- 1Centro de Biotecnología y Genómica de Plantas (UPM-INIA), Universidad Politécnica de Madrid, Madrid, Spain
- 2EcoLab, Université de Toulouse, CNRS, Toulouse, France
- 3Department of Chemistry and Biochemistry, Worcester Polytechnic Institute, Worcester, MA, United States
- 4Estación Experimental de Aula Dei, Consejo Superior de Investigaciones Científicas, Zaragoza, Spain
- 5Noble Research Institute, Ardmore, OK, United States
- 6ID21 Beamline, European Synchrotron Radiation Facility, Grenoble, France
- 7Instituto de Ciencias Agrarias, Consejo Superior de Investigaciones Científicas, Madrid, Spain
- 8Escuela Técnica Superior de Ingeniería Agronómica, Alimentaria y de Biosistemas, Universidad Politécnica de Madrid, Madrid, Spain
Symbiotic nitrogen fixation carried out by the interaction between legumes and diazotrophic bacteria known as rhizobia requires relatively large levels of transition metals. These elements are cofactors of many key enzymes involved in this process. Metallic micronutrients are obtained from soil by the roots and directed to sink organs by the vasculature, in a process mediated by a number of metal transporters and small organic molecules that facilitate metal delivery in the plant fluids. Among the later, nicotianamine is one of the most important. Synthesized by nicotianamine synthases (NAS), this molecule forms metal complexes participating in intracellular metal homeostasis and long-distance metal trafficking. Here we characterized the NAS2 gene from model legume Medicago truncatula. MtNAS2 is located in the root vasculature and in all nodule tissues in the infection and fixation zones. Symbiotic nitrogen fixation requires of MtNAS2 function, as indicated by the loss of nitrogenase activity in the insertional mutant nas2-1, phenotype reverted by reintroduction of a wild-type copy of MtNAS2. This would result from the altered iron distribution in nas2-1 nodules shown with X-ray fluorescence. Moreover, iron speciation is also affected in these nodules. These data suggest a role of nicotianamine in iron delivery for symbiotic nitrogen fixation.
Introduction
Nitrogen is one of the main limiting nutrients in the biosphere, in spite of N2 abundance (Smil, 1999; Hoffman et al., 2014). Nitrogenase is the only enzyme that can convert, fix, N2 into NH3 under physiological conditions, in an energy consuming process (Burk, 1934; Burgess and Lowe, 1996). This enzyme is only expressed by the small group of diazotrophic archaea and bacteria, some of them participating in symbiosis with other organisms (Boyd and Peters, 2013). Arguably, one of the best characterized symbiosis with diazotrophic bacteria is the one established between rhizobia and legumes (Brewin, 1991; Downie, 2014). This symbiosis is the basis for legume use in crop rotation strategies and their potential as an alternative to polluting and expensive synthetic nitrogen fertilizers (Johnson and Mohler, 2009; Mus et al., 2016).
Symbiotic nitrogen fixation by the legume-rhizobia system is carried out in root nodules (Downie, 2014). These are differentiated organs that develop after a complex exchange of chemical signals between the symbionts (Oldroyd, 2013). Detection of the nodulation factors released by the rhizobia, triggers cell proliferation in the pericycle-inner cortex of the root to originate nodule primordia (Xiao et al., 2014). As nodules grow, rhizobia from the root surface are directed by infection threads to the nodule cells (Gage, 2002). There, they are released in an endocytic-like process, originating pseudo-organelles known as symbiosomes (Roth and Stacey, 1989; Catalano et al., 2006). Within the symbiosomes, rhizobia differentiate into bacteroids and express the enzymatic machinery required for nitrogen fixation (Kondorosi et al., 2013). Nodule development follows either an indeterminate or a determinate growth pattern, based on whether they maintain an apical meristem to sustain growth (Vasse et al., 1990). As this meristem allows for sustained growth in indeterminate nodules, four developmental zones appear: the meristematic region or zone I; the infection-differentiation zone or zone II, where rhizobia are released in the cell and start differentiating; the fixation zone or zone III, where nitrogenase is active; and the senescent zone or zone IV, where symbiosomes are degraded and nutrients recycled (Burton et al., 1998). In addition, some authors define a transition interzone between zones II and III (Roux et al., 2014).
Nutrient exchange between the symbionts enables nitrogen fixation (Udvardi and Poole, 2013). Availability of fixed nitrogen forms in soils inhibits nodulation (Streeter, 1987). Similarly, low levels of photosynthates, phosphate, or sulphate transfer from the host plant decrease nodulation and nitrogen fixation rates (Singleton and van Kessel, 1987; Valentine et al., 2017; Schneider et al., 2019). Transition metals such as iron, copper, zinc, or molybdenum are also critical for nodulation and nitrogen fixation as cofactors in many of the involved enzymes (González-Guerrero et al., 2014; González-Guerrero et al., 2016). This includes not only nitrogenase (Rubio and Ludden, 2005), but also nicotinamide adenine dinucleotide phosphate (NADPH)-oxidases that participate in nodule signaling (Montiel et al., 2016), leghemoglobin that maintains nodule O2 homeostasis (Appleby, 1984), high-affinity cytochrome oxidases providing energy to the bacteroids (Preisig et al., 1996), as well as many enzymes involved in free radical control (Dalton et al., 1998; Santos et al., 2000; Rubio et al., 2007). Consequently, deficiencies in the uptake of these nutrients or alterations in the metal delivery pathways lead to defects in nodulation and/or nitrogen fixation (Tang et al., 1991; O'Hara, 2001; Senovilla et al., 2018; Gil-Díez et al., 2019).
To reach the bacteroids, metals must first cross from soil into the roots using the general mechanisms common to all dicots (Kobayashi and Nishizawa, 2012; Curie and Mari, 2017). Metal uptake is facilitated by soil acidification, the release of phenolics/coumarins and flavins, and cation reduction when required (Jain et al., 2014). Metals are then introduced into the root epidermis and symplastically or apoplastically reach the root endodermis, to cross into the vasculature, and delivered to sink organs. In model legume Medicago truncatula, metals are released from the vessels into the apoplast of the infection-differentiation zone of nodules (Rodríguez-Haas et al., 2013). These nutrients will be introduced in rhizobia-infected cells and targeted to symbiosomes for nitrogen fixation. In recent years, many of the membrane transporters participating in metal transfer from the plant to the bacteroids have been identified. For instance, iron transfer to nitrogen-fixing cells is facilitated by plasma membrane iron uptake protein MtNramp1 (Tejada-Jiménez et al., 2015), and its transport across the symbiosome membrane by MtSEN1 and MtFPN2 (Hakoyama et al., 2012, Escudero et al., 2019b). However, little is known on how metals are sorted intracellularly and on the speciation of these elements.
Unlike alkali or alkali-earth elements, transition metals are not “free,” hydrated, in physiological solutions. Instead, they are bound to a plethora of organic molecules that maintain them soluble under different pH, prevent metal-catalyzed production of free radicals in Fenton-style reactions, and avoid mis-metallation of enzymes (Finney and Halloran, 2003; Rellán-Álvarez et al., 2008; Flis et al., 2016). Systematic studies of the nature of these chemical species in the sap of model plants have revealed the importance of citrate and nicotianamine in this role (von Wiren et al., 1999; Durrett et al., 2007; Roschzttardtz et al., 2011; Schuler et al., 2012). Citrate is the main iron chelator in xylem and facilitates iron delivery across symplastically disconnected tissues (Durrett et al., 2007; Rellán-Álvarez et al., 2010; Roschzttardtz et al., 2011). It has also been associated with iron trafficking to nodules (LeVier et al., 1996). Citrate efflux proteins LjMATE1 and MtMATE67 are required for iron allocation to nodules and contribute to nitrogen fixation (Takanashi et al., 2013; Kryvoruchko et al., 2018). Citrate efflux is relevant for iron delivery to bacteroids, as indicated by the symbiosome localization of nodule-specific protein MtMATE67 (Kryvoruchko et al., 2018).
Nicotianamine is also an important player in plant metal homeostasis. This molecule is a non-proteinogenic amino acid synthesized by nicotianamine synthases (NAS) from S-adenosyl methionine (Higuchi et al., 1999). Nicotianamine-metal complexes mediate long-distance metal trafficking, particularly along the phloem, as well as participate in vacuolar metal storage (von Wiren et al., 1999; Haydon et al., 2012; Flis et al., 2016). A nodule-specific NAS gene was identified in senescent nodules of L. japonicus, likely participating in the metal redistribution to the developing flowers and embryos, as orthologues do with older leaves (Hakoyama et al., 2009; Schuler et al., 2012). No such nodule-specific NAS gene can be found in transcriptomic databases from indeterminate type nodules, but tentative evidence shows that a M. truncatula NAS protein, MtNAS1, might be responsible for iron allocation to these organs (Avenhaus et al., 2016). Here, we have characterized a second NAS protein, MtNAS2, identified in a screening of M. truncatula Tnt1-insertion mutants. This gene, although primarily expressed in roots, is important for metal allocation for symbiotic nitrogen fixation.
Materials and Methods
Biological Material and Growth Conditions
M. truncatula Gaertn R108 and nas2-1 (NF15101) seeds were scarified in concentrated sulfuric acid (96%) for 7.5 min. After removing the acid, the seeds were washed eight times with cold water, and surface-sterilized with 50% (v/v) bleach for 90 s. Seeds were embedded overnight in the dark at room temperature in sterile water, and transferred to 0.8% water-agar plates for 48 h at 4°C (stratification). Germination was carried out at 22°C in the dark. Seedlings were planted on sterile perlite pots, and inoculated with Sinorhizobium meliloti 2011 or the same bacterial strain transformed with pHC60 (Cheng and Walker, 1998). Plants were grown in a greenhouse under 16 h light/8 h dark at 25/20°C conditions. In the case of perlite pots, plants were watered every 2 days with Jenner's solution or water alternatively (Brito et al., 1994). Nodules were obtained at 28 dpi. Plants growing in non-symbiotic conditions were watered every 2 weeks with Jenner's solution supplemented with 20 mM NH4NO3. For hairy-root transformation experiments, M. truncatula seedlings were transformed with Agrobacterium rhizogenes strain ARqua1, fused to the appropriate binary vector as described (Boisson-Dernier et al., 2001). Briefly, the root tips of M. truncatula seedlings were removed and the wound was placed in contact with a fresh solid culture of A. rhizogenes ARqua1 containing the binary vector of interest. These seedlings were placed in Fahraeus medium (Fahraeus, 1957) containing 50 μg/ml kanamycin to select for transformed roots, and transferred a germination chamber at 16 h light/8 h dark at 25/20°C conditions. After 3 weeks, plants that had developed roots were placed in perlite pots and inoculated as indicated above.
