- 1Department of Environmental Sciences, Botany, Zurich-Basel Plant Science Center, University of Basel, Basel, Switzerland
- 2Laboratoire de Recherche en Sciences Végétales, Université de Toulouse, UPS, CNRS, Castanet-Tolosan, France
- 3Department of Biology at the Swiss Federal Institute of Technology Zurich, Zurich, Switzerland
- 4Max Planck Institute of Molecular Plant Physiology, Potsdam-Golm, Germany
- 5Agroécologie, AgroSup Dijon, CNRS, INRAE, Univ. Bourgogne, Univ. Bourgogne Franche-Comté, Dijon, France
In arbuscular mycorrhizal (AM) symbiosis, key components of nutrient uptake and exchange are specialized transporters that facilitate nutrient transport across membranes. As phosphate is a nutrient and a regulator of nutrient exchanges, we investigated the effect of P availability to extraradical mycelium (ERM) on both plant and fungus transcriptomes and metabolomes in a symbiocosm system. By perturbing nutrient exchanges under the control of P, our objectives were to identify new fungal genes involved in nutrient transports, and to characterize in which extent the fungus differentially modulates its metabolism when interacting with two different plant species. We performed transportome analysis on the ERM and intraradical mycelium of the AM fungus Rhizophagus irregularis associated to Populus trichocarpa and Sorghum bicolor under high and low P availability in ERM, using quantitative RT-PCR and Illumina mRNA-sequencing. We observed that mycorrhizal symbiosis induces expression of specific phosphate and ammonium transporters in both plants. Furthermore, we identified new AM-inducible transporters and showed that a subset of phosphate transporters is regulated independently of symbiotic nutrient exchange. mRNA-Sequencing revealed that the fungal transportome was not similarly regulated in the two host plant species according to P availability. Mirroring this effect, many plant carbohydrate transporters were down-regulated in P. trichocarpa mycorrhizal root tissue. Metabolome analysis revealed further that AM root colonization led to a modification of root primary metabolism under low and high P availability and to a decrease of primary metabolite pools in general. Moreover, the down regulation of the sucrose transporters suggests that the plant limits carbohydrate long distance transport (i.e. from shoot to the mycorrhizal roots). By simultaneous uptake/reuptake of nutrients from the apoplast at the biotrophic interface, plant and fungus are both able to control reciprocal nutrient fluxes.
Highlight
Gene expression analysis in arbuscular mycorrhizal symbiosis upon phosphate variations reveals imbalanced fungal gene regulation between perennial/C3 and annual/C4 host, highlights new mycorrhiza-inducible transporters and suggests active fungal carbohydrate uptake.
Introduction
Phosphorus (P), and nitrogen (N), are among the most essential nutrients for plants. As P is present in many biological compounds and is involved in many key metabolic processes, it can make up to 0.2% of the dry weight of a plant (Schachtman et al., 1998). In living plants, the cellular inorganic phosphate (Pi) concentration ranges between 1 and 10 mM whereas the soil water Pi concentration, is about 10,000-times less (Rausch and Bucher, 2002; Ai et al., 2009; Branscheid et al., 2010): Due to its negative charge and its low mobility, Pi is rapidly sequestered by cations and organic substances in the soil (e.g. clay) and therefore only barely accessible to plants (Poirier and Bucher, 2002; Aung et al., 2006; Chiou et al., 2006; Javot et al., 2007a; Tatry et al., 2009). To prevent mineral nutrient deficiency, and P deficiency in particular, a majority of land plants form symbioses with the so called arbuscular mycorrhizal (AM) fungi. In the AM symbiosis the AM fungus provides macro and micro nutrients to the plants and in return receives carbohydrates from the host plant (Smith and Read, 2008). AM fungi have a broad host range: each fungal species, each genet can colonize several plant individuals, also from different species, connected each other and forming a so called common mycorrhizal network (Walder et al., 2012; Fellbaum et al., 2014). The extraradical mycelium (ERM) of the AM fungi engaged in such a network is able to acquire nutrients that are out of reach or not accessible to the plant partners. For a given plant, this makes the mycorrhizal uptake pathway often more effective than the direct uptake pathway (Wipf et al., 2019). The nutrients taken up by the ERM are transported to the hyphal network inside the host root via the intraradical mycelium (IRM), which forms highly branched tree-like structures (arbuscules) inside the root cortical cells. The arbuscules are still surrounded by the plant cell-derived periarbuscular membrane and the inter-membrane interstice, the periarbuscular space. Within this structure, the mineral nutrients acquired by the AM fungus are exported to the periarbuscular space, and taken up by plant nutrient transporters of the periarbuscular membrane (Smith and Smith, 2011).
With respect to Pi, the extent to which plants cover their Pi-demand through the AM fungus ranges from only a small percentage to 100% (Paszkowski, 2006; Javot et al., 2007a). The Pi taken up by the ERM is incorporated into poly-P, transported to the arbuscules, hydrolyzed and translocated to the periarbuscular space (Ezawa et al., 2002; Javot et al., 2007a; Kikuchi et al., 2016). Essential key players in this process are transporters and permeases that facilitate uptake and transport of nutrients across membranes. The expression of transporters is regulated by nutrient availability. In this way, a steady and efficient translocation of nutrients adapted to given environmental conditions can be guaranteed (Smith and Smith, 2011; Courty et al., 2015; Garcia et al., 2016; Wipf et al., 2019).
In AM fungi, only few phosphate transporters (PT) were characterized so far: one in Funneliformis mosseae (GmosPT, Benedetto et al., 2005) and Glomus versiforme (GvPT, Harrison and Buuren 1995), and seven in Rhizophagus irregularis (formerly Glomus intraradices, RiPT1-RiPT7; Maldonado-Mendoza et al., 2001; Walder et al., 2016). Expression of the three high affinity transporters, RiPT1, GvPT, and GmosPT, is dependent on external Pi concentrations in the ERM (Harrison and Buuren, 1995; Maldonado-Mendoza et al., 2001; Benedetto et al., 2005). Reduced expression of GmosPT in the IRM compared to the ERM suggested a concentration-dependent regulation of PTs in the symbiotic root tissue.
In plants, the family of PTs can be divided into three subfamilies. Subfamily I transporters (Pht1) are membrane bound H+/P symporters driven by an H+ gradient. They are a subgroup of the major facilitator superfamily to which most of the PTs known to date belong (Pao et al., 1998). Subfamily II members are located in the plastids and function as antiporters (Poirier and Bucher, 2002; Rausch and Bucher, 2002; Javot et al., 2007b); members of the subfamily III are located in the mitochondrial inner membrane and are predicted to function as H+/P symporters or as P/OH- antiporters (Takabatake et al., 1999; Javot et al., 2007b). In mycorrhizal plants, some Pht1 PT are specifically induced during symbiotic interaction. The first mycorrhiza-inducible PT was identified in Solanum tuberosum (StPT3) and was localized in arbusculated root sections (Rausch et al., 2001). Then, more mycorrhiza-inducible transporters were identified in several other plants (Harrison et al., 2002; Glassop et al., 2005; Nagy et al., 2005; Loth-Pereda et al., 2011). StPT3 and MtPT4 from Medicago truncatula were present in the periarbuscular membrane only. Furthermore, it was demonstrated that MtPT4-deficient plants accumulated Pi as poly-P in the arbuscules, which resulted in a premature collapse of the arbuscules and in inefficient symbiosis (Javot et al., 2007a; Breuillin-Sessoms et al., 2015).
For N, it was long assumed that AM symbiosis plays only a minor role in plant nutrition. In the soil, inorganic N is mostly present as nitrate or ammonium, both of which are readily mobile in the soil. Therefore, it was assumed that AM fungi take up N with the same efficiency as plants (Marschner and Dell, 1994; Hodge et al., 2010; Smith and Smith, 2011). But, it was shown that AM fungi can contribute up to 42% of the plant N demand (Frey and Schüepp, 1993; Mäder et al., 2000; Govindarajulu et al., 2005). In addition to the uptake of ammonium and nitrate, it was shown that AM fungi can take up N in the form of organic molecules (i.e. small peptides and amino acids) (Bago et al., 1996; Hawkins et al., 2000; Govindarajulu et al., 2005; Jin et al., 2005) and possibly also in the form of more complex organic compounds (Leigh et al., 2009; Hodge et al., 2010). However, in plants as well as in AM fungi, it was shown that ammonium is the preferred N source, as it can be directly incorporated into glutamine by the glutamine synthetase/glutamine oxoglutarate aminotransferase (GS/GOGAT) pathway whereas nitrate needs to be reduced to ammonium before N assimilation in the GS/GOGAT pathway (Villegas et al., 1996; Hawkins et al., 2000; Toussaint et al., 2004). In the ERM of AM fungi, the glutamine is further metabolized into amino acids such as arginine, alanine and asparagine for transport. Studies using 15N showed that arginine was the most common labelled amino acid in the ERM of AM fungi (Govindarajulu et al., 2005). Arginine is then transported from the ERM to the IRM where it is cleaved by arginases in the arbuscules. The released ammonium is transported to the periarbuscular space where it can be taken up by the plant ammonium transporters (AMT). So far, only six transporters have been identified and functionally characterized in Glomeromycotina, three in R. irregularis GintAMT1, GintAMT2 and GintAMT3 (López-Pedrosa et al., 2006; Pérez-Tienda et al., 2011; Calabrese et al., 2016), and three in Geosiphon pyriformis (GpAmt1, GpAmt2, GpAmt3; Ellerbeck et al., 2013).
In plants, the family of AMT can be divided into two subfamilies: subfamily I and subfamily II (reviewed in Courty et al., 2015). While members of the subfamily I were found to be mostly expressed in roots, members of the subfamily II were preferentially expressed in shoots (Couturier et al., 2007). Several mycorrhiza-inducible AMTs have been identified through gene expression analysis in several plant species (Gomez et al., 2009; Guether et al., 2009), including PtAMT1.2 in poplar (Selle et al., 2005; Couturier et al., 2007) and SbAMT3.1 and SbAMT4 in sorghum (Koegel et al., 2013).
In return for the mineral nutrient, the AM fungi receives photosynthetates from the plants. In absence of gene encoding fatty acid synthase in their genome (Wewer et al., 2014; Tang et al., 2016), AM fungi are dependent to host fatty acids. Although the mechanism of transfer remains unknown, it was demonstrated that palmitic acid is transferred from plant roots to symbiotic mycelium, putatively esterified as mono-acyl-glycerol (Bravo et al., 2017; Jiang et al., 2017; Luginbuehl et al., 2017). Research on sugar transporter (SUT) expression in plants is not as consistent. Mycorrhization caused either increased or decreased expression of SUTs in root and shoots of the host plants (Ge et al., 2008; Boldt et al., 2011; Doidy et al., 2012a). A new class of SUT, the SWEETs, was identified. These transporters are located in the plasma membrane and have been shown to function as bidirectional sugar uniporters (Chen et al., 2010). Due to their involvement in rhizobial symbiosis it is assumed that they may also play a role in other biotrophic plant symbioses such as the AM symbiosis (Gamas et al., 1996; Doidy et al., 2012b).
In the AM fungus R. irregularis, four carbohydrate transporters have been identified (Helber et al., 2011). The gene encoding the monosaccharide transporter (MST) RiMST2 was the most highly expressed transporter in symbiotic tissue, and it could be localized in the arbuscules and in the IRM. Within this line, An et al. (2019) recently showed that the expression of the gene encoding M. truncatula SWEET1b transporter is strongly enhanced in arbuscule-containing cells compared to roots and localizes to the peri-arbuscule membrane.
A comprehensive view of symbiosis under environmental and nutritional stress is important in times of climate change and resource shortening, as AM symbiosis is a key component of nutrient uptake and exchange through their transport systems. However, despite accumulating knowledge about transporter expression and metabolic activity in AM symbiosis, we still lack a precise understanding about their regulation and their importance for metabolism. Here, our objectives were to define the effects of P availability on two plants connected through a mycorrhizal network. Therefore, we analyzed the effects of mycorrhization and contrasting P nutrition on the transporter expression and metabolite accumulation in Populus trichocarpa (poplar) and Sorghum bicolor (sorghum) when colonized by the AM fungus R. irregularis. Our main focus was on the regulatory role of the mycorrhization and P nutrition on the expression of the Pht1 PTs in the plants and in the AM fungus. Further we assessed the effect of the applied conditions on AMTs and carbohydrate transporters. In the AM fungus R. irregularis, we determined expression of PTs, AMTs, MST, and fatty acid metabolism in the ERM and in the IRM of colonized poplar and sorghum roots. We identified new specific mycorrhiza-inducible PTs and AMTs in poplar and sorghum. Moreover, our data allowed us to gain further insight into symbiotic carbon exchange.
Material and Methods
Experimental Set-Up
Experiments were performed with P. trichocarpa cuttings (clone 10174, Orléans, France) and S. bicolor (L.) Moench, cv Pant-5. Sorghum seeds were kindly provided by Indian Grassland and Fodder Research Institute (Chaudhary Charan Singh Agriculture University of Hissar, Haryana, India) and Govind Ballabh Pant University of Agriculture and Technology (Pantanagar, Uttaranchal, India). Seeds were surface-sterilized in 2.5% KClO for 10 min, rinsed several times with sterile deionized water and soaked overnight in sterile deionized water. Seeds were germinated in the dark at 25°C for 3 days. Plants were inoculated with 1 ml liquid inoculum of R. irregularis, isolate BEG75 (Inoculum Plus, Dijon, France), in 0.01 M citrate buffer (pH 6) with about 110 spores/ml. The microcosms were set up in tripartite compartments (mycorrhizal treatment) or single compartments (non-mycorrhizal treatments). Compartments were filled with an autoclaved (120°C, 20 min) quartz sand (Alsace, Kaltenhouse, Trafor AG, Basel): zeolite (Symbion, Czech Republic) substrate (1:1, w:w). In the tripartite compartment system poplar cuttings were planted in the left compartment and sorghum seedlings in the right compartment. Both plants were inoculated with R. irregularis to create a common mycorrhizal network and to increase poplar root colonization (Figure S1). Compartments were separated by two 21 µm meshes and one 3 mm mesh, to allow the AM fungus to grow from one compartment to the other but to avoid plant roots protruding the neighboring compartment. As control, non-inoculated poplar and sorghum plantlets grew in single compartments receiving the Pi containing fertilizer treatments directly to their roots. Plants were fertilized once a week with 10 ml Hoagland solution without P, until all plants showed signs of P depletion, indicated by anthocyan accumulation. From the 22nd week high-P (560 µM) or low-P (28 µM) containing Hoagland solution was applied to the first compartment for 9 weeks, to obtain ERM and to ensure that P was delivered via the mycorrhizal uptake pathway. Control plants received fertilizer treatment directly to their root systems.
Harvest
The ERM was harvested by dispersing the substrate with tap water and fishing it from the surface using a 32 µM mesh. These steps were repeated several times. Afterwards the cleaned ERM samples were snap frozen in liquid N and stored at -80°C.
