- 1State Key Laboratory of Forest Genetics and Breeding, Northeast Forestry University, Harbin, China
- 2School of Pharmacy, Harbin University of Commerce, Harbin, China
- 3Agriculture College of Yanbian University, Yanji, China
The application of drought stress-regulating transcription factors (TFs) offers a credible way to improve drought tolerance in plants. However, many drought resistant TFs always showed unintended adverse effects on plant growth or other traits. Few studies have been conducted in trees to evaluate and overcome the pleiotropic effects of drought tolerance TFs. Here, we report the dose-dependent effect of the Limonium bicolor LbDREB6 gene on its overexpression in Populus ussurensis. High- and moderate-level overexpression of LbDREB6 significantly increased drought tolerance in a dose-dependent manner. However, the OE18 plants showed stunted growth under normal conditions, but they were also more sensitive to Marssonina brunnea infection than wild type (WT) and OE14 plants. While, OE14 showed normal growth, the pathogen tolerance of them was not significantly different from WT. Many stress-responsive genes were up-regulated in OE18 and OE14 compared to WT, especially for OE18 plants. Meanwhile, more pathogen tolerance related genes were down-regulated in OE18 compared to OE14 and WT plants. We achieved improved drought tolerance by adjusting the increased levels of exogenous DREB genes to avoid the occurrence of growth reduction and reduced disease tolerance.
Introduction
Drought is among the most serious environmental stressors resulting in substantial damage annually to the global agricultural and forestry industries (Kudo et al., 2017). Thus, improving drought tolerance of plants is urgently needed to stabilize the global productivity of crops. Vascular plants have evolved complex molecular strategies to cope with water deficit (Lata and Prasad, 2011; Li et al., 2014). In particular, vascular plants mediate some stress responses to drought via transcription factors (TFs), which serve as master regulators of many stress-response genes (Singh et al., 2002; Nakashima et al., 2014). Therefore, TFs may comprise critical targets for transgenic engineering to modify the tolerances of plants to abiotic stressors (Quan et al., 2010). However, the application of TFs to improve drought tolerance frequently comes at the cost of introducing undesired phenotypes, such as dwarfism, decreased pathogen tolerance, and decreased grain yield (Lata and Prasad, 2011; Bhargava and Sawant, 2013; Cabello et al., 2014; Shavrukov et al., 2016). These negative effects constrain the practical utilization of drought-resistant transgenic plants based on TFs. Thus, to evaluate the drought tolerance conferred by TFs, it is necessary to simultaneously predict and avoid unintended effects in transgenic plants.
These TFs, especially the dehydration responsive element binding (DREB) factor family member, occur in many plant species and confer tolerance to abiotic stress (Lin et al., 2008; Liao et al., 2017). In Arabidopsis thaliana (L.) Heynh., the DREB subfamily is divided into six subgroups (A-1 to A-6) based on the structural characteristics of the proteins (Agarwal et al., 2017). Among the subgroups, A-1 and A-2 contain DREB1 and DREB2 TFs, which are known to be involved in stress responses to low temperature, drought, and high salt (Du et al., 2018). In Arabidopsis, DREB2 positively regulates the expression of drought-response genes (Sakuma et al., 2006). Overexpression of Glycine max DREB1 (GmDREB1) improved drought tolerance and responses to other abiotic stressors in transgenic Arabidopsis and wheat (Kidokoro et al., 2015; Zhou et al., 2020). Moreover, ectopic expression of GhDREB1 from cotton yielded stronger tolerance to chilling in transgenic Nicotiana tabacum L. (tobacco) compared to the wild type (WT) (Shan et al., 2007).
DREBs of the A-6 subgroup have also been identified from several plant species, and their functions in stress responses and development have been characterized. For example, expression of GhDBP2 is greatly up-regulated during drought, salt exposure, low temperature, and abscisic acid (ABA) treatments in the cotyledons of cotton plants (Huang et al., 2008), and overexpression of CmDREB6 in chrysanthemum enhanced heat tolerance (Du et al., 2018). Similarly, JcDREB, an A-6 subgroup member from Jatropha curcas L., a biodiesel plant, was up-regulated by cold, salt, and drought stress, and overexpression of JcDREB in transgenic Arabidopsis enhanced salt and freezing tolerance (Tang et al., 2011). In tobacco, overexpression of SsDREB, an A-6 DREB from halophilic Suaeda salsa (L.) Pall., increased salt and drought tolerance compared to WT plants (Zhang et al., 2015).
Although DREBs of several subgroups are known to increase drought tolerance, they have also been shown to inhibit growth in transgenic plants (Huang et al., 2009). For example, ZmDREB4.1 from maize, repressed cell division and constrained leaf extension and hypocotyl, petiole and stem elongation both natively in transgenic tobacco (Li et al., 2018). Likewise, overexpression of AtDREB1A in soybeans led to dwarfism and delayed flowering (Suo et al., 2016).
DREBs are also reported to be involved in the biotic stress signaling pathway. For example, the overexpression of At DREB1 in Solanum tuberosum L. (potato) enhanced tolerance to the fungal pest, Fusarium solani (Mart.) Sacc. (1881) (Charfeddine et al., 2015). In contrast, in transgenic Arabidopsis, overexpression of MsDREB2C improved drought stress tolerance but caused greater sensitivity to the pathogenic bacterium, Pst DC3000 (Pseudomonas syringae Van Hall, 1904 pv. tomato DC3000), and to the pathogenic fungus, Alternaria mali Roberts (1914), compared to WT plants (Zhao et al., 2013). Therefore, a more comprehensive evaluation of DREBs is needed to assess their performance in transgenic plants under combined effects from abiotic and biotic stressors.
Although there are significant advances in our understanding of drought tolerance in trees, especially through model systems such as poplars, there remains a limited number of field-based studies on water stress in transgenic trees, particularly under natural conditions. With respect to DREBs specifically, there have been few studies on the A-6 subgroup in woody plants representing either model or non-model systems. In a study on apples, overexpression of an A-6 subfamily member, MsDREB6.2, was found to confer drought tolerance (Liao et al., 2017), similarly to its homolog in Arabidopsis, RAP2.4 (Lin et al., 2008). This result suggests that A-6 DREBs may be involved in stress tolerance in woody plants as they are in herbaceous species. Nevertheless, data are largely lacking, and almost nothing is known about the negative effects of DREBs on growth in transgenic trees or the interactions of DREBs with the biotic stress response. Overall, more tree transgenesis is urgently needed to link physiology, systems biology, and field performance to the benefit of silviculture industries.
In the present study, we investigated the effect of different expression levels of LbDREB6, a DREB of the A-6 clade from Limonium bicolor (Bunge) Kuntze, on plant growth, drought tolerance, and pathogen susceptibility in transgenic Populus ussuriensis Komarov under natural water-deficient conditions. We found that moderate overexpression of LbDREB6 increased drought tolerance but did not affect plant growth and pathogen susceptibility, while high levels of overexpression of LbDREB6 caused stunted growth and increased sensitivity to pathogen infections compared to the WT. Our study provides a basis for using genetic modification to improve drought tolerance in poplar while avoiding other adverse traits such as growth inhibition and reduction of disease tolerance, which may have negative implications for woody crop plants.
Materials and Methods
Plant Materials and Growth Conditions
Populus ussuriensis clone Donglin plants were grown in vitro on 1/2 MS semi-solid medium, with 0.6% w/v agar and 2% w/v sucrose, under irradiation of 16 h-light/8 h-dark cycles with PPFD of 46 μmol m–2⋅s–1 at 25°C. The cuttings were subcultured at 4-week intervals.
Vector Construction and Populus Transformation
The coding region of LbDREB6 (Ban et al., 2011) was fused into ProkII vector under the control of CaMV35S promoter. The binary vector ProkII carried the gene encoding neomycin phosphotransferase as a selection marker. The vector was introduced into Agrobacterium strain EHA105 prior to transformation by leaf discs using the method as described by Zhao et al., 2017. Transformants were selected on media containing 50 mg/L kanamycin. All transgenic plants were confirmed by genomic PCR and by mRNA qRT-PCR. qRT-PCR was used to quantify DREB6 transcripts using a conserved sequence (F: TAAGTGGGTGGCTGAGAT; R: CCTTCGGAACCGAATACTG). The P. ussurensis PuActin gene (GeneBank accession number: MH644084) was used as the reference gene. All the primers are listed in Supplementary Table 1.
All regenerated WT and transgenic plantlets with well-developed leaf and root systems were transferred to the same size pots containing an autoclaved sand and soil mixture (1:3 v/v) at the same growth period. Potted plants were covered with transparent plastic to maintain high humidity and incubated in a growth chamber at 21°C under a 16 h photoperiod for 2 weeks, and then the cover was removed. The growth characterization was recorded after 3 months. The high-level LbDREB6 overexpression lines (OE18, OE32, and OE35) and moderate-level LbDREB6 overexpression lines (OE10, OE14, and OE30) plants were used in the following assay.
Western Blotting Analysis
Western blotting was performed as described by Chen et al. (2008). An anti-LbDREB6 polyclonal antibody (goat anti-mouse biotinylated immunoglobulin) was used to recognize LbDREB6. The antibody was prepared by the Institute of Genetics and Developmental Biology, Chinese Academy of Sciences. Immunoreactive polypeptides were visualized by using the Western ECL blotting substrate BeyoECL Moon (Beyotime, China). Imaging was performed using a LAS-4000 imaging system (Fijifilm Life Science, United States).
Growth Characteristics
Hand cut sections of the youngest fully developed leaves of WT, OE18, and OE14 plants that were cultured in a flask were cut transversely at the middle of the leaf and fixed with 4% formaldehyde in phosphate-buffered saline with 0.5% TritonX-100 for 4 h at 23°C in a tube roller. After three 10-min washes, sections were mounted in 0.1 mg⋅mL–1 Calcofluor White in water and imaged using Olympus microscopy (SZX7). Perimeters of leaf parenchyma cells were manually traced in Olympus Fluoview software and plotted. Using the same software, the area of hand cut sections of WT and transgenic plant stems was calculated. The same method was used for cross sections of the stems. For scanning electron microscopy (SEM), stem segments of 1-year-old plants grown in a greenhouse (OE18 and OE14) were glued onto aluminum stubs and placed on a chamber stage that had been precooled to −120°C. The samples were viewed using a VP-SEM instrument (S-3500N, Hitachi, Tokyo, Japan). After the regenerated plantlets were transferred to a greenhouse for 2 months, their height and leaf width of were investigated. Meanwhile, the gibberellic acid (GA) content of shoot tip samples was analyzed following the protocol instructions of the Plant Gibberellic Acid, GA, ELISA kit (San Diego, CA, United States). For GA treatment, plantlets transplanted for 2 months in a greenhouse under natural sunlight were sprayed daily with 100 μM GA3 for 15 days. After 1 month, the height of WT, OE14, and OE18 plantlets was measured. For each experiment, 10 samples were used with three replicates. Data are presented as means of three biological replicates and error bars represent ±SD.
Drought Tolerance Assay
For plant growth analysis under drought stress, 6-month-old plants grown in pots in the greenhouse were used to conduct experiments. WT, OE14, and OE18 plants were grown under drought conditions for 2 weeks, and re-watered under normal conditions for a 2-week recovery period. The relative water content (RWC,%), chlorophyll content and gas exchange were investigated under drought stress. The growth height of WT and transgenic plant lines were also investigated after re-watering for 2 weeks. Additionally, we cultured the sterile cutting seedling in a flask under 7% PEG6000 treatment for 2 days. All the experiments were repeated three times.
Physiological and Biochemical Analysis
Before conducting the following experiments, plants were subjected to drought contains for 5 days in a greenhouse. Leaf RWC and chlorophyll were extracted and analyzed according to a previously published method (Wang et al., 2016). Leaf gas exchange was measured using a portable open gas exchange system (Li-6400; Li-Cor, Inc., Lincoln, NE, United States) equipped with a light source (Li-6200-02B LED; Li-Cor). The transpiration rate (Tr), stomatal conductance (Gs), and net CO2 assimilation were measured in mature leaves between 0 and 5 days after drought stress. Environmental conditions in the leaf chamber consisted of a photosynthetic photon flux density of 1,400 μmol m–2⋅s–1, an air temperature of 25°C, and an ambient CO2 concentration of 400 μmol mol–1. Leaf malondialdehyde (MDA) content was determined as described by Chen et al. (2008). The electrolyte leakage (EL,%) was used to estimate cellular membrane stability, which was detected following the methods described by Gu et al. (2017). Leaf hydrogen peroxide levels were determined as described by Yin et al. (2010). The absorption of the supernatant was read at 390 nm, and the content of H2O2 was quantified based on the standard curve. All the experiments were repeated three times.
