- 1Department of Plant Physiology, Poznań University of Life Sciences, Poznań, Poland
- 2Department of Plant Ecophysiology, Faculty of Biology, Adam Mickiewicz University in Poznań, Poznań, Poland
- 3Department of Plant Anatomy and Cytology, Faculty of Biology and Environmental Protection, The University of Silesia in Katowice, Katowice, Poland
We provide evidence that alterations in DNA methylation patterns contribute to the regulation of stress-responsive gene expression for an intergenerational resistance of β-aminobutyric acid (BABA)-primed potato to Phytophthora infestans. Plants exposed to BABA rapidly modified their methylation capacity toward genome-wide DNA hypermethylation. De novo induced DNA methylation (5-mC) correlated with the up-regulation of Chromomethylase 3 (CMT3), Domains rearranged methyltransferase 2 (DRM2), and Repressor of silencing 1 (ROS1) genes in potato. BABA transiently activated DNA hypermethylation in the promoter region of the R3a resistance gene triggering its downregulation in the absence of the oomycete pathogen. However, in the successive stages of priming, an excessive DNA methylation state changed into demethylation with the active involvement of potato DNA glycosylases. Interestingly, the 5-mC–mediated changes were transmitted into the next generation in the form of intergenerational stress memory. Descendants of the primed potato, which derived from tubers or seeds carrying the less methylated R3a promoter, showed a higher transcription of R3a that associated with an augmented intergenerational resistance to virulent P. infestans when compared to the inoculated progeny of unprimed plants. Furthermore, our study revealed that enhanced transcription of some SA-dependent genes (NPR1, StWRKY1, and PR1) was not directly linked with DNA methylation changes in the promoter region of these genes, but was a consequence of methylation-dependent alterations in the transcriptional network.
Introduction
Plants exposed to adverse environmental conditions are forced to develop multiple and diverse strategies to sense the stress and mount defense responses against it. Defense priming is postulated as the physiological state of a plant where adaptive responses, initiated by various stimuli, trigger its metabolism so that the plant responds more effectively when second and more severe stress is encountered (Prime-A-Plant Group et al., 2006). It has been well documented that the non-protein amino acid, namely β-aminobutyric acid (BABA), effectively mediates the conditioning of induced plant defense mechanisms leading to enhanced protection against various pathogens (Cohen et al., 2016). Although BABA pre-treatment or other stimuli may prime a plant against future stress (Floryszak-Wieczorek et al., 2015; Meller et al., 2018), there is also evidence that such stress memory establishment is a relatively rare event or priming is reset during the period of stress recovery (Zimmerli et al., 2000; Pecinka et al., 2009; Crisp et al., 2016; Hilker et al., 2016).
The duration of the priming memory may often be short (days or weeks), but in some cases, it may extend to the first stress-free generation (F1), designated as “intergenerational memory,” or to at least two stress-free generations (F2), designated as “transgenerational memory” (Lämke and Bäurle, 2017). Recent findings revealed that primed parent could transmit to its offspring an improved response to potential future stress, in a process that is of epigenetic nature (Avramova, 2015; Conrath et al., 2015; Mauch-Mani et al., 2017).
Epigenetic regulation of gene expression relies on DNA methylation, small RNA activity and histone modifying complexes, which regulate chromatin structure. Alterations in DNA methylation have been suggested to be involved in the process of heritable stress adaptation and correlate with changes in stress-responsive gene expression (Zhu et al., 2015; Crisp et al., 2016; Seidl et al., 2016). DNA methylation (5-mC) is a type of epigenetic mark that modifies chromatin structure and gene expression, resulting often (but not always) in compacted chromatin and transcriptional gene silencing (TGS). It is well known that DNA methylation in plants is found in all cytosine sequence contexts (CG, CHG, CHH; where H = A, C or T) and the different types may serve as different functions. DNA methylation is relatively stable and may be maintained during cell division and differentiation. The transposon silencing and prevention of paramutation are essential to ensure cell function. However, under unfavorable conditions, DNA methylation patterns might be prone to changes, which may contribute to the exhibition of new resistance traits, or may give rise to abnormalities in the patterns of 5-mC associated with plant diseases. The genome-wide methylation pattern is dynamically regulated by three independent processes: maintenance of methylation, de novo methylation and DNA demethylation (Zhang et al., 2018).
An important component in the activated methyl cycle (AMC) determining the cellular methylation potential under normal and stress conditions is S-adenosyl-L-homocysteine hydrolase (SAHH), which catalyzes the reversible hydrolysis of S-adenosylhomocysteine (SAH) to adenosine and homocysteine (Palmer and Abeles, 1979). Subsequently, homocysteine is then converted, through methionine, to S-adenosylmethionine (SAM) and acts as a methyl donor for methyltransferases (MTs) in trans-methylation reactions of various acceptors, i.e., DNA, proteins and lipids. The regeneration of SAM from methionine, driven by SAM-synthase (SAMS), is the last step of the AMC cycle. However, several lines of evidence have indicated that SAHH plays a key role in the maintenance of the methylation potential under physiological or pathological conditions through regulating the inter- and intra-cellular SAH/SAM ratio (Rahikainen et al., 2018).
Plant DNA methyltransferases (DRMs) are grouped into three families: CG methylation is mediated mainly by Methyltransferases (METs), CG and CHG methylation is regulated by Chromodomain methyltransferases or CMTs, respectively, while CHH methylation is mainly catalyzed by Domains Rearranged DRMs. De novo DNA methylation is predominantly controlled in Arabidopsis by the RNA-directed DNA methylation (RdDM) pathway, in which methylated siRNAs are loaded onto ARGONAUTE4 (AGO4) to form the RNA-induced silencing complex that promotes recruitment of DRM2 to the target locus (Henderson et al., 2010; Saze et al., 2012; Matzke and Mosher, 2014). The current state of DNA methylation patterns under normal or stress circumstances is often the effect of cooperation or competition of these three methyltransferases and the RdDM pathway with the DNA demethylation machinery (Lei et al., 2015).
The active DNA demethylation in Arabidopsis is performed by four DNA glycosylases, DEMETER (DME), DME-like (DML2 and DML3) and Repressor of silencing (ROS1), with the latter to be preferentially involved in counteracting de novo DNA methylation established by the RdDM pathway (Zhu, 2009; López Sánchez et al., 2016).
Plants under stress have to balance between the stable and flexible DNA methylation status tuned with the transcriptional repressive or active state of stress-responding genes. The concept of memory-type transcription during repeated stresses indicates that sometimes the information on priming is stored in the form of epigenetic marks which contribute to the regulation of subsequent transcription of salicylic acid (SA) dependent stress-related genes (Pecinka et al., 2009; Jaskiewicz et al., 2011; Avramova, 2015). An elevated level of SA, a key component of defense-related signal transduction, induces SA-dependent changes that are mainly controlled by the central immune regulator Non-expressor of Pathogenesis-Related Genes 1 (NPR1). The NPR1 as a transcription coactivator, upon interaction with transcription factors TGA and WRKY (recognizing the W-box regulatory element), is required for SA-dependent regulation of Pathogenesis-Related (PR) gene expression and the establishment of the priming state (Fu and Dong, 2013). The StWRKY1 transcription factor is associated with potato immunity to Phytophthora infestans, the causal agent of late blight disease of potato, and the StWRKY1-silenced potato leaves exhibited an increase in the pathogen biomass and correlated with a lower level of late blight resistance (Yogendra et al., 2015).
In turn, genome instability connected with the altered DNA methylation status in the promoter regions of respective resistance (R) gene clusters could contribute to phenotypic plasticity in response to pathogens promoting the formation of new patterns of resistance genes regulation (Alvarez et al., 2010; Spoel and Dong, 2012). The group of the R genes coding for the nucleotide-binding site, leucine-rich repeat (NBS-LRR) proteins, that recognize pathogen effectors, is one of the largest families found to be regulated by micro RNAs (miRNAs) that trigger secondary siRNA production (Richard et al., 2018). At least 11 race-specific late blight R genes, deriving from Solanum demissum have been incorporated into various potato cultivars, belong to the family of NBS-LRR encoding resistance genes (Vleeshouwers et al., 2011). The R3a resistance gene has been widely introduced into potato cultivars and it is essential for plant immunity by encoding R3a protein recognizing the P. infestans effector Avr3a (Bos et al., 2010).
It was generally accepted that host plant harboring specific R genes encode proteins recognizing the corresponding pathogen-produced effector proteins (avirulence factors or Avr) and as a result inducing effector-triggered immunity (ETI) in the form of a strong defense response. In turn, the recognition of the host plant conserved pathogen-associated molecular patterns (PAMPs) results in PAMP-triggered immunity (PTI), which elicits a “general defense response” or basal immunity. Because pathogens have evolved a broad range of effectors to dampen PAMP-triggered signals and attenuate PTI, this type of defense response is much weaker than ETI (Pedley and Martin, 2003; Jones and Dangl, 2006).
The priming stimulus or pathogen ingress typically involves host genome hyper- or hypomethylation, which can influence the biogenesis of small RNAs, together with the expression of the R genes (Alvarez et al., 2010; Weiberg and Jin, 2015). However, to date, no model has been proposed for the DNA methylation state associated with its silencing or activating role in the expression of stress-responsive genes. In rice, chemically induced hypomethylation revealed suppression of R gene silencing (Xa21G) concomitant with enhanced and heritable resistance to Xanthomonas oryzae pv. oryzae (Akimoto et al., 2007). Reduction in the DNA methylation level may affect the stability of the R genes belonging to the NBS-LRR defense gene family; members of the family are clustered in chromosome regions abundant in repetitive sequences and transposon elements (TEs), rich in repressive chromatin modifications, such as DNA and H3K9me3 methylation (Saze et al., 2012; Du et al., 2015). Together with small RNAs, these epigenetic modifications preserve transcriptionally silent chromatin and prevent TEs expression. Transcription of the R genes needs to be strictly controlled to avoid inappropriate activation, since redundant R gene expression, in the absence of the pathogen, could affect plant growth and development (Xia et al., 2013). In turn, after pathogen recognition some 5-mC marks may be erased from these regions that can promote TEs activation, while the R genes (although not all) are moderately upregulated, presumably for pathogen recognition, amplify immune signals and enhance or reset resistance against the aggressor (Navarro et al., 2004).
The global genome methylation level significantly varies between species, ranging from 6–14% of total cytosines in Arabidopsis, 20% in wheat, 25% in maize, to more than 35% in potato (Kakutani et al., 1999; Steward et al., 2002; Xin et al., 2015; Viggiano and de Pinto, 2017). Surprisingly, the few organisms lacking an efficient 5-mC DNA methylation system include Drosophila (<0.0002%), Caenorhabditis elegans, yeast and oomycetes (Capuano et al., 2014). P. infestans is a member of the oomycetes, a group of organisms phylogenetically separated from fungi and grouped with the golden-brown algae in the stramenopiles (Burki et al., 2007). In this study, the changes in the DNA methylation status of potato in its interaction with P. infestans were examined.
Recently we found that the offspring of BABA-primed potato cv. Sarpo Mira maintained a higher resistance to virulent P. infestans MP977 over one BABA-untreated generation. The H3K4me2 epigenetic mark was identified in high numbers on the gene body of StWRKY1, PR1, and PR2 concomitant with transcript downregulation detected in leaves of primed potato and its descendants before the triggering stress. Moreover, our previous study revealed that the primed state in potato was associated with the transcriptional memory to post-infection histone acetyltransferase (HAT) activation in the next generation after the challenge inoculation with P. infestans (Meller et al., 2018). In the present study, with the same experimental design as mentioned above, we investigated how DNA methylation and demethylation in potato contribute to BABA-primed changes associated with transcriptional memory to improve resistance against the virulent P. infestans MP977.
Materials and Methods
Plant Material and BABA Treatment
Plants of the tetraploid potato cv. Sarpo Mira came from the Potato Genebank (Plant Breeding and Acclimatization Institute – IHAR-PIB in Bonin, Poland). Potato cultivar Sarpo Mira (carrying the R genes: R3a, R3b, R4, Rpi-Smira1, and Rpi-Smira2) is highly resistant to avr P. infestans. Potato seedlings, propagated through in vitro culture, were transferred to the soil and were grown to the stage of ten leaves in a phytochamber with 16 h of light (180 μmol⋅m-2⋅s-1) at 18 ± 2°C and 60% humidity. Then the plants were immunized by spraying leaves with 5 mM of BABA (5 ml per plant). The non-immunized plants were sprayed with water. Three days after BABA treatment (at 72 h) potato plants were challenge inoculated with virulent (vr) P. infestans (isolate MP977). Plants were kept for 24 h at 100% humidity and 18°C to facilitate disease development and then transferred to normal growth conditions. Leaves were collected at 1, 3, 6, 24 and 48 h after P. infestans challenge inoculation and frozen in liquid nitrogen until analysis.
The F1 plants, i.e., vegetative (from tubers) and generative (from seeds) progeny, were obtained from non-primed and BABA-primed selfed parental plants (F0). According to the experimental design (Supplementary Figure S1), the parental line (F0) was treated once with 5 mM BABA and the progeny (F1) was only inoculated with P. infestans MP977 at the 10 compound leaf stage.