For RNA extractions, two leaves from the top of Poplar plants and two young leaves of Sorghum plants were snap frozen in liquid N and stored at -80°C. The rest of the shoots was harvested and dried in an oven at 55°C for 4 days for total P measurement.
Roots were removed from substrate under tap water and cut into ∼1 cm small pieces. Two subsamples of about 100 mg were immediately frozen in liquid N and stored at -80°C. One subsample of about 100 mg was taken for root colonization measurements. The remaining roots were placed in a paper bag and dried at 55°C for 3.5 days for determination of total P and N content.
Colonization Measurements and P Extraction
Roots were immersed in 10% KOH and stored at 4°C overnight. The next day, the roots were rinsed with tap water and immersed in 2% HCl for 1 h at room temperature. Then, the roots were rinsed with tap water, immersed in 0.005% trypan blue (w:v in lactic-acid: glycerol: water, 1:1:1, v:v:v) and stored at 4°C o/n. The next day, the roots were rinsed and immersed in lactic-acid glycerol water (1:1:1, v:v:v) for destaining (Brundrett et al., 1984). Total colonization count was performed using the magnified intersection method (McGonigle et al., 1990). Differences between means of variables were assessed by t-test (p < 0.05), using Microsoft Excel 2010.
For determination of P concentration in the plants, dried root and shoot samples of six biological replicates were ground using a ball mill. Up to 500 mg were used for the modified P extraction method by Murphy and Riley (1962).
RNA Extraction
Total RNA was extracted from six biological replicates per plant species and mycelium, respectively. Total RNA was extracted from lyophilized extraradical mycelia, root and leaf samples using the RNeasy Plant Mini Kit (Qiagen, Courtaboeuf, France). RNA extracts were DNase treated with the DNA-free™ Kit, DNase Treatment and Removal Reagents (AMBION® by life technologies). Total RNA was quantified with the Qbit RNA BR Assay kit and purity was estimated using the Nanodrop (ND-1000, Witec, Switzerland).
Reverse Transcription and qRT-PCR
cDNAs from three biological replicates were obtained using the iScript™ cDNA Synthesis Kit (BIO RAD Laboratories, Paolo Alto, CA, United States), using 200 ng of total RNA per reaction, using same RNA extracts as for mRNA sequencing. For quantification a two-step quantitative RT-PCR approach was used. Gene specific primers were designed in Primer 3 (http://frodo.wi.mit.edu/cgi-bin/primer3/primer3_www.cgi) and tested as well in amplify 3.1 (http://engels.genetics.wisc.edu/amplify). Target gene expressions were normalized to the expression of the reference gene ubiquitin in Poplar (Potri.015G013600) and Sorghum (Sb10g026870) and translation elongation factor in R. irregularis, respectively. All primers used are listed in Table S1. qRT-PCRs were run in a 7500 real-time PCR system (Roche) using the following settings: 95°C for 3 min and then 40 cycles of 95°C for 30 s, 60°C for 1 min and 72°C for 30 s. There were three biological and three technical replicates per treatment. Differences in gene expression between applied conditions were tested by a one-way ANOVA using SPSS Statistics, version 22 (IBM, Chicago, USA).
RNA Sequencing and Data Analysis
Total RNA sequencing was done for three biological replicates per condition. Eighteen libraries were prepared and paired-end Illumina HiSeq mRNA sequencing (2x100bp RNA-Seq) was performed by Beckman Coulter Genomics France (Grenoble, France), which produced around 2 × 80 million reads per library in average. After quality check using FastQC, adaptor sequences were removed using FASTX-Toolkit. Only inserts of at least 30-nt were conserved for further analysis. Reads were mapped with CLC Genomics Workbench 11 (CLC Bio workbench, Qiagen, Aarhus, Denmark) using manufacturer’s recommendations on Poplar genome and gene annotation Ptrichocarpa_210_v3.0 (Tuskan et al., 2006), on Sorghum genome and gene annotation Sbicolor_313_v3.1 (McCormick et al., 2018), and on R. irregularis gene annotation Rhiir2-1 (Morin et al., 2019). The mapped reads for each transcript were calculated and normalized as RPKM for calculating gene expression (reads per kilobase of transcripts per million reads mapped—Mortazavi et al., 2008). Intact and broken pairs were both counted as one. The RPKMs of each transcript in different conditions were compared using proportion-based test statistics (Baggerly et al., 2003) implemented in CLC genomic Workbench suite. This beta-binomial test compares the proportions of counts in a group of samples against those of another group of samples. Different weights are given to the samples, depending on their sizes (total counts). The weights are obtained by assuming a Beta distribution on the proportions in a group, and estimating these, along with the proportion of a binomial distribution, by the method of moments. The result is a weighted t-type test statistic. We then calculated false discovery rate correction for multiple-hypothesis test (Benjamini and Hochberg, 1995). Only genes showing a difference of 10 reads between compared conditions were considered as significantly expressed. Genes were considered as differentially expressed when meeting the requirements of fold change ≥|2| and false discovery rate ≤ 0.05.The expression was then normalized using RPKM. Raw RNAseq data and global mapping data analyses were deposited at GEO (GSE138316).
Metabolite Profiling and Data Analysis
For extraction of soluble metabolites about 90 mg of deep frozen poplar root and ERM samples (three biological replicates per condition) were pulverized in liquid N. Metabolite profiling was performed as described in (Dethloff et al., 2014) by gas chromatography coupled to electron impact ionization/time-of-flight mass spectrometry (GC-EI/TOF-MS) using an Agilent 6890N24 gas chromatograph (Agilent Technologies, Böblingen, Germany; http://www.agilent.com). Guidelines for manually supervised metabolite identification were the presence of at least 3 specific mass fragments per compound and a retention index deviation < 1.0% (Strehmel et al., 2008). For quantification purposes all mass features were evaluated for best specific, selective and quantitative representation of observed analytes. Laboratory and reagent contaminations were evaluated and removed according to non-sample control experiments. Metabolites were routinely assessed by log2-transformed relative changes expressed as response ratios. Statistical testing, namely two-way analysis of variance (ANOVA) and Wilcoxon–Mann–Whitney testing of significance were performed using relative abundances or log2-transformed ratios. Statistical assessments and data visualizations were performed using the multi-experiment viewer software, MeV (Version 4.9; http://www.tm4.org/mev.html; Saeed et al., 2006) and the Microsoft-Excel 2010 program.
Results
Colonization, and N and P Measurements
AM colonization of roots was between 79 and 87% (poplar) and about 93% (sorghum) (Table S2). Non-AM plants were not colonized. The hyphal colonization and the percentage of vesicles were not significantly different between low-P and high-P treatments in sorghum and poplar plants. However, significantly, sorghum roots contained three times more arbuscules in the low-P treatment, indicating that P starvation supported mycorrhization.
P treatment had significant effects on P content in the shoots and roots of poplar and sorghum (Figure S2). High-P treatment on ERM increased P content in roots and shoots, except for the roots from non-AM sorghum plants. Under the same P supply conditions, non-AM poplar accumulated more P than AM poplar whereas in AM sorghum, P accumulation was comparable to non-AM plants.
P treatment had significant effects on N content in the shoots of poplar, but marginally on sorghum (Figure S2). High-P treatment increased N content in poplar shoots. Under the same P supply conditions, AM poplar accumulated more P than non-AM poplar whereas in AM sorghum, N accumulation was comparable to non-AM plants.
Patterns of Extraradical Mycelium and Intraradical Mycelium Expressomes in the Symbiocosm
Incidence of Phosphate Availability
The symbiotic part of the mycelium in roots (IRM) showed different gene expression patterns according to phosphate concentration and plant host. The total number of differentially expressed genes (DEG) differs around 10 fold between the two plants with 298 genes differentially regulated in S. bicolor plants against 3,162 in P. trichocarpa in response to phosphate treatment (Figure 1A). High phosphate concentration has a fewer incidence on the IRM transcriptome in S. bicolor than in P. trichocarpa whereas the fungus is connected to both plants, indicating that the allocation of mineral nutrients is more tightly regulated in P. trichocarpa than in S. bicolor. In both plants, the number of up regulated fungal genes is 10 times the number of down regulated fungal genes in high-P condition compared to low-P. This indicates that the fungus is metabolically more active in high-P condition, consistent with the fact that adding a high-P solution in the common mycorrhizal network compartment enhances the fungal-dependent Pi acquisition pathway. Only two fungal genes were significantly up regulated in both plants in response to high-P treatment and are involved in vesicular cargo. In Sorghum, the few up regulated genes were involved in vesicular trafficking, lipid binding, nitrate reduction and general transport. In P. trichocarpa, the genes up regulated by high-P belonged to vesicle metabolism, fatty acid metabolism and transport of N, Pi, sugars, and water. Interestingly, only one gene (a short chain dehydrogenase) was significantly up regulated in both plants and in the ERM in response to high-P treatment.
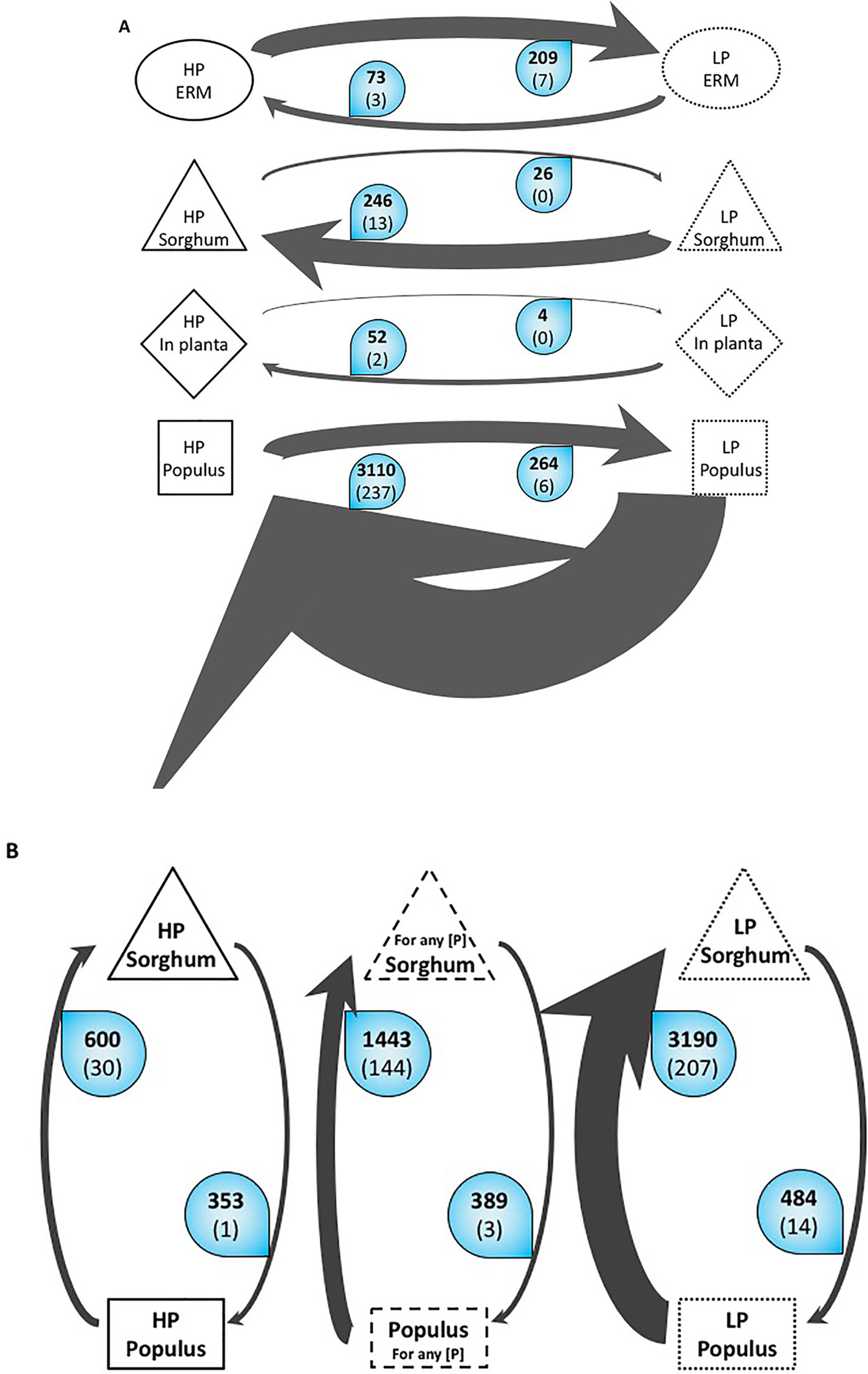
Figure 1 Overall variations of the number of Differentially Expressed Genes (DEG) of R. irregularis (A) Variations according to P. The arrows represent the number of differentially expressed genes in R. irregularis. Black bold numbers represent the number of genes with a fold change ≥2 and a false discovery rate ≤ 0.05. White numbers in brackets represent the number of genes involved in the transportome. The width of the arrow is proportional to the number of up-regulated genes. For example, the top arrow means that in the ERM grown in Low Phosphate, 209 genes are significantly up regulated at least two times compared to the ERM grown in High Phosphate. Among these 209 genes, seven are involved in the transportome. (B). Variations according to host plant. The arrows represent number of differentially expressed genes in R. irregularis between the two compared conditions. Black bold numbers represent the number of genes with a fold change ≥2 and a false discovery rate ≤ 0.05. White numbers in brackets represent the number of genes involved in the transportome. The width of the arrow is proportional to the number of up-regulated genes. ERM, extraradical mycelium; HP, high phosphate; LP, low phosphate; in planta, intraradical mycelium of both sorghum and poplar.
It is noteworthy that on the one hand, while cultivated in high-P, 3,408 genes were overexpressed in IRM (either in S. bicolor, or in P. trichocarpa or in both host plants) against 73 in the ERM compartment. On the other hand, while grown in low-P, the number of up regulated genes in planta dropped to 294 against 209 in the ERM. These overall expressome patterns suggest that when Pi is abundant in soil, the fungus invests little energy in recruiting the Pi but is highly active in exchanging this nutrient with the plants. On the opposite, when Pi is scarce in the medium, the extra radicle mycelium has to put a lot of energy in recovering the mineral in the soil but has fewer nutrients to exchange with the plants.
Incidence of Host Plant
We correlated the difference of DEG in the IRM in the two host plants connected by the same fungus at a given time point to the amount of nutrient resources that the fungus allocates to the two plants. Even though AM fungus has a very broad host range, their expressome differs according to host independently to P concentration (Figure 1B). In poplar, only 389 DEG were found among which only three are involved in the transportome (one vesicle transport and two transporters). In S. bicolor, a set of 1,443 fungal genes were found up-regulated, indicating active exchanges. A tenth of them (144) is involved in the transportome, representing all metabolisms (fatty acids, N, phosphate, sugars, potassium, general transporters, water, and vesicle transport). This difference indicates that AM symbiosis is more active and more tightly regulated in sorghum than in poplar (Figure 1B). The total P concentrations measured in the two mycorrhizal plants is consistent with this observation as more P was accumulated in mycorrhizal sorghum than in mycorrhizal poplar under low P fertilization (Figure S2).