Ribonucleic Acid Extraction and Quantitative Real-Time Polymerase Chain Reaction
RNA was extracted from 28 dpi plants using TRI-reagent (Life Technologies), treated using DNase turbo (Life Technologies), and cleaned with RNeasy Mini-Kit (Qiagen). Complementary DNA (cDNA) was obtained from 500 ng RNA using PrimeScript RT reagent Kit (Takara). Expression studies were carried out by real-time reverse transcription polymerase chain reaction (RT-qPCR; StepOne plus, Applied Biosystems) using the Power SyBR Green Master Mix (Applied Biosystems). The primers used are indicated in Supplementary Table 2. cDNA levels were normalized by using the ubiquitin conjugating enzyme E2 (Medtr7g116940) gene as internal standard. Real time cycler conditions have been previously described (González-Guerrero et al., 2010). Expression levels were determined in three independent experiments with four pooled plants.
β-Glucuronidase Staining
MtNAS2 promoter region was obtained by amplifying the 1,940 bp upstream of the start codon using the primers indicated in Supplementary Table 2, and cloned by Gateway Cloning Technology (Invitrogen) in pDONR207 (Invitrogen) and transferred to destination vector pGWB3 (Nakagawa et al., 2007). Hairy-root transformations of M. truncatula seedlings were carried out with A. rhizogenes ARqua1 as described by Boisson-Dernier et al. (2001). After 3 weeks on Fahraeus media plates with kanamycin (50 µg/ml), plant transformants were transferred to sterilized perlite pots and inoculated with S. meliloti 2011. GUS activity was determined in 28 dpi plants as described (Vernoud et al., 1999). Whole nodule and root images were taken with a Leica MZ10F dissecting microscope using the Leica AF software. Some nodules and roots were embedded in 6% agarose and sectioned in 100 μm slides using a Vibratome 1000 Plus. These sections were observed in a Zeiss Axiophot microscope using the Leica AF software.
Immunolocalization and Imaging
The coding sequence region of MtNAS2 and 1,940 bp upstream of its start codon were cloned in pGWB13 vector (Nakagawa et al., 2007) using Gateway Cloning Technology (Invitrogen). This fuses three HA epitopes to C-terminus of the protein. Hairy-root M. truncatula transformants were transferred to sterilized perlite pots and inoculated with S. meliloti 2011 containing the pHC60 plasmid that constitutively expresses GFP. Nodules and roots were collected from 28 dpi plants and fixed at 4°C overnight in 4% para-formaldehyde and 2.5% sucrose in phosphate-buffered saline (PBS). Fixative was removed by washing for 5 min in PBS and 5 min in water. Nodule and roots were included in 6% agarose for sectioning with a Vibratome 1000 Plus. Sections were dehydrated by serial incubation with methanol (30, 50, 70, and 100% in PBS) for 5 min and then rehydrated following the same methanol series in reverse order. Cell wall permeabilization was carried out by incubation with 2% (w/v) cellulase in PBS for 1 h and 0.1% (v/v) Tween 20 for 15 min. Sections were blocked with 5% (w/v) bovine serum albumin in PBS and then incubated with 1:50 anti-HA mouse monoclonal antibody (Sigma) in PBS at room temperature for 2 h. Primary antibody was washed three times with PBS for 15 min and subsequently incubated with 1:40 Alexa 594-conjugated anti-mouse rabbit monoclonal antibody (Sigma) in PBS at room temperature for 1 h. Secondary antibody was washed three times with PBS for 10 min. DNA was stained using 4′,6-diamidino-2-phenylindol (DAPI). Images were obtained with a confocal laser-scanning microscope (Leica SP8) using excitation light at 488 nm to GFP and 561 nm for Alexa 594, collecting the emission at 500–554 nm for GFP and at 597-654 for Alexa594.
Acetylene Reduction Assays
Nitrogenase activity assay was measured by acetylene reduction test (Hardy et al., 1968). Wild-type and nas2-1 nodulated roots from 28 dpi were separately introduced in 30 ml vials. Each tube contained four or five independently transformed plants. Three milliliters of air from each bottle was replaced by the same volume of acetylene, tubes were subsequently incubated for 30 min at room temperature. Gas samples were measured by analyzing 0.5 ml of ethylene from each bottle in a Shimadzu GC-8A gas chromatograph using a Porapak N column. The amount of the ethylene produced was determined by measuring the ethylene peaks relative to the standards. Acetylene reduction was measured in duplicate from three sets of three-four pooled plants.
Chlorophyll Content Assays
Total chlorophyll content was determined as previously described with some modifications (Inskeep and Bloom, 1985). Leaves were collected from 28 dpi plants and poled to obtain 50 mg of fresh material. Chlorophyll was extracted with 500 µl of di-methyl-formamide at 4°C overnight. Leaves were centrifuged for 5 min at 600 g at room temperature. After transferring the supernatant to another vial, the chlorophyll extraction was repeated with the same leave using strong vortexing. After spinning for 5 min at 600 g, the supernatant was pooled with the previous one. Chlorophyll was quantified at 647 and 664 nm in a Ultrospec 3300 Spectrophotometer (Amersham Bioscience). Data are the mean ± SE of three sets of five pooled plants.
Metal Content Determination
Shoots, roots, and nodules were collected from 28 dpi plants and mineralized with 15.6 M HNO3 (trace metal grade) at 75°C for 3 h and 2 M H2O2 at 20°C overnight. Metal quantifications were performed in duplicate by Atomic Absorption Spectroscopy, using a Perkin Elmer PinAAcle 900Z GF-AAS equipment. Metal concentration was normalized against fresh tissue weight. Three sets of three-four pooled organs were analyzed.
Synchrotron Radiation X-Ray Fluorescence Spectroscopy and X-Ray Absorption Near-Edge Spectroscopy
X-ray fluorescence spectroscopy (XRF) hyperspectral images and µXANES spectra were acquired on the beamline ID21 of the European Synchrotron Radiation Facility (Cotte et al., 2017), at 110 K in the liquid nitrogen (LN2) cooled cryostat of the Scanning X-ray Micro-spectroscopy end-station. Seven sections from M. truncatula R108 nodules and five from nas2-1 nodules were obtained from independent nodules embedded in optimal cutting temperature (OCT) medium and cryo-fixed by plunging in isopentane chilled with LN2. The 25 µm-thick sections of frozen samples were obtained using a Leica LN2 cryo-microtome and accommodated in a Cu sample holder cooled with LN2, sandwiched between Ultralene (SPEX SamplePrep) foils. The beam was focused to 0.4×0.9 µm2 with a Kirkpatrick-Baez (KB) mirror system. The emitted fluorescence signal was detected with energy-dispersive, large area (80 mm2) SDD detector equipped with Be window (SGX from RaySpec). Images were acquired at the fixed energy of 7.2 keV, by raster-scanning the sample in the X-ray focal plane, with a step of 3×3 µm2 and 100 ms dwell time. Elemental mass fractions were calculated from fundamental parameters with the PyMCA software package, applying pixel-by-pixel spectral deconvolution to hyperspectral maps normalized by the incoming flux (Solé et al., 2007). The incoming flux was monitored using a drilled photodiode previously calibrated by varying the photon flux at 7.2 keV obtaining a response of 1,927.9 charges/photon with a linear response up to 200 kcps. In PyMCA the incoming flux and XRF detector parameters were set to 2x109 photons/s, 0.7746 cm2 active area, and 4.65 cm sample to XRF detector distance. Sample matrix was assumed to be amorphous ice (11% H, 89% O, density 0.92 g/cm3), the sample thickness set at 25 µm obtained with the use of a cryo-microtome. Iron average mass fraction values from pixels in zone III and apical region from three wild type and three nas2-1 nodule samples were obtained using a mask tool that removes pixels with mass fractions below a threshold of 2 10−6 μg iron.
Fe-K edge (7.050 to 7.165 keV energy range, 0.5 eV step) µXANES spectra were recorded in regions of interest of the fluorescence maps acquired on ID21 beamline. Individual spectra were processed using Orange software with the Spectroscopy add-on (Demsar et al., 2013). The pre-processing step consisted of vector normalization and a Savitzky-Golay filter for the smoothing. Then a principal component analysis was performed on the second derivative of the spectra to highlight potential differences among genotypes within a given region of the nodule. A reference library was used for linear combination fitting (LCF) procedure. This library consisted of: Fe-foil [Fe(0)], Fe(II)-nicotianamine, Fe(II)S2 (http://ixs.iit.edu/database/), Fe(III)-haem (50 mM, pH7, bought from Sigma, CAS number: 16009-13-5), Fe(III)-cellulose (5 mM FeCl3 + 50 mM cellulose, pH 5.8, bought from Sigma, CAS number: 9004-34-6), Fe(III) glutamic acid (5 mM FeCl3 + 50 mM glutamic acid, pH 7, bought from Sigma, CAS number: 56-86-0), and Fe(III) ferritin (bought from Sigma, CAS number: 9007-73-2, 50 mM, pH7).Reference compounds were classified as Fe(II)-S (FeS2), Fe(II)-O/N (Fe-NA) and Fe(III)-O (Fe-cellulose, Fe-glutamic acid, and ferritin). XANES data treatment was performed using Athena software (Ravel and Newville, 2005) as previously described (Larue et al., 2014).
Statistical Tests
GraphPad Prism was used for the statistical analyses. Data were analyzed by ANOVA to test the differences between wild type, mutant and complemented M. truncatula plants. Tukey's or Student's t tests were used to calculate statistical significance of observed differences. Test results with p-values < 0.05 were considered as statistically significant
For nodule structure analysis and nicotianamine concentration determination see Supplementary Materials and Methods.
Results
Medicago truncatula Tnt1 Line NF15101 Phenotype Is Due to Transposon Insertion in MtNAS2
A search for metal-related symbiotic phenotypes of the mutants available at the Noble Research Institute LLC M. truncatula Mutant Database (Sun et al., 2019; https://medicago-mutant.noble.org/mutant/index.php) showed NF15101 as one of the available mutants with a nitrogen fixation deficient phenotype. This line has 22 Tnt1 insertions, 10 of which interrupted different M. truncatula genes (Supplementary Table 1), Medtr2g070310 among them. This gene encodes a protein with 52% identity and 67% similarity to Arabidopsis thaliana NAS2 protein, and consequently was renamed MtNAS2. MtNAS2 was expressed at similar levels in roots from plants inoculated or non-inoculated with S. meliloti (Figure 1A). Significantly, lower expression was observed in nodules, and no signal was detected in shoots from either inoculated or non-inoculated plants. Tnt1 was inserted in position +760 of MtNAS2 (Figure 1B), interrupting the reading frame of its only exon. Homozygous plants from a R2 seed population were identified by a PCR reverse screening (Cheng et al., 2014). These homozygous plants were considered as null mutants for MtNAS2, since MtNAS2 expression levels were below our detection limit (Figure 1C). As expected, NF15101, nas2-1 in this report, had reduced biomass production in nitrogen fixation conditions (Figures 2A, B). While nodule development and nodule number were not significantly altered in nas2-1 compared to wild type (Figures 2C, D, Supplementary Figure 1), nitrogenase activity was reduced three-fold in nas2-1 plants (Figure 2E). No significant differences in nicotianamine concentration were observed between wild-type and mutant plants (Supplementary Figure 2). The nas2-1 phenotype was reverted when a wild-type copy of MtNAS2 regulated by its own promoter was reintroduced in nas2-1 (Figure 2). No significant changes in nitrogenase activity were observed either when iron and/or zinc were removed from the nutrient solution or when they were added at higher concentrations (Supplementary Figure 3) The data indicate that among all the Tnt1 insertions, loss of MtNAS2 function was determinant for the reduction of nitrogenase activity and overall growth alterations.
