- Crop Genome Engineering, Applied Science and Technology, Corteva Agriscience, Johnston, IA, United States
Use of the morphogenic genes Baby Boom (Bbm) and Wuschel2 (Wus2), along with new ternary constructs, has increased the genotype range and the type of explants that can be used for maize transformation. Further optimizing the expression pattern for Bbm/Wus2 has resulted in rapid maize transformation methods that are faster and applicable to a broader range of inbreds. However, expression of Bbm/Wus2 can compromise the quality of regenerated plants, leading to sterility. We reasoned excising morphogenic genes after transformation but before regeneration would increase production of fertile T0 plants. We developed a method that uses an inducible site-specific recombinase (Cre) to excise morphogenic genes. The use of developmentally regulated promoters, such as Ole, Glb1, End2, and Ltp2, to drive Cre enabled excision of morphogenic genes in early embryo development and produced excised events at a rate of 25–100%. A different strategy utilizing an excision-activated selectable marker produced excised events at a rate of 53–68%; however, the transformation frequency was lower (13–50%). The use of inducible heat shock promoters (e.g. Hsp17.7, Hsp26) to express Cre, along with improvements in tissue culture conditions and construct design, resulted in high frequencies of T0 transformation (29–69%), excision (50–97%), usable quality events (4–15%), and few escapes (non-transgenic; 14–17%) in three elite maize inbreds. Transgenic events produced by this method are free of morphogenic and marker genes.
Introduction
The use of the morphogenic genes Bbm and Wus2 significantly increased transformation frequencies and reduced genotype dependence in many cereal crops (Lowe et al., 2016; Mookkan et al., 2017; Anand et al., 2018; Lowe et al., 2018). Morphogenic genes have enabled the development of a rapid transformation method involving direct formation of somatic embryos and T0 plants from immature scutella (Lowe et al., 2018). This approach has facilitated transformation (Lowe et al., 2016; Mookkan et al., 2017) and CRISPR/Cas-mediated editing (Chilcoat et al., 2017) in numerous elite maize inbreds, and enabled use of alternate explants, such as embryo slices from mature seeds or leaf segments (Lowe et al., 2016; Lowe et al., 2018). However, ectopic expression of the morphogenic genes causes pleiotropic effects including abnormal shoots/roots and infertile plants (Lowe et al., 2016). The use of promoters that drive high expression levels during the transformation process, but lower expression levels in the vegetative plant, somewhat ameliorates these problems (Lowe et al., 2018) but some negative effect and the presence of morphogenic genes is undesirable in commercial products. Transgenic plants regenerated through de novo meristem induction stimulated by morphogenic gene expression also showed developmental abnormalities (Maher et al., 2020), and without removal also raise concerns that non-visible pleiotropic effects are possible. Therefore, it is desirable to generate transgenic plants free from morphogenic genes. Previously, a method using a non-integrating Wus2 gene approach recovered fertile T0 plants free-off morphogenic genes, however this method needed a plant selectable marker gene (SMG) for regenerating events (Hoerster et al., 2020). Here we report an approach that allows excision of both the morphogenic gene and the SMG used in transformation at the same time. As an added benefit this method eliminates any adverse effect from the non-trait (e.g. SMGs) genes in commercial products.
Several strategies have been developed to remove SMGs following plant transformation. One approach is co-transformation with two constructs, one with the SMG and one with the gene of interest. In a transgenic plant with independent insertions of each of these constructs, the selectable marker can be segregated genetically (Hare and Chua, 2002; Puchta, 2003; Darbani et al., 2007; Ling et al., 2016). Alternatively, SMGs can be removed by excision via homologous recombination (Puchta, 2000; Zubko et al., 2000), elimination by transposition (Maeser and Kahmann, 1991; Gao et al., 2015) or, by the use of recombinases to excise unwanted DNA. Several recombination systems have been used to excise SMGs, including Cre/lox from bacteriophage P1 (Hoess et al., 1982; Hoess and Abremski, 1985), Flp/frt from Saccharomyces cerevisiae (Cox, 1983; Senecoff et al., 1985), R/RS from Zygosaccharomyces rouxii (Araki et al., 1985), and Gin/gix from bacteriophage (Klippel et al., 1988). Recombinases have been delivered via retransformation (Odell et al., 1990; Dale and Ow, 1991), sexual crosses (Bayley et al., 1992; Kilby et al., 1995; Kerbach et al., 2005), or transient expression (Gleave et al., 1999; Kopertekh et al., 2004; Kopertekh and Schiemann, 2005; Jia et al., 2006). In most of these systems, SMG removal takes place after the T0 generation and requires screening multiple plants. An alternative method is to induce excision prior to T0 regeneration. One approach is to design a construct containing SMG and the recombinase genes that are flanked by recombination sites. This has been referred to as “auto-excision” (Verweire et al., 2007; Moravčíková et al., 2008), and allows generation of SMG-free events. Placing the recombinase under the regulation of an inducible/chemical promoter, an expression system that allowed spatial and temporal control (regulated by external or intrinsic signals) was shown to be faster and less resource-intensive (Chong-Pérez and Angenon, 2013; Yau and Stewart, 2013).
We evaluated different strategies for auto-excision prior to regeneration to recover stable T0 plants free of morphogenic genes and in some cases the SMG as well: 1) an auto-excision system using developmentally regulated promoters, 2) an excision-activated marker gene system, and 3) an auto-excision system using an inducible promoter. These excision strategies were evaluated for 1) high transformation frequency, 2) quality event (QE, single-copy of T-DNA, backbone and morphogenic gene free) frequency, 3) ability to generate marker-free T0 plants, and 4) applicability to multiple elite maize inbreds. The use of developmentally regulated promoters driving Cre enabled auto-excision of morphogenic genes but resulted in low transformation frequency and QE recovery. These limitations were addressed using heat-shock inducible promoters driving expression of Cre, that resulted in higher frequencies of T0 transformation, gene-excision and QE recovery.
Materials and Methods
Plant Material
Pioneer temperate maize inbreds (HC69, PH2RT, PH85E, and PH84Z) were used in this study. All plants used for source immature embryos were grown in the greenhouse. One of the inbred lines (HC69) is nonproprietary and publicly available. The other three inbred lines described here are proprietary (PH2RT, PH85E, and PH84Z). In order to protect Corteva Agriscience proprietary germplasm, such germplasm will not be made available except at the discretion of Corteva Agriscience and then only in accordance with all applicable governmental regulations.
Donor Material and Tissue Culture
Seeds were germinated and grown in a greenhouse at temperature set-points of 25.5/20.0°C (day/night), and 16 h daylight. After 21 d, seedlings were transplanted into 5.9 L pots containing a soil-less substrate composed of 38% Canadian sphagnum peat, 51% composted bark, 8% perlite, and 3% vermiculite by volume and adjusted with lime to a pH of 6.0. Maize ears from the Pioneer inbred lines HC69, PH2RT, PH84Z, and PH85E were collected from the greenhouse (Johnston, Iowa) at 10 to 11 d after pollination, when the immature embryos were 1.5–2.0 mm in length. Ears were sterilized with 20% Clorox (final sodium hypochlorite concentration of 1.65%) for 15 min and rinsed three times with sterile distilled water.
Culture Media Used for Transformations and Plant Regeneration
Briefly, maize immature embryos (1.5–2 mm) were harvested and used for Agrobacterium-mediated transformation, using the media, selection and regeneration methods described previously (Lowe et al., 2018; Chu et al., 2019; Hoerster et al., 2020). For selection, 0.1 mg/L imazapyr was supplemented to somatic embryo formation medium or 150 mg/L G418 was substituted for imazapyr.
Agrobacterium-Mediated Transformation
Constructs used in these experiments are illustrated in Figures 1–3 and the individual expression components such as promoters, structural genes and terminators are listed in Table S1. The materials reported in this article contain selectable markers (HRA and NPTII) and reporter genes (ZS-Green and Zs-Yellow) owned by third parties. Authors may not be able to provide materials including third party genetic elements to the requestor because of certain third-party contractual restrictions placed on the author’s institution. In such cases, the requester will be required to obtain such materials directly from the third party. The authors and authors’ institution do not make any express or implied permission(s) to the requester to make, use, sell, offer for sale, or import third party proprietary materials.
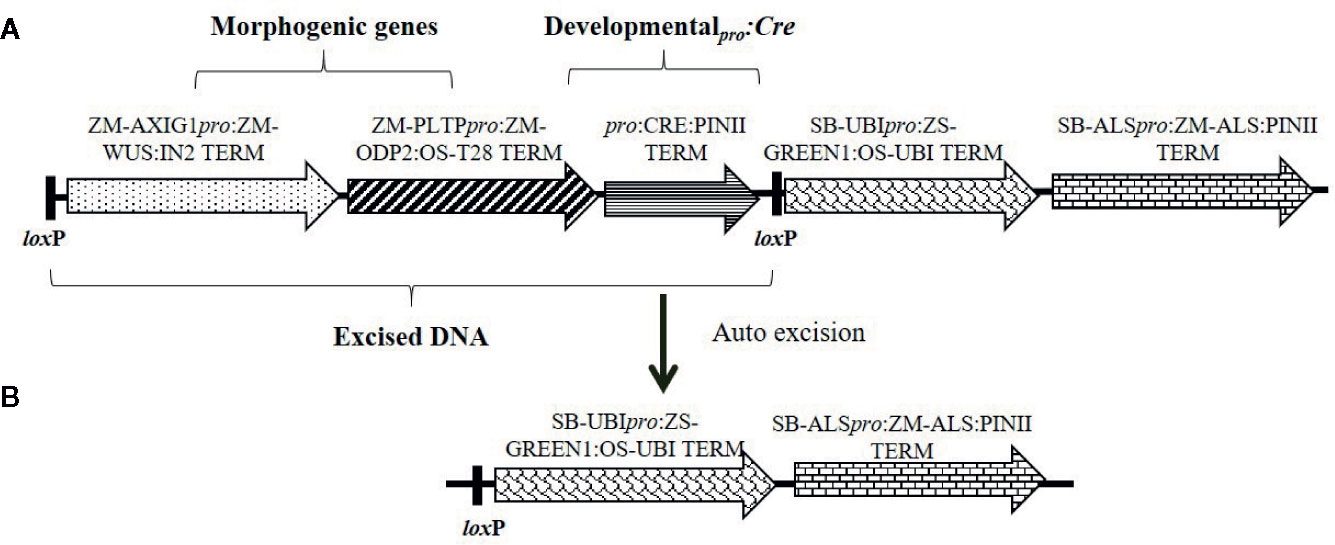
Figure 1 Schematic representation of an auto-excision construct design used for testing different developmentally regulated or stress-inducible promoters to achieve excision of morphogenic genes. (A) The excision construct with different promoter combinations driving Cre expression (represented by pro:CRE) and the DNA fragment to be excised flanked by two directly oriented loxP recombination sites. (B) The excised product following auto-excision. Refer to Table S-1 for description of construct components used in T-DNA construction.
