- 1Hydro Technology Institute, Tokyo, Japan
- 2Institute for Studies of the Global Environment, Sophia University, Tokyo, Japan
- 3Research and Development Center, Nippon Koei, Tsukuba, Japan
- 4Department of Environmental Science, Saitama University, Saitama, Japan
Egeria densa is an often-found invasive species in Japan, which has spread widely in the past two decades in rivers where no macrophytes had previously been found. As a result, these ecosystems have now become dominated by E. densa. The habitat preference for E. densa colony formation was investigated using the tissue concentrations of hydrogen peroxide (H2O2: a reactive oxygen species) under varying conditions in rivers and laboratory conditions. The empirical equations that can describe the macrophyte tissue H2O2 formation under various velocity and light conditions were produced. The H2O2 concentrations of dark-adapted plants are proportional to the flow velocity, and the surplus H2O2 concentration in the light-exposed condition corresponded to the photosystems produced H2O2. When the H2O2 concentration exceeds 16 μmol/gFW, plant tissue starts to deteriorate, and biomass declines, indicating the critical values required for long-term survival of the plant. The empirically obtained relationships between flow velocity or light intensity and the analysis of H2O2 concentration for different slopes and depths of channels found that the H2O2 value exceeds the critical H2O2 concentration in channels with above 1/100 at around 0.6 m depth. This agrees with the observed results where colonies were not found in channels with slopes exceeding 1/100, and biomass concentration was the largest at depths of 0.6 to 0.8 m. H2O2 concentration is quite applicable to understanding the macrophyte condition in various kinds of macrophyte management.
Introduction
The mid-streams of large Japanese rivers were characterized as gravel beds during the post-World War II era. Fine sediment beds were extremely limited. Thus, the ecosystems of gravel beds, characterized by rich hyporheic flows and biota, such as insect larvae and salmonid fish, were maintained for long periods (Hauer et al., 2016). Except for some emergent species, Phragmites japonica (Asaeda et al., 2009), almost no submerged macrophyte colonies existed in the main streams of major rivers (Kadono, 2004). Since then, dams and weirs have been frequently constructed and most of the waterways have been regulated. Therefore, almost all the gravel particles introduced upstream are trapped before entering the midstream, thus the supply of gravel to the midstream and downstream is completely curtailed. In addition, gravel was mined for use as construction materials from the 1960s to 1970s. The amount of gravel, therefore, substantially reduced in the midstream, compared to that of previous years (Asaeda and Sanjaya, 2017). In contrast, fine sediment inflows continued from the mid to downstream catchments. They were transported and settled, filling interstices on downstream gravel beds. Thus, the midstream beds are now partially covered with fine sediments that bury stones.
In the past two decades, invasive macrophyte Egeria densa began to form colonies in many rivers (Ministry of Lands Infrastructure Transportation and Tourism in Japan [MLIT], 2016). It often covers extensive areas of the channel bed and completely changes the ecosystem there (Collier et al., 1999; Yarrow et al., 2009). Financially, this causes substantial losses to inland fisheries, particularly in the yield of Ayu fish (Plecoglossus altivelis altivelis), a grazer of benthic algae (Asaeda et al., 2018). E. densa was cultivated in aquariums in the early 19th century, but it was disposed into natural freshwater bodies and became naturalized in the 1940s. However, it had not spread into rivers as they were gravelly in those days and were not in a suitable condition to support submerged macrophytes, however, it has been found in some lakes of western Japan since the 1970s (Kadono, 2004). Besides Japan, E. densa, spread widely in other continents (Champion and Tanner, 2000; Santos et al., 2011). It affected stream ecosystems extremely, retarding flow velocity, increasing sedimentation (Collier et al., 1999), and exile of native species (Santos et al., 2011; Gillard et al., 2017). Therefore, though the effects were particularly eminent in Japanese gravel rivers, the invasion of E. densa is a worldwide problem.
As several changes occur simultaneously in natural rivers, it is not easy to elucidate the primary reason that prevented earlier macrophyte colonization or their increase today. The habitat preferences of macrophytes are normally evaluated by monitoring their growth rate or biomass (Barko et al., 1991; Riis et al., 2012; O’Hare et al., 2018). However, there are various potentially influential factors in the natural environment and each factor changes from time to time during the period of the macrophytes’ growth. The existing conditions are, thus, considered to be a result of the integrated environmental conditions experienced previously, and the casual observation that is mainly practiced in vegetation management is not necessarily appropriate for evaluating their habitat preference.
In natural water, macrophytes are subjected to environmental stresses, such as flow velocity, high solar radiation, excessive high or low temperature, etc. In cell organelles, then, reactive oxygen species (ROS) are generated based on the intensity of the stresses, photosynthesis and metabolic activities (Zaman and Asaeda, 2013; Asaeda and Rashid, 2017; Parveen et al., 2017a). A part of these ROS is scavenged relatively quickly by antioxidant activities, and the homogeneity of ROS in tissues is maintained by a balance between the ROS and the antioxidants. However, under excessive stress, this balance collapses as oxidative stress surpasses the antioxidant capacity of the plant. The existence of ROS in plant tissues leads to oxidative stress, and when critical levels are exceeded, the plants tend to deteriorate (Sharma et al., 2012; Choudhury et al., 2017). The most common ROS is hydrogen peroxide (H2O2), which is generated by the superoxide dismutase by in the superoxide (Asada, 2006; Sharma et al., 2012). The H2O2 concentration is relatively stable and can be easily analyzed chemically (Satterfield and Bonnell, 1995; Zhou et al., 2006). The amount of tissue H2O2 concentration, therefore, has potential for use as an indicator to monitor the instantaneous environmental stress intensity on macrophytes (Asaeda et al., 2018).
During the daytime, the total amount of H2O2 generated in plant tissues is, therefore, primarily the sum of the H2O2 generated in response to environmental stress, photosynthesis, and other nonstress metabolic products. This process can be generalized as the following simple equation:
Although there are some interactions between the different environmental stressors and opposing trends in some combinations of stressors (Rivero et al., 2014), the share of H2O2 concentration of stresses is separated from metabolic, respiration, and photosynthesis produced H2O2 (Mittler, 2002). However, the plant oxidative stress is determined by cumulative H2O2 content present in the cells regardless of the source.
Several types of stressors are acting on submerged macrophytes in natural rivers. In the relatively steep non-polluted rivers, the major stressors include the mechanical stress introduced by high current velocity/turbulence, solar radiation, and temperature (Yarrow et al., 2009; Riis et al., 2012). As these are based on different physical quantities, it is difficult to compare the magnitude of each stressor on the submerged macrophytes. However, it is possible to differentiate photosynthesis produced H2O2 from the total accumulated H2O2 by dark adapting the plants (Asaeda et al., 2018). In addition, when other stresses are eliminated under controlled conditions in the laboratory, it is possible to quantify each type of stress by the produced H2O2.
Considering the facts that, it can be hypothesized that (1) there is a relationship between induced H2O2 concentration in E. densa and the intensity of each stress given by the habitat metrics, such as water velocity, temperature, and the solar radiation of the habitat. (2) E. densa growth reduced and is deteriorated in the condition in which H2O2 concentration exceeds a threshold value. (3) The habitat metric condition for E. densa, therefore, remains to be a H2O2 concentration less than the threshold value. Then, the habitat preference and adaptability of E. densa is studied in terms of H2O2 formation under varying riverine conditions and the controlled conditions in the laboratory, focusing on obtaining empirical relationships of factors on the tissue H2O2 contents.
As the tissue presence of H2O2 can be used to evaluate the plant condition, and the plant H2O2 content can be evaluated in a short period, it has the potential to be adopted in macrophyte monitoring practices. In the present study, we focused the H2O2 production of E. densa over various field and laboratory conditions. However, the methodology is widely applicable for various types of macrophyte managements, such as the identification of the optimum condition in the endangered species’ restoration, or in the extermination of alien species.
Materials and Methods
Field Observations
Several rivers that are highly colonized by E. densa were selected from the species distribution records in Japan (Ministry of Lands Infrastructure Transportation and Tourism in Japan [MLIT], 2016). In 2016 and 2017, observations were conducted in rivers for location data of E. densa colonization. Sampling activities were conducted on fine days (days with clear sky and no rain expected) in different seasons from Eno (Go), Saba and Hii Rivers, including their tributaries (Table 1). In each river, the surveys reached approximately 20 to 50 km from the upstream to the downstream area, and five to ten sites where more than one third of the bed was covered with pure E. densa colonies were selected for the study, including the most upstream colony in the main channel.
At each sampling site, there were several E. densa patches, and each patch was composed of several plants. Thus, more than five samples were collected from overlying shoots of different plants of a same patch in light-exposed (under natural conditions) and dark-adapted conditions to differentiate photosynthesis generated H2O2 from environmental factors and metabolism induced H2O2 of tissues. The dark exposure treatment was performed by placing a black plastic sheet (3 m × 3 m) floating over the E. densa colonies for 30 min. The 30 min pre-dark period was determined from laboratory experiments, which were conducted to determine the optimum pre-darkness duration. The plastic sheets were tied to fixed metal poles that were inserted in the riverbed, allowing the sheets to float on the water surface without causing mechanical disturbances to the macrophytes or altering the water flow. The PAR intensity under the sheet was found to be zero. The light-exposed samples were collected adjacent to the darkness treated samples. The collected samples were put in resealable plastic bags and quickly stored in a cool box containing dry ice until they were transferred to the laboratory to be stored at −80°C. Biomass was obtained from a 50 cm × 50 cm quadrant of each sampling point.
