- 1National Institute of Amazonian Research (INPA), Manaus, Brazil
- 2Climate and Ecosystem Sciences Division, Lawrence Berkeley National Laboratory, Berkeley, CA, United States
- 3Department of Integrative Biology, University of California, Berkeley, Berkeley, CA, United States
- 4Embrapa Amazônia Oriental, Belém, Brazil
- 5Environmental Sciences Division and Climate Change Science Institute, Oak Ridge National Laboratory, Oak Ridge, TN, United States
- 6Earth and Environmental Sciences Division, Los Alamos National Laboratory, Los Alamos, NM, United States
- 7Pacific Northwest National Laboratory, Richland, WA, United States
- 8Department of Geography, University of California, Berkeley, Berkeley, CA, United States
Current climate change scenarios indicate warmer temperatures and the potential for more extreme droughts in the tropics, such that a mechanistic understanding of the water cycle from individual trees to landscapes is needed to adequately predict future changes in forest structure and function. In this study, we contrasted physiological responses of tropical trees during a normal dry season with the extreme dry season due to the 2015–2016 El Niño-Southern Oscillation (ENSO) event. We quantified high resolution temporal dynamics of sap velocity (Vs), stomatal conductance (gs) and leaf water potential (ΨL) of multiple canopy trees, and their correlations with leaf temperature (Tleaf) and environmental conditions [direct solar radiation, air temperature (Tair) and vapor pressure deficit (VPD)]. The experiment leveraged canopy access towers to measure adjacent trees at the ZF2 and Tapajós tropical forest research (near the cities of Manaus and Santarém). The temporal difference between the peak of gs (late morning) and the peak of VPD (early afternoon) is one of the major regulators of sap velocity hysteresis patterns. Sap velocity displayed species-specific diurnal hysteresis patterns reflected by changes in Tleaf. In the morning, Tleaf and sap velocity displayed a sigmoidal relationship. In the afternoon, stomatal conductance declined as Tleaf approached a daily peak, allowing ΨL to begin recovery, while sap velocity declined with an exponential relationship with Tleaf. In Manaus, hysteresis indices of the variables Tleaf-Tair and ΨL-Tleaf were calculated for different species and a significant difference (p < 0.01, α = 0.05) was observed when the 2015 dry season (ENSO period) was compared with the 2017 dry season (“control scenario”). In some days during the 2015 ENSO event, Tleaf approached 40°C for all studied species and the differences between Tleaf and Tair reached as high at 8°C (average difference: 1.65 ± 1.07°C). Generally, Tleaf was higher than Tair during the middle morning to early afternoon, and lower than Tair during the early morning, late afternoon and night. Our results support the hypothesis that partial stomatal closure allows for a recovery in ΨL during the afternoon period giving an observed counterclockwise hysteresis pattern between ΨL and Tleaf.
Introduction
Evapotranspiration by terrestrial ecosystems delivers an estimated 62,000 km3 of water to the atmosphere every year, with the majority associated with plant transpiration (Jasechko et al., 2013). In the Amazon Basin, an estimated 25–50% of precipitation is recycled back to the atmosphere through forest transpiration (Eltahir and Bras, 1994; Chambers and Artaxo, 2017), with important implications for the interactions between the biosphere and atmosphere (Araújo et al., 2002; Negrón-Juárez et al., 2007). Under climate change scenarios, vegetation resilience will depend on the capacity to exploit water resources (Grossiord et al., 2017), and a mechanistic understanding of the water cycles from individual trees to landscape scales is necessary in order to predict changes in the forest structure (Chambers et al., 2014).
At the leaf level, transpiration flux is a function of vapor pressure deficit (VPD) between the leaf and the air and stomatal conductance (gs), according to Fick’s law of diffusion (Costa et al., 2010). Although numerous environmental factors influence gs, net radiation, VPD and soil moisture are often considered the most important (Jarvis, 1976; Jones, 1998; Lloyd and Farquhar, 2008; Daloso et al., 2017). High leaf temperatures (Tleaf) and VPD are known to induce stomatal closure in order to minimize excessive water loss (Farquhar, 1978; Meinzer et al., 1993; Tinoco-Ojanguren and Pearcy, 1993; Oren et al., 1999b; McAdam et al., 2016; Brodribb et al., 2017). The degree of stomatal closure is a balancing act between preventing hydraulic damage while still allowing enough CO2 influx for carbon fixation to avoid carbon-starvation (Adams et al., 2017). In addition, stomatal closure limits water lost through transpiration, and thereby indirectly regulates leaf temperatures. Given that the tropics have among the narrowest seasonal temperature range of any biome globally, they may be particularly sensitive to even small increases in temperature associated with climate change (Field et al., 2014). Indeed, rising temperature and VPD are environmental factors clearly associated with increased tree mortality in the tropics (McDowell et al., 2018), with more pronounced impacts during extreme drought events in the Amazon forest, such as the El Niño-Southern Oscillation (ENSO). This reinforces the importance of having more studies that investigate the effect of these variables (temperature and VPD) in the tropics, especially focusing on comparisons between two distinct periods, such as normal years (control scenario) and years with ENSO. This kind of approach can be considered as a “natural experiment” and allow to expand our understanding of the coupling of tree water use (and concurrent carbon uptake) and the environmental factors that affect stomatal conductance – solar radiation, CO2, air temperature, leaf temperature and humidity.
The water potential gradient that regulates water movement through trees is anchored by soil moisture availability on one end, and atmospheric moisture availability VPD on the other. VPD is indirectly estimated from measurements of relative humidity (RH) and air temperature (Tair) using micrometeorological sensors (Ewers and Oren, 2000). However, as Tleaf and Tair can differ by several degrees, the use of Tleaf instead of Tair to calculate VPD (ΔVPD) results in a more accurate representation of the true water vapor pressure gradient between the substomatal cavity and the boundary layer of the air near the leaf surface (Ewers and Oren, 2000). Therefore, Tleaf measurements are vital for better interpretation of plant hydraulic responses to environmental drivers in order to develop more accurate earth system models (ESMs) (Michaletz et al., 2016). However, sap velocity, Tleaf and environmental drivers are rarely measured together, especially in the tropics where the canopy layers are often hard to access (Chave et al., 2005; Segura and Kanninen, 2005). Thus, the response of a plant’s transpiration to changes in environmental and physiological conditions remains highly uncertain in ESMs (Jasechko et al., 2013).
In relation to environmental drivers, clockwise hysteresis patterns between sap flow and VPD have been reported with higher sap flow rates during the morning period relative to the afternoon (O’Brien et al., 2004; Zeppel et al., 2004; Zhang et al., 2014). In addition, a counterclockwise hysteresis pattern has been observed in tropical and temperate forests when sap flow is plotted as function of irradiance (O’Brien et al., 2004; Zeppel et al., 2004; Bretfeld et al., 2018; Brum et al., 2018). In the case of transpiration, it has been established that the hysteresis phenomena are influenced by the temporal lag between solar radiation, which tends to peak in the late morning to mid-day, and VPD which tends to peak in the early afternoon (O’Brien et al., 2004; Zeppel et al., 2004; Zhang et al., 2014; Novick et al., 2016). Also, hysteresis between sap flux and environmental drivers are influenced by the stored stem water and the time lag between basal sap velocity and upper canopy transpiration, as an effect of hydraulic capacitance and resistance (Phillips et al., 1997; Ward et al., 2012). However, coupled field observations of physiological and environmental variables that include not only diurnal sap velocity and environmental driving data, but also concurrent leaf level data such as gs, Tleaf and ΨL has been very limited in the tropics. Yet such data, are needed to verify the relationships between Vs and environmental/physiological drivers in tropical forests.
In this study, we present in situ field observations of environmental (direct solar radiation, Tair and VPD) and physiological (Vs, gs, and ΨL) variables and their correlations with Tleaf during the 2015–2016 ENSO. In order to observe the interactions between physiological variables and fast changing environmental conditions, we collected high temporal frequency data (15–60 min) in two primary rainforest sites located in the Eastern (Santarém) and in the Central (Manaus) Amazon. Since the 2015–2016 ENSO event was the warmest period in the Amazon forest over the past 13 years (Fontes et al., 2018), we expected peak Tleaf to increase and subsequently hysteretic behavior of water use vs. Tleaf to become more pronounced. In this study, we explored the mechanisms that regulate tree transpiration and the diurnal hysteresis patterns between physiological and environmental variables to contrast different tree species responses to the extreme 2015 dry season (ENSO) and a normal 2017 dry season (“control scenario”).
Materials and Methods
Study Area
The field activities occurred in two sites near the cities of Manaus and Santarém, Brazil (Supplementary Figure S1). Near the city of Manaus, trees with leaves accessible from the K-34 walkup tower were selected for study. The 50-meter tall K-34 tower is located at the Reserva Biológica do Cuieiras, also known as ZF-2, and contains roughly 22,000 ha adjacent to extensive areas of undisturbed tropical forest (Araújo et al., 2002). The mean value of rainfall is ∼2,500 mm year-1 with the driest months of the year concentrated from July to September (Araújo et al., 2002). Field data were collected at the K-34 tower site between July 01, 2015 and December 01, 2017.
Near the city of Santarém, four trees near the K-67 triangle tower were selected for study, located in the Tapajós National Forest with approximately 527,000 ha near the Santarém-Cuiabá highway (BR-163). The K-67 tower is located ∼6 km west of the BR-163 and ∼6 km east of Tapajós river, in an area of largely contiguous forest from north to south (Hutyra et al., 2007). The site receives ∼2,000 mm year-1 of rainfall and has a five-month dry season from mid-July to mid-December (Saleska et al., 2003; Wu et al., 2017). In Santarém, field data were collected during the period of April 01, 2016 to December 31, 2016.
Species Selection
Different species were selected in a plateau area of Tapajós National Forest (Santarém) and Reserva Biológica do Cueiras (ZF-2 – Manaus) (eight species in total; Table 1). Tree selection criteria were based on the proximity of the crowns to the two canopy access towers (K-34 and K-67). This approach enabled measurement of physiological variables including sap velocity at breast height, Tleaf, gs, and ΨL from leaves at the top of the crowns, together with environmental variables including direct solar radiation, Tair, and RH above the canopy (Supplementary Figure S2).
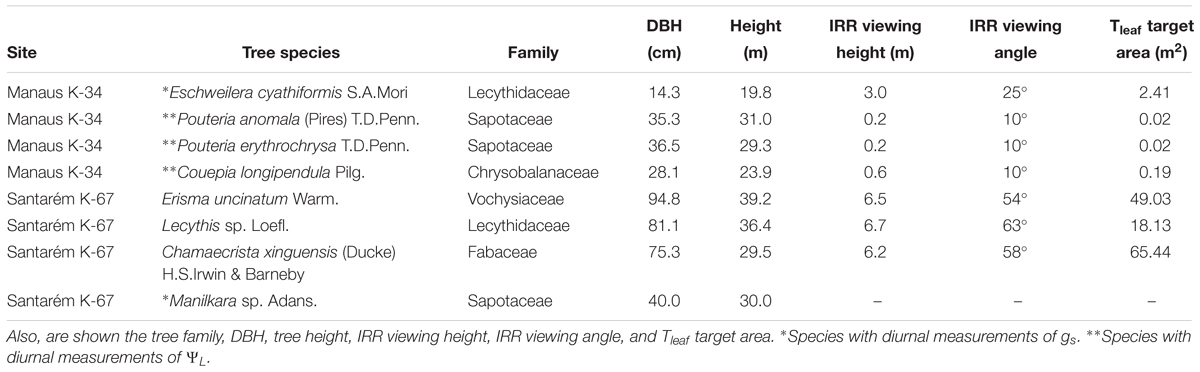
Table 1. List of tree species instrumented with sap velocity and Tleaf sensors in Manaus and Santarém.
Sap Velocity (Vs) Measurements
One heat pulse sap velocity sensor (SFM1, ICT international®) was installed per tree near breast height (DBH) following the protocols previously described by Christianson et al. (2017). The SFM1 sensor consists of a heater and two temperature-sensing probes to determine sap velocity (cm h-1) at 0.75 cm depth in the stem using the heat ratio method (Burgess et al., 2001; Green et al., 2003; Steppe et al., 2010). The heater needle was configured to emit a 20 Joule pulse of thermal energy every 15 min (sap heat ratio measurements for 5 min 32 s following the pulse). Biophysical characteristics (diameter and bark thickness) for each tree were used as input into the Sap Flow Tool version 1.4.1 (Phyto-IT®) to calculate sap velocity from raw data downloaded from the SFM1 sensors in the field.
