- 1Laboratory of Photobiology, Małopolska Centre of Biotechnology, Jagiellonian University, Krakow, Poland
- 2Department of Plant Biotechnology, Faculty of Biochemistry, Biophysics and Biotechnology, Jagiellonian University, Krakow, Poland
- 3Institute of Biochemistry and Biophysics, Polish Academy of Sciences, Warsaw, Poland
We examined the impact of UV-B irradiation on chloroplast movements in Arabidopsis leaves. Directional chloroplast movements induced by blue light have been described in multiple plant species. In weak light, chloroplasts accumulate at periclinal cell walls to increase light capture. In strong light, chloroplasts exhibit the avoidance response, as they move towards anticlinal walls to protect the photosynthetic apparatus from light-induced damage. In Arabidopsis, chloroplast movements are triggered by phototropins, phot1 and phot2, which are known as blue/UV-A photoreceptors. We found that irradiation with UV-B of 3.3 µmol·m−2·s−1 induced chloroplast accumulation in wild-type plants. UV-B-triggered accumulation was dependent on the presence of phototropins, especially phot1, but not on UVR8 (the canonical UV-B photoreceptor). Irradiation with strong UV-B of 20 µmol·m−2·s−1 did not induce substantial chloroplast relocations in wild-type leaves. However, in the jac1 mutant, which is defective in chloroplast accumulation, strong UV-B elicited chloroplast avoidance. This indicated that UV-B can also activate signaling to the avoidance response. To assess the possibility of indirect effects of UV-B on chloroplast movements, we examined the impact of UV-B on the actin cytoskeleton, which serves as the motile system for chloroplast movements. While irradiation with UV-B of 3.3 µmol·m−2·s−1 did not affect the actin cytoskeleton, strong UV-B disrupted its structure as shown using an Arabidopsis line expressing Lifeact-green fluorescent protein (GFP). In wild-type plants, pretreatment with strong UV-B attenuated chloroplast responses triggered by subsequent blue light irradiation, further indicating that this UV-B intensity also indirectly affects chloroplast movements. Taken together, our results suggest that the effect of UV-B on chloroplast movement is twofold: it directly induces phototropin-mediated movements; however, at higher intensities, it attenuates the movements in a nonspecific manner.
Introduction
Apart from visible light, the solar spectrum contains ultraviolet radiation, by convention divided into the UV-A (315–400 nm), UV-B (280–315 nm), and UV-C range (200–280 nm). As UV-C is absorbed by the ozone layer, only UV-A and UV-B reach the Earth’s surface (Aphalo et al., 2012). UV-B is an important environmental cue for plants (Robson et al., 2019), but strong UV-B causes damage to cellular constituents (Schuch et al., 2017). The main plant photoreceptor for UV-B is UVR8 (Jenkins, 2017). The UVR8 absorption spectrum exhibits a band between 260 and 300 nm, with a maximum at 282 nm and a shoulder at 290 nm (Yang et al., 2015). UVR8 is a dimeric protein. Upon UV-B exposure, it monomerizes (Rizzini et al., 2011) and moves from the cytoplasm to the nucleus (Yin et al., 2016). It is involved in protection against damage caused by excessive UV-B radiation through regulation of gene expression related to flavonoid biosynthesis, antioxidant activities, and DNA repair (Yin and Ulm, 2017). UVR8 participates also in UV-B-induced phototropic bending. However, its activity in this reaction is repressed in wild-type (WT) plants. The main receptors for UV-B-induced phototropism are phototropins (Vandenbussche et al., 2014; Vanhaelewyn et al., 2016).
Phototropins are known as blue/UV-A light photoreceptors, which control responses aimed at improving photosynthetic efficiency in plants. Two phototropins, phot1 and phot2, are found in Arabidopsis thaliana. They share highly redundant functions. Both control phototropism, stomata opening, chloroplast movements, leaf expansion, and positioning (Christie et al., 2015). In reactions controlled by both phototropins, phot1 is more light sensitive than phot2 (Sakai et al., 2001; Harada and Shimazaki, 2007). Phototropin expression is regulated by light. In mature Arabidopsis leaves, PHOT1 mRNA levels are reduced after blue and red light treatments, in contrast to PHOT2 mRNA levels, which are elevated (Łabuz et al., 2012). Blue light decreases the level of phototropin1 protein (Sakamoto and Briggs, 2002); however, the expression of phototropin2 at the protein level does not change under similar conditions (Kong et al., 2006). Phot2 is continuously degraded and re-synthesized in darkness. Blue light also causes its degradation, but at a slower rate (Aggarwal et al., 2014). The phototropin molecule consists of two parts, an N-terminal photosensory part with two light, oxygen, and voltage (LOV)-regulated domains and a C-terminal serine/threonine kinase domain. Each LOV domain contains a flavin mononucleotide (FMN) chromophore. Absorption spectra of LOV domains feature a peak in UV-A, around 370–380 nm. In the blue part of the spectrum, the absorption maximum of LOV domains is around 445–449 nm (Salomon et al., 2000; Christie et al., 2002; Kasahara et al., 2002b). Pure FMN in solution is characterized by absorbance maxima in the UV range at 220, 266, 370 nm and in the visible range at 450 nm (Copeland and Spiro, 1986). Light absorption leads to conformational changes in the LOV2 domain that result in the activation of the phototropin kinase domain (Tokutomi et al., 2008). Phototropins are considered as blue/UV-A photoreceptors; however, only recently has it been shown that they mediate UV-B-induced phototropism in etiolated Arabidopsis seedlings, in a manner dependent on their kinase activity and NPH3 (NONPHOTOTROPIC HYPOCOTYL3) dephosphorylation (Vanhaelewyn et al., 2016).
Blue light induces directional movements of chloroplasts in plants. In a few species of green algae, mosses, and ferns [e.g., Mougeotia scalaris (Suetsugu et al., 2005b), Physcomitrella patens (Kadota et al., 2000), and Adiantum capillus-veneris (Kawai et al., 2003)], also red light is known to trigger chloroplast relocations. Blue light signaling to chloroplast movements depends on phototropins (Banaś et al., 2012). In weak light, chloroplasts gather at the cell walls perpendicular to the direction of incident light, leading to a decrease in total leaf transmittance. This reaction, called the accumulation response, improves photosynthetic efficiency (Zurzycki, 1955; Gotoh et al., 2018). Chloroplast accumulation is controlled redundantly by both phototropins in Arabidopsis. In WT Arabidopsis, chloroplasts accumulate at blue light intensities between ∼0.08 and 20 μmol·m−2·s−1. In the phot2 mutant, in which only phot1 is active, chloroplasts accumulate at any intensity greater than ∼0.08 μmol·m−2·s−1 of blue light. In the phot1 mutant, in which only phot2 is active, chloroplast accumulation occurs in the range of 2–20 μmol·m−2·s−1 of blue light (Jarillo et al., 2001; Kagawa et al., 2001; Sakai et al., 2001). In strong blue light, chloroplasts position at cell walls parallel to the direction of incident light. This avoidance response results in an increase of leaf transmittance. It protects chloroplasts against photodamage in stress conditions (Kasahara et al., 2002a; Sztatelman et al., 2010). In WT plants, sustained chloroplast avoidance, controlled by phototropin2, occurs at light intensities higher than 20 μmol·m−2·s−1 of blue light (Sakai et al., 2001). In the phot2 mutant, only small, transient chloroplast avoidance is observed (Luesse et al., 2010), (Sztatelman et al., 2016). In land plants, chloroplast movements rely predominantly on actin filaments (Kandasamy and Meagher, 1999; Krzeszowiec et al., 2007), in particular cp-actin (chloroplast-actin) (Kadota et al., 2009; Yamashita et al., 2011; Tsuboi and Wada, 2012).
Chloroplast movements induced by the UV radiation were investigated only in a few species of non-vascular plants and aquatic monocots. UV-B induces chloroplast clumping in the aquatic monocot Halophila stipulacea (Sharon et al., 2011) and abolishes chloroplast rhythmical movements in the green alga Ulva petrosa (Han et al., 1998). The impact of UV on directional chloroplast movements was investigated in the aquatic monocot Lemna trisulca and the moss Funaria hygrometrica. In Lemna, UV irradiation containing the UV-C range induced chloroplast accumulation at low intensities and inhibited the movements at higher intensities (Zurzycki, 1962). In Funaria, the action spectrum of chloroplast movements from the dark position to the walls perpendicular to the direction of radiation featured three maxima, around 266, 366, and 454 nm. The peak at 266 nm was the greatest (Zurzycki, 1967).
In this work, we show that in the model plant A. thaliana, UV-B induces chloroplast movements in a phototropin-dependent manner. UVR8 is not involved in this response. Irradiation with strong UV-B disrupts the actin cytoskeleton architecture in the epidermal and palisade cells of Arabidopsis leaves. In line with this, pretreatment of WT leaves with strong UV-B reduces the magnitude of chloroplast responses to subsequent illumination with blue light.
Materials and Methods
Plants and Growth Conditions
WT Col-0 Arabidopsis, phot1: SALK_088841 (Lehmann et al., 2011), uvr8-6: SALK_033468 (Favory et al., 2009), jac1-3: WiscDsLox457-460P9 plants were purchased from Nottingham Arabidopsis Stock Centre. The phot2: npl1-1 mutant (Jarillo et al., 2001) was gifted by Jose Jarillo (Madrid, Spain). Seeds of Arabidopsis expressing Lifeact–green fluorescent protein (GFP) (Smertenko et al., 2010) were a gift from Tim Hawkins (Durham, United Kingdom). The phot1phot2 double mutant was selected from crosses (SALK_088841 with npl1-1) (see Supplementary Figure 1 for genotyping). The jac1-3 mutant (WiscDsLox457-460P9) was selected in this study (see Supplementary Figure 2 for line characteristics). Seeds were sown in Jiffy-7 pots (Jiffy Products International AS) and vernalized at 4°C for 2 days. Plants were transferred to a growth chamber (Sanyo MLR 350H) at 23°C, 80% relative humidity, with a photoperiod of 10-h light and 14-h darkness, at 70 μmol·m−2·s−1 of light supplied by fluorescent lamps (FL40SS.W/37, Sanyo, Japan).
UV-B and Light Treatments
In experiments on chloroplast movements and the actin cytoskeleton structure, leaves were detached from 4- to 5-week-old plants, dark adapted for at least 16 h. The upper side of leaves was irradiated with UV-B of 3.3 (1.3 W·m−2) or 20 µmol·m−2·s−1 (7.9 W·m−2) for 1 h, supplied by USHIO UV-B G8T5E fluorescent tubes. UV-B was filtered through UG-11 (Knight Optical, UK) and ZUS0325 (Asahi Spectra Co, Japan) filters, as well as two layers of cellulose acetate foil (95 µm thick, Rachow Kunststoff-Folien, Germany) (the filtered spectrum shown in Supplementary Figure 3A). In the experiments with blue light irradiation (455 nm, LED, LXHL-PR09, Ledium Ltd., Hungary), the intensities of 3.3, 20, or 120 µmol·m−2·s−1 were used (the spectrum shown in Supplementary Figure 3B). Control leaves were given a mock irradiation (kept in the irradiation chamber but covered with aluminum foil). Changes of leaf transmittance at 660 nm, shown in Figures 1 and 2, were measured before and immediately after irradiation, with a custom-made photometer (Walczak and Gabrys, 1980). For expression studies, dark-adapted plants were irradiated with UV of 0.2 µmol·m−2·s−1 for 3 h through WG305 cut-off filters in a growth chamber equipped with USHIO UV-B Lamps G8T5E as in Banaś et al. (2018).