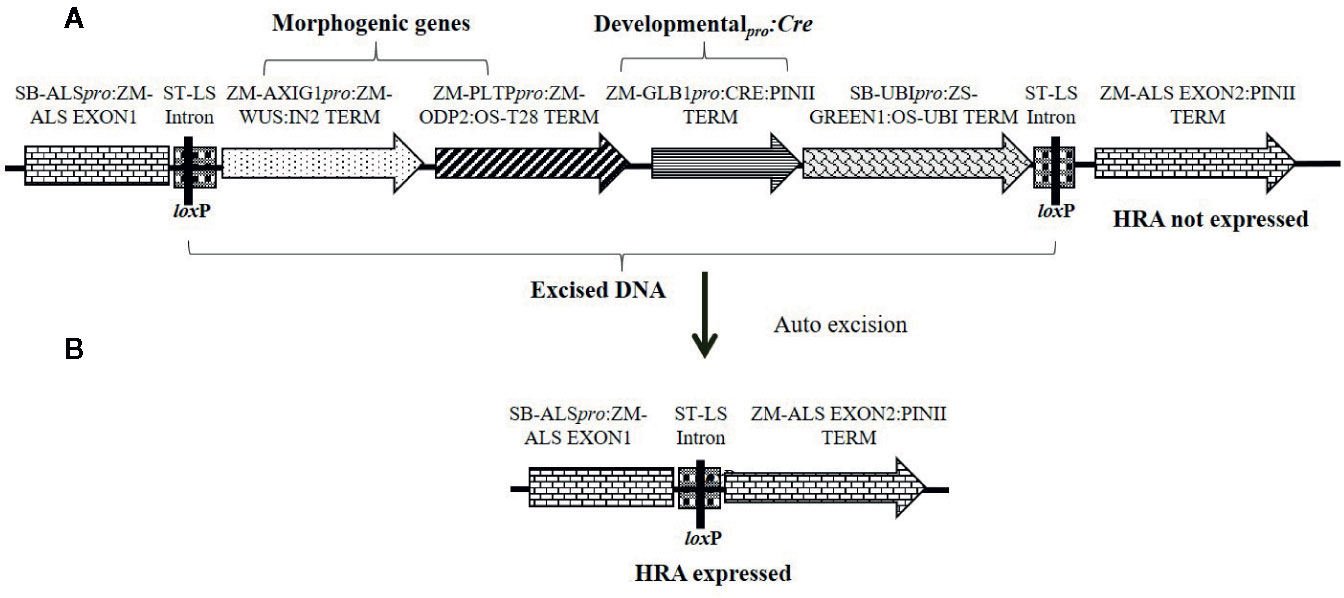
Figure 2 Schematic representation of an auto-excision construct design used for testing developmentally regulated promoters driving Cre expression (represented by pro:CRE) for excision-activated SMG expression. (A) An excision-activated selectable marker construct design with the DNA fragment to be excised flanked by two directly oriented loxP recombination sites. (B) Following excision, the HRA gene is activated and events are selected on a media supplemented with 0.1 mg/L imazapyr. Refer to Table S1 for description of construct components used in T-DNA construction.
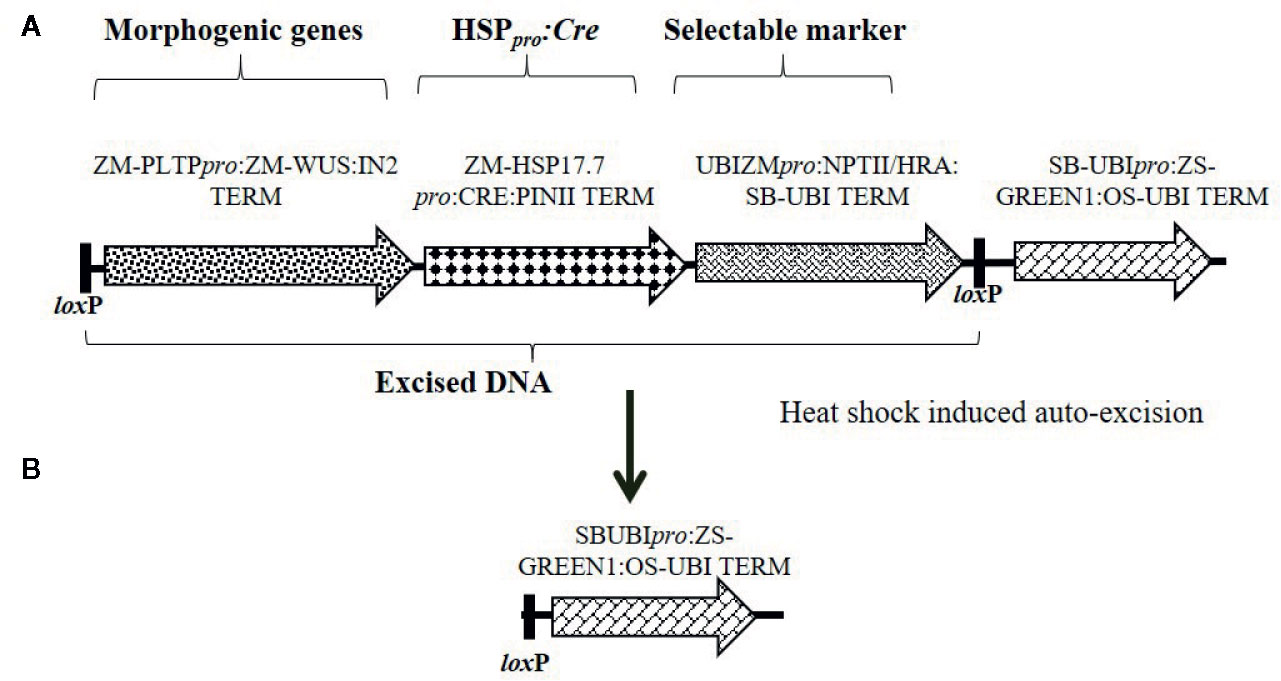
Figure 3 Schematic representation of an auto-excision construct design used for testing elimination of a morphogenic gene and a marker gene using heat shock promoter driving Cre expression for controlled gene excision. (A) Construct design depicting the order of cassettes including morphogenic genes, Hsp17.7pro driving Cre expression, and the selectable marker (HRA or NPTII) flanked by directly oriented loxP sites (a) which will be excised upon Cre expression. (B) Following excision, the DNA piece containing the ZS-GREEN expression cassette is left in the T0 event for visual confirmation of excision. Refer to Table S-1 for description of construct components used in T-DNA construction.
All transformations were done using the thymidine auxotrophic Agrobacterium tumefaciens strain LBA4404 THY- containing the pVIR helper plasmid, pPHP71539 (Anand et al., 2018) at OD550 of 0.5. The conditions for Agrobacterium suspension culture preparation following embryo isolation and infection have been previously described (Lowe et al., 2018; Hoerster et al., 2020). Two selectable markers were used in experiments: HRA (Green et al., 2009), a sulfonylurea herbicide resistance marker, driven by the sorghum Als promoter for selection with 0.1 mg/L imazapyr in culture medium, or the Ubipro:NPTII gene for selection with 150 mg/L G418 in culture medium.
Excision Conditions
For the developmentally regulated pro:Cre testing, no optimization was required. These experiments were performed on two inbreds, HC69 and PHR2HT. The previously described maize transformation protocol (Chu et al., 2019; Hoerster et al., 2020) was used with the transformation stage presented in Figure 4. After 3 weeks of selection in maturation media supplemented with either 0.1 mg/L imazapyr (HRA) or 150 mg/L G418 (NPTII), the plates with somatic embryos were subjected to heat shock treatment in a pre-heated incubator at different temperatures with 70% relative humidity for different time periods. Following heat-shock treatment, the plates were immediately returned to regeneration media with or without selectable marker for 2 weeks in the tissue culture chamber. Regenerated plants with healthy roots were transferred to soil. The initial heat shock treatment involved three different conditions: no heat shock (control), heat shock at 37°C for 1 day, or 42°C for 2 h/day for 3 consecutive days. The heat shock condition was further optimized using following treatments, 1) 42°C, 2 h/day for 2 d, 2) 42°C for 24 h, or 3) 45°C for 2 h/day to identify the best condition.