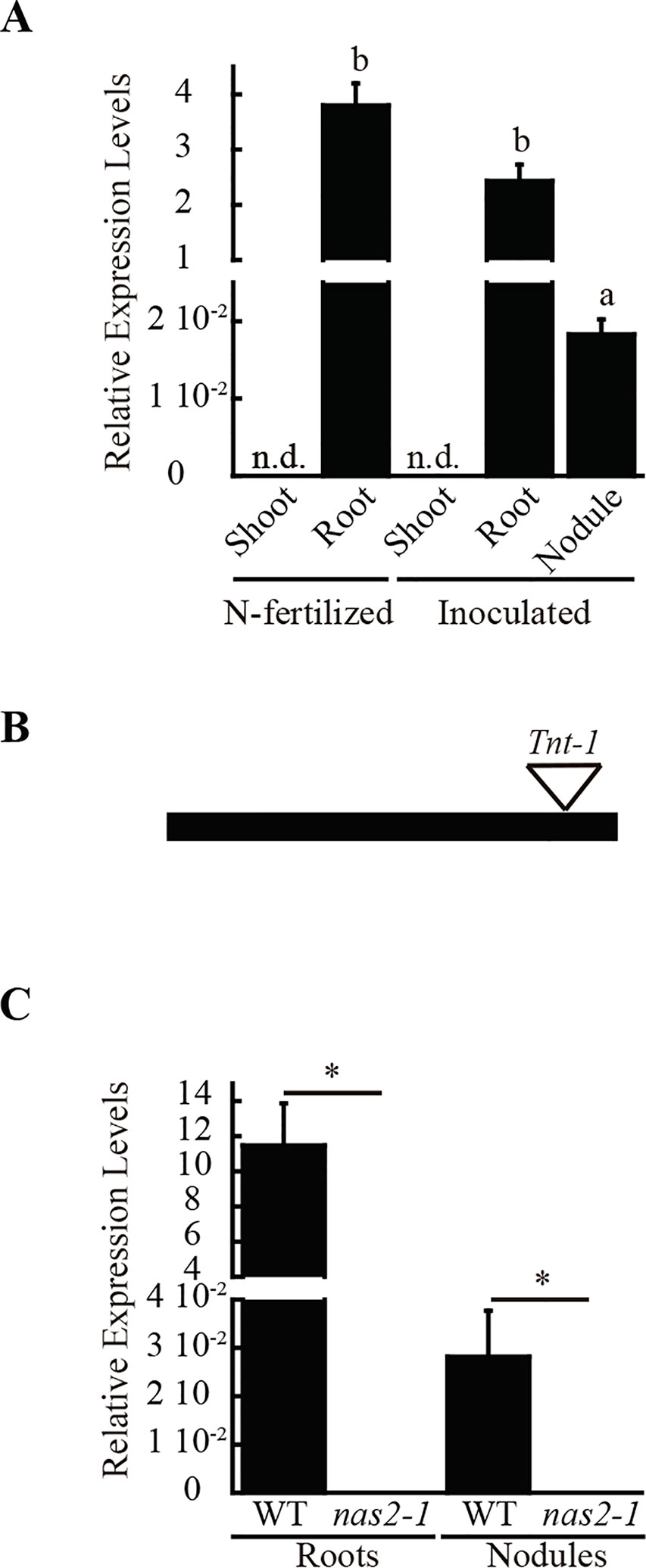
Figure 1 MtNAS2 is expressed in roots and nodules of M. truncatula. (A) Expression data was normalized to the expression of ubiquitin conjugating enzyme E2 gene (Medtr7g116940) as standard. Data are the mean ± SE of three independent experiments with 4 pooled plants. Different letters indicate statistical differences at P > 0.05 (Tukey t-test). (B) Tnt1 insertion in the only exon of MtNAS2 causes loss of MtNAS2 transcripts. (C) MtNAS2 expression was determined in 28 dpi roots and nodules of wild-type (WT) and nas2-1 plants. Data was relativized to the expression of ubiquitin conjugating enzyme E2 (Medtr7g116940) and expressed as mean ± SE of three independent experiments with 4 pooled plants. *indicates statistical differences at P > 0.05 (Student t-test).
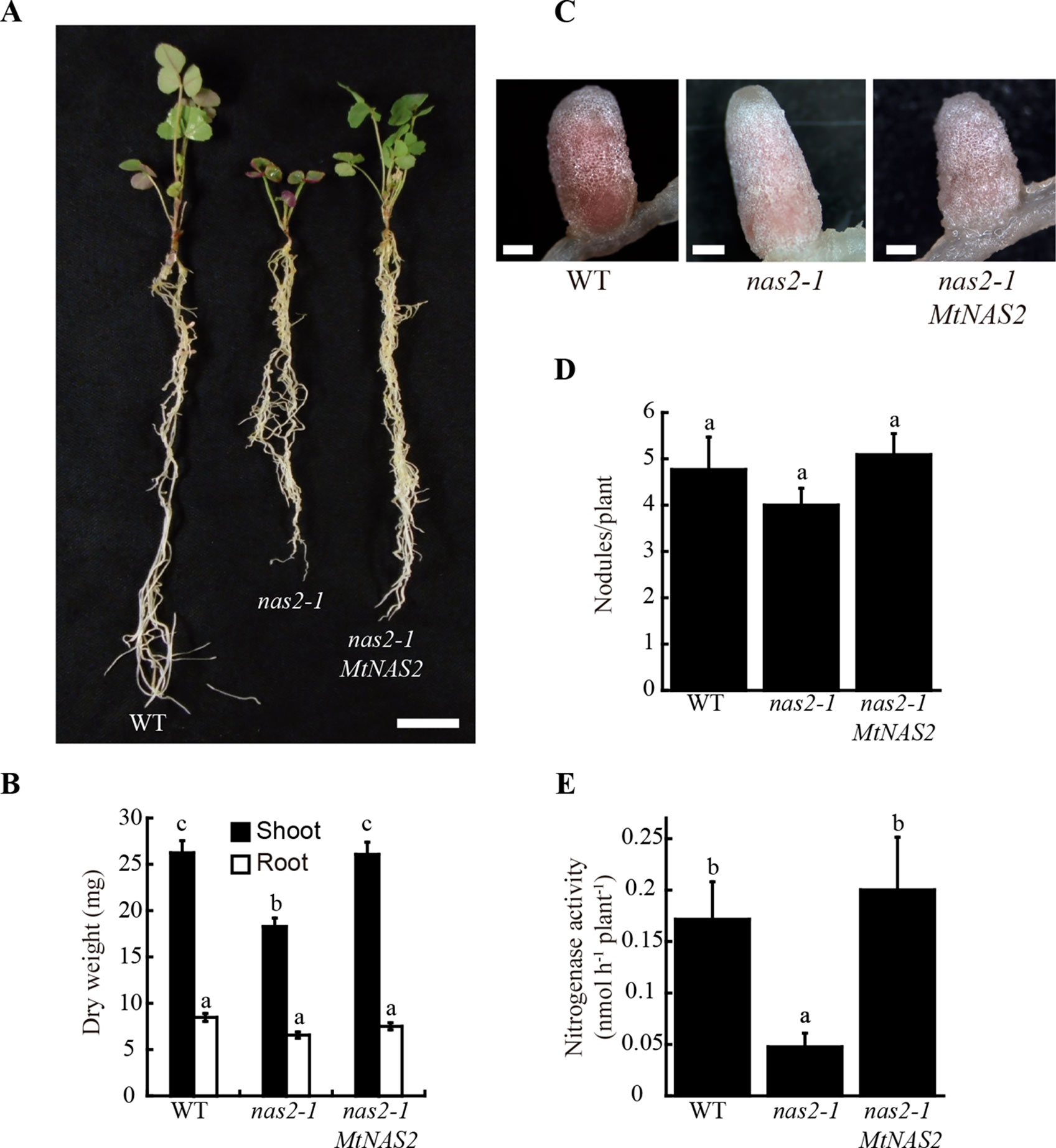
Figure 2 MtNAS2 is required for nitrogen fixation. (A) Growth of representative wild-type (WT), nas2-1, and nas2-1 plants transformed with MtNAS2 controlled by its own promoter (nas2-1 MtNAS2). Bar = 1.5 cm. (B) Dry weight of WT, nas2-1, and nas2-1 MtNAS2 plants. Data are the mean ± SE of at least 9 transformed plants. (C) Detail of representative nodules of WT, nas2-1, and nas2-1 MtNAS2 plants (n = 40–50 nodules). Bars = 500 μm. (D) Number of nodules in 28 dpi WT, nas2-1, and nas2-1 MtNAS2 plants. Data are the mean ± SE of at least nine transformed plants. Different letters indicate statistical differences at P < 0.05 (Tukey t-test). (E) Nitrogenase activity in 28 dpi nodules from WT, nas2-1, and nas2-1 MtNAS2 plants. Acetylene reduction was measured in duplicate from three sets of three-four pooled plants. Data are the mean ± SE. Different letters indicate statistical differences at P < 0.05 (Tukey t-test).
MtNAS2 Is Not Required for Plant Growth Under Non-Symbiotic Conditions
To determine whether the symbiotic phenotype of nas2-1 was the result of additional physiological processes being affected, these plants and their controls were grown in the same conditions as above, but supplemented with ammonium nitrate in the nutrient solution to compensate for the lack of rhizobial inoculation. In these conditions, no significant differences were found in plant growth, biomass production, or chlorophyll content between wild-type and nas2-1 plants (Figure 3). Considering the role of nicotianamine in plant iron homeostasis (von Wiren et al., 1999; Inoue et al., 2003) and the added pressure of symbiotic nitrogen fixation on iron nutrition (Terry et al., 1991), nas2-1 phenotype was also studied under non-symbiotic, low-iron conditions (no iron added to the nutrient solution). Low-iron supply did not lead to different growth between control and nas2-1 plants (Supplementary Figure 4).