Pathogen Culture and Plant Inoculation
The vr P. infestans (Mont.) de Bary isolate MP977 (A1 mating type, race 1.2.3.4.6.7.10) and the avirulent P. infestans isolate MP946 (A1 mating type, race 1.3.4.7.10.11) were kindly provided by the Plant Breeding Acclimatization Institute (IHAR), Młochów Research Centre, Poland. The MP946 avirulent isolate of P. infestans encoding AvrR3a in the presence of the corresponding R3a gene product of cv. Sarpo Mira elicits ETI, a strong response associated with the hypersensitive reaction (HR) in the host plant, which prevents pathogen colonization. In contrast, the virulent isolate MP977 of P. infestans triggers PTI, resulting in late blight symptom development in cv. Sarpo Mira. The pathogen was grown on a rye medium (Caten and Jinks, 1968) and transferred through a potato tuber two times before infection. Sporangia of P. infestans were obtained by washing 14-day-old cultures with cold distilled water. Zoospores were released by incubating the sporangia in water at 4°C for 2 h. Potato plants were inoculated by spraying 3 ml of a freshly isolated suspension of sporangia and zoospores with approximately 2.5 × 105 sporangia per ml. The mock-inoculated plants were sprayed with water.
Quantification of P. infestans
The P. infestans translation elongation factor 1a (Pitef1) gene, a single copy constitutively expressed gene, was used to quantify P. infestans in inoculated potato leaves using RT-qPCR analysis. For all the samples, the transcript levels of the Pitef1 gene were calculated relative to the expression levels of the potato ef1α and 18S rRNA (van’t Klooster et al., 2000; Orłowska et al., 2012).
Assessment of Disease Development
Disease symptoms were determined at day 5 after inoculation (dpi), based on the estimation of trypan-blue stained leaves (Wilson and Coffey, 1980) inoculated with zoospore suspension. Imaging was performed by scanning leaf discs with a LIDE 210 Scanner (Canon). The blue stain corresponded to the area covered by P. infestans mycelium and was analyzed using the ImageJ 1.47v open source software (Wayne Rasband National Institutes of Health, United States).
Global 5-mC Detection
Global 5-mC detection was performed with the 5-mC ELISA kit (Zymo Research, United States) according to the manufacturer’s protocol. All DNA samples were isolated from 200 mg of frozen leaf tissue using the Wizard® Genomic DNA Purification Kit (Promega, United States) following the manufacturer’s protocol.
Imaging of DNA Methylation
The immunostaining procedure was carried out as described by Braszewska-Zalewska et al. (2014). All probes were fixed in 3:1 methanol/glacial acetic acid to detect DNA methylation, subsequently macerated in 20% pectinase (Sigma Aldrich) and 2% cellulase (Onozuka) in 0.01 mM sodium citrate buffer (pH 4.8). After digestion, the material was washed again with sodium citrate buffer. Then the vascular bundles and the epidermis were removed. Cell suspensions were transferred to fresh 1.5 ml tubes and centrifuged (10000× g). The supernatant was removed and cells were washed with 45% acetic acid, centrifuged and suspended in acetic acid and heated up to 65°C for a minute. Next, the cells were transferred to a glass slide, covered with a coverslip and frozen in liquid nitrogen for 30 s. After freezing and removal of the coverslips, the slides were dried. Global cytosine methylation was immunodetected with mouse antibodies raised against 5-mC (Abcam; 1:200 in 1% BSA in 1 × PBS). Samples were denatured in 70% formamide in 2 × SSC for 2 min at 70°C. Then samples were dehydrated in 70 and 100% ethyl alcohol for 5 min and air-dried. Next, probes were blocked with 5% BSA and incubated with the primary antibody at 37°C for 1 h and afterward, they were washed in PBS and incubated with the secondary antibody (Alexa Fluor488 goat anti-mouse IgG; Invitrogen, Molecular Probes) applied under the same conditions. Chromosomes were counterstained with 2.5 mg/ml 4′,6-diamino-2-phenylindole (DAPI, Sigma Aldrich) in the Vectashield (Vector Laboratories). The quantitative acquisition and analysis were performed using a high-content screening system (ScanˆR, Olympus) based on a wide-field Olympus IX81 microscope, equipped with a CCD ORCA-ER camera (Hamamatsu Photonics, Japan) and an MT20 illumination system based on a 150-W xenon-mercury lamp. Automated segmentation of nuclei was based on threshold values (a border value of the fluorescence intensity of pixels between the background and the object). ImageJ (ver.1.52a1) was performed assuming the following parameters of fluorescence intensities: “total” (the sum of pixel intensity divided by the area of the object) and “mean” (total intensity divided by the area of the object). Normality of the signal intensity was assessed for each analyzed group (the Chi-square goodness-of-fit test, p<0.05). As all the samples were large (N = 780 to 3,870), the t-test for independent statistical samples was used to verify significant differences between the control and the treated samples.
Gene Expression Analysis
Gene expression analysis was performed for SAMS and SAHH that constitute important elements of the plant activated cellular methyl cycle (AMC). Maintenance of the DNA methylation/demethylation status of the potato genome was verified by transcript abundance analyses for the following DRMs MET1, CMT3, DRM2 and demethylases StDML2 (ortholog to Arabidopsis At3g47830) and ROS1. Moreover, RT-qPCR analysis was carried out for the key potato resistance R3a gene and SA-dependent NPR1, StWRKY1, PR1 stress-responding genes. RNA from 150 mg of frozen leaf tissue was extracted using the TRI Reagent® (Sigma, United States). DNA was removed using a Deoxyribonuclease Kit (Sigma, United States) and reverse transcription of 1 μg RNA was performed using a Reverse Transcription Kit (Thermo Fisher Scientific, United States). The RT-qPCR assays were performed applying the following conditions: 10 min at 95°C, followed by 35 cycles of 10 s at 95°C, 5 s at the annealing temperature of each specific primer (Supplementary Table S1) and 10 s at 72°C. The reaction mixture contained 0.1 μM of each primer, 1 μl of 5 × diluted cDNA, 10 μl of the Power SYBR Green PCR Master mix (Applied Biosystems, United States) and DEPC treated water to the total volume of 20 μl. The PicoReal Thermocycler (Thermo Fisher Scientific, United States) was used for RT-qPCR analyses. Primers were designed based on the available databases of NCBI (National Center of Biotechnology Information, United States) or PGSC (Potato Genome Sequencing Consortium) using the Primer-Blast software and are listed in Supplementary Table S1. The data were normalized to the reference genes, namely the elongation factor ef1α (AB061263) and the 18S rRNA (X67238). The Ct values were determined with the use of a Real-time PCR Miner (Zhao and Fernald, 2005) and the relative gene expression was calculated with the use of the efficiency corrected calculation models proposed by Pfaffl (2001) and Tichopad et al. (2004).
Evaluation of Specific DNA Methylation Levels Using Methylation-Sensitive High Resolution Melting (MS-HRM) Analysis
High-Resolution Melting (HRM) PCR is a commonly used method in genotyping or single nucleotide polymorphism detection (Simko, 2016). In combination with bisulfite treatment (methylation sensitive HRM, MS-HRM), where conversion of non-methylated cytosine to uracil causes changes in the DNA melting curve, HRM technique may be applied to detect qualitative changes in DNA methylation of targeted sequences both in human cancer diagnostic research (Wojdacz et al., 2008) and in plants (Tricker et al., 2012). Potato DNA (1 μg) was treated with sodium bisulfite using the EZ DNA Methylation-Gold Kit (Zymo Research, United States). Primers specific to bisulfite-converted antisense DNA were selected to amplify a region of a CpG island in the promoter regions for the NPR1, PR1, R3a and StWRKY1 genes using the MethPrimer 2.0 software. The gene sequences were obtained from the NCBI database (Supplementary Table S2). MS-HRM was performed in 20 μl reaction mixtures containing 10 μl of 2 × Luminaris Color HRM Master Mix, 200 ng of bisulfite-treated DNA, and 0.5 μM of each primer. MS-HRM was initiated by incubation for 5 min at 95°C to denature the DNA, followed by 50 cycles of 5 s at 95°C, 10 s at the annealing temperature of each specific primer and 15 s at 72°C. Fluorescence was measured on a PicoReal Thermocycler once per cycle to monitor template melting during a linear temperature transition from 65 to 95°C at 0.1°C/s. Fluorescence data were converted into melting peaks by the PicoReal software (ver. 2.2) to plot the negative derivative of fluorescence over temperature vs. temperature (-dF/dT vs. T) (Supplementary Table S1). The control was selected as a reference curve, while the difference between each curve and the reference was plotted against temperature to give a “difference curve” plot (Wittwer et al., 2003). The original reference curve became a horizontal line at zero, while the differentially methylated genotypes clustered along different paths to facilitate visual discrimination with the positive/negative values on the Y-axis for mean hypermethylation/hypomethylation, respectively. From the obtained data mean and standard deviations were calculated for each temperature point.
Statistical Analysis
All the results were based on three independent experiments, each with at least three biological replicates. For each experiment, means of the obtained values were calculated along with standard deviations. The analysis of variance was conducted and the least significant differences (LSDs) between means were determined using Tukey’s test at the level of significance α = 0.05 and 0.01.
Results
BABA-Primed Protection Against Late Blight Disease
The durability of BABA-primed resistant responses in potato cv. Sarpo Mira was evaluated on the basis of pathogen gene expression in the inoculated parental line and the vegetative progeny of primed plants derived from tubers and progeny derived from seeds. The BABA-priming efficiency as expressed by the transcript level of Pitef1 and cytochemical assay also corresponded to the reduced level of leaf colonization by P. infestans, as described previously (Meller et al., 2018). BABA treatment, prior to the challenge inoculation, resulted in a more pronounced resistance against late blight disease in potato (F0), since a 4-fold lower level of Pitef1 transcription at 48 hpi was observed when compared to unprimed inoculated plants (Figure 1). Descendants of BABA-primed potato (F1), grown from tubers, showed approx. 2.5-fold reduction in Pitef1 transcript accumulation at 48 hpi. Intergenerational resistance against P. infestans MP977 was also found in the progeny obtained from seeds (F1). The protection of this generation was reflected in the Pitef1 transcript being approx. 2-fold lower at 48 hpi than in unprimed inoculated plants. The less of pathogen biomass in the F0 and F1 lines of BABA-primed plants positively correlated with reduced disease symptoms detected by trypan blue staining of P. infestans mycelium (Figure 1). Our results revealed that potato exposed to BABA displayed enhanced resistance to P. infestans and produced offspring that were more resistant against the late blight disease than those from unprimed plants regardless of the means of reproduction.
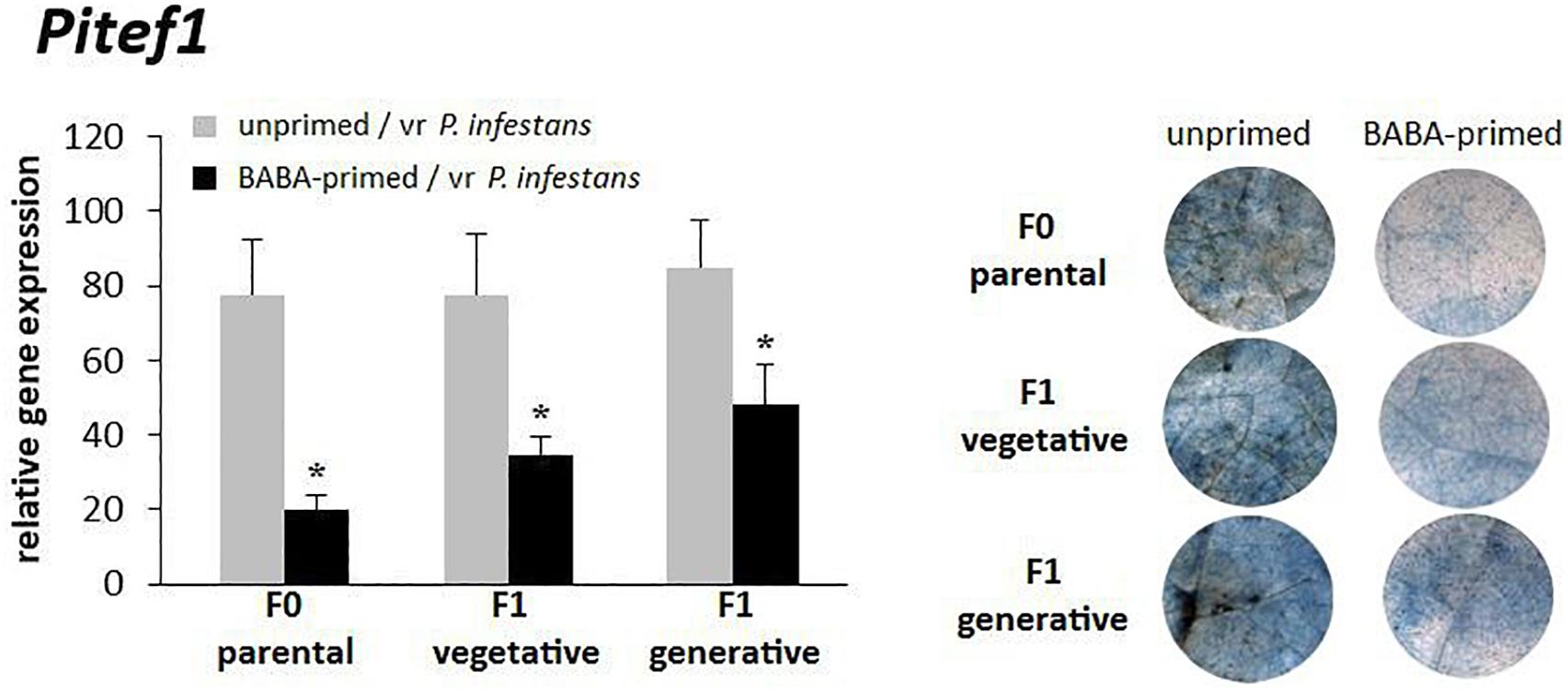
Figure 1. Intergenerational resistance of potato against P. infestans. BABA-induced resistance against P. infestans in the parental line (F0); intergenerational resistance in the vegetative F1 progeny and the generative F1 progeny. Three days after BABA treatment potato leaves were challenged with P. infestans and Pitef1 gene expression at 48 hpi and trypan-blue stained leaves at day 5 (dpi) were analyzed. Values represent means of at least three independent experiments, each with at least three biological replicates. Asterisks (∗) indicate values that differ significantly from unprimed and vr P. infestans-inoculated potato leaves at α<0.05, respectively.