At each sampling point, the water velocity was measured with an ultrasonic velocimeter and recorded for more than 1 min (Tokyo Keisoku Co. Ltd., Japan), at 20% (reference velocity) and 80% (depth of the colony) of the total water depth. Turbulence velocity component was calculated as a root mean square deviation from the mean velocity, from the velocity record. Photosynthetically active radiation intensity (PAR intensity) in the water was measured with a portable quantum flux meter (Apogee, MQ-200, United States) at 10 cm depth intervals.
On October 23, 2018, the lateral configuration of a channel was investigated to derive the effect of the E. densa colony on the environment, including the distributions of depth, sand depositions thickness, E. densa biomass, particle size, and the E. densa burying condition in trapped sediments at a point 45 km upstream from the river mouth of the Yahagi River. During the summers of 2016, 2017, and 2018, surveys were conducted from the upstream to the downstream areas in other rivers where the existence of E. densa was recorded, then the locations and the depth of the rivers were recorded and the channel slope was obtained from a topographic map [Geographical Survey Institute of Japan, the (Gsi), 2018].
Laboratory Experiments
The pre-darkness period in the field observation was investigated as follows. The apical cuttings with an average length of 10 cm were obtained from the stock culture and planted in two tanks (50 cm × 35 cm × 35 cm) with thoroughly washed commercial river sand (90% of <0.2 mm particle size; washed using tap water several times until all organic materials are washed away and finally, washed using distilled water to further remove nutrients). In each tank, twenty E. densa cuttings were planted and maintained in a temperature-controlled room maintained at a constant 23 ± 3°C temperature. Each tank was exposed to approximately 100 μmol/m2/s PAR with a 12 h/12 h light and dark period. Nutrients were supplied via a 5% Hoagland nutrient solution. After a two-month acclimatization period, one of the two tanks was covered entirely by a black plastic sheet, providing darkness. E. densa tissues from different samples were collected at 10 min intervals for 2 h and then collected at 6, 12, and 24 h. Light-exposed samples were collected, simultaneously. To avoid the stress of cutting, the tissues were collected from fresh tips during each sampling activity. The experiment was conducted in triplicates with different samples. The H2O2 concentrations of tissues were then analyzed. The H2O2 concentration of E. densa gradually declined with the dark duration, taking the lowest value at 30 min, then slightly increasing later for all cases. Therefore, in the field experiment, 30 min of darkness was adopted for the dark-adapted samples.
The effect of temperature on H2O2 generation was investigated, using four tanks similar to those in the previous experiments. After an acclimatization period of 2 months, the temperature regimes of the tanks were set to 10, 15, 25, and 30°C, respectively, using an aquarium water temperature controlling system (Aquarium cooler ZC-100α, Zensui Corporation, Japan). Light intensities, 220, 320 and 680 μmol/m2/s were obtained with the combination of several LED lamps. Then, a 5 m long flume equipped with a straightening plate at the upstream end was used to check the velocity effect. The central part was lightened with 200 μmol/m2/s of PAR intensity by a LED lamp. The velocity was adjusted at 23 cm/s to maintain the low turbulence intensity condition, less than 2 cm/s of turbulence velocity, following Asaeda et al. (2018), and the normal flow velocity of the E. densa habitat (Champion and Tanner, 2000). The temperature conditions were maintained for 7 days, and plants were sampled for the chemical analyses. The experiment was conducted in triplicates with different samples for each condition.
Chemical and Biomass Analyses
The tissue H2O2 was estimated colorimetrically using spectrophotometry (Asaeda et al., 2018). Plant chemicals were extracted into ice-cold phosphate buffers (50 μmol/L, pH 6.0) by crushing approximately 100 mg of the plant in the presence of polyvinylpyrrolidone (PVP). The extractions were centrifuged at 5000 g for 15 min at 4°C. The enzyme extraction of 750 μL was then mixed with 2.5 mL of 0.1% titanium sulfate in 20% (v/v) H2SO4, and the mixture was centrifuged at 2500 g for 15 min at 20°C. The optical absorption at a wavelength of 410 nm was measured using spectrophotometry (UV-1200, UV-Visible Spectrophotometer, Shimadzu, Japan), and the H2O2 concentrations (μmol/gFW) were estimated using a standard curve.
The dry weight of biomass was estimated by oven drying the collected biomass samples at 70°C for 72 h or until the weight became stable. The dried biomass was weighed and expressed in units of gDW/m2.
Statistical Comparison
The linear or power law (for solar radiation intensity) correlation between parameters was tested by Pearson’s correlation analysis and the statistical significance between observations were tested with Student’s t-test. The statistical comparisons of field data were performed to obtain the relationship between the H2O2 content and the external factors (such as velocity, turbulence velocity, light intensity, biomass, and depth), and the relationship between the factors (turbulence and mean flow). For H2O2 concentration and velocity or temperature relationship, statistical comparisons were performed for the different study sites and/or sampling time groups, which have different temperatures and solar radiations, to obtain the interaction between stresses and the H2O2 concentration. The gradient of the regression line was obtained for the whole set of data in the relation between each stress component and H2O2 concentration. Then, for each study site and sampling time groups, statistical analysis was conducted to check the significance of the regression. For the relationship between H2O2 concentration and light intensity, the power law regression of the excessive H2O2 concentration of the light exposed samples and dark-adapted samples was conducted to obtain the light intensity at zero H2O2 concentration. Then the data scattering was compared with the standard deviation.
All the statistical tests were performed using IBM SPSS Statistics Version 25. The regression lines and equations were obtained using the inbuilt regression function feature of Microsoft Excel 2016.
Results
The relationship between H2O2 concentration and water temperature, obtained from both laboratory experiments (PARs were 220, 320, and 680 μmol/m2/s with 0 cm/s of velocity; 200 μmol/m2/s of PAR and 23 cm/s of flow velocity), and dark-adapted condition of the field observation (PAR = 0 μmol/m2/s) is shown in the Figure 1. The recorded temperatures of field studies ranged between 10 and 25°C depending on the sampling seasons and rivers, and the fluctuations were observed to be 1–2°C in the same sampling condition groups. Thus, the extrapolated regressed lines of each group to zero velocity were used here (Figure 1). H2O2 concentration has a negative correlation with temperature in the 10 to 30oC temperature range and was regressed to lines with a gradient of −0.316 μmol/gFW/degree, for all light intensity groups. The regression equations are shown in Figure 1 compared to observed data (R = 0.982, P < 0.01 for 220 μmol/m2/s PAR; R = 0.963, P < 0.01 for field observation, 0 μmol/m2/s PAR). There was no overlapping among data from different groups. Thus, the effect of interaction between temperature and light intensity is sufficiently small.
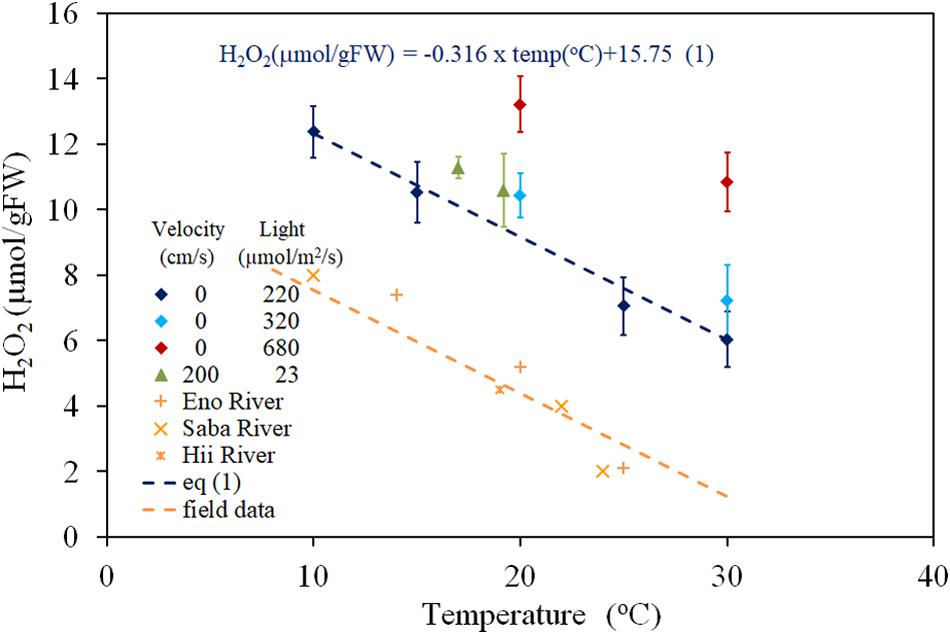
Figure 1. The concentration of H2O2 in E. densa tissue as a function of water temperature. The error bars represent the standard deviation (n = 3). Eno, Hii, and Saba Rivers data are values extrapolated to zero velocity of the regression line with –0.316 (μmol/gFW cm/s) gradient, shown by dashed lines in Figure 2.
Figure 2 presents the H2O2 contents of the field observation samples with respect to turbulence velocity. Under light exposure, the H2O2 contents are always higher than those in the corresponding dark-adapted samples by 5–10 μmol/gFW. However, the scattering was greater than that in the dark-adapted samples. Dark-adapted samples are composed of different temperature groups, which depend on the sampling time and rivers.