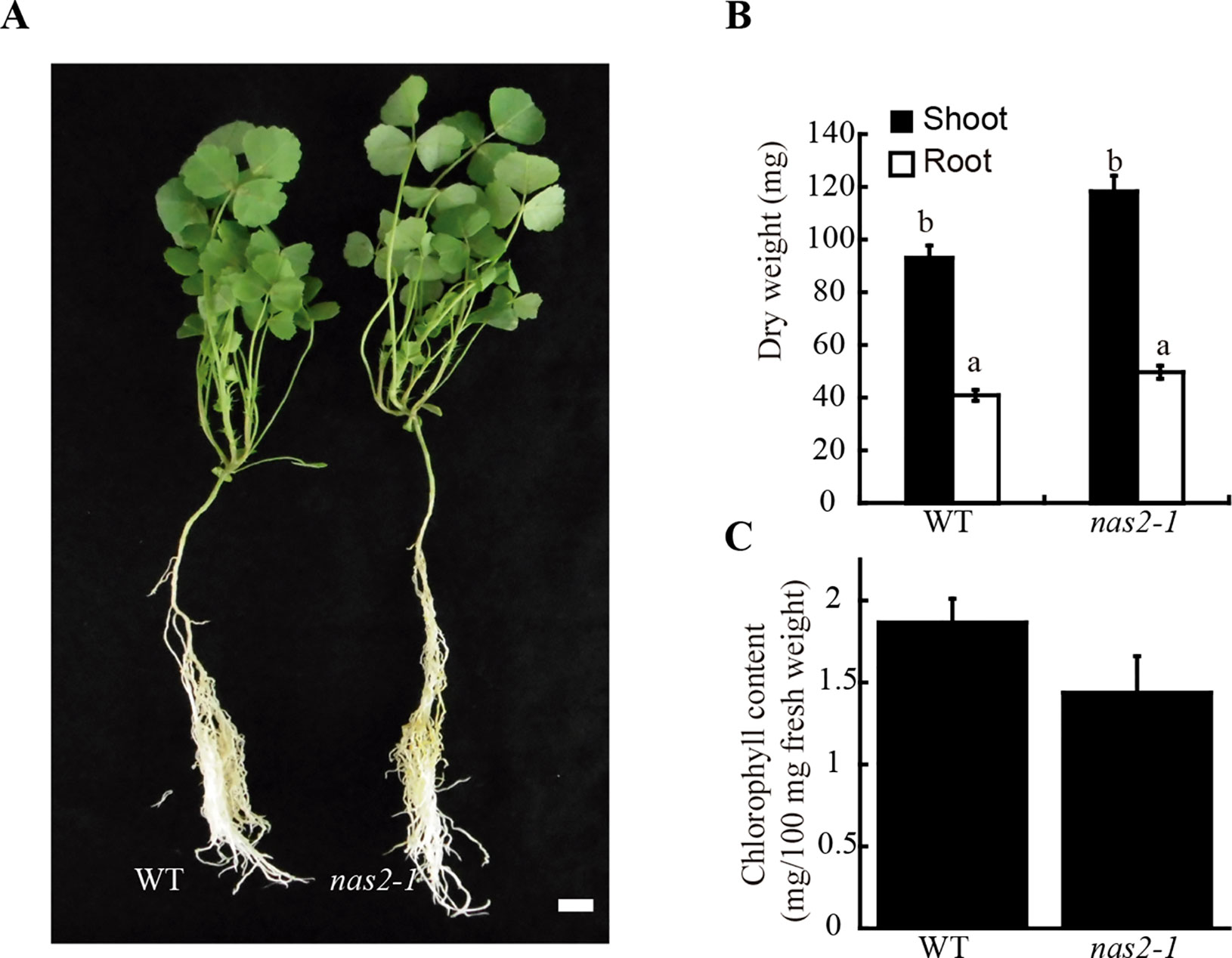
Figure 3 MtNAS2 is not required for plant growth under non-symbiotic conditions. (A) Growth of representative wild-type (WT) and nas2-1 plants when watered with a nutrient solution supplemented with ammonium nitrate and not inoculated with Sinorhizobium meliloti (n = 10–15 plants). Bar = 1.5 cm. (B) Dry weight of WT and nas2-1 plants. Data are the mean ± SE (n = 10 plants). Different letters indicate statistical differences at P < 0.05 (Tukey t-test). (C) Chlorophyll concentration of wild-type and nas2-1 plants. Data are the mean ± SE of three sets of five pooled plants. No differences at P < 0.05 were observed (Student t-test).
MtNAS2 Is Expressed in the Xylem Parenchyma in Roots and in the Nodule Differentiation and Fixation Zones
The physiological role of MtNAS2 is determined by its differential tissue and cellular expression. To establish the gene tissue expression, M. truncatula plants were transformed with a binary vector containing the MtNAS2 promoter region driving the β-glucuronidase (gus) gene transcription and GUS activity visualized using X-Gluc. MtNAS2 was expressed in roots and nodules (Figure 4A), in agreement with the transcript data (Figure 1). Longitudinal section of the nodules showed GUS activity in cells from the late zone II to the fixation zone of the nodule (Figure 4B). Nodule cross-sections showed expression in all nodule tissues (Figure 4C). In roots, MtNAS2 promoter was active in vasculature cells (Figure 4D).
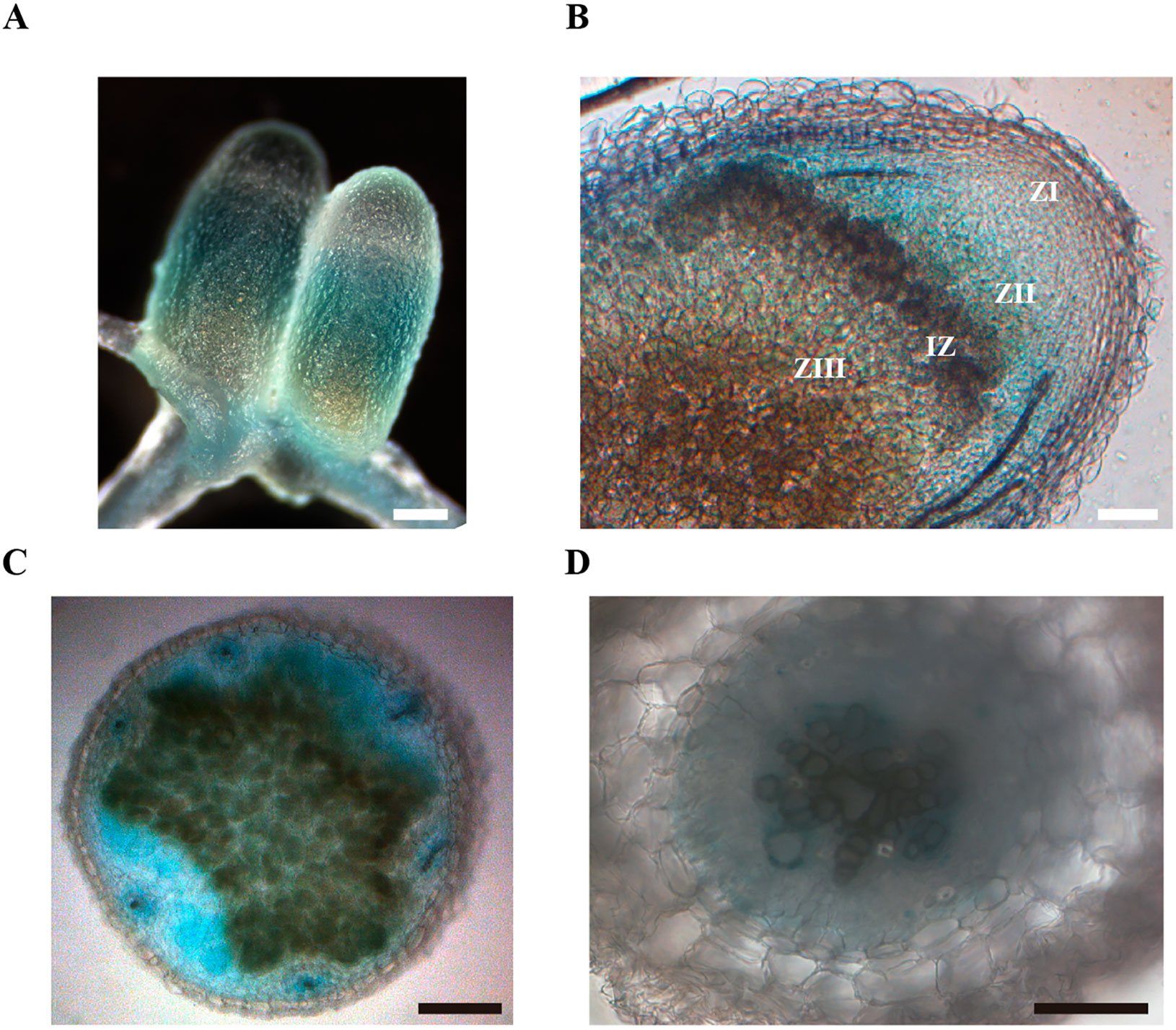
Figure 4 MtNAS2 is expressed in the root vasculature and in the late zone II, interzone, zone III, and vessels in nodules. (A) β-glucuronidase (GUS) staining of 28 dpi Medicago truncatula roots and nodules expressing the gus gene under the control of MtNAS2 promoter region (n = 10–20 plants). Bar = 100 μm. (B) Longitudinal section of a GUS-stained 28 dpi M. truncatula nodule expressing the gus gene under the control of MtNAS2 promoter region (n = 30 sections from 10 to 20 plants). ZI indicates zone I; ZII, zone II; IZ, interzone; and ZIII, zone III. Bar = 100 μm. (C) Cross section of the fixation zone of a GUS-stained 28 dpi M. truncatula nodule expressing the gus gene under the control of MtNAS2 promoter region (n = 30 sections from 10 to 20 plants). Bar = 200 μm. (D) Cross section of a GUS-stained 28 dpi M. truncatula root expressing the gus gene under the control of MtNAS2 promoter region (n = 30 sections from 10 to 20 plants). Bar = 100 μm.
Supporting the gene expression results, immunolocalization of HA-tagged MtNAS2 under control of its own promoter showed that the protein was located in cells neighboring the late zone II, interzone, and fixation zone (Figure 5A). At higher magnification, we could observe that MtNAS2-HA had a homogenous distribution within the cells, and it did not seem to cluster in any particular location (Figure 5B). Analysis of nodule vasculature showed MtNAS2-HA in endodermal cells (Figure 5C). However, in the root vasculature, MtNAS2-HA was detected at the center of the root, in the region comprised between the endodermis and the xylem (Figure 5D). At higher magnification, the root MtNAS2-HA signal was detected in small cells associated to the xylem (Supplementary Figure 5). Controls were carried out to ensure that the data did not stem from autofluorescence (Supplementary Figure 6). In addition, the antibodies used for immunolocalization do not cross react with M. truncatula proteins (Tejada-Jiménez et al., 2015).