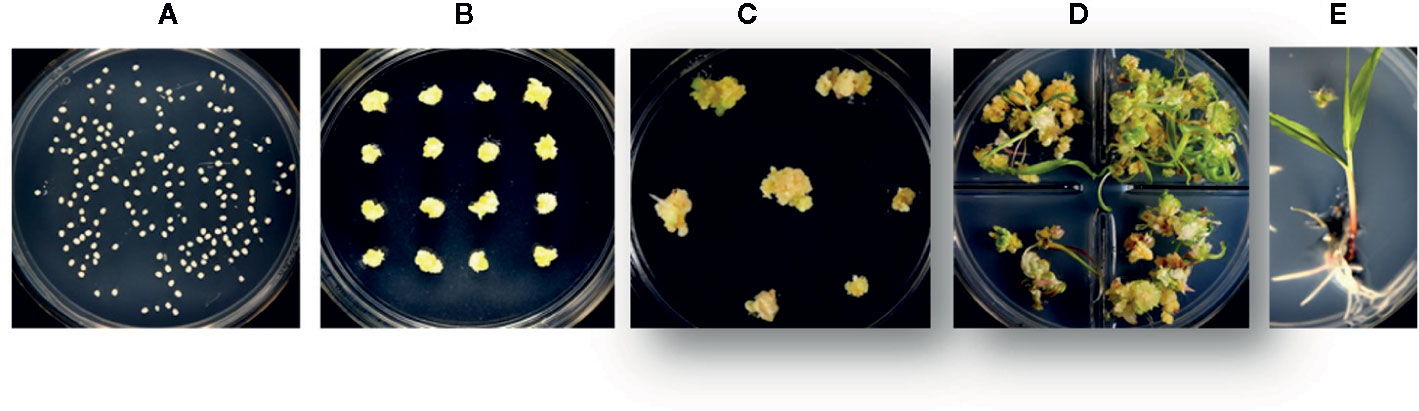
Figure 4 The different stages in rapid maize transformation and heat shock treatment. (A) immature zygotic embryos are isolated and infected with Agrobacterium tumefaciens, (B) transgenic somatic embryos are placed for 3 weeks on selection media based on selectable marker used (HRA or NPTII), (C) somatic embryos are heat shock treated and transferred to maturation media, (D) transgenic plants are regenerated with or without selection pressure for 2 weeks and, (E) regenerated plants are placed on a rooting media for 2–3 weeks.
Molecular Analyses
All molecular analysis and transgene copy number determination methods were previously described (Wu et al., 2014; Lowe et al., 2016; Hoerster et al., 2020). qPCR data was used to confirm recombinase-mediated excision based on the absence of the transgenes flanked by the loxP sites, determine the copy number of structural genes outside the excision DNA, and to screen for the presence of Agrobacterium binary construct backbone integration. Genomic DNA samples were extracted from a single piece (200 ng) of fresh leaf tissue from each plant (Truett et al., 2000). Non-transgenic maize inbred lines were used as the negative controls. Quantification was based on detection of amplified gene sequences using gene-specific forward and reverse primers, along with the corresponding gene-specific FAM™ or Vic®-based MGB fluorogenic probes (Applied Biosystems). The 2− ΔΔCT method (Livak and Schmittgen, 2001) was used to estimate copy number. Events which are single copy for all the transgenes and excised was used to calculate the excision frequency. Events which were excised with a single copy (SC) of all the transgenes without vector backbone integration were defined as a QE. The usable event (UE) frequency was calculated as transformation frequency times QE frequency. To determine intactness of the excised T-DNA at the insertion site, fifteen QE T0 plants were selected and subjected to Southern-by-Sequencing (Zastrow-Hayes et al., 2015).
Data collected from different experiments were analyzed separately by analysis of variance (ANOVA), with mean separation by LSD (P = 0.05) using JMP Pro 12.2.0 Statistical Discovery software package (SAS Institute Inc., Cary, NC).
Results
Excision via Developmentally-Regulated Promoters
The presence of morphogenic genes in transgenic events is undesirable because of unpredictable phenotypes (Lowe et al., 2016). Auto-excision of morphogenic genes occurs early in the transformation process which enables trait evaluation in T0 generation and reduces attrition due to T0 sterility. We evaluated several auto-excision designs, using Cre driven by various promoters. These included seven different developmentally regulated (embryo or meristem) promoters, the constitutive maize ubiquitin (ZM-Ubi) promoter, and the Agrobacterium nopaline synthase (Nos) promoter (Table 1). To facilitate excision, the morphogenic genes (Wus2 and Bbm) and the Cre gene cassette were flanked with a single pair of directly oriented loxP sites (Figure 1A). Following excision morphogenic-gene free events were regenerated as seen in Figure 1B. We evaluated two different inbreds (HC69 and PH2RT) to identify pro:Cre combinations that produced high frequencies of both transformation and excision. Molecular event data is presented in Table 2. All constructs tested produced stable transgenic events with some number of properly excised events. The Olepro : Cre had the highest transformation frequencies (27.2–37.1%), while the Glb1pro:Cre construct produced events with higher QE frequencies (8.6–18.4%).
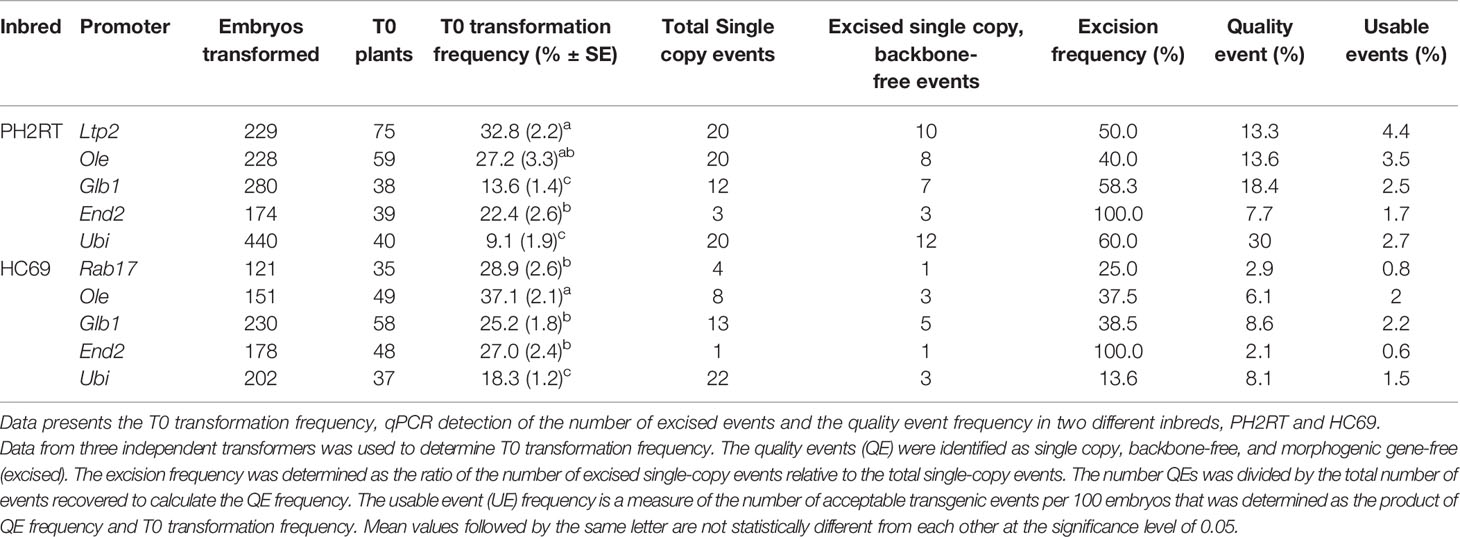
Table 2 Transformation results with different developmentally regulated promoters driving Cre expression for auto-excision of morphogenic genes using construct design described in Figure 1.
Excision via Marker Gene Activation
Although we achieved auto-excision with all developmentally regulated promoters tested, even for the best construct the usable events rate was around 2% and 80–90% of events were not excised quality events. To improve efficiency, we designed constructs in which the SMG was activated only upon excision of the morphogenic genes. This approach selected directly for excised events and was expected to increase QE frequency. A similar construct design was previously used to optimize tissue culture conditions for recovering high quality maize transgenic events (Chu et al., 2019). A schematic design of the construct is depicted in Figure 2A and the quality excised product in Figure 2B. For these experiments, either the Glb1 or the Ole promoters were used to drive Cre expression. The data from side-by-side testing of these two promoters using the construct design described in Figure 2 are summarized in Table 3. The construct containing Glb1pro:Cre improved T0 transformation and QE frequencies (1.8 and 1.4-fold), compared to the developmentally regulated gene-excision approach. When Olepro : Cre was used, the T0 transformation frequency was similar (>1.1-fold) while the QE frequency increased approximately 1.7-fold. The excision frequency was higher when excision-activated selection was used, with excision frequencies of 53.3 (Olepro : Cre) and 68.4% (Glbpro : Cre) when compared to the previous approach. Additionally, no null events (escapes) were identified by qPCR analysis.
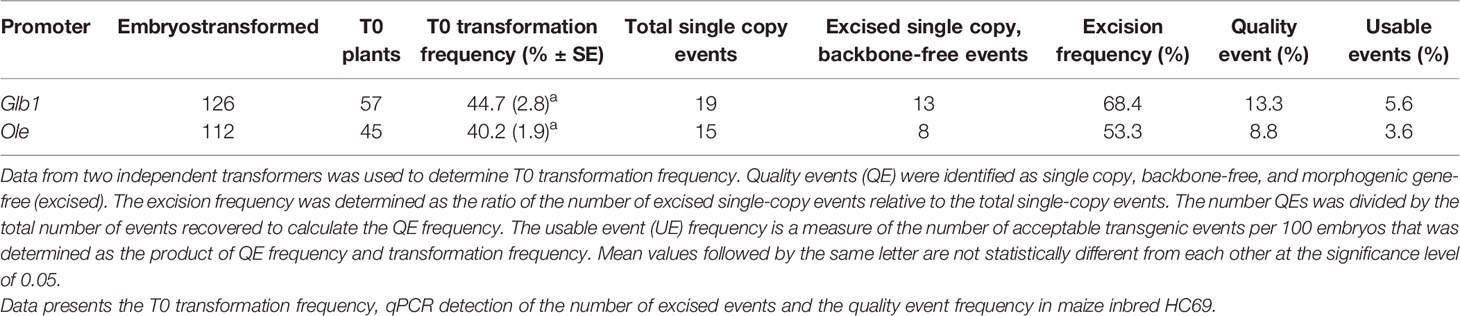
Table 3 Transformation results from excision-activated marker gene selection using either the Glb1pro or the Olepro driving Cre expression using construct design described in Figure 2.