Inoculation of Poplar With Marssonina Brunnea
Individuals of approximately equal size from WT, OE10, OE14, OE30, OE18, OE32, and OE35 lines after transplantation for 2 months in a growth chamber (25°C temperature, 70% humidity, 16 h/8 h light/dark cycle) were used for inoculation experiments. Plants were inoculated by thoroughly spraying a spore suspension of M. brunnea (±105 spores mL–1) onto the abaxial leaf surfaces according to the method used by Ullah et al. (2017). Immediately after spraying, each plant was covered with a polyethylene terephthalate bag to maintain high humidity; this was kept in the dark to facilitate spore germination. After 24 h, the bags were removed. Control seedlings were sprayed with distilled water. Leaf infection grade was set according to Zhao et al. (2017). All the experiments were repeated three times.
Transcriptome Analysis
We performed transcriptome analysis on two groups. One group was the fully expanded leaves, respectively, collected from WT and transgenic plant lines (OE14 and OE18) after drought treatment for 7 days in a greenhouse, and the other group was the apical meristem with two unexpanded young leaves which were harvested from WT, OE14, and OE18 plants after being inoculated with the pathogen M. brunnea, for 2 days; samples were immediately frozen in liquid nitrogen for RNA extraction. For each sample, ∼20 μg total RNA of each sample was sent to Annoroad Gene Technology Co., Ltd., Beijing, China for high throughout Illumina HiSeq 4000 sequencing (Illumina, San Diego, CA, United States). Each sample was conducted with three biological replicates and three technical replicates. The clean reads were mapped to P. trichocarpa mRNA reference sequence using TopHat (ver. 2.0.9) software (Trapnell et al., 2009). Transcript expression level is measured as fragments per kilobase of transcript per million mapped reads by Cufflinks (Trapnell et al., 2010). The reads per kilobase million (RPKM) value was used as the threshold for judging whether the gene was expressed; | log2FC| >1.0, P < 0.01, and a false discovery rate (FDR) < 0.01 were used as a threshold for the differential expression of genes to screen for DEGs. The DEGs and their encoded proteins were annotated by comparing them against NCBI, the Swiss-Prot database, NCBI non-redundant nucleotide sequence database, and non-redundant protein database. Then, the DEGs were imported into the Blast2 GO program (Götz et al., 2008) to identify gene ontology (GO) terms. Singular enrichment analysis in agriGO database1 was performed to identify significantly enriched GO terms. KEGG Orthology-Based Annotation System 2.0 (KOBAS,2) was utilized to identify significantly enriched pathways (Wu et al., 2006; Xie et al., 2011).
qRT-PCR Analysis
RNA was extracted from leaves of WT and transgenic lines of Populus exposed to drought stress for 7 days. Randomly selected 17 drought responsive genes in OE14 and OE18 compared to WT plants and six genes related to drought stress in OE18 compared to OE14 plants were verified by qRT-PCR, respectively. The primers used are listed in Supplementary Table 1. Furthermore, the selected 14 genes related to disease tolerance (PP2C, LRR-1, RPS2, RPM1, PYL, ABP19a, WRKY49, WRKY9, and NR) and GA20ox1 were verified by qRT-PCR. All the experiments were repeated three times. The primer sets used in this study to validate transcriptome data are given in the Supplementary Table 2. The raw sequence data of drought stress and pathogen infection RNA-seq experiments have been deposited in the Gene Expression Omnibus with the accession numbers GSE139373 and GSE120118, respectively.
Data Analysis
Statistical testing was performed with IBM SPSS Statistics 21 (IBM Corporation, Armonk, NY, United States). The data were tested by Student’s t-test (∗P < 0.05 or ∗∗P < 0.01).
Results
Effect of High-Level and Moderate-Level LbDREB6 Overexpression on Growth in Transgenic Populus
A total of 11 independent LbDREB6 overexpressing transgenic poplar lines were obtained by Agrobacterium-mediated genetic transformation and were analyzed through PCR. All lines had the expected 1,071 bp PCR product for the LbDREB6 gene (Supplementary Figure 1A). The qRT-PCR results indicated that the expression levels of LbDREB6 in transgenic plants were significantly higher than that in WT plants (Figure 1A). After transplantation for 2 months, the phenotyping experiment demonstrated that plants of most lines that had overexpression levels greater than 10 times that of WT plants were significantly shorter. In comparison, other transgenic lines with overexpression levels lower than 10 times that of the WT showed normal growth (Figure 1B). We chose three relatively moderate-level (OE10, OE14, and OE30) and three high-level (OE18, OE32, and OE35) LbDREB6 overexpression lines to conduct the following assays (Supplementary Figure 1B). The western blotting analysis using an anti-LbDREB6 antibody revealed that OE18 had a high LbDREB6 protein expression compared to OE14, which was consistent with the RT-PCR result (Supplementary Figure 1C).
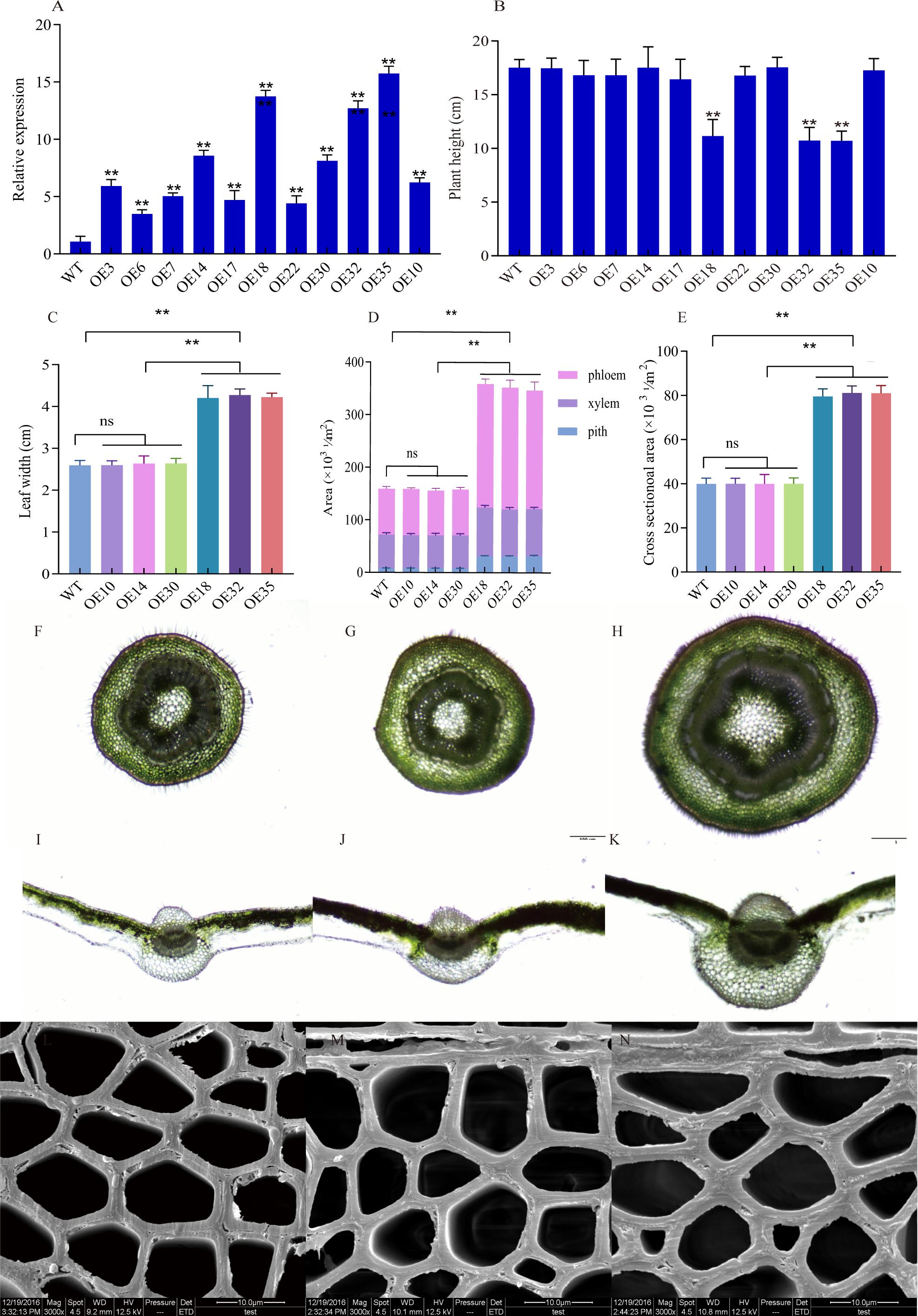
Figure 1. Growth characteristics, cross-section, and scanning electron microscopy (SEM) observation of high-level (OE18) and moderate-level (OE14) LbDREB6 overexpression lines and wild type (WT) P. ussuriensis plants. (A) qRT-PCR validations of OE18, OE14, and WT plants. PtrActin was used as an internal control. Data are presented as means of four biological replicates, and error bars represent ±SD. (B) Heights of OE18, OE14, and WT plants grown in greenhouse for 2 months. (C) Leaf widths of WT, OE18, and OE14 plants. (D) The quantification of sectioned areas representing bark, wood, and pith regions. (E) Midrib cross-sectional areas of WT, OE18, and OE14 plants. Samples were collected at 1 cm from the stem foundation. Cross-section of the stem in WT (F), OE14 (G), and OE18 (H) plants. Cross-section of the leaf midrib-xylem in WT (I), OE14 (J), and OE18 (K) plants. SEM observation of cell walls in WT (L), OE14 (M), and OE18 (N) plants. For (B–E), each value represents the mean of 20 plants with three biological replicates, and error bars represent ±SD. Asterisks indicate significant differences, *P < 0.05 and **P < 0.01.
Leaf width of OE18 plants was significantly larger than that of WT plants (Figure 1C), while the leaf width was not considerably different between OE14 and WT plants (Figure 1C). Leaf and stem sizes are dependent on both the number and the size of cells in the organ (Zhou et al., 2013). Cross sections of stems revealed that the primary and secondary xylem rings of OE14 (Figures 1D,G) were not significantly different from WT plants (Figures 1D,F), while OE18 stems (Figures 1D,H) increased significantly compared to the WT plants (Figures 1D,F). As quantified by the cross-sectional area measurements, the leaf veins of OE14 (Figures 1E,J) was not significantly different from WT plants (Figures 1E,I), but the leaf veins of OE18 (Figures 1E,K) were 40∼50% larger than the WT plants (Figures 1E,I). SEM observation showed that cell walls of OE14 (Figure 1M) were not significantly different from WT plants (Figure 1L). However, the cell walls of OE18 (Figure 1N) were considerably thicker than WT plants (Figure 1L).
Comparison of High-Level and Moderate-Level LbDREB6 Overexpression on Drought Tolerance
We examined the effects of drought stress on WT and transgenic poplar plants after growth in a greenhouse for 6 months. Under well-watered conditions, performance did not differ between the WT and the transgenic lines. However, after 7 days of drought stress, leaves of WT plants exhibited extensive dehydration symptoms, but only slight wilting was observed in OE14 and no effect was seen in OE18 (Figure 2A). After 14 days of drought stress, most of the WT leaves were chlorotic, while OE14 plant lines had less damage compared to WT plants, and most of the OE18 lines were green and vigorous (Figures 2B–D). After re-watering for 10 days, OE14 plants recovered faster than the WT plants (Figures 2E–H).