In the mesocosm grown in low-P, the fungus does not allocate nutrients equally between the different host plants. The number of genes up regulated in S. bicolor is 6.5 times the number of genes overexpressed in P. trichocarpa (3,190 and 484 respectively). Not all these genes are supposed to be involved in trophic exchanges but the genes involved in transportome between the two plants showed a similar pattern. In S. bicolor, 207 fungal genes were up regulated, corresponding to all the different metabolisms and transporters we investigated (fatty acids, N, phosphate, sugars, transporters, water and vesicle transport, with the exception of potassium). In P. trichocarpa however, only 14 genes belonged to our transportome list and were involved in sugar transport, vesicle trafficking, and general transporters. In low-P condition, the AMF seems to favor the exchanges with the Sorghum at the expense of the Poplar. This observation correlates with the total phosphate measured in the two plants (Figure S2).
Under high-P, the difference in gene expression is lower than in low-P. We observe only a 2-fold difference between the number of over expressed genes in S. bicolor and P. trichocarpa (600 and 353 respectively). Moreover, the number of genes involved in transportome is much lower. Only one gene involved in vesicular trafficking is specifically expressed in P. trichocarpa. In S. bicolor, 30 genes involved in fatty acid metabolism, sugar transport, vesicle transport and general transport were recovered. The high-P makes it easily recoverable by the AM fungus and improves symbiotic exchanges with both plants, thus minimizing the preference for S. bicolor over P. trichocarpa. This balanced fungal transcriptome is supported by plant P concentration as the measurements in the two plants are very close, with a slight abundance in poplar.
Regulation of Phosphate Transporter Expression
Gene Expression of Plant Pht1 Transporters
Using qRT-PCR, we measured the expression of the twelve Pht1 PT in roots and shoots of AM and non-AM poplar plants grown in high-P and low-P conditions. PtPT8 and PtPT10 were induced in AM-roots only, which implies an important role of these two transporters in symbiotic Pi uptake at the periarbuscular space (Figure S3).
Low-P treatment and mycorrhization induced expression of PtPT1.1, PtPT1.2, PtPT1.4, PtPhT1.7, and PtPT1.11 in roots (Figure S3B). PtPT1.2, PtPT1.4 and PtPT1.11 were strongly induced in shoots, suggesting that these transporters are involved in intercellular Pi transfer and Pi transport over long distances, respectively (Figure S3A). PtPT1.3 and PtPT1.6 were neither expressed in roots nor in shoots. mRNA-Seq analysis independently confirmed our results for Pht1 expression (Figure S4).
In sorghum, SbPT1.8 and SbPT1.10 were induced in AM roots like PtPT1.8 and PtPT1.10 (Figure S5). SbPT1.1, SbPT1.2, SbPT1.4, SbPT1.6, and SbPT1.7 were significantly induced in the non-AM low-P treatment. It seems that mycorrhization complemented sufficiently the P deficiency by increased Pi transfer to its host plant (Figure S2). In comparison to poplar Pht1, sorghum Pht1 was more susceptible to mycorrhization than to Pi concentration.
Gene Expression of Mycorrhizal Phosphate Transporters
In mycorrhizal poplar plants, qRT-PCR analysis of PT in the AM fungus R. irregularis in the ERM and IRM revealed expression of RiPT1, RiPT3, RiPT5, and RiPT7, with highest expression values for RiPT1 (Figure 2A). RiPT1 was significantly more expressed in the IRM compared to the ERM in low-P treatment. RiPT1 expression was significantly higher under low-P treatment in the ERM and the IRM compared to high-P treatment. RiPT7 tended to be highly expressed in the IRM compared to the ERM and RiPT3 was lowly expressed in the ERM and induced in high-P treatment in the IRM. In Sorghum, we observed similar expression patterns except for RiPT3 and RiPT7, significantly induced in the IRM than in the ERM (Figure 2B).
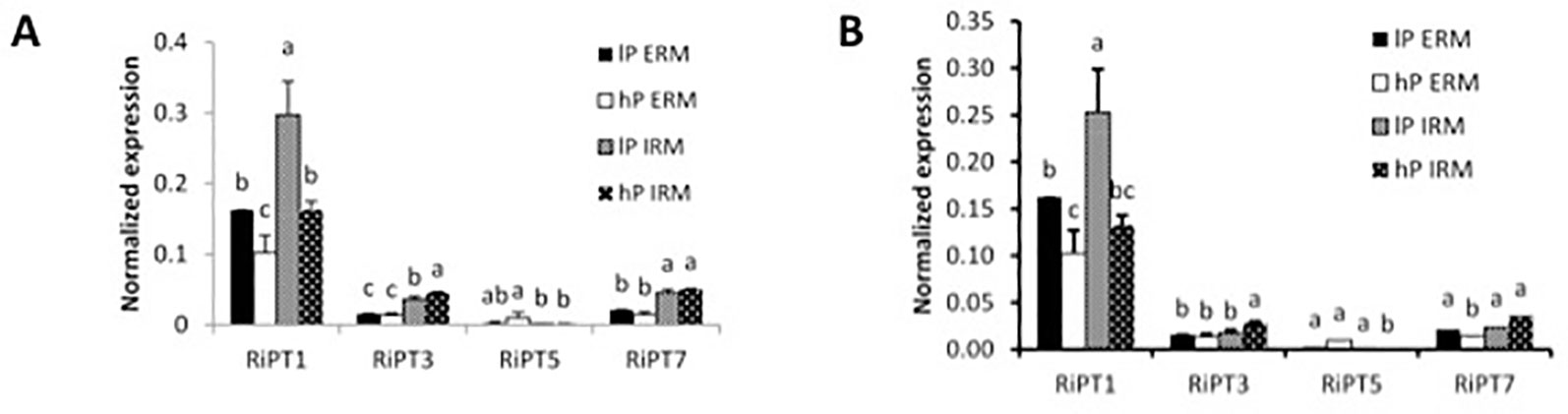
Figure 2 Quantification by qRT-PCR of the transcript abundances of phosphate transporters in R. irregularis when associated with P. trichocarpa (A) and S. bicolor (B). Quantification of transcript levels of transporters in the ERM and in the IRM of the host plant under high (hP) and low (lP) availability of P. Values are means of three biological and three technical replicates. Error bars represent the SE. Translational elongation factor was used as a reference transcript. Statistical analysis was performed by analysis of variance for each gene, followed by Tuckey honest significant difference. Lower case letters indicate significant difference(Tukey’s t-test; p < 0.05).
Regulation of Transporters Involved in Nitrogen Exchange
Gene Expression of Plant Ammonium Transporters
As N is a major component of AM symbiosis, transcript abundances of AMTs in poplar were analyzed (Figure S6). The expression of three of them (PtAMT1.1, PtAMT1.2, and PtAMT3.1) was dependent of either mycorrhizal symbiosis of P-treatment or both. We then measured their expression in poplar by qRT-PCR. While PtAMT1.1 and PtAMT1.2 were described as induced in poplar upon mycorrhization with the ectomycorrhizal fungi Paxillus involutus (Couturier et al., 2007) and Amanita muscaria (Selle et al., 2005), no previous expression data were available for PtAMT3.1. Interestingly, PtAMT3.1 clustered next to the three AM-inducible transporters GmAMT3.1 (Kobae et al., 2010), SbAMT3.1 (Koegel et al., 2013), and OsAMT3.1 (Pérez-Tienda et al., 2014) (Figure S6). Here, PtAMT1.1 was induced in the AM low-P treatment and the PtAMT1.2 and PtAMT3.1 were induced in the AM treatments (Figure 3). In addition, PtAMT1.2 and PtAMT3.1 were even higher expressed in the high-P condition, suggesting that both AMTs play a major role in symbiotic N transfer. Higher expression of these transporters under AM high-P condition might point to an increased N transfer when the AM fungus has access to more Pi. In shoots, we PtAMT1.1 has a similar expression pattern as in roots. PtAMT3.1 was only marginally expressed and PtAMT1.2 was not expressed in leaves (Figure 3). mRNA-Seq confirmed our observations for AMT expression levels in the roots of Poplar (Figure S6). In a previous transcriptome study (Calabrese et al., 2017), in which AM poplar plants were exposed to N deficiency, PtAMT4.1, PtAMT4.2, and PtAMT4.3 were AM-induced. Consistent with this previous study, we observed a specific induction of PtAMT2.2, PtAMT4.1, PtAMT4.2, and PtAMT4.3 upon mycorrhization even though these transporters were expressed at lower levels compared to PtAMT1.2 and PtAMT3.1.
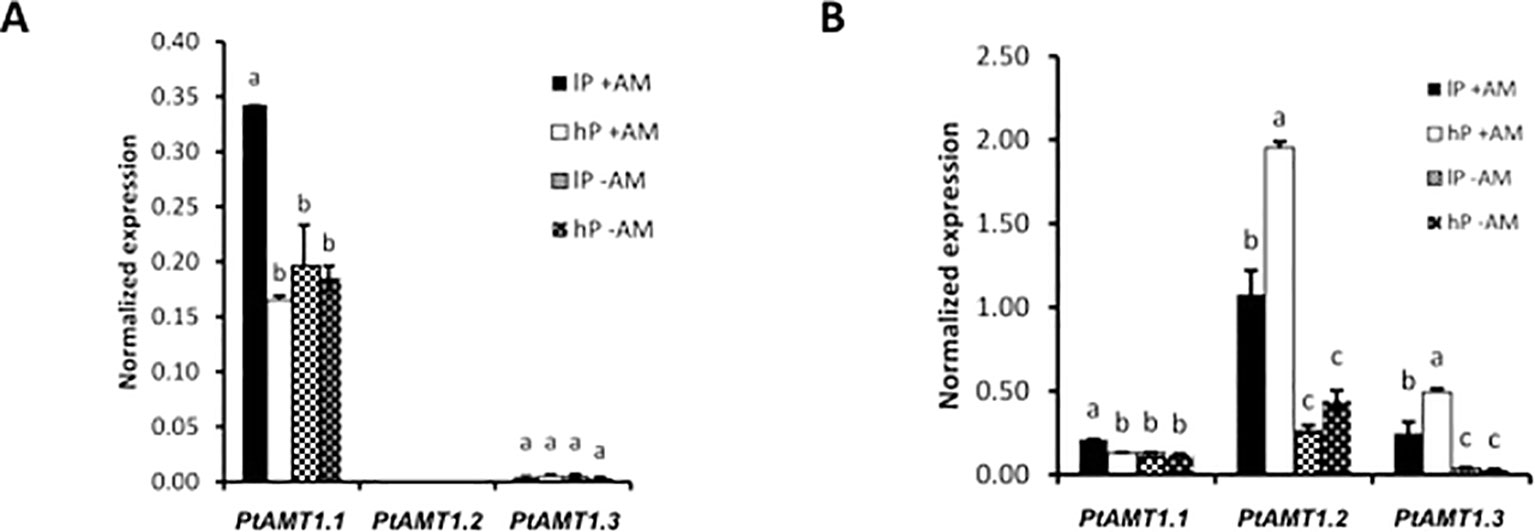
Figure 3 Quantification by qRT-PCR of the transcript abundances of the three ammonium transporters of poplar PtAMT1.1, PtAMT1.2, and PtAMT3.1 in the shoot (A) and root (B) of mycorrhized (+AM) and non-mycorrhized (-AM) P. trichocarpa under low P (lP) and high P (hP) condition. Values are the means of three biological replicates and three technical replicates, each. Error bars represent the SE. Ubiquitin was used as reference transcript. Statistical analysis was performed by analysis of variance (ANOVA) per gene, followed by Tuckey honest significant difference test (Tuckey HSD; p < 0.05). Lower case letters indicate significant difference (Tukey’s t-test; p < 0.05).
In Sorghum, SbAMT3.1 was specifically induced in AM-roots, and SbAMT1.1 and SbAMT1.2 were induced in the non-AM low-P treatment (Figure S7). However, SbAMT1.1 and SbAMT1.2 were nearly twice more expressed in shoots compared to roots.
Gene Expression Ammonium Transporters in Rhizophagus Irregularis
Quantitative expression analysis of the three AMTs in the AM fungus revealed that their expression is not significantly different in the ERM between the low-P and high-P treatments. The expression of GintAMT3 was significantly higher in the IRM compared to the ERM (Calabrese et al., 2017). GintAMT2 and GintAMT1 were equally expressed in the ERM and IRM in poplar and sorghum (Figure 4). GintAMT2 was significantly highly expressed in the IRM of sorghum compared to poplar. Specific induction of GintAMT3 in the IRM might indicate a possible localization of the transporter at the arbuscular side for the transfer of ammonium to the periarbuscular side to enable ammonium uptake for the plant.
