- 1Shaanxi Engineering Research Center for Viti-Viniculture, College of Enology, Northwest A&F University, Yangling, China
- 2Wine Research Centre, The University of British Columbia, Vancouver, BC, Canada
- 3Department of Botany, The University of British Columbia, Vancouver, BC, Canada
Abscisic acid (ABA) is a plant hormone that can mitigate heavy metal toxicity. Exogenous ABA and ABA mimic 1 (AM1) were applied to study the influence on Zn uptake and accumulation in Vitis vinifera L. cv. Merlot seedlings exposed to excess Zn. The seedlings were treated with either normal or excess levels of Zn in combination with applications of ABA and AM1. Excess Zn exposure resulted in decreased lateral root length, decreased photosynthesis, elevated uptake, and accumulation of Zn in roots, trunks, and stems, decreased jasmonic acid content in roots and leaves, and induced the expression of Zn transportation- and detoxification-related genes. Remarkably, in the presence of toxic amounts of Zn, the exogenous application of ABA, but not of AM1, reduced the uptake and accumulation of Zn in roots and induced higher expression of both ZIP genes and detoxification-related genes in root and leaf. These results indicate that exogenous ABA enhances the tolerance of grape seedlings to excess Zn and that AM1 is not a suitable ABA mimic compound for Zn stress alleviation in grapes.
Introduction
Wine grapes are produced in more than 40 countries. Climates and soils of different regions contribute to the diversity of quality and type of the grape and wines (Anderson and Aryal, 2015). In the last decades, wine grape cultivation has been rapidly developed in tropical and subtropical areas, including Mexico, Venezuela, Peru, Brazil, India, Thailand, and South China (Intarapichet et al., 2007; Jogaiah et al., 2013; Terra et al., 2013; dos Santos Lima et al., 2014), driven by local wine market demand (Anderson and Aryal, 2015; Anderson and Nelgen, 2015). However, soils in these areas are often susceptible to heavy metal toxicity because of low pH and pollution.
Zinc (Zn) is an essential micronutrient for plants. It plays a key role in photosynthetic redox reactions, and it is an essential cofactor for many enzymes involved in nitrogen metabolism and protein synthesis (Hafeez et al., 2013). Similar to other plant micronutrients, Zn is beneficial in a narrow range of concentrations, and its bioavailability in soils increases at low pH (Alloway, 2012). In unpolluted soils, the amount of Zn is generally below 125 ppm (Baccio et al., 2003; Hussain et al., 2010). However, environmental pollution due to industrial and agricultural activities including excessive application of Zn-containing fertilizers and pesticides, manures, sewage sludge, smelters, incinerators, mines, and galvanized products has increased the concentration of Zn in many agricultural soils above the threshold of phytotoxicity (Robson, 1993a).
According to the no observed effect concentrations (NOECs), the highest Zn dose that can be added to soils without affecting plants is 32–400 mg/kg (fresh weight). Toxic effects are identified at total Zn concentrations of 100 to >1000 mg/kg (Alloway, 2012), and a tissue concentration ≥400 mg/kg (dry weight) of Zn is considered toxic for nearly all plants (Sofo et al., 2013). Generally, Zn phytotoxicity inhibits the growth of roots and stems, modifies leaf morphology, induces chlorosis, reduces photosynthesis, interferes with the uptake of other nutrients, induces stress phytohormones, and alters the expression of genes related to Zn accumulation and detoxification (Rout and Das, 2009).
Abscisic acid (ABA) is a plant hormone with important functions as a stress alleviator, particularly in responses to drought, salt, and chilling stress (Bari and Jones, 2009). ABA levels in plants are tightly controlled by environmental conditions, and high ABA concentration activates signaling cascades of other phytohormones, such as salicylic acid (SA) and jasmonic acid (JA) (Shi et al., 2015). Exogenous ABA application causes an alleviating effect on plants under heavy metal stresses (Hsu and Kao, 2003; Wang et al., 2013), and a recent study by Shi et al. (2015) suggests that exogenous ABA applications can decrease the phytotoxic effect of Zn in Populus × canescens tissues by modulating the transcriptional activity of key genes involved in Zn transport and detoxification, and by activating the antioxidative defense system.
Since the application of ABA in agricultural practice is limited by ABA’s chemical instability, costly production, and rapid catabolism, a small molecule, ABA mimic (AM1), has been recently identified as an ABA surrogate based on its structural analogy to ABA (Cao et al., 2013). Similar to ABA, AM1 activates multiple members of the ABA receptor family, such as pyrabactin resistance 1 (PYR1) and PYR1-like (PYL) protein, and enhances the tolerance of plants to drought and cold stress (Cao et al., 2013; Cheng et al., 2016). However, compared with ABA, AM1 is easier to synthesize and more resistant to photolysis. Therefore, it has the potential to become an ABA replacement in agricultural practice.
In the present study, the effects of ABA and AM1 in heavy metal stress alleviation were tested by studying the uptake and translocation of Zn in “Merlot” grapevines grown under excess Zn stress. Leaf area, photosynthesis and foliar pigments, Zn localization and concentration, phytohormone level, and expression of Zn-related genes were measured to elucidate the physiological and molecular response underlying the potential mitigating effects of ABA on Zn uptake and on physiology in grapevine, and to explore strategies to mitigate Zn phytotoxicity in vineyards.
Materials and Methods
Plant Cultivation and Treatments
One-year-old hardwood cuttings of “Merlot” (V. vinifera L.) with 4–6 nodes were pre-rooted in a thermostatically controlled heated container (26°C at the base of the cuttings) in a cold room (4°C) for 40 days. The cuttings were then transferred to pots and cultivated for 5 weeks in the Horticulture Greenhouse at The University of British Columbia (26°C day and 20°C night, 16 h photoperiod). Afterward, the rooted seedlings were transferred into 4 L plastic pots filled with clean sand and cultivated for 10 more weeks. The plants were irrigated with 50 mL distilled water or Hoagland solution alternately at each sunset. Thirty-two plants with similar heights were randomly divided into four groups and treated with either basal (0.765 μM Zn2+, 0.22 mg/L) or excess levels (10 mM Zn2+, 2880 mg/L) of zinc sulfate (ZnSO4.7H2O) dissolved in aqueous solution. To 2 of 3 excess Zn treatments, 10 μM ABA or 10 μM AM1 solutions were applied to the roots. This resulted in four treatments: Basal Zn, Excess Zn, Excess Zn + ABA, and Excess Zn + AM1. The treatments were applied in combination with the Hoagland solution every day for 10 days. Eight grapevines per treatment were considered. Four plants per treatment were harvested on the 4th day after the treatment began (DAT); the rest of the plants were harvested on the 10th DAT. Each plant was regarded as a biological replicate, so four biological replicates were included in each treatment.
Leaf Area, Root Length, and Gas Exchange Measurement
For each plant, the lengths of the shoot and of the main vein of each leaf were measured using a ruler at 0, 4, 10 DAT. A regression line between the length of the main vein and the total leaf area was calculated by measuring 50 leaves of different sizes using a ruler and leaf area meter (LI-3100, LI-COR, NE, United States) (Sivilotti et al., 2016). The regression was used to estimate the leaf area of each leaf of the plants in a non-destructive manner, and the total leaf area per plant was calculated by summing the area of each leaf of the plant. The length of lateral roots was also measured at 10 DAT.
Before each sampling point, the leaf gas exchange was determined for each plant. Measurements were conducted from 9:00 am to 1:00 pm. Mature leaves with plastochron 7–9 (Lamoreaux et al., 1978) were selected for the measurement of the net photosynthetic rate (A), the stomatal conductance (gs), and the transpiration rate (E) using a Li-Cor-6400 portable photosynthesis system (Li-COR Inc., NE, United States). The light source from the lamp was set at 1500 μmol/m2/s, air flow through the sample chamber was 500 μmol/s, reference cell CO2 concentration was 400 μmol/mol, and the leaf temperature was 22.0°C.
Sampling of Plant Material
Lateral roots, trunks, and stems between the 5th and 9th nodes from the shoot tip, and leaves between the 1st and 10th node, were harvested at 4 and 10 DAT. Subsamples of fine fresh root, trunk, stem, and leaf were harvested for histochemical analysis. The rest of the samples were frozen in liquid nitrogen and ground into powder using RNase-free mortars and pestles, and then stored at -80°C. This plant material was used for Zn and hormone determination and gene expression analysis. Additional leaves with plastochron 7–9 were collected and used for pigment determination.
Analysis of Foliar Pigment
The leaves were cleaned with distilled water after collection. The veins were removed, and an aliquot of 0.5 g of leaf sample was added into a mortar and ground with 80% acetone. The homogenate was washed out with 80% acetone, filtered into a 10 mL volumetric flask, and then filled to volume. The concentrations of chlorophylls were determined spectrophotometrically as previously described (Wellburn, 1994).