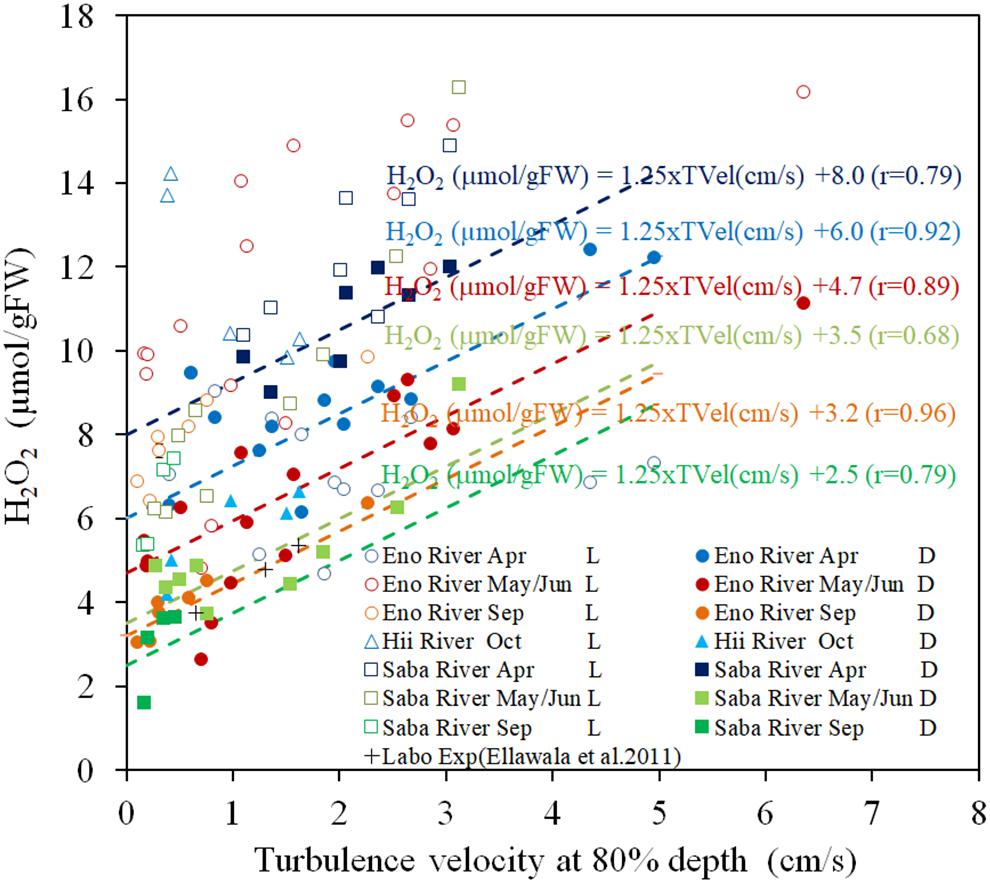
Figure 2. The concentration of H2O2 in E. densa tissue as a function of turbulence velocity at 80% depth in light-exposed (L) and dark-adapted (D) conditions. The “Labo Exp” represents data from the laboratory experiment (Ellawala et al., 2011). The dashed lines indicate the linear regressed lines with the gradient of 1.25 μmol/[gFW (cm/s)] of the dark-adapted conditions of the same colored symbols of as those of the sampling rivers and seasons, respectively. H2O2 is given in units of μmol/gFW, and while the TVel (turbulence velocity) are in cm/s. The dates May, Sep (September), and Oct (October) represent data obtained in 2016 while Jun (June) and Apr (April) represent data of 2017. Eno, Saba, and Hii represent Eno, Saba, and Hii rivers including their tributaries, respectively.
The H2O2 contents of dark-adapted samples were highly correlated with the turbulence velocity, with a gradient of 1.25 μmol/[gFW (cm/s)] (R = 0.796, P < 0.01). The regression lines with the same gradient are shown in Figure 2 for the dark-adapted samples of different sampling time and river groups, compared to observed data. For each group, the H2O2 contents were highly regressed to the lines (R = 0.917, 0.885, 0.964 for April, May/June and September sampling at the Eno River, respectively, 0.76 for the Hii River, and 0.84, 0.68, and 0.63, respectively, for the sampling during each season, respectively, at the Saba River for all P < 0.01). This finding indicates that the H2O2 concentration dependence on turbulence velocity is independent of temperature.
There is a significant positive correlation between the mean flow and turbulence velocity (R = 0.722, P < 0.01), as shown in Figure 3. Also, the correlation between the H2O2 content of dark-adapted samples with the mean velocity is also positive and significant (Figure 4, R = 0.571, P < 0.01). The relationships can be explained with linear regression equations [Eq. (2) for the 0–60 cm/s mean flow velocity range]. The line in Figure 4 included the cases in which the turbulence intensity was particularly high because of the large gravel beds. Although these values provide slightly higher H2O2 concentrations compared to those at normal sites, the relatively proportional relationship with turbulence velocity indicates that the mean flow velocity is available as a reference of the mechanical stress due to flow velocity (Asaeda et al., 2017).
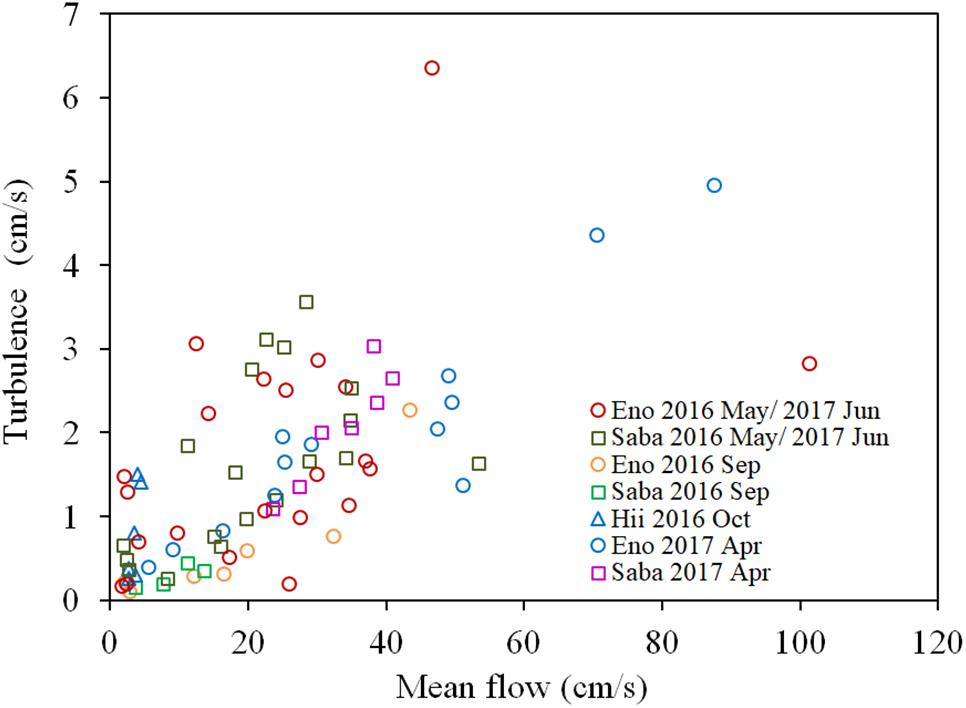
Figure 3. Relationship between the turbulence velocity and the mean flow velocity of each sampling site of different rivers. The sample collected month is represented by the month indicated in the legend. Eno, Saba, and Hii represent Eno, Saba, and Hii rivers, including their tributaries, respectively.
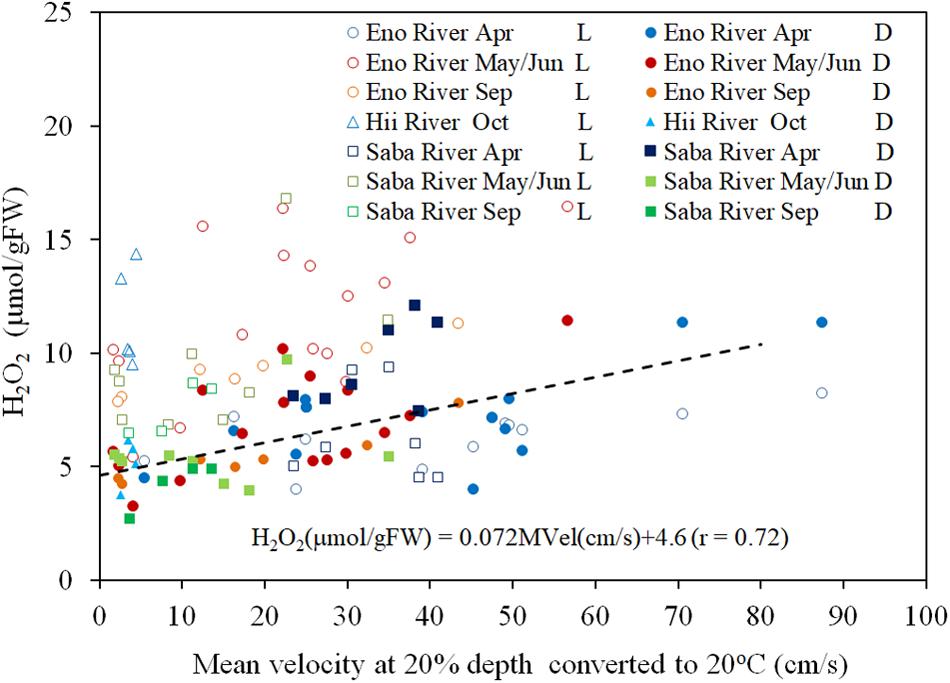
Figure 4. The concentration of H2O2, converted to 20°C, in E. densa tissue as a function of the mean velocity at 20% deep thin light-exposed (L) and dark-adapted (D) conditions. The dashed line indicates the linear trend of the dark-adapted condition. The dates May, Sep (September), and Oct (October) represent data obtained in 2016 while Jun (June), Apr (April) represent data of 2017. Eno, Saba, and Hii represent Eno, Saba, and Hii rivers including their tributaries, respectively. H2O2 is given in units of μmol/gFW, and the MVel (mean velocity) is in cm/s.
