- 1School of Biological Science and Engineering, Shaanxi University of Technology, Hanzhong, China
- 2School of Chemical and Environmental Science, Shaanxi University of Technology, Hanzhong, China
Salinity stress from soil or irrigation water can significantly limit the growth and development of plants. Emerging evidence suggests that hydrogen sulfide (H2S), as a versatile signal molecule, can ameliorate salt stress-induced adverse effects. However, the possible physiological mechanism underlying H2S-alleviated salt stress in cucumber remains unclear. Here, a pot experiment was conducted with an aim to examine the possible mechanism of H2S in enhancement of cucumber salt stress tolerance. The results showed that H2S ameliorated salt-induced growth inhibition and alleviated the reduction in photosynthetic attributes, chlorophyll fluorescence and stomatal parameters. Meanwhile H2S increased the endogenous H2S level concomitant with increased activities of D/L-cysteine desulfhydrase and β-cyanoalanine synthase and decreased activities of O-acetyl-L-serine(thiol)lyase under excess NaCl. Notably, H2S maintained Na+ and K+ homeostasis via regulation of the expression of PM H+-ATPase, SOS1 and SKOR at the transcriptional level under excess NaCl. Moreover, H2S alleviated salt-induced oxidative stress as indicated by lowered lipid peroxidation and reactive oxygen species accumulation through an enhanced antioxidant system. Altogether, these results demonstrated that application of H2S could protect cucumber seedlings against salinity stress, likely by keeping the Na+/K+ balance, controlling the endogenous H2S level by regulating the H2S synthetic and decomposition enzymes, and preventing oxidative stress by enhancing the antioxidant system under salinity stress.
Introduction
Salinization of soil is pervasive throughout the globe, which is gradually becoming an earnest threat to world agriculture, affecting approximately 20% of arable irrigated land and consequently leading to the loss of United States$ 27.5 billion per annum (Abdel Latef et al., 2019). Salt stress is a major environmental factor that leads to significant inhibition of plant growth and decrease of productivity. Salt stress can greatly inhibit plant growth by decreasing biomass production and reducing the Pn and Tr (Chen et al., 2016). Chlorophyll fluorescence is considered a tool for interpreting stress tolerance in plants. Measurement of the chlorophyll fluorescence from PSII, the primary reactions of photosynthesis, provides an assessment of plant stress (Diao et al., 2014). Under adverse conditions, Fo is an important indicator of damage to the PSII reaction center, while the Fv/Fm and Fv/Fo ratios reflect its maximum photo-energy conversion efficiency and its potential photochemical efficiency. The Fv/Fm and ΦPSII represent the primary and actual light energy conversion capacities, respectively. Any decreases in Fv/Fm and ΦPSII indicate decline in photochemical activity of PSII (Kumar and Parsad, 2015). In general, the Fv/Fm, ΦPSII and qL parameters have been calculated to reflect photochemical quenching, while NPQ is a non-photochemical-quenching parameter (Zhang et al., 2009). The factor qP represents the reduced state of the primary electron acceptor QA (Kumar and Parsad, 2015). Under saline conditions, there is a general decrease in qP parameters (Fv/Fm and Fo) and in the ETR, but increases in qP, ΦPSII and NPQ.
It is well known that salt stress results in the over-accumulation of Na+ in plants cells, which competitively inhibit the uptake of K+, thus leading to a deficiency of K+ (Zhu, 2003; Munns and Tester, 2008). Plants have evolved varying mechanisms to adapt to salinity, including reducing Na+ influx into the root, control of Na+ xylem loading, Na+ retrieval from the xylem, Na+ recirculation in the phloem, Na+ efflux from the root, intracellular compartmentation of Na+ into the vacuoles, and Na+ secretion from the leaf (Cui et al., 2011; Kronzucker and Britto, 2011). The best way to limit Na+ accumulation in plants would be to reduce Na+ influx into the root in the first place, leading to improved salt tolerance of crop plants. The SOS pathway is essential for salt stress tolerance and maintaining ion homeostasis in the cytoplasm (Zhu, 2002). For example, a plasma membrane Na+/H+ antiporter (SOS1) is able to remove excess Na+ from cells (Feki et al., 2014). The PM H+-ATPase also plays an important role in the regulation of ion homeostasis in the cytosol when plants encountered salt stress (Binzel, 1995). On the other hand, the prevention of Na+-caused K+ leakage is also critical for plant salt tolerance (Lai et al., 2014). K+ channels or transporters are responsible for the K+ transport into or out of root cells. Several reports showed that the outward-rectifying K+channel such as SKOR is involved in K+ efflux (Lai et al., 2014). The SKOR is expressed in the root stele and responsible for K+ release to the xylem (Gaymard et al., 1998).
Plants under salinity stress usually produce excessive amounts of ROS, such as O2•-, OH, 1O2, and H2O2 (Yin et al., 2016). And the excessive accumulation of ROS is potentially harmful to proteins, DNA and lipids, leading to impairment of membrane integrity, enzyme inhibition and chlorophyll degradation. Therefore, ROS scavenging is important for plant survival and growth under salinity stress conditions. To combat with excessive accumulation of ROS, plants have evolved a protective strategy by activating antioxidant system, which includes non-enzymatic antioxidants, such as acid ASA and GSH, and enzymatic antioxidants, such as SOD (EC1.15.1.1), CAT (EC 1.11.1.6), POD (EC 1.11.1.7), GPX (EC 1.11.1.9), APX (EC 1.11.1.11), and GR (EC 1.6.4.2). The functions of ASA and GSH are closely related to their redox states (Kocsy et al., 2001) and plants can adjust redox states of ASA and GSH by modulating their regeneration and biosynthesis.
For a long time, H2S has been considered as merely a toxic by product of cell metabolism, but nowadays it is emerging as a novel gaseous signal molecule, which participates in seed germination, plant growth and development, as well as the acquisition of stress tolerance including cross-adaptation in plants (Li et al., 2016). H2S homeostasis is closely regulated by L-CD, EC 4.4.1.1, D-CD, EC 4.4.1.15, CAS, EC 4.4.1.9, OAS-TL, EC 2.5.1.47, and so on (Li et al., 2016). L-CD and D-CD can catalyze the degradation of L-/D-cysteine to produce H2S, amine and pyruvate. Additionally, H2S can be released from cysteine in the presence of hydrogen cyanide by CAS. On the other hand, OAS-TL can incorporate H2S into O-acetyl-L-serine to form cysteine, and its reverse reaction can release H2S. Generally, plants synthesize H2S via L-CD or D-CD, which respond to environment stress to promote stress tolerance.
Evidence in some plant species has proven that H2S participates in plant responses to salt stresses. Wang et al. (2012) reported that H2S can enhance Medicago sativa tolerance against salinity via the NO pathway, which is also confirmed by Chen et al. (2015) in barley seedling. It is reported that H2S can coordinate regulation of the SOS pathway to overcome the deleterious effects of salt stress in strawberry (Christou et al., 2013). Deng et al. (2016) also confirmed that H2S kept a lower Na+ concentration via the regulation the SOS1 pathway to alleviate growth inhibition in wheat seedlings under NaCl stress. Subsequently, Lai et al. (2014) reported that endogenous H2S can enhance salt tolerance by the establishment of redox homeostasis and preventing salt-induced K+ loss in seedlings of Medicago sativa. In addition, H2S can also synergistically regulate Na+/K+ balance, mineral homeostasis and oxidative metabolism in rice under excessive salt stress (Mostofa et al., 2015b). But these studies are commonly involved in one aspect, either Na+/K+ balance or antioxidation system, either in roots or leaves, and there are limited reports on the overall changes in morphology, photosynthesis, stomatal responses, ROS accumulation and the correlation between changes in the leaves and roots following exogenous H2S treatment. Furthermore, the underlying mechanisms through which H2S regulates salt tolerance, especially the changes in H2S homeostasis, are still elusive, requiring in-depth analysis at the physiological and biochemical levels. Cucumber (Cucumis sativus L.) is an economically important crop as well as a model plant for systematic investigations on many aspects.
In this study, a pot experiment was conducted with an aim to evaluate the possible mechanism by which H2S enhances tolerance of salinity stress in the important vegetable crop cucumber, with particular emphasis on maintenance of the Na+/K+ balance, and regulating of H2S metabolism and oxidative stress response.
Materials and Methods
Plant Culture and Treatments
Under salt stress, the morphological characters and physiological indexes of sensitive cultivars are more obvious than tolerant cultivars. When the sensitive plants were treated with H2S, the ameliorative effects of H2S will be easy to judge. Therefore, the sensitive cucumber (C. sativus L.) cultivar “Chunxiaqiuwang” was used in our experiments. Uniform seeds were sterilized in 10 % (v/v) sodium hypochlorite solution for 5 min followed by washing three times with distilled water. Sterilized seeds were germinated on moist filter paper in petri dishes at 25°C in the dark. After germination, seedlings were transplanted into perlite-filled plastic pots (240 mL, 65 × 45 × 70 mm, a seedling per pot) and watered with Hoagland’s solution. 10 mL Hoagland’s solution was applied for every other day after germination, which contained 5 mM KNO3, 5 mM Ca(NO3)2, 1 mM NH4H2PO4, 2 mM MgSO4, 10 μM MnSO4, 50 μM H3BO3, 0.7 μM ZnSO4, 0.2 μM CuSO4, 0.01 μM (NH4)6Mo7O24 and 70 μM Fe-EDTA-Na2. The seedlings of cucumber were maintained in a controlled growth chamber with a light/dark regime of 14/10 h, relative humidity of 70%, temperature of 25°C and a PAR of 800 μmol.m-2.s-1. When the first true leaf emerged, uniform and healthy seedlings were selected and divided into three groups for the following treatments. (i) Hoagland’s nutrient solution (as Control); (ii) Hoagland’s nutrient solution + 200 mM NaCl (as NaCl); (iii) Hoagland’s nutrient solution + 200 mM NaCl + 5 or 10, or 15 or 20 μM NaHS (as NaCl + NaHS). In our preliminary experiment, the phenotypic characteristics of plants with treated by H2S alone were no significant difference compared with the control group (data not shown). Thus there is no only H2S treatment group in our study. NaHS is commonly used as an H2S donor since it dissociates to water produce HS- and Na+, and then combination of HS- with H+ produces H2S (Lin et al., 2012). NaHS is responsible for induction of stress tolerance, but not Na2S, Na2SO4, NaHSO4, Na2SO3, NaHSO3, or CH3COONa (Deng et al., 2016). Each treatment was replicated three times under the same experimental conditions. The NaHS was purchased from Sigma (St Louis, MO, United States) and used as H2S donor. The remaining treatment solution, which was not fully absorbed by plants, was removed and 10 mL fresh treatment solution was irrigated every other day at 9:00 AM during the whole culture process. After 7 days of treatment, the parameters of photosynthesis and chlorophyll fluorescence were determine using the first true leaf of seedlings with different treatments. And finally, at least 24 seedlings per treatment group were harvested and weighed separately to determine various physiological and biochemical parameters, while other samples were immediately frozen in liquid N2 and then stored in a -80°C freezer until use.
Morphological Assessment
Root length, seedling length (including aboveground and underground parts) and leaf area index were determined after 7 days of treatment. Leaf area index was measured using graph paper according to the method of Gupta et al. (2017). Plant roots were gently removed from the perlite, and then washed three times with deionized water to remove adhered perlite particles. After absorbing moisture from the root surface with a clean filter paper, the fresh weights of root and shoot were recorded, respectively. Shoot dry weight and root dry weight were, respectively, recorded after drying the same plants in an oven at 60 ° C until the weight became constant. Survival rate (%) was calculated as the number of survival seedlings / number of total seedlings. The rate of second leaf expansion (%) was calculated as the number of seedlings with appearance of the second leaf / number of total seedlings.
Measurements of Photosynthesis, Chlorophyll Fluorescence, and Chlorophyll Content
The first true leaf was chosen to determine the parameters of photosynthesis, chlorophyll fluorescence and chlorophyll Content. And the Pn and Tr were measured using an LI-6400 portable photosynthesis system (LI-COR, Lincoln, NE, United States). The leaf chlorophyll fluorescence parameters were measured using a portable chlorophyll fluorometer (OS-30p+, Opti-Science, Inc., Tyngsboro, MA, United States). The Fo emission was determined on dark-adapted leaves. The Fm was obtained during a subsequent saturating light pulse. A second saturating pulse of white light was imposed to determine the Fm′. The Fo′ was determined by illuminating the leaf with far-red light for 3 s. The fluorescence parameters were calculated according to Hu and Yu (2014): maximal quantum yield of PSII photochemistry, Fv/Fm = (Fm-Fo)/Fm; effective quantum-use efficiency of PSII in light-adapted state, Fv′/Fm′ = (Fm′-Fo′)/Fm′; quantum yield of PSII photochemistry, ΦPSII = (Fm′-Ft)/Fm′; qP = (Fm′-Ft)/(Fm′-Fo′); and NPQ = (Fm-Fm′)/Fm′. The contents of Chlorophyll a (Ca), chlorophyll b (Cb), and Cx+c were measured on fresh leaves as described by Lichtenthaler and Wellburn (1983).
