- 1Department of Biochemistry, Molecular Biology, Entomology and Plant Pathology, Mississippi State University, Starkville, MS, United States
- 2Department of Biological Sciences, Mississippi State University, Starkville, MS, United States
- 3Department of Entomology and Plant Pathology, Auburn University, Auburn, AL, United States
- 4Center for Computational Sciences High Performance Computing Collaboratory, Mississippi State University, Starkville, MS, United States
The conserved oligomeric Golgi (COG) complex, functioning in retrograde trafficking, is a universal structure present among eukaryotes that maintains the correct Golgi structure and function. The COG complex is composed of eight subunits coalescing into two sub-complexes. COGs1–4 compose Sub-complex A. COGs5–8 compose Sub-complex B. The observation that COG interacts with the syntaxins, suppressors of the erd2-deletion 5 (Sed5p), is noteworthy because Sed5p also interacts with Sec17p [alpha soluble NSF attachment protein (α-SNAP)]. The α-SNAP gene is located within the major Heterodera glycines [soybean cyst nematode (SCN)] resistance locus (rhg1) and functions in resistance. The study presented here provides a functional analysis of the Glycine max COG complex. The analysis has identified two paralogs of each COG gene. Functional transgenic studies demonstrate at least one paralog of each COG gene family functions in G. max during H. glycines resistance. Furthermore, treatment of G. max with the bacterial effector harpin, known to function in effector triggered immunity (ETI), leads to the induced transcription of at least one member of each COG gene family that has a role in H. glycines resistance. In some instances, altered COG gene expression changes the relative transcript abundance of syntaxin 31. These results indicate that the G. max COG complex functions through processes involving ETI leading to H. glycines resistance.
Introduction
Heterodera glycines [soybean cyst nematode (SCN)] is the most economically important pathogen of Glycine max (soybean). The infection of G. max by H. glycines accounts for a 7–10% decrease in yield and causes more economic loss than the rest of its pathogens combined losses (Wrather et al., 2001; Wrather and Koenning, 2006). G. max may show clear signs of H. glycines parasitism, including chlorosis and stunting. However, in some cases, no adverse signs of parasitism may be observed, except an approximately 15% decrease in yield (Wang et al., 2003). The life cycle of H. glycines is 30 or more days, dependent on ambient temperatures (Lauritis et al., 1983). During its obligate parasitic life cycle, H. glycines females become a hardened cyst, which is its carcass that contains 250–500 fertilized eggs. While within the cyst in the soil, the eggs may remain dormant for up to 9 years. Once the proper conditions occur, H. glycines eggs hatch as second-stage juveniles (J2s). Then, the J2s migrate toward and then burrow into the root. As they burrow, the J2s slice through root cells including epidermal, cortex, and endodermal cells with a mouth apparatus known as a stylet that is both rigid and tubular. It takes approximately 24 h for the J2 to reach its site of parasitism (Endo, 1965; Endo, 1991). Another function of the H. glycines stylet is to deliver effectors into a G. max pericycle or neighboring cell that it will parasitize. During this process, occurring over a period of days, the cell walls of the H. glycines-parasitized root cells dissolve. The cell walls dissolve through enzymatically driven processes facilitated by the nematode. As a result, 200–250 neighboring root cells are incorporated into a common cytoplasm producing a multinucleate syncytium. Notably, the syncytium is also where the localized defense response occurs, a process involving components of effector triggered immunity (ETI) and pathogen-activated molecular pattern (PAMP)-triggered immunity (PTI) (Ross, 1958; Endo, 1965, 1991; Jones and Dangl, 2006; Matsye et al., 2011, 2012; Pant et al., 2014; McNeece et al., 2017, 2019) (Figure 1).
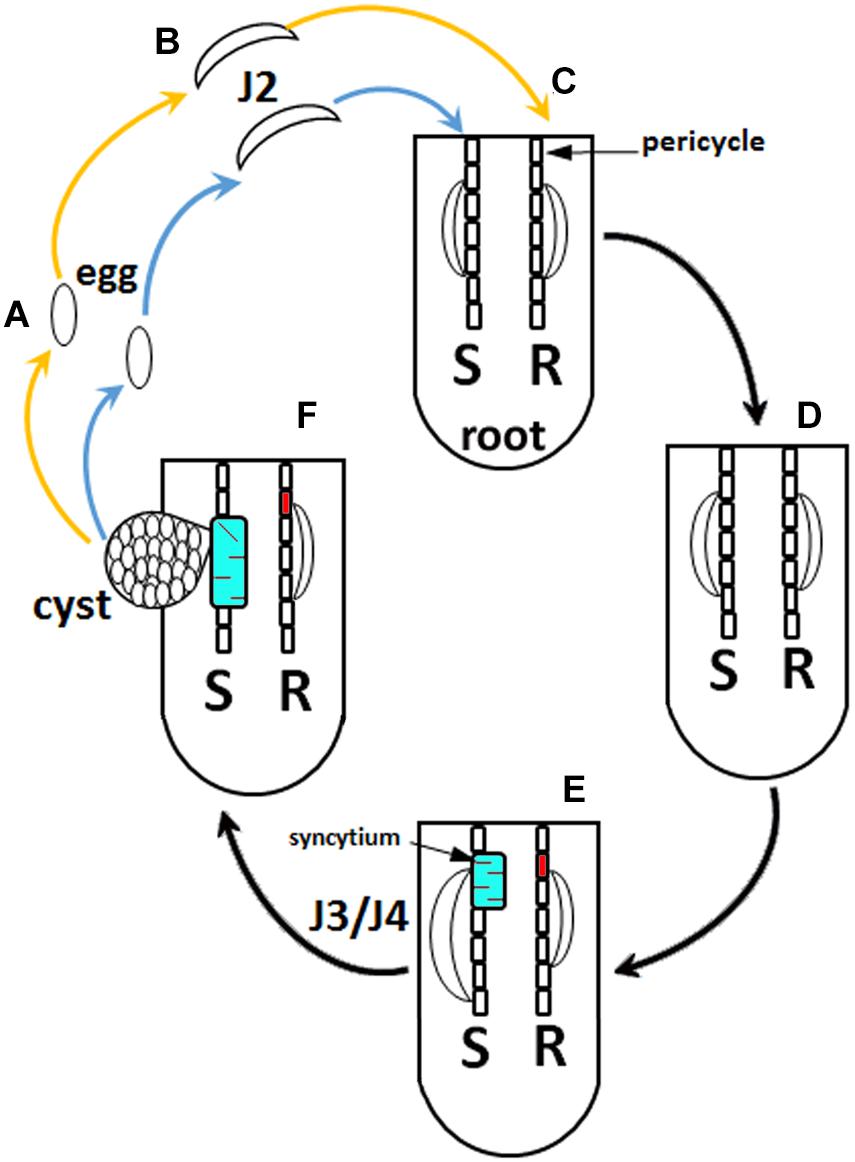
Figure 1. Heterodera glycines life cycle. (A) Eggs. (B) Second-stage juveniles (J2). (C) Root infected with J2 at 1 day post infection (dpi). (D) 3 dpi. (E) 6 dpi. (F) 30 dpi. The blue arrow indicates the nematode that will experience a compatible reaction, leading to plant susceptibility (S). The orange arrow indicates a nematode that will experience an incompatible reaction, leading to resistance (R). H. glycines development halts at the J2 stage during a resistant reaction in G. max[Peking/PI 548402]. Syncytia have been distinguished where, at 3 dpi, they are undergoing susceptible or resistant reactions that appear similar cytologically, showing features that include hypertrophy, an enlargement of nuclei, the development of dense cytoplasm, and an increase in endoplasmic reticulum (ER) and ribosome content. Due to these similarities, the 6 dpi time point is indicated, distinguishing a susceptible (red) and resistant (cyan) reaction because the syncytia undergoing a susceptible reaction are characterized by hypertrophy of nuclei and nucleoli, proliferation of cytoplasmic organelles, a reduction and dissolution of the vacuole, and cell expansion by incorporating adjacent cells. The resistant reaction, in contrast, shows cytoplasmic characteristics that are genotype-specific. The 6 dpi G. max[Peking/PI 548402] reaction has cell wall appositions, structures that aggregate cytoplasmic components through actin polarization and vesicle-mediated delivery of cargo, the production of a necrotic layer of cells that surrounds the syncytium, and the accumulation of ER. The G. max[Peking/PI 548402] resistant reaction leads to H. glycines development being blocked at the J2 stage. The G. max[PI 88788] resistant reaction lacks cell wall appositions and lacks a necrotic layer of cells that surrounds the syncytium during the resistant reaction while having an accumulation of ER. The G. max[PI 88788] resistant reaction leads to H. glycines development being blocked at J3–J4 stage.
Experiments performed in G. max show that part of its major resistance locus to the parasitic nematode H. glycines, rhg1, contains alpha soluble NSF attachment protein (α-SNAP) in tandemly copied arrays also including an amino acid transporter and a wound inducible protein (Caldwell et al., 1960; Matsye et al., 2011; Cook et al., 2012). Subsequent experiments have shown α-SNAP has a role in resistance (Matsye et al., 2012; Cook et al., 2012; Sharma et al., 2016). However, the genetic diversity of H. glycines has both complicated and facilitated the analysis of the rhg1 locus and resistance in general (Golden et al., 1970; Riggs and Schmitt, 1988). These observations are consistent with experiments showing membrane fusion apparatus proteins participating in vesicle transport having a role in the plant defense to pathogens (Collins et al., 2003). In those experiments, the SNARE protein syntaxin 121 (SYP121) functions in impairing fungal haustorial penetration, and thus, the mutant locus was first called penetration1 (pen1) (Collins et al., 2003). Since those initial observations, numerous other membrane fusion proteins found at various subcellular membrane-enveloped structures have been shown to function in defense processes (Lipka et al., 2005). More recent experiments performed in Hordeum vulgare (wheat) identified its COG3 (HvCOG3) functions during its defense response to cellular penetration by the fungal pathogen Blumeria graminis f.sp. hordei. (Ostertag et al., 2013). A number of G. max homologs of membrane fusion proteins, including 20S particle components and myosin XI, have been shown to function during the resistant reaction it has to parasitic nematodes (Matsye et al., 2012; Sharma et al., 2016; Klink et al., 2017; Austin et al., 2019). However, the COG complex has not yet been studied.
The α-SNAP gene was originally identified in Saccharomyces cerevisiae as Sec17 in a genetic screen designed to find proteins that function in secretion (Novick et al., 1980). The α-SNAP gene is part of a larger complex known as the 20S particle that functions in the fusion of vesicles to target membranes, leading to the secretion of cargo (Söllner et al., 1993a,b). Experiments performed in S. cerevisiae show Sec17p binds to the suppressors of the erd2-deletion 5 (Sed5p), known in plants as syntaxin 31 (Hardwick and Pelham, 1992; Lupashin et al., 1997; Bubeck et al., 2008). Both α-SNAP and syntaxin 31 have roles in the defense process G. max has to H. glycines (Matsye et al., 2012; Cook et al., 2012; Pant et al., 2014; Sharma et al., 2016). Sed5p is involved in retrograde trafficking through its interaction with the conserved oligomeric Golgi (COG) complex (Shestakova et al., 2007). Therefore, an indirect link exists between COG and α-SNAP through Sed5p and implicates the COG complex as having an important role in the defense process that G. max has toward H. glycines parasitism.
The COG complex is universal among eukaryotes, maintaining the correct structure and function of the Golgi apparatus (Ungar et al., 2002; Vukašinović and Žárský, 2016). An important facet of the COG complex is its role in retrograde trafficking occurring between the Golgi cisternae (Ungar et al., 2002). Furthermore, as part of its function, the COG complex plays an important role in the homeostasis of enzyme glycosylation (Ungar et al., 2002).
The COG complex is composed of eight subunits (Whyte and Munro, 2001; Ungar et al., 2002; Willett et al., 2013). The eight COG complex subunits coalesce into two sub-complexes (Fotso et al., 2005; Ungar et al., 2005). Sub-complex A is composed of COGs1–4, while sub-complex B is composed of COGs5–8 (Ungar et al., 2002). Components of the COG complex interact with the soluble N-ethylmaleimide-sensitive factor attachment protein receptor (SNARE), which is part of the 20S particle, to effect membrane fusion (Söllner et al., 1993a,b; Cottam and Ungar, 2012; Jahn and Fasshauer, 2012; Willett et al., 2013). The COG complex also functions along with other SNARE interacting proteins, Rabs, tethers containing coiled-coil proteins and various molecular motors to perform its functions (Cottam and Ungar, 2012; Willett et al., 2013). Consequently, the COG complex performs a central role in the retrograde movement of materials between Golgi cisternae.
Much understanding of the COG complex function has come from mutant analyses in S. cerevisiae. For example, S. cerevisiae mutants of COGs1–3 (i.e., sec36, sec35, and sec34, respectively) exhibit very slow growth, while COG4 (cod1/sec38) mutants are inviable (Whyte and Munro, 2001; Ram et al., 2002). In contrast, mutants of COGs5–8 (cod4, sec37, cod6, and dor1, respectively) are viable. The growth deficiencies are caused by various impairments of Golgi function involving retrograde trafficking.
In plants, the Arabidopsis thaliana embryo yellow (eye) mutant phenotype, which has impaired cell expansion and meristem organization, results from a mutation in the AtCOG7 gene AT5G51430 (Ishikawa et al., 2008). A surprising observation in A. thaliana is that AtCOG2 (AT4G24840) performs a role in recruiting the exocyst complex to xylem cell sites of secondary cell wall deposition (Oda et al., 2015). This process appears to involve the targeting of the exocyst to microtubules through the direct interaction of exocyst subunits with the COG2 protein (Vukašinović et al., 2017). COG3 (AT1G73430) and COG8 (AT5G11980) perform a number of functions including modulating the morphology of the Golgi apparatus and homeostasis during vesicle trafficking and also being essential for pollen tube growth (Tan et al., 2016). COG6 (AT1G31780) is essential for the maintenance of the Golgi structure and pollen tube growth (Rui et al., 2020). No clear role has been shown for A. thaliana COG1 (AT5G16300), COG4 (AT4G01400), or COG5 (AT1G67930). For a detailed treatise on the plant COG complex and the plant vesicle transport system in general, the reviewer is directed to Vukašinović and Žárský (2016). Consequently, in contrast to what is understood in S. cerevisiae, very little is known about the COG complex in plants and even less regarding its role during pathogenic interactions. Therefore, the interaction between G. max and H. glycines presents an opportunity to better understand plant defense responses, especially since α-SNAP and a COG-interacting protein, syntaxin, function in the resistant reaction (Cook et al., 2012; Matsye et al., 2012; Pant et al., 2014).