The H2O2 concentration of the light-exposed samples fluctuated heavily, but always exceeded the value of the corresponding dark-adapted samples. The excessive H2O2 content is light induced H2O2 content, postulated as a function of light intensity in Figure 5. There is a positive correlation between the light intensity and the H2O2 concentration, however, the increasing rate of the excessive H2O2 decreases with increasing light intensity. When the light intensity is lower than 40 μmol/m2/s, the excessive H2O2 was nearly 0, similar to experimental results obtained by Hussner et al. (2010) and Rodrigues and Thomaz (2010). Therefore, power low regression analyses were conducted for the excessive H2O2 concentration with respect to the surplus light intensity from 40 μmol/m2/s, as Eq. (3) in the figure (R = 0.738, P < 0.05). Although, the scattering is large, most of the data are distributed within the standard deviation from the Eq. (3) (1.57 μmol/gFW) without any systematic deviation regardless of rivers and sampling seasons, where the solar radiation intensity ranged from 40 to 600 μmol/m2/s, and temperature from 10 to 25oC.
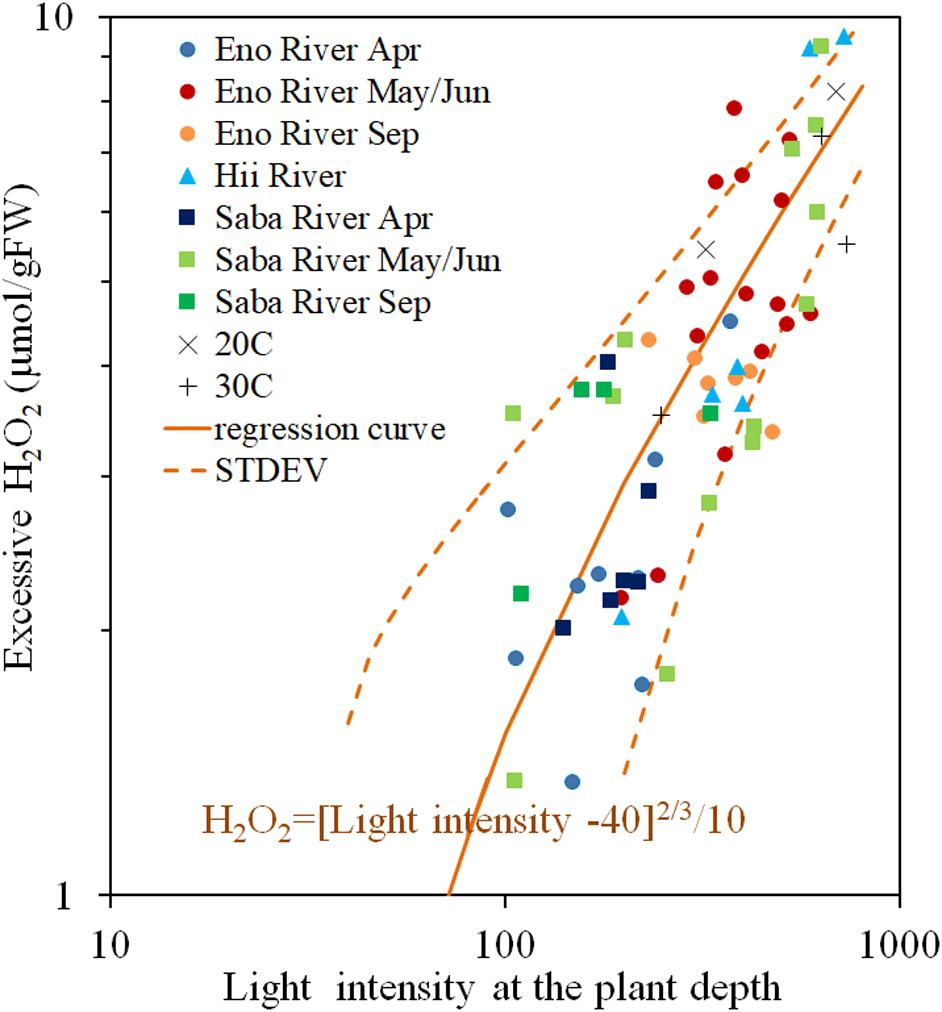
Figure 5. Photosynthesis-produced H2O2 concentration (Excessive H2O2) of E. densa tissue as a function of light intensity. The sample collected month is represented by the year and the month indicated in the legend. Eno, Saba, and Hii represent Eno, Saba, and Hii Rivers, including their tributaries, respectively. When the light intensity is lower than 40 μmol/m2/s, the excessive H2O2 concentration was 0 μmol/gFW. The regression curve in the excessive H2O2 concentration and the excessive light intensity from 40 μmol/m2/s condition is postulated by a rigid line, and the standard deviation is shown by the dashed line. “Light” is the light intensity at the sample depth (μmol/m2/s), and the excessive H2O2 concentration, H2O2rad (Temp), is expressed in μmol/gFW. The “H2O2rad” denotes the light induced H2O2 content. The Exp 20oC and Exp 30oC represent the excessive H2O2 concentration quantified in experiments under 20 and 30°C controlled temperatures.
Diurnal H2O2 Variation of E. densa
In the diurnal observation, the light intensity during the 2018 sampling was higher for the 2018 observation day (∼1500 μmol/m2/s at the shoot height) compared to that for the 2017 observation day (<1000 μmol/m2/s at the shoot height). This high solar radiation intensity condition continued for several weeks before the 2018 observation day, while rainy or cloudy conditions had persisted for several weeks before the 2017 observation day. Therefore, samples from the 2018 observation were exposed to high solar radiation for several weeks, as opposed to samples from the 2017 observation (Figure 6A).
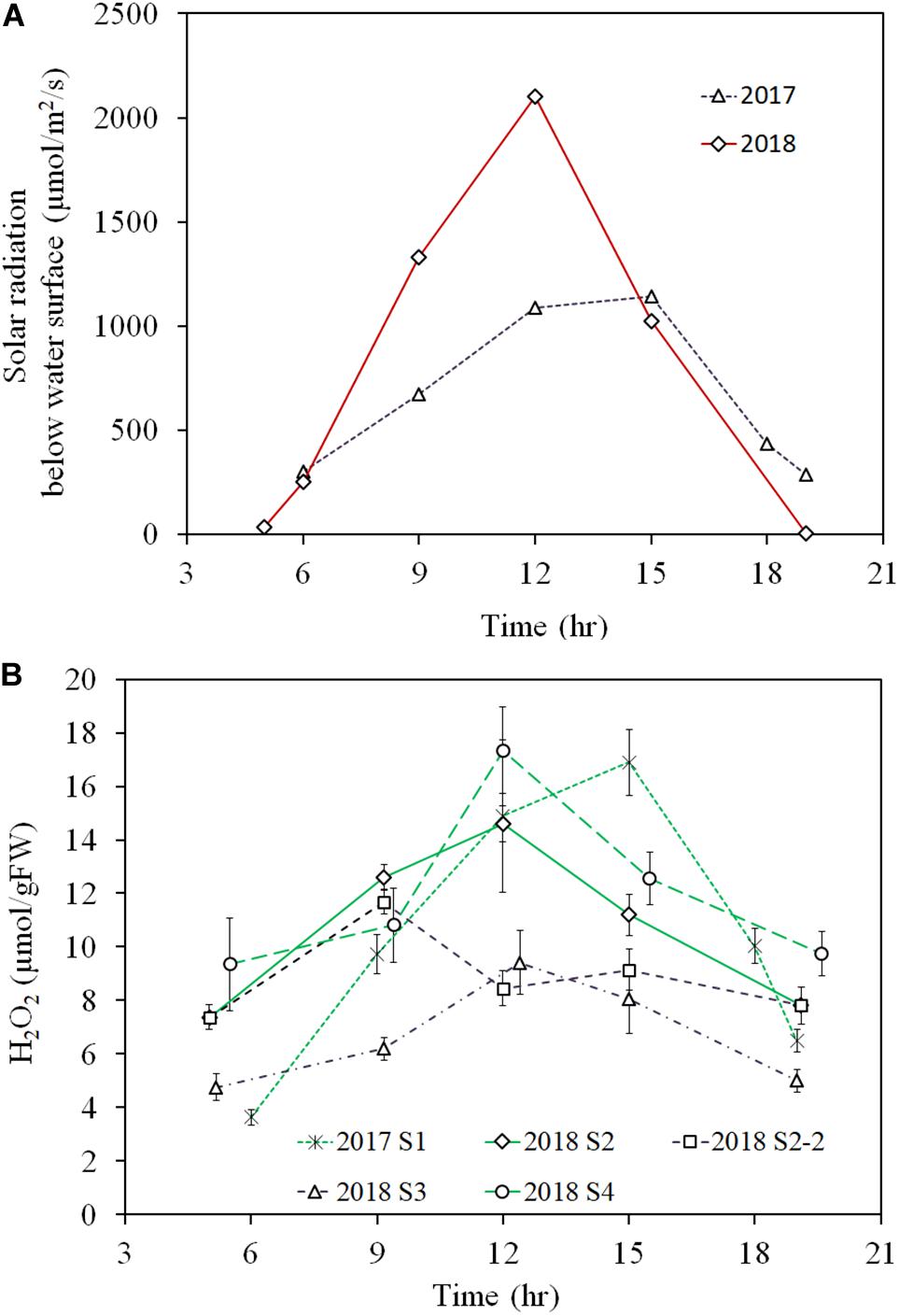
Figure 6. Diurnal variation of light intensity just below the water surface of 2017 and 2018 observations (A) and E. densa tissue H2O2 concentration (B), observed in the years 2017 (moderate radiation), and 2018 (excessive radiation). 2017 S1 represent the healthy shoots of 2017 observation. 2018 S2-2 and 2018 S3 represent degraded shoots under high solar radiation exposure at shallow water (<10 cm deep) in 2018, and 2018 S2 and 2018 S4 represent healthy shoots exposed to moderate radiation in deep depth sites (S2 30 cm deep and S4 50 cm deep) of the observation. The error bars represent the standard deviation.
