- 1Instituto de Ciencias Agrarias, Consejo Superior de Investigaciones Cientificas, Madrid, Spain
- 2Centro de Estudios Avanzados en Zonas Áridas, La Serena, Chile
Cadmium (Cd) pollution in soils is an increasing problem worldwide, and it affects crop production and safety. We identified Cd-tolerant and -sensitive cultivars by testing 258 accessions of Medicago truncatula at seedling stage, using the relative root growth (RRG) as an indicator of Cd tolerance. The factorial analysis (principal component analysis method) of the different growth parameters analyzed revealed a clear differentiation between accessions depending on the trait (tolerant or sensitive). We obtained a normalized index of Cd tolerance, which further supported the suitability of RRG to assess Cd tolerance at seedling stage. Cd and elements contents were analyzed, but no correlations with the tolerance trait were found. The responses to Cd stress of two accessions which had similar growth in the absence of Cd, different sensitivity to the metal but similar Cd accumulation capacity, were analyzed during germination, seedling stage, and in mature plants. The results showed that the Cd-tolerant accession (CdT) displayed a higher tolerance than the sensitive cultivar (CdS) in all the studied stages. The increased gene expression of the three main NADPH recycling enzymes in CdT might be key for this tolerance. In CdS, Cd stress produced strong expression of most of the genes that encode enzymes involved in glutathione and phytochelatin biosynthesis (MtCYS, MtγECS, and MtGSHS), as well as GR, but it was not enough to avoid a redox status imbalance and oxidative damages. Our results on gene expression, enzyme activity, antioxidant content, and lipid peroxidation indicate different strategies to cope with Cd stress between CdS and CdT, and provide new insights on Cd tolerance and Cd toxicity mechanisms in M. truncatula.
Introduction
Cadmium (Cd) is one of the most toxic substances for all living organisms, and its accumulation represents an increasing problem in agricultural soils due to anthropogenic activities, such as the extensive use of soil amendments and phytochemicals in agriculture, mining and industrial activities, transportation, uncontrolled dumping, and bad practices in waste treatment (Clemens et al., 2013). Plants are the main entry for Cd into the food chain (Peralta-Videa et al., 2009), presenting a risk both to the environment and to animal and human health. FAO is alerting of the hidden reality of soil pollution, especially in countries in development (Rodríguez-Eugenio et al., 2018). As an example, Cd levels in some areas of China have increased up to 250% in the last 30 years (Rodríguez-Eugenio et al., 2018), and in large cultivated areas, Cd accumulates to health-threatening levels in the so-called “cadmium rice” (Holdaway and Wang, 2018). Therefore, it is becoming of outmost interest the identification of crops and cultivars that are capable to tolerate moderate Cd stress and do not accumulate this heavy metal in their edible parts.
Legumes are together with cereals the main agricultural crops. They do not depend on nitrogen fertilization and are able to grow in poor and degraded soils, including heavy metal-polluted soils (Coba de la Peña and Pueyo, 2012). Medicago truncatula is a forage legume crop closely related to alfalfa, and a model legume plant. It constitutes an appropriate source for genetic improvement and an ideal model to study metal tolerance mechanisms in legumes. Cd-tolerant M. truncatula cultivars which do not accumulate Cd in the aerial part could be cultivated as a forage crop in moderately Cd-polluted soils, while cultivars with high metal accumulation could be used in phytoremediation of Cd-contaminated soils, due to its high biomass and good soil coverage. The identification of such cultivars in M. truncatula germplasm represents a potential tool to remediate the problems derived from Cd accumulation in both, arable lands and wild ecosystems. We have shown before the feasibility of identifying heavy metal-tolerant varieties in a M. truncatula germplasm by phenotyping a limited number of accessions (García de la Torre et al., 2013).
The effect of Cd stress on M. truncatula has been studied scarcely, mainly at the seedling stage (Aloui et al., 2009, 2011; Xu et al., 2010; Saeidi et al., 2012; Rahoui et al., 2014, 2015, 2017). Heavy metal tolerance and/or toxicity depend on the developmental stage of the plant, the metal concentration, and the time of exposure (Sanità di Toppi and Gabbrielli, 1999). In plants, Cd toxicity promotes growth reduction, macro and micronutrients uptake disturbance, and restriction in reserves mobilization at germination, among other detrimental effects (Benavides et al., 2005). Cd produces oxidative stress and lipid peroxidation (Romero-Puertas et al., 2012). In fact, the overproduction of reactive oxygen species (ROS) is considered the origin of the damage following Cd exposure (Cuypers et al., 2010). Cd does not take part in Fenton reactions for generating ROS but indirectly contributes to ROS formation by altering the antioxidant machinery of cells (Sharma and Dietz, 2009). Under Cd stress conditions, ROS play a dual role acting as toxic agents, but also as signal molecules, therefore the fine tuning of ROS homeostasis is crucial for the plant. The production of ROS and reactive nitrogen species with signaling functions are now considered strategies to avoid Cd toxicity (Romero-Puertas et al., 2019). Plants have several enzymatic and non-enzymatic antioxidant defense systems that allow ROS scavenging.
The first line of defense against ROS is the dismutation of O2– to H2O2 through superoxide dismutase enzymes (SODs) and then catalases and peroxidases detoxify H2O2. There are also important antioxidant enzymes of the ascorbate-glutathione (ASC-GSH) cycle that allow maintaining the redox state of the cell. Some of these reactions are NADPH-dependent, and this molecule has been described as a limiting factor for the plant antioxidant capacity (Foyer and Noctor, 2011). NADPH is mainly produced by three enzymes, glucose 6-phosphate dehydrogenase (G6PDH), 6-phosphogluconate dehydrogenase (6PGDH), and NADP+-dependent isocitrate dehydrogenase (ICDH). Differential responses of these enzymes have been reported in stressed plants; and ICDH has been described as a key enzyme in NADPH recycling, and essential for the antioxidant defense under stress conditions (Marino et al., 2007). NADPH-recycling dehydrogenases are activated in response to Cd (Marino et al., 2013; Pérez-Chaca et al., 2014). There are several reports that study ROS production under Cd stress; however, most of them do not compare the differences between metal-tolerant and sensitive cultivars. Studies that analyze the antioxidant defense at different developmental stages are also scarce.
The aims of this work were to evaluate Cd tolerance in a Medicago truncatula germplasm, and to identify Cd-tolerant and Cd-sensitive genotypes. We also comparatively assessed Cd effects on tolerant and sensitive accessions at different developmental stages. Metal accumulation, nutritional status, and several markers for Cd tolerance, related with antioxidant defenses and Cd-induced oxidative stress, were analyzed. Our results suggest different strategies to cope with Cd stress depending on the Cd tolerance trait, and provide new insights on Cd response mechanisms in M. truncatula.
Materials and Methods
Plant Material
Medicago truncatula cv. Parabinga was used to set up the experimental conditions for the tolerance assays. Two hundred and fifty-eight M. truncatula accessions obtained from the National Plant Germplasm System of the United States Department of Agriculture (ARS-USDA) were used for the Cd-tolerance screening.
Screening for Cadmium Tolerance
Seeds were scarified, sterilized, and germinated, and seedlings were transferred to a miniaturized hydroponic culture system containing modified Hoagland solution, pH 5.4, as previously described (García de la Torre et al., 2013). Seedlings were acclimatized during 24 h prior to Cd treatment.
Medicago truncatula cv. Parabinga seedlings were exposed to different Cd concentrations (0–40 μM CdCl2) for 48, 72, and 96 h under growth chamber conditions as described by García de la Torre et al. (2013). In subsequent experiments, seedlings of 258 M. truncatula accessions were exposed to 0 and 10 μM CdCl2 for 48 h. Thus, seedlings were 3 days old at harvest. Ten to thirty seedlings per cultivar were analyzed per treatment (control or Cd stress). Seedling relative root growth (RRG) was calculated as previously described (Sledge et al., 2005; García de la Torre et al., 2013), and it was used as an indicator of tolerance.
Screening Validation in Growth Pouches
Two-day-old seedlings were acclimatized for 24 h prior to Cd exposure in growth pouches (CYG Seed Germination Pouches, Mega International, Minneapolis, MN, United States) containing 50 mL of nutrient solution. To set up the assay conditions, M. truncatula cv. Parabinga plantlets were grown in a range of Cd concentrations (0–200 μM CdCl2) for 12 days, under growth chamber conditions (García de la Torre et al., 2013). Nutrient solution was changed every 48 h. In subsequent experiments, seedlings of four potentially Cd-tolerant and four potentially Cd-sensitive accessions were exposed to 0 or 100 μM CdCl2 for 12 days. Thus, plants were 15 days old at harvest. Five to ten pouches, each containing five plants per cultivar and treatment, were analyzed. Root length, root and shoot fresh and dry weights, and number of leaves were measured, and relative parameters (RX) were calculated as follows: RX = (Parameter XCd/Parameter XC) × 100.
Cadmium and Nutrients Content
The content of Cd and nutrients was analyzed. At harvest, plants were dipped in 10 mM Na2EDTA, and then washed twice in ultrapure water. Roots and shoots were collected and stove-dried at 60°C for 3 days, and digested with nitric-perchloric acid (7:3). Contents of Cd and nutrients were determined using ICP-OES (Perkin-Elmer Optima 4300 DV). Three pouches, each containing five plants, per cultivar and treatment were analyzed.
Analysis of Two Genotypes With Contrasting Tolerance
The tolerant PI 516929 (CdT) and sensitive PI 660497 (CdS) genotypes were selected to study the Cd effect on the germination process, gene expression, glutathione content, SOD and catalase (CAT) activities, and lipid peroxidation.
Scarified and sterilized CdT and CdS seeds were transferred into Petri dishes with a sterile filter paper imbibed with 5 mL of sterile water or 5 mL 200 μM CdCl2 for 72 h (25/19°C, 16/8 h) in the dark. Germination percentage was recorded on the third day. The seedlings radicles were removed and the cotyledons used in the α-amylase analysis. Four to six replicates (25 seeds per replicate) were assayed for each accession and treatment.
Three-day-old seedlings were transferred to the miniaturized hydroponic system for 72 h for acclimatization, prior to Cd treatment. Then, seedlings were exposed to 0 or 50 μM CdCl2 for 12 h. Five to ten seedlings per cultivar and treatment were used for histochemical detection of lipid peroxidation and loss of plasma membrane integrity. The rest of the roots were used for the gene expression analyses and determination of glutathione content.
Finally, 3-day-old CdT and CdS germinated seedlings were acclimatized for 7 days in vermiculite pots (350 mL), and then exposed to 0 or 100 μM CdCl2 for 15 days. Plants were 25 days old at harvest. Shoots and roots were analyzed for lipid peroxidation and CAT and SOD enzymatic activities. For each determination, four biological replicates per cultivar and treatment were analyzed.
Alpha-Amylase Activity
Cotyledons were ground in liquid nitrogen and homogenized (0.1 g FW mL–1) in 2 mM imidazole-HCl buffer (pH 7.0). The homogenates were centrifuged (15,000 g, 1 h, at 0°C), and the supernatant was used to determine the α-amylase activity as described by Smith and Roe (1949). The reaction mixture (100 μL supernatant, 550 μL phosphate buffer, 0.9 μg μL–1 starch, and 0.5 M NaCl) was incubated during 30 min at 37°C, and the reaction was stopped with 80 μL of 1 N HCl. The remaining starch was stained with 700 μL of diluted lugol (1:6). A blank for each sample was prepared using 100 μL of supernatant, 650 μL of deionized water, and 700 μL of diluted lugol. The starch content was monitored at 620 nm. The α-amylase activity was expressed as μg hydrolyzed starch g–1 fresh weight min–1.
RNA Isolation and Quantitative Real-Time PCR Analysis
Total RNA was isolated from seedling roots using the TRIZol (Invitrogen) method and treated with RNase-free DNase I (Thermo Fisher Scientific). RNA concentration was determined with a Nanodrop ND1000 spectrophotometer (Thermo Fisher Scientific). One microgram of DNA-free RNA was used for reverse transcription, using SuperScript® II Reverse Transcriptase (Invitrogen), and oligo-d(T)18 as primer. The cDNAs were diluted 10-fold before performing qPCR. The Primer3 software1 was used to design specific primers for all genes tested (Supplementary Table 1).
Quantitative PCR reactions were run in a 7300 Real-Time PCR Sequence Detection System (PE Applied Biosystems). Each reaction contained 1 μL cDNA, 5 μL SYBR Green PCR master mix (PE Applied Biosystems), and 0.5 μM (final concentration) primer, in a total volume of 10 μL. Initial denaturing time (10 min, 95°C) was followed by 40 PCR cycles (95°C, 15 s; 60°C, 90 s; 72°C, 30 s), and a melting curve (95°C, 15 s; 60°C, 60 s; 95°C, 15 s). Relative gene expression was calculated according to the primer efficiency method (Pfaffl, 2001). Only fold-changes greater than two were considered as significant. Four biological replicates (10 roots each) per cultivar and treatment were analyzed.
