- 1Institut Jean-Pierre Bourgin, INRA, AgroParisTech, CNRS, Université Paris-Saclay, Versailles, France
- 2Laboratoire de Biologie Moléculaire et Cellulaire, Institut de Biologie, Université de Neuchâtel, Neuchâtel, Switzerland
The XPF-ERCC1 complex, a highly conserved structure-specific endonuclease, functions in multiple DNA repair pathways that are pivotal for maintaining genome stability, including nucleotide excision repair, interstrand crosslink repair, and homologous recombination. XPF-ERCC1 incises double-stranded DNA at double-strand/single-strand junctions, making it an ideal enzyme for processing DNA structures that contain partially unwound strands. Here, we have examined the role of the XPF-ERCC1 complex in the model bryophyte Physcomitrella patens which exhibits uniquely high gene targeting frequencies. We undertook targeted knockout of the Physcomitrella ERCC1 and XPF genes. Mutant analysis shows that the endonuclease complex is essential for resistance to UV-B and to the alkylating agent MMS, and contributes to the maintenance of genome integrity but is also involved in gene targeting in this model plant. Using different constructs we determine whether the function of the XPF-ERCC1 endonuclease complex in gene targeting was removal of 3′ non-homologous termini, similar to SSA, or processing of looped-out heteroduplex intermediates. Interestingly, our data suggest a role of the endonuclease in both pathways and have implications for the mechanism of targeted gene replacement in plants and its specificities compared to yeast and mammalian cells.
Introduction
The XPF-ERCC1 complex is a highly conserved heterodimeric structure-specific endonuclease (SSE) composed of the XPF catalytic subunit and the ERCC1 DNA binding subunit that is involved in DNA repair and maintenance of chromosome stability (Dehé and Gaillard, 2017; Faridounnia et al., 2018). The protein sequences of the different ERCC1 homologs (ERCC1 in Drosophila melanogaster, Rad10 in Saccharomyces cerevisiae and Swi10 in Schizosaccharomyces pombe) and XPF homologs (MEI-9 in D. melanogaster, Rad1 in S. cerevisiae and Rad16 in S. pombe) are highly conserved as well as their capacity for heterodimerization, which insures stability and functionality of the complex. Consistent with the importance of heterodimerization of the two proteins for their function is that individual mutants in the ERCC1 and XPF genes exhibit similar phenotypes (Gregg et al., 2011). The endonuclease activity of the XPF-ERCC1 complex is responsible for DNA cleavage near junctions between single-stranded and double-stranded DNA, where the single strand departs 5′ to 3′ from the junction.
XPF-ERCC1 is essential for nucleotide excision repair (NER) of DNA, a mechanism that removes DNA damage induced by ultraviolet (UV) radiation and by mutagenic chemicals, or chemotherapeutic drugs that form bulky DNA adducts. In mammalian cells, null mutants of the ERCC1 or XPF genes are lethal and weaker mutations can result in xeroderma pigmentosum (XP), trichothiodystrophy (TTD), and Cockayne syndrome (CS), genetic disorders that are typical of mutations in genes required for NER (Gregg et al., 2011). However, there is evidence that the ERCC1 and XPF proteins have functions distinct from NER. Indeed, it was demonstrated that XPF-ERCC1 participates in the Fanconi Anemia Pathway of DNA interstrand crosslinks repair (Bhagwat et al., 2009) and recently the XPF-ERCC1 complex has been shown to be involved in a sub-pathway of long-patch base excision repair (BER) involving 5′ gap formation (Woodrick et al., 2017).
In addition to involvement in NER, BER and interstrand crosslink repair, there is evidence for a role of XPF-ERCC1 in double strand break (DSB) repair (Ahmad et al., 2008). Resolution of a DSB can be done by non-homologous end joining or by homology-directed repair (HDR) (Symington and Gautier, 2011). The XPF-ERCC1 complex and its S. cerevisiae homolog, the RAD1-RAD10 complex have been shown to participate to non-homologous repair of DSB and the RAD1 protein of S. cerevisiae and the mammalian ERCC1 protein play a major role in Alt-EJ (also known as MMEJ), a Ku-independent sub-pathway of NHEJ, that is error-prone, and that utilizes short stretches of homology to join two broken DNA ends (Ma et al., 2003; Ahmad et al., 2008). The RAD1-RAD10/XPF-ERCC1 complex participates also to intra or extra chromosomal HR between sequence repeats in S. cerevisiae (Klein, 1988; Schiestl and Prakash, 1988, 1990; Fishman-Lobell and Haber, 1992; Ivanov and Haber, 1995; Prado and Aguilera, 1995), in mammalian cells (Sargent et al., 1997, 2000; Al-Minawi et al., 2008) and in plants (Dubest et al., 2002, 2004). The function in HR of the RAD1-RAD10 endonuclease, is the removal of non-homologous 3′ termini of single-stranded overhangs of broken ends to facilitate single-strand annealing (SSA), an error-prone sub-pathway of HR (Bardwell et al., 1994; Pâques and Haber, 1997). Interestingly, the function of the RAD1-RAD10/XPF-ERCC1 complex has been shown to be also important for HR mediated gene targeting (GT) in S. cerevisiae and in mammalian cells (Schiestl and Prakash, 1988; Saffran et al., 1994; Adair et al., 2000; Niedernhofer et al., 2001; Langston and Symington, 2005; Rahn et al., 2011). There are two general methods for gene targeting, based on the two arrangements of donor DNA that can be used for gene targeting, called ends-in and ends-out (Hastings et al., 1993). They differ in whether the double-strand break is within the region of homology (ends-in) or at the ends (ends-out) leading to targeted gene replacement (TGR). Analysis of the capacity for GT using ends-in or ends-out substrates of recombination of mutants affected in the XPF-ERCC1 complex suggest that this complex could have different roles during gene targeting and that these roles could differ from one species to another.
In flowering plants, the study of the mechanisms of recombination is far behind that of mammals and yeast (Waterworth et al., 2011) and the low level of gene targeting efficiency, even if it can be increased to 1% by using a CRISPR-Cas9 based strategy (Wolter et al., 2018), makes the deciphering of this important mechanism difficult. However, in the plant kingdom the moss Physcomitrella patens is an exception to this rule and exhibits rates of gene targeting (GT) comparable to S. cerevisiae allowing advances in the understanding of DNA metabolism in plants (Schaefer and Zrÿd, 1997; Kamisugi et al., 2005, 2006; Odahara et al., 2007; Trouiller et al., 2007, 2006; Schaefer et al., 2010; Kamisugi et al., 2012; Wendeler et al., 2015; Collonnier et al., 2017; Odahara and Sekine, 2018; Wiedemann et al., 2018). It must be noticed that the mechanisms underlying targeted DNA integration are not necessarily conserved between P. patens and S. cerevisiae (Schaefer et al., 2010) and these differences can probably account for the differences that can be observed concerning the nature of the final products of ends-out constructs integration in the two species. First, ends-out construct integration producing single copy gene conversion (TGR), similar to what is observed in yeast, can be found in Physcomitrella but the majority (more than 80%) of the targeted integration events comprises head-to-tail concatemers of the transforming DNA fragment (Schaefer, 2002; Kamisugi et al., 2006), that could result from episomally replicating DNA (Murén et al., 2009). Second, a significant proportion (around 50%) of gene targeting events in Physcomitrella result in insertion of the ends-out construct adjacent to the target locus (targeted gene insertion-TGI). Typically, TGI comprises an HR event at one end of the integrant, accompanied by an apparent NHEJ event at the other (Schaefer, 2002; Kamisugi et al., 2005, 2006; Collonnier et al., 2017). This profile of integration is rarely observed in S. cerevisiae but frequent for GT events in flowering plants and animal cells (Adair et al., 1998; Hanin et al., 2001).
To investigate the potential role of the XPF-ERCC1 complex in DSB repair and gene targeting mechanisms in Physcomitrella we produced mutants for the PpERCC1 and PpXPF homologous genes. Our data show that loss of ERCC1 and/or XPF functions generates a strong mutator phenotype and hypersensitivity to UV-B and methyl methanesulfonate (MMS) induced DNA lesions, suggesting an active role of this complex in the moss NER and BER repair pathways. Using different constructs we further revealed that the moss XPF-ERCC1 complex is required for HR between both ends-out or ends-in constructs and genomic loci.
Materials and Methods
Plant Material and Growth Conditions
Physcomitrella patens (Hedw.) B.S.G. “Gransden” was used in this study. Individual strains were vegetatively propagated as lawns of protonemal filaments on rich agar PpNH4 medium (PpNO3 medium supplemented with 2.7 mM NH4-tartrate) overlaid with cellophane, or cultured as “spot inocula” on minimal medium (PpNO3) for phenotypic analyses and sporogenesis as previously described (Trouiller et al., 2006). Protoplast isolation, PEG mediated transformation and selection of transformed plants were performed according to (Schaefer et al., 2010).
Gene Identification and Isolation
Genomic DNA and total RNA were isolated from Physcomitrella as previously described (Trouiller et al., 2006). Physcomitrella genomic sequences encoding the ERCC1 and XPF genes were identified by BLAST search1. The available gene models were used for the design of PCR primers to amplify cognate genomic sequences, which were cloned in the TOPO®-TA (life technologies, United States) or pBluescript (Stratagene, United States) plasmids. PCR primers used are listed in Supplementary Table S1. In order to obtain a correct gene model for each sequence, full-length cDNA were amplified from Physcomitrella polyribosome-derived RNA by RT-PCR (Kamisugi et al., 2012) and sequenced. Predicted polypeptide sequences were aligned with the orthologous genes from other eukaryotes using CLUSTALW.
Generation of Deletion Mutants
The KO vector pERCC1 delta contains a 654 bp 5′-targeting fragment (chrom6: 19338880–19339533) and an 865 bp 3′-targeting fragment (chrom6: 19341999–19342863) flanking a LoxP-HygroR-LoxP marker in vector pBHRF (Schaefer et al., 2010). The KO vector pXPF delta contains a 974 bp 5′-targeting fragment (chrom18: 8489087–8490060) and a 1096 bp 3′-targeting fragment (chrom18: 8493588–8494683) flanking a LoxP-NeoR-LoxP marker in vector pBNRF (Schaefer et al., 2010). Moss protoplasts were transformed with pERCC1 delta digested with AvrII and PacI, or with pXPF delta digested with XbaI and PacI. Transformed plants carrying targeted gene replacement were identified by PCR genotyping and subsequent deletion of the selection marker was obtained by transient Cre recombinase expression (Trouiller et al., 2006). The double ercc1/xpf mutant was generated by retransforming a PpErcc1Δ deletion line with pXPF delta and selecting for targeted gene replacement at the PpXPF locus among neoR plants.