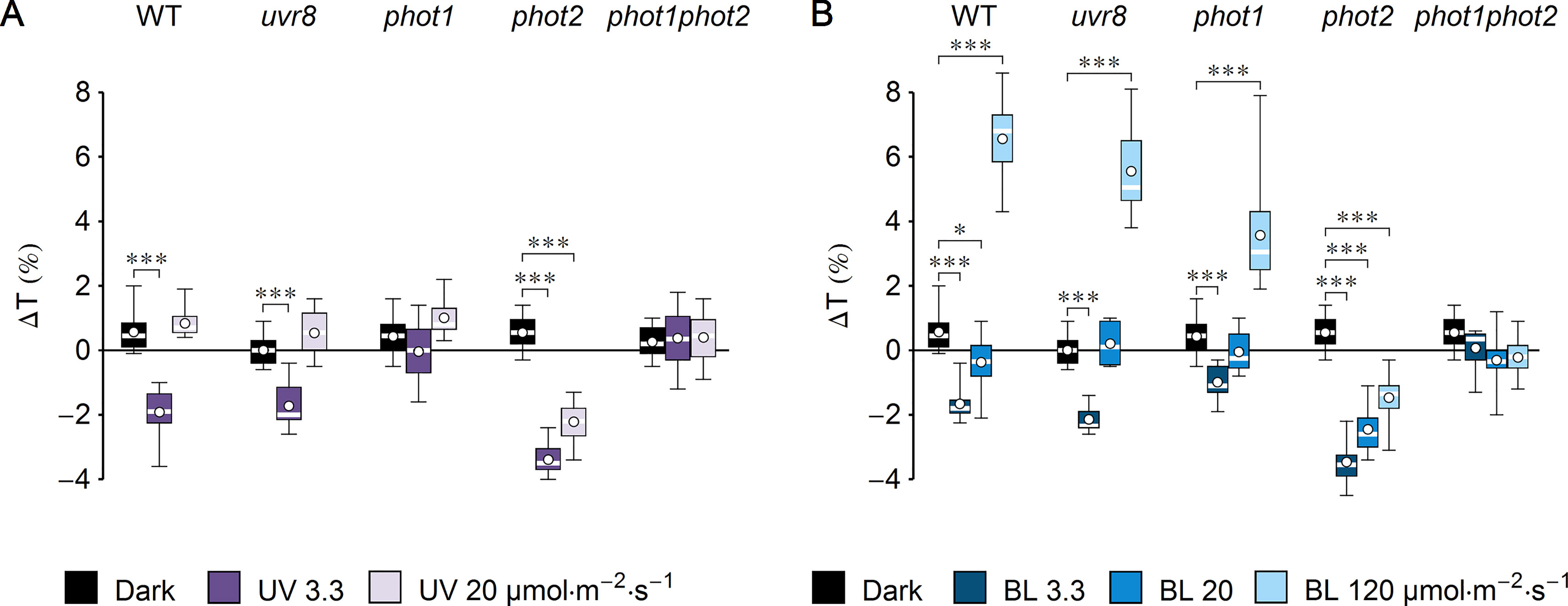
Figure 1 Effect of UV-B and blue light (BL) on leaf transmittance in dark-adapted Arabidopsis leaves of wild-type (WT), uvr8, phot1, phot2, and phot1phot2 mutant plants. (A) Leaves were irradiated for 1 h with UV-B (violet boxes) of 3.3 or 20 µmol·m−2·s−1 or kept in darkness (black boxes). (B) Leaves were irradiated for 1 h with blue light (blue boxes) of 3.3 or 20 or 120 µmol·m−2·s−1 or kept in darkness (black boxes). The difference ΔT between leaf transmittance after and before the treatment was measured for red light (660 nm). Plots in (A) and (B) share the dark controls. The leaves irradiated with UV-B and those irradiated with blue light were taken from the same batch of plants. Asterisks indicate statistically significant differences between means for WT and mutant lines (adjusted p values: *0.01 < p < 0.05, ***p < 0.001). Each box represents 12 measurements.
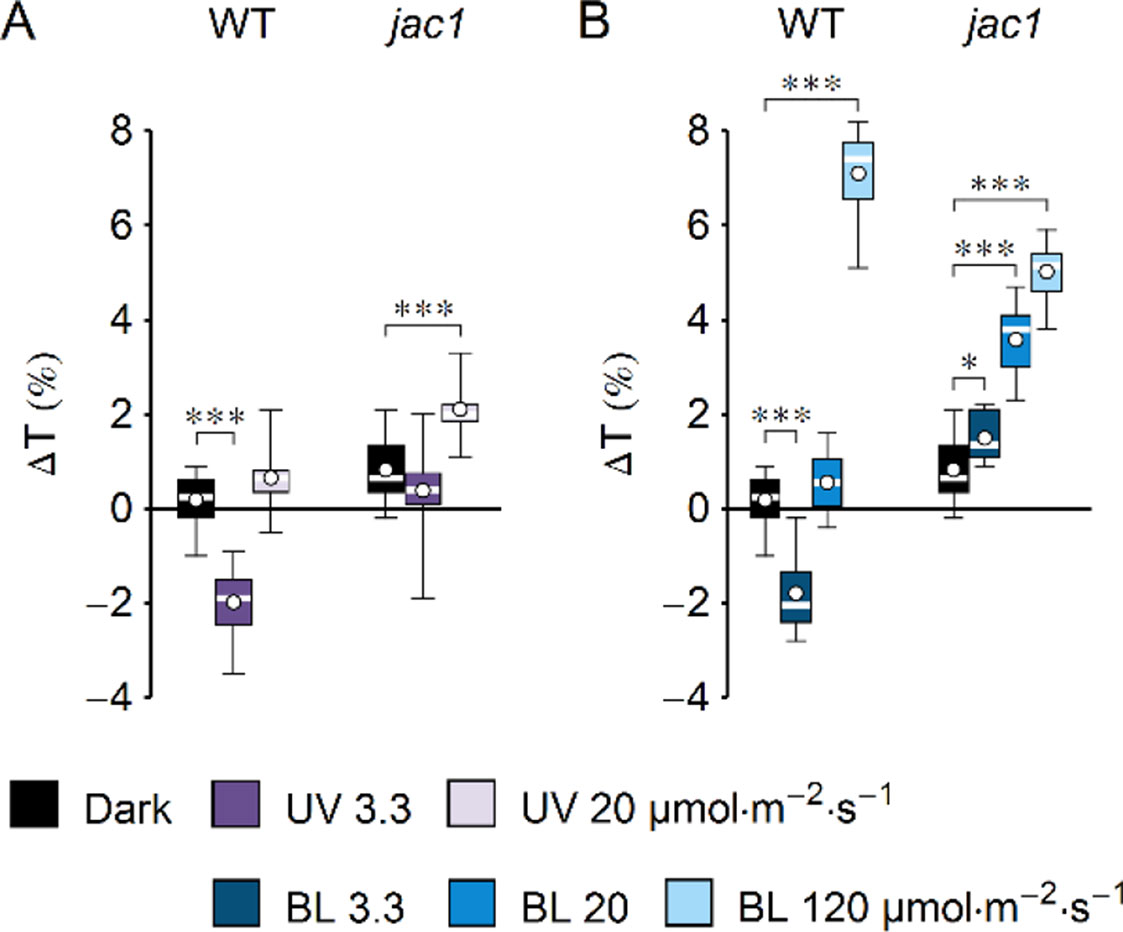
Figure 2 Effect of UV-B and blue light (BL) on leaf transmittance in dark-adapted Arabidopsis leaves of wild-type (WT) and jac1 mutant plants. (A) Leaves were irradiated for 1 h with UV-B (violet boxes) of 3.3 or 20 µmol·m−2·s−1 or kept in darkness (black boxes). (B) Leaves were irradiated for 1 h with blue light (blue boxes) of 3.3 or 20 or 120 µmol·m−2·s−1 or kept in darkness (black boxes). The difference ΔT between leaf transmittance after and before the treatment was measured for red light (660 nm). Plots in (A) and (B) share the dark controls. The leaves irradiated with UV-B and those irradiated with blue light were taken from the same batch of plants. Asterisks indicate statistically significant differences between means for WT and jac1 (adjusted p values: *0.01 < p < 0.05, ***p < 0.001). Each box represents 12 measurements.
Photometric Method
Chloroplast movements triggered by blue light were assessed using the photometric method according to Gabryś et al. (2017), which relies on measurements of changes in leaf transmittance. Chloroplast responses to continuous blue light (Luxeon Star Royal Blue LXHL-FR5C LED, 460 nm) of 1.6 or 120 μmol·m−2·s−1 were measured in detached leaves of overnight dark-adapted 4- to 5-week-old plants. Amplitudes and velocities of chloroplast responses after light treatments were calculated based on the photometric curves, using a custom-written Mathematica (Wolfram Research, US) package.
Confocal Microscopy
Microscopic observations were performed with the Axio Observer.Z1 inverted microscope (Carl Zeiss, Jena, Germany) and the LSM 880 confocal module. Plan-Neofluar 40×, NA 1.3, objective was used with oil immersion. The actin cytoskeleton was observed in the upper epidermis and the upper parts of palisade cells in 4- to 5-week-old Lifeact-GFP rosette leaves. The Ar laser line of 488 nm was used to excite GFP and chlorophyll. The emission in the range of 494–597 nm was recorded as the green channel. The emission in the range of 647–721 nm was recorded as the magenta channel. Chloroplast positioning was observed in the palisade parenchyma of WT Arabidopsis leaves as well as uvr8, phot1, phot2, phot1phot2, and jac1 mutants. The 633-nm He–Ne laser was used to excite chlorophyll, and the emission in the range of 647–721 nm was recorded as the magenta channel.
To examine the effect of UV-B on chloroplast positioning, stacks were collected on the upper surface of WT and mutant leaves, with the interval between slices set to 1.06 μm. Projection images were calculated from slices corresponding to the first 40 μm of stacks, starting from the upper surface of the epidermis. This range included the epidermis and upper parts of palisade cells. Images were then segmented using Otsu’s thresholding in ImageJ. The fraction of the image area occupied by chloroplasts was used as a measure of chloroplast relocation in response to irradiation. To examine the effect of UV-B on the actin cytoskeleton, Z-stacks were recorded on the upper epidermis of WT Arabidopsis leaves. The interval between stack slices was 1.06 μm. Maximum intensity projections were calculated from the slices spanning ∼30 μm, starting from the upper epidermis (to visualize pavement cells), or from slices spanning ∼10 μm, starting from the top of the palisade cells. Prior to quantification of the images of the epidermis, the sliding paraboloid method was used to subtract the background from the projection images, using ImageJ. Contrast was enhanced by histogram equalization. Actin fibers were marked in the images using the ridge detection method (Steger, 1998), implemented in an ImageJ plugin (Wagner et al., 2017). The areas occupied by guard cells and the contours of cells visible in the projection images were marked manually and excluded from the analysis.