Glutathione Determination
Reduced glutathione (GSH) and total glutathione (GSH + GSSG) were measured by means of the glutathione recycling assay (Floreani et al., 1997), essentially as described by Shvaleva et al. (2010) with the following modifications: Roots were ground in liquid nitrogen and homogenized in 5% (w/v) sulfosalicylic acid (0.2 g FW mL–1). Homogenates were centrifuged (15,000 g, 5 min, 4°C), and the supernatant was used for determination of total glutathione. Reaction mixture (1 mL) included 25 μL of supernatant, 80 mM TEA (pH 8), 0.6 mM 5,5′-dithio-bis (2-nitrobenzoic acid) (DTNB, Sigma), 0.21 mM NADPH (Sigma), and 1 unit glutathione reductase (Roche, Branchburg, NJ, United States). DTNB, NADPH, and glutathione reductase were dissolved or diluted in 150 mM K-phosphate buffer (pH 7.4), 6.3 mM EDTA. The 2-nitro-5-thiobenzoic acid (TNB) generation was monitored spectrophotometrically at 412 nm for 1 min at 25°C. For GSSG determination, GSH was derivatized by adding 6 μL of 2-vinyl-pyridine and 10 μL 1 M triethanolamine (pH 8.0) to 90 μL of supernatant, for 30 min. The final pH of the reaction was between 6.0 and 7.0. Derivatized samples were assayed as described above. GSH was determined as the difference between total glutathione and GSSG. Four biological replicates (10 roots each) per cultivar and treatment were analyzed.
Histochemical Analyses
Lipid peroxidation and loss of plasma membrane integrity detection were performed on intact seedling roots as described previously (Yamamoto et al., 2001). For lipid peroxidation, roots were stained for 20 min with Schiff’s reagent, which detects aldehydes originated from lipid peroxides, and kept in a solution containing 0.5% (w/v) K2S2O5 in 0.1 N HCl to retain the color.
The loss of plasma membrane integrity was detected by staining the roots with Evans blue solution [0.025% (w/v) Evans blue in 100 μM CaCl2, pH 5.6] for 10 min and washed several times with 100 μM CaCl2 (pH 5.6) until the dye no longer eluted from the roots.
The stained roots were observed under a stereomicroscope (Carl Zeiss Stemi 2000C, Fisher Scientific) with an attached digital camera (Leica DFC 420C). Color density was measured using the ImageJ software2. Five to ten seedlings per cultivar and treatment were analyzed.
Lipid Peroxidation
Assessment of malondialdehyde (MDA) was performed using the thiobarbituric acid method (Singh et al., 2007). Shoots and roots were ground in liquid nitrogen, homogenized in 1 mL of 0.1% TCA (0.1 g FW mL–1 for shoots and 0.25 g FW mL–1 for roots), and analyzed as described by Redondo et al. (2009). Four biological replicates per cultivar and treatment were analyzed.
Superoxide Dismutase and Catalase Activities
Shoots and roots (0.04 and 0.1 g FW mL–1, respectively) were ground in liquid nitrogen and homogenized in 50 mM K-phosphate buffer (pH 7.8) containing 0.1 mM EDTA, 1% (w/v) PVP-10, and 0.1% (v/v) Triton X-100. After centrifugation (20,000 g, 4°C, 30 min), the supernatant was used for the spectrometric determination of SOD and CAT activities, as described by Redondo et al. (2009).
Statistical Analyses and Tolerance Index
The statistical analyses were performed with the IBM SPSS Statistics 20 software (SPSS Inc., Chicago, IL, United States). The root lengths from the screening assay were analyzed by ANOVA (p < 0.05; n ≥ 10). Cultivars that did not show significant differences or displayed a significantly higher root growth under Cd treatment than under control conditions were considered potentially Cd-tolerant. A Pearson’s correlation analysis was performed comparing the results obtained here with those previously reported on mercury and aluminum tolerance for the same M. truncatula germplasm (Sledge et al., 2005; García de la Torre et al., 2013). The relative parameters, fresh weight root/shoot ratio, and elements content were analyzed by ANOVA (p < 0.05). Fisher LSD or Tukey HSD tests were applied for pair-wise comparison. Data were combined considering the different accessions as replicates of potentially tolerant or sensitive plants, and a factorial analysis (principal component analysis method, PCA) was conducted with the different relative parameters. The resulting components (PC1 and PC2) were plotted to check the associations. For each accession, a tolerance index was calculated and normalized (nTI) as described by García de la Torre et al. (2013). Germination percentage was analyzed by the Pearsons’s chi-squared test. The glutathione content, lipid peroxidation, α-amylase activity, and SOD and CAT activities were analyzed by ANOVA (p < 0.05), and the Tukey HSD test was applied for pair-wise comparisons.
Results
Medicago truncatula Germplasm Screening for Cd Tolerance
The appropriate Cd treatment (concentration and time) for the screening assays was determined based on the root growth response of M. truncatula cv. Parabinga, a Cd-sensitive cultivar (Supplementary Figure 1). The treatment with 10 μM CdCl2 for 48 h had a clear effect on this cultivar without completely arresting root growth (RRG ≈ 30%), and it was selected for M. truncatula germplasm screening.
Seedlings of 258 M. truncatula accessions were tested for Cd tolerance. Root growth results are shown in Supplementary Table 2. The response to the metal of the different genotypes followed a normal distribution (Figure 1), suggesting that the number of tested accessions and the assay conditions were adequate. Most accessions displayed low RRG values, ranging from 10 to 50% (225 accessions), suggesting that M. truncatula is in general sensitive to Cd. Twenty-six accessions displayed values from 60 to 80%, and eight accessions exhibited RRG values above 80% (Supplementary Table 2). Cd had not a detrimental effect on the root growth of six of the accessions tested, as either there were not significant differences between control and Cd-treated seedlings, or the root growth values of Cd-treated seedlings were significantly higher than those of untreated seedlings (Supplementary Table 2).
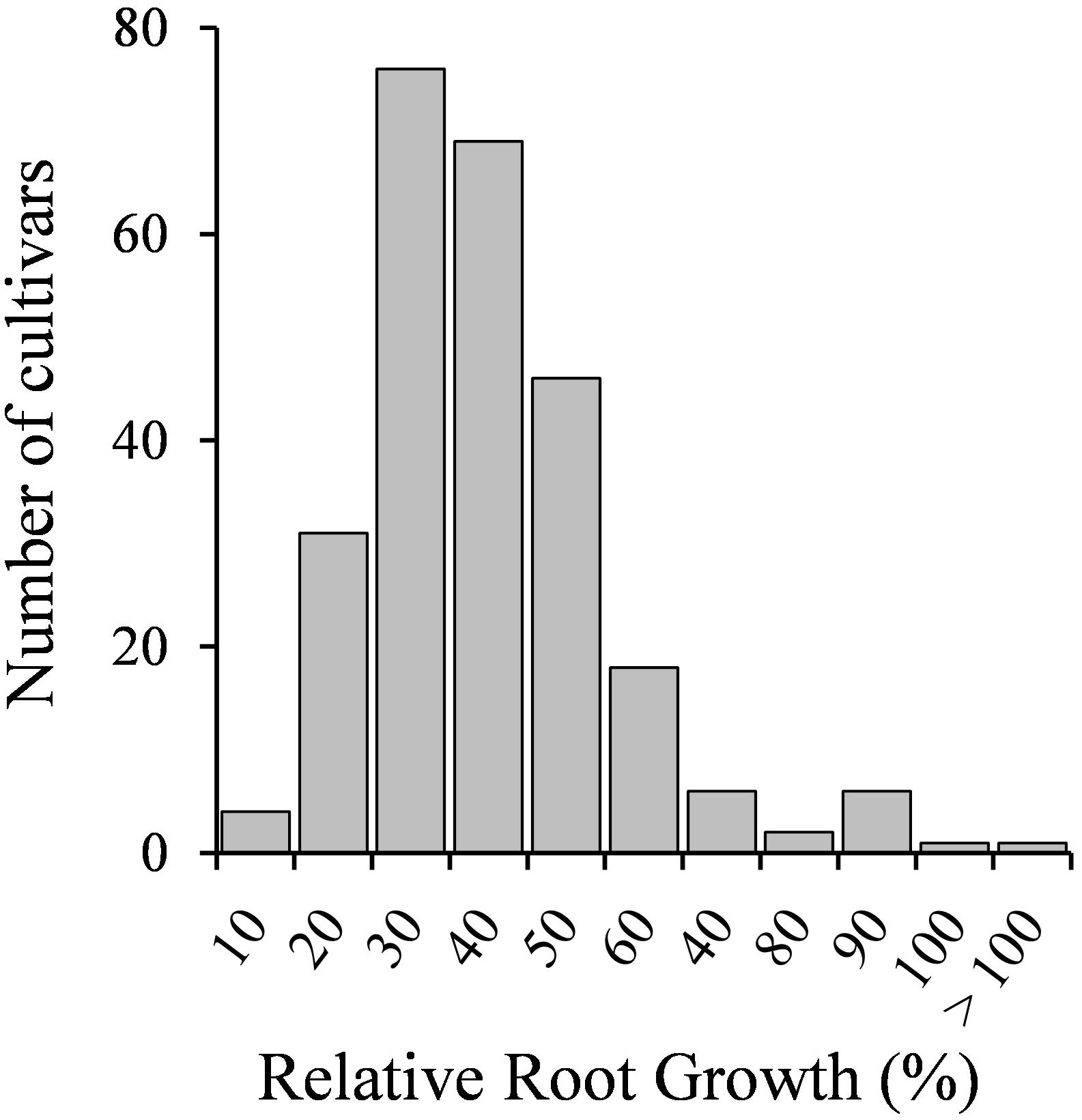
Figure 1. Seedling relative root growth (RRG) distribution of 258 M. truncatula accessions grown in the absence or presence of Cd stress (10 μM CdCl2, 48 h).
Plants might have both metal-specific tolerance mechanisms and common mechanisms for different metals. A Pearson’s correlation analysis revealed no correlation between Al and Cd tolerances but a significant positive correlation between Cd and Hg tolerances (r = 0.486, p < 0.01) (Figure 2).
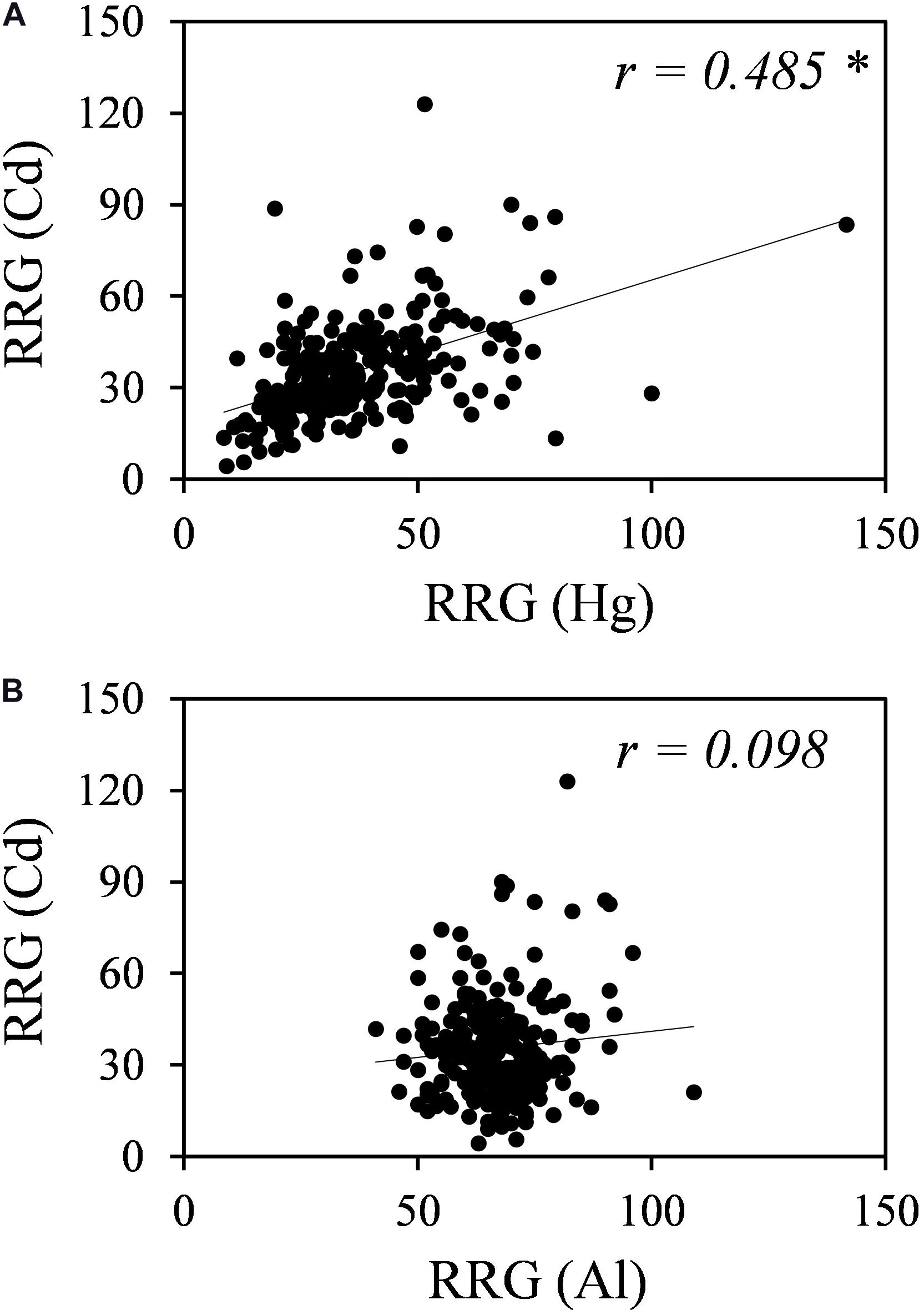
Figure 2. Correlation analyses for Cd and Hg tolerances (A) and for Cd and Al tolerances (B). RRG values for Hg were obtained from García de la Torre et al. (2013), and RRG values for Al were obtained from Sledge et al. (2005). * Indicates significant correlation at p-value < 0.01.
