- 1Graduate School of Human and Environmental Studies, Kyoto University, Kyoto, Japan
- 2Institute of Evolutionary Biology, Faculty of Biology, Biological and Chemical Research Centre, University of Warsaw, Warsaw, Poland
- 3Japan Agency for Marine-Earth Science and Technology, Yokosuka, Japan
- 4Graduate School of Agriculture, Kyoto University, Kyoto, Japan
Organisms that have lost their photosynthetic capabilities are present in a variety of eukaryotic lineages, such as plants and disparate algal groups. Most of such non-photosynthetic eukaryotes still carry plastids, as these organelles retain essential biological functions. Most non-photosynthetic plastids possess genomes with varied protein-coding contents. Such remnant plastids are known to be present in the non-photosynthetic, bacteriovorous alga Pteridomonas danica (Dictyochophyceae, Ochrophyta), which, regardless of its obligatory heterotrophic lifestyle, has been reported to retain the typically plastid-encoded gene for ribulose-1,5-bisphosphate carboxylase/oxygenase (RuBisCO) large subunit (rbcL). The presence of rbcL without photosynthetic activity suggests that investigating the function of plastids in Pteridomonas spp. would likely bring unique insights into understanding the reductive evolution of plastids, their genomes, and plastid functions retained after the loss of photosynthesis. In this study, we demonstrate that two newly established strains of the non-photosynthetic genus Pteridomonas possess highly reduced plastid genomes lacking rbcL gene, in contrast to the previous report. Interestingly, we discovered that all plastid-encoded proteins in Pteridomonas spp. are involved only in housekeeping processes (e.g., transcription, translation and protein degradation), indicating that all metabolite synthesis pathways in their plastids are supported fully by nuclear genome-encoded proteins. Moreover, through an in-depth survey of the available transcriptomic data of another strain of the genus, we detected no candidate sequences for nuclear-encoded, plastid-directed Fe–S cluster assembly pathway proteins, suggesting complete loss of this pathway in the organelle, despite its widespread conservation in non-photosynthetic plastids. Instead, the transcriptome contains plastid-targeted components of heme biosynthesis, glycolysis, and pentose phosphate pathways. The retention of the plastid genomes in Pteridomonas spp. is not explained by the Suf-mediated constraint against loss of plastid genomes, previously proposed for Alveolates, as they lack Suf genes. Bearing all these findings in mind, we propose the hypothesis that plastid DNA is retained in Pteridomonas spp. for the purpose of providing glutamyl-tRNA, encoded by trnE gene, as a substrate for the heme biosynthesis pathway.
Introduction
Plastids came into existence by endosymbiosis between a cyanobacterium and an ancient eukaryotic host that is currently believed to be the common ancestor of Archaeplastida. Plastids show the punctate distribution in various branches of the eukaryotic tree of life, as they have been laterally transferred through multiple independent secondary and/or higher order endosymbioses (Archibald, 2009; Keeling, 2010). These photosynthetic organisms are capable of efficiently converting solar energy to biochemical energy, which is subsequently utilized for various metabolic processes, such as carbon fixation, amino acid synthesis, nitrogen and sulfur metabolism, carotenoid synthesis, heme and chlorophyll synthesis, and a wide array of other metabolite synthesis pathways (Yasuno and Wada, 2002; Kleffmann et al., 2004; DellaPenna and Pogson, 2006; Hörtensteiner and Kräutler, 2011; Terashima et al., 2011; Przybyla-Toscano et al., 2018). An overwhelming part of the aforementioned pathways involves redox reactions, and these require proteins containing iron–sulfur (Fe–S) clusters as a cofactor for electron transport (Przybyla-Toscano et al., 2018).
Regardless of the beneficial aspects of photosynthesis as a mechanism to support autotrophic lifestyles by utilizing an easily accessible energy source, many organisms descending from photosynthetic ancestors have lost the ability of photosynthesis, therefore becoming obligate heterotrophs. Such secondarily non-photosynthetic organisms are known in land plants, green and red algae, ochrophytes, dinoflagellates and many other lineages; in fact, haptophytes, glaucophytes, and chlorarachniophytes are the only major plastid-bearing groups with no cases of photosynthesis loss known to date (Maciszewski and Karnkowska, 2019). Within photosynthetic lineages, photosynthesis losses may occur repeatedly, either in distantly related organisms or in close relatives. For example, all of the Apicomplexa (one of the main lineages of Alveolata) are descendants of a single ancestor that lost its photosynthetic capabilities, while in other lineages, such as diatoms, cryptophytes, or euglenids, losses of photosynthesis are documented to have occurred more than once in a single genus (Marin et al., 2003; Kamikawa et al., 2015; Salomaki and Kolisko, 2019).
With only a few exceptions, secondarily non-photosynthetic organisms still retain plastids, along with their genomes. As a prominent part of plastid genomes’ coding content constitutes photosynthesis-related genes, loss of photosynthesis is strictly tied with reductive evolution of plastid genomes, thus resulting in a varying degree of reduction in its size and gene repertoire. It is, however, worth noting that the patterns of gene loss and retention are extremely diverse across the tree of life. In many cases, non-photosynthetic plastids still possess genes for performing processes typically connected to photosynthesis, even though they forfeited the ability to absorb light. For example, certain secondarily non-photosynthetic lineages (e.g., the chlorophytes Prototheca spp. and the diatoms Nitzschia spp.) have retained the plastid-encoded ATP synthase complex genes (Kamikawa et al., 2015; Severgnini et al., 2018; Suzuki et al., 2018), while the gene for the large subunit of ribulose-1,5-bisphosphate carboxylase/oxygenase (RuBisCO) is present in non-photosynthetic plastids of Cryptomonas paramecium (Cryptophyta) and Euglena longa (Euglenophyta) (Donaher et al., 2009; Záhonová et al., 2016). In contrast, apicoplast genomes (plastid genomes of apicomplexans) carry no gene related to photosynthesis, but only the sufB gene, involved in Fe–S cluster assembly, and a complement of housekeeping genes, enabling transcription and translation of sufB (Salomaki and Kolisko, 2019).
There are, however, many secondarily non-photosynthetic organisms whose plastids’ functionality remains undescribed. One of these organisms is Pteridomonas danica, a non-photosynthetic, plastid-bearing bacteriovorous species of Dictyochophyceae (Ochrophyta). Knowledge on this organism’s plastid seems to be limited to its rbcL gene, retained in a similar manner to the aforementioned C. paramecium and E. longa (Sekiguchi et al., 2002), although in contrast to those two, no full plastid genome sequence from P. danica, or any other colorless dictyochophycean, has been reported so far. It is noteworthy that the phylogenetic analysis of P. danica’s rbcL demonstrated that its branch length was comparable to those of its photosynthetic relatives, suggesting that the gene’s evolutionary rate is not significantly increased. Therefore, it has not undergone remarkable degeneration, in contrast to photosynthesis-related genes in many non-photosynthetic organisms (Sekiguchi et al., 2002; Dorrell et al., 2019). Based on this observation, we hypothesized that the plastid genome of Pteridomonas might reflect the early stages of the reductive evolution of plastid genomes following the loss of photosynthesis.
Here, we report the complete plastid genomes of two strains of Pteridomonas spp. and show that in sharp contrast to the previous expectations, they are among the most functionally reduced plastid genomes reported to date. Our findings also demonstrate that even among very closely related species, the fates of genomes undergoing reduction can vary substantially through differential gene losses. Finally, we propose the most likely functional evolutionary constraint against complete loss of plastid genome after forfeiture of photosynthesis in Pteridomonas.
Materials and Methods
Isolation, Cultivation, and DNA Sequencing
A plankton net sample was collected at the port of JAMSTEC headquarters, Yokosuka, Kanagawa, Japan (35°19′09′′N, 139°39′02′′E) on January 9, 2013. A small aliquot of the net sample was initially added to the KLB medium (Yabuki and Tame, 2015) and incubated at 19–20°C under the 14-h light/10-h dark cycle condition. A single cell of Pteridomonas sp. was isolated from the enriched culture by micropipetting. The established strain YPF1301 was maintained under the same culture conditions and also deposited in the National Institute for the Environmental Sciences (NIES), Tsukuba, Japan as NIES-3357.
The other sample was collected at the Clover Point, Victoria, British Columbia, Canada (48°24′16″N, 123°21′02″W) on April 3, 2017, from the sand just beneath the water line in lower intertidal/upper subtidal layer. The established strain P. danica NY0221 was maintained using F/2 saltwater medium (prepared according to the guidelines from UTEX culture collection website), supplemented with vitamin B12 (to a total concentration of 100 μg l–1) and a single autoclaved barley seed (Hordeum vulgare). The strain was maintained in the same environmental conditions as strain YPF1301.
Using the DNeasy Plant mini kit (QIAGEN) following the manufacturer’s instruction, total DNA of Pteridomonas sp. YPF1301 was extracted from 1.32 × 107 cells that were harvested from 15 flasks of 2-weeks-old 75 ml culture in total by 1,000 × g for 5 min. The procedure produced 50 μl of the DNA solution with 42.8 ng/μl concentration. The total DNA was sent to Hokkaido System Science Co., Ltd. (Japan) for 100 bp paired-end sequencing by the Illumina HiSeq2500 platform using the 350-bp library constructed with TruSeq Nano DNA Library Prep Kit (Illumina) following the manufacturer’s instructions. The sequencing produced 54.0 million reads. Adapter trimming and quality filtering were performed with FASTX-Toolkit1. Reads with quality scores > 20 for at least 75% of their length were retained after quality filtering, resulting in 49.4 million paired-end reads. The filtered short reads were subjected to SPAdes-3.10.0 (Bankevich et al., 2012) with default settings for assembling.
In case of P. danica NY0221, total DNA was isolated from cell pellets obtained by centrifugation of two 10 ml culturing tubes using the same kit as above, resulting in a 50 μl DNA sample with 23 ng/μl concentration. This sample was handed to a different external company (Genomed S.A., Warsaw, Poland) for high-throughput sequencing using Illumina MiSeq technology, producing six million paired-end, 300 bp long reads. Raw data quality control was performed using FastQC tool2; additional trimming was not necessary due to adapter removal and quality filtering being parts of data handling by the sequencing company. Genome assembly was performed identically as in case of the strain YPF1301.