Analysis of Stomatal Parameters by Scanning Electron Microscope
The first true leaves were selected to measure the stomatal opening by scanning electron microscopy (S-3400N; Hitachi, Tokyo, Japan). The leaf epidermis and stomata were observed and photographed under 15 kv. The diameter of the stomata was measured by Motic Images Advanced 3.2 (Notic China Group Co., Ltd., China) software at 300× magnification.
Analysis of Electrolyte Leakage and Malondialdehyde Content
Membrane integrity was evaluated in the leaves and roots by measuring electrolyte leakage. Fresh leaf or root samples (50 mg) were cut in small pieces followed by repeated washings with double distilled water. And then the samples were incubated at 28°C for 2 h in test tubes containing 10 mL of double distilled water for determining EC1 using a conductivity meter (Lei-Ci, DDSJ-308F, Shanghai, China). The same samples were again kept in water bath at 100°C for 20 min and the EC2 was recorded at room temperature. The electrolyte leakage was calculated as electrolyte leakage (%) = (EC1/EC2) × 100%. MDA content in the leaves or roots was measured by TBA reaction according to Shi et al. (2014) with modifications. Leaves or roots tissue (0.5 g) was homogenized in 10 mL of 0.1% (w/v) TCA, and the extract was centrifuged at 5, 000 × g for 10 min at 4°C. To measure MDA, 1.5 mL of the supernatant was added into 1.5 mL of 0.5% (w/v) TBA made in 5 % TCA. The mixture was heated at 100°C for 20 min and then quickly cooled in an ice bath. After centrifuging at 7, 888 × g for 10 min, the absorbance of the supernatant was measured at 450 nm, 532 nm, and 600 nm, respectively. The MDA content of leaves or roots (per 0.5 g, FW) was calculated using the formula: C (nmol. g-1 FW) = 20 × [6.45 (OD532 – OD600) – 0.56 OD450].
Determination of Endogenous O2•- and H2O2 Levels
The production of O2•- was determined using the protocol described by Jiao et al. (2011) with some modifications. Samples (0.5 g) were homogenized in an ice bath with 1.2 mL of phosphate buffer (pH 7.8) and centrifuged at 5, 000 × g for 10 min at 4°C. The supernatant was reacted with 1 mL of hydroxylamine hydrochlorides for 1 h, then 1 mL of p-aminobenzene sulfonic acid and 1 ml of α-naphthylamine were added. The solution was kept at 25°C for 20 min. The optical density value of the solution was measured with a spectrophotometer at 530 nm using NaNO2 as the standard curve and the corresponding calibration curves was Y = 552.16 X + 4.365 (R2 = 0.9903) H2O2 was measured spectrophotometrically according to Jiang et al. (2012). Roots and leaves (0.2 g) were homogenized in an ice bath with 1 mL of 0.1% TCA and centrifuged at 12, 000 × g for 20 min at 4°C. The supernatant was used for measuring H2O2 content. The reaction mixture consisted of 0.5 mL of the extracted supernatant, 0.5 mL of 10 mM potassium phosphate buffer (pH 7.0) and 1 mL of 1 M potassium iodide. The reaction was developed for 1 h in darkness before the absorbance was measured at 390 nm. The blank was 0.1% TCA in the absence of root extract. The amount of H2O2 was calculated using a standard curve prepared with known concentrations of H2O2. The corresponding calibration curves was Y = 82.69 X – 1.11 (R2 = 0.9959).
Determination of Endogenous H2S Level and the Activities of L-CD, D-CD, CAS, and OAS-TL
Endogenous H2S content was determined by the formation of methylene blue from dimethyl-p-phenylenediamine in H2SO4 according to the method described previously (Zhang et al., 2008). Samples (0.5 g) were extracted in 1 mL phosphate buffer solution (50 mM, pH 6.8) containing 0.2 M ASA and 0.1 M EDTA. Subsequently, 1 M HCl (0.5 mL) was added to the homogenate in a test tube for releasing H2S, and 0.5 mL of 1% (w/v) zinc acetate was used to absorb H2S. After 30 min reaction, 0.3 mL 5 mM N,N-dimethyl-p-phenylenediamine dihydrochloride dissolved in 3.5 mM H2SO4 was injected into the trap. Then 0.3 mL of 50 mM ferric ammonium sulfate in 100 mM H2SO4 was injected into the trap. The amount of H2S in zinc acetate traps was determined spectrophotometrically at 670 nm, after leaving the mixture for 15 min at room temperature. Solutions with different concentrations of Na2S were prepared, treated in the same way as the assay samples and were used for the quantification of H2S.
Samples (0.3 g) were ground with a mortar and pestle in liquid nitrogen and soluble proteins were extracted with 1.5 mL of cold extraction buffer containing 20 mM Tris–HCl (pH 8.0), 0.1% (w/v) DTT and 0.2% (w/v) sodium ascorbate. The homogenate was centrifuged at 13, 000 × g at 4°C for 15 min. The resulting supernatant was used for the determination of the activities of L/D-CD, CAS, and OAS-TL. Total L-CD activity was determined by the release of H2S from L-cysteine as described in Riemenschneider et al. (2005) with minor modification. The assay contained in a total volume of 1 mL: 0.1 mL of 10 mM L-cysteine, 0.8 mL 100 mM Tris–HCl (containing 2.5 mM DTT, pH 9.0) and 0.1 mL of protein solution. After incubation for 60 min at 30° C, the reaction was terminated by adding 0.1 mL of 30 mM FeCl3 dissolved in 1.2 N HCl and 0.1 mL of 20 mM N, N-dimethyl-p-phenylenedi-amine dihydrochloride dissolved in 7.2 N HCl. The formation of methylene blue was determined at 670 nm, and known concentrations of Na2S were used in the calibration curve. D-CD activity was determined in the same way as L-CD activity except with D-cysteine instead of L-cysteine and the pH of the Tris–HCl buffer was 8.0. One unit of enzyme activity is defined as the amount of enzyme that produces 1.0 mmol/min H2S under the stated assay conditions and expressed as U g-1 FW. The total OAS-TL activity was determined following the method described Bloem et al. (2004). CAS activity was measured by the release of H2S from L-cysteine in the same way as L-CD activity with the following modifications: the 1.0 mL volume of reaction mixture contained 0.1 mL of 10 mM L-cysteine, 0.7 mL 100 mM Tris–HCl (pH 9.0), 0.1 mL of 7.5 mM KCN and 0.1 mL of protein solution, as described in Meyer et al. (2003).
Determination of Na+ and K+ Content and Na+/K+
Dried plant material (200 mg) was subjected to acid digestion in 10 mL digestion mixture [HClO4: HNO3: H2SO4 (5: 1: 1, v/v)] on a hot plate (120°C) until the volume was reduced to 1 mL. After diluting with double distilled water, the Na+ and K+ contents were estimated using the flame photometer (Horneck and Hanson, 1998).
Extraction of Total RNA and Real-Time RT-PCR Analysis
To study the effect of NaHS treatment on the expression of the SOS1, SKOR, and PM H+-ATPase genes in cucumber leaves and roots in response to salt stress, 18-day-old seedlings treated with either Hoagland’s nutrient solutions (control group), 200 mM NaCl, or 200 mM NaCl + 15.0 μM NaHS for a week were harvested. Total RNA of cucumber leaves or roots (100 mg) was isolated by grinding with mortar and pestle in liquid nitrogen to a fine powder and using TriZol Reagent (Invitrogen) in accordance with the manufacturer’s protocol. The total RNA samples were treated with RNAase-free DNase (TaKaRa Bio Inc., Dalian, China) to eliminate traces of DNA, followed by quantification using the NanoDrop 2000 (Thermo Fisher Scientific, Wilmington, DE, United States). Total RNA (2 μg) was reverse-transcribed using an oligo d (T) primer (50 μM, 1 μL) and M-MLV reverse transcriptase (200 U/μL, 1 μL) (BioTeke, Beijing, China). Real-time quantitative RT-PCR reactions were performed using an ABI 7000 (Applied Biosystems) with SYBR®Premix ExTaqTM (TaKaRa Bio Inc., China) and the cycling conditions of denaturation at 95°C for 5 min, followed by 40 cycles of denaturation at 95°C for 15 s, annealing at 60°C for 30 s, and extension at 72°C for 15 s. Using specific primers (Supporting information, Supplementary Table S1), the expression levels of the genes were presented as values relative to the corresponding control samples under the indicated conditions, with normalization of data to the geometric average of internal control gene GAPDH (a housekeeping genes). Three independent replicates were performed for each sample. The comparative threshold cycle (Ct) method was used to determine the relative amount of gene expression. Relative gene expression levels were calculated via the 2-ΔΔCT method. The relative gene expression was determined as previously described by Livak and Schmittgen (2001).
Measurements of ASA, GSH, SOD, CAT, GR, and POD
The ASA content was determined using the DCIP method. Samples (0.50 g) were homogenized in 3.0 mL of 2 % oxalic acid supplemented with 0.50 mL of 30 % ZnSO4 and 0.50 mL of 15% K4Fe(CN)6⋅3H2O. The volume of the extract was adjusted to 10.0 mL with 1% oxalic acid, followed by centrifugation at 10, 000 × g for 10 min. Then 3 mL of the supernatant was mixed with 2.0 mL of dye solution (0.25% DCIP and 0.20% NaHCO3) and 3 mL of dimethylbenzene. The absorbance was read at 500 nm, and the content of ASA was calculated using a standard curve. Total GSH was estimated using a kit (Jiancheng Bioengineering Institute, Nanjing, China). The absorbance of GSH was measured at 420 nm according to manufacturer’s instructions. CAT activity was measured according to the methods described by Velikova et al. (2000) using 10 mM potassium buffer (pH 7.00), with 100 μL of enzyme extract, and 33 mM H2O2. H2O2 was assayed from the decrease in absorbance in optical density at 240 nm, and the activity was calculated using the extinction coefficient of 40 mM cm-1 of H2O2. GR activity was determined by measuring the absorbance change at 340 nm due to oxidation of NADPH (Foyer and Halliwell, 1976). SOD and POD activities were assayed as described by Chen et al. (2013).
Statistical Analysis
Data were analyzed by one-way ANOVA using SPSS (version 21.0.0; IBM, Armonk, NY, United States). Different letters on the graphs or in the tables indicated that the mean values were statistically different at the P < 0.05 level.
Results
H2S Alleviates NaCl-Induced Inhibition of Cucumber Plant Growth
The morphology, survival rate, and expansion rate of the second leaf were determined after cucumber seedlings exposed to 200 mM NaCl for 7 days. Under salt stress, most seedlings began to show leaf wilting, lodging and inhibition of the second leaves compared with control group (Supporting information, Supplementary Figure S1). In order to analyze the alleviation effect of NaHS on growth inhibition induced by NaCl in a dose-dependent manner, series of concentrations of NaHS were added to the nutrient solution. In cucumber seedlings, 15 or 20 μM NaHS significantly alleviated the inhibition of plant growth during salt stress (Supplementary Figure S1A). Salinity treatment significantly (P < 0.05) reduced the survival rate by 89 %, the rate of expansion of the second leaf by 62 % (Supplementary Figures S1B,C) and significantly (P < 0.05) affected general growth (Figure 1A). Compared with the control, the shoot fresh weight, root fresh weight, seedling fresh weight, seedling total length, shoot dry weight, root dry weight, leaf area and root length were significantly (P < 0.05) reduced by 66%, 58%, 65%, 43%, 50%, 53%, 90%, and 48% under salt stress, respectively (Figures 1B–I). However, all growth parameters showed different degree ameliorating effects with application of different concentrations of NaHS (Figure 1 and Supplementary Figure S1). For example, the combination NaCl+15 μM NaHS significantly (P < 0.05) reduced shoot fresh weight, root fresh weight, seedling fresh weight, seedling total length, shoot dry weight, root dry weight, leaf area and root length by 28%, 40%, 31%, 14%, 17%, 42%, 41%, and 15%, respectively, as compared to the control (Figures 1B–I). Thus, these results indicated that exogenous supplementation with 15 μM NaHS was the most ameliorating effect in boosting cucumber tolerance to NaCl stress.
