- 1MOA Key Laboratory of Crop Ecophysiology and Farming System in the Middle Reaches of the Yangtze River, College of Plant Science and Technology, Huazhong Agricultural University, Wuhan, China
- 2Hubei Collaborative Innovation Center for Grain Industry, Yangtze University, Jingzhou, China
Field and pot experiments were conducted to investigate the control effects of parasitoid wasps (Chelonus munakatae Munakata) on striped rice stem borers and their impacts on N2O and CH4 emissions from paddy fields. Three treatments including no insect (NI), striped stem borer (CS) and parasitoid wasp + striped stem borer (CS+CM) were implemented. The abundance of GHG-related microorganisms in soils was determined by absolute real-time qPCR. Compared with NI, CS and CS+CM significantly increased the ratio of dead tillers, inhibited the growth and vitality of rice roots, and decreased the rice grain yield, while they significantly reduced the seasonal cumulative emissions of N2O and CH4 by 17.7–24.6 and 13.6–35.1%, and decreased the total seasonal global warming potential (GWP) by 13.6–34.7%, respectively. Moreover, compared with CS, CS+CM significantly enhanced the growth and vitality of rice roots, decreased the ratio of dead tillers, improved the rice grain yield, as well as increased the seasonal cumulative CH4 emissions and the total seasonal GWP. Principal component analysis indicated that the morphological features of rice roots play a more important role in regulating GHG emissions than GHG-related microorganisms. The results suggested that C. munakatae can effectively control the outbreak of C. suppressalis and alleviate crop damage with acceptably higher GHG emissions. It is concluded that it can be recommended as an effective, environment-friendly and sustainable approach to prevent and control C. suppressalis.
Introduction
Striped rice stem borer (Chilo suppressalis Walker) is one of the major pests that severely affect rice production (Herdt, 1991). Currently, the control and prevention of stem borers mainly depend on chemical and biological approaches (Duan et al., 2012). Although chemical pesticides are highly effective, their long-term and extensive application involve a series of drawbacks such as high costs, pesticide resistance and environmental pollution (Duan et al., 2012). Moreover, the population of natural enemies of stem borers has drastically decreased due to environmental deterioration and cannot be restored naturally, which further aggravates the outbreak of stem borers (Duan et al., 2012). It has been generally recognized that biological diversity plays an important role in the sustainable development of agriculture (Kennedy and Smith, 1995; Brussaard et al., 2007). Among the various natural enemies of striped rice stem borers in China, parasitoid wasp (Trioxys complanatus) is the dominant species, which has been thoroughly studied and widely used as a secure and reliable method to prevent and control stem borers (Chen et al., 2010; Wang and Ma, 2015). In the 1970s, two species of Trichogramma wasps, Trichogramma japonicum (Ashmead) and Trichogramma chilonis (Ishii), were utilized for the control of striped rice stem borers in China, which had brought fairly satisfactory results in some areas (Huang et al., 2018). Considering that ecological restoration and reconstruction are currently advocated worldwide, it is critical to study the control effects of parasitoid wasps on striped rice stem borers to reduce the application of pesticides for sustainable agriculture.
Nitrous oxide (N2O) and methane (CH4) are two major greenhouse gases (GHGs), and paddy fields are one of the major sources. Methane emissions from paddy fields account for approximately 12% of the global anthropogenic CH4 emissions (Intergovernmental Panel on Climate Change [IPCC], 2013). Rice plants are a vital factor affecting the production and emissions of N2O and CH4 (Yu et al., 1997). Nitrous oxide emissions from soils are regulated by rice plants mainly through changing rhizosphere pH, redox potential, and soil structure (Xu et al., 2000; Yan et al., 2000). Over 80% of the total N2O emissions are transported via rice plants in submerged paddy fields, while less than 20% are transported via aerial plant organs (Seiler et al., 1984; Conrad, 1996; Yan et al., 2000). Moreover, the CH4 transported through the plants during the rice growing season accounts for nearly 90% of the total CH4 emissions from paddy fields (Cicerone et al., 1983; Banker et al., 1995).
Given the critical role that the root aerenchyma tissues play in the GHG transportation from rhizosphere to atmosphere (Lu et al., 1999), and the major impact of root exudation on microbial processes such as nitrification, denitrification, methanogenesis and methanotrophy (Jia and Cai, 2003), the damage inflicted upon rice plants by striped rice stem borers will undoubtedly affect the N2O and CH4 emissions from paddy fields. Although the effects of striped rice stem borers and parasitoid wasps on the growth of rice plants have been well documented, and parasitoid wasps have been proved to be highly effective in controlling the outbreak of stem borers while reducing the environmental pollution caused by chemical pesticides, their impacts on GHG emissions have been rarely studied. Therefore, this study evaluated the control effects of Chelonus munakatae (Munakata) against C. suppressalis, as well as their impacts on N2O and CH4 emissions from a double-rice cropping system, aiming to provide a theoretical basis for promoting this biological pest control method in the future. The hypothesis was that C. munakatae could effectively control C. suppressalis, and their interaction may have significant effects on N2O and CH4 emissions from paddy soils.
Materials and Methods
Experimental Site
The experimental site is located in Huazhong Agricultural University, Wuhan City, Hubei Province, China (30°28′21″N latitude, 114°20′48″E longitude; 28 m above sea level). It has a subtropical monsoon climate with an average annual temperature of 23.6°C and an average annual precipitation of 906.8 mm. Field and pot experiments were simultaneously carried out with a double-rice cropping system (Oryza sativa L.; early rice LY287 and late rice J036) in 2018. The main soil properties before the experiments were examined by measuring a composite sample derived from mixing and homogenizing equal amounts of soil samples collected from each plot or each pot in May 2018 (Supplementary Table S1).
Experimental Design and Field Management
Three treatments including no insect (NI), striped rice stem borers (C. suppressalis) (CS), and striped rice stem borers (C. suppressalis) + parasitoid wasps (C. munakatae) (CS+CM) were applied following a random complete block design with three replications for both field and pot experiments. The eggs of C. suppressalis were reared in advance in the lab until they developed into second-instar larvae, and then placed evenly on the rice plants under CS and CS+CM treatments (80 larvae per plot in field experiment and 12 larvae per pot in pot experiment) successively on May 16th 2018 for early rice and August 20th in 2018 for late rice. For CS+CM treatment, the eggs of C. suppressalis were exposed to the mature wasps of C. munakatae at the beginning and only the ones with wasp eggs laid inside were kept for later rearing.
The experimental field was 216.09 m2 (14.7 × 14.7 m) in area, and the area of each plot was 8.41 m2 (2.9 × 2.9 m). The plots were separated from each other by 50 cm high and 50 cm wide ridges. Water leakage and fertilizer transference were prevented by covering the ridges with plastic films and digging 1 m wide ditches around each plot. Besides, supporting frames (3 × 3 × 3 m) over each plot were covered by 100 mesh nets to prevent the transference of insects across different plots. As for pot experiment, the soil was obtained by mixing and homogenizing equal amounts of silty and sandy soils followed by sieving (2 mm). Each pot (0.8 × 0.6 × 0.5 m) contained 80 kg soil, which was then watered and compacted. Similar to the field experiment, each pot was covered by a 100 mesh net to prevent insect spreading.