The study presented here is devoted to the characterization of the entire COG complex gene family of G. max as it relates to determining a potential role during its resistant reaction to the parasitic nematode H. glycines. The experiments presented here began by determining whether the COG complex gene family members are present in the genome of G. max. The 16 G. max COG complex genes have been cloned and genetically engineered for their overexpression in the H. glycines-susceptible genotype G. max[Williams 82/PI 518671] to see if that susceptible genotype became resistant to parasitism. In contrast, the entire COG complex gene family has been cloned and genetically engineered for its suppressed expression by RNA interference (RNAi) in the H. glycines-resistant genotype G. max[Peking/PI 548402] to see if that resistant genotype became susceptible to parasitism. COG gene expression assays using reverse transcriptase quantitative PCR (RT-qPCR) demonstrate the appropriate increased or decreased transcript abundances of the COG complex genes as a consequence of their transgenically driven overexpression or RNAi, respectively. The experiments demonstrate the involvement of specific COG complex gene family members functioning during the engineered resistance reaction, while their suppressed transcription by RNAi leads to engineered susceptibility to H. glycines parasitism. Furthermore, treatment of G. max with the bacterial elicitor harpin leads to induced expression of COG genes that function in resistance to H. glycines. This result indicates that ETI plays a role in activating the COG gene expression that functions in G. max resistance to H. glycines. Lastly, in some cases, COG gene expression appears to regulate the expression of syntaxin 31.
Materials and Methods
Experiment Details
Three independent biological replicates have been used for each experiment with each independent biological replicate having 10–25 experimental replicate plants. For RT-qPCR studies, three independent biological replicate lines were compared. For the female index (FI) studies using transgenic COG-overexpressing (OE), COG-RNAi, and their respective pRAP15-ccdB and pRAP17-ccdB control lines, the analysis used three independent biological replicates, each independent biological replicate having 10–25 experimental replicate plants. For the harpin effector RT-qPCR analyses, three independent biological replicates have been used, employing the same RNA that had been used in Aljaafri et al. (2017). A total of 10 plants were studied in each biological replicate and control in the experiments of Aljaafri et al. (2017). For the syntaxin 31 RT-qPCR analyses, three independent biological replicates have been used. Consequently, three independent biological replicates have been used in these analyses. Furthermore, a minimum of three independent biological replicates have been used in order to enable an assessment of significance.
Conserved Oligomeric Golgi Gene Identification
The G. max genome sequence, assembly, and annotation are housed at Phytozome1 (Goodstein et al., 2012). The G. max proteome has been queried with the conceptually translated A. thaliana COG gene sequences using the Basic Local Alignment Search Tool (BLAST) (Altschul et al., 1990). The comparative analyses have been performed in Phytozome using the default settings, including Target type: Proteome; Program: BLASTP-protein query to protein database; Expect (E) threshold: −1; Comparison matrix: BLOSUM62; Word (W) length: default = 3; number of alignments to show: 100 allowing for gaps and filter query.
Gene Cloning and Generation of Transgenic G. max
The more recent Glycine max Wm82.a2.v1 annotation has been used in the design of PCR primer sequences (Supplementary Table 1) (Lawaju et al., 2018). COG gene amplicons are generated by PCR using the Accuprime Taq Polymerase System (Invitrogen) according to the manufacturer’s instructions. The PCR reaction contents are run on a 1% agarose gel with the COG gene amplicons purified using the Wizard SV Gel and PCR Clean-Up System (Promega) according to the manufacturer’s instructions. Cloning of the COG amplicons is accomplished through ligation into the pENTR/D-TOPO entry vector using the pENTR/D-TOPO Cloning Kit (Invitrogen) according to the manufacturer’s instructions. Subsequently, the ligation reaction contents undergo transformation into One Shot TOP10 Chemically Competent Escherichia coli (TOP 10) (Invitrogen) cells according to the manufacturer’s instructions. Chemical selection occurs on Luria–Bertani (LB) agar plates that contain 50 μg/ml kanamycin. After 14 h, colonies are picked and grown in 3 ml of liquid LB containing 50 μg/ml kanamycin. After 14 h, the colonies undergo plasmid isolation using the Wizard Plus SV Minipreps DNA Purification System (Promega) according to the manufacturer’s instructions. The confirmed COG gene amplicons are ligated into the Gateway-compatible overexpression (pRAP15) or RNAi (pRAP17) destination vectors using the Gateway LR Clonase Enzyme mix (Invitrogen) according to their instructions (Curtis and Grossniklaus, 2003; Klink et al., 2009; Matsye et al., 2012). The promoter driving the expression of the overexpression and RNAi cassettes is the figwort mosaic virus (FMV) sub-genomic transcript (Sgt) promoter (Bhattacharyya et al., 2002). The FMV-Sgt promoter consists of a 301-bp FMV Sgt promoter fragment [sequence −270 to +31 from the transcription start site (TSS)] (Bhattacharyya et al., 2002). The promoter has been used in the design of the pRAP15 and pRAP17 vectors (Klink et al., 2009; Matsye et al., 2012). The RNAi cassette of pRAP17 is designed to produce a hairpin RNA having inverted repeats (Curtis and Grossniklaus, 2003; Klink et al., 2009). The experimental controls are the un-engineered pRAP15 or pRAP17 vectors. However, these vectors have the ccdB gene that is positioned where, otherwise, the COG gene amplicon is directionally inserted during the LR clonase reaction. Consequently, this feature makes the un-engineered vectors [pRAP15-ccdB (overexpression control) and pRAP17-ccdB (RNAi control)] suitable as controls for any non-specific effects from gene overexpression or RNAi (McNeece et al., 2019). The LR reaction contents undergo transformation into chemically competent E. coli TOP 10 cells, having been performed according to the manufacturer’s instructions. Chemical selection occurs on LB agar plates that contain 5 μg/ml tetracycline. COG gene-specific primers are used in PCR reactions to confirm the presence of the targeted gene (Supplementary Table 1). The COG gene-containing pRAP15/17 destination vectors undergo transformation into chemically competent Agrobacterium rhizogenes K599 (K599) using the freeze–thaw transformation procedure (Hofgen and Willmitzer, 1988; Haas et al., 1995; Collier et al., 2005; Lawaju et al., 2018).
Production of Transgenic Plants for Functional Experiments
Transgenic plants have been generated according to Lawaju et al. (2018). The 250-ml culture of K599 that is transformed with the COG-containing plasmid is pelleted through centrifugation in a Sorvall RC6 Plus Superspeed Centrifuge at 4oC for 20 min. The resulting K599 pellet is resuspended in Murashige and Skoog medium including vitamins (MS) (Duchefa Biochemie) containing 3.0% sucrose, pH 5.7 (MS media). The COG genes have been engineered to undergo overexpression in the H. glycines-susceptible genotype G. max[Williams 82/PI 518671]. In contrast, the COG genes have been engineered to undergo RNAi in the H. glycines-resistant genotype G. max[Peking/PI 548402]. Transgenic G. max plants are made by cutting off the root of a 1-week-old plant at the hypocotyl using a new, sterile razor blade. The plants are immersed in the K599 solution in a Petri dish prior to cutting. This step provides the transformed K599 immediate access into the wound site made by the removal of the root. Then, 25 rootless plants are placed in a 140-ml glass beaker containing 25 ml of transformed K599 in MS media solution. Infiltration of the plant tissue, having the transformed K599, occurs under a vacuum for 5 min. The pump is turned off after 5 min, and the plants are left in the vacuum for an additional 10 min. The vacuum is then slowly released, allowing the transformed K599 further entry into the plant tissue. After the vacuum is completely released, the cut ends of G. max are placed individually into fresh coarse Vermiculite Grade A-3 (Palmetto Vermiculite) in 50-cell 725602C Propagation Trays held in 710245C Standard Flats with holes in the bottom (T.O. Plastics) at a depth of 3–4 cm deep. The plant trays are then placed in a Sterilite, 25 Qt/23 L Modular Latch Box (Sterilite). The container is then covered with its lid. The covered containers are placed under lights at a distance of 20 cm from standard fluorescent cool white 4,100 K, 32-watt bulbs. The bulbs, emitting 2,800 lumens (Sylvania), are used to illuminate the plants for 5 days at ambient lab temperatures (22°C) on a 16/8 light/dark cycle. The plants are subsequently transferred to the greenhouse. The plants are removed from the container, allowing their recovery for 1 week. Visual selection of transgenic G. max roots occurs with the enhanced green fluorescent protein (eGFP) visual reporter (Haseloff et al., 1997). Visualization occurs using the Dark Reader Spot Lamp (SL10S) (Clare Chemical Research). Roots exhibiting the eGFP reporter expression will also possess the COG gene expression cassette. The eGFP and COG genes each have their own promoter and terminator sequences. COG gene transfer occurs because K599 shuttles the DNA cassette located between the left and right borders of the pRAP15 and pRAP17 destination vectors into the root cell chromosomal DNA. As a consequence, the gene is stably integrated into the root somatic cell chromosome, even though the DNA cassette is not incorporated into the germline (Tepfer, 1984; Haas et al., 1995; Collier et al., 2005). The resultant root develops from the base of a non-transgenic shoot stock, leading to the production of a genetically mosaic plant. Therefore, each individual transgenic root system is an independent transformant line. Culture of the transgenic plants occurs in Ray Leach “Cone-tainer” (SC10) pots (Stuewe and Sons, Inc.). These pots are secured in a Ray Leach Tray (RL98) (Stuewe and Sons, Inc.). The soil is a sandy (93.00% sand, 5.75% silt, and 1.25% clay) mixture. The plants are then allowed to recover for 2 weeks prior to the start of the experiments. The effect that the genetic constructs have on G. max COG gene expression is confirmed by RT-qPCR. (Please refer to RT-qPCR-related section.)
PCR and Reverse Transcriptase Quantitative PCR of Conserved Oligomeric Golgi Complex Gene Family Members
The determination of the extent of COG gene expression in transgenic G. max is accomplished by RT-qPCR according to Lawaju et al. (2018). The analysis procedure uses Taqman 6-carboxyfluorescein (6-FAM)-labeled probes and Black Hole Quencher (BHQ1) (MWG Operon) (Supplementary Table 1) according to the manufacturer’s instructions. The control used in the RT-qPCR experiments is designed from a ribosomal S21 (RPS21) protein coding gene (Glyma.15G147700) (Supplementary Table 1). The relative change in gene expression that is caused by the genetic engineering event is calculated using 2–ΔΔCT (Livak and Schmittgen, 2001). The p-values have been calculated using a Student’s t-test for the replicated RT-qPCR reactions (Yuan et al., 2006). Experiments and statistical analyses have been performed from three independent biological replicates.
Assaying the Effect Altered Conserved Oligomeric Golgi Gene Expression Has on Nematode Parasitism
Heterodera glycines infections of transgenic plants are performed according to the procedures described in Lawaju et al. (2018). H. glycines[NL1–Rhg/HG–type 7/race 3] eggs are obtained from cysts, collected from 60-day-old greenhouse-grown G. max stock plants grown in 500-cm3 polystyrene pots. Extraction of the H. glycines cysts from the stock G. max plants occurs by sucrose flotation. The roots containing the H. glycines cysts are then washed. Washing occurs through nested 850-μm-pore and 250-μm-pore sieves. Collection of the H. glycines cysts occurs from the 250-μm-pore sieve. A mortar and pestle are used to grind the H. glycines cysts to release eggs. Gravitational sieving and subsequent sucrose centrifugation are used to obtain the H. glycines eggs. Nested 75-μm-pore over 25-μm-pore sieves are used to recover the H. glycines eggs. Subsequently, H. glycines J2s are collected from hatched eggs. This occurs using a modified Baermann funnel that is placed on a Slide Warmer (Model 77) (Marshall Scientific) at 28°C. Hatching H. glycines egg occurs after 4–7 days. Subsequently, H. glycines J2s are collected. Collection occurs on a 25-μm-pore sieve, placed in 1.5-ml tubes. Centrifugation of the tube and its contents occurs at 10,000 rpm for 1 min. This step is followed by washing the contents with sterile distilled water and subsequent centrifugation again. Concentrating the J2s occurs by centrifugation in an IEC clinical centrifuge. This step is done for 30 s at 1,720 rpm to obtain a final optimized concentration of 2,000 pi-J2/ml. Subsequently, each plant having the transgenic root is inoculated with 1 ml of H. glycines. The H. glycines concentration is 2,000 J2s/ml per root system (per plant). Infection of the transgenic root systems by H. glycines is allowed to proceed for 30 days. At the experiment’s conclusion, the cysts are collected over nested 20- and 100-mesh sieves. To ensure collection of all cysts for enumeration of the female index (FI), the soil is washed several times and the rinse water sieved.
The community-accepted standard representation of the obtained data is the FI; FI = (Nx/Ns) × 100 (Golden et al., 1970). In the experiments presented here, Nx is the pRAP-COG gene-transformed (experimental) line. Ns is the pRAP-ccdB (control) line. For the analysis purposes presented here, the FI is calculated as cysts per whole root system (wr) grown within 100 cc of soil and also cysts per gram (pg) of root system. Each analysis has its own purpose. From a historical perspective, the wr system analysis determines the FI regardless of any differences in G. max or H. glycines genotype regarding root performance prior to or during infection. In contrast, the cyst pg of root system analysis accounts for possible altered root growth that is being caused by the influence of the overexpression or RNAi of the candidate G. max COG gene and/or nematode. For every experiment, three biological replicates are made for each construct. Furthermore, each biological replicate has 10–25 individual transgenic plants each that serve as experimental replicates. The pRAP15-ccdB overexpression control had 943.09 ± 275.47 cysts per root system in 100 cc of soil (p < 0.001). The pRAP17-ccdB RNAi had 7.77 ± 2.29 cysts per root system in 100 cc of soil (p < 0.001). Statistical analyses have employed the Mann–Whitney–Wilcoxon (MWW) rank-sum test, which is a non-parametric test of the null hypothesis not requiring the assumption of normal distributions (p < 0.05 cutoff) (Mann and Whitney, 1947). Root mass has been determined from fresh weight and analyzed using MWW (p < 0.05 cutoff). Experiments and statistical analyses have been performed from three independent biological replicates, with each biological replicate having 15–25 individual transgenic plants.
Results
Identification of G. max Conserved Oligomeric Golgi Complex Genes and Expression
The purpose of the analysis presented here is to understand whether, in G. max, its COG complex functions during the resistant reaction to H. glycines parasitism. Protein sequences of the eight COG complex subunits have been identified in A. thaliana (Table 1). The A. thaliana protein sequences have been used to query the proteome of G. max using protein BLAST analyses. The outcome of those analyses is the identification of the G. max COG complex homologs (Table 1).
Functional Analysis of the Conserved Oligomeric Golgi Complex Gene Family
The overexpression of the 16 COG genes has been accomplished in the H. glycines-susceptible G. max[Williams 82/PI 518671], along with the appropriate pRAP15-ccdB control. In contrast, RNAi of the 16 COG genes has been accomplished in the H. glycines-resistant G. max[Peking/PI 548402], along with the appropriate pRAP17-ccdB control. RNA has been isolated from the transgenic lines and converted to cDNA for its use in RT-qPCR experiments. As expected, the overexpression of the COG genes leads to an increase in their relative transcript abundance as compared to their pRAP15-ccdB root tissue control when using the RPS21 gene as a gene expression control (p < 0.05, Student’s t-test) (Figure 2). In contrast, RNAi of the COG genes leads to a decrease in their relative transcript abundance as compared to their pRAP17-ccdB root tissue control when using the RPS21 gene as a gene expression control (p < 0.05, Student’s t-test) (Figure 2). Consequently, the roots are functioning as expected regarding the relative transcript abundance of the COG genes. Therefore, the roots could be used for functional experiments to determine the effect the gene cassette has on H. glycines parasitism.