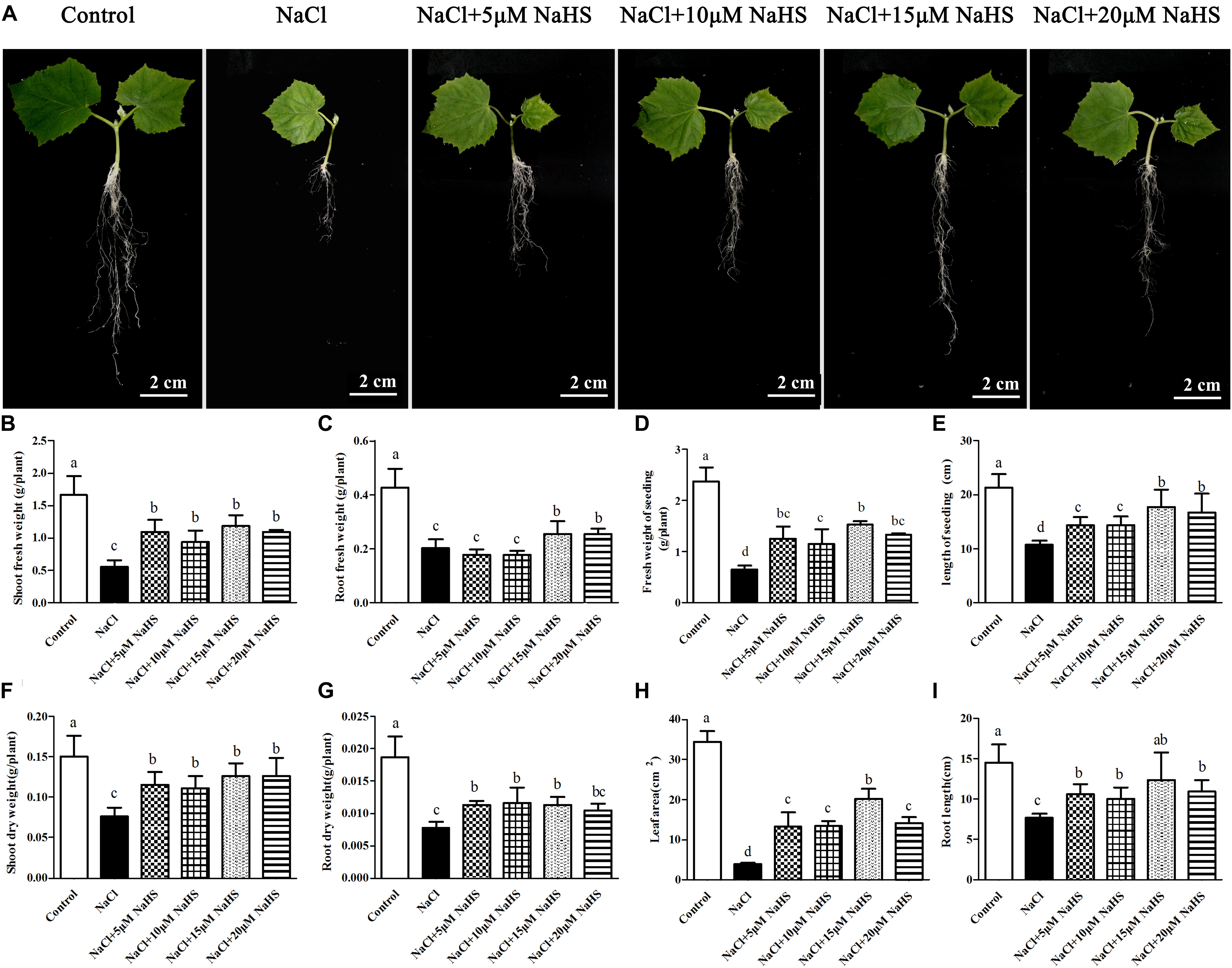
Figure 1. Effects of NaHS treatment on the NaCl-induced changes in cucumber seedling growth (A). Plant growth was evaluated by the indexes of shoot fresh weight (B), root fresh weight (C), fresh weight of seedling (D), length of seedling (E), shoot dry weight (F), root dry weight (G), leaf area (H) and root length (I) in Cucumis sativus L. cv. Chunxiaqiuwang grown under 200 mM NaCl. Each value is the mean of three biological replicates, and the vertical bars represent the standard errors. Values sharing the same lower case letters are insignificant as per Duncan’s test at P < 0.05.
Effects of H2S on Photosynthesis Under Excess NaCl
In comparison with the control, a significant (P < 0.05) decline in the Pn (by 14.3%) and a sharp decline in the Tr (by 94%) were recorded in the leaves of salt-stressed cucumber plants (Table 1). The combination of NaCl and 15 μM NaHS showed a decline in the Pn (by 2.4%) and Tr (by 90.4%) compared with the control, respectively (Table 1). Nevertheless, 5 μM NaHS did not significantly affect the Tr under salt stress. In addition, 20 μM NaHS alleviated the declines of Pn, but did not cause a significant change of Tr under salt stress.
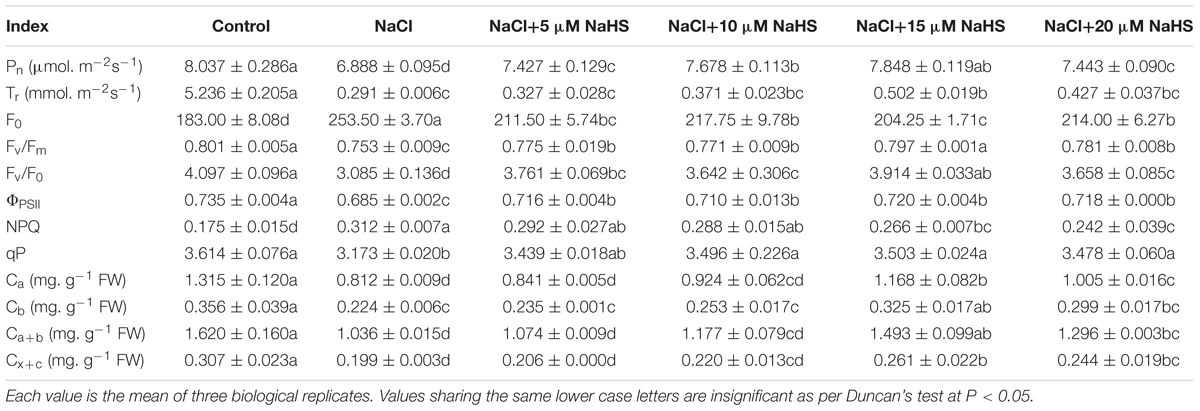
Table 1. Effects of NaHS treatment on the Pn, Tr, F0, Fv/Fm, Fv/F0, ΦPSII, NPQ, and qP values and the contents of Ca, Cb, Ca+b, and Cx+c in Cucumis sativus L. cv. Chunxiaqiuwang grown under 200 mM NaCl.
As shown in Table 1, salt stress significantly (P < 0.05) reduced Fv/Fm (by 6%), Fv/Fo (by 25%),ΦPSII (by 7%) and qP (by 12%) compared with the control, while 15 μM NaHS treatment reduced these by 0.5%, 4.5%, 2% and 3.1%, respectively, compared with the control. In contrast, comparing with the control, the parameters of Fo and NPQ significantly (P < 0.05) increased by 39% and 78% under salt stress. As expected, 15 μM NaHS significantly reversed the increase in Fo (by 10.4%) and NPQ (by 34.2%) under salt stress (Table 1).
Salt stress resulted in a decrease in the contents of the photosynthetic pigments Ca, Cb, Ca+b and Cx+c compared to the non-treated plants. Compared with the control, salt stress reduced Ca, Cb, Ca+b and Cx+c content by 38%, 37%, 36%, and 35%, respectively, while 15 μM NaHS treatment reduced Ca, Cb, Ca+b, and Cx+c content by 11.2%, 8.7%, 7.8% and 15% (Table 1).
Effects of H2S on the Stomatal Index Under Excess NaCl Conditions
To determine the effects of H2S on stomata in cucumber, the abaxial leaf surface was observed by a scanning electron microscope to analyze several stomatal indexes (Figures 2A–C). Salt stress induced stomatal closure and resulted in abnormal morphology of the epidermal and guard cells compared to the untreated control plants, whereas application of 15 μM NaHS significantly reversed these damages. Salt stress caused a noticeable reduction in stomatal opening rate by 67% (Figure 2D) and stomatal width by 74% (Figure 2E), and a significant increase in stomatal density by 41% (Figure 2F) and stomatal length by 36% (Figure 2G) compared with control plants. However, 15 μM NaHS treatment reduced stomatal opening rate by 30.8% (Figure 2D) and stomatal width by 52.8% (Figure 2E), and increased stomatal density by 12.6% (Figure 2F) and stomatal length by 12% (Figure 2G) compared with control plants. The diverse responses of the stomata indicated that there is co-ordination of the physiological (i.e., aperture) and morphological (i.e., stomatal density) changes induced by H2S that control gas exchange and Tr when encountering unfavorable environments.
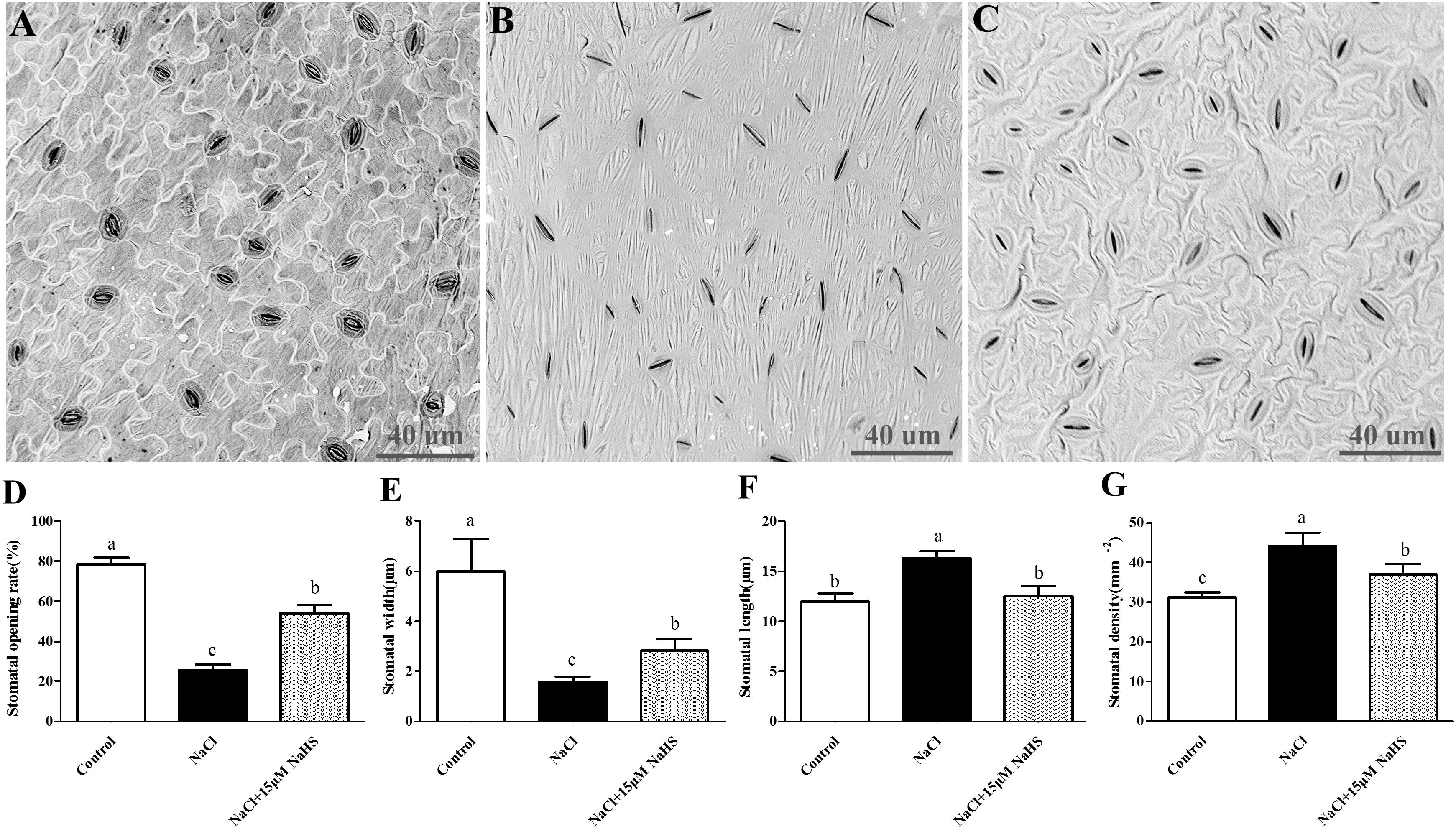
Figure 2. Scanning electron micrographs of abaxial leaf surfaces of cucumber showing the numbers and opening of stoma in control group (A), 200 mM NaCl treatment group (B) and the 15 μM NaHS + 200 mM NaCl treatment group (C). Effects of NaHS treatment on the changes of stomatal opening rate (D), stomatal width (E), stomatal length (F), and stomatal density (G) of Cucumis sativus L. cv. Chunxiaqiuwang under different treatments. Each value is the mean of three biological replicates, and the vertical bars represent the standard errors. Values sharing the same lower case letters are insignificant as per Duncan’s test at P < 0.05.