The diurnal variation of tissue H2O2 concentration followed the diurnal solar radiation intensity in 2017 and for healthy samples at 2018 S2 and S4 in 2018 (Figure 6B). Then, during the day, the H2O2 concentration rose up to nearly 16 μmol/gFW by noon and then, declined in the afternoon with the decline in solar radiation. In contrast, the values were substantially lower for the degraded samples in the 2018 observation. Then, samples at 2018 S2-2 and 2018 S3, showed less than 10 μmol/gFW of H2O2 concentration around noon. E. densa colonies remained healthy in the 2017 observation, while in the 2018 observation, shoots close to the water surface were degraded and appeared to be starting to die at S2-2 and S3.
As per Figures 7A,B, the H2O2 content for both the light-exposed and dark-adapted samples were negatively correlated with the biomass (R = −0.474, P < 0.01 for light-exposed and R = −0.504, P < 0.01 for dark-adapted). It was observed that there were no samples with an H2O2 content exceeding the 16 μmol/gFW range. The approximate channel slope of the river in which large colonies of E. densa were formed in the running water was between 1/120 and 1/1800. No E. densa colony was found in the further upstream reaches, with channels steeper than 1/100, unless weirs were constructed to regulate the water flow (Table 1). The stress on plants generated by the flow velocity is more intensified at deeper sites; however, a high biomass concentration was found in deeper zones in the channel rather than in the shallow zones. The biomass of the colonies was highly correlated with the depth of the water column, peaking at a depth of 80 cm, and gradually declining as the depth increased further (Figure 8). The biomass distribution exhibited a common trend irrespective of the river or the observation site.
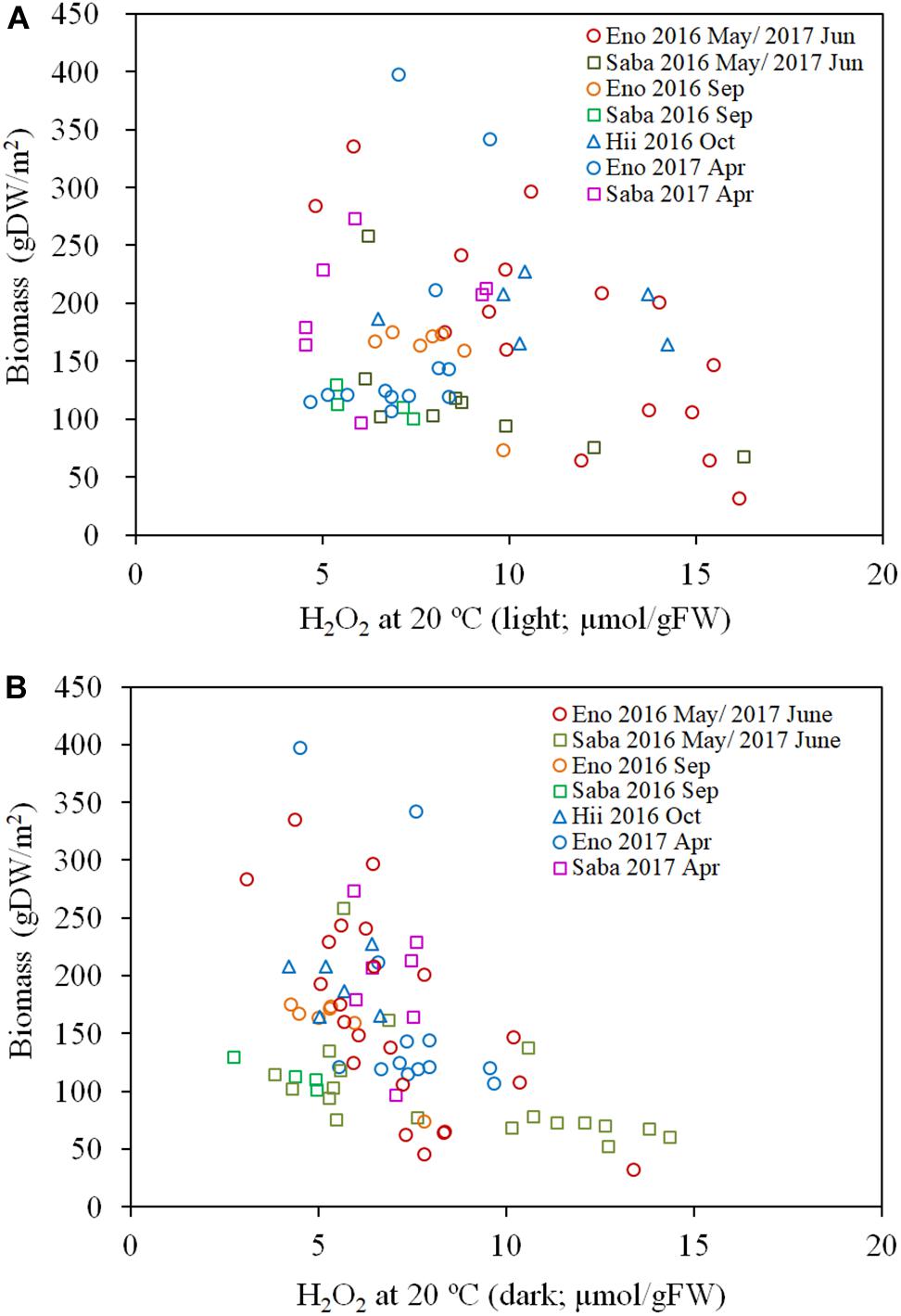
Figure 7. Biomass of E. densa as a function of light exposed H2O2 concentration (A) and dark-adapted H2O2 concentration (B). The H2O2 concentration was corrected to 20°C. The sample collected month is represented by the year and the month indicated in the legend. Eno, Saba, and Hii represent Eno, Saba, and Hii rivers, including their tributaries, respectively.
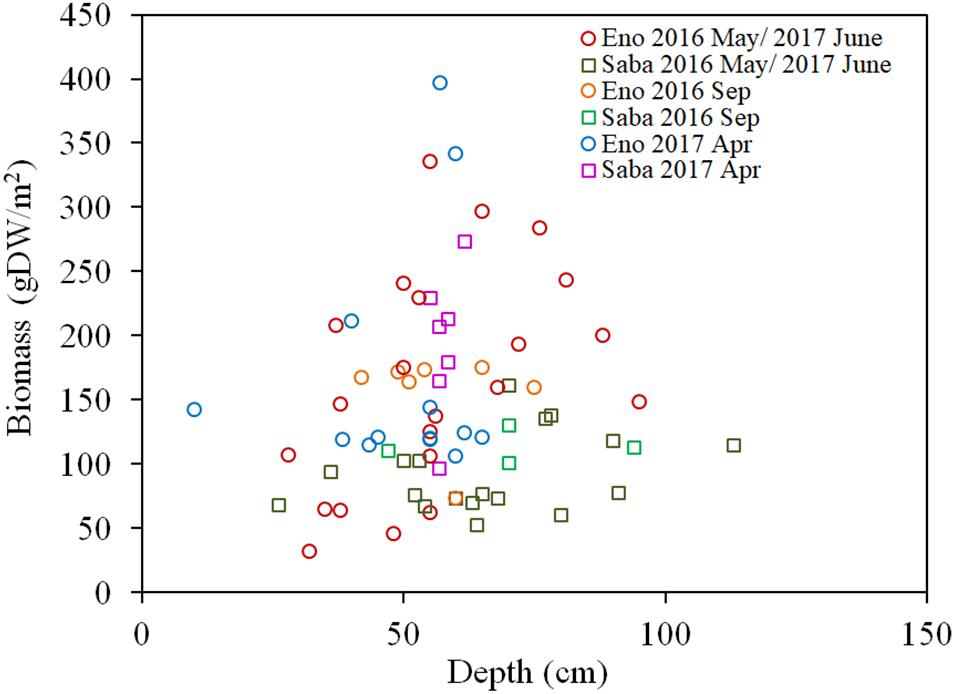
Figure 8. Biomass of E. densa as a function of water depth. The sample collected month is represented by the month indicated in the legend. Eno and Saba represent Eno and Saba rivers, including tributaries, respectively.
Discussion
Environmental Stressors on E. densa
There are several types of stressors acting on submerged macrophytes in natural rivers. As these are based on different physical quantities, it is difficult to compare the magnitude of the effect of each stressor on the submerged macrophytes. The difference in H2O2 concentrations between the continuous light-exposed and 30 min pre-shaded samples differentiate the stress-induced H2O2 from photosynthesis generated H2O2. Based on the outcome of the laboratory experiment, we developed a relationship between temperature and the H2O2 generation in plant tissues. When the fractions of H2O2 corresponding to photosynthesis and temperature effects were eliminated from the continuous light-exposed samples of field studies, the observed trend of H2O2 was similar to the result of the oscillating grid laboratory experiment, in which the amount of H2O2 in macrophyte tissues was proportional to the root mean square velocity of the turbulence as is shown in Figure 2 (Asaeda et al., 2017).
