- 1Laboratório de Genômica de Plantas e Bioenergia (PGEMBL), Departamento de Biotecnologia, Escola de Engenharia de Lorena, Universidade de São Paulo, Lorena, Brazil
- 2Lytic Solutions, LLC, Madison, WI, United States
- 3U.S. Dairy Forage Research Center, Agricultural Research Service, U.S. Department of Agriculture, Madison, WI, United States
- 4Department of Biochemistry, and The Department of Energy’s Great Lakes Bioenergy Research Center, The Wisconsin Energy Institute, University of Wisconsin, Madison, WI, United States
- 5Laboratório de Biologia Computacional, Evolutiva e de Sistemas, Centro de Energia Nuclear na Agricultura, Universidade de São Paulo, Piracicaba, Brazil
- 6Departamento de Genética e Evolução, Instituto de Biologia, Universidade Estadual de Campinas, Campinas, Brazil
- 7Laboratório de Ciências da Madeira, Departamento de Biotecnologia, Escola de Engenharia de Lorena, Universidade de São Paulo, Lorena, Brazil
The purification of hydroxycinnamic acids [p-coumaric acid (pCA) and ferulic acid (FA)] from grass cell walls requires high-cost processes. Feedstocks with increased levels of one hydroxycinnamate in preference to the other are therefore highly desirable. We identified and conducted expression analysis for nine BAHD acyltransferase ScAts genes from sugarcane. The high conservation of AT10 proteins, together with their similar gene expression patterns, supported a similar role in distinct grasses. Overexpression of ScAT10 in maize resulted in up to 75% increase in total pCA content. Mild hydrolysis and derivatization followed by reductive cleavage (DFRC) analysis showed that pCA increase was restricted to the hemicellulosic portion of the cell wall. Furthermore, total FA content was reduced up to 88%, resulting in a 10-fold increase in the pCA/FA ratio. Thus, we functionally characterized a sugarcane gene involved in pCA content on hemicelluloses and generated a C4 plant that is promising for valorizing pCA production in biorefineries.
Introduction
Grasses have great importance to worldwide agriculture, as huge volumes are used as food, animal feed, and bioenergy sources. The processing of grasses in agroindustry generates several non-grain products, such as stover or straw. The composition of these lignocellulosic materials makes them suitable feedstocks for the production of second-generation fuels and other value-added products, in a biorefinery concept (Chandel et al., 2012; Jin et al., 2018). Hydroxycinnamic acids, both ferulic acid (FA) and p-coumaric acid (pCA), are high-value chemicals that could be produced in this context. These phenolic acids present several health benefits due to their antioxidant, anti-inflammatory, and antimicrobial properties, being widely used in food, pharmaceutical, and cosmetics industries (Ou and Kwok, 2004; Pei et al., 2016). Currently, FA is often extracted from rice bran oil (20-25% wt of rice bran), which contains 0.9-2.9% of esters of trans-ferulic acid. pCA can be extracted from plants, such as Hedyotis diffusa, an herb used in Chinese medicine (Ou and Kwok, 2004; Cheung et al., 2006; Lerma-García et al., 2009; Karlen et al., 2020). Nonetheless, the production of these acids is limited by the amount of hydroxycinnamate esters in the feedstock, by the plant productivity, and by the extraction and purification processes. Using even part of the massive agroindustry grass biomass leftovers to isolate these phenolic acids could significantly decrease the costs of production (Karlen et al., 2020).
Grass cell walls are excellent sources of FA and pCA (Harris and Hartley, 1980; Hatfield et al., 2017). Glucuronoarabinoxylans (GAX) are mainly substituted with FA on arabinosyl units (FA-Ara), although pCA linked to arabinosyl units (pCA-Ara) has also been found at lower levels (Mueller-Harvey et al., 1986; Lapierre et al., 2018). In contrast, pCA is predominantly ester-linked to lignin, mostly to S units (Ralph et al., 1994a; Hatfield et al., 2009; Ralph, 2010). Among widely cultivated crops, Saccharum spp., sorghum, and maize are particularly good sources of pCA, as they have high levels of pCA in their culms, ranging from 3 to 6% of the dry weight, predominating over (releasable) FA (0.5–2% weight) (Masarin et al., 2011; del Río et al., 2015; Hatfield et al., 2017; Fanelli et al., 2020). Furthermore, these are C4 grasses, with highly efficient photosynthetic rates, having great potential as bioenergy crops (Carpita and McCann, 2008; van der Weijde et al., 2013; Hoang et al., 2015).