The Glbpro : Cre construct design was further evaluated in two additional inbreds, PH84Z and PH85E, alongside HC69 for comparison (Table 4). QEs were recovered in all three inbreds, which were free of the morphogenic genes with no escapes. Excision frequency was similar (55–58%) across all the inbreds; QE frequencies varied by genotype: 8.7 (HC69), 27.7 (PH85E), and 6.7% (PH84Z) leading to differences in usable quality event frequency (UE, quality events per 100 embryos): 4.3 (HC69), 3.6 (PH85E), and 1.9% (PH84Z).
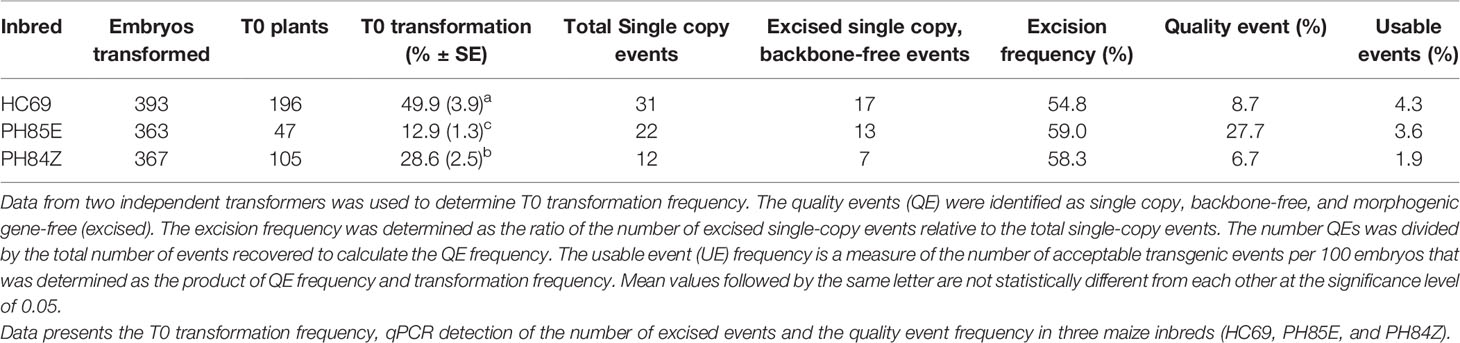
Table 4 Transformation results from excision-activated marker gene selection using Glbpro driving Cre expression using construct design described in Figure 2.
Excision via Stress-Inducible Promoters
To further improve efficiency, a series of stress-inducible promoters were tested for excision of morphogenic genes. The promoters were selected from a set of genes induced by heat (maize Hsp17.7 and Hsp26) and drought (ZmRab17, SiRAB21, BdDRP1, and BdDRP12). The construct design is identical to that described in Figure 1, where stress-inducible promoters drive Cre expression as represented by pro:Cre. In preliminary screening, embryos derived from HC69 were infected with an Agrobacterium strain containing one of the six constructs and, subsequently subjected to one of three different conditions: no heat shock (control), heat shock at 37°C for 1 day, or 42°C for 2 h/day for 3 consecutive days. The different steps in maize immature embryo transformation process included embryo infection with Agrobacterium strain containing the construct (Figure 4A), selection of transgenic events on media supplemented with selectable marker for 3 weeks (Figure 4B), heat-shock treatment step (Figure 4C) followed by immediate transfer to regeneration media with selection pressure (Figure 4D) and rooting (Figure 4E), before the events were sent to greenhouse. The auto-excision frequencies under induced and non-induced conditions were determined by qPCR analysis. Somatic embryos on maturation media (18 dpi) with 0.1 mg/L imazapyr were subjected to one of the heat conditions and moved onto a rooting media with 0.1 mg/L imazapyr following heat shock (Figure 4C).
All promoters except Hsp26 were leaky under non-induced conditions, resulting in gene-excision rates from 3.4 (Rab17pro) to 36% (BdRab21pro) compared to zero in the Cre-minus construct (Table 5). For a subset of the promoters ( HSP 17.7, Hsp26, Drp1, and Drp12), higher excision frequencies ranging from 43 to 100%, were observed in the 42°C, 2 h/day for 3 days heat treatment. Longer exposure of the somatic embryos at 37°C adversely effected T0 event recovery, compared to a short pulse of heat shock at 42°C (2 h/day for 3 days). Based on the recovery of excised T0 events with Hsp26pro construct at 42°C treatment compared to 37°C treatment, this promoter appeared to be induced only at higher temperatures.
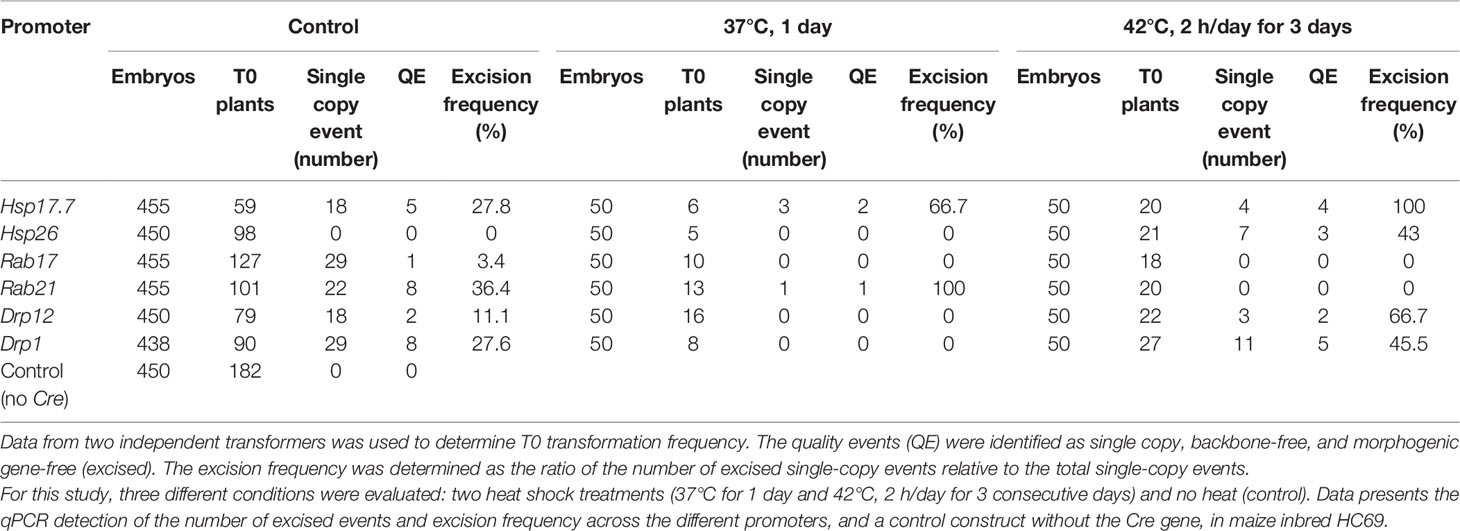
Table 5 Transformation results from screening of six different inducible promoters driving Cre expression for controlled gene excision.
Additional experiments were performed to further evaluate gene excision and optimize heat shock conditions using three of the inducible promoters (Hsp17.7, Hsp26, and Drp12). HC69 embryos infected with the three constructs were subjected to heat shock treatment at the maturation stage (Figure 4C). One of three different treatments were applied 1) no heat shock (control), 2) 42°C for 2 h, and 3) 42°C, 2 h on 3 consecutive days to determine frequencies of excision and UE recovery (Table 6). Consistent with the previous observation, Hsp17.7pro driving Cre expression under both heat treatments resulted in higher excision rates (62.5–69.2%) producing higher UE rates (10 to 18%) compared to Hsp26 pro and Drp12 pro. Based on the data, we identified Hsp17.7pro as the preferred promoter for auto-excision with heat shock of 42°C for 2 h.
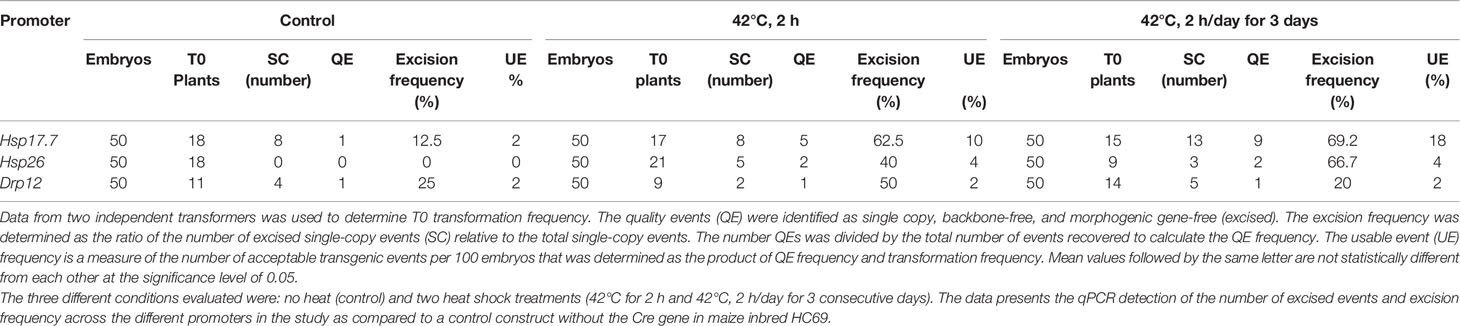
Table 6 Transformation results optimizing the heat shock conditions for controlled gene excision using three inducible promoters driving Cre expression.
