- 1Division Quality and Sensory of Plant Products, Georg-August-Universität Göttingen, Göttingen, Germany
- 2Institute of Plant Biology, Biological Research Centre, Hungarian Academy of Sciences, Szeged, Hungary
- 3Leibniz Institute of Vegetable and Ornamental Crops e.V., Grossbeeren, Germany
- 4Department of Plant Biology, University of Pécs, Pécs, Hungary
Ultraviolet-B (UV-B; 280–315 nm) radiation induces the biosynthesis of secondary plant metabolites such as flavonoids. Flavonoids could also be enhanced by blue (420–490 nm) or green (490–585 nm) light. Flavonoids act as antioxidants and shielding components in the plant’s response to UV-B exposure. They are shown to quench singlet oxygen and to be reactive to hydroxyl radical. The aim was to determine whether treatment with blue or green light can alter flavonoid profiles after pre-exposure to UV-B and whether they cause corresponding biological effects in Brassicaceae sprouts. Based on their different flavonoid profiles, three vegetables from the Brassicaceae were selected. Sprouts were treated with five subsequent doses (equals 5 days) of moderate UV-B (0.23 kJ m–2 day–1 UV-BBE), which was followed with two subsequent (equals 2 days) doses of either blue (99 μmol m–2 s–1) or green (119 μmol m–2 s–1) light. In sprouts of kale, kohlrabi, and rocket salad, flavonoid glycosides were identified by HPLC-DAD-ESI-MSn. Both Brassica oleracea species, kale and kohlrabi, showed mainly acylated quercetin and kaempferol glycosides. In contrast, in rocket salad, the main flavonol glycosides were quercetin glycosides. Blue light treatment after the UV-B treatment showed that quercetin and kaempferol glycosides were increased in the B. oleracea species kale and kohlrabi while—contrary to this—in rocket salad, there were only quercetin glycosides increased. Blue light treatment in general stabilized the enhanced concentrations of flavonoid glycosides while green treatment did not have this effect. Blue light treatment following the UV-B exposure resulted in a trend of increased singlet oxygen scavenging for kale and rocket. The hydroxyl radical scavenging capacity was independent from the light quality except for kale where an exposure with UV-B followed by a blue light treatment led to a higher hydroxyl radical scavenging capacity. These results underline the importance of different light qualities for the biosynthesis of reactive oxygen species that intercept secondary plant metabolites, but also show a pronounced species-dependent reaction, which is of special interest for growers.
Introduction
Plants have photosensory mechanisms to detect ultraviolet-B (UV-B; 280–315 nm) radiation (Heijde and Ulm, 2012; Jenkins, 2014) and therefore protect and repair sensitive targets from direct and indirect UV-induced injury (Britt, 1996; Jansen and Bornman, 2012). One line of defense is the increased biosynthesis of flavonoids and hydroxycinnamic acids (Jansen et al., 2008; Neugart and Schreiner, 2018). However, the impact of moderate UV-B, below ecologically summer day doses, during cultivation is less investigated (Schreiner et al., 2012; Neugart and Schreiner, 2018). Changes in the biosynthesis of flavonoid glycosides and hydroxycinnamic acid derivatives in response to UV-B depends on the chemical structure of the compounds (Olsson et al., 1998; Neugart et al., 2012a, 2014; Harbaum-Piayda et al., 2016). Light-emitting diodes (LEDs) are the most innovative light sources and create new possibilities for indoor cultivation. Blue and red LEDs were the first choice for manufacturers as these wavelengths are efficiently absorbed by chlorophylls (Kaiser et al., 2019). It is a widespread but erroneous belief that plants simply prefer blue and red light and poorly absorb green light (Smith et al., 2017). Less than 50% of green light (500–600 nm range) is reflected by plant chloroplasts, and green light reaches deeper leaf tissues more efficiently than blue or red wavelengths, which are shielded with chlorophyll-containing layers (Terashima et al., 2009). It was shown recently that flavonoid biosynthesis can be enhanced by blue light (420–490 nm) or green light (490–585 nm), but species differ in their response. While blue light treatment (30–100 μmol m–2 s–1) of lettuce resulted in higher total phenols (Johkan et al., 2010; Samuoliene et al., 2017), higher doses of blue light within the light spectrum (200 μmol m–2 s–1) resulted in a reduction of total phenolics in lamb’s lettuce (Wojciechowska et al., 2015). For green light treatment (30 μmol m–2 s–1) of lettuce, an increase of total phenolics was reported by the same authors. While UV-B radiation activates the UVR8 photoreceptor, blue and partly green light trigger the cryptochromes among other blue light receptors (Fuglevand et al., 1996; Heijde and Ulm, 2012; Jenkins, 2014; Tissot and Ulm, 2020). Extraordinarily, the light signaling of these photoreceptors, both UVR8 and cryptochromes, is mediated through COP1 operating as signaling center (Lau and Deng, 2012). While indoor farming offers the chance to tailor make light spectra in the visible spectrum, UV LEDs are not commonly used due to higher costs. One idea to overcome this issue is to have UV bulbs for the UV-B treatment and later on use visible light to stabilize or further increase the UV effects. There are no information how structurally different flavonol glycosides and hydroxycinnamic acids respond to different light qualities after pre-exposure with UV-B. Therefore, some basic principles need to be investigated for the later development of recipes.
The effect of light conditions during growth on flavonoids is of special importance, because these compounds act as antioxidants and shielding components in the plant’s response to UV-B exposure (Edreva, 2005; Agati et al., 2013; Waterman et al., 2017) and are well-known antioxidants in human nutrition (Pan et al., 2010). Flavonoids are structurally diverse polyphenols that are ubiquitous in plants where they naturally occur as glycosides. Brassica vegetable leaves have high concentrations of flavonoids and hydroxycinnamic acids (Neugart et al., 2012a). The main flavonoids of Brassicaceae are quercetin and kaempferol glycosides. In kale, kaempferol glycosides show different antioxidant activities dependent on their chemical structure (Fiol et al., 2012). Rocket salad is known for high concentrations of quercetin glycosides (Martínez-Sánchez et al., 2007).
Flavonoids, as antioxidants, can quench singlet oxygen in vivo (Triantaphylides and Havaux, 2009) and are also reactive to other reactive oxygen species (ROS) such as hydroxyl radicals (Husain et al., 1987). Glycosylation reduces the antioxidant activity of flavonoids and affects the accumulation, stability, and solubility of flavonoids (Gachon et al., 2005; Bowles et al., 2006). The intracellular accumulation at sites of ROS production underlines the important antioxidant properties of flavonoids (Hernandez et al., 2009). Remarkably, it was previously shown that hydroxycinnamic acids act as scavengers of ROS induced by UV-B radiation (Edreva, 2005). Many studies investigated the overall antioxidant activity (such as TEAC) among different Brassica vegetables (Podsedek, 2007) or focus on total phenolic content and specific radical scavenging capacities (Li et al., 2011). Nevertheless, there are less scavenging information on plant-specific ROS such as singlet oxygen and hydroxyl radical and their correlation to flavonoid glycosides.
The ability of plants to accumulate protective UV-absorbing compounds such as flavonoids in response to days or weeks of exposure to UV is well known (Searles et al., 2001). It was shown that epidermal flavonoids are more important in response to UV-A and UV-B than mesophyll flavonoids and even epidermal hydroxycinnamic acids (Burchard et al., 2000; Kolb et al., 2001). However, it was also shown that flavonoids can absorb light in the lower wavelength region of the visible spectrum, e.g., blue light (Gitelson et al., 2017), which can result in higher flavonoid concentrations (Matysiak and Kowalski, 2019). In addition, the absorption spectra of flavonoids depend on their chemical structure (Stochmal and Oleszek, 2007). Nevertheless, flavonoids such as rutin (absorption maxima at 260 and 360 nm) and hydroxycinnamic acids such as chlorogenic acid (absorption maximum at 320 nm) are relevant UV-absorbing compounds (Solovchenko and Merzlyak, 2008). How specific complex flavonoid glycosides and hydroxycinnamic acids contribute to the absorption capacity is still largely unclear, but will support non-destructive measurements of flavonoids in the future.
We hypothesize that (i) UV-B pre-exposure leads to an increase in flavonoid glycosides and hydroxycinnamic acids with high antioxidant activity, (ii) blue and green light after UV-B pre-exposure contributes to obtain the higher concentrations of flavonoid glycosides and hydroxycinnamic acids, (iii) these light-induced changes in flavonoid and hydroxycinnamic acid profiles result in an increased ROS scavenging capacity and increased absorption ability, and (iv) there are specific species within the Brassicaceae family. This knowledge will be transferable to recipes for indoor use and subsequent storage conditions, as it is important to increase not only the yield but also phenolic compounds that can act as color, flavor, and health-promoting compounds.
Materials and Methods
Three Brassicaceae vegetables, namely, kale (Brassica oleracea var. sabellica) kohlrabi (Brassica oleracea var. gongylodes L.), and rocket salad (Diplotaxis tenuifolia), were cultivated in a controlled environment, in a greenhouse, for the UV treatment. Afterward, they were transferred to climate chamber for the LED treatment. The first part of this experiment was carried out in the greenhouse at Grossbeeren (Germany, 52.37°N 13.33°E) under partly overcast skies with glass that is blocking UV. PAR was on average 306 μmol m–2 s–1 with intra- and inter-day variations [natural sunlight plus Lamps (SON-T Agro 400)]. Temperature was set at 22°C from 8:00 to 21:00 and 18°C from 21:00 to 8:00. The sprouts (3 days after sowing) were treated with five subsequent doses of moderate UV-B provided by UV-B fluorescence light sources (TL 40W 12 RS, Philips, Hamburg, Germany). The UV-B dose was 0.23 kJ m–2 day–1 UV-BBE per day (total: 1.15 kJ m–2 day–1 UV-BBE) at a distance of 60 cm from 10:30 to 12:00 each day. The lamps were moving above the samples being applied to an irrigation system, which at the same time results in an balanced UV radiation treatment among the samples. To calculate the biologically effective UV-B, the generalized plant action spectrum (Caldwell, 1971) was used. UV-B radiation was determined using an UV-B sensor (type DK-UVB 1.3-051, deka Sensor + Technologie, Teltow, Germany) with a spectral range of 265–315 nm. Afterward, a subset of the 8-day-old sprouts pre-treated with UV got two subsequent doses (6 h each from 8:00 to 14:00) of blue (450 nm–99 μmol m–2 s–1) or green (510 nm–119 μmol m–2 s–1) light (Figure 1). In addition to the blue or green LEDs, white LEDs (400–700 nm) were present in the climate chamber with 100 μmol m–2 s–1 from 6:00 to 18:00 to ensure further growth of the plants (Figure 1). Temperature was 22°C from 8:00 to 21:00 and 18°C from 21:00 to 8:00. A sampling fraction of three biological replicates, each one tray of sprouts grown from 3 g of seeds, was harvested after an acclimatization period of 24 h after the last treatment.