In biorefinery designs, commonly used pretreatment methods are autohydrolysis, acid catalysis, or alkaline processes (Galbe and Wallberg, 2019). When these treatments are applied to grass biomass, such as sugarcane bagasse or corn stover, part of the ester-linked pCA and FA is released into the pretreatment liquors (Gómez et al., 2014; van der Pol et al., 2015). Nevertheless, purification of pCA and FA from these pretreatment liquors requires high-cost processes that are especially complex when both acids are present together (Saboe et al., 2018; Karlen et al., 2020). Genetically engineered feedstocks with increased levels of one hydroxycinnamate in preference to the other are therefore highly desirable (Karlen et al., 2020). In this context, there is considerable potential in targeting high levels of ester-linked pCA onto hemicelluloses, as it has been shown in grasses that an increase in GAX-pCA is usually accompanied by a decrease in FA (Hartley, 1972; Bartley et al., 2013; Li et al., 2018). Another advantage of diminishing FA levels is improving biomass digestibility by reducing cell wall recalcitrance (Himmel et al., 2007), as some grass cell wall components are cross-linked by ferulates (Grabber et al., 1998a, 2000; Ralph et al., 1998, 2004; Ralph, 2010; Hatfield et al., 2017).
The genes encoding enzymes involved with hydroxy-cinnamate incorporation into grass cell walls belong to a specific clade, namely, the Mitchell clade, of the BAHD acyltransferases family (Mitchell et al., 2007; Cesarino et al., 2016; Zhong et al., 2019). Phylogenetic analysis revealed 20 rice genes in this clade, which were named AT1 to AT20 (AT = acyltransferase) (Bartley et al., 2013). Expression levels of genes belonging to this clade from several grasses have been associated with pCA and FA levels in lignin and GAX. It has been shown that AT3 and AT4 enzymes (also known as PMT) have a p-coumaryl-CoA monolignol transferase activity and are involved with pCA incorporation into lignin in rice, maize, and Brachypodium (Withers et al., 2012; Marita et al., 2014; Petrik et al., 2014). More recently, it was shown that an increase in expression levels of OsAT5 (OsFMT) was followed by an increase in FA specifically ester-linked to lignin in rice (Karlen et al., 2016). Regarding hydroxycinnamate incorporation into hemicelluloses, silencing of AT9 resulted in decreased FA-Ara levels in Setaria viridis and sugarcane cell walls (de Souza et al., 2018, 2019), and there is evidence that BdAT1 expression levels are also associated with FA content in Brachypodium cell walls (Buanafina et al., 2016). Nonetheless, more recently, a study involving the suppression of SvAT1 in S. viridis has evidenced a main role for this gene in the p-coumaroylation of arabinose in xylans. The silenced lines had a significant decrease in pCA-Ara levels, but also exhibited a small decrease in FA-Ara content in leaves, suggesting that SvAT1 enzyme could participate in GAX feruloylation as well, to a lesser extent (Mota et al., 2020). Furthermore, overexpression of a rice gene (OsAT10) influenced pCA levels in rice, resulting in pCA increase and FA decrease in xylans from mature straw (Bartley et al., 2013). A similar effect was observed for the heterologous overexpression of OsAT10 in switchgrass, which resulted in increased levels of total ester-linked pCA, but only in the green leaves (Li et al., 2018). Recently, an ortholog of OsAT10 in barley, HvAT10, was shown to influence the pCA and FA levels in the whole grain (Houston et al., 2020). Therefore, there is considerable potential in manipulating the expression of the Mitchell clade genes to generate biomass feedstocks that are simultaneously less recalcitrant and more suitable for hydroxycinnamic acid generation in biorefineries. In this context, manipulating AT10 genes seems suitable for pCA production.
Although BAHD acyltransferases enzymes were shown to be involved with hydroxycinnamate incorporation in grasses, several aspects still need to be investigated, especially in large-statured crops, such as sugarcane and maize. For sugarcane, the gene encoding an enzyme related to pCA incorporation into its GAX is yet to be identified and characterized. This would allow a better understanding of pCA incorporation into grass cell walls and could have applications in biorefineries, in which both biofuels and this high-valued chemical could be produced. In this work, genomic, transcriptomic, and expression analyses permitted to identify sugarcane genes belonging to the Mitchell clade. Overexpression of sugarcane ScAT10, an ortholog of OsAT10, in maize allowed to functionally characterize this gene, which affected hydroxycinnamate levels of arabinoxylan hemicelluloses increasing up to 10 times the total pCA/FA ratio in culms.