Determination of Phototropin Expression
Phototropin expression at mRNA and protein levels was examined in leaves of Arabidopsis WT and uvr8 mutant plants in at least five biological replicates. Each sample contained leaves from two plants frozen in liquid nitrogen immediately after treatment. RNA isolation and real-time polymerase chain reaction (PCR) were performed as described in Łabuz et al. (2012), with the exception of the oligodT primers used for RNA reverse transcription. Primer sequences can be found in Łabuz et al. (2012) (for PHOT1 and PHOT2) and in Czechowski et al. (2005) (for reference genes UBC, PDF2, and SAND). The relative expression of each gene in a sample was determined in three technical replicates. The mean value of Ct for samples from all experimental groups quantified simultaneously was subtracted from individual Ct values. Expression levels were then normalized using factors calculated with geNorm v3.4 (Vandesompele et al., 2002). Protein extraction was performed according to (Sakamoto and Briggs, 2002). Samples were homogenized and weighed to adjust to equal mass; 7.5% polyacrylamide gels were used for sodium dodecyl sulfate–polyacrylamide gel electrophoresis (SDS-PAGE) followed by a semi-dry transfer (Biorad). Western blot was performed as in Sztatelman et al. (2016). Anti-PHOT1 (AS10 720) and anti-PHOT2 (AS10 721) antibodies were prepared by Agrisera (see Łabuz et al., 2015). Anti-PHOT2 antibodies were used at a dilution of 1:5,000, and anti-PHOT1 antibodies at a dilution of 1:300 (a purified fraction). Secondary antibodies (goat anti-rabbit horseradish peroxidase (HRP)-conjugated IgG, Agrisera) were applied at a dilution of 1:25,000. Signal detection was performed with a Clarity Western ECL Blotting Substrate (Bio-Rad), using the BioSpectrum Imaging System (UVP Ultra-Violet Products Ltd). Intensities of the chemiluminescent signal were normalized to actin levels in each sample. Membranes were stripped with Restore Plus Western Blot Stripping Buffer (Thermo Scientific) and probed with an anti-actin antibody (AS132640, Agrisera) at a dilution of 1:2,500 at room temperature for 1 h, followed by secondary antibody incubation and enhanced chemiluminescence (ECL) detection. Densitometric quantification was performed with ImageJ.
Statistical Analysis
Statistical calculations were performed using the R software. The mRNA and protein levels were log-transformed before statistical analysis; other measurements were not transformed. Significance of the effects of the plant line and irradiation was assessed with two-way ANOVAs. As the interaction terms were significant, the pairwise comparisons between means of groups were performed, using the glht command of the multcomp package. To account for unequal variances between groups, the sandwich package was used. For data shown in Figures 1, 2, and 7, as well as in Supplementary Figures 7 and 8, the significance of the differences of means between the control group (mock-irradiated or dark samples) and the irradiated samples was assessed for each plant line. For data in Supplementary Figure 1D, the differences between WT and phot1phot2, as well as between glabra1 and phot1phot2glabra1, were tested. For data in Supplementary Figures 2B, C, the differences between plant lines (WT vs jac1) were tested for each irradiation condition. The p values reported with the asterisks are adjusted for multiple comparison using the Holm method. All comparisons shown in a single plot were treated as a family of comparisons for the purpose of the adjustment of p values.
A different procedure was used for the statistical analysis of the results of quantification of the actin cytoskeleton (shown in Supplementary Figure 5). In each repetition of this experiment, three similar leaves were chosen for dark control and for UV irradiation. Thus, pairs of observations can be distinguished. The means obtained for the control leaves were compared with those calculated for the UV-irradiated samples, using the paired sample t test in the R software. The Bonferroni correction was used to adjust the p values.
In all box plots, the central rectangle marks the range between the first and third quartiles, the central band is the median, and the ends of whiskers represent the lowest and greatest values. Means are marked with white circles.
Results
To investigate the impact of UV-B on chloroplast movements in the model plant Arabidopsis thaliana, detached leaves of dark-adapted plants were irradiated for 1 h with UV-B of 3.3 µmol·m−2·s−1 (1.3 W·m−2) or strong UV-B of 20 µmol·m−2·s−1 (7.9 W·m−2). Control leaves were subjected to mock irradiation (darkness). Leaf transmittance was measured before and after mock/UV-B treatment with the photometric method (Figure 1A). The difference of transmittance ΔT was used to assess chloroplast relocations quantitatively. To identify the photoreceptor responsible for the observed effects, a set of mutants was used: uvr8, phot1, phot2, and phot1phot2. A substantial (∼2%) decrease of transmittance, indicative of chloroplast accumulation, was observed in the WT and uvr8 leaves irradiated for 1 h with UV-B of 3.3 µmol·m−2·s−1. An even greater (3.4%) decrease in transmittance was recorded for the phot2 mutant. No substantial changes of leaf transmittance were observed in the phot1 and phot1phot2 mutants. This suggests that UV-B irradiation did not induce chloroplast accumulation in those lines. To check the dose dependency of UV-B responses, Arabidopsis leaves were treated with higher UV-B intensity of 20 µmol·m−2·s−1 for 1 h. In WT, uvr8, phot1, and phot1phot2 plants, the effect of UV-B irradiation on leaf transmittance was not statistically different from the effect of mock irradiation. Only in the phot2 mutant was a larger (2%) decrease of transmittance observed. To compare the magnitude of chloroplast responses with different wavelengths, equimolar blue light (455 nm) was used (Figure 1B). Leaf transmittance was measured in dark-adapted WT plants and photoreceptor mutants treated with blue light of 3.3, 20, and 120 µmol·m−2·s−1, which elicits full chloroplast avoidance. In WT and uvr8 mutant plants, treatment with blue light of 3.3 µmol·m−2·s−1 caused a 2% decrease in transmittance, which is consistent with chloroplast accumulation. The phot1 mutant showed only a 1% decrease in transmittance, whereas the change in the phot2 mutant was the largest, over 3%. Blue light of 20 µmol·m−2·s−1 did not induce substantial changes in transmittance of WT and uvr8, phot1, and phot1phot2 mutant leaves. Only in in the phot2 mutant was a large (3%) decrease of transmittance observed. Blue light of 120 µmol·m−2·s−1, which saturates chloroplast avoidance, triggered an increase in leaf transmittance in WT plants, as well as in uvr8 and phot1 mutants (∼6%, 5%, and 3%, respectively). In the phot2 mutant, this blue light intensity induced a 1.5% decrease of transmittance. In the phot1phot2 mutant, there were no statistically significant differences between the effects of blue light treatments and of mock irradiation.
UV-B intensity of 20 µmol·m−2·s−1 is much higher than the intensities occurring in field conditions, yet it is insufficient to induce chloroplast avoidance in WT Arabidopsis plants. This observation could be explained in two ways: strong UV-B is not capable of producing the signal to chloroplast avoidance, or it generates both signals to accumulation and avoidance, but the one leading to accumulation is prevailing. To distinguish between those scenarios, changes of leaf transmittance were investigated in the jac1 mutant. JAC1 (J-DOMAIN PROTEIN REQUIRED FOR CHLOROPLAST ACCUMULATION RESPONSE 1) is required for signaling to chloroplast accumulation; thus, jac1 shows chloroplast avoidance even at the blue light intensity of 1.6 µmol·m−2·s−1, which elicits chloroplast accumulation in WT plants (Suetsugu et al., 2005a) (Supplementary Figures 2B–E). In the jac1 mutant, UV-B of 3.3 µmol·m−2·s−1 did not induce changes in leaf transmittance; however, UV-B treatment of 20 µmol·m−2·s−1 caused a 2% increase (Figure 2A). This result suggests that UV-B can trigger chloroplast avoidance, which can be observed in mutant lines defective in signaling to chloroplast accumulation. In WT plants, signaling to avoidance is masked by the prevailing accumulation response. Irradiation with blue light elicited an increase in the transmittance of jac1 leaves. The magnitude of this increase was proportional to the light intensity (Figure 2B).
To confirm that transmittance changes resulted from chloroplast movements, chloroplast positioning was further examined under the confocal microscope in palisade mesophyll cells using chlorophyll autofluorescence (Figure 3). After UV-B treatment of 3.3 µmol·m−2·s−1, chloroplasts localized at the top periclinal cell walls of WT palisade cells. This relocation was not observed in leaves irradiated with UV-B of 20 µmol·m−2·s−1. The uvr8 mutant showed similar chloroplast arrangements as WT under all experimental treatments. In the phot1 mutant, no substantial relocations were observed in response to UV-B of 3.3 or 20 µmol·m−2·s−1. The phot2 mutant exhibits altered dark positioning of chloroplasts (Suetsugu et al., 2005a), which form characteristic rows under the top periclinal cell walls of palisade cells (Figure 3). UV-B of both intensities triggered dispersion of chloroplast clusters beneath the periclinal walls. The distance between chloroplasts increased, so that they became evenly distributed. In phot1phot2 plants, chloroplasts formed clusters beneath the top periclinal walls, and no substantial differences were observed between dark and UV-B treated leaves. The dark positioning of chloroplasts in palisade cells of the jac1 mutant differed from the positioning in WT plants. In jac1, chloroplasts gathered almost exclusively at the anticlinal cell walls. UV-B treatment did not trigger chloroplast rearrangements in the upper parts of palisade cells in the jac1 mutant (Figure 3). To relate microscopic observations with the results obtained by the photometric method, the area occupied by chloroplasts in the projection images was quantified (Supplementary Figure 4). An increase in the area occupied by chloroplasts, corresponding to chloroplast accumulation recorded with the photometric method, was observed in UV-B-irradiated WT, uvr8, and phot2 leaves. The dark positioning of chloroplasts was altered in jac1, phot2, and phot1phot2 mutants.