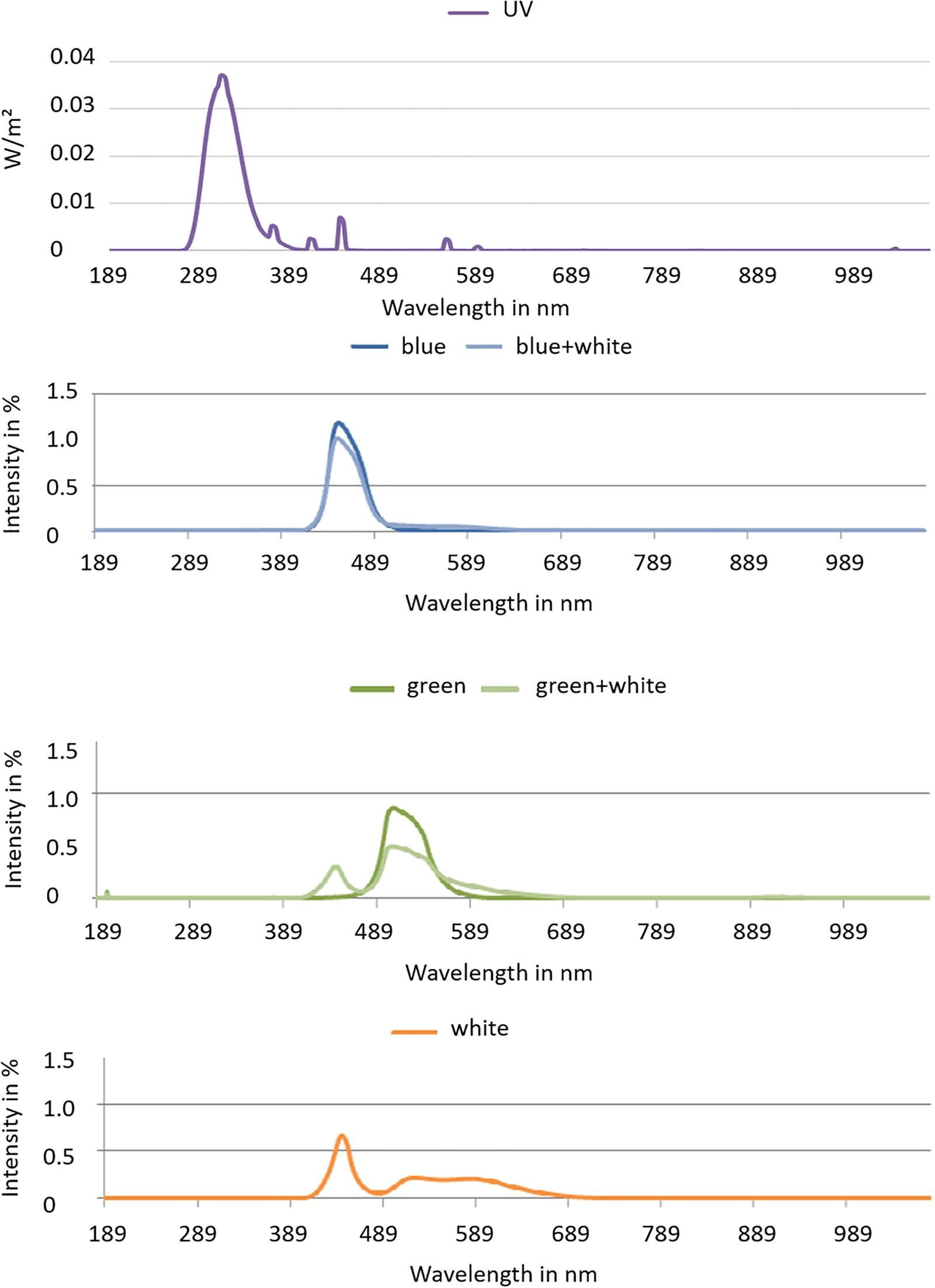
Figure 1. Spectra of blue and green LEDs with and without white light and the white light spectrum used in the climate chambers.
Flavonoid glycosides and hydroxycinnamic acids were identified and quantified by HPLC-ESI-MSn (Neugart et al., 2012b). The modified method follows in brief, and lyophilized samples (20 mg) were extracted with 1.2 ml of 60% aqueous methanol in three steps. The extract was evaporated to dryness and the residue was dissolved in 200 μl of 10% methanol and then filtered through a Spin-X filter (cellulose acetate membrane filter) for the HPLC analysis. An HPLC series 1100 from Agilent (Waldbronn, Germany) consisting of a degasser, binary pump, autosampler, column oven, and photodiode array detector was used to quantify the flavonoid glycosides. For identification purposes, an ion trap mass spectrometer (Agilent series 1100 MSD) was used with ESI as an ion source in negative ionization mode. Nitrogen was the dry gas (12 L/min, 350°C) and nebulizer gas (40 psi). Helium was the inert collision gas in the ion trap. The following gradient was used for eluent B (100% acetonitrile) at a temperature of 30°C: 5–7% (0–12 min), 7–9% (12–25 min), 9–12% (25–45 min), 12–15% (45–100 min), 15% isocratic (100–150 min), 15–50% (150–155 min), 50% isocratic (155–165 min), 50–5% (165–170 min), 5% isocratic (170–175 min). The flow was performed using 0.4 ml min–1, and the measured detector wavelength for the quantification was set at 370 nm for non-acylated flavonol glycosides and 330 nm for acylated flavonol glycosides. The standards quercetin-3-O-glucoside and the corresponding 3-O-glucosides of kaempferol and isorhamnetin (Carl Roth GmbH, Karlsruhe, Germany) were used to obtain an external calibration curve in the range of 0.1–10 mg 100 ml–1.
Singlet oxygen (1O2) and hydroxyl radical (∙OH) scavenging capacities by photospectrometric methods (Majer and Hideg, 2012). In singlet oxygen scavenging capacity measurements, p-nitrosodimethylaniline (RNO) is bleached by a product of the reaction between singlet oxygen and histidine, which can be followed by monitoring the decrease in RNO absorption at 440 nm. Reaction mixtures contained 10 mM methylene blue, 15 mM RNO, and 10 mM histidine in 50 mM phosphate buffer (pH 7.0). Singlet oxygen scavenging capacities of plant extracts were measured based on their abilities to inhibit the above reaction and were quantified as millimolar Trolox equivalents per gram of dry matter. The hydroxyl radical scavenging capacity was determined by measuring the ability of leaf extracts to inhibit the formation of the strongly fluorescent 2-hydroxyterephthalate (HTPA) in a reaction between terephthalate (1,4-benzenedicarboxylic acid, TPA) and hydroxyl radical. HTPA fluorescence was measured at room temperature with a Quanta Master QM-1 spectrofluorometer (Photon Technology Inc., Birmingham, NJ, United States), using 315-nm excitation and 420-nm emission. The 2.5-ml reaction mixture contained 500 mM TPA, 10 mM EDTA, 10 mM FeSO4, 100 mM AA, and 100 mM H2O2 in a 50 mM Na-phosphate buffer (pH 7.2). Hydroxyl radical scavenging of each plant extract was characterized by its half-inhibitory concentration on HTPA formation. The method was calibrated with ethanol, which is a strong hydroxyl radical scavenger, and specific hydroxyl radical neutralizing capacities of leaf extracts were given as millimolar ethanol equivalents per gram of dry matter.
The absorption spectra of the extracts were determined according to Mirecki and Teramura (1984). Twenty milligrams of freeze-dried powder was extracted with acidified methanol and used for spectrophotometric determination of total UV-B absorption (A280–315nm) which is linked to the phenolic content. All absorption measurements were carried out using a Shimadzu UV-1601 spectrophotometer.
All measurements were done on three independent biological replicates and two technical replicates in the lab.
To analyze variance (ANOVA), Tukey’s Honest Significant Difference (HSD) test was used to calculate significant differences at a significance level of p ≤ 0.05.
Results
Effect of Light Quality on Structurally Different Flavonoid Glycosides and Hydroxycinnamic Acids
The flavonol glycoside and hydroxycinnamic acid profiles varied within the three Brassicaceae vegetables. In total, kale (Figure 2 and Supplementary Table 1) and kohlrabi (Figure 2 and Supplementary Table 2) were characterized by acylated quercetin and kaempferol glycosides based on the 3-O-sophoroside-7-O-glycosides. Rocket salad (Figure 3 and Supplementary Table 3) had mainly acylated quercetin glycosides based on the quercetin-3,3′,4′-triglucoside. Generally, the response of flavonol glycosides and hydroxycinnamic acids to the different light treatments was distinctly dependent on their chemical structure. In kale, caffeoylquinic acid and feruloyl glucoside were increased by UV-B exposure and remain high after blue light treatment but not after green light treatment (Figure 2 and Supplementary Table 1). Interestingly, gentiobiosides acylated only with sinapoyl residues were found in high concentrations in kale plants, but mixtures of sinapoyl- and feruloyl-acylated gentiobiosides appeared in high concentrations in plants exposed to UV-B and subsequently to blue light treatment. For non-acylated flavonol glycosides, including quercetin, kaempferol, and isorhamnetin glycosides, an increase was found after UV-B exposure and was mostly stable after two doses of blue light but not green light (Figure 2 and Supplementary Table 1). The same was true for most of the acylated quercetin and kaempferol glycosides (Figure 2 and Supplementary Table 1). Nevertheless, for quercetin-3-feruloyl-sphoroside-7-glucoside, kaempferol-3-caffeoyl-sophoroside-7-glucoside, and kaempferol-3-caffeoyl-sophoroside-7-diglucoside, the highest concentrations were found after UV-B exposure followed by blue light treatment. Although kale and kohlrabi showed similar flavonoid and hydroxycinnamic acid profiles, the response of both to treatment was species-specific. In kohlrabi, hydroxycinnamic acids were rarely affected by UV-B exposure (Figure 2 and Supplementary Table 2). However, hydroxycinnamic acid monoglycosides, namely, caffeoyl-glucoside, sinapoyl-glucoside, and disinapoyl-glucoside, were increased after UV-B exposure followed by the exposure to green light. In contrast, mainly non-acylated quercetin glycosides (quercetin-3-sophoroside and quercetin-3-triglucoside) were increased by two- to threefold in response to UV-B (Figure 2 and Supplementary Table 2). Blue as well as green light after the UV-B exposure resulted in a decrease of the non-acylated quercetin glycosides. Furthermore, in kohlrabi, the most acylated quercetin and kaempferol triglycosides and tetraglycosides were increased by UV-B exposure and were still high after two subsequent doses of blue light, but no green light (Figure 2 and Supplementary Table 2). Nevertheless, quercetin and kaempferol pentaglycosides were not affected by UV-B exposure or blue and green light. The values are still high after two subsequent doses of blue light but not with green light instead. In rocket salad, several flavonol glycosides and hydroxycinnamic acids were increased by 1.2- to 2-fold due to the exposure with UV-B, e.g., quercetin-3′,4′-diglucoside-3′-(6-caffeoyl-glucoside) (Figure 3 and Supplementary Table 3). The values are still high after two subsequent doses of blue light, but not with green light instead.