Analysis of Gene Expression in Mutants
Transcript abundance in selected knockout lines was determined by RT-PCR. Total RNA was extracted using TRIZOL-reagent (Invitrogen) from 100 mg of protonemal tissue. Contaminating DNA was removed by DNaseI treatment with RNase Free DNase set (Qiagen) using spin columns of the Rneasy plant mini kit (Qiagen). RT-PCR was performed with RevertAid H Minus M-MuLV Reverse Transcriptase (Fermentas) on 500 ng RNA according to the supplier’s instructions. Quality control of DNA or RNA was performed using primers PpAPT#14 + PpAPT#19 (Supplementary Table S1). Detection of ERCC1 and XPF mRNA in mutant lines was performed by RT-PCR using primers indicated in Supplementary Table S1.
UV-B and MMS Sensitivity Assays
For UV-B sensitivity protoplasts of wild-type, ercc1Δ, xpfΔ, or xpfKΔ/ercc1Δ strains were spread (ca. 25000/plate) and regenerated on protoplast agar medium (PpNH4 + 0.5 g/L glucose + 6.6% mannitol). Plates were immediately exposed to UV-B light (60 J/m2/s) from a 312 nm TFX lamp. We calculated the flux with a UV-Elektronik GmbH dosimeter. The. Plates were immediately transferred to darkness for 24 h after treatment then to standard growth conditions for protoplast regeneration. Survival was determined as described previously (Kamisugi et al., 2012). For Methyl methanesulfonate (MMS) (Sigma-Aldrich) sensitivity, protoplasts were spread on protoplast agar medium freshly supplemented with different concentration of MMS and further regenerated under standard growth conditions. Cultures were transferred to PpNH4 medium without MMS after 6 days and survival was determined 2 weeks later by microscopic observation.
Evaluation of Spontaneous Mutation Frequency
To assess the mutator phenotype of ercc1Δ and xpfΔ mutants, we measured the level of spontaneous loss of function of the Adenine Phosphoribosyl Transferase gene (PpAPT) which confers resistance to 2-fluoroadenine (2-FA), as previously described (Trouiller et al., 2006). Several million of plants were regenerated from protoplasts of wild-type, ercc1Δ and xpfΔ mutants and then transferred to medium supplemented with 10 mM 2-FA (Fluorochem). After 2 weeks, the number of resistant plants was counted. Results were analyzed using the Fisher’s exact test.
Gene Targeting Assays
The different APT based gene targeting constructs used in this study are described in Figures 3, 4. GT efficiencies were assessed after transformation with vector PpAPT-KO2 (Schaefer et al., 2010) and selection for HygroR plants followed by selection for 2-FAR plants among them, as performed in Charlot et al. (2014), GT frequencies were assessed after transformation with vector PpAPT-KO2 or PpAPT-KO9 and direct selection of 2-FAR plants, a selection that only identifies targeted integration events in PpAPT. To evaluate the importance of the form of the transforming DNA, transformation was performed with PpAPT-KO9 digested with EcoRI + HindIII (ends-out) or with XbaI (ends-in) and direct selection for 2-FAR plants. To study the impact of heterologous sequences at the ends of the transforming DNA, transformation was performed with PpAPT-KO9 digested with ApaLI or with PpAPT-KO2 linearized within the hygromycin resistance cassette with AsiSI (long ends-in): in both cases transformation was followed by direct selection for 2-FAR clones. Experiments were repeated three to eight times and statistically analyzed using the Fisher exact test.
Analysis of Transformed Plants
In order to analyze large numbers of transformed plants for the nature of gene targeting events, PCR-based genotyping assays were used. Primers PpAPT#2 and PpAPT#20 located outside the genomic fragments present in the cassette (Supplementary Table S1 and Figure 3) were used to detect monocopy insertions. For detection of targeted gene replacement (TGR) and targeted gene insertion (TGI) integrations the 5′ and 3′ junctions of the integrations were characterized using primers PpAPT#2 + ProRev and PpAPT#20 + TerFwd (Supplementary Table S1 and Figure 3) respectively.
Cytology
Binocular observations were made with a Nikon SMZ1000.
Results
Identification of PpXPF and PpERCC1 and Generation of Deletion Mutants
The XPF-ERCC1 heterodimeric complex is a highly conserved structure endonuclease (Schwartz and Heyer, 2011). Sequence homology searches of the P. patens genome with the Arabidopsis homologs identified a single putative homolog for XPF (PpXPF, Pp3c18_11670) and ERCC1 (PpERCC1, Pp3c6_29610). PpXPF and PpERCC1 full length cDNAs were isolated and sequenced: this analysis confirmed the predicted structures (9 exons, Figure 1A) and protein sequences found in the database (Phytozome 12.0). A phylogenetic analysis, including plants, algae, animals and fungi established that PpXPF and PpERCC1 effectively belong to the XPF and ERCC1 plant clades among eukaryotic SSE of the XPF/MUS81 family (Supplementary Figure S1). The moss PpXPF protein is composed of 1047 amino acids and shares 50.3, 35.5, and 30.4% sequence identity with AtXPF, HsXPF, and SpRad16, respectively (Supplementary Figure S2). The homology between PpXPF and the XPF/RAD1 homologs is distributed throughout their length, but it is especially strong at the C-terminal end, in the region involved in the formation of the heterodimeric protein complex. Noticeably, the nuclease domain (restriction endonuclease type-II like PR011335) containing the specific motif ERKXXXD required for nuclease activity (Ciccia et al., 2008) and the RuvA_2-like domain (IPR010994) containing the duplicated Helix-hairpin-Helix (HhH) motif (PFAM:HHH_5) functioning as a scaffold for complex formation with ERCC1 (Tripsianes et al., 2005) can be identified in PpXPF (Supplementary Figure S2). The moss PpERCC1 protein is composed of 427 amino acids and shares 44.6, 27.6, and 23.3% sequence identity with AtERCC1, HsERCC1, and SpSwi10, respectively (Supplementary Figure S3). Sequence conservation is especially high within the central RAD10 domain (IPR004579) and the C-terminal RuvA 2-like domain, which are involved in DNA binding and complex formation in ERCC1 homologs (Manandhar et al., 2015). These features are consistent with our assumption that PpXPF and PpERCC1 encode the proteins forming the moss heterodimeric XPF/ERCC1 complex involved in several aspects of DNA repair.
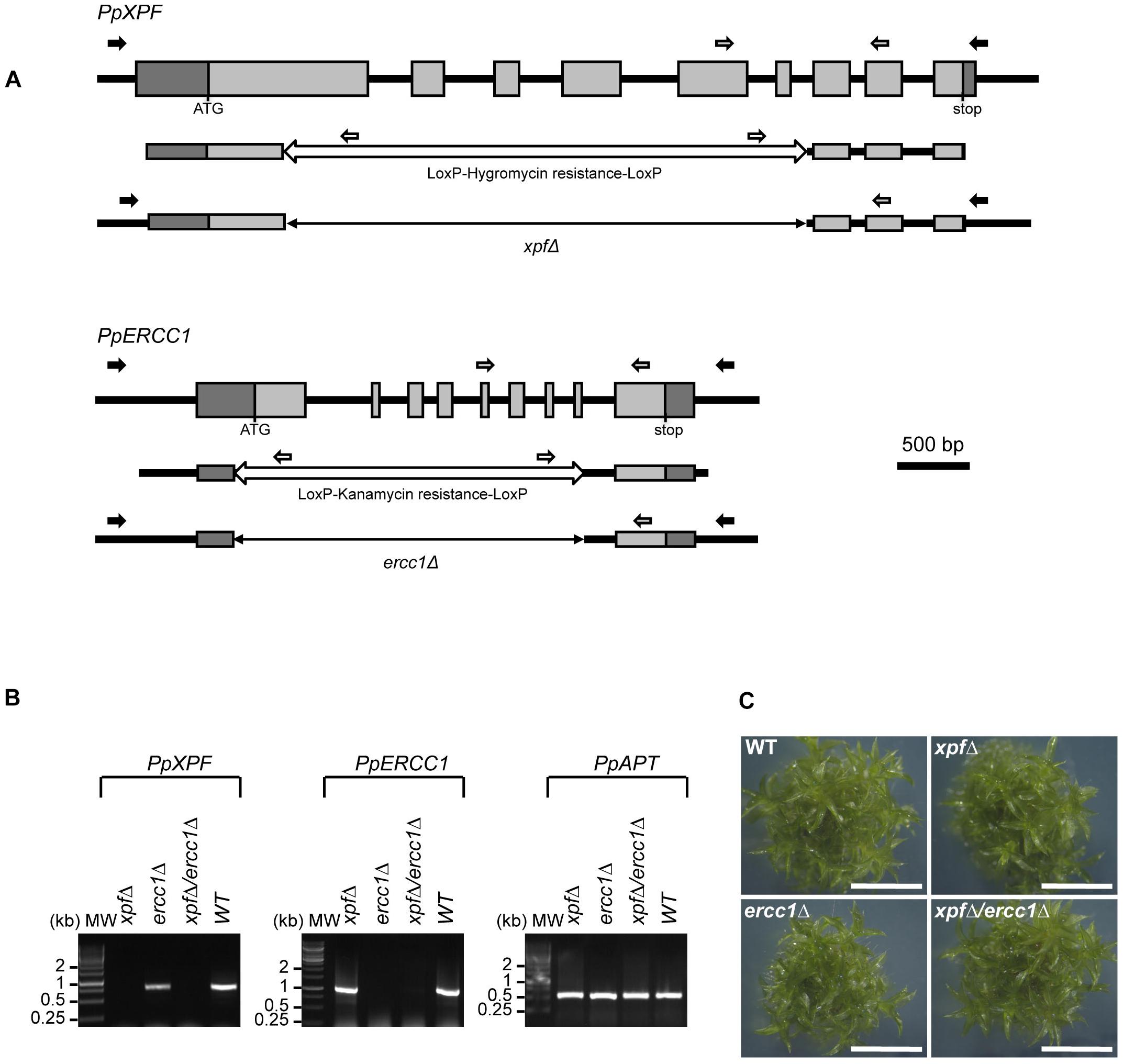
Figure 1. Structure and targeted disruption of Physcomitrella XPF and ERCC1 genes. (A) Structure of the WT, PpXPF, and PpERCC1 loci (top line), of the KO vector (middle) and of the deleted locus in the xpfΔ and ercc1Δ mutants (bottom). Exons are represented by gray boxes, with 5′- and 3′-UTR sequences in darker gray. The region deleted by cre-lox excision of a selection cassette is shown as a double arrow line. Arrows indicate the position of the primers used to genotype the plants by PCR (black and white) and RT-PCR (gray). (B) RT-PCR analysis of XPF and ERCC1 transcripts in wild-type and mutants plants. RNA was isolated from protonemal tissue of wild-type and mutants lines for cDNA synthesis and PCR amplification using gene-specific primers. The PpAPT transcript has been used as control. Primers are listed in Supplementary Table S1. (C) Morphology of plants of wild type of xpfΔ, ercc1Δ single mutants and xpfΔ/ercc1Δ double mutant. The picture was taken after 3 weeks of growth. scale bar = 4 mm.