Validation of the Rapid Screening Method for Cd Tolerance
To determine the validity of the screening results, treatment with 100 μM CdCl2 for 12 days in growth pouches was selected, as it was non-lethal but produced substantial differences in all growth parameters, in M. truncatula cv. Parabinga (data not shown).
Four potentially Cd-tolerant accessions (PI 660407, PI 660411, PI 516929, and PI 516933) with RRG values ranging from 66 to 90%, and four potentially Cd-sensitive accessions (PI 660497, PI 199257, PI 384634, and PI 516950) with RRG values ranging from 8 to 45% (Supplementary Table 2), were analyzed for morphological parameters. Results are shown in Table 1. No obvious phenotypic or anatomical differences were observed among accessions in the absence of Cd treatment. Root growth and root fresh weight were significantly affected in the presence of Cd in all accessions. While RRG and relative root fresh weight (RRFW) values of the potentially tolerant accessions were in general higher than those of the sensitive ones, two of the sensitive accession (PI 199257 and PI 660497) presented RRG values that were not significantly different from those of the tolerant accessions, and one tolerant accession (PI 516933) showed a similar RRFW value to those recorded for the sensitive varieties. No significant differences in relative root dry weight (RRDW) values were observed among varieties, and one sensitive accession (PI 660497) showed a significant reduction of the root dry weight after Cd treatment.
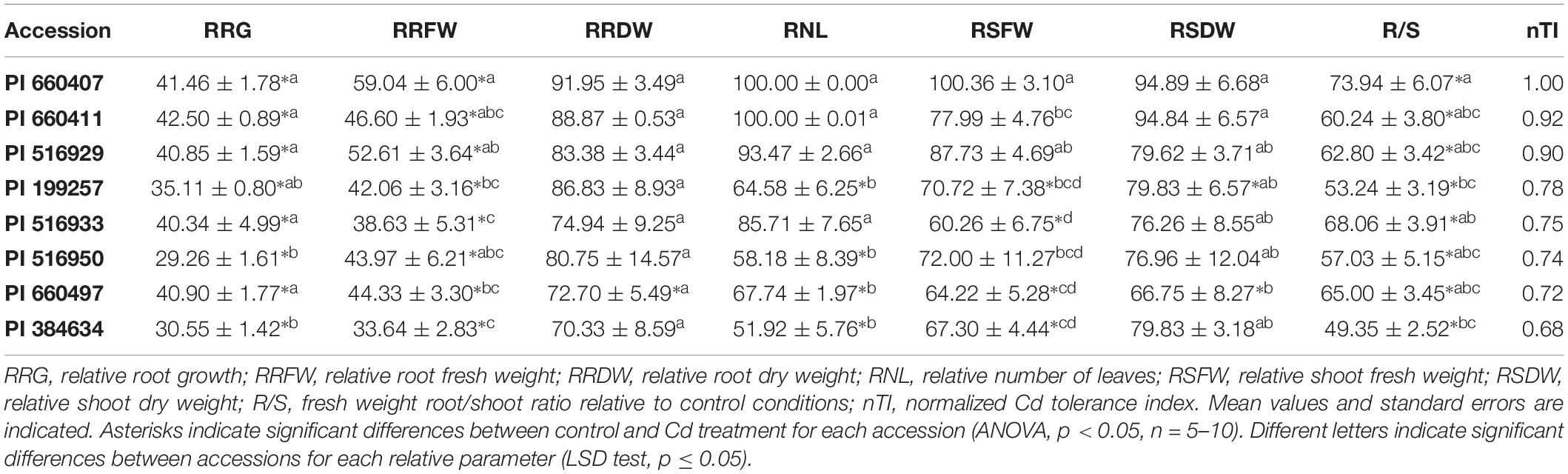
Table 1. Relative growth parameters and normalized tolerance indices for selected M. truncatula accessions grown in hydroponic pouches with 0 or 100 μM CdCl2 for 12 days.
Regarding the Cd effect on the aerial part growth, the number of leaves decreased significantly in sensitive accessions following exposure to Cd, while non-significant differences were found for all potentially tolerant accessions. Tolerant accessions had relative number of leaves (RNL) values above 85%, and significantly higher than those of the sensitive accessions, which remained below 68%. Cd significantly affected the relative shoot fresh weight (RSFW) of accession PI 516933 (initially ranked as Cd-tolerant), while this parameter was not significantly affected for accession PI 516950 (initially ranked as Cd-sensitive). Only one potentially tolerant accession, PI 660407, presented an RSFW value that was significantly different from the potentially sensitive accessions. No significant differences were found between RSFWs for the potentially tolerant accessions PI 660407 and PI 5162929. Cd stress produced a significant decrease in the shoot dry weight of two sensitive accessions. Significant differences for relative shoot dry weight (RSDW) were found between the sensitive accession PI 660497 and the two tolerant accessions PI 660407 and PI 660411, which displayed the highest RSDW values. Cd significantly affected the root/shoot ratio (R/S) of all accessions, and accession PI 660407 showed the highest R/S ratio.
A PCA analysis revealed two components that accounted for 83.42% of the total variance. PC1 accounted for 49.02% of the explained variance, with the following eigenvalues:
The second component accounted for 34.40% of the total variance, with the following eigenvalues:
Plotting the values for each component one against another, two different groups were identified, corresponding to tolerant and sensitive categories (Figure 3). These groups were associated with both components, and the tolerant accessions exhibited higher values than the sensitive accessions for the PCs. The RSDW and RRDW for PC1 and the RRG for PC2 were the main contributors to the maximum principal component variation. A normalized index of Cd tolerance (nTI), ranging from 0 to 1, was generated and a ranking of tolerance for the analyzed accessions was obtained (Table 1). The nTI values for the initially ranked as Cd-sensitive accessions were lower than 0.80. PI 660407 was the most Cd-tolerant among the accessions tested, and PI 516929 and PI 660411 could be confirmed as Cd-tolerant. The nTI values for those three tolerant accessions ranged from 1.00 to 0.90. Accession PI 516933, which was initially classified as Cd-tolerant according to the screening, presented an nTI value below 0.80, and therefore it had to be re-classified as a sensitive accession.
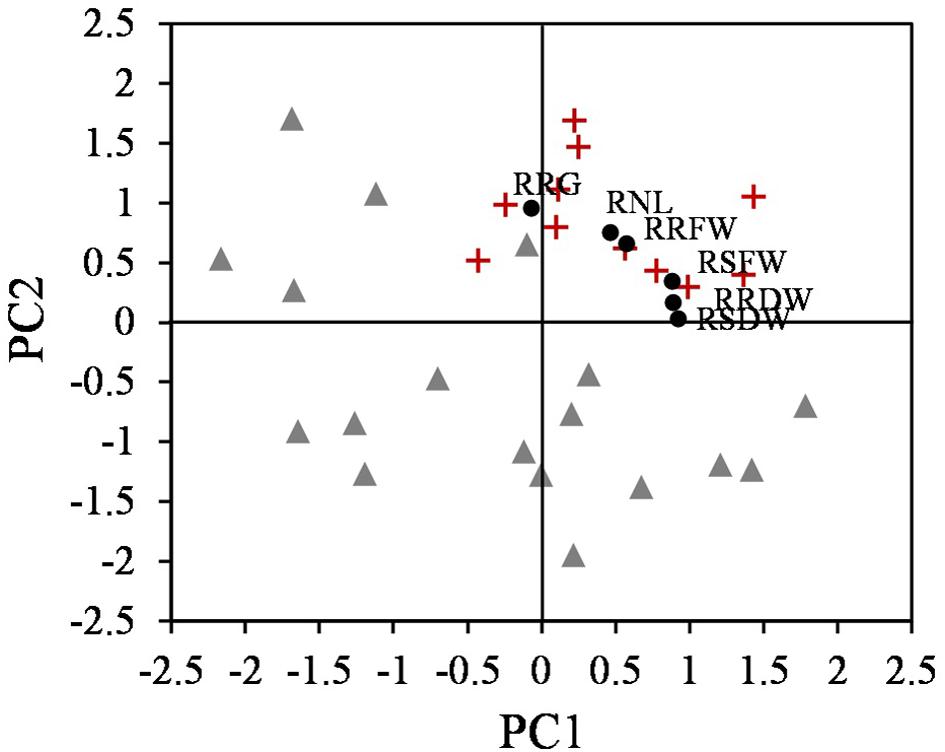
Figure 3. Scatter plot of the two components obtained from PCA that differentiate two groups, tolerant (+) and sensitive (Δ) accessions (n = 25), and the relative parameters according to the eigenvalues (∙).
To further characterize the eight selected accessions, Cd accumulation and nutrients content were determined in roots and shoots. Cd was mainly accumulated in roots and only low amounts of Cd were translocated to the shoots (Figure 4). Cd-tolerant accession PI 516929 accumulated the highest amount of Cd in roots (1802 mg kg–1) and shoots (32 mg kg–1), while Cd-tolerant accession PI 660407 had the lowest Cd content in roots (672 mg kg–1) and high Cd content in shoots (29 mg kg–1). Among the Cd-sensitive accessions, the highest Cd content in roots was found in PI 660497, which also had high Cd content in shoots. The lowest concentration of Cd in roots was observed in PI 516950, whose shoots showed the highest Cd content. Cd content in roots and shoots did not appear to correlate with the tolerance trait (Cd-tolerant or Cd-sensitive) and therefore, Cd accumulation cannot be considered as a suitable predictor for Cd tolerance.
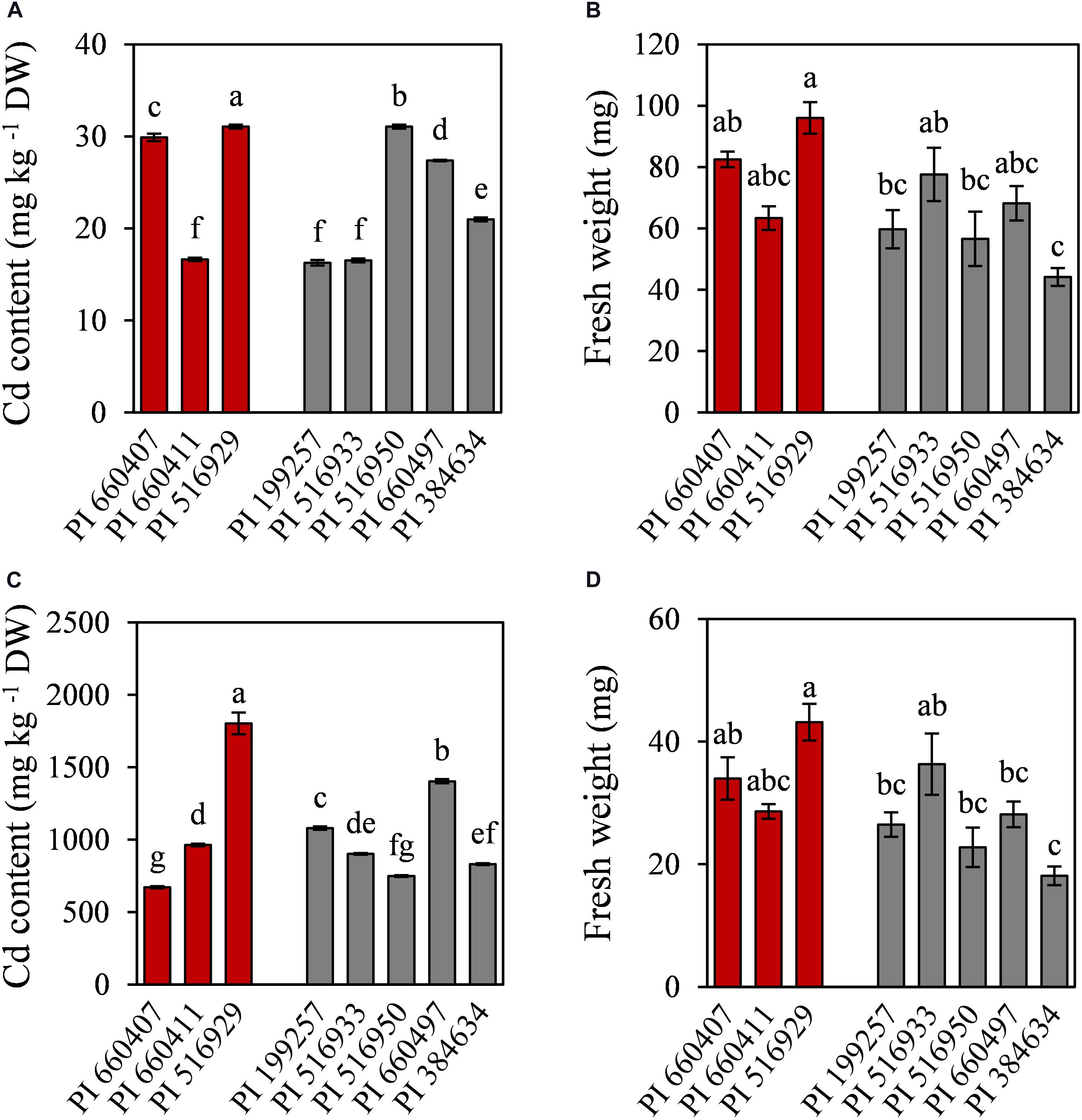
Figure 4. Cadmium content and fresh weight of shoots (A,B) and roots (C,D) of tolerant (red) and sensitive (gray) M. truncatula accessions grown in hydroponic pouches under Cd stress (100 μM CdCl2, 12 days). Mean values ± standard error are indicated, n = 3 for Cd content; n = 5–10 for fresh weight. Different letters indicate significant differences between cultivars (Tukey HSD test, p ≤ 0.05).