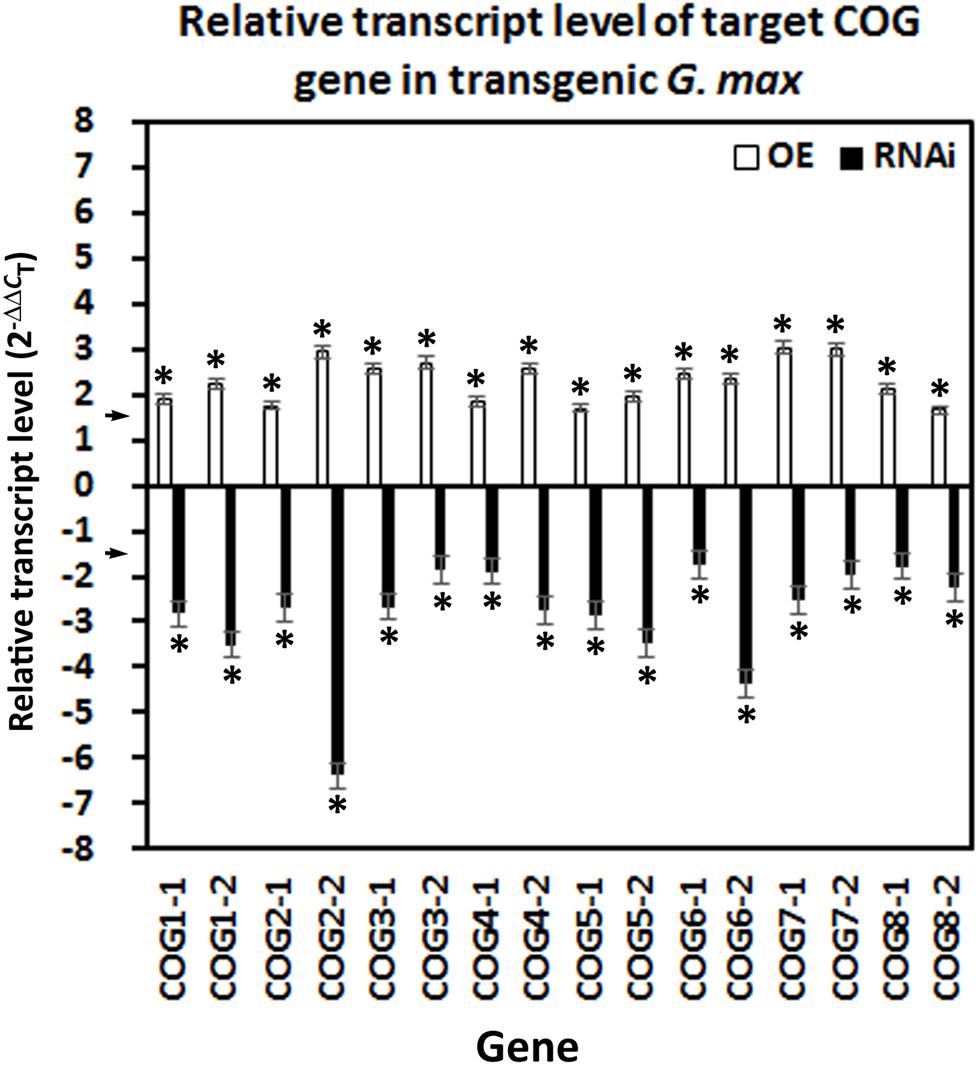
Figure 2. Confirmation of conserved oligomeric Golgi (COG) overexpressing (OE) and RNAi effect by RT-qPCR. In each transgenic line, gene expression has been altered accordingly with the overexpression lines increasing transcript abundance by ≥1.5-fold using the (2–ΔΔCT) method (Livak and Schmittgen, 2001) (p < 0.05, Student’s t-test) and the RNAi lines by ≤1.5-fold (p < 0.05, Student’s t-test). The arrows on the y-axis indicate the level where the experimental outcome is statistically significant [p < 0.05, Mann–Whitney–Wilcoxon (MWW)]. Experiments and statistical analyses have been performed from three independent biological replicates. Statistically significant p < 0.05.
Functional Experiments Examine the Conserved Oligomeric Golgi Gene Role During Resistance to H. glycines Parasitism
The number of experimental replicates (10–25 plants) within each biological replicate (n = 3) is provided (Supplementary Table 2). The soil containing the potted transgenic plants has been infested with 2,000 H. glycines J2s. Infection of the roots has been permitted to proceed for 30 days with subsequent extraction of cysts. Enumeration of cysts from the pRAP15-ccdB control and COG-overexpressing experimental lines has been performed. The analysis has resulted in the calculation of the FI from the overexpression lines for both cysts per wr system and cysts pg of wr system (Figure 3). The results of the analysis show that the overexpression of 14 of the 16 COG genes suppresses H. glycines parasitism by 50% or greater in the H. glycines-susceptible G. max[Williams 82/PI 518671] as compared to the pRAP15-ccdB control (p < 0.05, MWW). The only COG overexpression lines that did not have a statistically significant effect on H. glycines parasitism as compared to the pRAP15-ccdB control are COG6-2-OE and COG7-1-OE (p ≥ 0.05, MWW). These experiments are complimented by RNAi of each COG gene in the G. max[Peking/PI 548402] genotype, which is normally H. glycines-resistant. The enumeration of cysts from pRAP17-ccdB control and COG-RNAi experimental lines has been made in G. max[Peking/PI 548402], resulting in the calculation of the FI for both cysts per wr system and cysts pg of wr system (Figure 3). The results of the analysis show that the RNAi of nine COG genes, including COG1-2, COG2-2, COG3-1, COG4-2, COG5-1, COG6-1, COG7-1, COG7-2, and COG8-1, increases H. glycines parasitism by 1.5-fold (FI ≥ 150) or greater (p < 0.05, MWW) (Figure 3). In contrast, RNAi of COG1-1, COG2-1, COG3-2, COG4-1, COG5-2, COG6-2, and COG8-2 does not increase H. glycines parasitism in the H. glycines-resistant G. max[Peking/PI 548402] by our parameters (p ≥ 0.05, MWW). To satisfy our criteria of a COG gene functioning in resistance, a statistically significant decrease in H. glycines parasitism as determined by the FI result must be obtained in each overexpression replicate in both the cyst per wr system and cyst pg of wr system analyses and in each RNAi replicate in both the cyst per wr system and cyst pg of wr system analyses (p < 0.05, MWW). The results presented here show that those criteria have been met only for eight of the COG genes including COG1-2, COG2-2, COG3-1, COG4-2, COG5-1, COG6-1, COG7-2, and COG8-1 (Figure 3). Consequently, at least one paralog of each COG gene family satisfies our criteria as functioning in resistance to H. glycines parasitism.
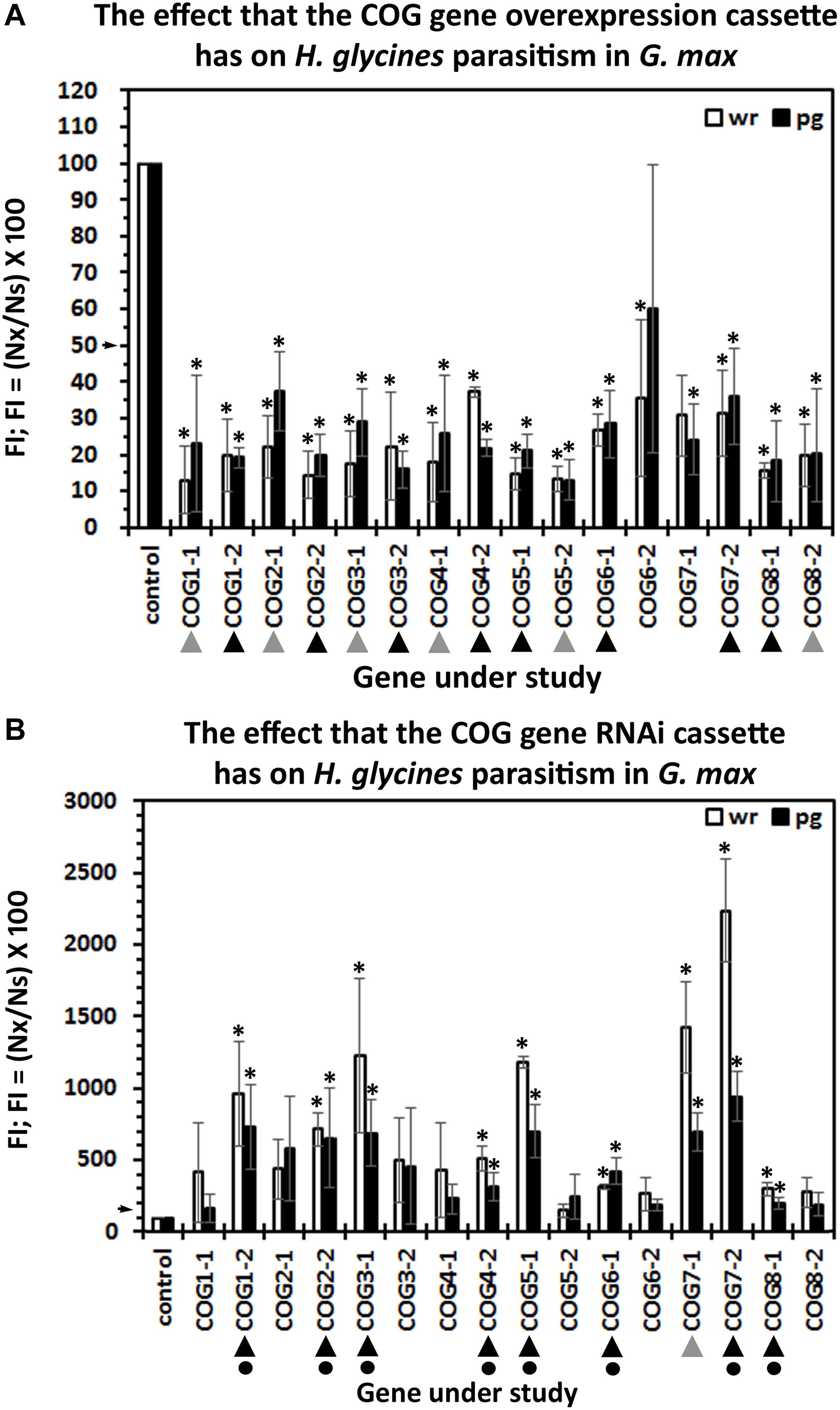
Figure 3. The female index (FI) of transgenic G. max. (A) Overexpression: roots engineered to increase the relative transcript abundance of the target conserved oligomeric Golgi (COG) gene through overexpression. (B) RNAi: The FI of transgenic G. max engineered to decrease the relative transcript abundance of the target COG gene; wr, whole root analysis; per gram, per gram analysis. Statistically significant (p < 0.05, Student’s t-test). The pRAP15-ccdB overexpression control had 943.09 ± 275.47 cysts per root system in 100 cc of soil (p < 0.001). The pRAP17-ccdB RNAi had 7.77 ± 2.29 cysts per root system in 100 cc of soil (p < 0.001). () Decreases H. glycines parasitism in the overexpressing (OE) lines or increases parasitism in the RNAi lines, but not both. Decreases H. glycines parasitism in the OE lines and increases parasitism in the RNAi lines (▲), and therefore, the gene is considered functioning in defense (
) because it meets the criteria of suppressing parasitism by 50% in the overexpression lines and increasing parasitism in the RNAi lines by 1.5-fold (p < 0.05, MWW). The arrows on the y-axis indicate the level where the experimental outcome is statistically significant (p < 0.05, MWW). Experiments and statistical analyses have been performed from three independent biological replicates each having 10–25 independent transgenic root systems. Statistically significant p < 0.05.
Differences occurring between the wr and pg analyses can be accounted for by root growth (Figure 4). As part of our analysis procedure, we enumerate root mass from fresh wet weight, allowing us to determine whether the expression of the COG gene cassette is influencing root growth. In these analyses, for a transgenic line to exhibit a statistically significant difference in root mass as compared to its respective control, all three biological replicates, each having between 10 and 25 experimental replicates (Supplementary Table 2), must be significantly different (p < 0.05, MWW). In the COG overexpression root mass analyses, COG3-1-OE, COG4-1-OE, and COG4-2-OE roots have a statistically significant decrease in root mass as compared to the pRAP15-ccdB control (p < 0.05, MWW). Conversely, COG5-1-OE root mass is statistically increased in comparison to the pRAP15-ccdB control (p < 0.05, MWW). In the RNAi transgenic lines, the COG3-1-RNAi, COG 4-1-RNAi, COG 5-1-RNAi, COG 7-1-RNAi, and COG 7-2-RNAi lines have a statistically significant increase in mass as compared to the pRAP17-ccdB control (p < 0.05, MWW). Among the studied COG genes, transgenic COG3-1 and COG4-1 roots are statistically significantly decreased in mass in the overexpression lines and increased in the RNAi lines (p < 0.05, MWW). In contrast, transgenic COG5-1 roots are statistically significantly increased in mass in both the overexpression and RNAi lines as compared to their pRAP15-ccdB and pRAP17-ccdB controls, respectively (p < 0.05, MWW). Consequently, by our analysis methods, COG3-1 and COG4-1 have an influence on G. max root growth.
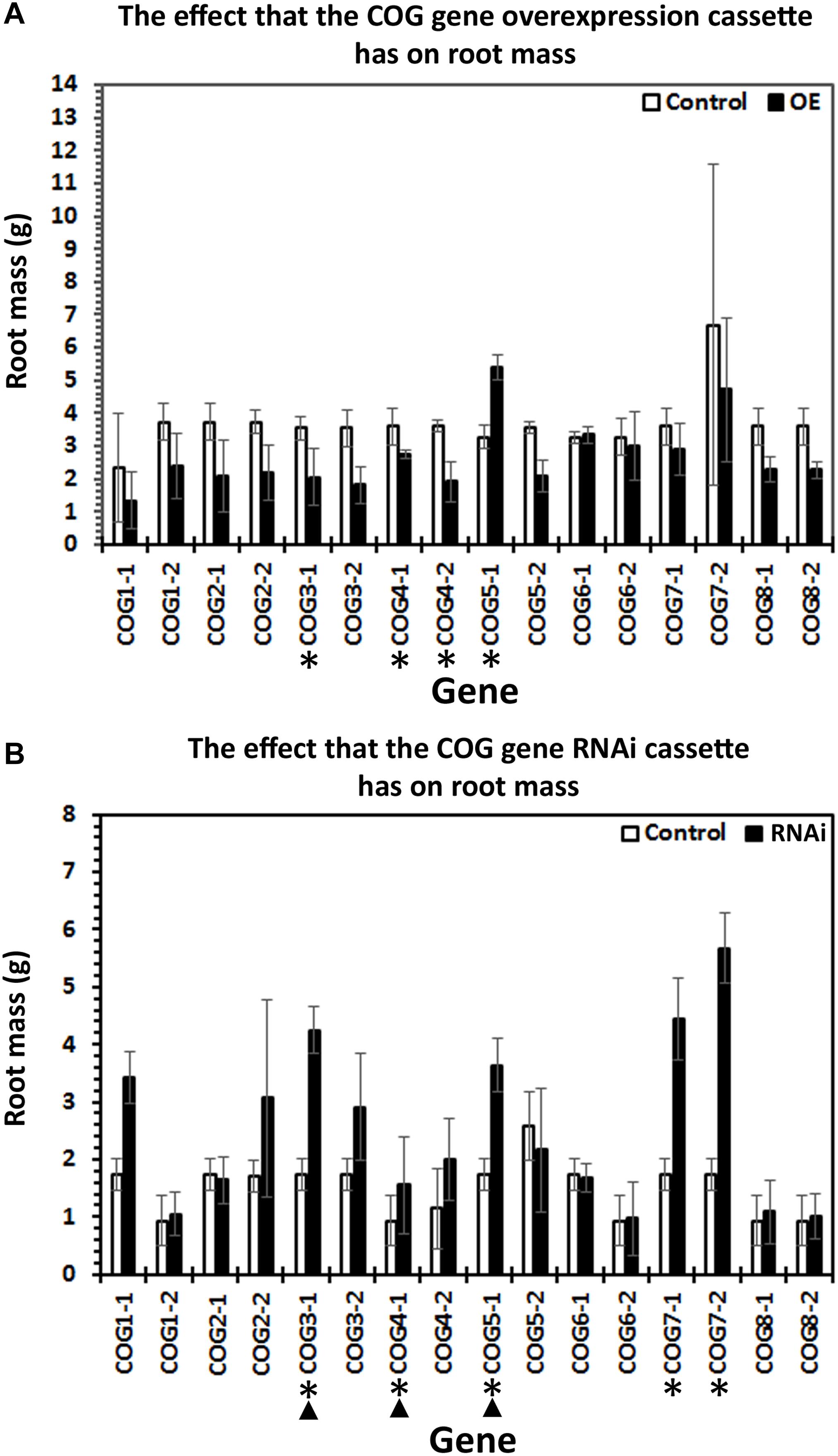
Figure 4. The effect the expression of the conserved oligomeric Golgi (COG) genetic cassette has on root mass. One-month-old transgenic root systems were weighed for their wet mass in grams (g) with three biological replicates with each biological replicate having between 10 and 25 root systems. Standard error is shown. (∗) Roots having statistically significant differences occurring between the control and COG-overexpressing (OE) or RNAi lines are indicated [p < 0.05, Mann–Whitney–Wilcoxon (MWW)]. (▲) Roots having statistically significant differences occurring between the control and COG-OE and the RNAi lines (p < 0.05, MWW). Experiments and statistical analyses have been performed from three independent biological replicates each having 10–25 independent transgenic root systems.
