- Molecular Plant Biology Unit, Department of Biochemistry, University of Turku, Turku, Finland
In plant science, 2,4-dinitrophenylether of iodonitrothymol (DNP-INT) is frequently used as an alternative to 2,5-dibromo-6-isopropyl-3-methyl-1,4-benzoquinone (DBMIB) to examine the capacity of plastoquinol and semiquinone to reduce O2. DNP-INT is considered to be an effective inhibitor of the photosynthetic electron transfer chain (PETC) through its binding at the Q0 site of Cyt-b6f. The binding and action of DNP-INT has been previously characterized spectroscopically in purified Cyt-b6f complex reconstituted with Plastocyanin, PSII membranes and plastoquinone, as well as in isolated thylakoids based on its property to block MV-mediated O2 consumption. Contrary to the conclusions obtained from these experiments, we observed clear reduction of P700+ in samples incubated with DNP-INT during our recent investigation into the sites of oxygen consumption in isolated thylakoids. Therefore, we carried out an extensive investigation of DNP-INT’s chemical efficacy in isolated thylakoids and intact leaves. This included examination of its capacity to block the PETC before PSI, and therefore its inhibition of CO2 fixation. P700 redox kinetics were measured using Dual-PAM whilst Membrane Inlet Mass Spectrometry (MIMS) was used for simultaneous determination of the rates of O2 evolution and O2 consumption in isolated thylakoids and CO2 fixation in intact leaves, using two stable isotopes of oxygen (16O2, 18O2) and CO2 (12C, 13C), respectively. Based on these investigations we confirmed that DNP-INT is unable to completely block the PETC and CO2 fixation, therefore its use may produce artifacts if applied to isolated thylakoids or intact cells, especially when determining the locations of reactive oxygen species formation in the photosynthetic apparatus.
Introduction
Life in all three domains is sustained by membrane protein complexes participating in circuits that couple redox reactions and proton pumping, with the generation of ATP and NAD(P)H. The strategic application of chemical inhibitors, in isolation and in concert, has proven invaluable to study the roles, components and mechanisms of electron transport chains. In studies of photosynthetic systems, 3-(3,4-dichlorophenyl)-1,1-dimethylurea (DCMU), inhibitor of plastoquinone (PQ) reduction by PSII (Trebst, 2007; see Figure 1) and 2,5-Dibromo-6-isopropyl-3-methyl-1,4-benzoquinone (DBMIB), inhibitor of PQ-oxidation by cytochrome (Cyt)-b6f complex (Trebst, 2007; see Figure 1) have been the most regularly used and commercially available inhibitors. A drawback of the quinol base of DBMIB is that it possesses intrinsic redox functionality that in excess concentrations provides endogenous electron transport capacity able to bypass Cyt-b6f (Chain and Malkin, 1979). To counter this, 2,4-dinitrophenylether of iodonitrothymol (DNP-INT) was synthesized as a redox inert surrogate with characteristics otherwise similar to DBMIB (Trebst et al., 1978).
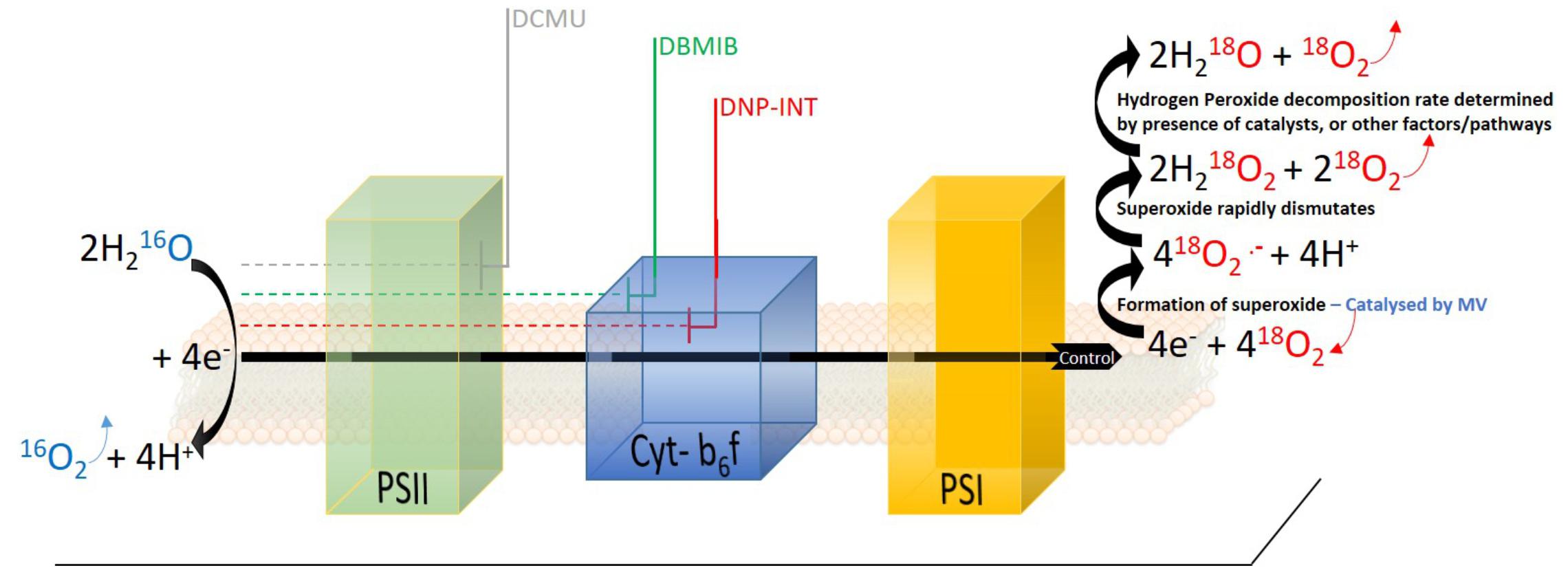
Figure 1. Schematic diagram of photosynthetic electron transport chain in isolated thylakoid samples when natural 16O2 isotope (Blue) was diminished significantly from the measuring chamber and enriched with the heavy 18O2 isotope (Red). One molecule of 16O2 (Blue) and four electrons are produced through oxidation of two water molecules at PSII. Electrons are transferred to four molecules of 18O2 at the acceptor side of PSI (potentially also within PQ pool), forming four superoxide molecules (step catalyzed by methyl viologen, MV). The superoxide dismutates rapidly into two hydrogen peroxide molecules, releasing two molecules of 18O2. Further decomposition of H2O2 to H2O and O2 is the final step in the electron transfer pathway of the water-water cycle. Approximate locations of inhibition of electron transport targeted by DCMU, DBMIB, and DNP-INT are indicated in the figure.
