- 1Laboratory of Crop Physiology, Department of Plant Biology, Institute of Biology, University of Campinas, Campinas, Brazil
- 2Laboratory of Plant Physiology “Coaracy M. Franco”, Center for Research & Development in Ecophysiology and Biophysics, Agronomic Institute, Campinas, Brazil
- 3Center for Natural and Human Sciences, Federal University of ABC, Santo André, Brazil
Nitric oxide (NO) is an important signaling molecule associated with many biochemical and physiological processes in plants under stressful conditions. Nitrate reductase (NR) not only mediates the reduction of NO3− to NO2− but also reduces NO2− to NO, a relevant pathway for NO production in higher plants. Herein, we hypothesized that sugarcane plants supplied with more NO3− as a source of N would produce more NO under water deficit. Such NO would reduce oxidative damage and favor photosynthetic metabolism and growth under water limiting conditions. Sugarcane plants were grown in nutrient solution and received the same amount of nitrogen, with varying nitrate:ammonium ratios (100:0 and 70:30). Plants were then grown under well-watered or water deficit conditions. Under water deficit, plants exhibited higher root [NO3−] and [NO2−] when supplied with 100% NO3−. Accordingly, the same plants also showed higher root NR activity and root NO production. We also found higher photosynthetic rates and stomatal conductance in plants supplied with more NO3−, which was associated with increased root growth. ROS accumulation was reduced due to increases in the activity of catalase in leaves and superoxide dismutase and ascorbate peroxidase in roots of plants supplied with 100% NO3− and facing water deficit. Such positive responses to water deficit were offset when a NO scavenger was supplied to the plants, thus confirming that increases in leaf gas exchange and plant growth were induced by NO. Concluding, NO3− supply is an interesting strategy for alleviating the negative effects of water deficit on sugarcane plants, increasing drought tolerance through enhanced NO production. Our data also provide insights on how plant nutrition could improve crop tolerance against abiotic stresses, such as drought.
Introduction
Nitric oxide (NO) is a diatomic radical gas and important signaling molecule in animals (Bogdan, 2015), fungi (Cánovas et al., 2016), bacteria (Crane et al., 2010), and plants (Mur et al., 2013). In plants, increasing evidence indicates NO as a key component of the signaling network, controlling numerous physiological and metabolic processes such as seed germination (Albertos et al., 2015), flowering (He et al., 2004), root growth (Fernandez-Marcos et al., 2011), respiration, stomatal conductance (Moreau et al., 2010; Wang et al., 2015), and adaptive responses to biotic and abiotic stresses (Shan et al., 2015; Fatma et al., 2016).
NO synthesis is increased in plants under drought and its role in promoting acclimation responses to cope with water deficit has been suggested (Cai et al., 2015; Silveira et al., 2017a). NO and NO-derived molecules play a critical role in intracellular redox signaling and in the activation of antioxidant defense mechanisms (Shi et al., 2014; Hatamzadeh et al., 2015; Silveira et al., 2015). For example, NO supply conferred drought tolerance to wheat seedlings, reducing membrane damage (García-Mata and Lamattina, 2001). Spraying S-nitrosogluthatione (GSNO)—a NO donor—on sugarcane plants resulted in higher photosynthesis under drought, promoting plant growth under stressful condition (Silveira et al., 2016).
The protective action of exogenous NO donors has been attributed to the elimination of superoxide (O2•-) and enhancement of the antioxidant system in sugarcane plants under drought (Silveira et al., 2017b). In addition, one of the main downstream effects of NO is the post-translational regulation involving thiols (Hancock and Neill, 2019). S-nitrosylation is a redox modification consisting in the reversible attachment of NO to the thiol group of a cysteine residue in a target protein leading to the formation S-nitrosothiols (SNOs) (Astier et al., 2012; Fancy et al., 2016). Then, S-nitrosylation may cause a conformational change in proteins, changing their activity or function. On the other hand, NO can react with reduced glutathione (GSH), producing S-nitrosoglutathione (GSNO)—an endogenous NO reservoir and an efficient NO donor (Jahnová et al., 2019).
While the mechanisms of NO synthesis in animals have been well documented, NO synthesis and its regulation in plants are complex and poorly understood. In animals, NO is bio-synthesized through NO synthase (NOS), which oxidizes L-arginine and produces L-citrulline and NO (Alderton et al., 2001). Although some evidence indicates the presence of NOS-like activity in many plant species, genes encoding NOS have not yet been identified in higher plants (Santolini et al., 2017; Hancock and Neill, 2019). NO production in plant species and under diverse biological conditions point to the co-existence of multiple pathways, likely functioning in distinct tissues/organs and subcellular compartments (León and Costa-Broseta, 2019).
One of the most important pathways for NO production in land plants is through nitrate reductase (NR) (Gupta et al., 2011; Fancy et al., 2016; Chamizo-Ampudia et al., 2017; León and Costa-Broseta, 2019), a multifunctional enzyme that catalyzes NO3− reduction to NO2−, which is then reduced to NH4+ during the N assimilatory pathway (Heidari et al., 2011). Arasimowicz-Jelonek et al. (2009) reported low NO concentration in cucumber seedlings treated with a NR inhibitor, suggesting its role in NO synthesis. In rice roots, NO production through NR was increased in response to NO3− supply (Sun et al., 2015). Furthermore, low NO production by Physcomitrella patens occurred when it received a NR inhibitor (Andrés et al., 2015). Although there are data supporting the association between NR activity and NO production in plants (Mur et al., 2013), some authors have argued that NO production through NR represents only a small fraction (1–2%) of total NO3− reduction (Yamasaki et al., 1999; Rockel et al., 2002). However, the role of such a NO production pathway and its sensitivity to small changes in NO3− supply in plants under water deficit remain unknown.
Nitrogen is the most influential plant nutrient in sugarcane cultivation (Meyer et al., 2007). Nitrate (NO3−), ammonium (NH4+), and urea (CO(NH2)2) are the main forms of fertilizers and, thus, are the main sources of N for crops (Esteban et al., 2016). Some crops have a preference for NH4+ uptake (Malagoli et al., 2000), but most studies have reported stress symptoms associated with NH4+ toxicity (Barreto et al., 2018; Boschiero et al., 2019). While Robinson et al. (2011) reported the sugarcane preference for NH4+, Pissolato et al. (2019a) found that increasing NH4+ supply causes biomass reduction and photosynthesis impairment of sugarcane plants. Changing the N source, NO3− supply has been shown to increase the tolerance to abiotic stresses in maize (Rios-Gonzales et al., 2002) and grass species (Wang and Macko, 2011).
The literature concerning NO3− supply and stress tolerance, taken together, led us to hypothesize that the increased plant performance under limiting conditions could be related to NO production through NR activity. Here, our aim was to test the hypothesis that sugarcane plants that receive NO3− and no NH4+ as sources of nitrogen will have higher NR activity and thereby produce more NO, compared to plants receiving the same amount of nitrogen but as a mixture of NO3− (70%) and NH4+ (30%). As a consequence of NO production, oxidative damage will be reduced under water deficit, favoring photosynthetic metabolism and plant growth.
Materials and Methods
Plant Material and Growth Conditions
Pre-sprouted sugarcane plants (Saccharum spp.) cv. IACSP95-5000 developed by the Sugarcane Breeding Program of the Agronomic Institute (ProCana, IAC, Brazil) were used. Six-week-old plants were transferred to plastic boxes (4 L) containing nutrient solution modified from De Armas et al. (1992): 5 mmol L−1 N (nitrate 90% + ammonium 10%); 9 mmol L−1 Ca; 0.5 mmol L−1 Mg; 1.2 mmol L−1 P; 1.2 mmol L−1 S; 24 µmol L−1 B; 16 µmol L−1 Fe; 9 µmol L−1 Mn; 3.5 µmol L−1 Zn; 1 µmol L−1 Cu; and 0.1 µmol L−1 Mo. Plants received this solution for 2 weeks until the establishment of treatments and the nutrient solution was renewed every 3 days throughout the experimental period.
Electrical conductivity of nutrient solution was maintained between 1.8 and 2.0 mS cm-¹ and pH at 5.9 ± 0.1. The pH was adjusted when necessary with 0.5 M citric acid or 0.5 M NaOH. Both variables were monitored on a daily basis using a portable electrical conductivity meter (mCA 150P, MS Tecnopon Instrumentação, Piracicaba SP, Brazil) and a portable pH meter (mPA 210P, MS Tecnopon Instrumentação, Piracicaba SP, Brazil). The nutrient solution volume was also checked daily and completed with water when necessary. The nutrient solution was aerated continuously by using an air compressor (Master Super II, Master, São Paulo SP, Brazil).
The experiment was carried in a growth chamber (Instalafrio, Brazil), with a 12 h photoperiod, air temperature of 30/20°C (day/night), air relative humidity of 80% and photosynthetic photon flux density (PPFD) about 800 µmol m−2 s−1.
Experiment I: Inducing NO Production Under Water Deficit Through NO3– Supply
Our previous study revealed that sugarcane plants can be supplied with 30% NH4+ in nutrient solution without compromising their photosynthesis and growth (Pissolato et al., 2019a). Thus, the NO3−:NH4+ ratios 100:0 and 70:30 were chosen to represent the treatments with more and less NO3−, while supplying the same amount of nitrogen and avoiding NH4+ toxicity. Plants were also subjected to varying water availability, according to the osmotic potential of nutrient solution: −0.15 MPa (reference, well-hydrated); and −0.75 MPa (water deficit, WD), totaling four treatments (2 NO3−:NH4+ ratios × 2 water treatments) with four biological replicates. The water deficit was induced by adding polyethylene glycol (Carbowax™ PEG-8000, Dow Chemical Comp, Midland MI, USA) to the nutrient solution, seven days after imposing NO3−:NH4+ ratios. To prevent osmotic shock, PEG-8000 was gradually added to the nutrient solution, reducing the osmotic potential of the solution by −0.20 MPa per day, i.e., −0.75 MPa was reached after three days (3th day of the experiment). Plants were allowed to recover from water deficit after returning them to control conditions on the 7th day of the experiment. They remained for four days under such conditions, when the experiment ended. For the biochemical analyzes, there were four biological replicates for both leaves and roots and samples were taken at the maximum water deficit (7th day) and at the end of the rehydration period (11th day). Samples were collected, immediately immersed in liquid nitrogen and then stored at −80°C. Biochemical analyzes were performed in technical triplicates.
Nitrate, Nitrite, and Ammonium
Fresh plant tissue samples (500 mg) were ground in liquid nitrogen and extraction medium containing methanol/chloroform/water (12:5:3 v/v). After centrifugation at 2,000 g for 5 min, the supernatants were collected, and chloroform and deionized water were added to them. The mixture was shaken vigorously and then centrifuged for 3 min at 2,000 g for phase separation. The upper aqueous phase was collected and maintained in a water bath at 37°C to remove traces of chloroform, and then the extracts were stored at −20°C (Bieleski and Turner, 1966).
For nitrate determination, an aliquot of the extract was pipetted into test tubes containing reaction medium (5% salicylic acid in conc. H2SO4). After 20 min, 2 N NaOH was added and the solution stirred. After cooling to room temperature, the absorbance was read in a spectrophotometer at 410 nm and the nitrate content calculated from a standard curve using KNO3 (100–1000 nmol) (Cataldo et al., 1975). For nitrite, an aliquot of the extract was added to 1% sulfanilamide solution in 3 N HCl and 0.02% N-naphthyl ethylenediamine solution. The tubes were allowed to stand for 30 min in the dark at room temperature. Deionized water was added and nitrite content quantified after reading the absorbance at 540 nm (Hageman and Reed, 1980). For ammonium, the extract was added to microtubes, where solution A (1% phenol and 0.005% sodium nitroprusside) was added and followed by solution B (0.5% sodium hydroxide containing 2.52% sodium hypochlorite). The tubes were incubated for 35 min in a water bath at 37°C, and the absorbance read at 625 nm after cooling to room temperature (McCullough, 1967). A standard curve of (NH4)2SO4 was used to estimate the ammonium content. Concentrations were corrected to sample water content and expressed on dry weight basis.
Nitrate Reductase Activity
Leaf and root nitrate reductase (NR, EC 1.7.1.1) activity was estimated as the rate of nitrite (NO2−) production (Cambraia et al., 1989). The enzyme extract was obtained from the macerate of 200 mg of fresh tissue with liquid nitrogen and homogenized with extraction medium containing 0.1 M tris-HCl buffer (pH 8.1), 4 mM NiSO4, 20 mM reduced glutathione (GSH), deionized water, and 0.5 mM PMSF. Then, the crude extracts were centrifuged at 10,000 g for 10 min at 4°C, and the supernatant was collected and maintained on ice. The extract was added to the assay medium containing 100 mM Tris-HCl buffer (pH 7.5), 10 mM KNO3, 0.05 mM NADH, and triton 1% X-100 (v/v), mixed and incubated at 30°C for 10 min. The reaction was quenched by adding 1% sulfanilamide solution in 1 M HCl and 0.01% N-naphthyl ethylenediamine. Nitrite production was determined by absorbance at 540 nm using a standard curve with KNO2. The NR activity was expressed as µmol NO2− min−1 mg−1 protein.