BABA Treatment Is Effective in Modifying the Cellular Methylation Potential
To examine whether the primed state providing better stress resistance in potato and its progeny, was due to the modification of the DNA methylation status, we first examined how BABA might alter gene expression of SAMS and SAHH, both being important components of the plant activated cellular methyl cycle.
The obtained data clearly indicated that BABA at a concentration of 5 mM enhanced SAHH transcription level starting from 1 to 24 h and slightly intensified SAMS expression at the same time after BABA supplied (Figures 2A,B). In turn, primed potato exposed to P. infestans MP977 inoculation showed abundant transcript accumulation mainly for SAMS (peaking at 6 hpi after pathogen attack) (Figures 2A,B). It cannot be excluded that the increase of SAMS expression after inoculation was a result of higher expression of SAHH and a consequently larger production of SAM precursors that had to be processed. Moreover, our experiment revealed that BABA-primed rather indirect epigenetic effect, through modulation of the SAHH/SAM ratio, caused instability in the cellular methylation potential with rather an opposite trend in changes upon challenge inoculation.
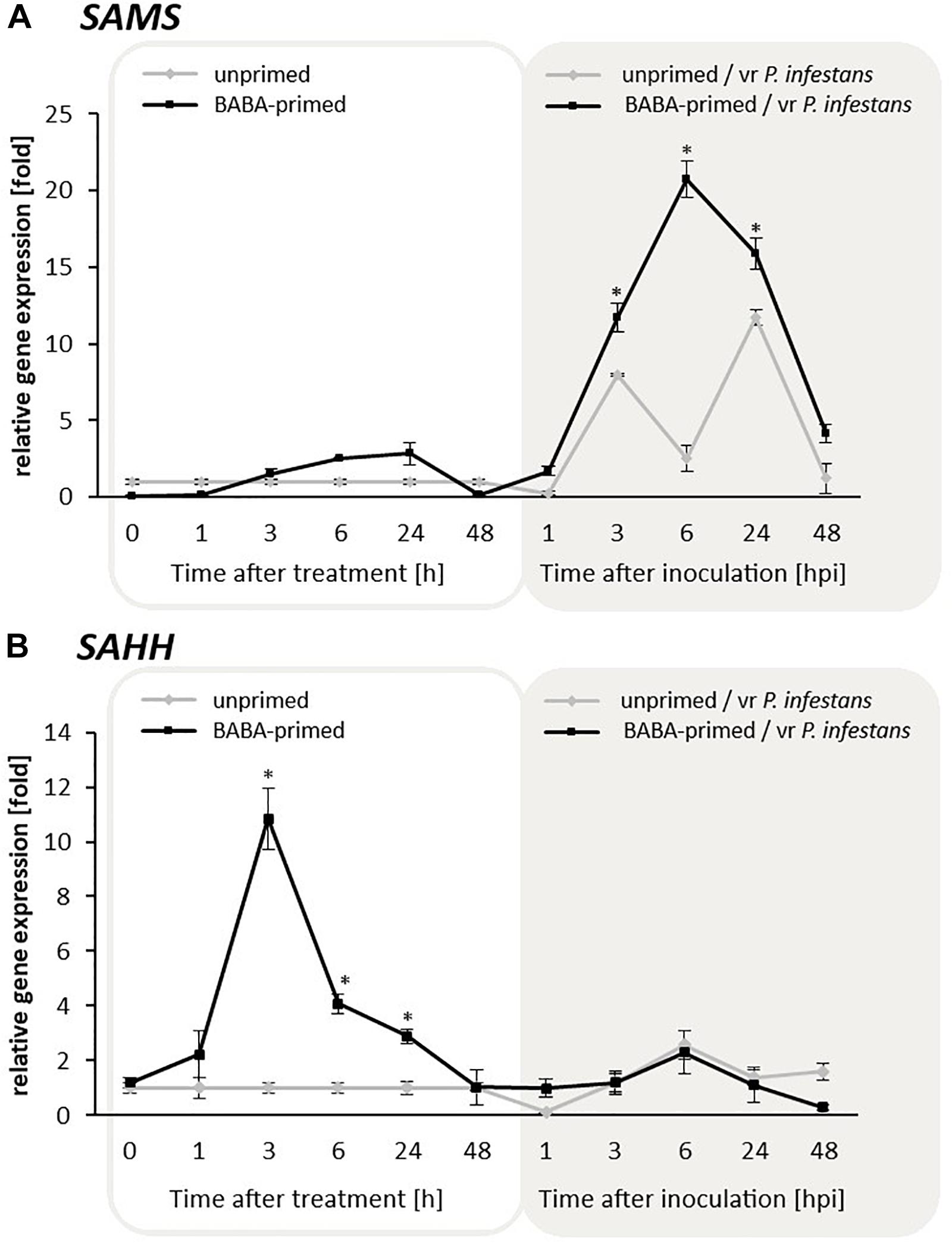
Figure 2. Expression patterns of SAMS (A) and SAHH (B) contributing to the potato cellular methylation potential in response to BABA treatment and challenge inoculation with P. infestans MP977 (3 days after BABA treatment). Analyses were performed at 1–48 h after 5 mM BABA exposure (white background) and 1–48 hpi after challenge inoculation with vr P. infestans (gray background). Light lines refer to unprimed and dark lines to primed plants. Values represent mean ± SD of at least three independent experiments. Asterisks (∗) indicate values that differ significantly from unprimed (water treated) or unprimed and P. infestans inoculated potato leaves at α<0.05, respectively.
DNA Methylation/Demethylation Genes Are Involved in the BABA-Primed Defense
Since DNA methylome plasticity is due to the presence of various enzymes modifying DNA methylation patterns, we first analyzed DRMs to investigate a potential association between cytosine methylation and transcription upon BABA-priming. RT-qPCR assays of MET1, required for global cytosine methylation maintenance in plants, did not show significant changes in its expression either after the BABA treatment or challenge inoculation. Thus, MET1 activity did not seem to be important for DNA reprogramming methylation induced by BABA (Figure 3A).
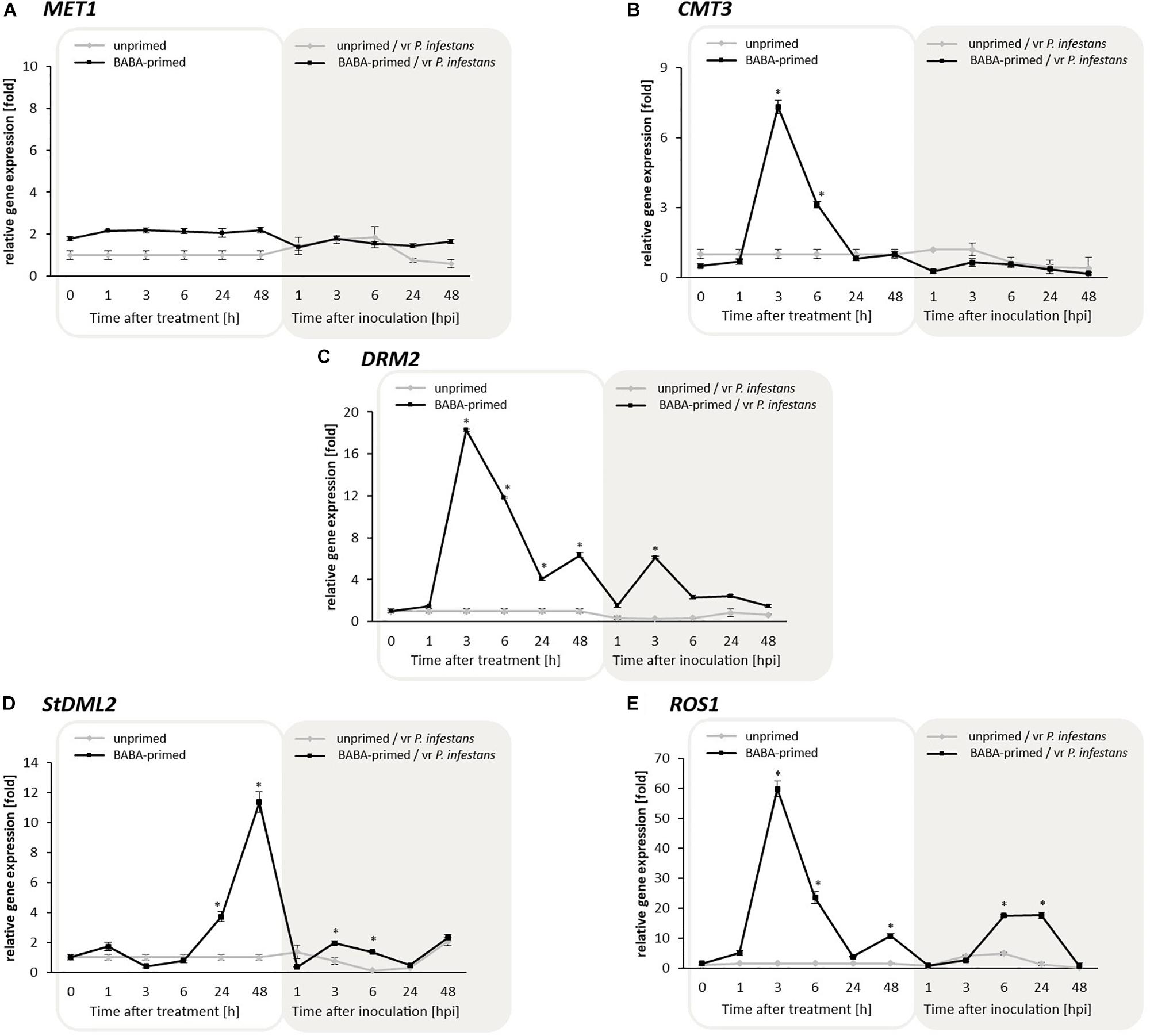
Figure 3. Transcriptional changes in potato DNA methylating genes MET1 (A), CMT3 (B), DRM2 (C); and DNA demethylating genes StDML2 (D), and ROS1 (E) in response to BABA and challenge inoculation with vr P. infestans MP977 (3 days after BABA treatment). Analyses were performed at 1–48 h after 5 mM BABA treatment (white background) and 1–48 hpi after challenge inoculation with P. infestans (gray background). Light lines and dark lines refer to unprimed and primed plants, respectively. Values represent mean ± SD of at least three independent experiments. Asterisks (∗) indicate values that differ significantly from unprimed (water-treated) or unprimed and P. infestans inoculated potato leaves at α<0.05 (∗), respectively.
In turn, an enhanced CMT3 gene expression (7-fold increase) was found at 3 h upon BABA treatment (Figure 3B). Interestingly, the 18-fold increase in DRM2 transcription was recorded at the same time after inducer exposure (Figure 3C). This data indicated the essential role and involvement of CMT3 and DRM2 in de novo DNA methylation associated with BABA priming.
Then we focused on the role of active DNA demethylation in BABA priming, driven by DNA glycosylase, namely DEMETER-like DNA demethylase (At3g47830), referred to as StDML2, and the Repressor of silencing 1 (ROS1) antagonists of RdDM to prevent DNA hypermethylation at specific loci. ROS1 underwent a strong 60- and 20-fold transcriptional increase at 3 and 6 h after BABA treatment, respectively; in the following hours the expression rapidly diminished (Figure 3E). Interestingly, ROS1 transcript level showed time-dependent kinetics similar to that of DRM2 expression upon BABA treatment and challenge inoculation with vr P. infestans, which confirmed their functional cooperation both controlled by RdDM. At the later phase of priming (from 24 to 48 h) an overproduction (12-fold increase) of StDML2 transcript was observed, suggesting active demethylation of the potato DNA methylome (Figure 3D). Obtained results revealed that BABA-induced wave of DNA methylation followed by removal of this methylation mark might change the target genomic region of key importance for long-lasting priming for defense.
Primed Potato Plants Exhibit a Shift in Global DNA Methylation Levels
To evaluate whether the genome-wide methylation status of potato was modified by BABA we performed global genome-methylation analyses using immunocytochemical (ICC) and ELISA immunoassay, as well as MS-HRM analysis in order to determine methylation patterns of the promoter region in the pathogen-responsive genes.
Global DNA Methylation Changes
The 5-mC DNA ELISA assay revealed that the DNA methylation status of potato leaves increased upon BABA treatment since it was found to be 2-fold higher (from 1 to 6 h); then it diminished slightly in the following hours (Figure 4A). This indicates that during the first 6 h after BABA treatment the DNA methylation increased to 80% (hypermethylation state), followed by a decrease to 35% of 5-mC. Upon the challenge with P. infestans a loss in potato DNA methylation was noted both in primed and unprimed potato plants subjected to P. infestans inoculation.