Rice seedlings were manually transplanted on April 23rd (early rice) and July 25th (late rice). For field experiment, the field was plowed by a rotary tiller and submerged for a week to exterminate the native pests, followed by seedling transplanting at the rate of 7.0 × 105 seedlings ha–1 (144 hills per plot with 3 plants hill–1). For pot experiment, rice seedlings were transplanted at the rate of 18 seedlings per pot (6 hills per pot with 3 plants hill–1). The weeding, irrigation and fertilization regimes were the same for both field and pot experiments. No chemical pesticides or herbicides were applied during the rice growing seasons, and weeds were removed manually. Except for being drained for 2 weeks, respectively, before tillering and harvest stages, the paddy field was irrigated regularly to keep the soil surface wet. As for fertilization, compound fertilizer (N:P2O5:K2O = 15%:15%:15%), urea (N of 46%) and potassium chloride (K2O of 60%) were applied to provide 195 kg N ha–1, 97.5 kg P2O5 ha–1 and 195 kg K2O ha–1 for early rice and 240 kg N ha–1, 120 kg P2O5 ha–1 and 240 kg K2O ha–1 for late rice throughout the rice growing season. During the process, P fertilizer was only applied as basal treatment. Half of the K fertilizer was applied as the basal fertilizer, while the other half was applied as a top dressing at the jointing stage. As for N fertilizer, 50, 30, and 20% were applied 2 days before the seedling transplanting, at the tillering stage and at the jointing stage, respectively.
Analysis of Plant Growth and Rice Yield
After the insect placement, the ratios of dead tillers of rice plants under CS and CS+CM treatments were measured by calculating the average ratio of dead tillers to total tillers of 36 hills of rice plants around the insect placement sites in each plot. The measurements were carried out every 2 days until they reached stable values. The calculating equation was as below,
where DR is the ratio of dead tillers, DT is the number of dead tillers, and TT is the total number of tillers per plant.
The vitality of rice roots at the harvest stage (July 8th for early rice and October 28th for late rice) was determined by bleeding sap flow method (Carvajal et al., 1996). One hill of rice plants was selected in each plot, and it was made sure that the growth status of the selected plants under the same treatment was similar to each other. With the tip cut off, the rest part of the roots was wrapped in water-proof sealing bags and the bleeding sap flow from the injured roots was then collected and weighed. In addition, another rice plant was selected in each plot at the harvest stage, and it was made sure that the growth status of the selected plants under the same treatment was similar to each other. This whole plant was dug out and washed clean, and then scanned by a root scanner (Fansheng, WinRHIZO, China) to analyze the root morphology. Moreover, the rice grain yield was evaluated at the harvest stage for both early and late rice in field experiment.
Gas Sampling and Analysis
The N2O and CH4 fluxes from paddy soil were determined by static chamber-gas chromatography method (Li et al., 2013). In pot experiment, box chambers (0.8 × 0.6 × 0.5 m or 0.8 × 0.6 × 1 m depending on plant height) made of stainless steel and covered with heat-insulation layers were used. A thermometer for temperature monitoring, four fans for air mixing and a vent tube for pressure equilibration were installed inside the chamber. Sampling was conducted every 7 days and every time after N fertilization throughout the rice growing seasons, with four samples successively collected per pot at the interval of 10 min (0, 10, 20, and 30 min) from the chamber headspace using a 30-mL gas-tight syringe. Gas samples were immediately transferred to 30-mL air-evacuated gas-tight glass vials for further analysis.
Nitrous oxide and CH4 concentrations were measured by a gas chromatography (Shimadzu, GC-14B, Japan), which was equipped with a stainless-steel column (Porapack N, length × inner diameter of 3 m × 2 mm), an electron capture detector (ECD) and a flame ionization detector (FID). The flow rates of the carrier (N2), fuel (H2) and supporting gas (zero air) were 30 mL min–1, 25 mL min–1 and 300 mL min–1, respectively, and the column and injector temperatures were adjusted to 50 and 100°C, respectively. The temperature of ECD for N2O analysis was adjusted to 330°C and that of FID for CH4 analysis was set to 200°C. The N2O and CH4 fluxes were calculated following the equation below (Zheng et al., 2006),
where F is the flux of N2O or CH4 (mg m–2 h–1), ρ is the density of N2O or CH4 standard gas (mg m–3), h is the height of the chamber headspace (m), dc/dt is the increasing rate of gas concentration in the chamber (mg m–3 h–1), and T is the mean temperature of the chamber in the unit of°C. The seasonal cumulative N2O and CH4 emissions per pot were computed according to Li et al. (2013) as shown below,
where CEPN2O is the seasonal cumulative N2O emissions per pot (mg pot–1 season–1), CEPCH4 is the seasonal cumulative CH4 emissions per pot (g pot–1 season–1), Fi and Fi+1 are the gas fluxes measured on two adjacent sampling dates (mg m–2 h–1), Di is the length of the ith sampling interval (d), n is the total number of sampling intervals and 0.48 is the surface area of the pot (m2).
The global warming potential (GWP) of N2O and CH4 is 298 and 34 times higher than that of carbon dioxide (CO2) over a 100-year period (Intergovernmental Panel on Climate Change [IPCC], 2013). Hence, the total seasonal GWP of N2O and CH4 was calculated as below,
where GWP is the total seasonal GWP of N2O and CH4 (t CO2-eq hm–2 season–1), CEN2O is the seasonal cumulative N2O emissions (kg hm–2 season–1), CECH4 is the seasonal cumulative CH4 emissions (kg hm–2 season–1), and CEPN2O and CEPCH4 are the same as mentioned above.
Soil Sampling and Chemical Analysis
Two soil samples were randomly taken from each pot during the five rice growing stages of seedling, tillering, booting, full heading and harvest (April 24th, May 12th, May 26th, June 3rd and July 8th for early rice, respectively; July 26th, August 19th, September 8th, September 25th and October 28th for late rice, respectively) from the depth of 0–5 and 0–20 cm, respectively. The two samples from the same pot and of the same depth were then immediately mixed and homogenized after the removal of stones and roots. The samples of 0–5 cm deep soil were stored at –20°C for chemical analysis, while the samples of 0–20 cm deep soil were stored at –80°C for further biological analysis.
The concentrations of soil ammonium-nitrogen (NH4+-N) and nitrate-nitrogen (NO3–-N) were measured by ultraviolet spectrophotometric method and colorimetric method with alpha-Naphthol Blue, respectively (Keeney and Nelson, 1982; Baethgen and Alley, 1989). Soil dissolved organic carbon (DOC) was extracted from soil-water solution using suction filtration, and then measured according to the Walkley–Black method (Allison, 1965).
Absolute Real-Time Quantitative PCR Analysis
Total genomic DNA was extracted from 0–20 cm deep soil samples collected at the tillering, booting, full heading and harvest stages (as mentioned above) using the Fast DNA SPIN Kit For Soil (MP Biomedicals LLC, United States) and purified by the QIAquick Gel Extraction Kit (QIAGEN Ltd., Shanghai, China). The genomic DNA was then analyzed using a NanoDrop spectrophotometer (NanoDrop Technologies Inc., Wilmington, DE, United States), and the A260/A280 ratios were determined to be above 1.8 and the A260/A230 ratios above 2.0.
Absolute real-time quantitative PCR (RT-qPCR) was performed using IQ5 Real-Time PCR detection system (Bio-Rad Laboratories Inc., United States) in 96-well PCR optical plates in triplicate per sample with AOA-amoA, AOB-amoA, nirS, nirK, pmoA, and mcrA as the target genes, following the PCR protocols shown in Supplementary Table S2. Standards for all assays were prepared from clones containing known numbers of DNA copies of the target genes as a plasmid insert (Angel et al., 2011). Standards were then diluted and used for the construction of standard curves in each reaction. Each reaction was composed of 12.5 μL of 2 × SYBR Premix Ex Taq TM (TaKaRa Inc., China), 0.5 μL of 10 pmol μL–1 appropriate forward and reverse primers, respectively, and 2 μL of 10-fold diluted template DNA in a final volume of 25 μL. Melting curve analysis was performed to examine the specificity of the amplified products. To avoid PCR inhibition, proper dilution factor of the template DNA was determined in advance by running qPCR with different dilutions of template DNA.