S-Nitrosogluthatione Reductase Activity
Leaf and root S-nitrosogluthatione reductase (GSNOR, EC 1.2.1.1) activity was determined spectrophotometrically at 25°C by monitoring the oxidation of NADH at 340 nm, based on Rodríguez-Ruiz et al. (2017). Briefly, 200 mg of fresh tissue were grounded with liquid nitrogen, resuspended in 20 mM HEPES buffer (pH 8.0), 10 mM EDTA, 0.5 mM PMSF, and centrifuged for 10 min at 10,000 g and 4°C. The enzyme extract was added in to the assay medium (20 mM HEPES buffer pH 8.0 and 1.8 mM NADH) at 25°C, and maintained in the dark. The reaction was started by adding 4 mM GSNO (Silveira et al., 2016) and the GSNOR activity followed by NADH oxidation at 340 nm. Activity was calculated using the NADH extinction coefficient (6.22 mM−1 cm−1 at 340 nm) and expressed as nmol NADH min−1 mg−1 protein.
S-Nitrosothiols Content
The total leaf and root proteins were extracted in deionized water and the resulting homogenate was used to estimate the S-nitrosothiol content through an amperometer, as described by Santos et al. (2016) and Zhang et al. (2000). Measurements were performed with the WPI amperometer TBR 4100/1025 (World Precision Instruments Inc., Sarasota FL, USA) and a specific nitric oxide (NO) sensor, ISO-NOP (2 mm). Aliquots of aqueous suspension were added to the sample compartment containing aqueous copper chloride solution (0.1 mol L−1). This condition allowed the detection of free NO released from the S-nitrosothiols present in the leaf and root protein homogenate. The samples were run in triplicate, and the calibration curve was obtained with newly prepared GSNO solutions. The data were compared with the standard curve obtained and normalized against fresh weight. The SNO content was expressed as nmol NO mg−1 protein.
Intracellular NO Detection
NO was assayed in leaf and root segments according to Silveira et al. (2019a). For the roots, it was collected approximately 1 cm from the middle part of secondary root. For the leaves, a thin cross section was made with the aid of a scalpel. The segments were incubated in MES-KCl buffer (10 mM MES, 50 mM KCl, 0.1 mM CaCl2, pH 6.15), at room temperature for 15 min. Then, these segments were incubated in solution of 10 µM DAF2-DA, mixing for 40 min in the dark at room temperature (Desikan et al., 2002; Bright et al., 2009). The samples were washed with buffer to remove the excess of DAF2-DA, placed onto a glass slide and covered with a glass slip before observing fluorescence using an inverted confocal microscope set for excitation at 488 nm and emission at 515 nm (Model Zeiss LSM510, Carl Zeiss AG, Germany). Photographs were taken with a 10x magnification, 15 s exposure and 1× gain. Images were analyzed using ImageJ software (NIH, Bethesda, MD, USA) and data were normalized by subtracting the values of the negative control (plants well-hydrated) and presented as mean pixel intensities.
Reactive Oxygen Species
The concentration of the superoxide anion (O2•−) was determined in 50 mg of fresh tissue incubated in an extraction medium consisting of 100 μM EDTA, 20 μM NADH, and 20 mM sodium phosphate buffer, pH 7.8. The reaction was initiated by adding 25.2 mM epinephrine in 0.1 N HCl. The samples were incubated at 28°C under stirring for 5 min and the absorbance was read at 480 nm over a further 5 min (Mohammadi and Karr, 2001). O2•− production was assessed by the accumulation of adrenochrome using a molar extinction coefficient of 4.0×103 M−1 cm−1 (Boveris, 1984). O2•− concentration was expressed as µmol O2•− g−1 dry weight.
The quantification of hydrogen peroxide (H2O2) was performed following Alexieva et al. (2001). Homogenates were obtained from 100 mg of fresh tissue ground in liquid nitrogen with the addition of polyvinylpolypyrrolidone (PVPP) and 0.1% of trichloroacetic acid (TCA) solution (w/v). The extract was centrifuged at 10,000 g and 4°C for 15 min. The reaction medium consisted of 1 mM KI, 0.1 M potassium phosphate buffer (pH 7.5) and crude extract. The microtubes were left on ice in the dark for 1 h. After this period, the absorbance was read at 390 nm. A standard curve was obtained with H2O2, and the results were expressed as µmol H2O2 g−1 dry weight.
Lipid Peroxidation
The concentration of malondialdehyde (MDA) was measured and used as a proxy of lipid peroxidation. 200 mg of fresh tissue were macerated in extraction medium containing 0.1% TCA (w/v) and centrifuged at 10,000 g for 15 min. The supernatant was added to 0.5% thiobarbituric acid (w/v) in 20% TCA (w/v), and the mixture incubated at 95°C for 20 min (Cakmak and Horst, 1991). After this time, the reaction was stopped in an ice bath. Then a new centrifugation was performed at 10,000 g for 10 min, and after 30 min at room temperature the absorbance was read at 532 and 600 nm, and the non-specific absorbance at 600 nm was discounted. The MDA concentration was calculated using an extinction coefficient of 155 mM−1 cm−1 (Heath and Packer, 1968) and results were expressed as µmol MDA g−1 dry weight.
Antioxidant Activity and Protein Extraction
The crude enzymatic extracts for the determination of superoxide dismutase activity (SOD), catalase (CAT) and ascorbate peroxidase (APX) were obtained from 100 mg of plant tissue in specific medium, followed by centrifugation at 12,000 g for 15 min at 4°C. The specific medium for CAT and SOD consisted of 0.1 M potassium phosphate buffer (pH 6.8), 0.1 mM EDTA, 1 mM PMSF and 1% PVPP, according to Peixoto et al. (1999). The specific medium for APX was composed of 50 mM potassium phosphate buffer (pH 7.0), 1 mM ascorbic acid, and 1 mM EDTA (Nakano and Asada, 1981).
Superoxide dismutase (SOD, EC 1.15.1.1) activity was determined according to Giannopolitis and Ries (1977). The crude extract was added to the reaction medium consisting of 100 mM sodium phosphate buffer (pH 7.8), 50 mM methionine, 5 mM EDTA, deionized water, 100 μM riboflavin, and 1 mM nitro blue tetrazolium chloride (NBT). A group of tubes was exposed to light (fluorescent lamp, 30 W) for 10 min, and another group remained in darkness. The absorbance was measured at 560 nm and one unit of SOD defined as the amount of enzyme required to inhibit NBT photoreduction by 50%, and activity expressed as U min−1 mg−1 of protein.
Catalase (CAT, EC 1.11.1.6) activity was quantified following the procedure described by Havir and McHale (1987). The crude extract was added to the reaction medium consisting of 100 mM potassium phosphate buffer (pH 6.8), deionized water, and 125 mM H2O2. The reaction was carried out in a water bath at 25°C for 2 min, and CAT activity was assessed by the decrease in absorbance at 240 nm, using the molar extinction coefficient of 36 M−1 cm−1 and expressed activity as nmol min−1 mg−1 of protein.
Ascorbate peroxidase (APX, EC 1.11.1.11) activity was evaluated as described by Nakano and Asada (1981). The crude extract was added in reaction medium consisting of 100 mM potassium phosphate buffer (pH 6.8), deionized water, 10 mM ascorbic acid, and 10 mM H2O2. The reaction was carried out at 25°C for 2 min and APX activity quantified by the decrease in absorbance at 290 nm, using the molar extinction coefficient of 2.8 M−1 cm−1 and expressing activity as μmol min−1 mg−1 of protein.
The protein levels were determined by the Bradford method (Bradford, 1976), using bovine serum albumin (BSA) as the standard. The extract used for this analysis was the same as for SOD and CAT enzymes.
Leaf Gas Exchange
Gas exchange and chlorophyll fluorescence of the first fully expanded leaf with visible ligule were measured throughout the experimental period using an infrared gas analyzer (Li-6400, Li-Cor, Lincoln NE, USA) equipped with a modulated fluorometer (6400-40 LCF, Li-Cor, Lincoln NE, USA). Net CO2 assimilation rate (An), stomatal conductance (gs) and the effective quantum efficiency of photosystem II (ϕPSII) were measured under PPFD of 2000 µmol m−2 s−1 and air CO2 concentration of 400 µmol mol−1. The measurements were performed between 10:30 and 12:30 h, as carried out previously (Pissolato et al., 2019a). The vapor pressure difference between leaf and air (VPDL) was 2.1 ± 0.2 kPa, and leaf temperature was 30 ± 0.4°C during the evaluations.
Photosynthetic Enzymes
The activity of ribulose-1,5-bisphosphate carboxylase/oxygenase (Rubisco, EC 4.1.1.39) was quantified in approximately 200 mg of leaves, which were macerated and homogenized in 100 mM bicine-NaOH buffer (pH 7.8), 1 mM ethylenediaminetetraacetic (EDTA), 5 mM MgCl2, 5 mM dithiothreitol (DTT), 1 mM phenylmethylsulfonyl fluoride (PMSF) and 10 µM leupeptin. The resulting solution was centrifuged at 14,000 g for 5 min at 4°C. An aliquot of leaf extract was incubated with the reaction medium containing 100 mM bicine-NaOH (pH 8.0) 10 mM NaHCO3, 20 mM MgCl2, 3.5 mM ATP, 5 mM phosphocreatine, 0.25 mM NADH, 80 nkat glyceraldehyde-3-phosphate dehydrogenase, 80 nkat 3-phosphoglyceric phosphokinase, and 80 nkat creatine phosphokinase, for 10 min at 25°C. The oxidation of NADH was initiated by adding 0.5 mM ribulose-1,5-bisphosphate (RuBP) and total Rubisco activity was measured. The reduction of absorbance at 340 nm was monitored for 3 min (Sage et al., 1988; Reid et al., 1997).
The activity of phosphoenolpyruvate carboxylase (PEPC, EC 4.1.1.31) was also evaluated in approximately 200 mg of leaves, which were macerated and homogenized in 100 mM potassium phosphate buffer (pH 7), 1 mM EDTA, 1 mM PMSF, and centrifuged at 14,000 g for 25 min at 4°C. The supernatant was collected, and the reaction medium for PEPC activity contained 50 mM Tris-HCl buffer (pH 7.8), 5 mM MgCl2, 5 mM glucose 6-phosphate, 10 mM NaHCO3, 33 nkat malic dehydrogenase, and 0.3 mM NADH. The reaction was initiated by adding 4 mM phosphoenolpyruvate at 30°C. The oxidation of NADH was monitored a 340 nm for 1 min (Degl’Innocenti et al., 2002).
Proteins were extracted from leaf samples with extraction buffer composed of 100 mM Tris, 1 mM EDTA, 5 mM DTT, 1 mM PMSF, and separated by SDS-PAGE (Laemmli, 1970). The first gel was stained with Comassie Brilliant Blue, and the second was used for Western blot. SDS-PAGE electrophoresis was performed with equal amounts of protein per lane. Soluble proteins were denatured using SDS, and they were electrophoretically transferred to a nitrocellulose membrane (Towbin et al., 1979). PEPC and Rubisco protein abundances were measured by detection of the PEPC subunit and Rubisco large subunit (RLS) using specific polyclonal antibodies (Agrisera Co, Sweden) according to the manufacturer’s instructions.
Chlorophyll Content and Leaf Relative Water Content (RWC)
A chlorophyll meter (CFL 1030, Falker, Porto Alegre RS, Brazil) was used to assess the relative chlorophyll content (Chl). The relative water content was calculated using the fresh (FW), turgid (TW) and dry (DW) weights of leaf discs, according to Jamaux et al. (1997): RWC=100×[(FW−DW)/(TW−DW)]. Leaf discs were collected during the morning (about 10:00 h) and measurements were taken at the maximum water deficit (7th day), and four days after returning plants to the control condition (re-watering period, 11th day).
Biometry
Leaf and root dry masses were quantified after drying samples in an oven (60°C) with forced-air circulation until constant weight. Leaf area of each plant was evaluated with a portable leaf area meter (model LI-3000, Li-Cor Inc., Lincoln NE, USA).
Experiment II: Using cPTIO to Offset the Benefits of NO in Plants Under Water Deficit
An additional experiment was performed to verify whether the benefits found in plants supplied with only NO3– and subjected to water deficit were in fact caused by NO. We used a NO scavenger, 2-(4-carboxyphenyl)-4,4,5,5-tetramethylimidazoline-1-oxyl-3-oxide (cPTIO). cPTIO is a stable organic radical developed by Akaike and Maeda (1996), which has been widely used as a control as it oxidizes the NO molecule to form NO2. In plants supplied with only NO3- as N source, the following treatments were evaluated: (a) well-watered condition, with an osmotic potential of the nutrient solution of −0.15 MPa; (b) water deficit, with an osmotic potential of nutrient solution of −0.75 MPa; and (c) same as b with 100 µM cPTIO.