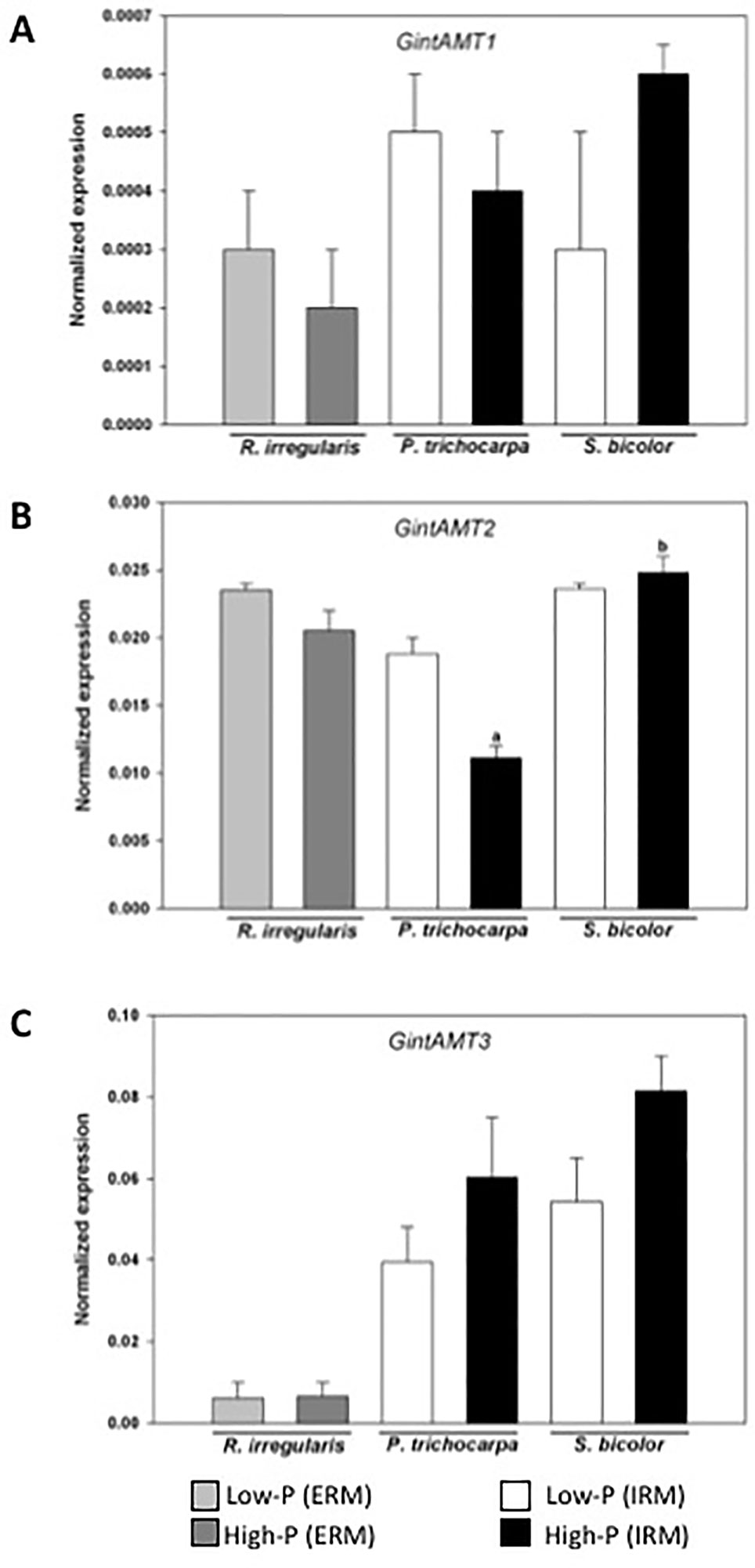
Figure 4 Quantification by qRT-PCR of the transcript abundances of ammonium transporters in R. irregularis. Transcript abundances were measured under low-P and high-P availability. Transcript abundances of the three transporters GintAMT1 (A), GintAMT2 (B), and GintAM3 (C) were measured in the extra-radical mycelium (ERM) and in the intraradical mycelium (IRM) when associated to the host plants poplar and sorghum. Values are means of three biological and three technical replicates. Translational elongation factor was used as a reference transcript. Statistical analysis was performed by analysis of variance for each gene, followed by Tuckey honest significant difference test (Tuckey HSD; p < 0.05). Lower case letters indicate significant difference(Tukey’s t-test; p < 0.05).
Gene Expression of Amino Acid and H+/Oligopeptide Transporters
In our study, we identified several amino acid transporters and H+/oligopeptide symporters that were either specifically induced or repressed upon mycorrhization (Table S3). Here, root colonization highly induced expression of two H+/oligopeptide transporters. Potri.005G233500, a homologous gene of AtPTR1 shown to transport di-/tripeptides with low selectivity in Arabidopsis thaliana, was highly induced. AtPTR1 is situated in the plasma membrane of vascular tissue which indicates a role in long-distance transport (Dietrich et al., 2004). AtPTR3, a homologue of Potri.002G258900, was induced upon salt stress and was shown to be regulated by methyl jasmonate, salicylic acid and abscisic acid. Further AtPTR3 was induced upon inoculation of the plant with pathogens. A reduced activation of AtPTR3 in hrpA mutant indicated that it is a defense related gene protecting the plant against abiotic and biotic stress (Karim et al., 2005; Karim et al., 2006). The differential expression of these transporters upon mycorrhization may suggest a role of the transporters in N uptake but also a role in AM root colonization.
Gene Expression Related to Carbon Exchange
Gene Expression of Sugar Transporters
Quantitative expression analysis using qRT-PCR of five SUT (SUT1 and SUT3 to SUT6) in poplar revealed that all were expressed (Figures S8 and S9). SUT1 was only marginally expressed while SUT4 was strongly expressed in roots and shoots. While SUT1 and SUT4 were not differentially expressed upon mycorrhization, surprisingly, SUT3 was down-regulated upon mycorrhization in roots and shoots of poplar. Interestingly, SUT6 was down-regulated in shoots by mycorrhization. In sorghum, SUT1 was also down-regulated (Figure S10). In Poplar, we screened our transcriptome data set for other carbohydrate transporters. We found three carbohydrate transporters induced and four repressed upon mycorrhization (Table S3). Among them, only the transporter/spinster transmembrane protein Potri.001G286600 presents characteristics for plasma membrane localization. The UDP-galactose transporter related proteins are localized at the lumen of the Golgi cisternae (Norambuena et al., 2002). On the fungal side, the MST GintMST2 was specifically induced in the IRM of mycorrhizal poplar and sorghum and its expression was not affected by P concentration (Figure 5). The induction of GintMST2 gene expression suggested a role in symbiotic carbohydrate transfer but functional properties still need to be determined. The down-regulation of plant MSTs expression in mycorrhizal conditions whereas the fungal GintMST2 is still expressed in the IRM indicate that the AM fungus obtains the sugar from the intercellular space without the cooperation of the plant itself, turning the AM root into a sink for sugars.
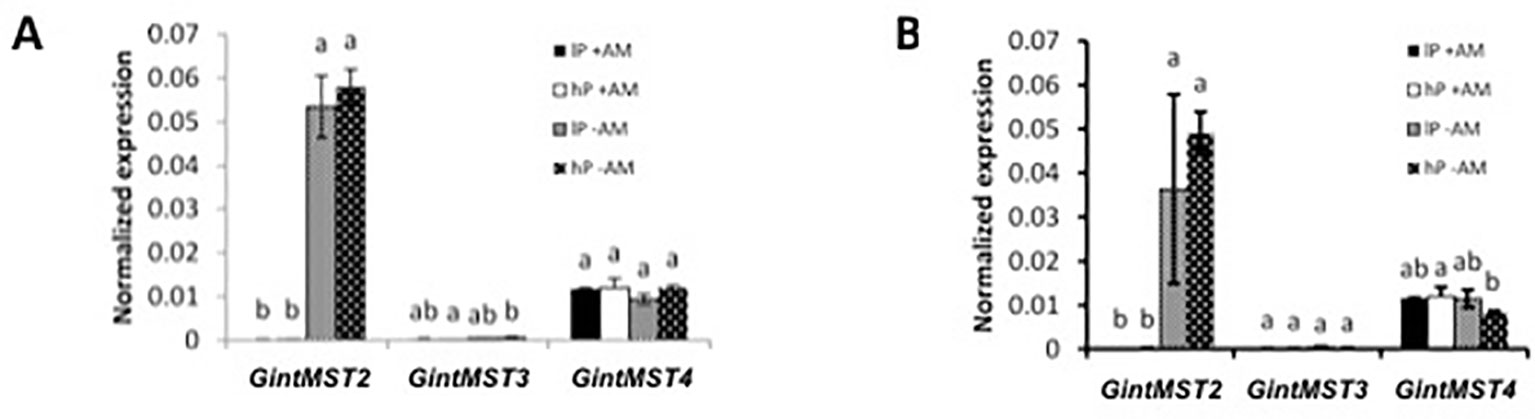
Figure 5 Quantification by qRT-PCR of the transcript abundances of monosaccharide transporters (MST) in R. irregularis when associated with P. trichocarpa (A) and S. bicolor (B). Quantification of transcript levels of transporters in the ERM and in the IRM of the host plant under high (hP) and low (lP) availability of P. Values are means of three biological and three technical replicates. Error bars represent the SE. Translational elongation factor was used as a reference transcript. Statistical analysis was performed by analysis of variance for each gene, followed by Tuckey honest significant difference. Lower case letters indicate significant difference(Tukey’s t-test; p < 0.05).
Expression of Fatty Acid Genes
Expression of different genes involved in fatty acid synthesis and transfer were screened. The expression of these genes is mandatory in order to accommodate the AM fungus and allow the formation of the arbuscules. Ram2, FatM, and STR1 were specifically expressed in mycorrhizal Sorghum and STR2, CCaMK, RAD1, Cyclops, and Castor/pollux were upregulated when the plant was in symbiosis (Table S4). These genes followed the same pattern of expression in poplar either in low-P or in high-P condition.
On the fungal side, no fatty acid transporter has been identified yet, suggesting another form of acquisition. However, many genes involved in fatty acid metabolism were overexpressed in the different plants. Multiple lipases and lipid recognition proteins are over expressed in planta compared to the ERM (Table S5). These might play a role both in managing the TAG stock or acquiring fatty acids from the host plant. Three desaturases and one elongase show a slight increase in expression in planta compared to ex planta. Pi addition had little effect on the ERM but increased the expression of several lipases in planta.
Primary Metabolism of Poplar and Sorghum Roots, and the ERM of R. Irregularis
To gain further insights into the changes of root primary metabolism caused by the interaction between poplar, sorghum and R. irregularis we conducted GC-MS metabolite profiling experiments. Under our experimental conditions, the ERM metabolite profile of R. irregularis was not significantly affected by P conditions (Tables 1 and S6). In the ERM, we observed slight but mostly non-significant increases of organic acids, glucose, trehalose, glycine and of amino acids with branched aliphatic side chains, with leucine as the only significant increase in our experiment (Tables 1 and S6).
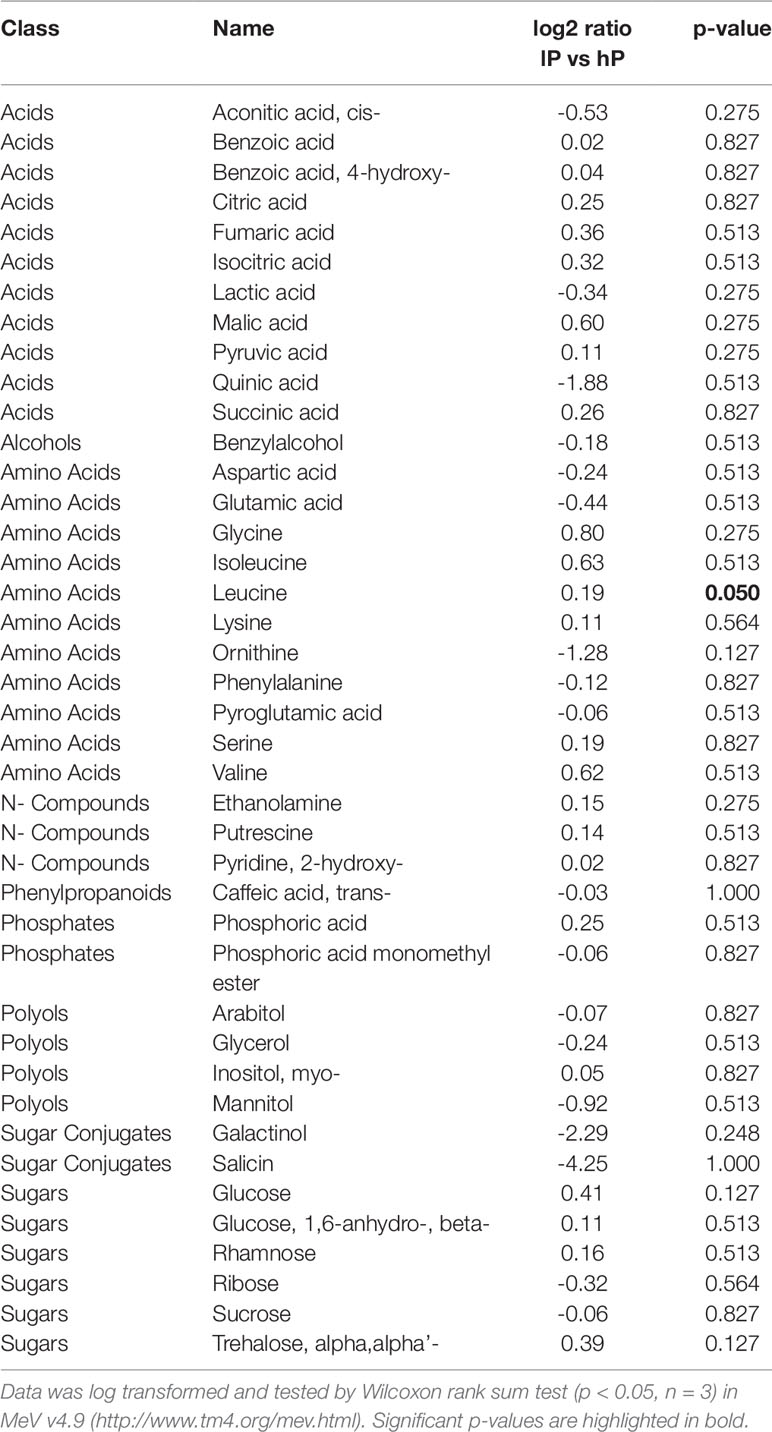
Table 1 Relative abundances of metabolites detected in the ERM of R. irregularis in response to low (lP) and high P (hP) concentrations.
In contrast, non-AM low-P treatment on poplar roots increased general organic acid and amino acid pools and coincidently decreased pools of glucose-6-phosphate or fructose-6-phosphate (Tables 2 and S7). Analysis of variance indicated significant general accumulation of only few metabolites, i.e., 4-amino-butanoic acid (GABA), isoleucine, phenylalanine, serine, threonic acid, ribonic acid, and arabinonic acid-1,4-lactone independently of the mycorrhizal status (Tables 2 and S7). AM colonization enhanced nutrient acquisition in the low-P treatment (Tables 2 and S7). In addition, mycorrhization modified the root primary metabolism under low-P and high-P and caused a general decrease of the metabolite pools. Thirty-eight out of 79 monitored primary metabolite pools were decreased with only one exception besides few still non-identified metabolites, namely trehalose (Tables 2 and S7). Trehalose is a major storage carbohydrate of AM fungi (Bécard et al., 1991). Mycorrhization not only decreased the main organic acids of the TCA cycle, e.g., malic acid, aconitic acid, 2-oxo-glutaric acid, succinic acid, and fumaric acid, but also many amino acids including aspartic and glutamic acid, as well as phenylalanine, glycine, serine, leucine, isoleucine and valine. In addition, mycorrhization decreased the glucose-6-phosphate, fructose-6-phosphate, myo-inositol, and galactinol pools and additional carbohydrates including maltose. For sorghum, the effect of P treatment on AM colonized plants was studied only. Compared to poplar, AM sorghum plants are slightly affected by the low-P treatment; we observed only a slight significant decrease of fructose-6-phosphate, glucose-6-phosphate, and phosphoric acid under low-P treatment (Table 3).