Reconstruction of Plastid DNA Sequences
Through homology-based search with plastid-encoded protein sequences of the dictyochophycean alga Florenciella parvula as queries, five AT-rich contigs highly likely derived from a plastid DNA was detected from the DNA assembly of Pteridomonas sp. YPF1301. By using PCR assays followed by the Sanger sequencing to fill the gap between termini of the contigs, we obtained a single DNA sequence of the plastid DNA with 54,809 bp in length for Pteridomonas sp. YPF1301. However, we could not map the genome as circular as no amplification occurred by PCR assays with multiple sets of primers bridging both termini of the single DNA sequence. Protein-coding genes were identified with MFannot3. The annotation was confirmed by BLASTP analyses of plastid-encoded protein sequences of Pteridomonas sp. YPF1301 against the GenBank non-redundant (nr) database. Transfer RNA genes were identified with MFannot3 and tRNAscan-SE (Lowe and Chan, 2016).
In contrast to YPF1301 strain, an analogous plastid-encoded protein homology-based search led to the identification of only one, 33,539 bp-long candidate plastid contig in the assembly of P. danica NY0221. A visualization of the contig using Bandage software (Wick et al., 2015) revealed the contig maps as circular, therefore most likely representing a full plastid genome molecule; additionally, attempts to elongate the putative plastid genome assembly using the same software tool failed, as it retrieved no additional contigs matching to any of the ends of the 33,539 bp-long contig. To further confirm that the circularly mapped sequence is the complete plastid genome, we also remapped the reads onto the plastid DNA with Bowtie2 (Langmead and Salzberg, 2012), resulting in >20× coverage observed consistently throughout the sequence, including the original start position. These procedures indicated that there was no additional plastid DNA contig to be assembled, and the plastid DNA of NY0221 was a circularly mapped molecule. Annotation of the contig was carried out using cpGAVAS online tool (Liu et al., 2012), followed by the Annotation Transfer feature available in Geneious v10.2.6 (Kearse et al., 2012), using the four available dictyochophycean plastid genomes (Han et al., 2019) as references, and manual curation.
Phylogenetic Analysis of Plastid-Encoded Proteins
To see whether the plastid-encoded protein sequences of Pteridomonas spp. evolve as rapidly as those of the other non-photosynthetic Ochrophyta species (Kamikawa et al., 2015), we chose 38 proteins encoded in the plastid DNAs of both Pteridomonas sp. YPF1301 and P. danica NY0221. The same protein sequences were also retrieved from the previously sequenced plastid genomes of 44 species, including five non-photosynthetic species, in GenBank (Supplementary Material). Homologs of each protein were aligned by MAFFT (Katoh and Standley, 2013) with the L-INS-i option. Ambiguously aligned sites were removed manually by BioEdit (Hall, 1999). Datasets of each protein were concatenated, and the resulting dataset, composed of 44 taxa and 6,660 sites, was subjected to phylogenetic analysis using IQ-TREE 1.6.12 (Nguyen et al., 2015), under the LG + C60 + F + Γ-PMSF substitution model with 100 bootstrap analyses.
Survey of Iron–Sulfur Cluster Proteins and Metabolic Pathways in the Plastid of Pteridomonas danica Strain PT
We obtained the assembled transcriptome data of P. danica strain PT from the Marine Microbial Eukaryote Transcriptome Sequencing Project (MMETSP; Keeling et al., 2014). Iron–sulfur cluster proteins in P. danica PT were surveyed with TBLASTN using queries of homologs from Arabidopsis thaliana (Couturier et al., 2013; Przybyla-Toscano et al., 2018) and diatoms (Kamikawa et al., 2017). Detected iron–sulfur cluster homologs were then confirmed by BLASTP against the non-redundant protein database of GenBank. Organellar targeting sequences of the detected homologs were evaluated with ASAFind (Gruber et al., 2015) and MitoFates (Fukasawa et al., 2015).
We also surveyed plastid-targeted protein sequences which require the iron–sulfur cluster as a cofactor, such as iron–sulfur domain-containing protein NEET, flavodoxin, chaperone protein dnaJ C76, translocon at the inner envelope membrane of chloroplasts 55, and ferredoxin, using homologs from Arabidopsis thaliana and photosynthetic stramenopiles available in NCBI database. The queries of photosynthetic stramenopiles were obtained from iron–sulfur cluster-containing proteins of Arabidopsis thaliana (Przybyla-Toscano et al., 2018) with TBLASTN search (Supplementary Table S1). Detected homologs from photosynthetic stramenopiles were confirmed and evaluated their plastid-targeting signals as described above. Sequences for the other plastid metabolisms (Bock and Khan, 2004; DellaPenna and Pogson, 2006; Hiltunen et al., 2012; Kamikawa et al., 2017; Przybyla-Toscano et al., 2018) were also surveyed.
Detection of Nuclear-Encoded, Plastid-Targeted TufA
We performed TBLASTN search with Florenciella parvula plastid-encoded TufA protein sequence (GenBank accession number: YP_009684446.1) as a query against the DNA assembly of Pteridomonas sp. YPF1301. Through this procedure, we detected three contigs carrying genes for TufA. One of them was identified as mitochondrial TufA, as it possessed the N-terminal mitochondrial targeting sequence detected by MitoFates (Fukasawa et al., 2015). The others coded either the N-terminal or the C-terminal half of the TufA protein. Plastid targeting sequences were detected by ASAFind (Gruber et al., 2015) in both sequences. We also surveyed the mitochondria-targeted and plastid-targeted TufA sequences in the transcriptome data of P. danica PT by a homology-based search, with Pteridomonas sp. YPF1301 TufA protein sequences as queries. Mitochondrial targeting signals were detected by MitoFates (Fukasawa et al., 2015). Only the C-terminal half of TufA protein was detected in the transcriptome data of P. danica PT, and the sequence was revealed to have N-terminal plastid-targeting sequence detected by ASAFind (Gruber et al., 2015). Those sequences were aligned with plastid and mitochondria-targeting TufA proteins by MAFFT (Katoh and Standley, 2013). Ambiguously aligned sites were removed manually by BioEdit (Hall, 1999). The resulting dataset, comprising 147 taxa and 369 sites, was used for phylogenetic analysis using IQ-TREE 1.6.12 (Nguyen et al., 2015), under the LG + Γ + F substitution model with 100 bootstrap analyses.
PCR-Based Survey and Phylogenetic Analysis of the rbcL Gene
Total DNA of Pteridomonas sp. strain YPF1301 was extracted as described above. Total DNA of Phaeodactylum tricornutum UTEX642 was extracted with Wizard Genomic DNA Purification Kit (Promega) according to the manufacturer’s instruction. We designed two primer sets exactly matched to the rbcL gene sequence of P. danica (Sekiguchi et al., 2002): rbcLF1/R1 and rbcLF2/R2 (Supplementary Table S2). We also designed a degenerated rbcL primer set (stramenopiles_RbcL_F/R; Supplementary Table S2) on the basis of RbcL protein sequences from ten Ochrophyta species (Pedinella sp. AB081639, Pteridomonas danica AB081642, Pseudochattonella verruculosa AB280607, Synura borealis HG514235, Phaeodactylum tricornutum MH064125, Nitzschia palea MH113811, Florenciella parvula MK518352, Pseudopedinella elastica MK518353, Dictyocha speculum MK561359, Rhizochromulina marina MK561360). The actin primer set was specifically designed for the actin gene sequence retrieved from the DNA assembly data of YPF1301 (Supplementary Table S2). Exact match primers were also designed for the plastid-encoded rpl36 gene (Supplementary Table S2). For all the primer sets, PCR amplification was conducted for 30 cycles of: a denaturation step at 98°C for 10 s, an annealing step at 55°C for 30 s, and an elongation step at 72°C for 2 min.
The rbcL gene sequences of Pteridomonas danica (Sekiguchi et al., 2002) were aligned with available sequence data of Dictyochophyceae in the GenBank nucleotide database using MAFFT (Katoh and Standley, 2013). Ambiguously aligned sites were removed manually by BioEdit (Hall, 1999). The resulting dataset, comprising 24 taxa and 1,364 sites, was subjected to phylogenetic analysis using IQ-TREE 1.6.12 (Nguyen et al., 2015), under the GTR + Γ + I + F substitution model with 100 bootstrap replicates.
Phylogenetic Analysis of 18S rRNA Gene
Nuclear 18S rRNA gene sequences of Pteridomonas sp. YPF1301 and P. danica NY0221 were retrieved from the DNA assembly data, and that of P. danica PT was retrieved from the transcriptome data. The sequences obtained were aligned with available sequence data of Dictyochophyceae in GenBank using MAFFT (Katoh and Standley, 2013). Ambiguously aligned sites were removed manually by BioEdit (Hall, 1999). The resulting dataset, comprising 42 taxa and 1,494 sites, was subjected to phylogenetic analysis using IQ-TREE 1.6.12 (Nguyen et al., 2015), under the GTR + Γ + I substitution model with 100 bootstrap replicates.
Results and Discussion
Plastid DNA in Pteridomonas spp.
In the initial stage of this project, we have established two strains of Pteridomonas spp., strain YPF1301 and strain NY0221. Both strains are closely related to the P. danica investigated in the study presented by Sekiguchi et al. (2002), and to the P. danica strain PT, from which transcriptomic data has been obtained in the Marine Microbial Eukaryote Transcriptome Sequencing Project (Keeling et al., 2014) (Figure 1).