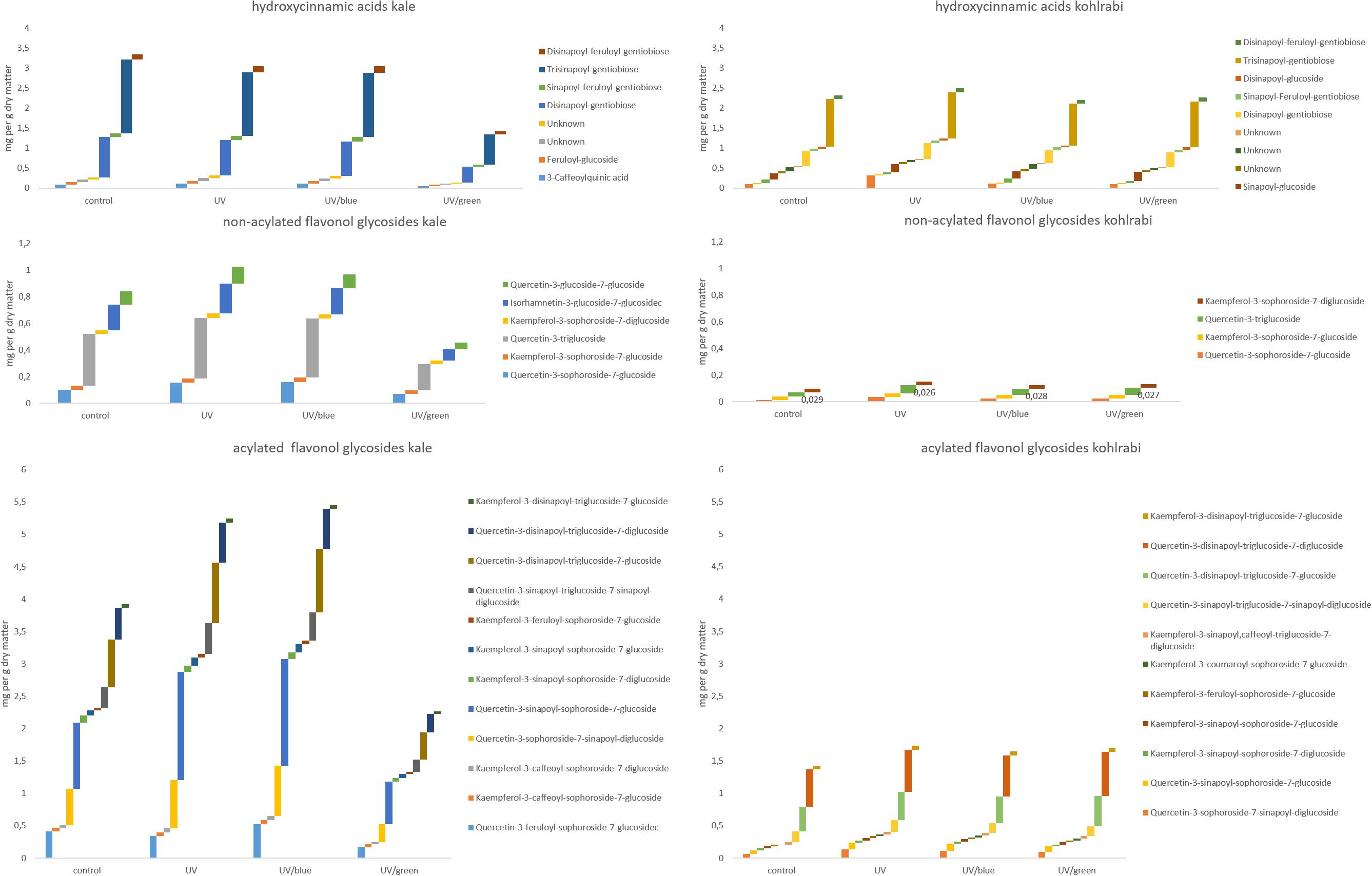
Figure 2. Hydroxycinnamic acids, non-acylated flavonol glycosides, and acylated flavonol glycosides of kale and kohlrabi grown under different irradiation qualities (control, UV, UV plus blue, and UV plus green). For statistics, see Supplementary Tables 1, 2.
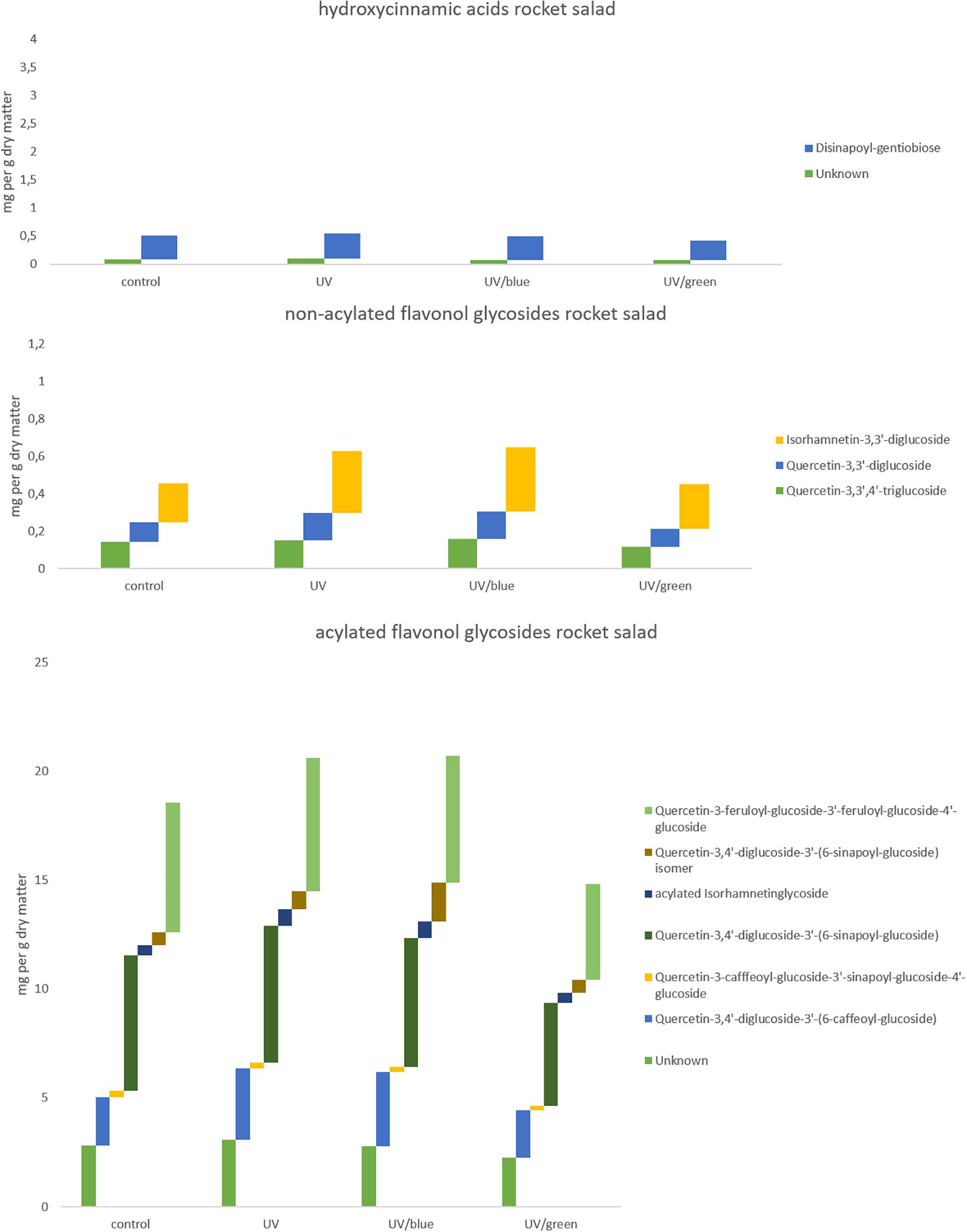
Figure 3. Hydroxycinnamic acids, non-acylated flavonol glycosides, and acylated flavonol glycosides of rocket salad grown under different irradiation qualities (control, UV, UV plus blue, and UV plus green). For statistics see Supplementary Table 3.
Effect of Light Quality on ROS Scavenging Capacity
In the present study, kale and kohlrabi, in general, showed a higher singlet oxygen scavenging capacity than rocket salad (Figure 4). Both kale and kohlrabi belong to the Brassica genus while rocket salad is Diplotaxis species. The results reveal a strong species-specific response of sprouts to the different light qualities regarding singlet oxygen scavenging capacity. For hydroxyl radical scavenging capacity, no species-specific response was detected in the Brassica sprouts. UV-B exposure resulted in higher singlet oxygen scavenging capacity in rocket but not kale and kohlrabi (Figure 4). Blue light treatment following the UV-B exposure did not result in a significant increase of singlet oxygen scavenging for kale and rocket but in a trend toward an increase. Green light treatment after UV-B exposure showed similar singlet oxygen scavenging as the control for all three Brassicaceae sprouts. The hydroxyl radical scavenging capacity was independent from the light quality except for kale, where an exposure with UV-B followed by a blue light treatment led to a higher hydroxyl radical scavenging capacity. In kale, non-acylated as well as acylated quercetin and kaempferol glycosides of very different chemical structures contributed to the singlet oxygen-scavenging capacity including kaempferol-3-caffeoyl-sophoroside-7-glucoside and its corresponding tetraglycoside (Table 1). In kohlrabi, however, there was not a single compound that contributed significantly to singlet oxygen scavenging capacity (Table 2), whereas in rocket salad, almost all the compounds measured, most of them quercetin glycosides and disinapoyl and trisinapoyl geniobiosides, have contributed to the singlet oxygen scavenging capacity (Table 3). None of the Brassicaceae species studied had a large amount of compounds with hydroxyl radical scavenging capacity (Tables 1–3).
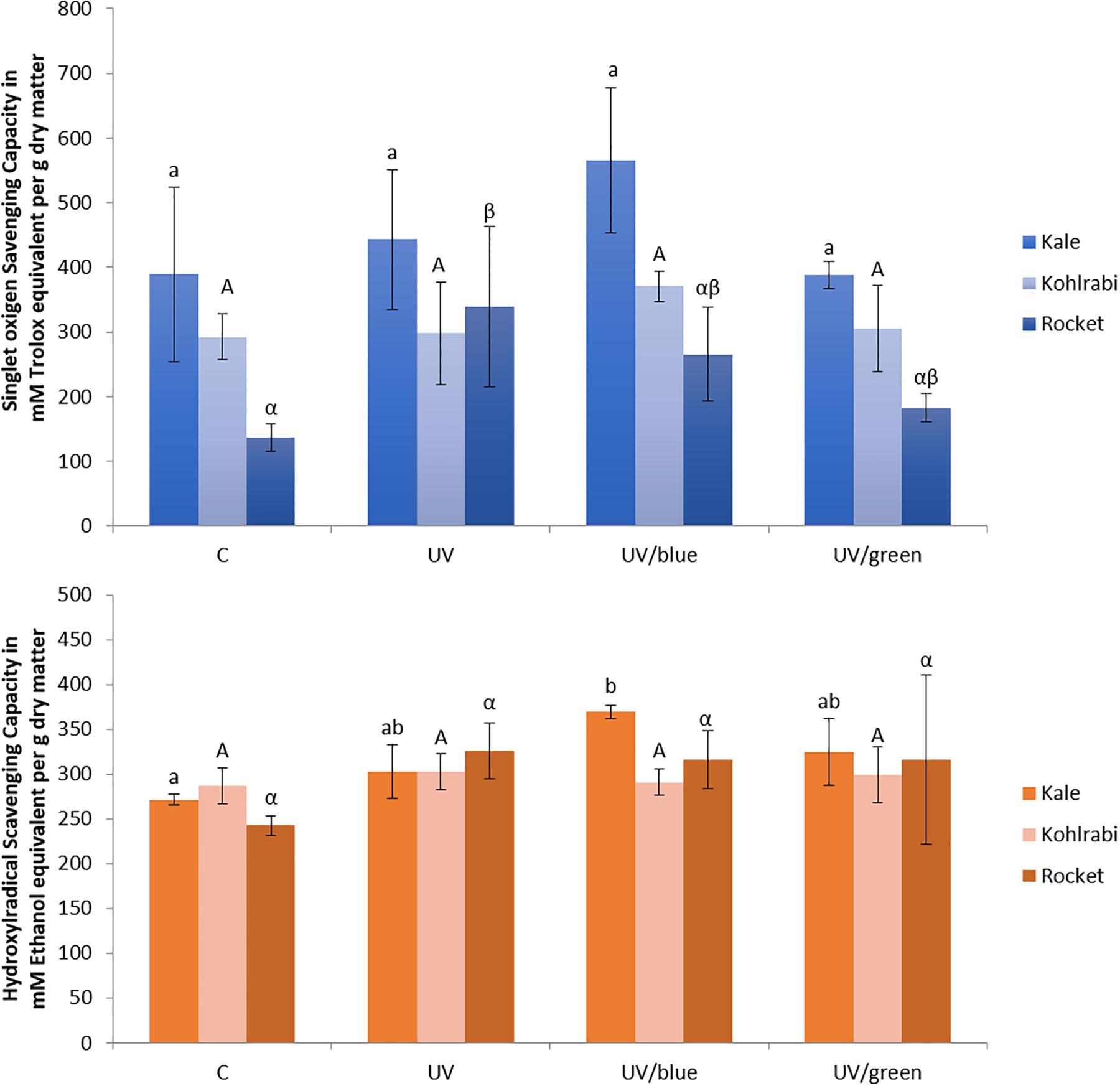
Figure 4. Singlet oxygen scavenging capacity and hydroxyl radical scavenging capacity of three Brassiceae species grown under different irradiation qualities (control, UV plus blue, and UV plus green). Different letters represent significant differences (p ≤ 0.05 by Tukey’s HSD test) between the treatment (n = 3).