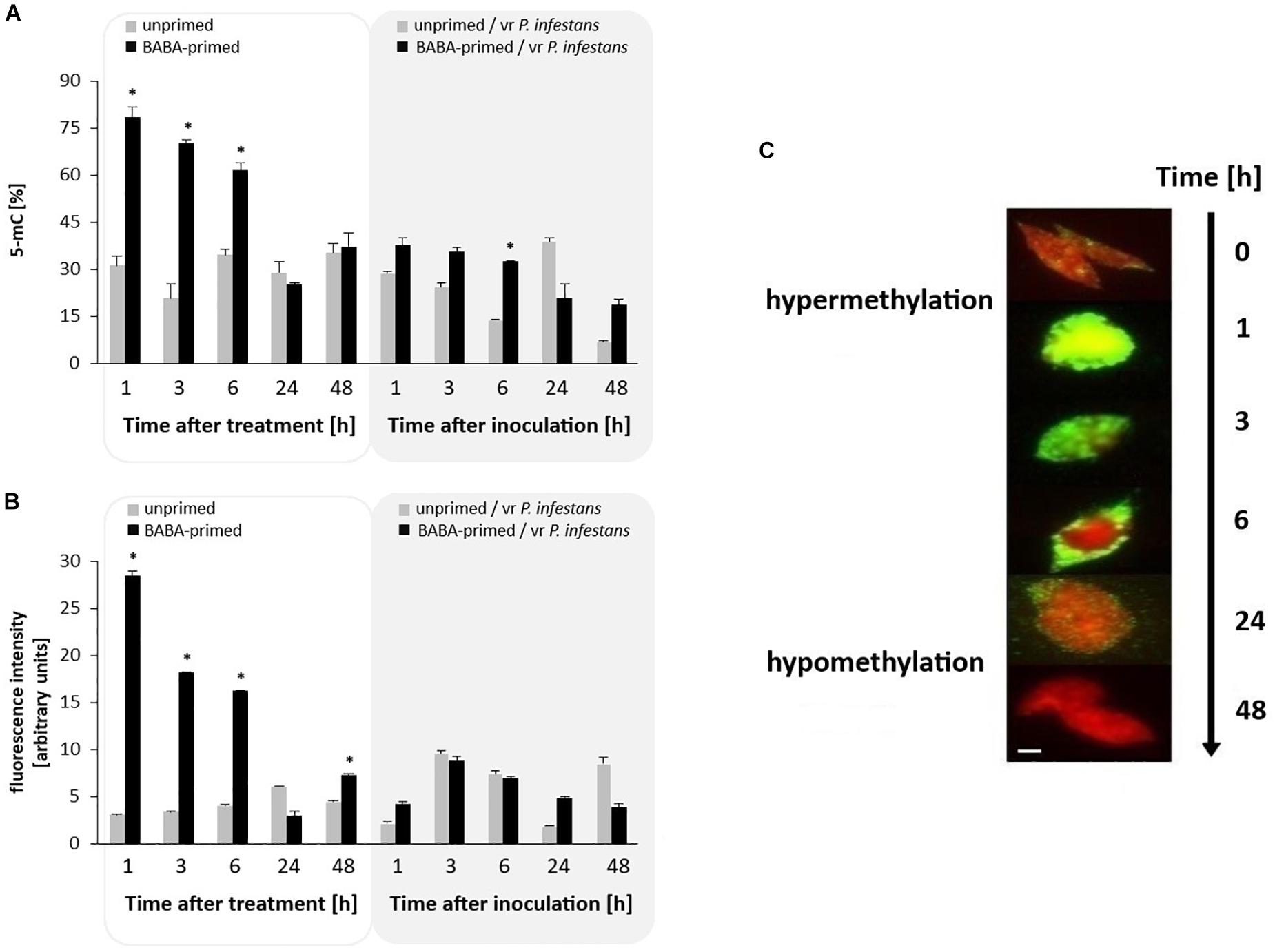
Figure 4. Global potato genome methylation in response to BABA treatment and challenge inoculation with P. infestans MP977 (at 72 hrs after BABA treatment). ELISA 5-mC assay (A); Immunodetection of 5-mC estimated as Alexa Fluor488 fluorescence intensity (B). Analyses were performed at 1–48 h after BABA (5 mM) treatment (white background) and 1–48 hpi (gray background) after challenge inoculation; the immunostaining pattern of representative nuclei at 0–48 h after BABA treatment (C). The red color: DAPI staining (indicating lack of methylation); the green color: methylated DNA immunodetected with anti-5-mC antibody and Alexa Fluor488 goat anti-mouse IgG. The fluorescence signals for nuclei and 5-mC were pseudocolored red and green, respectively, using the ImageJ program. Scale bar = 5 μm. Light columns and dark columns refer to unprimed and primed plants, respectively. The negative control was provided by immunostaining without the secondary or primary antibody. In both cases, we observed no fluorescence of Alexa Fluor488 on the slide of leaf nuclei, which means that the antibodies were specific and showed no unspecific binding. Values represent mean ± SD of at least three independent experiments. Asterisks (∗) indicate values that differ significantly from unprimed (water treated) or unprimed and P. infestans inoculated potato leaves at α<0.05, respectively.
Nuclei Methylation Changes
The immunostaining patterns corresponding to 5-mC were investigated in the nuclei of potato leaf tissue. BABA pretreatment was associated with early and drastic hypermethylation (5-fold increase) from 1 to 6 h, followed by a rapid hypomethylation of DNA in potato leaf nuclei when compared to unprimed plants (Figures 4B,C and Supplementary Figure S2). The highest level of this modification, visible as green nuclei (corresponding to Alexa488 fluorescence), was observed at 1 h post BABA treatment; in contrast, the lowest level of 5-mC methylation was detected at 24 h since nuclei were visible red (corresponding to DAPI fluorescence). After pathogen ingress, the observed level of methylation in nuclei slightly differed in immunofluorescence intensity between the P. infestans-challenged and BABA-primed inoculated leaf tissue at various time points after disease development (Figure 4B).
Stress-Responsive Gene Expression and Induced DNA Methylation in Promoter
DNA Methylation Does Not Influence the Transcription of SA-Dependent Genes
To evaluate the methylation patterns in the promoter regions of selected SA-dependent defense genes, i.e., NPR1, StWRKY1, and PR1, upon the BABA-inducible DNA methylation we employed MS-HRM analyses. Potato exposure to BABA caused time-dependent changes in the methylation levels of the NPR1 promoter. Initially, BABA induced a rapid increase in DNA methylation of the NPR1 promoter at 3 h (5–6% rise in fluorescence intensity), followed by a decrease at 48 h, reaching similar levels as in the unprimed potato (Figures 5A,B and Supplementary Figures S3A,B). Unexpectedly, the high 5-mC level of the NPR1 promoter at 3 h did not correlate with the high NPR1 gene expression upon BABA treatment (Figure 5C). At the later time point (48 h) the induced 5-mC mark level at the NPR1 promoter region reached the unprimed level since the transcript abundance of NPR1 (6–48 h) was comparable to that in the unprimed potato. The StWRKY1 promoter was partially methylated (6 h) based on the MS-HRM analysis and was not sufficiently effective in reducing StWRKY1 transcription (Figures 6A,B and Supplementary Figure S3C). Our data showed an enhanced expression of NPR1 and the StWRKY1 transcription factor during the onset of the priming state that was accompanied by methylated patterns of the promoter regions of these pivotal genes for potato defense. MS-HRM analysis showed that the methylation state of the PR1 promoter did not alter upon BABA exposure, whereas a higher accumulation of the PR1 transcript (6 h) was found at 3 h (Figures 7A,B and Supplementary Figure S3D).
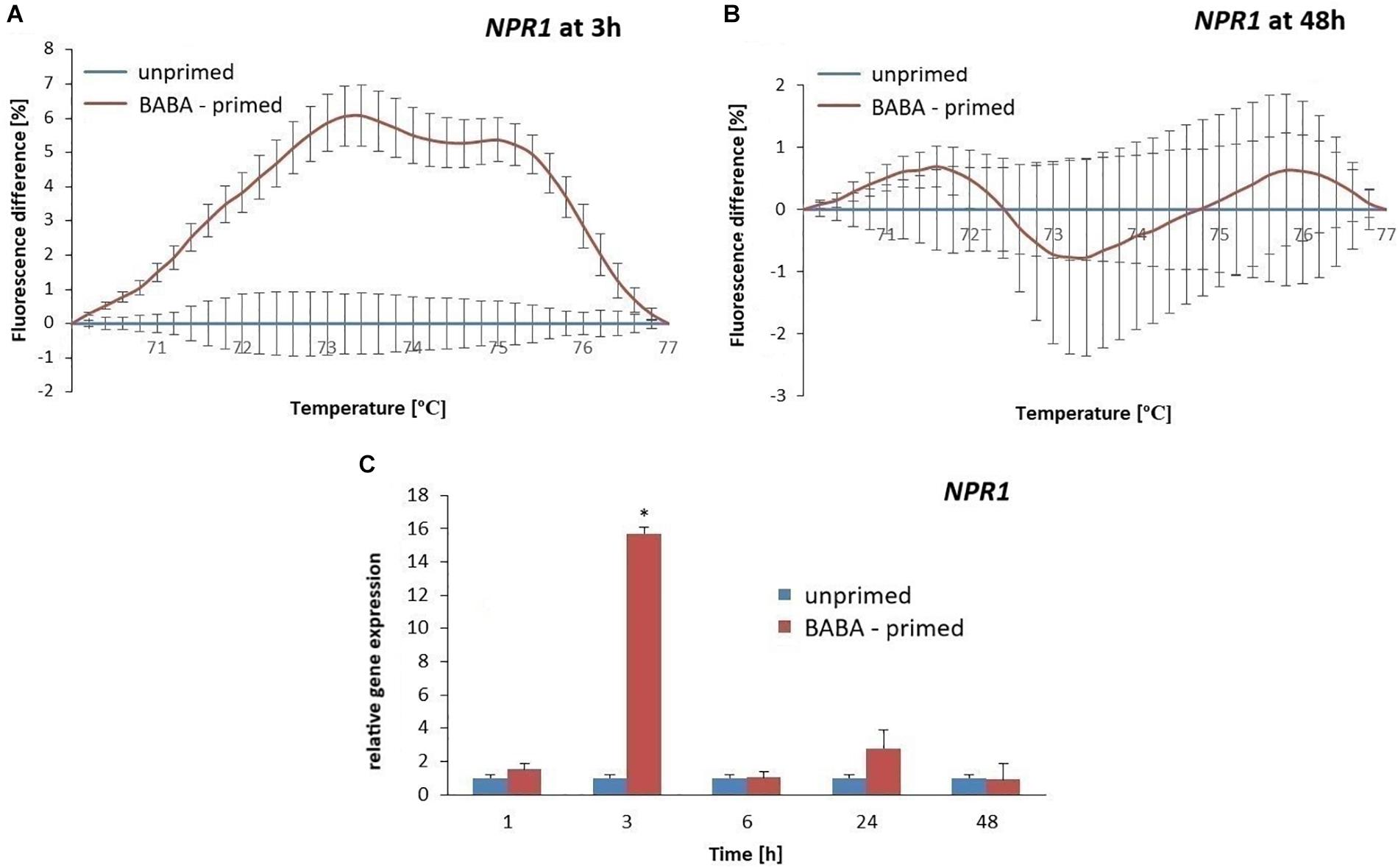
Figure 5. Gene expression and methylation level of NPR1 promoter after BABA treatment in potato. Melting curves of the MS-HRM assay at 3 h (A) and 48 h after BABA treatment (B). It is noted that higher fluorescence levels in BABA-treated plants compared to the unprimed showed enhanced DNA methylation of the NPR1 promoter at 3 h after BABA treatment. The RT-qPCR analysis of NPR1 expression in primed leaves of potato plants at 1–48 h after BABA treatment (C). Values represent mean ± SD of at least three independent experiments. Asterisks (∗) indicate values that differ significantly from unprimed (water-treated) potato leaves at α<0.05, respectively.
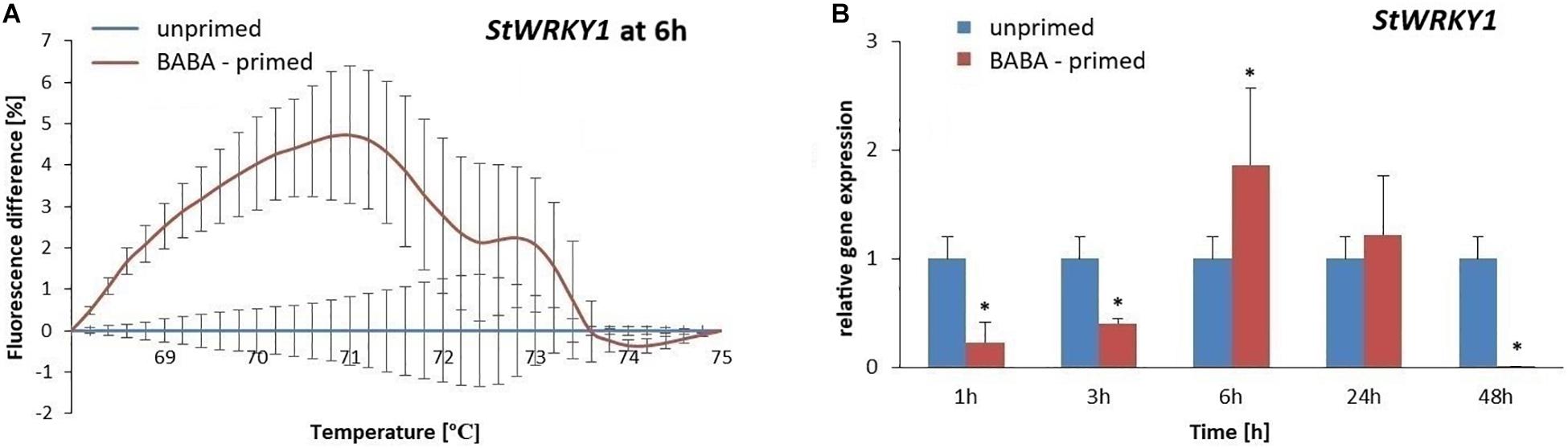
Figure 6. Gene expression and methylation level of StWRKY1 promoter after BABA treatment in potato. Melting curves of the MS-HRM assay at 6 h after BABA treatment (A). Details of the MS-HRM assay are given in the Materials and Methods. Higher fluorescence levels in BABA-treated plants compared to the unprimed showed enhanced DNA methylation of the StWRKY1 promoter at 6 h. RT-qPCR analysis of StWRKY1 expression in primed leaves of potato plants at 1–48 h after BABA treatment (B). Values represent mean ± SD of at least three independent experiments. Asterisks (∗) indicate values that differ significantly from unprimed (water-treated) potato leaves at α<0.05, respectively.