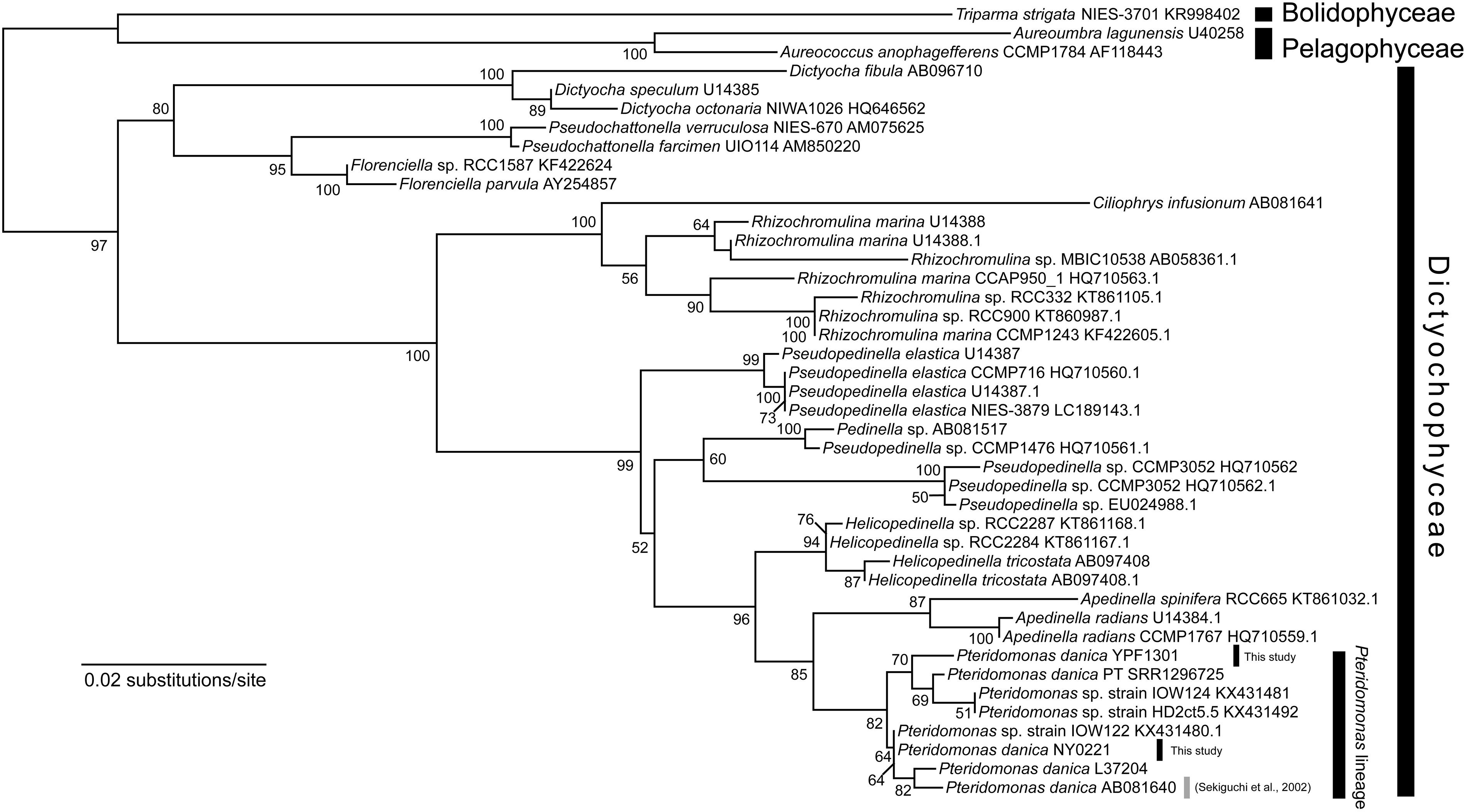
Figure 1. Maximum likelihood tree of 18S rRNA gene sequences of Dictyochophyceae. The tree was inferred with IQ-TREE 1.6.12 under the GTR + Γ + I model. Bootstrap values ≥50 are shown on branches. P. danica reported to bear rbcL is highlighted by gray line (Sekiguchi et al., 2002).
First, we sequenced the plastid DNA of the non-photosynthetic dictyochophyceaen Pteridomonas sp. strain YPF1301. The homology-based search of plastid-encoded proteins against the assembly of Pteridomonas sp. YPF1301, followed by PCR and the Sanger sequencing, resulted in a single, 54,809 bp-long, DNA sequence representing the plastid genome (Supplementary Figure S1). Regardless of numerous trials of PCR assay with multiple primer sets, we could not close the gap between termini of the plastid DNA sequence (data not shown). Instead, we detected a short, non-coding inverted repeat at both ends of the plastid genome sequence (Figure 2 and Supplementary Figure S1), similar to the ones reported from other linear-mapping organellar genomes (Nosek et al., 2004; Janouškovec et al., 2013; Oborník and Lukeš, 2015; Salomaki et al., 2015; Kamikawa et al., 2018). As no other contig has been detected as a candidate plastid genome fragment, we conclude that the 55 kb-long DNA covers the almost complete plastid genome of Pteridomonas sp. YPF1301. It remains unresolved whether the plastid DNA of Pteridomonas sp. YPF1301 is a linear molecule, or a circular molecule with an extended non-coding region, non-detectable by the homology-based methods. Both possibilities can equally well explain failures of amplification by our multiple PCR trials (data not shown). The plastid genome of Pteridomonas sp. YPF1301 carries 40 protein-coding genes, 24 transfer RNA genes, and two rRNA genes, as well as three functionally unassigned open reading frames (ORFs; Figure 2 and Table 1). The coding regions occupy 57.8% of the genome, excluding unassigned ORFs (62.3%, including those regions as coding ones) (Table 1). More than one-third of the genome is likely non-coding. The plastid genome is devoid of the tetrapartite structure, composed of a small single-copy region, a large single-copy region, and two inverted repeat copies of an rRNA operon, commonly observed in photosynthetic and non-photosynthetic plastid genomes of Ochrophyta (Han et al., 2019).
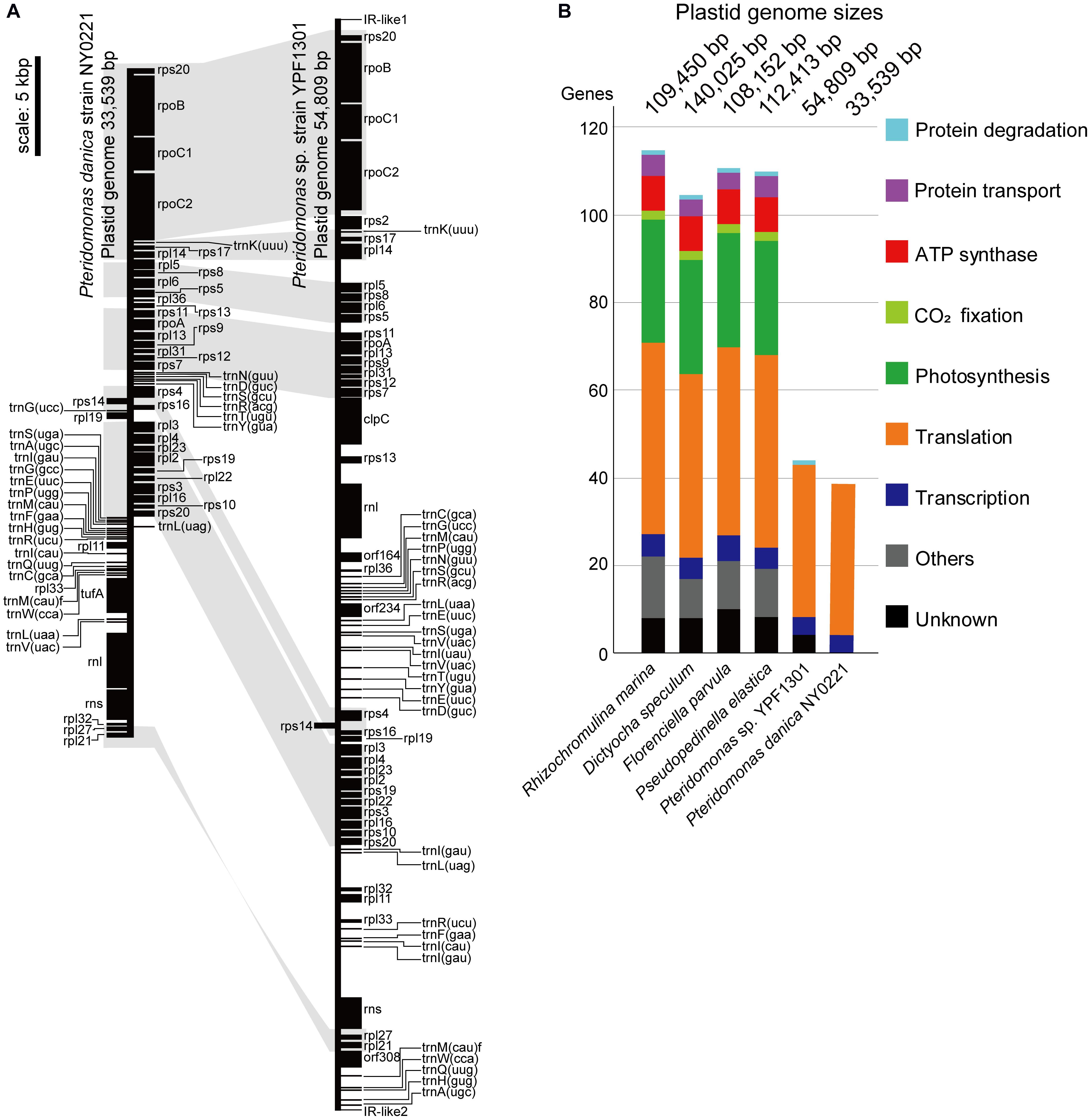
Figure 2. Plastid genomes of Pteridomonas sp. strain YPF1301 and Pteridomonas danica strain NY0221. (A) Plastid genome maps. The genomes are shown as linear for comparison of gene locations although the genome of strain NY0221 is a circularly mapping molecule (Supplementary Figure S1). Protein-coding and ribosomal RNA-coding regions are shown as black boxes. Transfer RNAs are shown as black lines. Conserved gene orders are highlighted in light gray. (B) Comparison of plastid-encoded genes and genome sizes among the photosynthetic and non-photosynthetic species of Dictyochophyceae (Han et al., 2019). Any color boxes show functional categories: light blue for protein degradation (clpC), violet for transport (sec, tatC), red for ATP synthase (atp), light green for CO2 fixation (rbcL), green for photosynthesis (psa, psb, pet), orange for translation (rps, rpl, tufA), blue for transcription (rpo), gray for other known functions, black for unknown functions (orf, ycf).
Subsequently, we sequenced the total DNA of Pteridomonas danica strain NY0221, and in contrast to YPF1301, we assembled a 33,539 bp-long circularly mapping molecule representing the plastid genome (Supplementary Figure S1). The plastid genome of P. danica NY0221 carries 39 protein-coding genes, 24 tRNA genes, and two rRNA genes (Table 1), and also lacks inverted repeats of the rRNA operon. The non-coding regions occupy less than 10% of this genome, in contrast with the expanded non-coding regions in the plastid genome of strain YPF1301. The gene-dense plastid genome of NY0221 strain is consistent with the previous observations of gene-dense plastid genomes of other non-photosynthetic members of Ochrophyta, usually with more than 80% of coding regions per genome (Kamikawa et al., 2018; Dorrell et al., 2019). Thus, the plastid genome of strain YPF1301, with less than 62% of coding regions, is an exception among those of the non-photosynthetic members of Ochrophyta.