Determination of Zn Concentration
Powder samples, approximately 5 g each, were dried at 60°C for 72 h to calculate the fresh to dry mass ratio. Afterward, 1 g of dried sample was weighed into a tared, oven-dried crucible. The samples were ashed in the muffle furnace for 1 h at 300°C and 4 h at 500°C. After the samples were cooled in a desiccator, 5 mL of 2 M HCl was carefully added. A warm sand bath was used to dissolve soluble constituents, and a tared Waterman #42 paper was used to filter the solution into a 100 mL volumetric flask. Distilled water was used to wash the filter paper and make up to 100 mL of solution. Subsequently, the concentrations of Zn and other mineral elements were determined using a Nu AttoM Inductively Coupled Plasma Mass Spectrometer (ICP-MS) (CAMECA, Gennevilliers, France) as described by Murray et al. (2000). For Zn levels in leaf, the concentrations were measured in the petiole, which has been widely used for diagnosis of Zn in previous research (Alloway, 2004; Romero et al., 2013).
Analysis of Zn Localization
Zn accumulation was histochemically detected in root, trunk, stem, and leaf tissues using the Zn chelating agent dithizone (diphenylthiocarbazone, 30 mg dissolved in 60 ml acetone, 20 ml distilled water and four drops of glacial acetic acid). Hand sections of fresh samples were stained for 2 min and rinsed several times with deionized H2O. Afterward, the sections were immediately analyzed with an Olympus AX-70 light microscope (Olympus Corporation, Tokyo, Japan). The red–purple Zn dithizonate complex was photographed under the light microscope at 10× or 20× magnification using an Olympus Fluoview 1000 scan head connected to a computer (Olympus Corporation, Tokyo, Japan).
Determination of Phytohormone Contents
Chemical Standards
ABA (cat. no. 013 2701) was purchased from OlChemIm Ltd. (Olomouc, Czechia), SA (cat. no. S5922) from Sigma-Aldrich (ON, Canada), and JA (cat. no. 88300) from Cayman Chemical (MI, United States). d6-ABA (cat. no. A110002) was purchased from Toronto Research Chemicals (ON, Canada), d4-SA (cat. no. CS-O-06948) from Clearsynth (ON, Canada), and d5-JA (cat. no. D-6936) from C/D/N Isotopes Inc. (QC, Canada).
Phytohormone Extraction
ABA, SA, and JA were extracted according to the method published by Shi et al. (2015) with minor modifications. An aliquot of 0.4 g of fresh tissue was extracted by 5 mL of 80% (v/v with water) methanol containing 200 mg/L of butylated hydroxytoluene and 500 mg/L of citric acid monohydrate. Aliquots of 100 ng of d6-ABA, d4-SA, and d5-JA each were added to the extraction buffer as internal standards (IS). The samples were shaken for 24 h at 4°C and subsequently centrifuged for 15 min at 10,000 × g under 4°C. The supernatant was collected and transferred into a 20 mL flat-bottom vial using a syringe (Luer-Lok Tip Syringe, Sigma-Aldrich, ON, Canada) and filter (0.22 μm × 13 mm, PVDF Millex Filter, Sigma-Aldrich, ON, Canada). Afterward, the supernatant was concentrated by a Thermo Savant’s Universal Vacuum System (UVS400) (Thermo Fisher Scientific, Waltham, MA, United States) and re-suspended in 250 μL of 80% methanol.
Identification and Quantification of Phytohormones
For the identification and quantification of ABA, SA, JA, and AM1, 5 μL of extract was injected into an Agilent 1100 Series high performance liquid chromatograph (HPLC) coupled to an LC/MSD Trap XCT Plus mass selective detector. Chromatography separation was carried out by an Agilent ZORBAX SB-C18 Column (1.8 μm, 4.6 mm × 50 mm). Mass spectrometry data were generated via electrospray ionization (ESI) in negative modes. The temperature of the column was maintained at 60°C. Mobile phases consisted of Solvent A and B. Solvent A was distilled water with 0.2% formic acid; solvent B was acetonitrile with 0.2% formic acid. The LC separation used a binary solvent gradient with a flow rate of 1.00 mL/min. The gradient conditions were 1.00 min, 10.0% solvent B; 5.00 min, 90.0% solvent B; 6.00 min, 90.0% solvent B; 6.10 min; 5.0% solvent B. Extracted ion chromatography was used for ABA, SA, and JA quantification. Specifically, [MS-H+] 263 was used for ABA, [MS-H+] 269 was used for d6-ABA, [MS-H+] 137 was used for SA, [MS-H+] 141 was used for d4-SA, [MS-H+] 209 was used for JA, [MS-H+] 214 was used for d5-JA. Hormone concentrations were calculated based on internal standard-based calibration curves (Supplementary Table S1) that were prepared for each analyte/IS pair, as described elsewhere (Ross et al., 2004). Samples were run in random order.
RNA Extraction and Analysis of Transcriptional Level by Real-Time PCR
Total RNA extraction and quantitative real-time PCR (qRT-PCR) were performed as described by Savoi et al. (2016). Total RNA was extracted with the “Spectrum Plant total RNA” kit (Sigma-Aldrich, ON, Canada) from 0.1 g of sample powder. The quantity and quality of the RNA were analyzed with an ND-1000 Spectrophotometer NanoDrop (Thermo Fisher Scientific, Wilmington, DE, United States). All RNA samples were digested to remove genomic DNA and reverse-transcribed in a 20 mL reaction mixture for cDNA synthesis using a DNase I, RNase-free, and RevertAid First Strand cDNA Synthesis Kit (Thermo Fisher Scientific, MA, United States), respectively, following the manufacturer’s manuals. The expression levels of 10 Zn-related genes, whose functions will be introduced in discussion, VviZIP2, VviZIP6, VviZIP7, VviZIP13, VviHMA2, VviNAS, VviNRAMP3, VviYSL1, VviPCR2, and VvibZIP23, were determined in root and leaf tissues by using an Applied BiosystemsTM 7500 Fast Dx Real-Time PCR Instrument (Thermo Fisher Scientific, MA, United States) and a PowerUP SYBR Green Master Mix (Thermo Fisher Scientific, TX, United States). The genes were selected according to whole-genome array data organized from publicly available RNA-sequencing experiments in our previous publication (Wong et al., 2018). The primers were designed with PrimerQuest Tool (Integrated DNA Technologies, IA, United States) (Supplementary Table S2), and the annealing temperature was 60°C for all primer pairs except VviUbiquitin, which annealed at 56°C.
Statistical Analysis
Mean and standard deviation of values were calculated using Excel 2016 (Microsoft, Washington, DC, United States). One-way analysis of variance (ANOVA) and two-way ANOVA were conducted using SPSS Statistics 20.0 (IBM, NY, United States). For experimental variables, the principal factors in two-way ANOVA were treatment and time of sampling. Statistical significance among averages was evaluated using the LSD’s test with P < 0.05. Figures were made using the drawing software Origin 9.0 (OriginLab, MA, United States).
Results
Leaf Area Index and Root Length
Shoot length and leaf area were measured at 0, 4, and 10 DAT. No difference of shoot length or leaf area among treatments was observed (Table 1). However, the Excess Zn and Excess Zn + ABA treatments promoted the increase (from 0 DAT to 4 and 10 DAT) of leaf area. As for the roots at 10 DAT, the average length in all the excess Zn treatments decreased compared with the Basal Zn treatment, and no alleviating effect was found for the ABA or AM1 addition treatments.
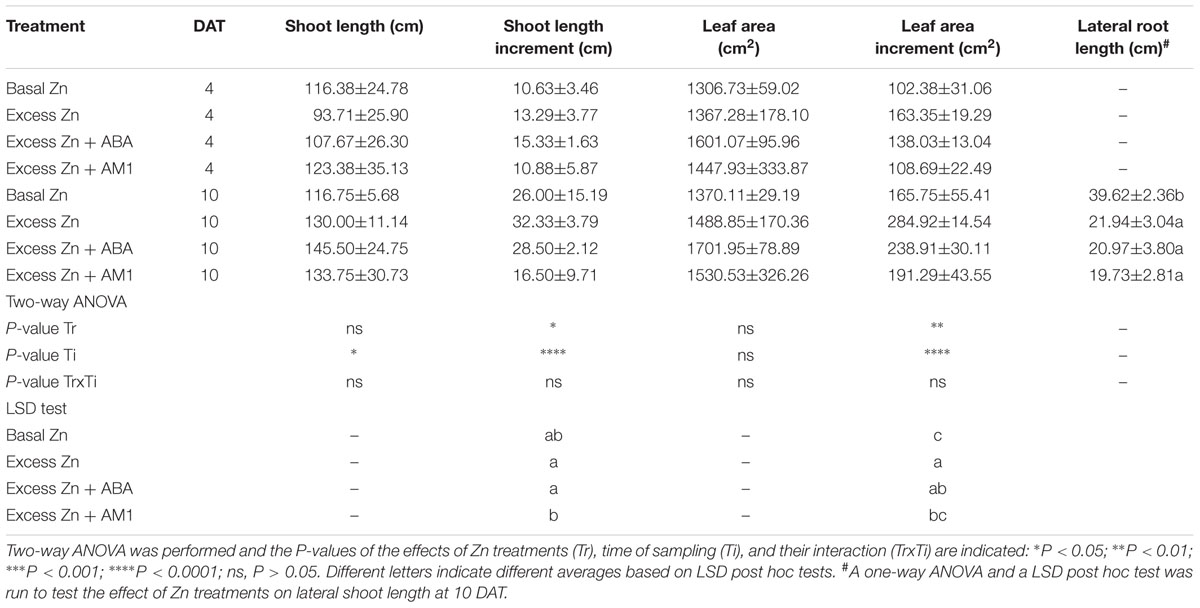
Table 1. Shoot length, shoot length increment from 0 days after the treatment (DAT), leaf area, leaf area increment, and lateral root length (cm) of “Merlot” (Vitis vinifera L.) seedlings exposed to basal and excess Zn in combination with ABA and AM1 (n = 4).