First, plants were moved and roots placed in a moist chamber, where they were sprayed with cPTIO and remained in the dark for 1 hour. After this treatment, the plants were returned to the boxes with the original nutrient solution. This procedure was performed for four consecutive days from the moment the water deficit (−0.75 MPa) was installed. We also evaluated the production of intracellular NO, plant biomass, net CO2 assimilation rate (An) and stomatal conductance (gs) as described previously.
Experimental Design and Statistical Analyses
The experiment was carried out in a completely randomized design and two causes of variation were analyzed: water availability and nitrogen source. Data were analyzed using Bayesian statistics and we used the JASP software (https://jasp-stats.org/). When significant differences were detected, the mean values (n=4) were compared using Bayes Factor (BF10). Our interpretation of Bayes Factor as evidence for alternative hypothesis (H1) was based on Raftery (1995): when 1 < BF10 < 3, there is a weak support to H1; 3 < BF10 < 20 indicates positive support to H1; and BF10 > 20 indicates strong support to the alternative hypothesis.
Results
Experiment I: Sugarcane Responses to Water Deficit as Affected by NO3– Supply
Nitrate, Nitrite, and Ammonium
We found no differences in leaf [NO3–] in plants subjected to water deficit (Figure 1A). Root [NO3–] was significantly higher in plants supplied with 100% NO3– under water deficit when compared to plants supplied with 70% NO3– under water deficit (Figure 1B). While leaf [NO2–] did not vary among treatments (Figure 1C), we found the highest root [NO2–] in plants supplied with 100% NO3– under water deficit (Figure 1D). We did not find significant changes in leaf and root [NH4+] due to NO3– supply under water deficit (Figures 1E, F). During the re-watering period, both previously stressed plants and the controls presented similar leaf and root [NO3–], [NO2–] and [NH4+] (Figure 1).
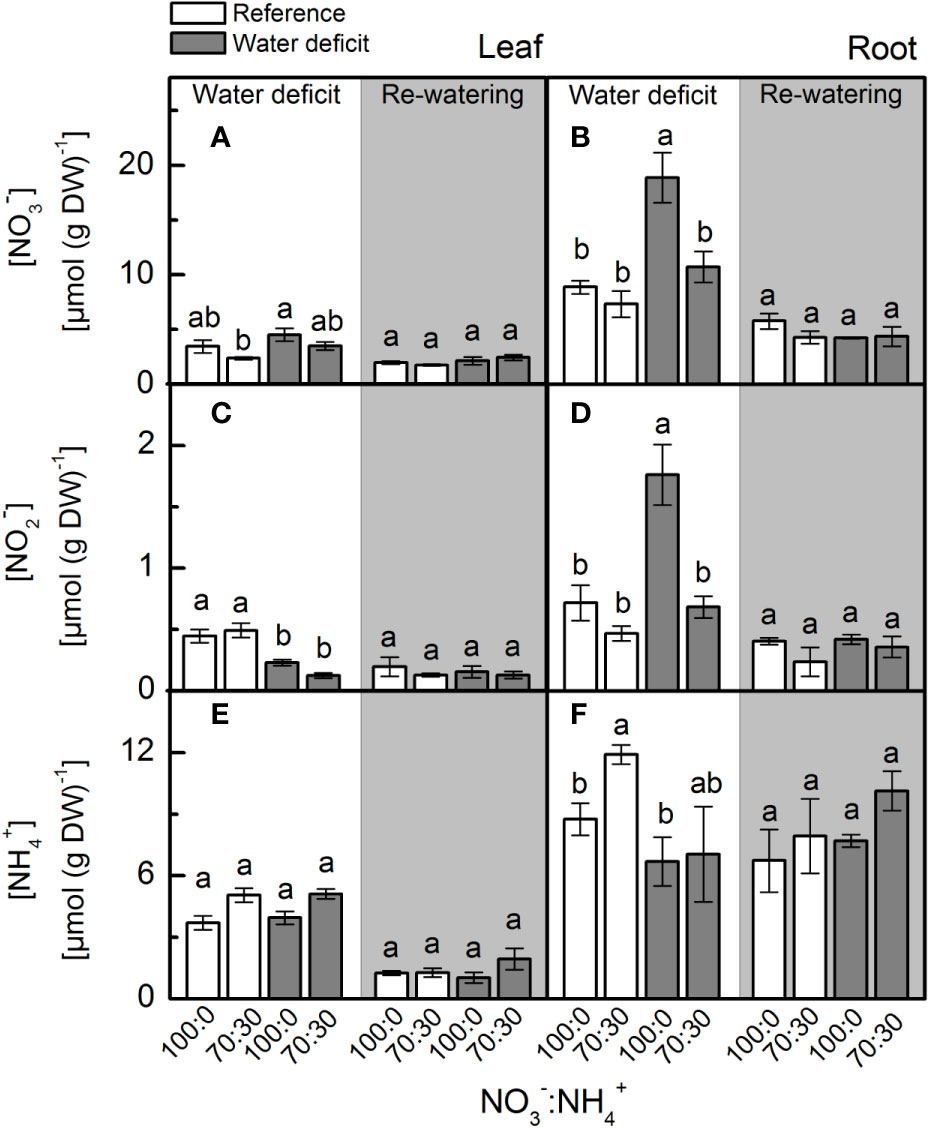
Figure 1 Concentration of nitrate (A, B), nitrite (C, D) and ammonium (E, F) in leaves (A, C, E) and roots (B, D, F) of sugarcane plants maintained well-hydrated (reference, white bars) or subjected to water deficit (gray bars) and supplied with varying NO3−:NH4+ ratios: 100:0 and 70:30. The white area indicates the period of water deficit, and the shaded area indicates the period of re-water. Bars represent the mean value of four biological replicates ± se. Different letters indicate statistical difference (BF10 > 3) among treatments in a given evaluation.
Nitrate Reductase, S-Nitrosoglutathione Reductase, and S-Nitrosothiols
Under low water availability, nitrate reductase (NR) activity was higher in plants supplied with 100% NO3– than those receiving 70% NO3–, regardless the plant organ (Figures 2A, B). While we did not notice differences among treatments for leaf NR activity during the re-watering period, root NR activity was higher under water deficit (Figure 2B). Under water deficit, plants supplied with 100% NO3– showed higher root GSNOR activity than those under 70% NO3– (Figure 2D). Non-significant differences were found in leaf SNO concentration while varying NO3– supply (Figure 2E). However, the lowest root S-nitrosothiols (SNO) concentration was observed in plants supplied with 100% NO3– under water deficit (Figure 2F).
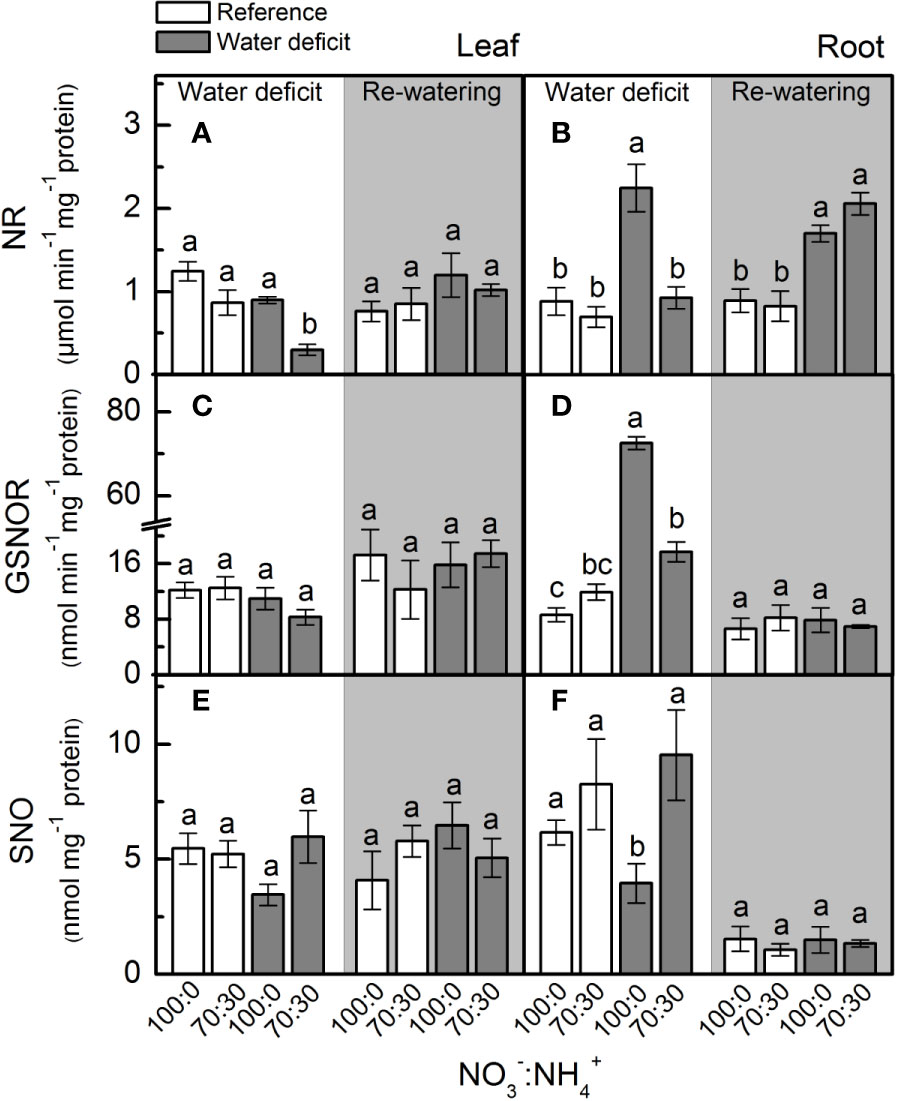
Figure 2 Nitrate reductase activity [NR, in (A, B)], S-nitrosoglutathione reductase activity [GSNOR, in (C, D)] and S-nitrosothiol concentration [SNO, in (E, F)] in leaves (A, C, E) and roots (B, D, F) of sugarcane plants maintained well-hydrated (reference, white bars) or subjected to water deficit (gray bars) and supplied with varying NO3−:NH4+ ratios: 100:0 and 70:30. The white area indicates the period of water deficit and the shaded area indicates the period of re-water. Bars represent the mean value of four biological replicates ± se. Different letters indicate statistical difference (BF10 > 3) among treatments in a given evaluation.
Intracellular NO Synthesis
When plants were facing low water availability, the intracellular NO was increased in both leaves and roots (Figure 3). However, roots receiving 100% NO3– exhibited higher NO production than those supplied with 70% NO3– (Figure 3B). Such a response did not occur in leaves (Figure 3A).
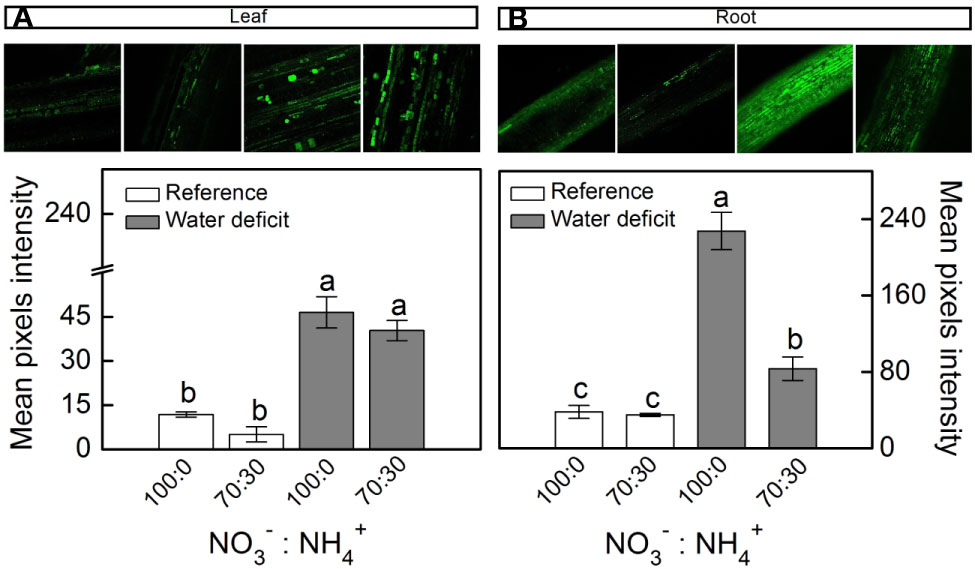
Figure 3 Confocal microscopy images showing intracellular NO synthesis in leaves (A) and roots (B) of sugarcane plants maintained well-hydrated (reference, white bars) or subjected to water deficit (gray bars) and supplied with varying NO3−:NH4+ ratios: 100:0 and 70:30. Mean pixel intensities are also shown. Bars represent the mean value of four biological replicates ± se. Different letters indicate statistical difference among treatments (BF10 > 3 for leaf; BF10 > 20 for root).