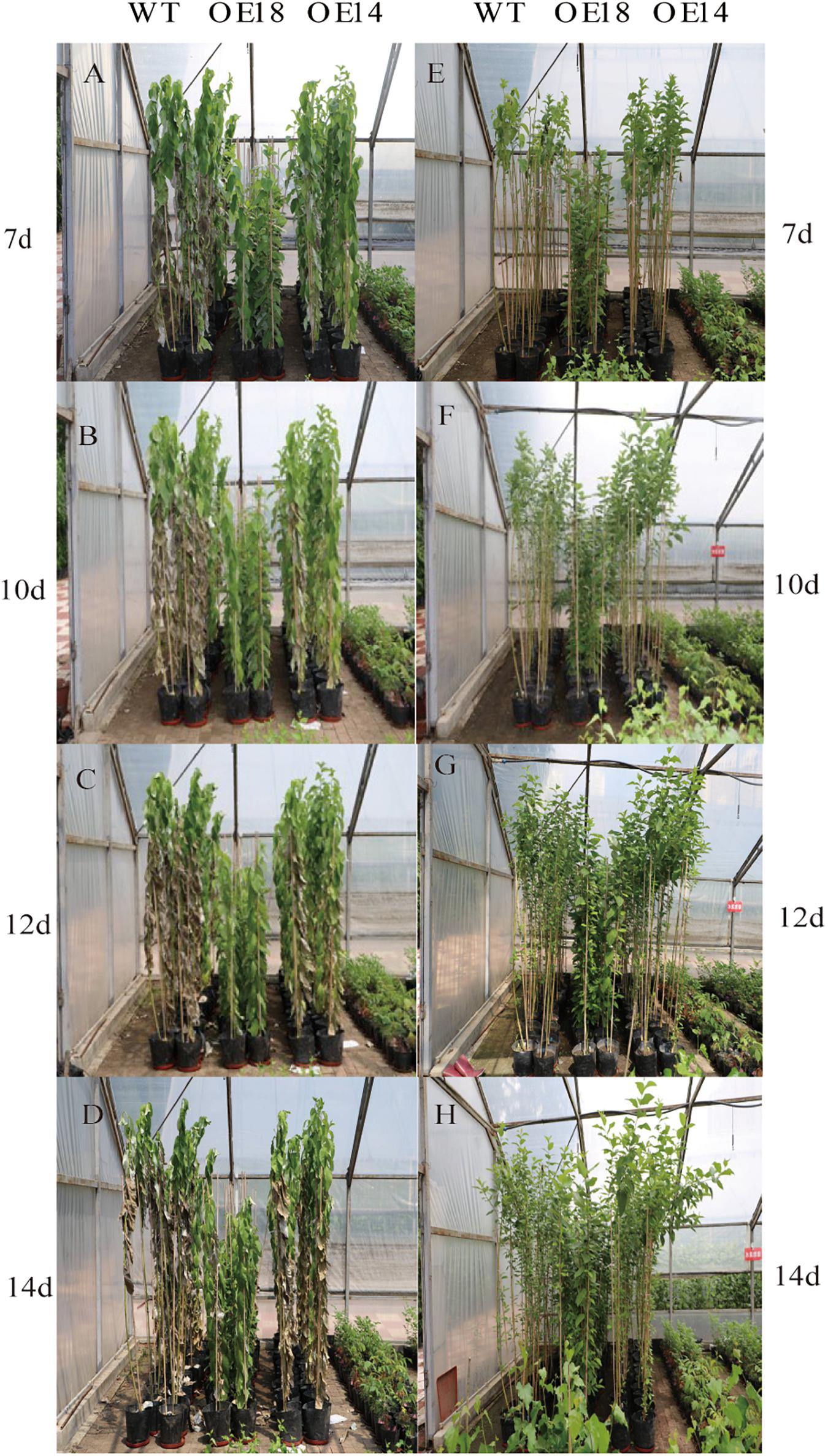
Figure 2. Phenotypic observation of WT and transgenic plant lines under drought stress for (A) 7d, (B) 10d, (C) 12d, (D) 14d. Phenotypic observation of WT and transgenic plant lines re-watered for (E) 7d, (F) 10d, (G) 12d, (H) 14d.
During the drought period, the leaf RWC (%) showed a downward trend, especially for WT plants (Figure 3A). Furthermore, the chlorophyll level of the transgenic and WT plants also decreased, but the transgenic plants still had a higher chlorophyll content than WT plants (Figure 3B). Additionally, the transgenic and WT poplars had remarkable variations in transpiration rate (Tr), stomatal conductance (Gs), and net CO2 assimilation during the 5 days of drought stress. The Tr and Gs in the transgenic and WT poplars showed an overall decreasing trend but they decreased faster in WT plants than that in transgenic poplars after 2 days of drought stress (Figures 3C,D). The net CO2 assimilation in the transgenic and WT poplars both decreased, but the net CO2 assimilation in WT poplars decreased much more rapidly than that in the transgenic poplars after drought treatment (Figure 3E).
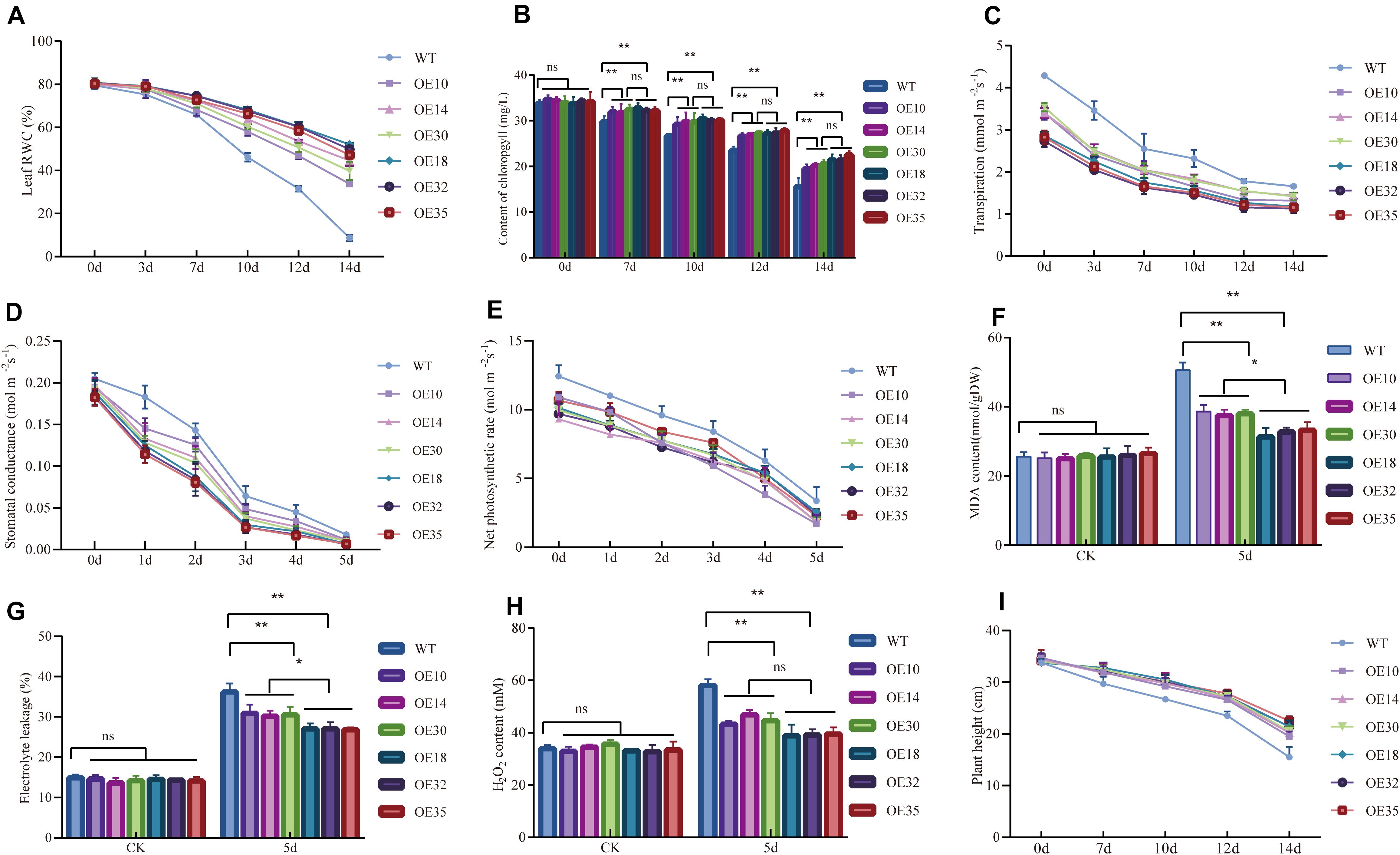
Figure 3. Physiological analysis of LbDREB6 overexpression lines and WT plants under drought stress. Measurement of leaf relative water content (RWC) (A), chlorophyll content (B), the transpiration (C), stomatal conductance (D), net CO2 assimilation (E), MDA content (F), electrolyte leakage (EL) (G), and H2O2 content (H). (I) Plant height after being re-watered for 2 weeks. For (I), each value represents the mean of 20 plants, and error bars represent ±SD. For (A) to (H), data are presented as means of three biological replicates, and error bars represent ±SD. Asterisks indicate significant differences, *P < 0.05 and **P < 0.01.
The MDA content (Figure 3F) and relative electrical conductance (EL) (Figure 3G) of WT and transgenic plants considerably increased after drought treatment. Among them, the MDA content (Figure 3F) and EL (Figure 3G) in transgenic plants decreased compared with WT plants. Meanwhile, MDA, and EL in high-level LbDREB6 overexpression plants were less than those in moderate-level LbDREB6 overexpression plants. The H2O2 content in the leaves of WT and transgenic plants was not significantly different compared to that before the treatment (Figure 3H). The H2O2 content in transgenic plant leaves was lower than that in WT plants after drought treatment, although both showed an increased H2O2 content (Figure 3H). Moreover, the H2O2 content was less in OE18, OE32, and OE35 compared to OE10, OE14, and OE30. After re-watering, the transgenic plants grew vigorously, and the height of OE14 was significantly higher than the WT plants (Figure 3I), while, WT plants were affected much more seriously and grew slowly (Figure 3I). We also verified the result in the flask, after 7% PEG6000 treatment for 2 days, the leaves of the WT (Supplementary Figure 2A) were damaged early and seriously, but the OE14 (Supplementary Figure 2B) and OE18 (Supplementary Figure 2C) plants still grew normally. This result was consistent with that of the experiment conducted in the greenhouse. Altogether, the transgenic poplars had better tolerance to drought stress than WT plants and high expression levels of LbDREB6 with less damage suffered from drought stress.
Expression Comparison of Downstream Target Genes Between OE14 and OE18 Leaf Transcriptomes in Response to Drought Stress
We used the Illumina sequencing platform to sequence the leaf transcriptomes of the WT and LbDREB6-transgenic plants under drought stress for 7 days. We identified a total of 20698 differentially expressed genes (DEGs) during drought stress (P < 0.05 and FDR < 0.001). Among these DEGs, there were 2,766 (1,654 up, 1,112 down, Supplementary Data 1) in OE14 and 9,442 (5,862 up, 3,580 down, Supplementary Data 2) in OE18 compared to the WT, respectively, and 8,490 (5,213 up, 3,277 down, Supplementary Data 3) were identified in OE18 compared to OE14 plants. Finally, a total of 879 DEGs were common in three groups (Supplementary Data 4 and Figure 4A). Of the common 879 DEGs, there were 707 up-regulated and 172 down-regulated genes; we found that multiple drought stress related downstream genes were differentially expressed, such as aquaporin (AQP) tonoplast intrinsic protein (TIP), glutathione S-transferase (GST), and some transcriptional factors. To verify the NGS data, the expression levels of eight genes were examined in WT, OE14, and OE18 plants with drought treatment by qRT-PCR (Figures 4B–H). It was found that the expression levels of all the genes tested were increased in the transgenic plants compared to the WT plants under drought treatment except ABA8’OH and WRKY46 in OE18 (Figure 4G). Among them, the expression levels of seven genes were higher in OE18 than those in OE14 plants (Figures 4B–F,H–L), and there were no significant differences in expression levels of the five genes between OE14 and OE18 plants (Supplementary Figures 4A–E) except WRKY46 (Supplementary Figure 4F). It was likely that these DEGs resulted in drought resistance of OE18 and OE14 when compared to WT plants. In OE18, we also found some DEGs that were probably related to the drought tolerance phenotype when compared to OE14 plants (Supplementary Data 3). Most of these DEGs were up-regulated, for example, major intrinsic protein (MIP) (Potri.003G050900, Potri.005G109300, and Potri.006G121700), cellulose synthase-like protein D5 (CSLD5) (Potri.014G125100), 2 galactinol synthase genes (GolS) (Potri.002G191600 and Potri.010G150400), and 5 late embryogenesis abundant (LEA) protein genes (Potri.018G052500, Potri.002G124600, Potri.009G003800, Potri.010G012100, and Potri.010G012100) responded to water deprivation; 2 peroxidase genes (Potri.007G122100 and Potri.018G015500), thioredoxin gene (Potri.001G028500), peptide methionine sulfoxide reductase B5 (MSRB5) (Potri.008G198600), L-ascorbate oxidase (Potri.004G010100), and peptide methionine sulfoxide reductase (MSRA) (Potri.T135400) respond to oxidative stress; 3 ABP19a (Potri.013G141900, Potri.001G169000, and Potri.003G065300), Metalloendoproteinase 2-MMP (Potri.019G073800), and 2 GST (Potri.011G140400 and Potri.011G140600) genes were involved in auxin-activated signaling pathways and development processes. We randomly selected some related genes to conduct qRT-PCR verification. The qRT-PCR analysis result showed that ABP19a (Potri.001G169000), TIP2-1 (Potri.003G050900), PIP2-8 (Potri.005G109300), LEA (Potri.018G052500), and Peroxidase 18 (Potri.018G015500) were highly induced in OE18 compared to OE14 except GolS (Potri.010G15040) (Supplementary Figure 5). These results were consistent with the transcriptome data.