The striking difference in size and gene density between the two plastid genomes of closely related non-photosynthetic Pteridomonas species might represent distinct fate or distinct evolutionary rate of plastid genome erosion after the abandonment of photosynthesis in their last common ancestor.
Recent Genome Rearrangements
Both genomes contain a similar gene set: the 40 proteins encoded in the YPF1301 plastid genome comprise 35 proteins for translation (i.e., ribosomal proteins), four proteins for transcription (i.e., RNA polymerases), and one gene for protein degradation (i.e., ClpC subunit), the latter missing from the plastid genome of strain NY0221. Apart from clpC, the plastid genome of the strain NY0221 also lacks two translation-related genes (rps2, rpl32), retained in strain YPF1301, but contains the translation elongation factor Tu gene (tufA), which is missing in strain YPF1301. Regardless of the previous report for rbcL gene in P. danica (Sekiguchi et al., 2002), we obtained no evidence of the corresponding gene sequence in the plastid genomes of our newly established strains (Figures 2A,B).
Interestingly, despite the differences in coding contents and size, the order of protein-coding genes in plastid genomes of Pteridomonas spp. is almost identical, with only a single, two protein-coding gene block (rpl33, rpl11) having inverse orientation. These genomes, however, are not fully syntenic, as the order of tRNA-encoding genes is drastically different (Figure 2 and Supplementary Figure S2). It would suggest that numerous rearrangements have taken place in their evolutionary past, but in contrast to other dictyochophyceans, they do not seem to affect the protein-coding gene order to a noticeable extent (Han et al., 2019). Although both plastid genomes presented in this work carry a single copy of the ribosomal RNAs, its organization differs substantially between the investigated strains. The small (rns) and large (rnl) ribosomal RNA subunit genes are clustered together in the same orientation in P. danica NY0221, which is a rather common feature in plastid genomes, as it has also been observed in another dictyochophyte, Dictyocha speculum (Han et al., 2019), as well as in certain diatoms (Cao et al., 2016), euglenids (Karnkowska et al., 2018) and chlorophytes (Turmel et al., 2017). In Pteridomonas sp. YPF1301, however, the ribosomal RNA small and large subunit genes are present in inverse orientation and separated by a cluster of 18 protein-coding genes, constituting almost half of the entire plastid genome; such a “broken” ribosomal operon has been identified so far exclusively in the plastid genome of the non-photosynthetic, parasitic green alga Helicosporidium sp. (de Koning and Keeling, 2006). The difference in rDNA organization between the examined strains of Pteridomonas can be interpreted as yet another indicator of the divergent evolution of plastid genomes in these two closely related organisms.
Extraordinarily Functionally Reduced Plastid DNAs of Pteridomonas spp.
When compared to other species of Dictyochophyceae (Han et al., 2019), the plastid genomes of Pteridomonas spp. are the smallest in size and gene content (Figure 2B). So far, plastid genomes of four photosynthetic Dictyochophyceae have been sequenced, and their sizes range from 108 to 140 kb, which are two or more times larger than the two Pteridomonas spp. plastid genomes analyzed here (Figure 2B). The photosynthetic plastid genomes of Dictyochophyceae sequenced to date carry 37 to 42 genes encoding proteins involved in photosynthesis, carbon fixation, and chlorophyll synthesis, 4 to 5 genes for protein transport, 42 to 44 genes for translation, 5 to 6 genes for transcription, and one gene for protein degradation. In contrast, functions encoded in the Pteridomonas spp. plastid genomes are dramatically reduced: all genes for photosynthesis, carbon fixation, chlorophyll synthesis, ATP synthesis/degradation, chaperonins (groEL), and Fe–S cluster assembly (sufB and sufC; Figure 2) are lost, and only genes for translation and transcription (and protein degradation in YPF1301) are retained. Moreover, their sequences are highly divergent and form long-branches on the phylogenetic tree, comparable to branches of other non-photosynthetic species of Ochrophyta (Supplementary Figure S3). The Pteridomonas plastid genomes encode fewer functions than other plastid genomes of non-photosynthetic species with red alga-derived plastids, including apicomplexan parasites and non-photosynthetic red algae (Figure 3A). Even among non-photosynthetic species, including green algal lineages and plants (Figure 3B), Pteridomonas plastid genomes are one of the most functionally reduced ones.
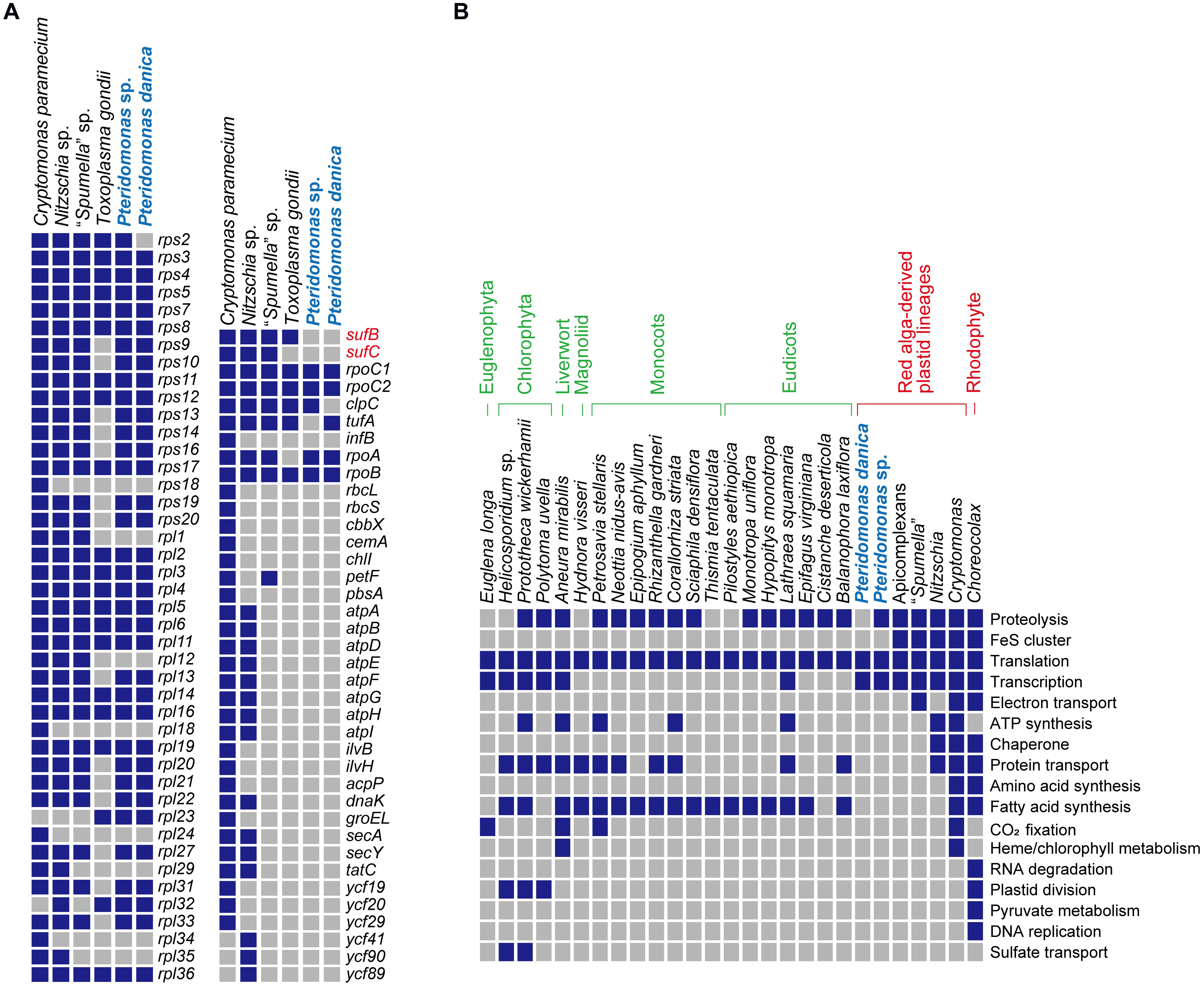
Figure 3. Comparison of non-photosynthetic plastid genomes. (A) Comparison of plastid gene contents from non-photosynthetic organisms with red alga-derived plastid. Nitzschia sp. NIES-3581 (Bacillariophyceae; GenBank no. AP018508; Kamikawa et al., 2015), Cryptomonas paramecium (Cryptophyta; GenBank no. GQ358203; Donaher et al., 2009), Toxoplasma gondii (Apicomplexa; GenBank no. U87145; Köhler et al., 1997), “Spumella” sp. NIES-1846 (Chrysophyceae; GenBank no. AP019363; Dorrell et al., 2019) were used for comparison. Presence and absence are shown by blue and gray boxes, respectively. (B) Comparison of the estimated functions encoded in non-photosynthetic plastid genomes. All the data except for Pteridomonas spp. highlighted in light blue are derived from Hadariová et al. (2018) and Dorrell et al. (2019).
The most unexpected results are the lack of rbcL, sufB, and sufC genes in both strains, and the punctate distribution of tufA and clpC genes in Pteridomonas spp. genomes. First, we reveal the punctate distribution of tufA gene in the plastid genomes of Pteridomonas spp., which is explained by the recent endosymbiotic gene transfer. Second, the strain of P. danica reported by Sekiguchi et al., the close relative of Pteridomonas spp. YPF1301 and NY0221 (Figure 1), retained the rbcL gene (Sekiguchi et al., 2002). Lastly, sufB and sufC genes are highly conserved among plastid genomes of photosynthetic Dictyochophyceae, and also non-photosynthetic, red alga-derived plastid-bearing species, with only a few exceptions (Janouškovec et al., 2015; Dorrell et al., 2019; Han et al., 2019; see also Figure 3). We discuss the above issues in more details below.