Optimization of Heat-Shock Conditions to Improve Auto-Excision
Further experiments were designed with Hsp17.7pro and Hsp26pro to optimize excision conditions. After three weeks of selection, somatic embryos at the maturation stage were subjected to one of three different heat conditions 1) 42°C, 2 h/day for 2 d, 2) 42°C for 24 h, or 3) 45°C for 2 h/day to determine frequencies of excision and UE. Across the treatments, transformation frequencies ranged from 35–54.9%, except in the 42°C for 24 h treatment of embryos with Hsp17.7pro driving Cre expression, which was lower (Table 7). The heat treatments increased excision rates, which varied with the conditions applied. Of the two Hsp promoters tested, Hsp17.7pro resulted in events with higher excision frequency (75% at 42°C for 24 h and 77.8% at 45°C for 2 h) compared to Hsp26pro (66.7 and 61.9%). The 45°C/2 h treatment worked worked best for both Hsp promoters.
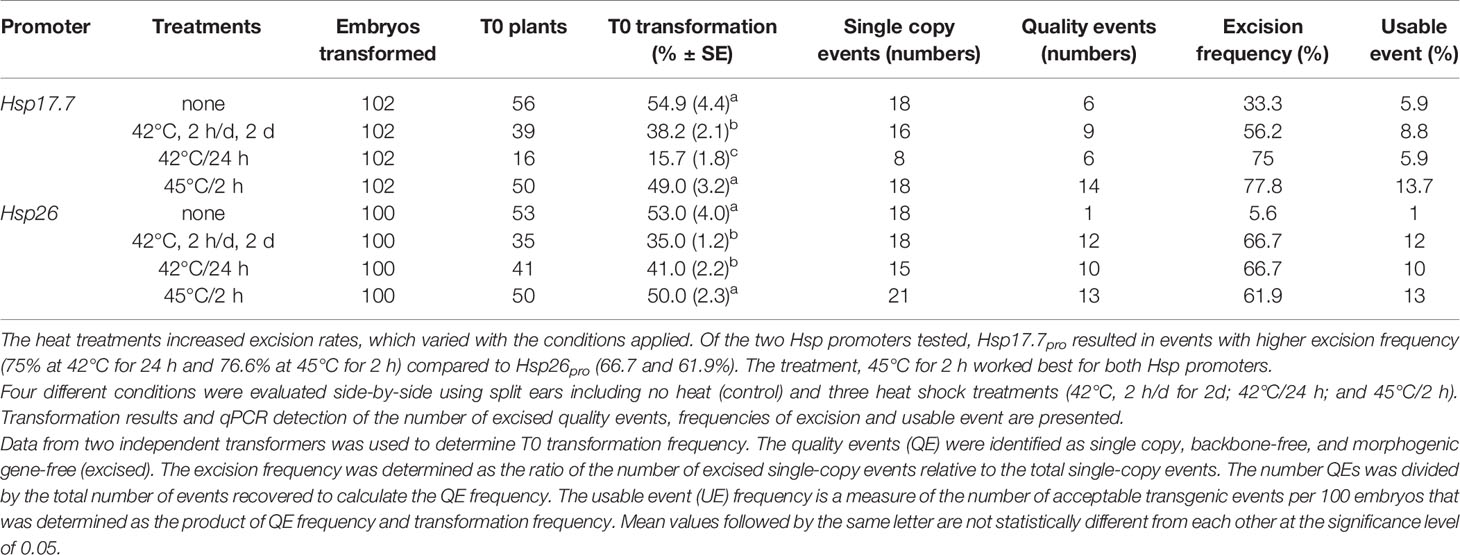
Table 7 Optimizing heat shock conditions for controlled gene excision using heat shock promoters Hsp17.7 and Hsp26 driving Cre expression.
Concurrent Elimination of Morphogenic and Plant Selectable Marker Genes
Next, we developed a strategy that simultaneously excised both the morphogenic genes and the SMG. Two different SMGs, HRA, and NPTII were tested. The construct design was slightly changed to enable excision of the SMG by including it as part of the excised DNA (morphogenic genes and Cre) flanked with a single pair of directly oriented loxP sites (Figure 3A) and the resulting excised events are free of SMG (Figure 3B). The binary construct designs with different selectable marker, morphogenic gene and a reporter gene Zs-GREEN is illustrated in Figure 3A. Following transformation and selection (either 0.1 mg/L imazapyr for the HRA gene or 150 mg/L G418 for the NPTII gene), the somatic embryos were heat-shock treated at 45°C for 2 h and moved into selection free regeneration and rooting media. Transformation data are presented in Table 8. Both HRA and NPTII constructs produced T0 plants free of morphogenic genes and SMG in the three inbreds tested. With the HRA construct, lower frequencies of QEs and UEs were observed and 2-fold more null events were produced compared to the NPTII construct. The excision frequency was comparable in both HRA and NPTII constructs. Irrespective of the differences, both selectable markers produced high frequencies of single copy, backbone-free events which are free of the morphogenic and marker genes.
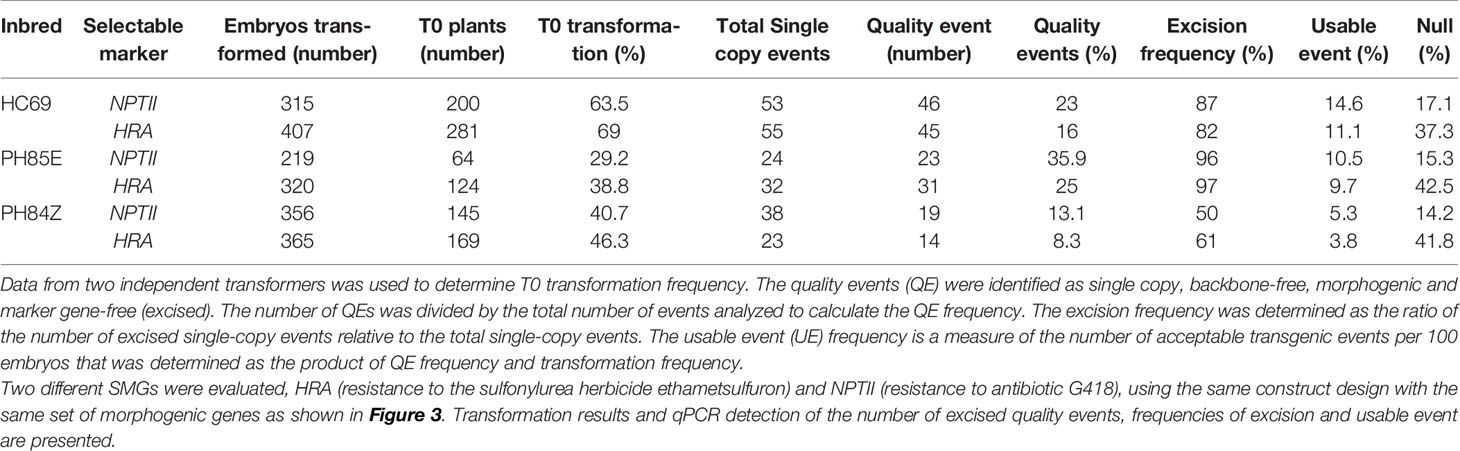
Table 8 Transformation results and molecular event data using the Hsp17.7 heat shock promoter for controlled excision of both morphogenic gene and marker gene in three maize inbreds (HC69, PH85E, and PH84Z).
To confirm the intactness of T-DNA following excision, 15 T0 quality events (five T0 plant per inbred, Table 8) transformed with the NPTII construct were analyzed with Southern-by-sequencing analysis (SbS, Zastrow-Hayes et al., 2015). SbS utilizes capture-based target enrichment of samples prior to next-generation sequencing (NGS) and is used to determine the insertion sequence and intactness of the inserted DNA at the insertion site. Twelve out of the fifteen T0 plants had intact single copy T-DNA insertion, free of morphogenic and marker genes. The other three plants failed SbS, two because they contained truncations of the T-DNA and one because an additional T-DNA fragment was inserted at a different location.
Progeny Analysis
To study the inheritance and segregation of the morphogenic and SMG-free events, we screened single-copy T0 plants free of morphogenic gene and SMG produced from the NPTII construct. Thirteen T0 QE plants, six plants from HC69, and seven plants from PHR84Z, were selected for progeny analysis. These plants were self-pollinated and all 13 events produced seeds. T1 plants were evaluated for zygosity using qPCR to evaluate copy number of Cre and NPTII genes (excised DNA). Twelve of the 13 events showed the expected Mendelian inheritance of a single copy T-DNA integration (1:2:1; chi-square p-value >0.05) in the T1 generation (Table 9). All T1 plants looked normal (Figure 5) and set seeds.