Effects of H2S Treatment on Electrolytic Leakage, Lipid Peroxidation, and ROS Accumulation Under Excess NaCl
The exposure of the cucumber plants to salinity significantly (P < 0.05) increased electrolyte leakage by 1.1 fold in leaves (Figure 3A) and by 87.2% in roots (Figure 3B), and increased the MDA content by 45% in leaves (Figure 3C) and by 1.0 fold in roots (Figure 3D) compared with the control. However, treatment of salt-stressed plants with 15 μM NaHS resulted in an increase in electrolyte leakage by 27.6% in leaves and by 46% in roots (Figures 3A,B) and an increase in the MDA content by 21% in leaves and by 5.4% in roots as compared to the control (Figures 3C,D). These results indicated that NaHS clearly reduces cell membrane injury.
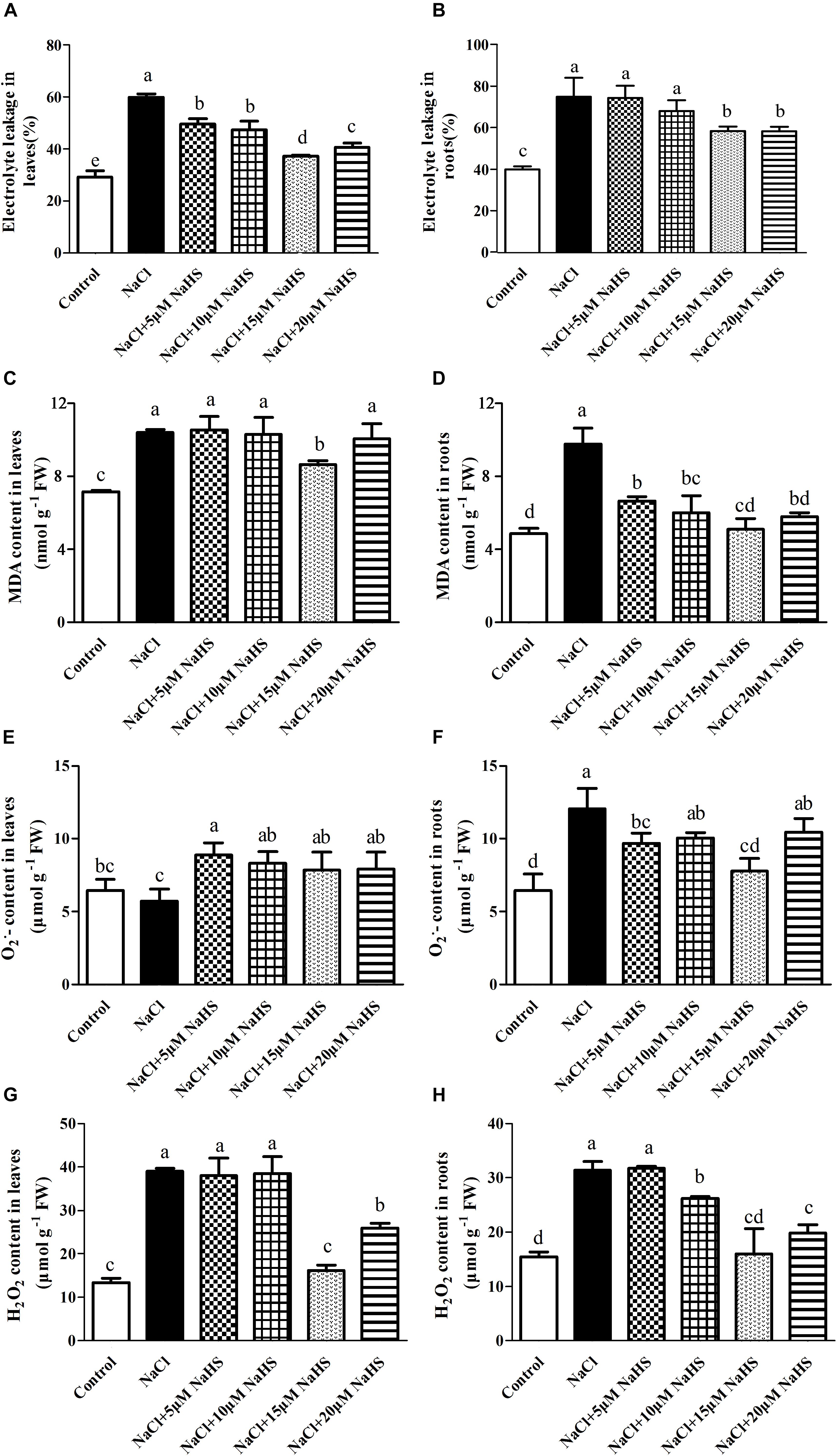
Figure 3. Effects of NaHS treatment on the NaCl-induced changes of electrolyte leakage in leaf (A) and root (B), MDA content in leaf (C) and root (D), O2•- in leaf (E) and root (F), and H2O2 content in leaf (G) and root (H) of Cucumis sativus L. cv. Chunxiaqiuwang. Each value is the mean of three biological replicates, and the vertical bars represent the standard errors. Values sharing the same lower case letters are insignificant as per Duncan’s test at P < 0.05.
Salt stress treatment did not change the O2•- accumulation in cucumber leaves (Figure 3E), but it did increase by nearly 2.1 fold in roots as compared to control plants (Figure 3F). While different concentration of NaHS treatment increased the accumulation of O2•- in cucumber leaves and roots as compared to the control (Figures 3E,F). Salt stress caused a dramatic increase in H2O2 by 1.92 fold in leaves (Figure 3G) and 1.04 fold in roots (Figure 3H) compared with the control. Treatment with 15 μM NaHS increased the H2O2 by 21.7% in leaves (Figure 3G) and 19.7% in roots compared with the control (Figure 3H). These results indicated that exogenous application of NaHS suppresses ROS accumulation, thereby protecting cucumber plants from NaCl-induced oxidative stress.
Effects of NaHS Treatment on Endogenous H2S Levels and H2S Metabolism Under Excess NaCl
In comparison with the control, a sharp decline in the content of endogenous H2S was recorded in leaves (by 49%) (Figure 4A) and roots (by 45%) (Figure 4B) of salt-treated cucumber plants, whereas 15 μM NaHS treatment improved the content of endogenous H2S by 9.9% in leaves (Figure 4A) and by 9.1% in roots (Figure 4B) compared with the control. Compared with the control, salt stress significantly (P < 0.05) decreased the activity of L-CD by 19% in leaves (Figure 4C) and 12% in roots (Figure 4D). The same change was found in the activity of D-CD after salt stress, which reduced by 13% in leaves (Figure 4E) and 33 % in roots compared with the control (Figure 4F), As expected, these changes were reverted by different concentrations of NaHS treatment. For example, treatment with 15 μM NaHS significantly (P < 0.05) improved the activities of L-CD (38.7% in roots) and D-CD (by 52.7% in leaves and 4.6 fold in roots) compared with the control (Figures 4C–F). These results indicated that NaHS treatment significantly enhanced the activities of L-CD and D-CD in cucumber seedlings under salt stress.
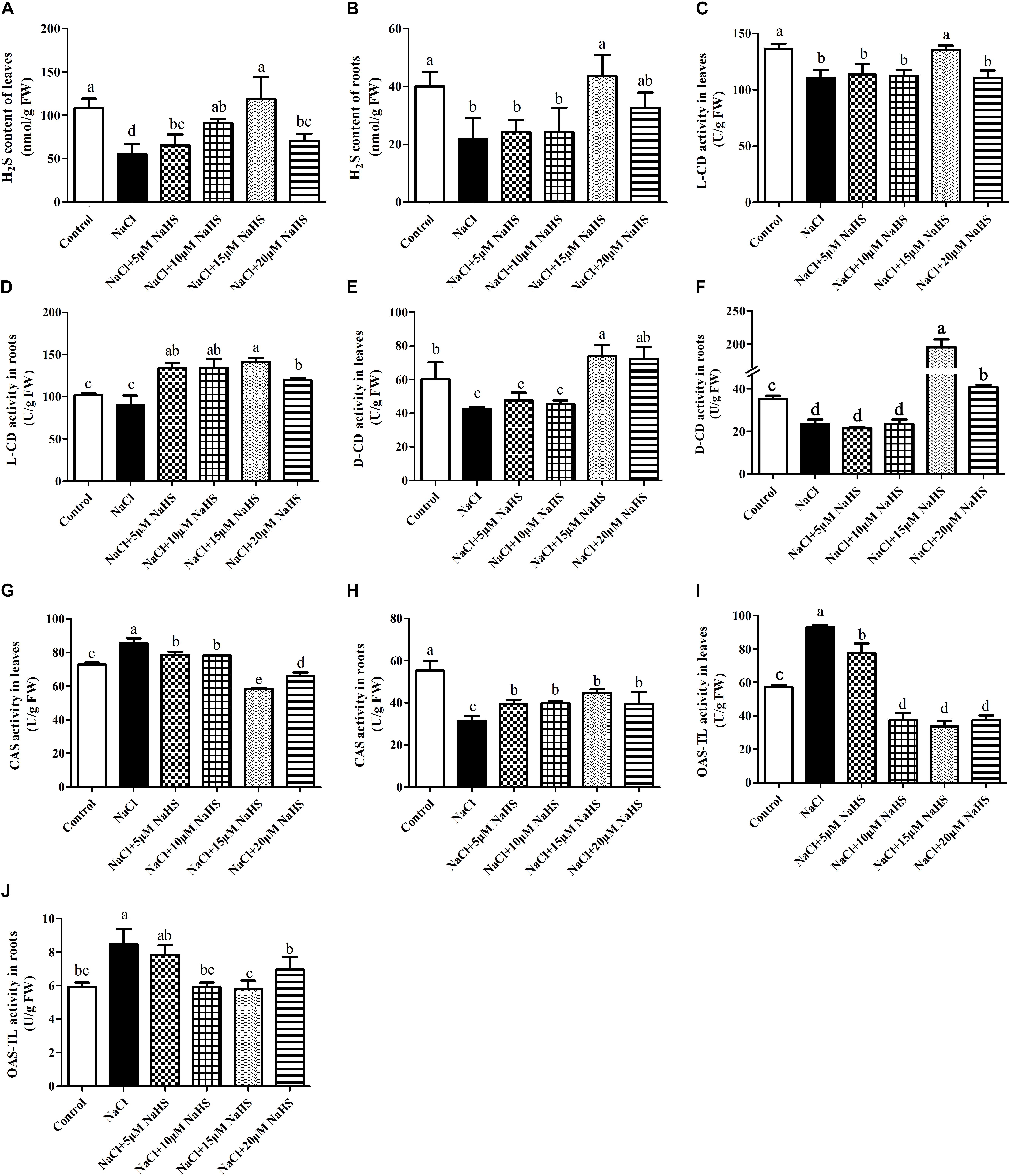
Figure 4. Effects of NaHS treatment on the NaCl-induced changes in endogenous H2S levels in leaf (A) and root (B) and activities of L-CD in leaf (C) and root (D), D-CD in leaf (E) and root (F), CAS in leaf (G) and root (H), and OAS-TL in leaf (I) and root (J) of Cucumis sativus L. cv. Chunxiaqiuwang. Each value is the mean of three biological replicates, and the vertical bars represent the standard errors. Values sharing the same lower case letters are insignificant as per Duncan’s test at P < 0.05.