Photosynthesis and Foliar Pigments
Leaf gas exchanges were affected by Zn treatments, but a significant Zn treatment × time of sampling interaction was also observed (Table 2). The CO2 assimilation rate (A) in the three excess Zn treatments was lower than that in the Basal Zn treatment at both 4 and 10 DAT. As for the stomatal conductance (gs) and the transpiration rate (E), the Excess Zn and Excess Zn + ABA treatments had higher gs than Basal Zn at 10 DAT, and the Excess Zn treatment had higher E than the Basal Zn treatment at 10 DAT. The foliar pigments were not affected by treatments.
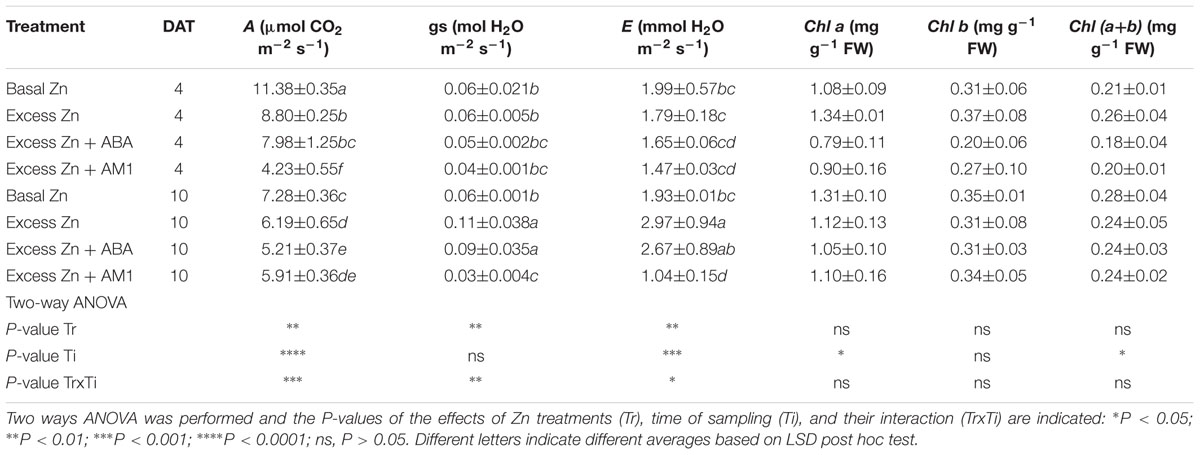
Table 2. Leaf photosynthesis (A), stomatal conductance (gs), transpiration rate (E) and photosynthetic pigments at 4 or 10 days after treatment (DAT) (n = 4).
Zn Concentrations and Localization
Zn concentrations in tissues of “Merlot” seedlings were measured at 4 and 10 DAT (Figure 1). Zn treatments affected Zn concentrations in roots, trunks, and stems, but not in leaves. Major effects were observed at 10 DAT. Overall, excess Zn applications increased the Zn concentration in root, trunk, and stem tissues. In the roots, excess Zn exposure led to an increase of Zn accumulation at 4 DAT, and AM1 addition further promoted Zn uptake. At 10 DAT, the Excess Zn and Excess Zn + AM1 treatments had a sharp increase of Zn concentration; the increase was much less in the Excess Zn + ABA treatment, indicating an alleviating effect of ABA on excess Zn accumulation. In the trunk, no effects were observed in the excess Zn treatments at 4 DAT, but increases of Zn levels were observed in the excess Zn treatments at 10 DAT with no mitigating effect of ABA and with a promoting effect of AM1. In the stems, all the excess Zn treatments increased the Zn concentration, and no effect of the time of sampling or of the interaction between the treatments and the time of sampling was observed.
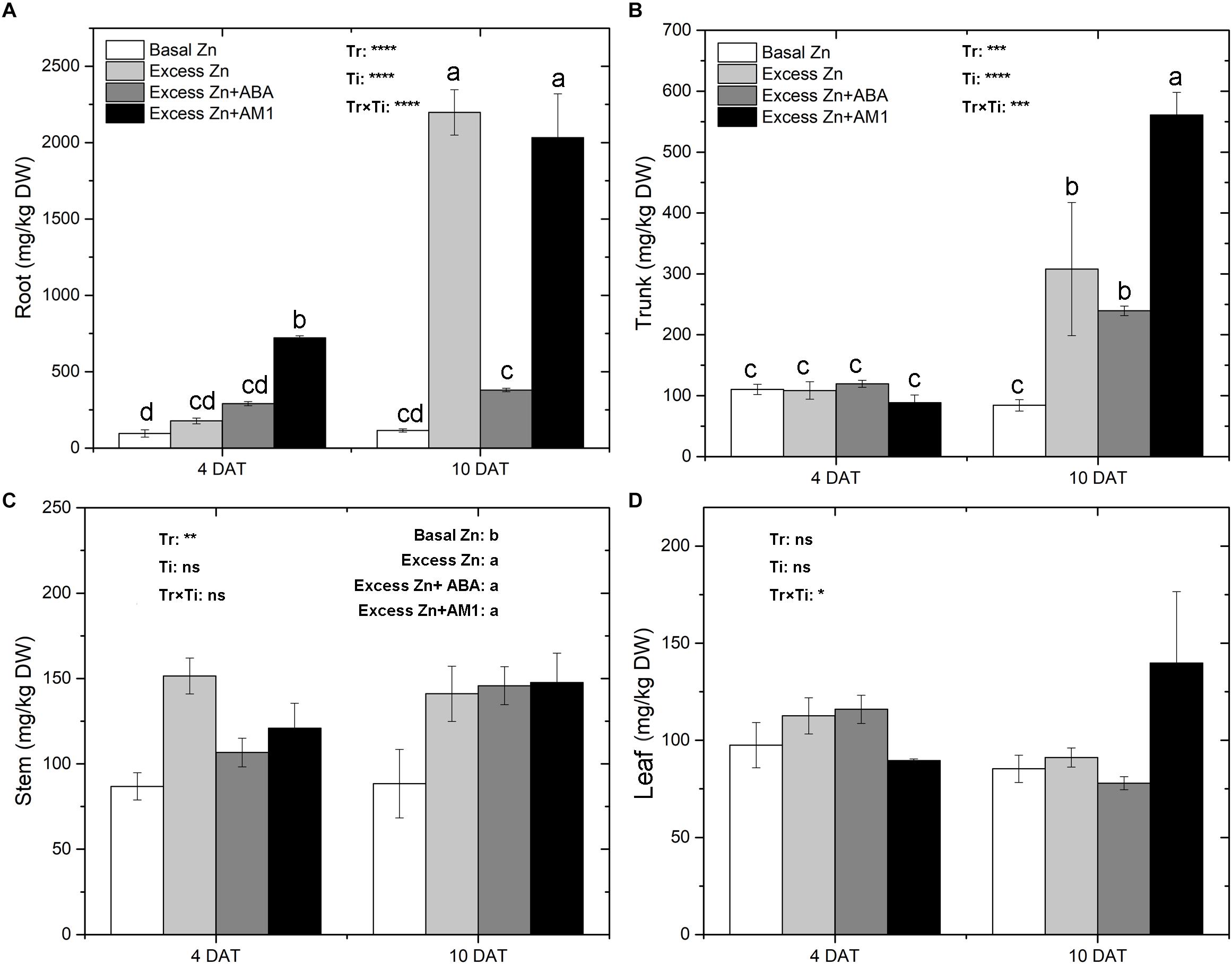
Figure 1. Zn concentrations (mg/kg DW) in root (A), trunk (B), stem (C), and leaf (D) tissues of “Merlot” (Vitis vinifera L.) seedlings exposed to Zn treatments at 4 or 10 days after treatment (DAT) (n = 4). Two-way ANOVA was performed and the P-values of the effects of Zn treatments (Tr), time of sampling (Ti), and their interaction (TrxTi) are indicated: ∗P < 0.05; ∗∗P < 0.01; ∗∗∗P < 0.001; ∗∗∗∗P < 0.0001; ns, P > 0.05. Different letters indicate significantly different averages based on LSD post hoc tests.
Zn-dithizone complexes were observed at 10 DAT in seedling tissues. As shown in Supplementary Figure S1, more Zn accumulated in epidermal cells in roots, pith rays, and phloem in trunks, stems, and petioles in the excess Zn treatments than in the Basal Zn treatment. According to the intensity of red–purple Zn-dithizone precipitates in the tissues, a sharp decline of Zn concentration was observed from lower (roots) to higher (petioles) tissues of the seedlings. Zn accumulation exhibited a similar pattern in the Excess Zn, Excess Zn + ABA, and Excess Zn + AM1 treatments.