The heat pulse method can be used for accurate measurements of sap flow (Lambers et al., 2008), but this method is unable to measure low rates due to its inability to distinguish heat-pulse velocities below a threshold velocity of 3–4 cm h-1 (Green et al., 2003). The probe spacing is also an important parameter and the sap velocity (Vs) is dependent upon the exact distance between needles as the following equation shows:
where: k is the thermal diffusivity of wet wood; x is the distance between the heat source (heater) and temperature sensors; v1 and v2 are the increases in temperature (from ambient) at equidistant points downstream and upstream from the heater.
In this study, we used the factory default setting of 5 mm of needle spacings, as recommended by the manufacturer (Burgess and Downey, 2014), using a metal drill guide to ensure equidistant sensor placing. This 5 mm spacing is suitable to a theoretical maximum of 54 cm h-1 (Burgess and Downey, 2014).
It should be noted that following the protocols of 5 mm probe spacing of the SFM1 sensors, it is possible that the instrumental maximum was reached at relatively modest flows (16 cm h-1 for Pouteria anomala for example) on some days. Other available methods to estimate sap flow like the thermal dissipation method and the heat field deformation also underestimate Vs, where the error tends to increase with further increases in Vs (Steppe et al., 2010). However, evidence that the maximum observed Vs was not due to a sensor saturation includes: (1) different maximum values of Vs between species (plateau in the scatter plots); (2) the state theoretical maximum of 54 cm h-1 of the ICT user manual; and (3) on the same trees (Grossiord et al., under review) found no statistical difference between sap velocities determined by ICT and Granier sensors.
Tleaf, Tair, VPD and Direct Solar Radiation Measurements
To measure Tleaf, a single infrared radiometer sensor (IRR SI-111 analogic for the species P. anomala, Pouteria erythrochrysa, and Couepia longipendula or IRR SI-131 digital for the species Eschweilera cyathiformis, Erisma uncinatum, Lecythis sp. and Chamaecrista xinguensis, Apogee®) was positioned from the tower’s structure with the field of view targeting the top of individual tree crowns (one IRR sensor per tree). Five-min averages of Tleaf were recorded using a CR-3000 (Campbell Scientific® for the SI-111 sensors) and EM-50 (Decagon® for the SI-131 sensors) dataloggers. The sensors were positioned with the viewing heights and viewing angles listed in Table 1. The field of view of each sensor (Tleaf target area) was calculated using the IRR calculator available in the website1. To validate the infrared radiometer sensors installed on the two sites, Tleaf measurements were made using Teflon insulated type T thermocouples (OM-CP-OCTTEMP-A Nomad®, Omega Engineering) directly attached to the abaxial side of the leaf using a breathable white tape and configured to register measurements every 15 s (Supplementary Figure S3). In addition, in Manaus direct solar radiation (W m-2) with 5-min averages were collected at 35.0 m above the canopy using a SPN1-Sunshine Pyranometer (Delta-T Devices®). Tair and RH data were obtained using a thermohygrometer (HC2S3, Campbell Scientific®) installed above the canopy at 51.1 m height on the K-34 tower structure.
In this study, a more accurate physiological approach to estimate VPD was applied. The Tetens equation was used to calculate the saturation vapor pressure of the air (eo) using the variables air temperature (Tair) and relative humidity of the air (RHo) (Eq. 2). To estimate the saturation vapor pressure inside the substomatal chamber (ei) the Tetens equation was also used replacing Tair by Tleaf (Eq. 3). The relative humidity inside the substomatal cavity (RHi) was assumed to be equal to 1 as demonstrated by many studies (Ward and Bunce, 1986; Buckley et al., 2017; Cernusak et al., 2018). With these variables it was possible to estimate the VPD difference between the substomatal chamber (ei × RHi) and the atmosphere (eo × RHo) (Eq. 4).
where: ΔVPD is the leaf-to-air water VPD (kPa); eo is the air saturated water vapor pressure (kPa); ei is the saturated water vapor pressure inside the substomatal chamber (kPa); RHi is the relative humidity inside the substomatal cavity which is assumed to be equal to 1. RHo is the relative humidity of the air near the leaf surface (expressed as a decimal); Tleaf is the leaf temperature in °C and Tair is the air temperature in °C.
Stomatal Conductance (gs) Measurements
Diurnal observations of gs were made on upper canopy leaves accessible from the walkup towers (K-34 in Manaus and a walkup tower 1 km from the K-67 triangle tower in Santarém). In Manaus, diurnal patterns of gs were measured from individual leaves at the top the main crown near the towers from 6:00 to 18:00 using a Li-Cor 6400 XT portable photosynthesis system (Li-Cor, Lincoln®, NE, United States). gs measurements on individual leaves were made for 10 min using Li-Cor 6400 XT. The CO2 reference concentration was held constant at 400 μmol mol-1. Tblock and photosynthetically active radiation values were set every 15 min to match environmental conditions. Using the Li-Cor 6400 XT we set the Tblock to achieve a target Tleaf, based on the infrared radiometers measurements recorded in the CR-3000 datalogger which have a screen that makes possible real time data reads without a computer. In Santarém, gs measurements on individual leaves were made every 2 min using the SD-1 leaf porometer system (Decagon Devices®, WA, United States) throughout the day.
Leaf Water Potential (ΨL) Measurements
In Manaus ΨL data were collected from three trees together with Tleaf measurements (Table 1) to access potential diurnal hysteresis patterns similar to those observed with sap velocity, gs, Tair, Tleaf, and ΔVPD. Hourly ΨL measurements (6:00 to 18:00 – 12 h) of healthy leaves without noticeable condensation on the surface of P. anomala, P. erythrochysa, and C. longipendula were performed in Manaus using a pressure chamber instrument (Model 1000, PMS Instrument Company®) connected to a high-pressure nitrogen cylinder. Small branches from the upper tree crowns were removed and a single leaf per tree was used to measure ΨL. The canopy position of each tree was also classified following the crown illumination index proposed by Synnott (1979) (Supplementary Table S1). In this study, the leaf water potential measurements were performed in a single day of September during both 2015 and 2017 dry season.
Data Analysis
Data were analyzed using IGOR Pro® version 6.3 (WaveMetrics, Inc., United States) and R v. 3.0.2 (R Development Core Team, 2013) software packages. In Manaus, 4-month time series (August to November) were plotted to observe the correlations between Tleaf and Tair during the 2015 dry season (ENSO). Additionally, the two-dimensional kernel density function (kde2d) was used to observe potential offsets between Tleaf and Tair during the 2015 dry season. In Manaus, the 2015 dry season (ENSO period) was compared with the 2017 dry season (“control scenario”) using hysteresis indexes (Hindex) of the normalized values of Tleaf, Tair, and ΨL for the species P. anomala, C. longipendula, and P. erythrochrysa. For the normalization of each variable the min-max feature scaling method was used to standardize the range of the raw data. Hysteresis indices were calculated using the shoelace formula (Eq. 5; Braden, 1986), and a paired t-test was performed (α = 0.05) to intercompare the Hindex of the species between the 2015 and 2017 dry seasons. The Hindex is a measure of the size of the hysteresis loop and enables quantitative comparisons of hysteresis behaviors during, for example, two contrasting periods like El Niño and regular season.
where: A is the area of the polygon, n is the number of sides of the polygon, and (xi, yi), i = 1, 2,…, n are the vertices (or “corners”) of the polygon.
Additionally, normalized sap velocity and Tleaf hysteresis parameters of the species E. cyathiformis, P. anomala, and P. erythrochysa were compared separating the morning and afternoon/night periods during both 2015 and 2017 dry seasons (ENSO and regular season). In the morning period sigmoidal curves were fitted using 15 min interval data of the variables Vs and Tleaf. The statistical parameters of the sigmoidal curves were used to compare the ENSO and the regular season between species. The same approach was done to compare the afternoon/night period of the variables Vs and Tleaf but using power curves instead of sigmoidal functions.
Results
Vs, Tleaf, and ΔVPD
Representative four-day time series of Vs as function of Tleaf and ΔVPD for E. cyathiformis in Manaus and Vs as function of Tleaf for Lecythis sp. in Santarém are presented in Figure 1. Despite expectations of a significant delay due to the large vertical distance between the observations of Vs and Tleaf, the two variables tightly track each other, during the day and night (Figures 1A,E). Additionally, normalized time series of Vs and Tleaf of six trees during a two-month period also show this tightly temporal track (Supplementary Figure S4). Moreover, temporal similarities were also graphically observed for the variables Vs and ΔVPD (Figure 1B).
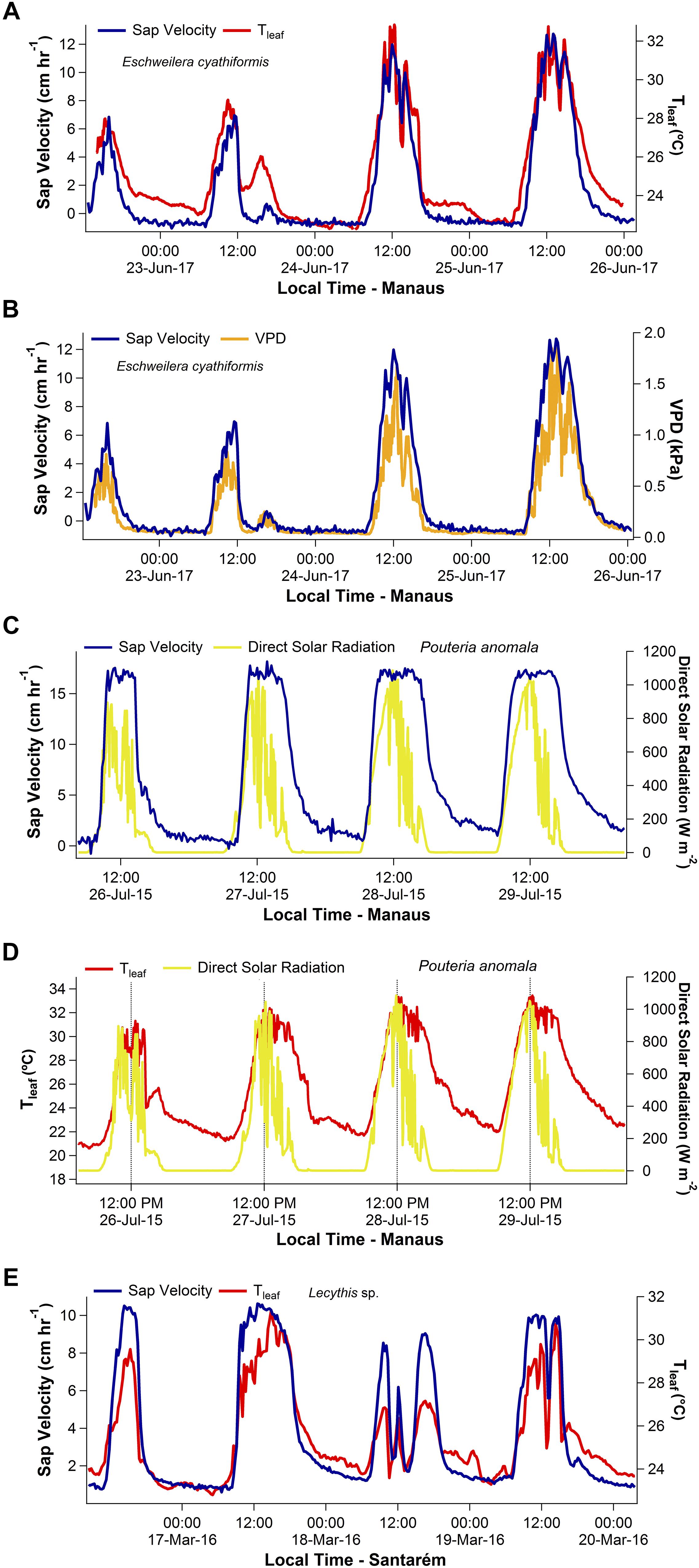
Figure 1. Four-day time series showing the daily patterns of sap velocity (Vs), leaf temperature (Tleaf), and vapor pressure deficit (ΔVPD) for the species Eschweilera cyathiformis, Pouteria anomala, and Lecythis sp. Example of temporal similarities between Vs and Tleaf are shown in (A,E). Temporal similarities were also observed for Vs and ΔVPD (B). A temporal decoupling during the afternoon period was observed between Vs and direct solar radiation (C). The contrasting patterns of direct solar radiation and Tleaf are also shown in (D).
Diurnal patterns of direct solar radiation differed from those of Tleaf in Manaus, especially during the afternoon period (Figure 1D). Direct solar radiation peaked about mid-day, then declined during the afternoon. In contrast, Tleaf and ΔVPD patterns peaked later, during the early afternoon and maintained high values until late afternoon even as solar radiation declined (Figures 1A–E). Thus, for Manaus, on average, a lag of 2 h and 22 min delay occurred between the peaks of direct solar radiation and Tleaf.