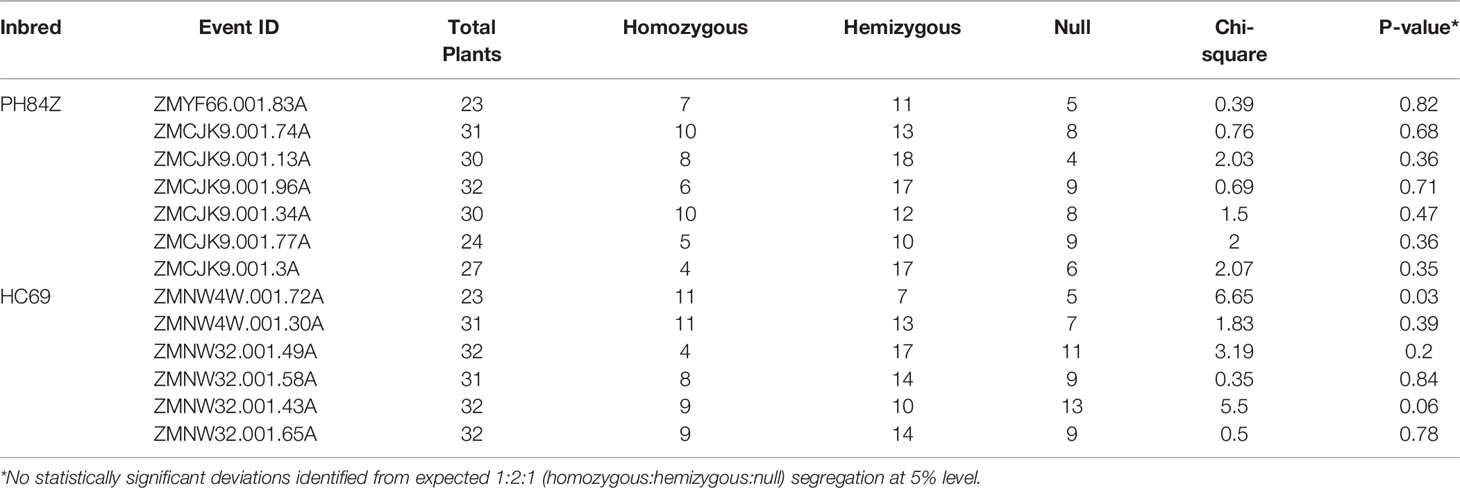
Table 9 Observed and expected number of homozygous, hemizygous, and null plants for T-DNA integration copy number in in T1 generation of 13 SC excised quality events across two maize inbreds (PH84Z and HC69).
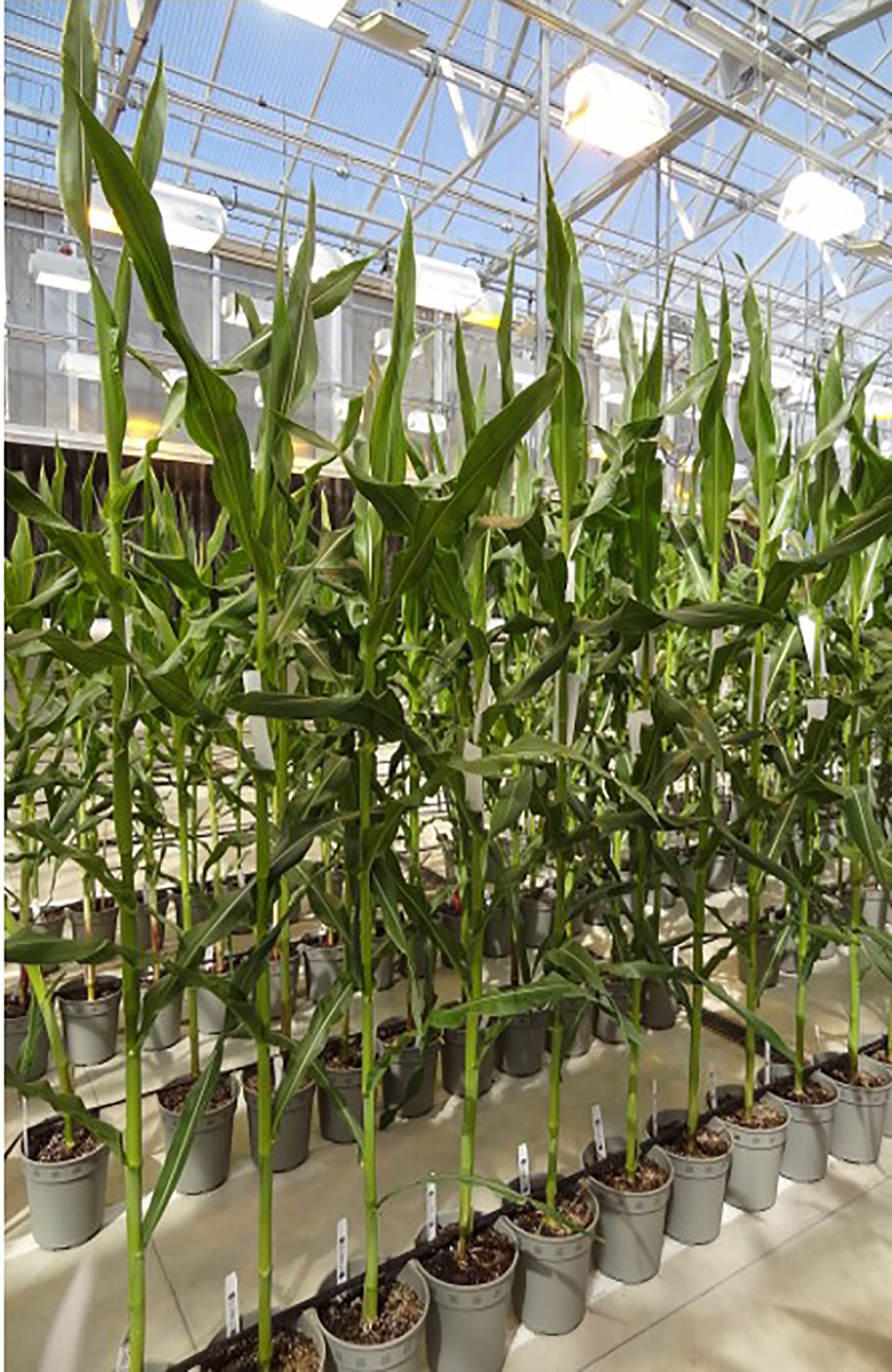
Figure 5 The T1 plants of PHR84Z. Homozygous T1 plants from PH84Z which were determined to be quality events, free of marker and morphogenic genes exhibited normal plant phenotype.
Discussion
In maize, direct induction of somatic embryos capable of rapidly germinating from immature embryos (without a callus phase) has been demonstrated using the auxin-inducible promoter Axig1 driving Wus2 expression in combination with Bbm driven by a maize PLTP promoter (Lowe et al., 2018). However, the presence of morphogenic genes in regenerated plants led to abnormal phenotypes (Lowe et al., 2016). Therefore, removing morphogenic genes is imperative for accurate event evaluation and product development. We achieved this by developing a gene excision system. Morphogenic gene excision was accomplished using a drought-inducible Rab17 promoter driving Cre recombinase expression (Vilardell et al., 1991). Although this approach worked, the desiccation step reduced event recovery and not all event achieved excision (Lowe et al., 2016).
In order to develop a more efficient systems, promoters of seven developmentally regulated genes, the Knotted-1 (Kn1) (Bolduc et al., 2012), Leafy cotyledon1 (Lec1) (Pelletier et al., 2017), barley Lipid transfer protein2 (Ltp2) (Kalla et al., 1994), an early embryo response gene (End2) (Casper et al., 2005), Globulin1 (Glb1) (Belanger and Kriz, 1991), and Olesin (Ole) (Anand et al., 2017b) were evaluated for their ability to express Cre and in order to excise morphogenic genes. Unlike inducible promoters these developmentally regulated promoters do not require physical or chemical induction. Morphogenic gene removal was observed using developmentally regulated promoters, but with lower QE frequencies. This may be attributable to premature expression of Cre, leading to untimely excision of the developmental genes.
We developed a method for regenerating events that are free of morphogenic genes using an excision-activated marker gene approach (Chu et al., 2019). Developmentally regulated promoters Glb1 and Ole that are active during late embryo development (Kriz et al., 1990; Anand et al., 2017b), were used to express Cre, leading to auto-excision. After excision, the HRA gene is activated (Chu et al., 2019). This strategy improved event recovery, however a large proportion of T0 events were multi-copy and non-excised. Rapid maize transformation was previously shown to produce high frequency of multi-copy events (Lowe et al., 2018) and our data is consistent with the observation. Another possibility is the restricted activation of the developmental promoters leading to partial/incomplete excision, which does not work in rapid maize transformation for enriching quality events.
Inducible promoters can be used to regulate expression of recombinase genes. These inducible promoters predominantly fall into two categories; 1) heat shock- or stress-inducible promoters (Kilby et al., 1995; Cuellar et al., 2006; Zhang et al., 2006; Du et al., 2019), and 2) chemical inducible promoters (Gatz, 1996; Zuo and Chua, 2000). Among the stress-inducible promoters tested, Hsp17.7pro and Hsp26pro worked best based on a higher frequency of T0 transformation, gene excision and UE rate. In maize, the regulation of Hsp promoters in response to stresses has been described (Pegoraro et al., 2011), including accumulation of Hsp proteins under temperatures over 32–33°C (Ristic et al., 1991; Vierling, 1991) and enhanced Hsp70 synthesis under drought and/or heat (Hu et al., 2010). The heat-inducible auto-excision system was previously described using a construct design that involves Hsp70pro driving the Cre recombinase for elimination of the SMG (egfp) while a second marker gene, expressing the anthocyanin pigmentation (Rsc) gene, was used for event sorting (Du et al., 2019). While successful, the strategy has limited practical application requiring tracking of transgenes in the T1 generation and subsequent segregation, which is resource-and time-intensive.
We developed a reliable and efficient method to obtain morphogenic gene-free events at high frequencies (66–77% of the total events generated). The overall objective was to develop an efficient auto-excision system for rapid maize transformation, with the purpose of eliminating both morphogenic and marker genes, that is highly efficient to meet the needs of high throughput maize transformation. The method we developed resulted in the elimination of morphogenic and marker genes at the maturation stage of transformation at high frequencies (ranging from 60–97%) in multiple elite inbreds. This was achieved by optimizing tissue culture conditions, optimization of heat shock treatment and identifying a versatile SMG. The stably transformed plants were normal, produced seeds and showed stable transmission of the integrated T-DNA to the next generation.