Phytohormone and AM1 Concentrations
Excess Zn exposure without ABA addition did not lead to changes of ABA in roots and leaves at 4 DAT but slightly increased ABA in roots at 10 DAT (Figures 2A,B). Excess Zn + ABA remarkably increased ABA in roots at both 4 DAT and 10 DAT, and increased ABA in leaves at 10 DAT; however, interactions between the treatments and the time of sampling were observed. Excess Zn + AM1 promoted ABA in roots at 4 DAT. No effect of excess Zn was observed on SA in roots or leaves (Figures 2C,D). Excess Zn decreased JA in the roots compared with the Basal Zn treatment (Figure 2E). The addition of exogenous ABA and AM1 alleviated the effect of excess Zn. No difference of JA in roots was found among the Excess Zn + ABA, Excess Zn + AM1, and Basal Zn treatments. In the leaves at 4 DAT, the Excess Zn treatment slightly reduced JA compared with the Basal Zn treatment, while the addition of exogenous ABA severely decreased JA compared with the Basal Zn and Excess Zn treatments (Figure 2F). At 10 DAT, Excess Zn + ABA induced the highest JA concentration among treatments.
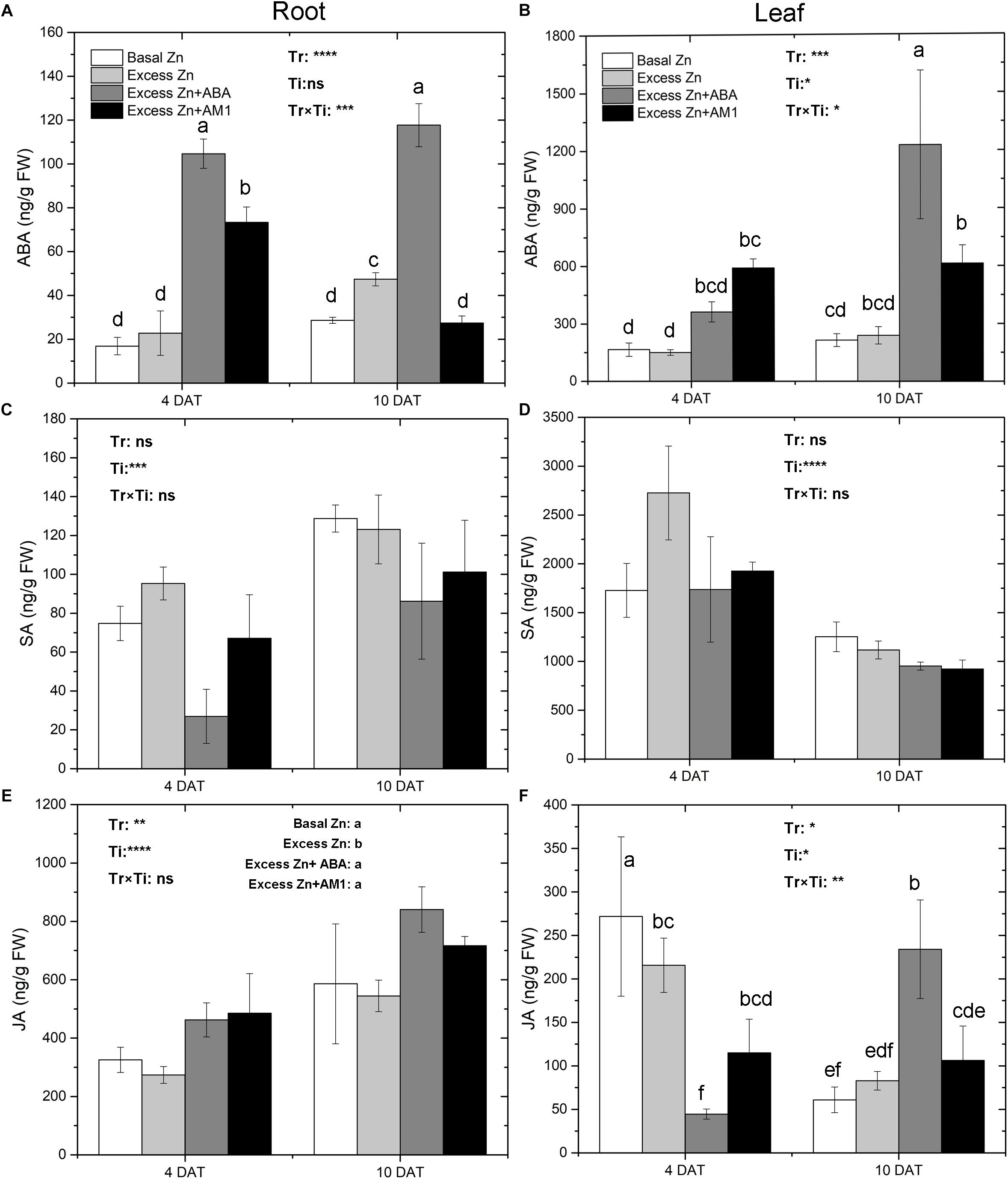
Figure 2. Abscisic acid (ABA), salicylic acid (SA) and jasmonic acid (JA) in the root (A,C,E) and leaf (B,D,F) tissues of “Merlot” (Vitis vinifera L.) seedlings exposed to Zn treatments at 4 or 10 days after treatment (DAT) (n = 4). Two-way ANOVA was performed and the P-values of the effects of Zn treatments (Tr), time of sampling (Ti), and their interaction (TrxTi) are indicated: ∗P < 0.05; ∗∗P < 0.01; ∗∗∗P < 0.001; ∗∗∗∗P < 0.0001; ns, P > 0.05. Different letters indicate significantly different averages based on LSD post hoc tests.
AM1 was detected in roots of plants treated with Excess Zn + AM1, at both 4 DAT and 10 DAT (Supplementary Figure S2), and the concentration at 10 DAT (283.33 μg/g FW) was higher than that at 4 DAT (63.89 μg/g FW). No AM1 was detected in leaf samples at 4 or 10 DAT.
Expression of Genes Involved in Zn Uptake and Translocation
To study the effect of excess Zn and ABA or AM1 addition on the expression of Zn uptake- and translocation-related genes, 10 genes, including the members of the ZIP family of transporters VviZIP2, VviZIP6, VviZIP7, and VviZIP13, heavy metal detoxification-related genes VviHMA2 (Heavy Metal ATPases of the P1B-type ATPases), VviNAS (NA Synthase), VviNRAMP3 (Natural Resistance-Associated Macrophage Protein), VviYSL1 (Yellow Stripe-Like), and VviPCR2 (Plant Cadmium Resistance), as well as the basic-region leucine zipper (bZIP) transcription factor VvibZIP23, were considered. Based on whole-genome array data (Supplementary Table S3 and Figure S3), relative expression of a total of 68 grapevine homologs of the above genes was analyzed across tissues and experimental conditions, and the 10 selected genes were the most expressed among the homologs.
The expression of most genes was notably affected by excess Zn exposure, ABA or AM1 addition, and the sampling time in both roots and leaves (Figure 3). In the roots, excess Zn induced higher expression of all the ZIP genes but VviZIP13 at 4 DAT, as well as some of the heavy metal detoxification-related genes, such as VviHMA2, VviNAS, VvibZIP23, and VviPCR2. VviHMA2 and VviNAS were the most affected by the Excess Zn treatment – seven and five times higher than Basal Zn treatment, respectively. At 10 DAT, most of the above genes were similarly expressed in both the Basal Zn and Excess Zn treatments, but VviZIP2, VviZIP13, and VviHMA2 were lower in expression in the Excess Zn than in the Basal Zn treatment. Excess Zn + ABA generally induced the expression of ZIP genes and detoxification-related genes than in Basal Zn and Excess Zn at 4 DAT. The detoxification genes VviHMA2, VviNAS, and VviPCR2 were the most influenced – 10 times more expressed in Excess Zn + ABA than in Basal Zn treatment. As compared with the Excess Zn treatment, the ABA addition led to a long-lasting induced expression of these genes at 10 DAT. The Zn detoxification gene VviYSL1 was the most induced by Excess Zn + ABA at 10 DAT. Expression of other genes, VviZIP6, VviZIP7, VvibZIP23, VviNRAMP3, and VviPCR2 was also upregulated in the Excess Zn + ABA treatment as compared with the Basal Zn and Excess Zn treatments. Excess Zn + AM1 affected the expression of VviZIP2, VviZIP6, VviZIP13, VvibZIP23, VviYSL1, and VviPCR2 at 4 DAT when compared with Basal Zn. The effect of AM1 addition on the expression of most genes was rarely consistent with the effect of ABA addition.