Significant effort has been committed to characterize the inhibitory action of DNP-INT in the photosynthetic electron transfer chain (PETC). Absorption spectroscopy measurements of purified Cyt-b6f complex reconstituted with plastocyanine (PC), PQ, photosystem (PS)I and PSII in the reaction medium (Lam and Malkin, 1983; O’Keefe, 1983), along with low temperature electron paramagnetic resonance (EPR) spectroscopy of purified Cyt-b6f complex (Malkin, 1986; Roberts and Kramer, 2001) have shown that DNP-INT blocks oxidation of plastoquinol and reduction of PC by binding at the Q0 site of Cyt-b6f. Furthermore, functional measurements have been used to demonstrate the effectiveness of DNP-INT in blocking linear electron transfer in more complex samples, such as isolated thylakoids. To this end, the oxygen (O2) consumption rate upon exposure of thylakoid samples to light was measured with a Clarke type O2 electrode in the absence of artificial electron acceptors (Khorobrykh and Ivanov, 2002). These experiments demonstrated similar O2 uptake rates between untreated and DNP-INT incubated thylakoid samples, yet the addition of methyl viologen (MV), a strong catalyst of O2 reduction at PSI acceptor side (see Figure 1), increased Net O2 uptake rates only in samples lacking DNP-INT. This was interpreted as strong evidence that DNP-INT blocks electron flow via PSI to MV, and therefore the chemical effectively truncates PSI from the PETC. Furthermore, similarity in O2 uptake rates observed between untreated and DNP-INT treated samples suggested the presence of superoxide forming pathways, similar to that in PSI, also associated with the reduced PQ pool and Cyt-b6f complex (Khorobrykh and Ivanov, 2002; Mubarakshina and Ivanov, 2010; Borisova-Mubarakshina et al., 2018).
The results from O2 electrode measurements with isolated thylakoids were interpreted as the ability of DNP-INT to allow semiquinone formation, whilst simultaneously blocking Cyt-f reduction (Mubarakshina and Ivanov, 2010), which made DNP-INT a critical tool in truncating the PETC at Cyt-b6f. It has resulted in the wide use of DNP-INT as an alternative to DBMIB for truncation of PSI from the PETC, particularly in works to explore reactive oxygen species (ROS) formation and scavenging pathways involving both reduced PQ-pool and semiquinones (Khorobrykh and Ivanov, 2002; Heyno et al., 2009; Mubarakshina and Ivanov, 2010; Borisova-Mubarakshina et al., 2018). Based on these characterizations, use of DNP-INT has provided a range of significant findings in plant (Stepien and Johnson, 2009) and algal physiology (Barbagallo et al., 1999), bioenergetics (Malnoe et al., 2011), bio-fuel applications (Mus et al., 2005), biochemical characterizations of PETC components (Krieger-Liszkay et al., 2000) and efforts to determine sites and activity of ROS formation/quenching (Khorobrykh and Ivanov, 2002; Heyno et al., 2009; Mubarakshina and Ivanov, 2010; Vetoshkina et al., 2017; Borisova-Mubarakshina et al., 2018).
In our current work, we attempted to determine the contribution of PSI in overall light-induced O2 reduction from isolated thylakoids and intact leaf discs. For this, we planned to truncate the PETC with inhibitors such as DBMIB and DNP-INT. As a control for successful and complete truncation of PSI from the PETC under our specific experimental conditions, we measured both isolated thylakoid and intact leaf samples with a Dual PAM to record the redox kinetics of P700. However, DNP-INT failed to block linear electron transport to PSI in both sample types. After controlling for the chemical structure of our commercially procured DNP-INT with 1HNMR spectroscopy, and following our successful reproduction of the frequently published O2 electrode results (Khorobrykh and Ivanov, 2002; Vetoshkina et al., 2017) described above, we embarked on a thorough investigation of PSI redox kinetics and photosynthetic gas exchange in DNP-INT treated samples. To confirm whether DNP-INT truly truncates PSI from the PETC, we compared the redox kinetics of P700 measurements incubated with DNP-INT and other well characterized PETC inhibitors. MIMS was used to distinguish the O2 consuming and O2 producing reactions occurring simultaneously in isolated thylakoid samples to test conclusions drawn from O2 electrode data, and was used then to measure rates of CO2 fixation in leaf discs infiltrated with DNP-INT.
Materials and Methods
Leaf Samples and Isolation of Thylakoids
Thylakoids were isolated from 6 week old Arabidopsis thaliana plants, grown at 16/8 h dark/light cycle at 120 μmol photons m2 s1 at atmospheric CO2. Thylakoids were isolated as described earlier (Tiwari et al., 2016) except all measurements were performed with freshly isolated thylakoids in buffer containing: 330 mM Sorbitol, 5 mM MgCl2, 10 mM NaCl, 5 mM NH4Cl, 50 mM Hepes (pH 7.6). Leaf measurements in MIMS and Dual-PAM were taken from the same plants.
Inhibitor Stocks
The inhibitors DCMU (Sigma, United States), DBMIB (Sigma, United States) were prepared as standard in ethanol. We purchased two separate stocks of DNP-INT (caymann chemicals batch no. 0468094-2, 0468093-2, 0524164-2) with the second being sent for analytical analysis to confirm purity via 1HNMR. In addition we received another DNP-INT stock from the original Trebst preparation (donated by Anja Krieger). All DNP-INT stock solutions were prepared in dimethyl sulfoxide and used separately to repeat experiments as stated in the text. For conducting each experiment, all stock solutions used were freshly prepared and the concentrations of all inhibitors used were based on those experimentally demonstrated in literature to completely block electron transfer process.
MIMS Measurements of Isolated Thylakoid Membranes
Freshly isolated thylakoid samples of known chlorophyll concentration were stored on ice in darkness. A Sentinel-PRO magnetic sector mass spectrometer (Thermo Fisher Scientific, United States) was employed to collect masses 32 and 36 with a total cycle time of approximately 4.5 s. For each run sufficient measurement buffer (containing: 330 mM Sorbitol, 5 mM MgCl2, 10 mM NaCl, 5 mM NH4Cl, 50 mM Hepes pH 7.6) was loaded into the cuvette equilibrated and calibrated to 25°C, thylakoids were added (equivalent to approximately 50 μg chlorophyll) to a final volume of 1000 μl. In darkness the sample was briefly purged with N2 to reduce the background 16O2 signal before a bubble of 18O2 (Cambridge Isotope Laboratories Inc., United Kingdom) was loaded into the stirring liquid, bringing the concentration of the heavier isotope up to approximately 150 nmol ml1. The bubble was removed and inhibitors were injected at this moment (either 10 μM DCMU, 10 μM MV, 10 μM DBMIB, 10 μM DNP-INT) and samples equilibrated in darkness for 5 min before data acquisition was started. Samples were illuminated via halogen lamp (Dolan Jenner, United States) at 120 μmol photons m2 s1. At the end of each run, the Chl concentration of the sample was determined in triplicate using the Porra Method (Porra et al., 1989) in 90% MeOH to ensure accurate normalization of rates between samples. The cuvette was washed thoroughly with multiple rinses of 70% Ethanol followed by MQ H2O when changing between inhibitors to avoid cross contamination. This was checked by running controls. All data was analyzed and fluxes calculated with equations described in Beckmann et al. (2009).