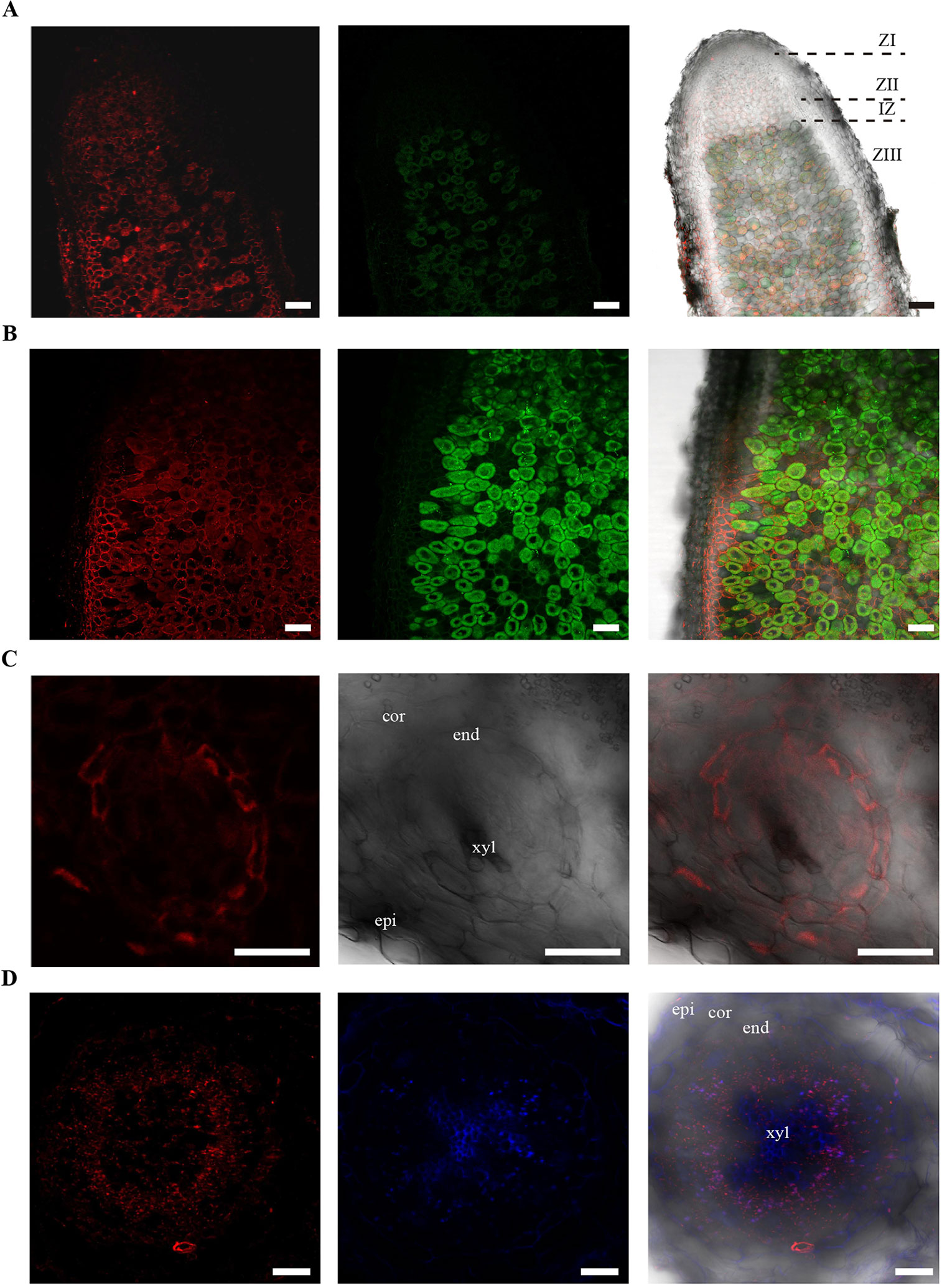
Figure 5 MtNAS2 is located in the nodule core cells, in the endodermis of the nodule vessels, and in cells surrounding the xylem in the root vasculature. (A) Longitudinal section of a 28 dpi M. truncatula nodule expressing MtNAS2-HA under its own promoter (n = 30 sections from 10 to 20 plants). The three C-terminal HA epitopes were detected using an Alexa594-conjugated antibody (red, left panel). Transformed plants were inoculated with a GFP-expressing Sinorhizobium meliloti (green, middle panel). Both images were overlaid with the transillumination image (right panel). ZI indicates zone I; ZII, zone II; IZ, interzone; and ZIII, zone III. Bars = 100 μm. (B) Detail of the zone III of a 28 dpi M. truncatula nodule expressing MtNAS2-HA under its own promoter. Left panel corresponds to the Alexa594 signal used to detect the HA-tag, middle panel corresponds to the GFP channel showing S. meliloti, and the two were overlaid with the bright field channel in the right panel (n = 30 sections from 10 to 20 plants). Bars = 50 μm (C) Cross section of a nodule vessel from a 28 dpi M. truncatula nodule expressing MtNAS2-HA under its own promoter. Left panel corresponds to the Alexa594 signal used to detect the HA-tag, middle panel corresponds to the bright field channel, and the two were overlaid in the right panel (n = 30 sections from 10 to 20 plants). epi indicates nodule epidermis; cor, nodule cortex; end, nodule vascular endodermis; and xyl, nodule vascular xylem. Bars = 50 μm. (D) Cross section from a 28 dpi M. truncatula root expressing MtNAS2-HA under its own promoter. Left panel corresponds to the Alexa594 signal used to detect the HA-tag, middle panel corresponds to autofluorescence signal of xylem, and the two were overlaid with the bright field channel in the right panel (n = 30 sections from 10 to 20 plants). epi indicates root epidermis; cor, root cortex; end, root endodermis; and xyl, xylem. Bars = 100 μm.
MtNAS2 Is Required for Efficient Metal Allocation for Symbiotic Nitrogen Fixation
Nicotianamine is required for metal allocation from source to sink tissues (Schuler et al., 2012). Alterations in nicotianamine synthesis typically lead to reduced metal delivery to sink tissues. To determine whether this was the case for nas2-1, iron, copper, and zinc levels in roots, shoots, and nodules from 28 days-post-inoculation (dpi) plants were determined. No significant changes in these levels were observed (Figure 6A). However, metal allocation might be altered while not affecting total nodule metal content. To assess this possibility, synchrotron-based X-ray fluorescence studies were carried out to determine iron distribution in nas2-1 compared to wild type (Figure 6B). These experiments showed that iron distribution was altered in nas2-1 mutants, which presented a lower percentage of iron in the fixation zone than wild type nodules (Figure 6C). To further confirm that mutation of MtNAS2 affected iron distribution in nodules as a consequence of changes of iron speciation, X-ray Absorption Near-Edge Spectroscopy (XANES) analyses of iron speciation in the different nodule developmental zones were carried out (Figure 6D). Principal component analyses of these spectra showed that the iron complexes in the fixation zone were quite different (Figure 6E). Fitting of the obtained spectra to known standards showed that the proportion of Fe-S complexes had a dramatic drop in nas2-1 compared to wild-type plants, while the proportion of O/N complexes with iron had a larger increase (Table 1).
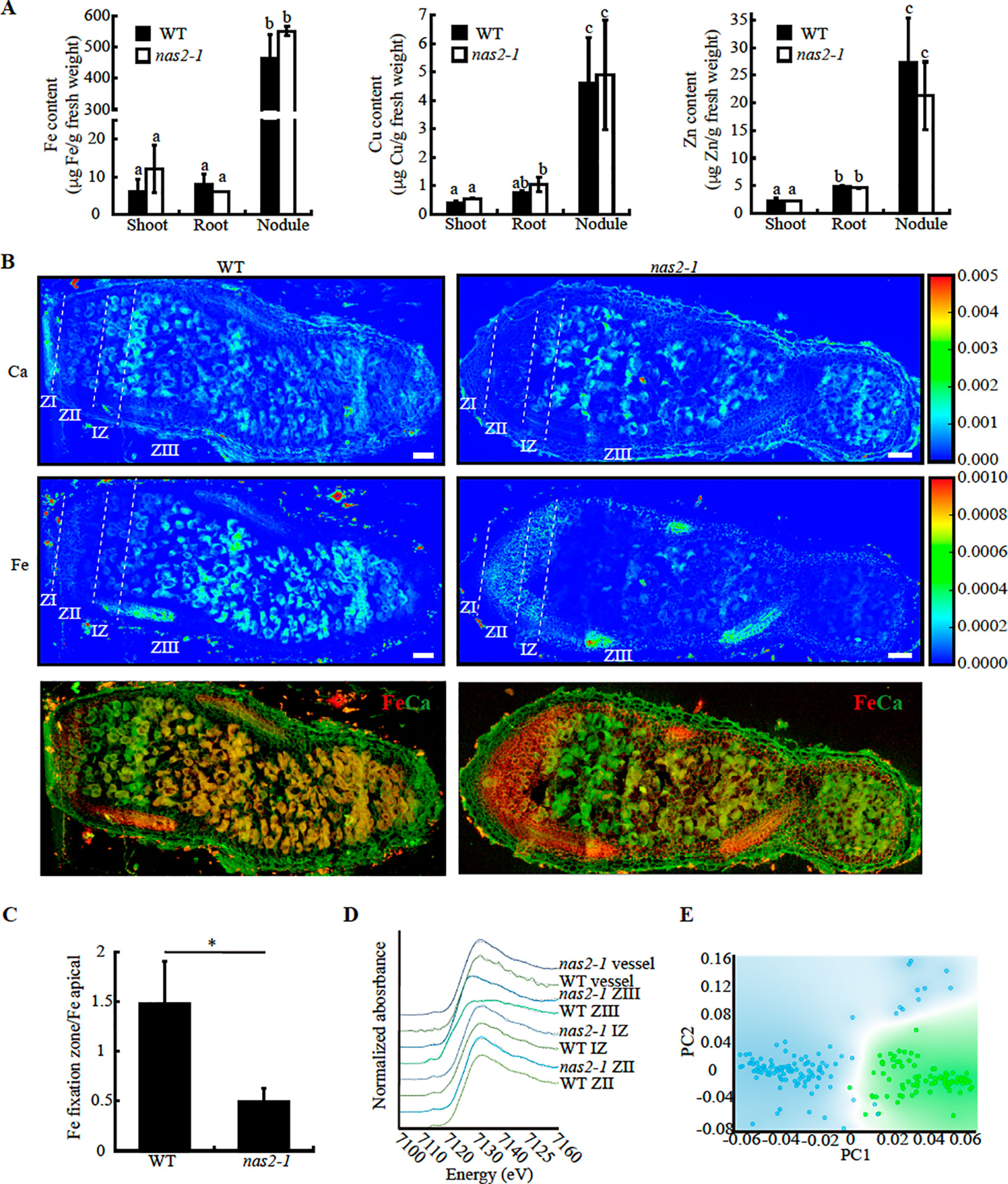
Figure 6 MtNAS2 is required for iron distribution and speciation in nodules. (A) Iron (left panel), copper (middle panel), and zinc (right panel) concentration in shoots, roots, and nodules from 28 dpi wild-type (WT) and nas2-1 plants. Data are the mean ± SE of three sets of three-four pooled organs. Different letters indicate statistical differences at P < 0.05 (Tukey t-test). (B) Synchrotron-based X-ray fluorescence images of WT (left panels) or nas2-1 (right panels) showing calcium (top panels) or iron (center panels) distribution in 28 dpi nodules. Lower panels are the overlaid iron and calcium distribution (iron is indicated in red and calcium in green). ZI indicates zone I; ZII, zone II; IZ, interzone; and ZIII, zone III (n = 5–7 nodules). Bars = 100 μm. (C) Iron content in fixation zone relative to the apical zone in wild type and nas2-1 nodules. Data are the mean ± SE (n = 3). * indicates statistical differences at P < 0.05 (Student t-test). (D) XANES spectra obtained from different regions of WT and nas2-1 nodules. (E) Decomposition of the zone III signal into its two principal components.