Statistical Analysis
Statistical analyses were carried out using SPSS (SPSS Inc., Chicago, IL, United States; Version 19.0) and Statistix (Analytical Software Inc., Tallahassee, FL, United States; Version 9.0). Normality and variance homogeneity of data were tested beforehand, and the qPCR datasets were log transformed to meet the requirements for analysis of variance (ANOVA). One-way ANOVA and two-way repeated measures ANOVA were conducted to analyze the data obtained only at harvest stages and at different growing stages, respectively, followed by least significant difference (LSD) test when an ANOVA result was significant. All levels of significance were defined at P ≤ 0.05. All plots were drawn with Origin (Origin Lab Corporation, United States; Version 2016).
To elucidate the dynamic mechanisms of GHGs and their relationships with rice plants, soil chemical properties and GHG-related microorganisms, principal component analysis (PCA) was carried out using Canoco (Biometris-Plant Research International, the Netherlands; Version 5.0). GHGs (denoted by red hollow arrow) were represented by the total seasonal GWP of N2O and CH4. The growth of rice plants (denoted by green arrows) was represented by the attributes of root morphology and vitality as well as the ratio of dead tillers. The soil chemical properties (denoted by red solid arrows) were represented by the NH4+-N and NO3–-N concentrations. The GHG-related soil microorganisms (denoted by black arrows) were represented by the abundance of functional genes including AOA-amoA, AOB-amoA, nirS, nirK, pmoA, and mcrA.
Results
Plant Growth and Rice Grain Yield
Striped stem borers significantly inhibited the growth and vigor of rice plants. Compared with CS, CS+CM significantly decreased the ratio of dead tillers by 32.5–33.6% (Supplementary Table S3). Moreover, compared with NI, CS significantly reduced the root length, surface area, volume and bleeding sap flow of both early and late rice by 38.0–46.1, 37.4–48.8, 31.9–53.4, and 32.8–47.5%, respectively, while CS+CM only significantly decreased the root length, surface area and volume of late rice by 18.1, 43.2, and 18.4%, respectively (Table 1). Compared with CS, CS+CM enhanced the root length, surface area and volume of late rice by 52.1, 10.8, and 74.9%, respectively (Table 1).
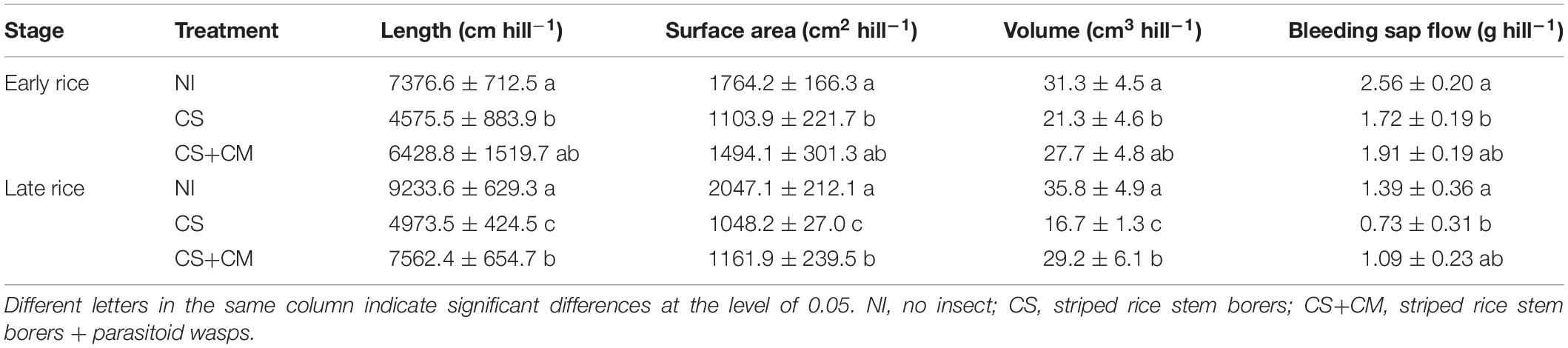
Table 1. Morphology and vitality of rice roots at harvest stage under different treatments in field experiment.
Compared with NI, the insect treatments significantly decreased the grain yield of both early and late rice by 10.3–22.1% (Supplementary Table S4). Particularly, the grain yield of late rice under CS+CM was significantly higher than that under CS by 15.1%, while no significant difference was observed in the grain yield of early rice between CS and CS+CM (Supplementary Table S4).
N2O and CH4 Emissions
The variations of N2O fluxes throughout the growing seasons were similar between early and late rice (Figure 1A). Three peaks of N2O fluxes were observed after the application of N fertilizers and field drying, respectively, at the seedling (April 24th for early rice and July 26th for late rice), tillering (May 4th for early rice and August 12th for late rice) and jointing (May 26th for early rice and September 8th for late rice) stages. The N2O fluxes ranged from –0.02 to 0.19 mg m–2 h–1 under NI, from –0.01 to 0.16 mg m–2 h–1 under CS, and from –0.01 to 0.16 mg m–2 h–1 under CS+CM, with the average values of 0.05, 0.04 and 0.05 mg m–2 h–1, respectively. Furthermore, the seasonal cumulative N2O emissions under CS of both early and late rice were significantly lower than those under NI by 17.7–24.6%, while no significant differences were found between NI and CS+CM or between CS and CS+CM (Table 2).
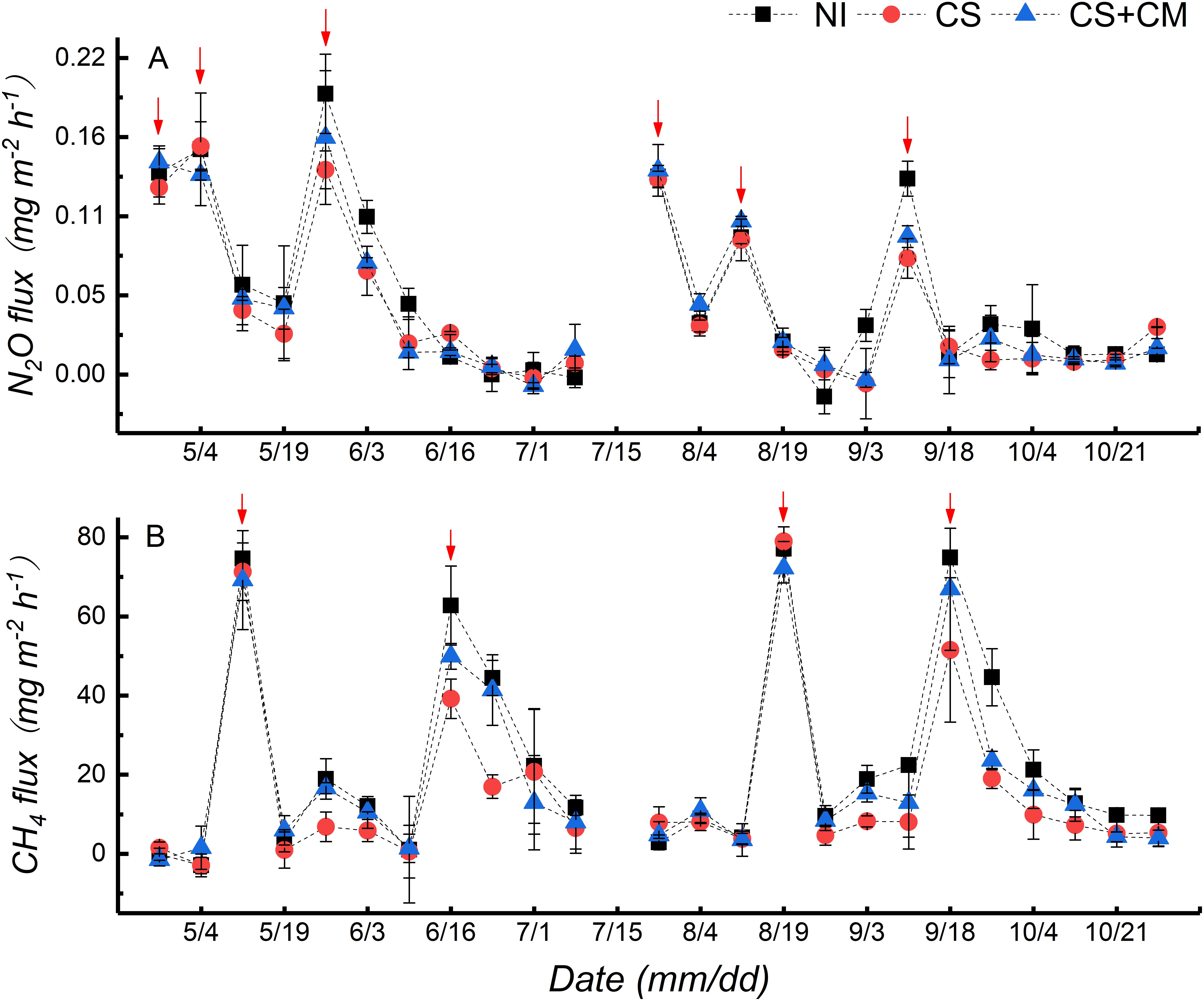
Figure 1. N2O (A) and CH4 (B) fluxes from paddy soil under different treatments throughout rice growing season in pot experiment. NI, no insect; CS, striped rice stem borers; CS+CM, striped rice stem borers + parasitoid wasps. Red arrows denote the peaks of N2O and CH4 fluxes.