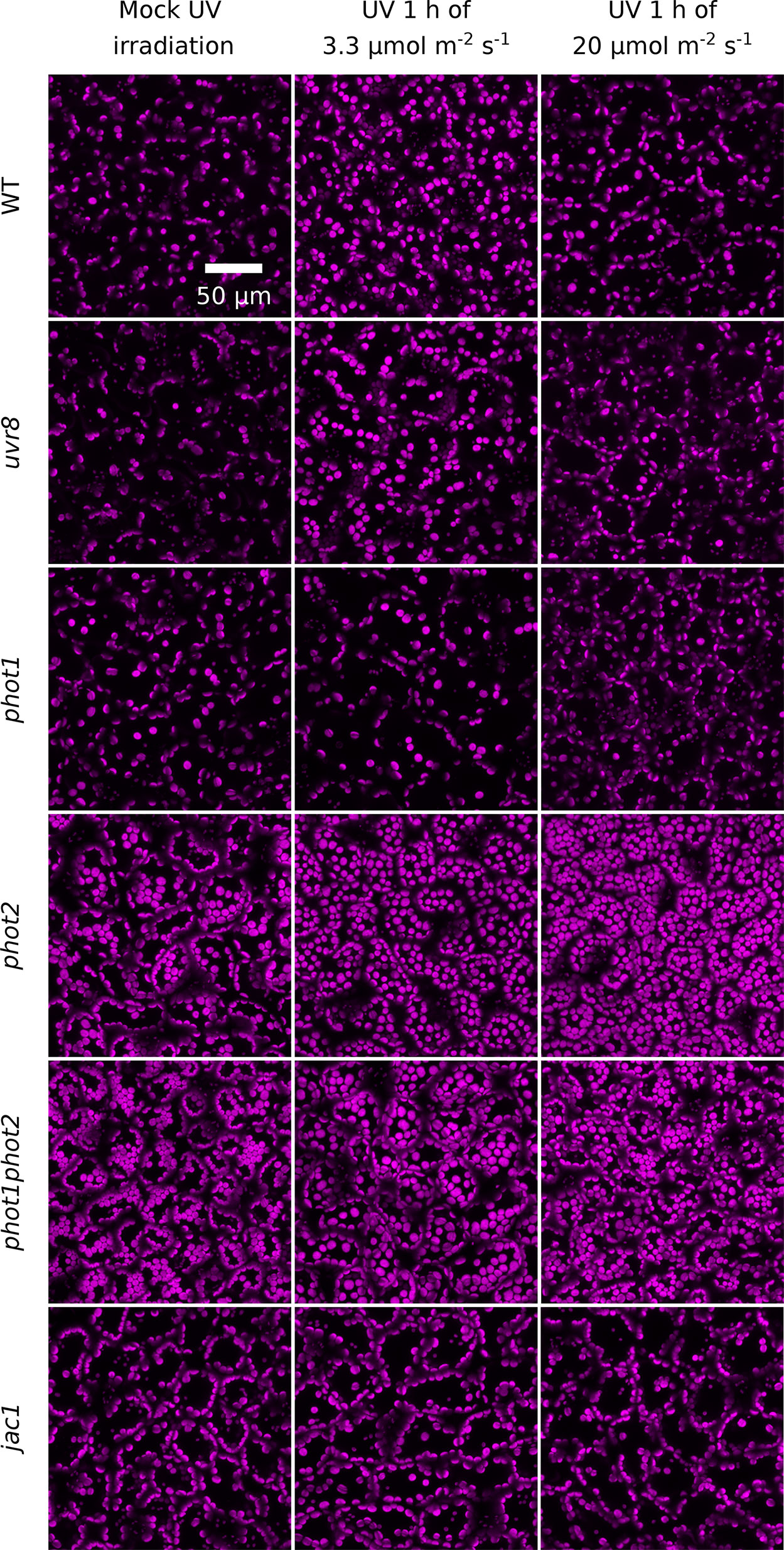
Figure 3 UV-B-induced chloroplast movements in palisade cells of Arabidopsis leaves of WT, uvr8, phot1, phot2, phot1phot2, and jac1 mutant plants. For 1 h, leaves were kept in darkness (mock irradiation) or irradiated with UV-B (280–320 nm) of 3.3 or 20 µmol·m−2·s−1. Chloroplast arrangements were then imaged with a confocal microscope, using chlorophyll autofluorescence (in magenta). Maximum intensity projections were calculated from Z-stacks, recorded for 40 µm, starting from the leaf upper surface.
To assess whether irradiation with UV-B has prolonged effects on chloroplast movements, we examined its impact on the actin cytoskeleton and investigated blue light-induced chloroplast movements in UV-B-pretreated leaves. Arabidopsis plants expressing Lifeact, an actin binding peptide fused with GFP, were exposed to different UV-B intensities and observed under the confocal microscope. Projected images were recorded for the upper epidermis (Figure 4) and the top parts of the palisade cells (Supplementary Figure 6). The cp-actin, which is specific for chloroplast movements, was too faint for analysis of the effects of UV-B. No substantial differences in the architecture of the cortical actin cytoskeleton in the pavement or palisade cells were observed between leaves kept in darkness and those irradiated with UV-B of 3.3 µmol·m−2·s−1 (Figure 4, Supplementary Figure 6). The effect of UV-B on the cortical actin cytoskeleton in the pavement cells of the upper epidermis was quantified. The average length of actin filaments and their length per area of the projection image did not differ significantly between these conditions (Supplementary Figures 5A, B). After treatment with strong UV-B of 20 µmol·m−2·s−1, actin filaments were still visible, but long, thick fibers were fewer, in both the pavement and palisade cells (Figure 4, Supplementary Figure 6). The level of fluorescence in the cytoplasm, the nucleus, and the vacuole increased (Figure 4). The results of quantification for the pavement cells indicated that the average length of actin filaments and their length per area of the projection image were smaller than in non-irradiated samples (Supplementary Figures 5A, B), which suggests that strong UV-B irradiation disrupted actin filaments. No cytoplasmic, vacuolar, or nuclear green fluorescence was observed in WT leaves, regardless of the conditions (Figure 4).
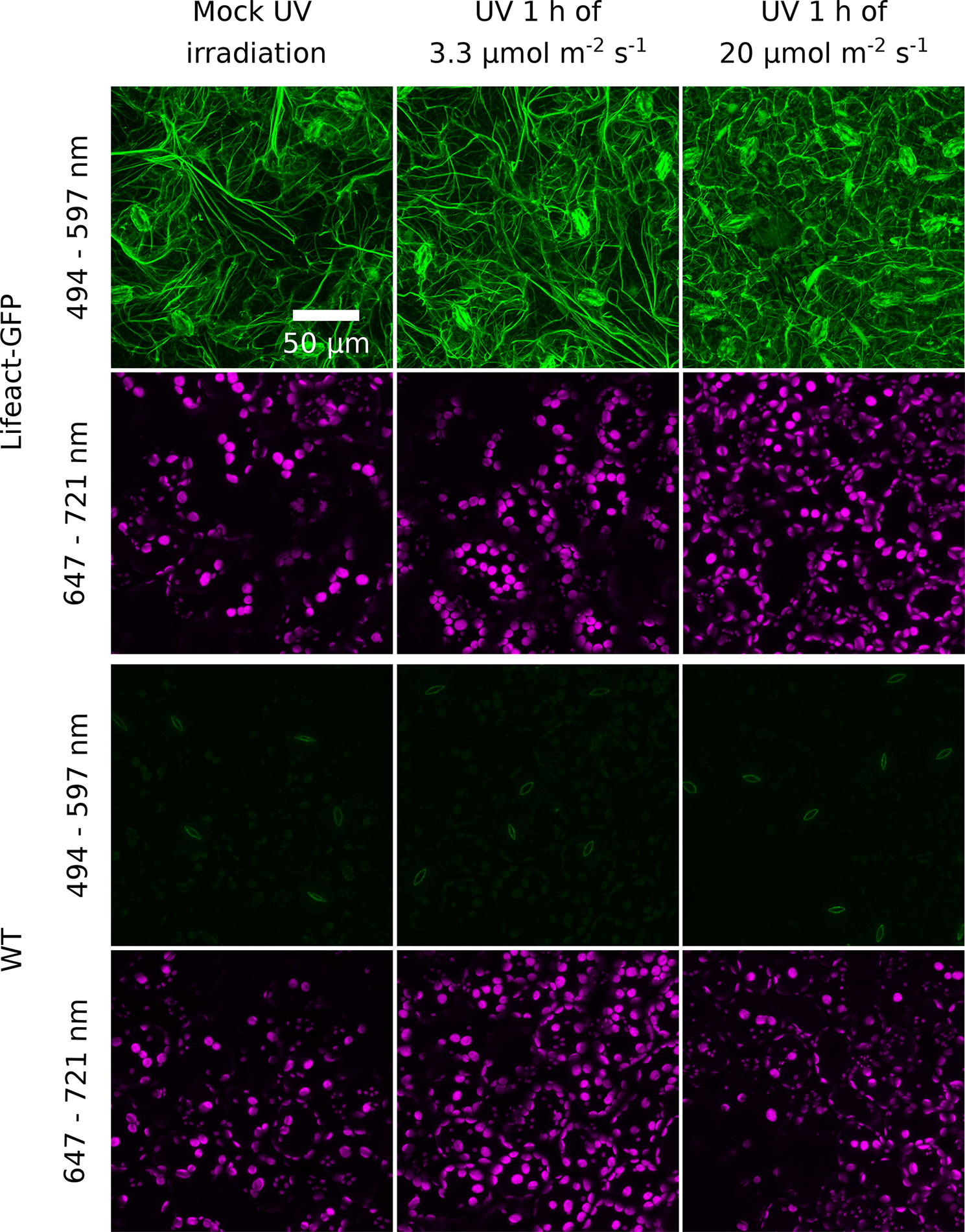
Figure 4 Effect of UV-B irradiation on the actin cytoskeleton visualized with Lifeact-green fluorescent protein (GFP) in the epidermal cells of Arabidopsis leaves. Wild-type (WT) plants serve as a control of nonspecific green fluorescence. Leaves were mock irradiated or irradiated with UV-B (280–320 nm) of 3.3 or 20 µmol·m−2·s−1 for 1 h. Cells were then imaged with a confocal microscope. The emission was recorded in the 494- to 597-nm range for GFP visualization and in the 647- to 721-nm range to visualize chloroplasts. Maximum intensity projections were calculated from Z-stacks, recorded for 30 µm, starting from the leaf upper surface.
Chloroplast responses to blue light after pretreatment with UV-B of 3.3 or 20 µmol·m−2·s−1 for 1 h were examined in WT and phot2 mutant plants using the photometric method. Blue light intensities of 1.6 µmol·m−2·s−1, eliciting chloroplast accumulation, and of 120 µmol·m−2·s−1, triggering chloroplast avoidance in WT plants, were used.
In WT plants, the amplitude of blue light-induced chloroplast accumulation was more than twice smaller in leaves pretreated with UV-B of 3.3 µmol·m−2·s−1 than in those kept in darkness (Figure 5A). However, the final transmittance was not affected by the pretreatment (Supplementary Figure 7A). This suggests that the small amplitude of blue light-induced accumulation in leaves irradiated with UV-B was merely due to the fact that in those leaves chloroplasts had already partially accumulated in response to UV-B. Similarly, UV-B pretreated leaves and those kept in darkness reached the same transmittance during the avoidance response induced by strong blue light of 120 µmol·m−2·s−1 (Figure 5B, Supplementary Figure 7B). In the phot2 mutant, which shows sustained chloroplast accumulation regardless of the blue light intensity, pretreatment with UV-B of 3.3 µmol·m−2·s−1 did not affect the final transmittance after subsequent illumination with any blue light intensity (Figures 5C, D). Both in WT and the phot2 mutant, the velocities of blue light-induced chloroplast accumulation were lower in leaves pretreated with UV-B of 3.3 µmol·m−2·s−1 than in the mock-irradiated ones (Supplementary Figures 7C, D). The differences in velocities may stem from the partial accumulation in UV-B irradiated leaves. The velocity of chloroplast avoidance in WT was not affected by the UV-B pretreatment (Supplementary Figure 7D).