Tleaf and Tair Relations
The highest Tleaf values during the ENSO in the year of 2015 (2015 dry season – August to November) were observed during the months of September and October (Figure 2). On some days during the 2015 dry season, the differences between Tleaf and Tair were close to 8°C for some species (average difference between Tleaf and Tair for all species: 1.65 ± 1.07°C). For the species C. longipendula (Figure 2A) the maximum observed Tleaf value was 40.78°C (September 12, 2015 – 11:30 local time) and the difference between Tleaf and Tair was on average 1.70 ± 1.20°C (maximum observed difference 7.43°C); for the species P. anomala (Figure 2B) the maximum observed Tleaf value was 40.09°C (September 22, 2015 – 15:30 local time) and the difference between Tleaf and Tair was in average 1.49 ± 0.92°C (maximum observed difference 7.26°C); for the species P. erythrochrysa (Figure 2C) the maximum observed Tleaf value was 39.67°C (October 4, 2015 – 13:30 local time) and the difference between Tleaf and Tair was in average 1.75 ± 1.06°C (maximum observed difference 6.39°C). At the end of the 2015 dry season (November), there were some days when Tair reached higher values compared to Tleaf for the species C. longipendula and P. erythrochrysa (Figures 2D,F). The 1:1 baseline presented in Figures 2A–C provides a reference to examine deviations of Tleaf from Tair, where the densest observations are between 23 and 26°C with the majority of Tleaf values lower than Tair. This pattern is observed during the night period, when the lowest Tair and Tleaf values are recorded. In addition, hysteresis patterns between Tleaf and Tair were observed for the species P. anomala, C. longipendula, and P. erythrochrysa in Manaus during the 2015 and 2017 dry season (Figure 3). The area of the hysteresis loops (Hindex) of P. anomala, C. longipendula and P. erythrochrysa during the 2015 dry season (ENSO) were statically larger (p < 0.01, α = 0.05, paired t-test) than the 2017 dry season (“control scenario”; Figure 3). The Hindex of the normalized variables Tleaf and Tair of the species P. anomala, C. longipendula and P. erythrochrysa during the 2015 dry season was, respectively: 0.0633; 0.0747; 0.0904. Moreover, the Hindex calculated for P. anomala, C. longipendula, and P. erythrochrysa for the 2017 dry season was, respectively: 0.0355; 0.0406; 0.0523.
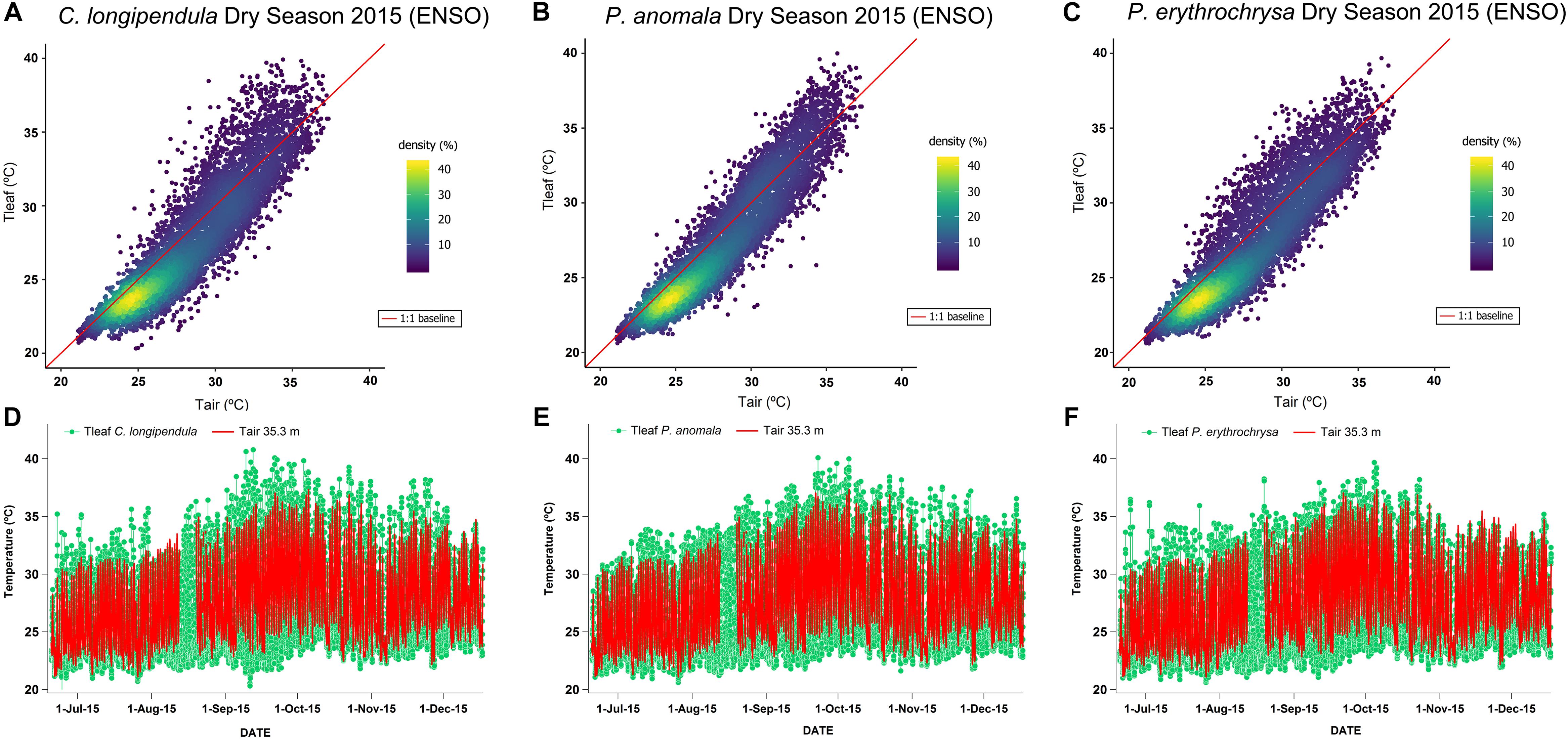
Figure 2. Relationship between Tleaf and Tair for the species C. longipendula, P. anomala, and P. erythrochrysa during the 2015 ENSO dry season (August to November) near K-34 tower (Manaus). The scatter plot using the kernel density method allowed to visualize that the observations between Tleaf and Tair overlaps at maximum rates around 25°C of temperature (40% of density), with the majority of Tleaf values lower than Tair (A–C). During the months of September and October of 2015, Tleaf reached, in some days, more than 40°C for all studied species (D–F). In general, during the 2015 ENSO, maximum values of Tleaf for all species were higher than maximum values of Tair. However, during some days at the end of the 2015 dry season, Tair was higher than Tleaf for the species C. longipendula and P. erythrochrysa (D,F).
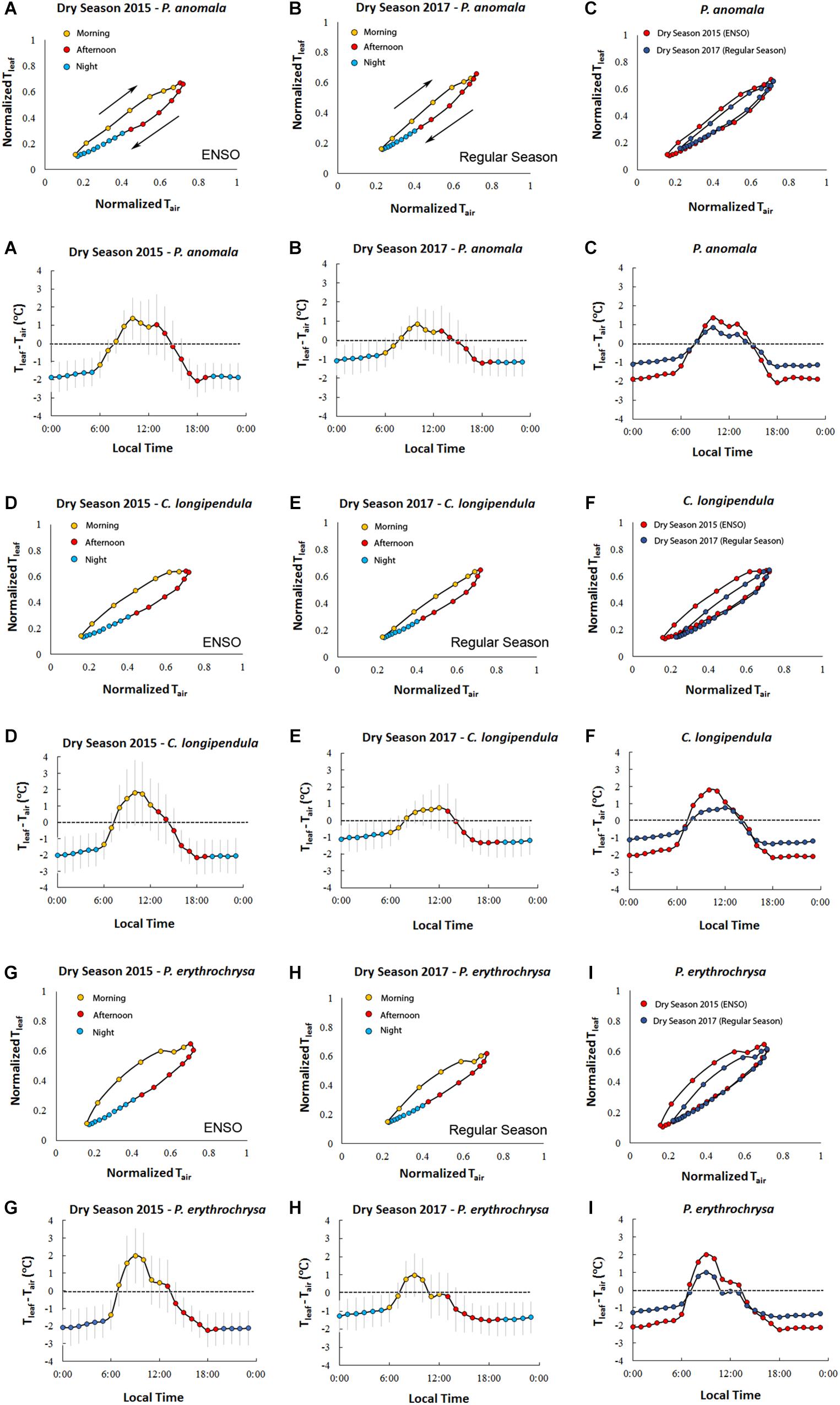
Figure 3. Hourly averages of Tleaf and Tair for the species C. longipendula, P. anomala, and P. erythrochrysa in Manaus site during the 2015 dry season (ENSO) and 2017 dry season (“control scenario”). The clockwise hysteresis pattern between Tleaf and Tair was observed for all the studied trees in Manaus. The orange dots represent the morning period (6:00–12:00), the red dots represent the afternoon period (13:00–19:00), and the blue dots represent the night period (20:00–5:00) (A,B,D,E,G,H). A significant difference (p < 0.01) of Tleaf and Tair hysteresis loops (Hindex) was observed when the 2015 dry season (ENSO period) was compared with the 2017 dry season (“control scenario”) (C,F,I). On average, in both 2015 and 2017 dry season, Tleaf was higher than Tair during the middle morning to early afternoon, and Tair was higher than Tleaf in the middle afternoon, night and early morning. The exception was for the species P. erythrochrysa where, on average, during the 2017 dry season Tleaf was higher than Tair only during the morning period (08:00–10:00) (H).
Using hourly averages for all the analyzed species it was possible to observe that generally Tleaf was higher than Tair from the middle of the morning period until the early afternoon in both the 2015 and 2017 dry seasons (Figure 3). In contrast, Tair was predominantly higher than Tleaf in the early morning, middle afternoon, and throughout the night in both the 2015 and 2017 dry seasons.
Vs-Tleaf, Vs-ΔVPD, and Vs-Direct Solar Radiation Diurnal Hysteresis
As an example, 1 week of Vs data were plotted as function of Tleaf, ΔVPD and direct solar radiation (Figures 4, 5). The clockwise hysteresis in Vs-Tleaf and Vs-ΔVPD was evident with morning periods showing higher temperature sensitivities than afternoon and night periods and in this study is referred to as the “gs effect” (Figures 4, 5). In Manaus, the scatter plot of Vs-direct solar radiation revealed a counterclockwise hysteresis pattern, on the same day as the Vs-ΔVPD clockwise hysteresis (Figure 5). For the same direct solar radiation values, higher Vs values in the afternoon were observed relative to the morning period, and in this study this pattern is referred to as the “VPD effect.”