As for the activity of CAS, a completely opposite trend was found between leaves and roots. Salt stress significantly (P < 0.05) improved the activity of CAS in leaves by 19% (Figure 4G) and reduced it by 43% in roots (Figure 4H) compared with the control. Application of 5 or 10 μM NaHS enhanced the activity of CAS by 9.4% or 8.9% in leaves, in contrast, 15 or 20 μM NaHS reduced it by 31.7% or 22.8% compared with the control. In the roots, 5∼20 μM NaHS all reduced the activity of CAS in different degree compared with the control group (Figures 4G,H). In addition, the OAS-TL activity significantly (P < 0.05) increased in both leaves (by 65%) (Figure 4I) and roots (43%) (Figure 4J) under salt stress. Similarly, these effects were reverted by different concentrations of NaHS as compared to the plants receiving salinity treatment alone. For example, 15 μM NaHS treatment reduced the activity of OAS-TL by 42% in leaves and 2.4% in roots as compared to the control (Figures 4I,J).
Effects of H2S on Na+ and K+ Homeostasis Under Excess NaCl
In plants, maintaining a lower Na+/K+ ratio is critical to its ability to tolerate salt stress. Compared with the control, a sharp increase in the Na+ content was observed in leaves (by 2.13 fold) and roots (1.35 fold) under salt stress (Figures 5A,B). On the contrary, a significant decrease in the K+ content was recorded in leaves (by 30%) and roots (by 41%) under salt stress as compared to the control (Figures 5C,D). Accordingly, the Na+/K+ ratio significantly increased in leaves (by 3.4 fold) and roots (by 3 fold) of salt-treated plants than the control (Figures 5E,F). As expected, treatment with 15 μM NaHS improved the Na+ content in leaves by 1.9 fold and roots by 1.0 fold, and reduced the K+ content in leaves by 17.8% and roots by 28% compared with the control, indicating that 15 μM NaHS significantly reversed the increase of Na+ and the decrease of K+ under salt stress. In addition, the Na+/K+ ratio was significantly lower in the NaHS-treated plants when compared with the plants treated with salt alone (Figures 5E,F). Thus, H2S eases excessive Na+ uptake and maintains the Na+/K+ homeostasis at the cellular level in cucumber plants under salt stress.
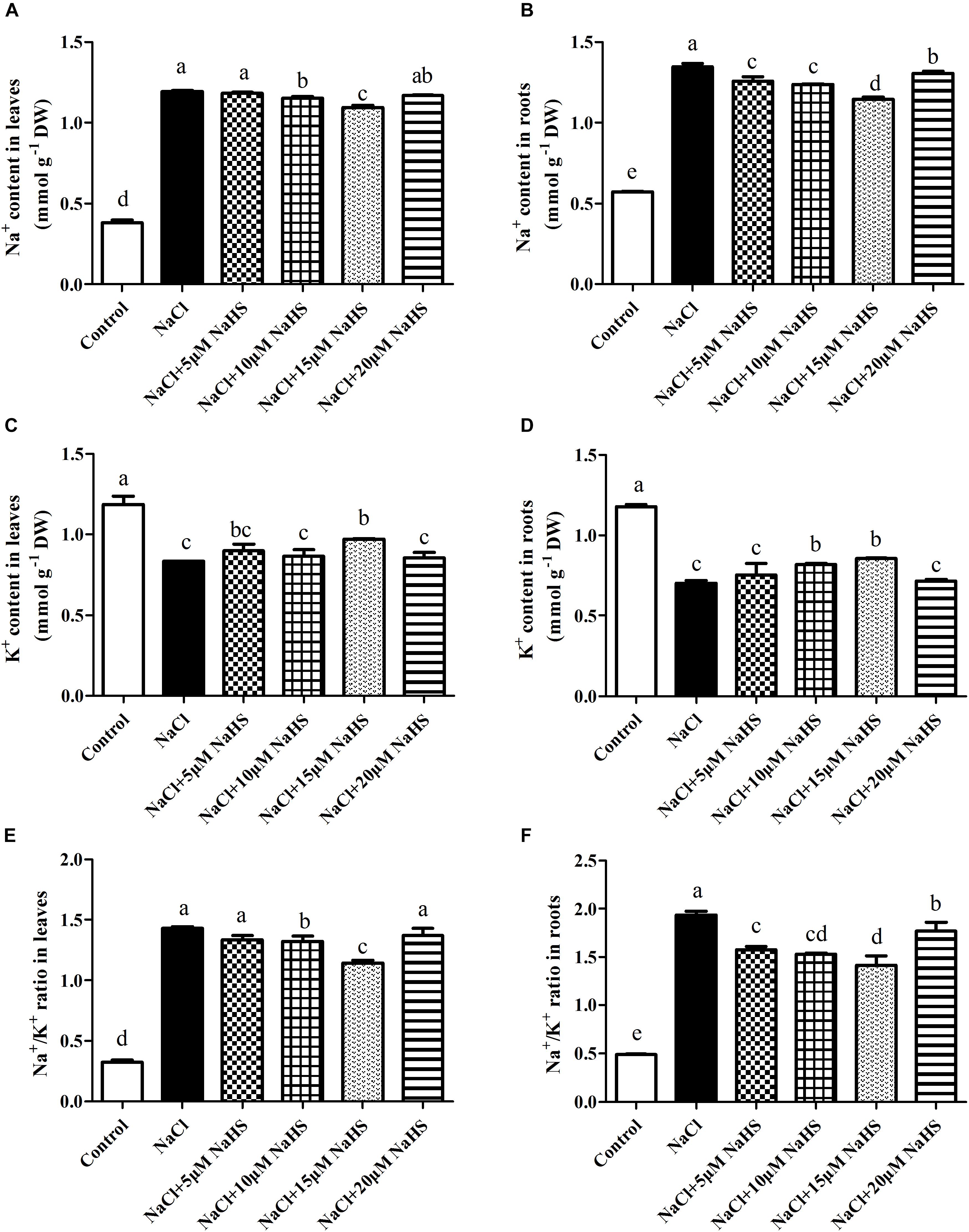
Figure 5. Effects of NaHS treatment on the NaCl-induced changes of Na+ content (A,B), K+ content (C,D), and Na+/K+ ratio (E,F) in leaf and root of Cucumis sativus L. cv. Chunxiaqiuwang grown under 200 mM NaCl. Each value is the mean of three biological replicates, and the vertical bars represent the standard errors. Values sharing the same lower case letters are insignificant as per Duncan’s test at P < 0.05.
Effects of H2S on Expression of PM H+-ATPase, SOS1, and SKOR Under Excess NaCl
To further characterize the effect of H2S on the modulation of Na+/K+ homeostasis in cucumber seedlings upon salt stress, the transcript levels of PM H+-ATPase, SOS1 and SKOR were analyzed. Real-time RT-PCR analysis showed that NaCl treatment resulted in the significant decreases in the transcripts levels of PM H+-ATPase by 66.9% (Figure 6A), SOS1 by 42.7% (Figure 6C) and SKOR by 72.4% (Figure 6E) in cucumber leaves compared with the controls, respectively. In contrast, the relative expression of PM H+-ATPase, SOS1 and SKOR were significantly increased by 72.6% (Figure 6B), 2.3 fold (Figure 6D) and 1.17 fold (Figure 6F) in the roots under NaCl stress compared with the control. The NaCl-induced increases in PM H+-ATPase, SOS1 and SKOR transcripts were significantly reversed by 15 μM NaHS treatments in the cucumber roots. As shown in Figure 6, compared with the control, treatment with 15 μM NaHS+NaCl reduced the relative expression of PM H+-ATPase, SOS1 and SKOR by 57.1%, 59.5% and 75.1% in leaves, respectively. On the contrary, treatment with 15 μM NaHS+NaCl improved the relative expression of PM H+-ATPase, SOS1 and SKOR by 2.1 fold, 2.59 fold and 3.21 fold in leaves, respectively, as compared to the control.
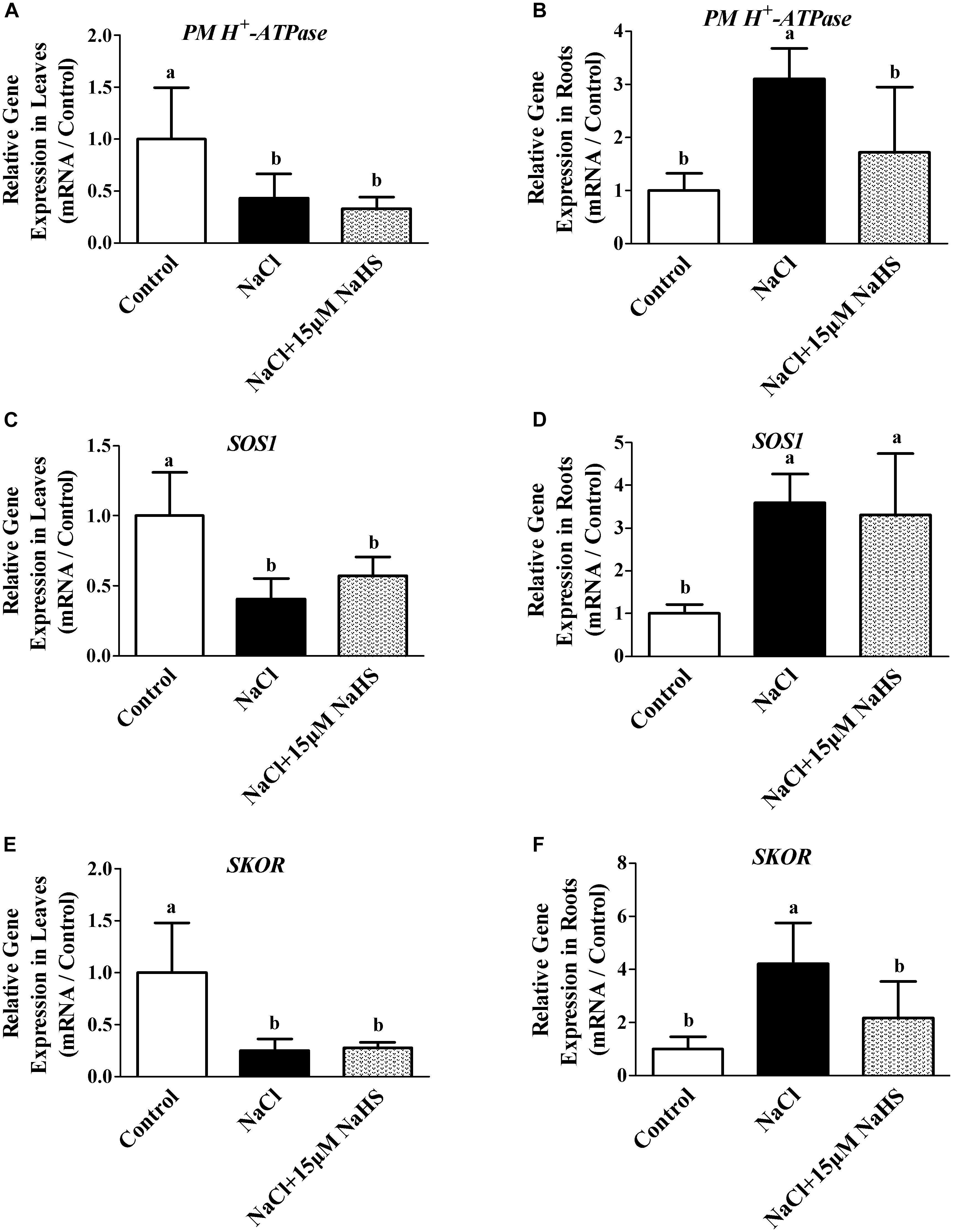
Figure 6. Effects of NaHS treatment on the NaCl-induced changes in expression of PM H+-ATPase in leaf (A) and root (B), SOS1 in leaf (C) and root (D), and SKOR in leaf (E) and root (F) in Cucumis sativus L. cv. Chunxiaqiuwang grown under different treatments. Each value is the mean of three biological replicates, and the vertical bars represent the standard errors. Values sharing the same lower case letters are insignificant as per Duncan’s test at P < 0.05. Total RNAs were extracted from roots and leaves and subjected to reverse transcription followed by real-time PCR. GAPDH was used as an internal control. The transcript level in roots and leaves in the control group was defined as “1” for each gene, and other treated samples were expressed relative to the corresponding control. The columns labeled with different letters are significantly different at P < 0.05. Error bars are SE (n = 3).