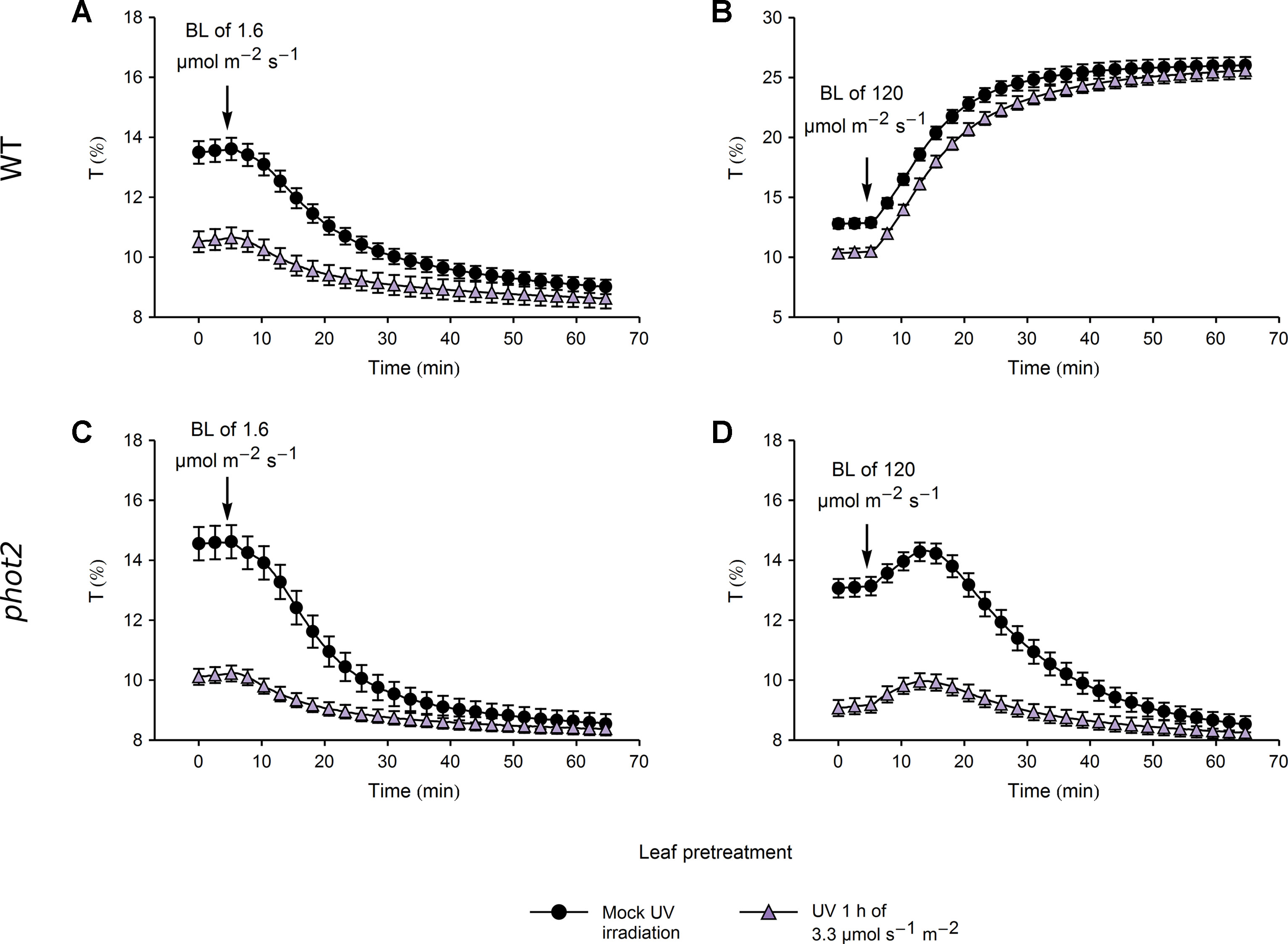
Figure 5 Chloroplast movements induced by blue light (BL) in control and UV-B pretreated leaves of wild-type (WT) (A, B) and phot2 plants (C, D). Changes in transmittance T induced by blue light of 1.6 (A, C) or 120 µmol·m−2·s−1 (B, D) were recorded for leaves previously irradiated with UV-B of 3.3 µmol·m−2·s−1 (triangles) or kept in darkness (disks). Each curve is an average of 20 recordings. Error bars show standard error (SE).
In WT plants, pretreatment with UV-B of 20 µmol·m−2·s−1 caused a substantial reduction in the magnitude of subsequent blue light-induced chloroplast accumulation and avoidance, even though the transmittance of UV-B and mock-irradiated leaves did not differ (Figures 6A, B). In line with this, the total transmittance change, calculated as the sum of transmittance changes induced by pretreatment and blue light illumination, differed significantly between the UV-B and mock-irradiated leaves (Supplementary Figures 8A, B). This indicates a nonspecific attenuation of chloroplast movements by strong UV-B. In the phot2 mutant, the transmittance reached during blue light-induced chloroplast accumulation did not differ between leaves pretreated with strong UV-B and the mock-irradiated ones (Supplementary Figures 8C, D). However, the velocities of chloroplast responses to blue light were substantially diminished in WT and phot2 leaves pretreated with strong UV-B (Supplementary Figures 8C, D).
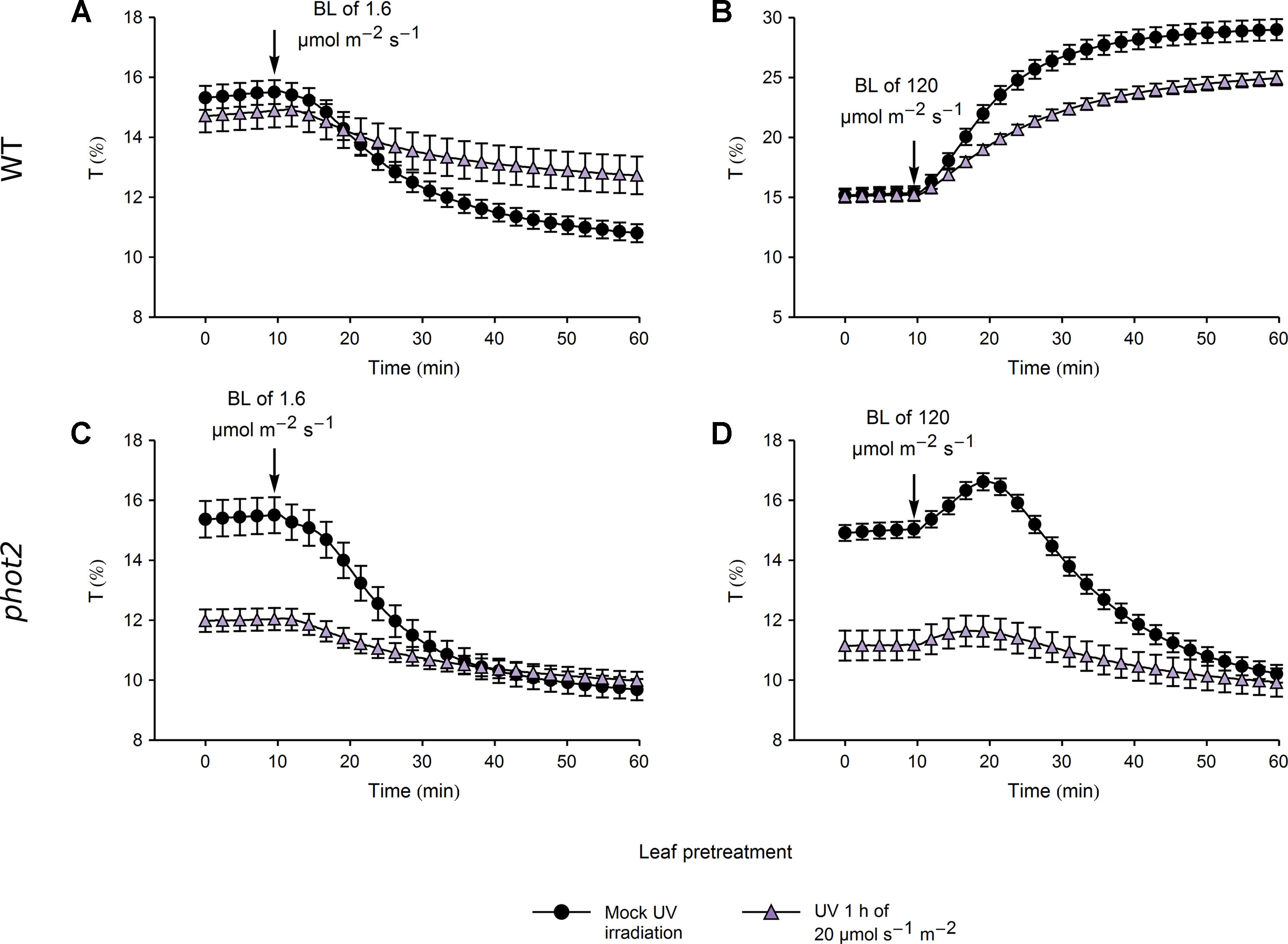
Figure 6 Chloroplast movements induced by blue light in control and UV-B pretreated leaves of wild-type (WT) (A, B) and phot2 plants (C, D). Changes in transmittance T induced by blue light of 1.6 (A, C) or 120 µmol·m−2·s−1 (B, D) were recorded for leaves previously irradiated with UV-B of 20 µmol·m−2·s−1 (triangles) for 1 h or mock-irradiated (disks). Each curve is an average of 14 recordings. Error bars show standard error (SE).
Several studies suggest that chloroplast movements depend on the expression level of the photoreceptor. Phot1-controlled chloroplast accumulation depends on its protein level (Doi et al., 2004). The velocity of chloroplast avoidance depends on phot2 protein expression (Kagawa and Wada, 2004; Kimura and Kagawa, 2009). To check whether UV-B irradiation may also affect chloroplast movements indirectly by controlling the amount of photoreceptors, phototropin1 and phototropin2 expression was investigated at the mRNA and protein levels in Arabidopsis leaves (Figure 7). WT and uvr8 plants were either treated with UV of 0.2 µmol·m−2·s−1 to induce the UVR8-regulated gene expression pathway (Brown and Jenkins, 2007) or kept in darkness for 3 h. UV slightly decreased phototropin1 expression at the mRNA and protein levels (Figures 7A, C) in both plant lines. PHOT2 mRNA level was up-regulated by UV in WT plants. This effect was less prominent in the uvr8 mutant (Figure 7B). At the protein level, the impact of UV treatment was observed neither in WT nor in uvr8 mutant plants (Figure 7D).