The Cd effect on the macro and micronutrients contents in roots and shoots of the different tested accessions was diverse (Tables 2, 3), but common features could be observed. In all accessions, Cd caused a significant decrease in Ca and S contents in shoots, and in Mn content in roots. The P content in roots and shoots was also decreased by Cd in all accessions, although it was not statistically significant for PI 660411 roots. Cd negatively affected the content of Mg in shoots of all accessions, although the decrease of Mg content was not statistically significant for PI 516929 and PI 384634. Following Cd stress, S content increased significantly in roots of tolerant accessions as well as in the sensitive accession PI 199257, and it decreased or did not change in the roots of the rest of the sensitive accessions. For the rest of nutrients, the effect of Cd was quite heterogeneous and did not appear to correlate with tolerance.
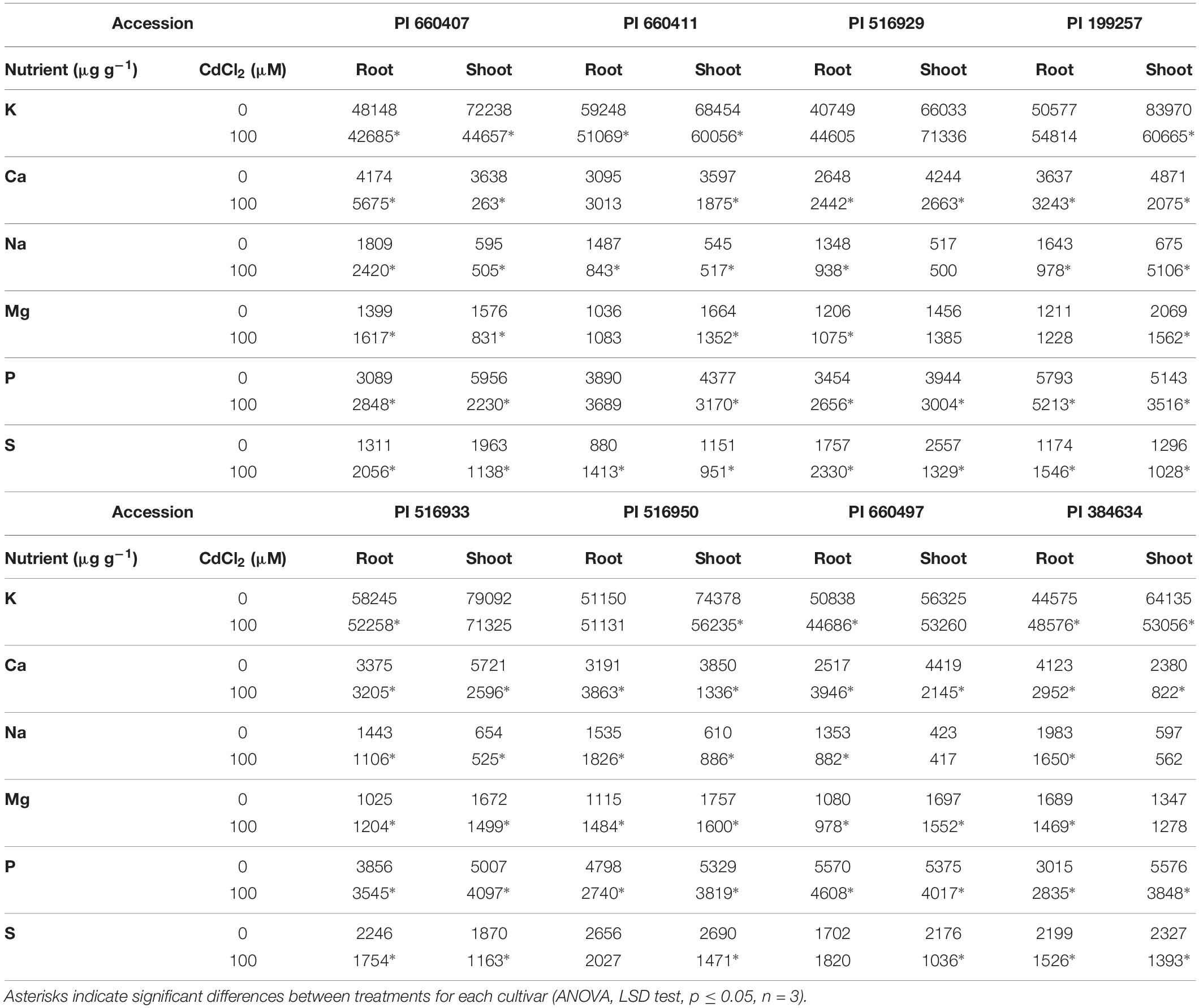
Table 2. Macronutrients content in Cd-tolerant and Cd-sensitive accessions of M. truncatula grown in hydroponic pouches with 0 or 100 μM CdCl2 for 12 days.
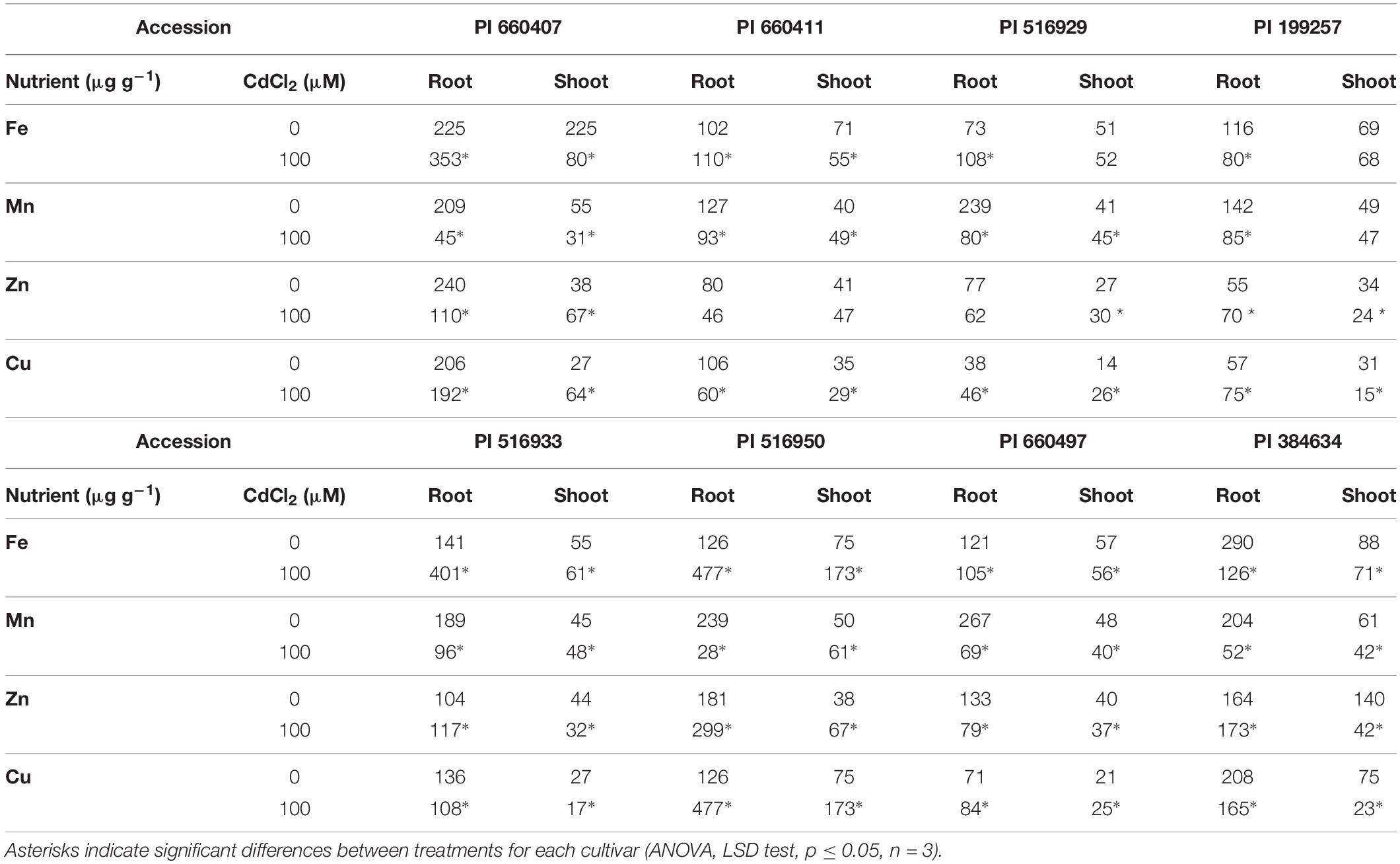
Table 3. Micronutrients content in Cd-tolerant and Cd-sensitive accessions of M. truncatula grown in hydroponic pouches with 0 or 100 μM CdCl2 for 12 days.
Contrasting Responses to Cd Stress of Two Accessions With Different Sensitivity to Cd
The responses to Cd stress were analyzed in two accessions classified as Cd-tolerant (CdT; PI 516929) and Cd-sensitive (CdS; PI 660497), respectively. Both accessions were similar in size in the absence of Cd and presented similar accumulation of Cd in roots and in shoots following Cd exposure.
We analyzed the effect of Cd on germination and on the α-amylase activity in cotyledons, as an indicator of reserves mobilization. Cd promoted a significant reduction of CdS germination (23%), but it did not affect the germination of CdT (Figure 5A). In the absence of the metal, CdS was able to hydrolyze a significantly higher quantity of starch than CdT (Figure 5B). Cd induced a significant reduction in starch hydrolysis in both accessions, although this reduction was significantly higher in CdS (64%) than in CdT (26%).
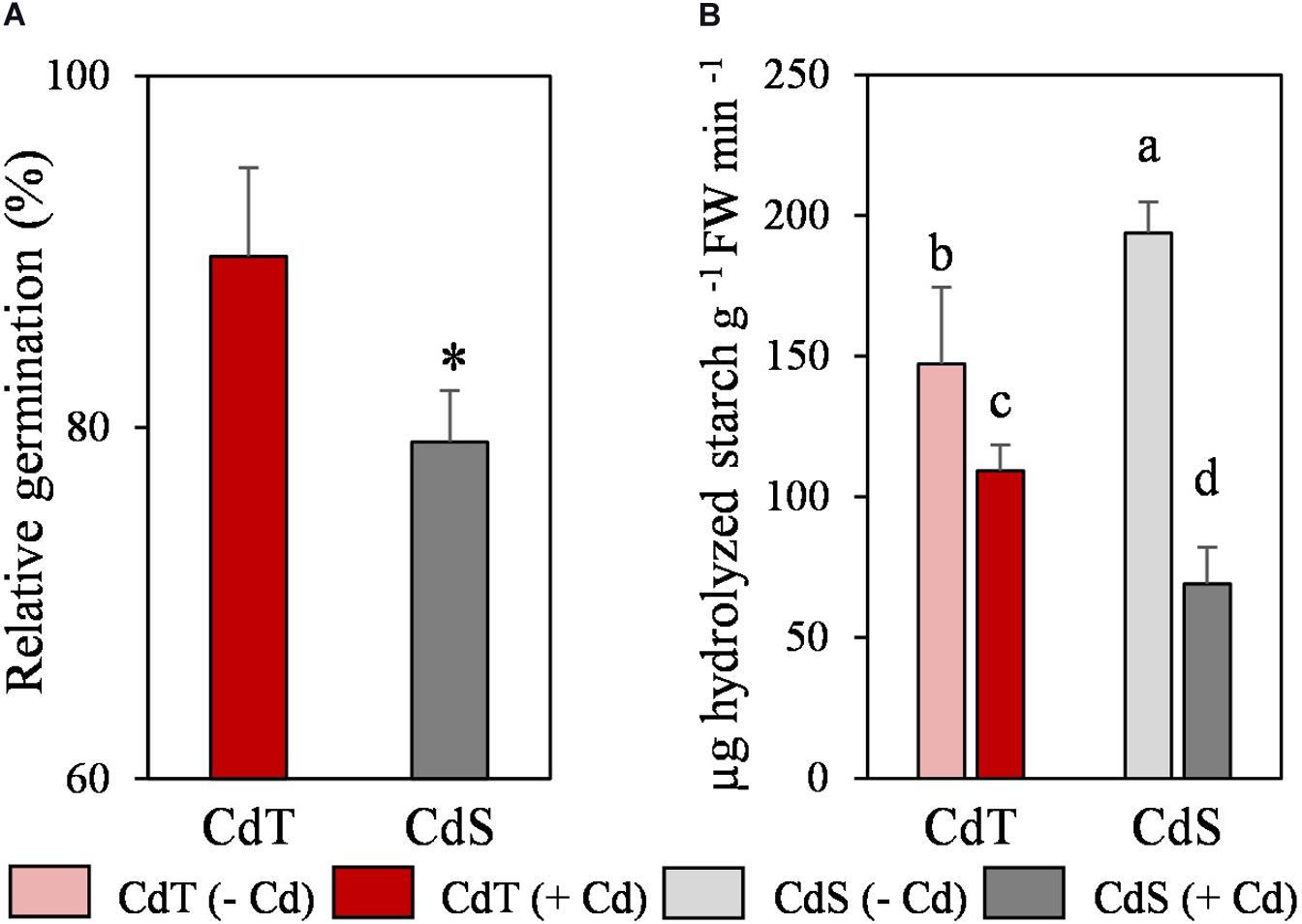
Figure 5. Relative germination in the presence of Cd (200 μM CdCl2, 72 h) (A) and α-amylase activity in cotyledons (B) of M. truncatula cultivars CdT (tolerant) and CdS (sensitive). Mean values ± standard deviation are represented. * Indicates significant differences between presence and absence of Cd for each accession [Pearsons’s chi-squared test (χ2), n = 4]. Different letters indicate significant differences between cultivars and treatments (Tukey HSD test, p ≤ 0.05, n = 4–6).