Effects of H2S on the Antioxidant System
To evaluate the role of H2S in mediating salt-induced antioxidant system, the levels of non-enzymatic antioxidants (ASA and GSH) and the activities of antioxidant enzymes (SOD, CAT, GR, and POD) were analyzed in leaves and roots of cucumber plants. As show in Table 2, compared with the control, the contents of ASA and GSH and the activity of GR were significantly increased by 98%, 73%, and 61% in the leaves, but they were decreased by 88%, 23%, and 2.25 fold, in the roots under NaCl treatment. However, application of exogenous NaHS ameliorated the effect of salt stress. For example, 15 μM NaHS treatment significantly increased the ASA content by 42.6% and the GR activity by 9.1%, and decreased GSH by 44% in the leaves as compared to the control. On the contrary, 15 μM NaHS treatment decreased the ASA content by 23.9% and the GR activity by 27.8%, and increased GSH by 48.7% in the roots as compared to the control. Compared with the control, SOD activity was increased under salt stress in the leaves by 23% and roots by 43%. Application of NaHS showed a synergistic effect resulting in decreased SOD activity in the leaves and roots under NaCl stress. The application 15 μM NaHS significantly decreased SOD activity in the leaves by 19% and roots by 15% as compared to NaCl stress alone. The NaCl treatment significantly decreased the activity of CAT in the leaves by 6 % and roots by 59% as compared to control, but increased the POD activity in the leaves by 25% and roots by 10%. Application of NaHS reversed these changes in the leaves and roots induced by salt stress.
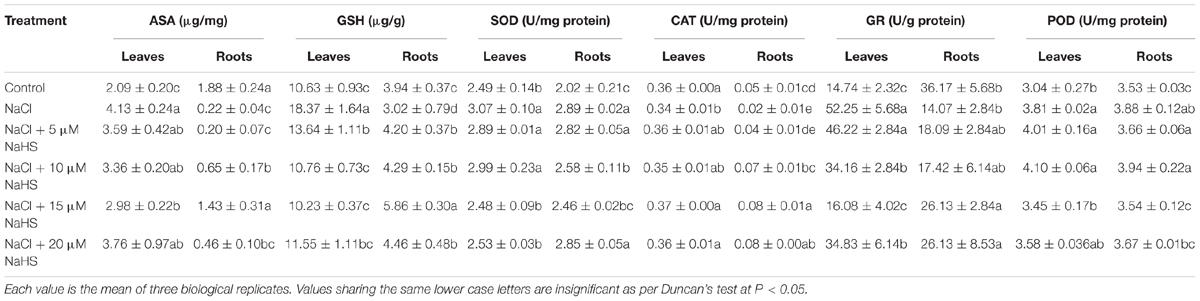
Table 2. Effects of NaHS treatment on ASA and GSH contents and the activities of SOD, CAT, GR, and POD in leaves and roots of Cucumis sativus L. cv. Chunxiaqiuwang grown under 200 mM NaCl stress.
Discussion
In plants, some small biological molecules, such as phytohormones and signal molecules could be considered as a powerful tool in modifying plants’ adaptability against adverse environment (Mostofa et al., 2015a). By exogenously application of the H2S donor NaHS, numerous results demonstrated that H2S participates in plant adaptive responses against multiple abiotic stresses (Lai et al., 2014). Ameliorative effects of H2S treatment are a trending topic among researchers that work on abiotic stress in plants, accordingly there are various studies in the literature that investigate how this molecule increase plant stress tolerance. However, most of the studies are limited to a certain topic such as only photosynthesis or Na+ and K+ homeostasis or antioxidant defense. A comprehensive study that investigates several issues in the same experimental setup and provides evidence on how H2S alleviates detrimental effects of salt stress is still limited. Our hydroponically based study aids in understanding how plants respond under NaCl-toxicity and how the use of H2S is capable of reverting this toxicity.
H2S Alleviates NaCl-Induced Inhibition of Plant Growth
Results showed that salinity stress inhibited plant growth performance as judged by decreased shoot fresh weight, root fresh weight, shoot dry weight, root dry weight, leaf area and root length compared with control, but this inhibition was significantly alleviated by H2S donor NaHS (Figure 1). Similarly, the reversion of salt stress responses by H2S has been observed in other plant species, like Medicago sativa (Lai et al., 2014), Oryza sativa (Mostofa et al., 2015b) and Triticum aestivum (Deng et al., 2016). However, it is well-known that H2S must be within a rather narrow concentration range to act as a signal molecule and that high concentrations of H2S induce a toxicity response. In our study, the capacity of H2S to enhance salt tolerance firstly increased and then decreased with the concentration improvement. Ameliorative effects of phenotypes were seen from 5 to 15 μM NaHS, but plants exhibited slight toxicity under the 20 μM NaHS treatment. It may be that excessive high levels of H2S might lead to the ROS over-production, which further leads to the inhibition of the mitochondrial electron transport chain (Mancardi et al., 2009). In our study, 20 μM NaHS treatment indeed caused the ROS over-production and cell membrane injury than 15 μM NaHS as judged by the parameters of electrolyte leakage, MDA, O2•- and H2O2 (Figure 3). Hence, the use of H2S is a double-edged sword, and the maintenance of a suitable level of intracellular H2S is key conferring salt tolerance in cucumber seedlings.
H2S Alleviates the NaCl-Induced Declines of Photosynthetic Attributes
In this study, the Fv/Fm was significantly decreased under salt stress, and they were remarkably improved with addition of 15 μM NaHS (Table 1). Similarly, the results of Christou et al. (2013) showed that 100 mM NaCl stress significantly decreased the values of Fv/Fm, while 100 μM NaHS significantly reversed the decline of Fv/Fm. In addition, a phenomenon that H2S prevented the reduction of photosynthetic pigments Ca, Cb, Ca+b, and Cx+c induced by salt stress was also observed in our study (Table 1). The results are consistent with these of Mostofa et al. (2015b), who reported that H2S improved overall growth and biomass of salt-stressed rice plants which could be attributed to its role in protecting Chla, Chlb and Cx+c from salt-induced damage. As the photosynthetic parameters Pn and Tr, our results showed that H2S also alleviated the decrease in the photosynthetic parameters Pn and Tr. These results are consistent with these of Dawood et al. (2012), who reported that H2S reduced the Aluminum-induced inhibition of Pn, Gs and Tr. Another study also demonstrated a similar result that NaHS treatment increased the Pn and Gs values in Spinacia oleracea seedlings under drought (Chen et al., 2016). Together these results suggest that H2S plays a role in plant photosynthesis.
Exogenous H2S Changes Endogenous H2S Accumulation Under Excess NaCl
Earlier investigations demonstrated that hypoxia, salt and Cd stress induce notable increases in endogenous H2S production in different plant species, such as pea, strawberry, alfalfa and Chinese cabbage (Cheng et al., 2013; Christou et al., 2013; Lai et al., 2014; Zhang et al., 2015). The activation of endogenous H2S level after stress treatments indicated that H2S might also be an important secondary messenger of stress sensing which in turn modulated plant physiological changes and downstream gene expressions (Christou et al., 2013). Surprisingly, a remarkable decline in endogenous H2S production was observed in cucumber leaves and roots upon treatment with 200 mM NaCl for 7 days in our experiment. Further investigation is still required to detail the reason. Although different kinds of environmental stress factors caused differential dynamic changes in endogenous H2S metabolism, exogenously applied NaHS, a well-known H2S donor, all greatly enhanced H2S concentration.
Generally, synthesize H2S in plants is associated with the enzymes L-CD, D-CD, OAS-TL or CAS, often in response to environmental stress, leading to accumulation of endogenous H2S and the acquisition of stress tolerance. The activities of L-CD in leaves and D-CD in both roots and leaves all showed a significantly decreased after salt stress treatment as compared to the control (Figures 4C–F). Lai et al. (2014) found that NaCl could induce L-CD in Medicago sativa roots, while the total activity of D-CD was not significantly altered. Meanwhile the expression of L-CD and D-CD at the transcripts level were strongly induced by drought stress in Arabidopsis thaliana (Jin et al., 2011). In our study, exogenously applied 15 μM NaHS significantly enhanced the activities of D-CD in both roots and leaves and L-CD in roots compared with the control or salt stress group (Figures 4D–F). However, Cheng et al. (2013) found that exogenously applied NaHS decreased the total activity of L-CD and D-CD in Pisum sativum L. seedlings under hypoxia stress. In addition, NO could induce the activities of to L-CD, OAS-TL and CAS regulate the endogenous H2S biosynthesis in maize roots (Peng et al., 2016). In our study, salt-stress significantly improved the activity of CAS in leaves and OAS-TL in leaves and roots and significantly reduced the activity of CAS in roots (Figures 4G–J). This is also in line with a report of Cheng et al. (2013), and their results showed that exogenously applied NaHS enhanced the activity of CAS and OAS-TL in Pisum sativum L. seedlings under hypoxia stress (Cheng et al., 2013).
H2S Maintains Na+ and K+ Homeostasis by Regulating Expression of PM H+-ATPase, SOS1, and SKOR Genes Under Excess NaCl
Maintenance of Na+ and K+ ion homeostasis is critically important for plants to survive under salt stress. Our study provided firm evidence that H2S prevented Na+ uptake and improved K+ uptake in both leaves and roots, thereby restraining the salt-induced decrease of the Na+/K+ ratio (Figure 5). Similarly, Lai et al. (2014) observed that the biologically beneficial role of NaHS was due to its specific ability to retain K+, thus preventing the increase of the Na+/K+ ratio in stellar cells under salt stress. In addition, Christou et al. (2013) showed that H2S regulates the expression of SOS pathway genes and kept the balance of Na+ and K+ in strawberry plants under salt stress. Deng et al. (2016) also found that H2S significantly improved the salt tolerance of wheat seedlings by regulating the membrane-bound translocation proteins of the SOS1 pathways to increase Na+ extrusion and decrease Na+ uptake. Interestingly, salt stress induced the up-regulation of PM H+-ATPase, SOS1 and SKOR at the transcript levels in roots compared with the control, but a completely opposite trend in leaves. This is also in line with a report of Wang et al. (2013), and their results showed that the expression of CsSOS1 increased in roots and decreased in leaves with the concentration of NaCl increasing in cucumber. Perhaps the root irrigation treatment method in this study affected the expression of genes or some signal first in roots. In our study, H2S alleviated the NaCl-induced increases in PM H+-ATPase expression in roots. It has been known that NaCl stress also up-regulates the expression of genes encoding PM H+-ATPase in roots (Shi et al., 2000). Moreover, it is well known that PM H+-ATPase can sustain an H+ gradient by promoting Na+ efflux and H+ influx to drive Na+/H+ antiport across the plasma membrane. It was also found that the administration of NaHS effectively prevented the NaCl-triggered K+ efflux in the mature zone of alfalfa seedling roots, which might be partially related to the SKOR channel (Lai et al., 2014). These studies suggested that salt-tolerant need plants have an ability to prevent transport of Na+ from roots to shoots and an improved ability to exclude Na+ from the roots (Takahashi et al., 2007a,b).
H2S Regulates the Antioxidant System and Reduces Oxidative Stress and Membrane Lipid Peroxidation Under Excess NaCl
Plants suffering from NaCl toxicity often exhibit symptoms associated with oxidative stress and membrane lipid peroxidation, which can result in accumulation of ROS and MDA (Hossain et al., 2005). In this study, high salinity triggered overproduction of O2•- and H2O2, increased the electrolyte leakage, and caused accumulation of MDA in both cucumber leaves and roots (Figure 3). H2S alleviated the salinity-induced oxidative damage, as evident by reduced levels of O2•-, H2O2, MDA and electrolyte leakage in both cucumber leaves and roots (Figure 3). This is in line with a report of Mostofa et al. (2015b), and their results showed that H2S reduced the salt-induced increase in the levels of O2•-, H2O2 and MDA in rice. Similar results were also reported by Chen et al. (2016), who demonstrated that NaHS treatment counteracted the accumulation of ROS and increased the MDA content of Spinacia oleracea L. seedlings under drought. The balance between ROS production and antioxidant defense determines the extent of oxidative damage within the plant. In our study, NaHS treatment significantly alleviated the improvement of ASA and GSH contents and SOD, GR, and POD activities in leaves of cucumber under salt stress (Table 2). In cucumber roots, H2S also successfully counteracted the decline in the content of ASA and GSH and the activities of CAT, GR and POD under salt stress (Table 2), indicating that H2S may enhance the ability of cucumber roots to scavenge ROS so that the plant can maintain membrane integrity under salt stress. The similar result was reported by Wang et al. (2012), who founded that NaHS pretreatment significantly activated the antioxidant enzymes activities including SOD, CAT, POD, and APX and their expression at the transcripts level under 100 mM NaCl stress in Medicago sativa L., thus resulting in the alleviation of oxidative damage induced by NaCl.