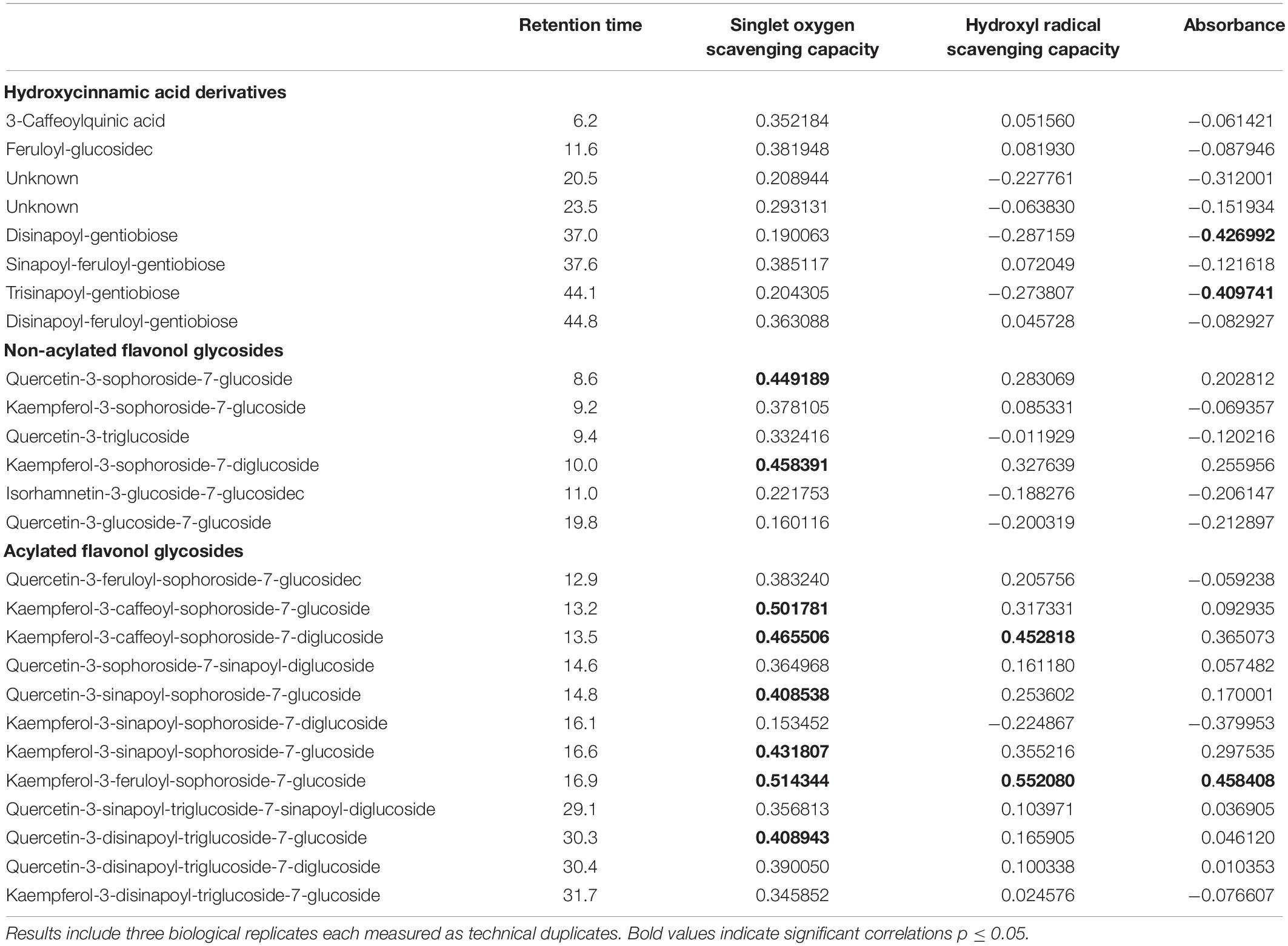
Table 1. Correlation of hydroxycinnamic acid derivatives and flavonol glycosides (mg g–1 dry weight) and biological functions as antioxidants (singlet oxygen scavenging capacity and hydroxyl radical scavenging capacity) and ultraviolet radiation shielding (absorbance) in kale.
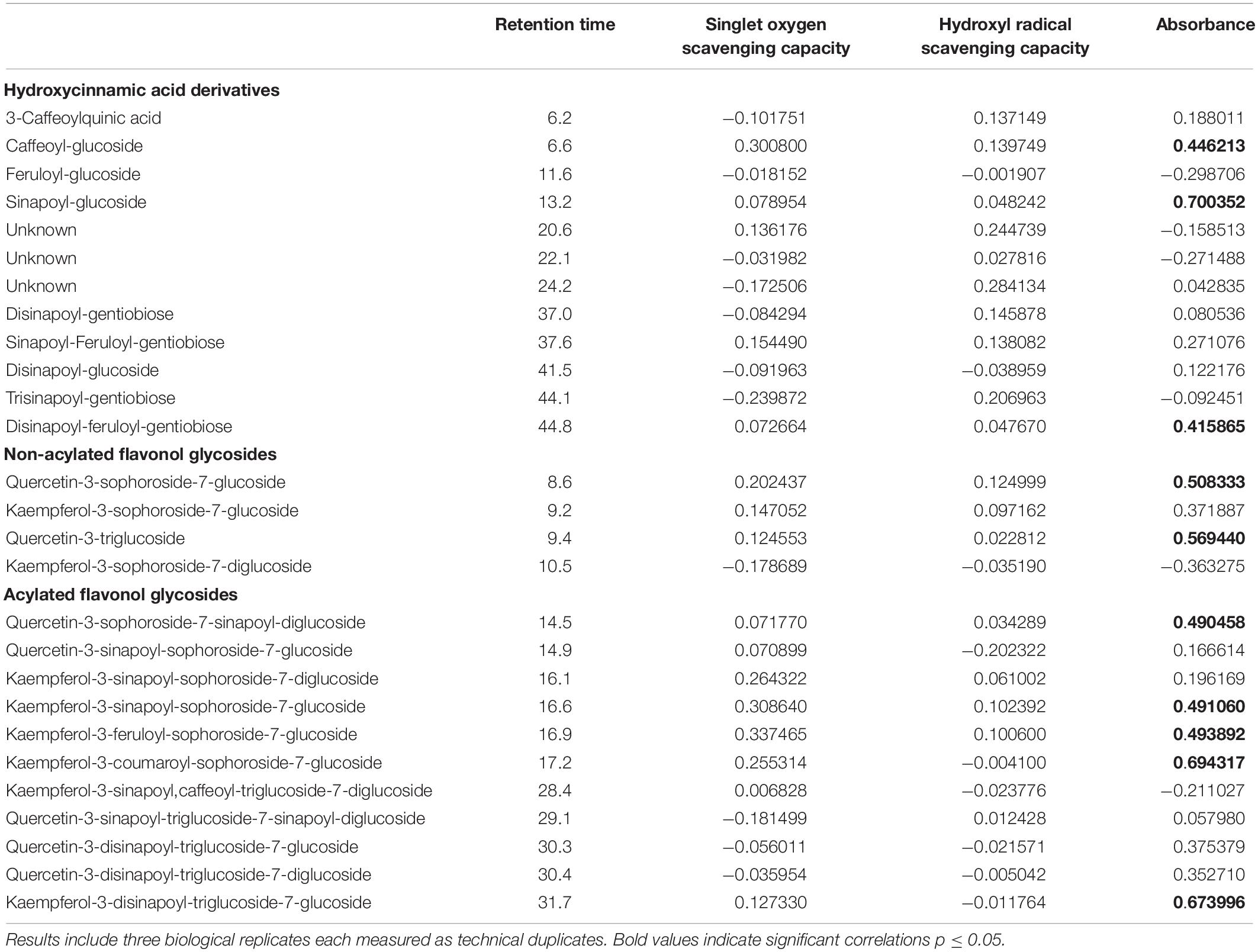
Table 2. Correlation of hydroxycinnamic acid derivatives and flavonol glycosides (mg g–1 dry weight) and biological functions as antioxidants (singlet oxygen scavenging capacity and hydroxyl radical scavenging capacity) and ultraviolet radiation shielding (absorbance) in kohlrabi.