Antioxidant Metabolism
Plants supplied with less NO3– presented higher leaf [O2•-] when compared to ones supplied with 100% NO3– under water deficit (Figure 4A). When plants faced water deficit, the highest root [H2O2] was found under 70% NO3– supply (Figure 4D). Although showing higher accumulation of O2•- and H2O2 in leaves and roots, plants supplied with 70% NO3– did not show higher MDA content than those under 100% NO3– (Figures 4E, F).
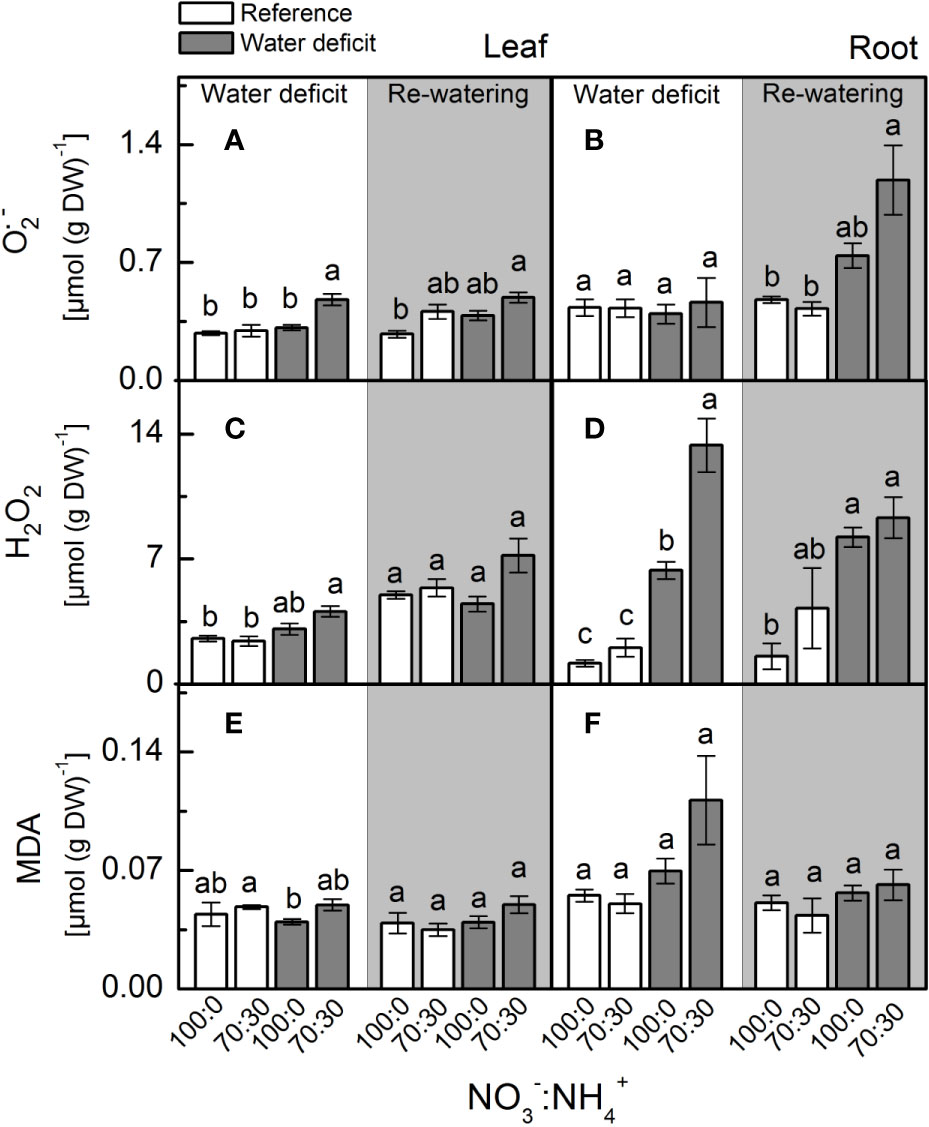
Figure 4 Concentration of superoxide anion [O2•−, in (A, B)], hydrogen peroxide (H2O2, in (C, D)] and malondialdehyde [MDA, in (E, F)] in leaves (A, C, E) and roots (B, D, F) of sugarcane plants maintained well-hydrated (reference, white bars) or subjected to water deficit (gray bars) and supplied with varying NO3−:NH4+ ratios: 100:0 and 70:30. The white area indicates the period of water deficit and the shaded area indicates the period of re-water. Bars represent the mean value of four biological replicates ± se. Different letters indicate statistical difference (BF10 > 3) among treatments in a given evaluation.
At the maximum water deficit, we found no differences in leaf superoxide dismutase (SOD) activity due to changes in NO3– supply (Figure 5A), but the highest SOD activity was observed in roots supplied with 100% NO3– (Figure 5B). Plants supplied with 100% NO3– showed higher root ascorbate peroxidase (APX) and leaf catalase (CAT) activities under water deficit (Figures 5D, E), while root catalase (CAT) activity was not changed by NO3– supply and water deficit (Figure 5F).
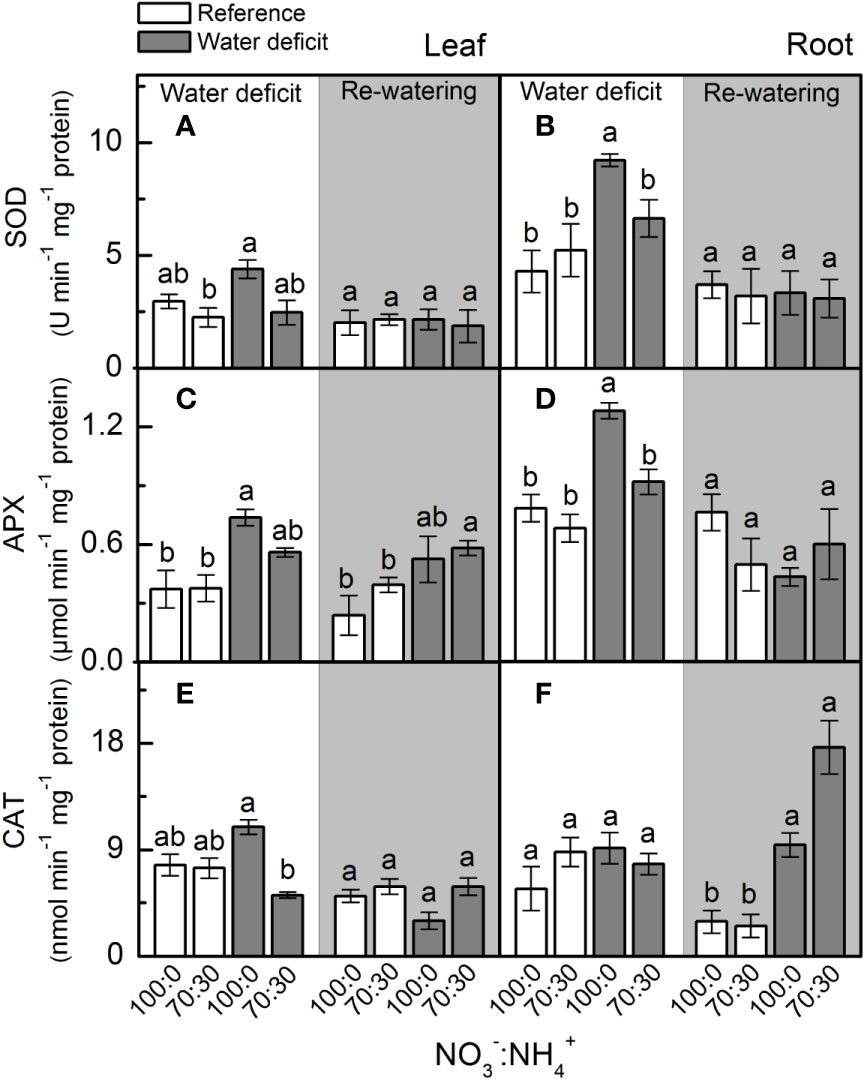
Figure 5 Superoxide dismutase activity [SOD, in (A, B)], ascorbate peroxidase activity [APX, in (C, D)] and catalase activity [CAT, in (E, F)] in leaves (A, C, E) and roots (B, D, F) of sugarcane plants maintained well-hydrated (reference, white bars) or subjected to water deficit (gray bars) and supplied with varying NO3−:NH4+ ratios: 100:0 and 70:30. The white area indicates the period of water deficit and the shaded area indicates the period of re-water. Bars represent the mean value of four biological replicates ± se. Different letters indicate statistical difference (BF10 > 3) among treatments in a given evaluation.
Photosynthesis and Relative Water Content
Low water availability caused a large reduction in net CO2 assimilation rate (An), however, plants supplied with more NO3– exhibited higher photosynthetic rates than those under NO3–:NH4+ 70:30 (Figure 6A). In addition, those plants showed a faster recovery of An after re-watering when compared to ones receiving 70% NO3– (Figure 6A). Similar results were found for stomatal conductance (Figure 6B) and effective quantum efficiency of PSII (Figure 6C). A significant reduction in leaf relative water content was found under water deficit, as compared to well-watered conditions (Figure 6D). We did not observe any significant difference among treatments for the PEPC abundance and activity at maximum water deficit (Supplementary Figures S1A, C). However, both Rubisco abundance and activity were decreased under water deficit, regardless of the variation in NO3– supply (Supplementary Figures S1B, D) The relative chlorophyll content was also reduced at the maximum water deficit, with no differences induced by NO3– supply (data not shown).
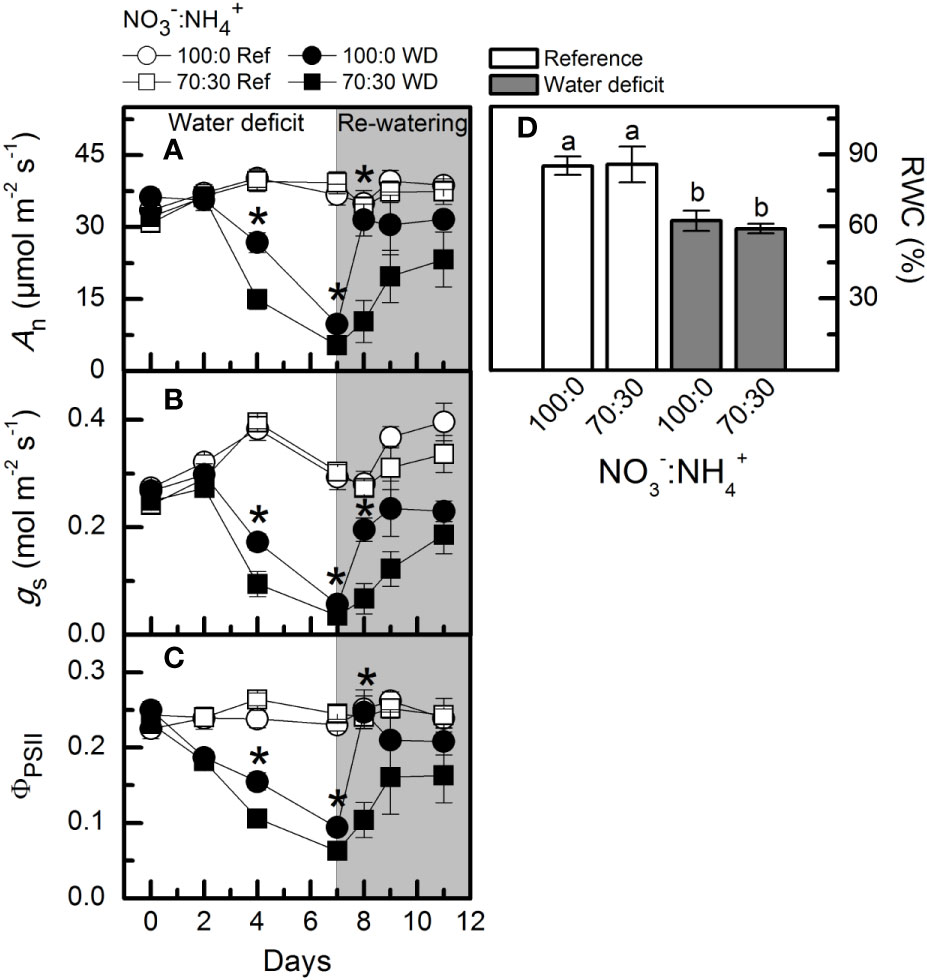
Figure 6 Net CO2 assimilation rate [An, in (A)], stomatal conductance [gS, in (B)], effective quantum efficiency of PSII [ΦPSII, in (C)] and leaf relative water content [RWC, in (D)] in sugarcane plants maintained well-hydrated (ref, white symbols and bars) or subjected to water deficit (WD, black symbols and gray bars) and supplied with varying NO3−:NH4+ ratios: 100:0 and 70:30. The white area indicates the period of water deficit and the shaded area indicates the period of re-water. Symbols and bars represent the mean value of four biological replicates ± se. Asterisks indicate significant differences between treatments under water deficit and different letters indicate statistical difference (BF10 > 3) among treatments in a given evaluation.