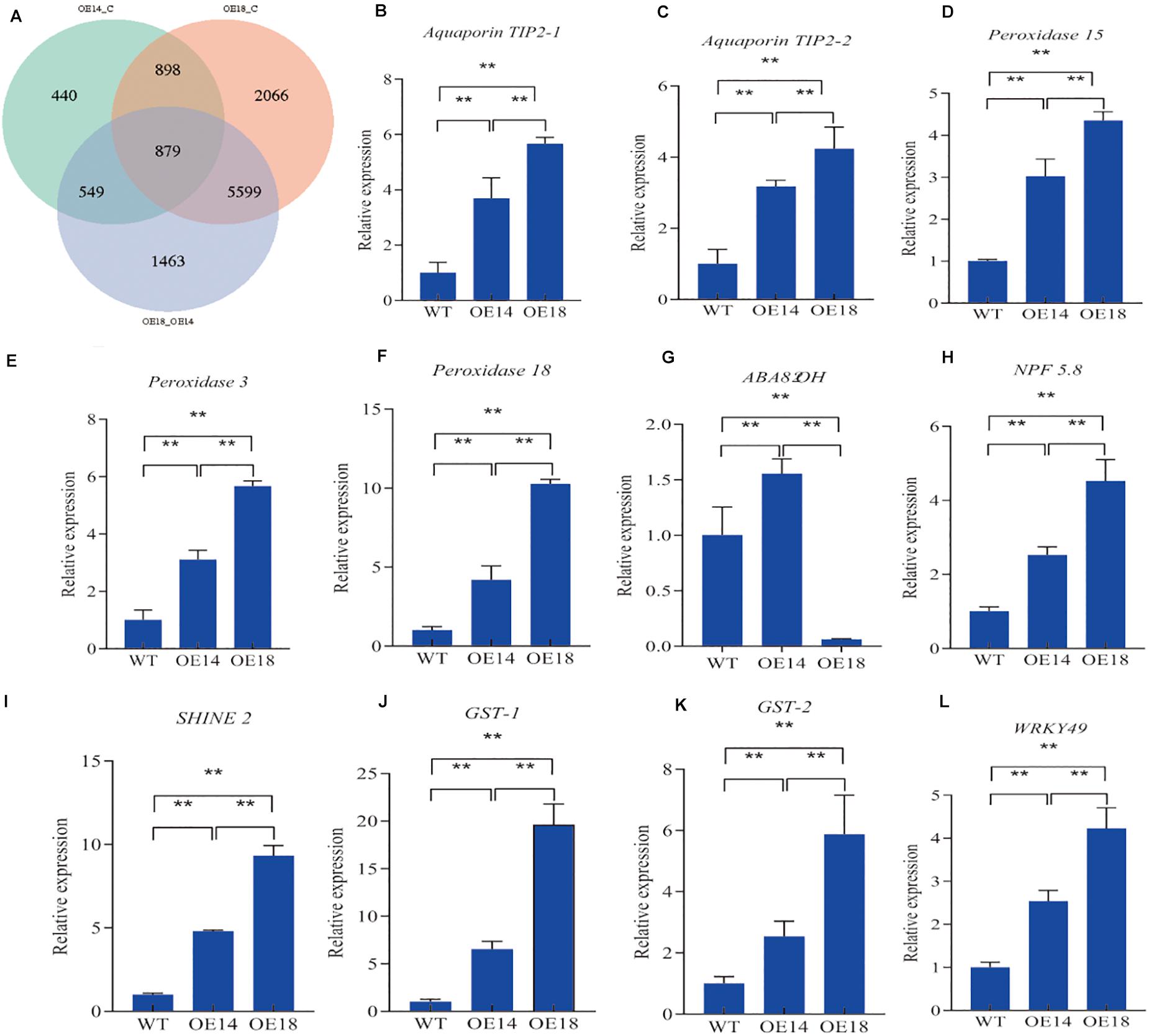
Figure 4. Quantitative real-time PCR analyses of the transcript levels of eight selected DEGs co-up-regulated in high-level (OE18) and moderate-level (OE14) LbDREB6 overexpression lines compared with WT plants under drought stress for 5 days. (A) Venn diagram among WT and OE14, WT and OE18, and OE14 and OE18 plants. Quantitative real-time PCR analyses of the transcript levels of (B) Aquaporin TIP2-1, (C) Aquaporin TIP2-2, (D) Peroxidase 15, (E) Peroxidase 3, (F) Peroxidase 18, (G) ABA8’OH, (H) NPF 5.8, (I) SHINE2, (J) GST-1, (K) GST-2, (L) WRKY49. Data are presented as means of three biological replicates, and error bars represent ±SD. Asterisks indicate significant differences, *P < 0.05 and **P < 0.01.
Effect of High- and Moderate-Level LbDREB6 Overexpression on Susceptibility to Marssonina Brunnea
After inoculation with the fungus M. brunnea, the OE14 line (Figures 5B,D) displayed no significant difference in phenotype compared to the WT (Figures 5A,D), while the lesion area on OE18 was larger than that of the WT (Figures 5C,D). This suggests that the enhanced expression level of LbDREB6 affects the susceptibility of poplar to the fungus. The MDA content of WT, OE14, and OE18 lines considerably increased after pathogen treatment (Figure 5E); the MDA content in OE18 plants was greater than that in WT plants compared to OE14 plants (Figure 5E). In addition, the relative EL showed the same trend of change (Figure 5F), indicating the leaves of OE18 plants showed severe membrane damage compared to OE14 plants. Overall, the susceptibility to M. brunnea infection in OE18 increased and that in OE14 was not altered compared to WT plants.
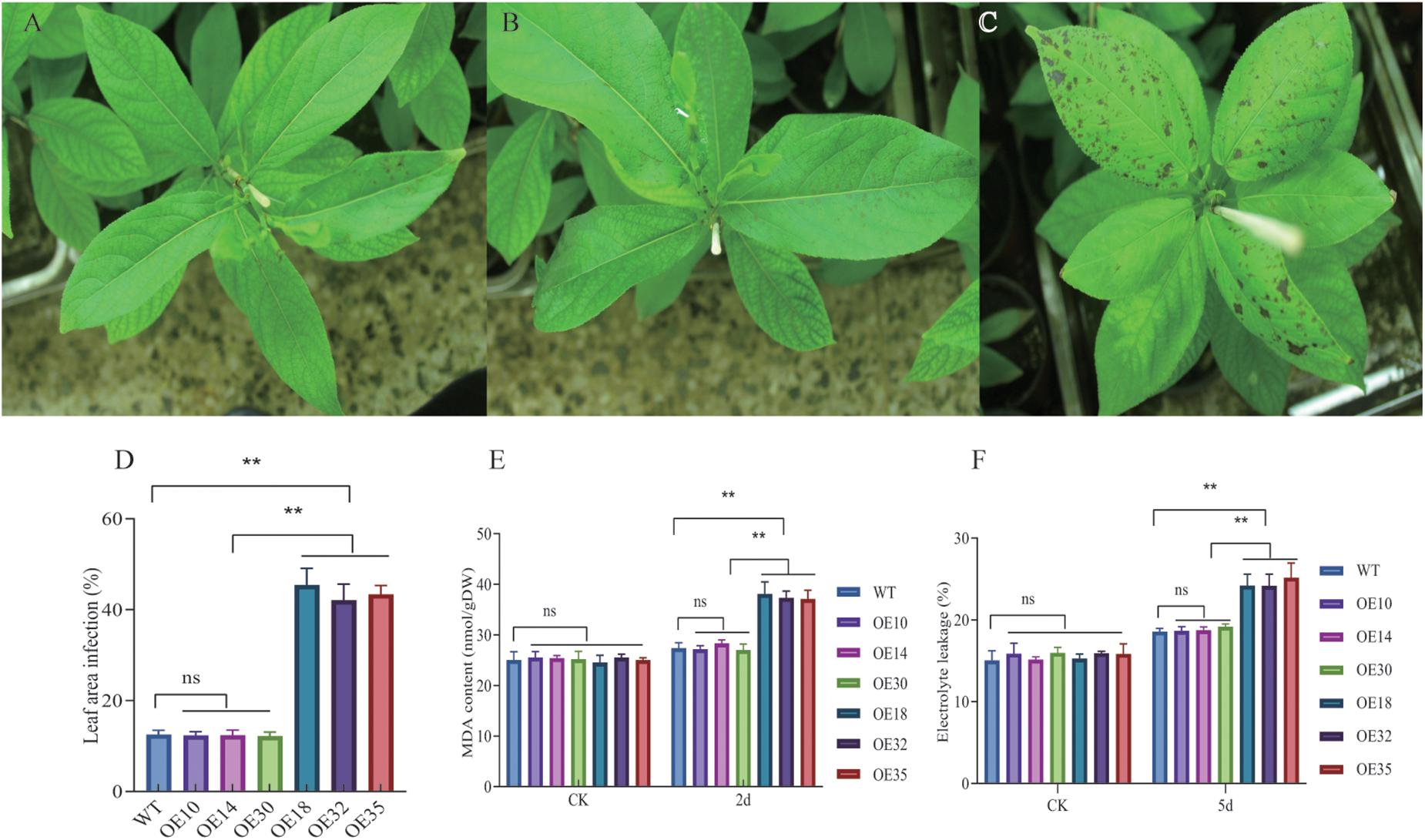
Figure 5. Comparison of LbDREB6 overexpression lines and WT leaves after Marssonina brunnea infection. The WT (A), OE14 (B), and OE18 (C) plants after being infected with M. brunnea for 2 days. Bar = 2cm. (D) Measurement of leaf disease indices. Each value represents the mean of 20 plants, and error bars represent ±SD. Measurement of MDA content (E) and electrolyte leakage (EL) (F) after being infected with M. brunnea for 2 days. Data are presented as means of three biological replicates, and error bars represent ±SD. Asterisks indicate significant differences, *P < 0.05 and **P < 0.01.
Comparison of OE18 and OE14 on Expression of Genes Involved in Disease Tolerance Under Pathogen Infection
To determine the possible pathways of LbDREB6 in pathogen defense, we conducted transcriptomic sequencing of leaf inoculated with the pathogen M. brunnea, for two days, from which we obtained 53.20 Gb clean reads (18.2–24.7 million per library, Q30 ≥ 95.02%). Approximately 68.3–70.42% clean reads per library could be mapped to the P. trichocarpa genome (Supplementary Table 3). The quality of the assembled transcriptome was appropriate for functional annotation and further analysis compared with the WT.