Materials and Methods
Identification, Annotation, and Phylogenetic Analysis of Mitchell Clade BAHD Family Members
To identify putative sugarcane BAHD acyltransferases from the Mitchell clade, we searched in different databases of both genomic and transcriptomic sequences. Two sugarcane genomes were used, from cultivars SP80-3280 (Riaño-Pachón and Mattiello, 2017) and R570 BAC (bacterial artificial chromosome), as well as R570 STP (single tilling path) (Garsmeur et al., 2018). Transcriptomics data used were SUCEST (Sugarcane EST project) dataset (Vettore et al., 2003; Vicentini et al., 2012) and RNA-seq from internodes (Vicentini et al., 2015) and leaves (Cardoso-Silva et al., 2014). We performed a BLASTp (Altschul et al., 1990) search into predicted protein databases, using Mitchell clade sequences from other grasses as a query (Bartley et al., 2013). For transcriptomic databases that consisted of DNA sequences only, we performed BLASTn search, and the DNA annotated sequences were then translated to protein using the Expasy translate tool1. To obtain higher sensitivity in the identification of sequences belonging to the BAHD acyltransferases family, we also conducted HMMER searches in the above-mentioned datasets, using hmmsearch and jackhmmer algorithms (Eddy, 2011). Furthermore, we updated the annotation of sorghum Mitchell clade proteins, performing BLASTp and HMMER searches into a more recent version of the genome, S. bicolor 313 v3.1 (McCormick et al., 2018), which was downloaded from Phytozome 122. The proteins containing the Pfam transferase domain (PF02458) were annotated and aligned with previously published proteins in the Mitchell clade from rice, maize, Brachypodium, S. viridis, and Arabidopsis (Bartley et al., 2013; de Souza et al., 2018). A maximum likelihood phylogenetic analysis was performed using PhyML 3.0 (Guindon et al., 2010). Parameters were JTT + G + F substitution model, selected using smart model selection (Lefort et al., 2017), and aLRT as the test method. The phylogenetic tree was visualized using the iTOL software3. After identification of sugarcane and sorghum proteins belonging to the Mitchell clade, a new phylogeny of specifically this clade was performed, selecting one sugarcane representative sequence for each ortholog group, using the same parameters. Proteins identified as belonging to the AT10 sub-clade were aligned using ClustalW, and an identity matrix was generated in the BioEdit® software. We also analyzed the expression profile of AT10 orthologs in rice (Jain et al., 2007), Brachypodium (Winter et al., 2007; Sibout et al., 2017), and maize (Hoopes et al., 2019) using the eFP Browser tool4.
Mitchell Clade Gene Expression Analysis in Sugarcane H321 by RT-qPCR
Sugarcane hybrids (Masarin et al., 2011) were cultivated from May 2015 to May 2016, in an experimental field located at Lorena, São Paulo, Brazil (22°43′51″ S, 45°07′29″ W). Plants were grown in 0.60 m × 1.0 m rows with 3.0 m × 2.0 m spacing between hybrids. Culms from 1-year-old first ratoon hybrid H321 plants were harvested. For each culm, the first internode (from top to bottom), in which superior and inferior nodes were clearly identified, was sampled as previously described (Collucci et al., 2019). These internodes were cut in 25 mm circles. The first 2 mm external section, containing epidermal cells, was removed. The remaining material was divided into three fractions corresponding to the rind, pith–rind interface, and pith (Costa et al., 2013). All leaves were sampled from 3-month-old H321 sugarcane plants, collected in 2015. The harvested tissues were immediately frozen in liquid nitrogen and then stored at −80°C until use. All samples were ground in a cryogenic grinder (2010 Geno/Grinder®, Spex SamplePrep®), always keeping the material in touch with liquid nitrogen. RNA was extracted using Concert™ Plant RNA Reagent (Invitrogen™). RNA quantity and quality were accessed using Biodrop duo (Biochrom), whereas integrity was checked with electrophoresis in 0.8% agarose gel. RNA samples were treated with DNAse RQ1 RNAse-free DNAse® (Promega Co.), and the first-strand cDNA was synthesized using SuperScript III Reverse Transcriptase® (Invitrogen®) following the manufacturer’s instructions. Primers specific for each of the nine sugarcane genes identified in the Mitchell clade were designed in the last exon and 3′UTR region, using both genomic and transcriptomic sequences (Supplementary Table 1), except for the GAPDH reference gene previously characterized (Bottcher et al., 2013). All quantitative real-time PCR (qRT-PCR) reactions were conducted in 96-well plates with SYBR™ GREEN PCR master mix (Applied Biosystems™, ThermoFisher Scientific™), in a 7,500 Fast Real-time PCR system (Applied Biosystems™, ThermoFisher Scientific™) using the program 95°C (20 s)/95°C (3 s)/40 cycles 60°C (30 s). Primer specificity was accessed through melting curve analysis, with program 95°C (20 s)/60°C (1 min)/95°C (15 s)/60°C (15 s) (Supplementary Figure 1A). For expression quantification, the efficiency of each primer pair was estimated using the LinRegPCR software (Ramakers et al., 2003; Ruijter et al., 2009; Supplementary Table 2A). Relative expression was calculated including efficiency correction and normalization with reference genes (Pfaffl, 2001; Vandesompele et al., 2002), using the Relative Quantification software (Thermo Fisher Cloud™).