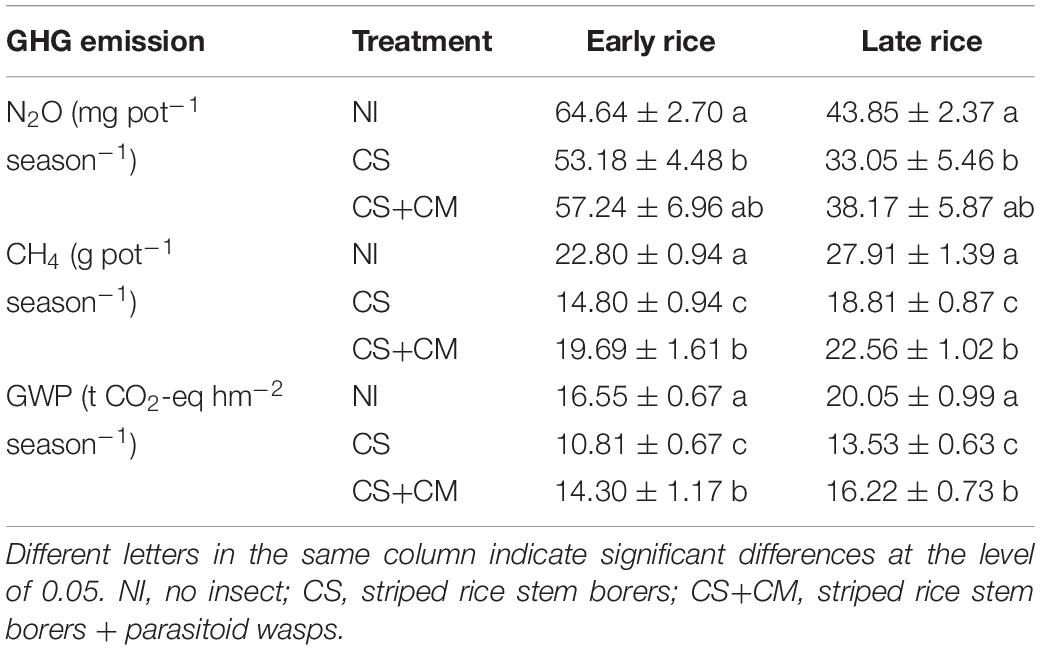
Table 2. Seasonal cumulative emissions of N2O and CH4 per pot and total seasonal GWP under different treatments in pot experiment.
The variations of CH4 fluxes throughout the growing seasons were similar between early and late rice (Figure 1B). Two peaks were observed at the tillering (May 12th for early and August 19th for late rice) and full heading (June 16th for early rice and September 18th for late rice) stages. The CH4 fluxes ranged from –2.92 to 77.14 mg m–2 h–1 under NI, from –2.95 to 78.98 mg m–2 h–1 under CS, and from –1.50 to 72.19 mg m–2 h–1 under CS+CM, with the average values of 23.55, 16.06, and 19.63 mg m–2 h–1, respectively. Moreover, the seasonal cumulative CH4 emissions under insect treatments of both early and late rice were significantly lower than those under NI by 13.6–35.1% (Table 2). However, CS+CM significantly increased the seasonal cumulative CH4 emissions by 19.9–33.0% compared with CS (Table 2).
Compared with NI, the insect treatments significantly reduced the total seasonal GWP of N2O and CH4 from paddy soil by 13.6–34.7% (Table 2). However, the total seasonal GWP of N2O and CH4 under CS+CM was significantly higher than that under CS by 19.9–32.3% (Table 2).
Ammonium-N, NO3–-N and DOC Concentrations
For early rice, both soil NH4+-N and NO3–-N concentrations increased and peaked at the booting stage, followed by a dramatic decrease thereafter, while they continuously declined throughout the growing season of late rice (Supplementary Figure S1). Compared with NI, CS significantly increased the soil NH4+-N concentrations at the later stages of rice growing season by 38.4–88.3%, while nearly no significant difference was observed between NI and CS+CM (Supplementary Figure S1A). Moreover, the soil NH4+-N concentrations under CS+CM were significantly lower than those under CS by 34.0–36.2% at the later stages of the rice growing season (Supplementary Figure S1A). In contrast, CS significantly decreased the soil NO3–-N concentrations at the later growing stages of the early rice and at the booting stage of the late rice by 23.7–32.6% relative to NI, and there were no significant differences between NI and CS+CM or between CS and CS+CM (Supplementary Figure S1B).
The DOC concentrations in paddy soil of early and late rice both peaked at the booting stage (Supplementary Figure S2). Furthermore, under CS, the soil DOC concentrations at the later stages of the rice growing season were significantly lower than those under NI by 13.9–39.5%; while under CS+CM, only the DOC concentrations at the booting stage of early rice and full heading stage of late rice were significantly lower than those under NI by 11.0–19.7% (Supplementary Figure S2). No significant difference in DOC concentrations was found between CS and CS+CM (Supplementary Figure S2).
Abundance of Microbial Functional Genes
The abundance of AOA-amoA and AOB-amoA genes that regulate the nitrification in paddy soil both peaked at the booting and full heading stages, ranging from 1.92 × 108 to 3.53 × 108 copies (g soil) –1 and 2.00 × 107 to 3.92 × 107 copies (g soil)–1, respectively (Figures 2A,B). Moreover, their abundance was significantly affected by the insect treatments (Figures 2A,B). Compared with that under NI, the abundance of AOA-amoA at the booting, full heading and harvest stages under CS was 21.0–37.5% lower, while that under CS+CM was reduced by 9.9–25.4%. Besides, CS+CM significantly enhanced the abundance of AOA-amoA at the booting, full heading and harvest stages by 13.8–19.4% compared with CS. On the other hand, the abundance of AOB-amoA under CS at the booting and full heading stages was significantly lower than that under NI by 24.5–37.1%, whereas nearly no significant difference in the abundance of AOB-amoA was observed between NI and CS+CM. Furthermore, compared with CS, CS+CM significantly enhanced the abundance of AOB-amoA at the booting and full heading stages by 20.8–45.1%.