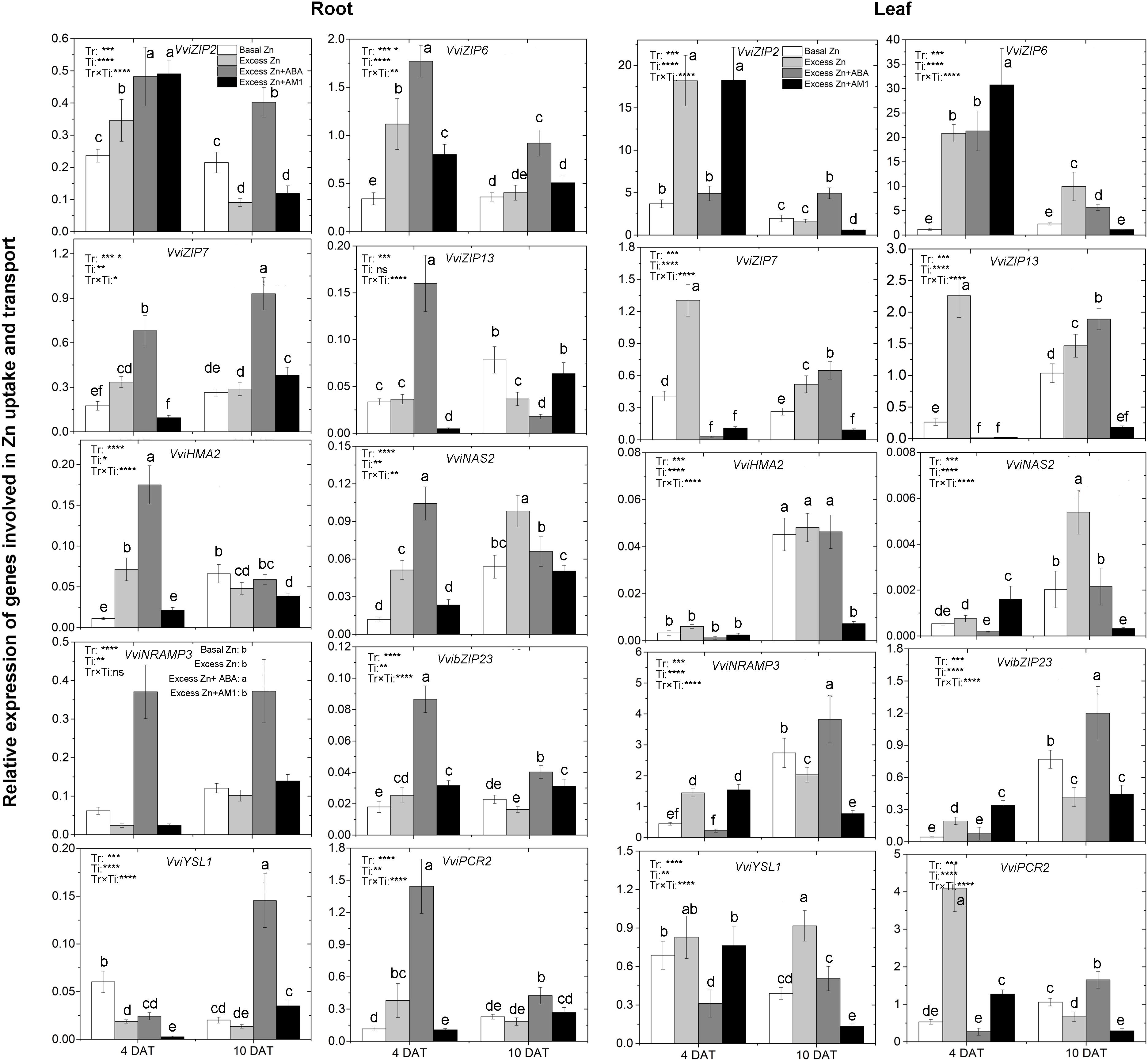
Figure 3. Relative expression of genes involved in Zn uptake and transport in the root (left panels) and leaf (right panels) tissues of “Merlot” (Vitis vinifera L.) seedlings exposed to Zn treatments at 4 or 10 days after treatment (DAT) (n = 4). Two-way ANOVA was performed and the P-values of the effects of Zn treatments (Tr), time of sampling (Ti), and their interaction (TrxTi) are indicated: ∗P < 0.05; ∗∗P < 0.01; ∗∗∗P < 0.001; ∗∗∗∗P < 0.0001; ns, P > 0.05. Different letters indicate significantly different averages based on LSD post hoc tests.
In the leaves, elevated expression of all the ZIP genes, VviNRAM3, and VviPCR2 was found in the Excess Zn treatment at 4 DAT. The Excess Zn + ABA treatment alleviated the increase of expression of VviZIP2, VviZIP7, VViZIP13, VviNRAM3, VvibZIP23, and VviPCR2 observed in Excess Zn at 4 DAT. Moreover, Excess Zn + ABA decreased VviZIP7, VviZIP13, and VviYSL1 expression as compared with the Basal Zn treatment. In the Excess Zn + AM1 treatment, the expression of VviZIP2, VviZIP6, VviNRAM3, VvibZIP23, and VviPCR2 was similar to that in the Excess Zn treatment and higher than that in the Basal Zn treatment at 4 DAT. At 10 DAT, in the Excess Zn treatment, the expression of VviZIP6, VviZIP7, VviZIP13, VviNAS2, and VviYSL1 was higher than in the Basal Zn treatment, while the expression of VviNRAMP3, VvibZIP23, and VviPCR2 was lower than Basal Zn treatment. The expression of most ZIP genes (VviZIP2, VviZIP7, VviZIP13), VviNRAMP3, VvibZIP23, and VviPCR2 in the Excess Zn + ABA treatment was higher than that in the Basal Zn and Excess Zn treatments. Moreover, in the Excess Zn + AM1 treatment, the expression of most genes except for VviZIP6 was down-regulated compared with the Basal Zn treatment at 10 DAT.
Discussion
ABA Alleviates Physiological Stress on: “Merlot” Grapevines Responding to Excess Zn
Reduced growth of roots and stunted shoot growth are general physiological responses to Zn phytotoxicity in plants (Sresty and Rao, 1999; Hirt and Shinozaki, 2004; Richard et al., 2011). In this study, we show that grapevines exposed for 10 days to toxic concentrations of Zn display reduced root length but show no growth defects or visible toxic symptoms in shoots. This result is in accordance with White et al. (1979) and suggests that shoots are more tolerant to long-term Zn exposure than roots.
Though excess Zn exposure did not affect the growth or the total chlorophyll content in grapevine leaves, it was associated with the inhibition of the net photosynthetic rate as previously reported in poplar (Shi et al., 2015) and beans (Tsonev and Cebola-Lidon, 2012). Given that the Mg2+ cofactor can be replaced by Zn2+ in chlorophylls (Wettstein et al., 1995), we hypothesize that the Zn-mediated inhibition of photosynthesis in grapevine is due to the impaired functioning of the electron transport within the light harvesting complexes rather than a reduction in the total content of photosynthetic pigments in the antenna.
There is evidence that with excess Zn exposure, higher proportions of the total plant Zn accumulate in the roots, and the excess Zn mainly accumulates in root cortical cell walls or vacuoles (Robson, 1993b; Bringezu et al., 1999). A higher intensity of zinc-dithizone precipitates was consistently observed in root cortical cells, and a larger increase of Zn concentration in roots as compared with other organs analyzed under excess Zn exposure was observed in grapevine seedlings (Supplementary Figure S1 and Figure 1). In the first 4 days of the excess Zn treatments, Zn levels in trunks, stems and leaves generally remained similar to Zn levels in the Basal Zn treatment, indicating that the roots prevented the upper tissues from accumulating potentially toxic Zn levels by accumulating the excess Zn (Figure 1). At 10 DAT, the higher Zn concentrations in roots, trunks, and stems under excess Zn treatments showed the extension of Zn toxicity from the roots to the aerial parts of grapevine seedlings. The addition of exogenous ABA mitigated the accumulation of Zn in roots. This result is consistent with those reported for poplar (Shi et al., 2015). However, in our study the difference between Excess Zn and Excess Zn + ABA was not significant for upper organs.
Plant hormones play important roles in diverse biotic and abiotic stress responses (Bari and Jones, 2009). Although the complex network of interactions of hormones and heavy metal toxicity is not fully understood, evidence has shown that ABA, SA, and JA are involved in the detoxification of heavy metals in plants (Hirt and Shinozaki, 2004; Clemens, 2006; Bari and Jones, 2009; Shi et al., 2015). Interestingly, the response of endogenous ABA concentrations to excess Zn stress varied among studies, including decrease, no change, and increase of ABA in the plant tissues (Zengin, 2006; Yang et al., 2011; Sofo et al., 2013; Shi et al., 2015). In the present study, ABA concentration was not affected by excess Zn, except in the root at 10 DAT, where it was higher in the Excess Zn than in the Basal Zn treatment (Figures 2A,B). On the other hand, exogenous ABA addition consistently increased ABA in roots and leaves, which coincides with previous research (Hsu and Kao, 2003; Shi et al., 2015). As in previous studies that investigated the mitigating effects of ABA on heavy metal toxicity (Hsu and Kao, 2003, 2005; Shi et al., 2015), exogenous ABA was applied simultaneously with excess Zn, and this continuous feeding of the seedlings with ABA (Excess Zn + ABA) produced a high level of ABA in the tissues considered in comparison with the Basal Zn and Excess Zn treatments. The high ABA levels in the leaves might have affected leaf physiology. However, despite a small but significant reduction in leaf photosynthesis observed in the exposed Zn + ABA treatment when compared with the Excess Zn treatment (Table 2), the other leaf parameters assessed were not affected by ABA addition. Transient applications of ABA have also been shown to alleviate lead stress (Zhao et al., 2009; Wang et al., 2013); hence, it would be worth assessing if transient applications would also alleviate Zn toxicity and for how long the alleviating affect would persist in grapevine.
