- 1Department of Soil and Crop Sciences, Colorado State University, Fort Collins, CO, United States
- 2Department of Crop and Soil Science, Oregon State University, Corvallis, OR, United States
- 3Department of Agronomy and Horticulture, University of Nebraska-Lincoln, Lincoln, NE, United States
- 4Limagrain Europe, Clermont-Ferrand, France
- 5Field Crop Development Centre, Alberta Agriculture and Forestry, Lacombe, AB, Canada
- 6Consejo Superior de Investigaciones Científicas (CSIC), Aula Dei Experimental Station, Zaragoza, Spain
- 7Bayer – Crop Science, Woodland, CA, United States
- 8Saatzucht Ackermann GmbH & Co. KG, Irlbach, Germany
- 9Secobra Recherches, Centre de Bois Henry, Maule, France
- 10Bavarian State Research Center for Agriculture, Institute for Crop Science, Freising, Germany
- 11United States Department of Agriculture-Agricultural Research Service (USDA-ARS), Aberdeen, ID, United States
- 12Department of Molecular Breeding, Center for Agricultural Research, Martonvásár, Hungary
- 13Division of Field Crops and Horticulture Research Tohoku Agricultural Research Center National Agriculture and Food Research Organization (NARO), Morioka, Japan
- 14Institute of Plant Science and Resources, Okayama University, Kurashiki, Japan
- 15Department of Agronomy and Plant Genetics, University of Minnesota, St. Paul, MN, United States
- 16Department of Horticulture and Crop Science, The Ohio State University/Ohio Agricultural Research and Development Center (OARDC), Wooster, OH, United States
- 17The James Hutton Institute (JHI), Invergowrie, United Kingdom
One option to achieving greater resiliency for barley production in the face of climate change is to explore the potential of winter and facultative growth habits: for both types, low temperature tolerance (LTT) and vernalization sensitivity are key traits. Sensitivity to short-day photoperiod is a desirable attribute for facultative types. In order to broaden our understanding of the genetics of these phenotypes, we mapped quantitative trait loci (QTLs) and identified candidate genes using a genome-wide association studies (GWAS) panel composed of 882 barley accessions that was genotyped with the Illumina 9K single-nucleotide polymorphism (SNP) chip. Fifteen loci including 5 known and 10 novel QTL/genes were identified for LTT—assessed as winter survival in 10 field tests and mapped using a GWAS meta-analysis. FR-H1, FR-H2, and FR-H3 were major drivers of LTT, and candidate genes were identified for FR-H3. The principal determinants of vernalization sensitivity were VRN-H1, VRN-H2, and PPD-H1. VRN-H2 deletions conferred insensitive or intermediate sensitivity to vernalization. A subset of accessions with maximum LTT were identified as a resource for allele mining and further characterization. Facultative types comprised a small portion of the GWAS panel but may be useful for developing germplasm with this growth habit.
Introduction
To meet the challenges of climate change, there has been increasing interest in the agronomic potential of fall-sown barley in northern latitudes (Fisk et al., 2013; Cuesta-Marcos et al., 2015). Although fall-sown barley in regions with winter precipitation patterns can have significant yield advantages compared with spring-sown barley, there is a heightened risk of low temperature-induced crop injury. This risk is problematic at two stages of development: at the vegetative stage, which is the focus of this research, and at flowering. For recent research on low temperature injury at flowering in cereals, please see Trevaskis et al. (2007); Chen et al. (2009), and Powell et al. (2012). Low temperature tolerance (LTT) at the vegetative stage is an inducible trait that involves a complex gene regulon (reviewed by Cuesta-Marcos et al., 2015), and it is a key component of the mega-phenotype known as “winter-hardiness.” Recognizing that a range of factors can determine winter-hardiness, in this report, we will focus on LTT, as measured by winter survival (WS). In this report, we will use LTT and WS synonymously. Additional traits that may contribute to winter-hardiness are sensitivity to vernalization (VRN) (Hayes et al., 1993) and sensitivity to short-day photoperiod (sd-PPD) (Fowler et al., 2001). The sd-PPD phenotype is defined as the case where the recessive “sensitive” (null) allele confers slow or no growth under short-day conditions and the “insensitive” (dominant) allele confers normal, or near-normal, growth rates under short-day conditions. It is difficult to measure VRN sensitivity under field conditions. This trait can be approximated by measuring the days to flowering (DTF) of unvernalized plants under controlled environment conditions and is the approach we follow in this report. Although barley and other cereals are considered long-day plants, there is phenotypic variation in barley for flowering time under short-day conditions; this variation is due to allelic variation at PPD-H2 (Karsai et al., 2008; Kikuchi et al., 2012). The functional allele allows for flowering under sd-PPD; the non-functional allele delays flowering under sd-PPD. We use “sensitivity” in this context to refer to the non-functional allele. Sensitivity to sd-PPD is a challenge to measure as a phenotype. Under field conditions, the flowering time of fall-planted cereals is determined by multiple genes responding to a range of environmental signals. Sensitivity to sd-PPD can be measured under controlled environment conditions, but the requirement that experimental materials be exposed only to short photoperiod for an extended time makes it challenging to assess the phenotypic responses of larger germplasm arrays. Therefore, potential sensitivity to sd-PPD is most easily assessed based on the allele-specific genotyping of PPD-H2 (Kikuchi et al., 2012). Understanding the genetics and physiology of LTT, VRN, and sd-PPD will, therefore, lead to more efficient breeding of fall-planted barley and utilization of the growth habit type “facultative.”
Facultative growth habit, as defined by von Zitzewitz et al. (2011), is defined as maximum LTT, sd-PPD sensitivity, and no VRN sensitivity. The theoretical advantage of facultative growth habit in the context of climate change and climate volatility is that it could give growers and processors maximum flexibility because the same variety could be planted in the fall or in the spring. If planted in the fall, the variety would be capable of achieving maximum LTT, and sd-PPD would ensure a timely vegetative to reproductive transition once day length reached a critical threshold. If planted in the spring, the inducible LTT regulon would not be triggered, and thus there would be no cost in reproductive fitness to the variety. Likewise, day length would be sufficiently long that sd-PPD would not be a factor. Genetic keys to the facultative growth habit scenario are the loss-of-function deletion of the VRN-H2 complex locus, or at least critical elements of it, and the presence of the winter allele in VRN-H1 with the intact, full intron 1 region (Karsai et al., 2005; von Zitzewitz et al., 2005; Rizza et al., 2016).
There is an extensive literature on the association of VRN sensitivity with LTT (reviewed by Cuesta-Marcos et al., 2015). VRN sensitivity will delay the vegetative to reproductive transition and is therefore a potential mechanism for ensuring maximum LTT. However, a problem with VRN sensitivity in the context of climate change is that it cannot be relied upon to delay the vegetative to reproductive transition: the VRN requirement can be met–and the vegetative to reproductive transition initiated–long before the risk of low temperature injury is past. In contrast, sd-PPD sensitivity should be a much better “insurance” against precocious transition to reproductive growth because climate change is not altering day length. There is no known cost to VRN sensitivity under fall-sown conditions, and there are reported yield advantages whose physiological basis is not clear (Casao et al., 2011a). However, if it can be demonstrated that there is no intrinsic penalty in terms of reproductive fitness, elimination of VRN sensitivity within an overall genome architecture of maximum LTT and sd-PPD sensitivity could be beneficial. Variety development could be streamlined, and commercial production simplified. The breeder could reduce breeding cycle time by bypassing the need for vernalization; the grower could plant the same variety whenever field and/or market conditions were optimum; seed companies could potentially reduce the number of cultivars they need to have available; and the end-user would have the assurance of similar quality/processing attributes, regardless of production season.
There is accumulating evidence that facultative growth habit, as defined, is feasible and viable. Bi-parental quantitative trait locus (QTL) mapping (Fisk et al., 2013) and genome-wide association studies (GWAS) (von Zitzewitz et al., 2011) confirm that maximum LTT is possible with the deletion of VRN-H2. Cuesta-Marcos et al. (2015) used near-isogenic lines and a suite of genomics tools to make the same point. A brief review of the key genes discovered in these studies provides essential perspective and framework for the research described in this report. The QTLs and genes (when known) related to LTT are: FR-H1 (HvBM5a) (Fu et al., 2005; von Zitzewitz et al., 2005; Dhillon et al., 2010), FR-H2 (a cluster of CBF transcription factors) (Skinner et al., 2005, 2006), and FR-H3 (Fisk et al., 2013). The VRN genes are VRN-H1 (HvBM5a) (Danyluk et al., 2003; Trevaskis et al., 2003; Yan et al., 2003; von Zitzewitz et al., 2005; Cockram et al., 2007; Dhillon et al., 2010), VRN-H2 (ZCCT-Ha,b,c) (Yan et al., 2004), and VRN-H3 (HvFT1) (Yan et al., 2006; Faure et al., 2007; Kikuchi et al., 2009). Sd-PPD is determined by PPD-H2 (Pan et al., 1994; Fowler et al., 2001; Faure et al., 2007; Cuesta-Marcos et al., 2008; Kikuchi et al., 2009; Casao et al., 2011b; Kikuchi et al., 2012). PPD-H1 (Karsai et al., 1999; Turner et al., 2005) determines flowering time under long-day conditions and is therefore not directly relevant to facultative growth habit but could be important for facultative varieties under spring-sown conditions. The cited studies were based on a relatively narrow sample of barley germplasm.
To further our understanding of LTT, VRN sensitivity, and facultative growth habit, we assembled a large (n = 882) panel of diverse barley germplasm and assessed it for WS under field conditions in a large number of field tests (24 locations over a 2-year period). The panel was also characterized for VRN sensitivity, measured as DTF, under controlled environment conditions. We conducted a GWAS meta-analysis based on Fisher’s test of combined probabilities to integrate WS data from multiple environments. This approach increases the power of detection and reduces false-positive associations (Evangelou and Ioannidis, 2013). A subset of lines were then re-genotyped for targeted alleles at known LTT, VRN, and PPD loci in order to test the predictive utility of the genetic model for facultative growth habit.
Materials and Methods
Plant Materials
An array of 882 barley accessions composed of cultivars, landraces, and advanced generation experimental lines contributed by 21 barley breeding and/or genetics programs from around the world was used to evaluate LTT (as WS) and VRN sensitivity (as DTF). Information on the accessions and programs contributing to germplasm is listed in Supplementary Table S1. With the goal of excluding spring accessions that would serve only to confirm the value of alleles at the known major LTT QTLs, a criterion for inclusion in the array was the expectation of some degree of LTT. The largest contributors to the panel were: (1) Oregon State University (OSU) and the University of Minnesota (UM), both United States via the Facwin-6 (Belcher et al., 2015), and (2) the James Hutton Institute (JHI) and the University of Dundee (Scotland, United Kingdom) via the Association Genetics of UK Elite Barley (AGOUEB) (Cockram et al., 2010) and Genomics-Assisted Exploration of Barley Diversity (ExBarDiv) projects (Tondelli et al., 2013).