MIMS Measurements of Leaf Discs
Leaf discs (14 mm) were cut from detached leaves and floated in darkness in either H2O (control) or H2O + 10 μM DBMIB or H2O + 10 μM DNP-INT. Samples were incubated overnight in darkness at 25°C. For measurements, all excess water from leaf surface was removed and a smaller 12.5 mm disc was cut from the larger disc to remove the old edge. This was loaded into an in-house built stainless steel cuvette of 1000 μl volume, equilibrated and calibrated at 25°C, using Teflon (Hansatech) membrane to separate the sample space from the high vacuum line of the Mass Spectrometer. With the disc maintained in darkness, the cuvette was purged with N2 to remove atmospheric 16O2 and 12CO2 before 18O2 (as above) and 13CO2 (Sigma-Aldrich, United States) were injected to approximately 3 and 2% by volume, respectively. Discs were kept for approximately 5 min in darkness inside the cuvette to ensure isotopic equilibrium in the system before the measurement started. The instrument recorded m/z 32, 36, 44, and 46 with a time resolution of approximately 6.5 s. After 4 min dark a halogen light directed via a liquid light guide illuminated the samples at 120 then 520 μmol photons m–2 s–1. All data was analyzed and fluxes calculated with equations described in Beckmann et al. (2009). Leaf discs are often used as a surrogate for intact leaf measurements. Although experimental limitations relating to altered physiological responses do exist between a leaf disc and an intact leaf, for the purposes of demonstrating functional PSI activity via CO2 fixation, these limitations do not apply to this set of experiments.
P700 Redox Kinetics Measurements
P700 redox kinetics were measured with detached leaves and isolated thylakoids using Dual-PAM-100 or Dual-Klass NIR (Walz, Germany). Isolated thylakoids in Dual Pam were measured at 100 μg Chl ml–1 using liquid sample holder. The P700 was oxidized under continuous far red (FR) light. Activating two short pulses of saturating actinic light i.e., single turnover (ST) (50 μs) and multiple turnover (MT) (50 ms) pulses, over the FR light oxidized P700, induced partial P700 re-reduction by electrons from PSII (Tiwari et al., 2016). Infiltration of inhibitors was conducted in the same manner as described for MIMS, however, the incubation time for leaves was only 1 h.
O2 Electrode Measurements
Thylakoid membranes prepared for MIMS measurements were also submitted to the Clark-type O2 electrode (Hansatech, United Kingdom) measurements according to the methods described (Khorobrykh and Ivanov, 2002). 15 μg Chlorophyll ml–1 of sample was injected to 1000 μl of the same measurement buffer used in MIMS measurements. Inhibitors were added as described in the text. Samples were loaded in darkness and after one minute of dark data acquisition the halogen lamp was turned on (800 μmol photons m–2 s–1) for 4 min (total 5 min).
Results and Discussion
P700 Redox Kinetics Suggested DNP-INT Does Not Truncate PSI From the PETC
In attempts to differentiate rates of O2 reduction between the acceptor side of PSI and the reduced PQ pool/Cyt-b6f complex, we have tested the efficacy of DBMIB and DNP-INT, two well characterized chemicals used to truncate PSI from the PETC, to validate that PSI was not reduced during our measurements. To examine this question we used a Dual PAM to measure the redox kinetics of P700 from both intact leaf and isolated thylakoid samples incubated with these inhibitors, and further compared them with DCMU as an unambiguous control for the inhibition of all intersystem electron transport between PSII and PSI (Figures 2A,B).
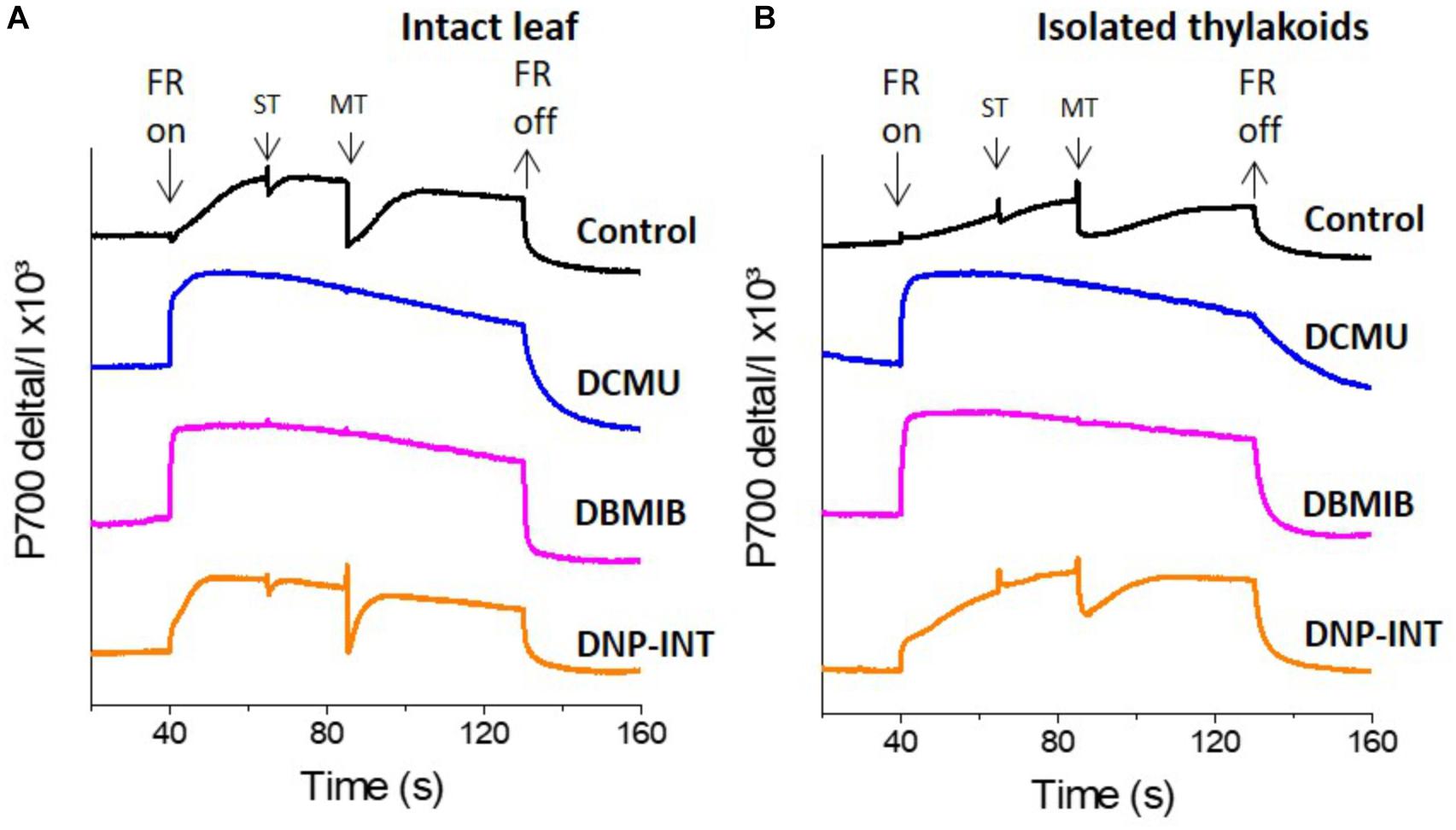
Figure 2. Comparison of P700 redox kinetics from DCMU, DBMIB and DNP-INT infiltrated intact leaf and isolated thylakoid samples, measured with Dual Pam. (A) P700 redox kinetics of intact leaves; (B) P700 redox kinetics of isolated thylakoids. Uncoupled thylakoid samples were measured in liquid sample holder cuvette of Dual Pam at a chl concentration of 100 μg Chl ml–1 in buffer containing only 330 mM Sorbitol, 5 mM MgCl2, 10 mM NaCl, 5 mM NH4Cl, 50 mM Hepes pH 7.6 or with each chemical modulator at 10 μM as indicated in figure. Leaves were infiltrated in darkness using water for the control sample and all inhibitors were used at 10 μM concentration. Representative curves are average of minimum n = 5 samples. FR = P700 oxidizing Far Red light. ST = Single turnover (50 μs) actinic light pulse, MT = multiple turnover (50 ms) saturating light pulse.