To investigate the differences between CdT and CdS in the antioxidant defense response to Cd stress, the expression of some genes that codify for enzymes involved in the antioxidant machinery and PC biosynthesis was studied in seedling roots exposed to 0 or 50 μM CdCl2 for 12 h.
In the absence of Cd, the two accessions showed different expression levels for some genes (Figure 6A). Different expression patterns were also observed in CdT and CdS after Cd exposure. The roots of Cd-treated CdT seedlings displayed enhanced expression of MtCAT, MtγECS, and MtMR and of the three genes encoding the NADPH-generating enzymes MtG6PDH, Mt6PGDH, and MtICDH, as compared to control unstressed seedling roots (Figure 6B). In contrast, Cd significantly reduced transcript accumulation of MtCuZnSOD a and c and MtGalLDH in CdS roots. Most of the players in GSH and phytochelatin biosynthesis strongly increased their expression in CdS roots (MtCYS, MtγECS, MtGSHS, MtGR, and MtG6PDH) (Figure 6C). The different Cd tolerance of the two accessions might be related to these variations in gene expression.
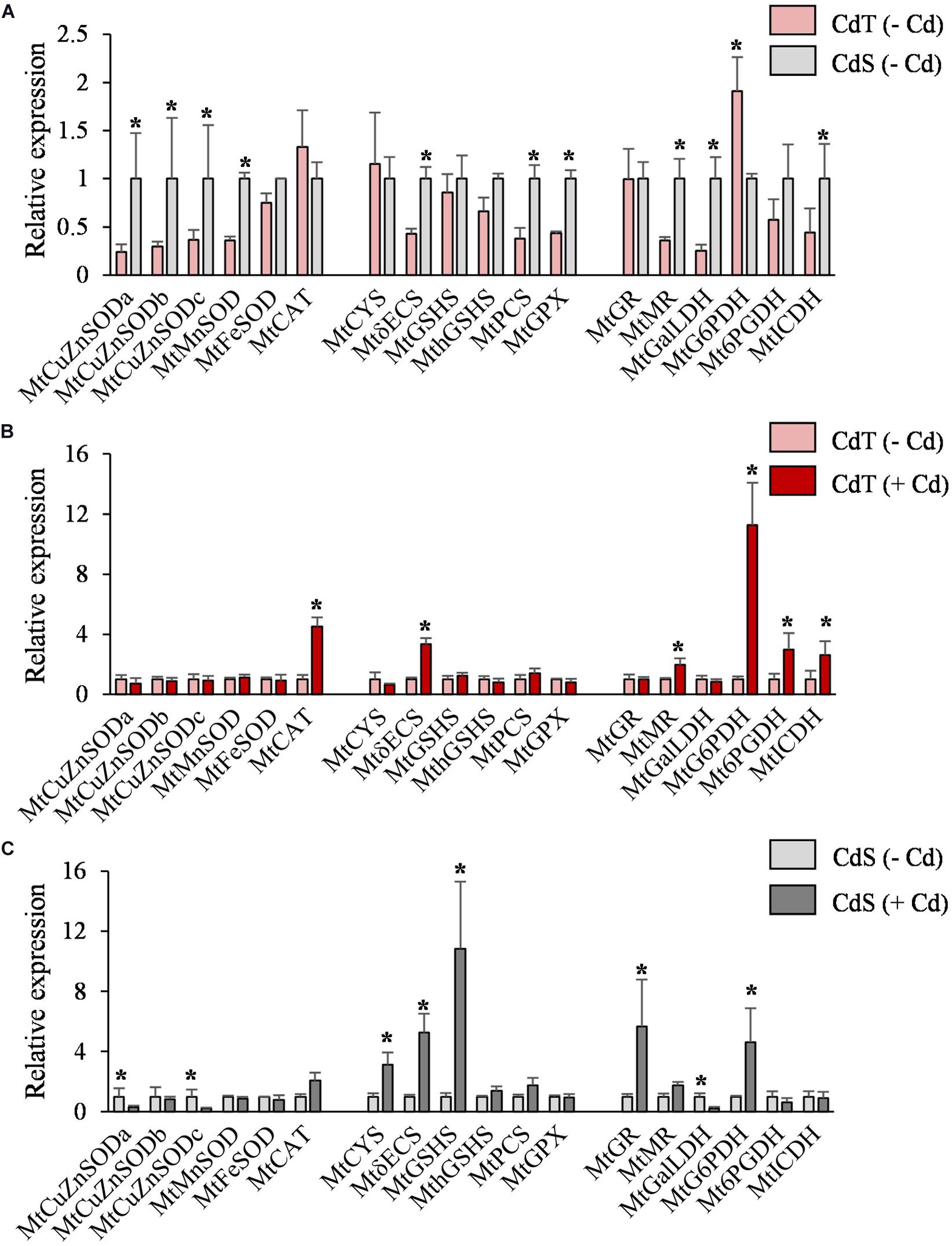
Figure 6. Transcript accumulation of genes involved in the antioxidant machinery and NADPH biosynthesis in seedling roots of M. truncatula cultivars CdT (tolerant) and CdS (sensitive) grown in glass containers in the absence or presence of 50 μM CdCl2 for 12 h. (A) Transcript accumulation for CdT and CdS in absence of Cd. (B) Effect of Cd on transcript accumulation in CdT roots. (C) Effect of Cd on transcript accumulation in CdS roots. Mean values ± standard deviation are represented (n = 4). * Denotes fold-changes greater than two.
To obtain deeper insight into the redox state of the plants, oxidized glutathione (GSSG), reduced glutathione (GSH), and GSH/GSSG ratio were analyzed in CdT and CdS seedling roots (Figure 7). In the absence of Cd, the GSH/GSSG ratio was significantly higher in CdS roots. Cd promoted a significant reduction in glutathione content in both accessions, but the GSH/GSSH ratio increased significantly in Cd-treated CdT roots when compared with control roots, while the ratio did not change upon Cd stress in CdS roots.

Figure 7. Oxidized glutathione (GSSG) (A), reduced glutathione (GSH) (B), and GSH/GSSG ratio (C) in seedling roots of M. truncatula cultivars CdT (tolerant) and CdS (sensitive) grown in glass containers in the absence or presence of 50 μM CdCl2 for 12 h. Mean values ± standard deviation are represented. Different letters indicate significant differences (Tukey HSD test, p ≤ 0.05, n = 4). * Denotes significant differences between control and Cd treatment.
We estimated the oxidative damage caused by Cd on CdT and CdS seedling roots by histochemical detection of lipid peroxidation and plasma membrane integrity. Cd promoted lipid peroxidation in both cultivars but with different intensity and localization (Figures 8A,B). In Cd-treated CdS roots, the staining was found along the entire root and was especially intense in the first 5 mm of the root apex, while in CdT-treated roots, lipid peroxidation was located on the first millimeter of the root apex, suggesting lower oxidative damage than that observed in CdS roots. Cd also had a contrasting effect on the plasma membrane integrity of CdT and CdS seedling roots (Figures 8C,D). Cd-treated CdS roots showed loss of plasma membrane integrity along the whole root. Contrariwise, Cd-treated CdT roots displayed minimal loss of plasma membrane integrity along the root surface and the root apex was not affected (around 4 mm), suggesting again less oxidative damage produced by Cd in the roots of the tolerant cultivar.
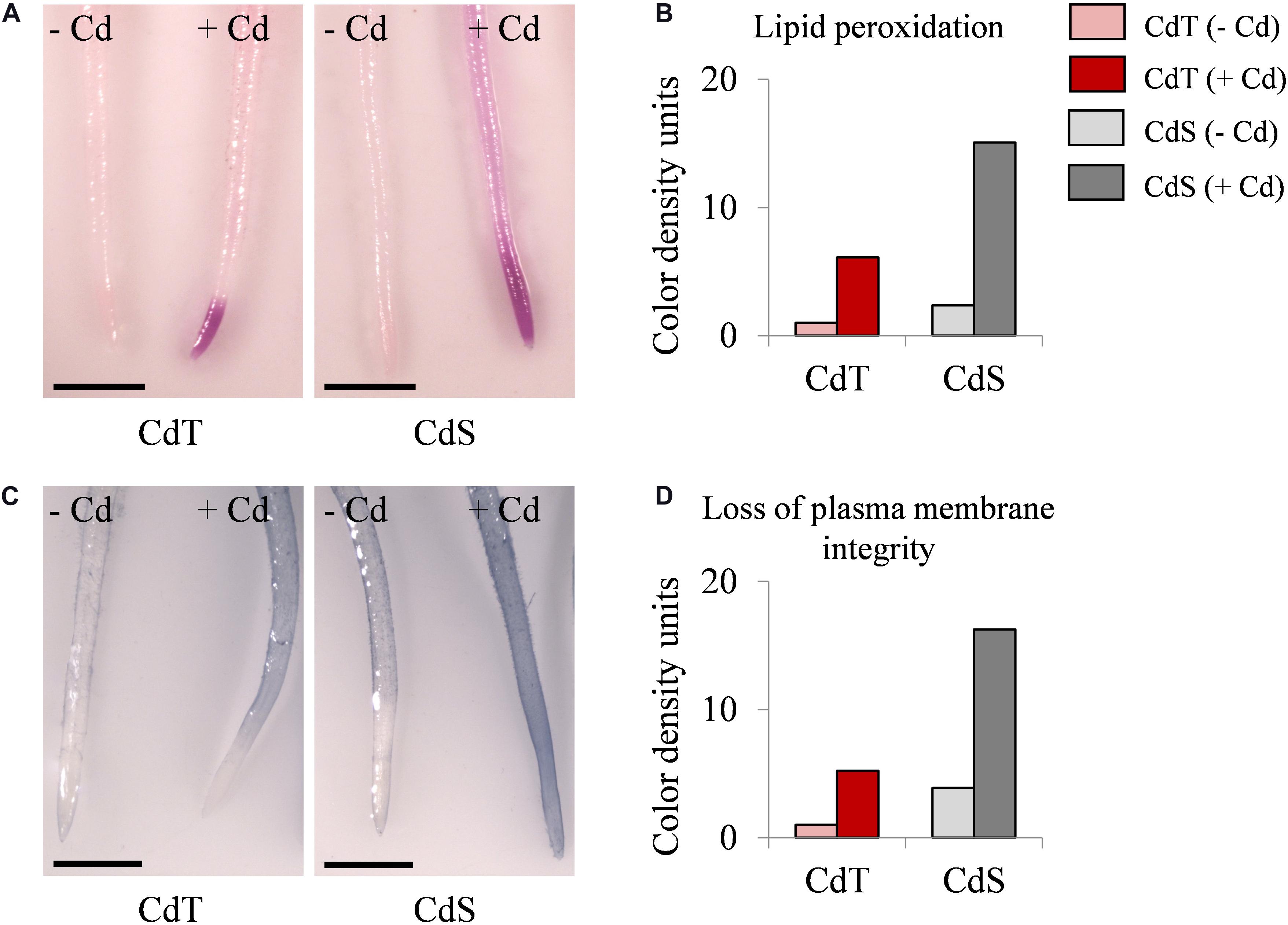
Figure 8. Lipid peroxidation and loss of plasma membrane integrity in seedling roots of M. truncatula cultivars CdT (tolerant) and CdS (sensitive) grown in glass containers in the absence or presence of 100 μM CdCl2 for 12 h. (A) Staining with Schiff’s reagent for lipid peroxidation assessment. (C) Staining with Evans Blue for plasma membrane integrity assessment. Quantification of color developed with Schiff’s reagent (B) and Evans Blue (D) on 1 cm root tips. Scale bar corresponds to 1 mm.
To determine whether CdT and CdS presented differences in their response after a longer exposure to Cd, CdT and CdS plants were grown in the presence or absence of Cd stress for 15 days. Clear differences in plant growth were observed between both cultivars after Cd stress (Figure 9). The lipid peroxidation (MDA content) and the SOD and CAT enzymatic activities were analyzed in roots (Figures 10A–C) and shoots (Figures 10D–F). Cd induced a significant increase of MDA levels in roots of both cultivars. In the absence of Cd, CdS presented a significantly higher MDA content in shoots compared with CdT, and Cd caused a significant increase in MDA content only in CdS shoots.