The Harpin Elicitor Influences the Expression of Conserved Oligomeric Golgi Genes
Prior RT-qPCR-based experiments demonstrated that G. max seeds treated with a harpin α/β elicitor, in addition to suppressing H. glycines parasitism, leads to an increase in the relative transcript abundance of several resistance genes including α-SNAP (Aljaafri et al., 2017). The α-SNAP gene, located on chromosome 18, is a component of the rhg1 locus and generally believed to be at least among the genes responsible for the rhg1 phenotype (Matsye et al., 2011, 2012; Cook et al., 2012; Sharma et al., 2016). The α-SNAP gene has first been identified in S. cerevisiae as Sec17p (α-SNAP) (Novick et al., 1980). α-SNAP binds the syntaxin, Sed5p, which is involved in retrograde trafficking through its interaction with the COG complex (Shestakova et al., 2007). Therefore, an indirect link exists between COG and α-SNAP through Sed5p. In experiments presented here, RT-qPCR of cDNA template synthesized from RNA isolated from roots growing from harpin α/β-treated germinating seedlings results in an increase in the relative transcript abundances of >1.5-fold (p < 0.05, Student’s t-test) for 13 COG genes, including COG1-2, COG2-1, COG2-2, COG3-1, COG3-2, COG4-1, COG4-2, COG5-1, COG5-2, COG6-1, COG6-2, COG7-2, COG8-1 (Figure 5) (Aljaafri et al., 2017). The results are consistent with those obtained for other genes functioning in G. max resistance to H. glycines whose relative transcript abundances have been examined from harpin α/β-treated seedlings (Aljaafri et al., 2017; Lawaju et al., 2018; McNeece et al., 2019).
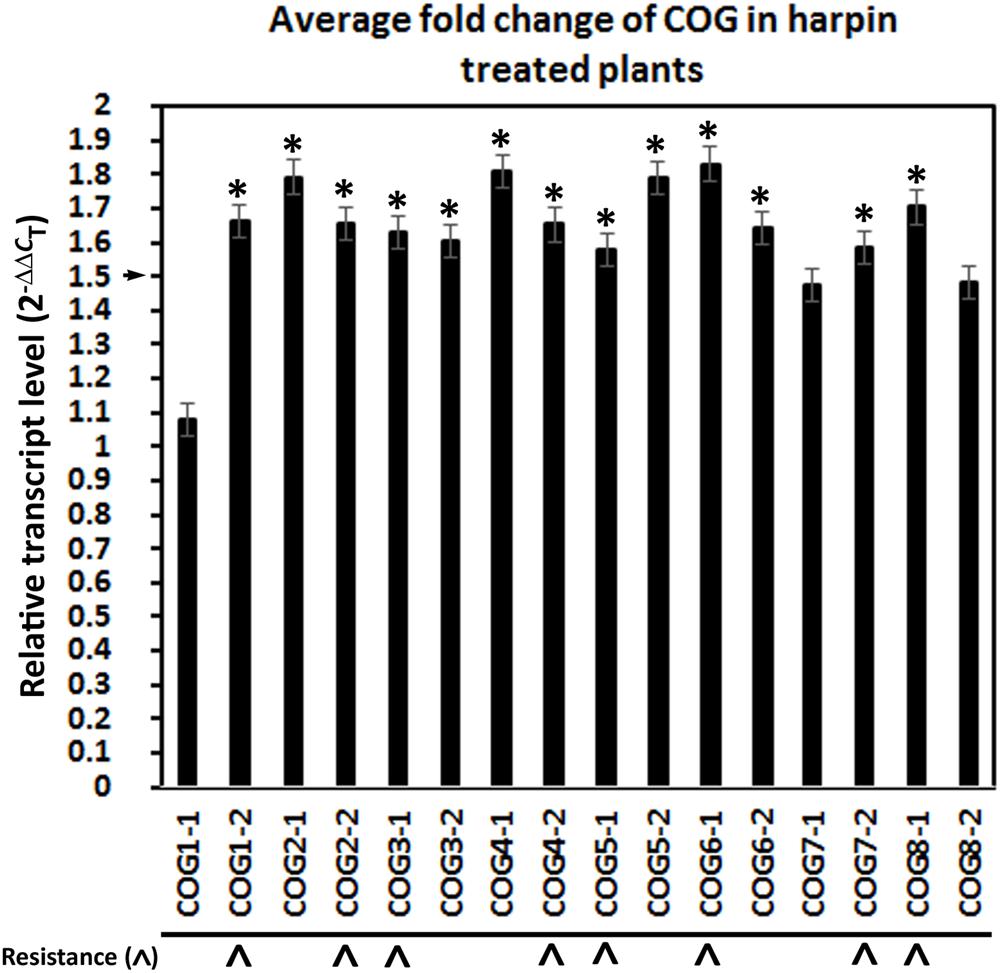
Figure 5. Treatment of G. max seeds with the bacterial effector harpin α/β results in the induction of conserved oligomeric Golgi (COG) gene expression. Gene expression calculated using the (2–ΔΔCT) method (Livak and Schmittgen, 2001). (*) The gene is considered induced in expression if the relative level of expression is induced by 1.5-fold (p ≤ 0.05, Student’s t-test). (∧) COG genes having a function in resistance by the criteria set and demonstrated in the genetic engineering studies. RT-qPCR performed as already described.
Altered Conserved Oligomeric Golgi Gene Expression Influences Syntaxin 31 (SYP38) Transcript Abundance
Prior experiments performed on G. max have shown that α-SNAP and syntaxin 31 (SYP38) (Glyma.14G064300) gene expression are co-regulated (Pant et al., 2014; Sharma et al., 2016). Analyses presented here have been performed to determine whether the relative transcript abundance of the G. max Sed5p homolog, syntaxin 31, is influenced by COG overexpression or RNAi. The results of those experiments are presented (Figure 6). In general, COG overexpression increases the relative transcript abundance of syntaxin 31 using RPS21 as a gene expression control (p < 0.05, Student’s t-test). Among these COG transgenic lines, however, the relative transcript abundance of syntaxin 31 is increased in the COG overexpression lines while also decreased in the COG RNAi lines only for COG4-2 and COG5-1 OE and RNAi transgenic lines (Figure 6). Therefore, COG4-2 and COG5-1 appear to influence the relative transcript abundance of syntaxin 31 at some level.
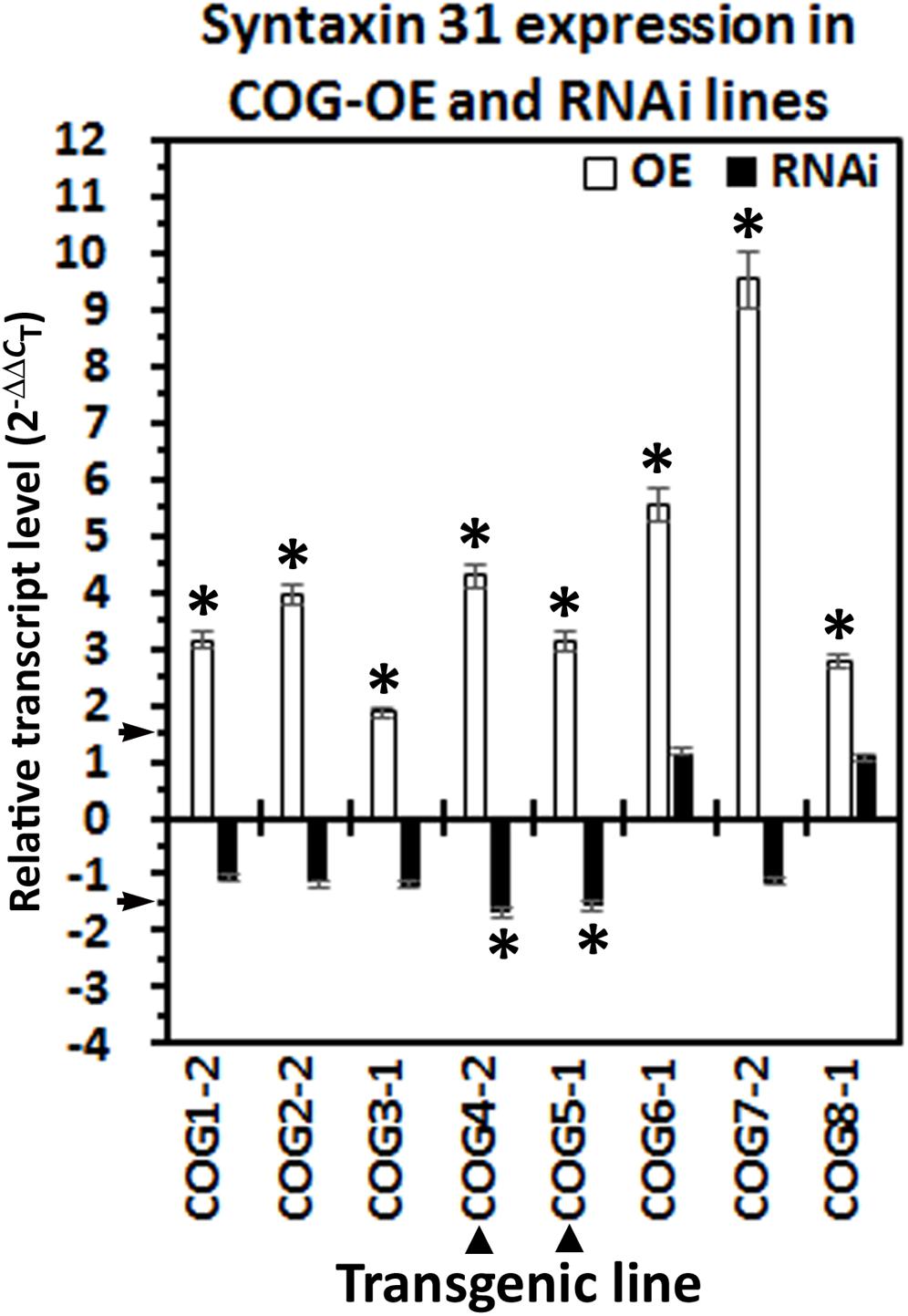
Figure 6. Syntaxin 31 expression in the transgenic conserved oligomeric Golgi (COG) lines. The cDNA made from the transgenic COG lines has been used in RT-qPCR experiments to determine the relative level of expression of syntaxin 31 (SYP38). (∗) Increased or decreased in its relative level of expression calculated using (2–ΔΔCT) (Livak and Schmittgen, 2001). (▲) Syntaxin 31 is increased in its relative transcript abundance in the transgenic COG-overexpressing (OE) lines and decreased in its relative transcript abundance in the transgenic COG-RNAi lines. RT-qPCR performed as already described.
Discussion
The G. max COG complex has been functionally characterized as it relates to its involvement in resistance to parasitism by H. glycines. The experiments have been performed because components of the vesicle trafficking machinery (myosin XI), vesicle tethering (Sec4), exocyst, and the membrane fusion complex (20S particle including SNARE) function in resistance to H. glycines (Cook et al., 2012; Matsye et al., 2012; Pant et al., 2014; Sharma et al., 2016, 2020; Klink et al., 2017). Among these genes is α-SNAP, a component of the “resistance to heterodera glycines” (rhg1) major H. glycines resistance locus (Matsye et al., 2011, 2012; Cook et al., 2012; Sharma et al., 2016). The observations are important from the standpoint that within these analyses occurred the identification of the G. max syntaxin 31 (SYP38), also functioning effectively in resistance (Pant et al., 2014). Syntaxin 31 is homologous to the S. cerevisiae Sed5p, a component of the SNARE complex functioning at the Golgi apparatus. In relation to the functional analysis presented here, Sed5p interacts with COG4 and COG6, which provides additional support to the hypothesis that the COG complex functions in the defense response that G. max has to H. glycines (Shestakova et al., 2007). Furthermore, Sed5p binds another protein, Sec17p (Hardwick and Pelham, 1992; Lupashin et al., 1997; Bubeck et al., 2008). Sec17 is the founding member of the α-SNAP gene family later identified in humans and then other organisms including plants (Novick et al., 1980; Clary et al., 1990; Sanderfoot et al., 2000). The α-SNAP gene is generally believed to be at least among the genes functioning at rhg1 during resistance to H. glycines parasitism (Matsye et al., 2011, 2012; Cook et al., 2012, 2014; Bekal et al., 2015; Bayless et al., 2016, 2018; Sharma et al., 2016; Lakhssassi et al., 2017; Butler et al., 2019). However, its role within the complex, expressed rhg1 locus containing various copies of three genes including α-SNAP, an amino acid transporter, and a wound inducible protein, copia retrotransposon, and various other structural elements are under continued study, which is important due to the nature of the locus (Melito et al., 2010; Cook et al., 2012, 2014; Bayless et al., 2016, 2018; Lakhssassi et al., 2017; Butler et al., 2019). Within this study, the principal findings are that the G. max COG complex families each has two members, one component of each gene family functions in defense, the expression of some of the family members are inducible by the bacterial effector harpin and some appear to influence the expression of syntaxin 31. These findings are discussed.