Following a dark period, addition of a constant FR light oxidized P700 to P700+ in all samples (Figures 2A,B). Transient re-reduction of P700+ to P700 by PSII derived electrons was achieved in inhibitor-free controls (Black lines, Figures 2A,B) via superimposition of saturating ST and MT actinic light pulses over the oxidizing FR light. Such transient re-reduction requires, and therefore tested, the function of the entire intersystem PETC (Fan et al., 2016; Tiwari et al., 2016). Extinguishing the FR light allowed P700+ to re-reduce to P700, at varying rates, in all samples. In a clear demonstration of successful PSI truncation from the rest of the PETC, the level of P700+ was largely unaffected following the ST and MT actinic pulses in both leaf and thylakoid samples treated with 10 μM DCMU (Blue lines) and 10 μM DBMIB (Pink lines). Unexpectedly, leaf and thylakoid samples treated with 10 μM DNP-INT (Orange lines) behaved in a manner resembling untreated controls. Although smaller in amplitude as compared to the controls, these dips (re-reduction of P700+) produced by ST and MT actinic pulses in the P700+ curves of DNP-INT treated leaf and thylakoid samples were undeniably present.
This suggested that electrons liberated by PSII were able to transiently reduce P700+ to P700, whilst the smaller amplitude of the dips suggested that the number of electrons transferred to P700+ in DNP-INT treated samples was impaired, compared to untreated samples. We also used a Dual Klass NIR to measure the redox kinetics of PC (Supplementary Figure S1), which accepts electrons exclusively from Cyt-f. For all treatments, the PC kinetics essentially matched with those discussed for the P700 data. This suggests that use of DNP-INT at 10 μM in isolated thylakoid samples did not block reduction of Cyt-f, and therefore P700 was still actively coupled to PSII, as opposed to the clear inhibition of PC and P700+ re-reduction produced by the treatment of samples with DCMU and DBMIB.
Our DNP-INT Stocks and Isolated Thylakoids Reproduce Published Data
The concentration, quality and activity of our DNP-INT stocks were checked before making further investigations into the results of the Dual PAM data. Although the 10 μM DNP-INT concentration used in our protocol was at the high end of published protocols, and five times the “minimum concentration required for complete inhibition” of 2 μM (Trebst et al., 1978), we tested the possibility that we had under-dosed the DNP-INT samples by conducting a concentration response curve (see Supplementary Figure S1). In this experiment, DNP-INT failed to block the transient re-reduction of P700 caused by actinic light pulses in both the leaf and thylakoid samples from 2 to 500 μM concentration. That is 1000× the published minimum concentration required and 100× the concentration generally reported when DNP-INT is used to truncate PSI from the PETC (Krieger-Liszkay et al., 2000; Khorobrykh and Ivanov, 2002; Borisova-Mubarakshina et al., 2018). Another important question was to assure the purity of the DNP-INT we had procured. Historically DNP-INT has only been available to researchers through collaboration with its creator, Prof Achim Trebst. However, companies have recently started to synthesize and market this chemical as an inhibitor of PQ oxidation. Although our supplier, Cayman Chemicals, provided a full analytical analysis of their product to validate its purity, we repeated our experiments with a second stock purchased from a separate batch and independently verified the structure of the product. The Turku University Instrumentation Center conducted 1H NMR on our DNP-INT stock, confirming the chemical’s structure and that the two benzene rings were connected (see Supplementary Figure S2). Additionally, we have repeated the P700 redox kinetics experiment with a sample of the original DNP-INT stock synthesized by Prof Achim Trebst, kindly donated to us by Anja Krieger. Significantly, the original stock of DNP-INT did not block electron flow to PSI in isolated thylakoids or intact leaves (see Supplementary Figure S3), validating the conclusions we have based on the commercially procured chemical.
To further test the reliability of our DNP-INT samples, and to confirm the proper functionality of the isolated thylakoid preparations, we decided to replicate published measurements that purportedly demonstrate effectiveness of DNP-INT in truncating PSI from the PETC (Trebst et al., 1978; Khorobrykh and Ivanov, 2002).
In this measurement, a Clark-type O2 electrode was used to compare Net O2 fluxes from isolated thylakoid samples (Figure 3), initially in the absence of artificial electron acceptors but with and without DNP-INT. The experiment was repeated with similar samples but MV was injected after one minute of illumination to observe its capacity to increase Net O2 uptake fluxes. The results (Figure 3F) faithfully reproduce the published outcome (Trebst et al., 1978; Khorobrykh and Ivanov, 2002). Compared to the untreated control (Black line), addition of DNP-INT (10 μM) to isolated thylakoid samples decreased rates of illuminated Net O2 uptake (Blue line). The addition of MV to the DNP-INT treated samples had no effect on this impaired flux (Pink line), in stark contrast to MV’s large effect of increasing the net O2 flux of untreated control samples (Red line). The fact that MV cannot increase O2 uptake rates in the presence of DNP-INT is traditionally interpreted as functional evidence for truncation of PSI from the PETC by 10 μM DNP-INT. Based on the following conclusions: (a) our independent 1H NMR analysis of the commercially procured DNP-INT stock (b) that we could faithfully re-produce the published O2 electrode result with the commercially procured DNP-INT stock and our preparation of thylakoid material, and (c) the successful reproduction of our initial P700 experiments using an original DNP-INT stock synthesized by Prof Trebst, we concluded that redox kinetics of PSI presented in Figure 2 were not the result of any artifacts and warranted an in-depth investigation.