Salicylic acid is also known as one of the key hormones that regulates plant response to biotic and abiotic stress (Maksymiec, 2007; Bari and Jones, 2009), and it plays a key role in the activation of pathogen-induced systemic acquired resistance (SAR) (Kachroo and Robin, 2013). Although there is evidence that an inverse relationship exists between SA-dependent resistance and JA-dependent resistance (Gupta et al., 2000; Turner et al., 2002), the concentration of SA was generally not affected by the treatments while that of JA was (Figures 2C,E). It is worth noticing that ABA treatment decreased SA in roots at 4 DAT compared with the Excess Zn treatment, even though the difference was only marginally significant (P = 0.07), indicating that in the grapevine roots exposed to excess Zn, SA signaling could be negatively regulated by ABA as reported for the plant immune response (de Torres Zabala et al., 2009; Kim et al., 2011).
Jasmonic acid plays an important role in plant defense. It is commonly biosynthesized in response to several biotic and abiotic stresses, including toxic action of heavy metals. Many studies have shown that heavy metal stress is closely connected with JA signaling (Xiang and Oliver, 1998; Cobbett, 2000; Maksymiec et al., 2005, Maksymiec, 2007; Bari and Jones, 2009), and it is likely that JA mediates heavy metal-induced gene expression. Additionally, exogenous JA could induce a cross-tolerance to several heavy metal stresses (Xiang and Oliver, 1998; Maksymiec and Krupa, 2006). However, a study of the aquatic plant Wolffia arrhiza indicates that exogenous JA acts in a concentration-dependent manner, in which a high concentration of JA enhances heavy metal toxicity (Piotrowska et al., 2009). In this study, the decreased accumulation of JA by excess Zn in roots confirms the role of JA in the adaptation response to heavy metal toxicity (Figure 2E). Furthermore, ABA addition inhibited the decrease of JA concentration in the roots. In addition, it has been reported that JA could protect the photosynthetic apparatus against heavy metal stress (Piotrowska et al., 2009). In our study, Excess Zn + ABA promoted a strong increase of JA concentration in the leaves at 10 DAT; however, this did not mitigate the reduction of photosynthesis determined by excess Zn.
ABA Induced the Expression Level of Zn-Related Genes in “Merlot” Grapevines Responding to Excess Zn
Zn uptake and transport play pivotal roles in Zn homeostasis in plants, and the Zrt and Irt-like protein (ZIP) family is well characterized for its critical role in Zn uptake, transport, and homeostasis (Astudillo et al., 2013). Our analysis showed that VviZIP2, VviZIP6, VviZIP7, and VviZIP13 homologs are highly expressed in different tissues in grapevine (Supplementary Figure S3). The induced expression of VviZIP genes in the Excess Zn treatment supported the fast accumulation of Zn in the roots at 4 DAT. In plants, basic region/leucine zipper motif (bZIP) transcription factors regulate diverse processes including stress signaling (Jakoby et al., 2002) and constitute a group of ABA-response regulators (Hirt and Shinozaki, 2004). bZIP19 and bZIP23 were reported to regulate the uptake of Zn (Assunção et al., 2010). The highly induced expression of VvibZIP23 by ABA addition regardless of treatment time hints at its function as an ABA-response regulator, and it corresponds with the up-regulated expression of ZIP genes in seedlings of the same treatment. The NRAMP family in plants encompasses metal transporters, and transporting ions into the vacuole is one way of reducing toxic metal levels in the cytosol (Hall, 2002). Besides, the transporter NRAMP3 is localized in the vacuolar membrane, and the elevated expression of NRAMP3 in Thlaspi caerulescens was suggested to be necessary for tolerating high Zn concentration (Oomen et al., 2009). In this study, the expression of VviNRAMP3 was consistently up-regulated in response to the Excess Zn + ABA treatment. However, no induction by excess Zn was found. The detoxification function of VviNRAMP3 might be induced by ABA alone. There are different mechanisms of tolerance to excess Zn, and there are various proteins involved in these mechanisms (Rout and Das, 2009; Sinclair and Krämer, 2012), which include the chelation of metals in the cytosol (NAS and YSL), the transport of Zn2+ from the cytoplasm into vacuoles (HMA), and the pumping of Zn2+ out of the cytosol for xylem loading (PCR) (Song et al., 2010; Ueno et al., 2011; Deinlein et al., 2012). The up-regulated expression of these genes by excess Zn in the roots also verified the toxicity of our excess Zn treatments. As for the ABA addition, the stronger and longer-lasting induction of the expression of detoxification genes showed the alleviating effect of exogenous ABA on Zn toxicity in grape seedlings.
Previous research has shown that the activity of antioxidant enzymes highly correlates with Zn levels in plants (Shi et al., 2015), and exogenous ABA causes increased activities of antioxidative enzymes (SOD, APX, CAT, and POD) in plants, relieving the oxidative stress caused by heavy metals (Zhao et al., 2009; Wang et al., 2013). These mechanisms and enzymes might also be involved in the response of grapevines to excess Zn.
Root Application of AM1 Does Not Alleviate Excess Zn Stress in “Merlot” Grapevines
AM1 was not effective in mitigating Zn uptake. AM1 has been reported as a promising analog of ABA due to its ability to activate ABA receptors. Although, like ABA, AM1 could enhance the tolerance of plants to drought and cold stress, we did find contrasting effects between AM1 and ABA treatments in response to excess Zn in grapevine. Various factors could have led to this inconsistency. First, the exogenous ABA was possibly taken up by the roots and transported into upper organs. On the contrary, seedlings only accumulated AM1 in roots, and there was no evidence of transport of AM1 to other tissues. This possibly contributed to limiting its effects on Zn absorbance and transportation. Second, AM1 is an agonist only for a selective subset of ABA receptors (Cao et al., 2013). Moreover, AM1 is less potent than ABA in binding to certain ABA receptors due to their structural differences. Recently, a series of AM1 fluorine derivatives (AMFs) were verified to be more potent ABA analogs (Cao et al., 2017). Testing the effect of these AMFs on alleviating excess Zn stress in grapevines will provide useful knowledge for their potential application in agriculture.
Conclusion
Excess Zn exposure led to shorter lateral roots, decreased photosynthesis, fast and high uptake and accumulation of Zn in roots, trunks and stems, decreased concentration of the heavy metal-related endogenous hormone JA, and induced expression of Zn transporter- and detoxification-related genes. Exogenous ABA addition mitigated the uptake and accumulation of Zn and led to higher induced expression of both VviZIP genes and detoxification-related genes. These results demonstrate that exogenous ABA could enhance the tolerance of grapevine to excess Zn, likely by regulating the expression of genes involved in Zn uptake and detoxification. As a heavy metal mitigating strategy, treatments with AM1 could not effectively alleviate the accumulation of Zn. Future studies should test if the observed responses are consistent across genotypes and in grafted grapevines.
Author Contributions
CS and YY performed the experiments. ZZ and SC provided all financial support and critical intellectual input in the design of the study. CS completed the manuscript in collaboration with SC and AR. All authors read and approved the final version of the manuscript.
Funding
This work was supported by the China Agriculture Research System for Grape Industry (CARS-29-zp-06) and the National Sciences and Engineering Research Council (NSERC) Discovery Program (10R23082). The China Scholarship Council (CSC) supported the international cooperation of this work.
Conflict of Interest Statement
The authors declare that the research was conducted in the absence of any commercial or financial relationships that could be construed as a potential conflict of interest.
Acknowledgments
The authors thank Dr. Leslie Lavkulich for helping with the Zn analysis as well as the China Scholarship Council (CSC) and the NSERC for financially supporting the project.
Supplementary Material
The Supplementary Material for this article can be found online at: https://www.frontiersin.org/articles/10.3389/fpls.2019.00872/full#supplementary-material
FIGURE S1 | Zn localization in root, trunk, stem, and petiole of “Merlot” (Vitis vinifera L.) seedlings exposed to Basal, Excess Zn, and Excess Zn with ABA and AM1 additions by dithizone staining at 10 days after treatment. Well stained samples with zinc-dithizone precipitates (red–purple), arrows point to Zn-dithizone precipitates.
FIGURE S2 | ABA mimic 1 (AM1) in the root and leaf tissues of “Merlot” (Vitis vinifera L.) seedlings in Excess Zn + AM1 treatment at 4 or 10 days after treatment (n = 4). Different letters indicate significantly different averages based on LSD post hoc tests.
FIGURE S3 | Relative expression of genes (Zrt and Irt-like protein, ZIP; Heavy Metal ATPases of the P1B-type ATPases, HMA; NA Synthase, NAS; Natural Resistance-Associated Macrophage Protein, NRAMP; Yellow Stripe-Like, YSL; Plant Cadmium Resistance, PCR; basic-region leucine zipper transcription factor, bZIP) involved in Zn uptake and transport in different tissues according to whole-genome array data from 14 publicly available experiments. VIT_03s0017g02170, VviZIP2; VIT_06s0004g05070, VviZIP6; VIT_06s0004g06940, VviZIP7; VIT_19s0015g00190, VviZIP13; VIT_11s0103g00370, VviHMA2; VIT_14s0060g01190, VviNAS2; VIT_07s0129g00620, VviNRAMP3; VIT_13s0158g00380, VvibZIP23; VIT_02s0025g02500, VviYSL1; VIT_01s0011g05470, VviPCR2.