The Conserved Oligomeric Golgi Complex Function
Original experiments that have been performed in S. cerevisiae led to the identification of the COG complex (Wuestehube et al., 1996; VanRheenen et al., 1998; Whyte and Munro, 2001; Ram et al., 2002). These findings were important to the analysis presented here because it has led to an understanding of how a number of components acting in vesicle transport may function in resistance in the G. max–H. glycines pathosystem. The COG complex functions in endosome-to-trans Golgi network (TGN) retrograde transport and is present in most eukaryotes (Koumandou et al., 2007). Therefore, it is expected to be involved in many cellular physiological processes, including protein stability, protein folding, protein–protein interactions, glycan-dependent quality control processes in the ER, among others (Moremen et al., 2012; Hebert et al., 2014). Consistent with this idea is the demonstration that, in A. thaliana, more than a thousand different N-glycosylated proteins have been identified with high confidence (Zielinska et al., 2010, 2012; Song et al., 2013; Strasser, 2016). As expected, mutations in COG genes generally lead to defects in glycosylation (Pokrovskaya et al., 2011). This defect occurs because of the impairment of recycling of enzymes functioning in glycosylation that would normally occur between the Golgi apparatus cisternae (Pokrovskaya et al., 2011). In S. cerevisiae, the COG complex interacts with the syntaxin Sed5p through its interactions with COG4 and COG6 (Shestakova et al., 2007). These observations mean that Sed5p interacts with both COG sub-complexes, indicating an important interaction that is central to cellular function. Observations also made in S. cerevisiae show that Sed5p interacts with Sec17p, the yeast homolog of α-SNAP (Hardwick and Pelham, 1992; Lupashin et al., 1997). Consequently, it could be expected that the COG complex would also perform a role in the resistant reaction that G. max has to H. glycines. The analysis presented here has been done because no functional analyses have been performed on COG complex genes as it relates to plant parasitic nematodes.
The G. max Conserved Oligomeric Golgi Complex
The analysis presented here has identified the G. max COG complex genes. There are two G. max COG paralogs for each A. thaliana COG gene. This observation is consistent with the allotetraploid nature of the G. max genome (Schmutz et al., 2010). However, no localized gene copy number amplification has been observed for any of the COG complex gene families like what has been observed for another vesicle transport complex, the exocyst (EXOC) (Cvrčková et al., 2012). As already described, localized gene duplication has been reported to be important to the resistant reaction that G. max has to H. glycines (Cook et al., 2012, 2014; Lakhssassi et al., 2017). Like the COG complex, the plant exocyst is also composed of eight subunits (EXOC1–8), but the plant EXOC7 gene has experienced an extensive proliferation into many subtypes that likely have specialized functions (Cvrčková et al., 2012). The structure of the COG complex found in G. max, therefore, is also similar to that found for S. cerevisiae where its COG genes have been identified in four genetic screens (Wuestehube et al., 1996; VanRheenen et al., 1998; Whyte and Munro, 2001; Ram et al., 2002). Analyses have shown that while it is clear that COG components exist in plants, their level of conservation is low, with comparative analyses to human COG protein sequences occurring in the range of 20–34% (Latijnhouwers et al., 2005). The G. max COG complex protein sequence homology to its homologs in A. thaliana spans from a low of 62% between A. thaliana COG1 and G. max COG1-1 to a high of 82% occurring between the A. thaliana COG3 and G. max COG3-1 and A. thaliana COG8 and G. max COG8-2.
Functional Analysis of G. max Conserved Oligomeric Golgi Complex Genes Root Growth
A functional analysis of three biological replicates, each having 10–25 experimental replicates, for the eight COG complex gene families has been performed for all 16 of its corresponding genes. The combination of impairing the susceptible reaction through COG gene overexpression in the otherwise H. glycines-susceptible G. max[Williams 82/PI 518671] and facilitating parasitism in the otherwise H. glycines-resistant (G. max[Peking/PI 548402]) is taken as evidence supporting that the COG gene performs some role in the resistant reaction (Pant et al., 2014). The functional experiments revealed by RT-qPCR of each targeted gene demonstrate that it is possible to retrieve transgenic roots for each COG complex gene. Earlier studies have indicated that, in some cases, it is not possible to obtain transgenic roots for genes targeted for overexpression (myosin XI) possibly due to toxic effects caused by the transgenic cassettes (Austin et al., 2019). The toxic nature of even specific variant components of the rhg1 locus, itself, and their direct binding partners has been reported (Bayless et al., 2016, 2018). These observations are consistent with the observation of the co-regulated nature of genes functioning in resistance in this pathosystem (Pant et al., 2014; Sharma et al., 2016; McNeece et al., 2019).
Part of the functional experiments used to determine the effect that the targeted resistance gene (COG complex component) has on H. glycines parasitism is to weigh the wet mass of each root. COG3-1-OE, COG4-1-OE, and COG5-1-OE roots have a 40.96, 23.68, and 46.8% decrease in root mass, respectively. In contrast, COG5-1 roots have a 1.64-fold increase in root mass. Complementary experiments using RNAi have also identified altered root mass in certain cases. For example, RNAi roots for COG3-1, COG4-1, COG5-1, COG7-1, and COG7-2 have increased masses of 2.45-fold, 1.66-fold, 2.09-fold, 2.56–fold, and 3.26-fold, respectively. The rest of the transgenic lines show no statistically significant effects in root mass regarding COG gene overexpression or RNAi. In comparisons of the transgenic experiments on the COG genes, COG3-1, COG4-1, and COG5-1 show effects in both the overexpression and RNAi lines. COG3-1 and COG4-1 show contrasting effects where their overexpression decreases root mass, while the RNAi increases root mass. COG5-1 shows an increase in both overexpression and RNAi lines. As has been reported for the G. max vesicle transport genes functioning in resistance to H. glycines parasitism, there appears to be a balance that is important to the function of the structure, and the balance in some cases appears to be manifested through co-regulated transcription and in its absence may be cytotoxic (Pant et al., 2014; Sharma et al., 2016; Bayless et al., 2016, 2018; Austin et al., 2019). Similar observations have been made in A. thaliana for COG7 embryo yellow (EYE) gene that functions in maintenance of the meristem (Ishikawa et al., 2008). The eye mutants are bushy, have shoot apical meristems with aberrant organization, and have an altered composition of their cell walls (Ishikawa et al., 2008). This is an important observation because the secreted hemicellulose-modifying gene xyloglucan endotransglycosylase/hydrolase (XTH) of G. max has been shown to have a significant role in the resistant reaction to H. glycines (Pant et al., 2014). Even the heterologous expression of the G. max XTH43 gene in Gossypium hirsutum (cotton) has led to a high degree of resistance to a different parasitic nematode, Meloidogyne incognita (Niraula et al., 2020). XTH is targeted to the Golgi apparatus prior to its secretion into the apoplast where it functions in cell wall modification (Fry, 1989; Fry et al., 1992; Nishitani and Tominaga, 1992; Nishitani, 1998). The Golgi apparatus, thus, serves prominently in processes involving cell wall modification, requiring the import of enzymes and glycoproteins from the ER to the Golgi via transition vesicles (Zhao and Colley, 2008; Handford et al., 2012). However, the synthesis of xyloglucan and modification of xyloglucan, itself, occur in the Golgi apparatus, first in the cisternae then moving to the medial- and trans-Golgi as XyG matures (Cocuron et al., 2007; Chevalier et al., 2010). Transport of the matrix polysaccharides and enzymes to the cell membrane then occurs through secretory vesicles (Kim and Brandizzi, 2016). The COG complex is also involved in homeostasis, pollen tube growth, and basic aspects of cell wall metabolism, so impairing individual components of the structure would be expected to have observable effects (Oda et al., 2015; Tan et al., 2016; Vukašinović et al., 2017; Rui et al., 2020).
G. max Conserved Oligomeric Golgi Genes and a Role in the Resistance to H. glycines Parasitism
The results of the functional studies demonstrate that COG complex gene overexpression has the general capability to impair the ability of H. glycines to parasitize G. max roots, except for COG6-2 and COG7-2. The overexpression of the remaining G. max COG complex genes in analyses of H. glycines cysts in the wr system analyses leads to a low 62.68% decrease in H. glycines parasitism in the COG4-2-OE transgenic lines to a high 87.12% decrease in parasitism in the COG1-2-OE transgenic lines. In contrast, the RNAi of the COG complex genes has less of a general ability to affect H. glycines parasitism, occurring for nine of the 16 studied COG genes. The COG complex genes shown in RNAi experiments to function in resistance span a low 3-fold increase in H. glycines parasitism in COG8-1 to a high 22.1-fold in COG7-2. In all, through the combination of overexpression and RNAi, one member of each COG gene family has been shown to function in the resistance. The genes include COG1-2, COG2-2, COG3-1, COG4-2, COG5-1, COG6-1, COG7-2, and COG8-1. The demonstration that COG3-1 functions in the resistant reaction is consistent with the observations that the hvCOG3 functions in resistance to fungal penetration in wheat (Ostertag et al., 2013). The conclusion drawn from the analysis presented here is that at least one gene from each COG complex gene family appears to function in defense. Furthermore, there appears to be a level of specificity regarding the genes that do participate in the resistant reaction since the remaining paralogs do not perform a role in defense. The results indicate that each component is important to the process, and it is possible that, by removing even just one component, the function of the whole structure is impaired. If this concept is true, the COG complex functions in a manner that is analogous to the exocyst by requiring each of its component parts for function. Furthermore, from the experiments presented here and results presented in other systems, we expect G. max COG genes to be expressed during the resistant reaction (TerBush et al., 1996; VanRheenen et al., 1998; Whyte and Munro, 2001; Ram et al., 2002; Kulich et al., 2010, 2015, 2018; Ostertag et al., 2013).
How the Conserved Oligomeric Golgi Complex Results Relate to Defense in the G. max–H. glycines Pathosystem
Prior work in the G. max–H. glycines pathosystem accomplished the transcriptional mapping of the major resistance locus, rhg1 (Matsye et al., 2011). Subsequent experiments have identified a number of variants within the locus and complex interactions occurring with their direct binding partners and even H. glycines effectors that may impair the function of these proteins (Cook et al., 2012; Bekal et al., 2015; Bayless et al., 2016, 2018; Lakhssassi et al., 2017). Subsequent functional studies demonstrated that the overexpression of α-SNAP in the H. glycines-susceptible genotype G. max[Williams 82/PI 518671] leads to those susceptible plants exhibiting an approximately 60% decrease in parasitism (Matsye et al., 2012; Sharma et al., 2016). The results of those analyses have implicated that a functional vesicle transport apparatus plays an important defense role in the G. max–H. glycines pathosystem (Matsye et al., 2012; Pant et al., 2014; Sharma et al., 2016).
The α-SNAP gene has been first identified as the Secretion (Sec) gene (Sec17) in a mutant screen aimed to understand secretion (Novick et al., 1980, 1981). The α-SNAP gene name had been later denoted in studies of human cells and found to be homologous to Sec17 (Clary et al., 1990). The α-SNAP protein interacts with syntaxin 31, mediating the delivery of transition vesicles to the cis face of the Golgi apparatus (Hardwick and Pelham, 1992; Lupashin et al., 1997; Bubeck et al., 2008; Matsye et al., 2012; Pant et al., 2014). Subsequent work has demonstrated the involvement of α-SNAP at all sites where vesicle and target membrane fusion occur, making it a universal component of membrane fusion (Zick et al., 2015). The importance of a functional 20S particle to pathogenesis has been revealed in a number of studies that have identified microbial neurotoxins that target SNARE proteins in animal systems and thus inhibit secretion (Schiavo et al., 1992a,b, 1994; Pellegrini et al., 1995; Chai et al., 2006; Jin et al., 2006; Strotmeier et al., 2012; Bennett et al., 2013). The COG complex relates to this structure because it interacts with a protein known as golgin-84 (Bascom et al., 1999; Sohda et al., 2010). Golgin-84 is a coiled-coil, integral membrane protein of the Golgi apparatus (Bascom et al., 1999). The interaction between the COG complex and golgin-84 is required for the assembly of a SNARE complex that functions in intra-Golgi retrograde transport (Sohda et al., 2010). Other interactions occurring between the COG complex and SNARE have also been observed, further strengthening the hypothesis that the COG complex performs an important function in intramembrane vesicle movement through specific target and vesicle membrane protein interactions (Suvorova et al., 2002; Shestakova et al., 2007; Laufman et al., 2011). The specific interactions include how the COG complex interacts directly with Syntaxin 6 as it positively regulates the endosome-TGN to facilitate retrograde transport (Laufman et al., 2011). Another interaction includes the COG complex and its interactions with Golgi SNAREs to facilitate intra-Golgi trafficking (Suvorova et al., 2002). The COG complex also interacts with syntaxin 5a, also known as Sed5p, to enhance intra-Golgi stability of the SNARE complex (Shestakova et al., 2007). Notably, Sed5p binds specifically to α-SNAP (Sec17p) at the cis face of the Golgi apparatus to facilitate vesicle fusion that ultimately leads to secretion (Hardwick and Pelham, 1992). Furthermore, other bacterial effectors that target the COG complex directly alter the movement of membrane to facilitate pathogen biology (Miller et al., 2017). The results presented here provide more context for those observations and show that by increasing the amount of individual COG complex gene expression, it is possible to facilitate an effective defense response. This process may happen because the other vesicle transport proteins are already being induced in their expression to accommodate a resistance reaction. In contrast, by impairing COG complex transcription, a normally H. glycines-resistant genotype like G. max[Peking/PI 548402] facilitates parasitism.
Components of the Conserved Oligomeric Golgi Complex Are Inducible by the Harpin Elicitor
The expression of the G. max COG genes can be induced by the bacterial elicitor harpin.
The harpin protein has been first identified from the causative agent of fire blight disease, Erwinia amylovora, which infects species of the Rosaceae (Wei et al., 1992). Harpins are heat-stable, glycine-rich proteins found in several gram-negative plant pathogenic bacteria and are secreted by the bacterial type III secretion system (Wei and Beer, 1993; Bogdanove et al., 1996; Choi et al., 2013). Harpins can function during the hypersensitive response but may also function through a systemic defense response (Wei et al., 1992; Dong et al., 1999; Neyt and Cornelis, 1999; Aljaafri et al., 2017). Harpin is known to function through ETI (Jones and Dangl, 2006). These interactions then lead to the transduction of signal through the mitogen-activated protein kinase (MAPK) cascade to elicit a defense response (Mindrinos et al., 1994; Grant et al., 1995; van der Biezen and Jones, 1998; Desikan et al., 1999, 2001; Lee et al., 2001; Mackey et al., 2002, 2003; Coppinger et al., 2004). Harpin, NDR1, and MAPKs have all been shown to be capable of functioning in the resistant reaction that G. max has to H. glycines and lead to the induced expression of α-SNAP, among other resistance genes (Aljaafri et al., 2017; McNeece et al., 2017, 2019). The genes also function to impair the pathogenicity of other pathogens of G. max (Lawaju et al., 2018). The results presented here showing the harpin-induced expression of COG genes functioning in resistance are consistent with observations that harpin can be effective in eliciting a resistant reaction (Aljaafri et al., 2017). However, observations have been made that show harpin can be deactivated through the activities of other bacterial effectors that specifically target it (O’Neill et al., 2018).
