb/erq241
Nagata, N., Asami, T., and Yoshida, S. (2001). Brassinazole, an inhibitor of brassinosteroid biosynthesis, inhibits development of secondary xylem in cress plants (Lepidium sativum). Plant Cell Physiol. 42, 1006–1011. doi: 10.1093/pcp/pce122
Schmidt, G. W., and Delaney, S. K. (2010). Stable internal reference genes for normalization of real-time RT-PCR in tobacco (Nicotiana tabacum) during development and abiotic stress. Mol. Genet. Genomics 283, 233–241. doi: 10.1007/s00438-010-0511-1
Segonzac, C., Macho, A. P., Sanmartin, M., Ntoukakis, V., Sanchez-Serrano, J. J., and Zipfel, C. (2014). Negative control of BAK1 by protein phosphatase 2A during plant innate immunity. EMBO J. 33, 2069–2079. doi: 10.15252/embj.201488698
Shi, Q. M., Yang, X., Song, L., and Xue, H. W. (2011). Arabidopsis MSBP1 is activated by HY5 and HYH and is involved in photomorphogenesis and brassinosteroid sensitivity regulation. Mol. Plant 4, 1092–1104. doi: 10.1093/mp/ssr049
Siebers, M., Brands, M., Wewer, V., Duan, Y., Holzl, G., and Dormann, P. (2016). Lipids in plant-microbe interactions. Biochim. Biophys. Acta 1861, 1379–1395. doi: 10.1016/j.bbalip.2016.02.021
Song, L., Shi, Q. M., Yang, X. H., Xu, Z. H., and Xue, H. W. (2009). Membrane steroid-binding protein 1 (MSBP1) negatively regulates brassinosteroid signaling by enhancing the endocytosis of BAK1. Cell Res. 19, 864–876. doi: 10.1038/cr.2009.66
Symons, G. M., Davies, C., Shavrukov, Y., Dry, I. B., Reid, J. B., and Thomas, M. R. (2006). Grapes on steroids. Brassinosteroids are involved in grape berry ripening. Plant Physiol. 140, 150–158. doi: 10.1104/pp.105.070706
Szekeres, M., Nemeth, K., Koncz-Kalman, Z., Mathur, J., Kauschmann, A., Altmann, T., et al. (1996). Brassinosteroids rescue the deficiency of CYP90, a cytochrome P450, controlling cell elongation and de-etiolation in Arabidopsis. Cell 85, 171–182. doi: 10.1016/s0092-8674(00)81094-6
Tofighi, C., Khavari-Nejad, R. A., Najafi, F., Razavi, K., and Rejali, F. (2017). Responses of wheat plants to interactions of 24-epibrassinolide and Glomus mosseae in saline condition. Physiol. Mol. Biol. Plants 23, 557–564. doi: 10.1007/s12298-017-0439-6
Trouvelot, A., Kough, J. L., and Gianninazzi-Pearson, V. (1986). “Mesure du taux de mycorrhization VA d’un système radiculaire. Recherche de méthodes d’estimation ayant une signification fonctionelle,” in Physiological and Genetical Aspects of Mycorrhizae, eds V. Gianinazzi-Pearson and S. Gianinazzi (Paris: INRA).
Wewer, V., Dombrink, I., Vom Dorp, K., and Dormann, P. (2011). Quantification of sterol lipids in plants by quadrupole time-of-flight mass spectrometry. J. Lipid Res. 52, 1039–1054. doi: 10.1194/jlr.D013987
Witzel, K., Matros, A., Moller, A. L. B., Ramireddy, E., Finnie, C., Peukert, M., et al. (2018). Plasma membrane proteome analysis identifies a role of barley membrane steroid binding protein in root architecture response to salinity. Plant Cell Environ. 41, 1311–1330. doi: 10.1111/pce.13154
Yang, X., Song, L., and Xue, H. W. (2008). Membrane steroid binding protein 1 (MSBP1) stimulates tropism by regulating vesicle trafficking and auxin redistribution. Mol. Plant 1, 1077–1087. doi: 10.1093/mp/ssn071
Yang, X. H., Xu, Z. H., and Xue, H. W. (2005). Arabidopsis membrane steroid binding protein 1 is involved in inhibition of cell elongation. Plant Cell 17, 116–131. doi: 10.1105/tpc.104.028381
Zhang, D. W., Deng, X. G., Fu, F. Q., and Lin, H. H. (2015). Induction of plant virus defense response by brassinosteroids and brassinosteroid signaling in Arabidopsis thaliana. Planta 241, 875–885. doi: 10.1007/s00425-014-2218-8
Zhang, Q., Blaylock, L. A., and Harrison, M. J. (2010). Two Medicago truncatula half-ABC transporters are essential for arbuscule development in arbuscular mycorrhizal symbiosis. Plant Cell 22, 1483–1497. doi: 10.1105/tpc.110.074955
Keywords: BR signaling, sucrose transport, arbuscular mycorrhiza, endocytosis, SUT2, MSBP1, Solanum lycopersicum, Nicotiana tabacum
Citation: von Sivers L, Jaspar H, Johst B, Roese M, Bitterlich M, Franken P and Kühn C (2019) Brassinosteroids Affect the Symbiosis Between the AM Fungus Rhizoglomus irregularis and Solanaceous Host Plants. Front. Plant Sci. 10:571. doi: 10.3389/fpls.2019.00571
Received: 28 February 2019; Accepted: 15 April 2019;
Published: 15 May 2019.
Edited by:
Brigitte Mauch-Mani, Université de Neuchâtel, SwitzerlandReviewed by:
Maria J. Pozo, Spanish National Research Council (CSIC), SpainLuisa Lanfranco, University of Turin, Italy
Copyright © 2019 von Sivers, Jaspar, Johst, Roese, Bitterlich, Franken and Kühn. This is an open-access article distributed under the terms of the Creative Commons Attribution License (CC BY). The use, distribution or reproduction in other forums is permitted, provided the original author(s) and the copyright owner(s) are credited and that the original publication in this journal is cited, in accordance with accepted academic practice. No use, distribution or reproduction is permitted which does not comply with these terms.
*Correspondence: Christina Kühn, a3VlaG5jaHJAYmlvbG9naWUuaHUtYmVybGluLmRl; a3VlaG5jaHJAY21zLmh1LWJlcmxpbi5kZQ==