Based on the transcriptome annotation, a total of 688 DEGs were identified in OE18 when compared to both the WT and OE14 plants (Figure 6A and Supplementary Data 3). In total, 58 DEGs related to disease tolerance were identified in OE18 plants including 41 up-regulated and 17 down-regulated genes (Supplementary Data 3). Plants have two different plant–pathogen interaction sub-pathways, pattern triggered immunity (PTI) and effector-triggered immunity (ETI) (He et al., 2015). In PTI, there were 17 genes differently expressed in OE18 when compared to both the WT and OE14 plants, including translated nucleotide-binding leucine-rich repeat (NB-LRR) kinase EFR, two WRKY TFs (WRKY49 and WRKY7), three ubiquitin E3 ligases, and some receptor-like proteins involved in immune response and regulating the expression downstream defense-related genes. In ETI, we found that two disease tolerance proteins RPM1 and one disease tolerance protein RPS2 were down-regulated. In addition, two nitrite reductase differentially induced genes were down-regulated in OE18 when compared to both the WT and OE14 plants. KEGG enrichment revealed that DEGs related to plant hormone signal transduction, followed by plant–pathogen interactions and biosynthesis of amino acids were enriched in OE18 plants when compared to both the WT and OE14 plants (Figure 6B); similarly, plant hormone signal transduction and plant–pathogen interactions were enriched in OE18 plants compared with both WT and OE14 plants (Figure 6C). In plant hormone pathways, one PYR/PYL family protein, two protein phosphatases 2C (PP2C), and two auxin-binding proteins ABP19a were also differentially expressed.
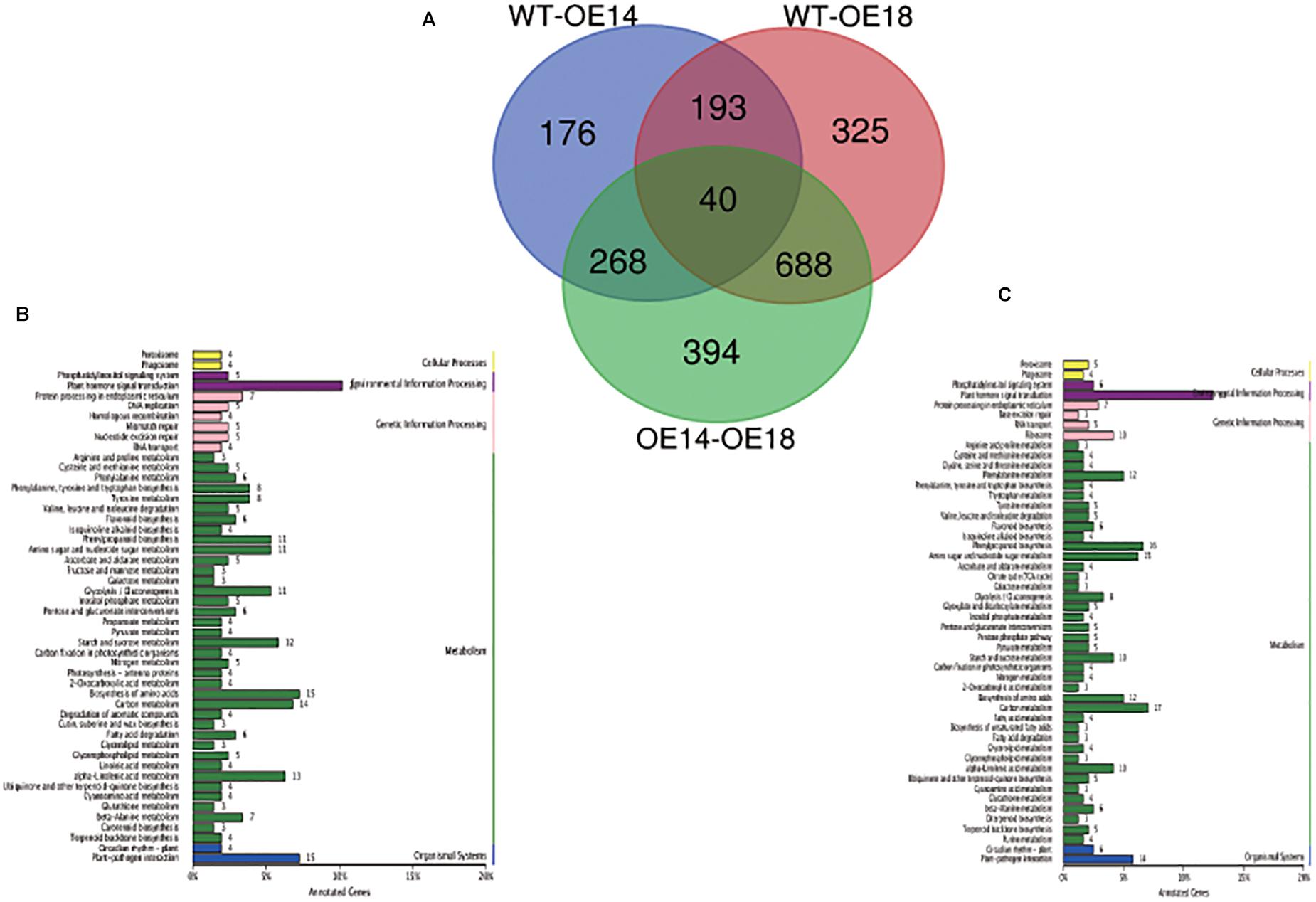
Figure 6. Venn diagram and KEGG classification of the DEGs among high-level (OE18) and moderate-level (OE14) LbDREB6 overexpression lines and WT P. ussuriensis plants after infection with Marssonina brunnea. (A) Venn diagram among WT and OE14, WT and OE18, and OE14, and OE18 plants. (B) KEGG classification of differentially expressed genes between WT and OE18 lines. (C) KEGG classification of differentially expressed genes between OE14 and OE18 lines.
We selected some DEGs involved in plant–pathogen interaction pathways and ABA regulation to conduct qRT-PCR analysis. The result showed PP2C-1, NB-LRR-1, NB-LRR-2, RPS2, RPM1-1, RPM1-2, PYL4, WRKY49, and NR genes were down-regulated in OE18 plants when compared to both the WT and OE14 plants (Figure 7).
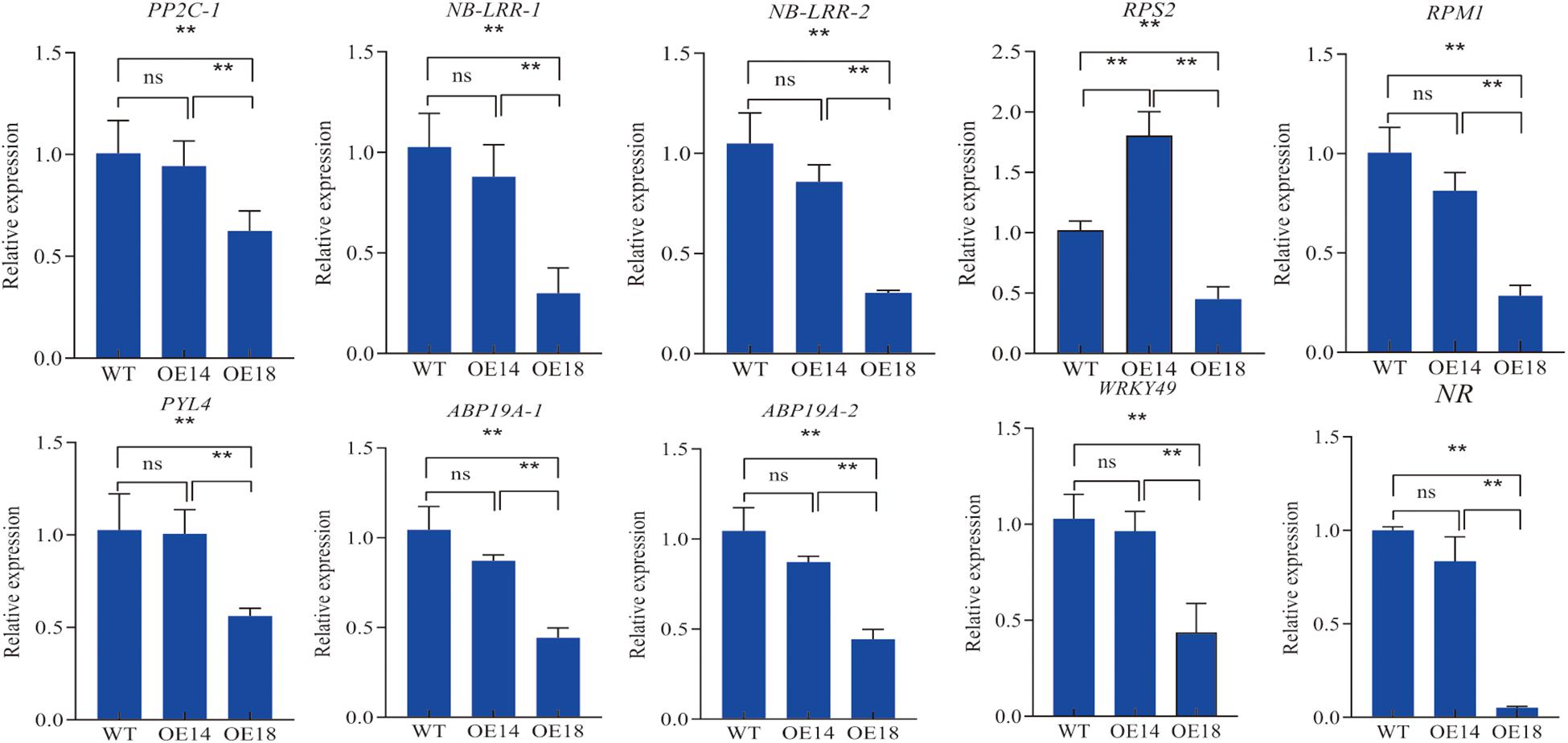
Figure 7. Quantitative real-time PCR analyses of the transcript levels of disease tolerance related genes in leaves of high-level (OE18) and moderate-level (OE14) LbDREB6 overexpression lines and WT P. ussuriensis plants under M. brunnea infection treatment. Data are presented as means of three biological replicates, and error bars represent ±SD. Asterisks indicate significant differences, *P < 0.05 and **P < 0.01.
Effect of High-Level and Moderate-Level LbDREB6 Overexpression on GA20ox1 Gene Expression
We found that the GA 20-oxidase 1 (GA20ox1) gene was down-regulated in OE18 plants when compared to both WT and OE14 plants from the shoot tip transcriptome dataset. The qRT-PCR results showed that the expression level of GA20ox1 in OE18 was decreased to ∼50 and 49% when compared to the WT plants and OE14, respectively (Figure 8A). However, the expression of GA20ox1 was not significantly different between the OE14 and WT plants (Figure 8A). To investigate if there is a difference in GA content between WT and transgenic plants, all samples of shoot tips were harvested after plants were transplanted into pots for 2 months. There was no significant difference between the WT or moderate-level LbDREB6 overexpression plants, but GA in high-level LbDREB6 overexpression plants was significantly lower, by ∼25%, when compared to the WT plants (Figure 8B). This was consistent with the gene expression pattern of GA20ox1 in the samples (Figure 8A). To examine whether the dwarf phenotype was caused by GA deficiency, we cultivated WT and transgenic plantlets transplanted in a greenhouse under natural sunlight for 2 months and they were sprayed daily with 100 μM GA3 for 15 days. Under these conditions, the height of the transgenic as well as the WT plants increased rapidly, especially for OE18 plants (Supplementary Figure 3). The increased ratio of OE18 plant height was higher than that of WT plants (Supplementary Figure 3). These results demonstrated that high-level overexpression of LbDREB6 decreased growth rate, probably by inhibiting GA synthesis, while moderate overexpression of LbDREB6 did not change the normal growth of transgenic P. ussuriensis.