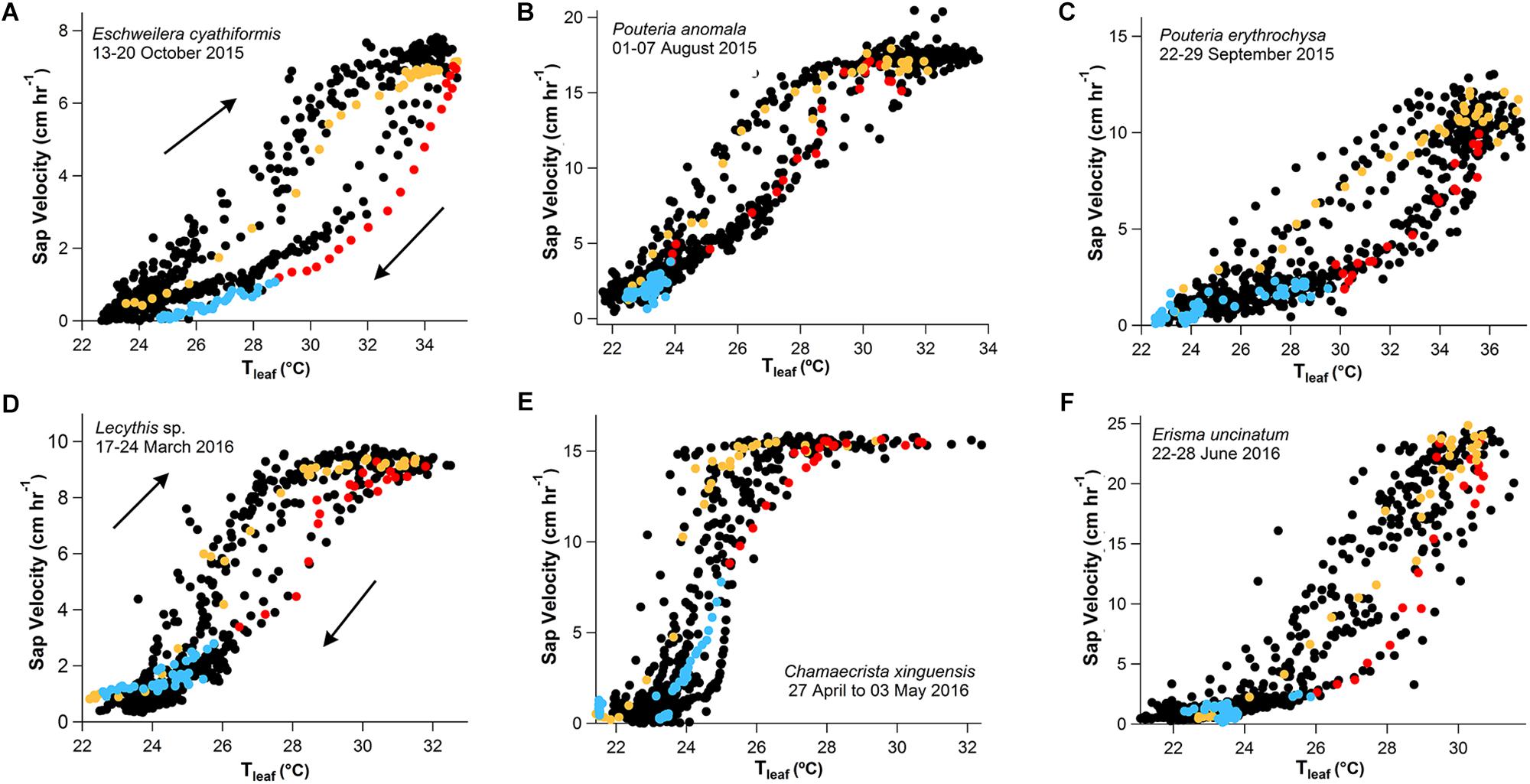
Figure 4. One-week scatter plot data with 15-min observation intervals (black dots) showing the clockwise hysteresis of Vs and Tleaf for three trees of different species in Manaus (A–C), and Santarém (D–F). The hysteresis phenomenon is represented by a single day of data randomly selected, separated by morning (orange values, 6:00–14:00), afternoon (red values, 14:15–19:00), and nighttime (blue values, 19:15–5:45) periods.
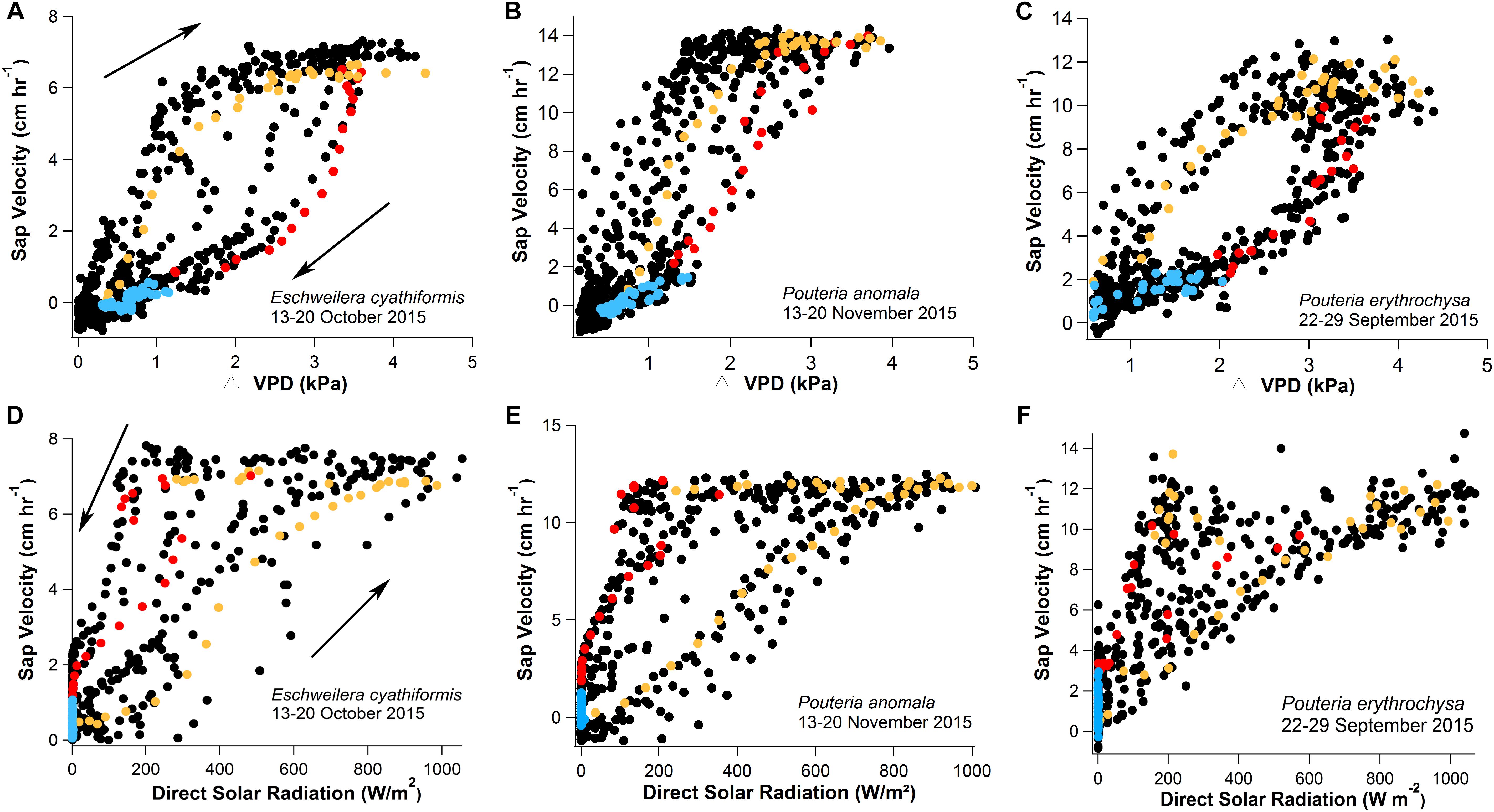
Figure 5. One-week data with 15-min observation intervals showing clockwise hysteresis of Vs and ΔVPD (A–C) and counterclockwise hysteresis of Vs and direct solar radiation (D–F) for the species E. cyathiformis, P. anomala, and P. erythrochrysa. The clockwise and counterclockwise hysteresis phenomena were observed for each tree species using the data of the exact same week (black dots) and day (color dots) separated by morning (orange values, 6:00–14:00), afternoon (red values, 14:15–19:00), and nighttime periods (blue values, 19:15–5:45). During the morning period the Vs and ΔVPD clockwise hysteresis pattern showed higher temperature sensitivities compared to afternoon/night periods. In this study, this pattern was called the “gs effect.” During the afternoon period the counterclockwise hysteresis relationship between Vs and direct solar radiation showed higher temperature sensitivities compared to morning/night periods and it was called the “VPD effect.” Both observed effects are a result of the Fick’s law of diffusion (transpiration = gs × VPD). For this interpretation, direct solar radiation was considered to have similar temporal patterns with gs by circadian cycles.
Vs showed a sigmoid dependence on Tleaf and ΔVPD including a rapid increase, an inflection point, and a plateau. When Vs reached the maximum values for each species, it was insensitive to further increases in Tleaf and ΔVPD (Figure 6 and Supplementary Figure S5). The sigmoid pattern was observed in all studied trees (Supplementary Figure S5), although the maximum Vs differed between species from 8 to 24 cm h-1; detailed daily patterns of Vs-Tleaf revealed a sigmoid increase in Vs during the morning period followed by an exponential decrease in the afternoon and throughout the night (Figure 6). During the morning period the curve’s maximum values (max) of the sigmoid function for the variables Tleaf and Vs were lower when the 2015 dry season (ENSO) was compared to the 2017 dry season (regular) for the species E. cyathiformis and P. erythrochrysa (Figure 7) (curve’s maximum values (max): E. cyathiformis ENSO (2015): 0.6379 ± 0.017; E. cyathiformis regular dry season (2017): 0.9376 ± 0.028; P. erythrochrysa ENSO (2015): 0.6992 ± 0.029; P. erythrochrysa regular dry season (2017): 0.8837 ± 0.029). For the species P. anomala the max was statistically equal in both periods (curve’s maximum values (max): P. anomala ENSO (2015): 0.7386 ± 0.082; P. anomala regular dry season (2017): 0.6960 ± 0.024). The inflection point (xhalf) of the sigmoidal curves also revealed different patterns between species. The xhalf values were lower for the species E. cyathiformis and P. anomala during the 2015 ENSO in comparison to the 2017 regular dry season (inflection point (xhalf): E. cyathiformis ENSO (2015): 0.3433 ± 0.006; E. cyathiformis regular dry season (2017): 0.5401 ± 0.006; P. anomala ENSO (2015): 0.2648 ± 0.024; P. anomala regular dry season (2017): 0.3423 ± 0.008. In contrast the xhalf value for the species P. erythrochrysa was higher during the ENSO in comparison to the 2017 regular dry season (inflection point (xhalf): P. erythrochrysa ENSO (2015): 0.4253 ± 0.012; P. erythrochrysa regular dry season (2017): 0.3064 ± 0.007). The statistical values of the power function during the afternoon/night period was relatively similar in both ENSO and regular dry season for the species E. cyathiformis and P. anomala (Figure 7). The exception was the species P. erythrochrysa which the exponent parameter (pow) were higher during ENSO compared to the 2017 regular dry season.