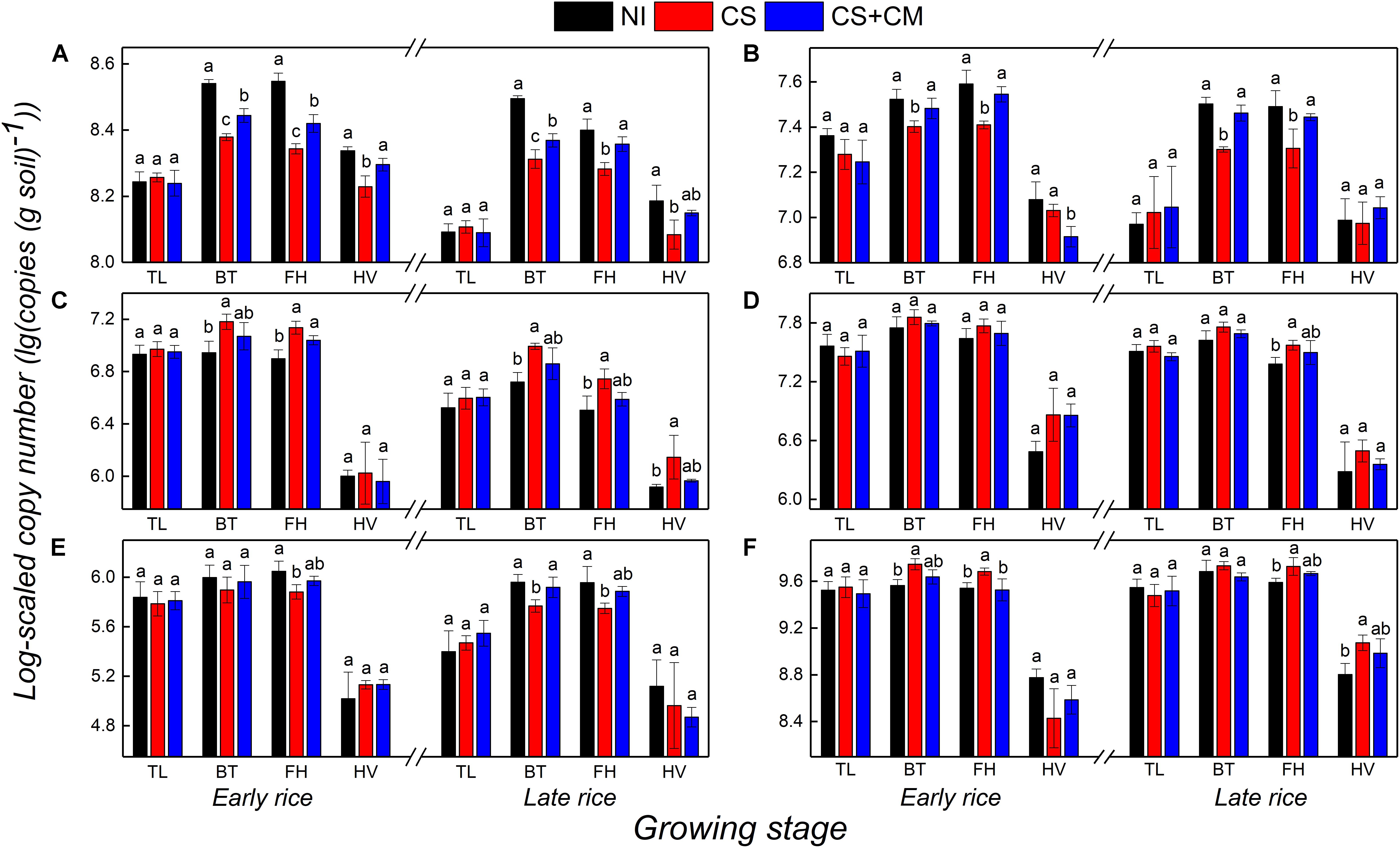
Figure 2. Abundance of AOA-amoA (A), AOB-amoA (B), nirS (C), nirK (D), pmoA (E) and mcrA (F) genes at log-scale in paddy soil under different treatments at tillering, booting, full heading and harvest stages in pot experiment. NI, no insect; CS, striped rice stem borers; CS+CM, striped rice stem borers + parasitoid wasps. TL, tillering stage; BT, booting stage; FH, full heading stage; HV, harvest stage. The abundance of genes was measured in triplicate per sample. Different letters indicate significant differences at the level of 0.05.
The abundance of both nirS and nirK genes that regulate the denitrification in paddy soil peaked at the booting stages, and ranged from 5.31 × 106 to 1.53 × 107 copies (g soil) –1 and from 4.27 × 107 to 7.31 × 107 copies (g soil) –1, respectively (Figures 2C,D). In addition, the insect treatments only imposed significant impacts on the abundance of nirS (Figures 2C,D). The abundance of nirS under CS at the booting, full heading and harvest stages was significantly higher than that under NI by 71.3–85.8%, while there were no significant differences between NI and CS+CM or between CS and CS+CM.
The abundance of both pmoA and mcrA genes, which, respectively, regulate the oxidization and production of CH4 in paddy soils, peaked at the booting and full heading stages, ranging from 5.63 × 105 to 1.13 × 106 copies (g soil) –1 and 3.41 × 109 to 5.59 × 109 copies (g soil) –1, respectively (Figures 2E,F). Compared with NI, CS significantly lowered the abundance of pmoA at the booting and full heading stages by 32.1–39.7%, while nearly no significant differences were observed between NI and CS+CM or CS and CS+CM (Figure 2E). On the contrary, the abundance of mcrA under CS at the booting, full heading and harvest stages was significantly higher than that under NI by 38.3–85.0%, whereas there was nearly no significant difference between NI and CS+CM or CS and CS+CM (Figure 2F).
As shown in the PCA plot, two axes accounted for 70.1 and 22.1% of the variance (Figure 3). GHGs were positively correlated with the morphological indexes of rice roots, soil DOC and NO3–-N concentrations, and the abundance of nitrifiers harboring AOB-amoA and methanogens harboring mcrA, while were negatively correlated with the ratio of dead tillers, soil NH4+-N concentrations, and the abundance of denitrifiers harboring nirS and nirK and methanotrophs harboring pmoA (Figure 3).