To investigate PpXPF and PpERCC1 functions we generated xpf and ercc1 deletion mutants, named xpfΔ and ercc1Δ respectively, using targeted gene disruption followed by Cre/lox mediated elimination of the resistance cassette (Figure 1A and section “Materials and Methods”). Deletion of exons 1–8 in PpERCC1 and of exons 1–6 in PpXPF was confirmed by PCR genotyping and sequence analysis (data not shown). A double knock-out mutant named xpfΔ/ercc1Δ was produced by re-transformation of an ercc1Δ mutant with the pXPF delta vector. RT-PCR analysis established that the full-length transcripts were no longer produced in these mutants (Figure 1B). For all further experiments, we used two independent xpfΔ, ercc1Δ, or xpfΔ/ercc1Δ strains and both alleles of the same mutants show similar phenotypes.
The xpfΔ, ercc1Δ, and xpfΔ/ercc1Δ Mutants Show No Developmental Defects
In Bryophytes, the life cycle is dominated by the haploid gametophyte. Haploid spores of P. patens germinate to form a juvenile filamentous network of tip growing cells, the protonema. One week after germination, initials of leafy shoots called buds differentiate from protonemal branch initials and further develop by meristematic growth into the leafy gametophores. After 1 month, each individual plant is composed of several dozens of gametophores. Short day length and low temperature induces the differentiation of the reproductive organs at the shoot apex. Finally fertilization of the egg cell by flagellated antherozoids give rise to the epiphytic diploid sporophyte in which meiosis takes place to produce new haploid spores, reviewed in Bonhomme et al. (2013) and Kofuji and Hasebe (2014).
The phenotype of the xpfΔ, ercc1Δ, and xpfΔ/ercc1Δ mutants was assessed throughout the entire life cycle. Protonemal growth, bud differentiation and leafy shoots development were similar in the three mutants compared to the wild-type and the xpfΔ, ercc1Δ, and xpfΔ/ercc1Δ strains are fertile (Figure 1C and Supplementary Figure S4). These findings show that the XPF/ERCC1 complex plays no direct role in either vegetative or reproductive moss development.
The xpfΔ, ercc1Δ, and xpfΔ/ercc1Δ Mutants Are Impaired in Repairing Exogenous and Endogenous DNA Damage
The XPF/ERCC1 complex is involved in several DNA repair pathways in eukaryotes: it is a major factor of NER and also contributes to ICL, SSA, and HR. We therefore evaluated the sensitivity of the mutants to DNA damage induced by UV-B and methyl methanesulfonate (MMS). To assess the sensitivity of the xpfΔ, ercc1Δ, and xpfΔ/ercc1Δ strains to UV-B light, which generates DNA damage essentially repaired by the NER pathway, we monitored the ability of UV-B-treated protoplasts to regenerate into plants. Our data show that both xpfΔ and ercc1Δ mutants display an extremely high UV-B sensitivity which is not further increased in the double xpfΔ/ercc1Δ strain (Figure 2 and Supplementary Figure S5). With a lethal dose 50 around 8 mJ/cm2, these mutants are ca. 10-fold more sensitive than the rad51-1-2 double mutant strain (Schaefer et al., 2010; Charlot et al., 2014) and 20-fold more sensitive than the WT (Figure 2). Such a strong sensitivity to UV-B provides evidence for a key involvement of the moss XPF/ERCC1 complex in the repair of UV-induced DNA damage, most likely by the NER pathway. We further evaluate the implication of the XPF/ERCC1 complex in the repair of DNA damage induced by the alkylating agent MMS, which is believed to stall replication forks. In this assay, fresh protoplasts are regenerated for 6 days on MMS containing medium, and their ability to regenerate into plants is evaluated after a further 14-days of growth on standard medium. Our analyses show that the xpfΔ, ercc1Δ, and xpfΔ/ercc1Δ strains display a similar increased sensitivity to MMS compared to the WT (Figure 2 and Supplementary Figure S5). This finding indicates that the moss XPF/ERCC1 complex contributes to the repair of DNA damages induced by an MMS treatment. Further experiments are needed to characterize the exact nature of these damages.
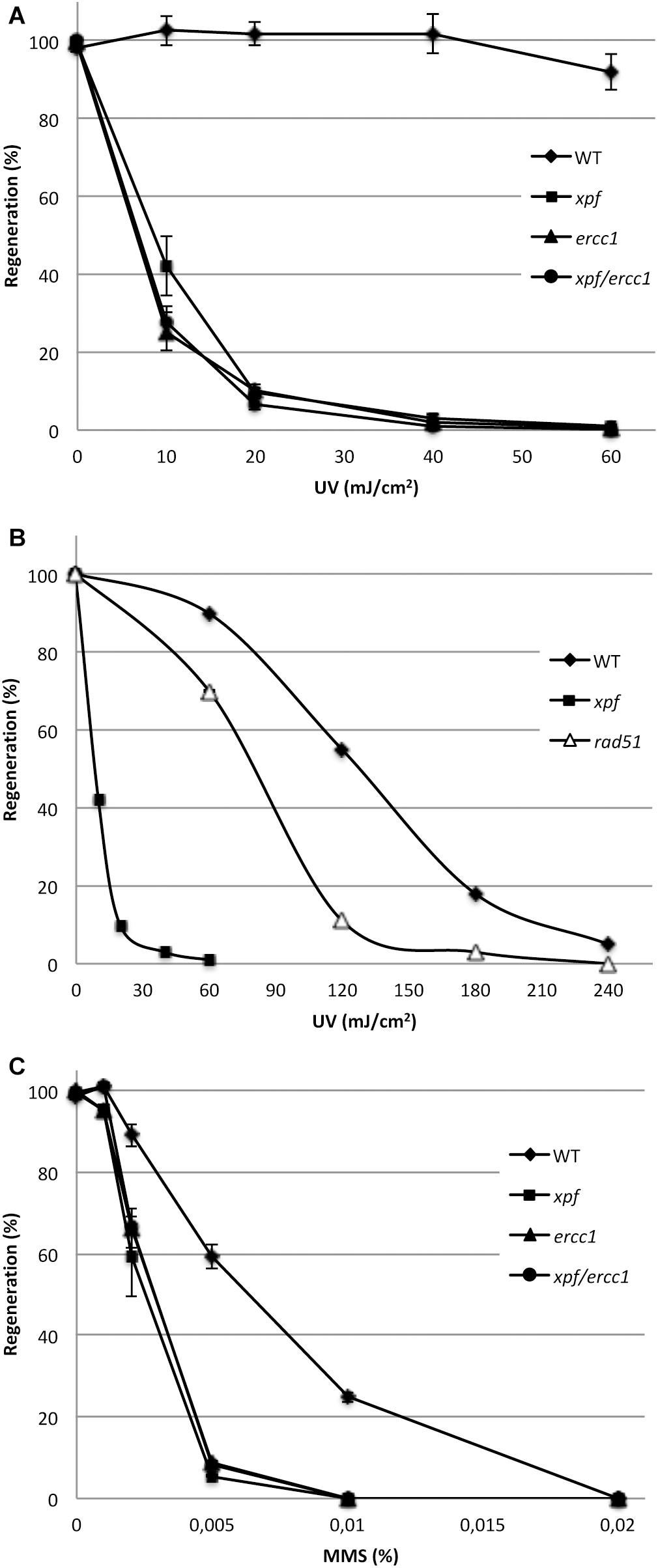
Figure 2. Sensitivity of the wild-type (WT) and of the xpf, ercc1, and xpf/ercc1 mutants toward genotoxic agents. (A) Survival curves of the WT, xpf and ercc1 (n = 4), and xpf/ercc1 strains (n = 2) in response to low doses of UV-B light (scale bar = standard deviation). (B) The survival curves of WT, xpf and rad51 strains in response to higher UV-B doses are shown (references: this study and Charlot et al., 2014). (C) Survival rates of protoplasts after exposure to MMS: survival is expressed as the percentage of regenerated protoplasts relative to untreated samples. The experiment was performed two times except for xpf/ercc1 (scale bar = standard deviation).
Finally to evaluate the level of contribution of the XPF/ERCC1 complex to the repair of endogenous DNA damage, we measured the level of spontaneous loss of function of the PpAPT reporter gene (Trouiller et al., 2006) and assess the mutator phenotype of these mutants. More than 2 million protoplasts of WT, xpfΔ and ercc1Δ strains were regenerated and selected for their resistance to 2-fluoroadenine. No 2-FA resistant plants were recovered in the WT, and a total of 5 and 4 2-FA resistant plants were identified in the xpfΔ and ercc1Δ mutant, respectively (Table 1). We previously showed that the APT mutation rate in WT was around 10−8 (Charlot et al., 2014), this analysis shows that the APT mutation rate is increased approximately 100-fold in the xpfΔ (2.5 × 10−6) and ercc1Δ (1.8 × 10−6) strains. These data support a direct involvement of the moss XPF-ERCC1 complex in repairing naturally occurring DNA damage to prevent the accumulation of mutations in the genome. Taken together the above data demonstrate that the moss XPF-ERCC1 complex plays an important role in the repair of both endogenous and exogenous DNA damage.
Gene Targeting Using an Ends-Out Construct Is Reduced in the xpfΔ and ercc1Δ Mutants
In yeast and mouse ES cells, the RAD1-RAD10 (or XPF-ERCC1) complex is involved in gene targeting using ends-out constructs (Niedernhofer et al., 2001; Langston and Symington, 2004, 2005). In order to investigate the involvement of the moss XPF-ERCC1 complex in gene targeting, we determined gene targeting rates in wild-type, xpfΔ and ercc1Δ mutants cells after transformation with an ends-out targeting substrate with homologous ends designed to inactivate the PpAPT gene and containing an hygromycin resistance cassette (PpAPT-KO2) (Figure 3). The gene targeting efficiency (GTE), determined as the frequency of 2-FA resistant plants amongst transformed plants (HygR) (Table 2), reaches 69.5% in the wild-type and is reduced by 1.7 and 1.5-folds in the xpfΔ, and ercc1Δ mutants respectively (Table 2, Fisher’s test p ≤ 0.003). These experiments demonstrate that PpXPF and PpERCC1 contribute to but are not essential for gene targeting.