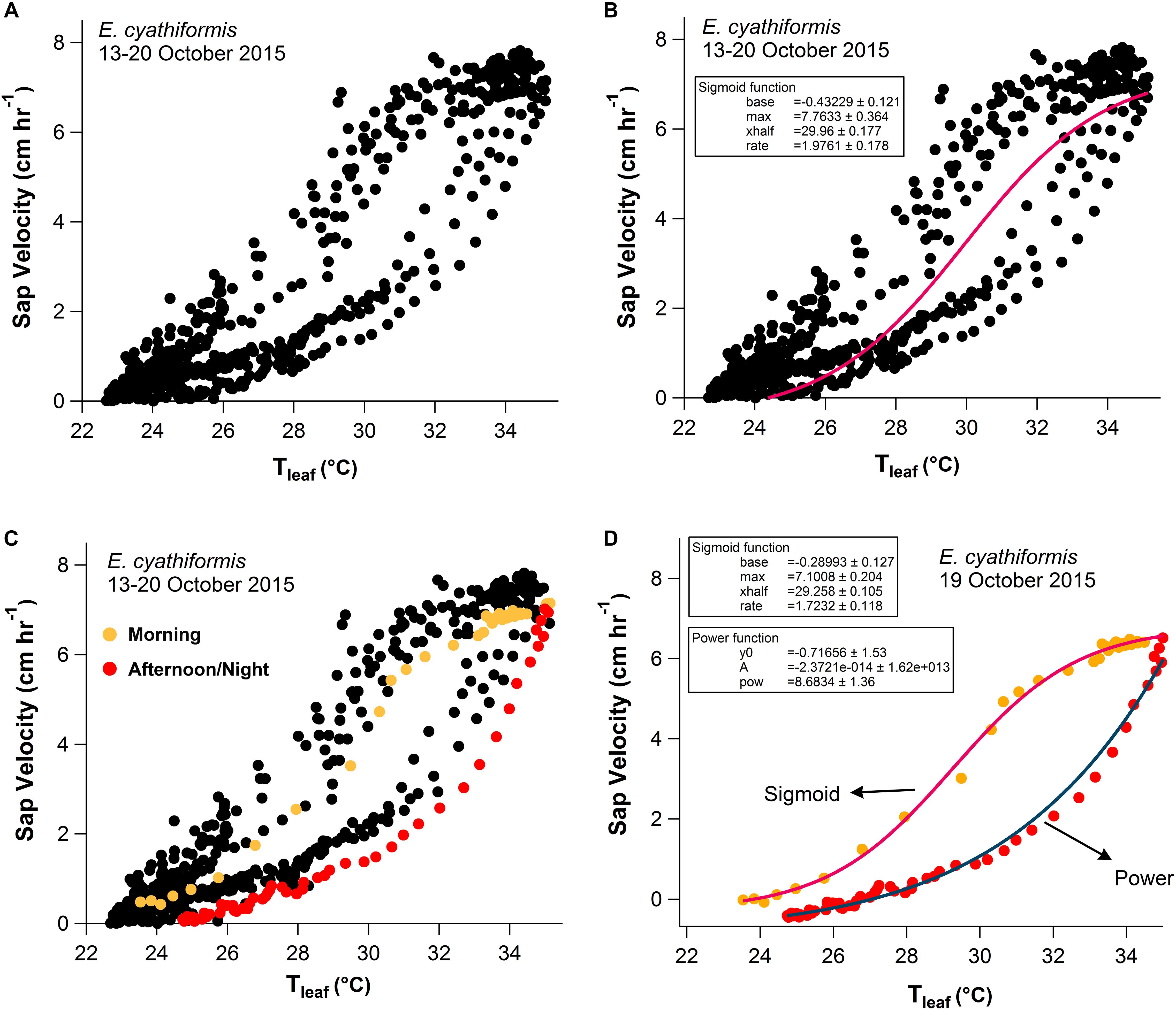
Figure 6. One-week scatter plot data (13–20 October 2015) with 15-min observation intervals for E. cyathiformis (A). The sigmoid function presented the best fit for one-week data of sap velocity (Vs) and Tleaf (A,B). A single day data randomly selected, separated by two periods, presented different patterns (C,D). During the morning period (orange dots 6:00–14:00) the sigmoid function presented the best fit y = base + and during the afternoon/night period (red dots 14:15–5:45) the power function presented the best fit y = y0 + Axpow (D).
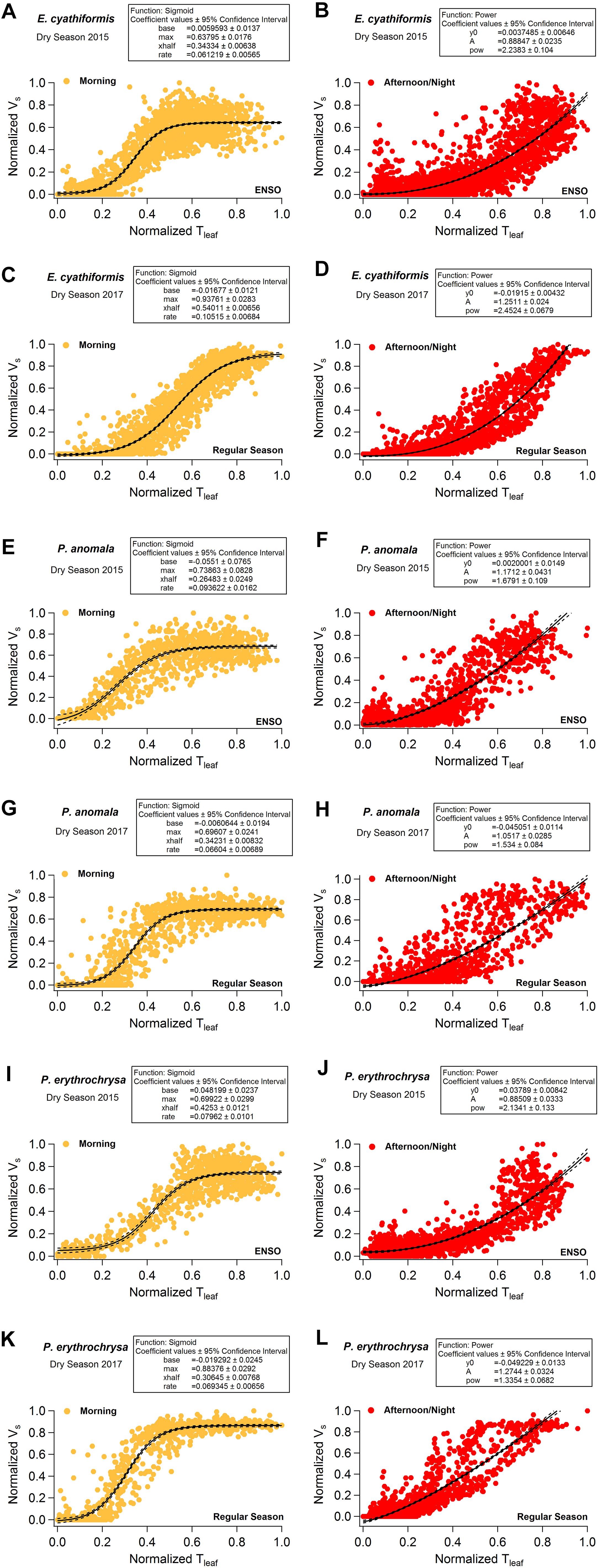
Figure 7. Intercomparison of the hysteretic behavior of the normalized variables Vs and Tleaf for the species E. cyathiformis, P. anomala, and P. erythrochrysa during 2015 (ENSO) and 2017 (regular) dry seasons. During the morning period the curve’s maximum values (max) of the sigmoid function for variables Vs and Tleaf were lower during the 2015 dry season (ENSO) in comparison to the 2017 dry season (Regular) for the species E. cyathiformis and P. erythrochrysa and statically equal for the species P. anomala (A,C,E,G,I,K). The inflection point (xhalf) of the sigmoidal curves also revealed different patterns for the species when the 2015 dry season was compared to the 2017 dry season. The xhalf values were lower for the species E. cyathiformis and P. anomala during the 2015 ENSO in comparison to the 2017 regular dry season (A,C,E,G). In contrast the xhalf value for the species P. erythrochrysa was higher during the ENSO in comparison to the 2017 regular dry season (I,K). The statistical values of the power function during the afternoon/night period were relative similar in both ENSO and regular dry season for the species E. cyathiformis and P. anomala (B,D,F,H). The exception was the species P. erythrochrysa which the exponent parameter (pow) where higher during ENSO compared to the 2017 regular dry season (J,L).
gs-Tleaf and ΨL-Tleaf Diurnal Hysteresis
Clockwise hysteresis patterns were observed for gs-Tleaf in Manaus and Santarém (Supplementary Figure S6). For the same Tleaf, the observed gs values of E. cyathiformis and Manilkara sp. were greater during the morning period than the afternoon. The maximum observed gs values occurred at a Tleaf of 33.3°C in Manaus and 32.6°C in Santarém (Supplementary Figure S6).
A counterclockwise hysteresis pattern was observed between ΨL and Tleaf (Figure 8). At the same Tleaf values, ΨL were more negative in the morning compared to the afternoon period. During September 2015 (peak of the El Niño) the Hindex of the variables ΨL and Tleaf were significantly higher compared to September 2017 (regular dry season) (p < 0.01, α = 0.05). The Hindex calculated for the species P. anomala, C. longipendula, and P. erythrochrysa during the 2015 dry season was, respectively: 0.2379; 0.3116; 0.1605. Moreover, the Hindex calculated for P. anomala, C. longipendula, and P. erythrochrysa for the 2017 dry season was, respectively: 0.0642; 0.1596; 0.0324.
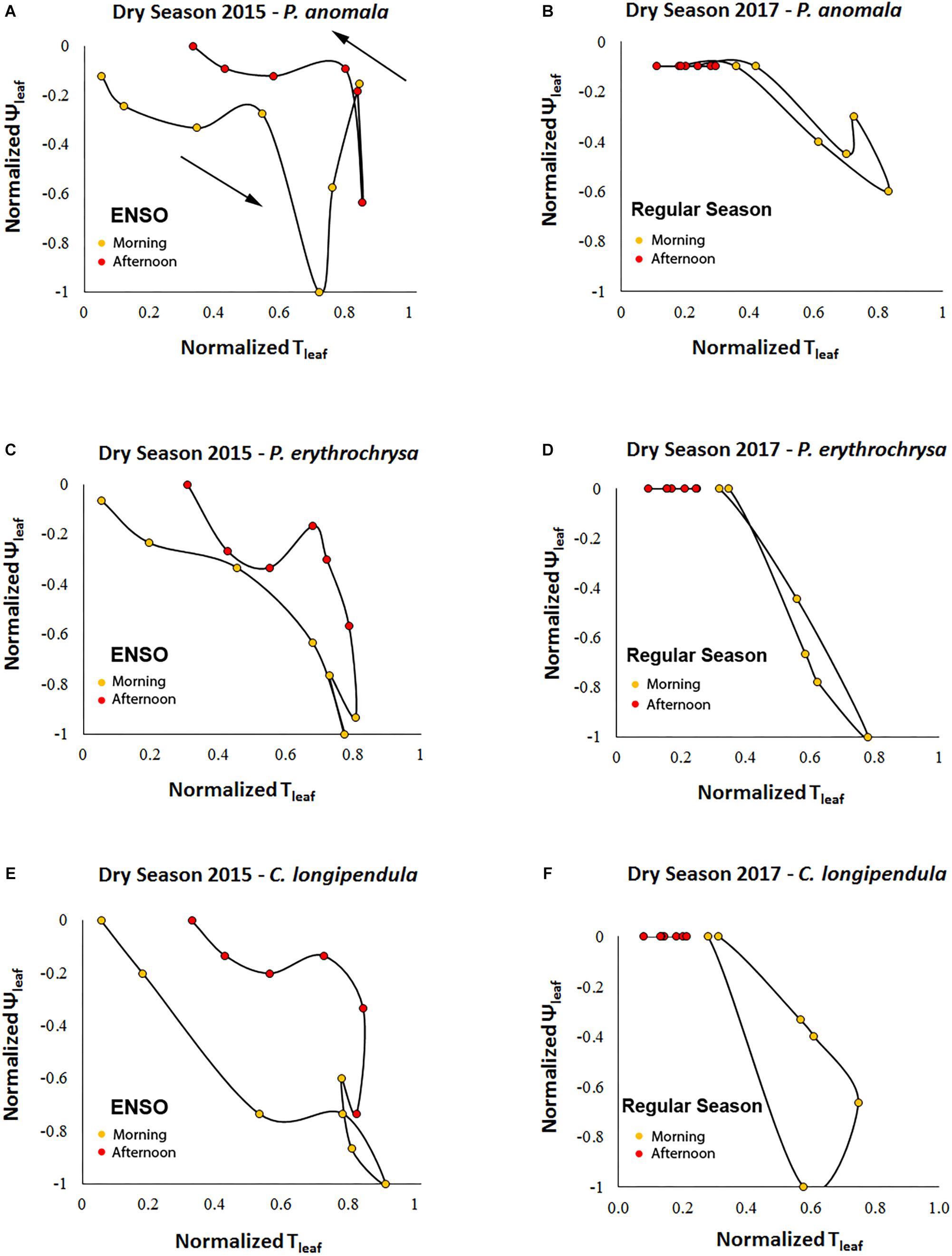
Figure 8. Counterclockwise hysteresis of ΨL and Tleaf for the species C. longipendula, P. anomala, and P. erythrochrysa in Manaus during the 2015 and 2017 dry seasons. The stomata resistance acts to minimize the water loss during the afternoon periods given the observed counterclockwise pattern for ΨL–Tleaf. During September 2015 (peak of the El Niño) the Hindex of the variables ΨL and Tleaf was significant higher (A,C,E) compared to September 2017 (regular dry season) (p < 0.01, α = 0.05) (B,D,F).