Plant Growth
The lowest values for shoot dry mass (Figure 7A) and leaf area (Figure 7C) were found in plants supplied with less NO3– under low water availability. In addition, the root dry mass of plants supplied with 70% NO3– was significantly reduced under water deficit (Figure 7B).
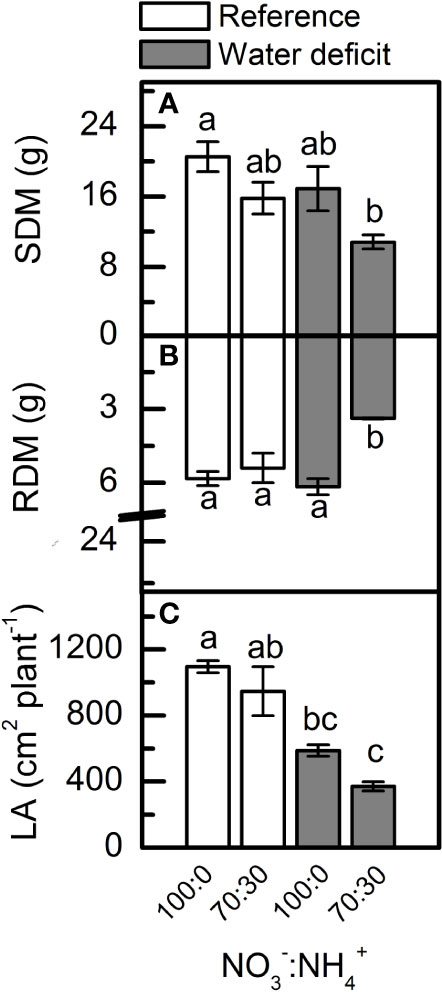
Figure 7 Shoot [SDM, in (A)] and root [RDM, in (B)] dry mass and leaf area [LA, in (C)] of sugarcane plants maintained well-hydrated (reference, white bars) or subjected to water deficit (gray bars) and supplied with varying NO3−:NH4+ ratios: 100:0 and 70:30. Bars represent the mean value of four biological replicates ± se. Different letters indicate statistical difference among treatments (BF10 > 3 for SDM and LA; BF10 > 20 for RDM).
Experiment II: Offsetting the Benefits of NO Synthesis Induced by NO3− Supply
cPTIO—a NO scavenger—was sprayed on roots supplied with 100% NO3– and facing water deficit. As consequence, the intracellular NO synthesis was reduced in leaves and roots (Figures 8A, B) and plants showed lower photosynthetic rates and stomatal conductance under water deficit as compared to ones not sprayed with cPTIO (Figures 9A, B). As found in experiment I, plants presented decreases in root dry mass due to water deficit when cPTIO was sprayed (Figure 9D; Supplementary Figure S2).
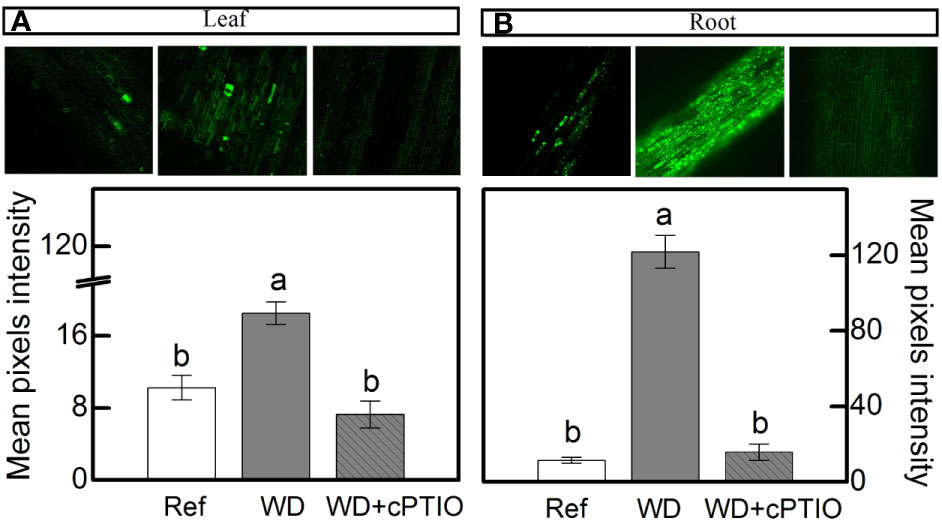
Figure 8 Confocal microscopy images showing intracellular NO synthesis in leaves (A) and roots (B) of sugarcane plants supplied with only NO3− (100:0 NO3−:NH4+) and maintained well-hydrated (reference, white bars), subjected to water deficit (WD, gray bars) and subjected to water deficit and sprayed with cPTIO (WD+cPTIO, gray striped bars). Mean pixel intensities are also shown. Bars represent the mean value of four biological replicates ± se. Different letters indicate statistical difference among treatments (BF10 > 3 for leaf; BF10 > 20 for root).
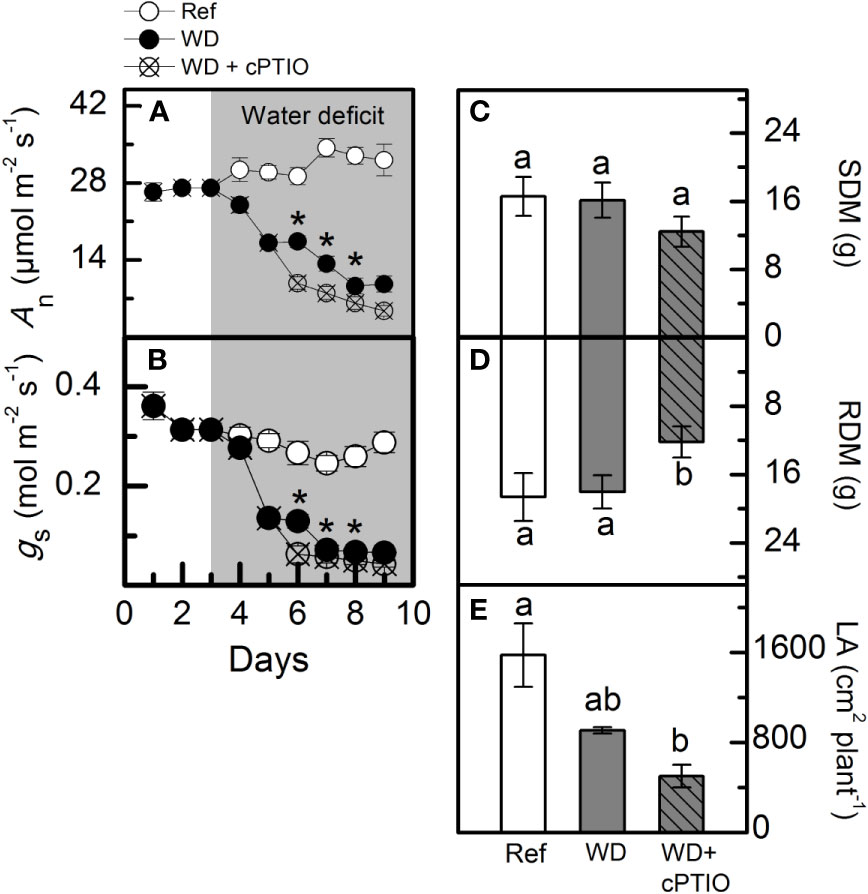
Figure 9 Net CO2 assimilation rate [An, in (A)], stomatal conductance [gS, in (B)], shoot [SDM, in (C)] and root [RDM, in (D)] dry mass and leaf area [LA, in (E)] of sugarcane plants supplied with only NO3− (100:0 NO3−:NH4+) and maintained well-hydrated (reference, white symbols and bars), subjected to water deficit (WD, black symbols and gray bars) and subjected to water deficit and sprayed with cPTIO (WD+cPTIO, crossed symbols and gray striped bars). Symbols and bars represent the mean value of four biological replicates ± se. Asterisks indicate significant differences between treatments under water deficit (A, B) and different letters indicate statistical difference (BF10 > 3) among treatments in a given evaluation.
Discussion
Nitrate Supply Stimulates Root NO Production, Improving Photosynthesis and Antioxidant Metabolism of Sugarcane Under Water Deficit
Our findings revealed that nitrate reductase is an important enzymatic pathway for NO synthesis and also that sugarcane plants supplied with 100% NO3− presented enhancement of drought tolerance. Here, we found higher NO3− accumulation in roots under water deficit and receiving only NO3− as source of nitrogen (Figure 1B), which caused higher NO2− production when compared to roots exposed to 70% NO3− and 30% NH4+ (Figure 1D). Such findings are supported by higher root nitrate reductase (NR) activity (Figure 2B), which reduces NO3− to NO2− during the N assimilation pathway (Heidari et al., 2011). As an alternative reaction, NR may also reduce NO2− to NO (Fancy et al., 2016). In fact, the highest NO accumulation was found in roots under water deficit and supplied with only NO3− (Figure 3B) and it is known that NO3− and NO2− play a key role in NO synthesis through NR (Vanin et al., 2004; Yamasaki, 2005; Sun et al., 2015). In Physcomitrella patens, low NR activity was associated with drastic reductions in NO synthesis, further evidence that NR is an important pathway for NO production in plants (Andrés et al., 2015). It is worth noting that NO synthesis is low under non-limiting conditions, even in plants supplied with only NO3− (Figure 3). In general, increases in NO synthesis are expected under stressful conditions, when NO2− accumulation occurs (Mur et al., 2012). Not only NR is involved in NO synthesis but also a NO-forming nitrite reductase (NOFNiR) found recently in Chlamydomonas reinhardtii. When supplying such algae with NO3−, NO was synthetized through NOFNiR either in vitro or in vivo conditions (Chamizo-Ampudia et al., 2016; Chamizo-Ampudia et al., 2017). As NR would have a significant role in NO synthesis under high NO2− and low NO3− availability (Kolbert et al., 2019), it is reasonable to suggest that NO synthesis could occur through the dual system NR : NOFNiR, a hypothesis to be tested in higher plants.
In the last decades, rapidly increasing evidence has indicated NO as an important player in plant responses to environmental constraining conditions by inducing the antioxidant defenses (Hatamzadeh et al., 2015; Silveira et al., 2017b). During cell detoxification, O2•− is dismuted to H2O2 by superoxide dismutase, which is rapidly eliminated by catalase and ascorbate peroxidase, producing H2O and O2 (Lázaro et al., 2013). Here, we observed higher superoxide dismutase activity in roots under water deficit and supplied with 100% NO3− (Figure 5B), with root [O2•−] remaining similar among treatments (Figure 4B). Interestingly, there was lower O2•− accumulation in leaves under water deficit and supplied with only NO3− (Figure 4A), even with superoxide dismutase showing similar activity to the one found in plants supplied with 70% NO3− and 30% NH4+ (Figure 5A). As a possible explanation, such low leaf [O2•−] may be related to the interaction of this radical with NO, generating peroxynitrite (ONOO−), which in turn can lead to the formation of NO2− and the hydroxyl radical. ONOO− can also add a nitro group to tyrosine residues—a process known as tyrosine nitration (Wullf et al., 2009; Begara-Morales et al., 2014). Although tyrosine nitration was originally considered as indicative of stress conditions, recent evidence suggests its role in cell signaling (Mengel et al., 2013).
Root [H2O2] was lower in plants under water deficit that received 100% NO3− as compared to ones supplied with 70% NO3− and 30% NH4+ (Figure 4D), indicating an efficient detoxification through increased root ascorbate peroxidase activity (Figure 5D). In fact, the activation of antioxidant mechanisms to maintain ROS homeostasis often involves NO (Hatamzadeh et al., 2015; Silveira et al., 2015). Many reports show that exogenous NO improves abiotic stress tolerance, causing decreases in [H2O2] and lipid peroxidation (Gross et al., 2013). Exogenous NO supply inhibits ROS accumulation in many plant species under stress conditions (Verma et al., 2013), such as cucumber and rice under drought (Farooq et al., 2009). Sugarcane plants supplied with GSNO—a NO donor—showed increases in the activity of antioxidant enzymes, such as superoxide dismutase in leaves and catalase in roots under water deficit (Silveira et al., 2017b). In addition, the S-nitrosylation has a role in mediating the interplay between NO and other reactive signaling mechanisms, such as those involving ROS. For instance, S-nitrosylation of RBOHD causes its inactivation, and thus reduces ROS formation through this pathway (Yu et al., 2012). Such findings revealed that NO has an important role in controlling endogenous ROS levels.