Discussion
Metallic micronutrient delivery to nodules is essential for symbiotic nitrogen fixation, as they are cofactors in many of the involved enzymes (Brear et al., 2013; González-Guerrero et al., 2014). In recent years, studies have shown how metals are exported to the apoplast in the infection/differentiation zone of M. truncatula nodules (Rodríguez-Haas et al., 2013), and transmembrane transporters introduce metals into rhizobia-infected cells (Tejada-Jiménez et al., 2015; Abreu et al., 2017; Tejada-Jiménez et al., 2017; Senovilla et al., 2018), or deliver iron to the bacteroids (Escudero et al., 2019b). In this transport, citrate participates in maintaining iron solubility in the apoplast, and as the preferred iron source for bacteroids (Moreau et al., 1995; LeVier et al., 1996; Kryvoruchko et al., 2018). Here we show that nicotianamine synthesis is also important for correct iron allocation to M. truncatula nodules.
Interrupting MtNAS2 expression with a transposon insertion led to reduced plant growth in symbiotic conditions, a consequence of lower nitrogenase activity. Although several genes were affected in the studied Tnt1 line, reintroduction of a wild-type copy of MtNAS2 was sufficient to restore wild-type growth. Consequently, the mutation of this gene was mainly responsible for the observed phenotype. While important under symbiotic conditions, MtNAS2 seemed to be playing a secondary role when plants were not inoculated but watered with an ammonium nitrate-supplemented nutrient solution instead. This is in contrast to the substantially higher expression levels of MtNAS2 in roots than in nodules. This observation would suggest a predominant role in nicotianamine synthesis in roots. However, studies in A. thaliana reveal the existence of a high redundancy rate in the NAS family, where a quadruple nas mutant was required to observe a substantial phenotype, including limited growth (Klatte et al., 2009). Similarly, no significant changes in nicotianamine content were observed in single nas A. thaliana lines, as neither was observed in M. truncatula nas2-1. Two possible causes might explain the symbiosis-specific phenotype of nas2-1 plants. One of them is that MtNAS2 would be required to compensate for the enhanced iron requirements of nodulated plants. This additional nutritional pressure would trigger the observed nas2-1 phenotype. If so, we should have also observed a similar phenotype when plants were watered with an iron-restricted nutrient solution, which has been shown in the past to elicit the iron deficiency response in M. truncatula (Andaluz et al., 2009; Tejada-Jiménez et al., 2015). However, this was not observed. Alternatively, in a more parsimonious mechanism, neofunctionalization of pre-existing genes during the development of symbiotic nitrogen fixation might have led to the loss of functional redundancy. Similar observations have been made when studying other M. truncatula metal homeostasis genes that, although expressed in roots and in nodules, exhibit phenotypes limited to nodulation and nitrogen fixation (Tejada-Jiménez et al., 2015; Abreu et al., 2017; León-Mediavilla et al., 2018).
In roots, MtNAS2 was expressed at high levels in xylem parenchyma cells, similarly to rice NAS2 (Inoue et al., 2003). Vascular localization of NAS proteins is not unusual, since they have been associated to long distance metal trafficking (von Wiren et al., 1999; Kumar et al., 2017). In nodules, MtNAS2 was also associated to the vessels. However, the cellular localization of the protein is different to what was observed in roots; in nodules, most of vascular MtNAS2 was confined to the endodermis. This alternative distribution of MtNAS2 in vessels could be indicative of differential functions. Root vascular localization could indicate a role in metal loading of the vascular fluids, while endodermal localization in nodules might mediate either uptake from saps or intracellular metal trafficking. In any case, it seems unlikely that the nicotianamine synthesized by nodule endodermal cells would end up in the apoplast, since citrate-iron complexes seem to be formed in this compartment at a pH that does not facilitate iron-nicotianamine association (Rellán-Álvarez et al., 2008).
MtNAS2 expression in nodule core cells in the late zone II, interzone, and zone III also indicates a role of nicotianamine in metal homeostasis of nitrogen fixing cells. It has been previously described that nicotianamine can participate in intracellular metal trafficking and in cell-to-cell metal delivery, as well as serve as intracellular storage of metals (Haydon et al., 2012). Mutation of MtNAS2 did not significantly alter iron, copper, or zinc levels in any of the plant organs analyzed, but a major shift in iron distribution was observed in nodules, with a significant decrease of iron accumulation in the interzone and early fixation zone. This would indicate that iron trafficking in these cells is altered. However, MtNAS2-mediated iron trafficking would only affect a subset of the nodule iron-proteome, since delivery to the fixation zone was not completely blocked as attested by the red color of nodules, indicative that leghemoglobin (an important iron sink) was being produced in addition to a residual nitrogenase activity. This could suggest the existence of differential metalation pathways in nodules that might serve different subsets of proteins, which could partially complement each other under stress conditions. Supporting this hypothesis, mutation of MtNAS2 did not equally affect all the iron species in the fixation zone. While the percentage of iron-sulfur complexes detected by XANES was significantly lower than in control plants, iron coordinated by nitrogen or oxygen atoms was increased. Considering the high demand for iron-sulfur clusters for nitrogenase assembly (Rubio and Ludden, 2005), its decrease could explain the reduction of nitrogenase activity observed. The changes in iron speciation were particularly severe in the fixation zone, which is consistent with MtNAS2 distribution, with the observed reduction of nitrogenase activity, and with the iron distribution data. It is important to indicate that we cannot rule out similar effects on copper or zinc speciation and distribution, since the synchrotron setup available to us at the European Synchrotron Radiation Facility prevented us to carry out similar analyses on those two elements.
This work highlights the importance of MtNAS2 in iron delivery for symbiotic nitrogen fixation. This is not the only NAS gene that might be involved in the process, since total nicotianamine production is sustained in nodules, and other family members have been shown to be expressed in these organs, such as MtNAS1 (Avenhaus et al., 2016) or MtNAS3 (Medtr7g112130) according to the Symbimics database (Roux et al., 2014). However, no MtNAS4 (Medtr2g034240) expression in roots or nodules is reported in this database. The localization of MtNAS2 indicates that nicotianamine would be involved in intracellular iron trafficking that is highly important for nitrogenase functioning. This role would not be directly providing the element to the bacteroid, since iron-citrate seems to be the key here, but perhaps would shuttle this element in the cytosol. However, to better define this possibility, new tools in elemental imaging and speciation with higher resolution within a cell need to be established to track iron and other elements. In addition, the roles of zinc-induced facilitator (ZIF)-like (Haydon et al., 2012) and yellow stripe-like (YSL) proteins (Waters et al., 2006) in symbiotic nitrogen fixation must be determined. Finally, other NAS proteins might facilitate iron recycling in M. truncatula nodules, as it occurs in L. japonicus (Hakoyama et al., 2009).
Data Availability Statement
All datasets generated for this study are included in the article/Supplementary Material.
Author Contributions
VE carried out most of the experimental work. IA, CL, and HC-M carried out the synchrotron-based work. IA, JC-G, JA, and AÁ-F were responsible for the nicotianamine content determination. ES performed the phenotypic analyses under changing metal concentrations and participated in the complementation assays. MT-J carried out the initial expression analyses. JW and KM obtained the nas2-1 mutant. LN-A and JMA determined metal concentrations. JI and MG-G were responsible for experimental design, data analyses, and wrote the manuscript with contributions from all authors.
Funding
This research was funded by a European Research Council Starting Grant (ERC-2013-StG-335284) and a Ministerio de Economía y Competitividad (MINECO) grant (AGL2015-65866-P), to MG-G, and a MINECO grant (AGL2016-75226-R) to JA and AÁ-F. VE was partially funded by the Severo Ochoa Programme for Centres of Excellence in R&D from Agencia Estatal de Investigación of Spain (grant SEV-2016-0672) to CBGP. IA is recipient of a Juan de la Cierva- Formación postodoctoral fellowship from Ministerio de Ciencia, Innovación y Universidades (FJCI-2017-33222). Development of M. truncatula Tnt1 mutant population was, in part, funded by the National Science Foundation, USA (DBI-0703285) to KM.
Conflict of Interest
The authors declare that the research was conducted in the absence of any commercial or financial relationships that could be construed as a potential conflict of interest.
Acknowledgments
We would like to thank Dr. Marine Cotte and Dr Juan Reyes-Herrera for assistance in using beamline ID21 during experiments EV246 and EV323. We would also like to acknowledge the other members of laboratory 281 at Centro de Biotecnología y Genómica de Plantas (UPM-INIA) for their support and feedback in preparing this manuscript. This manuscript has been released as a pre-print at BioRxiv (Escudero et al. BioRxiv doi 10.1101/717983).
Supplementary Material
The Supplementary Material for this article can be found online at: https://www.frontiersin.org/articles/10.3389/fpls.2019.01780/full#supplementary-material
References
Abreu, I., Saez, A., Castro-Rodríguez, R., Escudero, V., Rodríguez-Haas, B., Senovilla, M., et al. (2017). Medicago truncatula Zinc-Iron Permease6 provides zinc to rhizobia-infected nodule cells. Plant Cell Environ. 40, 2706–2719. doi: 10.1111/pce.13035
Andaluz, S., Rodríguez-Celma, J., Abadía, A., Abadía, J., López-Millán, A.-F. (2009). Time course induction of several key enzymes in Medicago truncatula roots in response to Fe deficiency. Plant Physiol. Biochem. 47, 1082–1088. doi: 10.1016/j.plaphy.2009.07.009
Appleby, C. A. (1984). Leghemoglobin and Rhizobium respiration. Annu. Rev. Plant Physiol. 35, 443–478. doi: 10.1146/annurev.pp.35.060184.002303
Avenhaus, U., Cabeza, R. A., Liese, R., Lingner, A., Dittert, K., Salinas-Riester, G., et al. (2016). Short-term molecular acclimation processes of legume nodules to increased external oxygen concentration. Front. Plant Sci. 6, 1012. doi: 10.3389/fpls.2015.01133
Boisson-Dernier, A., Chabaud, M., Garcia, F., Bécard, G., Rosenberg, C., Barker, D. G. (2001). Agrobacterium rhizogenes-transformed roots of Medicago truncatula for the study of nitrogen-fixing and endomycorrhizal symbiotic associations. Mol. Plant Microbe Interact. 14, 695–700. doi: 10.1094/MPMI.2001.14.6.695
Boyd, E. S., Peters, J. W. (2013). New insights into the evolutionary history of biological nitrogen fixation. Front. Microbiol. 4, 201. doi: 10.3389/fmicb.2013.00201
Brear, E. M., Day, D. A., Smith, P. M. C. (2013). Iron: an essential micronutrient for the legume–rhizobium symbiosis. Front. Plant Sci. 4, 359. doi: 10.3389/fpls.2013.00359
Brewin, N. J. (1991). Development of the legume root nodule. Annu. Rev. Cell Biol. 7, 191–226. doi: 10.1146/annurev.cb.07.110191.001203
Brito, B., Palacios, J. M., Hidalgo, E., Imperial, J., Ruíz-Argüeso, T. (1994). Nickel availability to pea (Pisum sativum L.) plants limits hydrogenase activity of Rhizobium leguminosarum bv. viciae bacteroids by affecting the processing of the hydrogenase structural subunits. J. Bacteriol. 176, 5297–5303. doi: 10.1128/jb.176.17.5297-5303.1994
Burgess, B., Lowe, D. (1996). Mechanism of molybdenum nitrogenase. Chem. Rev. 96, 2983–3011. doi: 10.1021/cr950055x
Burton, J. W., Harlow, C., Theil, E. C. (1998). Evidence for reutilization of nodule iron in soybean seed development. J. Plant Nutr. 5, 913–927. doi: 10.1080/01904169809365453
Catalano, C. M., Czymmek, K. J., Gann, J. G., Sherrier, D. J. (2006). Medicago truncatula syntaxin SYP132 defines the symbiosome membrane and infection droplet membrane in root nodules. Planta 225, 541–550. doi: 10.1007/s00425-006-0369-y
Cheng, H. P., Walker, G. C. (1998). Succinoglycan is required for initiation and elongation of infection threads during nodulation of alfalfa by Rhizobium meliloti. J. Bacteriol. 180, 5183–5191.