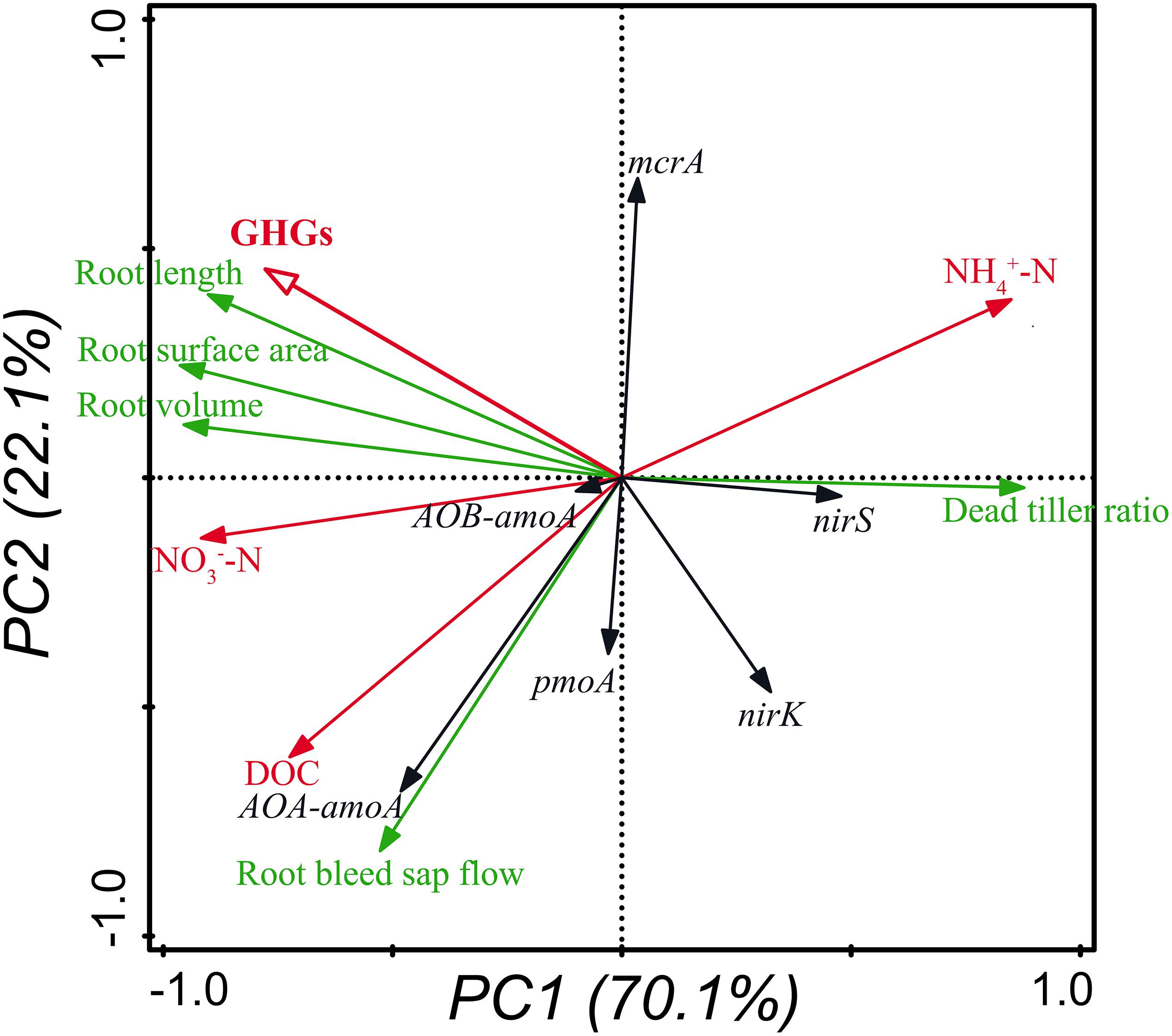
Figure 3. Relationships of GHGs with the growth of rice plants, soil chemical properties and GHG-related microorganisms shown by PCA. GHGs and soil chemical properties are denoted by hollow and solid red arrows, respectively. Attributes of rice plants are denoted by green arrows, and soil microorganisms are denoted by black arrows. The abundance of genes was measured in triplicate per sample.
Discussion
Impacts of C. suppressalis and C. munakatae on Rice Growth and Grain Yield
Khush and Toenniessen (1991) claimed that stem borer damage could result in a 10–30% loss of total rice grain yield. Our results also showed that C. suppressalis significantly decreased rice grain yield (Supplementary Table S4) compared with no insect treatment, possibly owing to the increased ratio of dead tillers (Supplementary Table S3), dead shoots and the resultant formation of dead hearts and white heads caused by C. suppressalis. This explanation is supported by previous studies (Pathak, 1968; Dale, 1994), which reported that larval feeding of C. suppressalis on rice plants could lead to the yellowing and dying of growing shoots, fewer tillers and more panicles with unfilled grains. In addition, the inhibited growth and vitality of rice roots (Table 1) induced by nutrient consumption and tissue damage in rice plants under the C. suppressalis treatment, may limit the uptake and transport of nutrients through the roots and negatively affect the grain filling process, thus reducing grain yield (Pathak et al., 1971).
Moreover, numerous studies have concluded that natural enemies could effectively control the outbreak of pests (Beddington et al., 1978; Settle et al., 1996; Losey and Vaughan, 2006). Our results also indicated that C. munakatae is highly effective in controlling C. suppressalis to significantly decrease the ratio of dead tillers (Supplementary Table S3), improve the growth of roots (Table 1), and increase the grain yield (Supplementary Table S4). It has been reported that C. munakatae can impair the activity of C. suppressalis through parasitism, thereby alleviating their damage on rice plants and ultimately enhancing the rice yield (Maki, 1930).
Impacts of C. suppressalis and C. munakatae on GHG Emissions and Related Microorganisms
In this study, N2O fluxes from paddy soils peaked three times just after the application of N fertilizers and field drying throughout the rice growing season (Figure 1A), suggesting that N2O emissions are highly correlated with N fertilization and field drying. Similarly, Yao et al. (2000) and Wang et al. (2011) revealed that N fertilization could stimulate N2O emissions by providing more N substrates for the microbial nitrification and denitrification in soils. Moreover, Zhang et al. (2015) concluded that field drying increases N2O emissions from paddy fields by facilitating microbial nitrification due to the improvement of soil aeration. As for CH4 fluxes from paddy soil in this study, two peaks were observed at the tillering and full heading stages, respectively (Figure 1B). This may be attributed to the increased aerenchyma cells in rice plants at the tillering stage, which could facilitate CH4 transport (Bhattacharya et al., 2013) and the production of more root exudates at the full heading stage, and higher CH4 production by supplying more nutrients for methanogens (Lu et al., 2000).
This study shows that insect treatments could significantly decrease the fluxes (Figure 1) and seasonal accumulative emissions (Table 2) of N2O and CH4, as well as the total seasonal GWP (Table 2) compared with no insect treatment, probably because the growth of rice roots and shoots was inhibited by C. suppressalis parasitism (Supplementary Table S3 and Table 1), which limited the gas transport through root and shoot aerenchyma. The PCA results also imply that GHGs were highly positively correlated with the growth of rice roots (Figure 3). This conclusion is supported by numerous studies, which suggests that plant-mediated transport is a major pathway for gas efflux in submerged soil (Hosono and Nouchi, 1997; Groot et al., 2005; Reid et al., 2015) and more than 80% of both N2O and CH4 is emitted through rice plants (Yu et al., 1997; Watanabe et al., 1999; Yan et al., 2000). Similarly, the relatively higher fluxes (Figure 1) and seasonal accumulative emissions (Table 2) of N2O and CH4 as well as the significantly higher total seasonal GWP (Table 2) under CS+CM than under CS may also be owing to the lower damage on rice plants under CS+CM (Supplementary Table S3 and Table 1) as mentioned above.
As shown by the results of this study, the abundance of both nitrifiers and methanotrophs was significantly lower under the C. suppressalis treatment than under no insect treatment (Figures 2A,B,E), whereas that of both denitrifiers and methanogens was significantly higher (Figures 2C,F), which could be explained by the reduction of oxygen released from rice roots due to the root damage under the C. suppressalis treatment (Table 1), and the subsequent activation of anaerobic microorganisms including denitrifiers and methanogens. The PCA results also support this speculation, illustrating that the abundance of both nitrifiers harboring AOA-amoA and AOB-amoA and methanotrophs harboring pmoA is positively correlated with the growth and vitality of rice roots (Figure 3).