Phenotyping
The array (or subsets thereof) was phenotyped for WS under field conditions at 13 locations in 2013–2014 [Canada, France, Germany (two sites), Hungary, Japan, Scotland, Spain, and the United States (five sites)] and 11 locations in 2014–2015 [Canada, France, Germany, Hungary, Scotland, Spain, and the United States (five sites)]. Details on the specific test sites are provided in Supplementary Table S2. Data from the locations that grew the full array were used for this report; data from the locations that grew the subsets of the array were not used. A Type II modified augmented design (Lin and Poushinsky, 1983, 1985) was used at each location. In this design, a single replicate of the accessions was distributed among blocks, each containing three replicated checks. The primary check was “Alba” (winter growth habit), and the secondary checks were “Maja” (facultative growth habit) and “Full Pint” (spring growth habit). Each entry was grown in a single row, 1-m long plot, and WS was assessed visually as the percentage of plants that survived the winter. This design allows entry values to be adjusted based on the primary and secondary checks. Based on the relative efficiency, there was no advantage to either primary or secondary adjustments: therefore, un-adjusted WS values were used for all subsequent analyses.
The array was phenotyped for VRN sensitivity, by measuring DTF, under greenhouse conditions in 2015 at OSU, Corvallis, Oregon (United States). A single unvernalized plant of each accession was grown in a six cell-pack, where each plant had a total soil volume of 85 cm3. The Alba, Maja, and Full Pint checks were each replicated 10 times. The greenhouse was maintained at 18 ± 2°C. Natural daylight was supplemented with high intensity lighting to ensure a photoperiod regime of 16 h light/24 h. DTF was recorded for each plant when the first inflorescence was 50% emerged from the boot. The experiment was terminated at 154 days after planting, and any accession that had not flowered was assigned a DTF value of 154. Twenty-one accessions were not included in the VRN phenotyping due to the lack of seed, and three accessions that were included did not germinate. These 24 accessions were not included in subsequent analyses of DTF data. Growth habit classification data (winter, facultative, spring), when available, were obtained for these accessions from USDA-GRIN1. Based on DTF, accessions were separated into three groups: those with vernalization sensitivity (DTF ≥ 130), those with vernalization insensitivity (DTF ≤ 73), and those with intermediate sensitivity (DTF ≥ 74 and ≤ 129). A DTF threshold value ≤ 73 for vernalization insensitive germplasm was based on “Dicktoo,” a well-characterized facultative barley (Karsai et al., 2005) and accession 06OR-20, as described in the “Results” section. Inflorescence type (2-row, 6-row) was recorded for each accession. The WS and DTF phenotype data for each accession are provided in Supplementary Table S1.
Genotyping
The 882 accessions were genotyped with the barley 9K iSelect array (Comadran et al., 2012). Genotyping of the AGOUEB and ExBarDiv germplasm was conducted at TraitGenetics GmbH in Gatersleben, Germany, whereas the remaining germplasm was genotyped at the USDA-ARS Small Grains Genotyping Center in Fargo, ND, United States. After combining all available data and filtering for data quality (>20% missing data) and minor allele frequency (MAF) <0.05, there were 5,725 single-nucleotide polymorphism (SNP) loci assayed on each of 882 accessions. Physical coordinates of iSelect SNPs on the barley reference genome (Mascher et al., 2017) were retrieved from BARLEX2 (Colmsee et al., 2015). Of the 5,275 SNPs, 4,875 have an assigned physical position on the reference genome. The complete genotype data are provided in Supplementary Table S3.
A KASP genotyping assay (LGC Genomics, Teddington, United Kingdom) targeting specific loci involved in growth habit and vernalization was performed in a selected subset of 93 accessions based on the first year of field WS data. The targeted loci were PPD-H1, PPD-H2, VRN-H1, and VRN-H2. Data are provided in Supplementary Table S4. After a second year of WS data were obtained, 23 of the original 93 accessions chosen in Year 1 were in the overall top 5% based on the average of the 10 environments, across 2 years, where there was differential WS. In this report, we focus on these 23 accessions (Table 3).
Data Analysis
Principal component analysis (PCA) and linkage disequilibrium (LD) analyses were conducted in TASSEL v.5 (Bradbury et al., 2007) using SNPs with MAF >0.05. LD was estimated as the correlation coefficient r2 between pairs of SNPs within each chromosome. The decay of LD over physical distance was investigated by plotting pair-wise r2 values and generating a locally weighted scatterplot smoothing (LOESS) curve. LD decay distance was determined when r2 fell to the critical threshold estimated from the 99th percentile r2 distribution for unlinked markers. For GWAS, the mixed-linear model (Zhang et al., 2010) implemented in TASSEL v.5 was used, with a principal component analysis (3 PCs) accounting for population structure in the dataset. For WS, GWAS was first performed on each individual environment. In order to statistically pool GWAS results from the different environments and to detect gene by environment interactions, a meta-analysis was performed (Manning et al., 2011; Kang, 2015). We used Fisher’s method (Fisher, 1925) for combining p-values across environments, as follows:
where pi is the p-value for the ith environment. Then, the new Fisher’s p-value was calculated using the formula
where is a chi-square variable with 2k degrees of freedom, and k is the number of environments being combined. A false discovery rate (FDR; Benjamini and Hochberg, 1995) threshold of 0.01 was used to identify significant associations. Genes within significant regions were retrieved from the barley reference genome (Mascher et al., 2017) available in BARLEX (see footnote 2).
To assess two locus interactions for VRN sensitivity and WS, a likelihood ratio test (LRT) was performed using a model that includes the two markers tested, their interactions, and population structure based on PCs and a model including only PCs (Cuesta-Marcos et al., 2010). The LRT for VRN sensitivity was based on SNPs with a q-value ≤0.1, based on association analysis. The significant SNPs from this analysis were then used to identify the interactions between loci associated with WS. A phylogenetic tree of the 5% accessions with maximum WS (using the average of 10 environments) was generated using the neighbor-joining clustering method implemented in TASSEL v.5.
Results
LTT and VRN Sensitivity
We used data from the 10 of the 24 environments where there was differential WS: Minnesota (MN) and Ohio (OH) (United States) and Alberta (AB) (Canada) in 2014 and 2015; 2014 Germany (DE); and 2015 Idaho (ID), Scotland (United Kingdom), and Spain (ES). In 13 environments, there was either 100% WS, no detectable difference in WS, or the experiment was compromised in some fashion. In one environment (2014 Nebraska), no entries survived. The frequency distribution of average WS was normal (Figure 1A) and revealed substantial phenotypic variation, with a low of 11% (Mishima 41), a high of 74% (PI87835), and an average of 38%. Considering the checks, the winter (Alba) and facultative (Maja) checks had similar winter survival levels: 46 and 47%, respectively. The spring check (Full Pint) had a WS value of 19%.
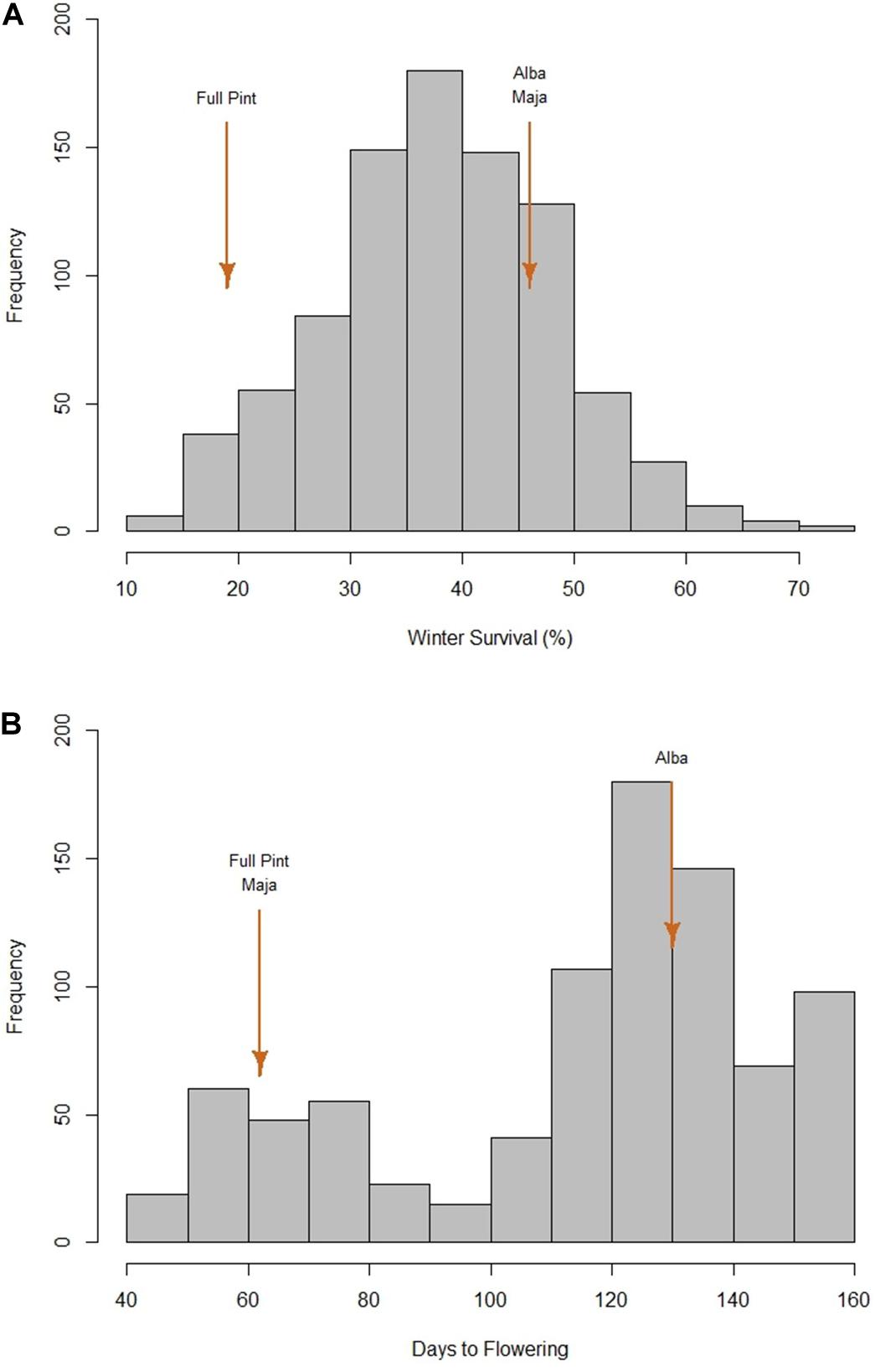
Figure 1. Phenotypic frequency distributions for 882 barley accessions. (A) Average winter survival across 10 locations. (B) Days to flowering under greenhouse conditions without vernalization. Full Pint, Maja, and Alba are the spring, facultative, and winter checks, respectively.