Generation of Ubi:ScAT10 Maize Lines
A plant transformation construct whereby ScAT10 would be expressed from the strong constitutive maize ubiquitin promoter was made as follows. The identified ScAT10 coding sequence (1362 bp) flanked by attb1/attb2 recombinant regions (25 bp each) was synthesized in the puc57 vector (FASTBIO). Following Gateway cloning® instructions (Invitrogen™), a PCR product consisting of ScAT10 CDS flanked by attb regions was generated (Supplementary Table 1), gel purified, and recombined with plasmid pDONR221 in a BP reaction (BP clonase, Invitrogen™). The generated entry vector was confirmed by restriction enzyme digestion (New England BioLabs™) and recombined with destination vector Ox:pzp221b (Ox—overexpression) (Marita et al., 2014), through LR reaction (LR clonase, Invitrogen™). The resulting pzp221b:Ox:ScAT10 was confirmed by restriction enzyme digestion, and ScAT10 CDS insert was confirmed by sequencing (Eurofins) (Supplementary Figure 2). Maize HiII lines were transformed with the pzp221b:Ox:ScAT10 construct, using Agrobacterium tumefaciens, at the Plant Transformation Facility, Iowa State University5, following their standard procedures. Ten biological independent transgenic events were received and regenerated in Petri dishes with selective media containing Bialaphos. These plantlets were transplanted to 5 cm pots in a growth chamber with a 24 h light regime. After a month, they were transplanted to larger pots (11.5 liters) in a greenhouse with a 16 h light regime (Supplementary Figure 3). Plants were periodically watered and fertilized with soluble fertilizer (nitrogen–phosphorus–potassium 18–2–18). The 10 independent lines were further analyzed, both genotypically and phenotypically. DNA was isolated from the 4th leaf (before complete expansion) of transgenic plants after a month of growth, as previously described (Marita et al., 2014). PCR genotyping was conducted using specific primers targeting ScAT10 (Supplementary Table 1). To avoid annealing with endogenous maize ZmAT10 genomic sequences, we designed primers in the exon–exon junction, specifically targeting the inserted sugarcane CDS (Supplementary Figure 4).
Maize Ubi:ScAT10 Lines Gene Expression Levels by RT-qPCR
The base of the first fully developed leaf after ear development was harvested from 3-month-old greenhouse-grown plants. RNA was extracted from these tissues using Spectrum™ plant total RNA kit (protocol A; Sigma Aldrich®). RNA quantity/quality was inferred via spectrophotometry, and integrity was checked by electrophoresis on a 0.8% agarose gel. One microgram of RNA was treated with RNAse-free DNAse (Promega Co.). First-strand cDNA was synthesized with GoScript Reverse Transcription System (Promega Co.), using 400 ng of DNAse-treated RNA and OligodT primers, following the manufacturer’s instructions. For RT-qPCR analysis, primers were designed for target gene ScAT10, as well as for reference genes ZmLUG and ZmMEP (Manoli et al., 2012) using Primer3plus (Supplementary Table 1). ScAT10 primers were designed in a region encompassing part of ScAT10 CDS and the T-DNA insert before the NOS transcriptional termination site (Supplementary Figure 2), conferring specificity. Maize reference gene primers were designed in the last exon and 3′UTR region. All RT-qPCR reactions and relative expression calculations were conducted as described above (Supplementary Figure 1B and Supplementary Table 2B).