Discussion
Correlations Between Sap Velocity, Tleaf, Tair and ΔVPD
With a fine-scale measurement resolution of 15 min, temporal similarities between Vs and Tleaf were observed for all studied species. Delays between Vs-Tleaf and Vs-ΔVPD, are expected due to the large differences in the heights of the measurements (basal sap velocity versus Tleaf in the upper canopy). This type of delays are related to the capacitance of the stems, where the evaporative demand in the branches near the crown are greater than the basal portions of the trunk, with an observed lag in the morning period between these two portions of the tree (Meinzer et al., 2003). Future research should aim to quantify these delays, using for example, simultaneous measurements of Vs at DBH height and Vs in the branches near the crown, as in Goldstein et al. (1998). At a small time resolution, similar results were previously observed using Granier sap flow system and micrometeorological sensors in French Guiana (Granier et al., 1996), and North Carolina, United States (Oren et al., 1999a). At both sites (F. Guiana and N. Carolina), similar temporal correlations were observed between sap flow and VPD using a measurement resolution of 30 min. A recent study of Bretfeld et al. (2018) showed that Vs is largely in phase with VPDair in an 80 year-old-forest which is similar to the observed results of this study. In addition, sigmoid patterns between Vs-VPD and Vs-Tleaf were also observed for all studied trees (Supplementary Figure S5), as also observed by O’Brien et al. (2004) in tropical species of Costa Rica, and da Costa et al. (2018) in the eastern Amazon. In this study, sap velocity displayed species-specific diurnal hysteresis patterns with a sigmoidal increase during the morning period and an exponential decrease during the afternoon/night period, with statistical differences between the extreme 2015 dry season (ENSO) and a normal 2017 dry season (“control scenario”) (Figures 6, 7).
Given the large diurnal Tleaf variation and the exponential dependence of VPD on Tleaf (Eqs 2, 3), changes in VPD during the daytime are largely driven by changes in Tleaf (Jackson et al., 1981; Ewers and Oren, 2000). The relationship between Tleaf and Tair represented by Tleaf-Tair offset is a good indicator of water stress in plants (Jackson et al., 1981). Over short time scales (e.g., 10 s) Tleaf is also partially related to the leaf mass per area (Michaletz et al., 2016), which can provide new insights about the water dynamics like capacitance. Low temperature values during rainstorms are associated with low VPD and high RH in the Amazon forest (Moradi et al., 2016). Periods of days with the lowest observed Vs are related to the lowest observed values of Tleaf and ΔVPD. This observation is consistent with previous studies in the Amazon where rain, cloud coverage, and reduced direct solar radiation were found to be the major factors that reduced xylem sap flow rates (Kunert et al., 2017). In addition, other researchers in tropical sites like Costa Rica found that significant leaf wetness also reduces sap flow by up to 28% by impacting VPD (Aparecido et al., 2016).
The positive nighttime Vs observed in Manaus and Santarém also apparently followed Tleaf, similar to that observed by Burgess and Dawson (2004) which found strong positive similarities between nighttime sap flow and VPD in Sequoia sempervirens. These observations of positive nighttime Vs in the first hours of the night are probably related to the capacitance of stem tissues and radial water transport (Steppe et al., 2012), as well as incomplete stomatal closure (Snyder et al., 2003; Barbour and Buckley, 2007; Dawson et al., 2007) which also allows trees to increase their water content through foliar water uptake (Eller et al., 2013). Another explanation for nighttime transpiration is the water outlet through lenticels, small pores on stem surfaces of many tropical tree species (Roth, 1981). However, it should be noted that no treatment was done to correct Vs for small potential offsets (less than 1 cm h-1 – lower scales) to estimate the standard deviation of Vs, and the possibility of positive nighttime water flow where, in this study, the Vs values are generally close to zero.
Hysteresis Patterns
In the current study, clockwise hysteresis patterns were observed for Vs-Tleaf and Vs-ΔVPD (Figures 4, 5). Similar results were previously described for sap flow and VPD in tropical forests of Costa Rica (O’Brien et al., 2004) and in tropical secondary forests in Panama (Bretfeld et al., 2018), in temperate forests of Australia (Zeppel et al., 2004), in eastern Amazon trees (Brum et al., 2018) and in a grass-land ecosystem (Zhang et al., 2014). The observed hysteresis phenomenon for sap velocity has been described as a result of the temporal offset of gs that tends to peak, in the tropics, during late morning to mid-day (10:30–12:00) (Slot and Winter, 2017a) (Supplementary Figure S6) and VPD that tends to peak in the early afternoon (13:00–14:30). The hysteresis phenomenon can also be visualized with variables Tleaf and direct solar radiation (irradiance). In normal conditions gs respond positively to irradiance (Hennessey and Field, 1991; Gorton et al., 1993; Motzer et al., 2005) and the temporal similarities between these two variables are supposed to be associated with circadian cycles (daily patterns), as demonstrated in some hysteretic behaviors of this study separating the morning, afternoon, and night periods.
In terms of temperature, gs was found to reach a maximum at a Tleaf of 31–33°C, which relates with the optimum temperature for photosynthesis (Topt) previously determined for many tropical species (Slot and Winter, 2017b). Interestingly, this Topt range seems to match with the inflection point (xhalf) in the Vs-Tleaf hysteresis plots in Manaus (Figures 4–7). Thus, we speculate that it may be possible to determine Topt using Vs-Tleaf diurnal hysteresis plots for individual trees. The observed inflection points patterns of Vs-Tleaf during the morning period for the species E. cyathiformis, P. anomala, and P. erythrochrysa (Figure 7) seems to be influenced by the canopy position and the resistance to drought once, in this study, species-specific shifts emerged when the 2015 ENSO was compared to the 2017 dry season. Additionally, the calculation of an index to quantify the hysteresis loops (Hindex) was performed by this study, similar to a previous approach of Zuecco et al. (2016) which described hysteresis patterns of hydrological variables and runoff events. A quantitative comparison of hysteresis loops from a large number of species using an index approach would be a way of constraining ranges of hysteresis effects in models. Satellite observations revealed that September 2015 exhibited the warmest monthly averaged surface air temperature of any other month over the past 13 years in the Central Amazon (Fontes et al., 2018). These extreme temperatures also modified Tleaf patterns, as observed by this study (Figure 3). Higher Tleaf values were reflected in a significantly higher Hindex for the variables Tleaf-Tair and ΨL-Tleaf when the 2015 dry season (ENSO period) was compared to the 2017 dry season (“control scenario”). Even though Hindex for Vs was not calculated in this study, we found species-specific shifts in diurnal sap velocity dynamics in both 2015 and 2017 dry seasons contradicting the expected higher values of transpiration during 2015 ENSO compared to other periods due to the high evaporative demand. Brum et al. (2018), for example, found significant differences in the Hindex for transpiration between dry and wet seasons during the 2015–2016 ENSO in the Eastern Amazon. Nevertheless, the effect of gs during drought events needs to be clarified because trees are expected to show a strong stomatal control under drought conditions, which would offset the expected increase in transpiration Hindex. The higher Tleaf values compared to Tair during the middle morning to early afternoon observed by this study for the species P. anomala, C. longipendula, and P. erythrochrysa also contradicts with the expected decrease in Tleaf due the transpiration effect. One possible explanation for this pattern is the leaf flushing which is, in the Central Amazon, concentrated in the five driest months (Lopes et al., 2016). On this period, it is possible that the leaf photosynthesis apparatus and tissues aren’t fully developed, reflecting directly in the gs and transpiration patterns. Also, the increasing of light availability in the Amazon forest during the dry season can, maybe, exert an overriding effect in Tleaf patterns relative to the cooling effect of transpiration.
Changes in gs are associated with changes in ΨL via their mutual effects on the balance between Vs and transpiration rates. Consistent with the clockwise hysteresis between Vs-Tleaf and Vs-ΔVPD, a counterclockwise hysteresis pattern was observed between ΨL-Tleaf. At the same Tleaf, ΨL were more negative during morning than afternoon suggesting that partial stomatal closure in the afternoon allows the leaf to recover to less negative ΨL (Figure 8; Jarvis, 1976). The results suggest that during 2015–2016 ENSO the trees of this study located in a plateau area have a strong isohydric behavior, by reducing stomatal conductance in the warm afternoon periods in order to reduce transpiration rates, thereby minimizing the chances for embolism (Sade et al., 2012; Roman et al., 2015). The same process can be observed in the clockwise hysteresis between Vs-Tleaf, and Vs-ΔVPD where the partial stomatal closure in the afternoon period reduce the Vs rates compared to the morning (Figures 4, 5). In this study this pattern was called the “gs effect.” It should be emphasized that the daily hysteresis patterns with the morning, afternoon and night periods separated by colors make it possible to observe both the “gs effect” and “VPD effect” (Figure 5).
Interestingly, the counterclockwise hysteresis pattern of Vs-direct solar radiation is not driven by the partial stomatal closure in the late morning until the afternoon period, but by the high ΔVPD. At the same solar radiation intensity, higher Vs occurs during the afternoon relative to the morning and in this study was called the “VPD effect” (Figure 5). This observations suggests that partial stomatal closure in the afternoon can be offset by the effect of high ΔVPD in maintaining elevated transpiration rates under high afternoon temperatures, as previously shown in other ecosystems (O’Brien et al., 2004; Zeppel et al., 2004; Zhang et al., 2014; Novick et al., 2016; Bretfeld et al., 2018; Brum et al., 2018). Also, these results support recent findings that heat waves can be associated with sustained transpirational cooling as a key mechanism of thermotolerance (Drake et al., 2018). However, the mechanisms of transpiration cooling can be exceeded by the heat intensity during El Niño events, which increase tree mortality in the Amazon forest (Aleixo et al., 2019). Finally, the range of Hindex for the variables Tleaf and Tair presented for the first time in this study, can be a useful tool to predict future impacts on tree mortality during extreme drought events in comparison to other periods.
The observed differences of the maximum Vs rates (curve’s maximum values (max) of sigmoidal functions during the morning period – Figure 7)) between species can be related to the diameter of the vessels (Dünisch and Morais, 2002), wood density (Eller et al., 2018) and with the susceptibility to embolism (Lovisolo and Schubert, 1998). Tree height is also an important factor which influences sun exposure and therefore the temperature of the leaves and transpiration rates (Goldstein et al., 1998). In Manaus, E. cyathiformis was the thinnest and shortest studied tree with 14.3 cm of DBH and 19.8 m of height and was the tree with the lowest observed Vs rates (∼8 cm h-1) during ENSO (Figure 4). In contrast, the Pouteria genus (P. anomala and P. erythrochysa) have large DBHs (35.3 cm and 36.5 cm) and high rates of Vs during ENSO (18 and 12 cm h-1, respectively) (Figure 4). This is consistent with other studies where DBH, height and sap flow showed a positive correlation (Motzer et al., 2005). Likewise, the same correlations were observed in Santarém, where the larger DBHs showed the higher sap velocity. Also, the observed differences in curve’s maximum values (max) of sigmoidal curves observed for Vs and Tleaf during ENSO period and the regular dry season (Figure 7), may be associated with the high range of functional traits and susceptibility to embolism of some trees in the Amazon forest along hydro-topographic gradients (Cosme et al., 2017; Oliveira et al., 2019). Other important issue is that mortality rates during droughts are substantially higher for large DBH’s (Meakem et al., 2017), and maybe this is potentially aggravated by high transpiration rates and the crown exposure to the direct light. Another study of rain exclusion in the eastern Amazon by Rowland et al. (2015) support this observation. However, other factors are also involved since it has been shown that early-successional forests experienced more drought stress than trees in late-successional forests (Bretfeld et al., 2018). In fact, more studies are needed in the tropics, especially in the Amazon, due to the large diversity of terrestrial plants (Ter Steege et al., 2013), the wide range of functional traits and evolutionary strategies to avoid cavitation, carbon starvation, and other aspects related to drought which can modify Tleaf and Vs patterns.