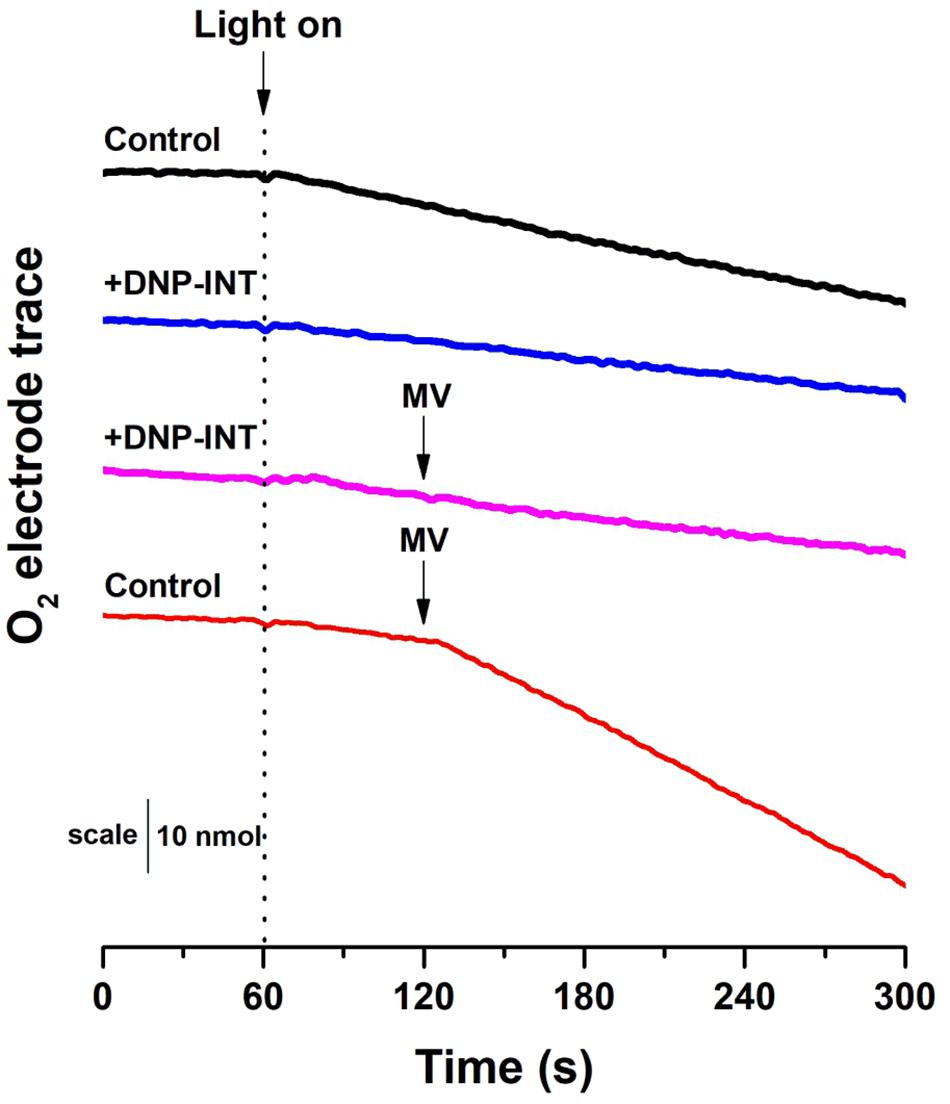
Figure 3. Raw traces from O2 electrode to demonstrate that our materials and methods can reproduce O2 electrode data historically used to show the efficacy in DNP-INT. Raw [O2] vs time traces were generated with thylakoids isolated from Arabidopsis thaliana. All measurements were conducted at a starting [O2] of approximately 250 nmol ml–1. Light intensity was 800 μmol photons m–2 s–1, Thylakoids equivalent to 15 μg Chl ml1. Each curve is an average of n = 6 replicate measurements.
MIMS Data Showed That O2 Electrode Assumptions Are Not Always Correct
Interpretation of data in Figure 2 surprised us by suggesting that DNP-INT did not fully inhibit the re-reduction of P700+ by actinic light pulses, as was expected and observed in samples incubated with DBMIB and DCMU (Figure 2). At the same time, the O2 electrode data in Figure 3 has been interpreted to imply that DNP-INT effectively blocks electron donation to MV (Figure 3) and therefore PSI is effectively truncated from the PETC by DNP-INT. We inferred the best explanation for this discrepancy to rest with the inherent limitations of the Clark-type O2 electrode, which can only measure Net O2 fluxes. This is particularly problematic when the stoichiometry of O2 production and consumption is not clearly known, as is the case when O2 is both produced and consumed in the same sample, at unknown relative rates depending on the characteristics of the O2 reduction pathways active under different conditions (see Figure 1). To investigate whether this limitation of O2 electrodes has potentially contributed to miscalculate/misinterpret the efficacy of DNP-INT’s activity in isolated thylakoid samples, we employed the resolving power of MIMS. MIMS is able to discriminate between O2 isotopologues to independently and simultaneously measure activity of PSII, and concomitant reduction of O2 in isolated thylakoids (Furbank and Badger, 1983). By independently measuring the activity of PSII it was possible to unambiguously judge whether MV added to the DNP-INT inhibited samples could increase the rate of O2 evolution or not, providing a clear test of whether or not PSI is truncated from the PETC in isolated thylakoids. As a final test of the capacity for DNP-INT to impair electron donation to PSI in intact leaves, we used the MIMS to measure whether or not leaf discs infiltrated with DNP-INT could still fix CO2 in comparison to those infiltrated with DBMIB.
In both isolated thylakoid and leaf disc samples, PSII activity was measured as the Gross O2 evolution rate, [or production of 16O2 derived from PSII splitting of H216O (see Figure 1)]. The reduction of O2 was measured directly through enrichment of the samples with the stable 18O2 isotope, which following an N2 purge to reduce 16O2 became the primary electron acceptor in the isolated thylakoid samples. Leaf disc samples were purged with N2 gas before being enriched to approximately 2% with 13CO2, used as the terminal electron acceptor, and 3% 18O2 to monitor O2 reduction rates. Photorespiration in leaf discs was minimized by maintaining an elevated CO2 partial pressure, which left mitochondrial respiration and potentially the Mehler reaction as primary O2 uptake pathways. Monitoring 12CO2 efflux rates derived from mitochondrial respiration enabled an estimate of illuminated respiration rates, minus any CO2 re-fixation (Busch et al., 2013; Busch, 2018) to offset its contribution to 18O2 consumption, whilst the latter was minimized through low O2 partial pressure.