The frequency distribution of DTF, without vernalization, clearly separates two groups of accessions, with the dividing point at 95 DTF (Figure 1B). A DTF of 95 under controlled environment conditions, however, is not necessarily predictive of agronomically relevant DTF under field conditions (data not shown). Both Full Pint (the spring check) and Maja (the facultative check) had DTF values of 62, as compared with Alba, the winter check, at 130. In this research, we defined the 139 vernalization-insensitive (spring or potentially facultative) accessions as those with DTF values ≤ 73 based on Dicktoo (DTF = 72) and one accession (O6OR-20) with a DTF of 73. There were 386 accessions with intermediate vernalization sensitivity (≥ 74 and ≤ 129 DTF). The remaining 333 accessions where DTF data were obtained were classified as vernalization sensitive and are defined, for the purposes of this research, as having winter growth habit (Supplementary Table S1).
LTT and Its Relationship With Test Environments, VRN Sensitivity, and Inflorescence Type
The relationships between LTT, VRN sensitivity, and spike morphology were assessed using principal component analysis. The first four principal components accounted for 56.6% of the variation for WS. PC1 accounted for 22.1% of the variation, and PC2 accounted for 14.1% of the variation (Figure 2A). The 10 differential environments were clustered into three groups: 1) UK-15, ES-15, and DE-14, 2) OH-15, ID-15, AB-14, AB-15, and MN-14, and 3) OH-14 and MN-15, of which AB-14 and AB-15 were the most similar to each other, and UK-15 was the most unique. The overlay of WS values for the top and bottom 5% of accessions (Figure 2B) showed that accessions with the highest survival values (n = 44) were grouped with the Alba and Maja checks, whereas those with the lowest survival (n = 44) were grouped with the Full Pint check. The overlay of VRN sensitivity–classified as sensitive, intermediate, and insensitive–showed that most of the VRN-insensitive accessions grouped with Full Pint and the accessions with the lowest LTT, although some VRN-insensitive accessions grouped with the accessions with the highest winter survival. Accessions with intermediate VRN sensitivity, and VRN-sensitive accessions, are found throughput the PCA (Figure 2C). There was a relationship of spike morphology (2-row vs. 6-row) with LTT (Figure 2D): 84% of accessions within the top 5% for LTT were 6-rows. In the full array, there are 529 6-rows and 350 2-rows.
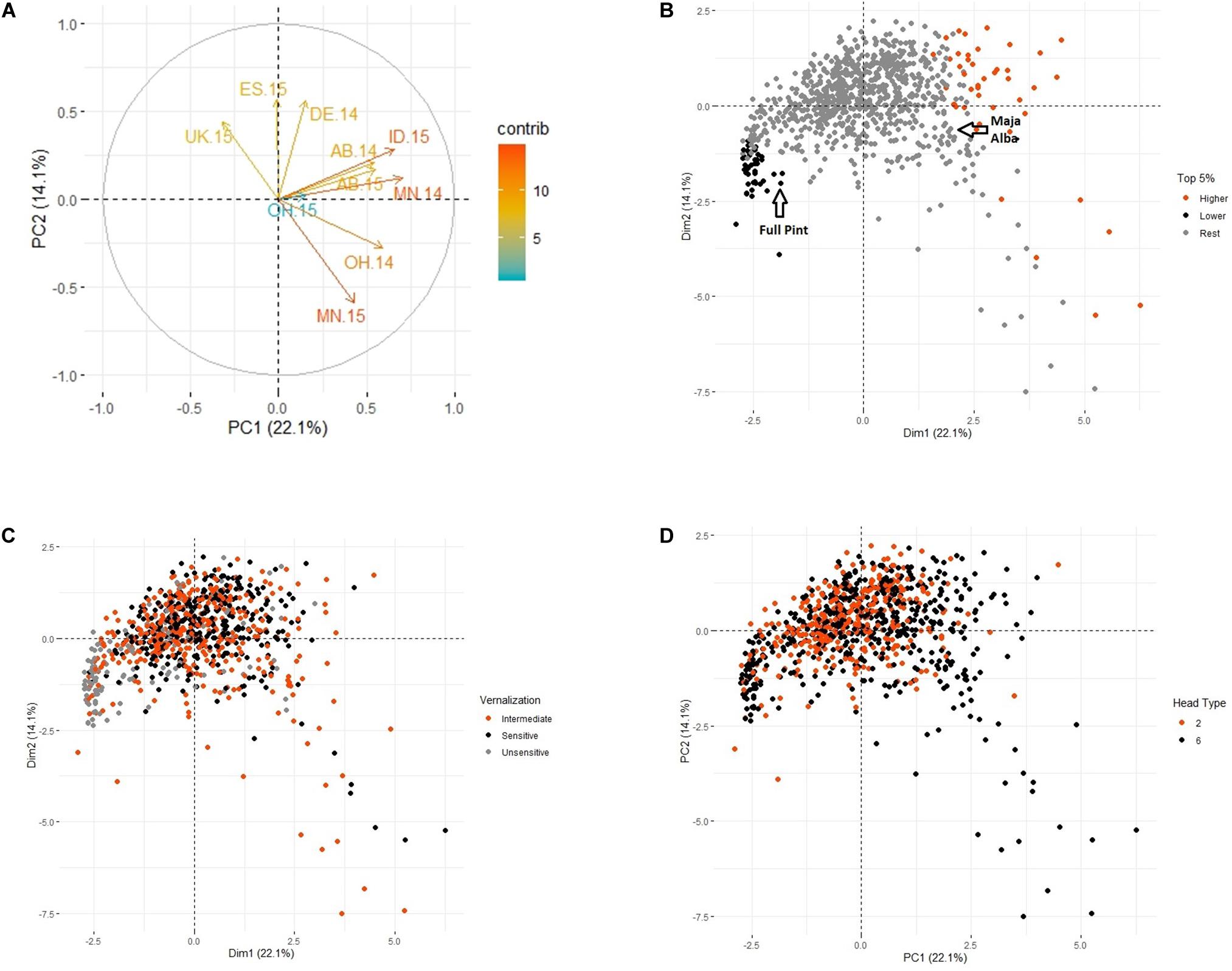
Figure 2. Principal component analysis of average winter survival across 10 locations. (A) Contribution of each location to variation across all sites (locations are defined in the text). (B) Overlay of the top 5%, bottom 5%, and rest of the accessions for winter survival. (C) Overlay of vernalization sensitivity (see text for definitions). (D) Overlay of head type (2-row, 6-row).
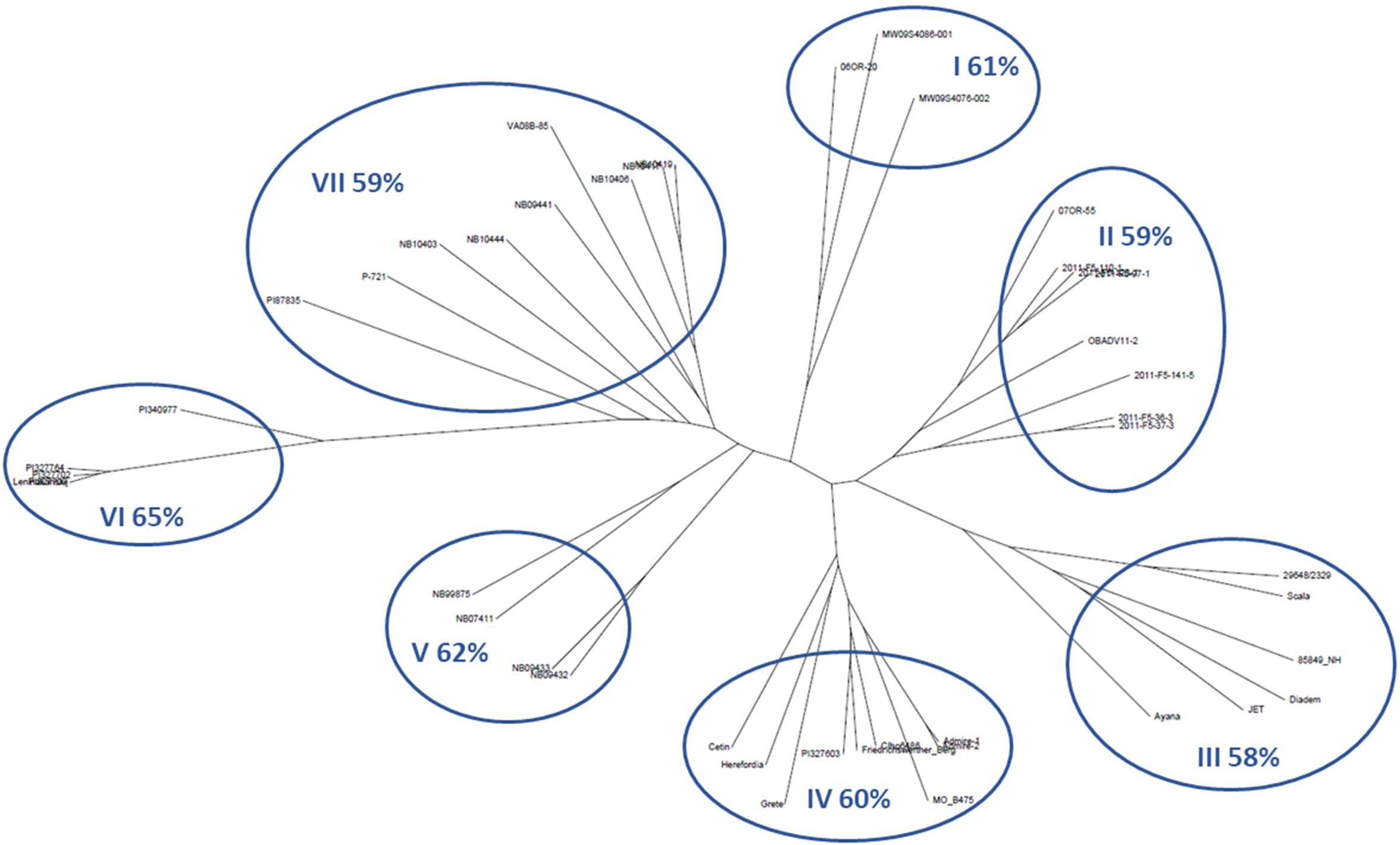
Figure 3. Diversity dendrogram based on SNP genotype data for the top 5% lines of barley accessions for average winter survival across 10 locations. Clades are numbered clockwise, starting with I. The% values are average winter survival for the clade. See Table 1 for more data on the accessions within each clade.