Recent Endosymbiotic Gene Transfer
Punctate distribution of clpC and tufA might be a result of gene loss or endosymbiotic gene transfer. We did not detect any candidate sequence of the clpC gene in the assembly of strain NY0221. The gene for ClpC has been lost in NY0221 after divergence from the lineage leading to YPF1301. However, we retrieved two GC-rich contigs carrying tufA gene fragments, one of which contains a spliceosomal intron, suggesting that the tufA gene is most likely encoded in the nuclear genome of YPF1301 strain. Those results imply a recent plastid-to-nucleus endosymbiotic gene transfer of the tufA gene in Pteridomonas sp. YPF1301. One of the two fragments encodes N-terminal half of the plastid TufA protein, while the other codes its C-terminal half. It would be worth noting that both of the two tufA gene fragments encode bipartite plastid-targeting signals, comprising signal peptides followed by transit peptide-like regions (Supplementary Figures S4A,B), targeting the nuclear-encoded plastid proteins to the organelle (Gruber et al., 2015). Presence of the targeting signal in both sequences suggests that tufA is encoded in two distinct loci and imported into the plastid as two peptides. The phylogenetic analysis of organellar TufA proteins recovered the plastid-encoded sequence of P. danica NY0221 and the nuclear-encoded N-terminal half sequences of Pteridomonas sp. YPF1301 as monophyletic although its bootstrap support was moderate (64%; Supplementary Figure S4C). The monophyletic relationship would suggest a recent endosymbiotic gene transfer to the nucleus. Similarly, the nuclear-encoded C-terminal half sequences of P. danica strain PT and Pteridomonas sp. YPF1301 formed a monophyletic group (bootstrap value = 61%), and the clade was then nested in Ochrophyta, although the position of Pteridomonas sequences was not strongly or even moderately supported. Nevertheless, the lower supports on branches in the tree are most likely due to insufficient phylogenetic signals in the single protein dataset used for the analysis. This finding might represent a possible example that reduced non-photosynthetic plastid genomes are shaped not only by gene losses but also gene flow from the organelle to the nucleus, still ongoing even after photosynthesis loss.
Loss of the Canonical rbcL Gene
To investigate whether rbcL gene is present in the nuclear genome, it was further surveyed by homology-based search, with the previously reported rbcL sequence of P. danica (GenBank accession number: AB081642.1) as a query against the genome assembly of YPF1301, but this procedure did not detect any candidate sequence of rbcL. Similar results were also obtained in the PCR assays. We designed multiple primer sets, including exact match primers to P. danica rbcL and degenerate primers for the conserved regions of RuBisCO large subunit (RbcL; Supplementary Table S2). The PCR assays with these primer sets did not amplify any product for the Pteridomonas sp. YPF1301 DNA (Supplementary Figure S5). These experiments show that rbcL gene is most likely absent in the nuclear genome of Pteridomonas sp. YPF1301 or its sequence is too divergent to be detectable. The rbcS gene for RuBisCO small subunit was also undetectable by the homology-based survey. Similarly, by the homology-based survey, the rbcL and rbcS genes were undetected from the assembled DNA data of P. danica NY0221. These findings might raise a possibility that rbcL genes of P. danica and another non-photosynthetic dictyochophycean species might not be simply derived from a vertical inheritance from photosynthetic ancestors but of certain artifact such as a contamination. Otherwise, these findings might represent ongoing strain-specific rbcL gene losses in strain YPF1301, and most likely also in the strain NY0221.
To evaluate these possibilities, we performed phylogenetic analysis of the rbcL gene, with a richer taxon sampling than that of Sekiguchi et al. (2002) (Figure 4). Consequently, the rbcL gene of P. danica (Sekiguchi et al., 2002) formed a monophyletic clade with those of the photosynthetic dictyochophycean species Pseudopedinella sp. CCMP1476 and Pedinella sp. (90% bootstrap value; Figure 4), inconsistent with the sister relationship between Pteridomonas and Apedinella in the 18S rRNA tree (Figure 1). Similarly, the rbcL gene of the non-photosynthetic dictyochophycean Ciliophrys infusionum nested in the Rhizochromulina clade (Figure 4; 100% bootstrap value), and specifically, formed a monophyletic clade with Rhizochromulina marina (100% bootstrap value; Figure 4). Given the inconsistency between the organismal (18S rRNA) phylogeny and the rbcL phylogeny, we suggest the rbcL genes in P. danica as well as C. infusionum might not be derived from a simple vertical inheritance from their photosynthetic ancestors. As it cannot be concluded whether they are derived of contaminations, possible strain-specific losses of rbcL genes in Pteridomonas spp. should be investigated after isolation of new strains closely related to the previously reported rbcL-bearing P. danica (Sekiguchi et al., 2002).
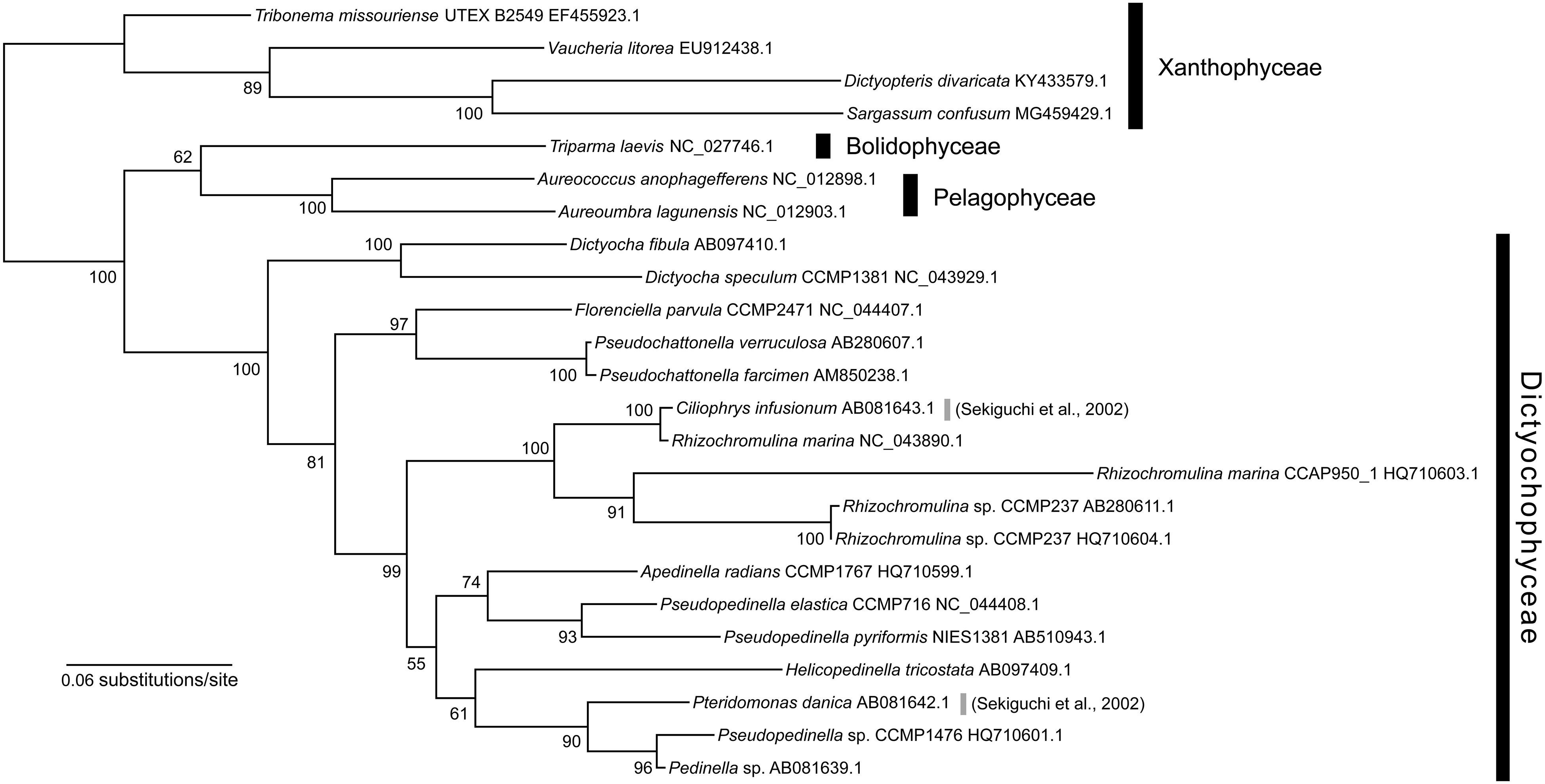
Figure 4. Maximum likelihood tree of rbcL gene sequences of Dictyochophyceae. The tree was inferred with IQ-TREE 1.6.12 under the GTR + Γ + I + F model. Bootstrap values ≥50 are shown on branches.
Loss of Genes for Iron–Sulfur Cluster Assembly
Fe–S cluster assembly involves plastid-encoded proteins SufB and SufC, as well as nuclear-encoded SufA, SufD, SufE, and SufS (Lill, 2009; Balk and Schaedler, 2014; Przybyla-Toscano et al., 2018). Given the absence of sufB and sufC in the plastid contig, we searched the contigs representing nuclear genome for genes encoding Suf system proteins, using homologs from Arabidopsis thaliana (Przybyla-Toscano et al., 2018) and homologs of photosynthetic Ochrophyta species as queries. Using this procedure, we did not detect any Suf family homologs in the assembly of Pteridomonas sp. YPF1301 or in the MMETSP-originated transcriptome of P. danica strain PT (Keeling et al., 2014) (Figure 5). In contrast, genes encoding mitochondrial and cytosolic Fe–S cluster assembly systems were present in the PT transcriptome data (Lill, 2009; Balk and Schaedler, 2014) (Supplementary Figure S6 and Supplementary Table S3). Therefore, we conclude that the Fe–S cluster assembly Suf system is most likely absent from the plastids of Pteridomonas spp.