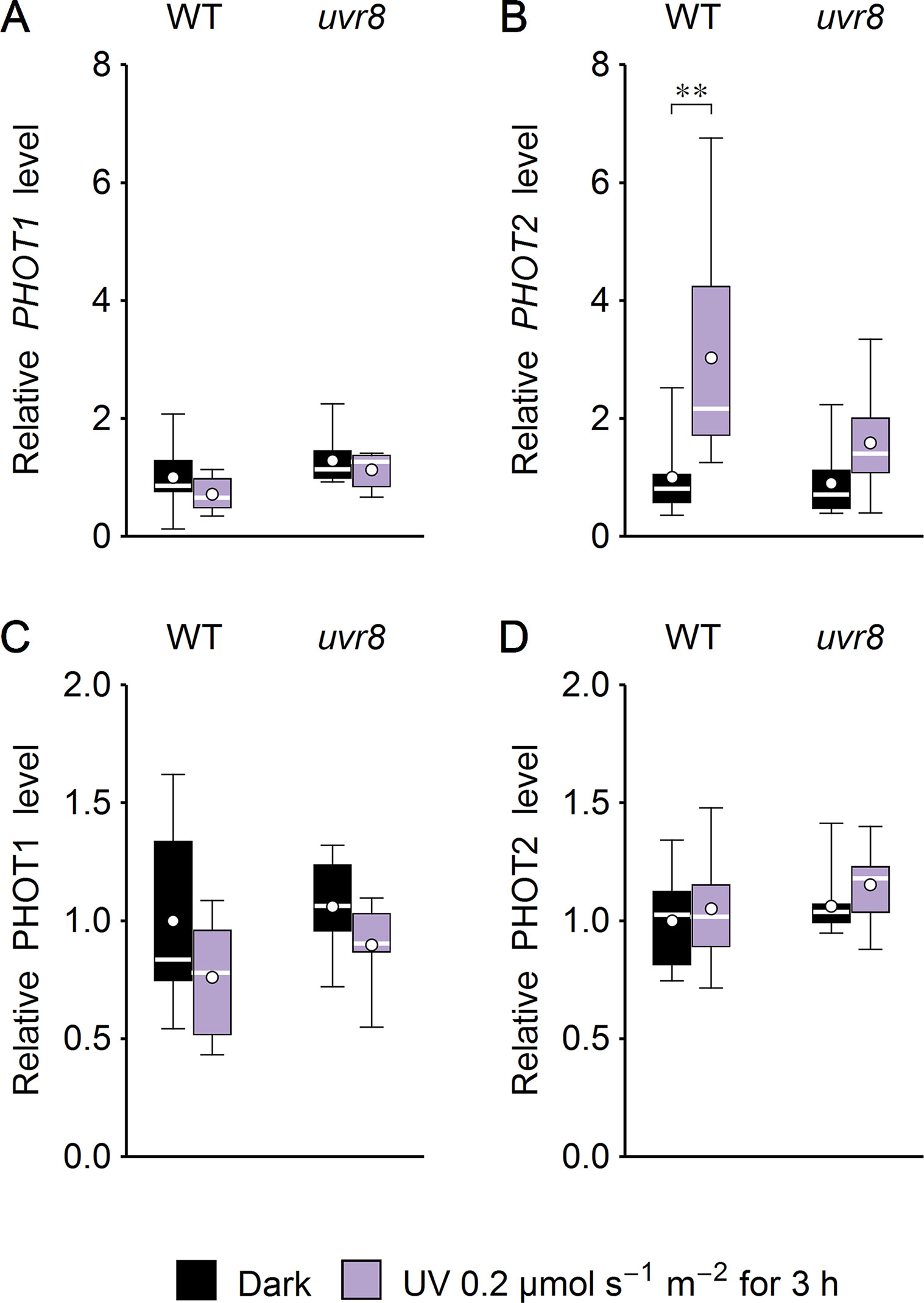
Figure 7 Effect of UV on the expression of phototropins in Arabidopsis leaves of wild type (WT) and the uvr8 mutant. Whole plants were irradiated for 3 h with UV of 0.2 µmol·m−2·s−1. The expression of phototropin1 (A, C) and phototropin2 (B, D) was examined at the mRNA level, using quantitative real-time polymerase chain reaction (PCR) (A, B) and immunoblotting followed by densitometry (C, D). Each box represents 7 and 8 (A, B), 7 (C), or 10 (D) biological replicates. Asterisks indicate statistically significant differences between illuminated and dark-adapted samples (adjusted p values: **0.001 < p < 0.01).
Discussion
UV-B induces chloroplast directional movements in the model plant Arabidopsis thaliana. Irradiation with UV-B of 3.3 µmol·m−2·s−1 triggers substantial chloroplast accumulation in WT, uvr8, and phot2 plants, but not in phot1 and phot1phot2 mutants (Figure 1A). Chloroplast responses to equimolar blue light are similar (Figure 1B). Chloroplast accumulation induced by UV-B relies on the presence of a protein necessary for blue light-induced accumulation, JAC1 (Figure 2A). JAC1 is responsible for inhibiting chloroplast avoidance triggered by phot2, as in strong blue light, the phenotype of the phot2jac1 double mutant resembles that of the phot2 single mutant (Kodama et al., 2010). Dependence of both UV-B- and blue light-induced chloroplast accumulation on JAC1 suggests that the signaling pathways used for these responses are the same or at least share some of their components. Chloroplast accumulation after UV-B of 3.3 µmol·m−2·s−1 is more prominent in the phot2 mutant than in WT (Figure 1A). This parallels the observations of increased blue light sensitivity of this mutant in the chloroplast accumulation response (Luesse et al., 2010). The enhancement of phot1-mediated chloroplast accumulation after blue light pulses, observed in the absence of phot2, was described in Sztatelman et al. (2016). This effect may result from physical interactions of phototropins. It appears that the presence of phot2 attenuates chloroplast accumulation triggered by short pulses of strong blue light in WT plants. In the phot2 mutant, only phot1 is present, so the attenuation is not observed. Phototropin interactions, predominantly through their N-terminal parts, may be responsible for this effect, as phototropin levels remain unaffected (Sztatelman et al., 2016). It appears that the postulated ability of phototropins to form homodimers and heterodimers influences chloroplast responses to UV-B. Similarities between UV-B- and blue light-induced movements are also apparent when high intensities are used. UV-B of 20 µmol·m−2·s−1 induces chloroplast accumulation only in the phot2 mutant (Figure 1A). Likewise, blue light of the same intensity induces accumulation only in this mutant (Figure 1B).
Although the results of quantification of microscopic images (Supplementary Figure 4) roughly agree with the results obtained with the photometric method, direct comparison is not straightforward. Microscopic observations are performed for palisade cells. The photometric method detects chloroplast relocations throughout the leaf tissue, including cell layers that are located deeper in the mesophyll and thus receive less light. The gradient of UV-B in the tissue is probably much higher than that of visible light, due to its increased absorption. In Funaria, the transmittance of the whole cell (excluding chloroplasts) is high (∼90%) in the visible range and UV longer than 330 nm. At shorter wavelengths, the transmittance is reduced, decreasing to 62% at 300 nm (Zurzycki, 1967).
The increase in leaf transmittance upon strong UV-B irradiation observed in the jac1 mutant indicates that UV-B can elicit also chloroplast avoidance (Figure 2A). Only phot2 is capable of mediating sustained avoidance. It seems to be activated by UV-B, though its sensitivity in this range is lower than to blue light (Figure 1). Thus, both phototropins may trigger chloroplast relocations upon UV-B irradiation. In field conditions, UV-B intensity does not exceed 3 W·m−2 (Aphalo et al., 2012). If no other radiation is present, such intensities of UV-B induce only chloroplast accumulation in WT Arabidopsis plants. It may seem counterintuitive that the potentially protective chloroplast avoidance cannot be induced in WT plants even by strong UV-B, which induces oxidative stress and directly causes DNA lesions. However, in the field, UV-B is always accompanied by UV-A and blue light, and thus, those different wavelengths probably act additively to elicit chloroplast relocations. The intensity of UV-B is usually much smaller than the intensity of accompanying UV-A and blue light. The avoidance response is sensitive to UV-A and blue light, which may have reduced the selective pressure for UV-B-induced avoidance. Low-intensity UV-B can act as an environmental signal. Chloroplast movements are induced mainly in plants that grow in changing light conditions, not in full sunlight (Augustynowicz and Gabryś, 1999; Higa and Wada, 2016). The UV-B to UV-A ratio changes depending on the light conditions and is higher in the tree shade as compared with full sunlight (Parisi and Kimlin, 1999). This suggests that under the canopy, the activation of phototropins by the UV-B part of the spectrum may contribute to the induction of chloroplast accumulation and to optimization of photosynthesis.
The differences in chloroplast responses to blue light observed between leaves pretreated with UV-B of 3.3 µmol·m−2·s−1 and mock-irradiated ones (Figure 5) can be explained by the initial, UV-B induced movement of chloroplasts towards the periclinal cell walls. This is not the case when leaves are pretreated with strong UV-B of 20 µmol·m−2·s−1 (Figure 6). In WT plants, initial transmittance changes do not differ between UV-B and mock-irradiated samples, but chloroplast responses to blue light of 1.6 (Figure 6A) and 120 µmol·m−2·s−1 (Figure 6B) are diminished, in terms of both amplitudes and rates (Supplementary Figure 8). This indicates that strong UV-B impairs both chloroplast accumulation and avoidance in an unspecific manner, probably due to cell damage. Microscopic examination of the actin cytoskeleton in epidermal cells (Figure 4) and the upper parts of the palisade cells (Supplementary Figure 6) supports this assumption. In wheat protoplasts, UV-B irradiation disturbs the actin cytoskeleton, which forms foci at different stages of the cell cycle. Those effects are accompanied by the formation of apoptotic bodies (Chen and Han, 2016). UV-B-induced programmed cell death has also been reported for BY2 cells (Lytvyn et al., 2010). Thus, chloroplast movement inhibition after strong UV-B may result from actin disruption caused not directly by irradiation but by programmed cell death induction. On the other hand, strong UV-B intensity used in this study still induces chloroplast accumulation in the phot2 mutant (Figure 1A) and avoidance in jac1 (Figure 2A).