The relationships of LTT, VRN sensitivity, and spike morphology were explored in greater depth using the 5% of accessions with the highest WS across environments. The average WS for this group was 60% (Table 1 and Figure 3). Figure 3 shows an unrooted neighbor-joining dendrogram for these 44 accessions, based on 5,725 SNPs. Each of the seven clades is numbered from the top of the figure, proceeding clockwise, and the average survival of the accessions within each clade is given. The accessions in clade I are all VRN-insensitive 6-rows and trace to the UM or OSU programs. All entries in clade II are 6-rows from the OSU program and are VRN sensitive or are intermediate in their VRN sensitivity. Clade III is composed of 2-rows and 6-rows from European programs, all with winter habit. Clade IV is composed of 6-rows from the US GRIN germplasm collection, of European origin, and accessions contributed by the JHI. All are winters or have intermediate VRN sensitivity, except for Grete, which is VRN insensitive. No DTF data are available for two of the USDA accessions. Clade V is composed of 6-row winter types from the University of Nebraska, Lincoln (UNL) breeding program. Clade VI consists of 6-row germplasm accessions from the USDA collection, all tracing to Russia except for one accession from Armenia. The one accession for which DTF data are available is a winter. Clade VII consists of VRN-sensitive 6-rows from the UNL breeding program, a USDA accession tracing to North Korea, and one accession from the Virginia Polytechnical Institute and State University (VPI-SU) program.
GWAS of LTT and VRN Sensitivity
Prior to conducting GWAS, we assessed population structure and linkage disequilibrium in the LTT panel of 882 accessions. Structure was evaluated by performing PCA on 5,725 SNPs. As expected, there was strong population structure associated with spike morphology, with the first principal component of the PCA (PC1) mainly separating 2-row from 6-row barley accessions (Figure 4A). Accessions in the first two PCs were largely clustered based on their geographical origin, with PC1 mainly differentiating European from North American barleys, which largely correspond to 2-row and 6-row accessions, respectively, and PC2 separating Asian accessions from the rest (Figure 4B). On a genome-wide level, LD decayed within 24,300 kb (Supplementary Figure S1), although LD varied significantly across the genome, with centromeric and pericentromeric regions generally having higher LD (Supplementary Figure S2). Considering only polymorphic SNPs with a MAF >0.05, there is, on average, one SNP every 793 kb. However, the SNP density is also much higher in more distal, low-LD regions (Supplementary Figure S2).
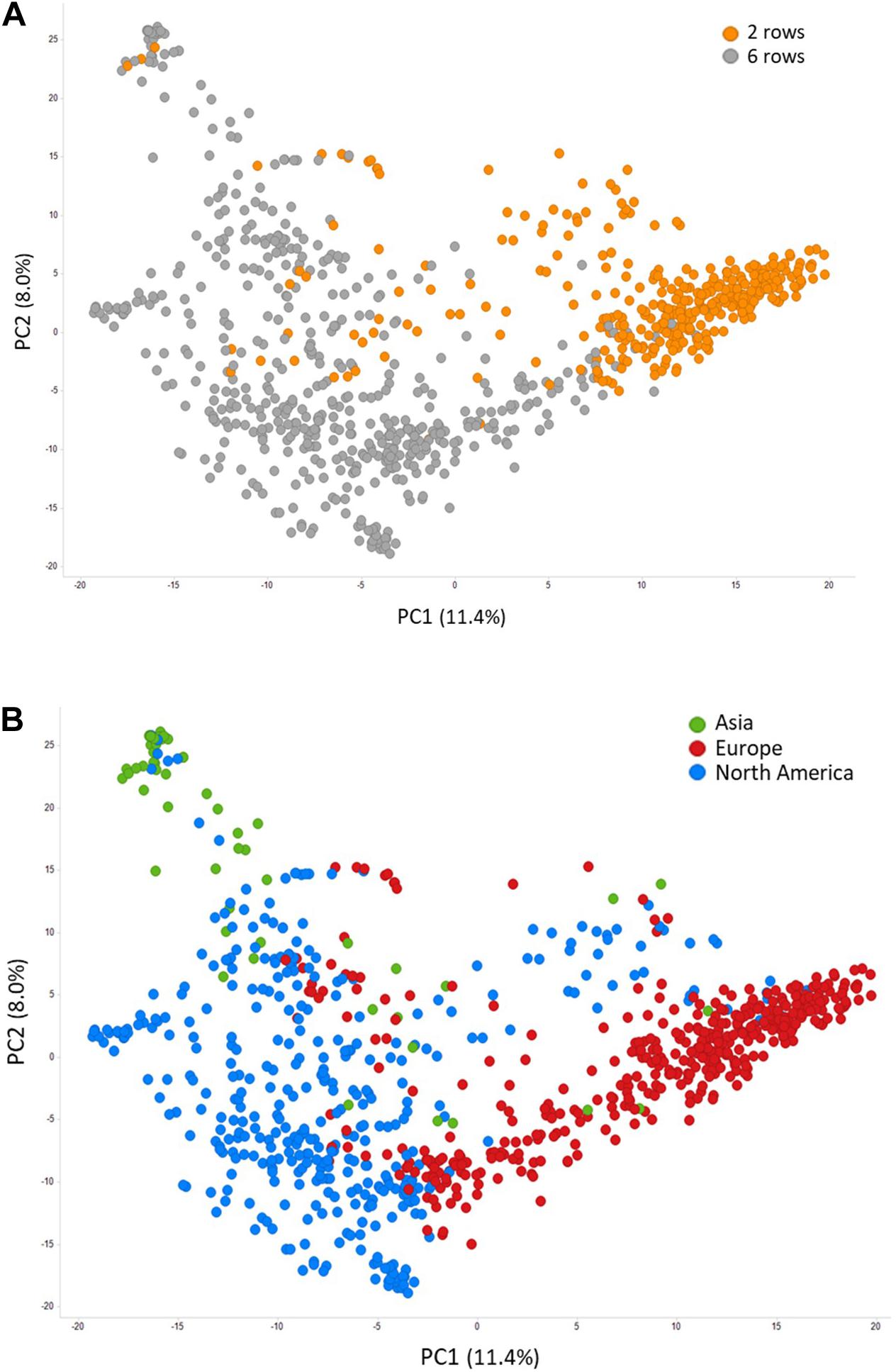
Figure 4. Principal component analysis of 882 barley accessions colored by head type (A) and accession origin (B).
GWAS of WS was first performed using data from each of the 10 individual environments, and results were then combined via meta-analysis (see section “Materials and Methods” and Supplementary Table S5). A summary of QTL effects across individual environments is shown in Supplementary Table S5. The meta-analysis revealed 15 significant loci on all barley chromosomes except 3H (Figure 5A and Table 2). Five of these loci correspond to previously reported QTLs/genes (FR-H3, PPD-H1, VRN-H2, FR-H2, and FR-H1/VRN-H1), whereas the other 11 are novel (Figure 5A and Table 2). The major determinants of WS were FR-H2 and FR-H1/VRN-H1 (Figure 5A and Table 2), which were also the loci detected in the highest number of individual environments (6/10 environments). Both loci were detected at a very high resolution, with the peak SNP for FR-H1/VRN-H1 (BOPA1_1501-353) located inside the causative MADS-box transcription factor gene (HORVU5Hr1G095630), and the highly significant SNPs on FR-H2 BOPA2_12_30845 and BOPA2_12_30852 (Supplementary Table S5) contained inside the gene cluster of C-repeat-binding factors (CBFs). VRN-H2 and the 2H locus with the highest significance level (BOPA2_12_21527; Table 2) were also identified in two individual environments (Supplementary Table S5), whereas another four loci (BOPA2_12_10938 on 1H, PPD-H1 on 2H, BOPA2_12_10278 on 6H, and BOPA2_12_30645 on 7H; Table 2) were identified in one individual environment (Supplementary Table S5). Each of these eight loci showed gene by environment interactions (Manning et al., 2011; Kang, 2015). The remaining loci, including FR-H3, were only identified in the meta-analysis. Three candidate genes for FR-H3 were identified: HORVU1Hr1G012690, which contains the peak SNP (SCRI_RS_114047) and encodes a tetraspanin family protein; HORVU1Hr1G012680, which encodes a protein belonging to UDP-glycosyltransferase superfamily; and HORVU1Hr1G012710, at 259 kb from the peak SNP, encoding the low temperature-induced protein lt101.2.