Conclusion
Our objective is to remove morphogenic and selectable marker genes from the events generated from rapid maize transformation. The first generation of rapid maize transformation method was designed to improve the transformation rates and to extend transformation capabilities to many genotypes. Subsequently, we demonstrated a viable second-generation alternative, using a mixture of an Agrobacterium strains, one with non-integrating Wus2 gene and the other with a combination of structural genes to regenerate transgenic plants free of morphogenic genes. Even though this simplifies vector construction, however, the process still relies on a SMG for recovery of stable transgenic events. This study demonstrated a viable third alternative, relying on inducible promoters for auto-excision of both the morphogenic genes and the SMG in the early stages of maize transformation. The stable transformed plants recovered by this method are free of the morphogenic genes and marker genes, a desirable quality for transgene evaluation and in commercial products.
Data Availability Statement
The datasets presented in this study can be found in online repositories. The names of the repository/repositories and accession number(s) can be found in the article/Supplementary Material.
Author Contributions
AA, EW, KL, WG-K, TJ, and NC conceived the research idea. AA, EW, KL, and WG-K designed constructs and research. NW, MA, GH, and LR conducted maize transformation and optimization. EW and AA, performed data analysis. AA, WG-K, TJ, and NC wrote the manuscript.
Conflict of Interest
NW, MA, GH, LR, EW, KL, WG-K, and AA are inventors on pending applications on this work and a related work are current employees of Corteva Agriscience who owns the pending patent applications. TJ and NC are current employees of Corteva Agriscience.
Acknowledgments
We thank the internal support groups, Super-Vector (SV) team for their support with vector construction and PCR Analysis and Characterization (PAC) team for molecular event quality analysis. Scott Betts with program support, Terry Hu for maize transformation support. Special thanks to Tracy Fisher and Scott Betts for critical reading of the manuscript and Kara Califf for the art work.
Supplementary Material
The Supplementary Material for this article can be found online at: https://www.frontiersin.org/articles/10.3389/fpls.2020.01298/full#supplementary-material
Abbreviations
Bbm, Babyboom; Cre, CRE recombinase; HSP, Heat-shock promoters; SMG, Selectable marker-free; Pro, promoters; QE, Quality events; UE, Usable event; Wus2, Wuschel2.
References
An, G., Mitra, A., Choi, H. K., Costa, M. A., An, K., Thornburg, R. W., et al. (1989). Functional analysis of the 3’ control region of the potato wound-inducible proteinase inhibitor II gene. Plant Cell 1, 115–122. doi: 10.1105/tpc.1.1.115
An, G. (1986). Development of plant promoter expression vectors and their use for analysis of differential activity of nopaline synthase promoter in transformed tobacco cells. Plant Physiol. 81, 86–91. doi: 10.1104/pp.81.1.86
Anand, A., Arling, M. L., Da Silva Conceicao, A., Gordon-Kamm, W. J., Hastings, C. E., Hoerster, G. M., et al. (2017a). Methods and compositions for rapid plant transformation. USPTO. United States patent US20170121722A1.
Anand, A., Bass, S. H., Bertain, S. M., Cho, H. J., Kinney, A. J., Klein, T. M., et al. (2017b). Ochrobactrum-mediated transformation of plants. International Patent application. International patent WO2017040343A1.
Anand, A., Bass, S. H., Wu, E., Wang, N., Mcbride, K. E., Annaluru, N., et al. (2018). An improved ternary vector system for Agrobacterium-mediated rapid maize transformation. Plant Mol. Biol. 97, 187–200. doi: 10.1007/s11103-018-0732-y
Araki, H., Jearnpipatkul, A., Tatsumi, H., Sakurai, T., Ushio, K., Muta, T., et al. (1985). Molecular and functional organization of yeast plasmid pSR1. J. Mol. Biol. 182, 191–203. doi: 10.1016/0022-2836(85)90338-9
Bayley, C. C., Morgan, M., Dale, E. C., Ow, D. W. (1992). Exchange of gene activity in transgenic plants catalyzed by the Cre-lox site-specific recombination system. Plant Mol. Biol. 18, 353–361. doi: 10.1007/BF00034962
Belanger, F. C., Kriz, A. L. (1991). Molecular basis for allelic polymorphism of the maize Globulin-1 gene. Genetics 129, 863–872.
Bolduc, N., Yilmaz, A., Mejia-Guerra, M. K., Morohashi, K., O’connor, D., Grotewold, E., et al. (2012). Unraveling the KNOTTED1 regulatory network in maize meristems. Genes Dev. 26, 1685–1690. doi: 10.1101/gad.193433.112
Busk, P. K., Jensen, A. B., Pagès, M. (1997). Regulatory elements in vivo in the promoter of the abscisic acid responsive gene rab17 from maize. Plant J. 11, 1285–1295. doi: 10.1046/j.1365-313X.1997.11061285.x
Casper, L., Lappegard, K. K., Abbitt, S. E., Martino-Catt, S. J., Olsen, O. A. (2005). Seed-preferred promoters from end genes. USPTO. United States patent US6903205B2.
Chilcoat, D., Liu, Z. B., Sander, J. (2017). Use of CRISPR/Cas9 for crop improvement in maize and soybean. Prog. Mol. Biol. Transl. Sci. 149, 27–46. doi: 10.1016/bs.pmbts.2017.04.005
Chong-Pérez, B., Angenon, G. (2013). “Strategies for generating marker-free transgenic plants,” in Genetic Engineering. Ed. Sithole-Niang, I. (Intech Open). 2, 17–48.
Christensen, A. H., Sharrock, R. A., Quail, P. H. (1992). Maize polyubiquitin genes: structure, thermal perturbation of expression and transcript splicing, and promoter activity following transfer to protoplasts by electroporation. Plant Mol. Biol. 18, 675–689. doi: 10.1007/BF00020010
Chu, U. C., Adelberg, J., Lowe, K., Jones, T. J. (2019). Use of DoE methodology to optimize the regeneration of high-quality, single-copy transgenic Zea mays L. (maize) plants. In Vitro Cell. Dev. Biol. Plant 55, 678–694. doi: 10.1007/s11627-019-10002-w
Cox, M. M. (1983). The FLP protein of the yeast 2-microns plasmid: expression of a eukaryotic genetic recombination system in Escherichia coli. Proc. Natl. Acad. Sci. U.S.A. 80, 4223–4227. doi: 10.1073/pnas.80.14.4223
Cuellar, W., Gaudin, A., Solórzano, D., Casas, A., Ñopo, L., Chudalayandi, P., et al. (2006). Self-excision of the antibiotic resistance gene nptII using a heat inducible Cre-loxP system from transgenic potato. Plant Mol. Biol. 62, 71–82. doi: 10.1007/s11103-006-9004-3
Dale, E. C., Ow, D. W. (1991). Gene transfer with subsequent removal of the selection gene from the host genome. Proc. Natl. Acad. Sci. U. S. A. 88, 10558–10562. doi: 10.1073/pnas.88.23.10558
Darbani, B., Eimanifar, A., Stewart, C. N., Jr., Camargo, W. N. (2007). Methods to produce marker-free transgenic plants. Biotechnol. J. 2, 83–90. doi: 10.1002/biot.200600182
Du, D., Jin, R., Guo, J., Zhang, F. (2019). Construction of marker-free genetically modified maize using a heat-inducible auto-excision vector. Genes 10, 374. doi: 10.3390/genes10050374
Gao, X., Zhou, J., Li, J., Zou, X., Zhao, J., Li, Q., et al. (2015). Efficient generation of marker-free transgenic rice plants using an improved transposon-mediated transgene reintegration strategy. Plant Physiol. 167, 11–24. doi: 10.1104/pp.114.246173
Gatz, C. (1996). Chemically inducible promoters in transgenic plants. Curr. Opin. Biotechnol. 7, 168–172. doi: 10.1016/S0958-1669(96)80008-5
Gleave, A. P., Mitra, D. S., Mudge, S. R., Morris, B. A. M. (1999). Selectable marker-free transgenic plants without sexual crossing: transient expression of cre recombinase and use of a conditional lethal dominant gene. Plant Mol. Biol. 40, 223–235. doi: 10.1023/A:1006184221051
Green, J. M., Hale, T., Pagano, M. A., Andreassi, J. L., Gutteridge, S. A. (2009). Response of 98140 corn with Gat4621 and HRA transgenes to glyphosate and ALS-inhibiting herbicides. Weed Sci. 57, 142–148. doi: 10.1614/WS-08-152.1
Hare, P. D., Chua, N. H. (2002). Excision of selectable marker genes from transgenic plants. Nat. Biotechnol. 20, 575–580. doi: 10.1038/nbt0602-575
Hoerster, G., Wang, N., Ryan, L., Wu, E., Anand, A., McBride, K., et al. (2020). Use of non-integrating Zm-Wus2 vectors to enhance maize transformation. In Vitro Cell. Dev. Biol. Plant. 56, 265–279. doi: 10.1007/s11627-019-10042-2
Hoess, R. H., Abremski, K. (1985). Mechanism of strand cleavage and exchange in the Cre-lox site-specific recombination system. J. Mol. Biol. 181, 351–362. doi: 10.1016/0022-2836(85)90224-4
Hoess, R. H., Ziese, M., Sternberg, N. (1982). P1 site-specific recombination: nucleotide sequence of the recombining sites. Proc. Natl. Acad. Sci. U. S. A. 79, 3398–3402. doi: 10.1073/pnas.79.11.3398