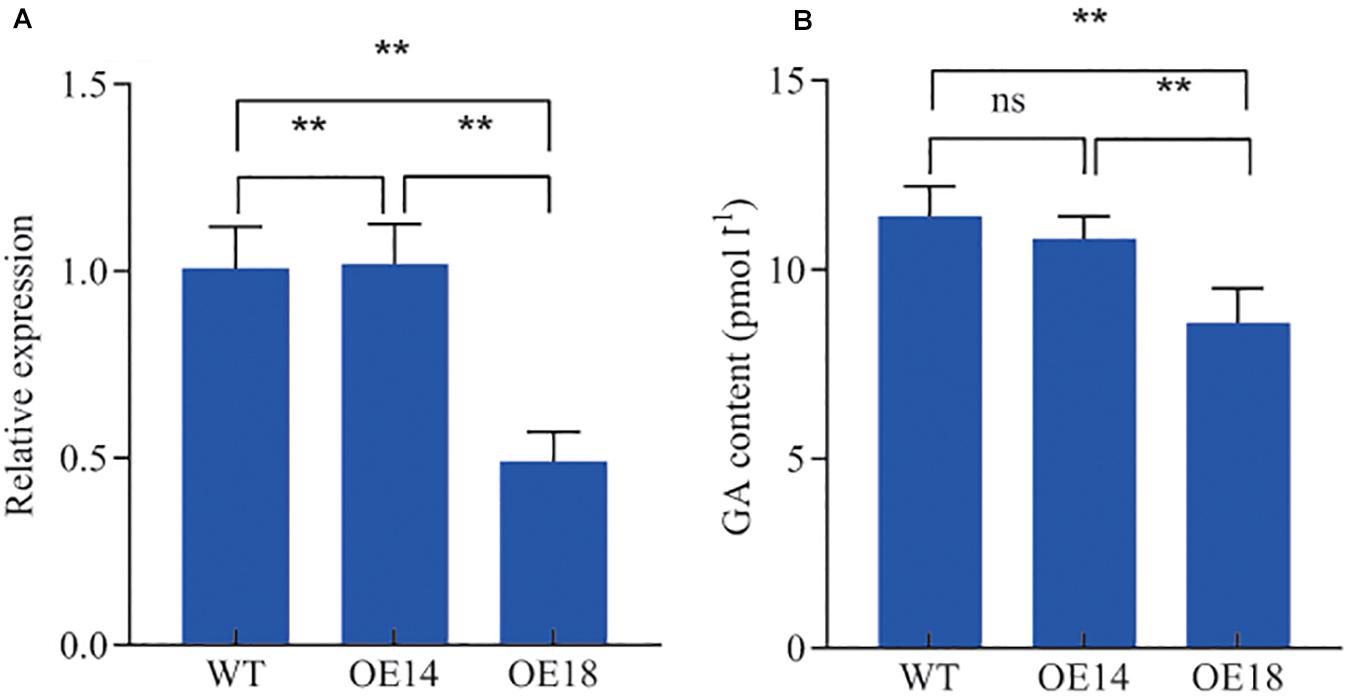
Figure 8. Quantitative real-time PCR analyses of the transcript levels of the GA20ox1 gene and GA content detection in leaves of high-level (OE18) and moderate-level (OE14) LbDREB6 overexpression lines and WT P. ussuriensis plants. (A) qRT-PCR analysis of the GA20ox1 gene. (B) GA content determination in leaves of WT and transgenic plants. Data are presented as means of three biological replicates, and error bars represent ±SD. Asterisks indicate significant differences, *P < 0.05 and **P < 0.01.
Discussion
Increasingly, numerous studies are showing that DREBs have crucial roles in regulating plant development and responses to both abiotic and biotic stresses. However, there have been only a few studies to date to investigate the multi-directional effects of DREBs in trees. In the present study, we overexpressed LbDREB6 in poplar to investigate the role of this A-6 DREB TF in regulating plant growth, drought tolerance, and disease tolerance. We found that in the transgenic line of poplar with high levels of overexpression of LbDREB6, drought tolerance was greatly improved, but growth was inhibited, and there was a decreased tolerance to fungal pathogens. In contrast, in the plants having a moderate level of overexpression of LbDREB6, we observed drought tolerance along with normal growth and no effects on pathogen tolerance. Our study suggests that drought tolerance can be improved by carefully adjusting the levels of overexpression of DREB TF genes to avoid the occurrence of unfavorable effects.
The abilities of plants to respond to drought stress can sometimes be predicted by their varying capacities to modulate key physiological and biochemical responses at the cellular level, including changes in membrane integrity, internal water balance, and the accumulation of osmolytes and antioxidants (Kang et al., 2011). MDA, the final product of lipid-peroxidation, is one well-known marker of cell membrane injury (Taulavuori et al., 2001; Anjum et al., 2015), while accumulation of reactive oxygen species (ROS) can be scavenged by antioxidants (Royer and Noctor, 2003). Our results showed that the levels of MDA, H2O2, and ROS, in plants overexpressing LbDREB6 under water deficit conditions were lower compared to WT plants. The lower levels of H2O2 may indicate that LbDREB6 conferred enhanced protection from oxidative damage and a better ROS scavenging ability in the transgenic plants. Lower MDA levels seem to indicate that the transgenic plants had greater membrane integrity to withstand cellular-level effects of water loss. Our results were similar to observations in 35S:MsDREB6.2-transgenic apple, which had improved tolerance to drought stress (Liao et al., 2017) and coincided with lower levels of ROS and MDA. Another measure of membrane integrity is EL (Gu et al., 2017). In this study, the lower rate of increase in EL in plants overexpressing LbDREB6 suggests that less cell damage occurred from drought stress. This physiological change within the overexpression lines may account for the higher RWC compared to WT plants under drought stress. These results indicate that the LbDREB6-overexpressing plants achieved better water balance to alleviate water deficiency and had higher drought tolerance compared to the non-transgenic WT plants. Additionally, both OE14 and OE18 showed better growth compared to WT plants under drought stress, though the OE18 line exhibited greater drought tolerance than OE14.
When the plants were subjected to drought stress, a large number of genes were differentially expressed compared to plants under normal growth conditions, and subsequently, many protein or metabolic products were generated to help protect the plant from drought stress. In this study, overexpression of LbDREB6 yielded increased transcription of many downstream genes. This is expected because TFs like LbDREB6 are significant upstream regulatory proteins, which play a major role in multiple pathways when plants are subjected to drought stress (Ning et al., 2011). Prior studies also revealed that transcription of downstream genes was increased when DREB TFs were up-regulated. For example, MdSHINE2 from apple (a homolog of AtSHINE2 in Arabidopsis), an A-6 DREB, conferred drought tolerance by regulating wax biosynthesis (Zhang et al., 2019). Similarly, Arabidopsis transformed with GmWRKY54, an A-6 DREB from soybean, conferred salt and drought tolerance, possibly through the regulation of DREB2A and STZ/Zat10 (Zhou et al., 2008). Additionally, RAP2.4 in Arabidopsis regulated the expression of the plasma membrane intrinsic protein (PIP) and TIP subfamilies of AQPs, which are integral membrane proteins, in response to drought stress (Rae et al., 2011). AQPs, especially PIP and TIP, are believed to play key roles in maintaining water homeostasis (Alexandersson et al., 2005; Rae et al., 2011).
In the current study, we found differential expression of several genes involved in drought response pathways between the transgenic lines and the WT. Notably, we found that two GST genes, which constitute part of an antioxidant defense system, were induced in transgenic LbDREB6 overexpression lines. Activation of the GST antioxidant system occurred in response to ROS (Noctor et al., 2014; Fox et al., 2017) and indicates that the poplar trees were protected from ROS that resulted from drought. We also found that a key regulator of ABA catabolism, ABA8’OH (Potri.004G235400), was down-regulated in the OE18 line when compared to OE14. ABA is an important phytohormone that regulates plant water use, and thus, it is correlated with drought tolerance. While ABA can be applied exogenously to facilitate drought tolerance, this approach has limited utility because ABA is rapidly inactivated. Therefore, genes that regulate levels of endogenous ABA are of critical importance to develop drought resistant crops (Takeuchi et al., 2016). Down-regulation of ABA8’OH should decrease catabolism of ABA, and therefore probably improves drought tolerance in the OE18 line. Taken together, these results suggest that LbDREB6 can regulate large groups of stress-related genes, and subsequently, promote enhanced tolerance against drought stress.
Other genes known for their involvement in drought response, such as MIP, TIP, GolS, and LEA, showed a greater change in expression levels in the OE18 plants compared to OE14. GolS of Arabidopsis, GolSAt2, has been reported to confer drought tolerance and increase grain yield in transgenic rice (Oryza sativa L.) under dry field conditions (Selvaraj et al., 2017). LEA of wheat, TaLEA3, overexpressed in Phellodendrons amurense yielded tolerance to drought stress by rapid stomatal closure (Yang et al., 2018). Differential expression of homologs of these genes in OE18 may help to explain why this line is more drought resistant than OE14 at the transcriptional level.
Gibberellic acid, the most important hormone regulating shoot elongation, plays an important role in determining plant height (Richards et al., 2001). One critical gene family that participates in GA synthesis and degradation is GA20ox, an oxidase (Hedden and Phillips, 2000; Wuddineh et al., 2015). Decreasing the level of expression of GA20ox causes severe dwarf phenotypes in some species due to reduced levels of active GAs. In tomato, SlDREB is known to act as a positive regulator in drought stress responses, but overexpression caused the dwarf phenotype via down-regulation of GA20oxs as well as ent-copalyl diphosphate synthase (SlCPS), another gene in the GA synthesis pathway (Li et al., 2012). The dwarf phenotype also affected DREB1B/CBF1 transgenic tomato, which could be rescued by exogenous application of GA3 (Hsieh et al., 2002). The expression levels of GA biosynthetic genes, including OsGA20ox1, were significantly reduced in rice plants overexpressing JcDREB2 of Jatropha curcas Tang et al., 2017. Similarly, here, we found that the expression level of GA20ox1 in OE18 was decreased by ∼50% when compared to WT and OE14 plant lines, and OE18 showed lower GA content. The expression level of GA20ox1 was not significantly different between the WT and OE14, which were also similar in height. Therefore, it appears that dwarfism due to overexpression of LbDREB6 results from an interaction that down-regulates expression of the GA20ox gene family, and consequently, reduces the levels of GAs.
The poplar rust fungus, Marssonina brunnea (Ellis and Everh.) Magnus causes significant yield reduction and severe economic losses in commercial poplar plantations. In our study, OE18 was more sensitive to M. brunnea infection compared to both OE14 and WT plants, while we detected no difference between OE14 and WT plants. This suggests that tolerance to fungal infection was reduced by overexpression of LbDREB6. Briefly, plants have two tiers of responses to microbial and fungal pathogens: pattern triggered immunity (PTI) at epidermal layers in response to the pathogens themselves and effector triggered immunity (ETI), which responds to metabolites produced by pathogens after an infection is established (Boller and He, 2009). Both PTI and ETI recognize pathogen infection according to arrays of receptors that include kinases, TFs, and plant hormones (He et al., 2015). Additionally, pathogenic response in plants is mediated by plant hormones, such as salicylic acid, jasmonic acid, and ABA, which act as secondary messengers (Mauch-Mani and Mauch, 2005; He et al., 2015). We found that multiple pattern recognition receptors that participate in the PTI system, especially EF-Tu receptors (ETRs), were differentially expressed in the OE18 line, in which they were largely down-regulated compared to OE14 and WT plants. In particular, we observed that the ETRs, WRKY (WRKY49 and WRKY7), RPM1, RPS2, and NOS were down-regulated in OE18. WRKY TFs are known to play important roles in transcriptional reprogramming in response to various stressors including pathogen infection in plants. For example, in rice, OsWRKY67 improved tolerance against two pathogens, Magnaporthe oryzae (T. T. Hebert) M. E. Barr and Xanthomonas oryzae oryzae (Vo et al., 2018). NOS genes regulate the production of nitric oxide, which is involved in the plant pathogenic response (Arasimowicz-Jelonek and Floryszak-Wieczorek, 2014). Thus, down-regulation of NOS in OE18 likely led to reduced nitric oxide, and therefore, decreased pathogen tolerance. Additionally, several genes affecting levels of ABA were also down-regulated in OE18. Specifically, we found that ABA receptors, including one PYL4 and one PP2C, were down-regulated in OE18 as well as ABA responsive element binding factor (ABF), which encodes a basic leucine zipper TF. The PYR/PYL family of proteins positively regulate ABA response in various tissues by inhibiting the interaction of PP2C with a protein kinase, SnRK2 (Umezawa et al., 2009). Then, this complex stimulates the expression of ABF, which enhances tolerance to necrotrophic pathogens (Umezawa et al., 2009). Thus, we proposed that high levels of overexpression of LbDREB6 yielded decreased tolerance to M. brunnea due to effects on the PTI system and actions of ABA. However, this behavior of LbDREB6 merits further study.