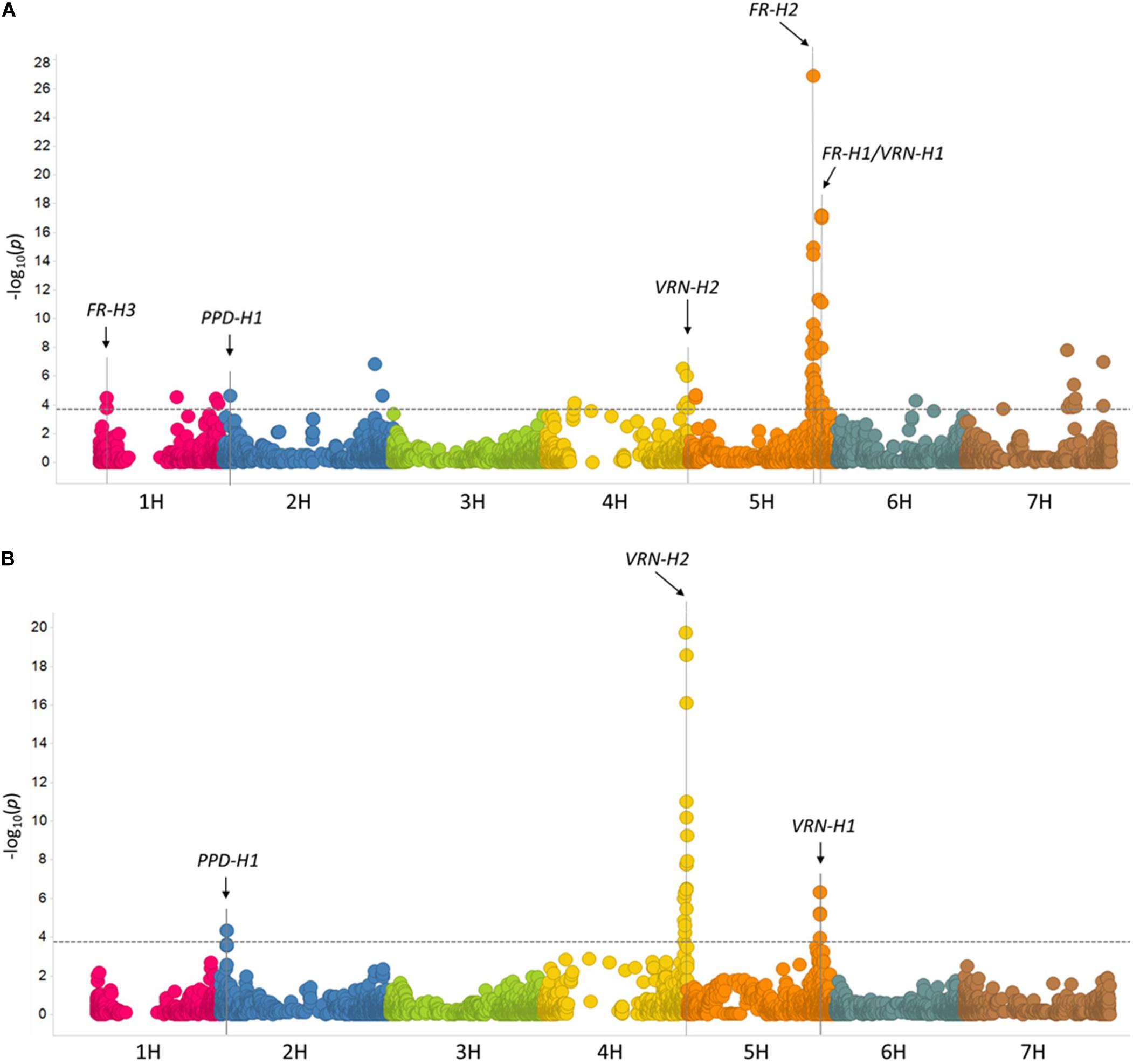
Figure 5. Manhattan plots from the GWAS of 882 accessions. (A) Meta-analysis of winter survival. (B) Days to flowering without vernalization. -log10 (p-values) are shown for 4,875 SNPs with physical coordinates in the barley reference genome (Mascher et al., 2017). The dashed lines indicate the 0.01 FDR-corrected threshold [3.70 for (A) and 3.75 for (B)].
Three loci were significantly associated with DTF: VRN-H2 on 4H, VRN-H1 on 5H, and PPD-H1 on 2H (Figure 5B, Table 2, and Supplementary Table S5). Peak SNPs on PPD-H1 (BOPA2_12_30871) and VRN-H1 (BOPA1_1501-353 and BOPA2_12_30930) are within the pseudo-response regulator encoded by HORVU2Hr1G013400 and the MADS box transcription factor encoded by HORVU5Hr1G095630, respectively. As expected, the zinc-finger/CCT domain transcription factor encoded by VRN-H2 could not be identified by significant SNPs on 4H since the reference genome “Morex” carries a VRN-H2 deletion.
Two Locus Interactions
For WS, interactions between VRN sensitivity and QTL/genes associated with LTT were assessed using data from the 6/10 environments where FR-H2 had a significant effect in the GWAS. Different two-locus combinations were significant at each of the six locations including interactions between VRN-H2/FR-H2, VRN-H1/FR-H2, and PPD-H1/FR-H2. There was no significant VRN-H1/VRN-H2 interaction in any environment. For VRN sensitivity, highly significant interactions were found between VRN-H2, VRN-H1, and PPD-H1 (Supplementary Table S6). The most significant interaction was between VRN-H1 and VRN-H2, and a large -log10 (p-value) was also found for the VRN-H2/PPD-H1 interaction.
Allele-Specific Genotyping for Growth Habit-Related Genes
The results of the allele-specific genotyping of PPD-H2, PPD-H1, VRN-H1, and VRN-H2 on accessions with the highest winter survival are shown in Table 3. At the PPD-H2 locus, three of the VRN-insensitive accessions have the allele conferring sensitivity to sd-PPD, where the plant exhibits delayed flowering under short days. One accession (MW09S4086-001) has the sd-PPD insensitive (functional) allele, where flowering occurs under short-day conditions. Of the remaining VRN intermediate and VRN-sensitive accessions, 11 have the short-day sensitive allele, and 7 have the short-day insensitive allele. A novel deletion at this locus was found in one accession (Leninakanskij). At the PPD-H1 locus, 22 accessions carry the dominant allele, which promotes flowering under long-day conditions (Turner et al., 2005); only Leninakanskij has the recessive allele, which delays flowering under long-day conditions. All 23 accessions have the “intact” VRN-H1 winter allele, which is reported to be associated with LTT (Rizza et al., 2016). At VRN-H2, two of the four VRN-insensitive accessions have a deletion of all three ZCCT-H genes. Two accessions, tracing to the UMN program, have partial deletions at this locus. Complete deletions were observed in two of the accessions with intermediate VRN sensitivity, both from the OSU program. Partial deletions of VRN-H2 were observed in four accessions, two in the intermediate group (MOB-475 and Cetin) and two in the winter growth habit group (Jet and Diadem).
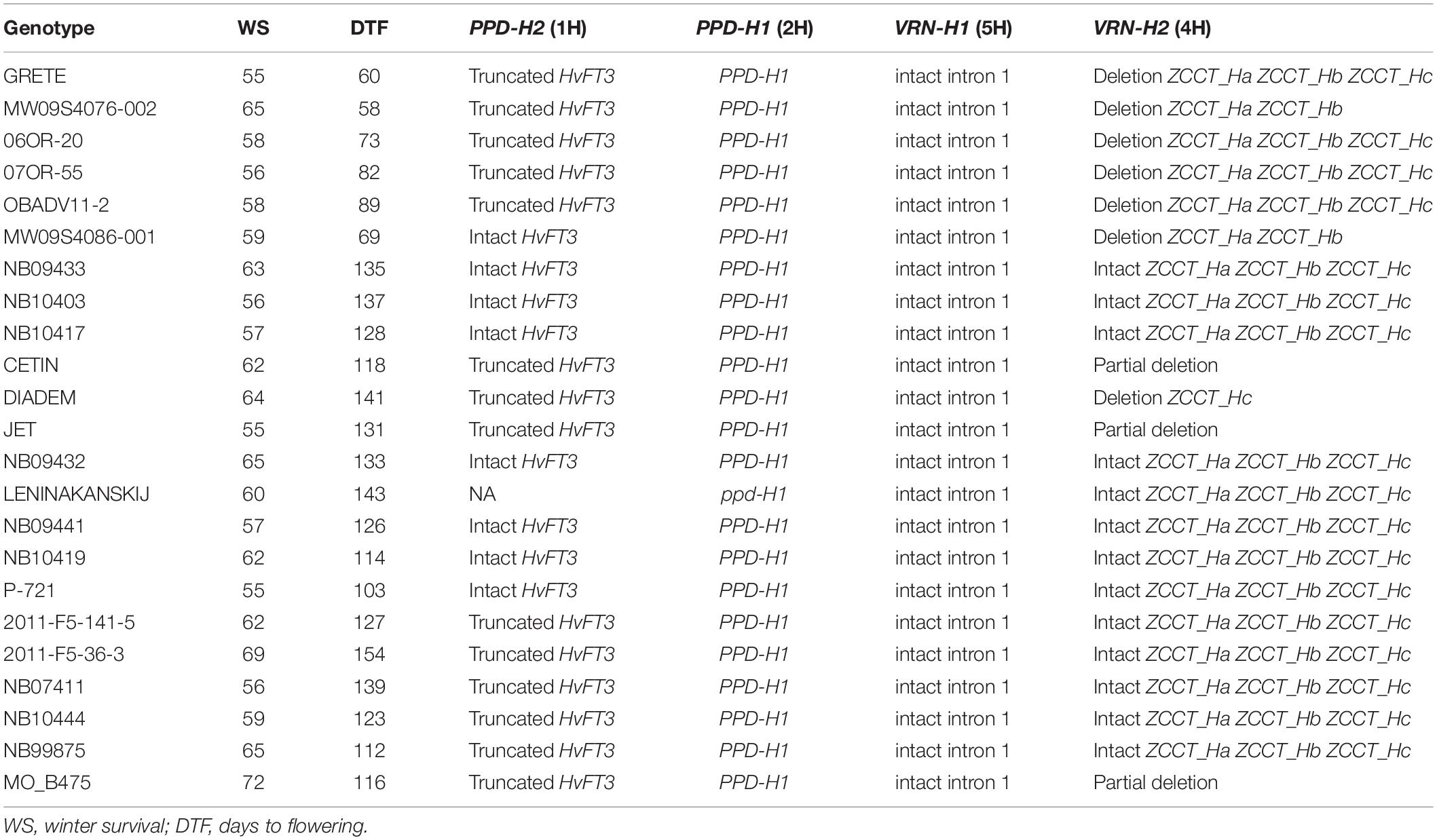
Table 3. Allele types at PPD-H1, PPD-H2, VRN-H1, and VRN-H2 for 23 barley accessions in the top 5% for winter survival across 10 locations.
Discussion
The Challenges of Phenotyping LTT and VRN Sensitivity
There are advantages and disadvantages to assessing LTT and VRN sensitivity under field and/or controlled environment conditions. Assessment of a GWAS panel for LTT is feasible only under field conditions, but naturally occurring low temperature events are rare, and variables besides low temperature can determine survival. In this study, fewer than half of the test sites provided useful data for GWAS of LTT, and yet every test site has, at some point in the past 30 years, generated useful data for mapping the genetic determinants of this phenotype. The implication is that a large number of test sites need to be sampled in order to ensure a reasonable chance of obtaining useful field data. Therefore, some future research could be directed at smaller subsets of informative germplasm, such as the top 5% identified in this panel. At each field site, perhaps selected based on the probability of generating representative differential survival (e.g., Figure 2A), assessment of WS using replicated large plots is recommended. For example, there was 100% mortality in the GWAS panel single rows at the Nebraska test site in 2014. The nine accessions that were also included in an adjacent replicated yield trial with four, 3 m row plots had WS values ranging from 40 to 81% (Baenziger, personal communication). Furthermore, we recommend that comprehensive weather data be obtained at each site. These multi-location data, coupled with genotype and phenotype data, will be useful in validating and enhancing climate models, such as the one recently reported by Byrns et al. (2020). Smaller subsets would also be amenable to controlled environment tests, where functional genomics approaches can complement GWAS (Fowler et al., 2001; Stockinger et al., 2007).