MIMS data from isolated thylakoid samples are presented in Figures 4A–D. When illuminated at 120 μmol photons m–2 s–2 little difference in PSII activity (Blue lines, Figure 4) could be observed between control and DNP-INT treated thylakoids (Figures 4A,C). However, Gross O2 uptake rates (Red Lines, Figure 4) were slightly lower in the latter, resulting in Net O2 fluxes (Gray Lines, Figure 4), calculated from the difference between the two Gross O2 rates, being smaller (less negative) in the DNP-INT samples. The difference in Net O2 fluxes matches the results from the Clark-type O2 electrode in Figure 3. However, the observation that O2 evolution rates were actually very similar between the two conditions was unexpected. The smaller O2 uptake rates in DNP-INT treated samples (Figure 4C) suggests that less H2O2 accumulated in the presence of this chemical, potentially due to unknown complexities of ROS scavenging pathways within the thylakoid membrane.
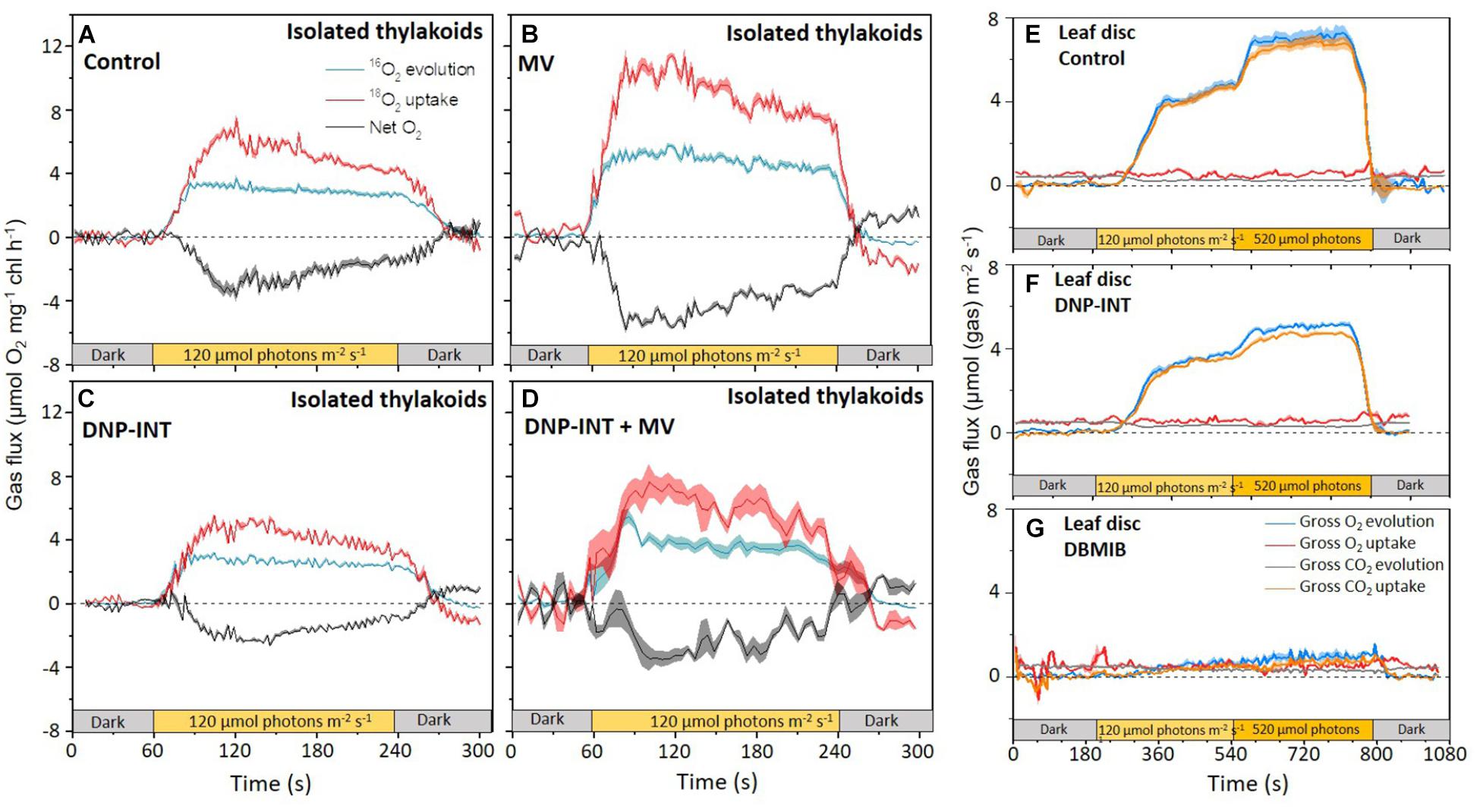
Figure 4. Integrated gas flux versus time plots of isolated thylakoid and intact leaf disc samples (±SE) Simultaneously measured rates of O2 Evolution and Consumption from isolated thylakoids (50 μg chlorophyll ml–1) (A–D), with the addition of CO2 consumption and production rates from intact 12.5 mm leaf discs (E–G) measured with MIMS. Thylakoid samples measured under similar conditions used in Dual-PAM, see Figure 2. Leaf discs were floated overnight in water, or water containing 10 μM DNP-INT or 10 μM DBMIB in darkness. The rates of all isolated thylakoids were offset to zero in pre-illuminated darkness. Isolated thylakoid curves comprise averaged data from n = 7 (Control), n = 8 (DNP-INT), n = 8 (MV) and n = 4 (DNP-INT + MV) independent replicates. Leaf disc curves comprise the averaged data of n = 4 (control), n = 4 (DNP-INT) n = 3 (DBMIB) independent replicates.
Addition of MV to thylakoids (Figure 4B) resulted in a large increase to rates of PSII O2 evolution and Gross O2 uptake, compared to the control, demonstrating that PSII activity is dictated by the strength of the acceptors in isolated thylakoid samples. The proportionate increase in both O2 fluxes resulted in an increased difference between them, producing a larger negative Net O2 flux, which also strongly reflected the Clark-type O2 electrode data in Figure 3. Whilst it was clear that DNP-INT impeded rates of MV photoreduction (Figure 4D), it was also clear that MV increased rates of PSII activity in the DNP-INT inhibited samples (for a direct comparison refer to Supplementary Figure S4 where we plot the Gross O2 evolution rates measured under the four conditions on the same axis). This suggests that in-spite of the presence of DNP-INT, MV was able to increase the acceptor side capacity, which strongly supports the conclusions from P700 data in Figure 2, implying that DNP-INT does not truncate PSI from the PETC. In addition, the data shows that O2 uptake rates did not increase proportionately with the increased PSII activity. This resulted in a smaller increase in the negative Net O2 flux than would be predicted from a measure of PSII O2 evolution alone, which again fits the O2 electrode data in Figure 3 and suggests that DNP-INT treated samples seem to generate less stable H2O2 than samples lacking this compound. Overall, deconvolution of O2 generation and uptake afforded by MIMS has revealed that the Net O2 fluxes observed do not necessarily correlate to the activity of PSII for a given condition. This suggests that the Net rates reported by Clark-type O2 electrodes are unable to provide sufficient information to accurately interpret the site-specific complexities of ROS formation and scavenging in isolated thylakoid membrane samples lacking artificial donors and acceptors.