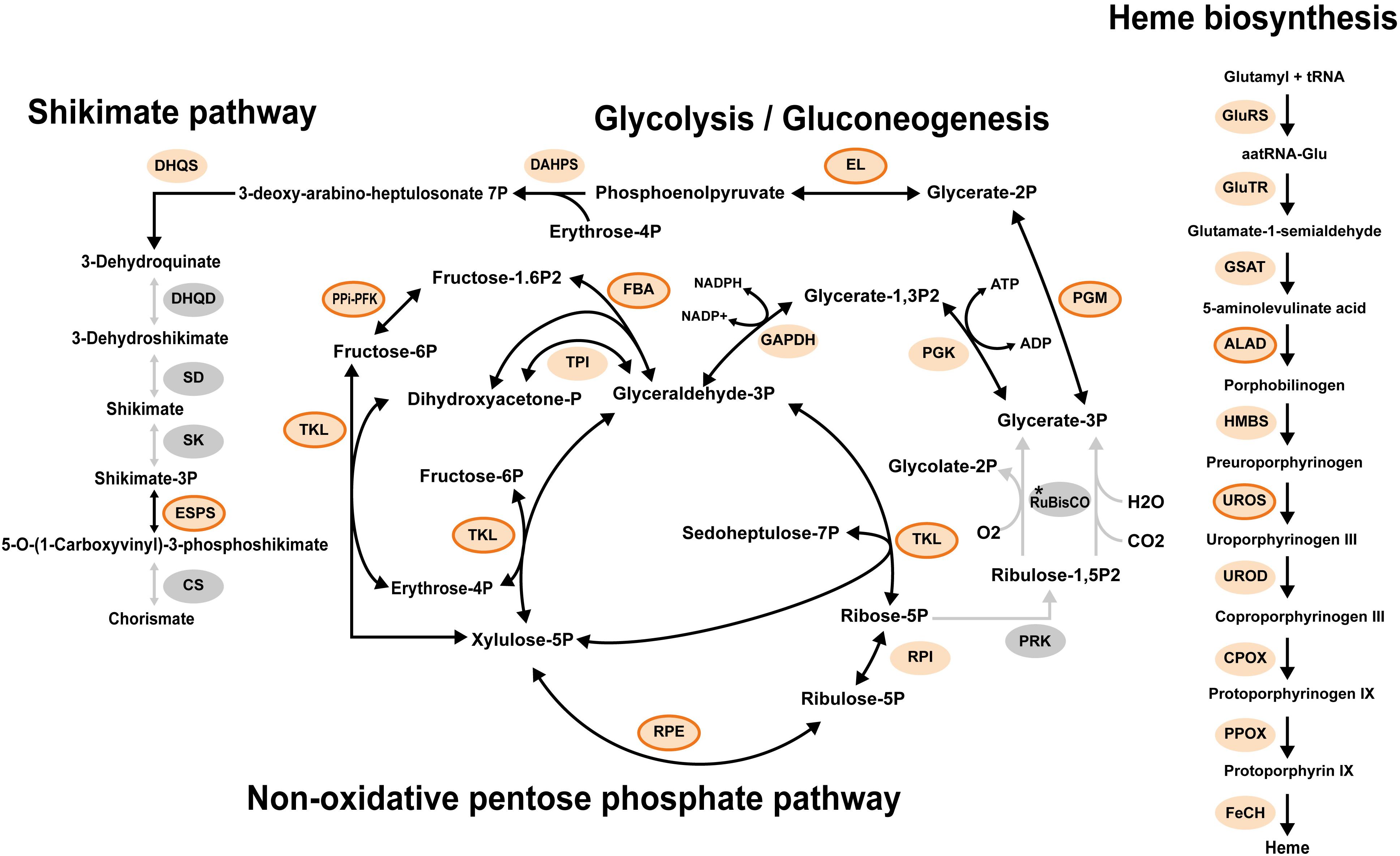
Figure 5. Representative metabolisms of the plastid in Pteridomonas danica strain PT. Light orange circles enclosed by an orange line show proteins with the detectable plastid targeting signals. Light orange circles with no line show proteins with no detectable plastid targeting signal probably due to lack of 5′ termini of sequences in the transcriptome data. Gray circles show proteins not detected. The asterisk shows that it is unclear whether RuBisCO is present as both large and small subunits of RuBisCO are plastid-encoded proteins; A plastid genome of strain PT is unavailable. ALAD, delta-aminolevulinic acid dehydratase; CPOX, coproporphyrinogen oxidase; CS, chorismate synthase; DAHPS, 3-deoxy-7-phosphoheptulonate synthase; DHQD, bifunctional 3-dehydroquinate dehydratase; DHQS, 3-dehydroquinate synthase; EL, enolase; ESPS, 3-phosphoshikimate 1-carboxyvinyltransferase; FBA, fructose 1,6-bisphosphate aldolase; FeCH, ferrochelatase; GAPDH, glyceraldehyde 3-phosphate dehydrogenase; GluRS, glutamyl tRNA synthase; GluTR, glutamyl tRNA reductase; GSAT, glutamate-1-semialdehyde aminotransferase; HMBS, hydroxymethylbilane synthase; PGK, phosphoglycerate kinase; PGM, phosphoglycerate mutase; PPi-PFK, pyrophosphate-dependent phosphofructokinase; PPOX, protoporphyrinogen oxidase; PRK, phosphoribulokinase; RuBisCO, ribulose-1,5-bisphosphate carboxylase/oxygenase large and small subunits; RPE, ribulose 5-phosphate 3-epimerase; RPI, ribose 5-phosphate isomerase; SD, shikimate dehydrogenase; SK, shikimate kinase; TAL, transaldolase; TKL, transketolase; TPI, triosephosphate isomerase; UROD, uroporphyrinogen decarboxylase; UROS, uroporphyrinogen III synthase.
The Fe–S cluster proteins are essential for various metabolic pathways in plastids, such as photosynthesis, amino acid synthesis, nitrogen and sulfur metabolism, carotenoid synthesis, vitamin and thiazole synthesis, chlorophyll synthesis and degradation, or lipoic acid synthesis (Przybyla-Toscano et al., 2018). Therefore, Suf pathway components (sufB and sufC) are among the most widespread and conserved plastid-encoded proteins. In alveolates, plastid sufB gene is proposed to play a crucial role as the constraint against loss of plastid DNA after loss of photosynthesis (Janouškovec et al., 2015, 2019).
To gain insight into the evolution of the Suf system in the non-photosynthetic dictyochophycean Pteridomonas spp., we further surveyed the transcriptome of P. danica strain PT for plastid-targeted proteins requiring Fe–S clusters (Supplementary Table S1). Intriguingly, we did not identify any of those sequences (Supplementary Figure S7), as none of the pathways reconstructed in the plastid metabolic map of P. danica PT, such as chorismate synthesis, heme synthesis, glycolysis, and the non-oxidative pentose phosphate pathway, require Fe–S clusters (Figure 5, Supplementary Table S4, and Supplementary Figure S7). Given these observations, it is most likely that Pteridomonas spp. not only lost the plastid Suf system for Fe–S cluster assembly, but also forfeited all plastid pathways requiring Fe–S clusters as a cofactor after the loss of photosynthesis. The functional reduction of plastids in the bacteriovorous Pteridomonas spp. is consistent with a previously proposed hypothesis that parasitic and phagotrophic species could achieve a deep functional reduction of plastids after loss of photosynthesis, while osmotrophic species would maintain many plastidial biosynthetic functions (Kamikawa et al., 2017; Dorrell et al., 2019).
Possible Roles of the Pteridomonas Plastid Genome
Our comparative plastid genome analyses provide insight into the evolutionary principle of organellar genome retention even after the loss of photosynthesis. Interestingly, although we sequenced the plastid genome of two non-photosynthetic species of Dictyochophyceae, Pteridomonas sp. YPF1301 and P. danica NY0221, the only genes they share encode the proteins involved in gene expression (Figure 2).
Based on the observation that sufB gene is conserved in almost all non-photosynthetic plastid genomes in Alveolata, it was proposed that this gene would be a possible constraint against loss of plastid genome in non-photosynthetic algae (Janouškovec et al., 2015). Our findings of Pteridomonas spp. plastid genomes lacking any genes for the plastid Suf system indicate that sufB gene is not always a constraint against loss of plastid DNA. Therefore, which factors might make the plastid genome indispensable in? The strain YPF1301 carries a plastid-encoded clpC gene, which was proposed as a possible constraint against loss of the apicoplast genome in the non-photosynthetic apicomplexan parasite parva (Janouškovec et al., 2015, 2019). T. parva also retains the clpC gene but it lacks sufB gene and any other genes involved in plastid metabolism (Janouškovec et al., 2015, 2019). Thus, the clpC gene might be one of factors mediating the retention of plastid DNA in Pteridomonas sp. YPF1301, possibly for correct assembly of the organellar multisubunit complex (Choquet and Vallon, 2000; Wostrikoff et al., 2004). However, this does not apply to P. danica strain NY0221, as it lacks the clpC gene, as well as any other non-housekeeping protein-coding genes.
For Pteridomonas spp., we propose that the plastid-encoded trnE gene is the most likely constraint against loss of plastid DNA (Barbrook et al., 2006; Hadariová et al., 2018). The plastid heme biosynthesis pathway begins with tRNA-Glu, synthesized from a trnE gene transcript and glutamate by tRNA-Glu synthase (Barbrook et al., 2006). This role of trnE is the only gene expression-unrelated function encoded in the entire plastid genome of P. danica strain NY0221. As trnE gene is conserved in all of the known non-photosynthetic plastid genomes (Barbrook et al., 2006; Wicke et al., 2016; Hadariová et al., 2018), the trnE-mediated constraint would be typical for all non-photosynthetic plastid genomes except apicoplasts. Genes for transcription (i.e., rpoA, rpoB, rpoC1 and rpoC2 genes) and for translation (i.e., tufA, rps, rpl, rRNA, and tRNA genes) could be basically retained to transcribe trnE gene in Pteridomonas sp. NY0221. It is suggested that some non-photosynthetic land plants need to import certain tRNA species from another compartment because they lost the corresponding tRNA genes from the plastid DNA (Barbrook et al., 2006; Wicke et al., 2016). In fact, some plastid-bearing non-photosynthetic algae and land plants completely lack plastid genomes, but retain tRNA-Glu-dependent heme biosynthesis in the plastids, suggesting import of tRNA-Glu into their plastids (e.g., Dorrell et al., 2019). After non-photosynthetic plastids establish efficient import of tRNAs, tRNA genes including trnE might become unnecessary to be present in and transcribed from the plastid DNAs, which may be followed by loss of plastid genomes, as seen in a few plastid genome-lacking algae/land plants (e.g., Dorrell et al., 2019).
The reason behind the apicoplasts being exceptional would be that the heme biosynthetic pathway in apicomplexan parasites begins in mitochondria, and it involves synthesis of the precursor of heme from glycine and succinate, but not from tRNA-Glu (Oborník and Green, 2005).
Conclusion
The data presented in this work can serve as an important insight into the scarcely studied phenomenon of loss of photosynthetic function in plastids of free-living organisms, as opposed to the considerably well-studied remnant plastids of parasites. We demonstrate that Pteridomonas spp. possess highly functionally reduced plastid genomes, lacking sufB and sufC genes, the former of which has been thought to have a role as the possible constraint against the loss of plastid genome. Instead, we propose that the main reason for the retention of the plastid genome in Pteridomonas is the tRNA-Glu gene (trnE), essential for the plastid heme biosynthesis pathway.