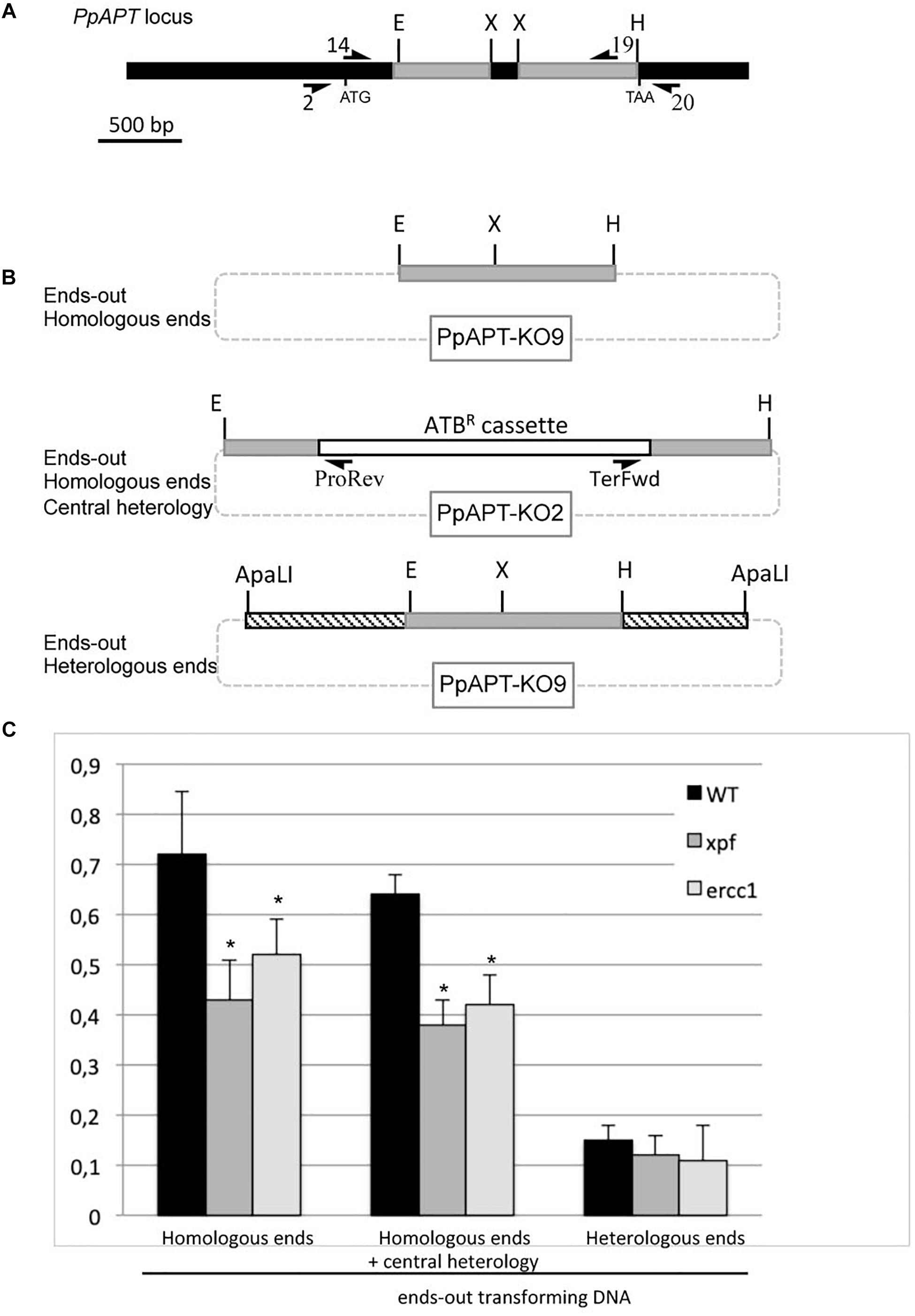
Figure 3. Gene targeting frequency in WT and xpf and ercc1 mutants using ends-out type vectors. (A) PpAPT WT locus. Regions targeted using ends-out or ends-in vectors are in gray. Primers used in this study are referenced in Supplementary Table S1. Different forms of the transforming DNA used. (B) Digestion of PpAPT-KO9 with EcoRI + HindIII give rise to ends-out transforming DNA, with double strand breaks at the edges of APT sequences. Digestion of PpAPT-KO2 with EcoRI + HindIII give rise to ends-out transforming DNA, with double strand breaks at the edges of APT sequences and a central heterologous region. Digestion of PpAPT-KO9 with ApaLI generates ends-out transforming DNA with heterologous sequence at the DSB (dashed bars: heterologous stretches, thin lines: plasmid sequences, dotted lines: plasmid sequences absent from the transforming DNA). (C) Gene targeting frequency of the APT gene in the wild type, and xpf and ercc1 mutants using different ends-out types transforming DNA. Asterisks indicate significant differences with the WT (Fisher exact test p-value ≤0.01).
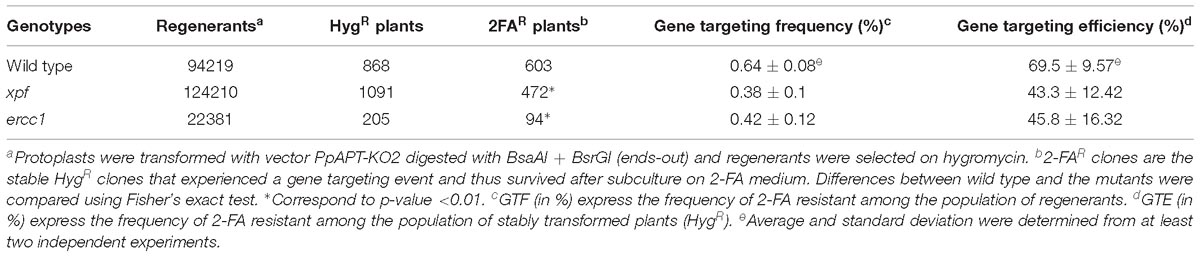
Table 2. Comparison of transformation and gene targeting efficiencies using an ends-out type targeting construct containing an heterologous selectable marker.
Gene Targeting Decrease in the xpfΔ and ercc1Δ Mutants Is Not Due to the Heterologous Selectable Marker
The linearized ends-out targeting constructs PpAPT-KO2 used in the previous experiment does not contain non-homologous tails at its 3′ ends. For this reason the decrease of GT in the xpfΔ or ercc1Δ mutants cannot be attributed to the role of the PpXPF-PpERCC1 complex in removing 3′ overhangs of non-homologous sequences as described for SSA or for targeted integration of ends-in targeting constructs in CHO cells. Nevertheless, in yeast and mouse the endonuclease complex has been shown to be also involved in the resolution of the large loop of mismatches that forms between the targeted gene and the central region of heterology corresponding to the selectable marker (Niedernhofer et al., 2001; Langston and Symington, 2004, 2005). In order to determine if the moss XPF-ERCC1 complex has a role in handling the heterology generated by the presence of the selectable marker (hygromycin resistance cassette in this case), GT frequencies (GTF) of an ends-out targeting substrate with homologous ends but lacking a selection marker (Figure 3, PpAPT-KO9 digested by EcoRI/HindIII), were measured. This substrate confers 2-FA resistance upon targeted integration at the PpAPT locus, generating a 159 bp deletion. The gene targeting frequency was determined as the frequency of 2-FA resistant plants amongst regenerated protoplasts (Table 2 and Supplementary Table S2). GTF observed in the wild type using this ends-out targeting construct is slightly but significantly higher (Fisher exact test p-value = 0.01) compared to GTF observed using the ends-out targeting construct containing the selection marker that creates a central region of heterology (Figure 3). GTFs observed using this ends-out targeting construct in the xpfΔ and ercc1Δ mutants were reduced by 1.7 and 1.4-fold respectively compared to wild type (Figure 3). This reduction is very similar to the one observed using the ends-out targeting construct containing the selectable marker. These results show that the ERCC1 and XPF proteins are necessary for gene targeting of an ends-out type targeting construct even in the absence of a large loop of mismatches in its central region.
Gene Targeting Using an Ends-In Construct Is Affected in the xpfΔ and ercc1Δ Mutants
In S. cerevisiae and in hamster cells (CHO), the RAD1-RAD10/XPF-ERCC1 is involved in removing long non-homologous tails from the 3′ ends of invading strands from ends-in gene targeting constructs (Schiestl and Prakash, 1988, 1990; Adair et al., 2000; Sargent et al., 2000). In Arabidopsis, XPF (RAD1) and ERCC1 (RAD10) have been shown to play a role in intermolecular recombination between plasmids by removing non-homologous 3′ ends from recombination intermediates (Dubest et al., 2002, 2004). In order to test the role of the moss XPF-ERCC1 complex in gene targeting using an ends-in targeting substrate with homologous ends GT frequencies (GTF) of an ends-in targeting construct (Figure 4, PpAPT-KO9 digested by XbaI), were measured in the wild type and xpfΔ or ercc1Δ mutants. GTF observed in the wild type using this ends-in targeting construct is slightly (1.2-fold) but significantly lower (Supplementary Table S2, Fisher exact test p-value = 0.014) compared to GTF observed using the ends-out targeting construct. GTF, reaches 0.59% in the wild-type and is reduced by 1.2 and 1.4-folds in the xpfΔ, and ercc1Δ mutants respectively (Figure 4 and Supplementary Table S2, Fisher’s test p-value ≤0.05). Use of an ends-in targeting substrate with heterologous ends strongly decrease the GTF in the wild type and the two mutants (Figure 4 and Supplementary Table S2). These results show that the ERCC1 and XPF proteins are also involved in gene targeting of an ends-in type targeting construct and that presence of heterologous sequences at the 5′ and 3′ extremities of this type of construct is very detrimental for the efficiency of gene targeting.