Syntaxin 31 Transcript Levels Correlate to Conserved Oligomeric Golgi Gene Expression
Prior experiments have demonstrated the co-regulated nature of various G. max vesicle transport components involved in the resistant reaction to H. glycines (Pant et al., 2014; Sharma et al., 2016). The demonstration that Sed5p binds to Sec17p, COG4, and COG6 led to experiments to examine the relative transcript abundance of syntaxin 31 in the transgenic COG-overexpressing and RNAi lines (Hardwick and Pelham, 1992; Lupashin et al., 1997; Shestakova et al., 2007; Bubeck et al., 2008). The results of those experiments have revealed that there is an increased transcript level of syntaxin 31 in each of the transgenic COG-overexpressing lines that have been shown to impair H. glycines parasitism. These observations are consistent with experiments showing that several of the vesicle transport genes are co-regulated or that a balanced level of expression is important (Pant et al., 2014; Sharma et al., 2016; Bayless et al., 2016, 2018). However, the reciprocal experiments determining COG gene expression in syntaxin 31-overexpressing roots have not been performed. In contrast, suppressed syntaxin 31 expression is observed only in the COG4-2-RNAi and COG5-1-RNAi lines. In the other COG-RNAi lines, there is no observed effect on syntaxin 31 expression. This result, in itself, is interesting since it is these G. max[Peking/PI 548402] transgenic lines that become susceptible to H. glycines parasitism. From these experiments, only the transgenic COG4-2 and COG5-1 are experiencing modulated syntaxin 31 expression that correlates to the respective COG overexpression (induced syntaxin 31 transcription) or RNAi lines (suppressed syntaxin 31 transcription). Notably, COG4 and COG5 occur on each of the two lobes of the COG complex so signals relating to each lobe of the COG complex may be important in this regulated transcription. Furthermore, prior experiments have shown that Sed5p binds COG4p (Shestakova et al., 2007).
Summary
Genes composing the G. max COG complex have been identified. Furthermore, transgenic functional analyses, a harpin elicitor study, and the effect that COG component overexpression/RNAi have on syntaxin 31 expression have been performed (Table 2). Furthermore, the combination of COG gene overexpression in the H. glycines-susceptible G. max[Williams 82/PI 518671] (along with the appropriate pRAP15-ccdB control) and RNAi in the H. glycines-resistant G. max[Peking/PI 548402] (along with the appropriate pRAP17-ccdB control) has led to the identification of eight COG genes that perform a role in resistance. An RT-qPCR analysis targeting each of the 16 G. max COG genes using RNA isolated from harpin α/β-treated seeds has resulted in the determination that 12 of them are increased in their relative transcript abundance. Lastly, altered COG expression affects the transcript abundance of syntaxin 31. The overall results are consistent with those observed in other protein complexes that relate to vesicle transport. Specifically, the exocyst requires each component for the assembly and function of the structure. Furthermore, the balanced expression, possibly through regulated co-expression of components relating to the resistance reaction that G. max has to H. glycines, is also receiving similar treatment. The further analyses of these and additional genes that relate to the vesicle transport process will undoubtedly identify further intricacies regarding their involvement during the resistant reaction. Some of these interactions may come from yet unexplored membrane components. For example, experiments have shown the COG complex protein COG2 with EXOC70A1 via vesicle tethering 1 (VETH1) and VETH2 to promote xylem vessel differentiation (Oda et al., 2015). These recent results indicate that the cross-talk that is occurring between these different components functioning in vesicle trafficking is more prevalent and more important than previously understood. Furthermore, the results indicate it is possible that H. glycines may specifically target other Golgi-associated proteins for its benefit like what it already does for a-SNAP.
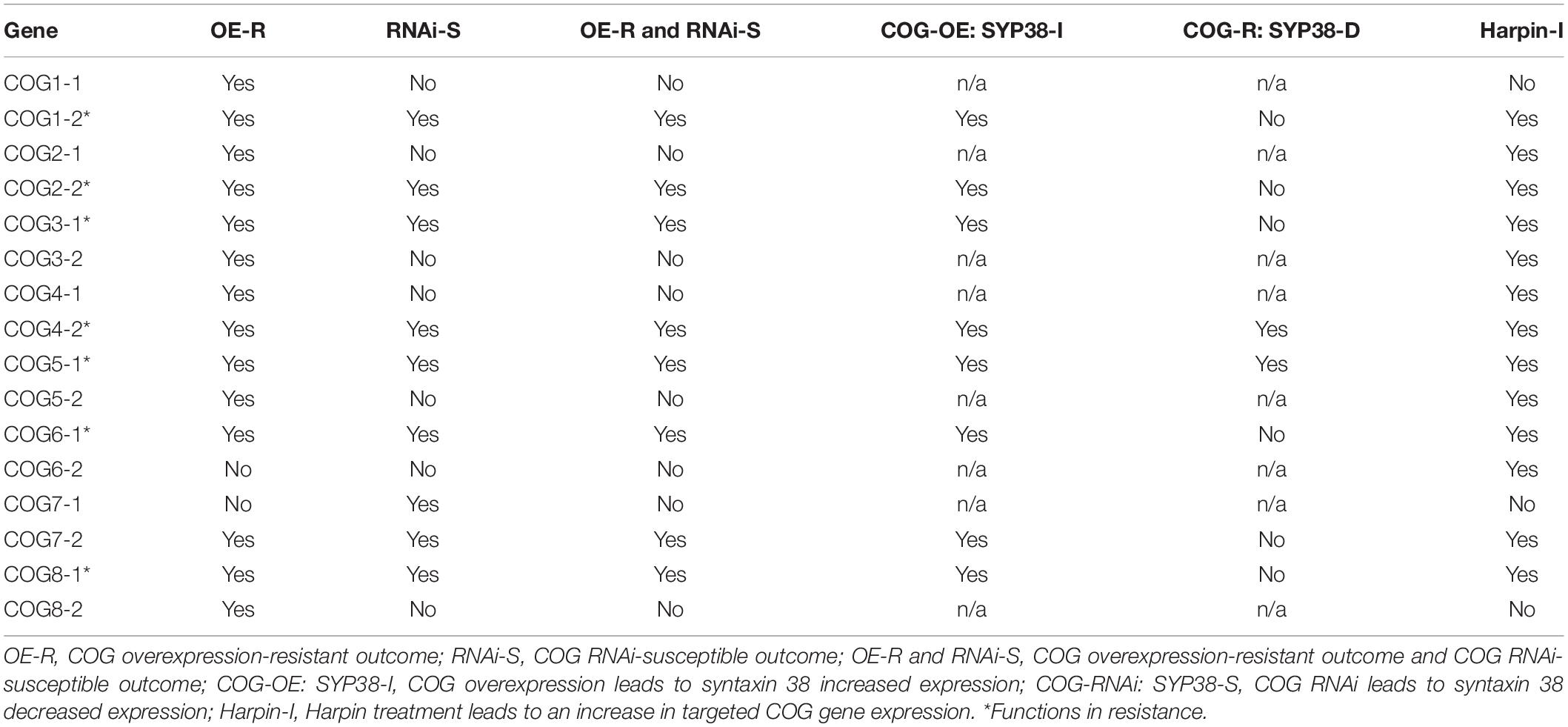
Table 2. Summary of the data pertaining to this analysis of the G. max conserved oligomeric Golgi (COG) complex.
Data Availability Statement
The original contributions presented in the study are included in the article/Supplementary Material, further inquiries can be directed to the corresponding author.
Author Contributions
BL: conceptual planning, identifying COG genes, comparative analyses to A. thaliana proteome, PCR primer design, cloning genes, plant genetic transformation, nematode infections, cyst extractions, qRT-PCR, quantitative analyses, and manuscript preparation. PN: affymetrix data extraction and analysis, qRT-PCR, and quantitative analyses. GL and KL: conceptual planning, nematode infections, cyst extractions, and quantitative analysis. VK: conceptual planning, quantitative analyses, and manuscript preparation. All authors contributed to the article and approved the submitted version.
Conflict of Interest
The authors declare that the research was conducted in the absence of any commercial or financial relationships that could be construed as a potential conflict of interest.
Acknowledgments
The authors are thankful for start-up support provided by the Department of Biological Sciences, Mississippi State University. The authors are thankful for an awarded competitive Special Research Initiative grant from the College of Arts and Sciences at Mississippi State University (VK). The authors are thankful to the Department of Biochemistry, Molecular Biology, Entomology and Plant Pathology, Mississippi State University, for greenhouse space. The authors are grateful to Yixiu Pinnix for her technical help.
Supplementary Material
The Supplementary Material for this article can be found online at: https://www.frontiersin.org/articles/10.3389/fpls.2020.564495/full#supplementary-material
Supplementary Table 1 | PCR and RT-PCR primers used in the analysis.
Supplementary Table 2 | Number of experimental and biological replicates employed in the analysis.
Abbreviations
COG, conserved oligomeric Golgi; ETI, effector triggered immunity.
Footnotes
References
Aljaafri, W. A. R., McNeece, B. T., Lawaju, B. R., Sharma, K., Niruala, P. M., Pant, S. R., et al. (2017). A harpin elicitor induces the expression of a coiled-coil nucleotide binding leucine rich repeat (CC-NB-LRR) defense signaling gene and others functioning during defense to parasitic nematodes. Plant Physiol. Biochem. 121, 161–175. doi: 10.1016/j.plaphy.2017.10.004
Altschul, S. F., Gish, W., Miller, W., Myers, E. W., and Lipman, D. J. (1990). Basic local alignment search tool. J. Mol. Biol. 215, 403–410. doi: 10.1016/S0022-2836(05)80360-2
Austin, H. W., McNeece, B. T., Sharma, K., Niraula, P. M., Lawrence, K. S., and Klink, V. P. (2019). An expanded role of the SNARE-containing regulon as it relates to the defense process that Glycine max has to Heterodera glycines. J. Plant Interact. 14, 276–283. doi: 10.1080/17429145.2019.1622043
Bascom, R. A., Srinivasan, S., and Nussbaum, R. L. (1999). Identification and characterization of golgin-84, a novel Golgi integral membrane protein with a cytoplasmic coiled-coil domain. J. Biol. Chem. 274, 2953–2962. doi: 10.1074/jbc.274.5.2953
Bayless, A. M., Smith, J. M., Song, J., McMinn, P. H., Teillet, A., August, B. K., et al. (2016). Disease resistance through impairment of α-SNAP-NSF interaction and vesicular trafficking by soybean Rhg1. Proc. Natl. Acad. Sci. U.S.A. 113, E7375–E7382. doi: 10.1073/pnas.1610150113
Bayless, A. M., Zapotocny, R. W., Grunwald, D. J., Amundson, K. K., Diers, B. W., and Bent, A. F. (2018). An atypical N-ethylmaleimide sensitive factor enables the viability of nematode-resistant Rhg1 soybeans. Proc. Natl. Acad. Sci. U.S.A. 115, E4512–E4521. doi: 10.1073/pnas.1717070115
Bekal, S., Domier, L. L., Gonfa, B., Lakhssassi, N., Meksem, K., and Lambert, K. N. (2015). A SNARE-like protein and biotin are implicated in soybean cyst nematode virulence. PLoS One 10:e0145601. doi: 10.1371/journal.pone.0145601
Bennett, T. L., Kraft, S. M., Reaves, B. J., Mima, J., O’Brien, K. M., and Starai, V. J. (2013). LegC3, an effector protein from Legionella pneumophila, inhibits homotypic yeast vacuole fusion in vivo and in vitro. PLoS One 82:e56798. doi: 10.1371/journal.pone.0056798
Bhattacharyya, S., Dey, N., and Maiti, I. B. (2002). Analysis of cis-sequence of subgenomic transcript promoter from the figwort mosaic virus and comparison of promoter activity with the cauliflower mosaic virus promoters in monocot and dicot cells. Virus Res. 90, 47–62. doi: 10.1016/S0166-0934(02)00146-5
Bogdanove, A. J., Wei, Z. M., Zhao, L., and Beer, S. V. (1996). Erwinia amylovora secretes harpin via a type III pathway and contains a homolog of yopN of Yersinia spp. J. Bacteriol. 178, 1720–1730. doi: 10.1128/JB.178.6.1720-1730.1996
Bubeck, J., Scheuring, D., Hummel, E., Langhans, M., Viotti, C., Foresti, O., et al. (2008). The syntaxins SYP31 and SYP81 control ER-Golgi trafficking in the plant secretory pathway. Traffic 9, 1629–1652. doi: 10.1111/j.1600-0854.2008.00803.x
Butler, K. J., Chen, S., Smith, J. M., Wang, X., and Bent, A. F. (2019). Soybean resistance locus Rhg1 confers resistance to multiple cyst nematodes in diverse plant species. Phytopathology 109, 2107–2115. doi: 10.1094/PHYTO-07-19-0225-R
Caldwell, B. E., Brim, C. A., and Ross, J. P. (1960). Inheritance of resistance of soybeans to the soybean cyst nematode, Heterodera glycines. Agron. J. 52, 635–636. doi: 10.2134/agronj1960.00021962005200110007x
Chai, Q., Arndt, J. W., Dong, M., Tepp, W. H., Johnson, E. A., Chapman, E. R., et al. (2006). Structural basis of cell surface receptor recognition by botulinum neurotoxin B. Nature 444, 1096–1100. doi: 10.1038/nature05411
Chevalier, L., Bernard, S., Ramdani, Y., Lamour, R., Bardor, M., Lerouge, P., et al. (2010). Subcompartment localization of the side chain xyloglucan-synthesizing enzymes within Golgi stacks of tobacco suspension-cultured cells. Plant J. 64, 977–989. doi: 10.1111/j.1365-313X.2010.04388.x
Choi, M. S., Kim, W., Lee, C., and Oh, C. S. (2013). Harpins, multifunctional proteins secreted by gram-negative plant-pathogenic bacteria. Mol. Plant. Microbe. Interact. 26, 1115–1122. doi: 10.1094/MPMI-02-13-0050-CR
Clary, D. O., Griff, I. C., and Rothman, J. E. (1990). SNAPs, a family of NSF attachment proteins involved in intracellular membrane fusion in animals and yeast. Cell 61, 709–721. doi: 10.1016/0092-8674(90)90482-T
Cocuron, J.-C., Lerouxel, O., Drakakaki, G., Alonso, A. P., Liepman, A. H., Keegstra, K., et al. (2007). A gene from the cellulose synthase-like C family encodes a beta-1,4 glucan synthase. Proc. Natl. Acad. Sci. U.S.A. 104, 8550–8555. doi: 10.1073/pnas.0703133104
Collier, R., Fuchs, B., Walter, N., Lutke, K. W., and Taylor, C. G. (2005). Ex vitro composite plants: an inexpensive, rapid method for root biology. Plant J. 43, 449–457. doi: 10.1111/j.1365-313X.2005.02454.x
Collins, N. C., Thordal-Christensen, H., Lipka, V., Bau, S., Kombrink, E., Qiu, J. L., et al. (2003). SNARE-protein mediated disease resistance at the plant cell wall. Nature 425, 973–977. doi: 10.1038/nature02076
Cook, D. E., Bayless, A. M., Wang, K., Guo, X., Song, Q., Jiang, J., et al. (2014). Distinct copy number, coding sequence, and locus methylation patterns underlie Rhg1-mediated soybean resistance to soybean cyst nematode. Plant Physiol. 165, 630–647. doi: 10.1104/pp.114.235952
Cook, D. E., Lee, T. G., Guo, X., Melito, S., Wang, K., Bayless, A. M., et al. (2012). Copy number variation of multiple genes at Rhg1 mediates nematode resistance in soybean. Science 338, 1206–1209. doi: 10.1126/science.1228746
Coppinger, P., Repetti, P. P., Day, B., Dahlbeck, D., Mehlert, A., and Staskawicz, B. J. (2004). Overexpression of the plasma membrane-localized NDR1 protein results in enhanced bacterial disease resistance in Arabidopsis thaliana. Plant J. 40, 225–237. doi: 10.1111/j.1365-313X.2004.02203.x
Cottam, N. P., and Ungar, D. (2012). Retrograde vesicle transport in the Golgi. Protoplasma 249, 943–955. doi: 10.1007/s00709-011-0361-7
Curtis, M. D., and Grossniklaus, U. (2003). A gateway cloning vector set for high-throughput functional analysis of genes in planta. Plant Physiol. 133, 462–469. doi: 10.1104/pp.103.027979
Cvrčková, F., Grunt, M., Bezvoda, R., Hála, M., Kulich, I., Rawat, A., et al. (2012). Evolution of the land plant exocyst complexes. Front. Plant Sci. 3:159. doi: 10.3389/fpls.2012.00159
Desikan, R., Clarke, A., Atherfold, P., Hancock, J. T., and Neill, S. J. (1999). Harpin induces mitogen-activated protein kinase activity during defence responses in Arabidopsis thaliana suspension cultures. Planta 210, 97–103. doi: 10.1007/s004250050658
Desikan, R., Hancock, J. T., Ichimura, K., Shinozaki, K., and Neill, S. J. (2001). Harpin induces activation of the Arabidopsis mitogen-activated protein kinases AtMPK4 and AtMPK6. Plant Physiol. 126, 1579–1587. doi: 10.1104/pp.126.4.1579
Dong, H., Delaney, T. P., Bauer, D. W., and Beer, S. V. (1999). Harpin induces disease resistance in Arabidopsis through the systemic acquired resistance pathway mediated by salicylic acid and the NIM1 gene. Plant J. 20, 207–215. doi: 10.1046/j.1365-313x.1999.00595.x
Endo, B. Y. (1965). Histological responses of resistant and susceptible soybean varieties, and backcross progeny to entry development of Heterodera glycines. Phytopathology 55, 375–381.