We also observed that Pteridomonas spp., despite their close kinship, exhibit a divergent pattern of gene loss and retention in their non-photosynthetic plastid genomes. This pattern, however, is not at all unique in secondarily non-photosynthetic algae and land plants, as it has been described in other groups – e.g., the parasitic land plants Orobanchaceae (Wicke et al., 2013), the cryptophyte genus Cryptomonas (Tanifuji et al., 2020), the diatom genus Nitzschia (Kamikawa et al., 2018) and the chlorophyte genus Prototheca (Figueroa-Martinez et al., 2015; Severgnini et al., 2018; Suzuki et al., 2018). In each of these groups except for Orobanchaceae, loss of photosynthesis occurred more than once, leading to diverse outcomes in relatively closely related organisms, although the case of Nitzschia is still controversial (Onyshchenko et al., 2019).
The findings from our study suggest that the existence of universal constraints against genome loss in non-photosynthetic plastids is unlikely. Instead, we are convinced that the fate of the organellar genome and the conservation of its functions vary with each lineage’s evolutionary background, ecological context, and a wide array of unpredictable occurrences. As a result, even in very close relatives, the vestigial plastids can be far from identical.
It is, however, important to note that these observations are based solely on our investigation of organellar genomes and transcriptomic data of a related strain, and do not fully account for the nuclear-encoded components of plastids’ functionality, which still await a detailed examination in a separate study. Additionally, the importance of trnE for plastid genome retention must be further evaluated by future biochemical studies on the acquisition of tRNA-Glu for heme biosynthesis in completely DNA-deprived vestigial plastids (Oborník and Green, 2005; Barbrook et al., 2006; Wicke et al., 2016; Hadariová et al., 2018).
Data Availability Statement
Plastid genome sequences of Pteridomonas spp. were deposited to data banks under the following accession numbers (DDBJ LC580440 for Pteridomonas sp. YPF1301 and GenBank MT909785 for the Pteridomonas danica NY0221). The raw reads of YPF1301 were also deposited to DDBJ (PRJDB10481). The datasets used for the phylogenetic analyses can be found in the Supplementary Material.
Author Contributions
RK and AK conceived the project. MK, KM, and AY sequenced, assembled, and annotated plastid genomes. MK analyzed the data and performed phylogenetic analyses. AK, HM, and RK provided reagents and materials. MK, KM, AK, and RK wrote the manuscript. All the authors checked and approved the final version.
Funding
This work was in part supported by JSPS Grants-in-Aid for Scientific Research (B) awarded to RK (Grant No. 19H03274), National Science Centre, Poland (SONATA Grant Number 2016/21/D/NZ8/01288 to AK), and Polish Ministry of Science and Higher Education scholarship for outstanding young researchers to AK.
Conflict of Interest
The authors declare that the research was conducted in the absence of any commercial or financial relationships that could be construed as a potential conflict of interest.
Acknowledgments
We thank Dr. Naoji Yubuki for kindly allowing us to sequence his culture strain of Pteridomonas danica NY0221.
Supplementary Material
The Supplementary Material for this article can be found online at: https://www.frontiersin.org/articles/10.3389/fpls.2020.602455/full#supplementary-material
Footnotes
- ^ http://hannonlab.cshl.edu/fastx_toolkit/
- ^ http://www.bioinformatics.babraham.ac.uk/projects/fastqc/
- ^ https://megasun.bch.umontreal.ca/cgi-bin/dev_mfa/mfannotInterface.pl
References
Archibald, J. M. (2009). The puzzle of plastid evolution. Curr. Biol. 19, R81–R88. doi: 10.1016/j.cub.2008.11.067
Balk, J., and Schaedler, T. A. (2014). Iron cofactor assembly in plants. Annu. Rev. Plant Biol. 65, 125–153. doi: 10.1146/annurev-arplant-050213-035759
Bankevich, A., Nurk, S., Antipov, D., Gurevich, A. A., Dvorkin, M., Kulikov, A. S., et al. (2012). SPAdes: a new genome assembly algorithm and its applications to single-cell sequencing. J. Comput. Biol. 19, 455–477. doi: 10.1089/cmb.2012.0021
Barbrook, A. C., Howe, C. J., and Purton, S. (2006). Why are plastid genomes retained in non-photosynthetic organisms? Trends Plant Sci. 11, 101–108. doi: 10.1016/j.tplants.2005.12.004
Bock, R., and Khan, M. S. (2004). Taming plastids for a green future. Trends Biotechnol. 22, 311–318. doi: 10.1016/j.tibtech.2004.03.005
Cao, M., Yuan, X. L., and Bi, G. (2016). Complete sequence and analysis of plastid genomes of Pseudo-nitzschia multiseries (Bacillariophyta). Mitochondrial DNA 27, 2897–2898. doi: 10.3109/19401736.2015.1060428
Choquet, Y., and Vallon, O. (2000). Synthesis, assembly and degradation of thylakoid membrane proteins. Biochimie 82, 615–634. doi: 10.1016/S0300-9084(00)00609-X
Couturier, J., Touraine, B., Briat, J. F., Gaymard, F., and Rouhier, N. (2013). The iron-sulfur cluster assembly machineries in plants: current knowledge and open questions. Front. Plant Sci. 4:259. doi: 10.3389/fpls.2013.00259
de Koning, A. P., and Keeling, P. J. (2006). The complete plastid genome sequence of the parasitic green alga Helicosporidium sp. is highly reduced and structured. BMC Biol. 4:12. doi: 10.1186/1741-7007-4-12
DellaPenna, D., and Pogson, B. J. (2006). Vitamin synthesis in plants: tocopherols and carotenoids. Annu. Rev. Plant Biol. 57, 711–738. doi: 10.1146/annurev.arplant.56.032604.144301
Donaher, N., Tanifuji, G., Onodera, N. T., Malfatti, S. A., Chain, P. S. G., Hara, Y., et al. (2009). The complete plastid genome sequence of the secondarily Nonphotosynthetic Alga Cryptomonas paramecium: reduction, compaction, and accelerated evolutionary rate. Genome Biol. Evol. 1, 439–448. doi: 10.1093/gbe/evp047
Dorrell, R. G., Azuma, T., Nomura, M., de Kerdrel, G. A., Paoli, L., Yang, S., et al. (2019). Principles of plastid reductive evolution illuminated by nonphotosynthetic chrysophytes. Proc. Natl. Acad. Sci. U. S. A. 116, 6914–6923. doi: 10.1073/pnas.1819976116
Figueroa-Martinez, F., Nedelcu, A. M., Smith, D. R., and Reyes-Prieto, A. (2015). When the lights go out: the evolutionary fate of free-living colorless green algae. New Phytol. 206, 972–982. doi: 10.1111/nph.13279
Fukasawa, Y., Tsuji, J., Fu, S. C., Tomii, K., Horton, P., and Imai, K. (2015). MitoFates: improved prediction of mitochondrial targeting sequences and their cleavage sites. Mol. Cell. Proteomics 14, 1113–1126. doi: 10.1074/mcp.M114.043083
Gruber, A., Rocap, G., Kroth, P. G., Armbrust, E. V., and Mock, T. (2015). Plastid proteome prediction for diatoms and other algae with secondary plastids of the red lineage. Plant J. 81, 519–528. doi: 10.1111/tpj.12734
Hadariová, L., Vesteg, M., Hampl, V., and Krajčovič, J. (2018). Reductive evolution of chloroplasts in non-photosynthetic plants, algae and protists. Curr. Genet. 64, 365–387. doi: 10.1007/s00294-017-0761-0
Hall, T. A. (1999). BioEdit: a user-friendly biological sequence alignment editor and analysis program for windows 95/98/NT. Nucleic Acids Symp. Ser. 41, 95–98.