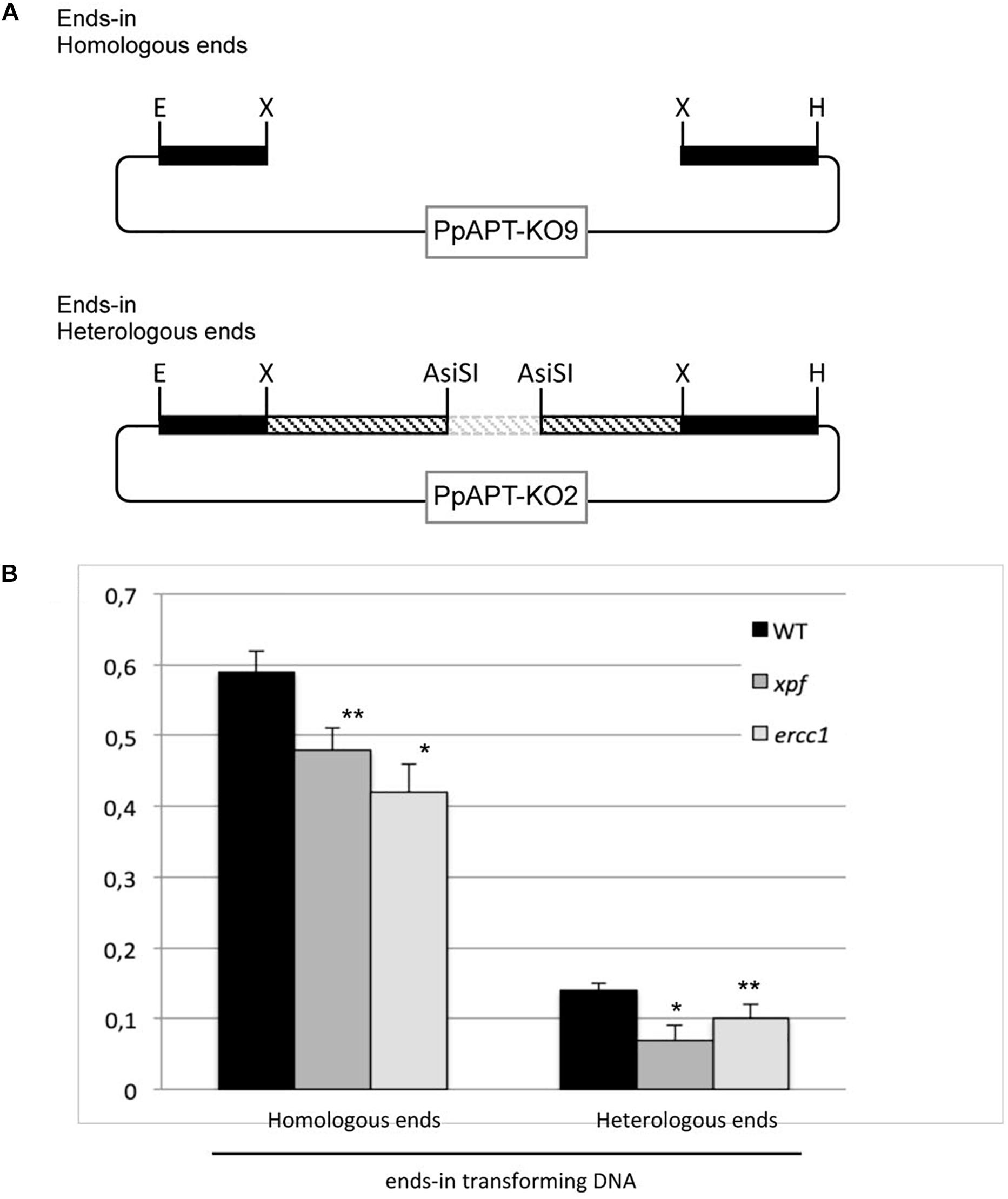
Figure 4. Gene targeting frequency in WT and xpf and ercc1 mutants using ends-in type vectors. (A) Different forms of the transforming DNA used. Digestion of PpAPT-KO9 with XbaI give rise to ends-in transforming DNA, with double strand breaks at the edges of APT sequences. Digestion of PpAPT-KO2 with AsiSI generates ends-in transforming DNA with heterologous sequence at the double strand breaks (dashed bars: heterologous stretches, thin lines: plasmid sequences, dotted lines: plasmid sequences absent from the transforming DNA). (B) Gene targeting frequency of the APT gene in the wild type, and xpf and ercc1 mutants using different ends-in types transforming DNA. Asterisks indicate significant differences with the WT (∗Fisher exact test p-value ≤0.01; ∗∗Fisher exact test p-value ≤0.05).
The Nature of Targeted Integration Is Modified in the xpfΔ and ercc1Δ Mutants
If, as in yeast, P. patens stable transformants can result from a double recombination at both ends of the ends-out type targeting fragment leading to TGR, another type of integration, named targeted gene insertions (TGI, Supplementary Figure S6), can be found in P. patens, like in ES cells (Kamisugi et al., 2006). In order to test the role of the PpXPF-PpERCC1 complex in the formation of TGI, we measured, using a PCR based approach, the ratio of targeted gene replacement (TGR) versus targeted gene insertion (TGI) in 2FAR plants obtained after transformation with the ends-out targeting substrate (PpAPT-KO2 digested EcoRI/HindIII, Table 2 and Figure 3) in the wild-type and xpf backgrounds. For the wild-type, 79% of targeted transformants were identified as TGR and 21% as TGI, while in the xpfΔ mutant, a statistically higher number of the transformants (Fisher’s exact test P = 0.005) were identified as TGR (93%) and 7% as TGI (Figure 5A). These findings show that XPF and probably the XPF-ERCC1 complex is an important factor in the mechanism leading to TGI in P. patens.
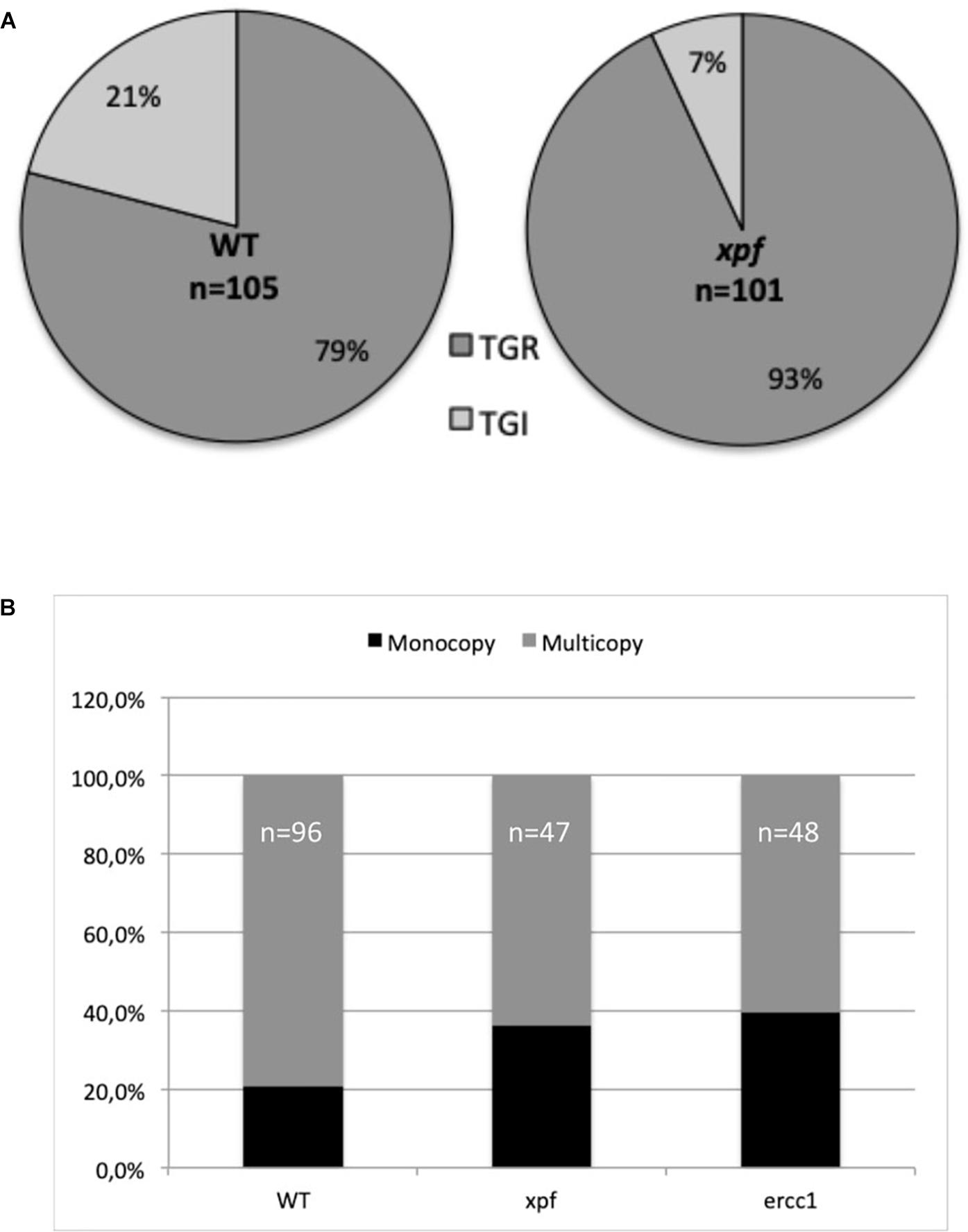
Figure 5. Type of integrations at the APT locus using an ends-out construct for the wild-type (WT) and mutants. (A) Rate of targeted gene replacement (TGR) vs. targeted gene insertion (TGI) integrations was estimated using primers specific to the PpAPT-KO2 cassette and primers located on the PpAPT gene but outside the genomic fragments present on the donor DNA cassette in WT and xpf mutant (see section “Materials and Methods,” Figure 3 and Supplementary Figure S6). (B) Rate of monocopy vs. multicopy insertions at the targeted locus was estimated in WT and xpf and ercc1 mutants, using primers located outside the sequences homologous to the gene fragments present in the PpAPT-KO9 donor DNA template (see section “Materials and Methods,” Figure 3 and Supplementary Figure S6).
Another marked difference between S. cerevisiae and P. patens concerning the type of integration is the fact that insertion of concatenated copies of the donor cassettes is frequent in GT experiments in P. patens (Supplementary Figure S6; Kamisugi et al., 2006). These concatenates result probably from episomally replicating DNA (Murén et al., 2009) a characteristic that have been used recently for complementation of an auxotrophic marker in P. patens (Ulfstedt et al., 2017). In order to clarify the role of the PpXPF-PpERCC1 complex in the formation of targeted gene replacement with head-to-tail multicopy, we measured, using a PCR based approach, the number of monocopy TGR (Supplementary Figure S6) in 2FAR plants obtained after transformation with the ends-out targeting substrate (PpAPT-KO9 digested EcoRI/HindIII, Figure 3) in the wild-type and mutants backgrounds. The percentage of monocopy integration in the WT and in the xpfΔ and ercc1Δ mutants was 21, 36, and 40% respectively (Figure 5B). Therefore the proportion of monocopy TGR is significantly more important in the xpfΔ and ercc1Δ mutants compared to the wild-type (chi-squared test P < 0.05).
We can conclude from these results that the nature of the targeted integrations is altered in these mutants. The PpERCC1 and PpXPF proteins are involved in the mechanism that results in the integration of the ends-out targeting construct via TGI and in the integration of concatemers. Nevertheless, TGI type integrations and concatemers integrations can be detected in the mutants contexts, implying that other nucleases can partially complement the absence of XPF and ERCC1 for these mechanisms in P. patens.