Under the zero-turbulence velocity, the tissue H2O2 concentration corresponds to a combination of photosynthesis, metabolic activities, and environmental stresses. The difference between light and dark treatment experiments distinguishes the amount of H2O2 generated by photosynthesis from the remaining stressors. Further, the variation in solar radiation intensity during the day is reflected in the H2O2 concentrations, which varied according to the light intensity from approximately 2 to 10 μmol/gFW (Figure 5). There were parallel relationships between the H2O2 concentration and the temperature for different light intensity groups with the same velocity (Figure 1), which explains the independency of H2O2-temperature relationship trend from the light intensity but simultaneously elevates the trend due to excessive H2O2 production (Figure 5). The temperature-H2O2 dependency linear relationship provides the temperature generated H2O2 concentration, which is reportedly around ∼10 μmol/gFW in the E. densa habitat temperature range of 10–35oC, in the present observation and previous reports (Hanamoto and Ikushima, 1988.; Yarrow et al., 2009; Gillard et al., 2017).
H2O2 concentration of dark-adapted samples had linearly increasing trends with respect to turbulence velocity, regardless of different sampling months and river groups, which differentiate the temperatures (Figure 2). The H2O2 concentration extrapolated to zero velocity had the same relationship with the temperature dependency on the H2O2 concentration (Figure 1). Thus, the increasing rate of the H2O2 concentration with respect to turbulence effects indicates the turbulence induced H2O2.
These H2O2-solar radiation and H2O2-temperature relationships exhibit almost the same trends for photosynthetic rates obtained by the outdoor experiments with different temperatures and light intensities (Riis et al., 2012). Therefore, the H2O2 relationship with the light intensity and temperature conditions, can be considered as common trends for E. densa. In the practical application, H2O2 concentration can be applicable to determine the different types of stressors in the same manner and to compare their relative magnitudes.
Compared to the H2O2 induced by metabolic activities, which is ∼4 μmol/gFW given in the field experiments of dark-adapted samples at zero velocity, in the relatively steep non-polluted rivers, the major stressors include the mechanical stress introduced by high current velocity/turbulence, solar radiation, and temperature. The river water quality is relatively good, and there is no salinity in the midstream of Japanese rivers. However, in eutrophic water, organic matter accumulates at the bottom of stagnant zones, which creates an anoxic zone in the sediment layer; however, the bottom sediment anoxia contributed only ∼5 μmol/gFW of H2O2 (Parveen et al., 2017a). As for the biotic stress, toxic strains of cyanobacteria, Microcystis, for instance, generate only 1.5 μmol/gFW of H2O2 (Amorin et al., 2017). Therefore, the amount of H2O2 generated by photosynthesis, temperature and flow velocity is relatively large compared to other stresses, and these stresses are considered as major stresses, which control its colonization.
The contribution of each stress to the total H2O2 of the plant can be distinguished with this method and can be adapted to determine the total level of environmental stress on macrophytes. The combination of different stresses sometimes imposes two opposing demands on the plant (Choudhury et al., 2017) or interact each other. However, the parallel relationship among the major stresses in natural rivers, namely, solar radiation, flow velocity, and water temperature and the interactive effects seems to be sufficiently small. The reason for the relatively lower interactive effects among stressors is not clear.
H2O2 is generated by the surplus number of electrons. In the photosynthesis process, the surplus amount of electrons are generated on the thylakoid membrane by strong energy (Asada, 2006), while the consumption of electrons is decreased under low temperature due to the suppressed CO2 fixation by the inactivation of Rubisco in Calvin cycle (Nishiyama and Murata, 2014), or the mechanical damage of organelles in turbulent flow (Atapaththu et al., 2015). Therefore, the sites that can cause the electron surplus is different between stresses.
Threshold Condition of E. densa Mortality
Egeria densa colonies of 2017 observation remained healthy, while in the 2018 observation, shoots close to the water surface were degraded and appeared to be starting to die. The intensive oxidative stress caused by high solar radiation for several days should be the reason for the degradation of 2018 colonies. The results indicate the depression of plant metabolism owing to solar radiation exceeding the tolerable levels. When the H2O2 concentration became higher than 16 μmol/gFW in hypoxia and hydrogen sulfate exposure experiments for E. densa, plants deteriorated, and the total chlorophyll concentration and the H2O2 concentration substantially declined compared to other samples (Parveen et al., 2017a,b,c). Also, with the exposure of Fe, E. densa exhibited lowest growth rate, chlorophyll content and photosystem efficiency at around 16 μmol/gFW of H2O2 content and beyond the level, healthy plants did not exist (unpublished data). Same as the other observations, the 2018 observations show the H2O2 level of colonies peaked beyond the 16 μmol/gFW during the daytime. Therefore, the H2O2 concentration of 16 μmol/gFW can be considered as a critical value for the survival of E. densa, regardless of the types of stressors. Exceeding the threshold level, would lead to the deterioration of plants due to oxidative damage.
Empirical Expression of Habitat Preference and Colonizable Conditions Simulation
The H2O2 concentration of macrophytes can be used to explain the expected macrophyte distribution in a river. When Eq. (4) is considered, the H2O2 generated by each stressor can be expressed as follows:
The total H2O2 concentration (H2O2tot) at a particular temperature (Temp) is given by:
where H2O2rad is the H2O2 generated by solar radiation exposure, H2O2vel is the H2O2 generated by the flow velocity, and H2O2met is the H2O2 generated by metabolism.
The light intensity at a particular depth of water, z, can be calculated using Eq. (5) (Middelboe and Markager, 1997):
where k is the attenuation coefficient in water, which was 0.035 (/cm) in the observed rivers. The solar-radiation-induced H2O2 content has an increasing relationship with the light intensity, given by Eq. (3). Considering Eq. (3), the H2O2 concentration generated by the solar radiation under a particular temperature (within the temperature range 10–30°C), therefore, can be expressed as Eq. (6):
Direct mechanical stress is generated by turbulence rather than the mean flow (Atapaththu et al., 2015; Asaeda and Rashid, 2017; Asaeda et al., 2017); however, there is a close relationship between these two quantities, particularly in straight uniform channels with uniform roughness. It is assumed that the mean velocity U (m/s) under the uniform flow is empirically given by Manning’s law [Eq. (7)]:
where R is the hydraulic radius, approximately given by the depth “H” (m), the channel bed slope “S,” and Manning’s roughness coefficient “n.” The effects of longitudinal configuration, vegetation, etc., are added in “n.” Therefore, considering Eq. (2), the H2O2 accumulation due to velocity stress and metabolism at a particular temperature (within the temperature range 10–30°C) can be expressed as follows (Eq. 8):
Subsequently, the total H2O2 concentration within the temperature range 10–30°C can be given as follows (Eq. 9):
for I0 exp (-kz) ≥ 10 μmol/m2/s;
for I0 exp (-kz) < 10 μmol/m2/s.
Figure 9 shows the simulated H2O2 concentration generated by using Eq. (9), for different channel slopes and depths. I0 is assumed to be 2000 μmol/m2/s, as the highest solar radiation experienced in the observation area on a fine day during summer and when the canopy top was assumed to be located at the 80% depth, which is the average canopy height of the observed E. densa colonies. Manning’s roughness coefficient for the channels is approximated at 0.08. Channel slope was obtained from the topographic map (ArcGIS, 2019, Esri, New York Street, Redlands, California). The target area was characterized by steep basins, and the difference in elevation between the channel bed and the riparian zone is nearly constant thus the riverbed gradient is nearly the same as that of the riparian zone. The simulated results of Figure 9 are consistent with the observed data of the rivers. The H2O2 concentration generally declines with depth, due to declining light intensity. In the steep channel, the H2O2 concentration increases again with a further increase in depth as the flow velocity rises. On gentler slopes, due to the lower flow velocity, H2O2 concentration is lower, and there is a wider range of depths in which E. densa colonies potentially form. However, the shallow depths become unsuitable for colonization of E. densa due to the higher light intensity.
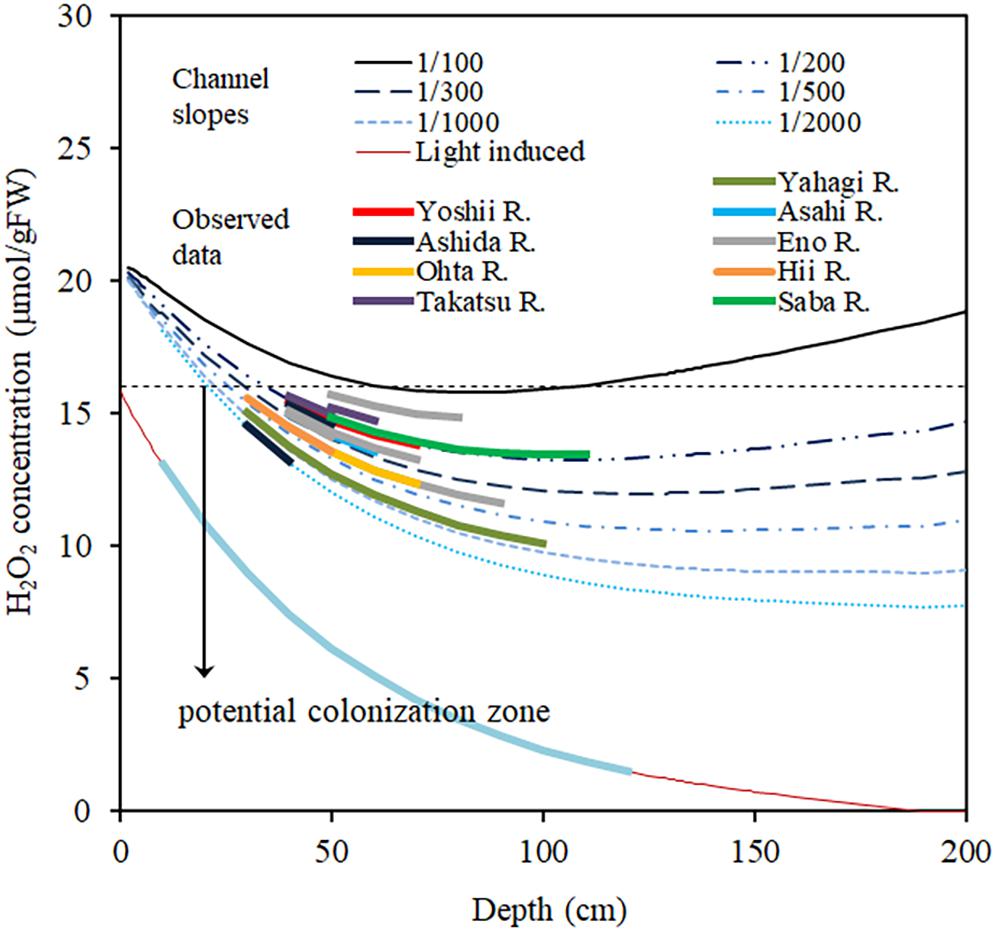
Figure 9. Simulated H2O2 concentration in the plant body of E. densa changes with the water column depth for different channel slopes and actual slope data of several Japanese rivers. The line “Light-induce” represents the H2O2 concentration induced by light and change with the depth. The downward arrow “potential colonization zone” represents the depth zone of different channel slopes suitable for E. densa colonization.