Conclusion
For the first time in the Amazon forest, the quantitative differences and the hysteresis pattern between Tleaf and Tair were demonstrated and compared during the 2015 (ENSO) and 2017 (“control scenario”) dry seasons. The relationship between Tleaf and Tair was significantly different between these two periods and, in general, Tleaf was higher than Tair during the middle morning to early afternoon. The use of the variable Tleaf together with Tair are extremely important to ecophysiological observations due to the differences in terms of magnitude and temporal patterns. Also, Tleaf is an important variable to estimate the true water vapor pressure gradient between the substomatal cavity and the boundary layer of the air near the leaf surface (ΔVPD). Moreover, sap velocity displayed species-specific diurnal hysteresis patterns that were strongly linked to gs and VPD and reflected by changes in Tleaf. In the morning, gs was linearly related to Tleaf and sap velocity displayed a sigmoidal relationship with Tleaf. In the afternoon, stomatal conductance declined as Tleaf approached a daily peak, allowing ΨL to begin recovery, while sap velocity declined with an exponential relationship with Tleaf. Hysteresis indices (Tleaf : Tair and Tleaf : ΨL) were much more pronounced during the ENSO event than during a typical dry season and varied between species, which reflects species-specific capacitance and tree hydraulic traits. Future research may address a new modeling approach using the magnitude of the hysteresis loops (Hindex) to measure, for example, the intensity of the droughts and how it impacts plant communities. Finally, the hysteretic behavior of the transpiration separated by morning, afternoon and night periods is the key to understand the complexity of this process in a changing climate and improve the current models.
Author Contributions
BG, KJ, RN-J, IS-F, CF, LC, JH, JC, NH, TD, and NM performed the experiments and analyzed the data. JC, NH, TD, and NM planned and designed the experiments. BG and KJ wrote the manuscript. CF, AA, JW, BN, CV, DC, GS, CK, and NM improved the manuscript.
Funding
This material is based upon work supported as part of the Next Generation Ecosystem Experiments-Tropics (NGEE-Tropics), as part of DOE’s Terrestrial Ecosystem Science Program – Contract No. DE-AC02-05CH11231. Additional funding for this research was provided by the Coordenação de Aperfeiçoamento de Pessoal de Nível Superior (CAPES).
Conflict of Interest Statement
The authors declare that the research was conducted in the absence of any commercial or financial relationships that could be construed as a potential conflict of interest.
Acknowledgments
The authors are thankful to the logistical and scientific support provided by the Laboratório de Manejo Florestal (LMF) and the Large-Scale Biosphere-Atmosphere Program (LBA) at the National Institute of Amazonian Research (INPA).
Supplementary Material
The Supplementary Material for this article can be found online at: https://www.frontiersin.org/articles/10.3389/fpls.2019.00830/full#supplementary-material
Footnotes
References
Adams, H. D., Zeppel, M. J., Anderegg, W. R., Hartmann, H., Landhäusser, S. M., Tissue, D. T., et al. (2017). A multi-species synthesis of physiological mechanisms in drought-induced tree mortality. Nat. Ecol. Evol. 1, 1285–1291. doi: 10.1038/s41559-017-0248-x
Aleixo, I., Norris, D., Hemerik, L., Barbosa, A., Prata, E., Costa, F., et al. (2019). Amazonian rainforest tree mortality driven by climate and functional traits. Nat. Clim. Change 9, 384–388. doi: 10.1038/s41558-019-0458-0
Aparecido, L. M. T., Miller, G. R., Cahill, A. T., and Moore, G. W. (2016). Comparison of tree transpiration under wet and dry canopy conditions in a costa rican premontane tropical forest. Hydrol. Process. 30, 5000–5011. doi: 10.1002/hyp.10960
Araújo, A., Nobre, A., Kruijt, B., Elbers, J., Dallarosa, R., Stefani, P., et al. (2002). Comparative measurements of carbon dioxide fluxes from two nearby towers in a central amazonian rainforest: the manaus LBA site. J. Geophys. Res. Atmos. 107:8090. doi: 10.1029/2001JD000676
Barbour, M. M., and Buckley, T. N. (2007). The stomatal response to evaporative demand persists at night in Ricinus communis plants with high nocturnal conductance. Plant Cell Environ. 30, 711–721. doi: 10.1111/j.1365-3040.2007.01658.x
Bretfeld, M., Ewers, B. E., and Hall, J. S. (2018). Plant water use responses along secondary forest succession during the 2015–2016 El Niño drought in panama. New Phytol. 219, 885–899. doi: 10.1111/nph.15071
Brodribb, T. J., McAdam, S. A., and Carins Murphy, M. R. (2017). Xylem and stomata, coordinated through time and space. Plant Cell Environ. 40, 872–880. doi: 10.1111/pce.12817
Brum, M., Gutiérrez López, J., Asbjornsen, H., Licata, J., Pypker, T., Sanchez, G., et al. (2018). ENSO effects on the transpiration of eastern amazon trees. Philos. Trans. R. Soc. B Biol. Sci. 373:20180085. doi: 10.1098/rstb.2018.0085
Buckley, T. N., John, G. P., Scoffoni, C., and Sack, L. (2017). The sites of evaporation within leaves. Plant Physiol. 173, 1763–1782. doi: 10.1104/pp.16.01605
Burgess, S., and Downey, A. (2014). SFM1 Sap Flow Meter Manual. Armidale, NSW: ICT international Pty Ltd.
Burgess, S. S., Adams, M. A., Turner, N. C., Beverly, C. R., Ong, C. K., Khan, A. A., et al. (2001). An improved heat pulse method to measure low and reverse rates of sap flow in woody plants. Tree Physiol. 21, 589–598. doi: 10.1093/treephys/21.9.589
Burgess, S. S. O., and Dawson, T. E. (2004). The contribution of fog to the water relations of Sequoia sempervirens (D. Don): foliar uptake and prevention of dehydration. Plant Cell Environ. 27, 1023–1034. doi: 10.1111/j.1365-3040.2004.01207.x
Cernusak, L. A., Ubierna, N., Jenkins, M. W., Garrity, S. R., Rahn, T., Powers, H. H., et al. (2018). Unsaturation of vapour pressure inside leaves of two conifer species. Sci. Rep. 8:7667. doi: 10.1038/s41598-018-25838-2
Chambers, J., Davies, S., Koven, C., Kueppers, L., Leung, R., McDowell, N., et al. (2014). Next Generation Ecosystem Experiment (NGEE) Tropics. Berkeley, CA: Lawrence Berkeley National Laboratory.
Chambers, J. Q., and Artaxo, P. (2017). Biosphere-atmosphere interactions: deforestation size influences rainfall. Nat. Clim. Change 7, 175–176. doi: 10.1038/nclimate3238
Chave, J., Andalo, C., Brown, S., Cairns, M., Chambers, J., Eamus, D., et al. (2005). Tree allometry and improved estimation of carbon stocks and balance in tropical forests. Oecologia 145, 87–99. doi: 10.1007/s00442-005-0100-x
Christianson, D. S., Varadharajan, C., Christoffersen, B., Detto, M., Faybishenko, B., Gimenez, B. O., et al. (2017). A metadata reporting framework (FRAMES) for synthesis of ecohydrological observations. Ecol. Inform. 42, 148–158. doi: 10.1016/j.ecoinf.2017.06.002
Cosme, L. H., Schietti, J., Costa, F. R., and Oliveira, R. S. (2017). The importance of hydraulic architecture to the distribution patterns of trees in a central Amazonian forest. New Phytol. 215, 113–125. doi: 10.1111/nph.14508
Costa, M. H., Biajoli, M. C., Sanches, L., Malhado, A. C. M., Hutyra, L. R., da Rocha, H. R., et al. (2010). Atmospheric versus vegetation controls of amazonian tropical rain forest evapotranspiration: are the wet and seasonally dry rain forests any different? J. Geophys. Res. Biogeosci. 115:G04021. doi: 10.1029/2009JG001179
da Costa, A. C., Rowland, L., Oliveira, R. S., Oliveira, A. A., Binks, O. J., Salmon, Y., et al. (2018). Stand dynamics modulate water cycling and mortality risk in droughted tropical forest. Glob. Chang. Biol. 24, 249–258. doi: 10.1111/gcb.13851
Daloso, D. M., Medeiros, D. B., Anjos, L., Yoshida, T., Araújo, W. L., and Fernie, A. R. (2017). Metabolism within the specialized guard cells of plants. New Phytol. 216, 1018–1033. doi: 10.1111/nph.14823
Dawson, T. E., Burgess, S. S., Tu, K. P., Oliveira, R. S., Santiago, L. S., Fisher, J. B., et al. (2007). Nighttime transpiration in woody plants from contrasting ecosystems. Tree Physiol. 27, 561–575. doi: 10.1093/treephys/27.4.561
Drake, J. E., Tjoelker, M. G., Vårhammar, A., Medlyn, B. E., Reich, P. B., Leigh, A., et al. (2018). Trees tolerate an extreme heatwave via sustained transpirational cooling and increased leaf thermal tolerance. Glob. Chang. Biol. 24, 2390–2402. doi: 10.1111/gcb.14037
Dünisch, O., and Morais, R. R. (2002). Regulation of xylem sap flow in an evergreen, a semi-deciduous, and a deciduous meliaceae species from the amazon. Trees 16, 404–416. doi: 10.1007/s00468-002-0182-6
Eller, C. B., Barros, F. V., Bittencourt, P. R. L., Rowland, L., Mencuccini, M., and Oliveira, R. S. (2018). Xylem hydraulic safety and construction costs determine tropical tree growth. Plant Cell Environ. 41, 548–562. doi: 10.1111/pce.13106
Eller, C. B., Lima, A. L., and Oliveira, R. S. (2013). Foliar uptake of fog water and transport belowground alleviates drought effects in the cloud forest tree species, Drimys brasiliensis (winteraceae). New Phytol. 199, 151–162. doi: 10.1111/nph.12248
Eltahir, E. A., and Bras, R. L. (1994). Precipitation recycling in the Amazon basin. Q. J. R. Meteorol. Soc. 120, 861–880. doi: 10.1002/qj.49712051806
Ewers, B. E., and Oren, R. (2000). Analyses of assumptions and erros in the calculation of stomatal conductance from sap flux measurements. Tree Physiol. 20, 579–589. doi: 10.1093/treephys/20.9.579
Farquhar, G. (1978). Feedforward responses of stomata to humidity. Funct. Plant Biol. 5, 787–800. doi: 10.1071/PP9780787
Field, C. B., Barros, V. R., Mach, K., and Mastrandrea, M. (2014). Climate Change 2014: Impacts, Adaptation, and Vulnerability. Cambridge: Cambridge University Press.
Fontes, C. G., Dawson, T. E., Jardine, K., McDowell, N., Gimenez, B. O., Anderegg, L., et al. (2018). Dry and hot: the hydraulic consequences of a climate change–type drought for amazonian trees. Philos. Trans. R. Soc. B Biol. Sci. 373:20180209. doi: 10.1098/rstb.2018.0209
Goldstein, G., Andrade, J., Meinzer, F., Holbrook, N., Cavelier, J., Jackson, P., et al. (1998). Stem water storage and diurnal patterns of water use in tropical forest canopy trees. Plant Cell Environ. 21, 397–406. doi: 10.1046/j.1365-3040.1998.00273.x
Gorton, H. L., Williams, W. E., and Assmann, S. M. (1993). Circadian rhythms in stomatal responsiveness to red and blue light. Plant Physiol. 103, 399–406. doi: 10.1104/pp.103.2.399
Granier, A., Huc, R., and Barigah, S. (1996). Transpiration of natural rain forest and its dependence on climatic factors. Agric. For. Meteorol. 78, 19–29. doi: 10.1016/0168-1923(95)02252-X
Green, S., Clothier, B., and Jardine, B. (2003). Theory and practical application of heat pulse to measure sap flow. Agron. J. 95, 1371–1379. doi: 10.2134/agronj2003.1371
Grossiord, C., Sevanto, S., Borrego, I., Chan, A. M., Collins, A. D., Dickman, L. T., et al. (2017). Tree water dynamics in a drying and warming world. Plant Cell Environ. 40, 1861–1873. doi: 10.1111/pce.12991
Hennessey, T. L., and Field, C. B. (1991). Circadian rhythms in photosynthesis: oscillations in carbon assimilation and stomatal conductance under constant conditions. Plant Physiol. 96, 831–836. doi: 10.1104/pp.96.3.831
Hutyra, L. R., Munger, J. W., Saleska, S. R., Gottlieb, E., Daube, B. C., Dunn, A. L., et al. (2007). Seasonal controls on the exchange of carbon and water in an Amazonian rain forest. J. Geophys. Res. Biogeosci. 112:G03008. doi: 10.1029/2006JG000365
Jackson, R. D., Idso, S., Reginato, R., and Pinter, P. (1981). Canopy temperature as a crop water stress indicator. Water Resour. Res. 17, 1133–1138. doi: 10.1029/WR017i004p01133
Jarvis, P. (1976). The interpretation of the variations in leaf water potential and stomatal conductance found in canopies in the field. Philos. Trans. R. Soc. Lond. B Biol. Sci. 273, 593–610. doi: 10.1098/rstb.1976.0035
Jasechko, S., Sharp, Z. D., Gibson, J. J., Birks, S. J., Yi, Y., and Fawcett, P. J. (2013). Terrestrial water fluxes dominated by transpiration. Nature 496, 347–350. doi: 10.1038/nature11983
Jones, H. G. (1998). Stomatal control of photosynthesis and transpiration. J. Exp. Bot. 49, 387–398.