Conclusion
In conclusion, exogenous application of H2S alleviates the NaCl-induced toxicity via improving the growth and photosynthetic parameters in cucumber, which could be attributed to three mechanisms: (1) H2S increases endogenous H2S levels via increasing the activities of L-CD and D-CD and decreasing the activities of CAS and OAS-TL in leaves or roots; (2) H2S maintains the Na+ and K+ balance through regulating the expression of PM H+-ATPase, SOS1 and SKOR; and (3) H2S enhances the accumulation of antioxidants and the activities of antioxidant enzymes, leading to decline ROS accumulation and membrane lipid peroxidation. As summarized in Figure 7, H2S alleviates salt stress in cucumber through cross talk among the mechanisms that maintain Na+/K+ homeostasis and regulating H2S metabolism and oxidative stress response. In addition, the roots and leaves of cucumber showed different responses to exogenous H2S treatment. Taken together, suitable concentration H2S could be used to alleviate NaCl-stress induced toxicity in cucumber seedlings.
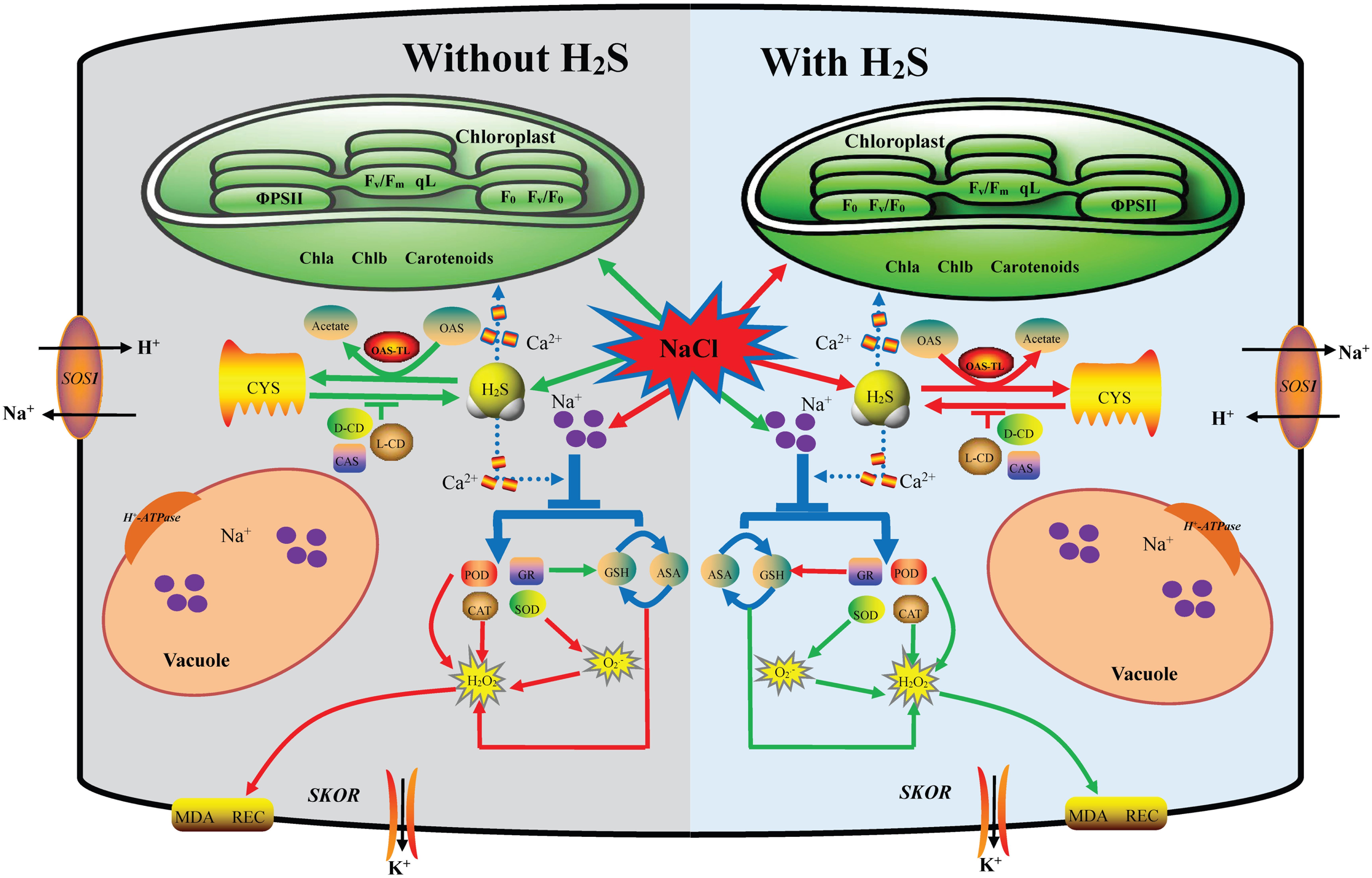
Figure 7. A proposed model for H2S-mediated salt stress responses in cucumber. This model depicting how H2S regulates the enhanced salt tolerance by mediating maintaining the Na+/K+ balance and regulating endogenous H2S metabolism and oxidative stress response. Under salt stress, on the one hand, the application of NaHS reversed the expression up-regulation of PM H+-ATPase, SOS1, and SKOR in roots to keep Na+ and K+ balance. On the other hand, the application of NaHS increase L/D-CD activity and decrease CAS and OAS-TL activities to regulating endogenous H2S metabolism. Additionally H2S altered the accumulation of antioxidants and the activities of antioxidant enzymes, leading to reduced ROS accumulation and membrane lipid peroxidation. The red arrow indicated up-regulation or increase and the green arrow indicated down-regulation or decrease in this model.
Author Contributions
J-LJ designed the experiment and wrote the manuscript. YT and X-MR conducted the experiment. MY and R-PH helped in data analysis and presentation. LL revised the manuscript. All authors listed have made a substantial, direct and intellectual contribution to the work.
Funding
This research was supported by the National Natural Science Foundation of China (31660153), Shaanxi Province Key Research and Development Project (2018NY-042), China, and Key Research Project of Shaanxi Provincial Department of Education (18JS017), China.
Conflict of Interest Statement
The authors declare that the research was conducted in the absence of any commercial or financial relationships that could be construed as a potential conflict of interest.
Acknowledgments
We thank Anita K. Snyder, M.Sc. for the helpful suggestions and language polishing on this manuscript.
Supplementary Material
The Supplementary Material for this article can be found online at: https://www.frontiersin.org/articles/10.3389/fpls.2019.00678/full#supplementary-material
FIGURE S1 | Effects of NaHS treatment on the morphological changes (A), survival rate (B), and expanding rate of the second leaf (C) in seedlings of Cucumis sativus L. cv. Chunxiaqiuwang grown under 200 mM NaCl. Each value is the mean of three biological replicates, and the vertical bars represent the standard errors. Values sharing the same lower case letters are insignificant as per Duncan’s test at P < 0.05.
Abbreviations
ΦPSII, Quantum yield of photosystem II photochemistry; 1O2, Singlet oxygen; ANOVA, One-way analysis of variance; APX, Ascorbate peroxidase; ASA, Ascorbic acid; CAS, Cyanoalanine synthase; CAT, Catalase; CO, Carbon monoxide; Cx+c, Carotenoid; D-CD, D-cysteine desulfhydrase; DCIP,2,6-dichlorophenolindophenol; DTT, DL-dithiothreitol; EC1, Initial electrical conductivity; EC2, Second electrical conductivity; EDTA, Ethylenediaminetetraacetic acid; ETR, Electron transport rate; Fm, Maximal chlorophyll fluorescence; Fm′, Maximum fluorescence level in the light-adapted state; Fo, Minimal chlorophyll fluorescence; Fo′, Minimal fluorescence level in the light-adapted state; Fv/Fo, Ratio of variable to minimal fluorescence; Fv/Fm, Ratio of variable to maximum fluorescence; FW, Fresh weight; GPX, Glutathione peroxidase; GR, Glutathione reductase; GSH, Glutathione; H+-ATPase, ATP-dependent proton pump; H2O2, Hydrogen peroxide; H2S, Hydrogen sulfide; K+, Potassium ions; L-CD, L-cysteine desulfhydrase; MDA, Malondialdehyde; Na+, Sodium ions; NADPH, Nicotinamide adenine dinucleotide phosphate; NaHS, Sodium hydrosulfide; NO, Nitric oxide; NPQ, Non-photochemical quenching parameters; NSCCs, Non-selective cation channels; O2•-, Superoxide radical anions; OAS-TL, O-acetyl-(thiol)-serinelyase; OH, Hydroxyl radicals; PAR, Photosynthetically active radiation; PM H+-ATPase, ATP-dependent proton pump of the plasma membrane; Pn, Net photosynthetic rate; POD, Peroxidase; PSII, Photosystem II; QA, primary electron acceptor of PSII; qP, Photochemical quenching; qRT-PCR, Quantificational real-time polymerase chain reaction; ROS, Reactive oxygen species; RT-PCR, Real-time polymerase chain reaction; SKOR, Shaker-like K+ outward-rectifying channel; SOD, Superoxide dismutase; SOS, Salt overly sensitive; TBA, Thiobarbituric acid; TCA, Trichloroacetic acid; Tr, Transpiration rate.
References
Abdel Latef, A. A. H., Mostofa, M. G., Rahman, M. M., Abdel-Farid, I. B., and Tran, L. S. P. (2019). Extracts from yeast and carrot roots enhance maize performance under seawater-induced salt stress by altering physio-biochemical characteristics of stressed plants. J. Plant Growth Regul. 1–14. doi: 10.1007/s00344-018-9906-81-14
Binzel, M. L. (1995). NaCl induced accumulation of tonoplast and plasma membrane H+-ATPase message in tomato. Physiol. Plant 94, 722–728. doi: 10.1111/j.1399-3054.1995.tb00990.x
Bloem, E., Riemenschneider, A., Volker, J., Papenbrock, J., Schmidt, A., Salac, I., et al. (2004). Sulphur supply and infection with Pyrenopeziza brassicae influence L-cysteine desulphydrase activity in Brassica napus L. J. Exp. Bot. 55, 2305–2312. doi: 10.1093/jxb/erh236
Chen, J., Shang, Y. T., Wang, W. H., Chen, X. Y., He, E. M., Zheng, H. L., et al. (2016). Hydrogen sulfide-mediated polyamines and sugar changes are involved in hydrogen sulfide-induced drought tolerance in Spinacia oleracea seedlings. Front. Plant Sci. 7:1173. doi: 10.3389/fpls.2016.01173
Chen, J., Wang, W. H., Wu, F. H., He, E. M., Liu, X., Shangguan, Z. P., et al. (2015). Hydrogen sulfide enhances salt tolerance through nitric oxide-mediated maintenance of ion homeostasis in barley seedling roots. Sci. Rep. 5:12516. doi: 10.1038/srep12516
Chen, J., Wang, W. H., Wu, F. H., You, C. Y., Liu, T. W., Dong, X. J., et al. (2013). Hydrogen sulfide alleviates aluminum toxicity in barley seedlings. Plant Soil 362, 301–318. doi: 10.1007/s11104-012-1275-7
Cheng, W., Zhang, L., Jiao, C. J., Su, M., Yang, T., Zhou, L. N., et al. (2013). Hydrogen sulfide alleviates hypoxia-induced root tip death in Pisum sativum. Plant Physiol. Biochem. 70, 278–286. doi: 10.1016/j.plaphy.2013.05.042
Christou, A., Manganaris, G. A., Papadopoulos, I., and Fotopoulos, V. (2013). Hydrogen sulfide induces systemic tolerance to salinity and non-ionic osmotic stress in strawberry plants through modification of reactive species biosynthesis and transcriptional regulation of multiple defence pathways. J. Exp. Bot. 64, 1953–1966. doi: 10.1093/jxb/ert055
Cui, J. X., Zhou, Y. H., Ding, J. G., Xia, X. J., Shi, K., Chen, S. C., et al. (2011). Role of nitric oxide in hydrogen peroxide-dependent induction of abiotic stress tolerance by brassinosteroids in cucumber. Plant Cell Environ. 34, 347–358. doi: 10.1111/j.1365-3040.2010.02248.x
Dawood, M., Cao, F. B., Jahangir, M. M., Zhang, G. P., and Wu, F. B. (2012). Alleviation of aluminum toxicity by hydrogen sulfide is related to elevated ATPase, and suppressed aluminum uptake and oxidative stress in barley. J. Hazard. Mater. 20, 121–128. doi: 10.1016/j.jhazmat.2011.12.076
Deng, Y. Q., Bao, J., Yuan, F., Liang, X., Feng, Z. T., and Wang, B. S. (2016). Exogenous hydrogen sulfide alleviates salt stress in wheat seedlings by decreasing Na+ content. Plant Growth Regul. 79, 391–399. doi: 10.1007/s10725-015-0143-x
Diao, M., Ma, L., Wang, J. W., Cui, J. X., Fu, A. F., and Liu, H. Y. (2014). Selenium promotes the growth and photosynthesis of tomato seedlings under salt stress by enhancing chloroplast antioxidant defense system. J. Plant Growth Regul. 33, 671–682. doi: 10.1007/s00344-014-9416-2
Feki, K., Quintero, F. J., Khoudi, H., Leidi, E. O., Masmoudi, K., Pardo, J. M., et al. (2014). A constitutively active form of a durum wheat Na+/H+ antiporter SOS1 confers high salt tolerance to transgenic Arabidopsis. Plant Cell Rep. 33, 277–288. doi: 10.1007/s00299-013-1528-9
Foyer, C. H., and Halliwell, B. (1976). The presence of glutathione and glutathione reductase in chloroplasts: a proposed role in ascorbic acid metabolism. Planta 133, 21–25. doi: 10.1007/BF00386001
Gaymard, F., Pilot, G., Lacombe, B., Bouchez, D., Bruneau, D., Boucherez, J., et al. (1998). Identification and disruption of a plant shaker-like outward channel involved in K+ release into the xylem sap. Cell 94, 647–655. doi: 10.1016/S0092-8674(00)81606-2
Gupta, P., Srivastava, S., and Seth, C. S. (2017). 24-Epibrassinolide and sodium nitroprusside alleviate the salinity stress in Brassica juncea L. cv. Varuna through cross talk among proline, nitrogen metabolism and abscisic acid. Plant Soil 411, 483–498. doi: 10.1007/s11104-016-3043-6
Horneck, D. A., and Hanson, D. (1998). “Determination of potassium and sodium by flame emission spectrophotometry,” in Handbook of Reference Methods for Plant Analysis, ed. Y. P. Karla (Washington DC: CRC Press), 158–160.