The final experiment to test the efficacy of DNP-INT to block electron transport to PSI in infiltrated leaves was to measure the capacity of intact leaves, infiltrated with 10 μM DNP-INT, to fix CO2 during illumination using MIMS. Again, DBMIB was used as a comparison when electron transport at the Cyt-b6f complex was significantly inhibited. After 5 min darkness, leaf discs were illuminated 5 min at 120 μmol photons m–2 s–1, then 5 min at 520 μmol photons m–2 s–1 before extinguishing the actinic light. As expected, photosynthetic activity was severely impaired by infiltration with DBMIB (Figure 4G) compared to untreated controls (incubated in water, Figure 4E). However, samples incubated with DNP-INT (Figure 4F) exhibited only a small reduction, of approximately 30%, in CO2 fixation rates during illumination at both irradiances. In all samples, rates of mitochondrial respiration (CO2 efflux, gray lines Figures 4E–G) were similar.
Conclusion
In efforts to assess the contribution of PSI-independent O2 reduction pathways operating during illumination within the PETC, it is necessary to disconnect PSI completely from the rest of the PETC with effective inhibitors. Whilst DCMU and DBMIB functioned as expected in this regard, we were surprised when our initial results suggested that DNP-INT failed to block re-reduction of P700+ at concentrations up to 1000× the published minimum concentration required. To verify that our materials were not producing an artifact, we confirmed the structure of our commercially procured DNP-INT stocks through 1H NMR and we successfully reproduced a published O2 electrode experiment used historically to support the contention that DNP-INT effectively truncates PSI from the PETC in isolated thylakoid samples. Then we again compared the rates of P700+ re-reduction measured with a Dual-PAM between untreated controls, the inhibitors DCMU, DBMIB and both the commercially procured and original stocks of DNP-INT. Whilst DBMIB and DCMU clearly blocked complete re-reduction of P700+ in both isolated thylakoid and leaf disc samples, neither stock of DNP-INT was able to replicate this result. This suggests that DNP-INT can only slow electron transfer through the PETC. The implication of this conclusion is that DNP-INT fails to block the Mehler reaction during in vitro experiments, or the carbon reduction cycle in intact samples. Using MIMS to independently and simultaneously measure the rates of O2 production and consumption in isolated thylakoids, we demonstrated that MV added to DNP-INT treated samples was able to increase rates of PSII activity. However, the O2 uptake rate did not increase commensurately, potentially due to unknown complexities of ROS scavenging within thylakoid membranes. As such, the Net O2 flux did not appear to be different from the DNP-INT treated samples lacking MV. Through these experiments, we demonstrated that measurements of Net O2 fluxes with an O2 electrode are insufficient for measurements of the site-specific O2 reduction pathways operating within thylakoid membranes, due to the complexities of the competing routes of superoxide formation and quenching that potentially invalidate some assumptions underpinning the interpretation of Net O2 fluxes. Finally, we showed that leaf discs incubated with DNP-INT could still fix CO2 at rates impaired by approximately 30%, whereas DBMIB severely impaired all photosynthetic fluxes from similar samples. We suggest that DNP-INT should no longer be used as an inhibitor of PSI reduction and that results reliant on the assumption that DNP-INT had effectively inhibited the activity of PSI must be revisited. Nonetheless, the chemical may still find use for its apparent ability to slow electron transport between the PQ pool and PSI. This could be useful as a tool to artificially replicate the induction of photosynthetic control, imparted by the Cyt-b6f complex by an acidified lumen, potentially in the absence of NPQ or state transitions. It may also find use with researchers trying to modulate electron transfer rates in biophotovoltaic applications (Tschörtner et al., 2019). Finally, the disagreement in results between characterization studies of DNP-INT in purified complexes and our findings from intact samples may point to currently unknown aspects of Cyt-b6f function. Perhaps DNP-INT only partially blocks Cyt-b6f due to competitive inhibition with some other substrate that may now be studied with DNP-INT. Or perhaps the results reflect a sub-population of DNP-INT sensitive Cyt-b6f which may correlate to some spatial distribution in the thylakoid membrane, or its participation in super or mega complexes. Further work to explain the apparent discrepancies of DNP-INT function between spectroscopic measurements in highly purified samples and our results obtained with isolated thylakoids and intact leaf discs may yield significant information about photosynthesis in the future.
Data Availability Statement
The datasets generated for this study are available on request to the corresponding author.
Author Contributions
AT, DF, and E-MA conceptualized and designed the work. DF and AT carried out the experimental work, the data analysis and interpretation of the data. DF drafted the manuscript. AT and E-MA revised the content for final submission.
Funding
Research was funded by the Center of Excellence program of the Academy of Finland (project no 307335) and by the Jane and Aatos Erkko Foundation.
Conflict of Interest
The authors declare that the research was conducted in the absence of any commercial or financial relationships that could be construed as a potential conflict of interest.
Acknowledgments
Thanks to Tuomas Karskela at the Turku instrument Center for 1H NMR measurement and analysis. We thank Dr. Anja Krieger-Liszkay for providing original DNP-INT synthesized by Prof. Achim Trebst.