Higher superoxide dismutase and ascorbate peroxidase in roots facing water deficit and receiving only NO3− (Figures 5B, D) may be a consequence of S-nitrosylation. In pea (Pisum sativum), S-nitrosylation increased the activity of cytosolic ascorbate peroxidase (Begara-Morales et al., 2014). However, we noticed higher levels of S-nitrosothiols (SNOs) in roots under water deficit and supplied with NO3− and NH4+ (Figure 2F). At this point, one should consider that NO-mediated post-translational modifications on target proteins may be positive or negative (Nabi et al., 2019). Some of these modifications may alter signaling pathways mediated by other ROS (Holzmeister et al., 2014). According to Clark et al. (2000), S-nitrosylation can inhibit catalase activity, which implies that low level of S-nitrosylation can increase catalase activity during stress conditions, thus increasing ROS detoxification. In this way, higher [SNO] found in plants that received less nitrate (Figures 2E, F) is associated with changes in the antioxidant system that lead to increases in leaf [O2•−] and root [H2O2] (Figures 4A, D). It has been proposed that S-nitrosylation can regulate [H2O2] in plants, controlling both the antioxidant defense system and the ROS-producing enzymes (Ortega-Galisteo et al., 2012; Yu et al., 2012).
Here, we found high GSNOR activity in roots under water deficit that received 100% NO3− and low accumulation of SNOs (Figures 2D, F). GSNOR can break down GSNO—a SNO, reducing GSNO levels and consequently decreasing the total cellular level of S-nitrosylation (Feechan et al., 2005). Thus, it indirectly controls the overall SNOs within cells (Feechan et al., 2005), suggesting that GSNOR may be crucial in regulating the cellular SNO pool. In fact, increases in GSNOR activity contributed to the reduction of S-nitrosylation in pea plants under salt stress (Camejo et al., 2013). As GSNO is an NO donor, we can argue that increases in root GSNOR activity under water deficit and supplied with only NO3− (Figure 2D) are related to the reduction of GSNO levels and linked to high NO synthesis in roots (Figure 3B). High levels of reactive nitrogen species (RNS) may be harmful to plants (Nabi et al., 2019), and the absence of GSNOR activity in plants results in a significant increase in levels of SNOs and impairment of plant immunity (Feechan et al., 2005), plant growth, and development (Kwon et al., 2012). Gong et al. (2015) demonstrated that absence of GSNOR activity increased the sensitivity of Solanum lycopersicum to alkaline stress due to the excessive accumulation of NO and SNOs, causing higher levels of endogenous S-nitrosylation and turning stomata insensitive to ABA.
Stomatal closure is the primary response of plants to water deficit, reducing the CO2 supply for photosynthesis and then decreasing biomass production (Machado et al., 2009; Ribeiro et al., 2013). Although water deficit had reduced the stomatal conductance, higher NO3− supply alleviated such negative effects (Figure 6B). Due to higher stomatal conductance, sugarcane plants supplied with 100% NO3− showed an improvement in photosynthesis under water deficit (Figure 6A). By integrating CO2 assimilation throughout the experimental period, plants supplied with only NO3− fixed about 1.5 times more carbon than those supplied with NO3− and NH4+. Such a response was also related to improvement of primary photochemistry, with plants showing higher conversion of light energy into chemical energy at the PSII level (Figure 6C).
Under water deficit, plants supplied with 70% NO3− and 30% NH4+ presented reduced root biomass as compared to those supplied with 100% NO3−, which were not affected by low water availability (Figure 7B). Such increase in root growth was associated with higher NO content (Figure 6B), as found by Silveira et al. (2016). At maximum water deficit, high NO synthesis was found in the root meristematic zone of plants supplied with 100% NO3− (Supplementary Figure S3). Several reports indicate that NO is involved in the regulation of root growth and developmental processes (Correa-Aragunde et al., 2004; Lombardo and Lamattina, 2012; Sun et al., 2015). The root system is able to perceive low water availability and to produce chemical signals that regulate the water flow from roots to shoots. NO is one of those chemical signals that stimulates root expansion and development (Silveira et al., 2016; Xu et al., 2017). Given the effects of NO on root growth, it is reasonable to assume a potential influence of NO mediating auxin signaling in roots. Correa-Aragunde et al. (2006) demonstrated that auxin-dependent cell cycle gene regulation was dependent on NO during lateral root formation in tomato plants. NO also modulates the auxin response during adventitious root formation in cucumber plants (Pagnussat et al., 2002) and Arabidopsis thaliana (Lombardo et al., 2006).
Overall, increases in NO content can trigger root development and improve water uptake, reducing the impact of low water availability on leaf water status and allowing higher stomatal conductance and photosynthesis, as noticed herein and also by Silveira et al. (2017b; 2019b). The novelty here is that we were able to induce NO synthesis in sugarcane plants by changing the nitrogen source. Such a finding has a practical consequence for sugarcane in the field as endogenous NO synthesis can be stimulated by increasing NO3− supply. Apart from economic issues, our data give insights on how stress tolerance can be managed by common practices in agricultural systems and further development on this technique should be carried out with field-grown plants, where interactions among nutrients, soil-root interactions, and soil nitrogen dynamics determine plant performance.
Is Sugarcane Performance Under Water Deficit Really Improved by NO?
Herein, we used 2-(4-carboxyphenyl)-4,4,5,5-tetramethylimidazoline-1-oxyl-3-oxide (cPTIO)—an endogenous NO scavenger (Akaike and Maeda, 1996)—to check if benefits induced by increasing NO3− supply were related to NO. cPTIO drastically reduced the DAF-2DA in plants under water deficit, indicating lower NO accumulation in both leaves and roots (Figures 8A, B). As consequence, plants showed even lower stomatal conductance and net CO2 assimilation rate when compared to plants under water deficit and not supplied with cPTIO (Figures 9A, B). cPTIO sprays also reduced root growth (Figure 9D), as found previously (Figure 7B). Taken together, our data clearly show that the improved performance of sugarcane plants supplied with only NO3− was due to stimulation of NO synthesis under water deficit.
Conclusion
Sugarcane plants grown in nutrient solution containing only NO3− as nitrogen source were more tolerant to water deficit, and this response was associated with increased NO production and high nitrate reductase activity in roots. Herein, increasing NO3− supply was enough to stimulate NO synthesis and alleviate the effects of water deficit on sugarcane plants by increasing the activity of antioxidant enzymes, photosynthesis, stomatal conductance, and root growth. From a broad perspective, our data show that supplying more NO3− during nitrogen fertilization may improve sugarcane tolerance and be beneficial to field-grown sugarcane.
Data Availability Statement
All datasets generated for this study are included in the article/Supplementary Material.
Author Contributions
MDP executed the experiments, analyzed the data, and drafted the manuscript. NS assisted in the execution of biochemical analyzes, data analysis, and revised the manuscript. PP assisted in the analysis of antioxidant metabolism, nitrate reductase, and S-nitrosoglutathione. AS and MTP performed the analysis of S-nitrosothiols and nitrite. EM, LS, and RR edited and revised the manuscript.
Funding
This work was supported by the São Paulo Research Foundation (FAPESP, Grants #2017/11279-7, #2017/05029-8, and #2018/08194-2); the Coordination for the Improvement of Higher Education Personnel (CAPES, Grant Code Codes #001; #88882.317451/2019-01); and the National Council for Scientific and Technological Development (CNPq, Grants #302460/2018-7, #404815/2018-9, #313117/2019-5, and #311345/2019-0).
Conflict of Interest
The authors declare that the research was conducted in the absence of any commercial or financial relationships that could be construed as a potential conflict of interest.
Acknowledgments
The authors are also very grateful to the National Institute of Science and Technology of Photonics applied to Cell Biology (INFABIC) for guiding our analyses with the confocal microscope. This manuscript has been released as a pre-print at bioRxiv (Pissolato et al., 2019b) and contains part of the first author’s Master thesis (Pissolato, 2019).
Supplementary Material
The Supplementary Material for this article can be found online at: https://www.frontiersin.org/articles/10.3389/fpls.2020.00970/full#supplementary-material
References
Akaike, T., Maeda, H. (1996). Quantitation of nitric oxide using 2-phenyl-4, 4, 5, 5-tetramethylimidazoline-1-oxyl 3-oxide (PTIO). Methods Enzymol. 268, 211–221. doi: 10.1016/s0076-6879(96)68023-9
Albertos, P., Romero-Puertas, M. C., Tatematsu, K., Mateos, I., Sánchez-Vicente, I., Nambara, E., et al. (2015). S-nitrosylation triggers ABI5 degradation to promote seed germination and seedling growth. Nat. Commun. 6, 8669. doi: 10.1038/ncomms9669
Alderton, W. K., Cooper, C. E., Knowles, R. G. (2001). Nitric Oxide Synthases: structure, function and inhibition. Biochem. J. 357, 593–615. doi: 10.1042/bj3570593
Alexieva, V., Sergiev, I., Mapelli, S., Karanov, E. (2001). The effect of drought and ultraviolet radiation on growth and stress markers in pea and wheat. Plant Cell Environ. 24, 1337–1344. doi: 10.1046/j.1365-3040.2001.00778.x
Andrés, R. M., Peralta, A. S., Vázquez, J. P. S., Mendivil, S. N., Cabrera, J. A. P., Torres, M. E. S., et al. (2015). The nitric oxide production in the moss Physcomitrella patens is mediated by nitrate reductase. PloS One 10, e0119400. doi: 10.1371/journal.pone.0119400
Arasimowicz-Jelonek, M., Floryszak-Wieczorek, J., Kubiś, J. (2009). Involvement of nitric oxide in water stress-induced responses of cucumber roots. Plant Sci. 177, 682–690. doi: 10.1016/j.plantsci.2009.09.007
Astier, J., Kulik, A., Koen, E., Besson-Bard, A., Bourque, S., Jeandroz, S., et al. (2012). Protein S-nitrosylation: what’s going on in plants? Free Radic. Biol. Med. 53, 1101–1110. doi: 10.1016/j.freeradbiomed.2012.06.032
Barreto, R. F., Cruz, F. J. R., Gaion, L. A., Prado, R. M., Carvalho, R. F. (2018). Accompanying ions of ammonium sources and nitrate: ammonium ratios in tomato plants. J. Plant Nutr. Soil Sci. 181, 382–387. doi: 10.1002/jpln.201700413
Begara-Morales, J. C., Sánchez-Calvo, B., Chaki, M., Valderrama, R., Mata-Pérez, C., Jaramillo-López, J., et al. (2014). Dual regulation of cytosolic ascorbate peroxidase (APX) by tyrosine nitration and S-nitrosylation. J. Exp. Bot. 65, 527–538. doi: 10.1093/jxb/ert396
Bieleski, R. L., Turner, N. A. (1966). Separation and estimation of amino acids in crude plant extracts by thin-layer electrophoresis and chromatography. Anal. Biochem. 17, 278–293. doi: 10.1016/0003-2697(66)90206-5