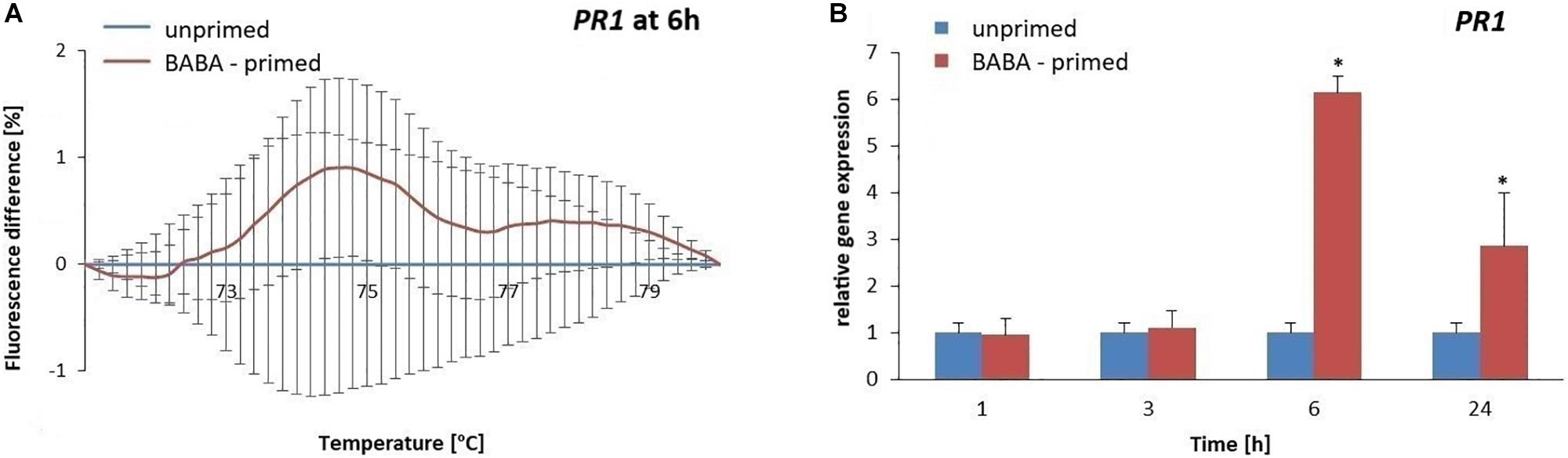
Figure 7. Gene expression and methylation level of PR1 promoter after BABA treatment in potato. Melting curves of the MS-HRM assay at 6 h after BABA treatment (A). Details of the MS-HRM assay are given in the Materials and Methods section. Fluorescence levels in BABA-treated plants compared to the unprimed showed no significant differences in the PR1 promoter methylation upon BABA exposure. RT-qPCR analysis of PR1 expression in primed leaves of potato plant at 1–48 h after BABA treatment (B). Values represent mean ± SD of at least three independent experiments. Asterisks (∗) indicate values that differ significantly from unprimed (water treated) potato leaves at α<0.05, respectively.
The obtained results revealed no association between promoter DNA methylation of SA-dependent genes and their expression levels in BABA-primed potato. This unexpected DNA methylation of the promoter regions results, reported in this study, might indicate that other epigenetic modifications may be associated with gene expression increase.
R3a Gene Promoter Loci-Specific Methylation Patterns
DNA methylation patterns of the R3a promoter became rapidly altered following BABA treatment and it was evident as divergent melting curve profiles were observed in the primed and unprimed potato (Supplementary Figure S4). The methylation level of the R3a promoter increased rapidly (15–20% rise in fluorescence intensity) immediately after the priming stimulus was recognized by the potato BABA receptor (6 h after BABA treatment) and such a promoter DNA methylation may have contributed to the observed downregulation of R3a expression (Figures 8A,B). In turn, after challenge inoculation with P. infestans MP977 the methylation level of the R3a promoter drastically diminished, since the melting curve profiles in the primed and unprimed potato plants were very similar (Supplementary Figure S4). In addition, the expression of R3a significantly increased in relation to the unprimed potato 3 h after P. infestans infection. The immediate upregulation (5-fold increase at 3 hpi) of R3a, playing a key role in the potato immune system, was a good indicator of potato in a primed state.
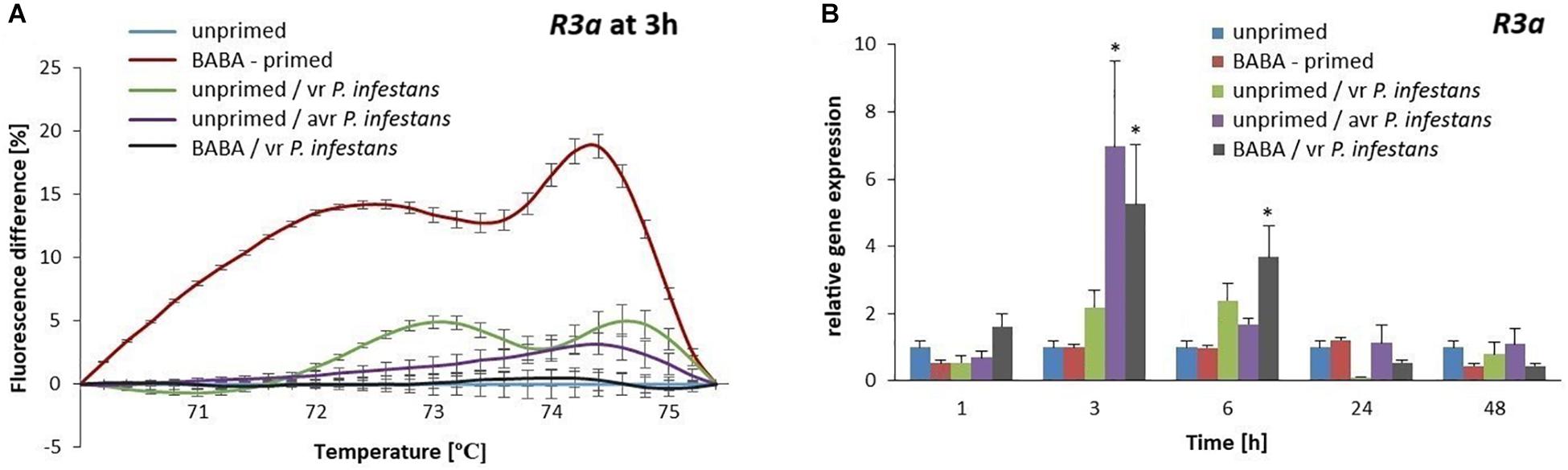
Figure 8. Gene expression and methylation level of R3a promoter after BABA treatment in potato. MS-HRM curves of the R3a promoter at 3 h after BABA or water treatment (unprimed) indicating its DNA methylated status, at 3 h after sequential treatment of BABA/vr P. infestans MP977 (primed-challenged), at 3 h after vr P. infestans MP977 inoculated (PTI-type of resistance) or Avr3a-encoding P. infestans MP946 inoculated (ETI-type of resistance) (A), respectively. Values represent means of data ± SD of at least three independent experiments. RT-qPCR analysis of R3a gene expression in BABA-primed potato leaves followed by challenge inoculation with vr P. infestans MP977 or in unprimed potato only subjected to virulent P. infestans MP977 or Avr3a-encoding P. infestans MP946 inoculation (B). Values represent mean ± SD of at least three independent experiments. Asterisks (∗) indicate values that differ significantly from those of unprimed (water treated) plants.
In order to better understand the putative correlation between the R3a gene expression and the methylated state of its promoter in relation to the type of immune response, we compared both ETI and PTI, defense responses, with BABA-primed ones (Figures 8A,B and Supplementary Figures S4A–D). Differences in DNA methylation patterns of the R3a promoter among the types of immune responses entail the speed and magnitude of R3a gene induction after inoculation. As early as 3 hpi after the challenge with the Avr3a-encoding P. infestans MP946 (ETI-type of defense), the highest (7-fold) increase in the R3a gene expression was observed with the lowest methylation level of its promoter (reflected by the percentage of fluorescence intensity shown in Figure 8A). A comparable, rapid and high (approx. 5-fold) increase in R3a gene expression with a low methylation level of its promoter was found in BABA-primed potato at 3 hpi after P. infestans MP977 inoculation. In turn, the unprimed potato inoculated with the virulent P. infestans MP977 (PTI-type of defense) showed only a 2-fold increase in gene transcription with a more methylated promoter sequence of R3a compared to the ETI and BABA-primed types of defense. These results indicate that a suitable DNA methylation level in the R3a gene promoter could be essential for its expression, which as a result may contribute to plant immunity in potato.
DNA Methylation Level Is Correlated With Intergenerational Priming for Defense in Potato
We analyzed whether changes in the DNA methylation patterns after BABA priming might provoke an intergenerational shift in the R3a gene expression. Indeed, the offspring of BABA-primed plants derived from tubers or seeds exhibited high levels of the R3a gene expression that correlated with an intergenerational resistance to vr P. infestans when compared with the unprimed potato (Figures 9C,D).
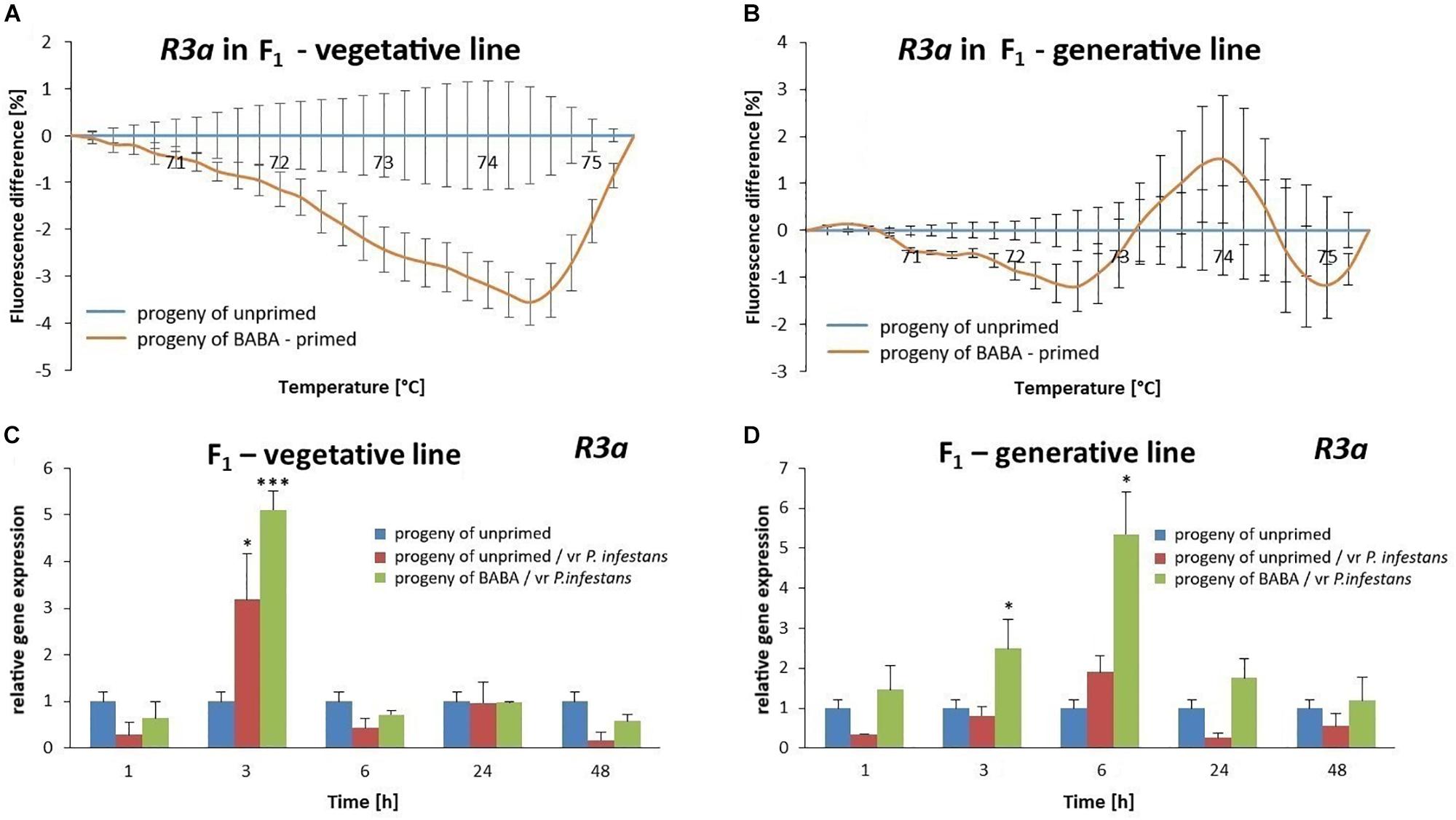
Figure 9. DNA methylation of the R3a promoter region involved in intergenerational priming for defense against vr P. infestans in potato. The MS-HRM melting curve of the R3a promoter region showed hypomethylation in the vegetative progeny of BABA-primed parents (A) and less methylated promoter of this gene in the generative progeny obtained from seeds (B). Values represent means of data ± SD of at least three independent experiments. The RT-qPCR analysis of R3a gene expression after vr P. infestans inoculation in the offspring of BABA-primed plants obtained from tubers (C) or seeds (D). Values represent mean ± SD of at least three independent experiments. Asterisks (∗) or (∗∗∗) indicate values that differ significantly from the progeny of unprimed (water-treated) potato leaves at α<0.05 and α<0.01, respectively, at each time point.