Discussion
We report here the identification and characterization of the P. patens mutant for the XPF and ERCC1 genes. The xpf and ercc1 P. patens mutants are viable and show no phenotypic defect under normal conditions, in agreement with what was observed for the Arabidopsis xpf and ercc1 mutants (Hefner et al., 2003; Preuss and Britt, 2003; Dubest et al., 2004), but in contrast with the situation observed in mammalian cells, where null mutants of the ERCC1 or XPF genes are lethal (McWhir et al., 1993; Núñez et al., 2000; Hsia et al., 2003; Tian et al., 2004). Like their Arabidopsis counterparts the xpf and ercc1 P. patens mutants are fully fertile suggesting that in plants, like in S. cerevisiae, this complex has only a minor role, if one, in meiosis which is in contrast with what is observed in Drosophila or C. elegans where the homologs of XPF1 have been shown to be involved in meiotic crossover formation (Sekelsky et al., 1995; Radford et al., 2005; Agostinho et al., 2013; O’Neil et al., 2013; Saito et al., 2013).
The P. patens xpf and ercc1 mutants present a strong increase in sensitivity to UV-B compared with the wild type. These results are consistent with previous studies on this complex in yeast (Prakash et al., 1993), animals (Gregg et al., 2011), and in Arabidopsis thaliana (Fidantsef et al., 2000; Liu et al., 2000; Hefner et al., 2003; Dubest et al., 2004; Biever et al., 2014) and confirm the requirement of the XPF-ERCC1 complex in the NER pathway in plants. Interestingly, the frequency of spontaneous mutations (mutator rate) in the P. patens xpf and ercc1 mutants is very high compared to wild type (100-fold increase) and is even higher than the one found in the rad51 mutant background, depleted for homologous recombination (Schaefer et al., 2010). This is reminiscent of what is observed in the S. cerevisiae rad1 mutant where an increases in the frequencies of single-base-pair substitution, single-base-pair deletion and insertion of the yeast retrotransposon Ty have been described (Kunz et al., 1990). It must be noticed that the fold increase in spontaneous mutations in the xpf/rad1 mutants backgrounds is significantly higher in P. patens compared to S. cerevisiae (Kunz et al., 1990; Doetsch et al., 2001). These data demonstrate the essential role of the XPF/ERCC1 complex in genome stability in P. patens and could potentially reflect a more prominent role of the NER pathway in genome stability in P. patens compared to S. cerevisiae. In addition, we have shown here that XPF-ERCC1 is also important for the response to MMS and the recent observation in P. patens that the rad51 mutant is more sensitive to MMS compared to WT (Goffová et al., 2019) reinforces the hypothesis that the HR machinery, and the XPF-ERCC1 complex would contribute to the repair of damages that would result from an MMS treatment, the exact nature of these damages being still unclear (Wyatt and Pittman, 2006). The role of the P. patens XPF/ERCC1 complex in these repair pathways could also be the cause of the important genetic instability observed in the corresponding mutants background.
There is good evidence for a role of XPF-ERCC1 in repair of double strand break through homologous recombination (Ahmad et al., 2008). We show here that the P. patens XPF-ERCC1 complex is involved in gene targeting using an ends-in construct and is also, and potentially even more important, for gene targeting using an ends-out construct. Concerning the ends-in construct the role of the P. patens XPF-ERCC1 complex could be, as in S. cerevisiae and in hamster cells (CHO), to remove the non-homologous tails from the 3′ ends of invading strands (Schiestl and Prakash, 1988, 1990; Adair et al., 2000; Sargent et al., 2000), reminiscent of the function of the endonuclease complex in SSA. It is more difficult to propose this function to explain the importance of the complex in gene targeting of the ends-out construct during TGR. This role of the XPF-ERCC1 complex for ends-out gene targeting in P. patens is of particular interest and should be consider in the light of other observations in other models. Indeed, in Arabidopsis ERCC1 has been proposed to be involved not only in SSA recombination as measured by a plasmid assay, but also in gene conversion/crossing over in chromosomal DNA (Dubest et al., 2004) and in mouse cells this complex is essential for ends-out gene targeting in the absence of non-homologous overhangs (Niedernhofer et al., 2001), implying a more general role for this endonuclease in recombination than the removal of non-homologous DNA overhangs from recombination intermediates. The endonuclease complex has also been shown to be important for TGR using ends-out targeting constructs in S. cerevisiae and separate studies have reported TGR efficiency decrease, ranging from a 3- to 40-fold reduction in rad1 and rad10 mutants (Schiestl and Prakash, 1988, 1990; Schiestl et al., 1994; Saparbaev et al., 1996; Symington et al., 2000; Langston and Symington, 2005). When using a classical ends-out gene targeting construct one possible role for the endonuclease could be the resolution of the large loop of mismatches that forms between the targeted gene and the heterologous selectable marker (that separate de 5′ and 3′ regions of homology of an ends-out construct) during the two ends invasion process of TGR (Niedernhofer et al., 2001; Langston and Symington, 2004, 2005). We could show here that the importance of the P. patens XPF-ERCC1 complex for targeted integration of the ends-out construct is not affected by the presence or the absence of an heterologous selectable marker.
In order to better understand the role of the P. patens XPF-ERCC1 complex in TGR using an ends-out construct we have compared the nature of the TGR events found in the wild type and in the mutants. As observed previously (Kamisugi et al., 2006), we could identify several types of gene targeting event (Supplementary Figure S6): (i) TGR, in which the targeted locus is replaced by a single copy of the transforming DNA (HR/HR) (ii) this may involve insertion of multiple copies (concatemers) of the targeting construct and (iii) “one-end gene targeting” or TGI, that may result from an homologous recombination event at one end of the construct accompanied by an apparent non-homologous end-joining event at the other (HR/NHEJ) (iv) this process may also involve insertion of multiple copies of the targeting construct. We could show here that the XPF-ERCC1 complex is more specifically involved in the mechanism that results in the integration of the targeting construct via TGI and in the integration of concatemers. The decrease in the number of concatemers and TGI events in the xpf or ercc1 mutants context could explain, at least for a part, the general decrease in gene targeting efficiency observed using a ends-out type construct in absence of the XPF-ERCC1 complex. One hypothesis to explain the role of the complex in the formation of TGI and concatemers events could be its potential role in the handling of the looped-out heteroduplex intermediates (Supplementary Figure S6) formed during the invasion process in presence of the concatemers that are produced before integration at the targeted APT locus. In this context, and taking into consideration our recent data showing the involvement of the POLQ protein in TGI events formation in P. patens (Mara et al., 2019), it would be interesting to check for a potential interaction between the XPF-ERCC1 complex and the Alt-EJ pathway for the targeted integration of ends-out construct in this moss. Such a cross-talk between the RAD1-RAD10 complex and the Alt-EJ repair pathway, that, like the SSA pathway, involves the removal of non-homologous 3′ tails, has already been proposed in yeast and more recently in animals (Ma et al., 2003; Sallmyr and Tomkinson, 2018).
We have shown here an essential role of the XPF-ERCC1 endonuclease complex in genetic stability of the model plant P. patens. Moreover, we have shown for the first time in plants, the implication of this endonuclease in gene targeting through ends-in or ends-out constructs. If the role of this complex in ends-in construct integration can be easily explained by the capacity of this endonuclease to remove non-homologous 3′ ends tails the exact role of the complex in integration of ends-out type constructs is still puzzling and further work is needed. Different functions of the XPF-ERCC1 complex and at different steps of the process of targeted integration of ends-out constructs could be involved. These functions could be shared by other organisms, like yeast and animal cells, where this endonuclease has also been shown to be important. However, more specific roles, due to specificities of the mechanism of targeted integration in the different species, could be involved. One of these could consist in the removal of the “apparent” heterologous regions formed during concatemers production in P. patens. Existence of this putative mechanism in other species, and especially in flowering plants is an open question and one must take into consideration that P. patens has an intrinsic high level of homologous recombination that could lead to functions of the XPF-ERCC1 complex that would be specific to this moss. Nevertheless, deciphering of the shared and specific roles of the XPF-ERCC1 complex in integration of the ends-out type constructs in different species is important to better understand the action of this endonuclease in genome maintenance and could have also potential applications in order to improve the efficiency and/or the quality of gene targeting for applied research.
Data Availability
All datasets generated for this study are included in the manuscript and/or the Supplementary Files.
Author Contributions
FN, DS, AG-D, and J-MN designed the research. AG-D and PR performed the research with the help of FC, AE, and DS. FN, AG-D, DS, and J-MN wrote the manuscript with contributions from all the authors.
Funding
This work was supported by the Agence Nationale de la Recherche (ANR-09-GENM-402 and ANR-11-BTBR-0001-GENIUS). The IJPB benefits from the support of the LabEx Saclay Plant Sciences-SPS (ANR-10-LABX-0040-SPS).
Conflict of Interest Statement
The authors declare that the research was conducted in the absence of any commercial or financial relationships that could be construed as a potential conflict of interest.