Bogdan, C. (2015). Nitric oxide synthase in innate and adaptive immunity: an update. Trends Immunol. 36, 161–178. doi: 10.1016/j.it.2015.01.003
Boschiero, B. N., Mariano, E., Azevedo, R. A., Trivelin, P. C. (2019). Influence of nitrate-ammonium ratio on the growth, nutrition, and metabolism of sugarcane. Plant Physiol. Biochem. 139, 246–255. doi: 10.1016/j.plaphy.2019.03.024
Boveris, A. (1984). Determination of the production of superoxide radicals and hydrogen peroxide in mitochondria. Methods Enzymol. 105, 429–435. doi: 10.1016/S0076-6879(84)05060-6
Bradford, M. N. (1976). A rapid and sensitive method for the quantitation of microgram quantities of protein utilizing the principle of protein dye binding. Anal. Biochem. 72, 248–254. doi: 10.1016/0003-2697(76)90527-3
Bright, J., Hiscoc, S. J., James, P. E., Hancock, J. T. (2009). Pollen generates nitric oxide and nitrite: a possible link to pollen-induced allergic responses. Plant Physiol. Biochem. 47, 49–55. doi: 10.1016/j.plaphy.2008.09.005
Cánovas, D., Marcos, J. F., Marcos, A. T., Strauss, J. (2016). Nitric oxide in fungi: is there NO light at the end of the tunnel? Curr. Genet. 62, 513–518. doi: 10.1007/s00294-016-0574-6
Cai, W., Liu, W., Wang, W. S., Fu, Z. W., Han, T. T., Lu, Y. T. (2015). Overexpression of rat neurons nitric oxide synthase in rice enhances drought and salt tolerance. PloS One 10, e0131599. doi: 10.1371/journal.pone.0131599
Cakmak, I., Horst, W. J. (1991). Effect of aluminium on lipid peroxidation, superoxide dismutase, catalase, and peroxidase activities in root tips of soybean (Glycine max). Physiol. Plant 83, 463–468. doi: 10.1111/j.1399-3054.1991.tb00121.x
Cambraia, J., Pimenta, J. A., Estevao, M. M., Sant’Anna, R. (1989). Aluminum effects on nitrate uptake and reduction in sorghum. J. Plant Nutr. 12, 1435–1445. doi: 10.1080/01904168909364048
Camejo, D., Romero-Puertas, M., Rodríguez-Serrano, M., Sandalio, L. M., Lázaro, J. J., Jiménez, A., et al. (2013). Salinity-induced changes in S-nitrosylation of pea mitochondrial proteins. J. Proteomics 79, 87–99. doi: 10.1016/j.jprot.2012.12.003
Cataldo, J. M., Haroom, M., Schrader, L. E., Youngs, V. L. (1975). Rapid calorimetric determination of nitrate in plant tissue by nitration of salicylic acid. Commun. Soil Sci. Plan. 6, 71–80. doi: 10.1080/00103627509366547
Chamizo-Ampudia, A., Sanz-Luque, E., Llamas, A., Ocaña-Calahorro, F., Mariscal, V., Carreras, A., et al. (2016). A dual system formed by the ARC and NR molybdoenzymes mediates nitrite-dependent NO production in Chlamydomonas. Plant Cell Environ. 10, 2097–2107. doi: 10.1111/pce.12739
Chamizo-Ampudia, A., Sanz-Luque, E., Llamas, A., Galvan, A., Fernandez, E. (2017). Nitrate reductase regulates plant nitric oxide homeostasis. Trends Plant Sci. 22, 163–174. doi: 10.1016/j.tplants.2016.12.001
Clark, D., Durner, J., Navarre, D. A., Klessig, D. F. (2000). Nitric oxide inhibition of tobacco catalase and ascorbate peroxidase. Mol. Plant Microbe Interact. 13, 1380–1384. doi: 10.1094/MPMI.2000.13.12.1380
Correa-Aragunde, N., Graziano, M., Lamattina, L. (2004). Nitric oxide plays a central role in determining lateral root development in tomato. Planta 218, 900–905. doi: 10.1007/s00425-003-1172-7
Correa-Aragunde, N., Graziano, M., Chevalier, C., Lamattina, L. (2006). Nitric oxide modulates the expression of cell cycle regulatory genes during lateral root formation in tomato. J. Exp. Bot. 57, 581–588. doi: 10.1093/jxb/erj045
Crane, B. R., Sudhamsu, J., Patel, B. A. (2010). Bacterial nitric oxide synthases. Annu. Rev. Biochem. 79, 445–470. doi: 10.1146/annurev-biochem-062608-103436
De Armas, R., Valadier, M. H., Champigny, M. L., Lamaze, T. (1992). Influence of ammonium and nitrate on the growth and photosynthesis of sugarcane. J. Plant Physiol. 140, 531–535. doi: 10.1016/S0176-1617(11)80783-2
Degl’Innocenti, E., Guide, L., Soldatini, G. F. (2002). Effect of chronic O3 fumigation on the activity of some Calvin cycle enzymes in two poplar clones. Photosynthetica 40, 121–126. doi: 10.1023/A:1020127231198
Desikan, R., Griffiths, R., Hancock, J. T., Neill, S. (2002). A new role for an old enzyme: nitrate reductase-mediated nitric oxide generation is required for abscisic acidinduced stomatal closure in Arabidopsis thaliana. Proc. Natl. Acad. Sci. U.S.A. 99, 16314–16318. doi: 10.1073/pnas.252461999
Esteban, R., Ariz, I., Cruz, C., Moran, J. F. (2016). Mechanisms of ammonium toxicity and the quest for tolerance. Plant Sci. 248, 92–101. doi: 10.1016/j.plantsci.2016.04.008
Fancy, N. N., Bahlmann, A. K., Loake, G. J. (2016). Nitric oxide function in plant abiotic stress. Plant Cell Environ. 40, 462–472. doi: 10.1111/pce.12707
Farooq, M., Basra, S. M. A., Wahid, A., Rehman, H. (2009). Exogenously applied nitric oxide enhances the drought tolerance in fine grain aromatic rice (Oryza sativa L.). J. Agro. Crop Sci. 195, 254–261. doi: 10.1111/j.1439-037X.2009.00367.x
Fatma, M., Masood, A., Per, T. S., Khan, N. A. (2016). Nitric oxide alleviates salt stress inhibited photosynthetic response by interacting with sulfur assimilation in mustard. Front. Plant Sci. 7, 521. doi: 10.3389/fpls.2016.00521
Feechan, A., Kwon, E., Yuri, B., Wang, Y., Pallas, J., Loake, G. (2005). A central role for S-nitrosothiols in plant disease resistance. Proc. Natl. Acad. Sci. USA 102, 8054–8059. doi: 10.1073/pnas.0501456102
Fernandez-Marcos, M., Sanz, L., Lewis, D. R., Muday, G. K., Lorenzo, O. (2011). Nitric oxide causes root apical meristem defects and growth inhibition while reducing PIN-FORMED1 (PIN1)-dependent acropetal auxin transport. Proc. Natl. Acad. Sci. U.S.A. 108, 18506–18511. doi: 10.1073/pnas.1108644108
García-Mata, C., Lamattina, L. (2001). Nitric oxide induces stomatal closure and enhances the adaptive plant responses against drought stress. Plant Physiol. 126, 1196–1204. doi: 10.1104/pp.126.3.1196
Giannopolitis, C. N., Ries, S. K. (1977). Superoxide dismutase: Occurrence in higher plants. Plant Physiol. 59, 309–314. doi: 10.1104/pp.59.2.309
Gong, B., Wen, D., Wang, X., Wei, M., Yang, F., Li, Y., et al. (2015). S-nitrosoglutathione reductase modulated redox signaling controls sodic alkaline stress responses in Solanum lycopersicum L. Plant Cell Physiol. 1, 790–802. doi: 10.1093/pcp/pcv007
Gross, F., Durner, J., Gaupels, F. (2013). Nitric oxide, antioxidants and prooxidants in plant defence responses. Front. Plant Sci. 4, 419. doi: 10.3389/fpls.2013.00419
Gupta, K. J., Fernie, A. R., Kaiser, W. M., Dongen, J. T. V. (2011). On the origins of nitric oxide. Trends Plant Sci. 16, 160–168. doi: 10.1016/j.tplants.2010.11.007
Hageman, R. H., Reed, A. J. (1980). Nitrate reductase from higher plants. Methods Enzymol. 69, 270–280. doi: 10.1016/S0076-6879(80)69026-0
Hancock, J. T., Neill, S. J. (2019). Nitric Oxide: Its generation and interactions with other reactive signaling compounds. Plants 8, 41. doi: 10.3390/plants8020041
Hatamzadeh, A., Nalousi, A. M., Ghasemnezhad, M., Biglouei, M. H. (2015). The potential of nitric oxide for reducing oxidative damage induced by drought stress in two turf grass species, creeping bent grass and tall fescue. Grass Forage Sci. 70, 538–548. doi: 10.1111/gfs.12135
Havir, E. A., McHale, N. A. (1987). Biochemical and developmental characterization of multiple forms of catalase in tobacco leaves. Plant Physiol. 84, 450–455. doi: 10.1104/pp.84.2.450
He, Y., Tang, R. H., Hao, Y., Stevens, R. D., Cook, C. W., Ahn, S. M., et al. (2004). Nitric oxide represses the Arabidopsis floral transition. Science 305, 1968–1971. doi: 10.1126/science.1098837
Heath, R. L., Packer, L. (1968). Photoperoxidation in isolated chloroplast. I. kinetics and stoichiometry of fatty acid peroxidation. Arch. Biochem. Biophys. 125, 189–198. doi: 10.1016/0003-9861(68)90654-1
Heidari, B., Matre, P., Nemie-Feyissa, D., Meyer, C., Rognli, O. A., Moller, S. G., et al. (2011). Protein phosphatase 2A B55 and A regulatory subunits interact with nitrate reductase and are essential for nitrate reductase activation. Plant Physiol. 156, 165–172. doi: 10.1104/pp.111.172734
Holzmeister, C., Gaupels, F., Geerlof, A., Sarioglu, H., Sattler, M., Durner, J., et al. (2014). Differential inhibition of Arabidopsis superoxide dismutases by peroxynitrite-mediated tyrosine nitration. J. Exp. Bot. 66, 989–999. doi: 10.1093/jxb/eru458
Jahnová, J., Luhová, L., Petřivalský, M. (2019). S-nitrosoglutathione reductase—the master regulator of protein S-nitrosation in plant no signaling. Plants 8, 48. doi: 10.3390/plants8020048
Jamaux, I., Steinmetz, A., Belhassen, E. (1997). Looking for molecular and physiological markers of osmotic adjustment in sunflower. New Phytol. 137, 117–127. doi: 10.1046/j.1469-8137.1997.00817.x
Kolbert, Z., Barroso, J. B., Brouquisse, R., Corpas, F. J., Gupta, K. J., Lindermayr, C., et al. (2019). A forty-year journey: The generation and roles of NO in plants. Nitric. Oxide 93, 53–70. doi: 10.1016/j.niox.2019.09.006
Kwon, E., Feechan, A., Yun, B. W., Hwang, B. H., Pallas, J. A., Kang, J. G., et al. (2012). AtGSNOR1 function is required for multiple developmental programs in Arabidopsis. Planta 236, 887–900. doi: 10.1007/s00425-012-1697-8
Lázaro, J. J., Jiménez, A., Camejo, D., Martí, M. C., Lázaro-Payo, A., Barranco-Medina, S., et al. (2013). Dissecting the integrative antioxidant and redox systems in plant mitochondria. Effect of stress and S-nitrosylation. Front. Plant Sci. 4, 460. doi: 10.3389/fpls.2013.00460
Laemmli, U. K. (1970). Cleavage of structural proteins during the assembly of the head of bacteriophage T4. Nature 227, 680–685. doi: 10.1038/227680a0
León, J., Costa-Broseta, Á. (2019). Present knowledge and controversies, deficiencies, and misconceptions on nitric oxide synthesis, sensing, and signaling in plants. Plant Cell Environ. 43, 1–15. doi: 10.1111/pce.13617
Lombardo, M. C., Lamattina, L. (2012). Nitric oxide is essential for vesicle formation and trafficking in Arabidopsis root hair growth. J. Exp. Bot. 63, 4875–4885. doi: 10.1093/jxb/ers166
Lombardo, M. C., Graziano, M., Polacco, J. C., Lamattina, L. (2006). Nitric oxide functions as a positive regulator of root hair development. Plant Signal. Behav. 1, 28–33. doi: 10.4161/psb.1.1.2398
Machado, R. S., Ribeiro, R. V., Marchiori, P. E. R., Machado, D. F. S. P., Machado, E. C., Landell, M. G. D. A. (2009). Biometric and physiological responses to water deficit in sugarcane at different phenological stages. Pesq. Agropec. Bras. 44, 1575–1582. doi: 10.1590/S0100-204X2009001200003
Malagoli, M., Canal, A. D., Quaggiotti, S., Pegoraro, P., Bottacin, A. (2000). Differences in nitrate and ammonium uptake between Scots pine and European larch. Plant Soil 221, 1–3. doi: 10.1023/A:1004720002898
McCullough, H. (1967). The determination of ammonia in whole blood by a direct colorimetric method. Clin. Chim. Acta 17, 297–304. doi: 10.1016/0009-8981(67)90133-7
Mengel, A., Chaki, M., Shekariesfahlan, A., Lidermayr, C. (2013). Effect of nitric oxide on gene transcription—S-nitrosylation of nuclear proteins. Front. Plant Sci. 4, 293. doi: 10.3389/fpls.2013.00293
Meyer, J. H., Schumann, A. W., Wood, R. A., Nixon, D. J., Berg, M. V. D. (2007). Recent advances to improve nitrogen use efficiency of sugarcane in the South African sugar industry. Proc. Int. Soc Sugar Cane Technol. 26, 238–246.