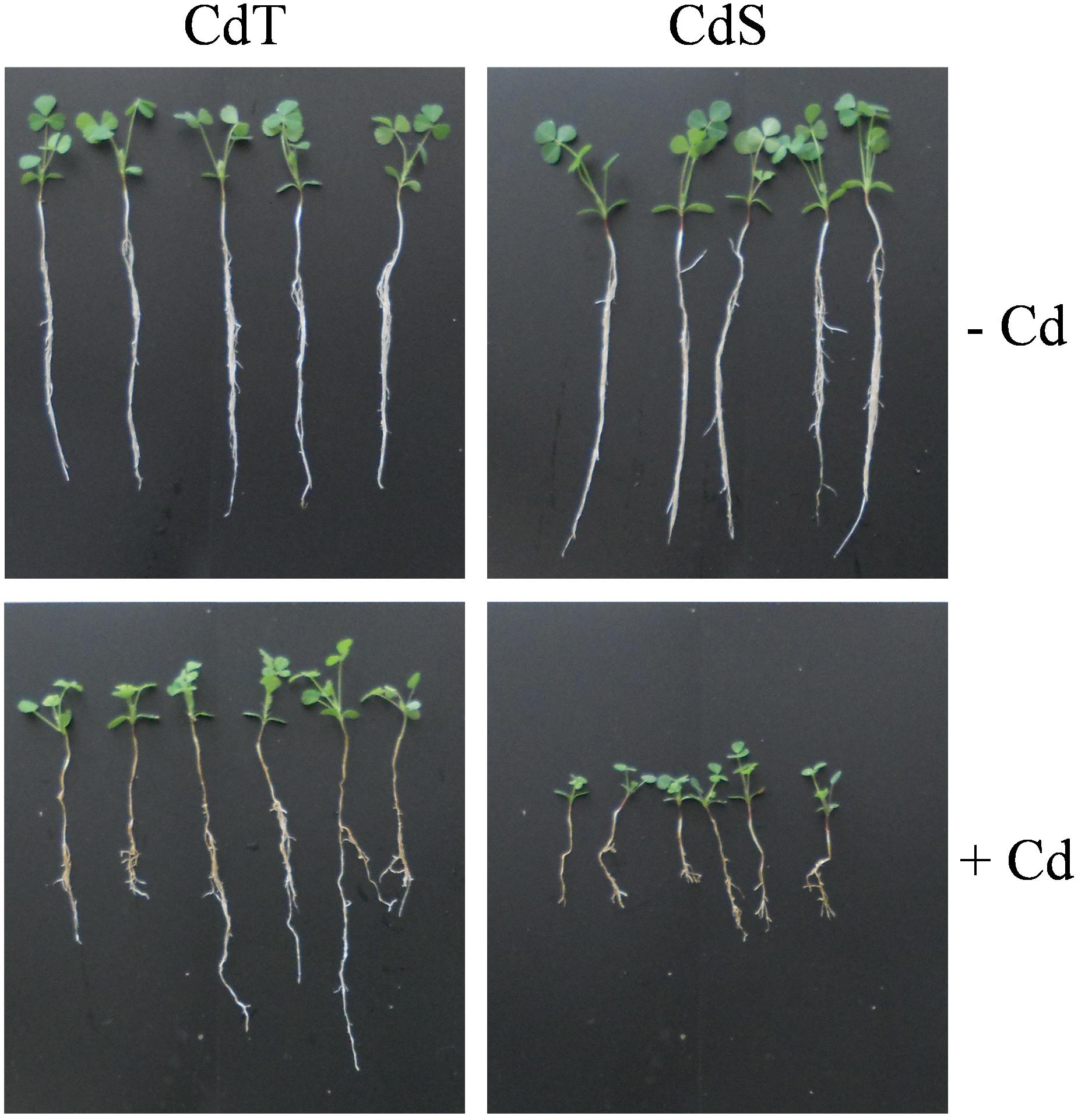
Figure 9. Representative image of the plant growth M. truncatula cultivars CdT (tolerant) and CdS (sensitive) grown in pots containing vermiculite with 0 or 100 μM CdCl2 for 15 days.
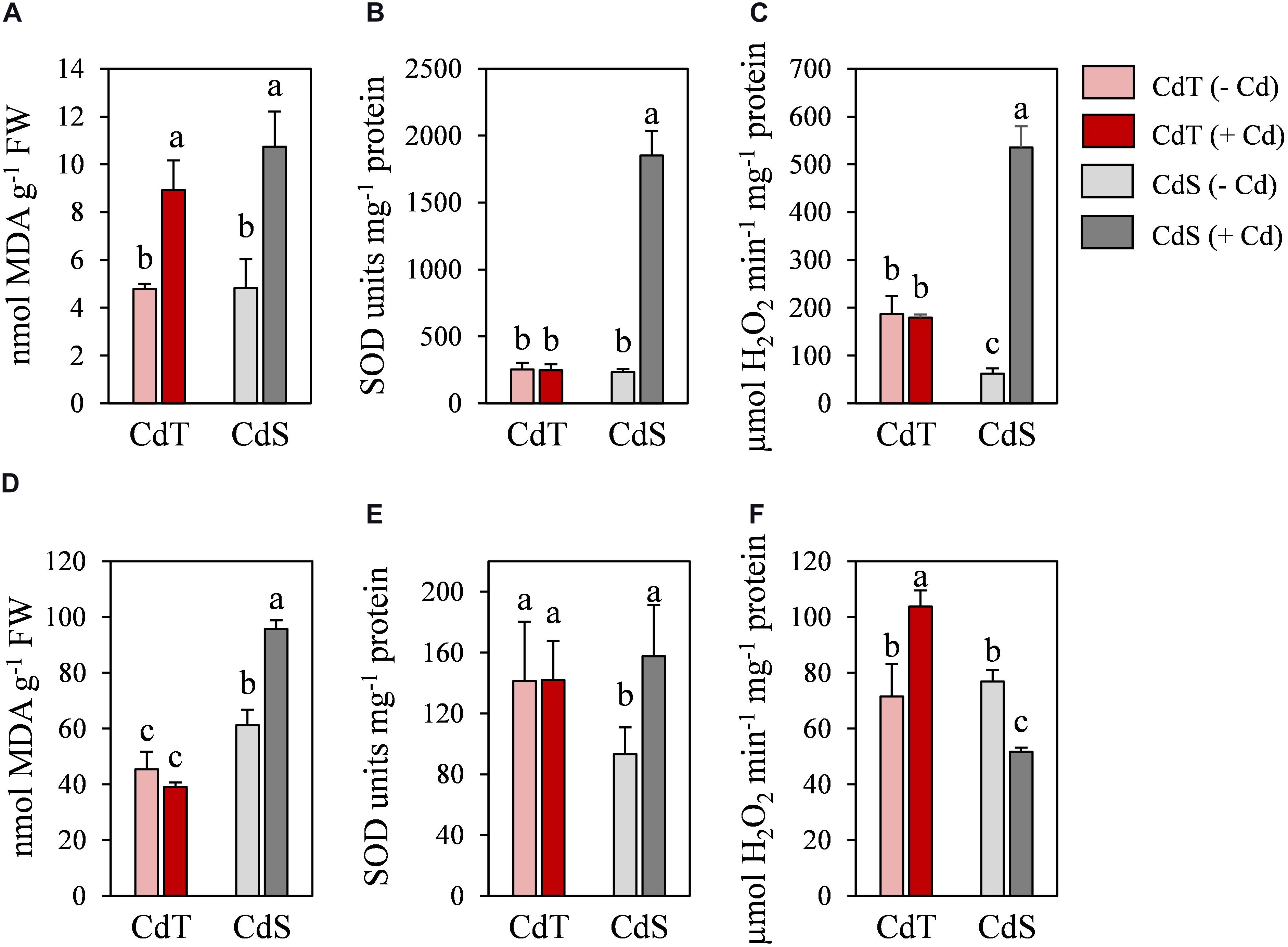
Figure 10. Lipid peroxidation (MDA), and SOD and catalase activities in M. truncatula cultivars CdT (tolerant) and CdS (sensitive) grown in pots containing vermiculite with 0 or 100 μM CdCl2 for 15 days. (A–C) Roots. (D–F) Shoots. Different letters indicate significant differences (Tukey HSD test, p ≤ 0.05, n = 4).
In the absence of Cd, no significant differences were observed in SOD activity in roots between accessions, while in shoots, CdT presented a significantly higher SOD activity compared with CdS. Cd induced a remarkable increase of the SOD activity in CdS roots and shoots, while CdT SOD activities were unaffected (Figures 10B,E).
In the absence of Cd, CAT activity was significantly higher in CdT roots than in CdS roots (Figures 10C,F). The Cd treatment induced a very significant CAT activity increment in CdS roots and CdT shoots and a significant decrease of CAT activity in CdS shoots (Figure 10F).
Discussion
Cd toxicity is directly associated to cellular redox imbalance and ROS accumulation. Plant tolerance mechanisms and responses to Cd vary considerably among species and cultivars (Rizwan et al., 2017; Hasanuzzaman et al., 2019; Romero-Puertas et al., 2019). In this work, we evaluated Cd tolerance in a M. truncatula germplasm and identified tolerant genotypes. We analyzed several Cd toxicity and Cd tolerance markers in accessions displaying contrasted sensitivity to Cd. Relative root growth (RRG) has been described as a very suitable indicator of heavy metal tolerance (Sledge et al., 2005; Saeidi et al., 2012; García de la Torre et al., 2013; Rahoui et al., 2014). The screening of 258 M. truncatula accessions performed here showed a notable intraspecific variability in the response to Cd within M. truncatula, which suggests that cultivars with even higher tolerance could be detected by screening larger germplasm collections.
We focused our study on two M. truncatula accessions, CdT (tolerant) and CdS (sensitive) that presented similar growth in control conditions, and similar Cd accumulation in their roots and shoots, but remarkable differences in their growth under Cd stress. We analyzed the effect of Cd stress at two stages of plant development: seed germination and vegetative growth (seedlings and 25-day-old plants). Germination is considered a very Cd-sensitive process in comparison with other plant developmental stages (Rahoui et al., 2008), although large differences can exit among plant species and also cultivars (Huybrechts et al., 2019). During germination, reserves mobilization is required to allow plant emergence, and Cd may provoke changes in membrane composition, antioxidant status, and electrical conductivity that might promote nutrient leakages during germination (Sfaxi-Bousbih et al., 2010). Here we showed that both germination and reserves mobilization (starch hydrolysis) were more affected in CdS than in CdT under Cd stress. Significant restrictions in starch mobilization have also been observed in seeds of various Cd-sensitive legume cultivars (Mihoub et al., 2005; Rahoui et al., 2008). Rahoui et al. (2015) suggested that genotype-dependent negative effects of Cd on reserve mobilization, respiration recovery, and nutrient transport during seed germination might explain the different susceptibility to Cd in several lines of M. truncatula.
Cadmium uptake causes an imbalance in the nutrient metabolism (uptake, transport, and use) at the root level, probably by competition with the absorption and transport of essential elements, in particular Ca2+, Fe2+, Mg2+, Mn2+, Cu2+, and Zn2+ (DalCorso et al., 2008; Loix et al., 2017; Ismael et al., 2019; Qin et al., 2020). Our results on nutrients contents suggest that Cd could be using different transporters, such as calcium, zinc, and/or iron transporters, as previously described (Thomine et al., 2000; Rodríguez-Serrano et al., 2009; Ismael et al., 2019). Stephens et al. (2011) reported that in M. truncatula, Zn uptake was inhibited by Cd. Sulfur is an essential macronutrient, key in protein synthesis, and an important structural component of many co-enzymes and thiol compounds that play important roles in stress tolerance (Ernst et al., 2008). The increase in S content under Cd stress found in CdT roots could contribute to its tolerance, as S assimilation activates the pathway leading to the synthesis of Cys, which is precursor of GSH and other thiol-containing compounds, and it is induced by Cd stress (Baig et al., 2019).
Antioxidant metabolism is critical in Cd tolerance (Terrón-Camero et al., 2020). SODs and CAT represent the first line of response to ROS to maintain the cell redox balance. Enhanced transcript accumulation of SODs after Cd treatment has been described in some M. truncatula cultivars, but also the contrary effect in a Cd-susceptible accession (Rahoui et al., 2014). In the present work, we observed repression of MtCuZnSODa and MtCuZnSODc in CdS seedlings, which could be related to the decrease in zinc content under Cd stress; SOD activity was not affected in CdT, and CAT expression was increased in CdT seedlings only. These results evidence differences in the response to Cd of both cultivars.
γ-ECS is a limiting enzyme for GSH synthesis; its activity is GSH regulated and dependent on Cys availability. It has been proposed that a high γECS expression could compensate GSH deficiency under heavy metal stress (Sobrino-Plata et al., 2014). In CdT seedlings, Cd increased MtγECS transcript accumulation, but the rest of transcripts related to GSH and PCs biosynthesis (MtCYS, MtGSHS, and MtPCS) remained unaffected. On the contrary, in CdS, Cd provoked an increase in transcript accumulation of MtCYS, MtγECS, MtGSHS, as well as MtGR, which codes for a glutathione reductase, a significant enzyme in maintaining redox homeostasis via a correct GSH/GSSG ratio. This suggest that Cd tolerance is not so much dependent on phytochelatin quelation in tolerant plants, which is a common response to Cd stress in sensitive species as previously reported (Schat et al., 2002).
Glutathione plays a central role as chelator, antioxidant, and signaling molecule (Jozefczak et al., 2012). Cd caused a reduction in GSSG and GSH contents in the roots of both accessions and an increase in GSH/GSSG ratio in CdT, but not in CdS. These results are in agreement with the decrease in glutathione content without changes in GSH/GSSG ratio reported for a Cd-sensitive M. sativa cultivar (Sobrino-Plata et al., 2009).