Dynamic Mechanisms of GHGs Under Insect Treatment
As shown by the PCA results, GHGs had much higher correlations with the morphological indexes of rice roots than with the GHG-related soil microorganisms (Figure 3), implying that rice roots play a more important role than GHG-related microorganisms in regulating the GHG emissions under insect treatment from paddy soil. This conclusion is also supported by another result: when rice roots were damaged by C. suppressalis parasitism (Table 1), the GHG emissions and total seasonal GWP were both significantly reduced (Figure 1 and Table 2), even though the abundance of denitrifiers and methanogens was significantly higher than that under no insect treatment (Figures 2C,F). Similarly, Zhang et al. (2016) observed that the underground part of rice plants is a major contributor to CH4 emissions from paddy fields. Das and Baruah (2008) also reported that the length and volume of rice roots are significantly positively correlated with GHG emissions.
Apart from the roots, soil organic carbon (SOC) is another vital factor affecting the GHGs from paddy soil (Tokida et al., 2011), as SOC affects the microbial activity directly by providing substrates and nutrients, or indirectly by altering the oxygen exchange through root by affecting the root growth (Lu et al., 2002; Cai et al., 2016). Jiang et al. (2018) revealed that the contribution of GHG-related microorganisms to GHG emissions is higher than that of root aerenchyma only when the SOC concentrations are high enough. The SOC concentrations in this study were relatively low due to the absence of straw incorporation. Hence, it was the roots rather than the GHG-related microorganisms that play a determining role in the dynamic mechanisms of GHGs from paddy soils.
Although this study provides interesting insight into the dynamic mechanisms of GHGs in paddy fields under insect treatment, a few shortcomings still exist. For example, only the data of 1 year were collected. There was no comparison between the biological and chemical approaches by including chemical pesticide application as a treatment, and no straw was incorporated into the soil. Therefore, further research should be carried out to address these problems to acquire a more thorough understanding of the effects of this biological approach.
Conclusion
This study focused on the control effects of C. munakatae against C. suppressalis and their impacts on rice plants and GHG emissions from paddy fields. Compared with no insect treatment, C. suppressalis treatment significantly increased the ratio of dead tillers, inhibited the growth and vitality of rice roots and decreased the grain yield. However, the GHG emissions from paddy soil under the C. suppressalis treatment were significantly lower than those under no insect treatment, due to the morphological changes of rice roots. Chelonus munakatae could effectively control the C. suppressalis outbreak and alleviate the pest damage by reducing the ratio of dead tillers and enhancing the rice grain yield without the application of chemical pesticides. The relatively higher GHG emissions under C. munakatae + C. suppressalis treatment compared with those under C. suppressalis treatment alone, were acceptable since they were still significantly lower than those under no insect treatment. Therefore, the prevention and control of C. suppressalis outbreak in paddy fields by C. munakatae is an effective, environment-friendly and sustainable approach that should be widely promoted.
Data Availability Statement
The raw data supporting the conclusions of this article has been made available by the authors, without undue reservation, to any qualified researcher (Supplementary Data Sheet S1).
Author Contributions
CL conceived and designed the research. HZ, DF, and TL provided the experimental data. DF and HZ performed the statistical analysis. DF wrote the manuscript. CL and CC commented on and revised the manuscript.
Funding
This work was funded by the National Key Research and Development Project of China (2017YFD0301400), the National Natural Science Foundation of China (31671637), and the Natural Science Foundation of Hubei Province (2018CFB608). The funding sources had no involvement in study design, the collection, analysis and interpretation of data, the writing of the report or the decision to submit the article for publication.
Conflict of Interest
The authors declare that the research was conducted in the absence of any commercial or financial relationships that could be construed as a potential conflict of interest.
Acknowledgments
We sincerely thank Victoria Fernández, the associate editor of the journal, and the two reviewers for the constructive comments.
Supplementary Material
The Supplementary Material for this article can be found online at: https://www.frontiersin.org/articles/10.3389/fpls.2020.00228/full#supplementary-material
References
Allison, L. E. (1965). “Organic carbon,” in Methods of Soil Analysis, Part 2: Chemical and Microbiological Properties, ed. C. A. Black (Madison, WI: American Society of Agronomy Inc.), 1367–1378.
Angel, R., Claus, P., and Conrad, R. (2011). Methanogenic archaea are globally ubiquitous in aerated soils and become active under wet anoxic conditions. ISME J. 6, 847–862. doi: 10.1038/ismej.2011.141
Baethgen, W. E., and Alley, M. M. (1989). A manual colorimetric procedure for measuring ammonium nitrogen in soil and plant Kjeldahl digests. Commun. Soil Sci. Plant Anal. 20, 961–969. doi: 10.1080/00103628909368129
Banker, B. C., Kludze, H. K., Alford, D. P., DeLaune, R. D., and Lindau, C. W. (1995). Methane sources and sinks in paddy rice soils: relationship to emissions. Agric. Ecosyst. Environ. 53, 243–251. doi: 10.1016/0167-8809(94)00578-3
Beddington, J. R., Free, C. A., and Lawton, J. H. (1978). Characteristics of successful natural enemies in models of biological control of insect pests. Nature 273, 513–519. doi: 10.1038/273513a0
Bhattacharya, P., Nayak, A. K., Mohanty, S., Tripathi, R., Mohammad, S., Anjani, K., et al. (2013). Greenhouse gas emission in relation to labile soil C, N pools and functional microbial diversity as influenced by 39 years long-term fertilizer management in tropical rice. Soil Tillage Res. 129, 93–105. doi: 10.1016/j.scitotenv.2019.135909
Brussaard, L., Ruiter, P. C. D., and Brown, G. G. (2007). Soil biodiversity for agricultural sustainability. Agric. Ecosyst. Environ. 121, 233–244. doi: 10.1016/j.agee.2006.12.013
Cai, Y., Zheng, Y., Bodelier, P. L. E., Conrad, R., and Jia, Z. (2016). Conventional methanotrophs are responsible for atmospheric methane oxidation in paddy soils. Nat. Commun. 7:11728. doi: 10.1038/ncomms11728
Carvajal, M., Cooke, D. T., and Clarkson, D. T. (1996). Responses of wheat plants to nutrition deprivation may involve the regulation of water-channel function. Planta 199, 372–381.
Chen, H. F., Huang, S. S., Zhang, Y. Z., Zeng, X., and Huang, Z. H. (2010). Control efficacy of Trichogramma japonicum against Chilo suppressalis and Chilaraea auricilia. Chin. J. Appl. Ecol. 21, 743–748.
Cicerone, R. J., Shetter, J. D., and Delwiche, C. C. (1983). Seasonal variations of methane flux from a California rice paddy. Geophys. Res. Lett. 88, 7203–7209.
Conrad, R. (1996). Soil microorganisms as controllers of atmospheric trace gases (H2CO, CH4, OCS, N2O, and NO). Microbiol. Rev. 60, 609–640. doi: 10.1128/mmbr.60.4.609-640.1996
Dale, D. (1994). “Insect pests of the rice plant—their biology and ecology: stem borers,” in Biology and Management of Rice Insects, ed. E. A. Heinrichs (New Delhi: Wily Eastern Ltd.), 388–408.
Das, K., and Baruah, K. K. (2008). Methane emission associated with anatomical and morphophysiological characteristics of rice (Oryza sativa) plant. Physiol. Plant. 134, 303–312. doi: 10.1111/j.1399-3054.2008.01137.x
Duan, M. C., Liu, Y. H., Zhang, X., Zeng, W. G., and Yu, Z. R. (2012). Agricultural disease and insect-pest control via agro-ecological landscape construction. Chin. J. Eco Agric. 20, 825–831. doi: 10.3724/sp.j.1011.2012.00825
Groot, T. T., Bodegom, P. M. V., Meijer, H. A. J., and Harren, F. J. M. (2005). Gas transport through the root–shoot transition zone of rice tillers. Plant Soil 277, 107–116. doi: 10.1007/s11104-005-0435-4
Hosono, T., and Nouchi, I. (1997). Effect of gas pressure in the root and stem base zone on methane transport through rice bodies. Plant Soil 195, 65–73.