E. densa Colonization in Rivers
In rivers steeper than 1/100, the upstream to midstream areas are originally filled with gravel or boulders, and fine sediment beds are rare as they are easily flushed away during flooding. The flow velocity depends on the channel slope, depth, and roughness. Roughness is determined by the bed sediment size as well as other factors related to the channel configuration, such as longitudinal morphology and bars (Parker and Peterson, 1980). The formation of E. densa colonies poses a physical disadvantage due to the high flow velocity and high light intensity as well as the high turbulence caused by the gravel bed as long as gravel sediments are supplied. However, when the gravel supply is decreased, the gravel-to-sand transition is extended further upstream (Singer, 2008), and the sand bed area increases in the former gravel zones. Sand is transported mostly as a suspended sediment load along the channel. E. densa communities accumulate suspended sediments efficiently, as they have a complex, dense stem structure that is widely distributed over the bed with thicker stems and denser whorls compared to similar native species, Hydrilla verticillata, and common native submerged species, Elodea sp., Myriophyllum spicatum, and Potamogeton crisps (Sand-Jensen, 1998; Vermaat, et al. 2000; Statzner et al., 2006). At the same time, water intake weirs have been frequently constructed along rivers in the last 30 years (personal communication). Then, stagnant water was produced in the upstream zone. Thick E. densa colonies were developed in the upstream area of many weirs in the observation (data are not shown). With the E. densa dispersal ability via fragmentation, it spread increasingly into the downstream (Casati et al., 2000, 2002; Redekop et al., 2016). Then, the dense shoot morphology leads to the formation of many sandy patches in the former complete gravel beds of the downstream. When fine sediments are supplied, it is easier to fix roots and take nutrients from the ground than from the stony bed (Barko et al., 1991). Buried by a sediment layer, the E. densa complex shoots reinforce the sand layer, which is otherwise easily washed away by floods. Once the E. densa colonies develop, the sandy spaces increase widely on the gravel bed, accommodating more macrophytes, including other species. Subsequently, the ecosystem changes to a macrophyte-dominated ecosystem. A similar phenomenon was observed, after the occurrence of a large flood in the Yahagi river (in July 2017), sandy sediments accumulated in colonies burying E. densa up to 20 to 30 cm on a bed that was originally gravelly (Supplementary Figure 1). The E. densa biomass was relatively dense, up to 150–400 gDW/m2, in these sites, as the accumulated sand layer was reinforced with E. densa stem structures. This layer was thus rigid and could not be easily flushed away during moderate floods (Supplementary Figure 2). In contrast to the original gravel bed, the sandy surface was smooth, and turbulence generation was also reduced. Further, in field observations, an accumulation of fine sediments was always found inside the E. densa colonies of all rivers.
Conclusion
The high concentrations of H2O2 introduced by high flow velocity and high solar radiation in summer inhibited the formation of large colonies in the gravel channel, owing to the high oxidative stress. The accumulation of H2O2 in E. densa showed a significant relationship for both flow velocity and solar radiation. The critical H2O2 concentration to maintain a healthy population of E. densa can be considered as 16 μmol/gFW, which corresponds to the termination of biomass accumulation. Under the strongest solar radiation on summer days, the H2O2 level often exceeds the critical condition, leading to the deterioration of E. densa and ultimately, the H2O2 concentrations decline as the plant tissues start to deteriorate. H2O2 concentrations of E. densa were estimated for channels with different slopes and different depths. The H2O2 concentration is higher than the critical value in shallow water and increases in steeper channels, exceeding the critical value at a channel slope larger than 1/100. Once colonized, E. densa accumulates sandy suspended sediment efficiently and creates a preferable environment for further colonization. The present methodology can be applied to predict the area that can be conveniently colonized by E. densa within a short time period, which has been determined based on a prolonged monitoring activity.
Data Availability Statement
All datasets generated for this study are included in the article/Supplementary Material.
Author Contributions
TA conceived the study. TA and LV performed the experiments. TA and MS analyzed and interpreted the data, and wrote the manuscript. All authors reviewed the final version of the manuscript.
Funding
This study was financially supported by a grant-in-aid from the Japan Society for the Promotion of Science, Scientific Research grant numbers (15H04045 and 19H02245), development grant from River Works Technology Research and Development Program from the Ministry of Land, Infrastructure, Transportation and Tourism, Japan, the River Fund from the River Foundation of Japan, and the Watershed Ecology Research Group organized by Water Resources Environment Center.
Conflict of Interest
The authors declare that the research was conducted in the absence of any commercial or financial relationships that could be construed as a potential conflict of interest.
Acknowledgments
We would like to express our deepest appreciation to the sampling team, comprising Dr. Kelum Sanjaya, Dr. Chandani Chalanika, Dr. Keerthi Atapaththu, Dr. Abner Barnuevo, Ms. Gulgina Muhtar, Ms. Chihiro Takeuchi, and Ms. Liping Xia, for their efforts.
Supplementary Material
The Supplementary Material for this article can be found online at: https://www.frontiersin.org/articles/10.3389/fpls.2020.00422/full#supplementary-material
References
Amorin, C. A., Ulisses, C., and Moura, A. N. (2017). Biometric and physiological responses of Egeria densa Planch. Cultivated with toxic and non-toxic strains of Microcystis. Aquat. Toxicol. 191, 201–208. doi: 10.1016/j.aquatox.2017.08.012
Asada, K. (2006). Production and scavenging of reactive oxygen species in chloroplasts and their functions. Plant Physiol. 141, 391–396. doi: 10.1104/pp.106.082040
Asaeda, T., and Rashid, M. (2017). Effects of turbulence motion on the growth and physiology of aquatic plants. Limnologica 62, 181–187. doi: 10.1016/j.limno.2016.02.006
Asaeda, T., and Sanjaya, K. (2017). The effect of the shortage of gravel sediment in midstream river channels on riparian vegetation cover. River Res. Appl. 33, 1107–1118. doi: 10.1002/rra.3166
Asaeda, T., Sanjaya, K., and Kaneko, Y. (2017). Effects of mechanical stressors caused by mean flow and turbulence on aquatic plants with different morphologies. Ecohydrology 10:e1873. doi: 10.1002/eco.1873
Asaeda, T., Senavirathna, M. D. H. J., Xia, L., and Barnuevo, B. (2018). Application of hydrogen peroxide as an environmental stress indicator for vegetation management. Engineering 4, 610–616. doi: 10.1016/j.eng.2018.09.001
Asaeda, T., Siong, K., Kawashima, T., and Sakamoto, K. (2009). Growth of Phragmites japonica on a sandbar of regulated river: morphological adaptation of the plant to low water and nutrient availability in the substrate. River Res. Appl. 25, 874–891. doi: 10.1002/rra.1191
Atapaththu, K., and Asaeda, T. (2015). Growth and stress responses of Nuttall’s waterweed Elodea nuttallii (Planch) St. John to water movements. Hydrobiologia 747, 217–233. doi: 10.1007/s10750-014-2141-9
Atapaththu, K., Miyagi, A., Atsuzawa, K., Kaneko, Y., Kawai-Yamada, M., and Asaeda, T. (2015). Effects of water turbulence on variations in cell ultrastructure and metabolism of amino acids in the submersed macrophyte, Elodea nuttallii (Planch.) H. St. John. Plant Biol. 17, 997–1004. doi: 10.1111/plb.12346
Barko, J. W., Gunnison, D., and Carpenter, S. R. (1991). Sediment interactions with submerged macrophyte growth and community dynamics. Aquat. Bot. 41, 41–65. doi: 10.1016/0304-3770(91)90038-7
Casati, P., Lara, M. V., and Andreo, C. S. (2000). Induction of a C4-like mechanism of CO2 fixation in Egeria densa, a submersed aquatic species. Plant Physiol. 123, 1611–1622. doi: 10.1104/pp.123.4.1611
Casati, P., Lara, M. V., and Andreo, C. S. (2002). Regulation of enzymes involved in C4 photosynthesis and the antioxidant metabolism by UV-B radiation in Egeria densa, a submersed aquatic species. Photosynth. Res. 71, 251–264.