Our results indicate that UVR8 is not involved in controlling chloroplast movements under UV-B (Figures 1A and 3). This agrees with its predominantly nuclear activity and relatively low expression level in the leaf mesophyll (Bernula et al., 2017). UVR8 enters the nucleus after UV-B treatment (Kaiserli and Jenkins, 2007), where it controls gene expression. Its role in the cytoplasm is not well understood. UVR8 is capable of triggering phototropism in seedlings in the absence of phototropins (Vandenbussche et al., 2014). However, phototropism relies on a gradient formation of signaling components across the whole tissue (Morrow et al., 2018), which can be obtained even with a photoreceptor localized mainly in the nucleus, such as UVR8. Chloroplast movements are very local. They can be induced in a small volume of the cell by microbeam irradiation and require photoreceptors that are spatially oriented inside the cell through their association with the plasma membrane (Sakamoto and Briggs, 2002; Kong et al., 2013a) or the chloroplast envelope (Kong et al., 2013b). Our results suggest that low-intensity UV up-regulates PHOT2 mRNA levels, and this process is at least partially mediated by the UVR8 photoreceptor (Figure 7B). However, UV-B has no impact on phototropin protein levels (Figures 7C, D).
We demonstrate that the UV-B part of the spectrum takes part in the regulation of chloroplast movements in Arabidopsis in a manner dependent on phototropins. Our results suggest that phototropin1 is required for efficient UV-B-induced chloroplast accumulation. The involvement of phototropins in UV-B sensing has been shown previously for phototropism (Vandenbussche et al., 2014; Vanhaelewyn et al., 2016). Perception of UV-B by phototropins may be important for plants growing under the canopy, where higher UV-B to UV-A ratios are observed. Thus, the phototropin pathway may contribute to the physiological effects of UV-B. However, the UV-B-induced chloroplast accumulation described in this work differs from typical responses to UV-B, mediated by UVR8, which include modulation of development and induction of mechanisms that protect the plant against damage. Our results add new insights into the diversity of plant responses to UV-B and its potential applications.
Data Availability Statement
All datasets generated for this study are included in the manuscript/Supplementary Files.
Author Contributions
PH, JŁ, and AKB designed the study. PH, JŁ, AKB, and OS performed the experiments. JŁ wrote the manuscript. PH, AKB, OS, and HG commented and improved the manuscript.
Conflict of Interest
The authors declare that the research was conducted in the absence of any commercial or financial relationships that could be construed as a potential conflict of interest.
Acknowledgments
This work was supported by the Polish National Science Centre grant no. UMO-2011/03/D/NZ3/00210 to AKB and grant no. UMO-2014/15/D/NZ2/02306 to JŁ and by the European Regional Development Fund in the framework of the Polish Innovation Economy Operational Program [contract no. POIG.02.01.00-12-167/08 for funds for the purchase of equipment in the project Małopolska Centre of Biotechnology (MCB)].
Supplementary Material
The Supplementary Material for this article can be found online at: https://www.frontiersin.org/articles/10.3389/fpls.2019.01279/full#supplementary-material
References
Aggarwal, C., Banaś, A. K., Kasprowicz-Maluśki, A., Borghetti, C., Łabuz, J., Dobrucki, J., et al. (2014). Blue-light-activated phototropin2 trafficking from the cytoplasm to Golgi/post-Golgi vesicles. J. Exp. Bot. 65, 3263–3276. doi: 10.1093/jxb/eru172
Aphalo, P. J., Albert, A., Bjorn, L. O., Mcleod, A. R., Robson, T. M., Rosenqvist, E. (2012). Beyond the visible: a handbook of best practice in plant UV photobiology. Ed. Aphalo, P.J. (Helsinki, Helsinki: University of Helsinki) doi: 10.31885/9789521083631
Augustynowicz, J., Gabry, H. (1999). Chloroplast movements in fern leaves: correlation of movement dynamics and environmental flexibility of the species. Plant Cell Environ. 22, 1239–1248. doi: 10.1046/j.1365-3040.1999.00487.x
Banaś, A. K., Aggarwal, C., Łabuz, J., Sztatelman, O., Gabryś, H. (2012). Blue light signalling in chloroplast movements. J. Exp. Bot. 63, 1559–1574. doi: 10.1093/jxb/err429
Banaś, A. K., Hermanowicz, P., Sztatelman, O., Łabuz, J., Aggarwal, C., Zgłobicki, P., et al. (2018). 6,4-PP photolyase encoded by AtUVR3 is localized in nuclei, chloroplasts and mitochondria and its expression is down-regulated by light in a photosynthesis-dependent manner. Plant Cell Physiol. 59, 44–57. doi: 10.1093/pcp/pcx159
Bernula, P., Crocco, C. D., Arongaus, A. B., Ulm, R., Nagy, F., Viczián, A. (2017). Expression of the UVR8 photoreceptor in different tissues reveals tissue-autonomous features of UV-B signalling. Plant Cell Environ. 40, 1104–1114. doi: 10.1111/pce.12904
Brown, B. A., Jenkins, G. I. (2007). UV-B signaling pathways with different fluence-rate response profiles are distinguished in mature Arabidopsis leaf tissue by requirement for UVR8, HY5, and HYH. Plant Physiol. 146, 576–588. doi: 10.1104/pp.107.108456
Chen, H., Han, R. (2016). Characterization of actin filament dynamics during mitosis in wheat protoplasts under UV-B radiation. Sci. Rep. 6, 1–8. doi: 10.1038/srep20115
Christie, J. M., Blackwood, L., Petersen, J., Sullivan, S. (2015). Plant flavoprotein photoreceptors. Plant Cell Physiol. 56, 401–413. doi: 10.1093/pcp/pcu196
Christie, J. M., Swartz, T. E., Bogomolni, R. A., Briggs, W. R. (2002). Phototropin LOV domains exhibit distinct roles in regulating photoreceptor function. Plant J. 32, 205–219. doi: 10.1046/j.1365-313X.2002.01415.x
Copeland, R. A., Spiro, T. G. (1986). Ultraviolet resonance Raman spectroscopy of flavin mononucleotide and flavin adenine dinucleotide. J. Phys. Chem. 90, 6648–6654. doi: 10.1021/j100283a011
Czechowski, T., Stitt, M., Altmann, T., Udvardi, M. K., Scheible, W. R. (2005). Genome-wide identification and testing of superior reference genes for transcript normalization in Arabidopsis. Plant Physiol. 139, 5–17. doi: 10.1104/pp.105.063743
Doi, M., Shigenaga, A., Emi, T., Kinoshita, T., Shimazaki, K. I. (2004). A transgene encoding a blue-light receptor, phot1, restores blue-light responses in the Arabidopsis phot1 phot2 double mutant. J. Exp. Bot. 55, 517–523. doi: 10.1093/jxb/erh044
Favory, J., Gruber, H., Rizzini, L., Oravecz, A., Funk, M., Albert, A., et al. (2009). Interaction of COP1 and UVR8 regulates stress acclimation in Arabidopsis. EMBO J. 28, 591–601. doi: 10.1038/emboj.2009.4
Gabryś, H., Banaś, A. K., Hermanowicz, P., Krzeszowiec, W., Leśniewski, S., Łabuz, J., et al. (2017). Photometric assays for chloroplast movement responses to blue light. Bio. Protocol. 7, 1–11. doi: 10.21769/BioProtoc.2310
Gotoh, E., Suetsugu, N., Yamori, W., Ishishita, K., Kiyabu, R., Fukuda, M., et al. (2018). Chloroplast accumulation response enhances leaf photosynthesis and plant. Plant Physiol. 178, 1358–1369. doi: 10.1104/pp.18.00484
Han, T., Chung, H., Kang, S. H. (1998). UV photobiology of marine macroalgae. Korean J. Polar Res. 9, 37–46.
Harada, A., Shimazaki, K. (2007). Phototropins and blue light-dependent calcium signaling in higher plants. Photochem. Photobiol. 83, 102–111. doi: 10.1562/2006-03-08-IR-837
Higa, T., Wada, M. (2016). Chloroplast avoidance movement is not functional in plants grown under strong sunlight. Plant Cell Environ. 39, 871–882. doi: 10.1111/pce.12681
Jarillo, J. A., Gabrys, H., Capel, J., Alonso, J. M., Ecker, J. R., Cashmore, A. R. (2001). Phototropin-related NPL1 controls chloroplast relocation induced by blue light. Nature 410, 952–954. doi: 10.1038/35073622
Jenkins, G. I. (2017). Photomorphogenic responses to ultraviolet-B light. Plant Cell Environ. 40, 2544–2557. doi: 10.1111/pce.12934
Kadota, A., Sato, Y., Wada, M. (2000). Intracellular chloroplast photorelocation in the moss Physcomitrella patens is mediated by phytochrome as well as by a blue-light receptor. Planta 210, 932–937. doi: 10.1007/s004250050700
Kadota, A., Yamada, N., Suetsugu, N., Hirose, M., Saito, C., Shoda, K., et al. (2009). Short actin-based mechanism for light-directed chloroplast movement in Arabidopsis. Proc. Natl. Acad. Sci. 106, 13106–13111. doi: 10.1073/pnas.0906250106
Kagawa, T., Wada, M. (2004). Velocity of chloroplast avoidance movement is fluence rate dependent. Photochem. Photobiol. Sci. 3, 592–595. doi: 10.1039/b316285k
Kagawa, T., Sakai, T., Suetsugu, N., Oikawa, K., Ishiguro, S., Kato, T., et al. (2001). Arabidopsis NPL1: A Phototropin Homolog Controlling the Chloroplast High-Light Avoidance Response. Sci. 291, 2138–2141. doi: 10.1126/science.291.5511.2138
Kaiserli, E., Jenkins, G. I. (2007). UV-B promotes rapid nuclear translocation of the Arabidopsis UV-B-specific signaling component UVR8 and activates its function in the nucleus. Plant Cell 19, 2662–2673. doi: 10.1105/tpc.107.053330
Kandasamy, M. K., Meagher, R. B. (1999). Actin–organelle interaction: association with chloroplast in Arabidopsis leaf mesophyll cells. Cell Motil. Cytoskeleton 44, 110–118. doi: 10.1002/(SICI)1097-0169(199910)44:2<110::AID-CM3>3.3.CO;2-F
Kasahara, M., Kagawa, T., Oikawa, K., Suetsugu, N., Miyao, M., Wada, M. (2002a). Chloroplast avoidance movement reduces photodamage in plants. Nature 420, 829–832. doi: 10.1038/nature01213
Kasahara, M., Swartz, T. E., Olney, M. A., Onodera, A., Mochizuki, N., Fukuzawa, H., et al. (2002b). Photochemical properties of the flavin mononucleotide-binding domains of the phototropins from Arabidopsis, rice, and Chlamydomonas reinhardtii. Plant Physiol. 129, 762–773. doi: 10.1104/pp.002410