Huang, X. L., Wu, Z. P., Jiang, T., Zhang, W. N., and Xiao, H. J. (2018). Research and application progresses of biological control of rice stem borer Chilo suppressalis using Parasitoids. Chin. J. Biol. Control 34, 148–155.
Intergovernmental Panel on Climate Change [IPCC], (2013). Climate Change 2013: The Physical Science Basis Working Group I Contribution to the Fifth Assessment Report of the Intergovernmental Panel on Climate Change. Cambridge: Cambridge University Press.
Jia, Z. J., and Cai, Z. C. (2003). Effects of rice plants on methane emissions from paddy fields. Chin. J. Appl. Ecol. 14, 2049–2053.
Jiang, Y., Guan, D. H., and Zhang, W. J. (2018). Effects of rice plant traits on methane emissions from rice paddies: a review. Chin. J. Eco Agric. 26, 175–181.
Keeney, D. R., and Nelson, D. W. (1982). “Nitrogen-inorganic forms,” in Methods of Soil Analysis. Part 2 – Chemical and Microbiological Properties, Agronomy 9, 2nd Edn, eds R. H. Miller and D. R. Keeney (Madison, WI: American society of Agronomy), 643–698.
Kennedy, A. C., and Smith, K. L. (1995). Soil microbial diversity and the sustainability of agricultural soils. Plant Soil 170, 75–86. doi: 10.1007/978-94-011-0479-1_6
Khush, G. S., and Toenniessen, G. H. (1991). Rice Biotechnology. Wallingford: C.A.B. International in Association with the International Rice Research Institute.
Li, C., Zhang, Z., Guo, L., Cai, M., and Cao, C. (2013). Emissions of CH4 and CO2 from double rice cropping systems under varying tillage and seeding methods. Atmos. Environ. 80, 438–444. doi: 10.1016/j.atmosenv.2013.08.027
Losey, J. E., and Vaughan, M. (2006). The economic value of ecological services provided by insects. Bioscience 56, 311–323.
Lu, Y., Wassmann, R., Heinz-Ulrich, R., Neue, H. U., and Huang, C. (2000). Dynamics of dissolved organic carbon and methane emissions in a flooded rice soil. Soil Sci. Soc. Am. J. 64, 2011–2017. doi: 10.2136/sssaj2000.6462011x
Lu, Y., Wassmann, R., Neue, H. U., and Huang, C. (1999). Impact of phosphorus supply on root exudation, aerenchyma formation and methane emission of rice plants. Biogeochemistry 47, 203–218. doi: 10.1007/bf00994923
Lu, Y., Watanbe, A., and Kimura, M. (2002). Contribution of plant-derived carbon to soil microbial biomass dynamics in a paddy rice microcosm. Biol. Fertil. Soils 36, 136–142. doi: 10.1007/s00374-002-0504-2
Maki, K. (1930). The Biology of Chelonus munakatae. Mats. Alumni Soc. Morioka Agric. For. Coll. 6, 43–47.
Pathak, M. D. (1968). Ecology of common insect pests of rice. Annu. Rev. Entomol. 13, 257–294. doi: 10.1146/annurev.en.13.010168.001353
Pathak, M. D., Andres, F., Galacgac, N., and Raros, R. (1971). Resistance of Rice Varieties to Striped Rice Borers. Manila: international Rice Research Institute.
Reid, M. C., Pal, D. S., and Jaffe, P. R. (2015). Dissolved gas dynamics in wetland soils: root-mediated gas transfer kinetics determined via push-pull tracer tests. Water Resour. Res. 51, 7343–7357. doi: 10.1002/2014wr016803
Seiler, W., Holzapfel-Pschorn, A., Conrad, R., and Scharffe, D. (1984). Methane emission from rice paddies. J. Atmos. Chem. 1, 241–268. doi: 10.1007/bf00058731
Settle, W. H., Ariawan, H., Astuti, E. T., Cahyana, W., Hakim, A. L., Hindayana, D., et al. (1996). Managing tropical rice pests through conservation of generalist natural enemies and alternative prey. Ecology 77, 1975–1988. doi: 10.2307/2265694
Tokida, T., Adachi, M., Cheng, W., Nakajima, Y., Fumoto, T., and Matsushima, M. (2011). Methane and soil CO2 production from current-season photosynthates in a rice paddy exposed to elevated CO2 concentration and soil temperature. Glob. Chang. Biol. 17, 3327–3337. doi: 10.1111/j.1365-2486.2011.02475.x
Wang, D., and Ma, X. H. (2015). Evaluation of control effect of Trichogramma on Chilo suppressalis. Jiangsu Agric. Sci. 43, 113–115.
Wang, J., Zhang, M., Xiong, Z., Liu, P., and Pan, G. (2011). Effects of biochar addition on N2O and CO2 emissions from two paddy soils. Biol. Fertil. Soils 47, 887–896. doi: 10.1007/s00374-011-0595-8
Watanabe, A., Takeda, T., and Kimura, M. (1999). Evaluation of origins of CH4 carbon emitted from rice paddies. J. Geophys. Res. Atmos. 104, 23623–23629. doi: 10.1029/1999jd900467
Xu, H., Xing, G. X., and Cai, Z. C. (2000). Effect of soil water regime and soil texture on N2O emission from rice paddy field. Acta Pedol. Sin. 37, 499–505.
Yan, X., Shi, S., Du, L., and Xing, G. (2000). Pathways of N2O emission from rice paddy soil. Soil Biol. Biochem. 32, 437–440. doi: 10.1016/s0038-0717(99)00175-3
Yao, H., Yagi, K., and Nouchi, I. (2000). Importance of physical plant properties on methane transport through several rice cultivars. Plant Soil 222, 83–93.
Yu, K. W., Wang, Z. P., and Chen, G. X. (1997). Nitrous oxide and methane transport through rice plants. Biol. Fertil. Soils 24, 341–343. doi: 10.1007/s003740050254
Zhang, G., Yu, H., Fan, X., Ma, J., and Xu, H. (2016). Carbon isotope fractionation reveals distinct process of CH4 emission from different compartments of paddy ecosystem. Sci. Rep. 6:27065. doi: 10.1038/srep27065
Zhang, Z. S., Guo, L. J., Liu, T. Q., Li, C. F., and Cao, C. G. (2015). Effects of tillage practices and straw returning methods on greenhouse gas emissions and net ecosystem economic budget in rice-wheat cropping systems in central China. Atmos. Environ. 122, 636–644. doi: 10.1016/j.atmosenv.2015.09.065
Keywords: Chilo suppressalis, Chelonus munakatae, CH4, N2O, paddy field
Citation: Fan D, Zhang H, Liu T, Cao C and Li C (2020) Control Effects of Chelonus munakatae Against Chilo suppressalis and Impact on Greenhouse Gas Emissions From Paddy Fields. Front. Plant Sci. 11:228. doi: 10.3389/fpls.2020.00228
Received: 15 October 2019; Accepted: 13 February 2020;
Published: 06 March 2020.
Edited by:
Victoria Fernandez, Polytechnic University of Madrid, SpainReviewed by:
Gong-yin Ye, Zhejiang University, ChinaYu Jiang, Nanjing Agricultural University, China
Copyright © 2020 Fan, Zhang, Liu, Cao and Li. This is an open-access article distributed under the terms of the Creative Commons Attribution License (CC BY). The use, distribution or reproduction in other forums is permitted, provided the original author(s) and the copyright owner(s) are credited and that the original publication in this journal is cited, in accordance with accepted academic practice. No use, distribution or reproduction is permitted which does not comply with these terms.
*Correspondence: Chengfang Li, bGljaGVuZ2ZhbmdAMTI2LmNvbQ==
†These authors have contributed equally to this work