VRN sensitivity is straightforward to measure as DTF under controlled environment conditions, but the data are only an approximation of DTF under field conditions, where there are complex interactions involving changing photoperiod duration, diurnal fluctuations in temperature, and other environmental signals affecting plant growth and development. A deeper understanding of VRN sensitivity can be obtained under field conditions with repeated plantings from fall to spring (Igartua et al., 1999) and in controlled environment tests involving factorial combinations of time, temperature, and photoperiod duration (Casao et al., 2011a). These approaches are constrained by the number of genotypes that can be tested: therefore, as with LTT, we recommend that an informative subset of genotypes be used to empirically assess vernalization response within a framework of complete monitoring of environmental conditions.
Insights Into LTT and VRN Sensitivity From GWAS
The large panel of accessions used in this study (Table 2 and Figure 5A) showed that the most significant determinants of WS were FR-H1 and FR-H2, confirming previous work (reviewed by Cuesta-Marcos et al., 2015). Implications of this finding range from the trivial to the exciting. The large number of accessions with WS values within 20% of the spring check (Figure 1A) provides the trivial explanation: despite the stated criteria that all submissions to the panel have maximum LTT, additional phenotypic and genotypic pre-screening may have been advisable. For example, every accession in the top 5% for LTT has the complete intron in VRN-H1 (Table 3), suggesting that this haplotype could have been a useful criterion for inclusion of accessions in the GWAS panel. The exciting implication of the finding that FR-H1 and FR-H2 are significant sources of variation is that there is much more to be learned about allelic variation at these loci, using locus in the broadest sense of the term. For example, FR-H2 is a complex locus, where copy number variation (CNV) in CBF gene family members is a driver of differences in degree of LTT (Stockinger et al., 2007; Knox et al., 2010; Francia et al., 2016). The significant effect of this complex locus on LTT could, therefore, represent an example of the importance of CNV in barley, a topic addressed in general by Muñoz-Amatriaín et al. (2013), and with specific reference to FR-H2 by Muñoz-Amatriaín and Mascher (2018). In the case of FR-H1, there are additional genes in physical proximity with HvBM5A whose annotation makes them intriguing possible determinants of LTT (Cuesta-Marcos et al., 2015). Allelic variation at this “super gene” complex could, therefore, account for the continued importance of this QTL.
The discovery of candidate genes for FR-H3 in this GWAS panel is a key finding. While all three genes merit additional investigation, and two or more of them could be acting in concert as a complex locus, HORVU1Hr1G012710 is the most obvious candidate, based on annotation. This gene encodes barley lipid transfer protein 101 (blt101), which is known to be induced by low temperatures (Brown et al., 2001) and may be involved in the differential WS of barley genotypes by slowing growth under low temperatures and increasing tolerance to water stress (Choi and Hwang, 2015). Choi and Hwang (2015) also showed that transgenic wheat overexpressing blt101 had greater LTT than the wild type. HORVU1Hr1G012680 encodes a protein in the UDP-glycosyltransferase superfamily. Members of this family are reported to be involved in LTT via flavonoid glycosylation (Schulz et al., 2016; Zhao et al., 2019). HORVU1Hr1G012690, which contains the most significantly associated SNP (SCRI_RS_114047), encodes a tetraspanin family protein. Tetraspanins are small transmembrane proteins with important roles in plant development and responses to biotic and abiotic stresses, including low temperatures (reviewed by Reimann et al., 2017).
The significant effect of VRN-H2 on LTT in this study is likely due to the prevalence of winter types with VRN-H2 and high LTT and accessions lacking VRN-H2 with low LTT (Figure 2C). This argument for non-causative correlation of VRN-H2 with LTT is supported by the equal WS values for Alba (winter check) and Maja (facultative check) (Figure 1A) and the presence of the facultative accessions in the top 5% with VRN-H2 deletions (Table 3). The significant main effect of PPD-H1 on LTT requires additional research. Vegetative stage low temperature injury is expected under field conditions to occur during winter, when there are short photoperiod conditions. However, there are reports involving differential LTT in controlled environment studies using long photoperiods (Stockinger et al., 2007; Dhillon et al., 2010).
In addition to identifying FR-H1, FR-H2, FR-H3, VRN-H2, and PPD-H1, the GWAS meta-analysis of WS revealed additional novel loci that merit further investigation (Table 2 and Supplementary Table S5). Most of these loci were not significant in any of the individual environments, supporting the power of the meta-analysis, which is used in human medicine for the identification of genetic risk factors for complex diseases (Evangelou and Ioannidis, 2013). By combining the results from different GWAS to increase sample size, this approach increases statistical power and reduces false positives (Wang and Xu, 2019). No WS QTLs were detected using the meta-analysis, or in the GWAS of individual environment data, in the vicinity of the Vrs1 locus on chromosome 2H. This locus is the primary determinant of row type in barley (Komatsuda et al., 2007), and its lack of effect on LTT supports the hypothesis that the association of the 6-row phenotype and high LTT in this study (Figures 2D, 5A) is due to unintentional bias in choosing accessions for inclusion. At the time the study was designed, the largest contributors of germplasm were breeding programs focusing on 6-rows. Given the current predominance of 2-row barley for malting and brewing, future LTT research should focus on this row type. Furthermore, our data suggest that the WS of 6-row barley can be transferred to 2-row barley.
With a complex trait, such as LTT, interactions will likely be the rule rather than the exception. We found significant two-locus interactions for VRN-H1, VRN-H2, and PPD-H1 with FR-H2 and novel QTLs. The lack of significant interaction between VRN-H1 and VRN-H2 for WS at any test site is further evidence that specific combinations of alleles at these loci are not required for maximum LTT—an encouraging prospect for the potential of facultative growth habit. Understanding the interaction of PPD-H1 with FR-H2 will require further research. The results of these investigations could lead to the addition of PPD-H1 as a criterion for facultative growth habit.
The GWAS of DTF under greenhouse conditions (Figure 5B) identified the principal determinants of vernalization response reported in the literature: VRN-H1 and VRN-H2 (reviewed by Cuesta-Marcos et al., 2015). Furthermore, these loci showed the largest two-locus interaction we detected in this study, as expected based on the model originally proposed by Takahashi and Yasuda (1971) and subsequently validated by Yan et al. (2003) and Szũcs et al. (2007). Interestingly, VRN-H3 was not a determinant of DTF in this array: either because of monomorphism in the germplasm or the environmental conditions of the greenhouse assay. Loscos et al. (2014) characterized CNVs at this locus in barley germplasm and identified associations with DTF. Extending this research to the current germplasm, or subsets thereof, is warranted. The significance of PPD-H1 in VRN sensitivity (Figure 5B) may be attributable to the long-day photoperiod (ld-PPD, 16 h light/8 h dark) used in the controlled environment assay. We also observed a significant interaction of PPD-H1 and VRN-H2, as reported in other germplasm (Laurie et al., 1995; Karsai et al., 2005; Turner et al., 2013), providing additional evidence for the interconnectedness of the vernalization and photoperiod pathways under long-day conditions.
Additional Insights From the Top 5% for LTT
The top 5% (Tables 1, 3 and Figure 3) will be a resource for introgressing alleles contributing to maximum LTT into the current barley germplasm and for deeper analysis of the genetics and physiology of LTT. Most of these accessions are 6-rows, and most are feed barley cultivars or land race accessions. Therefore, targeted allele introgression into elite 2-row malting germplasm will be required. The prevalence of the sd-PPD sensitivity allele at PPD-H2 in the top 5% is notable and suggests a fitness advantage to this phenotype, in the absence of direct selection. We hypothesize that significant QTL effects for this locus on LTT were not detected in the current data set because mid-winter warm temperature events did not occur in the test environments. The sd-PPD sensitivity phenotype, therefore, can be viewed as an insurance mechanism. Validation of its value will require controlled environment experiments and “fortuitous” field trials when winter warm temperature events occur. The presence of the sd-PPD insensitive allele in accessions with winter growth habit may be attributable to the historical dependency on VRN sensitivity to delay the vegetative to reproductive transition and/or to its importance in winter barleys from regions with mild winters (Casao et al., 2011b)
The prevalence of the ld-PPD sensitivity allele at PPD-H1 in all of the top 5% could be due to selection for early maturity in fall-planted barley and not to a direct effect on LTT. The widespread occurrence of the ld-PPD insensitive allele in spring habit barley is credited with enhancing yield by extending the grain-filling period (Turner et al., 2005). Introgressing the ld-PPD insensitive into fall-planted germplasm could, therefore, be one path to increasing grain yield. There is evidence of selection for this allele in germplasm from Southern Europe (Casao et al., 2011b).
Prospects for Facultative Growth Habit
Climate change makes it imperative to ensure that crops are resilient in the face of volatility in temperature and precipitation. Facultative varieties offer one path forward for barley: the capacity to acclimate and achieve maximum LTT without vernalization sensitivity, coupled with the “insurance of sd-PPD sensitivity,” will allow for flexibility in planting date. There is precedent in the literature that facultative growth habit types are capable of achieving the same LTT as winter growth habit types (Fowler et al., 2001; von Zitzewitz et al., 2011; Fisk et al., 2013; Rizza et al., 2016). In the current research, the facultative check Maja had a WS value comparable to the winter check Alba. The current GWAS panel was assembled to study LTT irrespective of growth habit. Therefore, it is encouraging that facultative types were among the top 5% for LTT. Marker assisted selection for loss-of-function alleles at VRN-H2 at PPD-H2 now provides an efficient path to target facultative growth habit. Rigorous assessment of the prospects for this growth habit type will (1) require extensive assessment of larger numbers of diverse facultative accessions, compared with winter checks, in multiple environments and (2) be facilitated by the development and deep characterization of isogenic lines developed through traditional introgression or, potentially, through genome editing.
Data Availability Statement
The datasets generated for this study can be found in the online repositories. The names of the repository/repositories and accession number(s) can be found in the article/ Supplementary Material.
Author Contributions
AC-M, PH, DH, SF, and LH designed the experiment, provided seed to the cooperators, curated the data, and conducted the preliminary analyses. MM-A and JH had primary responsibility for data analysis. JH, MM-A, and PH prepared the drafts, edited the drafts, and ensured co-author access to the evolving manuscript. Co-authors PH, FC, CE, AG, MH, GH, EI, IK, TN, KeS, ES, and WT grew the experiment and generated the WS data, they and all the other co-authors participated in reviewing and editing. All authors read and approved the manuscript.
Funding
Support was provided by the USDA-NIFA TCAP Project no. 2011-68002-30029.
Conflict of Interest
AB was employed by the company Limagrain Europe, AC-M was employed by Bayer Crop Science, CE was employed by Saatzucht Ackermann GmbH & Co. KG, and AG was employed by the company Secobra Recherches.
The remaining authors declare that the research was conducted in the absence of any commercial or financial relationships that could be construed as a potential conflict of interest.