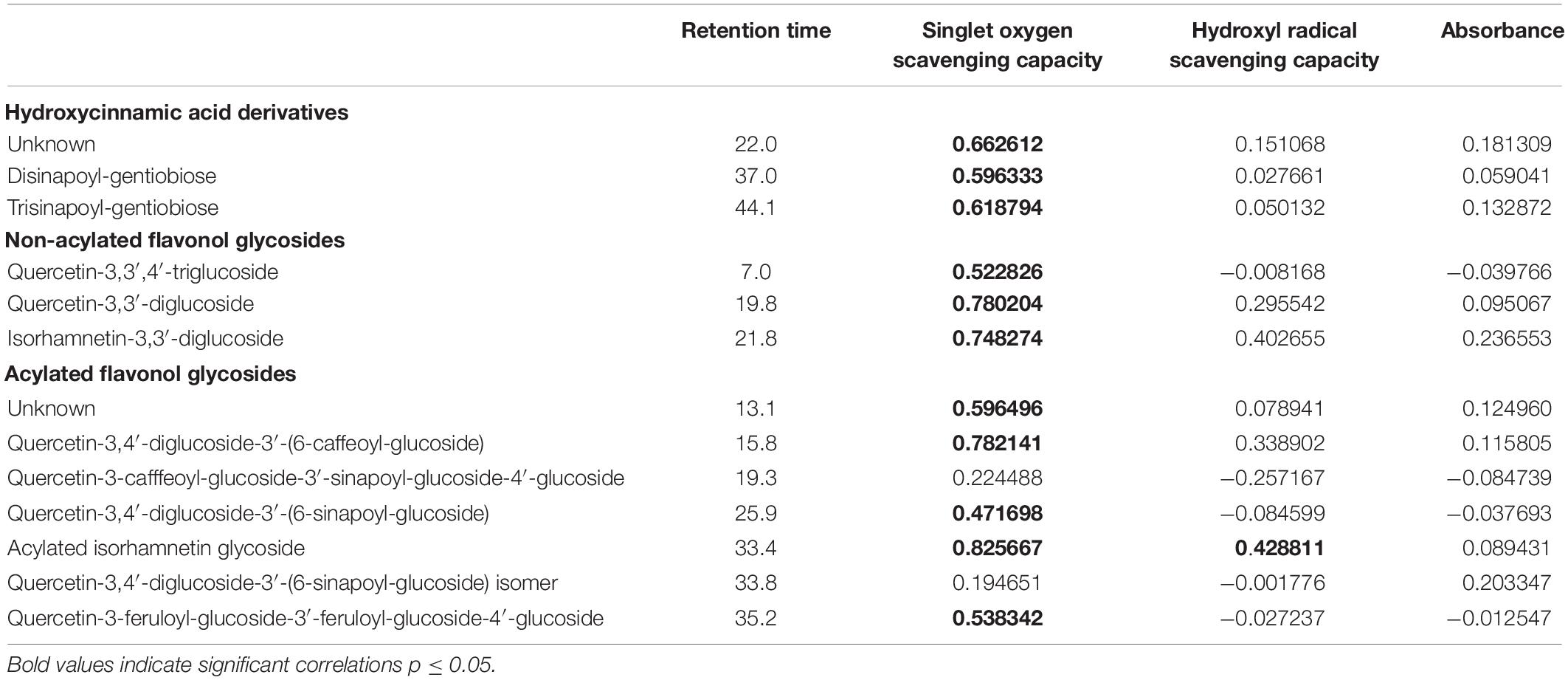
Table 3. Correlation of hydroxycinnamin acid derivatives and flavonol glycosides (mg g–1 dry weight) and biological functions as antioxidants (singlet oxygen scavenging capacity and hydroxyl radical scavenging capacity) and ultraviolet radiation shielding (absorbance) in rocket salad.
Effect of Light Quality on Absorption Ability
The extracts of Brassicaceae vegetables showed lower absorption intensities in kohlrabi compared to kale and rocket (Figure 5). In kale, the absorption was higher in UV-B-exposed plants and was additionally increased by the blue and green light treatment, whereas in kohlrabi, there was a clear UV-B exposure effect but no further increase following the blue and green light treatment. In rocket salad, the absorption was only slightly increased by UV-B exposure and not further increased by blue or green light treatment. Nevertheless, for kale, negative correlations of absorption and the hydroxycinnamic acid derivatives disinapoyl-gentiobioside as well as trisinapoly gentiobioside were found, whereas kaempferol-3-feruloyl-sophorroside-7-glucoside was the only flavonol glycoside to contribute to the absorption (Table 1). In contrast, in kohlrabi caffeoyl-glucoside, sinapoyl-glucoside and disnapoyl-feruloyl-gentiobioside contributed to the higher absorption alongside with several non-acylated quercetin glycosides and acylated quercetin and kaempferol glycosides (Table 2). In rocket salad, none of the soluble flavonol glycosides and hydroxycinnamic acid derivatives contributed to absorbance changes, which were very small (Table 3).
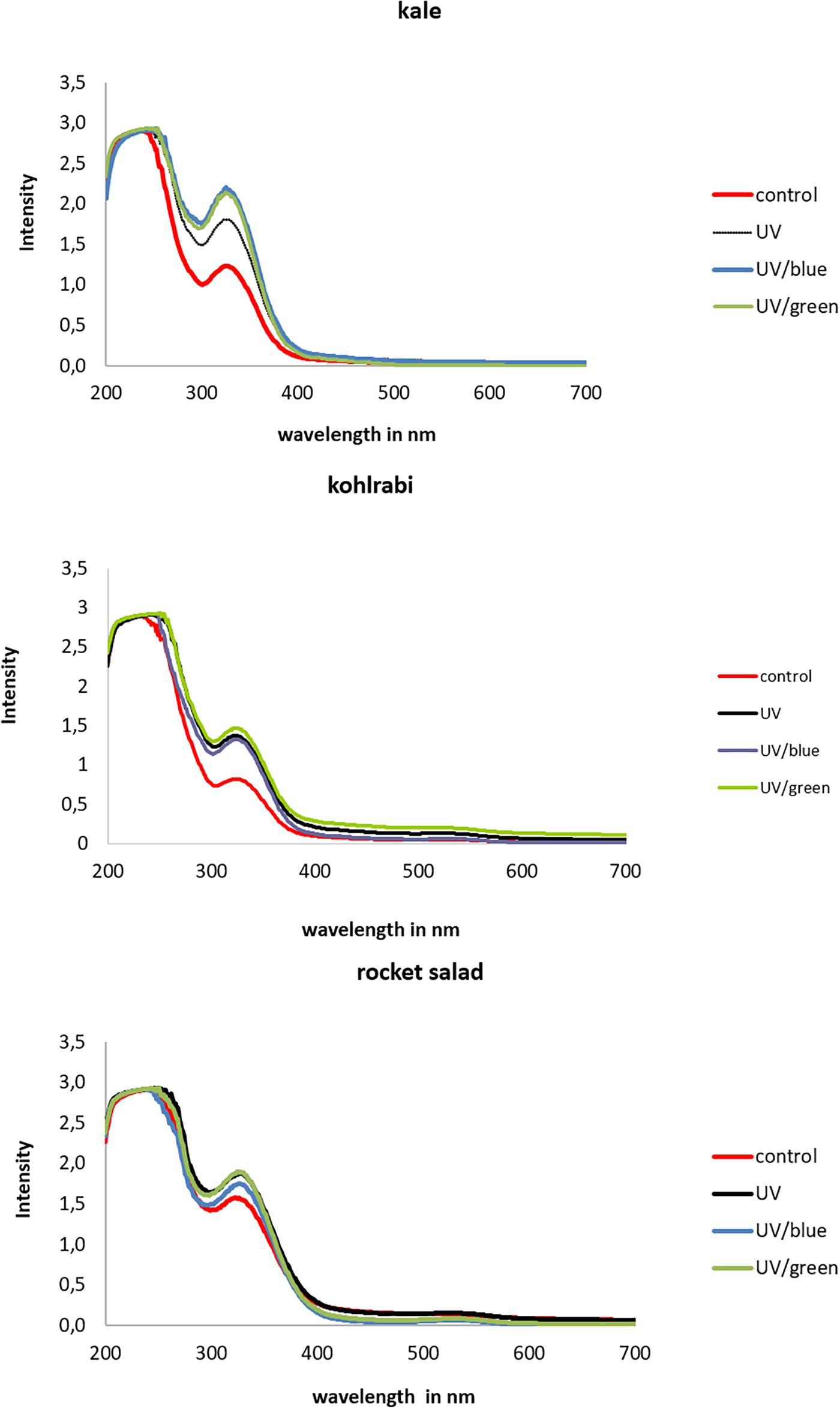
Figure 5. Absorption of three Brassiceae species grown under different irradiation qualities (control, UV, UV plus blue, and UV plus green).
Discussion
Effect of Light Quality on Structurally Different Flavonoid Glycosides and Hydroxycinnamic Acids
The results on the chemical structures identified and quantified are in line with the literature on Brassica species (Ferreres et al., 2009; Lin and Harnly, 2009; Olsen et al., 2009; Schmidt et al., 2010; Cartea et al., 2011; Kyriacou et al., 2020) and rocket salad (Martínez-Sánchez et al., 2007; Pasini et al., 2012; Bell and Wagstaff, 2014). UV-B exposure can lead to an up to 2 fold increase of phenolic compounds in Brassica species and rocket salad as previously found in the literature (Reifenrath and Mueller, 2007; Harbaum-Piayda et al., 2010; Mormile et al., 2019; Table 4). Additionally, an effect dependent on the specific chemical structure as well as the dose and duration of the UV-B treatment was shown (Olsson et al., 1998; Neugart et al., 2012a, 2014; Groenbaek et al., 2014; Rechner et al., 2016). The present study shows that kaempferol glycosides such as kaempferol-3-caffeoyl-sophoroside-7-glucoside of Brassica species and quercetin glycosides in rocket salad are involved in the UV and blue light response. Nevertheless, blue light was not able to further increase flavonoid glycosides and hydroxycinnamic acids in Brassicaceae sprouts. However, in broccoli, kaempferol-3-feruloyl-sphoroside-7-glucoside and kaempferol-3-caffeoyl-sophoroside-7-glucoside were increased after blue light treatment alone, without any UV pre-treatment (Rechner et al., 2017). Blue light was able to increase total phenolics of lettuce (Johkan et al., 2010). Furthermore, in basil, lettuce and arugula flavonoid glycosides and hydroxycinnamic acids reacted to blue light based on their chemical structure, mainly based on the aglycone as trigger (Taulavuori et al., 2016, 2018). An effect of the glycosylation pattern or the acylated hydroxycinnamic acid has not been found in the present study but was previously discussed for kale juvenile plants or broccoli juvenile plants treated with UV-B (Neugart et al., 2014; Rechner et al., 2016). Furthermore, blue light was able to increase sinapoyl derivatives and kaempferol glycosides of Arabidopsis thaliana with a clear pattern that kaempferol glycosides including glucose are increased, while those not including glucose but rhamnose are not (Brelsford et al., 2019). The fact that complex flavonoid glycosides, e.g., quercetin and kaempferol pentaglycosides from kohlrabi, are not affected by the light treatment was previously found in broccoli (Rechner et al., 2016). It is worth noting that the blue-to-red ratio should be 1:3 or higher to increase flavonoid glycosides and hydroxycinnamic acids (Pennisi et al., 2019). Such ratios were not reached in the present study and might explain why no further increase of flavonoid glycosides and hydroxycinnamic acid derivatives was found. However, green light seems to reduce plant response in general (Folta and Maruhnich, 2007), even though there had also been reports that green light in addition to blue and red light can increase total phenols in lettuce (Bian et al., 2018). In accordance with the results of the present study in broccoli plants radiated with green light without any treatment of UV-B, structurally different flavonoid glycosides and hydroxycinnamic acids were in the same concentrations of the control plants (Rechner et al., 2017). This is true although green light (119 μmol m–2 s–1) had a higher intensity than blue light (99 μmol m–2 s–1) due to technical limitations in the climate chamber. Generally, there is the assumption that higher intensities of blue and green light are needed to actually increase flavonoid glycosides and hydroxycinnamic acid derivatives.