Supplementary Material
The Supplementary Material for this article can be found online at: https://www.frontiersin.org/articles/10.3389/fpls.2019.00588/full#supplementary-material
Footnotes
References
Adair, G. M., Rolig, R. L., Moore-Faver, D., Zabelshansky, M., Wilson, J. H., and Nairn, R. S. (2000). Role of ERCC1 in removal of long non-homologous tails during targeted homologous recombination. EMBO J. 19, 5552–5561. doi: 10.1093/emboj/19.20.5552
Adair, G. M., Scheerer, J. B., Brotherman, A., McConville, S., Wilson, J. H., and Nairn, R. S. (1998). Targeted recombination at the Chinese hamster APRT locus using insertion versus replacement vectors. Somat. Cell Mol. Genet. 24, 91–105. doi: 10.1023/b:scam.0000007112.62928.d8
Agostinho, A., Meier, B., Sonneville, R., Jagut, M., Woglar, A., Blow, J., et al. (2013). Combinatorial regulation of meiotic holliday junction resolution in C. elegans by HIM-6 (BLM) helicase, SLX-4, and the SLX-1, MUS-81 and XPF-1 nucleases. PLoS Genet. 9:e1003591. doi: 10.1371/journal.pgen.1003591
Ahmad, A., Robinson, A. R., Duensing, A., van Drunen, E., Beverloo, H. B., Weisberg, D. B., et al. (2008). ERCC1-XPF endonuclease facilitates DNA double-strand break repair. Mol. Cell. Biol. 28, 5082–5092. doi: 10.1128/MCB.00293-08
Al-Minawi, A. Z., Saleh-Gohari, N., and Helleday, T. (2008). The ERCC1/XPF endonuclease is required for efficient single-strand annealing and gene conversion in mammalian cells. Nucleic Acids Res. 36, 1–9. doi: 10.1093/nar/gkm888
Bardwell, A. J., Bardwell, L., Tomkinson, A. E., and Friedberg, E. C. (1994). Specific cleavage of model recombination and repair intermediates by the yeast Rad1-Rad10 DNA endonuclease. Science 265, 2082–2085. doi: 10.1126/science.8091230
Bhagwat, N., Olsen, A. L., Wang, A. T., Hanada, K., Stuckert, P., Kanaar, R., et al. (2009). XPF-ERCC1 participates in the fanconi anemia pathway of cross-link repair. Mol. Cell. Biol. 29, 6427–6437. doi: 10.1128/MCB.00086-09
Biever, J. J., Brinkman, D., and Gardner, G. (2014). UV-B inhibition of hypocotyl growth in etiolated Arabidopsis thaliana seedlings is a consequence of cell cycle arrest initiated by photodimer accumulation. J. Exp. Bot. 65, 2949–2961. doi: 10.1093/jxb/eru035
Bonhomme, S., Nogué, F., Rameau, C., and Schaefer, D. G. (2013). Usefulness of Physcomitrella patens for studying plant organogenesis. Methods Mol. Biol. 959, 21–43. doi: 10.1007/978-1-62703-221-6_2
Charlot, F., Chelysheva, L., Kamisugi, Y., Vrielynck, N., Guyon, A., Epert, A., et al. (2014). RAD51B plays an essential role during somatic and meiotic recombination in Physcomitrella. Nucleic Acids Res. 42, 11965–11978. doi: 10.1093/nar/gku890
Ciccia, A., McDonald, N., and West, S. C. (2008). Structural and functional relationships of the XPF/MUS81 family of proteins. Annu. Rev. Biochem. 77, 259–287. doi: 10.1146/annurev.biochem.77.070306.102408
Collonnier, C., Epert, A., Mara, K., Maclot, F., Guyon-Debast, A., Charlot, F., et al. (2017). CRISPR-Cas9-mediated efficient directed mutagenesis and RAD51-dependent and RAD51-independent gene targeting in the moss Physcomitrella patens. Plant Biotechnol. J. 15, 122–131. doi: 10.1111/pbi.12596
Dehé, P.-M., and Gaillard, P.-H. L. (2017). Control of structure-specific endonucleases to maintain genome stability. Nat. Rev. Mol. Cell Biol. 18, 315–330. doi: 10.1038/nrm.2016.177
Doetsch, P. W., Morey, N. J., Swanson, R. L., and Jinks-Robertson, S. (2001). Yeast base excision repair: interconnections and networks. Prog. Nucleic Acid Res. Mol. Biol. 68, 29–39. doi: 10.1016/s0079-6603(01)68087-5
Dubest, S., Gallego, M. E., and White, C. I. (2002). Role of the AtRad1p endonuclease in homologous recombination in plants. EMBO Rep. 3, 1049–1054. doi: 10.1093/embo-reports/kvf211
Dubest, S., Gallego, M. E., and White, C. I. (2004). Roles of the AtErcc1 protein in recombination. Plant J. 39, 334–342. doi: 10.1111/j.1365-313x.2004.02136.x
Faridounnia, M., Folkers, G., and Boelens, R. (2018). Function and interactions of ERCC1-XPF in DNA damage response. Molecules 23:3205. doi: 10.3390/molecules23123205
Fidantsef, A. L., Mitchell, D. L., and Britt, A. B. (2000). The Arabidopsis UVH1 gene is a homolog of the yeast repair endonuclease RAD1. Plant Physiol. 124, 579–586. doi: 10.1104/pp.124.2.579
Fishman-Lobell, J., and Haber, J. E. (1992). Removal of nonhomologous DNA ends in double-strand break recombination: the role of the yeast ultraviolet repair gene RAD1. Science 258, 480–484. doi: 10.1126/science.1411547
Goffová, I., Vágnerová, R., Peška, V., Franek, M., Havlová, K., Holá, M., et al. (2019). Roles of RAD51 and RTEL1 in telomere and rDNA stability in Physcomitrella patens. Plant J [Epub ahead of print].
Gregg, S. Q., Robinson, A. R., and Niedernhofer, L. J. (2011). Physiological consequences of defects in ERCC1-XPF DNA repair endonuclease. DNA Repair. 10, 781–791. doi: 10.1016/j.dnarep.2011.04.026
Hanin, M., Volrath, S., Bogucki, A., Briker, M., Ward, E., and Paszkowski, J. (2001). Gene targeting in Arabidopsis. Plant J. 28, 671–677. doi: 10.1046/j.1365-313x.2001.01183.x
Hastings, P. J., McGill, C., Shafer, B., and Strathern, J. N. (1993). Ends-in vs. Ends-out recombination in yeast. Genetics 135, 973–980.
Hefner, E., Preuss, S. B., and Britt, A. B. (2003). Arabidopsis mutants sensitive to gamma radiation include the homologue of the human repair gene ERCC1. J. Exp. Bot. 54, 669–680. doi: 10.1093/jxb/erg069
Hsia, K.-T., Millar, M. R., King, S., Selfridge, J., Redhead, N. J., Melton, D. W., et al. (2003). DNA repair gene Ercc1 is essential for normal spermatogenesis and oogenesis and for functional integrity of germ cell DNA in the mouse. Development 130, 369–378. doi: 10.1242/dev.00221
Ivanov, E. L., and Haber, J. E. (1995). RAD1 and RAD10, but not other excision repair genes, are required for double-strand break-induced recombination in Saccharomyces cerevisiae. Mol. Cell. Biol. 15, 2245–2251. doi: 10.1128/mcb.15.4.2245
Kamisugi, Y., Cuming, A. C., and Cove, D. J. (2005). Parameters determining the efficiency of gene targeting in the moss Physcomitrella patens. Nucleic Acids Res. 33:e173. doi: 10.1093/nar/gni172
Kamisugi, Y., Schaefer, D. G., Kozak, J., Charlot, F., Vrielynck, N., Holá, M., et al. (2012). MRE11 and RAD50, but not NBS1, are essential for gene targeting in the moss Physcomitrella patens. Nucleic Acids Res. 40, 3496–3510. doi: 10.1093/nar/gkr1272
Kamisugi, Y., Schlink, K., Rensing, S. A., Schween, G., von Stackelberg, M., Cuming, A. C., et al. (2006). The mechanism of gene targeting in Physcomitrella patens: homologous recombination, concatenation and multiple integration. Nucleic Acids Res. 34, 6205–6214. doi: 10.1093/nar/gkl832
Klein, H. L. (1988). Different types of recombination events are controlled by the RAD1 and RAD52 genes of Saccharomyces cerevisiae. Genetics 120, 367–377.
Kofuji, R., and Hasebe, M. (2014). Eight types of stem cells in the life cycle of the moss Physcomitrella patens. Curr. Opin. Plant Biol. 17, 13–21. doi: 10.1016/j.pbi.2013.10.007
Kunz, B. A., Kohalmi, L., Kang, X., and Magnusson, K. A. (1990). Specificity of the mutator effect caused by disruption of the RAD1 excision repair gene of Saccharomyces cerevisiae. J. Bacteriol. 172, 3009–3014. doi: 10.1128/jb.172.6.3009-3014.1990
Langston, L. D., and Symington, L. S. (2004). Gene targeting in yeast is initiated by two independent strand invasions. Proc. Natl. Acad. Sci. U.S.A. 101,15392–15397. doi: 10.1073/pnas.0403748101
Langston, L. D., and Symington, L. S. (2005). Opposing roles for DNA structure-specific proteins Rad1, Msh2, Msh3, and Sgs1 in yeast gene targeting. EMBO J. 24, 2214–2223. doi: 10.1038/sj.emboj.7600698
Liu, Z., Hossain, G. S., Islas-Osuna, M. A., Mitchell, D. L., and Mount, D. W. (2000). Repair of UV damage in plants by nucleotide excision repair: Arabidopsis UVH1 DNA repair gene is a homolog of Saccharomyces cerevisiae Rad1. Plant J. 21, 519–528. doi: 10.1046/j.1365-313x.2000.00707.x
Ma, J., Kim, E. M., Haber, J. E., and Lee, S. E. (2003). Yeast Mre11 and Rad1 proteins define a Ku-independent mechanism to repair double-strand breaks lacking overlapping end sequences. Mol. Cell. Biol. 23, 8820–8828. doi: 10.1128/mcb.23.23.8820-8828.2003
Manandhar, M., Boulware, K. S., and Wood, R. D. (2015). The ERCC1 and ERCC4 (XPF) genes and gene products. Gene 569, 153–161. doi: 10.1016/j.gene.2015.06.026
Mara, K., Charlot, F., Guyon-Debast, A., Schaefer, D. G., Collonnier, C., Grelon, M., et al. (2019). POLQ plays a key role in repair of CRISPR/Cas9 induced double strand breaks in the moss Physcomitrella patens. New Phytol. 222, 1380–1391. doi: 10.1111/nph.15680
McWhir, J., Selfridge, J., Harrison, D. J., Squires, S., and Melton, D. W. (1993). Mice with DNA repair gene (ERCC-1) deficiency have elevated levels of p53, liver nuclear abnormalities and die before weaning. Nat. Genet. 5, 217–224. doi: 10.1038/ng1193-217
Murén, E., Nilsson, A., Ulfstedt, M., Johansson, M., and Ronne, H. (2009). Rescue and characterization of episomally replicating DNA from the moss Physcomitrella. Proc. Natl. Acad. Sci. U.S.A. 106, 19444–19449. doi: 10.1073/pnas.0908037106
Niedernhofer, L. J., Essers, J., Weeda, G., Beverloo, B., de Wit, J., Muijtjens, M., et al. (2001). The structure-specific endonuclease Ercc1-Xpf is required for targeted gene replacement in embryonic stem cells. EMBO J. 20, 6540–6549. doi: 10.1093/emboj/20.22.6540
Núñez, F., Chipchase, M. D., Clarke, A. R., and Melton, D. W. (2000). Nucleotide excision repair gene (ERCC1) deficiency causes G(2) arrest in hepatocytes and a reduction in liver binucleation: the role of p53 and p21. FASEB J. 14, 1073–1082. doi: 10.1096/fasebj.14.9.1073
Odahara, M., Inouye, T., Fujita, T., Hasebe, M., and Sekine, Y. (2007). Involvement of mitochondrial-targeted RecA in the repair of mitochondrial DNA in the moss, Physcomitrella patens. Genes Genet. Syst. 82, 43–51. doi: 10.1266/ggs.82.43
Odahara, M., and Sekine, Y. (2018). RECX interacts with mitochondrial RECA to maintain mitochondrial genome stability. Plant Physiol. 177, 300–310. doi: 10.1104/pp.18.00218
O’Neil, N. J., Martin, J. S., Youds, J. L., Ward, J. D., Petalcorin, M. I. R., Rose, A. M., et al. (2013). Joint molecule resolution requires the redundant activities of MUS-81 and XPF-1 during Caenorhabditis elegans meiosis. PLoS Genet. 9:e1003582. doi: 10.1371/journal.pgen.1003582
Pâques, F., and Haber, J. E. (1997). Two pathways for removal of nonhomologous DNA ends during double-strand break repair in Saccharomyces cerevisiae. Mol. Cell. Biol. 17, 6765–6771. doi: 10.1128/mcb.17.11.6765
Prado, F., and Aguilera, A. (1995). Role of reciprocal exchange, one-ended invasion crossover and single-strand annealing on inverted and direct repeat recombination in yeast: different requirements for the RAD1, RAD10, and RAD52 genes. Genetics 139, 109–123.