MS-HRM analysis showed that the R3a promoter region in the progeny potato derived from BABA-primed parents by propagation from tubers (F1) was highly hypomethylated, whereas progeny from seeds (F1) was only slightly hypomethylated when compared to potato plants from unprimed parents (Figures 9A,B and Supplementary Figure S5). Together, our data suggest that BABA-induced reversible changes in the methylation pattern of the R3a promoter that could contribute to improved functionality of R3a in parental plants, and probably also other R gene clusters in the tetraploid potato under biotic stress, and that these adaptive changes were passed onto their progeny.
Discussion
It is generally accepted that primed plants show a faster and stronger activation of defense responses against pathogens after challenge inoculation. Much less is known on the coordination of epigenetic mechanisms associated with gene transcriptional reprogramming during the establishment of the primed state. Recent reports have underlined that DNA methylation guarantees stabilization and protects the genome against unwanted rearrangements, while targeted hypomethylation of specific loci is related with the acquisition of new traits or release of suppression of silent genes improving plant resistance to biotic factors (Zhu et al., 2015; López Sánchez et al., 2016; Viggiano and de Pinto, 2017; Tamiru et al., 2018).
S-adenosyl-L-homocysteine hydrolase plays a key role in the cellular AMC. Unlike human cells, in which impaired SAHH activity leads to severe diseases as a consequence of homocysteine over-accumulation (Tehlivets et al., 2013), in plant cells suppression of SAHH activity tends to increase resistance to various viruses (Yang et al., 2011; Cañizares et al., 2013). Previously we found that down-regulation of SAHH is linked to potato resistance against P. infestans (Arasimowicz-Jelonek et al., 2013). Similarly, other data showed that co-silencing of tomato SAHH enhances immunity to Pseudomonas syringae pv. tomato DC3000 (Li et al., 2015).
It was documented by Rocha et al. (2005) that mutations in the AtSAHH1 gene caused a general reduction in the 5-mC level associated with the transcriptionally active chromatin structure in Arabidopsis. In transgenic tobacco, the expression of antisense RNA of SAHH resulted in DNA hypomethylation (Tanaka et al., 1997), while Arabidopsis supplied with a SAHH inhibitor (dihydroxypropyladenine) reduced the pool of methylated DNA (Baubec et al., 2010).
The results from the current study revealed that both SAHH (removing SAH) and SAMS (producing S-adenosylmethionine) were engaged in a modulation of the cellular methylating potential in BABA-primed potato. It is likely that BABA triggered epigenetic changes through modulation of the SAH/SAM ratio and initially potentiated the DNA methylation state in potato. In fact, drastic genome-wide DNA hypermethylation was observed shortly after BABA administration, as reflected by the 2-fold rise in global 5-mC detected by ELISA immunoassay (Figure 4A). The shift in DNA methylation was confirmed by immunostaining of primed potato leaf nuclei. In agreement with these observations, a rapid (3–6 h) up-regulation was noted for both CMT3 and DRM2, engaged in de novo DNA methylation, accompanied by enhanced ROS1 activation. Together, our results suggest that immediately after BABA treatment the primed potato reprogrammed the DNA methylation patterns toward hypermethylation. However, during the maintenance of the priming state, the excessive DNA methylation changed into demethylation with the active involvement of StDML2 and probably also other DNA glycosylases.
Changes in de novo DNA methylation in a plant genome can be dynamic during the priming events. Moreover, defense priming has been divided into a priming phase, a post-challenged primed state and an inter- or transgenerational primed state (Balmer et al., 2015). Thus performing analyses of DNA methylation from the whole genome bisulfite sequencing data (WGBS) might lead to a misinterpretation of epigenetic responses related to the transcriptional state of genes while we are focusing on one time-point of the priming event.
Interestingly, the Arabidopsis ros1 mutant showed enhanced RdDM and decreased plant resistance to bacteria (Yu et al., 2013). In addition, the triple Arabidopsis DNA demethylase mutant rdd (ros1 dml2 dml3) showed high susceptibility to Fusarium oxysporum with downregulation of many stress-responsive genes (Le et al., 2014).
Reports on DNA methylation and its relationship with plant resistance to pathogens seem to be of particular interest; so far, however, collected data are rather puzzling. It was found that three resistance inducers reduced the DNA methylation level providing resistance to powdery mildew in barley (Kraska and Schönbeck, 1993). In Arabidopsis virulent or avirulent strains of Pseudomonas syringae pv. tomato elicit the host plant DNA demethylation (Pavet et al., 2006). In contrast, Arabidopsis plants subjected to various types of biotic and abiotic stresses (i.e., salt, UVB, cold, heat, and flood) revealed a rise in global genome methylation correlated with stress adaptive changes that are transmitted to the progeny of stressed plants (Boyko et al., 2007, 2010; Bilichak et al., 2012). The analysis of total DNA methylation in two consecutive generations of TMV-infected tobacco showed a hypermethylated genome and an increased resistance to TMV, Pseudomonas syringae and Phytophthora nicotianae (Kathiria et al., 2010). However, in these findings, no clear correlation between DNA methylation and stress resistance was observed since the progeny of stressed tobacco or Arabidopsis were differentially methylated with the hypomethylated regions located in several LRR-containing loci or in euchromatic areas of nuclei (Boyko et al., 2010; Kathiria et al., 2010). Furthermore, genome-wide studies with various Arabidopsis mutants defective in CG (met1-3) or non-CG (ddc, drm1-2, drm-2-2, and cmt3-11) methylation showed enhanced resistance to P. syringae pv. tomato (Pst) DC3000 compared to the wild-type plant (Dowen et al., 2012). Also, Luna et al. (2012) documented that the hypomethylated DNA status in the ddc Arabidopsis mutant exhibited an intergenerational resistance phenotype to the bacteriumPstDC3000, but an enhanced susceptibility to the necrotrophic fungus A. brassicicolla.
Stress is known to induce the differential expression of various R-genes and defense-responsive genes, but the knowledge on the mechanism of de novo DNA methylation/active demethylation controlling their target recognition is still in its infancy (Ikeda and Kinoshita, 2009; Li et al., 2012; Bond and Baulcombe, 2015). Thus, we explored the impact of BABA treatment on the methylation state of the resistance gene R3a on potato, as well as the selected SA-dependent genes that might provide a background for long-term memory of host resistance to P. infestans. Time-dependent changes in the methylation status of the NPR1 promoter upon BABA treatment were observed. At the onset of priming, the high 5-mC methylation state in the NPR1 promoter negatively correlated with the cognate gene expression, but during the maintenance of the priming state, NPR1 exhibited a reduction in promoter methylation and transcriptional down-regulation. How promoter methylation might activate NPR1 gene expression remains to be investigated. One could speculate that DNA methylation may inhibit the binding of some transcription repressors or may promote the binding of some transcription activators (Wang et al., 2013).
Our results indicate that BABA treatment caused a 2-fold rise in StWRKY1 expression at 6 h with no significant changes in the DNA methylation level of its promoter. According to Song et al. (2012), stress-responsive transcription factors may be influenced by both DNA methylation and histone modification in a region-specific manner, suggesting heterogeneity in the genome for gene expression regulated via epigenetic modifications under stress. Generally, the correlation between the DNA methylation status and gene expression is a highly multifaceted event, greatly dependent upon the type of methylation as well as the pattern of methylation within or outside the gene sequence (Niederhuth and Schmitz, 2017).
Our findings support a model where BABA can prime PR1 gene expression without 5-mC alterations in its promoter region. This is in agreement with the study of Slaughter et al. (2012), where minimal changes in overall C-methylation in the PR1 promoter region were observed both in the parent and descendants of BABA-primed Arabidopsis; however, PR1 gene was highly responsive to priming and this effect was transferred to the next plant generation.
According to Dowen et al. (2012), inducible expression of some defense genes might not be directly caused by DNA methylation, but rather is the result of methylation-dependent alterations in the transcriptional network or hierarchic control of DNA methylation (Viggiano and de Pinto, 2017). Moreover, an important role devolves to cooperation between the stress-related gene expression and histone configuration, which may keep nucleosomes in the stand by state to facilitate rapid gene expression when needed (Alvarez-Venegas et al., 2007; Zhu et al., 2015). In support of the above, our previous data documented that both NPR1 and PR1, early upon BABA treatment, showed a transient rise in H3K4me2, although later it was replaced by the H3K9me2 (or H3K27me3) mark that correlated with gene expression, and this effect was passed to the progeny resulting in improved protection against P. infestans (Meller et al., 2018).
In contrast to several published data on the methylation status of defense-responsive genes, there has been limited research on the regulation of R-gene expression by methylation. Precise regulation of the R gene expression is pivotal to prevent fitness costs and autoimmune responses in the absence of the pathogen. Although R genes are expressed constitutively at low levels, a rapid overexpression of the R genes contributes to improved resistance under biotic stress (de Vries et al., 2015). Generally, a high resistance level was associated with rapid R gene induction (Gu et al., 2005; Römer et al., 2007; Kramer et al., 2009); however, the expression of some R genes was occasionally very low (Tan et al., 2007), did not increase after infection (Huang et al., 2005) and it was influenced by various environmental factors or the host’s genetic background (Cao et al., 2007).
In the present study BABA alone did not activate R3a gene expression (Figure 8B), but triggered methylation of the R3a promoter region (Figure 8A). However, in successive hours after BABA treatment, an excessive DNA methylation status in potato was changed into demethylation. When the primed potato was challenged with vr P. infestans MP977, the promoter of R3a showed the demethylation state that correlated with its enhanced transcription. Considering the amplitude of R3a gene expression with the 5-mC level of the promoter, the obtained results showed the kinetics and speed of R3a gene induction in BABA-primed defense to be similar to the ETI–response rather than the PTI one. This finding might lead to the overall conclusion that DNA methylation is one of the factors controlling the kinetics of expression of both R3a and other defensive genes, while certain 5-mC-mediated changes might be involved in the intergenerational stress memory, leading to the formation of new epialleles conferring a broad-spectrum resistance against various pathogens.
We provided evidence that DNA methylation is a flexible mechanism that ultimately is able to modify the R3a resistance gene expression and initiate a favorable balance between silent and active gene transcription. BABA transiently activated rapid DNA methylation of the R3a promoter and triggered down-regulation of this gene in the absence of the pathogen. Furthermore, descendants of BABA-primed potato derived from tubers (mitotic intergenerational inheritance) or seeds (meiotic intergenerational inheritance) carrying a less methylated R3a promoter showed higher transcription of R3a, which correlated with enhanced intergenerational resistance to vr P. infestans MP977 when compared to the inoculated progeny of unprimed potato.
The obtained results confirmed once again that plants could integrate and “store” various signals into stress memory for future use or prepare their offspring for potential future assaults. To the best of our knowledge, this is the first report on the potato R3a gene activation/suppression by the stress-induced differentially methylated region of its promoter modifying the level of potato resistance to P. infestans both in the same and the successive generations. Our future efforts will focus on the recognition of the hierarchy of events that drives DNA methylation reprogramming in potato upon BABA treatment, such as ROS1 involvement, which seems to be preferentially engaged in counteracting de novo DNA methylation established by RdDM and probably plays a key role in stress-responsive gene expression associated with plant immunity.
Author Contributions
JF-W and MA-J planned and designed the research. DK, BM, and AD performed the experiments, and collected and analyzed the data. AB-Z performed the immunostaining procedure. JF-W wrote the manuscript.
Funding
This work was supported by the grant of the Polish National Science Centre; project NCN No. 2013/11/B/NZ9/01903.
Conflict of Interest Statement
The authors declare that the research was conducted in the absence of any commercial or financial relationships that could be construed as a potential conflict of interest.
Acknowledgments
The authors are greatly thankful to Kacper Nowacki, a Bachelor of Engineering degree student, for his help in the MS-HRM analysis.
Supplementary Material
The Supplementary Material for this article can be found online at: https://www.frontiersin.org/articles/10.3389/fpls.2019.00650/full#supplementary-material
FIGURE S1 | Schematic representation of the experimental design. The parental line of “Sarpo Mira” potato genotype (F0) was treated once with 5 mM BABA (B0). The progeny (F1) grown from tubers or seeds were not again treated with BABA, but only inoculated with vr P. infestans- at the stage of 10 compound leaves. Abbreviations: the parental F0 line control plants – C0 and BABA-primed – B0, the offspring of control plants – CV and CG, the primed plants of the vegetative F1 line – BV and the generative F1 line – BG.
FIGURE S2 | Global potato nuclei methylation study in response to BABA exposure. A representative image gallery of approx. 168 nuclei at 1, 6 and 48 h upon BABA treatment, respectively. The red color: DAPI staining (lack of methylation); the green color: methylated DNA was immunodetected with anti-5-methylcytosine (5-mC) antibody and Alexa Fluor488 goat anti-mouse IgG. The red and green colors were added using ImageJ software. Scale bar = 5 μm.
FIGURE S3 | Methylation levels of NPR1, StWRKY1, and PR1 promoter after BABA treatment in primed potato. MS-HRM curves of the DNA methylated state of the NPR1 promoter at 3 and 48 h (A,B); the StWRKY1 promoter at 6 h (C); the PR1 promoter at 6 h after BABA treatment in primed potato (D). Fluorescence values of BABA-treated plants (red line) were compared to the unprimed ones (blue line) as shown in the figure. Values represent mean ± SD of at least three independent experiments.