NADPH is important in the defense against oxidative stress (Foyer and Noctor, 2011). It has been reported that Cd tolerance might be more dependent on NADPH availability than on its antioxidant capacity (León et al., 2002). The upregulation of gene expression and increased activities of NADPH-recycling dehydrogenases have been reported in M. truncatula nodules and soybean roots in response to Cd (Marino et al., 2013; Pérez-Chaca et al., 2014). The enhanced expression of the genes encoding three NADPH-recycling enzymes in CdT strongly suggests that it might represent an important defense mechanism, which appears to be absent in CdS.
Following Cd exposure, oxidative stress is considered as the origin of damage in plant tissues (Schützendübel et al., 2002; Rahoui et al., 2017). Cd-induced lipid peroxidation has been reported in M. truncatula (Sobrino-Plata et al., 2009; Rahoui et al., 2017). In CdS seedling roots, Cd produced higher lipid peroxidation and loss of cell membrane integrity than in CdT. Taken together, our results on seedlings indicate that Cd promoted a more severe oxidative damage in CdS than in CdT, which was able to better neutralize ROS production. In agreement with our results, a correlation between Cd tolerance and low oxidative stress at the seedling stage has been described in tolerant M. truncatula accessions (Rahoui et al., 2014).
Metal tolerance and/or toxicity also depend on the plant developmental stage. We analyzed the differences in tolerance of 25-day-old CdT and CdS plants after a long exposure to Cd. Lipid peroxidation (MDA content) and SOD and CAT activities were determined in roots and shoots. MDA content was unaffected in CdT shoots, and SOD activity was also not affected in CdT roots or shoots. Catalase activity significantly increased in CdT shoots, which could explain why lipid peroxidation did not increase in CdT shoots. In CdS roots, catalase activity increased, while a decrease was observed in shoots. The consequent accumulation of H2O2 seems to be key in inducing growth reduction associated to Cd stress (Schützendübel et al., 2002).
Figure 11 summarizes our results on gene expression, enzyme activity, and antioxidant content. A contrasting behavior of the two cultivars can be observed.
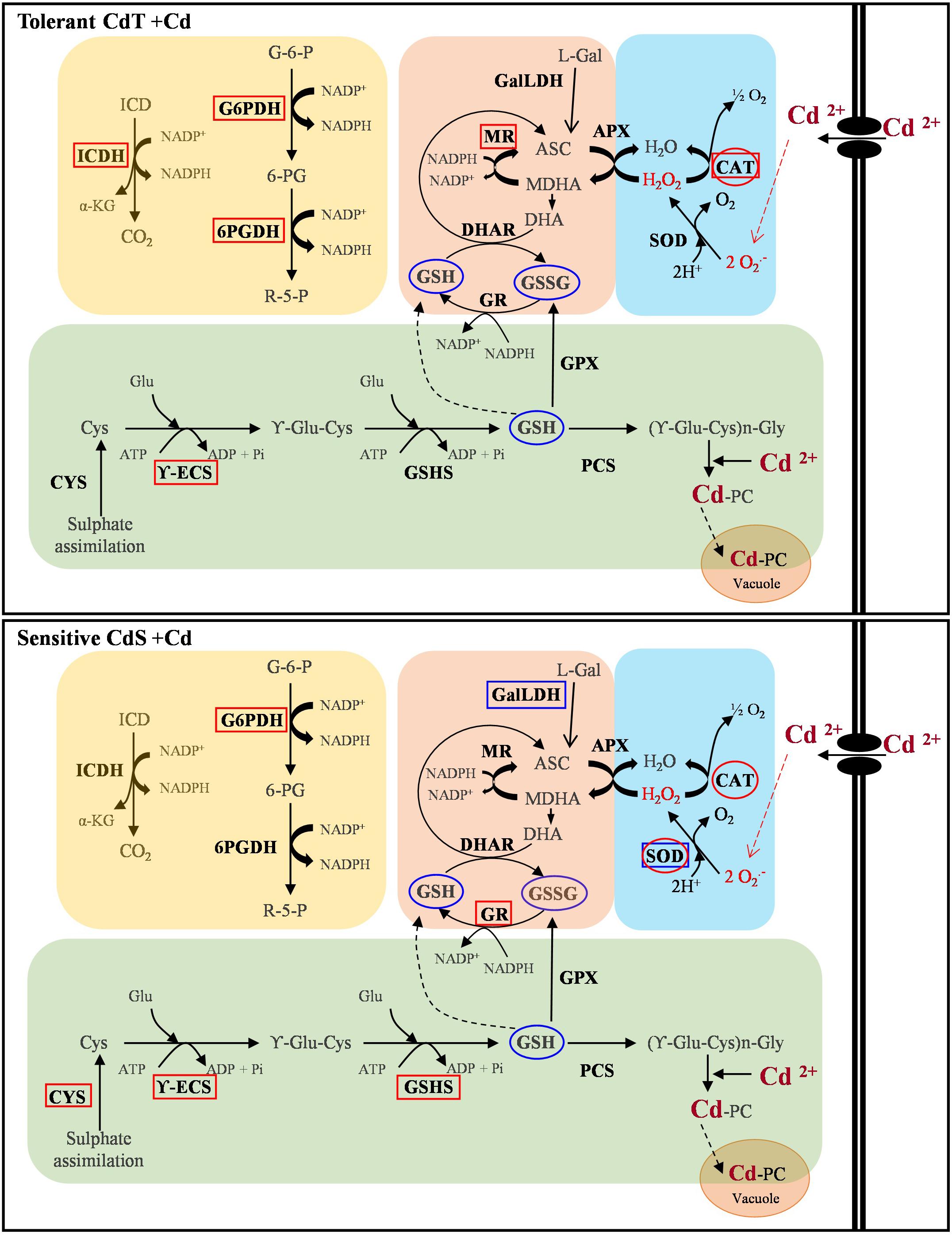
Figure 11. Schematic overview of the contrasting responses observed in M. truncatula cultivars CdT (tolerant) and CdS (sensitive) upon Cd stress relative to gene expression, enzyme activity, and antioxidant content. Analyzed parameter is in bold letter. Rectangles denote a Cd effect on the expression of the gene encoding the enzyme. Ovals indicate a Cd effect on the enzymatic activity or content of non-enzymatic antioxidants. Red and blue colors denote increase and decrease, respectively, as compared with non-Cd treated plants. ASC, ascorbate; CAT, catalase; Cys, cysteine; CYS, cysteine synthase; DHA, dehydroascorbate; DHAR, dehydroascorbate reductase; GalLDH, galactono-1,4-lactone dehydrogenase; Glu, glutamic; G6P, glucose-6-phosphate; G6PDH, glucose-6-phosphate dehydrogenase; GPX, glutathione peroxidase; GR, glutathione reductase; GSSG, oxidized glutathione; GSH, reduced glutathione; GSHS, glutathione synthetase; ICD, isocitrate; ICDH, isocitrate dehydrogenase; L-Gal, L-galactono-lactone; MDHA, monodehydroascorbate; MR, monodehydroascorbate reductase; PCS, phytochelatin synthase; R5P, ribulose-5-phosphate; SOD, superoxide dismutase; 6PGDH, 6-phosphogluconate dehydrogenase; γ-ECS, γ-glutamyl-cysteine synthetase; α-KG, α-ketoglutarate.
In summary, CdT was more tolerant to Cd stress than CdS at all developmental stages studied. Both cultivars shared an increased CAT activity. In CdS, SOD increased in response to primary oxidative stress, while it did not seem to play a crucial role in CdT response. Their additional response mechanisms were different. CdT ensured availability of NADPH by increasing expression of NADPH-recycling enzymes, and, unexpectedly, presented no induction of the PC biosynthesis pathway. On the contrary, CdS upregulated the expression of three of the four genes involved in PC biosynthesis, but not those responsible for NADPH recycling. NADPH availability appears as the crucial factor in Cd tolerance in M. truncatula. PCs represent a common response to heavy metals, but depending on the stress level, they might not be sufficient. Conversely, when alternative factors are activated, PCs increase might not be necessary.
Our results point at the possibility of identifying highly tolerant cultivars with additional traits, such as high or low accumulation and translocation. The number of M. truncatula accessions available in different collections worldwide is above 6,000. Forage legumes present an important potential to be used in heavy-metal phytoremediation. Cultivars with high levels of the enzymes involved in NADPH production could be used to transfer the tolerance trait to cultivated M. truncatula, alfalfa, or other legumes. Additionally, a collection of M. truncatula mutant lines is available from the Noble Research Institute3, and there are over 300 fully sequenced M. truncatula cultivars that can be obtained from the Medicago Hapmap Project Germplasm4. These resources will facilitate the identification and analysis of genes and loci involved in Cd tolerance and accumulation, and a better understanding of Cd tolerance and detoxifying mechanisms.
Data Availability Statement
The original contributions presented in the study are included in the article and the Supplementary Material. Further inquiries can be directed to the authors.
Author Contributions
VSGT, TCP, JJP, and MML conceived the experiments. VSGT performed the experiments and wrote the first draft. TCP contributed to the experimental part. JJP and MML supervised and contributed to the experiments. TCP, JJP, and MML wrote the last version of the manuscript. All authors read and approved the submitted version.
Funding
This work was supported by Comunidad de Madrid (Grant S2009/AMB-151 to MML, and a contract to TCP), MINECO (Grants AGL2013-40758-R to JJP and MML), AEI/FEDER-UE (Grant AGL2017-88381-R to JJP and MML), and Fundación Areces (Grant to JJP). VSGT was the recipient of an FPU fellowship from the Spanish Ministry of Education.
Conflict of Interest
The authors declare that the research was conducted in the absence of any commercial or financial relationships that could be construed as a potential conflict of interest.
Acknowledgments
We thank L. Barrios for her help with statistical analyses, and the National Plant Germplasm System, USDA for providing us with the M. truncatula accessions. We acknowledge support of 25% of the publication fee by the CSIC Open Access Publication Support Initiative through its Unit of Information Resources for Research (URICI).
Supplementary Material
The Supplementary Material for this article can be found online at: https://www.frontiersin.org/articles/10.3389/fpls.2021.595001/full#supplementary-material
Footnotes
- ^ https://bioinfo.ut.ee/primer3-0.4.0/
- ^ http://imagej.nih.gov/ij/
- ^ https://www.noble.org/
- ^ http://www.medicagohapmap.org/
References
Aloui, A., Recorbet, G., Gollotte, A., Robert, F., Valot, B., Gianinazzi-Pearson, V., et al. (2009). On the mechanisms of cadmium stress alleviation in Medicago truncatula by arbuscular mycorrhizal symbiosis: a root proteomic study. Proteomics 9, 420–433. doi: 10.1002/pmic.200800336
Aloui, A., Recorbet, G., Robert, F., Schoefs, B., Bertrand, M., Henry, C., et al. (2011). Arbuscular mycorrhizal symbiosis elicits shoot proteome changes that are modified during cadmium stress alleviation in Medicago truncatula. BMC Plant Biol. 11:75. doi: 10.1186/1471-2229-11-75
Baig, M. A., Ahmad, J., Ali, A. A., and Quresh, M. I. (2019). “Role of sulfur metabolism in cadmium tolerance,” in Cadmium Tolerance in Plants: Agronomic, Molecular, Signaling, and Omic Approaches, eds M. Hasanuzzaman, M. N. V. Prasad, and K. Nahara (Amsterdam: Elsevier Inc), 335–365.
Benavides, M., Gallego, S. M., and Tomaro, M. (2005). Cadmium toxicity in plants. Braz. J. Plant Physiol. 17, 21–34. doi: 10.1590/S1677-04202005000100003
Clemens, S., Aarts, M., Thomine, S., and Verbruggen, N. (2013). Plant science: the key to preventing slow cadmium poisoning. Trends Plant Sci. 18, 92–99. doi: 10.1016/j.tplants.2012.08.003
Coba de la Peña, T., and Pueyo, J. J. (2012). Legumes in the reclamation of marginal soils, from cultivar and inoculant selection to transgenic approaches. Agron. Sustain. Dev. 32, 65–91. doi: 10.1007/s13593-011-0024-2
Cuypers, A., Plusquin, M., Remans, T., Jozefczak, M., Keunen, E., Gielen, H., et al. (2010). Cadmium stress: an oxidative challenge. Biometals 23, 927–940. doi: 10.1007/s10534-010-9329-x
DalCorso, G., Farinati, S., Maistri, S., and Furini, A. (2008). How plants cope with cadmium: staking all on metabolism and gene expression. J. Integr. Plant Biol. 50, 1268–1280. doi: 10.1111/j.1744-7909.2008.00737.x
Ernst, W., Krauss, G., Verkleij, J. A. C., and Wesenberg, D. (2008). Interaction of heavy metals with the sulphur metabolism in angiosperms from an ecological point of view. Plant Cell Environ. 31, 123–143. doi: 10.1111/j.1365-3040.2007.01746.x
Floreani, M., Petrone, M., Debetto, P., and Palatini, P. (1997). A comparison between different methods for the determination of reduced and oxidized glutathione in mamalian tissues. Free Radic. Res. 26, 449–445. doi: 10.3109/10715769709084481
Foyer, C. H., and Noctor, G. (2011). Ascorbate and glutathione: the heart of the redox hub. Plant Physiol. 155, 2–18. doi: 10.1104/pp.110.167569
García de la Torre, V. S., Coba de la Peña, T., Lucas, M. M., and Pueyo, J. J. (2013). Rapid screening of Medicago truncatula germplasm for mercury tolerance at the seedling stage. Environ. Exp. Bot. 91, 90–96. doi: 10.1016/j.envexpbot.2013.03.004
Hasanuzzaman, M., Prasad, M. N. V., and Nahara, K. (2019). Cadmium Tolerance in Plants: Agronomic, Molecular, Signaling, and Omic Approaches. Amsterdam: Elsevier Inc.