Prakash, S., Sung, P., and Prakash, L. (1993). DNA repair genes and proteins of Saccharomyces cerevisiae. Annu. Rev. Genet. 27, 33–70. doi: 10.1146/annurev.genet.27.1.33
Preuss, S. B., and Britt, A. B. (2003). A DNA-damage-induced cell cycle checkpoint in Arabidopsis. Genetics 164, 323–334.
Radford, S. J., Goley, E., Baxter, K., McMahan, S., and Sekelsky, J. (2005). Drosophila ERCC1 is required for a subset of MEI-9-dependent meiotic crossovers. Genetics 170, 1737–1745. doi: 10.1534/genetics.104.036178
Rahn, J. J., Rowley, B., Lowery, M. P., Coletta, L. D., Limanni, T., Nairn, R. S., et al. (2011). Effects of varying gene targeting parameters on processing of recombination intermediates by ERCC1-XPF. DNA Repair. 10, 188–198. doi: 10.1016/j.dnarep.2010.10.011
Saffran, W. A., Greenberg, R. B., Thaler-Scheer, M. S., and Jones, M. M. (1994). Single strand and double strand DNA damage-induced reciprocal recombination in yeast. Dependence on nucleotide excision repair and RAD1 recombination. Nucleic Acids Res. 22, 2823–2829. doi: 10.1093/nar/22.14.2823
Saito, T. T., Lui, D. Y., Kim, H. M., Meyer, K., and Colaiácovo, M. P. (2013). Interplay between structure-specific endonucleases for crossover control during caenorhabditis elegans meiosis. PLoS Genet. 9:e1003586. doi: 10.1371/journal.pgen.1003586
Sallmyr, A., and Tomkinson, A. E. (2018). Repair of DNA double-strand breaks by mammalian alternative end-joining pathways. J. Biol. Chem. 293, 10536–10549. doi: 10.1074/jbc.TM117.000375
Saparbaev, M., Prakash, L., and Prakash, S. (1996). Requirement of mismatch repair genes MSH2 and MSH3 in the RAD1-RAD10 pathway of mitotic recombination in Saccharomyces cerevisiae. Genetics 142, 727–736.
Sargent, R. G., Meservy, J. L., Perkins, B. D., Kilburn, A. E., Intody, Z., Adair, G. M., et al. (2000). Role of the nucleotide excision repair gene ERCC1 in formation of recombination-dependent rearrangements in mammalian cells. Nucleic Acids Res. 28, 3771–3778. doi: 10.1093/nar/28.19.3771
Sargent, R. G., Rolig, R. L., Kilburn, A. E., Adair, G. M., Wilson, J. H., and Nairn, R. S. (1997). Recombination-dependent deletion formation in mammalian cells deficient in the nucleotide excision repair gene ERCC1. Proc. Natl. Acad. Sci. U.S.A. 94, 13122–13127. doi: 10.1073/pnas.94.24.13122
Schaefer, D. G. (2002). A new moss genetics: targeted mutagenesis in Physcomitrella patens. Annu. Rev. Plant Biol. 53, 477–501. doi: 10.1146/annurev.arplant.53.100301.135202
Schaefer, D. G., Delacote, F., Charlot, F., Vrielynck, N., Guyon-Debast, A., Le Guin, S., et al. (2010). RAD51 loss of function abolishes gene targeting and de-represses illegitimate integration in the moss Physcomitrella patens. DNA Repair. 9, 526–533. doi: 10.1016/j.dnarep.2010.02.001
Schaefer, D. G., and Zrÿd, J. P. (1997). Efficient gene targeting in the moss Physcomitrella patens. Plant J. 11, 1195–1206. doi: 10.1046/j.1365-313x.1997.11061195.x
Schiestl, R. H., and Prakash, S. (1988). RAD1, an excision repair gene of Saccharomyces cerevisiae, is also involved in recombination. Mol. Cell. Biol. 8, 3619–3626. doi: 10.1128/mcb.8.9.3619
Schiestl, R. H., and Prakash, S. (1990). RAD10, an excision repair gene of Saccharomyces cerevisiae, is involved in the RAD1 pathway of mitotic recombination. Mol. Cell. Biol. 10, 2485–2491. doi: 10.1128/mcb.10.6.2485
Schiestl, R. H., Zhu, J., and Petes, T. D. (1994). Effect of mutations in genes affecting homologous recombination on restriction enzyme-mediated and illegitimate recombination in Saccharomyces cerevisiae. Mol. Cell. Biol. 14, 4493–4500. doi: 10.1128/mcb.14.7.4493
Schwartz, E. K., and Heyer, W.-D. (2011). Processing of joint molecule intermediates by structure-selective endonucleases during homologous recombination in eukaryotes. Chromosoma 120, 109–127. doi: 10.1007/s00412-010-0304-7
Sekelsky, J. J., McKim, K. S., Chin, G. M., and Hawley, R. S. (1995). The drosophila meiotic recombination gene mei-9 encodes a homologue of the yeast excision repair protein Rad1. Genetics 141, 619–627.
Symington, L. S., and Gautier, J. (2011). Double-strand break end resection and repair pathway choice. Annu. Rev. Genet. 45, 247–271. doi: 10.1146/annurev-genet-110410-132435
Symington, L. S., Kang, L. E., and Moreau, S. (2000). Alteration of gene conversion tract length and associated crossing over during plasmid gap repair in nuclease-deficient strains of Saccharomyces cerevisiae. Nucleic Acids Res. 28, 4649–4656. doi: 10.1093/nar/28.23.4649
Tian, M., Shinkura, R., Shinkura, N., and Alt, F. W. (2004). Growth retardation, early death, and DNA repair defects in mice deficient for the nucleotide excision repair enzyme XPF. Mol. Cell. Biol. 24, 1200–1205. doi: 10.1128/mcb.24.3.1200-1205.2004
Tripsianes, K., Folkers, G., Ab, E., Das, D., Odijk, H., Jaspers, N. G. J., et al. (2005). The structure of the human ERCC1/XPF interaction domains reveals a complementary role for the two proteins in nucleotide excision repair. Structure 13, 1849–1858. doi: 10.1016/j.str.2005.08.01
Trouiller, B., Charlot, F., Choinard, S., Schaefer, D. G., and Nogué, F. (2007). Comparison of gene targeting efficiencies in two mosses suggests that it is a conserved feature of Bryophyte transformation. Biotechnol. Lett. 29, 1591–1598. doi: 10.1007/s10529-007-9423-5
Trouiller, B., Schaefer, D. G., Charlot, F., and Nogué, F. (2006). MSH2 is essential for the preservation of genome integrity and prevents homeologous recombination in the moss Physcomitrella patens. Nucleic Acids Res. 34,232–242. doi: 10.1093/nar/gkj423
Ulfstedt, M., Hu, G.-Z., Johansson, M., and Ronne, H. (2017). Testing of auxotrophic selection markers for use in the moss physcomitrella provides new insights into the mechanisms of targeted recombination. Front. Plant Sci. 8:1850. doi: 10.3389/fpls.2017.01850
Waterworth, W. M., Drury, G. E., Bray, C. M., and West, C. E. (2011). Repairing breaks in the plant genome: the importance of keeping it together. New Phytol. 192, 805–822. doi: 10.1111/j.1469-8137.2011.03926.x
Wendeler, E., Zobell, O., Chrost, B., and Reiss, B. (2015). Recombination products suggest the frequent occurrence of aberrant gene replacement in the moss Physcomitrella patens. Plant J. 81, 548–558. doi: 10.1111/tpj.12749
Wiedemann, G., van Gessel, N., Köchl, F., Hunn, L., Schulze, K., Maloukh, L., et al. (2018). RecQ helicases function in development, DNA repair, and gene targeting in Physcomitrella patens. Plant Cell 30, 717–736. doi: 10.1105/tpc.17.00632
Wolter, F., Klemm, J., and Puchta, H. (2018). Efficient in planta gene targeting in Arabidopsis using egg cell-specific expression of the Cas9 nuclease of Staphylococcus aureus. Plant J. 94, 735–746. doi: 10.1111/tpj.13893
Woodrick, J., Gupta, S., Camacho, S., Parvathaneni, S., Choudhury, S., Cheema, A., et al. (2017). A new sub-pathway of long-patch base excision repair involving 5’ gap formation. EMBO J. 36, 1605–1622. doi: 10.15252/embj.201694920
Keywords: XPF-ERCC1, gene targeting, Physcomitrella patens, DNA repair, recombination
Citation: Guyon-Debast A, Rossetti P, Charlot F, Epert A, Neuhaus J-M, Schaefer DG and Nogué F (2019) The XPF-ERCC1 Complex Is Essential for Genome Stability and Is Involved in the Mechanism of Gene Targeting in Physcomitrella patens. Front. Plant Sci. 10:588. doi: 10.3389/fpls.2019.00588
Received: 07 March 2019; Accepted: 18 April 2019;
Published: 09 May 2019.
Edited by:
Kaoru Okamoto Yoshiyama, Tohoku University, JapanReviewed by:
Karel J. Angelis, Institute of Experimental Botany (ASCR), CzechiaVasilissa Ivanova Manova, Institute of Plant Physiology and Genetics (BAS), Bulgaria
Copyright © 2019 Guyon-Debast, Rossetti, Charlot, Epert, Neuhaus, Schaefer and Nogué. This is an open-access article distributed under the terms of the Creative Commons Attribution License (CC BY). The use, distribution or reproduction in other forums is permitted, provided the original author(s) and the copyright owner(s) are credited and that the original publication in this journal is cited, in accordance with accepted academic practice. No use, distribution or reproduction is permitted which does not comply with these terms.
*Correspondence: Fabien Nogué, ZmFiaWVuLm5vZ3VlQGlucmEuZnI=