Plant Cell Wall Isolation for Chemical Compositional Analysis
The whole culms of 4-month-old senescent plants (age determined as after transferring from tissue culture) were harvested. Samples were ground in a UDY mill (Udy Corp., Fort Collins, CO., United States) with a 1 mm screen and dried overnight at 55°C. Cell wall was extracted as previously described (Hatfield et al., 2009). Ground tissue (5-10 g) was incubated in Nalgene centrifuge bottles with 50 mM tris acetate buffer (pH 6), shaken, and centrifuged (6,500 rpm for 20 min) three times. Samples were then extracted three times with 80% ethanol, using the same procedure. Pellets were extracted another three times with acetone and one time with chloroform:methanol (2:1 v/v). Samples were then washed with acetone to remove the chloroform:methanol mix. Final cell wall residues were air-dried in a fume hood, fully dried, and stored in a 55°C oven until further use for assays.
Ester-Linked pCA and FA Released by Mild Alkaline Treatment
The dried cell walls (100 mg) were incubated in 3 ml vials with 100 μl of 1 mg/l 2-hydroxycinnamic acid (oCA) as internal standard and 2.4 ml 2 M NaOH, at room temperature for about 20 h (Grabber et al., 1995). Samples were acidified to pH 2 with 12.1 M HCl. Phenolics were then extracted three times with 2.0 ml diethyl ether. Released phenolics were identified and quantified as trimethylsilyl derivatives (40 μl TMSI and 10 μl pyridine) by GLC-FID (Agilent Technologies 7890 GC system) on a ZB-1 column (Phenomenex, Zebron 100% dimethylpolysiloxane; 30 m × 0.25 mm, 0.25 μm film). The GLC conditions were injector 315°C, detector 300°C, and a temperature program of 200°C 1 min and 4°C min–1 to 248°C held for 2 min, followed by 30°C min–1 to 300°C before holding for 20 min. All GC temperature programs were run at 20 psi constant pressure and split ratio 30:1. Standards of FA and pCA were used to identify and quantify phenolic products.
Mild Acid Hydrolysis
The dried cell walls were treated by mild acidolysis according to a previous report (Lapierre et al., 2018). Hydrolysates were analyzed by HPLC. For this, the samples were quantified on a Shimadzu Nexara X2 HPLC equipped with a Phenomenex Kinetex C18 column (2.6 μm × 2.1 mm × 150 mm, P/N: 00F-4462-AN). The mobile phase was a binary gradient of solvent A: water + 0.1% formic acid and solvent B: acetonitrile + 0.1% formic acid. The detector was a photo-diode array scanning from λ = 250–600 nm, quantification was performed at λ = 270 nm, and a 5-point calibration curve was determined using authentic MeAra-pCA, MeAra-FA, and oCA (54 μg/ml, internal standard).
Cell Wall Total Neutral Sugars
Total neutral sugars in the cell walls were released according to a previous report (Rancour et al., 2012). Monosaccharides were converted to their alditol acetate derivatives following the protocol described by Blakeney et al. (1983). Sugar derivatives were quantified by GLC-FID on a Shimadzu GC-2010 using a 007-225 methylpolysiloxane column (30 m × 0.25 mm with 0.25 μm film thickness; Quadrex Corporation). GLC conditions were injector 220°C, detector 240°C, and a temperature program of 215°C for 2 min, 4°C min–1 to 230°C before holding for 11.25 min run at a constant linear velocity of 33.4 cm.s–1, and split ratio 25:1.