Cheng, X., Wang, M., Lee, H. K., Tadege, M., Ratet, P., Udvardi, M., et al. (2014). An efficient reverse genetics platform in the model legume Medicago truncatula. New Phytol. 201, 1065–1076. doi: 10.1111/nph.12575
Cotte, M. P. E., Salomé, M., Rivard, C., Nolf, W. D., Castillo-Michel, H., Fabris, T., et al. (2017). The ID21 X-ray and infrared microscopy beamline at the ESRF: status and recent applications to artistic materials. J. Anal. Atom. Spect. 32, 477–493. doi: 10.1039/C6JA00356G
Curie, C., Mari, S. (2017). New routes for plant iron mining. New Phytol. 214, 521–525. doi: 10.1111/nph.14364
Dalton, D. A., Joyner, S. L., Becana, M., Iturbe-Ormaetxe, I., Chatfield, J. M. (1998). Antioxidant defenses in the peripheral cell layers of legume root nodules. Plant Physiol. 116, 37–43. doi: 10.1104/pp.116.1.37
Demsar, J., Curk, T., Erjavec, A., Gorup, C., Hocevar, T., Milutinovic, M., et al. (2013). Orange: data mining toolbox in Python. J. Mach. Learn. Res. 14, 2349–2353.
Durrett, T. P., Gassmann, W., Rogers, E. E. (2007). The FRD3-mediated efflux of citrate into the root vasculature is necessary for efficient iron translocation. Plant Physiol. 144, 197–205. doi: 10.1104/pp.107.097162
Escudero, V., Abeu, I., del Sastre, E., Tejada-Jiménez, M., Larue, C., Novoa-Aponte, L., et al. (2019a). Nicotianamine synthase2 is required for symbiotic nitrogen fixation in Medicago truncatula nodules. bioRxiv. 717983. doi: 10.1101/717983
Escudero, V., Abreu, I., Tejada-Jiménez, M., Rosa-Núñez, E., Quintana, J., Isabel Prieto, R., et al. (2019b). Medicago truncatula Ferroportin2 mediates iron import into nodule symbiosomes. bioRxiv. 630699. doi: 10.1101/630699
Fahraeus, G. (1957). The infection of clover root hairs by nodule bacteria studied by a simple glass slide technique. J. Gen. Microbiol. 16, 374–381. doi: 10.1099/00221287-16-2-374
Finney, L. A., Halloran, T. V. (2003). Transition metal speciation in the cell: Insights from the chemistry of metal ion receptors. Science 300, 931. doi: 10.1126/science.1085049
Flis, P., Ouerdane, L., Grillet, L., Curie, C., Mari, S., Lobinski, R. (2016). Inventory of metal complexes circulating in plant fluids: a reliable method based on HPLC coupled with dual elemental and high-resolution molecular mass spectrometric detection. New Phytol. 211, 1129–1141. doi: 10.1111/nph.13964
Gage, D. J. (2002). Analysis of infection thread development using Gfp- and DsRed-expressing Sinorhizobium meliloti. J. Bacteriol. 184, 7042–7046. doi: 10.1128/jb.184.24.7042-7046.2002
Gil-Díez, P., Tejada-Jiménez, M., León-Mediavilla, J., Wen, J., Mysore, K. S., Imperial, J., et al. (2019). MtMOT1.2 is responsible for molybdate supply to Medicago truncatula nodules. Plant Cell Environ. 42, 310–320. doi: 10.1111/pce.13388
González-Guerrero, M., Raimunda, D., Cheng, X., Argüello, J. M. (2010). Distinct functional roles of homologous Cu+ efflux ATPases in Pseudomonas aeuginosa. Mol. Microbiol. 78, 1246–1258. doi: 10.1111/j.1365-2958.2010.07402.x
González-Guerrero, M., Matthiadis, A., Sáez, Á., Long, T. A. (2014). Fixating on metals: new insights into the role of metals in nodulation and symbiotic nitrogen fixation. Front. Plant Sci. 5, 45. doi: 10.3389/fpls.2014.00045
González-Guerrero, M., V., E., Sáez, Á., Tejada-Jiménez, M. (2016). Transition metal transport in plants and associated endosymbionts. Arbuscular mycorrhizal fungi and rhizobia. Front. Plant Sci. 7, 1088. doi: 10.3389/fpls.2016.01088
Hakoyama, T., Watanabe, H., Tomita, J., Yamamoto, A., Sato, S., Mori, Y., et al. (2009). Nicotianamine synthase specifically expressed in root nodules of Lotus japonicus. Planta 230, 309–317. doi: 10.1007/s00425-009-0944-0
Hakoyama, T., Niimi, K., Yamamoto, T., Isobe, S., Sato, S., Nakamura, Y., et al. (2012). The integral membrane protein SEN1 is required for symbiotic nitrogen fixation in Lotus japonicus nodules. Plant Cell Physiol. 53, 225–236. doi: 10.1093/pcp/pcr167
Hardy, R. W., Holsten, R. D., Jackson, E. K., Burns, R. C. (1968). The acetylene-ethylene assay for n(2) fixation: laboratory and field evaluation. Plant Physiol. 43, 1185–1207. doi: 10.1104/pp.43.8.1185
Haydon, M. J., Kawachi, M., Wirtz, M., Hillmer, S., Hell, R., Krämer, U. (2012). Vacuolar nicotianamine has critical and distinct roles under iron deficiency and for zinc sequestration in Arabidopsis. Plant Cell 24, 724–737. doi: 10.1105/tpc.111.095042
Higuchi, K., Suzuki, K., Nakanishi, H., Yamaguchi, H., Nishizawa, N. K., Mori, S. (1999). Cloning of nicotianamine synthase genes, novel genes involved in the biosynthesis of phytosiderophores. Plant Physiol. 119, 471–480. doi: 10.1104/pp.119.2.471
Hoffman, B. M., Lukoyanov, D., Yang, Z. Y., Dean, D. R., Seefeldt, L. C. (2014). Mechanism of nitrogen fixation by nitrogenase: The next stage. Chem. Rev. 114, 4041–4062. doi: 10.1021/cr400641x
Inoue, H., Higuchi, K., Takahashi, M., Nakanishi, H., Mori, S., Nishizawa, N. K. (2003). Three rice nicotianamine synthase genes, OsNAS1, OsNAS2, and OsNAS3 are expressed in cells involved in long-distance transport of iron and differentially regulated by iron. Plant J. 36, 366–381. doi: 10.1046/j.1365-313x.2003.01878.x
Inskeep, W. P., Bloom, P. R. (1985). Extinction coefficients of chlorophyll a and b in N,N-dimethylformamide and 80% acetone. Plant Physiol. 77, 483–485. doi: 10.1104/pp.77.2.483
Jain, A., Wilson, G. T., Connolly, E. L. (2014). The diverse roles of FRO family metalloreductases in iron and copper homeostasis. Front. Plant Sci. 5, 100. doi: 10.3389/fpls.2014.00100
Johnson, S. E., Mohler, C. L. (2009). Crop rotation on organic farms: A planning manual (Ithaca, NY: National Resource, Agricultrue and Engineering Services).
Klatte, M., Schuler, M., Wirtz, M., Fink-Straube, C., Hell, R., Bauer, P. (2009). The analysis of Arabidopsis nicotianamine synthase mutants reveals functions for nicotianamine in seed iron loading and iron deficiency responses. Plant Physiol. 150, 257–271. doi: 10.1104/pp.109.136374
Kobayashi, T., Nishizawa, N. K. (2012). Iron uptake, translocation, and regulation in higher plants. Annu. Rev. Plant Biol. 63, 131–152. doi: 10.1146/annurev-arplant-042811-105522
Kondorosi, E., Mergaert, P., Kereszt, A. (2013). A paradigm for endosymbiotic life: cell differentiation of Rhizobium bacteria provoked by host plant factors. Annu. Rev. Microbiol. 67, 611–628. doi: 10.1146/annurev-micro-092412-155630
Kryvoruchko, I. S., Routray, P., Sinharoy, S., Torres-Jerez, I., Tejada-Jiménez, M., Finney, L. A., et al. (2018). An iron-activated citrate transporter, MtMATE67, is required for symbiotic nitrogen fixation. Plant Physiol. 176, 2315–2329. doi: 10.1104/pp.17.01538
Kumar, R. K., Chu, H. H., Abundis, C., Vasques, K., Rodriguez, D. C., Chia, J.-C., et al. (2017). Iron-nicotianamine transporters are required for proper long distance iron signaling. Plant Physiol. 175, 1254. doi: 10.1104/pp.17.00821
Larue, C., Castilo-Michel, H., Sobanska, S., Cécillon, L., Bureau, S., Barthès, V., et al. (2014). Foliar exposure of the crop Lactuca sativa to silver nanoparticles: evidence for internalization and changes in Ag speciation. J. Hazard. Mat. 264, 98–106. doi: 10.1016/j.jhazmat.2013.10.053
León-Mediavilla, J., Senovilla, M., Montiel, J., Gil-Díez, P., Saez, Á., Kryvoruchko, I. S., et al. (2018). MtMTP2-facilitated zinc transport into intracellular compartments is essential for nodule development in Medicago truncatula. Front. Plant Sci. 9, 990. doi: 10.3389/fpls.2018.00990
LeVier, K., Day, D. A., Guerinot, M. L. (1996). Iron uptake by symbisomes from soybean root nodules. Plant Physiol. 111, 893–900. doi: 10.1104/pp.111.3.893
Montiel, J., Arthikala, M. K., Cárdenas, L., Quinto, C. (2016). Legume NADPH oxidases have crucial roles at different stages of nodulation. Int. J. Mol. Sci. 17, 680. doi: 10.3390/ijms17050680
Moreau, S., Meyer, J. M., Puppo, A. (1995). Uptake of iron by symbiosomes and bacteroids from soybean nodules. FEBS Lett. 361, 225–228. doi: 10.1016/0014-5793(95)00155-3
Mus, F., Crook, M. B., Garcia, K., Garcia Costas, A., Geddes, B. A., Kouri, E. D., et al. (2016). Symbiotic nitrogen fixation and the challenges to its extension to non-legumes. Appl. Environ. Microbiol. 82, 3698–3710. doi: 10.1128/AEM.01055-16
Nakagawa, T., Kurose, T., Hino, T., Tanaka, K., Kawamukai, M., Niwa, Y., et al. (2007). Development of series of gateway binary vectors, pGWBs, for realizing efficient construction of fusion genes for plant transformation. J. Biosci. Bioeng. 104, 34–41. doi: 10.1263/jbb.104.34
O'Hara, G. W. (2001). Nutritional constraints on root nodule bacteria affecting symbiotic nitrogen fixation: a review. Austr. J. Exp. Agr. 41, 417–433. doi: 10.1071/EA00087
Oldroyd, G. E. D. (2013). Speak, friend, and enter: signalling systems that promote beneficial symbiotic associations in plants. Nat. Rev. Microbiol. 11, 252–263. doi: 10.1038/nrmicro2990
Preisig, O., Zufferey, R., Thony-Meyer, L., Appleby, C., Hennecke, H. (1996). A high-affinity cbb3-type cytochrome oxidase terminates the symbiosis- specific respiratory chain of Bradyrhizobium japonicum. J. Bacteriol. 178, 1532–1538. doi: 10.1128/jb.178.6.1532-1538.1996
Ravel, B., Newville, M. (2005). Athena, artemis, hephaestus: data analysis for X-ray absorption spectroscopy using IFEFFIT. J. Synchr. Rad. 12, 537–541. doi: 10.1107/S0909049505012719
Rellán-Álvarez, R., Abadía, J., Álvarez-Fernández, A. (2008). Formation of metal-nicotianamine complexes as affected by pH, ligand exchange with citrate and metal exchange. A study by electrospray ionization time-of-flight mass spectrometry. Rapid Commun. Mass Spectrom. 22, 1553–1562. doi: 10.1002/rcm.3523
Rellán-Álvarez, R., Giner-Martínez-Sierra, J., Orduna, J., Orera, I., Rodríguez-Castrillón, J.Á., García-Alonso, J. I., et al. (2010). Identification of a tri-iron(III), tri-citrate complex in the xylem sap of iron-deficient tomato resupplied with iron: new insights into plant iron long-distance transport. Plant Cell Physiol. 51, 91–102. doi: 10.1093/pcp/pcp170
Rodríguez-Haas, B., Finney, L., Vogt, S., González-Melendi, P., Imperial, J., González-Guerrero, M. (2013). Iron distribution through the developmental stages of Medicago truncatula nodules. Metallomics 5, 1247–1253. doi: 10.1039/c3mt00060e
Roschzttardtz, H., Séguéla-Arnaud, M., Briat, J.-F., Vert, G., Curie, C. (2011). The FRD3 citrate effluxer promotes iron nutrition between symplastically disconnected tissues throughout Arabidopsis development. Plant Cell 23, 2725–2737. doi: 10.1105/tpc.111.088088
Roth, L. E., Stacey, G. (1989). Bacterium release into host cells of nitrogen-fixing soybean nodules: the symbiosome membrane comes from three sources. Eur. J. Cell Biol. 49, 13–23.