Endo, B. Y. (1991). Ultrastructure of initial responses of susceptible and resistant soybean roots to infection by Heterodera glycines. Revue Nématol. 14, 73–84.
Fotso, P., Koryakina, Y., Pavliv, O., Tsiomenko, A. B., and Lupashin, V. V. (2005). Cog1p plays a central role in the organization of the yeast conserved oligomeric Golgi complex. J. Biol. Chem. 280, 27613–27623. doi: 10.1074/jbc.M504597200
Fry, S. C. (1989). The structure and functions of xyloglucan. J. Exp. Bot. 40, 1–11. doi: 10.1093/jxb/40.1.1
Fry, S. C., Smith, R. C., Renwick, K. F., Martin, D. J., Hodge, S. K., and Matthews, K. J. (1992). Xyloglucan endotransglycosylase, a new wall-loosening enzyme activity from plants. Biochem. J. 282, 821–828. doi: 10.1042/bj2820821
Golden, A. M., Epps, J. M., Riggs, R. D., Duclos, L. A., Fox, J. A., and Bernard, R. L. (1970). Terminology and identity of infraspecific forms of the soybean cyst nematode (Heterodera glycines). Plant Dis. Rep. 54, 544–546.
Goodstein, D. M., Shu, S., Howson, R., Neupane, R., Hayes, R. D., Fazo, J., et al. (2012). Phytozome: a comparative platform for green plant genomics. Nucleic Acids Res. 40, D1178–D1186. doi: 10.1093/nar/gkr944
Grant, M. R., Godiard, L., Straube, E., Ashfield, T., Lewald, J., Sattler, A., et al. (1995). Structure of the Arabidopsis RPM1 gene enabling dual specificity disease resistance. Science 269, 843–846. doi: 10.1126/science.7638602
Haas, J. H., Moore, L. W., Ream, W., and Manulis, S. (1995). Universal PCR primers for detection of phytopathogenic Agrobacterium strains. Appl. Environ. Microbiol. 61, 2879–2884. doi: 10.1128/AEM.61.8.2879-2884.1995
Handford, M., Rodríguez-Furlán, C., Marchant, L., Segura, M., Gómez, D., Alvarez-Buylla, E., et al. (2012). Arabidopsis thaliana AtUTr7 encodes a golgi-localized UDP-glucose/UDP-galactose transporter that affects lateral root emergence. Mol. Plant 5, 1263–1280. doi: 10.1093/mp/sss074
Hardwick, K. G., and Pelham, H. R. (1992). SED5 encodes a 39-kD integral membrane protein required for vesicular transport between the ER and the Golgi complex. J. Cell Biol. 119, 513–521. doi: 10.1083/jcb.119.3.513
Haseloff, J., Siemering, K. R., Prasher, D. C., and Hodge, S. (1997). Removal of a cryptic intron and subcellular localization of green fluorescent protein are required to mark transgenic Arabidopsis plants brightly. Proc. Natl. Acad. Sci. U.S.A. 94, 2122–2127. doi: 10.1073/pnas.94.6.2122
Hebert, D. N., Lamriben, L., Powers, E. T., and Kelly, J. W. (2014). The intrinsic and extrinsic effects of N-linked glycans on glycoproteostasis. Nat. Chem. Biol. 10, 902–910. doi: 10.1038/nchembio.1651
Hofgen, R., and Willmitzer, L. (1988). Storage of competent cells for Agrobacterium transformation. Nucleic Acids Res. 16, 9877–9877. doi: 10.1093/nar/16.20.9877
Ishikawa, T., Machida, C., Yoshioka, Y., Ueda, T., Nakano, A., and Machida, Y. (2008). EMBRYO YELLOW gene, encoding a subunit of the conserved oligomeric Golgi complex, is required for appropriate cell expansion and meristem organization in Arabidopsis thaliana. Genes Cells 13, 521–535. doi: 10.1111/j.1365-2443.2008.01186.x
Jahn, R., and Fasshauer, D. (2012). Molecular machines governing exocytosis of synaptic vesicles. Nature 490, 201–207. doi: 10.1038/nature11320
Jin, R., Rummel, A., Binz, T., and Brunger, A. T. (2006). Botulinum neurotoxin B recognizes its protein receptor with high affinity and specificity. Nature 444, 1092–1095. doi: 10.1038/nature05387
Jones, J. D., and Dangl, J. L. (2006). The plant immune system. Nature 444, 323–329. doi: 10.1038/nature05286
Kim, S. J., and Brandizzi, F. (2016). The plant secretory pathway for the trafficking of cell wall polysaccharides and glycoproteins. Glycobiology 26, 940–949. doi: 10.1093/glycob/cww044
Klink, V. P., Kim, K.-H., Martins, V. E., MacDonald, M. H., Beard, H. S., Alkharouf, N. W., et al. (2009). A correlation between host-mediated expression of parasite genes as tandem inverted repeats and abrogation of the formation of female Heterodera glycines cysts during infection of Glycine max. Planta 230, 53–71. doi: 10.1007/s00425-009-0926-2
Klink, V. P., McNeece, B. T., Pant, S. R., Sharma, K., Nirula, P. M., and Lawrence, G. W. (2017). Components of the SNARE-containing regulon are co-regulated in root cells undergoing defense. Plant Signal. Behav. 12:e1274481. doi: 10.1080/15592324.2016.1274481
Koumandou, V. L., Dacks, J. B., Coulson, R. M., and Field, M. C. (2007). Control systems for membrane fusion in the ancestral eukaryote; evolution of tethering complexes and SM proteins. BMC Evol. Biol. 7:29. doi: 10.1186/1471-2148-7-29
Kulich, I., Cole, R., Drdová, E., Cvrcková, F., Soukup, A., Fowler, J., et al. (2010). Arabidopsis exocyst subunits SEC8 and EXO70A1 and exocyst interactor ROH1 are involved in the localized deposition of seed coat pectin. New Phytol. 188, 615–625. doi: 10.1111/j.1469-8137.2010.03372.x
Kulich, I., Vojtíková, Z., Glanc, M., Ortmannová, J., Rasmann, S., and Žárský, V. (2015). Cell wall maturation of Arabidopsis trichomes is dependent on exocyst subunit EXO70H4 and involves callose deposition. Plant Physiol. 168, 120–131. doi: 10.1104/pp.15.00112
Kulich, I., Vojtíková, Z., Sabol, P., Ortmannová, J., Neděla, V., Tihlaříková, E., et al. (2018). Exocyst subunit EXO70H4 has a specific role in callose synthase secretion and silica accumulation. Plant Physiol. 176, 2040–2051. doi: 10.1104/pp.17.01693
Lakhssassi, N., Liu, S., Bekal, S., Zhou, Z., Colantonio, V., Lambert, K., et al. (2017). Characterization of the soluble NSF attachment protein gene family identifies two members involved in additive resistance to a plant pathogen. Sci. Rep. 7:45226. doi: 10.1038/srep45226
Latijnhouwers, M., Hawes, C., and Carvalho, C. (2005). Holding it all together? Candidate proteins for the plant Golgi matrix. Curr. Opin. Plant Biol. 8, 632–639. doi: 10.1016/j.pbi.2005.09.014
Laufman, O., Hong, W., and Lev, S. (2011). The COG complex interacts directly with Syntaxin 6 and positively regulates endosome-to- TGN retrograde transport. J. Cell Biol. 194, 459–472. doi: 10.1083/jcb.201102045
Lauritis, J. A., Rebois, R. V., and Graney, L. S. (1983). Development of Heterodera glycines Inchoe on soybean, Glycine max. J. Nematol. 15, 272–280.
Lawaju, B. R., Lawrence, K. S., Lawrence, G. W., and Klink, V. P. (2018). Harpin-inducible defense signaling components impair infection by the ascomycete Macrophomina phaseolina. Plant Physiol. Biochem. 129, 331–348. doi: 10.1016/j.plaphy.2018.06.020
Lee, J., Klessig, D. F., and Nurnberger, T. (2001). A harpin binding site in tobacco plasma membranes mediates activation of the pathogenesis-related gene HIN1 independent of extracellular calcium but dependent on mitogen-activated protein kinase activity. Plant Cell 13, 1079–1093. doi: 10.1105/tpc.13.5.1079
Lipka, L., Dittgen, J., Bednarek, P., Bhat, R., Wiermer, M., Stein, M., et al. (2005). Pre- and postinvasion defenses both contribute to nonhost resistance in Arabidopsis. Science 310, 1180–1183. doi: 10.1126/science.1119409
Livak, K. J., and Schmittgen, T. D. (2001). Analysis of relative gene expression data using real-time quantitative PCR and the 2(-Delta Delta C(T)) method. Methods 25, 402–408. doi: 10.1006/meth.2001.1262
Lupashin, V. V., Pokrovskaya, I. D., McNew, J. A., and Waters, M. G. (1997). Characterization of a novel yeast SNARE protein implicated in Golgi retrograde traffic. Mol. Biol. Cell 8, 2659–2676. doi: 10.1091/mbc.8.12.2659
Mackey, D., Belkhadir, Y., Alonso, J. M., Ecker, J. R., and Dangl, J. L. (2003). Arabidopsis RIN4 is a target of the Type III virulence effector AvrRpt2 and modulates RPS2-mediated resistance. Cell 112, 379–389. doi: 10.1016/S0092-8674(03)00040-0
Mackey, D., Holt, B. F. III, Wiig, A., and Dangl, J. L. (2002). RIN4 interacts with Pseudomonas syringae type III effector molecules and is required for RPM1-mediated resistance in Arabidopsis. Cell 108, 743–754. doi: 10.1016/S0092-8674(02)00661-X
Mann, H. B., and Whitney, D. R. (1947). On a test of whether one of two random variables is stochastically larger than the other. Ann. Math. Stat. 18, 50–60. doi: 10.1214/aoms/1177730491
Matsye, P. D., Kumar, R., Hosseini, P., Jones, C. M., Tremblay, A., Alkharouf, N. W., et al. (2011). Mapping cell fate decisions that occur during soybean defense responses. Plant Mol. Bio. 77, 513–528. doi: 10.1007/s11103-011-9828-3
Matsye, P. D., Lawrence, G. W., Youssef, R. M., Kim, K.-H., Matthews, B. F., Lawrence, K. S., et al. (2012). The expression of a naturally occurring, truncated allele of an α-SNAP gene suppresses plant parasitic nematode infection. Plant Mol. Bio. 80, 131–135. doi: 10.1007/s11103-012-9932-z
McNeece, B. T., Pant, S. R., Sharma, K., Nirula, P. M., Lawrence, G. W., and Klink, V. P. (2017). A Glycine max homolog of NON-RACE SPECIFIC DISEASE RESISTANCE 1 (NDR1) alters defense gene expression while functioning during a resistance response to different root pathogens in different genetic backgrounds. Plant Physiol. Biochem. 114, 60–71. doi: 10.1016/j.plaphy.2017.02.022
McNeece, B. T., Sharma, K., Lawrence, K. S., Lawrence, G. W., and Klink, V. P. (2019). The mitogen activated protein kinase (MAPK) gene family functions as a cohort during the Glycine max defense response to Heterodera glycines. Plant Physiol. Biochem. 137, 25–41. doi: 10.1016/j.plaphy.2019.01.018
Melito, S., Heuberger, A. L., Cook, D., Diers, B. W., MacGuidwin, A. E., and Bent, A. F. (2010). A nematode demographics assay in transgenic roots reveals no significant impacts of the Rhg1 locus LRR-Kinase on soybean cyst nematode resistance. BMC Plant Biol. 10:104. doi: 10.1186/1471-2229-10-104
Miller, C. N., Smith, E. P., Cundiff, J. A., Knodler, L. A., Blackburn, J. B., Lupashin, V., et al. (2017). A brucella type IV effector targets the COG tethering complex to remodel host secretory traffic and promote intracellular replication. Cell Host Microbe 22, 317–329.e7. doi: 10.1016/j.chom.2017.07.017
Mindrinos, M., Katagiri, F., Yu, G. L., and Ausubel, F. M. (1994). The A. thaliana disease resistance gene RPS2 encodes a protein containing a nucleotide-binding site and leucine-rich repeats. Cell 78, 1089–1099. doi: 10.1016/0092-8674(94)90282-8
Moremen, K. W., Tiemeyer, M., and Nairn, A. V. (2012). Vertebrate protein glycosylation: diversity, synthesis and function. Nat. Rev. Mol. Cell. Biol. 13, 448–462. doi: 10.1038/nrm3383
Neyt, C., and Cornelis, G. R. (1999). Insertion of a Yop translocation pore into the macrophage plasma membrane by Yersinia enterocolitica: Requirement for translocators YopB and YopD, but not LcrG. Mol. Microbiol. 33, 971–981. doi: 10.1046/j.1365-2958.1999.01537.x
Niraula, P. M., Lawrence, K. S., and Klink, V. P. (2020). The heterologous expression of a soybean (Glycine max) xyloglucan endotransglycosylase/hydrolase (XTH) in cotton (Gossypium hirsutum) suppresses parasitism by the root knot nematode Meloidogyne incognita. PLoS One 15:e0235344. doi: 10.1371/journal.pone.0235344
Nishitani, K. (1998). Construction and restructuring of the cellulose-xyloglucan framework in the apoplast as mediated by the xyloglucan related protein family: a hypothetical scheme. J. Plant Res. 111, 159–166. doi: 10.1007/BF02507162
Nishitani, K., and Tominaga, R. (1992). Endo-xyloglucan transferase, a novel class of glycosyltransferase that catalyzes transfer of a segment of xyloglucan molecule to another xyloglucan molecule. J. Biol. Chem. 267, 21058–21064.