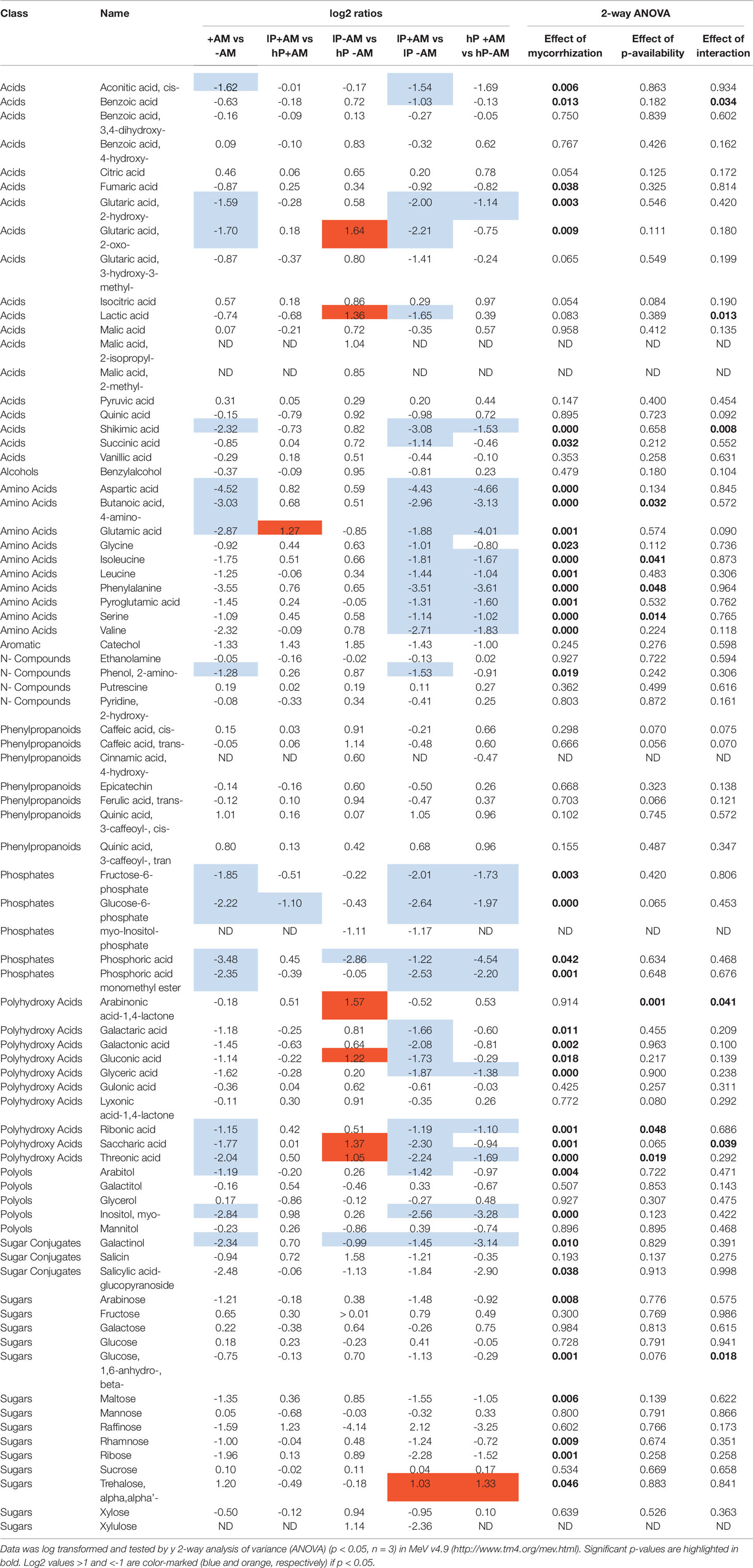
Table 2 Relative abundances of metabolites detected in poplar roots. Abundances were measured in the mycorrhized (+AM) and non-mycorrhized (-AM) poplar roots under high (hP) and low (lP) P availability.
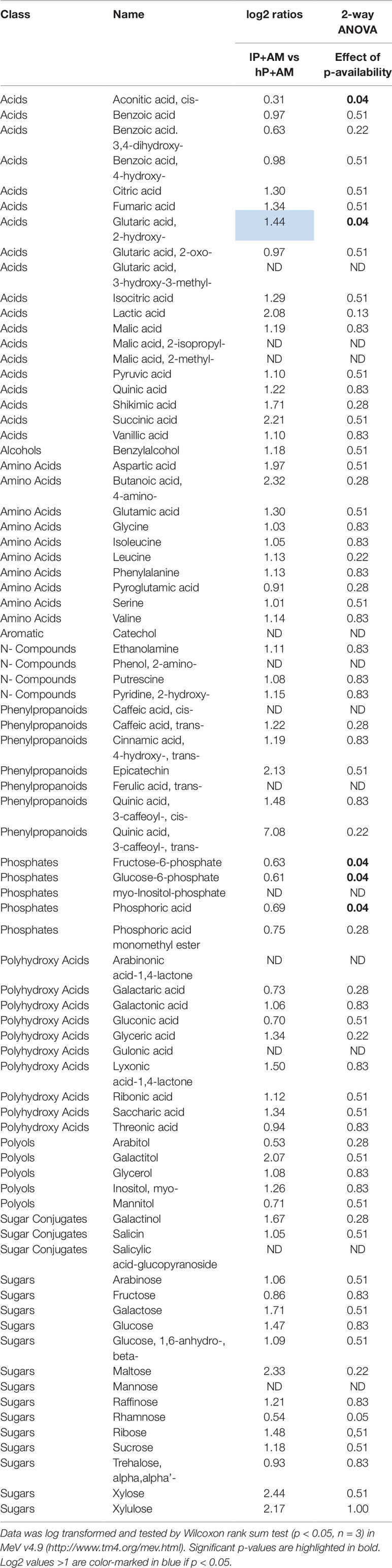
Table 3 Relative abundances of metabolites detected in sorghum roots. Abundances were measured in the mycorrhized (+AM) sorghum roots under high (hP) and low (lP) P availability.
Discussion
In this study, we described the effects of P supply and AM colonization on PTs, AMTs, carbohydrate transporters and lipid genes in poplar and sorghum, and in the ERM and IRM of the AM fungus R. irregularis.
Differential Resource Allocation in the Common Mycorrhizal Network
In the symbiocosm, we observed disequilibrium in fungal DEG patterns according to sorghum and poplar symbiotic tissues in response to high-P concentration supplied to ERM. Sorghum is an annual grass with a C4 metabolism, and poplar a perennial tree with a C3 metabolism. The fungal transportome in the fast growing Poaceae is always more important than in poplar in any given P concentration. It has been shown that the fungus is able to adapt its resource allocation to the best symbiont available (Fellbaum et al., 2014; Whiteside et al., 2019). Our data provide same conclusion at the transcriptomic level. However, we have no information about the extent of the ERM developed from roots of each of the two host plants, so differences observed between the two hosts may depend also on a differential ability of the symbiont to form functional ERM. Anyway, we could hypothesize that the perennial poplar plant is less mycorrhiza-dependent than the annual sorghum.
Symbiotic Phosphorus Exchange
In our study, we confirmed the expression of PtPT1.10 in Poplar AM roots only (Figure S3) as already reported (Loth-Pereda et al., 2011). In addition, PtPT1.8 was also expressed in AM roots only. In sorghum roots, AM colonization induced the specific expression of SbPT1.8, SbPT1.10 and partially of SbPT1.11 (Figure S5). The specific induction of PtPT1.8 and PtPT1.10 in poplar and of SbPT1.8, SbPT1.10 in sorghum upon mycorrhization strongly suggested that there is a symbiosis-dependent Pi uptake system. In M. truncatula, it has been shown that MtPT4 is specifically expressed in the periarbuscular membrane (Harrison et al., 2002). The specific induction of PtPT1.8 and PtPT1.10 in poplar and of SbPT1.8, SbPT1.10 in sorghum suggested that these transporters are localized at the periarbuscular membrane (Figure 6).
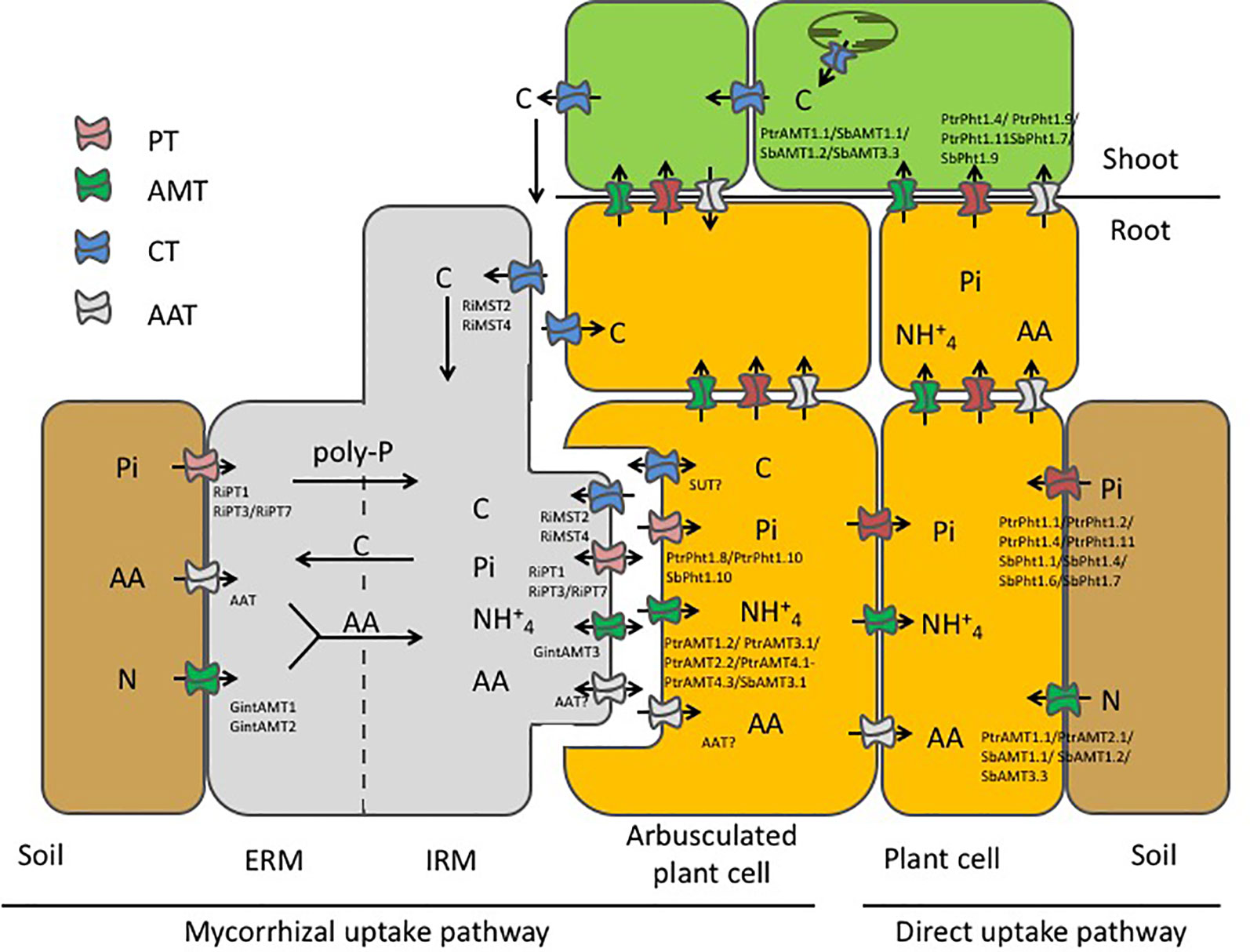
Figure 6 Schematic representation of the mycorrhizal nutrient uptake pathway and the direct nutrient uptake pathway in our model systems poplar and sorghum when colonized by R. irregularis. In the direct uptake pathway (right hand side) nutrients, i.e. inorganic phosphate (Pi) and nitrogen (N) are taken up from the rhizosphere by phosphate transporters (PT) and ammonium transporters (AMT) and are transported to the shoot. In symbiotic interaction, the AMF partially takes over nutrition of the plant. Nutrients are taken up by specialized transporters in the extraradical mycelium (ERM) and are further transported to the intraradical mycelium (IRM) where they are transferred to the periarbuscular space, to be taken up by plant transporters. N has been suggested to be additionally taken up from the soil in form of amino acids (AA) by predicted amino acid transporters (AAT). In exchange for the transfer of the mineral nutrients the mycorrhizal fungus is rewarded with essential carbohydrates from the plant. As some transporters were specifically induced by mycorrhization a possible localization at the periarbuscular membrane was assumed for the plant transporters. High induction of mycorrhizal transporters in the IRM compared to the extraradical mycelium ERM suggest that these transporters are mainly involved in nutrient exchange at the symbiotic interface. The reality is probably much more complex, with reuptake of nutrients (double-headed arrow) at the biotrophic interface, allowing both partners to, at least partially, control the exchanges.
On the fungal side, the PTs of R. irregularis were expressed in the ERM and in the IRM of poplar and sorghum roots. The high affinity transporter RiPT1 was previously found to be regulated in the ERM by external Pi concentration (Maldonado-Mendoza et al., 2001) and expressed at the arbuscular side (Fiorilli et al., 2013). Here, RiPT1 was up-regulated by external low-P concentrations in the ERM but also in the IRM. High expression of RiPT1 suggests that RiPT1 is the main transporter for Pi uptake and symbiotic Pi transfer in our conditions. Maldonado-Mendoza et al. (2001) predicted the existence of other PTs operating at high external P concentrations. Indeed, RiPT3 and RiPT7 were expressed but not affected by the nutrient conditions in the ERM. It might be that higher Pi concentrations are needed to increase expression of these possible high affinity transporters. Clearly, RiPT3 and RiPT7 were induced in the IRM with sorghum, with a similar tendency in poplar, suggesting that they participate also in Pi transfer/exchanges in the symbiosis.
P-Dependent Regulation of Phosphate Transporter Expression
Low-P conditions induced expression of PtPT1.1, PtPT1.2, PtPT1.4, and PtPT1.11 in poplar roots, showing that expression and regulation of PTs is dependent on Pi availability (Figure S3). In the mycorrhizal microcosm, the only Pi source was the AM fungal symbiont, and the absence of a direct Pi source further increased expression of these four transporters. As we still observed a Pi-dependent regulation of transporter expression, our data suggest that these PTs are regulated by external and internal Pi-concentrations and are regulated independently by the mycorrhizal pathway. Increased expression of PTs in low-P condition further suggests that the AM fungus supplies the host plant with more Pi if the fungus itself has increased access to Pi. By regulating these PTs independently poplar ensures a P nutrition uncoupled from the AM symbiont. Symbiosis-independent P-nutrition is necessary for perennial plants as mycorrhizal abundance varies in nature with the seasons (Courty et al., 2008; Dumbrell et al., 2011).
The fact that PtPT1.2, PtPT1.4, and PtPT1.11 expression was also induced upon P-limiting conditions in the shoots suggests that they function in Pi uptake at the root-soil interface as well as in intercellular distribution and translocation of Pi from root to shoot. PtPT1.9 was mainly expressed in the shoot, which suggests that it is mainly responsible for Pi allocation in the shoots.
Sorghum on the other hand turned out to be more mycorrhiza-dependent than poplar. Under mycorrhization PTs were equally low expressed as under a non-mycorrhizal high-P condition, indicating that the AM fungus was able to cover the Pi needs of sorghum. Induction of PT in the shoots in the non-mycorrhizal low-P condition further showed that the plant suffered of P deficiency and therefore probably reallocated Pi from old leaves (source) to young leaves (sink). The stronger dependency of sorghum to the AM fungus was also indicated by Pi-accumulation in mycorrhizal and non-mycorrhizal sorghum (Figure S2).