Conclusion
In previous studies, the overexpression of DREBs in transgenic plants improved drought tolerance but with varying levels of growth inhibition under non-drought conditions. Our results show that the higher the overexpression level of the LbDREB6 gene, the stronger the drought tolerance in Populus ussuriensis. However, under high levels of overexpression of LbDREB6, poplar trees exhibited growth inhibition and decreased tolerance to fungal infection. In contrast, under moderate levels of overexpression LbDREB6, poplar trees showed normal growth and no effect on fungal tolerance. These results provide novel insight into the regulation of plant growth by LbDREB6 and its roles in diverse responses to biotic and abiotic stressors. Additionally, our study provides a method to achieve improved drought tolerance by adjusting the levels of overexpression of DREB genes to avoid the occurrence of unfavorable traits, such as decreased growth rate and reduced disease tolerance.
Data Availability Statement
Publicly available datasets were analyzed in this study. This data can be found here: GSE139373 and GSE120118.
Author Contributions
CL designed and conceived the experiments. JY and HW performed the experiments. SZ and XL analyzed the data. XZ and WW revised the manuscript. CL and JY wrote the manuscript. All authors reviewed and approved the manuscript.
Funding
This work was supported by the National Natural Science Foundation of China (31870649), the 111 Project (B16010), the Fundamental Research Funds for the Central Universities (2572016AA01), and Heilongjiang Touyan Innovation Team Program (Tree Genetics and Breeding Innovation Team).
Conflict of Interest
The authors declare that the research was conducted in the absence of any commercial or financial relationships that could be construed as a potential conflict of interest.
Acknowledgments
The authors acknowledge TopEdit (www.topeditsci.com) for the linguistic editing and proofreading during the preparation of this manuscript.
Supplementary Material
The Supplementary Material for this article can be found online at: https://www.frontiersin.org/articles/10.3389/fpls.2020.528550/full#supplementary-material
Supplementary Figure 1 | Generation and transplantation of LbDREB6 transgenic Populus ussuriensis lines. (A) PCR validations on OE lines; M, DNA Marker DL2000; P, pROKII-GFP plasmid used as the positive control, N, gDNA of the wild type (WT) plant was utilized as the PCR templates for the negative control; lanes 4–12, gDNA of LbDREB6 overexpression lines were utilized as the PCR templates for lane 4–7. (B) Transplantation of in vitro plants with well-developed leaf and root systems in pots containing autoclaved sand and soil mixture (1:3 v/v) for 3 months. Left: WT, middle: OE14 line, right: OE18 line. (C) Detection of the LbDREB6 protein in transformed P. ussuriensis by Western blotting.
Supplementary Figure 2 | Exogenous GA 3 effectively reverses the GA-deficiency phenotype. Data are presented as means of three biological replicates, and error bars represent ±SD. Asterisks indicate significant differences, ∗P < 0.05 and ∗∗P < 0.01.
Supplementary Figure 3 | Quantitative real-time PCR analyses of the transcript levels of eight selected DEGs co-up-regulated in high-level (OE18) and moderate-level (OE14) LbDREB6 overexpression lines compared to WT plants under drought stress for 5 days. (A) DREB2C. (B) Peroxidase 6. (C) ATHB-13. (D) MFYA. (E) WRKY40. (F) WRKY46. Data are presented as means of three biological replicates, and error bars represent ±SD. Asterisks indicate significant differences, ∗P < 0.05 and ∗∗P < 0.01.
Supplementary Figure 4 | Quantitative real-time PCR analyses of the transcript levels of eight selected DEGs co-up-regulated in in high-level (OE18) and moderate-level (OE14) LbDREB6 overexpression lines compared to WT plants under drought stress for 5 days. Data are presented as means of three biological replicates, and error bars represent ±SD. Asterisks indicate significant differences, ∗P < 0.05 and ∗∗P < 0.01.
Supplementary Table 1 | Primers used in qRT-PCR analysis for genes responsive to drought.
Supplementary Table 2 | Primers used in qRT-PCR analysis for disease tolerance related genes.
Supplementary Table 3 | Statistical analysis of sequencing.
Supplementary Data 1 | The differentially expressed genes in OE14 compared to the WT plant.
Supplementary Data 2 | The differentially expressed genes in OE18 compared to the WT plant.
Supplementary Data 3 | The differentially expressed genes in OE18 compared to the OE14 plant.
Supplementary Data 4 | Genes related to disease tolerance in OE18 line compared to the WT and OE14 lines.
Footnotes
References
Agarwal, P. K., Gupta, K., Lopato, S., and Agarwal, P. (2017). Dehydration responsive element binding transcription factors and their applications for the engineering of stress tolerance. J. Exp. Bot. 68, 2135–2148. doi: 10.1093/jxb/erx118
Alexandersson, E., Fraysse, L., Sjovall-Larsen, S., Gustavsson, S., Fellert, M., Karlsson, M., et al. (2005). Whole gene family expression and drought stress regulation of aquaporins. Plant Mol. Biol. 59, 469–484. doi: 10.1007/s11103-005-0352-1
Anjum, N. A., Sofo, A., Scopa, A., Roychoudhury, A., and Gill, S. S. (2015). Lipids and proteins-major targets of oxidative modifications in abiotic stressed plants. Environ. Sci. Pollut. R. 22, 4099–4121. doi: 10.1007/s11356-014-3917-1
Arasimowicz-Jelonek, M., and Floryszak-Wieczorek, J. (2014). Nitric oxide: an effective weapon of the plant or the pathogen? Mol. Plant. Pathol. 15, 406–416. doi: 10.1111/mpp.12095
Ban, Q. Y., Liu, G. F., and Wang, Y. C. (2011). A DREB gene from Limonium bicolor mediates molecular and physiological responses to copper stress in transgenic tobacco. J. Plant Physiol. 168, 449–458. doi: 10.1016/j.jplph.2010.08.013
Bhargava, S., and Sawant, K. (2013). Drought stress adaption: metabolic adjustment and regulation of gene expression. Plant Breed. 132, 21–32. doi: 10.1111/pbr.12004
Boller, T., and He, S. Y. (2009). Innate immunity in plants: an arms race between pattern recognition receptors in plants and effectors in microbial pathogens. Science 324, 742–744. doi: 10.1126/science.1171647
Cabello, J. V., Lodeyro, A. F., and Zurbriggen, M. D. (2014). Novel perspectives for the engineering of abiotic stress tolerance in plants. Curr. Opin. Biotech. 26, 62–70. doi: 10.1016/j.copbio.2013.09.011
Charfeddine, M., Bouaziz, D., Charfeddine, S., Hammami, A., Ellouz, O. N., and Bouzid, R. G. (2015). Overexpression of dehydration-responsive element-binding 1 protein (DREB1) in transgenic Solanum tuberosum enhances tolerance to biotic stress. Plant Biotechnol. Rep. 9, 79–88. doi: 10.1007/s11816-015-0345-8
Chen, J. M., Gao, C., Shi, Q., Shan, B., Lei, Y. J., Dong, C. F., et al. (2008). Different expression patterns of CK2 subunits in the brains of experimental animals and patients with transmissible spongiform encephalopathies. Arch. Virol. 153, 1013–1020. doi: 10.1007/s00705-008-0084-z
Du, X., Li, W., Sheng, L., Deng, Y., Wang, Y., Zhang, W., et al. (2018). Over-expression of chrysanthemum CmDREB6 enhanced tolerance of chrysanthemum to heat stress. BMC Plant Biol. 18:178. doi: 10.1186/s12870-018-1400-8
Fox, H., Doron-Faigenboim, A., Ketty, G., Bourstein, R., Attia, Z., Zhou, J., et al. (2017). Transcriptome analysis of Pinus halepensis under drought stress and during recovery. Tree Physiol. 38, 423–441. doi: 10.1093/treephys/tpx137
Götz, S., García-Gomez, J. M., Terol, J., Williams, T. D., Nagaraj, S. H., Nueda, M. J., et al. (2008). High-throughput functional annotation and data mining with the Blast2GO suite. Nucleic Acids Res. 36, 3420–3435. doi: 10.1093/nar/gkn176
Gu, X. B., Gao, Z. H., Yan, Y. C., Wang, X. Y., Qiao, Y. S., and Chen, Y. H. (2017). RdreB1BI enhances drought tolerance by activating AQP-related genes in transgenic strawberry. Plant Physiol. Biochy. 119:33e42. doi: 10.1016/j.plaphy.2017.08.013
He, B., Gu, Y., Tao, X., Cheng, X., Wei, C., Fu, J., et al. (2015). De novo transcriptome sequencing of Oryza officinalis wall ex watt to identify disease-tolerance genes. Int. J. Mol. Sci. 16, 29482–29495. doi: 10.3390/ijms161226178
Hedden, P., and Phillips, A. L. (2000). Gibberellin metabolism: new insights revealed by the genes. Trends Plant Sci. 5, 523–530. doi: 10.1016/s1360-1385(00)01790-8
Hsieh, T. H., Lee, J. T., Yang, P. T., Chiu, L. H., Chamg, Y. Y., Wang, Y. C., et al. (2002). Heterology expression of the Arabidopsis C-repeat/dehydration response element binding factor 1 gene confers elevated tolerance to chiling and oxidative stresses in transgenic tomato. Plant Physiol. 129, 1086–1094. doi: 10.1104/pp.003442
Huang, B., Jin, L., and Liu, J. Y. (2008). Identification and characterization of the novel gene GhDBP2 encoding a DRE-binding peotein from cotton (Gossypium hirsutum). J. Plant Physiol. 165, 214–223. doi: 10.1016/j.jplph.2006.11.003
Huang, J. G., Yang, M., Liu, P., Yang, G. D., Wu, C. A., and Zheng, C. C. (2009). GhDREB1 enhances abiotic stress tolerance, delays GA-mediated development and represses cytokinin signalling in transgenic Arabidopsis. Plant Cell Environ. 32, 1132–1145. doi: 10.1111/j.1365-3040.2009.01995.x
Kang, Y., Han, Y., Torres-Jerez, I., Wang, M., Tang, Y., Monteros, M., et al. (2011). System responses to long-term drought and re-watering of two contrasting alfalfa varieties. Plant J. 68:871e889. doi: 10.1111/j.1365-313X.2011.04738.x
Kidokoro, S., Watanabe, K., Ohori, T., Moriwaki, T., Maruyama, K., Mizoi, J., et al. (2015). Soybean DREB1/CBF-type transcription factors function in heat and drought as well as cold stress-responsive gene expression. Plant J. 81, 505–518. doi: 10.1111/tpj.12746
Kudo, M., Kidokoro, S., Yoshida, T., Mizoi, J., Todaka, D., Fernie, A. R., et al. (2017). Double overexpression of DREB and PIF transcription factors improves drought stress tolerance and cell elongation in transgenic plants. Plant Biotechnol. J. 15, 458–471. doi: 10.1111/pbi.12644
Lata, C., and Prasad, M. (2011). Role of DREBs in regulation of abiotic stress responses in plants. J. Exp. Bot. 62, 4731–4748. doi: 10.1093/jxb/err210
Li, C., Yue, J., Wu, X., Xu, C., and Yu, J. (2014). An ABA-responsive DRE-binding protein gene from Setaria italica, SiARDP, the target gene of SiAREB, plays a critical role under drought stress. J. Exp. Bot. 65, 5415–5427. doi: 10.1093/jxb/eru302
Li, J., Sima, W., Ouyang, B., Wang, T., Ziaf, K., Luo, Z., et al. (2012). Tomato SlDREB gene restricts leaf expansion and internode elongation by downrefulatin key genes for gibberellin biosynthesis. J. Exp. Bot. 63, 6407–6420. doi: 10.1093/jxb/ers295