TABLE S1 | Calibration curves for quantification of phytohormones.
TABLE S2 | Primer list of tested genes involved in Zn uptake and transport in of “Merlot” (Vitis vinifera L.) seedlings.
TABLE S3 | Relative expression of 68 grapevine homologous genes across tissues and experimental conditions.
References
Alloway, B. J. (2004). “Symptoms of zinc deficiency in agricultural and horticultural crops,” in Zinc in Soils and Crop Nutrition, ed. L. Pletinck (Brussels: International Zinc Association), 59–70.
Alloway, B. J. (ed.) (2012). “Key heavy metals and metalloids,” in Heavy Metals in Soils: Trace Metals and Metalloids in Soils and their Bioavailability, (Berlin: Springer), 465–496.
Anderson, K., and Aryal, N. R. (2015). Which Winegrape Varieties are Grown Where? A Global Empirical Picture. Adelaide: University of Adelaide Press.
Anderson, K., and Nelgen, S. (2015). Global Wine Markets, 1961 to 2009: a Statistical Compendium. Adelaide: University of Adelaide Press.
Assunção, A. G., Herrero, E., Lin, Y.-F., Huettel, B., Talukdar, S., Smaczniak, C., et al. (2010). Arabidopsis thaliana transcription factors bZIP19 and bZIP23 regulate the adaptation to zinc deficiency. Proc. Natl. Acad. Sci. U.S.A. 107, 10296–10301. doi: 10.1073/pnas.1004788107
Astudillo, C., Fernandez, A. C., Blair, M. W., and Cichy, K. A. (2013). The Phaseolus vulgaris ZIP gene family: identification, characterization, mapping, and gene expression. Front. Plant Sci. 4:286. doi: 10.3389/fpls.2013.00286
Baccio, D. D., Tognetti, R., Sebastiani, L., and Vitagliano, C. (2003). Responses of Populusdeltoides x Populus nigra (Populus x euramericana) clone I-214 to high zinc concentrations. New Phytologist. 159, 443–452. doi: 10.1046/j.1469-8137.2003.00818.x
Bari, R., and Jones, J. D. (2009). Role of plant hormones in plant defence responses. Plant Mol. Biol. 69, 473–488. doi: 10.1007/s11103-008-9435-0
Bringezu, K., Lichtenberger, O., Leopold, I., and Neumann, D. (1999). Heavy metal tolerance of Silene vulgaris. J. Plant Physiol. 154, 536–546. doi: 10.1016/S0176-1617(99)80295-8
Cao, M., Liu, X., Zhang, Y., Xue, X., Zhou, X. E., Melcher, K., et al. (2013). An ABA-mimicking ligand that reduces water loss and promotes drought resistance in plants. Cell Res. 23, 1043–1054. doi: 10.1038/cr.2013.95
Cao, M. J., Zhang, Y.-L., Liu, X., Huang, H., Zhou, X. E., Wang, W.-L., et al. (2017). Combining chemical and genetic approaches to increase drought resistance in plants. Nat. Commun. 8:1183. doi: 10.1038/s41467-017-01239-3
Cheng, Z., Jin, R., Cao, M., Liu, X., and Chan, Z. (2016). Exogenous application of ABA mimic 1 (AM1) improves cold stress tolerance in bermudagrass (Cynodon dactylon). Plant Cell Tiss. Organ Cult. 125, 231–240. doi: 10.1007/s11240-016-0941-5
Clemens, S. (2006). Toxic metal accumulation, responses to exposure and mechanisms of tolerance in plants. Biochimie 88, 1707–1719. doi: 10.1016/j.biochi.2006.07.003
Cobbett, C. S. (2000). Phytochelatin biosynthesis and function in heavy-metal detoxification. Curr. Opin. Plant Biol. 3, 211–216. doi: 10.1016/s1369-5266(00)00066-2
de Torres Zabala, M., Bennett, M. H., Truman, W. H., and Grant, M. R. (2009). Antagonism between salicylic and abscisic acid reflects early host–pathogen conflict and moulds plant defence responses. Plant J. 59, 375–386. doi: 10.1111/j.1365-313x.2009.03875.x
Deinlein, U., Weber, M., Schmidt, H., Rensch, S., Trampczynska, A., Hansen, T. H., et al. (2012). Elevated nicotianamine levels in Arabidopsis halleri roots play a key role in zinc hyperaccumulation. Plant Cell 24, 708–723. doi: 10.1105/tpc.111.095000
dos Santos Lima, M., Silani, I. D. S., Toaldo, I. M., Corrêa, L. C., Biasoto, A. C. T., Pereira, G. E., et al. (2014). Phenolic compounds, organic acids and antioxidant activity of grape juices produced from new Brazilian varieties planted in the Northeast Region of Brazil. Food Chem. 161, 94–103. doi: 10.1016/j.foodchem.2014.03.109
Gupta, V., Willits, M. G., and Glazebrook, J. (2000). Arabidopsis thaliana EDS4 contributes to salicylic acid (SA)-dependent expression of defense responses: evidence for inhibition of jasmonic acid signaling by SA. Mol. Plant Microbe Interact. 13, 503–511. doi: 10.1094/mpmi.2000.13.5.503
Hafeez, B., Khanif, Y. M., and Saleem, M. (2013). Role of zinc in plant nutrition- a review. Am. J. Exp. Agric. 3, 374–391. doi: 10.9734/ajea/2013/2746
Hall, J. (2002). Cellular mechanisms for heavy metal detoxification and tolerance. J. Exp. Bot. 53, 1–11. doi: 10.1093/jexbot/53.366.1
Hirt, H., and Shinozaki, K. (2004). Plant Responses to Abiotic Stress. Berlin: Springer Science & Business Media.
Hsu, Y., and Kao, C. (2003). Role of abscisic acid in cadmium tolerance of rice (Oryza sativa L.) seedlings. Plant Cell Environ. 26, 867–874. doi: 10.1046/j.1365-3040.2003.01018.x
Hsu, Y. T., and Kao, C. H. (2005). Abscisic acid accumulation and cadmium tolerance in rice seedlings. Physiol. Plant. 124, 71–80. doi: 10.1111/j.1399-3054.2005.00490.x
Hussain, S., Maqsood, M. A., and Rahmatullah, M. (2010). Increasing grain zinc and yield of wheat for the developing world: a review. Emir. J. Food Agric. 22, 326–339. doi: 10.1016/j.jtemb.2009.05.002
Intarapichet, K. O., Woraratphoka, J., and Indrapichate, K. (2007). Phenolic compounds and antioxidative properties of selected wines from the northeast of Thailand. Food Chem. 104, 1485–1490. doi: 10.1016/j.foodchem.2007.02.020
Jakoby, M., Weisshaar, B., Dröge-Laser, W., Vicente-Carbajosa, J., Tiedemann, J., Kroj, T., et al. (2002). bZIP transcription factors in Arabidopsis. Trends Plant Sci. 7, 106–111. doi: 10.1016/s1360-1385(01)02223-3
Jogaiah, S., Oulkar, D. P., Vijapure, A. N., Maske, S. R., Sharma, A. K., and Somkuwar, R. G. (2013). Influence of canopy management practices on fruit composition of wine grape cultivars grown in semi-arid tropical region of India. Afr. J. Agric. Res. 8, 3462–3472. doi: 10.5897/ajar2013.7307
Kachroo, A., and Robin, G. P. (2013). Systemic signaling during plant defense. Curr. Opin. Plant Biol. 16, 527–533. doi: 10.1016/j.pbi.2013.06.019
Kim, T.-H., Hauser, F., Ha, T., Xue, S., Böhmer, M., Nishimura, N., et al. (2011). Chemical genetics reveals negative regulation of abscisic acid signaling by a plant immune response pathway. Curr. Biol. 21, 990–997. doi: 10.1016/j.cub.2011.04.045
Lamoreaux, R. J., Chaney, W. R., and Brown, K. M. (1978). The plastochron index: a review after two decades of use. Am. J. Bot. 65, 586–593. doi: 10.1002/j.1537-2197.1978.tb06113.x
Maksymiec, W. (2007). Signaling responses in plants to heavy metal stress. Acta Physiol. Plant. 29:177. doi: 10.1007/s11738-007-0036-3
Maksymiec, W., and Krupa, Z. (2006). The effects of short-term exposition to Cd, excess Cu ions and jasmonate on oxidative stress appearing in Arabidopsis thaliana. Environ. Exp. Bot. 57, 187–194. doi: 10.1016/j.envexpbot.2005.05.006
Maksymiec, W., Wianowska, D., Dawidowicz, A. L., Radkiewicz, S., Mardarowicz, M., and Krupa, Z. (2005). The level of jasmonic acid in Arabidopsis thaliana and Phaseolus coccineus plants under heavy metal stress. J. Plant Physiol. 162, 1338–1346. doi: 10.1016/j.jplph.2005.01.013
Murray, R., Miller, D. J., and Kryc, K. (2000). Analysis of major and trace elements in rocks, sediments, and interstitial waters by inductively coupled plasma–atomic emission spectrometry (ICP-AES). ODP Tech. 29, 1–27. doi: 10.2973/odp.tn.29.2000
Oomen, R. J., Wu, J., Lelièvre, F., Blanchet, S., Richaud, P., Barbier-Brygoo, H., et al. (2009). Functional characterization of NRAMP3 and NRAMP4 from the metal hyperaccumulator Thlaspi caerulescens. New Phytol. 181, 637–650. doi: 10.1111/j.1469-8137.2008.02694.x
Piotrowska, A., Bajguz, A., Godlewska-Żyłkiewicz, B., Czerpak, R., and Kamiǹska, M. (2009). Jasmonic acid as modulator of lead toxicity in aquatic plant Wolffia arrhiza (Lemnaceae). Environ. Exp. Bot. 66, 507–513. doi: 10.1016/j.envexpbot.2009.03.019
Richard, O., Pineau, C., Loubet, S., Chalies, C., Vile, D., Marques, L., et al. (2011). Diversity analysis of the response to Zn within the Arabidopsis thaliana species revealed a low contribution of Zn translocation to Zn tolerance and a new role for Zn in lateral root development. Plant Cell Environ. 34, 1065–1078. doi: 10.1111/j.1365-3040.2011.02305.x
Robson, A. D. (1993a). “Zinc phytotoxicity,” in Zinc in Soils and Plants (Dordrecht: Kluwer Academic Publishers), 135–144.