Symbiotic Nitrogen Exchange
As symbiotic N transfer is also an important aspect of AM symbiosis we analyzed the effects of mycorrhization and Pi availability on plant and AM fungal AMT expression (Figure 6). P-content has a stronger effect on poplar N contain than mycorrhization. Sorghum N nutrition is not affected by Pi availability. In poplar, mycorrhization induced expression of three AMTs. Our results are supported by previous studies which showed that PtAMT1.1 and PtAMT1.2 were mycorrhiza-inducible when poplar was mycorrhiza, suggesting a sensor role l with the ectomycorrhizal fungi P. involutus (Couturier et al., 2007) and A. muscaria (Selle et al., 2005) (Figure S6). In addition, we found that AMT3.1 is as well a mycorrhiza-inducible transporter in the roots, which is in contrast to the data of Couturier et al. (2007) who detected PtAMT3.1 solely in senescing leaves. In shoots, the expression of PtAMT1.1 is mediated by Pi availability under mycorrhizal conditions. Further, increased expression of PtAMT1.2 and PtAMT3.1 suggests an increased ammonium transfer when the fungal needs of Pi are accomplished. Analysis of the transcriptome dataset revealed that PtAMT4.1, PtAMT4.2, and PtAMT4.3 were also induced upon mycorrhization independently from the P supply of the fungus as it was the case in our previous study where mycorrhizal poplar was set under N stress (Calabrese et al., 2017).
As there is an ongoing debate on whether amino acids as an organic N source can be taken up by AM fungi and transferred from the fungus to the plant (reviewed in Hodge and Storer, 2015) we screened the transcriptome data of poplar and identified several amino acid transporters and H+/oligopeptide symporters that were either induced or repressed upon mycorrhization (Table S3). Specific induction of amino acid transporters and one of the H+/oligopeptide transporters indicate that amino acids are transferred from the AM fungus to the plant as an alternative N source. However, our metabolome analysis on mycorrhizal and non-mycorrhizal poplar roots showed that mycorrhization reduced the abundance of most metabolites including amino acids in the colonized roots tissue (Tables 2 and S7), suggesting high rates of metabolic turnover by the fungus or the host roots or, alternatively, transport to the shoots. Interestingly, an accumulation of relevant metabolites might be detectable in the shoots as it was demonstrated by Whiteside et al. (2012) for the amino acids phenylalanine, lysine, asparagine, arginine, histidine, cysteine, methionine, and tryptophan with quantum dot analysis.
In the well-established mycorrhizal symbiosis described here, GintAMT3 was significantly induced in the IRM in both hosts, poplar and sorghum (Figure 4), indicating a major participation of this transporter in the exchanges of ammonium at the arbuscule (Calabrese et al., 2017). High GinAMT2 expression levels independent of Pi-supply and localization in the ERM and IRM indicate that it is a low affinity transporter for ammonium. Moreover, GinAMT2 displays high sequence similarity to GintAMT3 which is a low affinity transporter (Calabrese et al., 2017). GintAMT1 on the other hand, a high affinity transporter, was expressed at low levels in the ERM and IRM independent of the P availability. Together, these findings indicate that GintAMT1 and GintAMT2 are mainly involved in the uptake of ammonium in the ERM and in the IRM, and that GintAMT3 is mainly involved in the exchanges/competition of/for ammonium at the biotrophic interface between the AMF and its host plant.
In sorghum, only SbAMT3.1 was induced in mycorrhizal roots (Figure S7). In contrast to Koegel et al. (2013)SbAMT4 was not expressed in our experimental conditions. Induced expression of SbAMT1.1 and SbAMT1.2 in the low-P condition suggests that upon sensing of nutrient stress the plant activates a general nutrient uptake program to avoid running short on one or more essential nutrients. In addition our data show that regulation of sorghum AMTs is less mycorrhiza-dependent than nutrient-dependent. Under mycorrhization the plant is supplied with sufficient P and N to keep the P and N level constant within the plant. This scenario may explain the unchanged expression of the SbAMTs. Interestingly, mycorrhization induced expression of SbAMT1.1 and SbAMT1.2 in the shoots in low-P and of SbAMT3.3 in high-P conditions. An increased translocation rate of ammonium from root to shoot upon mycorrhization may be a possible explanation. Moreover, SbAMT1.1 and SbAMT1.2 expression levels have a tendency to be also concentration dependent. Strong induction of SbAMT3.3 in the shoots indicated an increased ammonium flux during high-P and mycorrhizal condition.
Symbiotic Carbon Exchange
In order to shed some light on symbiotic carbon exchange we used qRT-PCR and mRNA-Seq analysis to identify possible transporters that enable carbohydrate transport from the plant to the fungus. Interestingly, quantitative RT-PCR expression analysis and mRNA-Seq analysis revealed downregulation of carbohydrate transporters in poplar (Figure S8 and Figure 6) and sorghum (Figure S9), which might indicate that carbohydrates are actively sequestered by the fungus. But we also identified two carbohydrate transporters induced upon mycorrhization (Table S3). The UDP-galactose transporters are intracellular transporters situated in the cisternae of the Golgi lumen where UDP-galactose is used for synthesis of non-cellulosic polysaccharides and glycoproteins (Norambuena et al., 2002). Only recently, it was shown that they also transport rhamnose (Rautengarten et al., 2014). In the Golgi-network and the early endosome the newly synthesized proteins are sorted either for the secretion to the plasma membrane, the extracellular matrix or for degradation (Feraru et al., 2012; Brandizzi and Barlowe, 2013; McFarlane et al., 2014). Induced expression of UDP-galactose therefore indicates an increased transport activity of proteins to colonized cells which goes along with increased plasma membrane synthesis. In M. truncatula, it has been shown that arbuscule development goes along with the synthesis of the periarbuscular membrane which is distinct from the plasma membrane (Pumplin and Harrison, 2009). Further it has been shown that AM-induced transporters are specifically directed to the periarbuscular membrane and an involvement of the trans-Golgi for PT has been implicated (Pumplin et al., 2012). The other transporter induced upon mycorrhization is a SUT/spinster transmembrane protein, a member of the major facilitator proteins, which makes it a candidate for being localized at the cell membrane eventually stimulating carbohydrate transport to the AM symbiont.
Interesting is also that a glucose-6-phosphate/phosphate and phosphoenolpyruvate/phosphate antiporter was induced. Glucose-6 phosphate/phosphate antiporters are normally expressed in non-green plastids and serves as carbohydrate importer. In amyloplasts glucose-6 phosphate/phosphate antiporters are located at the site of starch and fatty acids synthesis (Kammerer et al., 1998; Flügge, 2001). Phosphoenolpyruvate/phosphate antiporter mediates the transport of the transport of Phosphoenolpyruvate into the organelles as in amyloplasts, for the synthesis of aromatic amino acids and several secondary compounds. Increased activity of this transporter suggests a decrease of freely available sugars, of storing starch and of aromatic amino acids synthesis. These results shed light the link between carbon, phosphorus, but also N.
In the AM fungi G. pyriformis and R. irregularis several carbohydrate transporters were identified. For GpMST1 and RiMST2, it was shown that they specifically transport monosaccharides. RiMST2 was found to be expressed at the arbuscular site and in the intraradical hyphae (Schüßler et al., 2006; Helber et al., 2011). Helber et al. (2011) proposed a model in which the absorbed sugars might derive from cell wall degradation but this is contradictory to previous observations in which carbohydrate transfer varied with the source strength of the host plant (Olsson et al., 2010; Fellbaum et al., 2014; Zhang et al., 2015). We demonstrated that RiMST2 was induced in the IRM of its host plants poplar (Figure 3) and sorghum (Figure 3) and that its expression level remained unchanged by Pi availability. The metabolome analysis revealed further that mycorrhization significantly decreased the abundance of monosaccharides (i.e. glucose, rhamnose and ribose) in AM poplar roots. In AM fungi it has been shown that hexoses received from the host plants are transformed to glycogen, trehalose. Our observations are consistent with these findings that monosaccharides are taken up mainly by RiMST2 from the apoplast, converted into disaccharides (i.e. trehalose) for transport and hydrolyzed in the ERM into monosaccharides, ready for direct usage or for storage. The mycorrhizal-dependent downregulation of carbohydrate transporters and monosaccharide abundance in poplar roots, along with reduced RiMST2 expression at the arbuscular site and in the IRM suggests that the fungus actively sequesters the sugars on its own demand.
Taken together our data suggest that the plant strictly regulates carbohydrate allocation on mycorrhizal roots. With the Sugar transporter/spinster transmembrane protein a possible candidate for symbiotic carbon transfer from the plant to the periarbuscular space is found. The fungus may take up sugars from there by RiMST2.
AMF are fatty acid (FA) auxotrophs as their fatty acids are provided by their host plant (McLean et al., 2017). To date, mechanisms of FA transport are unknown. We observed that fungal lipid metabolism is highly expressed in the IRM. While many lipases, elongases, and desaturases are expressed both in the ERM and IRM, they might play a role in the storage and use of triglycerides in lipid droplets. However, their over-expression in planta could be a clue of the recovering process of FA from the plant.
Conclusion
Here, we demonstrated that mycorrhization leads to specific induction of a variety of transporters. As P and N are key elements of mycorrhizal symbiosis, we tested the expression of PT and AMT and show that mycorrhization specifically induces expression of a selection of PT and AMTs in poplar and in sorghum. Further, we identified one carbohydrate transporter specifically induced in mycorrhizal root tissue that might be a possible candidate for symbiotic carbon exchange. By contrast, other carbohydrate transporters were down-regulated upon mycorrhization indicating that the plant may not volunteer provision of carbohydrates in exchange for mineral nutrients. We further showed that some nutrient transporters are more strongly expressed in the IRM compared to the ERM, which indicates that they are directly involved in nutrient transfer at the arbuscular membrane or, as in the case of MST, in nutrient uptake into the intraradical hyphae (Figure 6). Nevertheless, despite its importance, the release of major nutrients taken up by the ERM into the root apoplasm occurs through widely unknown mechanisms, which must include the differentiation and polarization of the fungal membrane transport functions. Transport processes across the polarized membrane interfaces are of major importance in the functioning of the established mycorrhizal association as the symbiotic relation is based on an apparent ‘fair-trade’ between fungus and host plant. PTs and AMTs are active transporters and they are able to transport nutrients against the direction of concentration gradients using electrochemical potential differences build up by proton-pumping ATPases. In fact, the reality is probably much more complex, with reuptake of nutrients at the biotrophic interface allowing both partners to, at least partially, control the exchanges (Figure 6).
In agriculture, vast amounts of mineral fertilizers are applied to the field to increase crop yield. Indeed, the amount of applied fertilizer might actually exceed the plant needs for mineral nutrients. Collecting data such as ours will help to further deepen our understanding of the plant-AM symbiosis and may lead to development of a more sustainable agriculture with a reduced fertilizer input and improved adaptation to changing environmental conditions.
Data Availability Statement
Raw RNAseq data and global mapping data analyses were deposited at GEO (GSE138316).
Author Contributions
SC made the major part of the experiments. AS and LC made in silico analysis. JK and AE performed metabolite analysis. All co-authors participated in writing.
Funding
This project was supported by the Swiss National Science Foundation (grants no. PZ00P3_136651 to P-EC and no. 127563 to TB). The authors thank the following Institutions for financial support: the Burgundy Franche Comté Regional Council, the division of Plant Health and Environment of the French National Institute for Agricultural Research (INRA).
Conflict of Interest
The authors declare that the research was conducted in the absence of any commercial or financial relationships that could be construed as a potential conflict of interest.
Acknowledgments
We would like to thank De-Roman Yvan for his help in establishing the fungal transportome list. LRSV is part of the TULIP ‘Laboratoire d’Excellence’ (ANR-10-LABX-41). The authors are grateful to the anonymous reporters for constructive comments on the manuscript.
Abbreviations
AM, Arbuscular mycorrhiza; AMF, Arbuscular mycorrhizal fungi; AMT, Ammonium transporter; ERM, Extraradical mycelium; IRM, Intraradical mycelium; N, Nitrogen; P, Phosphorus; Pi, inorganic phosphate; PT, Phosphate transporter; GS/GOGAT, glutamine synthetase/glutamine oxoglutarate aminotransferase.
Supplementary Material
The Supplementary Material for this article can be found online at: https://www.frontiersin.org/articles/10.3389/fpls.2019.01617/full#supplementary-material
Table S1 | Primer used for qRT-PCR in P. trichocarpa, S. bicolor and R. irregularis.
Table S2 | Percentage of colonized P. trichocarpa and S. bicolor roots colonized by R. irregularis. Statistical analysis was performed on six biological replicates by T.TEST (p<0.05) for each plant species. Significant differences are indicated by lower case letters.
Table S3 | Differentially expressed amino acid and carbohydrate transporters in P. trichocarpa. Significant p-values (p<0.05) are highlighted in bold.
Table S4 | P. trichocarpa and S. bicolor transporters regulated by mycorrhizal symbiosis and P treatment.
Table S5 | Name of fungal genes regulated by P treatment and during the interaction with P. trichocarpa and S. bicolor.
Table S6 | Relative abundances of metabolites detected in the ERM of R. irregularis. Data was log transformed and tested by Wilcoxon rank sum test (p<0.05) in MeV v4.9 (http://www.tm4.org/mev.html). Significant p-values are highlighted in bold.
Table S7 | Relative abundances of metabolites detected poplar roots. Abundances were measured in the mycorrhized (+AM) and non-mycorrhized (-AM) poplar roots under high (hP) and low (lP) P availability. Data was log transformed and tested by y 2-way analysis of variance (ANOVA) (p<0.05) in MeV v4.9 (http://www.tm4.org/mev.html). Significant p-values are highlighted in bold.