Acknowledgments
We thank Shiaoman Chao (retired) USDA-ARS Fargo, North Dakota for Illumina 9K genotyping and allele calling and Sassoum Lo (University of California Davis) for technical support in the GWAS meta-analysis. We are grateful for the contributions of germplasm by all co-authors and M. Gotz, V. Korzun, K. J. Muller, L. Cattivelli, I. Romagosa, and R. Sharma.
Supplementary Material
The Supplementary Material for this article can be found online at: https://www.frontiersin.org/articles/10.3389/fpls.2020.585927/full#supplementary-material
Footnotes
References
Belcher, A. R., Graebner, R. C., Cuesta-Marcos, A., Fisk, S., Filichkin, T., Smith, K. P., et al. (2015). Registration of the TCAP FAC-WIN6 barley panel for genomewide association studies. J. Plant Regist. 9, 411–418. doi: 10.3198/jpr2014.12.0083crmp
Benjamini, Y., and Hochberg, Y. (1995). Controlling the false discovery rate: a practical and powerful approach to multiple testing. J. R. Stat. Soc. Ser. B 57, 289–300. doi: 10.1111/j.2517-6161.1995.tb02031.x
Bradbury, P. J., Zhang, Z., Kroon, D. E., Casstevens, T. M., Ramdoss, Y., and Buckler, E. S. (2007). TASSEL: software for association mapping of complex traits in diverse samples. Bioinformatics 23, 2633–2635. doi: 10.1093/bioinformatics/btm308
Brown, A. P., Dunn, M. A., Goddard, N. J., and Hughes, M. A. (2001). Identification of a novel low-temperature-response element in the promoter of the barley (Hordeum vulgare L) gene blt101.1. Planta 213, 770–780. doi: 10.1007/s004250100549
Byrns, B. M., Greer, K. J., and Fowler, D. B. (2020). Modelling winter survival in cereals: an interactive tool. Crop. Sci. 60, 2408–2419. doi: 10.1002/csc2.20246
Casao, M. C., Igartua, E., Karsai, I., Lasa, J. M., Gracia, M. P., and Casas, A. M. (2011a). Expression analysis of vernalization and day-length response genes in barley (Hordeum vulgare L.) indicates that VRNH2 is a repressor of PPDH2 (HvFT3) under long days. J. Exp. Bot. 62, 1939–1949. doi: 10.1093/jxb/erq382
Casao, M. C., Karsai, I., Igartua, E., Gracia, M. P., Veisz, O., and Casas, A. M. (2011b). Adaptation of barley to mild winters: a role for PPDH2. BMC Plant Biol. 11:164. doi: 10.1186/1471-2229-11-164
Chen, A., Gusta, L. V., Brûlé-Babel, A., Leach, R., Baumann, U., Fincher, G. B., et al. (2009). Varietal and chromosome 2H locus-specific frost tolerance in reproductive tissues of barley (Hordeum vulgare L.) detected using a frost simulation chamber. Theor. Appl. Genet. 119, 685–694. doi: 10.1007/s00122-009-1079-1
Choi, C., and Hwang, C. H. (2015). The barley lipid transfer protein, BLT101, enhances cold tolerance in wheat under cold stress. Plant Biotechnol. Rep. 9, 197–207. doi: 10.1007/s11816-015-0357-4
Cockram, J., Jones, H., Leigh, F. J., O’Sullivan, D., Powell, W., Laurie, D. A., et al. (2007). Control of flowering time in temperate cereals: genes, domestication, and sustainable productivity. J. Exp. Bot. 58, 1231–1244. doi: 10.1093/jxb/erm042
Cockram, J., White, J., Zuluaga, D. L., Smith, D., Comadran, J., Macaulay, M., et al. (2010). Genome-wide association mapping to candidate polymorphism resolution in the unsequenced barley genome. Proc. Natl. Acad. Sci. U.S.A. 107:21611. doi: 10.1073/pnas.1010179107
Colmsee, C., Beier, S., Himmelbach, A., Schmutzer, T., Stein, N., Scholz, U., et al. (2015). BARLEX - the barley draft genome explorer. Mol. Plant 8, 964–966. doi: 10.1016/j.molp.2015.03.009
Comadran, J., Kilian, B., Russell, J., Ramsay, L., Stein, N., Ganal, M., et al. (2012). Natural variation in a homolog of Antirrhinum centroradialis contributed to spring growth habit and environmental adaptation in cultivated barley. Nat. Genet. 44:1388. doi: 10.1038/ng.2447
Cuesta-Marcos, A., Casas, A. M., Yahiaoui, S., Gracia, M. P., Lasa, J. M., and Igartua, E. (2008). Joint analysis for heading date QTL in small interconnected barley populations. Mol. Breed. 21, 383–399. doi: 10.1007/s11032-007-9139-1
Cuesta-Marcos, A., Muñoz-Amatriaín, M., Filichkin, T., Karsai, I., Trevaskis, B., Yasuda, S., et al. (2015). The relationships between development and low temperature tolerance in barley near isogenic lines differing for flowering behavior. Plant Cell Physiol. 56, 2312–2324. doi: 10.1093/pcp/pcv147
Cuesta-Marcos, A., Szûcs, P., Close, T. J., Filichkin, T., Muehlbauer, G. J., Smith, K. P., et al. (2010). Genome-wide SNPs and re-sequencing of growth habit and inflorescence genes in barley: implications for association mapping in germplasm arrays varying in size and structure. BMC Genomics 11:707. doi: 10.1186/1471-2164-11-707
Danyluk, J., Kane, N. A., Breton, G., Limin, A. E., Fowler, D. B., and Sarhan, F. (2003). TaVRT-1, a putative transcription factor associated with vegetative to reproductive transition in cereals. Plant Physiol. 132, 1849–1860. doi: 10.1104/pp.103.023523
Dhillon, T., Pearce, S. P., Stockinger, E. J., Distelfeld, A., Li, C., Knox, A. K., et al. (2010). Regulation of freezing tolerance and flowering in temperate cereals: the VRN-1 connection. Plant Physiol. 153, 1846–1858. doi: 10.1104/pp.110.159079
Evangelou, E., and Ioannidis, J. P. A. (2013). Meta-analysis methods for genome-wide association studies and beyond. Nat. Rev. Genet. 14, 379–389. doi: 10.1038/nrg3472
Faure, S., Higgins, J., Turner, A., and Laurie, D. A. (2007). The flowering locus T-like gene family in barley (Hordeum vulgare). Genetics 176, 599–609. doi: 10.1534/genetics.106.069500
Fisk, S. P., Cuesta-Marcos, A., Cistué, L., Russell, J., Smith, K. P., Baenziger, S., et al. (2013). FR-H3: a new QTL to assist in the development of fall-sown barley with superior low temperature tolerance. Theor. Appl. Genet. 126, 335–347. doi: 10.1007/s00122-012-1982-8
Fowler, D. B., Breton, G., Limin, A. E., Mahfoozi, S., and Sarhan, F. (2001). Photoperiod and temperature interactions regulate low-temperature-induced gene expression in barley. Plant Physiol. 127, 1676–1681. doi: 10.1104/pp.010483
Francia, E., Morcia, C., Pasquariello, M., Mazzamurro, V., Milc, J. A., Rizza, F., et al. (2016). Copy number variation at the HvCBF4-HvCBF2 genomic segment is a major component of frost resistance in barley. Plant Mol. Biol. 92, 161–175. doi: 10.1007/s11103-016-0505-4
Fu, D., Szucs, P., Yan, L., Helguera, M., Skinner, J. S., von Zitzewitz, J., et al. (2005). Large deletions within the first intron in VRN-1 are associated with spring growth habit in barley and wheat. Mol. Genet. Genomics 273, 54–65. doi: 10.1007/s00438-004-1095-4
Hayes, P. M., Blake, T., Chen, T. H., Tragoonrung, S., Chen, F., Pan, A., et al. (1993). Quantitative trait loci on barley (Hordeum vulgare L.) chromosome 7 associated with components of winterhardiness. Genome 36, 66–71. doi: 10.1139/g93-009
Igartua, E., Casas, A. M., Ciudad, F., Montoya, J. L., and Romagosa, I. (1999). RFLP markers associated with major genes controlling heading date evaluated in a barley germ plasm pool. Heredity 83, 551–559. doi: 10.1046/j.1365-2540.1999.00589.x
Kang, H. (2015). Statistical considerations in meta-analysis. Hanyang. Med. Rev. 35, 23–32. doi: 10.7599/hmr.2015.35.1.23
Karsai, I., Mészáros, K., Szücs, P., Hayes, P. M., Láng, L., and Bedö, Z. (1999). Effects of loci determining photoperiod sensitivity (Ppd-H1) and vernalization response (Sh2) on agronomic traits in the ‘Dicktoo’בMorex’ barley mapping population. Plant Breed. 118, 399–403. doi: 10.1046/j.1439-0523.1999.00408.x
Karsai, I., Szũcs, P., Köszegi, B., Hayes, P. M., Casas, A., Bedõ, Z., et al. (2008). Effects of photo and thermo cycles on flowering time in barley: a genetical phenomics approach. J. Exp. Bot. 59, 2707–2715. doi: 10.1093/jxb/ern131
Karsai, I., Szũcs, P., Mészáros, K., Filichkina, T., Hayes, P., Skinner, J., et al. (2005). The Vrn-H2 locus is a major determinant of flowering time in a facultative× winter growth habit barley (Hordeum vulgare L.) mapping population. Theor. Appl. Genet. 110, 1458–1466. doi: 10.1007/s00122-005-1979-7
Kikuchi, R., Kawahigashi, H., Ando, T., Tonooka, T., and Handa, H. (2009). Molecular and functional characterization of PEBP genes in barley reveal the diversification of their roles in flowering. Plant Physiol. 149:1341. doi: 10.1104/pp.108.132134
Kikuchi, R., Kawahigashi, H., Oshima, M., Ando, T., and Handa, H. (2012). The differential expression of HvCO9, a member of the CONSTANS-like gene family, contributes to the control of flowering under short-day conditions in barley. J. Exp. Bot. 63, 773–784. doi: 10.1093/jxb/err299
Knox, A. K., Dhillon, T., Cheng, H., Tondelli, A., Pecchioni, N., and Stockinger, E. J. (2010). CBF gene copy number variation at frost resistance-2 is associated with levels of freezing tolerance in temperate-climate cereals. Theor. Appl. Genet. 121, 21–35. doi: 10.1007/s00122-010-1288-7
Komatsuda, T., Pourkheirandish, M., He, C., Azhaguvel, P., Kanamori, H., Perovic, D., et al. (2007). Six-rowed barley originated from a mutation in a homeodomain-leucine zipper I-class homeobox gene. Proc. Natl. Acad. Sci. U.S.A. 104, 1424–1429. doi: 10.1073/pnas.0608580104