FIGURE S4 | Methylation levels of R3a promoter in potato. MS-HRM curves of the DNA methylated state of the R3a promoter at 3 h after BABA treatment (A); 3 h after vr P. infestans MP977 inoculation (B); 3 h after vr P. infestans PM977 inoculation (C); or after sequential treatment of BABA/vr P. infestans MP977 (D), respectively. Fluorescence values of samples were compared to the control as shown in the figure. Values represent mean ± SD of at least three independent experiments.
FIGURE S5 | Methylation levels of R3a promoter in intergenerational potato. Melting curves of the MS-HRM promoter region of R3a before vr P. infestans MP977 inoculation in the vegetative progeny of BABA-primed parents (A), and the generative progeny obtained from seeds (B). Fluorescence values of samples were compared to the control (progeny of unprimed plants) as shown in the figure. Values represent mean ± SD of at least three independent experiments.
TABLE S1 | Numerical data of melting curves obtained from MS-HRM analysis for the tested genes.
TABLE S2 | Primers used for RT-qPCR and MS-HRM analyses.
Footnotes
References
Akimoto, K., Katakami, H., Kim, H.-J., Ogawa, E., Sano, C. M., Wada, Y., et al. (2007). Epigenetic inheritance in rice plants. Ann. Bot. 100, 205–217. doi: 10.1093/aob/mcm110
Alvarez, M. E., Nota, F., and Cambiagno, D. A. (2010). Epigenetic control of plant immunity. Mol. Plant Pathol. 11, 563–576. doi: 10.1111/j.1364-3703.2010.00621.x
Alvarez-Venegas, R., Abdallat, A. A., Guo, M., Alfano, J. R., and Avramova, Z. (2007). Epigenetic control of a transcription factor at the cross section of two antagonistic pathways. Epigenetics 2, 106–113. doi: 10.4161/epi.2.2.4404
Arasimowicz-Jelonek, M., Floryszak-Wieczorek, J., Gzyl, J., and Chmielowska-Bąk, J. (2013). Homocysteine over-accumulation as the effect of potato leaves exposure to biotic stress. Plant Physiol. Biochem. 63, 177–184. doi: 10.1016/j.plaphy.2012.11.025
Avramova, Z. (2015). Transcriptional ‘memory’ of a stress: transient chromatin and memory (epigenetic) marks at stress-response genes. Plant J. 83, 149–159. doi: 10.1111/tpj.12832
Balmer, A., Pastor, V., Gamir, J., Flors, V., and Mauch-Mani, B. (2015). The ‘prime-ome’: towards a holistic approach to priming. Trends Plant Sci. 20, 443–452. doi: 10.1016/j.tplants.2015.04.002
Baubec, T., Dinh, H. Q., Pecinka, A., Rakic, B., Rozhon, W., Wohlrab, B., et al. (2010). Cooperation of multiple chromatin modifications can generate unanticipated stability of epigenetic States in Arabidopsis. Plant Cell 22, 34–47. doi: 10.1105/tpc.109.072819
Bilichak, A., Ilnystkyy, Y., Hollunder, J., and Kovalchuk, I. (2012). The progeny of Arabidopsis thaliana plants exposed to salt exhibit changes in DNA methylation, histone modifications and gene expression. PLoS One 7:e30515. doi: 10.1371/journal.pone.0030515
Bond, D. M., and Baulcombe, D. C. (2015). Epigenetic transitions leading to heritable, RNA-mediated de novo silencing in Arabidopsis thaliana. Proc. Natl. Acad. Sci. U.S.A. 112, 917–922. doi: 10.1073/pnas.1413053112
Bos, J. I. B., Armstrong, M. R., Gilroy, E. M., Boevink, P. C., Hein, I., Taylor, R. M., et al. (2010). Phytophthora infestans effector AVR3a is essential for virulence and manipulates plant immunity by stabilizing host E3 ligase CMPG1. Proc. Natl. Acad. Sci. U.S.A. 107, 9909–9914. doi: 10.1073/pnas.0914408107
Boyko, A., Blevins, T., Yao, Y., Golubov, A., Bilichak, A., Ilnytskyy, Y., et al. (2010). Transgenerational adaptation of Arabidopsis to stress requires DNA methylation and the function of dicer-like proteins. PLoS One 5:e9514. doi: 10.1371/journal.pone.0009514
Boyko, A., Kathiria, P., Zemp, F. J., Yao, Y., Pogribny, I., and Kovalchuk, I. (2007). Transgenerational changes in the genome stability and methylation in pathogen-infected plants: (virus-induced plant genome instability). Nucleic Acids Res. 35, 1714–1725. doi: 10.1093/nar/gkm029
Braszewska-Zalewska, A., Tylikowska, M., Kwasniewska, J., and Szymanowska-Pulka, J. (2014). Epigenetic chromatin modifications in barley after mutagenic treatment. J. Appl. Genet. 55, 449–456. doi: 10.1007/s13353-014-0226-9
Burki, F., Shalchian-Tabrizi, K., Minge, M., Skjaeveland, A., Nikolaev, S. I., Jakobsen, K. S., et al. (2007). Phylogenomics reshuffles the eukaryotic supergroups. PLoS One 2:e790. doi: 10.1371/journal.pone.0000790
Cañizares, M. C., Lozano-Durán, R., Canto, T., Bejarano, E. R., Bisaro, D. M., Navas-Castillo, J., et al. (2013). Effects of the crinivirus coat protein–interacting plant protein SAHH on post-transcriptional RNA silencing and its suppression. MPMI 26, 1004–1015. doi: 10.1094/MPMI-02-13-0037-R
Cao, Y., Duan, L., Li, H., Sun, X., Zhao, Y., Xu, C., et al. (2007). Functional analysis of Xa3/Xa26 family members in rice resistance to Xanthomonas oryzae pv. oryzae. Theor. Appl. Genet. 115, 887–895. doi: 10.1007/s00122-007-0615-0
Capuano, F., Mülleder, M., Kok, R., Blom, H. J., and Ralser, M. (2014). Cytosine DNA methylation is found in Drosophila melanogaster but absent in Saccharomyces cerevisiae, Schizosaccharomyces pombe, and other yeast species. Anal. Chem. 86, 3697–3702. doi: 10.1021/ac500447w
Caten, C. E., and Jinks, J. L. (1968). Spontaneous variability of single isolates of Phytophthora infestans. I. Cultural variation. Can. J. Bot. 46, 329–348. doi: 10.1139/b68-055
Cohen, Y., Vaknin, M., and Mauch-Mani, B. (2016). BABA-induced resistance: milestones along a 55-year journey. Phytoparasitica 44, 513–538. doi: 10.1007/s12600-016-0546-x
Conrath, U., Beckers, G. J. M., Langenbach, C. J. G., and Jaskiewicz, M. R. (2015). Priming for enhanced defense. Annu. Rev. Phytopathol. 53, 97–119. doi: 10.1146/annurev-phyto-080614-120132
Crisp, P. A., Ganguly, D., Eichten, S. R., Borevitz, J. O., and Pogson, B. J. (2016). Reconsidering plant memory: intersections between stress recovery, RNA turnover, and epigenetics. Sci. Adv. 2:e1501340. doi: 10.1126/sciadv.1501340
de Vries, S., Kloesges, T., and Rose, L. E. (2015). Evolutionarily dynamic, but robust, targeting of resistance Genes by the miR482/2118 gene family in the Solanaceae. Genome Biol. Evol. 7, 3307–3321. doi: 10.1093/gbe/evv225
Dowen, R. H., Pelizzola, M., Schmitz, R. J., Lister, R., Dowen, J. M., Nery, J. R., et al. (2012). Widespread dynamic DNA methylation in response to biotic stress. Proc. Natl. Acad. Sci. U.S.A. 109, E2183–E2191. doi: 10.1073/pnas.1209329109
Du, J., Johnson, L. M., Jacobsen, S. E., and Patel, D. J. (2015). DNA methylation pathways and their crosstalk with histone methylation. Nat. Rev. Mol. Cell Biol. 16, 519–532. doi: 10.1038/nrm4043
Floryszak-Wieczorek, J., Arasimowicz-Jelonek, M., and Abramowski, D. (2015). BABA-primed defense responses to Phytophthora infestans in the next vegetative progeny of potato. Front. Plant Sci. 6:844. doi: 10.3389/fpls.2015.00844
Fu, Z. Q., and Dong, X. (2013). Systemic acquired resistance: turning local infection into global defense. Annu. Rev. Plant Biol. 64, 839–863. doi: 10.1146/annurev-arplant-042811-105606
Gu, K., Yang, B., Tian, D., Wu, L., Wang, D., Sreekala, C., et al. (2005). R gene expression induced by a type-III effector triggers disease resistance in rice. Nature 435, 1122–1125. doi: 10.1038/nature03630
Henderson, I. R., Deleris, A., Wong, W., Zhong, X., Chin, H. G., Horwitz, G. A., et al. (2010). The de novo cytosine methyltransferase DRM2 requires intact UBA domains and a catalytically mutated paralog DRM3 during RNA-directed DNA methylation in Arabidopsis thaliana. PLoS Genet. 6:e1001182. doi: 10.1371/journal.pgen.1001182
Hilker, M., Schwachtje, J., Baier, M., Balazadeh, S., Bäurle, I., Geiselhardt, S., et al. (2016). Priming and memory of stress responses in organisms lacking a nervous system: priming and memory of stress responses. Biol. Rev. 91, 1118–1133. doi: 10.1111/brv.12215
Huang, S., Van Der Vossen, E. A. G., Kuang, H., Vleeshouwers, V. G. A. A., Zhang, N., Borm, T. J. A., et al. (2005). Comparative genomics enabled the isolation of the R3a late blight resistance gene in potato: cloning the potato late blight R3a gene by synteny. Plant J. 42, 251–261. doi: 10.1111/j.1365-313X.2005.02365.x
Ikeda, Y., and Kinoshita, T. (2009). DNA demethylation: a lesson from the garden. Chromosoma 118, 37–41. doi: 10.1007/s00412-008-0183-3
Jaskiewicz, M., Conrath, U., and Peterhänsel, C. (2011). Chromatin modification acts as a memory for systemic acquired resistance in the plant stress response. EMBO Rep. 12, 50–55. doi: 10.1038/embor.2010.186
Jones, J. D. G., and Dangl, J. L. (2006). The plant immune system. Nature 444, 323–329. doi: 10.1038/nature05286
Kakutani, T., Munakata, K., Richards, E. J., and Hirochika, H. (1999). Meiotically and mitotically stable inheritance of DNA hypomethylation induced by ddm1 mutation of Arabidopsis thaliana. Genetics 151, 831–838.
Kathiria, P., Sidler, C., Golubov, A., Kalischuk, M., Kawchuk, L. M., and Kovalchuk, I. (2010). Tobacco mosaic virus infection results in an increase in recombination frequency and resistance to viral, bacterial, and fungal pathogens in the progeny of infected tobacco plants. Plant Physiol. 153, 1859–1870. doi: 10.1104/pp.110.157263
Kramer, L. C., Choudoir, M. J., Wielgus, S. M., Bhaskar, P. B., and Jiang, J. (2009). Correlation between transcript abundance of the RB gene and the level of the RB-mediated late blight resistance in potato. Mol. Plant Microbe Interact. 22, 447–455. doi: 10.1094/MPMI-22-4-0447
Kraska, T., and Schönbeck, F. (1993). About changes in the chromatin structure after resistance induction in Hordeum vulgare L. J. Phytopathol. 137, 10–14. doi: 10.1111/j.1439-0434.1993.tb01320.x
Lämke, J., and Bäurle, I. (2017). Epigenetic and chromatin-based mechanisms in environmental stress adaptation and stress memory in plants. Genome Biol. 18:124. doi: 10.1186/s13059-017-1263-6
Le, T.-N., Schumann, U., Smith, N. A., Tiwari, S., Au, P. C. K., Zhu, Q.-H., et al. (2014). DNA demethylases target promoter transposable elements to positively regulate stress responsive genes in Arabidopsis. Genome Biol. 15:458. doi: 10.1186/s13059-014-0458-3
Lei, M., Zhang, H., Julian, R., Tang, K., Xie, S., and Zhu, J.-K. (2015). Regulatory link between DNA methylation and active demethylation in Arabidopsis. Proc. Natl. Acad. Sci. U.S.A. 112, 3553–3557. doi: 10.1073/pnas.1502279112
Li, F., Pignatta, D., Bendix, C., Brunkard, J. O., Cohn, M. M., Tung, J., et al. (2012). MicroRNA regulation of plant innate immune receptors. Proc. Natl. Acad. Sci. U.S.A. 109, 1790–1795. doi: 10.1073/pnas.1118282109
Li, X., Huang, L., Hong, Y., Zhang, Y., Liu, S., Li, D., et al. (2015). Co-silencing of tomato S-adenosylhomocysteine hydrolase genes confers increased immunity against Pseudomonas syringae pv. tomato DC3000 and enhanced tolerance to drought stress. Front. Plant Sci. 6:717. doi: 10.3389/fpls.2015.00717
López Sánchez, A., Stassen, J. H., Furci, L., Smith, L. M., and Ton, J. (2016). The role of DNA (de)methylation in immune responsiveness of Arabidopsis. Plant J. 88, 361–374. doi: 10.1111/tpj.13252
Luna, E., Bruce, T. J. A., Roberts, M. R., Flors, V., and Ton, J. (2012). Next-generation systemic acquired resistance. Plant Physiol. 158, 844–853. doi: 10.1104/pp.111.187468
Matzke, M. A., and Mosher, R. A. (2014). RNA-directed DNA methylation: an epigenetic pathway of increasing complexity. Nat. Rev. Genet. 15, 394–408. doi: 10.1038/nrg3683
Mauch-Mani, B., Baccelli, I., Luna, E., and Flors, V. (2017). Defense priming: an adaptive part of induced resistance. Annu. Rev. Plant Biol. 68, 485–512. doi: 10.1146/annurev-arplant-042916-041132
Meller, B., Kuźnicki, D., Arasimowicz-Jelonek, M., Deckert, J., and Floryszak-Wieczorek, J. (2018). BABA-primed histone modifications in potato for intergenerational resistance to Phytophthora infestans. Front. Plant Sci. 9:1228. doi: 10.3389/fpls.2018.01228
Navarro, L., Zipfel, C., Rowland, O., Keller, I., Robatzek, S., Boller, T., et al. (2004). The transcriptional innate immune response to flg22. Interplay and overlap with Avr gene-dependent defense responses and bacterial pathogenesis. Plant Physiol. 135, 1113–1128. doi: 10.1104/pp.103.036749
Niederhuth, C. E., and Schmitz, R. J. (2017). Putting DNA methylation in context: from genomes to gene expression in plants. Biochim. Biophys. Acta 1860, 149–156. doi: 10.1016/j.bbagrm.2016.08.009
Orłowska, E., Fiil, A., Kirk, H.-G., Llorente, B., and Cvitanich, C. (2012). Differential gene induction in resistant and susceptible potato cultivars at early stages of infection by Phytophthora infestans. Plant Cell Rep. 31, 187–203. doi: 10.1007/s00299-011-1155-2
Palmer, J. L., and Abeles, R. H. (1979). The mechanism of action of S-adenosylhomocysteinase. J. Biol. Chem. 254, 1217–1226.