Kawai, H., Kanegae, T., Christensen, S., Kiyosue, T., Sato, Y., Imaizumi, T., et al. (2003). Responses of ferns to red light are mediated by an unconventional photoreceptor. Nature 421, 287–290. doi: 10.1038/nature01310
Kimura, M., Kagawa, T. (2009). Blue light-induced chloroplast avoidance and phototropic responses exhibit distinct dose dependency of PHOTOTROPIN2 in Arabidopsis thaliana. Photochem. Photobiol. 85, 1260–1264. doi: 10.1111/j.1751-1097.2009.00564.x
Kodama, Y., Suetsugu, N., Kong, S. G., Wada, M. (2010). Two interacting coiled-coil proteins, WEB1 and PMI2, maintain the chloroplast photorelocation movement velocity in Arabidopsis. Proc. Natl. Acad. Sci. 107, 19591–19596. doi: 10.1073/pnas.1007836107
Kong, S. G., Kagawa, T., Wada, M., Nagatani, A. (2013a). A C-terminal membrane association domain of phototropin 2 is necessary for chloroplast movement. Plant Cell Physiol. 54, 57–68. doi: 10.1093/pcp/pcs132
Kong, S. G., Suetsugu, N., Kikuchi, S., Nakai, M., Nagatani, A., Wada, M. (2013b). Both phototropin 1 and 2 localize on the chloroplast outer membrane with distinct localization activity. Plant Cell Physiol. 54, 80–92. doi: 10.1093/pcp/pcs151
Kong, S. G., Suzuki, T., Tamura, K., Mochizuki, N., Hara-Nishimura, I., Nagatani, A. (2006). Blue light-induced association of phototropin 2 with the Golgi apparatus. Plant J. 45, 994–1005. doi: 10.1111/j.1365-313X.2006.02667.x
Krzeszowiec, W., Rajwa, B., Dobrucki, J., Gabryś, H. (2007). Actin cytoskeleton in Arabidopsis thaliana under blue and red light. Biol. Cell 99, 251–260. doi: 10.1042/BC20060077
Łabuz, J., Hermanowicz, P., Gabryś, H. (2015). The impact of temperature on blue light induced chloroplast movements in Arabidopsis thaliana. Plant Sci. 239, 238–249. doi: 10.1016/j.plantsci.2015.07.013
Łabuz, J., Sztatelman, O., Banaś, A. K., Gabryś, H. (2012). The expression of phototropins in Arabidopsis leaves: developmental and light regulation. J. Exp. Bot. 63, 1763–1771. doi: 10.1093/jxb/ers061
Lehmann, P., Nöthen, J., Schmidt Von Braun, S., Bohnsack, M. T., Mirus, O., Schleiff, E. (2011). Transitions of gene expression induced by short-term blue light. Plant Biol. 13, 349–361. doi: 10.1111/j.1438-8677.2010.00377.x
Luesse, D. R., Deblasio, S. L., Hangarter, R. P. (2010). Integration of phot1, phot2, and PhyB signalling in light-induced chloroplast movements. J. Exp. Bot. 61, 4387–4397. doi: 10.1093/jxb/erq242
Lytvyn, D. I., Yemets, A. I., Blume, Y. B. (2010). UV-B overexposure induces programmed cell death in a BY-2 tobacco cell line. Environ. Exp. Bot. 68, 51–57. doi: 10.1016/j.envexpbot.2009.11.004
Morrow, J., Willenburg, K. T., Liscum, E. (2018). Phototropism in land plants: molecules and mechanism from light perception to response. Front. Biol. (Beijing) 13, 342–357. doi: 10.1007/s11515-018-1518-y
Parisi, A. V., Kimlin, M. G. (1999). Comparison of the spectral biologically effective solar ultraviolet in adjacent tree shade and sun. Phys. Med. Biol. 44, 2071–2080. doi: 10.1088/0031-9155/44/8/316
Rizzini, L., Nagy, F., Jenkins, G. I., Ulm, R., Baumeister, R., Favory, J. J., et al. (2011). Perception of UV-B by the Arabidopsis UVR8 protein. Science 332, 103–106. doi: 10.1126/science.1200660
Robson, T. M., Aphalo, P. J., Banaś, A. K., Barnes, P. W., Brelsford, C. C., Jenkins, G. I., et al. (2019). A perspective on ecologically relevant plant-UV research and its practical application. Photochem. Photobiol. Sci. 18, 970–988. doi: 10.1039/C8PP00526E
Sakai, T., Kagawa, T., Kasahara, M., Swartz, T. E., Christie, J. M., Briggs, W. R., et al. (2001). Arabidopsis nph1 and npl1: blue light receptors that mediate both phototropism and chloroplast relocation. Proc. Natl. Acad. Sci. 98, 6969–6974. doi: 10.1073/pnas.101137598
Sakamoto, K., Briggs, W. R. (2002). Cellular and subcellular localization of phototropin 1. Plant Cell 14, 1723–1735. doi: 10.1105/tpc.003293
Salomon, M., Christie, J. M., Knieb, E., Lempert, U., Briggs, W. R. (2000). Photochemical and mutational analysis of the FMN-binding domains of the plant blue light receptor, phototropin. Biochemistry 39, 9401–9410. doi: 10.1021/bi000585
Schuch, A. P., Moreno, N. C., Schuch, N. J., Menck, C. F. M., Garcia, C. C. M. (2017). Sunlight damage to cellular DNA: focus on oxidatively generated lesions. Free Radic. Biol. Med. 107, 110–124. doi: 10.1016/j.freeradbiomed.2017.01.029
Sharon, Y., Dishon, G., Beer, S. (2011). The effects of UV radiation on chloroplast clumping and photosynthesis in the seagrass Halophila stipulacea grown under high-PAR conditions. J. Mar. Biol. 2011, 1–6. doi: 10.1155/2011/483428
Smertenko, A. P., Deeks, M. J., Hussey, P. J. (2010). Strategies of actin reorganisation in plant cells. J. Cell Sci. 123, 3019–3028. doi: 10.1242/jcs.079749
Steger, C. (1998). An unbiased detector of curvilinear structures. IEEE Trans. Pattern Anal. Mach. Intell. 20, 113–125. doi: 10.1109/34.659930
Suetsugu, N., Kagawa, T., Wada, M. (2005a). An auxilin-like J-domain protein, JAC1, regulates phototropin-mediated chloroplast movement. Plant Physiol. 139, 151–162. doi: 10.1104/pp.105.067371
Suetsugu, N., Mittmann, F., Wagner, G., Hughes, J., Wada, M. (2005b). From the cover: a chimeric photoreceptor gene, NEOCHROME, has arisen twice during plant evolution. Proc. Natl. Acad. Sci. 102, 13705–13709. doi: 10.1073/pnas.0504734102
Sztatelman, O., Łabuz, J., Hermanowicz, P., Banaś, A. K., Bażant, A., Zgłobicki, P., et al. (2016). Fine tuning chloroplast movements through physical interactions between phototropins. J. Exp. Bot. 67, 4963–4978. doi: 10.1093/jxb/erw265
Sztatelman, O., Waloszek, A., Banaś, A. K., Gabryś, H. (2010). Photoprotective function of chloroplast avoidance movement: in vivo chlorophyll fluorescence study. J. Plant Physiol. 167, 709–716. doi: 10.1016/j.jplph.2009.12.015
Tokutomi, S., Matsuoka, D., Zikihara, K. (2008). Molecular structure and regulation of phototropin kinase by blue light. Biochim. Biophys. Acta - Proteins Proteomics 1784, 133–142. doi: 10.1016/j.bbapap.2007.09.010
Tsuboi, H., Wada, M. (2012). Distribution pattern changes of actin filaments during chloroplast movement in Adiantum capillus-veneris. J. Plant Res. 125, 417–428. doi: 10.1007/s10265-011-0444-8
Vandenbussche, F., Tilbrook, K., Fierro, A. C., Marchal, K., Poelman, D., Van Der Straeten, D., et al. (2014). Photoreceptor-mediated bending towards UV-B in Arabidopsis. Mol. Plant 7, 1041–1052. doi: 10.1093/mp/ssu039
Vandesompele, J., De Preter, K., Pattyn, F., Poppe, B., Van Roy, N., De Paepe, A., et al. (2002). Accurate normalization of real-time quantitative RT-PCR data by geometric averaging of multiple internal control genes. Genome Biol. 3, RESEARCH0034. doi: 10.1186/gb-2002-3-7-research0034
Vanhaelewyn, L., Schumacher, P., Poelman, D., Fankhauser, C., Van Der Straeten, D., Vandenbussche, F. (2016). REPRESSOR OF ULTRAVIOLET-B PHOTOMORPHOGENESIS function allows efficient phototropin mediated ultraviolet-B phototropism in etiolated seedlings. Plant Sci. 252, 215–221. doi: 10.1016/j.plantsci.2016.07.008
Walczak, T., Gabrys, H. (1980). New type of photometer for measurements of transmission changes corresponding to chloroplast movements in leaves. Photosynthetica 14, 65–72.
Yamashita, H., Sato, Y., Kanegae, T., Kagawa, T., Wada, M., Kadota, A. (2011). Chloroplast actin filaments organize meshwork on the photorelocated chloroplasts in the moss Physcomitrella patens. Planta 233, 357–368. doi: 10.1007/s00425-010-1299-2
Yang, X., Montano, S., Ren, Z. (2015). How does photoreceptor UVR8 perceive a UV-B signal. Photochem. Photobiol. 91, 993–1003. doi: 10.1111/php.12470
Yin, R., Skvortsova, M. Y., Loubéry, S., Ulm, R. (2016). COP1 is required for UV-B–induced nuclear accumulation of the UVR8 photoreceptor. Proc. Natl. Acad. Sci. 113, E4415–E4422. doi: 10.1073/pnas.1607074113
Yin, R., Ulm, R. (2017). How plants cope with UV-B: from perception to response. Curr. Opin. Plant Biol. 37, 42–48. doi: 10.1016/j.pbi.2017.03.013
Zurzycki, J. (1955). Chloroplast arrangements as a factor of photosynthesis. Acta Soc. Bot. Pol. XXIV, 27–63. doi: 10.5586/asbp.1955.003
Zurzycki, J. (1962). The action spectrum for the light depended movements of chloroplasts in Lemna trisulca L. Acta Soc. Bot. Pol. XXXI, 489–538. doi: 10.5586/asbp.1962.036
Keywords: phototropin1, phototropin2, chloroplast movements, UV-B, UVR8, Arabidopsis
Citation: Hermanowicz P, Banaś AK, Sztatelman O, Gabryś H and Łabuz J (2019) UV-B Induces Chloroplast Movements in a Phototropin-Dependent Manner. Front. Plant Sci. 10:1279. doi: 10.3389/fpls.2019.01279
Received: 11 April 2019; Accepted: 12 September 2019;
Published: 15 October 2019.
Edited by:
Filip Vandenbussche, Ghent University, BelgiumReviewed by:
Saijaliisa Kangasjärvi, University of Turku, FinlandRuohe Yin, Shanghai Jiao Tong University, China
Shin-ichiro Inoue, Nagoya University, Japan
Copyright © 2019 Hermanowicz, Banaś, Sztatelman, Gabryś and Łabuz. This is an open-access article distributed under the terms of the Creative Commons Attribution License (CC BY). The use, distribution or reproduction in other forums is permitted, provided the original author(s) and the copyright owner(s) are credited and that the original publication in this journal is cited, in accordance with accepted academic practice. No use, distribution or reproduction is permitted which does not comply with these terms.
*Correspondence: Justyna Łabuz, anVzdHluYS5zb2prYUB1ai5lZHUucGw=