Li, S. X., Zhao, Q., Zhu, D. Y., and Yu, J. J. (2018). A DREB-like transcription factors from maize (Zea mays), ZmDREB4.1 plays a negative role in plant growth and development. Front. Plant Sci. 9:395. doi: 10.3389/fpls.2018.00395
Liao, X., Guo, X., Wang, Q., Wang, Y., Zhao, D., Yao, L., et al. (2017). Overexpression of MsDREB6.2 results in cytokinin-deficient developmental phenotypes and enhances drought tolerance in transgenic apple plants. Plant J. 89, 510–526. doi: 10.1111/tpj.13401
Lin, R., Park, H., and Wang, H. (2008). Role of Arabidopsis RAP2.4 in regulating light- and ethylene-mediated developmental processes and drought stress tolerance. Mol. Plant 1, 42–57. doi: 10.1093/mp/ssm004
Mauch-Mani, B., and Mauch, F. (2005). The role of abscisic acid in plant–pathogen interactions. Curr. Opin. Plant Biol. 8, 409–414. doi: 10.1016/j.pbi.2005.05.015
Nakashima, K., Yamaguchi-Shinozaki, K., and Shinozaki, K. (2014). The transcriptional regulatory network in the drought response and its crosstalk in abiotic stress responses including drought, cold, and heat. Front. Plant Sci. 5:170. doi: 10.3389/fpls.2014.00170
Ning, Y., Jantasuriyarat, C., Zhao, Q., Zhang, H., Chen, S., Liu, J., et al. (2011). The SINA E3 ligase OsDIS1 negatively regulates drought response in rice. Plant Physiol. 157, 242–255. doi: 10.1104/pp.111.180893
Noctor, G., Mhamdi, A., and Foyer, C. H. (2014). The roles of reactive oxygen metabolism in drought: not so cut and dried. Plant Physiol. 164, 1636–1648. doi: 10.1104/pp.113.233478
Quan, R. D., Hu, S. J., Zhang, Z. L., Zhang, H. W., Zhang, Z. J., and Huang, R. F. (2010). Overexpression of an ERF transcription factor TSRF1 improves rice drought tolerance. Plant Biotechnol. J. 8, 476–488. doi: 10.1111/j.1467-7652.2009.00492.x
Rae, L., Lao, N. T., and Kavanagh, T. A. (2011). Regulation of multiple aquaporin genes in Arabidopsis by a pair of recently duplicated DREB transcription factors. Planta 234, 429–444. doi: 10.1007/s00425-011-1414-z
Richards, D. E., King, K. E., Ait-Ali, T., and Harberd, N. P. (2001). How gibberellin regulates plant growth and development: a molecular genetic analysis of gibberellin signaling. Annu. Rev. Plant Physiol. Plant Mol. Biol. 52, 67–88. doi: 10.1146/annurev.arplant.52.1.67
Royer, C. H., and Noctor, G. (2003). Redox sensing and signaling associated with reactive oxygen in chloroplasts, peroxisomes and mitochondria. Physiol. Planarum 119, 355–364. doi: 10.1034/j.1399-3054.2003.00223.x
Sakuma, Y., Maruyama, K., Osakabe, Y., Qin, F., Seki, M., Shinozaki, K., et al. (2006). Functional analysis of an Arabidopsis transcription factor, DREB2A, involved in drought-responsive gene expression. Plant Cell 18, 1292–1309. doi: 10.1105/tpc.105.035881
Selvaraj, M. G., Ishizaki, T., Valencia, M., Ogawa, S., Dedicova, B., Ogata, T., et al. (2017). Overexpression of an Arabidopsis thaliana galactinol synthase gene improves drought tolerance in transgenic rice and increased grain yield in the field. Plant Biotechnol. J. 15, 1465–1477. doi: 10.1111/pbi.12731
Shan, D. P., Huang, J. G., Yang, Y. T., Guo, Y. H., Wu, C. A., Yang, G. D., et al. (2007). Cotton GhDREB1 increases plant tolerance to low temperature and is negatively regulated by gibberellic acid. New Phytol. 176, 70–81. doi: 10.1111/j.1469-8137.2007.02160.x
Shavrukov, Y., Baho, M., Lopato, S., and Langridge, P. (2016). The TaDREB3 transgene transferred by conventional crossings to different genetic backgrounds of bread wheat improves drought tolerance. Plant Biotechnol. J. 14, 313–322. doi: 10.1111/pbi.12385
Singh, K. B., Foley, R. C., and Oñate-Sánchez, L. (2002). Transcription factors in plant defense and stress responses. Curr. Opin. Plant Biol. 5, 430–436. doi: 10.1016/s1369-5266(02)00289-3
Suo, H., Lü, J., Ma, Q., Yang, C., Zhang, X., Meng, X., et al. (2016). The AtDREB1A transcription factor up-regulates expression of a vernalization pathway gene, GmVRN1-like, delaying flowering in soybean. Acta Physiol. Plant 38:137. doi: 10.1007/s11738-016-2136-4
Takeuchi, J., Okamoto, M., Mega, R., Kanno, Y., Ohnishi, T., Seo, M., et al. (2016). Abscinazole-E3M, a practical inhinitor of abscisic acid 8’-hydroxylase for improvinig drought tolerance. Sci. Rep. 6:37060. doi: 10.1038/srep37060
Tang, M., Liu, X., Deng, H., and Shen, S. (2011). Over-expression of JcDREB, a putative AP2/EREBP domain-containing transcription factor gene in woody biodiesel plant Jatropha curcas, enhances salt and freezing tolerance in transgenic Arabidopsis thaliana. Plant Sci. 181, 623–631. doi: 10.1016/j.plantsci.2011.06.014
Tang, Y. H., Liu, K., Zhang, J., Li, X. L., Xu, K. D., Zhang, Y., et al. (2017). JcDREB2, a physic nut AP2/ERF gene, alters plant growth and salinity stress responses in transgenic rice. Front. Plant Sci. 8:306. doi: 10.3389/fpls.2017.00306
Taulavuori, E., Hellstrom, E. K., Taulavuori, K., and Laine, K. (2001). Comparison of two methods used to analyse lipid peroxidation from Vaccinium myrtillus (L.) during snow removal, reacclimation and cold acclimation. J. Exp. Bot. 52, 2375–2380. doi: 10.1093/jexbot/52.365.2375
Trapnell, C., Pachter, L., and Salzberg, S. L. (2009). TopHat: discovering splice junctions with RNA-Seq. Bioinformatics 25, 1105–1111. doi: 10.1093/bioinformatics/btp120
Trapnell, C., Williams, B. A., Pertea, G., Mortazavi, A., Kwan, G., van Baren, M. J., et al. (2010). Transcript assembly and quantification by RNA-Seq reveals unannotated transcripts and isoform switching during cell differentiation. Nat. Biotechnol. 28, 511–515. doi: 10.1038/nbt.1621
Ullah, C., Unsicker, S. B., Fellenberg, C., Constabel, C. P., Schmidt, A., and Gershenzon, J. A. (2017). Flavan-3-ols are an effective chemical defense against rust infection. Plant Physiol. 175, 1560–1578. doi: 10.1104/pp.17.00842
Umezawa, T., Sugiyama, N., Mizoguchi, M., Hayashi, S., Myouga, F., Yamaguchi-Shinozaki, K., et al. (2009). Type 2C protein phosphatases directly regulate abscisic acid-activated protein kinases in Arabidopsis. Proc. Natl. Acad. Sci. U.S.A. 106, 17588–17593. doi: 10.1073/pnas.0907095106
Vo, K. T. X., Kim, C. Y., Hoang, T. V., Lee, S. K., Shirsekar, G., Seo, Y. S., et al. (2018). OsWRKY67 plays a positive role in basal and XA21-mediated tolerance in rice. Front. Plant Sci. 8:2220. doi: 10.3389/fpls.2017.02220
Wang, C., Liu, S., Dong, Y., Zhao, Y., Geng, A. K., Xia, X. L., et al. (2016). PdEPF1 regulates water-use efficiency and drought tolerance by modulating stomatal density in poplar. Plant Biotechnol. J. 14, 849–860. doi: 10.1111/pbi.12434
Wu, J., Mao, X., Cai, T., Luo, J., and Wei, L. (2006). KOBAS server: a web-based platform for automated annotation and pathway identification. Nucleic Acids Res. 34, 720–724. doi: 10.1093/nar/gkl167
Wuddineh, W. A., Mazarrei, M., Zhang, J. Y., Poovaiah, C. R., Mann, D. G. J., Ziebell, A., et al. (2015). Identification and overexpression of gibberellin 2-oxidase (GA2ox) in switchgrass (Panicum virgatum L.) for improved plant architecture and reduced biomass recalcitrance. Plant Biotechnol. J. 13, 636–647. doi: 10.1111/pbi.12287
Xie, C., Mao, X., Huang, J., Ding, Y., Wu, J., Dong, S., et al. (2011). KOBAS 2.0: a web server for annotation and identification of enriched pathways and diseases. Nucleic Acids Res. 39, 316–322. doi: 10.1093/nar/gkr483
Yang, J., Zhao, S., Zhao, B., and Li, C. (2018). Overexpression of TaLEA3 induces rapid stomatal closure under drought stress in Phellodendron amurense Rupr. Plant Sci. 277, 100–109. doi: 10.1016/j.plantsci.2018.09.022
Yin, L., Wang, S., Eltayeb, A. E., Uddin, M. I., Yamamoto, Y., Tsuji, W., et al. (2010). Overexpression of dehydroascorbate reductase, but not monodehydroascorbate reductase, confers tolerance to aluminum stress in transgenic tobacco. Planta 231, 609–621. doi: 10.1007/s00425-009-1075-3
Zhang, X., Liu, X., Wu, L., Yu, G., Wang, X., and Ma, H. (2015). The SsDREB transcription factor from the succulent halophyte Suaeda salsa enhances abiotic stress tolerance in transgenic tobacco. Int. J. Genomics 2015, 1–13.
Zhang, Y. L., Zhang, C. L., Wang, G. L., Wang, Y. X., Qi, C. H., You, C. X., et al. (2019). Apple AP2/EREBP transcription factor MdSHINE2 confers drought resisitance by regulating wax biosynthesis. Planta 249, 1627–1643. doi: 10.1007/s00425-019-03115-4
Zhao, H., Jiang, J., Li, K. L., and Liu, G. F. (2017). Populus simonii× Populus nigra WRKY70 is involved in salt stress and leaf blight disease responses. Tree Physiol. 37, 1–18. doi: 10.1093/treephys/tpx020
Zhao, K., Shen, X. J., Yuan, H. Z., Liu, Y., Liao, X., Wang, Q., et al. (2013). Isolation and characterization of dehydration-responsive element-binding factor 2C (MsDREB2C) from Malus sieversii Roem. Plant Cell Physiol. 54, 1415–1430. doi: 10.1093/pcp/pct087
Zhou, M., Li, D., Li, Z., Hu, Q., Yang, C. H., Zhu, L. H., et al. (2013). Constitutive expresion of a miR319 gene alters plant developemnt and enhances salt and drought tolerance in transgenic creeping bentgrass. Plant Physiol. 161, 1375–1391. doi: 10.1104/pp.112.208702
Zhou, Q. Y., Tian, A. G., Zou, H. F., Xie, Z. M., Li, G., Huang, J., et al. (2008). Soybean WRKY-type transcription factor genes, GmWRKY13, GmWRKY21, anf GmWRKY54, confer differential tolerance to abiotic stresses in transgenic Arabidopsis plants. Plant Biotechnol. J. 6, 486–503. doi: 10.1111/j.1467-7652.2008.00336.x
Keywords: poplar, drought stress, dehydration responsive element binding transcription factor, dwarf, disease tolerance
Citation: Yang J, Wang H, Zhao S, Liu X, Zhang X, Wu W and Li C (2020) Overexpression Levels of LbDREB6 Differentially Affect Growth, Drought, and Disease Tolerance in Poplar. Front. Plant Sci. 11:528550. doi: 10.3389/fpls.2020.528550
Received: 21 January 2020; Accepted: 06 October 2020;
Published: 11 November 2020.
Edited by:
Sanushka Naidoo, University of Pretoria, South AfricaCopyright © 2020 Yang, Wang, Zhao, Liu, Zhang, Wu and Li. This is an open-access article distributed under the terms of the Creative Commons Attribution License (CC BY). The use, distribution or reproduction in other forums is permitted, provided the original author(s) and the copyright owner(s) are credited and that the original publication in this journal is cited, in accordance with accepted academic practice. No use, distribution or reproduction is permitted which does not comply with these terms.
*Correspondence: Chenghao Li, Y2hsaUBuZWZ1LmVkdS5jbg==; Y2hsaTBAMTYzLmNvbQ==
†These authors have contributed equally to this work