Laurie, D., Pratchett, N., Bezant, J., and Snape, J. (1995). RFLP mapping of five major genes and eight quantitative trait loci controlling flowering time in a winter x spring barley (Hordeum vulgare L.) cross. Genome 38, 575–585. doi: 10.1139/g95-074
Lin, C.-S., and Poushinsky, G. (1983). A modified augmented design for an early stage of plant selection involving a large number of test lines without replication. Biometrics 39, 553–561. doi: 10.2307/2531083
Lin, C.-S., and Poushinsky, G. (1985). A modified augmented design (type 2) for rectangular plots. Can. J. Plant Sci. 65, 743–749. doi: 10.4141/cjps85-094
Loscos, J., Igartua, E., Contreras-Moreira, B., Gracia, M. P., and Casas, A. M. (2014). HvFT1 polymorphism and effect-survey of barley germplasm and expression analysis. Front. Plant Sci. 5:251. doi: 10.3389/fpls.2014.00251
Manning, A. K., LaValley, M., Liu, C.-T., Rice, K., An, P., Liu, Y., et al. (2011). Meta-analysis of gene-environment interaction: joint estimation of SNP and SNP × environment regression coefficients. Genet. Epidemiol. 35, 11–18. doi: 10.1002/gepi.20546
Mascher, M., Gundlach, H., Himmelbach, A., Beier, S., Twardziok, S. O., Wicker, T., et al. (2017). A chromosome conformation capture ordered sequence of the barley genome. Nature 544, 427–433. doi: 10.1038/nature22043
Muñoz-Amatriaín, M., Eichten, S. R., Wicker, T., Richmond, T. A., Mascher, M., Steuernagel, B., et al. (2013). Distribution, functional impact, and origin mechanisms of copy number variation in the barley genome. Genome Biol. 14, R58. doi: 10.1186/gb-2013-14-6-r58
Muñoz-Amatriaín, M., and Mascher, M. (2018). “Sequence diversity and structural variation,” in The Barley Genome. Compendium of Plant Genomes, eds N. Stein and G. Muehlbauer (Cham: Springer), 109–122. doi: 10.1007/978-3-319-92528-8_8
Pan, A., Hayes, P. M., Chen, F., Chen, T. H. H., Blake, T., Wright, S., et al. (1994). Genetic analysis of the components of winterhardiness in barley (s L.). Theor. Appl. Genet. 89, 900–910. doi: 10.1007/BF00224516
Powell, N., Ji, X., Ravash, R., Edlington, J., and Dolferus, R. (2012). Yield stability for cereals in a changing climate. Funct. Plant Biol. 39, 539–552. doi: 10.1071/fp12078
Reimann, R., Kost, B., and Dettmer, J. (2017). TETRASPANINs in plants. Front. Plant Sci. 8:545. doi: 10.3389/fpls.2017.00545
Rizza, F., Karsai, I., Morcia, C., Badeck, F.-W., Terzi, V., Pagani, D., et al. (2016). Association between the allele compositions of major plant developmental genes and frost tolerance in barley (Hordeum vulgare L.) germplasm of different origin. Mol. Breed. 36:156. doi: 10.1007/s11032-016-0571-y
Schulz, E., Tohge, T., Zuther, E., Fernie, A. R., and Hincha, D. K. (2016). Flavonoids are determinants of freezing tolerance and cold acclimation in Arabidopsis thaliana. Sci. Rep. 6:34027. doi: 10.1038/srep34027
Skinner, J. S., Szucs, P., von Zitzewitz, J., Marquez-Cedillo, L., Filichkin, T., Stockinger, E. J., et al. (2006). Mapping of barley homologs to genes that regulate low temperature tolerance in Arabidopsis. Theor. Appl. Genet. 112, 832–842. doi: 10.1007/s00122-005-0185-y
Skinner, J. S., von Zitzewitz, J., Szucs, P., Marquez-Cedillo, L., Filichkin, T., Amundsen, K., et al. (2005). Structural, functional, and phylogenetic characterization of a large CBF gene family in barley. Plant Mol. Biol. 59, 533–551. doi: 10.1007/s11103-005-2498-2
Stockinger, E. J., Skinner, J. S., Gardner, K. G., Francia, E., and Pecchioni, N. (2007). Expression levels of barley Cbf genes at the Frost resistance-H2 locus are dependent upon alleles at Fr-H1 and Fr-H2. Plant J. 51, 308–321. doi: 10.1111/j.1365-313X.2007.0141.x
Szũcs, P., Skinner, J. S., Karsai, I., Cuesta-Marcos, A., Haggard, K. G., Corey, A. E., et al. (2007). Validation of the VRN-H2/VRN-H1 epistatic model in barley reveals that intron length variation in VRN-H1 may account for a continuum of vernalization sensitivity. Mol. Genet. Genomics 277, 249–261. doi: 10.1007/s00438-006-0195-8
Takahashi, R., and Yasuda, S. (1971). “Genetics of earliness and growth habit in barley,” in Proceedings of the 2nd International Barley Genetics Symposium, ed. R. Nilan (Washington, DC: Washington State University Press), 388–408.
Tondelli, A., Xu, X., Moragues, M., Sharma, R., Schnaithmann, F., Ingvardsen, C., et al. (2013). Structural and temporal variation in genetic diversity of european spring two-row barley cultivars and association mapping of quantitative traits. Plant Genome 6:Plantgenome2013.2003.0007. doi: 10.3835/plantgenome2013.03.0007
Trevaskis, B., Bagnall, D. J., Ellis, M. H., Peacock, W. J., and Dennis, E. S. (2003). MADS box genes control vernalization-induced flowering in cereals. Proc. Natl. Acad. Sci. U.S.A. 100:13099. doi: 10.1073/pnas.1635053100
Trevaskis, B., Tadege, M., Hemming, M. N., Peacock, W. J., Dennis, E. S., and Sheldon, C. (2007). Short vegetative phase-like MADS-box genes inhibit floral meristem identity in barley. Plant Physiol. 143, 225–235. doi: 10.1104/pp.106.090860
Turner, A., Beales, J., Faure, S., Dunford, R. P., and Laurie, D. A. (2005). The pseudo-response regulator Ppd-H1 provides adaptation to photoperiod in barley. Science 310, 1031–1034. doi: 10.1126/science.1117619
Turner, A. S., Faure, S., Zhang, Y., and Laurie, D. A. (2013). The effect of day-neutral mutations in barley and wheat on the interaction between photoperiod and vernalization. Theor. Appl. Genet. 126, 2267–2277. doi: 10.1007/s00122-013-2133-6
von Zitzewitz, J., Cuesta-Marcos, A., Condon, F., Castro, A. J., Chao, S., Corey, A., et al. (2011). The genetics of winterhardiness in barley: perspectives from genome-wide association mapping. Plant Genome 4, 76–91. doi: 10.3835/plantgenome2010.12.0030
von Zitzewitz, J., Szûcs, P., Dubcovsky, J., Yan, L., Francia, E., Pecchioni, N., et al. (2005). Molecular and structural characterization of barley vernalization genes. Plant Mol. Biol. 59, 449–467. doi: 10.1007/s11103-005-0351-2
Wang, M., and Xu, S. (2019). Statistical power in genome-wide association studies and quantitative trait locus mapping. Heredity 123, 287–306. doi: 10.1038/s41437-019-0205-3
Yan, L., Fu, D., Li, C., Blechl, A., Tranquilli, G., Bonafede, M., et al. (2006). The wheat and barley vernalization gene VRN3 is an orthologue of FT. Proc. Natl. Acad. Sci. U.S.A. 103:19581. doi: 10.1073/pnas.0607142103
Yan, L., Loukoianov, A., Blechl, A., Tranquilli, G., Ramakrishna, W., SanMiguel, P., et al. (2004). The wheat VRN2 gene is a flowering repressor down-regulated by vernalization. Science 303, 1640–1644. doi: 10.1126/science.1094305
Yan, L., Loukoianov, A., Tranquilli, G., Helguera, M., Fahima, T., and Dubcovsky, J. (2003). Positional cloning of the wheat vernalization gene VRN1. Proc. Natl. Acad. Sci. U.S.A. 100:6263. doi: 10.1073/pnas.0937399100
Zhang, Z., Ersoz, E., Lai, C.-Q., Todhunter, R. J., Tiwari, H. K., Gore, M. A., et al. (2010). Mixed linear model approach adapted for genome-wide association studies. Nat. Genet. 42, 355–360. doi: 10.1038/ng.546
Keywords: barley, low temperature tolerance, GWAS, meta-analysis, facultative, multi-environments
Citation: Muñoz-Amatriaín M, Hernandez J, Herb D, Baenziger PS, Bochard AM, Capettini F, Casas A, Cuesta-Marcos A, Einfeldt C, Fisk S, Genty A, Helgerson L, Herz M, Hu G, Igartua E, Karsai I, Nakamura T, Sato K, Smith K, Stockinger E, Thomas W and Hayes P (2020) Perspectives on Low Temperature Tolerance and Vernalization Sensitivity in Barley: Prospects for Facultative Growth Habit. Front. Plant Sci. 11:585927. doi: 10.3389/fpls.2020.585927
Received: 24 August 2020; Accepted: 01 October 2020;
Published: 09 November 2020.
Edited by:
Elizabeth Dennis, Commonwealth Scientific and Industrial Research Organisation (CSIRO), AustraliaReviewed by:
Ben Trevaskis, Commonwealth Scientific and Industrial Research Organisation (CSIRO), AustraliaMartin Mascher, Leibniz Institute of Plant Genetics and Crop Plant Research (IPK), Germany
Copyright © 2020 Muñoz-Amatriaín, Hernandez, Herb, Baenziger, Bochard, Capettini, Casas, Cuesta-Marcos, Einfeldt, Fisk, Genty, Helgerson, Herz, Hu, Igartua, Karsai, Nakamura, Sato, Smith, Stockinger, Thomas and Hayes. This is an open-access article distributed under the terms of the Creative Commons Attribution License (CC BY). The use, distribution or reproduction in other forums is permitted, provided the original author(s) and the copyright owner(s) are credited and that the original publication in this journal is cited, in accordance with accepted academic practice. No use, distribution or reproduction is permitted which does not comply with these terms.
*Correspondence: María Muñoz-Amatriaín, bWFyaWEubXVub3pfYW1hdHJpYWluQGNvbG9zdGF0ZS5lZHU=; Javier Hernandez, aGVybmFuZnJAb3JlZ29uc3RhdGUuZWR1
†These authors have contributed equally to this work