Supplementary Material
The Supplementary Material for this article can be found online at: https://www.frontiersin.org/articles/10.3389/fpls.2020.00382/full#supplementary-material
References
Barbagallo, R. P., Finazzi, G., and Forti, G. (1999). Effects of inhibitors on the activity of the cytochrome b(6)f complex: evidence for the existence of two binding pockets in the lumenal site. Biochemistry 38, 12814–12821. doi: 10.1021/bi990424+
Beckmann, K., Messinger, J., Badger, M. R., Wydrzynski, T., and Hillier, W. (2009). On-line mass spectrometry: membrane inlet sampling. Photosynthesis Res. 102, 511–522. doi: 10.1007/s11120-009-9474-7
Borisova-Mubarakshina, M. M., Naydov, placeI. A., and Ivanov, B. N. (2018). Oxidation of the plastoquinone pool in chloroplast thylakoid membranes by superoxide anion radicals. FEBS Lett. 592, 3221–3228. doi: 10.1002/1873-3468.13237
Busch, F. A. (2018). Photosynthetic gas exchange in land plants at the leaf level. Methods Mol. Biol. 1770, 25–44. doi: 10.1007/978-1-4939-7786-4_2
Busch, F. A., Sage, T. L., Cousins, A. B., and Sage, R. F. (2013). C3 plants enhance rates of photosynthesis by reassimilating photorespired and respired CO2. Plant Cell Environ. 36, 200–212. doi: 10.1111/j.1365-3040.2012.02567.x
Chain, R. K., and Malkin, R. (1979). On the interaction of 2,5-dibromo-3-methyl-6-isopropylbenzoquinone (DBMIB) with bound electron carriers in spinach chloroplasts. Arch. Biochem. Biophys. 197, 52–56. doi: 10.1016/0003-9861(79)90217-0
Fan, D. Y., Fitzpatrick, D., Oguchi, R., Ma, W., Kou, J., and Chow, W. S. (2016). Obstacles in the quantification of the cyclic electron flux around Photosystem I in leaves of C3 plants. Photosynth Res. 129, 239–251. doi: 10.1007/s11120-016-0223-4
Furbank, R. T., and Badger, M. R. (1983). Oxygen exchange associated with electron transport and photophosphorylation in spinach thylakoids. Biochim. Biophys. Acta 723, 400–409. doi: 10.1016/0005-2728(83)90047-6
Heyno, E., Gross, C. M., Laureau, C., Culcasi, M., Pietri, S., and Krieger-Liszkay, A. (2009). Plastid alternative oxidase (PTOX) promotes oxidative stress when overexpressed in tobacco. J. Biol. Chem. 284, 31174–31180. doi: 10.1074/jbc.M109.021667
Khorobrykh, S. A., and Ivanov, B. N. (2002). Oxygen reduction in a plastoquinone pool of isolated pea thylakoids. Photosynth Res. 71, 209–219.
Krieger-Liszkay, A., Kienzler, K., and Johnson, G. N. (2000). Inhibition of electron transport at the cytochrome b(6)f complex protects photosystem II from photoinhibition. FEBS Lett. 486, 191–194. doi: 10.1016/s0014-5793(00)02250-x
Lam, E., and Malkin, R. (1983). Characterization of electron transfer from water to plastocyanin catalyzed by resolved electron transfer complexes from chloroplasts. Arch. Biochem. Biophys. 224, 456–463. doi: 10.1016/0003-9861(83)90232-1
Malkin, R. (1986). Interaction of stigmatellin and DNP-INT with the Rieske iron-sulfur center of the chloroplast cytochrome b6-f complex. FEBS Lett. 208, 317–320. doi: 10.1016/0014-5793(86)81041-9
Malnoe, A., Wollman, F. A., de Vitry, C., and Rappaport, F. (2011). Photosynthetic growth despite a broken Q-cycle. Nat. Commun. 2:301. doi: 10.1038/ncomms1299
Mubarakshina, M. M., and Ivanov, B. N. (2010). The production and scavenging of reactive oxygen species in the plastoquinone pool of chloroplast thylakoid membranes. Physiol. Plant. 140, 103–110. doi: 10.1111/j.1399-3054.2010.01391.x
Mus, F., Cournac, L., Cardettini, V., Caruana, A., and Peltier, G. (2005). Inhibitor studies on non-photochemical plastoquinone reduction and H(2) photoproduction in Chlamydomonas reinhardtii. Biochim. Biophys. Acta 1708, 322–332. doi: 10.1016/j.bbabio.2005.05.003
O’Keefe, D. P. (1983). Sites of cytochrome b-563 reduction, and the mode of action of DNP-INT and DBMIB in the chloroplast cytochrome b-563/f complex. FEBS Lett. 162, 349–354. doi: 10.1016/0014-5793(83)80786-8
Porra, R. J., Thompson, W. A., and Kriedemann, P. E. (1989). Determination of accurate extinction coefficients and simultaneous equations for assaying chlorophylls a and b extracted with four different solvents: verification of the concentration of chlorophyll standards by atomic absorption spectroscopy. Biochim. Biophys. Acta 975, 384–394. doi: 10.1016/s0005-2728(89)80347-0
Roberts, A. G., and Kramer, D. M. (2001). Inhibitor “double occupancy” in the Q(o) pocket of the chloroplast cytochrome b6f complex. Biochemistry 40, 13407–13412. doi: 10.1021/bi015774m
Stepien, P., and Johnson, G. N. (2009). Contrasting responses of photosynthesis to salt stress in the glycophyte Arabidopsis and the halophyte thellungiella: role of the plastid terminal oxidase as an alternative electron sink. Plant Physiol. 149, 1154–1165. doi: 10.1104/pp.108.132407
Tiwari, A., Mamedov, F., Grieco, M., Suorsa, M., Jajoo, A., Styring, S., et al. (2016). Photodamage of iron-sulphur clusters in photosystem I induces non-photochemical energy dissipation. Nat. Plants 2:16035. doi: 10.1038/nplants.2016.35
Trebst, A. (2007). Inhibitors in the functional dissection of the photosynthetic electron transport system. Photosynth Res. 92, 217–224. doi: 10.1007/s11120-007-9213-x
Trebst, A., Wietoska, H., Draber, W., and Knops, H. (1978). The inhibition of photosynthetic electron flow in chloroplasts by the dinitrophenylether of bromo- or iodo-nitrothymol. Z Naturforsch 33c, 919–927. doi: 10.1515/znc-1978-11-1220
Tschörtner, J., Lai, B., and Krömer, J. O. (2019). Biophotovoltaics: green power generation from sunlight and water. Front. Microbiol. 10:866. doi: 10.3389/fmicb.2019.00866
Keywords: DNP-INT, superoxide, P700 spectroscopy, MIMS, photosynthetic electron transfer chain, reactive oxygen species, stable-isotopes
Citation: Fitzpatrick D, Aro E-M and Tiwari A (2020) A Commonly Used Photosynthetic Inhibitor Fails to Block Electron Flow to Photosystem I in Intact Systems. Front. Plant Sci. 11:382. doi: 10.3389/fpls.2020.00382
Received: 14 November 2019; Accepted: 17 March 2020;
Published: 15 April 2020.
Edited by:
Anja Liszkay, Centre National de la Recherche Scientifique (CNRS), FranceReviewed by:
Yuji Suzuki, Tohoku University, JapanToru Hisabori, Tokyo Institute of Technology, Japan
Copyright © 2020 Fitzpatrick, Aro and Tiwari A. This is an open-access article distributed under the terms of the Creative Commons Attribution License (CC BY). The use, distribution or reproduction in other forums is permitted, provided the original author(s) and the copyright owner(s) are credited and that the original publication in this journal is cited, in accordance with accepted academic practice. No use, distribution or reproduction is permitted which does not comply with these terms.
*Correspondence: Arjun Tiwari, YXJqdW4udGl3YXJpQHV0dS5maQ==