Novick, P., Ferro, S., and Schekman, R. (1981). Order of events in the yeast secretory pathway. Cell 25, 461–469. doi: 10.1016/0092-8674(81)90064-7
Novick, P., Field, C., and Schekman, R. (1980). Identification of 23 complementation groups required for post-translational events in the yeast secretory pathway. Cell 21, 205–215. doi: 10.1016/0092-8674(80)90128-2
Oda, Y., Iida, Y., Nagashima, Y., Sugiyama, Y., and Fukuda, H. (2015). Novel coiled-coil proteins regulate exocyst association with cortical microtubules in xylem cells via the conserved oligomeric golgi-complex 2 protein. Plant Cell Physiol. 56, 277–286. doi: 10.1093/pcp/pcu197
O’Neill, E. M., Mucyn, T. S., Patteson, J. B., Finkel, O. M., Chung, E. H., Baccile, J. A., et al. (2018). Phevamine A, a small molecule that suppresses plant immune responses. Proc. Natl. Acad. Sci. U.S.A. 115, E9514–E9522. doi: 10.1073/pnas.1803779115
Ostertag, M., Stammler, J., Douchkov, D., Eichmann, R., and Hückelhoven, R. (2013). The conserved oligomeric Golgi complex is involved in penetration resistance of barley to the barley powdery mildew fungus. Mol. Plant Pathol. 14, 230–240. doi: 10.1111/j.1364-3703.2012.00846.x
Pant, S. R., Matsye, P. D., McNeece, B. T., Sharma, K., Krishnavajhala, A., Lawrence, G. W., et al. (2014). Syntaxin 31 functions in Glycine max resistance to the plant parasitic nematode Heterodera glycines. Plant Mol. Biol. 85, 107–121. doi: 10.1007/s11103-014-0172-2
Pellegrini, L. L., O’Connor, V., Lottspeich, F., and Betz, H. (1995). Clostridial neurotoxins compromise the stability of a low energy SNARE complex mediating NSF activation of synaptic vesicle fusion. EMBO J. 14, 4705–4713. doi: 10.1002/j.1460-2075.1995.tb00152.x
Pokrovskaya, I. D., Willett, R., Smith, R. D., Morelle, W., Kudlyk, T., and Lupashin, V. V. (2011). Conserved oligomeric Golgi complex specifically regulates the maintenance of Golgi glycosylation machinery. Glycobiology 21, 1554–1569. doi: 10.1093/glycob/cwr028
Ram, R. J., Li, B., and Kaiser, C. A. (2002). Identification of Sec36p, Sec37p and Sec38p: components of the yeast complex that contains Sec34p and Sec35p. Mol. Biol. Cell 13, 1484–1500. doi: 10.1091/mbc.01-10-0495
Riggs, R. D., and Schmitt, D. P. (1988). Complete characterization of the race scheme for Heterodera glycines. J. Nematol. 20, 392–395.
Ross, J. P. (1958). Host-Parasite relationship of the soybean cyst nematode in resistant soybean roots. Phytopathology 48, 578–579.
Rui, Q., Wang, J., Li, Y., Tan, X., and Bao, Y. (2020). Arabidopsis COG6 is essential for pollen tube growth and golgi structure maintenance. Biochem. Biophys. Res. Commun. 528, 447–452. doi: 10.1016/j.bbrc.2020.05.189
Sanderfoot, A. A., Farhah, F., Assaad, F. F., and Raikhel, N. V. (2000). The Arabidopsis genome. An abundance of soluble N-ethylmaleimide-sensitive factor adaptor protein receptors. Plant Physiol. 124, 1558–1569. doi: 10.1104/pp.124.4.1558
Schiavo, G., Benfenati, F., Poulain, B., Rossetto, O., de Laureto, P. P., DasGupta, B. R., et al. (1992a). Tetanus and botulinum-B neurotoxins block neurotransmitter release by proteolytic cleavage of synaptobrevin. Nature 359, 832–835. doi: 10.1038/359832a0
Schiavo, G., Poulain, B., Rossetto, O., Benfenati, F., Tauc, L., and Montecucco, C. (1992b). Tetanus toxin is a zinc protein and its inhibition of neurotransmitter release and protease activity depend on zinc. EMBO J. 11, 3577–3583. doi: 10.1002/j.1460-2075.1992.tb05441.x
Schiavo, G., Rossetto, O., and Montecucco, C. (1994). Clostridial neurotoxins as tools to investigate the molecular events of neurotransmitter release. Semin. Cell Biol. 5, 221–229. doi: 10.1006/scel.1994.1028
Schmutz, J., Cannon, S. B., Schlueter, J., Ma, J., Mitros, T., Nelson, W., et al. (2010). Genome sequence of the palaeopolyploid soybean. Nature 463, 178–183. doi: 10.1038/nature08670
Sharma, K., Niraula, P. M., Troell, H. A., Adhikari, M., Alshehri, H. A., Alkharouf, N. W., et al. (2020). Exocyst components promote an incompatible interaction between Glycine max (soybean) and Heterodera glycines (the soybean cyst nematode). Sci Rep. 10:15003. doi: 10.1038/s41598-020-72126-z
Sharma, K., Pant, S. R., McNeece, B. T., Nirula, P. M., Burson, H. E., Lawrence, G. W., et al. (2016). Co-regulation of the Glycine max soluble N-ethylmaleimide-sensitive fusion protein attachment protein receptor (SNARE)-containing regulon occurs during defense to a root pathogen. J. Plant Interact. 11, 74–93. doi: 10.1080/17429145.2016.1195891
Shestakova, A., Suvorova, E., Pavliv, O., Khaidakova, G., and Lupashin, V. (2007). Interaction of the conserved oligomeric Golgi complex with t-SNARE Syntaxin5a/Sed5 enhances intra-Golgi SNARE complex stability. J. Cell Biol. 179, 1179–1192. doi: 10.1083/jcb.200705145
Sohda, M., Misumi, Y., Yamamoto, A., Nakamura, N., Ogata, S., Sakisaka, S., et al. (2010). Interaction of Golgin-84 with the COG complex mediates the intra-Golgi retrograde transport. Traffic 11, 1552–1566. doi: 10.1111/j.1600-0854.2010.01123.x
Söllner, T., Bennett, M. K., Whiteheart, S. W., Scheller, R. H., and Rothman, J. E. (1993a). A protein assembly-disassembly pathway in vitro that may correspond to sequential steps of synaptic vesicle docking, activation, and fusion. Cell 75, 409–418. doi: 10.1016/0092-8674(93)90376-2
Söllner, T., Whiteheart, S. W., Brunner, M., Erdjument-Bromage, H., Geromanos, S., Tempst, P., et al. (1993b). SNAP receptors implicated in vesicle targeting and fusion. Nature 362, 318–324. doi: 10.1038/362318a0
Song, W., Mentink, R. A., Henquet, M. G., Cordewener, J. H., van Dijk, A. D., Bosch, D., et al. (2013). N-Glycan occupancy of Arabidopsis N-glycoproteins. J. Proteomics. 93, 343–355. doi: 10.1016/j.jprot.2013.07.032
Strasser, R. (2016). Plant protein glycosylation. Glycobiology 26, 926–939. doi: 10.1093/glycob/cww023
Strotmeier, J., Willjes, G., Binz, T., and Rummel, A. (2012). Human synaptotagmin-II is not a high affinity receptor for botulinum neurotoxin B and G: increased therapeutic dosage and immunogenicity. FEBS Lett. 586, 310–313. doi: 10.1016/j.febslet.2011.12.037
Suvorova, E. S., Duden, R., and Lupashin, V. V. (2002). The Sec34/Sec35p complex, a Ypt1p effector required for retrograde intra-Golgi trafficking, interacts with Golgi SNAREs and COPI vesicle coat proteins. J. Cell Biol 157, 631–643. doi: 10.1083/jcb.200111081
Tan, X., Cao, K., Liu, F., Li, Y., Li, P., Gao, C., et al. (2016). Arabidopsis COG Complex subunits COG3 and COG8 modulate golgi morphology, vesicle trafficking homeostasis and are essential for pollen tube growth. PLoS Genet 12:e1006140. doi: 10.1371/journal.pgen.1006140
Tepfer, D. (1984). Transformation of several species of higher plants by Agrobacterium rhizogenes: sexual transmission of the transformed genotype and phenotype. Cell 37, 959–967. doi: 10.1016/0092-8674(84)90430-6
TerBush, D. R., Maurice, T., Roth, D., and Novick, P. (1996). The exocyst is a multiprotein complex required for exocytosis in Saccharomyces cerevisiae. EMBO 15, 6483–6494. doi: 10.1002/j.1460-2075.1996.tb01039.x
Ungar, D., Oka, T., Brittle, E. E., Vasile, E., Lupashin, V. V., Chatterton, J. E., et al. (2002). Characterization of a mammalian Golgi-localized protein complex, COG, that is required for normal Golgi morphology and function. J. Cell Biol. 157, 405–415. doi: 10.1083/jcb.200202016
Ungar, D., Oka, T., Vasile, E., Krieger, M., and Hughson, F. M. (2005). Subunit architecture of the conserved oligomeric Golgi complex. J. Biol. Chem. 280, 32729–32735. doi: 10.1074/jbc.M504590200
van der Biezen, E. A., and Jones, J. D. (1998). The NB-ARC domain: a novel signalling motif shared by plant resistance gene products and regulators of cell death in animals. Curr. Biol. 8, R226–R227. doi: 10.1016/S0960-9822(98)70145-9
VanRheenen, S. M., Cao, X., Lupashin, V. V., Barlowe, C., and Waters, M. G. (1998). Sec35p, a novel peripheral membrane protein, is required for ER to Golgi vesicle docking. J. Cell Biol. 141, 1107–1119. doi: 10.1083/jcb.141.5.1107
Vukašinović, N., Oda, Y., Pejchar, P., Synek, L., Peèenková, T., Rawat, A., et al. (2017). Microtubule-dependent targeting of the exocyst complex is necessary for xylem development in Arabidopsis. New Phytol. 213, 1052–1067. doi: 10.1111/nph.14267
Vukašinović, N., and Žárský, V. (2016). Tethering complexes in the Arabidopsis endomembrane system. Front. Cell. Dev. Biol. 4:46. doi: 10.3389/fcell.2016.00046
Wang, J., Niblack, T. L., Tremain, J. A., Wiebold, W. J., Tylka, G. L., Marett, C. C., et al. (2003). The soybean cyst nematode reduces soybean yield without causing obvious symptoms. Plant Dis. 87, 623–628. doi: 10.1094/PDIS.2003.87.6.623
Wei, Z. M., and Beer, S. V. (1993). HrpI of Erwinia amylovora functions in secretion of harpin and is a member of a new protein family. J. Bacteriol. 175, 7958–7967. doi: 10.1128/JB.175.24.7958-7967.1993
Wei, Z. M., Laby, R. J., Zumoff, C. H., Bauer, D. W., He, S. Y., Collmer, A., et al. (1992). Harpin, elicitor of the hypersensitive response produced by the plant pathogen Erwinia amylovora. Science 257, 85–88. doi: 10.1126/science.1621099
Whyte, J. R. C., and Munro, S. (2001). The SeC34/35 golgi transport complex is related to the exocyst, defining a family of complexes involved in multiple steps of membrane traffic. Dev. Cell 1, 527–537. doi: 10.1016/S1534-5807(01)00063-6
Willett, R., Ungar, D., and Lupashin, V. (2013). The Golgi puppet master: COG complex at center stage of membrane trafficking interactions. Histochem. Cell. Biol. 140, 271–283. doi: 10.1007/s00418-013-1117-6
Wrather, J. A., and Koenning, S. R. (2006). Estimates of disease effects on soybean yields in the United States 2003-2005. J. Nematol. 38, 173–180.
Wrather, J. A., Steinstra, W. C., and Koenning, S. R. (2001). Soybean disease loss estimates for the United States from 1996-1998. Can. J. Plant Pathol. 23, 122–131. doi: 10.1080/07060660109506919
Wuestehube, L. J., Duden, R., Eun, A., Hamamoto, S., Korn, P., Ram, R., et al. (1996). New mutants of Saccharomyces cerevisiae affected in the transport of proteins from the endoplasmic reticulum to the Golgi complex. Genetics 142, 393–406.
Yuan, J. S., Reed, A., Chen, F., and Stewart, C. N. (2006). Statistical analysis of real-time PCR data. BMC Bioinformatics 7:85. doi: 10.1186/1471-2105-7-85
Zhao, W., and Colley, K. J. (2008). “Nucleotide sugar transporters of the Golgi apparatus,” in The Golgi Apparatus, eds A. A. Mironov and M. Pavelka (Vienna: Springer). doi: 10.1007/978-3-211-76310-0_13
Zick, M., Orra, A., Schwartz, M. L., Merz, A. J., and Wickner, W. T. (2015). Sec17 can trigger fusion of trans-SNARE paired membranes without Sec18. Proc. Natl. Acad. Sci. U.S.A 112, E2290–E2297. doi: 10.1073/pnas.1506409112
Zielinska, D. F., Gnad, F., Schropp, K., Wiśniewski, J. R., and Mann, M. (2012). Mapping N-glycosylation sites across seven evolutionarily distant species reveals a divergent substrate proteome despite a common core machinery. Mol. Cell 46, 542–548. doi: 10.1016/j.molcel.2012.04.031
Keywords: conserved oligomeric Golgi (COG) complex, soybean, nematode, disease resistance, harpin, elicitor, effector triggered immunity (ETI)
Citation: Lawaju BR, Niraula P, Lawrence GW, Lawrence KS and Klink VP (2020) The Glycine max Conserved Oligomeric Golgi (COG) Complex Functions During a Defense Response to Heterodera glycines. Front. Plant Sci. 11:564495. doi: 10.3389/fpls.2020.564495
Received: 21 May 2020; Accepted: 02 October 2020;
Published: 11 November 2020.
Edited by:
Viktor Zarsky, Charles University, CzechiaReviewed by:
Fatima Cvrckova, Charles University, CzechiaCaiji Gao, South China Normal University, China
Copyright © 2020 Lawaju, Niraula, Lawrence, Lawrence and Klink. This is an open-access article distributed under the terms of the Creative Commons Attribution License (CC BY). The use, distribution or reproduction in other forums is permitted, provided the original author(s) and the copyright owner(s) are credited and that the original publication in this journal is cited, in accordance with accepted academic practice. No use, distribution or reproduction is permitted which does not comply with these terms.
*Correspondence: Vincent P. Klink, dmtsaW5rQGJpb2xvZ3kubXNzdGF0ZS5lZHU=; aGVhcnR3b29kMjdAaG90bWFpbC5jb20=
†Present address: Bisho Ram Lawaju, Department of Entomology and Plant Pathology, Auburn University, Auburn, AL, United States; Prakash Niraula, Department of Plant Pathology and Microbiology, Texas A&M AgriLife Research & Extension Center, Texas A&M University, Weslaco, TX, United States