References
Ai, P., Sun, S., Zhao, J., Fan, X., Xin, W., Guo, Q., et al. (2009). Two rice phosphate transporters, OsPht1;2 and OsPht1;6, have different functions and kinetic properties in uptake and translocation. Plant J. 57, 798–809. doi: 10.1111/j.1365-313X.2008.03726
An, J., Zeng, T., Ji, C., de Graaf, S., Zheng, Z., Xiao, T. T., et al. (2019). A Medicago truncatula transporter implicated in arbuscule maintenance during arbuscular mycorrhizal symbiosis. New Phytol. 224, 396–408. doi: 10.1111/nph.15975
Aung, K., Lin, S. I., Wu, C. C., Huang, Y. T., Su, C., Chiou, T. J. (2006). pho2, a phosphate overaccumulator, is caused by a nonsense mutation in a microRNA399 target gene. Plant Physiol. 141, 1000–1011. doi: 10.1104/pp.106.078063
Baggerly, K. A., Deng, L., Morris, J. S., Marcelo Aldaz, C. (2003). Differential expression in SAGE: accounting for normal between-library variation. Bioinformatics 19, 1477–1483. doi: 10.1093/bioinformatics/btg173
Bago, B., Vierheilig, H., Piché, Y., Azcón-Aguilar, C. (1996). Nitrate depletion and pH changes induced by the extraradical mycelium of the arbuscular mycorrhizal fungus Glomus intraradices grown in monoxenic culture. New Phytol. 133, 273–280. doi: 10.1111/j.1469-8137.1996.tb01894
Bécard, G., Doner, L., Rolin, D., Douds, D., Pfeffer, P. (1991). Identification and quantification of trehalose in vesicular-arbuscular mycorrhizal fungi by in vivo 13C NMR and HPLC analyses. New Phytol. 118, 547–552. doi: 10.1111/j.1469-8137.1991.tb00994.x
Benedetto, A., Magurno, F., Bonfante, P., Lanfranco, L. (2005). Expression profiles of a phosphate transporter gene (GmosPT) from the endomycorrhizal fungus. Glomus mosseae. Mycorrhiza 15, 620–627. doi: 10.1007/s00572-005-0006-9
Benjamini, Y., Hochberg, Y. (1995). Controlling the false discovery rate: a practical and powerful approach to multiple testing. J. R. Stat. Soc B. 57, 289–300. doi: 10.1111/j.2517-6161.1995.tb02031
Boldt, K., Pörs, Y., Haupt, B., Bitterlich, M., Kühn, C., Grimm, B., et al. (2011). Photochemical processes, carbon assimilation and RNA accumulation of sucrose transporter genes in tomato arbuscular mycorrhiza. J. Plant Physiol. 168, 1256–1263. doi: 10.1016/j.jplph.2011.01.026
Brandizzi, F., Barlowe, C. (2013). Organization of the ER-Golgi interface for membrane traffic control. Nat. Rev. Mol. Cell Biol. 14, 382–392. doi: 10.1038/nrm3588
Branscheid, A., Sieh, D., Pant, B. D., May, P., Devers, E. A., Elkrog, A., et al. (2010). Expression pattern suggests a role of miR399 in the regulation of the cellular response to local Pi increase during arbuscular mycorrhizal symbiosis. Mol. Plant-Microbe In. 23, 915–926. doi: 10.1094/MPMI-23-7-0915
Bravo, A., Brands, M., Wewer, V., Dörmann, P., Harrison, M. J. (2017). Arbuscular mycorrhiza-specific enzymes FatM and RAM2 fine-tune lipid biosynthesis to promote development of arbuscular mycorrhiza. New Phytol. 214, 1631–1645. doi: 10.1111/nph.14533
Breuillin-Sessoms, F., Floss, D. S., Gomez, S. K., Pumplin, N., Ding, Y., Levesque-Tremblay, V., et al. (2015). Suppression of arbuscule degeneration in Medicago truncatula phosphate transporter4 mutants is dependent on the ammonium transporter 2 family protein AMT2;3. Plant Cell 27, 1352–1366. doi: 10.1105/tpc.114.131144
Brundrett, M. C., Piché, Y., Peterson, R. L. (1984). A new method for observing the morphology of vesicular–arbuscular mycorrhizae. Can. J. Bot. 62, 2128–2134. doi: 10.1139/b84-290
Calabrese, S., Pérez-Tienda, J., Ellerbeck, M., Arnould, C., Chatagnier, O., Boller, T., et al. (2016). GintAMT3 – a low-affinity ammonium transporter of the arbuscular mycorrhizal. Rhizophagus irregularis. Front. Plant Sci. 7, art 679. doi: 10.3389/fpls.2016.00679
Calabrese, S., Kohler, A., Niehl, Veneault-Fourrey, C., Boller, T., Courty, P.-E. (2017). Transcriptome analysis of the Populus trichocarpa–Rhizophagus irregularis mycorrhizal symbiosis. regulation of plant and fungal transportomes under nitrogen starvation. Plant Cell Physiol. 58, 1003–1017. doi: 10.1093/pcp/pcx044
Chen, L. Q., Hou, B. H., Lalonde, S., Takanaga, H., Hartung, M. L., Qu, X. Q., et al. (2010). Sugar transporters for intercellular exchange and nutrition of pathogens. Nature 468, 527–532. doi: 10.1038/nature09606
Chiou, T. J., Aung, K., Lin, S. I., Wu, C. C., Chiang, S. F., Su, C. L. (2006). Regulation of phosphate homeostasis by microRNA in Arabidopsis. Plant Cell 18, 412–421. doi: 10.1105/tpc.105.038943
Courty, P. E., Franc, A., Pierrat, J. C., Garbaye, J. (2008). Temporal changes in the ectomycorrhizal community in two soil horizons of a temperate oak forest. Appl. Environ. Microb. 74, 5792–5801. doi: 10.1128/AEM.01592-08
Courty, P. E., Smith, P., Koegel, S., Redecker, D., Wipf, D. (2015). Inorganic nitrogen uptake and transport in beneficial plant root-microbe interactions. Crit. Rev. Plant Sci. 34, 4–16. doi: 10.1080/07352689.2014.897897
Couturier, J., Montanini, B., Martin, F., Brun, A., Blaudez, D., Chalot, M. (2007). The expanded family of ammonium transporters in the perennial poplar plant. New Phytol. 174, 137–150. doi: 10.1111/j.1469-8137.2007.01992
Dethloff, F., Erban, A., Orf, I., Alpers, J., Fehrle, I., Beine-Golovchuk, O., et al. (2014). Profiling methods to identify cold-regulated primary metabolites using gas chromatography coupled to mass spectrometry. In Plant Cold Acclimation. Springe 1166, 171–197. doi: 10.1007/978-1-4939-0844-8_14
Dietrich, D., Hammes, U., Thor, K., Suter-Grotemeyer, M., Flückiger, R., Slusarenko, A. J., et al. (2004). AtPTR1, a plasma membrane peptide transporter expressed during seed germination and in vascular tissue of Arabidopsis. Plant J. 40, 488–499. doi: 10.1111/j.1365-313X.2004.02224
Doidy, J., Grace, E., Kühn, C., Simon-Plas, F., Casieri, L., Wipf, D. (2012a). Sugar transporters in plants and in their interactions with fungi. Trends Plant Sci. 17, 413–422. doi: 10.1016/j.tplants.2012.03.009
Doidy, J., van Tuinen, D., Lamotte, O., Corneillat, M., Alcaraz, G., Wipf, D. (2012b). The Medicago truncatula sucrose transporter family: characterization and implication of key members in carbon partitioning towards arbuscular mycorrhizal fungi. Mol. Plant 5, 1346–1358. doi: 10.1093/mp/sss079
Dumbrell, A. J., Ashton, P. D., Aziz, N., Feng, G., Nelson, M., Dytham, C., et al. (2011). Distinct seasonal assemblages of arbuscular mycorrhizal fungi revealed by massively parallel pyrosequencing. New Phytol. 190, 794–804. doi: 10.1111/j.1469-8137.2010.03636
Ellerbeck, M., Schüßler, A., Brucker, D., Dafinger, C., Loos, F., Brachmann, A. (2013). Characterization of three ammonium transporters of the Glomeromycotan fungus. Geosiphon pyriformis. Eukaryot. Cell 12, 1554–1562. doi: 10.1128/EC.00139-13
Ezawa, T., Smith, S., Smith, F. A. (2002). P metabolism and transport in AM fungi. Plant Soil 244, 221–230. doi: 10.1023/A:1020258325010
Fellbaum, C. R., Mensah, J. A., Cloos, A. J., Strahan, G. E., Pfeffer, P. E., Kiers, E. T., et al. (2014). Fungal nutrient allocation in common mycorrhizal networks is regulated by the carbon source strength of individual host plants. New Phytol. 203, 646–656. doi: 10.1111/nph.12827
Feraru, E., Feraru, M. I., Asaoka, R., Paciorek, T., De Rycke, R., Tanaka, H., et al. (2012). BEX5/RabA1b regulates trans-Golgi network-to-plasma membrane protein trafficking in Arabidopsis. Plant Cell 24, 3074–3086. doi: 10.1105/tpc.112.098152
Fiorilli, V., Lanfranco, L., Bonfante, P. (2013). The expression of GintPT, the phosphate transporter of Rhizophagus irregularis, depends on the symbiotic status and phosphate availability. Planta 237, 1267–1277. doi: 10.1007/s00425-013-1842-z
Flügge, U. I. (2001). Plant chloroplasts and other plastids, in Encycl Life Sci (London: Macmillan).
Frey, B., Schüepp, H. (1993). Acquisition of nitrogen by external hyphae of arbuscular mycorrhizal fungi associated with Zea mays L. New Phytol. 124, 221–230. doi: 10.1111/j.1469-8137.1993.tb03811.x
Gamas, P., de Carvalho Niebel, F., Lescure, N., Cullimore, J. V. (1996). Use of a subtractive hybridization approach to identify new Medicago truncatula genes induced during root nodule development. Mol. Plant Microbe In. 9, 233–242. doi: 10.1094/mpmi-9-0233
Garcia, K., Doidy, J., Zimmermann, S., Wipf, D., Courty, P. E. (2016). Take a trip through the plant and fungal transportome of mycorrhiza. Trends Plant Sci. 21, 937–950. doi: 10.1016/j.tplants.2016.07.010
Ge, L., Sun, S., Chen, A., Kapulnik, Y., Xu, G. (2008). Tomato sugar transporter genes associated with mycorrhiza and phosphate. Plant Growth Regul. 55, 115–123. doi: 10.1007/s10725-008-9266-7
Glassop, D., Smith, S., Smith, F. (2005). Cereal phosphate transporters associated with the mycorrhizal pathway of phosphate uptake into roots. Planta 222, 688–698. doi: 10.1007/s00425-005-0015-0
Gomez, S. K., Javot, H., Deewatthanawong, P., Torres-Jerez, I., Tang, Y., Blancaflor, E., et al. (2009). Medicago truncatula and Glomus intraradices gene expression in cortical cells harboring arbuscules in the arbuscular mycorrhizal symbiosis. BMC Plant Biol. 9, 10. doi: 10.1186/1471-2229-9-10
Govindarajulu, M., Pfeffer, P. E., Jin, H., Abubaker, J., Douds, D. D., Allen, J. W., et al. (2005). Nitrogen transfer in the arbuscular mycorrhizal symbiosis. Nature 435, 819–823. doi: 10.1038/nature03610
Guether, M., Neuhäuser, B., Balestrini, R., Dynowski, M., Ludewig, U., Bonfante, P. (2009). A mycorrhizal-specific ammonium transporter from Lotus japonicus acquires nitrogen released by arbuscular mycorrhizal fungi. Plant Physiol. 150, 73–83. doi: 10.1104/pp.109.136390
Harrison, M. J., Buuren, M. L. (1995). A phosphate transporter from the mycorrhizal fungus Glomus versiforme. Nature 378, 626–629. doi: 10.1038/378626a0
Harrison, M. J., Dewbre, G. R., Liu, J. (2002). A phosphate transporter from Medicago truncatula involved in the acquisition of phosphate released by arbuscular mycorrhizal fungi. Plant Cell 14, 2413–2429. doi: 10.1105/tpc.004861
Hawkins, H. J., Johansen, A., George, E. (2000). Uptake and transport of organic and inorganic nitrogen by arbuscular mycorrhizal fungi. Plant Soil 226, 275–285. doi: 10.1023/A:1026500810385
Helber, N., Wippel, K., Sauer, N., Schaarschmidt, S., Hause, B., Requena, N. (2011). A versatile monosaccharide transporter that operates in the arbuscular mycorrhizal fungus Glomus sp is crucial for the symbiotic relationship with plants. Plant Cell 23, 3812–3823. doi: 10.1105/tpc.111.089813
Hodge, A., Storer, K. (2015). Arbuscular mycorrhiza and nitrogen: implications for individual plants through to ecosystems. Plant Soil 386, 1–19. doi: 10.1016/j.funeco.2010.02.002
Hodge, A., Helgason, T., Fitter, A. H. (2010). Nutritional ecology of arbuscular mycorrhizal fungi. Fungal Ecol. 3, 267–273. doi: 10.1016/j.funeco.2010.02.002
Javot, H., Penmetsa, R. V., Terzaghi, N., Cook, D. R., Harrison, M. J. (2007a). A Medicago truncatula phosphate transporter indispensable for the arbuscular mycorrhizal symbiosis. P. Natl. Acad. Sci. U.S.A. 104, 1720–1725. doi: 10.1073/pnas.0608136104
Javot, H., Pumplin, N., Harrison, M. J. (2007b). Phosphate in the arbuscular mycorrhizal symbiosis: transport properties and regulatory roles. Plant Cell Env. 30, 310–322. doi: 10.1111/j.1365-3040.2006.01617
Jiang, Y., Wang, W., Xie, Q., Liu, N., Liu, L., Wang, D., et al. (2017). Plants transfer lipids to sustain colonization by mutualistic mycorrhizal and parasitic fungi. Science 356, 1172–1175. doi: 10.1126/science.aam9970
Jin, H., Pfeffer, P. E., Douds, D. D., Piotrowski, E., Lammers, P. J., Shachar-Hill, Y. (2005). The uptake, metabolism, transport and transfer of nitrogen in an arbuscular mycorrhizal symbiosis. New Phytol. 168, 687–696. doi: 10.1111/j.1469-8137.2005.01536
Kammerer, B., Fischer, K., Hilpert, B., Schubert, S., Gutensohn, M., Weber, A., et al. (1998). Molecular characterization of a carbon transporter in plastids from heterotrophic tissues: the glucose 6-phosphate/phosphate antiporter. Plant Cell 10, 105–117. doi: 10.1105/tpc.10.1.105
Karim, S., Lundh, D., Holmström, K. O., Mandal, A., Pirhonen, M. (2005). Structural and functional characterization of AtPTR3, a stress-induced peptide transporter of Arabidopsis. J. Mol. Model. 11, 226–236. doi: 10.1007/s00894-005-0257-6
Karim, S., Holmström, K. O., Mandal, A., Dahl, P., Hohmann, S., Brader, G., et al. (2006). AtPTR3, a wound-induced peptide transporter needed for defence against virulent bacterial pathogens in Arabidopsis. Planta 225, 1431–1445. doi: 10.1007/s00425-006-0451-5
Keymer, A., Pimprikar, P., Wewer, V., Huber, C., Brands, M., Bucerius, S. L., et al. (2010). Localized expression of arbuscular mycorrhiza-inducible ammonium transporters in soybean. Plant Cell Physiol. 51, 1411–1415. doi: 10.1093/pcp/pcq099
Kikuchi, Y., Hijikata, N., Ohtomo, R., Handa, Y., Kawaguchi, M., Saito, K., et al. (2016). Aquaporin-mediated long-distance polyphosphate translocation directed towards the host in arbuscular mycorrhizal symbiosis: application of virus-induced gene silencing. New Phytol. 211, 1202–1208. doi: 10.1111/nph.14016
Koegel, S., Ait Lahmidi, N., Arnould, C., Chatagnier, O., Walder, F., Ineichen, K., et al. (2013). The family of ammonium transporters (AMT) in Sorghum bicolor: two AMT members are induced locally, but not systemically in roots colonized by arbuscular mycorrhizal fungi. New Phytol. 198, 853–865. doi: 10.1111/nph.12199
López-Pedrosa, A., González-Guerrero, M., Valderas, A., Azcón-Aguilar, C., Ferrol, N. (2006). GintAMT1 encodes a functional high-affinity ammonium transporter that is expressed in the extraradical mycelium of Glomus intraradices. Fung. Genet. Biol. 43, 102–110. doi: 10.1016/j.fgb.2005.10.005
Leigh, J., Hodge, A., Fitter, A. H. (2009). Arbuscular mycorrhizal fungi can transfer substantial amounts of nitrogen to their host plant from organic material. New Phytol. 181, 199–207. doi: 10.1111/j.1469-8137.2008.02630
Loth-Pereda, V., Orsini, E., Courty, P. E., Lota, F., Kohler, A., Diss, L., et al. (2011). Structure and expression profile of the phosphate Pht1 transporter gene family in mycorrhizal Populus trichocarpa. Plant Physiol. 156, 2141–2154. doi: 10.1104/pp.111.180646
Luginbuehl, L. H., Menard, G. N., Kurup, S., Van Erp, H., Radhakrishnan, G. V., Breakspear, A., et al. (2017). Fatty acids in arbuscular mycorrhizal fungi are synthesized by the host plant. Science 356, 1175–1178. doi: 10.1126/science.aan0081
Mäder, P., Vierheilig, H., Streitwolf-Engel, R., Boller, T., Frey, B., Christie, P., et al. (2000). Transport of 15N from a soil compartment separated by a polytetrafluoroethylene membrane to plant roots via the hyphae of arbuscular mycorrhizal fungi. New Phytol. 146, 155–161. doi: 10.1046/j.1469-8137.2000.00615
Maldonado-Mendoza, I. E., Dewbre, G. R., Harrison, M. J. (2001). A phosphate transporter gene from the extra-radical mycelium of an arbuscular mycorrhizal fungus Glomus intraradices is regulated in response to phosphate in the environment. Mol. Plant Microbe In. 14, 1140–1148. doi: 10.1094/MPMI.2001.14.10.1140
Marschner, H., Dell, B. (1994). Nutrient uptake in mycorrhizal symbiosis. Plant Soil 159, 89–102. doi: 10.1007/BF00000098
McCormick, R., Truong, S., Sreedasyam, A., Jenkins, J., Shu, S., Sims, D., et al. (2018). The Sorghum bicolor reference genome: Improved assembly, gene annotations, a transcriptome atlas, and signatures of genome organization. Plant J. 93 (2), 338–354.