Roux, B., Rodde, N., Jardinaud, M.-F., Timmers, T., Sauviac, L., Cottret, L., et al. (2014). An integrated analysis of plant and bacterial gene expression in symbiotic root nodules using laser-capture microdissection coupled to RNA sequencing. Plant J. 77, 817–837. doi: 10.1111/tpj.12442
Rubio, L. M., Ludden, P. W. (2005). Maturation of nitrogenase: a biochemical puzzle. J. Bacteriol. 187, 405–414. doi: 10.1128/JB.187.2.405-414.2005
Rubio, M. C., Becana, M., Sato, S., James, E. K., Tabata, S., Spaink, H. P. (2007). Characterization of genomic clones and expression analysis of the three types of superoxide dismutases during nodule development in Lotus japonicus. Mol. Plant Microbe Interact. 20, 262–275. doi: 10.1094/MPMI-20-3-0262
Santos, R., Hérouart, D., Puppo, A., Touati, D. (2000). Critical protective role of bacterial superoxide dismutase in Rhizobium–legume symbiosis. Mol. Microbiol. 38, 750–759. doi: 10.1046/j.1365-2958.2000.02178.x
Schneider, S., Schintlmeister, A., Becana, M., Wagner, M., Woebken, D., Wienkoop, S. (2019). Sulfate is transported at significant rates through the symbiosome membrane and is crucial for nitrogenase biosynthesis. Plant Cell Environ. 42, 1180–1189. doi: 10.1111/pce.13481
Schuler, M., Rellán-Álvarez, R., Fink-Straube, C., Abadía, J., Bauer, P. (2012). Nicotianamine functions in the phloem-based transport of iron to sink organs, in pollen development and pollen tube growth in Arabidopsis. Plant Cell 24, 2380–2400. doi: 10.1105/tpc.112.099077
Senovilla, M., Castro-Rodríguez, R., Abreu, I., Escudero, V., Kryvoruchko, I., Udvardi, M. K., et al. (2018). Medicago truncatula Copper Transporter1 (MtCOPT1) delivers copper for symbiotic nitrogen fixation. New Phytol. 218, 696–709. doi: 10.1111/nph.14992
Singleton, P. W., van Kessel, C. (1987). Effect of localized nitrogen availability to soybean half-root systems on photosynthate partitioning to roots and nodules. Plant Physiol. 83, 552. doi: 10.1104/pp.83.3.552
Smil, V. (1999). Nitrogen in crop production: an account of global flows. Global Biogeochem. Cycles 13, 647–662. doi: 10.1029/1999GB900015
Solé, V. A., Papillon, E., Cotte, M., Walter, P., Susini, J. A. (2007). A multiplatform code for the analysis of energy-dispersive X-ray fluorescence spectra. Spectrochim. Acta B 62, 63–68. doi: 10.1016/j.sab.2006.12.002
Streeter, J. G. (1987). Carbohydrate, organic acid, and amino acid composition of bacteroids and cytosol from soybean nodules. Plant Physiol. 85, 768–773. doi: 10.1104/pp.85.3.768
Sun, L., Gill, U. S., Nandety, R. S., Kwon, S., Mehta, P., Dickstein, R., et al. (2019). Genome-wide analyses of flanking sequences reveals that Tnt1 insertion is positively correlated with gene methylation in Medicago truncatula. Plant J. 98, 1016–1119. doi: 10.1111/tpj.14291
Takanashi, K., Yokosho, K., Saeki, K., Sugiyama, A., Sato, S., Tabata, S., et al. (2013). LjMATE1: a citrate transporter responsible for iron supply to the nodule infection zone of Lotus japonicus. Plant Cell Physiol. 54, 585–594. doi: 10.1093/pcp/pct019
Tang, C., Robson, A. D., Dilworth, M. J. (1991). Which stage of nodule initiation in Lupinus angustifolius L. is sensitive to iron deficiency? New Phytol. 117, 243–250. doi: 10.1111/j.1469-8137.1991.tb04905.x
Tejada-Jiménez, M., Castro-Rodríguez, R., Kryvoruchko, I., Lucas, M. M., Udvardi, M., Imperial, J., et al. (2015). Medicago truncatula natural resistance-associated macrophage protein1 is required for iron uptake by rhizobia-infected nodule cells. Plant Physiol. 168, 258–272. doi: 10.1104/pp.114.254672
Tejada-Jiménez, M., Gil-Diez, P., Leon-Mediavilla, J., Wen, J., Mysore, K. S., Imperial, J., et al. (2017). Medicago truncatula molybdate transporter type 1 (MOT1.3) is a plasma membrane molybdenum transporter required for nitrogenase activity in root nodules under molybdenum deficiency. New Phytol. 216, 1223–1235. doi: 10.1111/nph.14739
Terry, R. E., Soerensen, K. U., Jolley, V. D., Brown, J. C. (1991). The role of active Bradyrhizobium japonicum in iron stress response of soy-beans. Plant Soil 130, 225–230. doi: 10.1007/BF00011877
Udvardi, M., Poole, P. S. (2013). Transport and metabolism in legume-rhizobia symbioses. Annu. Rev. Plant Biol. 64, 781–805. doi: 10.1146/annurev-arplant-050312-120235
Valentine, A. J., Kleinert, A., Benedito, V. A. (2017). Adaptive strategies for nitrogen metabolism in phosphate deficient legume nodules. Plant Sci. 256, 46–52. doi: 10.1016/j.plantsci.2016.12.010
Vasse, J., de Billy, F., Camut, S., Truchet, G. (1990). Correlation between ultrastructural differentiation of bacteroids and nitrogen fixation in alfalfa nodules. J. Bacteriol. 172, 4295–4306. doi: 10.1128/jb.172.8.4295-4306.1990
Vernoud, V., Journet, E. P., Barker, D. G. (1999). MtENOD20, a Nod factor-inducible molecular marker for root cortical cell activation. Mol. Plant Microbe Interact. 12, 604–614. doi: 10.1094/MPMI.1999.12.7.604
von Wiren, N., Klair, S., Bansal, S., Briat, J.-F., Khodr, H., Shioiri, T., et al. (1999). Nicotianamine chelates both FeIII and FeII. Implications for metal transport in plants. Plant Physiol. 119, 1107–1114. doi: 10.1104/pp.119.3.1107
Waters, B. M., Chu, H.-H., DiDonato, R. J., Roberts, L. A., Eisley, R. B., Lahner, B., et al. (2006). Mutations in Arabidopsis Yellow Stripe-Like1 and Yellow Stripe-Like3 reveal their roles in metal ion homeostasis and loading of metal ions in seeds. Plant Physiol. 141, 1446–1458. doi: 10.1104/pp.106.082586
Keywords: iron, metal homeostasis, nicotianamine synthases, nodulation, metal nutrition
Citation: Escudero V, Abreu I, del Sastre E, Tejada-Jiménez M, Larue C, Novoa-Aponte L, Castillo-González J, Wen J, Mysore KS, Abadía J, Argüello JM, Castillo-Michel H, Álvarez-Fernández A, Imperial J and González-Guerrero M (2020) Nicotianamine Synthase 2 Is Required for Symbiotic Nitrogen Fixation in Medicago truncatula Nodules. Front. Plant Sci. 10:1780. doi: 10.3389/fpls.2019.01780
Received: 08 August 2019; Accepted: 20 December 2019;
Published: 30 January 2020.
Edited by:
Seçkin Eroğlu, Middle East Technical University, TurkeyReviewed by:
Pierre Frendo, University of Nice Sophia Antipolis, FranceEmre Aksoy, Niğde Ömer Halisdemir University, Turkey
Alexandra Lešková, Slovak Academy of Sciences (SAS), Slovakia
Copyright © 2020 Escudero, Abreu, del Sastre, Tejada-Jiménez, Larue, Novoa-Aponte, Castillo-González, Wen, Mysore, Abadía, Argüello, Castillo-Michel, Álvarez-Fernández, Imperial and González-Guerrero. This is an open-access article distributed under the terms of the Creative Commons Attribution License (CC BY). The use, distribution or reproduction in other forums is permitted, provided the original author(s) and the copyright owner(s) are credited and that the original publication in this journal is cited, in accordance with accepted academic practice. No use, distribution or reproduction is permitted which does not comply with these terms.
*Correspondence: Manuel González-Guerrero, bWFudWVsLmdvbnphbGV6QHVwbS5lcw==