Robson, A. D. (1993b). “Distribution and transport of zinc in plants,” in Zinc in Soils and Plants (Dordrecht: Kluwer Academic Publishers), 79–89.
Romero, I., García-Escudero, E., and Martín, I. (2013). Leaf blade versus petiole analysis for nutritional diagnosis of Vitis vinifera L. cv. Tempranillo. Am. J. Enol. Vitic. 64, 50–64. doi: 10.5344/ajev.2012.11004
Ross, A. R., Ambrose, S. J., Cutler, A. J., Feurtado, J. A., Kermode, A. R., Nelson, K., et al. (2004). Determination of endogenous and supplied deuterated abscisic acid in plant tissues by high-performance liquid chromatography-electrospray ionization tandem mass spectrometry with multiple reaction monitoring. Anal. Biochem. 329, 324–333. doi: 10.1016/s0003-2697(04)00177-0
Rout, G. R., and Das, P. (2009). “Effect of metal toxicity on plant growth and metabolism: I. Zinc,” in Sustainable Agriculture, eds E. Lichtfouse, M. Navarrete, P. Debaeke, S. Véronique, and C. Alberola (Dordrecht: Springer), 873–884. doi: 10.1007/978-90-481-2666-8_53
Savoi, S., Wong, D. C., Arapitsas, P., Miculan, M., Bucchetti, B., Peterlunger, E., et al. (2016). Transcriptome and metabolite profiling reveals that prolonged drought modulates the phenylpropanoid and terpenoid pathway in white grapes (Vitis vinifera L.). BMC Plant Biol. 16:67. doi: 10.1186/s12870-016-0760-1
Shi, W. G., Li, H., Liu, T. X., Polle, A., Peng, C. H., and Luo, Z. B. (2015). Exogenous abscisic acid alleviates zinc uptake and accumulation in Populus × canescens exposed to excess zinc. Plant Cell Environ. 38, 207–223. doi: 10.1111/pce.12434
Sinclair, S. A., and Krämer, U. (2012). The zinc homeostasis network of land plants. Biochim. Biophys. Acta 1823, 1553–1567. doi: 10.1016/j.bbamcr.2012.05.016
Sivilotti, P., Herrera, J. C., Lisjak, K., Česnik, H. B., Sabbatini, P., Peterlunger, E., et al. (2016). Impact of leaf removal, applied before and after flowering, on anthocyanin, tannin, and methoxypyrazine concentrations in ‘Merlot’ (Vitis vinifera L.) grapes and wines. J. Agric. Food Chem. 64, 4487–4496. doi: 10.1021/acs.jafc.6b01013
Sofo, A., Vitti, A., Nuzzaci, M., Tataranni, G., Scopa, A., Vangronsveld, J., et al. (2013). Correlation between hormonal homeostasis and morphogenic responses in Arabidopsis thaliana seedlings growing in a Cd/Cu/Zn multi-pollution context. Physiol. Plant. 149, 487–498. doi: 10.1111/ppl.12050
Song, W.-Y., Choi, K. S., Geisler, M., Park, J., Vincenzetti, V., Schellenberg, M., et al. (2010). Arabidopsis PCR2 is a zinc exporter involved in both zinc extrusion and long-distance zinc transport. Plant Cell 22, 2237–2252. doi: 10.1105/tpc.109.070185
Sresty, T., and Rao, K. M. (1999). Ultrastructural alterations in response to zinc and nickel stress in the root cells of pigeonpea. Environ. Exp. Bot. 41, 3–13. doi: 10.1016/s0098-8472(98)00034-3
Terra, M. F., Prado, G., Pereira, G. E., Ematné, H. J., and Batista, L. R. (2013). Detection of ochratoxin A in tropical wine and grape juice from Brazil. J. Sci. Food Agric. 93, 890–894. doi: 10.1002/jsfa.5817
Tsonev, T., and Cebola-Lidon, F. J. (2012). Zinc in plants - an overview. Emir. J. Food Agric. 24, 322–333.
Turner, J. G., Ellis, C., and Devoto, A. (2002). The jasmonate signal pathway. Plant Cell 14(Suppl. 1), S153–S164.
Ueno, D., Milner, M. J., Yamaji, N., Yokosho, K., Koyama, E., Clemencia Zambrano, M., et al. (2011). Elevated expression of TcHMA3 plays a key role in the extreme Cd tolerance in a Cd-hyperaccumulating ecotype of Thlaspi caerulescens. Plant J. 66, 852–862. doi: 10.1111/j.1365-313X.2011.04548.x
Wang, J., Chen, J., and Pan, K. (2013). Effect of exogenous abscisic acid on the level of antioxidants in Atractylodes macrocephala Koidz under lead stress. Environ. Sci. Pollut. Res. 20, 1441–1449. doi: 10.1007/s11356-012-1048-0
Wellburn, A. R. (1994). The spectral determination of chlorophylls a and b, as well as total carotenoids, using various solvents with spectrophotometers of different resolution. J. Plant Physiol. 144, 307–313. doi: 10.1016/s0176-1617(11)81192-2
Wettstein, D. V., Gough, S., and Kannangara, C. G. (1995). Chlorophyll biosynthesis. Plant Cell 7, 1039–1057. doi: 10.1105/tpc.7.7.1039
White, M., Chaney, R., and Decker, A. (1979). Role of roots and shoots of soybeans in tolerance to excess soil zinc. Crop Sci. 19, 126–128.
Wong, D. C. J., Zhang, L., Merlin, I., Castellarin, S. D., and Gambetta, G. A. (2018). Structure and transcriptional regulation of the major intrinsic protein gene family in grapevine. BMC Genomics 19:248. doi: 10.1186/s12864-018-4638-5
Xiang, C., and Oliver, D. J. (1998). Glutathione metabolic genes coordinately respond to heavy metals and jasmonic acid in Arabidopsis. Plant Cell 10, 1539–1550. doi: 10.1105/tpc.10.9.1539
Yang, Y., Sun, C., Yao, Y., Zhang, Y., and Achal, V. (2011). Growth and physiological responses of grape (Vitis vinifera “Combier”) to excess zinc. Acta Physiol. Plant. 33, 1483–1491. doi: 10.1007/s11738-010-0687-3
Zengin, F. K. (2006). The effects of Co2+ and Zn2+ on the contents of protein, abscisic acid, proline and chlorophyll in bean (Phaseolus vulgaris cv. Strike) seedlings. J. Environ. Biol. 27, 441–448.
Keywords: abscisic acid, ABA mimic, excess Zn stress, grape, heavy metal toxicity
Citation: Song C, Yan Y, Rosado A, Zhang Z and Castellarin SD (2019) ABA Alleviates Uptake and Accumulation of Zinc in Grapevine (Vitis vinifera L.) by Inducing Expression of ZIP and Detoxification-Related Genes. Front. Plant Sci. 10:872. doi: 10.3389/fpls.2019.00872
Received: 10 January 2019; Accepted: 18 June 2019;
Published: 05 July 2019.
Edited by:
Rosa M. Rivero, Spanish National Research Council (CSIC), SpainReviewed by:
Ruben Bottini, CONICET Mendoza, ArgentinaSergio Ruffo Roberto, State University of Londrina, Brazil
Lingfei Shangguan, Nanjing Agricultural University, China
Rongrong Guo, Guangxi Academy of Agricultural Sciences, China
Copyright © 2019 Song, Yan, Rosado, Zhang and Castellarin. This is an open-access article distributed under the terms of the Creative Commons Attribution License (CC BY). The use, distribution or reproduction in other forums is permitted, provided the original author(s) and the copyright owner(s) are credited and that the original publication in this journal is cited, in accordance with accepted academic practice. No use, distribution or reproduction is permitted which does not comply with these terms.
*Correspondence: Zhenwen Zhang, emhhbmd6aHc2MEBud3N1YWYuZWR1LmNu; Simone Diego, c2ltb25lLmNhc3RlbGxhcmluQHViYy5jYQ==">Castellarinc2ltb25lLmNhc3RlbGxhcmluQHViYy5jYQ==; c2RjYXN0ZWxAbWFpbC51YmMuY2E=