Pavet, V., Quintero, C., Cecchini, N. M., Rosa, A. L., and Alvarez, M. E. (2006). Arabidopsis displays centromeric DNA hypomethylation and cytological alterations of heterochromatin upon attack by Pseudomonas syringae. Mol. Plant Microbe Interact. 19, 577–587. doi: 10.1094/MPMI-19-0577
Pecinka, A., Rosa, M., Schikora, A., Berlinger, M., Hirt, H., Luschnig, C., et al. (2009). Transgenerational stress memory is not a general response in Arabidopsis. PLoS One 4:e5202. doi: 10.1371/journal.pone.0005202
Pedley, K. F., and Martin, G. B. (2003). Molecular basis of Pto-mediated resistance to bacterial speck disease in tomato. Annu. Rev. Phytopathol. 41, 215–243. doi: 10.1146/annurev.phyto.41.121602.143032
Pfaffl, M. W. (2001). A new mathematical model for relative quantification in real-time RT-PCR. Nucleic Acids Res. 29:e45.
Prime-A-Plant Group, Conrath, U., Beckers, G. J. M., Flors, V., García-Agustín, P., Jakab, G., et al. (2006). Priming: getting ready for battle. Mol. Plant Microbe Interact. 19, 1062–1071. doi: 10.1094/MPMI-19-1062
Rahikainen, M., Alegre, S., Trotta, A., Pascual, J., and Kangasjärvi, S. (2018). Trans-methylation reactions in plants: focus on the activated methyl cycle. Physiol. Plant. 162, 162–176. doi: 10.1111/ppl.12619
Richard, M. M. S., Gratias, A., Thareau, V., Kim, K. D., Balzergue, S., Joets, J., et al. (2018). Genomic and epigenomic immunity in common bean: the unusual features of NB-LRR gene family. DNA Res. 25, 161–172. doi: 10.1093/dnares/dsx046
Rocha, P. S. C. F., Sheikh, M., Melchiorre, R., Fagard, M., Boutet, S., Loach, R., et al. (2005). The Arabidopsis HOMOLOGY-DEPENDENT GENE SILENCING1 gene codes for an S-adenosyl-L-homocysteine hydrolase required for DNA methylation-dependent gene silencing. Plant Cell 17, 404–417. doi: 10.1105/tpc.104.028332
Römer, P., Hahn, S., Jordan, T., Strauss, T., Bonas, U., and Lahaye, T. (2007). Plant pathogen recognition mediated by promoter activation of the pepper Bs3 resistance gene. Science 318, 645–648. doi: 10.1126/science.1144958
Saze, H., Tsugane, K., Kanno, T., and Nishimura, T. (2012). DNA methylation in plants: relationship to small RNAs and histone modifications, and functions in transposon inactivation. Plant Cell Physiol. 53, 766–784. doi: 10.1093/pcp/pcs008
Seidl, M. F., Cook, D. E., and Thomma, B. P. H. J. (2016). Chromatin biology impacts adaptive evolution of filamentous plant pathogens. PLoS Pathog. 12:e1005920. doi: 10.1371/journal.ppat.1005920
Simko, I. (2016). High-resolution DNA melting analysis in plant research. Trends Plant Sci. 21, 528–537. doi: 10.1016/j.tplants.2016.01.004
Slaughter, A., Daniel, X., Flors, V., Luna, E., Hohn, B., and Mauch-Mani, B. (2012). Descendants of primed Arabidopsis plants exhibit resistance to biotic stress. Plant Physiol. 158, 835–843. doi: 10.1104/pp.111.191593
Song, Y., Ji, D., Li, S., Wang, P., Li, Q., and Xiang, F. (2012). The dynamic changes of DNA methylation and histone modifications of salt responsive transcription factor genes in soybean. PLoS One 7:e41274. doi: 10.1371/journal.pone.0041274
Spoel, S. H., and Dong, X. (2012). How do plants achieve immunity? Defence without specialized immune cells. Nat. Rev. Immunol. 12, 89–100. doi: 10.1038/nri3141
Steward, N., Ito, M., Yamaguchi, Y., Koizumi, N., and Sano, H. (2002). Periodic DNA methylation in maize nucleosomes and demethylation by environmental stress. J. Biol. Chem. 277, 37741–37746. doi: 10.1074/jbc.M204050200
Tamiru, M., Hardcastle, T. J., and Lewsey, M. G. (2018). Regulation of genome-wide DNA methylation by mobile small RNAs. New Phytol. 217, 540–546. doi: 10.1111/nph.14874
Tan, X., Meyers, B. C., Kozik, A., West, M. A. L., Morgante, M., St Clair, D. A., et al. (2007). Global expression analysis of nucleotide binding site-leucine rich repeat-encoding and related genes in Arabidopsis. BMC Plant Biol. 7:56. doi: 10.1186/1471-2229-7-56
Tanaka, H., Masuta, C., Uehara, K., Kataoka, J., Koiwai, A., and Noma, M. (1997). Morphological changes and hypomethylation of DNA in transgenic tobacco expressing antisense RNA of the S-adenosyl-L-homocysteine hydrolase gene. Plant Mol. Biol. 35, 981–986.
Tehlivets, O., Malanovic, N., Visram, M., Pavkov-Keller, T., and Keller, W. (2013). S-adenosyl-L-homocysteine hydrolase and methylation disorders: yeast as a model system. Biochim. Biophys. Acta 1832, 204–215. doi: 10.1016/j.bbadis.2012.09.007
Tichopad, A., Didier, A., and Pfaffl, M. W. (2004). Inhibition of real-time RT-PCR quantification due to tissue-specific contaminants. Mol. Cell. Probes 18, 45–50. doi: 10.1016/j.mcp.2003.09.001
Tricker, P. J., Gibbings, J. G., Rodríguez López, C. M., Hadley, P., and Wilkinson, M. J. (2012). Low relative humidity triggers RNA-directed de novo DNA methylation and suppression of genes controlling stomatal development. J. Exp. Bot. 63, 3799–3813. doi: 10.1093/jxb/ers076
van’t Klooster, J. W., van den Berg-Velthuis, G., van West, P., and Govers, F. (2000). tef1, a Phytophthora infestans gene encoding translation elongation factor 1α. Gene 249, 145–151. doi: 10.1016/S0378-1119(00)00151-7
Viggiano, L., and de Pinto, M. C. (2017). “Dynamic DNA methylation patterns in stress response,” in Plant Epigenetics RNA Technologies, eds N. Rajewsky, S. Jurga, and J. Barciszewski (Cham: Springer International Publishing), 281–302. doi: 10.1007/978-3-319-55520-1_15
Vleeshouwers, V. G. A. A., Raffaele, S., Vossen, J. H., Champouret, N., Oliva, R., Segretin, M. E., et al. (2011). Understanding and exploiting late blight resistance in the age of effectors. Annu. Rev. Phytopathol. 49, 507–531. doi: 10.1146/annurev-phyto-072910-095326
Wang, Y., An, C., Zhang, X., Yao, J., Zhang, Y., Sun, Y., et al. (2013). The Arabidopsis elongator complex subunit2 epigenetically regulates plant immune responses. Plant Cell Online 25, 762–776. doi: 10.1105/tpc.113.109116
Weiberg, A., and Jin, H. (2015). Small RNAs–the secret agents in the plant-pathogen interactions. Curr. Opin. Plant Biol. 26, 87–94. doi: 10.1016/j.pbi.2015.05.033
Wilson, U. E., and Coffey, M. D. (1980). Cytological evaluation of general resistance to phytophthora infestans in potato foliage. Ann. Bot. 45, 81–90.
Wittwer, C. T., Reed, G. H., Gundry, C. N., Vandersteen, J. G., and Pryor, R. J. (2003). High-resolution genotyping by amplicon melting analysis using LCGreen. Clin. Chem. 49, 853–860. doi: 10.1373/49.6.853
Wojdacz, T. K., Dobrovic, A., and Hansen, L. L. (2008). Methylation-sensitive high-resolution melting. Nat. Protoc. 3, 1903–1908. doi: 10.1038/nprot.2008.191
Xia, S., Cheng, Y. T., Huang, S., Win, J., Soards, A., Jinn, T.-L., et al. (2013). Regulation of transcription of nucleotide-binding leucine-rich repeat-encoding genes SNC1 and RPP4 via H3K4 trimethylation. Plant Physiol. 162, 1694–1705. doi: 10.1104/pp.113.214551
Xin, C., Hou, R., Wu, F., Zhao, Y., Xiao, H., Si, W., et al. (2015). Analysis of cytosine methylation status in potato by methylation-sensitive amplified polymorphisms under low-temperature stress. J. Plant Biol. 58, 383–390. doi: 10.1007/s12374-015-0316-1
Yang, X., Xie, Y., Raja, P., Li, S., Wolf, J. N., Shen, Q., et al. (2011). Suppression of methylation-mediated transcriptional gene silencing by βC1-SAHH protein interaction during geminivirus-betasatellite infection. PLoS Pathog. 7:e1002329. doi: 10.1371/journal.ppat.1002329
Yogendra, K. N., Kumar, A., Sarkar, K., Li, Y., Pushpa, D., Mosa, K. A., et al. (2015). Transcription factor StWRKY1 regulates phenylpropanoid metabolites conferring late blight resistance in potato. J. Exp. Bot. 66, 7377–7389. doi: 10.1093/jxb/erv434
Yu, A., Lepere, G., Jay, F., Wang, J., Bapaume, L., Wang, Y., et al. (2013). Dynamics and biological relevance of DNA demethylation in Arabidopsis antibacterial defense. Proc. Natl. Acad. Sci. U.S.A. 110, 2389–2394. doi: 10.1073/pnas.1211757110
Zhang, H., Lang, Z., and Zhu, J.-K. (2018). Dynamics and function of DNA methylation in plants. Nat. Rev. Mol. Cell Biol. 19, 489–506. doi: 10.1038/s41580-018-0016-z
Zhao, S., and Fernald, R. D. (2005). Comprehensive algorithm for quantitative real-time polymerase chain reaction. J. Comput. Biol. 12, 1047–1064. doi: 10.1089/cmb.2005.12.1047
Zhu, J.-K. (2009). Active DNA demethylation mediated by DNA glycosylases. Annu. Rev. Genet. 43, 143–166. doi: 10.1146/annurev-genet-102108-134205
Zhu, Q.-H., Shan, W.-X., Ayliffe, M. A., and Wang, M.-B. (2015). Epigenetic mechanisms: an emerging player in plant-microbe interactions. MPMI 29, 187–196. doi: 10.1094/MPMI-08-15-0194-FI
Keywords: potato acquired resistance, Phytophthora infestans, stress-responsive genes, DNA methylation/demethylation, intergenerational resistance
Citation: Kuźnicki D, Meller B, Arasimowicz-Jelonek M, Braszewska-Zalewska A, Drozda A and Floryszak-Wieczorek J (2019) BABA-Induced DNA Methylome Adjustment to Intergenerational Defense Priming in Potato to Phytophthora infestans. Front. Plant Sci. 10:650. doi: 10.3389/fpls.2019.00650
Received: 30 December 2018; Accepted: 30 April 2019;
Published: 31 May 2019.
Edited by:
Péter Poór, University of Szeged, HungaryReviewed by:
Victoria Pastor, Jaume I University, SpainShaojun Xie, Purdue University, United States
Copyright © 2019 Kuźnicki, Meller, Arasimowicz-Jelonek, Braszewska-Zalewska, Drozda and Floryszak-Wieczorek. This is an open-access article distributed under the terms of the Creative Commons Attribution License (CC BY). The use, distribution or reproduction in other forums is permitted, provided the original author(s) and the copyright owner(s) are credited and that the original publication in this journal is cited, in accordance with accepted academic practice. No use, distribution or reproduction is permitted which does not comply with these terms.
*Correspondence: Jolanta Floryszak-Wieczorek, am9sYW50YS5mbG9yeXN6YWtAdXAucG96bmFuLnBs