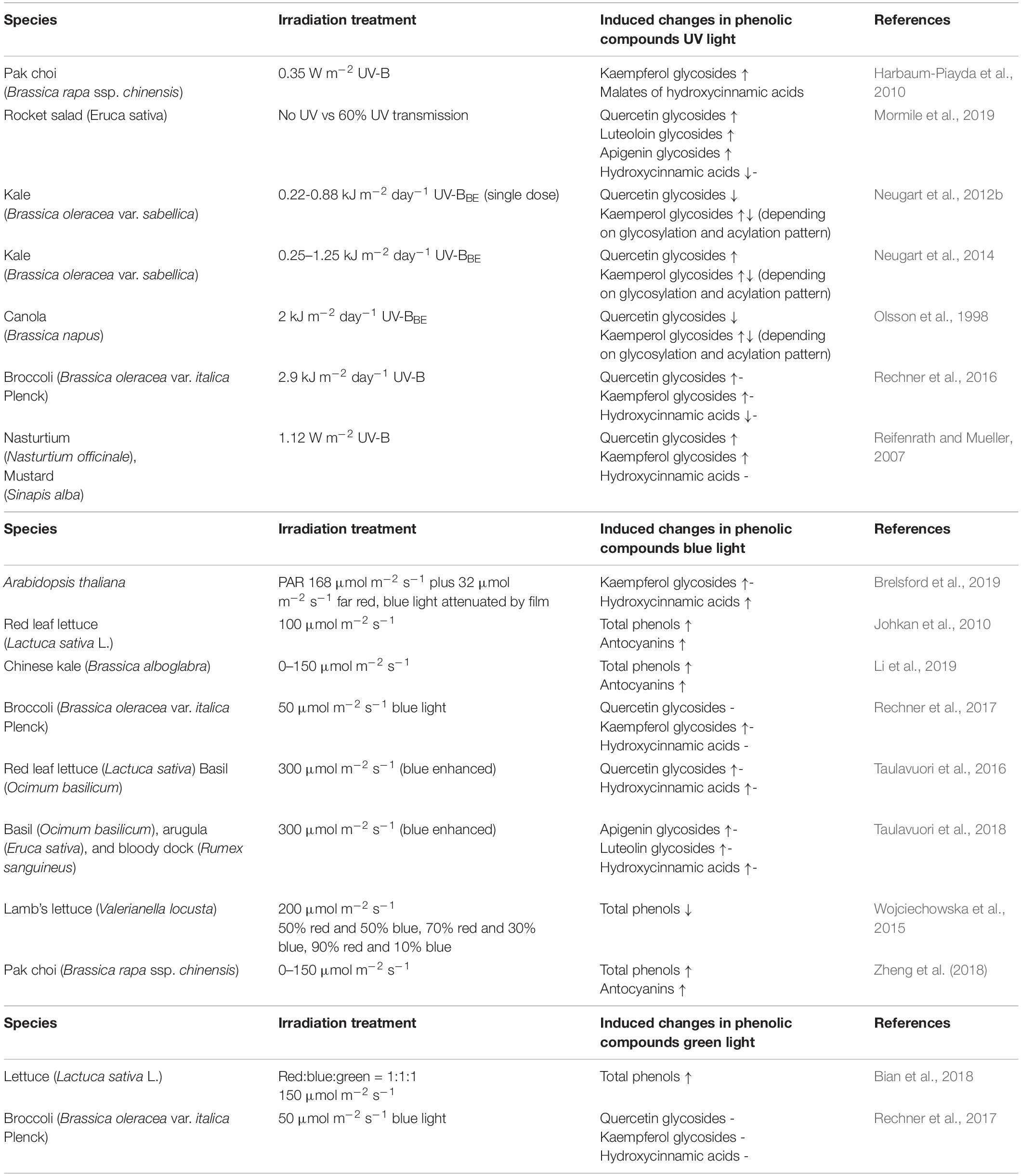
Table 4. Overview of the effects of UV, blue, and green light on flavonoid glycosides and hydroxycinnamic acid derivatives.
Even though kale and kohlrabi had similar flavonoid and hydroxycinnamic acid profiles, the response of both to the treatment was species-specific (Figure 1). In Chinese kale, 100 μmol m–2 s–1 of blue light treatment were not enough to increase total phenolics (Li et al., 2019), while in pak choi, it was enough to lead to an increase of total phenolics (Zheng et al., 2018). In lamb’s lettuce, the increase of blue light in the light spectrum even decreased the total phenolics (Wojciechowska et al., 2015). It is therefore possible that either the intensity of blue light was too low for kohlrabi or kohlrabi uses other defense mechanisms such as waxes.
Effect of Light Quality on ROS Scavenging Capacity
Leaves are good sources of flavonoid glycosides, which, alongside with other compounds such as carotenoids and vitamins, contribute to a high singlet oxygen scavenging capacity (Ribeiro et al., 2015). Ascorbic acid and quercetin contribute to the hydroxyl radical scavenging capacity of Indian olive (Panja et al., 2014). This leads to the conclusion that other compounds must be involved. In the present study, the results reveal a strong species-specific response of sprouts to the different light qualities dependent on the high variation of flavonoid glycosides and hydroxycinnamic acids within the species. Recent results showed a strong correlation between flavonols and singlet oxygen scavenging capacity, as shown here for kale and rocket salad, but also revealed that glycosides of flavonols are less effective as their aglycones (Agati et al., 2007; Majer et al., 2014; Csepregi and Hideg, 2018). Hydroxycinnamic acids had about 10% of singlet oxygen scavenging capacity compared to quercetin and kaempferol (Csepregi and Hideg, 2018). In the Brassicaceae sprouts, no correlation of hydroxycinnamic acids and the singlet oxygen scavenging capacity and hydroxyl radical scavenging capacity was found as previously described for hydroxycinnamic acids ferulic acid and p-coumaric acid of millet cultivars (Chandrasekara and Shahidi, 2011). In wild strawberries, different cultivars showed a strong correlation of singlet oxygen scavenging capacity and hydroxyl radical scavenging capacity (Wang et al., 2007), a trend that is also seen in the data presented here. Furthermore, in strawberries, numerous hydroxycinnamic acids as well as quercetin and kaempferol glycosides were increased with higher temperatures, in line with the singlet oxygen scavenging capacity and hydroxyl radical scavenging capacity (Wang and Zheng, 2001). None of the Brassicaceae species studied showed a large amount of compounds contributing to the hydroxyl radical scavenging capacity. Concomitantly, Majer et al. (2010) found no correlation between total phenolic content and hydroxyl radical scavenging capacity in tobacco leaves assuming other compounds involved in the quenching of hydroxyl radical. Hydroxycinnamic acids, although highly concentrated in the Brassica sprouts (Heinze et al., 2018), had no specific effects on the scavenging capacity to plant-specific ROS.
Effect of Light Quality on Absorption Ability
In addition to their role as antioxidants, flavonoids act as UV shielding components (Edreva, 2005). Kaempferol, quercetin, and isorhamnetin absorb longer wavelength in the UV radiation (350–400 nm) better than higher energized UV-B (280–315 nm). Absorption spectra of flavonol glycosides are shifted slightly toward shorter wavelengths compared to their corresponding aglycones (Majer et al., 2014). However, the role of structurally different flavonol glycosides in the UV shielding function of leaves is still not clear. UV-B as well as blue and green light treatment resulted in a slight shift of the band I assuming a better shielding activity (Edreva, 2005). Hydroxycinnamic acids with absorption maxima between 320 and 330 nm serve specific UV-B screening functions much better (Harborne and Williams, 2000). The results on kale and kohlrabi, once more underlines the species-specific response although the compounds were comparable in the two Brassica oleracea species. In the model plant Arabidopsis thaliana, the absorption at 330 nm was higher in a UV-tolerant mutant, in line with the reduced UV-B transmittance (Bieza and Lois, 2001). Kohlrabi, as a plant, might use also other defense mechanisms such as leaf hairs or waxes. In general, hydroxycinnamic acids have a higher UV-A and especially UV-B absorption compared to the flavonoid aglycones quercetin and kaempferol (Csepregi and Hideg, 2018). However, it is to be mentioned that glycosylation of flavonoid aglycones, which is commonly found in plants, resulted in a lower UV absorption capacity of these compounds (Csepregi and Hideg, 2018). Furthermore, in the absence of flavonoids, hydroxycinnamic acid derivatives were found to take over the absorption of UV radiation especially in the UV-A range (Stelzner et al., 2019). In total, the absorbance seemed to be less important in the defense against UV compared to the antioxidant activity as previously shown in linden leaves (Majer et al., 2014). Nevertheless, cell wall-bound phenolics were not investigated here and might play a major role in the UV response based on absorption.
Conclusion
UV radiation resulted in the enhancement of a number of hydroxycinnamic acids and quercetin as well as kaempferol glycosides in different Brassicaceae vegetable sprouts. In kale, the glycosylation pattern had no effect on the UV-B response. In kohlrabi, tri- and tetraglycosides are involved in the UV-B response, whereas pentaglycosides are not, which is clearly highlighting the effect of the glycosylation pattern. Blue light treatment after pre-exposure with UV resulted in constantly high concentrations of the most hydroxycinnamic acids and quercetin and kaempferol glycosides in three Brassicaceae sprouts, but no further increase. Green light, in general, resulted in a decrease of most flavonol glycosides and hydroxycinnamic acids to the control level. This result underlines the importance of different light qualities on the biosynthesis of flavonoids and hydroxycinnamic acids and their fast response to changing environments. The enhancement of the singlet oxygen scavenging capacity or the hydroxyl radical scavenging capacity was very low and often only a trend even though some hydroxycinnamic acids or quercetin and kaempferol glycosides were increased 1.2- to 2-fold. However, sprouts seem to be protected by high concentrations of hydroxycinnamic acids compared to adult plants. The shift in the absorption band highlights the role of flavonol glycosides and hydroxycinnamic acids as shielding compounds in the UV-A and UV-B range. Nevertheless, the investigated sprouts showed species-specific responses in the absorption also reflecting the chemically different structures of the hydroxycinnamic acids and quercetin and kaempferol glycosides, as well as other possible defense mechanisms. Regarding the use of UV light and blue or green light in vertical farming to improve secondary plant metabolites that correlate with quality traits, a combination of UV light and subsequent blue light is possible, but requires further specifications, especially regarding the intensity of blue light.
Data Availability Statement
The original contributions presented in the study are included in the article/Supplementary Material, further inquiries can be directed to the corresponding author/s.
Author Contributions
SN and MS designed the experiment. SN conducted the experiment and wrote the first draft of the manuscript. PM measured and evaluated the singlet oxygen and hydroxyl radical scavenging capacity as well as absorbance. ÉH contributed in the discussion of biological functions. All authors revised and approved the manuscript.
Funding
ÉH wishes to thank the National Research, Development, and Innovation Office for support (grant no. KH-129615). This work was supported by grant no. STSM-FA0906-9730.
Conflict of Interest
The authors declare that the research was conducted in the absence of any commercial or financial relationships that could be construed as a potential conflict of interest.
Acknowledgments
We thank Sarah Farrherr for support of the flavonoid analysis.