Champion, P. D., and Tanner, C. C. (2000). Seasonality of macrophytes and interaction with flow in a New Zealand lowland stream. Hydrobiologia 441, 1–12.
Choudhury, F. K., Rivero, R. M., Blumwald, E., and Mittler, R. (2017). Reactive oxygen species, abiotic stress and stress combination. Plant J. 90, 856–867. doi: 10.1111/tpj.13299
Collier, K. J., Champion, P. D., and Croker, G. F. (1999). Patch- and reach-scale dynamics of a macrophyte-invertebrate system in a New Zealand lowland stream. Hydorobiologia 392, 89–97.
De Silva, H. C. C., and Asaeda, T. (2018). Stress response and tolerance of the submerged macrophyte Elodea nuttallii(Planch) St. John to heat stress: a comparative study of shock heat stress and gradual heat stress. J. Plant Biosyst.- Int. J. Dealing Asp. Plant Biol. 152, 787–794. doi: 10.1080/11263504.2017.1338628
Ellawala, K. C., Asaeda, T., and Kawamura, K. (2011). The effect of flow turbulence on plant growth and several growth regulators in Egeria densa Planchon. Flora-Morphol. Distribut. Funct. Ecol. Plants 206, 1085–1091. doi: 10.1016/j.flora.2011.07.014
Geographical Survey Institute of Japan, the (GSI). (2018). The Topographic Map of Japan. Tsukuba: Geographical Survey Institute of Japan.
Gill, S. S., and Tuteja, N. (2010). Reactive oxygen species and antioxidant machinery in abiotic stress tolerance in crop plants. Plant Physiol. Biochem. 48, 909–930. doi: 10.1016/j.plaphy.2010.08.016
Gillard, M., Thiebaut, G., Deleu, C., and Leroy, B. (2017). Present and future distribution of three aquatic plants taxa across the world: decrease in native and increase in invasive ranges. Biol. Invasions 19, 2159–2170. doi: 10.1007/s10530-017-1428-y
Hanamoto, T., and Ikushima, I. (1988). Life cycle of Egeria densa planch., an aquatic plant naturalized in Japan. Aquat. Bot. 30, 389–403. doi: 10.1016/0304-3770(88)90070-8
Hauer, F. R., Locke, H., Dreitz, V. J., Hebblewhite, M., Lowe, W. H., Muhlfeld, C. C., et al. (2016). Gravel-bed river floodplains are the ecological nexus of glaciated mountain landscape. Sci. Adv. 2:e1600026. doi: 10.1126/sciadv.1600026
Hussner, A., Hoelken, H. P., and Jahns, P. (2010). Low light acclimated submerged freshwater plants show a pronounced sensitivity to increasing irradiances. Aquat. Bot. 93, 17–24. doi: 10.1016/j.aquabot.2010.02.003
Kadono, Y. (2004). Alien aquatic plants naturalized in Japan: history and present status. Global Environ. Res. 8, 163–169.
Middelboe, A. L., and Markager, S. (1997). Depth limits and minimum light requirements of freshwater macrophytes. Freshw. Biol. 37, 553–568. doi: 10.1046/j.1365-2427.1997.00183.x
Ministry of Lands, Infrastructure, Transportation and Tourism in Japan (2016). (2018). River Environmental Database. Available online at: http://mizukoku.nilim.go.jp/ksnkankyo/. (accessed October 2018). doi: 10.1046/j.1365-2427.1997.00183.x
Mittler, R. (2002). Oxidative stress, antioxidants and stress tolerance. Trends Plant Sci. 7, 405–410. doi: 10.1016/s1360-1385(02)02312-9
Nishiyama, Y., and Murata, N. (2014). Revised scheme for the mechanism of photoinhibition and its application to enhance the abiotic stress tolerance of the photosynthesis machinery. Appl. Microbiol. Biotechnol. 98, 8777–8796. doi: 10.1007/s00253-014-6020-0
O’Hare, M., Baattrup-Pedersen, A., Baumgarte, I., Freeman, A., Gunn, I. D. M., Lazar, A. N., et al. (2018). Responses of aquatic plants to eutrophication in rivers: a revised conceptual model. Front. Plant Sci. 9:1451. doi: 10.3389/fpls.2018.00451
Parker, G., and Peterson, A. W. (1980). Bar resistance of gravel-bed streams. J. Hydraul. Eng. 106, 1559–1575.
Parveen, M., Asaeda, T., and Rashid, M. D. H. (2017a). Biochemical adaptations of four submerged macrophytes under combined exposure to hypoxia and hydrogen sulphide. PLos ONE 12:e 0182691. doi: 10.1371/journal.pone.0182691
Parveen, M., Asaeda, T., and Rashid, M. D. H. (2017b). Effect of hydrogen sulfide exposure on the growth, oxidative stress and carbohydrate metabolism of Elodea nuttallii and Egeria densa. Fund. Appl. Limnol. 191:5.
Parveen, M., Asaeda, T., and Rashid, M. D. H. (2017c). Hydrogen sulfide induced growth, photosynthesis and biochemical responses in three submerged macrophytes. Flora 230, 1–11. doi: 10.1016/j.flora.2017.03.005
Redekop, P., Hofstra, D., and Hussner, A. (2016). Elodea Canadensis shows a higher dispersal capacity via fragmentation than Egeria densa and Lagarosiphon major. Aquat. Bot. 130, 45–49. doi: 10.1016/j.aquabot.2016.01.004
Riis, T., Olesen, B., Clayton, J. S., Lambertini, C., Brix, H., and Sorrell, B. K. (2012). Growth and morphology in relation to temperature and light availability during the establishment of three invasive aquatic plant species. Aquat. Bot. 102, 56–64. doi: 10.1016/j.aquabot.2012.05.002
Rivero, R. M., Mestre, T. C., Mittler, R., Rubio, F., Garcia-Sanchez, F., and Martinez, V. (2014). The combined effect of salinity and heat reveals a specific physiological, biochemical and molecular response in tomato plants. Plant Cell Environ. 37, 1059–1073. doi: 10.1111/pce.12199
Rodrigues, R. B., and Thomaz, S. M. (2010). Photosynthesis and growth responses of Egeria densa to Photosynthesis active radiation. Aquat. Bot. 92, 281–284. doi: 10.1016/j.aquabot.2010.01.009
Sand-Jensen, K. (1998). Influence of submerged macrophytes on sediment composition and near-bed flow in lowland streams. Freshw. Biol. 39, 663–679. doi: 10.1046/j.1365-2427.1998.00316.x
Santos, M. J., Anderson, L. W., and Ustin, S. L. (2011). Effective invasive species on plant communities: an example using submerged aquatic plants at the regional scale. Biol. Invasions 13, 443–457. doi: 10.1007/s10530-010-9840-6
Satterfield, C. N., and Bonnell, A. H. (1995). Interferences in the titanium sulfate method for hydrogen peroxide. Anal. Chem. 27, 1174–1175. doi: 10.1021/ac60103a042
Sharma, P., Jha, A. B., Dubey, R. S., and Pessarakli, M. (2012). Reactive oxygen species, oxidative damage, and antioxidative defense mechanism in plants under stressful conditions. J. Bot. 2012, 1–26. doi: 10.1155/2012/217037
Singer, M. B. (2008). Downstream patterns of bed material grain size in a large, lowland alluvial river subject to low sediment supply. Water Resour. Res. 44:W12202. doi: 10.1029/2008WR007183
Statzner, B., Lamouroux, N., Nikora, V., and Sagnes, P. (2006). The debate about drag and reconfiguration of freshwater macrophytes: comparing results obtained by three recently discussed approaches. Freshw. Biol. 51, 2173–2183. doi: 10.1111/j.1365-2427.2006.01636.x
Vermaat, J. E., Santamaria, L., and Roots, P. J. (2000). Water flow across and sediment trapping in submerged macrophyte beds of contrasting growth form. Archiv. Hydrobiol. 148, 549–562. doi: 10.1127/archiv-hydrobiol/148/2000/549
Yarrow, M., Marin, V. H., Finlayson, M., Tironi, A., Delgado, L. E., and Fisher, F. (2009). The ecology of Egeria densa Planchon (Liliopsida:Alismatales): a wetland ecosystem engineer? Rev. Chilena Historia Nat. 82, 299–313.
Zaman, T., and Asaeda, T. (2013). Effects of NH4–N concentrations and gradient redox level on growth and allied biochemical parameters of Elodea nuttallii (Planch.). Flora 208, 211–219. doi: 10.1016/j.flora.2013.02.009
Keywords: invasive species, environmental stress, oxidative stress, river vegetation, empirical equations
Citation: Asaeda T, Senavirathna MDHJ and Vamsi Krishna L (2020) Evaluation of Habitat Preferences of Invasive Macrophyte Egeria densa in Different Channel Slopes Using Hydrogen Peroxide as an Indicator. Front. Plant Sci. 11:422. doi: 10.3389/fpls.2020.00422
Received: 24 July 2019; Accepted: 23 March 2020;
Published: 30 April 2020.
Edited by:
Giuseppe Brundu, University of Sassari, ItalyReviewed by:
Emily Frances Strange, Leiden University, NetherlandsElisa Soana, University of Ferrara, Italy
Copyright © 2020 Asaeda, Senavirathna and Vamsi Krishna. This is an open-access article distributed under the terms of the Creative Commons Attribution License (CC BY). The use, distribution or reproduction in other forums is permitted, provided the original author(s) and the copyright owner(s) are credited and that the original publication in this journal is cited, in accordance with accepted academic practice. No use, distribution or reproduction is permitted which does not comply with these terms.
*Correspondence: Takashi Asaeda, asaeda@mail.saitama-u.ac.jp