McFarlane, H. E., Watanabe, Y., Yang, W., Huang, Y., Ohlrogge, J., Samuels, A. L. (2014). Golgi- and trans-Golgi network-mediated vesicle trafficking is required for wax secretion from epidermal cells. Plant Physiol. 164, 1250–1260. doi: 10.1104/pp.113.234583
McGonigle, T. P., Miller, M. H., Evans, D. G., Fairchild, G. L., Swan, J. A. (1990). A new method which gives an objective measure of colonization of roots by vesicular—arbuscular mycorrhizal fungi. New Phytol. 115, 495–501. doi: 10.1111/j.1469-8137.1990.tb00476.x
McLean, A. M., Bravo, A., Harrison, M. J. (2017). Plant signaling and metabolic pathways enabling arbuscular mycorrhizal symbiosis. Plant Cell 29, 2319–2335. doi: org/10.1105/tpc.17.00555
Morin, E., Miyauchi, S., San Clemente, H., Chen, E. C. H., Pelin, A., de la Providencia, I., et al. (2019). Comparative genomics of Rhizophagus irregularis, R. cerebriforme, R. diaphanus and Gigaspora rosea highlights specific genetic features in Glomeromycotina. New Phytol. 222, 1584–1598. doi: 10.1111/nph.15687
Mortazavi, A., Williams, B. A., McCue, K., Schaeffer, L., Wold, B. (2008). Mapping and quantifying mammalian transcriptomes by RNA-Seq. Nat. Methods 5, 621–628. doi: org/10.1038/nmeth.1226
Murphy, J., Riley, J. P. (1962). A modified single solution method for the determination of phosphate in natural waters. Anal. Chim. Acta 27, 31–36. doi: 10.1016/S0003-2670(00)88444-5
Nagy, R., Karandashov, V., Chague, V., Kalinkevich, K., Tamasloukht, M. B., Xu, G., et al. (2005). The characterization of novel mycorrhiza-specific phosphate transporters from Lycopersicon esculentum and Solanum tuberosum uncovers functional redundancy in symbiotic phosphate transport in solanaceous species. Plant J. 42, 236–250. doi: 10.1111/j.1365-313X.2005.02364
Norambuena, L., Marchant, L., Berninsone, P., Hirschberg, C. B., Silva, H., Orellana, A. (2002). Transport of UDP-galactose in plants: identification and functional characterization of AtUTr1, an Arabidopsis thaliana UDP-galactose/UDP-glucose transporter. J. Biol. Chem. 277, 32923–32929. doi: 10.1074/jbc.M204081200
Olsson, P. A., Rahm, J., Aliasgharzad, N. (2010). Carbon dynamics in mycorrhizal symbioses is linked to carbon costs and phosphorus benefits. FEMS Microbiol. Ecol. 72, 125–131. doi: 10.1111/j.1574-6941.2009.00833
Pao, S. S., Paulsen, I. T., Saier, M. H. (1998). Major facilitator superfamily. Microbiol. Mol. Biol. Rev. 62, 1–34.
Paszkowski, U. (2006). A journey through signaling in arbuscular mycorrhizal symbioses. New Phytol. 172, 35–46. doi: 10.1111/j.1469-8137.2006.01840
Pérez-Tienda, J., Testillano, P. S., Balestrini, R., Fiorilli, V., Azcón-Aguilar, C., Ferrol, N. (2011). GintAMT2, a new member of the ammonium transporter family in the arbuscular mycorrhizal fungus Glomus intraradices. Fung. Genet. Biol. 48, 1044–1055. doi: 10.1016/j.fgb.2011.08.003
Pérez-Tienda, J., Corrêa, A., Azcón-Aguilar, C., Ferrol, N. (2014). Transcriptional regulation of host transporters and GS/GOGAT pathway in arbuscular mycorrhizal rice roots. Plant Physiol. Biochem. 75, 1–8. doi: 10.1016/j.plaphy.2013.11.029
Poirier, Y., Bucher, M. (2002). Phosphate transport and homeostasis in Arabidopsis. in The Arabidopsis Book eds. Sommerville, C.R., Meyerowitz, E.M. (Rockville, Maryland: American Society of Plant Biologists).
Pumplin, N., Harrison, M. J. (2009). Live-cell imaging reveals periarbuscular membrane domains and organelle location in Medicago truncatula roots during arbuscular mycorrhizal symbiosis. Plant Physiol. 151, 809–819. doi: 10.1104/pp.109.141879
Pumplin, N., Zhang, X., Noar, R. D., Harrison, M. J. (2012). Polar localization of a symbiosis-specific phosphate transporter is mediated by a transient reorientation of secretion. P. Natl. Acad. Sci. U .S. A. 109, 665–672. doi: 10.1073/pnas.1110215109
Rausch, C., Bucher, M. (2002). Molecular mechanisms of phosphate transport in plants. Planta 216, 23–37. doi: 10.1007/s00425-002-0921-3
Rausch, C., Daram, P., Brunner, S., Jansa, J., Laloi, M., Leggewie, G., et al. (2001). A phosphate transporter expressed in arbuscule-containing cells in potato. Nature 414, 462–470. doi: 10.1038/35106601
Rautengarten, C., Ebert, B., Moreno, I., Temple, H., Herter, T., Link, B., et al. (2014). The Golgi localized bifunctional UDP-rhamnose/UDP-galactose transporter family of Arabidopsis. P. Natl. Acad. Sci. U.S.A. 111, 11563–11568. doi: 10.1073/pnas.1406073111
Saeed, A. I., Bhagabati, N. K., Braisted, J. C., Liang, W., Sharov, V., Howe, E. A., et al. (2006). TM4 microarray software suite. Method. Enzymol. 411, 134–193. doi: 10.1016/S0076-6879(06)11009-5
Schüßler, A., Martin, H., Cohen, D., Fitz, M., Wipf, D. (2006). Characterization of a carbohydrate transporter from symbiotic glomeromycotan fungi. Nature 444, 933–936. doi: 10.1038/nature05364
Schachtman, D. P., Reid, R. J., Ayling, S. M. (1998). Phosphorus uptake by plants: from soil to cell. Plant Physiol. 116, 447–453. doi: 10.1104/pp.116.2.447
Selle, A., Willmann, M., Grunze, N., Geßler, A., Weiß, M., Nehls, U. (2005). The high-affinity poplar ammonium importer PttAMT1.2 and its role in ectomycorrhizal symbiosis. New Phytol. 168, 697–706. doi: 10.1111/j.1469-8137.2005.01535
Smith, S. E., Smith, F. A. (2011). Roles of arbuscular mycorrhizas in plant nutrition and growth: new paradigms from cellular to ecosystem scales. Annu. Rev. Plant Biol. 62, 227–250. doi: 10.1146/annurev-arplant-042110-103846
Strehmel, N., Hummel, J., Erban, A., Strassburg, K., Kopka, J. (2008). Retention index thresholds for compound matching in GC–MS metabolite profiling. J. Chromatogr. B 871, 182–190. doi: 10.1016/j.jchromb.2008.04.042
Takabatake, R., Hata, S., Taniguchi, M., Kouchi, H., Sugiyama, T., Izui, K. (1999). Isolation and characterization of cDNAs encoding mitochondrial phosphate transporters in soybean, maize, rice, and Arabidopsis. Plant Mol. Biol. 40, 479–486. doi: 10.1023/A:1006285009435
Tang, N., San Clemente, H., Roy, S., Becard, G., Zhao, B., Roux, C. (2016). A survey of the gene repertoire of Gigaspora rosea unravels conserved features among glomeromycota for obligate biotrophy. Front. Microbiol. 7, 233. doi: 10.3389/fmicb.2016.00233
Tatry, M. V., El Kassis, E., Lambilliotte, R., Corratgé, C., Van Aarle, I., Amenc, L. K., et al. (2009). Two differentially regulated phosphate transporters from the symbiotic fungus Hebeloma cylindrosporum and phosphorus acquisition by ectomycorrhizal Pinus pinaster. Plant J. 57, 1092–1102. doi: 10.1111/j.1365-313X.2008.03749
Toussaint, J. P., St-Arnaud, M., Charest, C. (2004). Nitrogen transfer and assimilation between the arbuscular mycorrhizal fungus Glomus intraradices Schenck and Smith and Ri T-DNA roots of Daucus carota L. in an in vitro compartmented system. Can. J. Microbiol. 50, 251–260. doi: 10.1139/w04-009
Tuskan, G. A., Difazio, S., Jansson, S., Bohlmann, J., Grigoriev, I., Hellsten, U., et al. (2006). The genome of black cottonwood, Populus trichocarpa (Torr. and Gray). Science 313, 1596–1604. doi: 10.1126/science.1128691
Villegas, J., Williams, R. D., Nantais, L., Archambault, J., Fortin, J. A. (1996). Effects of N source on pH and nutrient exchange of extramatrical mycelium in a mycorrhizal Ri T-DNA transformed root system. Mycorrhiza 6, 247–251. doi: 10.1007/s005720050132
Walder, F., Niemann, H., Natarajan, M., Lehmann, M. F., Boller, T., Wiemken, A. (2012). Mycorrhizal networks: common goods of plants shared under unequal terms of trade. Plant Physiol. 159, 789–797. doi: 10.1104/pp.112.195727
Walder, F., Boller, T., Wiemken, A., Courty, P. E. (2016). Regulation of plants’ phosphate uptake in common mycorrhizal networks: role of intraradical fungal phosphate transporters. Plant Signal. Behav. 11, e1131372. doi: 10.1080/15592324.2015.1131372
Wewer, V., Brands, M., Dörmann, P. (2014). Fatty acid synthesis and lipid metabolism in the obligate biotrophic fungus Rhizophagus irregularis during mycorrhization of Lotus japonicus. Plant J. 79, 398–412. doi: 10.1111/tpj.12566
Whiteside, M. D., Garcia, M. O., Treseder, K. K. (2012). Amino acid uptake in arbuscular mycorrhizal plants. PloS One 7, e47643. doi: 10.1371/journal.pone.0047643
Whiteside, M. D., Werner, G. D. A., Caldas, V. E. A., van’t Padje, A., Dupin, S. E., Elbers, B., et al. (2019). Mycorrhizal fungi respond to resource inequality by moving phosphorus from rich to poor patches across networks. Curr. Biol. 29, 2043–2050. doi: 10.1016/j.cub.2019.04.061
Wipf, D., Krajinski, F., van Tuinen, D., Recorbet, G., Courty, P. E. (2019). Trading on the arbuscular mycorrhiza market: from arbuscule to common mycorrhizal networks. New Phytol. 223, 1127–1142. doi: 10.1111/nph.15775
Keywords: arbuscular mycorrhizal fungi, symbiocosm, extraradical mycelium, intraradical mycelium, phosphorus, ammonium, carbohydrates transporters, lipid metabolism
Citation: Calabrese S, Cusant L, Sarazin A, Niehl A, Erban A, Brulé D, Recorbet G, Wipf D, Roux C, Kopka J, Boller T and Courty P-E (2019) Imbalanced Regulation of Fungal Nutrient Transports According to Phosphate Availability in a Symbiocosm Formed by Poplar, Sorghum, and Rhizophagus irregularis. Front. Plant Sci. 10:1617. doi: 10.3389/fpls.2019.01617
Received: 31 July 2019; Accepted: 18 November 2019;
Published: 12 December 2019.
Edited by:
Raffaella Balestrini, Italian National Research Council (IPSP-CNR), ItalyReviewed by:
Philipp Franken, Friedrich Schiller University Jena, GermanyCristiana Sbrana, Istituto di Biologia e Biotecnologia Agraria (IBBA), Italy
Copyright © 2019 Calabrese, Cusant, Sarazin, Niehl, Erban, Brulé, Recorbet, Wipf, Roux, Kopka, Boller and Courty. This is an open-access article distributed under the terms of the Creative Commons Attribution License (CC BY). The use, distribution or reproduction in other forums is permitted, provided the original author(s) and the copyright owner(s) are credited and that the original publication in this journal is cited, in accordance with accepted academic practice. No use, distribution or reproduction is permitted which does not comply with these terms.
*Correspondence: Pierre-Emmanuel Courty, cGllcnJlLWVtbWFudWVsLmNvdXJ0eUBpbnJhLmZy
†These authors have contributed equally to this work