Supplementary Material
The Supplementary Material for this article can be found online at: https://www.frontiersin.org/articles/10.3389/fpls.2020.611247/full#supplementary-material
References
Agati, G., Brunetti, C., Di Ferdinando, M., Ferrini, F., Pollastri, S., and Tattini, M. (2013). Functional roles of flavonoids in photoprotection – new evidence, lessons from the past. Plant Physiol. Biochem. 72, 35–45. doi: 10.1016/j.plaphy.2013.03.014
Agati, G., Matteini, P., Goti, A., and Tattini, M. (2007). Chloroplast-located flavonoids can scavenge singlet oxygen. New Phytol. 174, 77–89. doi: 10.1111/j.1469-8137.2007.01986.x
Bell, L., and Wagstaff, C. (2014). Glucosinolates, myrosinase hydrolysis products, and flavonols found in rocket (Eruca sativa and Diplotaxis tenuifolia). J. Agric. Food Chem. 62, 4481–4492. doi: 10.1021/jf501096x
Bian, Z., Cheng, R., Wang, Y., Yang, Q., and Lu, C. (2018). Effect of green light on nitrate reduction and edible quality of hydroponically grown lettuce (Lactuca sativa L.) under short-term continuous light from red and blue light-emitting diodes. Environ. Exp. Bot. 153, 63–71. doi: 10.1016/j.envexpbot.2018.05.010
Bieza, K., and Lois, R. (2001). An Arabidopsis mutant tolerant to lethal ultraviolet-B levels shows constitutively elevated accumulation of flavonoids and other phenolics. Plant Physiol. 126, 1105–1115. doi: 10.1104/pp.126.3.1105
Bowles, D., Lim, E. K., Poppenberger, B., and Vaistij, F. E. (2006). Glycosyltransferases of lipophilic small molecules. Annu. Rev. Plant Biol. 57, 567–597. doi: 10.1146/annurev.arplant.57.032905.105429
Brelsford, C. C., Morales, L. O., Nezval, J., Kotilainen, T. K., Hartikainen, S. M., Aphalo, P. J., et al. (2019). Do UV-A radiation and blue light during growth prime leaves to cope with acute high light in photoreceptor mutants of Arabidopsis thaliana? Physiol. Plant. 165, 537–554. doi: 10.1111/ppl.12749
Britt, A. B. (1996). DNA damage and repair in plants. Annu. Rev. Plant Physiol. Plant Mol. Biol. 47, 75–100. doi: 10.1146/annurev.arplant.47.1.75
Burchard, P., Bilger, W., and Weissenbock, G. (2000). Contribution of hydroxycinnamates and flavonoids to epidermal shielding of UV-A and UV-B radiation in developing rye primary leaves as assessed by ultraviolet-induced chlorophyll fluorescence measurements. Plant Cell Environ. 23, 1373–1380. doi: 10.1046/j.1365-3040.2000.00633.x
Caldwell, M. M. (1971). “Solar UV irradiation and the growth and development of higher plants,” in Photophysiology VI, ed. Giese, 131–177.
Cartea, M. E., Francisco, M., Soengas, P., and Velasco, P. (2011). Phenolic compounds in Brassica vegetables. Molecules 16, 251–280. doi: 10.3390/molecules16010251
Chandrasekara, A., and Shahidi, F. (2011). Inhibitory activities of soluble and bound millet seed phenolics on free radicals and reactive oxygen species. J. Agric. Food Chem. 59, 428–436. doi: 10.1021/jf103896z
Csepregi, K., and Hideg, É (2018). Phenolic compound diversity explored in the context of photo-oxidative stress protection. Phytochem. Anal. 29, 129–136. doi: 10.1002/pca.2720
Edreva, A. (2005). The importance of non-photosynthetic pigments and cinnamic acid derivatives in photoprotection. Agric. Ecosyst. Environ. 106, 135–146. doi: 10.1016/j.agee.2004.10.002
Ferreres, F., Fernandes, F., Oliveira, J. M. A., Valentão, P., Pereira, J. A., and Andrade, P. B. (2009). Metabolic profiling and biological capacity of Pieris brassicae fed with kale (Brassica oleracea L. var. acephala). Food Chem. Toxicol. 47, 1209–1220. doi: 10.1016/j.fct.2009.02.014
Fiol, M., Adermann, S., Neugart, S., Rohn, S., Mügge, C., Schreiner, M., et al. (2012). Highly glycosylated and acylated flavonols isolated from kale (Brassica oleracea var. sabellica) — structure–antioxidant activity relationship. Food Res. Int. 47, 80–89. doi: 10.1016/j.foodres.2012.01.014
Folta, K. M., and Maruhnich, S. A. (2007). Green light: a signal to slow down or stop. J. Exp. Bot. 58, 3099–3111. doi: 10.1093/jxb/erm130
Fuglevand, G., Jackson, J. A., and Jenkins, G. I. (1996). UV-B, UV-A, and blue light signal transduction pathways interact synergistically to regulate chalcone synthase gene expression in Arabidopsis. Plant Cell 8, 2347–2357. doi: 10.1105/tpc.8.12.2347
Gachon, C. M. M., Langlois-Meurinne, M., and Saindrenan, P. (2005). Plant secondary metabolism glycosyltransferases: the emerging functional analysis. Trends Plant Sci. 10, 542–549. doi: 10.1016/j.tplants.2005.09.007
Gitelson, A., Chivkunova, O., Zhigalova, T., and Solovchenko, A. (2017). In situ optical properties of foliar flavonoids: implication for non-destructive estimation of flavonoid content. J. Plant Physiol. 218, 258–264. doi: 10.1016/j.jplph.2017.08.009
Groenbaek, M., Jensen, S., Neugart, S., Schreiner, M., Kidmose, U., and Lakkenborg Kristensen, H. (2014). Influence of cultivar and fertilizer approach on curly kale (Brassica oleracea L. var. sabellica). 1. Genetic diversity reflected in agronomic characteristics and phytochemical concentration. J. Agric. Food Chem. 62, 11393–11402. doi: 10.1021/jf503096p
Harbaum-Piayda, B., Palani, K., and Schwarz, K. (2016). Influence of postharvest UV-B treatment and fermentation on secondary plant compounds in white cabbage leaves. Food Chem. 197, 47–56. doi: 10.1016/j.foodchem.2015.10.065
Harbaum-Piayda, B., Walter, B., Bengtsson, G. B., Hubbermann, E. M., Bilger, W., and Schwarz, K. (2010). Influence of pre-harvest UV-B irradiation and normal or controlled atmosphere storage on flavonoid and hydroxycinnamic acid contents of pak choi (Brassica campestris L. ssp chinensis var. communis). Postharvest Biol. Technol. 56, 202–208. doi: 10.1016/j.postharvbio.2010.01.003
Harborne, J. B., and Williams, C. A. (2000). Advances in flavonoid research since 1992. Phytochemistry 55, 481–504. doi: 10.1016/s0031-9422(00)00235-1
Heijde, M., and Ulm, R. (2012). UV-B photoreceptor-mediated signalling in plants. Trends in Plant Sci. 17, 230–237. doi: 10.1016/j.tplants.2012.01.007
Heinze, M., Hanschen, F. S., Wiesner-Reinhold, M., Baldermann, S., Grafe, J., Schreiner, M., et al. (2018). Effects of developmental stages and reduced UVB and low UV conditions on plant secondary metabolite profiles in pak choi (Brassica rapa subsp. chinensis). J. Agric. Food Chem. 66, 1678–1692. doi: 10.1021/acs.jafc.7b03996
Hernandez, I., Alegre, L., van Breusegem, F., and Munne-Bosch, S. (2009). How relevant are flavonoids as antioxidants in plants? Trends Plant Sci. 14, 125–132. doi: 10.1016/j.tplants.2008.12.003
Husain, S. R., Cillard, J., and Cillard, P. (1987). Hydroxyl radical scavenging activity of flavonoids. Phytochemistry 26, 2489–2491. doi: 10.1016/s0031-9422(00)83860-1
Jansen, M. A. K., and Bornman, J. F. (2012). UV-B radiation: from generic stressor to specific regulator. Physiol. Plant. 145, 501–504. doi: 10.1111/j.1399-3054.2012.01656.x
Jansen, M. A. K., Hectors, K., O’Brien, N. M., Guisez, Y., and Potters, G. (2008). Plant stress and human health: do human consumers benefit from UV-B acclimated crops? Plant Sci. 175, 449–458. doi: 10.1016/j.plantsci.2008.04.010
Jenkins, G. I. (2014). The UV-B photoreceptor UVR8: from structure to physiology. Plant Cell 26, 21–37. doi: 10.1105/tpc.113.119446
Johkan, M., Shoji, K., Goto, F., Hashida, S., and Yoshihara, T. (2010). Blue light-emitting diode light irradiation of seedlings improves seedling quality and growth after transplanting in red leaf lettuce. Hortic. Sci. 45, 1809–1814. doi: 10.21273/hortsci.45.12.1809
Kaiser, E., Ouzounis, T., Giday, H., Schipper, R., Heuvelink, E., and Marcelis, L. F. M. (2019). Adding blue to red supplemental light increases biomass and yield of greenhouse-grown tomatoes, but only to an optimum. Front. Plant Sci. 9:2002. doi: 10.3389/fpls.2018.02002
Kolb, C. A., Kaser, M. A., Kopecky, J., Zotz, G., Riederer, M., and Pfundel, E. E. (2001). Effects of natural intensities of visible and ultraviolet radiation on epidermal ultraviolet screening and photosynthesis in grape leaves. Plant Physiol. 127, 863–875. doi: 10.1104/pp.010373
Kyriacou, M. C., El-Nakhel, C., Pannico, A., Graziani, G., Soteriou, G. A., Giordano, M., et al. (2020). Phenolic constitution, phytochemical and macronutrient content in three species of microgreens as modulated by natural fiber and synthetic substrates. Antioxidants (Basel) 9:252. doi: 10.3390/antiox9030252
Lau, O. S., and Deng, X. W. (2012). The photomorphogenic repressors COP1 and DET1: 20 years later. Trends Plant Sci. 17, 584–593. doi: 10.1016/j.tplants.2012.05.004
Li, H., Zhang, M., and Ma, G. (2011). Radical scavenging activity of flavonoids from Trollius chinensis Bunge. Nutrition 27, 1061–1065. doi: 10.1016/j.nut.2011.03.005
Li, Y., Zheng, Y., Liu, H., Zhang, Y., Hao, Y., Song, S., et al. (2019). Effect of supplemental blue light intensity on the growth and quality of Chinese kale. Hortic. Environ. Biotechnol. 60, 49–57. doi: 10.1007/s13580-018-0104-1
Lin, L.-Z., and Harnly, J. M. (2009). Identification of the phenolic components of collard greens, kale, and Chinese broccoli. J. Agric. Food Chem. 57, 7401–7408. doi: 10.1021/jf901121v
Majer, P., and Hideg, E. (2012). Developmental stage is an important factor that determines the antioxidant responses of young and old grapevine leaves under UV irradiation in a greenhouse. Plant Physiol. Biochem. 50, 15–23. doi: 10.1016/j.plaphy.2011.09.018
Majer, P., Neugart, S., Krumbein, A., Schreiner, M., and Hideg, É (2014). Singlet oxygen scavenging by leaf flavonoids contributes to sunlight acclimation in Tilia platyphyllos. Environ. Exp. Bot. 100, 1–9. doi: 10.1016/j.envexpbot.2013.12.001
Majer, P., Stoyanova, S., and Hideg, E. (2010). Do leaf total antioxidant capacities (TAC) reflect specific antioxidant potentials? A comparison of TAC and reactive oxygen scavenging in tobacco leaf extracts. J. Photochem. Photobiol. B Biol. 100, 38–43. doi: 10.1016/j.jphotobiol.2010.04.007