Holdaway, J., and Wang, W. (2018). From soil pollution to “cadmium Rice” to public health impacts: an interdisciplinary analysis of influencing factors and possible responses. J. Resour. Ecol. 9, 1–12. doi: 10.5814/j.issn.1674-764x.2018.01.001
Huybrechts, M., Cuypers, A., Deckers, J., Iven, V., Vandionant, S., Jozefczak, M., et al. (2019). Cadmium and plant development: an agony from seed to seed. Int. J. Mol. Sci. 20:3971. doi: 10.3390/ijms20163971
Ismael, M. A., Elyamine, A. M., Moussa, M. G., Cai, M., Zhao, X., and Hu, C. (2019). Cadmium in plants: uptake, toxicity, and its interactions with selenium fertilizers. Metallomics 11, 255–277. doi: 10.1039/c8mt00247a
Jozefczak, M., Remans, T., Vangronsveld, J., and Cuypers, A. (2012). Glutathione is a key player in metal-induced oxidative stress defenses. Int. J. Mol. Sci. 13, 3145–3175. doi: 10.3390/ijms13033145
León, A., Palma, J., Corpas, F., Gomez, M., Romero-Puertas, M., Chatterjee, D., et al. (2002). Antioxidative enzymes in cultivars of pepper plants with different sensitivity to cadmium. Plant Physiol. Biochem. 40, 813–820. doi: 10.1016/S0981-9428(02)01444-4
Loix, C., Huybrechts, M., Vangronsveld, J., Gielen, M., Keunen, E., and Cuypers, A. (2017). Reciprocal interactions between cadmium-induced cell wall responses and oxidative stress in plants. Front. Plant Sci. 8:1867. doi: 10.3389/fpls.2017.01867
Marino, D., Damiani, I., Gucciardo, S., Mijangos, I., Pauly, N., and Puppo, A. (2013). Inhibition of nitrogen fixation in symbiotic Medicago truncatula upon Cd exposure is a local process involving leghaemoglobin. J. Exp. Bot. 64, 5651–5660. doi: 10.1093/jxb/ert334
Marino, D., González, E. M., Frendo, P., Puppo, A., and Arrese-Igor, C. (2007). NADPH recycling systems in oxidative stressed pea nodules: a key role for the NADP+ -dependent isocitrate dehydrogenase. Planta 225, 413–421. doi: 10.1007/s00425-006-0354-5
Mihoub, A., Chaoui, A., and El Ferjani, E. (2005). Biochemical changes associated with cadmium and copper stress in germinating pea seeds (Pisum sativum L.). C. R. Biol. 328, 33–41. doi: 10.1016/j.crvi.2004.10.003
Peralta-Videa, J. R., Lopez, M. L., Narayan, M., Saupe, G., and Gardea-Torresdey, J. (2009). The biochemistry of environmental heavy metal uptake by plants: implications for the food chain. Int. J. Biochem. Cell Biol. 41, 1665–1677. doi: 10.1016/j.biocel.2009.03.005
Pérez-Chaca, M. V., Rodríguez-Serrano, M., Molina, A. S., Pedranzani, H. E., Zirulnik, F., Sandalio, L. M., et al. (2014). Cadmium induces two waves of reactive oxygen species in Glycine max (L.) roots. Plant Cell Environ. 37, 1672–1687. doi: 10.1111/pce.12280
Pfaffl, M. W. (2001). A new mathematical model for relative quantification in real-time RT-PCR. Nucleic Acids Res. 29, 2002–2007. doi: 10.1093/nar/29.9.e45
Qin, S. Y., Liu, H. E., Nie, Z. J., Rengel, Z., Gao, W., Li, C., et al. (2020). Toxicity of cadmium and its competition with mineral nutrients for uptake by plants: a review. Pedosphere 30, 168–180. doi: 10.1016/S1002-0160(20)60002-9
Rahoui, S., Ben, C., Chaoui, A., Martinez, Y., Yamchi, A., Rickauer, M., et al. (2014). Oxidative injury and antioxidant genes regulation in cadmium-exposed radicles of six contrasted Medicago truncatula genotypes. Environ. Sci. Pollut. Res. 21, 8070–8083. doi: 10.1007/s11356-014-2718-x
Rahoui, S., Chaoui, A., Ben, C., Rickauer, M., Gentzbittel, L., and El Ferjani, E. (2015). Effect of cadmium pollution on mobilization of embryo reserves in seedlings of six contrasted Medicago truncatula lines. Phytochemistry 111, 98–106. doi: 10.1016/j.phytochem.2014.12.002
Rahoui, S., Chaoui, A., and El Ferjani, E. (2008). Differential sensitivity to cadmium in germinating seeds of three cultivars of faba bean (Vicia faba L.). Acta Physiol. Plant. 30, 451–456. doi: 10.1007/s11738-008-0142-x
Rahoui, S., Martinez, Y., Sakouhi, L., Ben, C., Rickauer, M., El Ferjani, E., et al. (2017). Cadmium-induced changes in antioxidative systems and differentiation in roots of contrasted Medicago truncatula lines. Protoplasma 254, 473–489. doi: 10.1007/s00709-016-0968-9
Redondo, F. J., Coba de la Peña, T., Morcillo, C. N., Lucas, M. M., and Pueyo, J. J. (2009). Overexpression of flavodoxin in bacteroids induces changes in antioxidant metabolism leading to delayed senescence and starch accumulation in alfalfa root nodules. Plant Physiol. 149, 1166–1178. doi: 10.1104/pp.108.129601
Rizwan, M., Ali, S., Adrees, M., Ibrahim, M., Tsang, D. C. W., Zia-ur-Rehman, M., et al. (2017). A critical review on effects, tolerance mechanisms and management of cadmium in vegetables. Chemosphere 182:90e105. doi: 10.1016/j.chemosphere.2017.05.013
Rodríguez-Eugenio, N., McLaughlin, M., and Pennock, D. (2018). Soil Pollution: A Hidden Reality. Rome: FAO.
Rodríguez-Serrano, M., Romero-Puertas, M. C., Pazmiño, D. M., Testillano, P. S., Risueño, M. C., Del Río, L. A., et al. (2009). Cellular response of pea plants to cadmium toxicity: cross talk between reactive oxygen species, nitric oxide, and calcium. Plant Physiol. 150, 229–243. doi: 10.1104/pp.108.131524
Romero-Puertas, M. C., Ortega-Galisteo, A., Rodriguez-Serrano, M., and Sandalio, L. M. (2012). “Insights into cadmium toxicity: reactive oxygen and nitrogen species function,” in Metal toxicity in Plants: Perception, Signaling and Remediation, eds D. K. Gupta and L. M. Sandalio (Berlin: Springer), 91–117.
Romero-Puertas, M. C., Terrón-Camero, L. C., Peláez-Vico, M. A., Olmedilla, A., and Sandalio, L. M. (2019). Reactive oxygen and nitrogen species as key indicators of plant responses to Cd stress. Environ. Exp. Bot. 161, 107–119. doi: 10.1016/j.envexpbot.2018.10.012
Saeidi, G., Rickauer, M., and Gentzbittel, L. (2012). Tolerance for cadmium pollution in a core-collection of the model legume, Medicago truncatula L. at seedling stage. Aust. J. Crop Sci. 6, 641–648.
Sanità di Toppi, L., and Gabbrielli, R. (1999). Response to cadmium in higher plants. Environ. Exp. Bot. 41, 105–130. doi: 10.1016/S0098-8472(98)00058-6
Schat, H., Llugany, M., Vooijs, R., Jeanette, H., and Bleeker, P. (2002). The role of phytochelatins in constitutive and adaptive heavy metal tolerances in hyperaccumulator and non-hyperaccumulator metallophytes. J. Exp. Bot. 53, 2381–2392. doi: 10.1093/jxb/erf107
Schützendübel, A., Nikolova, P., Rudolf, C., and Polle, A. (2002). Cadmium and H2O2 -induced oxidative stress in Populus canescens roots. Plant Physiol. Biochem. 40, 577–584. doi: 10.1016/S0981-9428(02)01411-0
Sfaxi-Bousbih, A., Chaoui, A., and El Ferjanim, E. (2010). Cadmium impairs mineral and carbohydrate mobilization during the germination of bean seeds. Ecotoxicol. Environ. Saf. 73, 1123–1129. doi: 10.1016/j.ecoenv.2010.01.005
Sharma, S. S., and Dietz, K. J. (2009). The relationship between metal toxicity and cellular redox imbalance. Trends Plant Sci. 14, 43–50. doi: 10.1016/j.tplants.2008.10.007
Shvaleva, A., Coba de la Peña, T., Rincón, A., Morcillo, C. N., García de la Torre, V., Lucas, M. M., et al. (2010). Flavodoxin overexpression reduces cadmium-induced damage in alfalfa root nodules. Plant Soil 326, 109–121. doi: 10.1007/s11104-009-9985-1
Singh, M., Singh, D., and Rai, M. (2007). Assessment of growth, physiological and biochemical parameters and activities of antioxidative enzymes in salinity tolerant and sensitive basmati rice varieties. J. Agron. Crop Sci. 193, 398–412. doi: 10.1111/j.1439-037X.2007.00267.x
Sledge, M. K., Pechter, P., and Payton, M. E. (2005). Aluminum tolerance in Medicago truncatula germplasm. Crop Sci. 45, 2001–2004. doi: 10.2135/cropsci2004.0673
Smith, B. W., and Roe, J. H. (1949). A photometric method for the determination of α-amylase in blood and urine, with use of the starch-iodine color. J. Biol. Chem 179, 53–59.
Sobrino-Plata, J., Meyssen, D., Cuypers, A., Escobar, C., and Hernández, L. E. (2014). Glutathione is a key antioxidant metabolite to cope with mercury and cadmium stress. Plant Soil 377, 369–381. doi: 10.1007/s11104-013-2006-4
Sobrino-Plata, J., Ortega-Villasante, C., Flores-Cáceres, M. L., Escobar, C., Del Campo, F. F., and Hernández, L. E. (2009). Differential alterations of antioxidant defenses as bioindicators of mercury and cadmium toxicity in alfalfa. Chemosphere 77, 946–954. doi: 10.1016/j.chemosphere.2009.08.007
Stephens, B. W., Douglas, R., Cook, D. R., and Grusak, M. A. (2011). Characterization of zinc transport by divalent metal transporters of the ZIP family from the model legume Medicago truncatula. Biometals 24, 51–58. doi: 10.1007/s10534-010-9373-6
Terrón-Camero, L. C., del Val, C., Sandalio, L. M., and Romero-Puertas, M. C. (2020). Low endogenous NO levels in roots and antioxidant systems are determinants for the resistance of Arabidopsis seedlings grown in Cd. Environ. Pollut. 256:113411. doi: 10.1016/j.envpol.2019.113411
Thomine, S., Wang, R., Ward, J. M., Crawford, N. M., and Schroeder, J. I. (2000). Cadmium and iron transport by members of a plant metal transporter family in Arabidopsis with homology to Nramp genes. Proc. Natl. Acad. Sci. U.S.A. 97, 4991–4996. doi: 10.1073/pnas.97.9.4991
Xu, J., Wang, W., Yin, H., Liu, X., Sun, H., and Mi, Q. (2010). Exogenous nitric oxide improves antioxidative capacity and reduces auxin degradation in roots of Medicago truncatula seedlings under cadmium stress. Plant Soil 326, 321–330. doi: 10.1007/s11104-009-0011-4
Keywords: heavy metal stress, cadmium, reactive oxygen species, nutrient content, Medicago truncatula, germplasm, metal tolerance, NADPH recycling enzymes
Citation: García de la Torre VS, Coba de la Peña T, Pueyo JJ and Lucas MM (2021) Cadmium-Tolerant and -Sensitive Cultivars Identified by Screening of Medicago truncatula Germplasm Display Contrasting Responses to Cadmium Stress. Front. Plant Sci. 12:595001. doi: 10.3389/fpls.2021.595001
Received: 14 August 2020; Accepted: 08 February 2021;
Published: 11 March 2021.
Edited by:
Felipe Klein Ricachenevsky, Federal University of Rio Grande, BrazilReviewed by:
Mirza Hasanuzzaman, Sher-e-Bangla Agricultural University, BangladeshKamrun Nahar, Sher-e-Bangla Agricultural University, Bangladesh
Copyright © 2021 García de la Torre, Coba de la Peña, Pueyo and Lucas. This is an open-access article distributed under the terms of the Creative Commons Attribution License (CC BY). The use, distribution or reproduction in other forums is permitted, provided the original author(s) and the copyright owner(s) are credited and that the original publication in this journal is cited, in accordance with accepted academic practice. No use, distribution or reproduction is permitted which does not comply with these terms.
*Correspondence: M. Mercedes Lucas, bWx1Y2FzQGljYS5jc2ljLmVz
†These authors have contributed equally to this work and share senior authorship