Mohammadi, M., Karr, A. L. (2001). Superoxide anion generation in effective and ineffective soybean root nodules. J. Plant Physiol. 158, 1023–1029. doi: 10.1078/S0176-1617(04)70126-1
Moreau, M., Lindermayr, C., Durner, J., Klessig, D. (2010). NO synthesis and signaling in plants—where do we stand? Physiol. Plant 138, 372–383. doi: 10.1111/j.1399-3054.2009.01308.x
Mur, L. A., Sivakumaran, A., Mandon, J., Cristescu, S. M., Harren, F. J., Hebelstrup, K. H. (2012). Haemoglobin modulates salicylate and jasmonate/ethylene-mediated resistance mechanisms against pathogens. J. Exp. Bot. 63, 4375–4387. doi: 10.1093/jxb/ers116
Mur, L. A., Mandon, J., Persijn, S., Cristescu, S. M., Moshkov, I. E., Novikova, G. V., et al. (2013). Nitric oxide in plants: an assessment of the current state of knowledge. AoB Plants 5, pls052. doi: 10.1093/aobpla/pls052
Nabi, R. B. S., Tayade, R., Hussain, A., Kulkarni, K. P., Imran, Q. M., Mun, B. G., et al. (2019). Nitric oxide regulates plant responses to drought, salinity, and heavy metal stress. Environ. Exp. Bot. 161, 120–133. doi: 10.1016/j.envexpbot.2019.02.003
Nakano, Y., Asada, K. (1981). Hydrogen peroxide is scavenged by ascorbate-specific peroxidases in spinach chloroplasts. Plant Cell Physiol. 22, 867–880. doi: 10.1093/oxfordjournals.pcp.a076232
Ortega-Galisteo, A. P., Serrano, M. R., Pazmiño, D. M., Gupta, D. K., Sandalio, L. M., Puertas, M. C. R. (2012). S-Nitrosylated protein sinpea (Pisum sativum L.) leaf peroxisomes: changes under abiotic stress. J. Exp. Bot. 63, 2089–2103. doi: 10.1093/jxb/err414
Pagnussat, G. C., Simontacchi, M., Puntarulo, S., Lamattina, L. (2002). Nitric oxide is required for root organogenesis. Plant Physiol. 129, 954–956. doi: 10.1104/pp.004036
Peixoto, P. H. P., Cambraia, J., Sant’ana, R., Mosquim, P. R., Moreira, M. A. (1999). Aluminum effects on lipid peroxidation and on activities of enzymes of oxidative metabolism in sorghum. Braz. J. Plant Physiol. 11, 137–143.
Pissolato, M. D., Silveira, N. M., Machado, E. C., Zambrosi, F. C. B., Sodek, L., Ribeiro, R. V. (2019a). Photosynthesis and biomass accumulation in young sugarcane plants grown under increasing ammonium supply in nutrient solution. Theor. Exp. Plant Physiol. 31, 401–411. doi: 10.1007/s40626-019-00154-w
Pissolato, M. D., Silveira, N. M., Prataviera, P. J., Machado, E. C., Seabra, A. B., Pelegrino, M. T., et al. (2019b). Enhanced nitric oxide synthesis through nitrate supply improves drought tolerance of sugarcane plants. bioRxiv. doi: 10.1101/860544
Pissolato, M. D. (2019). Alleviating the effects of water deficit on sugarcane through nitrogen nutrition (Master’s thesis. Campinas (SP): University of Campinas).
Raftery, A. E. (1995). “Bayesian model selection in social research,” in Sociological Methodology. Ed. Marsden, P. V. (Cambridge: Blackwell), 111–196.
Reid, C. D., Tissue, D. T., Fiscus, E. L., Strain, B. R. (1997). Comparison of spectrophotometric and radio isotopic methods for the assay of Rubisco in ozone-treated plants. Physiol. Plant 101, 398–404. doi: 10.1111/j.1399-3054.1997.tb01014.x
Ribeiro, R. V., Machado, R. S., Machado, E. C., Machado, D. F. S. P., Magalhães Filho, J. R., Landell, M. G. A. (2013). Revealing drought-resistance and productive patterns in sugarcane genotypes by evaluating both physiological responses and stalk yield. Exp. Agric. 49, 212–224. doi: 10.1017/S0014479712001263
Rios-Gonzalez, K., Erdei, L., Lips, S. H. (2002). The activity of antioxidant enzymes in maize and sunflower seedlings as affected by salinity and different nitrogen sources. Plant Sci. 162, 923–930. doi: 10.1016/S0168-9452(02)00040-7
Robinson, N., Brackin, R., Vinall, K., Soper, F., Holst, J., Gamage, H., et al. (2011). Nitrate paradigm does not hold up for sugarcane. PloS One 6, e19045. doi: 10.1371/journal.pone.0019045
Rockel, P., Strube, F., Rockel, A., Wildt, J., Kaiser, W. M. (2002). Regulation of nitric oxide (NO) production by plant nitrate reductase in vivo and in vitro. J. Exp. Bot. 53, 103–110. doi: 10.1093/jexbot/53.366.103
Rodríguez-Ruiz, M., Mioto, P., Palma, J. M., Corpas, F. J. (2017). S-nitrosoglutathione reductase (GSNOR) activity is down-regulated during pepper (Capsicum annuum L.) fruit ripening. Nitric. Oxide 68, 51–55. doi: 10.1016/j.niox.2016.12.011
Sage, R. F., Sharkey, T. D., Seemann, J. R. (1988). The in vivo response of the ribulose-1,5-bisphosphate carboxylase activation state and the pool sizes of photosynthetic metabolites to elevated CO2 in Phaseolus vulgaris L. Planta 174, 407–416. doi: 10.1007/BF00959528
Santolini, J., André, F., Jeandroz, S., Wendehenne, D. (2017). Nitric oxide synthase in plants: Where do we stand? Nitric. Oxide 63, 30–38. doi: 10.1016/j.niox.2016.09.005
Santos, M. C., Seabra, A. B., Pelegrino, M. T., Haddad, P. S. (2016). Synthesis, characterization and cytotoxicity of glutathione-and PEG-glutathione-superparamagnetic iron oxide nanoparticles for nitric oxide delivery. Appl. Surf. Sci. 367, 26–35. doi: 10.1016/j.apsusc.2016.01.039
Shan, C., Zhou, Y., Liu, M. (2015). Nitric oxide participates in the regulation of the ascorbate-glutathione cycle by exogenous jasmonic acid in the leaves of wheat seedlings under drought stress. Protoplasma 252, 1397–1405. doi: 10.1007/s00709-015-0756-y
Shi, H., Ye, T., Zhu, J. K., Chan, Z. (2014). Constitutive production of nitric oxide leads to enhanced drought stress resistance and extensive transcriptional reprogramming in Arabidopsis. J. Exp. Bot. 65, 4119–4131. doi: 10.1093/jxb/eru184
Silveira, N. M., Oliveira, J. A. D., Ribeiro, C., Canatto, R. A., Siman, L., Cambraia, J., et al. (2015). Nitric oxide attenuates oxidative stress induced by arsenic in lettuce (Lactuca sativa) leaves. Water Air Soil Poll 226, 379. doi: 10.1007/s11270-015-2630-0
Silveira, N. M., Frungillo, L., Marcos, F. C., Pelegrino, M. T., Miranda, M. T., Seabra, A. B., et al. (2016). Exogenous nitric oxide improves sugarcane growth and photosynthesis under water deficit. Planta 244, 181–190. doi: 10.1007/s00425-016-2501-y
Silveira, N. M., Hancock, J. T., Frungillo, L., Siasou, E., Marcos, F. C., Salgado, I., et al. (2017a). Evidence towards the involvement of nitric oxide in drought tolerance of sugarcane. Plant Physiol. Biochem. 115, 354–359. doi: 10.1016/j.plaphy.2017.04.011
Silveira, N. M., Marcos, F. C., Frungillo, L., Moura, B. B., Seabra, A. B., Salgado, I., et al. (2017b). S-nitrosoglutathione spraying improves stomatal conductance, Rubisco activity and antioxidant defense in both leaves and roots of sugarcane plants under water deficit. Physiol. Plant 160, 383–395. doi: 10.1111/ppl.12575
Silveira, N. M., Machado, E. C., Ribeiro, R. V. (2019a). “Extracellular and intracellular NO detection in plants by diaminofluoresceins,” in Redox-mediated signal transduction: methods and protocols, methods in molecular biology. Eds. Hancock, J. T., Conway, M. (New York: Springer), 103–108.
Silveira, N. M., Seabra, A. B., Marcos, F. C., Pelegrino, M. T., Machado, E. C., Ribeiro, R. V. (2019b). Encapsulation of S-nitrosoglutathione into chitosan nanoparticles improves drought tolerance of sugarcane plants. Nitric. Oxide 84, 38–44. doi: 10.1016/j.niox.2019.01.004
Sun, H., Li, J., Song, W., Tao, J., Huang, S., Chen, S., et al. (2015). Nitric oxide generated by nitrate reductase increases nitrogen uptake capacity by inducing lateral root formation and inorganic nitrogen uptake under partial nitrate nutrition in rice. J. Exp. Bot. 66, 2449–2459. doi: 10.1093/jxb/erv030
Towbin, H., Staehelin, T., Gordon, J. (1979). Electrophoretic transfer of proteins from polyacrylamide gels to nitrocellulose sheets: procedure and some applications. Proc. Natl. Acad. Sci. USA 76, 4350–4356. doi: 10.1073/pnas.76.9.4350
Vanin, A. F., Svistunenko, D. A., Mikoyan, V. D., Serezhenkov, V. A., Fryer, M. J., Baker, N. R., et al. (2004). Endogenous superoxide production and the nitrite/nitrate ratio control the concentration of bioavailable free nitric oxide in leaves. J. Biol. Chem. 279, 24100–24107. doi: 10.1074/jbc.M312601200
Verma, K., Mehta, S. K., Shekhawat, G. S. (2013). Nitric oxide (NO) counteracts cadmium induced cytotoxic processes mediated by reactive oxygen species (ROS) in Brassica juncea: cross-talk between ROS, NO and antioxidant responses. Biometals 26, 255–269. doi: 10.1007/s10534-013-9608-4
Wang, L., Macko, S. A. (2011). Constrained preferences in nitrogen uptake across plant species and environments. Plant Cell Environ. 34, 525–534. doi: 10.1111/j.1365-3040.2010.02260.x
Wang, P., Du, Y., Hou, Y. J., Zhao, Y., Hsu, C. C., Yuan, F., et al. (2015). Nitric oxide negatively regulates abscisic acid signaling in guard cells by S-nitrosylation of OST1. Proc. Natl. Acad. Sci. USA 112, 613–618. doi: 10.1073/pnas.1423481112
Wullf, A., Oliveira, H. C., Saviani, E. E., Salgado, I. (2009). Nitrite reduction and superoxide-dependent nitric oxide degradation by Arabidopsis mitochondria: influence of external NAD(P)H dehydrogenases and alternative oxidase in the control of nitric oxide levels. Nitric. Oxide 21, 132–139. doi: 10.1016/j.niox.2009.06.003
Xu, X. T., Jin, X., Liao, W. B., Dawuda, M. M., Li, X. P., Wang, M., et al. (2017). Nitric oxide is involved in ethylene-induced adventitious root development in cucumber (Cucumis sativus L.) explants. Sci. Hortic. 215, 65–71. doi: 10.1016/j.scienta.2016.12.006
Yamasaki, H., Sakihama, Y., Takahashi, S. (1999). An alternative pathway for nitric oxide production in plants: new features of an old enzyme. Trends Plant Sci. 4, 128–129. doi: 10.1016/S1360-1385(99)01393-X
Yamasaki, H. (2005). The NO world for plants: achieving balance in an open system. Plant Cell Environ. 28, 78–84. doi: 10.1111/j.1365-3040.2005.01297.x
Yu, M., Yun, B. W., Spoel, S. H., Loake, G. J. (2012). A sleigh ride through the SNO: Regulation of plant immune function by protein S-nitrosylation. Curr. Opin. Plant Biol. 15, 424–430. doi: 10.1016/j.pbi.2012.03.005
Keywords: nitrate reductase, nitrite, photosynthesis, reactive oxygen species, S-nitrosylation
Citation: Pissolato MD, Silveira NM, Prataviera PJC, Machado EC, Seabra AB, Pelegrino MT, Sodek L and Ribeiro RV (2020) Enhanced Nitric Oxide Synthesis Through Nitrate Supply Improves Drought Tolerance of Sugarcane Plants. Front. Plant Sci. 11:970. doi: 10.3389/fpls.2020.00970
Received: 17 February 2020; Accepted: 15 June 2020;
Published: 30 June 2020.
Edited by:
Juan Carlos Begara-Morales, University of Jaén, SpainReviewed by:
Fábio M. DaMatta, Universidade Federal de Viçosa, BrazilByung-Wook Yun, Kyungpook National University, South Korea
Copyright © 2020 Pissolato, Silveira, Prataviera, Machado, Seabra, Pelegrino, Sodek and Ribeiro. This is an open-access article distributed under the terms of the Creative Commons Attribution License (CC BY). The use, distribution or reproduction in other forums is permitted, provided the original author(s) and the copyright owner(s) are credited and that the original publication in this journal is cited, in accordance with accepted academic practice. No use, distribution or reproduction is permitted which does not comply with these terms.
*Correspondence: Rafael V. Ribeiro, cnZyQHVuaWNhbXAuYnI=