Han, K. Y., Maciszewski, K., Graf, L., Yang, J. H., Andersen, R. A., Karnkowska, A., et al. (2019). Dictyochophyceae plastid genomes reveal unusual variability in their organization. J. Phycol. 55, 1166–1180. doi: 10.1111/jpy.12904
Hiltunen, H. M., Illarionov, B., Hedtke, B., Fischer, M., and Grimm, B. (2012). Arabidopsis RIBA proteins: two out of three isoforms have lost their bifunctional activity in riboflavin biosynthesis. Int. J. Mol. Sci. 13, 14086–14105. doi: 10.3390/ijms131114086
Hörtensteiner, S., and Kräutler, B. (2011). Chlorophyll breakdown in higher plants. Biochim. Biophys. Acta 1807, 977–988. doi: 10.1016/j.bbabio.2010.12.007
Janouškovec, J., Paskerova, G. G., Miroliubova, T. S., Mikhaiiov, K. V., Birley, T., Aieoshin, V. V., et al. (2019). Apicomplexan-like parasites are polyphyletic and widely but selectively dependent on cryptic plastid organelles. Elife 8:e49662. doi: 10.7554/eLife.49662
Janouškovec, J., Sobotka, R., Lai, D.-H., Flegontov, P., Koník, P., Komenda, J., et al. (2013). Split photosystem protein, linear-mapping topology, and growth of structural complexity in the plastid genome of Chromera velia. Mol. Biol. Evol. 30, 2447–2462. doi: 10.1093/molbev/mst144
Janouškovec, J., Tikhonenkov, D. V., Burki, F., Howe, A. T., Kolísko, M., Mylnikov, A. P., et al. (2015). Factors mediating plastid dependency and the origins of parasitism in apicomplexans and their close relatives. Proc. Natl. Acad. Sci. U. S. A. 112, 10200–10207. doi: 10.1073/pnas.1423790112
Kamikawa, R., Azuma, T., Ishii, K., Matsuno, Y., and Miyashita, H. (2018). Diversity of organellar genomes in non-photosynthetic diatoms. Protist 169, 351–361. doi: 10.1016/j.protis.2018.04.009
Kamikawa, R., Moog, D., Zauner, S., Tanifuji, G., Ishida, K.-I., Miyashita, H., et al. (2017). A non-photosynthetic diatom reveals early steps of reductive evolution in plastids. Mol. Biol. Evol. 34, 2355–2366. doi: 10.1093/molbev/msx172
Kamikawa, R., Yubuki, N., Yoshida, M., Taira, M., Nakamura, N., Ishida, K.-i., et al. (2015). Multiple losses of photosynthesis in Nitzschia (Bacillariophyceae). Phycol. Res. 63, 19–28. doi: 10.1111/pre.12072
Karnkowska, A., Bennett, M. S., and Triemer, R. E. (2018). Dynamic evolution of inverted repeats in Euglenophyta plastid genomes. Sci. Rep. 8:16071. doi: 10.1038/s41598-018-34457-w
Katoh, K., and Standley, D. M. (2013). MAFFT multiple sequence alignment software version 7: improvements in performance and usability. Mol. Biol. Evol. 30, 772–780. doi: 10.1093/molbev/mst010
Kearse, M., Moir, R., Wilson, A., Stones-Havas, S., Cheung, M., Sturrock, S., et al. (2012). Geneious Basic: an integrated and extendable desktop software platform for the organization and analysis of sequence data. Bioinformatics 28, 1647–1649. doi: 10.1093/bioinformatics/bts199
Keeling, P. J. (2010). The endosymbiotic origin, diversification and fate of plastids. Phil. Trans. R. Soc. B 365, 729–748. doi: 10.1098/rstb.2009.0103
Keeling, P. J., Burki, F., Wilcox, H. M., Allam, B., Allen, E. E., Amaral-Zettler, L. A., et al. (2014). The Marine Microbial Eukaryote Transcriptome Sequencing Project (MMETSP): illuminating the functional diversity of eukaryotic life in the oceans through transcriptome sequencing. PLoS Biol. 12:e1001889. doi: 10.1371/journal.pbio.1001889
Kleffmann, T., Russenberger, D., von Zychlinski, A., Christopher, W., Sjölander, K., Gruissem, W., et al. (2004). The Arabidopsis thaliana chloroplast proteome reveals pathway abundance and novel protein functions. Curr. Biol. 14, 354–362. doi: 10.1016/j.cub.2004.02.039
Köhler, S., Delwiche, C. F., Denny, P. W., Tilney, L. G., Webster, P., Wilson, R. J. M., et al. (1997). A plastid of probable green algal origin in Apicomplexan parasites. Science 275, 1485–1489. doi: 10.1126/science.275.5305.1485
Langmead, B., and Salzberg, S. L. (2012). Fast gapped-read alignment with Bowtie 2. Nat. Methods 9, 357–359. doi: 10.1038/nmeth.1923
Lill, R. (2009). Function and biogenesis of iron-sulphur proteins. Nature 460, 831–838. doi: 10.1038/nature08301
Liu, C., Shi, L., Zhu, Y., Chen, H., Zhang, J., Lin, X., et al. (2012). CpGAVAS, an integrated web server for the annotation, visualization, analysis, and GenBank submission of completely sequenced chloroplast genome sequences. BMC Genomics 13:715. doi: 10.1186/1471-2164-13-715
Lowe, T. M., and Chan, P. P. (2016). tRNAscan-SE On-line: integrating search and context for analysis of transfer RNA genes. Nucl. Acids Res. 44, W54–W57. doi: 10.1093/nar/gkw413
Maciszewski, K., and Karnkowska, A. (2019). Should I stay or should I go? Retention and loss of components in vestigial endosymbiotic organelles. Curr. Opin. Genet. Develop. 5, 33–39. doi: 10.1016/j.gde.2019.07.013
Marin, B., Palm, A., Klingberg, M., and Melkonian, M. (2003). Phylogeny and taxonomic revision of plastid-containing Euglenophytes based on SSU rDNA sequence comparisons and synapomorphic signatures in the SSU rRNA secondary structure. Protist 154, 99–145.
Nguyen, L. T., Schmidt, H. A., von Haeseler, A., and Minh, B. Q. (2015). IQ-TREE: a fast and effective stochastic algorithm for estimating maximum-likelihood phylogenies. Mol. Biol. Evol. 32, 268–274. doi: 10.1093/molbev/msu300
Nosek, J., Novotna, M., Hlavatovicova, Z., Ussery, D. W., Fajkus, J., and Tomaska, L. (2004). Complete DNA sequence of the linear mitochondrial genome of the pathogenic yeast Candida parapsilosis. Mol. Genet. Genomics 272, 173–180. doi: 10.1007/s00438-004-1046-0
Oborník, M., and Green, B. R. (2005). Mosaic origin of the heme biosynthesis pathway in photosynthetic eukaryotes. Mol. Biol. Evol. 22, 2343–2353. doi: 10.1093/molbev/msi230
Oborník, M., and Lukeš, J. (2015). The organellar genomes of Chromera and Vitrella, the phototrophic relatives of apicomplexan parasites. Annu. Rev. Microbiol. 69, 129–144. doi: 10.1146/annurev-micro-091014-104449
Onyshchenko, A., Ruck, E. C., Nakov, T., and Alverson, A. J. (2019). A single loss of photosynthesis in the diatom order Bacillariales (Bacillariophyta). Am. J. Bot. 106, 560–572. doi: 10.1002/ajb2.1267
Przybyla-Toscano, J., Roland, M., Gaymard, F., Couturier, J., and Rouhier, N. (2018). Roles and maturation of iron–sulfur proteins in plastids. J. Biol. Inorg. Chem. 23, 545–566. doi: 10.1007/s00775-018-1532-1
Salomaki, E. D., and Kolisko, M. (2019). There is treasure everywhere: reductive plastid evolution in apicomplexa in light of their close relatives. Biomolecules 9:378. doi: 10.3390/biom9080378
Salomaki, E. D., Nickles, K. R., and Lane, C. E. (2015). The ghost plastid of Choreocolax polysiphoniae. J. Phycol. 51, 217–221. doi: 10.1111/jpy.12283
Sekiguchi, H., Moriya, M., Nakayama, T., and Inouye, I. (2002). Vestigial chloroplasts in heterotrophic stramenopiles Pteridomonas danica and Ciliophrys infusionum (Dictyochophyceae). Protist 153, 157–167. doi: 10.1078/1434-4610-00094
Severgnini, M., Lazzari, B., Capra, E., Chessa, S., Luini, M., Bordoni, R., et al. (2018). Genome sequencing of Prototheca zopfii genotypes 1 and 2 provides evidence of a severe reduction in organellar genomes. Sci. Rep. 8:14637. doi: 10.1038/s41598-018-32992-0
Suzuki, S., Endoh, R., Manabe, R. I., Ohkuma, M., and Hirakawa, Y. (2018). Multiple losses of photosynthesis and convergent reductive genome evolution in the colourless green algae Prototheca. Sci. Rep. 8:940. doi: 10.1038/s41598-017-18378-8
Tanifuji, G., Kamikawa, R., Moore, C. E., Mills, T., Onodera, N. T., Kashiyama, Y., et al. (2020). Comparative plastid genomics of Cryptomonas species reveals fine-scale genomic responses to loss of photosynthesis. Genome Biol. Evol. 12, 3926–3937. doi: 10.1093/gbe/evaa001
Terashima, M., Specht, M., and Hippler, M. (2011). The chloroplast proteome: a survey from the Chlamydomonas reinhardtii perspective with a focus on distinctive features. Curr. Genet. 57, 151–168. doi: 10.1007/s00294-011-0339-1
Turmel, M., Otis, C., and Lemieux, C. (2017). Divergent copies of the large inverted repeat in the chloroplast genomes of ulvophycean green algae. Sci. Rep. 7:994. doi: 10.1038/s41598-017-01144-1
Wick, R. R., Schultz, M. B., Zobel, J., and Holt, K. E. (2015). Bandage: interactive visualization of de novo genome assemblies. Bioinformatics 31, 3350–3352. doi: 10.1093/bioinformatics/btv383
Wicke, S., Müller, K. F., de Pamphilis, C. W., Quandt, D., Wickett, N. J., Zhang, Y., et al. (2013). Mechanisms of functional and physical genome reduction in photosynthetic and nonphotosynthetic parasitic plants of the broomrape family. Plant Cell 25, 3711–3725. doi: 10.1105/tpc.113.113373
Wicke, S., Müller, K. F., DePamphilis, C. W., Quandt, D., Bellot, S., and Schneeweiss, G. M. (2016). Mechanistic model of evolutionary rate variation en route to a nonphotosynthetic lifestyle in plants. Proc. Natl. Acad. Sci. U. S. A. 113, 9045–9050. doi: 10.1073/pnas.1607576113
Wostrikoff, K., Girard-Bascou, J., Wollman, F. A., and Choquet, Y. (2004). Biogenesis of PSI involves a cascade of translational autoregulation in the chloroplast of Chlamydomonas. EMBO J. 23, 2696–2705. doi: 10.1038/sj.emboj.7600266
Yabuki, A., and Tame, A. (2015). Phylogeny and reclassification of Hemistasia phaeocysticola (Scherffel) Elbrächter & Schnepf, 1996. J. Eukaryot. Microbiol. 62, 426–429. doi: 10.1111/jeu.12191
Yasuno, R., and Wada, H. (2002). The biosynthetic pathway for lipoic acid is present in plastids and mitochondria in Arabidopsis thaliana. FEBS Lett. 517, 110–114. doi: 10.1016/S0014-5793(02)02589-9
Keywords: heme biosynthesis, Ochrophyta, plastid genome, reductive evolution, complex plastid
Citation: Kayama M, Maciszewski K, Yabuki A, Miyashita H, Karnkowska A and Kamikawa R (2020) Highly Reduced Plastid Genomes of the Non-photosynthetic Dictyochophyceans Pteridomonas spp. (Ochrophyta, SAR) Are Retained for tRNA-Glu-Based Organellar Heme Biosynthesis. Front. Plant Sci. 11:602455. doi: 10.3389/fpls.2020.602455
Received: 03 September 2020; Accepted: 03 November 2020;
Published: 27 November 2020.
Edited by:
Miroslav Obornik, Academy of Sciences of the Czech Republic (ASCR), CzechiaReviewed by:
Zoltan Fussy, Charles University, CzechiaLudek Koreny, University of Cambridge, United Kingdom
Copyright © 2020 Kayama, Maciszewski, Yabuki, Miyashita, Karnkowska and Kamikawa. This is an open-access article distributed under the terms of the Creative Commons Attribution License (CC BY). The use, distribution or reproduction in other forums is permitted, provided the original author(s) and the copyright owner(s) are credited and that the original publication in this journal is cited, in accordance with accepted academic practice. No use, distribution or reproduction is permitted which does not comply with these terms.
*Correspondence: Anna Karnkowska, YW5rYXJuQGJpb2wudXcuZWR1LnBs; Ryoma Kamikawa, a2FtaWthd2EucnlvbWEuN3ZAa3lvdG8tdS5hYy5qcA==