Martínez-Sánchez, A., Llorach, R., Gil, M. I., and Ferreres, F. (2007). Identification of new flavonoid glycosides and flavonoid profiles to characterize rocket leafy salads (Eruca vesicaria and Diplotaxis tenuifolia). J. Agric. Food Chem. 55, 1356–1363. doi: 10.1021/jf063474b
Matysiak, B., and Kowalski, A. (2019). White, blue and red LED lighting on growth, morphology and accumulation of flavonoid compounds in leafy greens. Zemdirbyste 103, 281–286. doi: 10.13080/z-a.2019.106.036
Mirecki, R. M., and Teramura, A. H. (1984). Effects of ultraviolet-B irradiance on soybean: the dependence of plant-sensitivity on the photosynthetic photon flux-density during and after leaf expansion. Plant Physiol. 74, 475–480. doi: 10.1104/pp.74.3.475
Mormile, P., Rippa, M., Graziani, G., and Ritieni, A. (2019). Use of greenhouse-covering films with tailored UV-B transmission dose for growing ‘medicines’ through plants: rocket salad case. J. Sci. Food Agric. 99, 6931–6936. doi: 10.1002/jsfa.9980
Neugart, S., Fiol, M., Schreiner, M., Rohn, S., Zrenner, R., Kroh, L. W., et al. (2014). Interaction of moderate UV-B exposure and temperature on the formation of structurally different flavonol glycosides and hydroxycinnamic acid derivatives in Kale (Brassica oleracea var. sabellica). J. Agric. Food Chem. 62, 4054–4062. doi: 10.1021/jf4054066
Neugart, S., Kläring, H.-P., Zietz, M., Schreiner, M., Rohn, S., Kroh, L. W., et al. (2012a). The effect of temperature and radiation on flavonol aglycones and flavonol glycosides of kale (Brassica oleracea var. sabellica). Food Chem. 133, 1456–1465. doi: 10.1016/j.foodchem.2012.02.034
Neugart, S., and Schreiner, M. (2018). UVB and UVA as eustressors in horticultural and agricultural crops. Sci. Hortic. 234, 370–381. doi: 10.1016/j.scienta.2018.02.021
Neugart, S., Zietz, M., Schreiner, M., Rohn, S., Kroh, L. W., and Krumbein, A. (2012b). Structurally different flavonol glycosides and hydroxycinnamic acid derivatives respond differently to moderate UV-B radiation exposure. Physiol. Plant. 145, 582–593. doi: 10.1111/j.1399-3054.2012.01567.x
Olsen, H., Aaby, K., and Borge, G. I. A. (2009). Characterization and quantification of flavonoids and hydroxycinnamic acids in curly kale (Brassica oleracea L. convar. acephala var. sabellica) by HPLC-DAD-ESI-MSn. J. Agric. Food Chem. 57, 2816–2825. doi: 10.1021/jf803693t
Olsson, L. C., Veit, M., Weissenbock, G., and Bornman, J. F. (1998). Differential flavonoid response to enhanced UV-B radiation in Brassica napus. Phytochemistry 49, 1021–1028. doi: 10.1016/s0031-9422(98)00062-4
Pan, M. H., Lai, C. S., and Ho, C. T. (2010). Anti-inflammatory activity of natural dietary flavonoids. Food Funct. 1, 15–31. doi: 10.1039/c0fo00103a
Panja, S., Chaudhuri, D., Baban Ghate, N., Le Minh, H., and Mandal, N. (2014). In vitro assessment of phytochemicals, antioxidant and DNA protective potential of wild edible fruit of Elaeagnus latifolia Linn. Fruits 69, 303–314. doi: 10.1051/fruits/2014019
Pasini, F., Verardo, V., Caboni, M. F., and D’Antuono, L. F. (2012). Determination of glucosinolates and phenolic compounds in rocket salad by HPLC-DAD-MS: evaluation of Eruca sativa Mill. and Diplotaxis tenuifolia L. genetic resources. Food Chem. 133, 1025–1033. doi: 10.1016/j.foodchem.2012.01.021
Pennisi, G., Blasioli, S., Cellini, A., Maia, L., Crepaldi, A., Braschi, I., et al. (2019). Unraveling the role of red:blue led lights on resource use efficiency and nutritional properties of indoor grown sweet basil. Front. Plant Sci. 10:305. doi: 10.3389/fpls.2019.00305
Podsedek, A. (2007). Natural antioxidants and antioxidant capacity of Brassica vegetables: a review. LWT Food Sci. Technol. 40, 1–11. doi: 10.1016/j.lwt.2005.07.023
Rechner, O., Neugart, S., Schreiner, M., Wu, S., and Poehling, H. M. (2016). Different narrow-band light ranges alter plant secondary metabolism and plant defense response to aphids. J. Chem. Ecol. 42, 989–1003. doi: 10.1007/s10886-016-0755-2
Rechner, O., Neugart, S., Schreiner, M., Wu, S., and Poehling, H.-M. (2017). Can narrow-bandwidth light from UV-A to green alter secondary plant metabolism and increase Brassica plant defenses against aphids? PLoS One 12:e0188522. doi: 10.1371/journal.pone.0188522
Reifenrath, K., and Mueller, C. (2007). Species-specific and leaf-age dependent effects of ultraviolet radiation on two Brassicaceae. Phytochemistry 68, 875–885. doi: 10.1016/j.phytochem.2006.12.008
Ribeiro, A. B., Berto, A., Chisté, R. C., Freitas, M., Visentainer, J. V., and Fernandes, E. (2015). Bioactive compounds and scavenging capacity of extracts from different parts of Vismia cauliflora against reactive oxygen and nitrogen species. Pharm. Biol. 53, 1267–1276. doi: 10.3109/13880209.2014.974063
Samuoliene, G., Virsile, A., Brazaityte, A., Jankauskiene, J., Sakalauskiene, S., Vastakaite, V., et al. (2017). Blue light dosage affects carotenoids and tocopherols in microgreens. Food Chem. 228, 50–56. doi: 10.1016/j.foodchem.2017.01.144
Schmidt, S., Zietz, M., Schreiner, M., Rohn, S., Kroh, L. W., and Krumbein, A. (2010). Identification of complex, naturally occurring flavonoid glycosides in kale (Brassica oleracea var. sabellica) by high-performance liquid chromatography diode-array detection/electrospray ionization multi-stage mass spectrometry. Rapid Commun. Mass Spectrom. 24, 2009–2022. doi: 10.1002/rcm.4605
Schreiner, M., Mewis, I., Huyskens-Keil, S., Jansen, M. A. K., Zrenner, R., Winkler, J. B., et al. (2012). UV-B-induced secondary plant metabolites – potential benefits for plant and human health. Crit. Rev. Plant Sci. 31, 229–240. doi: 10.1080/07352689.2012.664979
Searles, P. S., Flint, S. D., and Caldwell, M. M. (2001). A meta-analysis of plant field studies simulating stratospheric ozone depletion. Oecologia 127, 1–10. doi: 10.1007/s004420000592
Smith, H. L., McAusland, L., and Murchie, E. H. (2017). Don’t ignore the green light: exploring diverse roles in plant processes. J. Exp. Bot. 68, 2099–2110. doi: 10.1093/jxb/erx098
Solovchenko, A. E., and Merzlyak, M. N. (2008). Screening of visible and UV radiation as a photoprotective mechanism in plants. Russ. J. Plant Physiol. 55:719. doi: 10.1134/S1021443708060010
Stelzner, J., Roemhild, R., Garibay-Hernández, A., Harbaum-Piayda, B., Mock, H.-P., and Bilger, W. (2019). Hydroxycinnamic acids in sunflower leaves serve as UV-A screening pigments. Photochem. Photobiol. Sci. 18, 1649–1659. doi: 10.1039/c8pp00440d
Stochmal, A., and Oleszek, W. (2007). Effect of acylation of flavones with hydroxycinnamic acids on their spectral characteristics. Nat. Prod. Commun. 2, 571–574.
Taulavuori, K., Hyöky, V., Oksanen, J., Taulavuori, E., and Julkunen-Tiitto, R. (2016). Species-specific differences in synthesis of flavonoids and phenolic acids under increasing periods of enhanced blue light. Environ. Exp. Bot. 121, 145–150. doi: 10.1016/j.envexpbot.2015.04.002
Taulavuori, K., Pyysalo, A., Taulavuori, E., and Julkunen-Tiitto, R. (2018). Responses of phenolic acid and flavonoid synthesis to blue and blue-violet light depends on plant species. Environ. Exp. Bot. 150, 183–187. doi: 10.1016/j.envexpbot.2018.03.016
Terashima, I., Fujita, T., Inoue, T., Chow, W. S., and Oguchi, R. (2009). Green light drives leaf photosynthesis more efficiently than red light in strong white light: revisiting the enigmatic question of why leaves are green. Plant Cell Physiol. 50, 684–697. doi: 10.1093/pcp/pcp034
Tissot, N., and Ulm, R. (2020). Cryptochrome-mediated blue-light signalling modulates UVR8 photoreceptor activity and contributes to UV-B tolerance in Arabidopsis. Nat. Commun. 11:1323. doi: 10.1038/s41467-020-15133-y
Triantaphylides, C., and Havaux, M. (2009). Singlet oxygen in plants: production, detoxification and signaling. Trends Plant Sci. 14, 219–228. doi: 10.1016/j.tplants.2009.01.008
Wang, S. Y., Lewers, K. S., Bowman, L., and Ding, M. (2007). Antioxidant activities and anticancer cell proliferation properties of wild strawberries. J. Am. Soc. Hort. Sci. 132, 647–658. doi: 10.21273/JASHS.132.5.647
Wang, S. Y., and Zheng, W. (2001). Effect of plant growth temperature on antioxidant capacity in strawberry. J. Agric. Food Chem. 49, 4977–4982. doi: 10.1021/jf0106244
Waterman, M. J., Nugraha, A. S., Hendra, R., Ball, G. E., Robinson, S. A., and Keller, P. A. (2017). Antarctic moss biflavonoids show high antioxidant and ultraviolet-screening activity. J. Nat. Prod. 80, 2224–2231. doi: 10.1021/acs.jnatprod.7b00085
Wojciechowska, R., Bugosz-Grochowska, O., Kołton, A., and Żupnik, M. (2015). Effects of LED supplemental lighting on yield and some quality parameters of lamb’s lettuce grown in two winter cycles. Sci. Hortic. 187, 80–86. doi: 10.1016/j.scienta.2015.03.006
Keywords: LEDs (light emitting diode), kale, UV-radiation, kohlrabi, rocket salad
Citation: Neugart S, Majer P, Schreiner M and Hideg É (2021) Blue Light Treatment but Not Green Light Treatment After Pre-exposure to UV-B Stabilizes Flavonoid Glycoside Changes and Corresponding Biological Effects in Three Different Brassicaceae Sprouts. Front. Plant Sci. 11:611247. doi: 10.3389/fpls.2020.611247
Received: 28 September 2020; Accepted: 10 December 2020;
Published: 28 January 2021.
Edited by:
Marie-Theres Hauser, University of Natural Resources and Life Sciences Vienna, AustriaReviewed by:
Myung-Min OH, Chungbuk National University, South KoreaAlexei E. Solovchenko, Lomonosov Moscow State University, Russia
Copyright © 2021 Neugart, Majer, Schreiner and Hideg. This is an open-access article distributed under the terms of the Creative Commons Attribution License (CC BY). The use, distribution or reproduction in other forums is permitted, provided the original author(s) and the copyright owner(s) are credited and that the original publication in this journal is cited, in accordance with accepted academic practice. No use, distribution or reproduction is permitted which does not comply with these terms.
*Correspondence: Susanne Neugart, c3VzYW5uZS5uZXVnYXJ0QHVuaS1nb2V0dGluZ2VuLmRl