Hu, X., Liu, R., Li, Y., Wang, W., Tai, F., Xue, R., et al. (2010). Heat shock protein 70 regulates the abscisic acid-induced antioxidant response of maize to combined drought and heat stress. Plant Growth Regul. 60, 225–235. doi: 10.1007/s10725-009-9436-2
Jia, H., Pang, Y., Chen, X., Fang, R. (2006). Removal of the selectable marker gene from transgenic tobacco plants by expression of cre recombinase from a tobacco mosaic virus vector through agroinfection. Transgenic. Res. 15, 375–384. doi: 10.1007/s11248-006-0011-6
Kalla, R., Shimamoto, K., Potter, R., Nielsen, P. S., Linnestad, C., Olsen, O.-A. (1994). The promoter of the barley aleurone-specific gene encoding a putative 7 kDa lipid transfer protein confers aleurone cell-specific expression in transgenic rice. Plant J. 6, 849–860. doi: 10.1046/j.1365-313X.1994.6060849.x
Kerbach, S., Lörz, H., Becker, D. (2005). Site-specific recombination in Zea mays. Theor. Appl. Genet. 111, 1608–1616. doi: 10.1007/s00122-005-0092-2
Kilby, N. J., Davies, G. J., Snaith, M. R., Murray, J. A. H. (1995). FLP recombinase in transgenic plants: constitutive activity in stably transformed tobacco and generation of marked cell clones in Arabidopsis. Plant J. 8, 637–652. doi: 10.1046/j.1365-313X.1995.08050637.x
Klippel, A., Mertens, G., Patschinsky, T., Kahmann, R. (1988). The DNA invertase Gin of phage Mu: formation of a covalent complex with DNA via a phosphoserine at amino acid position 9. EMBO J. 7, 1229–1237. doi: 10.1002/j.1460-2075.1988.tb02935.x
Kopertekh, L., Schiemann, J. (2005). Agroinfiltration as a tool for transient expression of cre recombinase in vivo. Transgenic Res. 14, 793–798. doi: 10.1007/s11248-005-8293-7
Kopertekh, L., Jüttner, G., Schiemann, J. (2004). PVX-Cre-mediated marker gene elimination from transgenic plants. Plant Mol. Biol. 55, 491–500. doi: 10.1007/s11103-004-0237-8
Kriz, A. R., Wallace, M. S., Paiva, R. (1990). Globulin gene expression in embryos of maize viviparous mutants. Plant Physiol. 92, 538–542. doi: 10.1104/pp.92.2.538
Ling, F., Zhou, F., Chen, H., Lin, Y. (2016). Development of marker-free insect-resistant indica rice by Agrobacterium tumefaciens-mediated co-transformation. Front. Plant Sci. 7 (448), 1–10. doi: 10.3389/fpls.2016.01608
Liu, S., Kriz, A., Duncan, D., Widholm, J. (1998). Abscisic acid-regulated Glb1 transient expression in cultured maize P3377 cells. Plant Cell Rep. 17, 650–655. doi: 10.1007/s002990050459
Livak, K. J., Schmittgen, T. D. (2001). Analysis of relative gene expression data using real-time quantitative pcr and the 2–ΔΔCT method. Methods 25, 402–408. doi: 10.1006/meth.2001.1262
Lowe, K., Wu, E., Wang, N., Hoerster, G., Hastings, C., Cho, M.-J., et al. (2016). Morphogenic regulators baby boom and wuschel improve monocot transformation. Plant Cell 28, 1998–2015. doi: 10.1105/tpc.16.00124
Lowe, K., La Rota, M., Hoerster, G., Hastings, C., Wang, N., Chamberlin, M., et al. (2018). Rapid genotype “independent” Zea mays L. (maize) transformation via direct somatic embryogenesis. In Vitro Cell. Dev. Biol. Plant 54, 240–252. doi: 10.1007/s11627-018-9905-2
Maeser, S., Kahmann, R. (1991). The Gin recombinase of phage Mu can catalyse site-specific recombination in plant protoplasts. Mol. Gen. Genet. 230, 170–176. doi: 10.1007/BF00290665
Maher, M. F., Nasti, R. A., Vollbrecht, M., Starker, C. G., Clark, M. D., Voytas, D. F. (2020). Plant gene editing through de novo induction of meristems. Nat. Biotechnol. 38, 84–89. doi: 10.1038/s41587-019-0337-2
Mookkan, M., Nelson-Vasilchik, K., Hague, J., Zhang, Z. J., Kausch, A. P. (2017). Selectable marker independent transformation of recalcitrant maize inbred B73 and sorghum P898012 mediated by morphogenic regulators BABY BOOM and WUSCHEL2. Plant Cell Rep. 36, 1477–1491. doi: 10.1007/s00299-017-2169-1
Moravčíková, J., Vaculková, E., Bauer, M., Libantová, J. (2008). Feasibility of the seed specific cruciferin C promoter in the self excision Cre/loxP strategy focused on generation of marker-free transgenic plants. Theor. Appl. Genet. 117, 1325. doi: 10.1007/s00122-008-0866-4
Odell, J., Caimi, P., Sauer, B., Russell, S. (1990). Site-directed recombination in the genome of transgenic tobacco. Mol. Gen. Genet. 223, 369–378. doi: 10.1007/BF00264442
Pegoraro, C., Mertz, L. M., Da Maia, L. C., Rombaldi, C. V., De Oliveira, A. C. (2011). Importance of heat shock proteins in maize. J. Crop Sci. Biotechnol. 14, 85–95. doi: 10.1007/s12892-010-0119-3
Pelletier, J. M., Kwong, R. W., Park, S., Le, B. H., Baden, R., Cagliari, A., et al. (2017). LEC1 sequentially regulates the transcription of genes involved in diverse developmental processes during seed development. Proc. Natl. Acad. Sci. U. S. A. 114, E6710–E6719. doi: 10.1073/pnas.1707957114
Puchta, H. (2000). Removing selectable marker genes: taking the shortcut. Trends Plant Sci. 5, 273–274. doi: 10.1016/S1360-1385(00)01684-8
Puchta, H. (2003). Marker-free transgenic plants. Plant Cell Tissue Organ Cult. 74, 123–134. doi: 10.1023/A:1023934807184
Ristic, Z., Gifford, D. J., Cass, D. D. (1991). Heat shock proteins in two lines of Zea mays L. that differ in drought and heat resistance. Plant Physiol. 97, 1430–1434. doi: 10.1104/pp.97.4.1430
Senecoff, J. F., Bruckner, R. C., Cox, M. M. (1985). The FLP recombinase of the yeast 2-micron plasmid: characterization of its recombination site. Proc. Natl. Acad. Sci. U. S. A. 82, 7270–7274. doi: 10.1073/pnas.82.21.7270
Truett, G. E., Heeger, P., Mynatt, R. L., Truett, A. A., Walker, J. A., Warman, M. L. (2000). Preparation of PCR-quality mouse genomic dna with hot sodium hydroxide and tris (HotSHOT). BioTechniques 29, 52–54. doi: 10.2144/00291bm09
Verweire, D., Verleyen, K., De Buck, S., Claeys, M., Angenon, G. (2007). Marker-free transgenic plants through genetically programmed auto-excision. Plant Physiol. 145, 1220–1231. doi: 10.1104/pp.107.106526
Vierling, E. (1991). The roles of heat shock proteins in plants. Annu. Rev. Plant Physiol. Plant Mol. Biol. 42, 579–620. doi: 10.1146/annurev.pp.42.060191.003051
Vilardell, J., Mundy, J., Stilling, B., Leroux, B., Pla, M., Freyssinet, G., et al. (1991). Regulation of the maize rab17 gene promoter in transgenic heterologous systems. Plant Mol. Biol. 17, 985–993. doi: 10.1007/BF00037138
Wu, E., Lenderts, B., Glassman, K., Berezowska-Kaniewska, M., Christensen, H., Asmus, T., et al. (2014). Optimized Agrobacterium-mediated sorghum transformation protocol and molecular data of transgenic sorghum plants. In Vitro Cell. Dev. Biol. Plant 50, 9–18. doi: 10.1007/s11627-013-9583-z
Yau, Y. Y., Stewart, C. N., Jr. (2013). Less is more: strategies to remove marker genes from transgenic plants. BMC Biotechnol. 13, 36–36. doi: 10.1186/1472-6750-13-36
Zastrow-Hayes, G. M., Lin, H., Sigmund, A. L., Hoffman, J. L., Alarcon, C. M., Hayes, K. R., et al. (2015). Southern-by-Sequencing: a robust screening approach for molecular characterization of genetically modified crops. Plant Genome 8, 1. doi: 10.3835/plantgenome2014.08.0037
Zhang, Y., Li, H., Ouyang, B., Lu, Y., Ye, Z. (2006). Chemical-induced autoexcision of selectable markers in elite tomato plants transformed with a gene conferring resistance to lepidopteran insects. Biotechnol. Lett. 28, 1247–1253. doi: 10.1007/s10529-006-9081-z
Zubko, E., Scutt, C., Meyer, P. (2000). Intrachromosomal recombination between attP regions as a tool to remove selectable marker genes from tobacco transgenes. Nat. Biotechnol. 18, 442–445. doi: 10.1038/74515
Keywords: Agrobacterium, developmentally-regulated promoters, heat-shock promoters, morphogenic genes, marker-free events, rapid maize transformation
Citation: Wang N, Arling M, Hoerster G, Ryan L, Wu E, Lowe K, Gordon-Kamm W, Jones TJ, Chilcoat ND and Anand A (2020) An Efficient Gene Excision System in Maize. Front. Plant Sci. 11:1298. doi: 10.3389/fpls.2020.01298
Received: 24 April 2020; Accepted: 11 August 2020;
Published: 02 September 2020.
Edited by:
Brian Jones, The University of Sydney, AustraliaReviewed by:
Seiichi Toki, National Institute of Agrobiological Sciences, JapanSerena Varotto, University of Padua, Italy
Copyright © 2020 Wang, Arling, Hoerster, Ryan, Wu, Lowe, Gordon-Kamm, Jones, Chilcoat and Anand. This is an open-access article distributed under the terms of the Creative Commons Attribution License (CC BY). The use, distribution or reproduction in other forums is permitted, provided the original author(s) and the copyright owner(s) are credited and that the original publication in this journal is cited, in accordance with accepted academic practice. No use, distribution or reproduction is permitted which does not comply with these terms.
*Correspondence: Ajith Anand, YWppdGguYW5hbmRAY29ydGV2YS5jb20=