Hossain, M., Naher, F., and Shahabuddin, Q. (2005). Food security and nutrition in Bangladesh: progress and determinants. Electron. J. Agric. Dev. Econ. 2, 103–132. doi: 10.1111/mcn.12260
Hu, Y., and Yu, D. (2014). Brassinosteroid insensitive2 interacts with abscisic acid insensitive5 to mediate the antagonism of brassinosteroids to abscisic acid during seed germination in Arabidopsis. Plant Cell 26, 4394–4408. doi: 10.1105/tpc.114.130849
Jiang, J. L., Su, M., Wang, L. Y., Jiao, C. J., Sun, Z. X., Cheng, W., et al. (2012). Exogenous hydrogen peroxide reversibly inhibits root gravitropism and induces horizontal curvature of primary root during grass pea germination. Plant Physiol. Biochem. 53, 84–93. doi: 10.1016/j.plaphy.2012.01.017
Jiao, C. J., Jiang, J. L., Li, C., Ke, L. M., Cheng, W., Li, F. M., et al. (2011). Beta-ODAP accumulation could be related to low levels of superoxide anion and hydrogen peroxide in Lathyrus sativus L. Food Chem. Toxicol. 49, 556–562. doi: 10.1016/j.fct.2010.05.054
Jin, Z. P., Shen, J. J., Qiao, Z. J., Yang, G. D., Wang, R., and Pei, Y. X. (2011). Hydrogen sulfide improves drought resistance in Arabidopsis thaliana. Biochem. Biophys. Res. Commun. 414, 481–486. doi: 10.1016/j.bbrc.2011.09.090
Kocsy, G., Galiba, G., and Brunold, C. (2001). Role of glutathione in adaptation and signaling during chilling and cold acclimation in plants. Physiol. Plant 113, 158–164. doi: 10.1034/j.1399-3054.2001.1130202.x
Kronzucker, H. J., and Britto, D. T. (2011). Sodium transport in plants: acritical review. New Phytol. 189, 54–81. doi: 10.1111/j.1469-8137.2010.03540.x
Kumar, A., and Parsad, M. N. V. (2015). Lead-induced toxicity and interference in chlorophyll fluorescence in Talinum triangulare grown hydroponically. Photosynthetica 53, 66–71. doi: 10.1007/s11099-015-0091-8
Lai, D. W., Mao, Y., Zhou, H., Li, F., Wu, M. Z., Zhang, J., et al. (2014). Endogenous hydrogen sulfide enhances salt tolerance by coupling the reestablishment of redox homeostasis and preventing salt-induced K+ loss in seedlings of Medicago sativa. Plant Sci. 225, 117–129. doi: 10.1016/j.plantsci.2014.06.006
Li, Z. G., Min, X., and Zhou, Z. H. (2016). Hydrogen sulfide: a signal molecule in plant cross-adaptation. Front. Plant Sci. 7:1621. doi: 10.3389/fpls.2016.01621
Lichtenthaler, H. K., and Wellburn, A. R. (1983). Determination of total carotenoids and chlorophyll a and b of leaf extracts in different solvents. Biochem. Soc. Trans. 11, 591–592. doi: 10.1042/bst0110591
Lin, Y. T., Li, M. Y., Cui, W. T., Lu, W., and Shen, W. B. (2012). Haem oxygenase-1 is involved in hydrogen sulfide-induced cucumber adventitious root formation. J. Plant Growth Regul. 31, 519–528. doi: 10.1007/s00344-012-9262-z
Livak, K. J., and Schmittgen, T. D. (2001). Analysis of relative gene expression data using real-time quantitative PCR and the 2-ΔΔCT method. Methods 25, 402–408. doi: 10.1006/meth.2001.1262
Mancardi, D., Penna, C., Merlino, A., Del Soldato, P., Wink, D. A., and Pagliaro, P. (2009). Physiological and pharmacological features of the novel gasotransmitter: hydrogen sulfide. Biochim. Biophys. Acta 1787, 864–872. doi: 10.1016/j.bbabio.2009.03.005
Meyer, R., Burow, M., Bauer, M., and Papenbrock, J. (2003). Arabidopsis sulfurtransferases: investigation of their function during senescence and in cyanide detoxification. Planta 217, 1–10. doi: 10.1007/s00425-002-0964-5
Mostofa, M. G., Rahman, A., Ansary, M. M. U., Watanabe, A., Fujita, M., and Tran, L. P. (2015a). Hydrogen sulfide modulates cadmium-induced physiological and biochemical responses to alleviate cadmium toxicity in rice. Sci. Rep. 5:14078. doi: 10.1038/srep14078
Mostofa, M. G., Saegusa, D., Fujita, M., and Tran, L. S. (2015b). Hydrogen sulfide regulates salt tolerance in rice by maintaining Na+/K+ balance, mineral homeostasis and oxidative metabolism under excessive salt Stress. Front. Plant Sci. 6:1055. doi: 10.3389/fpls.2015.01055
Munns, R., and Tester, M. (2008). Mechanisms of salinity tolerance. Annu. Rev. Plant Biol. 59, 651–681. doi: 10.1146/annurev.arplant.59.032607.092911
Peng, R. Y., Bian, Z. Y., Zhou, L. N., Cheng, W., Hai, N., Yang, C. Q., et al. (2016). Hydrogen sulfide enhances nitric oxide-induced tolerance of hypoxia in maize (Zea mays L.). Plant Cell Rep. 35, 2325–2340. doi: 10.1007/s00299-016-2037-4
Riemenschneider, A., Nikiforova, V., Hoefgen, R., Kok, L. J. D., and Papenbrock, J. (2005). Impact of elevated H2S on metabolite levels, activity of enzymes and expression of genes involved in cysteine metabolism. Plant Physiol. Biochem. 43, 473–483. doi: 10.1016/j.plaphy.2005.04.001
Shi, H., Ishitani, M., Kim, C., and Zhu, J. K. (2000). The Arabidopsis thaliana salt tolerance gene SOS1 encodes a putative Na+/H+ antiporter. Proc. Natl. Acad. Sci. U.S.A. 97, 6896–6901. doi: 10.1073/pnas.120170197
Shi, Y., Zhang, Y., Yao, H. J., Wu, J. W., Sun, H., and Gong, H. J. (2014). Silicon improves seed germination and alleviates oxidative stress of bud seedlings in tomato under water deficit stress. Plant Physiol. Biochem. 78, 27–36. doi: 10.1016/j.plaphy.2014.02.009
Takahashi, R., Nishio, T., Ichizen, N., and Takano, T. (2007a). High-affinity K+ transporter PhaHAK5 is expressed only in salt-sensitive reed plants and shows Na+ permeability under NaCl stress. Plant Cell Rep. 26, 1673–1679. doi: 10.1007/s00299-007-0364-1
Takahashi, R., Nishio, T., Ichizen, N., and Takano, T. (2007b). Salt-tolerant reed plants contain lower Na+and higher K+ than salt-sensitive reed plants. Acta Physiol. Planta 29, 431–438. doi: 10.1007/s11738-007-0052-3
Velikova, V., Yordanov, I., and Edreva, A. (2000). Oxidative stress and some antioxidant systems in acid rain-treated bean plants: protective role of exogenous polyamines. Plant Sci. 151, 59–66. doi: 10.1016/s0168-9452(99)00197-1
Wang, S., Li, Z., Rui, R., Fan, G. S., and Lin, K. W. (2013). Cloning and characterization of a plasma membrane Na+/H+ antiporter gene from Cucumis sativus. Russ. J. Plant Phys. 60, 330–336. doi: 10.1134/S102144371303014X
Wang, Y. Q., Li, L., Cui, W. T., Xu, S., Shen, W. B., and Wang, R. (2012). Hydrogen sulfide enhances alfalfa (Medicago sativa) tolerance against salinity during seed germination by nitric oxide pathway. Plant Soil 351, 107–119. doi: 10.1007/s11104-011-0936-2
Yin, L. N., Wang, S. W., Tanaka, K., Fujihara, S., Itai, A., Den, X. P., et al. (2016). Silicon-mediated changes in polyamines participate in silicon-induced salt tolerance in Sorghum bicolor L. Plant Cell Environ. 39, 245–258. doi: 10.1111/pce.12521
Zhang, H., Hu, L. Y., Hu, K. D., He, Y. D., Wang, S. H., and Luo, J. P. (2008). Hydrogen sulfide promotes wheat seed germination and alleviates oxidative damage against copper stress. J. Integr. Plant Biol. 50, 1518–1529. doi: 10.1111/j.1744-7909.2008.00769.x
Zhang, H., Tang, J., Liu, X. P., Wang, Y., Yu, W., Peng, W. Y., et al. (2009). Hydrogen sulfide promotes root organogenesis in Ipomoea batatas, Salix matsudana and Glycine max. J. Integr. Plant Biol. 51, 1086–1094. doi: 10.1111/j.1744-7909.2009.00885.x
Zhang, L. P., Pei, Y. X., Wang, H. J., Jin, Z. P., Liu, Z. Q., Qiao, Z. J., et al. (2015). Hydrogen sulfide alleviates cadmium-induced cell death through restraining ROS accumulation in roots of Brassica rapa L. ssp. pekinensis. Oxid. Med. Cell Longev. 2015:804603 doi: 10.1155/2015/804603
Zhu, J. K. (2002). Salt and drought stress signal transduction in plants. Annu. Rev. Plant Biol. 53, 247–273. doi: 10.1146/annurev.arplant.53.091401.143329
Keywords: salt stress, hydrogen hulfide, Cucumis sativus L., lipid peroxidation, Na+/K+ balance, expression of SKOR gene
Citation: Jiang J-L, Tian Y, Li L, Yu M, Hou R-P and Ren X-M (2019) H2S Alleviates Salinity Stress in Cucumber by Maintaining the Na+/K+ Balance and Regulating H2S Metabolism and Oxidative Stress Response. Front. Plant Sci. 10:678. doi: 10.3389/fpls.2019.00678
Received: 05 January 2019; Accepted: 06 May 2019;
Published: 28 May 2019.
Edited by:
Vasileios Fotopoulos, Cyprus University of Technology, CyprusReviewed by:
Arafat Abdel Hamed Abdel Latef, South Valley University, EgyptBaris Uzilday, Ege University, Turkey
Copyright © 2019 Jiang, Tian, Li, Yu, Hou and Ren. This is an open-access article distributed under the terms of the Creative Commons Attribution License (CC BY). The use, distribution or reproduction in other forums is permitted, provided the original author(s) and the copyright owner(s) are credited and that the original publication in this journal is cited, in accordance with accepted academic practice. No use, distribution or reproduction is permitted which does not comply with these terms.
*Correspondence: Jing-Long Jiang, amlhbmdqaW5nbG9uZzUxMUAxNjMuY29t
†These authors have contributed equally to this work