Kunert, N., Aparecido, L. M. T., Wolff, S., Higuchi, N., dos Santos, J., de Araujo, A. C., et al. (2017). A revised hydrological model for the central amazon: the importance of emergent canopy trees in the forest water budget. Agric. For. Meteorol. 239, 47–57. doi: 10.1016/j.agrformet.2017.03.002
Lambers, H., Chapin, F. S., and Pons, T. L. (2008). “Plant water relations,” in Plant Physiological Ecology, eds L. P. Thijs, H. Lambers, and F. S. Chapin (New York, NY: Springer), 163–223.
Lloyd, J., and Farquhar, G. D. (2008). Effects of rising temperatures and [CO2] on the physiology of tropical forest trees. Philos. Trans. R. Soc. B Biol. Sci. 363, 1811–1817. doi: 10.1098/rstb.2007.0032
Lopes, A. P., Nelson, B. W., Wu, J., de Alencastro Graça, P. M. L., Tavares, J. V., Prohaska, N., et al. (2016). Leaf flush drives dry season green-up of the central amazon. Remote Sens. Environ. 182, 90–98. doi: 10.1016/j.rse.2016.05.009
Lovisolo, C., and Schubert, A. (1998). Effects of water stress on vessel size and xylem hydraulic conductivity in Vitis vinifera L. J. Exp. Bot. 49, 693–700. doi: 10.1093/jxb/49.321.693
McAdam, S. A., Sussmilch, F. C., and Brodribb, T. J. (2016). Stomatal responses to vapour pressure deficit are regulated by high speed gene expression in angiosperms. Plant Cell Environ. 39, 485–491. doi: 10.1111/pce.12633
McDowell, N., Allen, C. D., Anderson-Teixeira, K., Brando, P., Brienen, R., Chambers, J., et al. (2018). Drivers and mechanisms of tree mortality in moist tropical forests. New Phytol. 219, 851–869. doi: 10.1111/nph.15027
Meakem, V., Tepley, A. J., Gonzalez-Akre, E. B., Herrmann, V., Muller-Landau, H. C., Wright, S. J., et al. (2017). Role of tree size in moist tropical forest carbon cycling and water deficit responses. New Phytol. 219, 947–958. doi: 10.1111/nph.14633
Meinzer, F., Goldstein, G., Holbrook, N., Jackson, P., and Cavelier, J. (1993). Stomatal and environmental control of transpiration in a lowland tropical forest tree. Plant Cell Environ. 16, 429–436. doi: 10.1111/j.1365-3040.1993.tb00889.x
Meinzer, F. C., James, S. A., Goldstein, G., and Woodruff, D. (2003). Whole-tree water transport scales with sapwood capacitance in tropical forest canopy trees. Plant Cell Environ. 26, 1147–1155. doi: 10.1046/j.1365-3040.2003.01039.x
Michaletz, S. T., Weiser, M. D., McDowell, N. G., Zhou, J., Kaspari, M., Helliker, B. R., et al. (2016). The energetic and carbon economic origins of leaf thermoregulation. Nat. Plants 2:16129. doi: 10.1038/nplants.2016.129
Moradi, I., Arkin, P., Ferraro, R., Eriksson, P., and Fetzer, E. (2016). Diurnal variation of tropospheric relative humidity in tropical regions. Atmos. Chem. Phys. 16, 6913–6929. doi: 10.5194/acp-16-6913-2016
Motzer, T., Munz, N., Küppers, M., Schmitt, D., and Anhuf, D. (2005). Stomatal conductance, transpiration and sap flow of tropical montane rain forest trees in the southern ecuadorian andes. Tree Physiol. 25, 1283–1293. doi: 10.1093/treephys/25.10.1283
Negrón-Juárez, R. I., Hodnett, M. G., Fu, R., Goulden, M. L., and von Randow, C. (2007). Control of dry season evapotranspiration over the amazonian forest as inferred from observations at a southern Amazon forest site. J. Clim. 20, 2827–2839. doi: 10.1175/JCLI4184.1
Novick, K. A., Miniat, C. F., and Vose, J. M. (2016). Drought limitations to leaf-level gas exchange: results from a model linking stomatal optimization and cohesion–tension theory. Plant Cell Environ. 39, 583–596. doi: 10.1111/pce.12657
O’Brien, J., Oberbauer, S., and Clark, D. (2004). Whole tree xylem sap flow responses to multiple environmental variables in a wet tropical forest. Plant Cell Environ. 27, 551–567. doi: 10.1111/j.1365-3040.2003.01160.x
Oliveira, R. S., Costa, F. R., van Baalen, E., de Jonge, A., Bittencourt, P. R., Almanza, Y., et al. (2019). Embolism resistance drives the distribution of amazonian rainforest tree species along hydro-topographic gradients. New Phytol. 221, 1457–1465. doi: 10.1111/nph.15463
Oren, R., Phillips, N., Ewers, B., Pataki, D., and Megonigal, J. (1999a). Sap-flux-scaled transpiration responses to light, vapor pressure deficit, and leaf area reduction in a flooded Taxodium distichum forest. Tree Physiol. 19, 337–347. doi: 10.1093/treephys/19.6.337
Oren, R., Sperry, J., Katul, G., Pataki, D., Ewers, B., Phillips, N., et al. (1999b). Survey and synthesis of intra-and interspecific variation in stomatal sensitivity to vapour pressure deficit. Plant Cell Environ. 22, 1515–1526. doi: 10.1046/j.1365-3040.1999.00513.x
Phillips, N., Nagchaudhuri, A., Oren, R., and Katul, G. (1997). Time constant for water transport in loblolly pine trees estimated from time series of evaporative demand and stem sapflow. Trees 11, 412–419. doi: 10.1007/s004680050102
Roman, D., Novick, K., Brzostek, E., Dragoni, D., Rahman, F., and Phillips, R. (2015). The role of isohydric and anisohydric species in determining ecosystem-scale response to severe drought. Oecologia 179, 641–654. doi: 10.1007/s00442-015-3380-9
Rowland, L., da Costa, A. C. L., Galbraith, D. R., Oliveira, R., Binks, O. J., Oliveira, A., et al. (2015). Death from drought in tropical forests is triggered by hydraulics not carbon starvation. Nature 528, 119–122. doi: 10.1038/nature15539
Sade, N., Gebremedhin, A., and Moshelion, M. (2012). Risk-taking plants: anisohydric behavior as a stress-resistance trait. Plant Signal. Behav. 7, 767–770. doi: 10.4161/psb.20505
Saleska, S. R., Miller, S. D., Matross, D. M., Goulden, M. L., Wofsy, S. C., Da Rocha, H. R., et al. (2003). Carbon in amazon forests: unexpected seasonal fluxes and disturbance-induced losses. Science 302, 1554–1557. doi: 10.1126/science.1091165
Segura, M., and Kanninen, M. (2005). Allometric models for tree volume and total aboveground biomass in a tropical humid forest in costa rica. Biotropica 37, 2–8. doi: 10.1111/j.1744-7429.2005.02027.x
Slot, M., and Winter, K. (2017a). In situ temperature relationships of biochemical and stomatal controls of photosynthesis in four lowland tropical tree species. Plant Cell Environ. 40, 3055–3068. doi: 10.1111/pce.13071
Slot, M., and Winter, K. (2017b). In situ temperature response of photosynthesis of 42 tree and liana species in the canopy of two panamanian lowland tropical forests with contrasting rainfall regimes. New Phytol. 214, 1103–1117. doi: 10.1111/nph.14469
Snyder, K., Richards, J., and Donovan, L. (2003). Night-time conductance in C3 and C4 species: do plants lose water at night? J. Exp. Bot. 54, 861–865. doi: 10.1093/jxb/erg082
Steppe, K., Cochard, H., Lacointe, A., and Ameglio, T. (2012). Could rapid diameter changes be facilitated by a variable hydraulic conductance? Plant Cell Environ. 35, 150–157. doi: 10.1111/j.1365-3040.2011.02424.x
Steppe, K., De Pauw, D. J. W., Doody, T. M., and Teskey, R. O. (2010). A comparison of sap flux density using thermal dissipation, heat pulse velocity and heat field deformation methods. Agric. For. Meteorol. 150, 1046–1056. doi: 10.1016/j.agrformet.2010.04.004
Synnott, T. J. (1979). A Manual of Permanent Plot Procedures for Tropical Rainforests. Oxford: University of Oxford.
Ter Steege, H., Pitman, N. C., Sabatier, D., Baraloto, C., Salomão, R. P., Guevara, J. E., et al. (2013). Hyperdominance in the amazonian tree flora. Science 342:1243092. doi: 10.1126/science.1243092
Tinoco-Ojanguren, C., and Pearcy, R. W. (1993). Stomatal dynamics and its importance to carbon gain in two rainforest piper species. I. VPD effects on the transient stomatal response to lightflecks. Oecologia 94, 388–394. doi: 10.1007/BF00317115
Ward, D. A., and Bunce, J. A. (1986). Novel evidence for a lack of water vapour saturation within the intercellular airspace of turgid leaves of mesophytic species. J. Exp. Bot. 37, 504–516. doi: 10.1093/jxb/37.4.504
Ward, E. J., Bell, D. M., Clark, J. S., and Oren, R. (2012). Hydraulic time constants for transpiration of loblolly pine at a free-air carbon dioxide enrichment site. Tree Physiol. 33, 123–134. doi: 10.1093/treephys/tps114
Wu, J., Guan, K., Hayek, M., Restrepo-Coupe, N., Wiedemann, K. T., Xu, X., et al. (2017). Partitioning controls on Amazon forest photosynthesis between environmental and biotic factors at hourly to interannual timescales. Glob. Chang. Biol. 23, 1240–1257. doi: 10.1111/gcb.13509
Zeppel, M. J., Murray, B. R., Barton, C., and Eamus, D. (2004). Seasonal responses of xylem sap velocity to VPD and solar radiation during drought in a stand of native trees in temperate Australia. Funct. Plant Biol. 31, 461–470. doi: 10.1071/FP03220
Zhang, Q., Manzoni, S., Katul, G., Porporato, A., and Yang, D. (2014). The hysteretic evapotranspiration—vapor pressure deficit relation. J. Geophys. Res. Biogeosci. 119, 125–140. doi: 10.1002/2013JG002484
Keywords: tropical forests, sap velocity, stomatal conductance, direct solar radiation, vapor pressure deficit, leaf temperature, hysteresis
Citation: Gimenez BO, Jardine KJ, Higuchi N, Negrón-Juárez RI, Sampaio-Filho IdJ, Cobello LO, Fontes CG, Dawson TE, Varadharajan C, Christianson DS, Spanner GC, Araújo AC, Warren JM, Newman BD, Holm JA, Koven CD, McDowell NG and Chambers JQ (2019) Species-Specific Shifts in Diurnal Sap Velocity Dynamics and Hysteretic Behavior of Ecophysiological Variables During the 2015–2016 El Niño Event in the Amazon Forest. Front. Plant Sci. 10:830. doi: 10.3389/fpls.2019.00830
Received: 29 October 2018; Accepted: 07 June 2019;
Published: 28 June 2019.
Edited by:
Sebastian Leuzinger, Auckland University of Technology, New ZealandReviewed by:
Howard Scott Neufeld, Appalachian State University, United StatesMario Bretfeld, University of Wyoming, United States
Copyright © 2019 Gimenez, Jardine, Higuchi, Negrón-Juárez, Sampaio-Filho, Cobello, Fontes, Dawson, Varadharajan, Christianson, Spanner, Araújo, Warren, Newman, Holm, Koven, McDowell and Chambers. This is an open-access article distributed under the terms of the Creative Commons Attribution License (CC BY). The use, distribution or reproduction in other forums is permitted, provided the original author(s) and the copyright owner(s) are credited and that the original publication in this journal is cited, in accordance with accepted academic practice. No use, distribution or reproduction is permitted which does not comply with these terms.
*Correspondence: Bruno O. Gimenez, YnJ1bm8ub2xpdmEuZ2ltZW5lekBnbWFpbC5jb20=
†These authors have contributed equally to this work