Derivation Followed by Reductive Cleavage (DFRC)
The derivatization followed by reductive cleavage (DFRC) analysis was performed on maize samples as described for the optimized DFRC protocol (Regner et al., 2018). The maize sample (50 mg) was stirred in a two-dram vial, with PTFE pressure release cap (Chemglass CG-4912-02), in acetyl bromide/acetic acid (1/4 v/v, 3 ml) at 50°C for 2.5 h. The solvent was removed on a SpeedVac (Thermo Scientific SPD131DDA, 50°C, 35 min, 1.0 Torr, 35 Torr/min). The crude film was suspended in absolute ethanol (0.5 ml), and the ethanol was then removed on the SpeedVac (50°C, 15 min, 6.0 Torr, 35 Torr/min). The residue was suspended in 1,4-dioxane:acetic acid:water (5/4/1 v/v/v, 5 ml), and nano-powder zinc (150 mg) was added. The reaction was then sealed and sonicated for 1 h at room temperature. The reaction was spiked with a mixture of isotopically labeled internal standards (H-d8, G-d8, S-d8, G-DDpCA-d10, S-DDpCA-d10, G-DDFA-d10, and S-DDFA-d10) and quantitatively transferred using dichloromethane (DCM, 2 × 2 ml) to a separatory funnel charged with saturated ammonium chloride (10 ml). The organics were extracted with DCM (3 ml × 10 ml), combined, dried over anhydrous sodium sulfate, and filtered through qualitative filter paper, and the solvent was removed on a rotary evaporator (water bath at <50°C). The free hydroxyl groups were then acetylated for 30 min in pyridine and acetic anhydride (1/1 v/v, 5 ml), after which the solvent was removed by rotary evaporation to give a crude oily film. To remove most of the polysaccharide-derived products, the crude DFRC product was loaded onto an SPE cartridge (Supelco Supelclean LC-Si SPE tube, 3 ml, P/N: 505048) with DCM (3 ml × 1.0 ml). The products were eluted with n-hexane:ethyl acetate (1:1, v:v, 8 ml), and the combined solvents were removed on a rotary evaporator and transferred with DCM to a GC–MS vial for a final sample volume of ∼1 ml. The samples were analyzed on a triple-quadrupole GC–MS/MS (Shimadzu GCMS-TQ8030) operating in multiple-reaction-monitoring (MRM) mode using synthetic standards for authentication and calibration. Calibration curves were determined from the ratio of peak areas of the synthetic trans-product to the corresponding isotope-labeled internal standard vs. the ratio of their concentrations.
Enzymatic Digestibility of Ubi:ScAT10 Maize Lines
Approximately 50 mg of cell wall material was suspended in buffer [30 mM citrate/NaOH pH 4.5, 0.01% (w/v) NaN3] containing 5 U/ml of cellulase (Celluclast, Novozymes®) and 5 U/ml xylanase (Sigma-Aldrich, St. Louis, MO, United States), adjusting the volume to 1 U/mg of cell wall. Samples were incubated at 40°C in a shaker for 48 h. Tubes were centrifugated, and an aliquot of the supernatant was analyzed for total carbohydrate using the phenol-sulfuric method (DuBois et al., 1956). Percent of conversion was estimated by the ratio of sugars released and the sum of xylose, arabinose, and glucose in the cell walls determined for each event.
Statistical Analysis
For sugarcane expression profiling, RT-qPCR experiments were performed in technical triplicate for each biological duplicate, and statistically significant differences were determined using the unpaired Student’s t-test (α = 0.05). For maize transgenics characterization, RT-qPCR and mild acidolysis were performed in technical triplicate, whereas determination of ester-linked phenolics released by mild alkaline treatment, total cell wall neutral sugars, DFRC, and digestibility analysis was performed in technical duplicate. Statistically significant differences were determined using the unpaired Welch’s t-test (α = 0.05) comparing the mean of analyzed biological individuals for each group (Ubi:ScAT10 transgenic lines, n ≥ 4 and B73/empty vector control lines, n ≥ 3). All statistical analyses were conducted in R6.
Results
Identification and Phylogenetic Analysis of BAHD Family Members From Mitchell Clade A
We used a bioinformatics approach to identify sugarcane genes belonging to the Mitchell clade A (Mitchell et al., 2007; Molinari et al., 2013) of BAHD acyltransferases. BLAST (Altschul et al., 1990) and HMMer (Eddy, 2011) searches against genomic and transcriptomic sugarcane datasets, followed by a phylogenetic analysis for sugarcane, sorghum, Brachypodium, Setaria, rice, maize, and Arabidopsis (Figure 1A) revealed nine sugarcane unigenes in this clade with complete open reading frames (ORFs), which were named as ScAT1 to ScAT10 lacking of ScAT4, based on the nomenclature of Bartley et al. (2013) (Supplementary Tables 3A,B). We constructed a phylogenetic tree in which, for each sub-clade containing AT1 to AT10 ortholog genes, only one sugarcane identified sequence (unigene) was represented, prioritizing those from R570 and SP8032-80 genomes whenever possible (Figure 1A and Supplementary Tables 3A,B). Nonetheless, for the sub-clade containing AT7 orthologs, only sugarcane transcript sequences were identified in the analyzed datasets (Supplementary Table 3B). Therefore, the currently available sugarcane genomic sequences do not yet cover the full transcriptome for this crop, and thus RNA-seq remains a powerful tool to provide additional information for the identification of new genes in sugarcane.
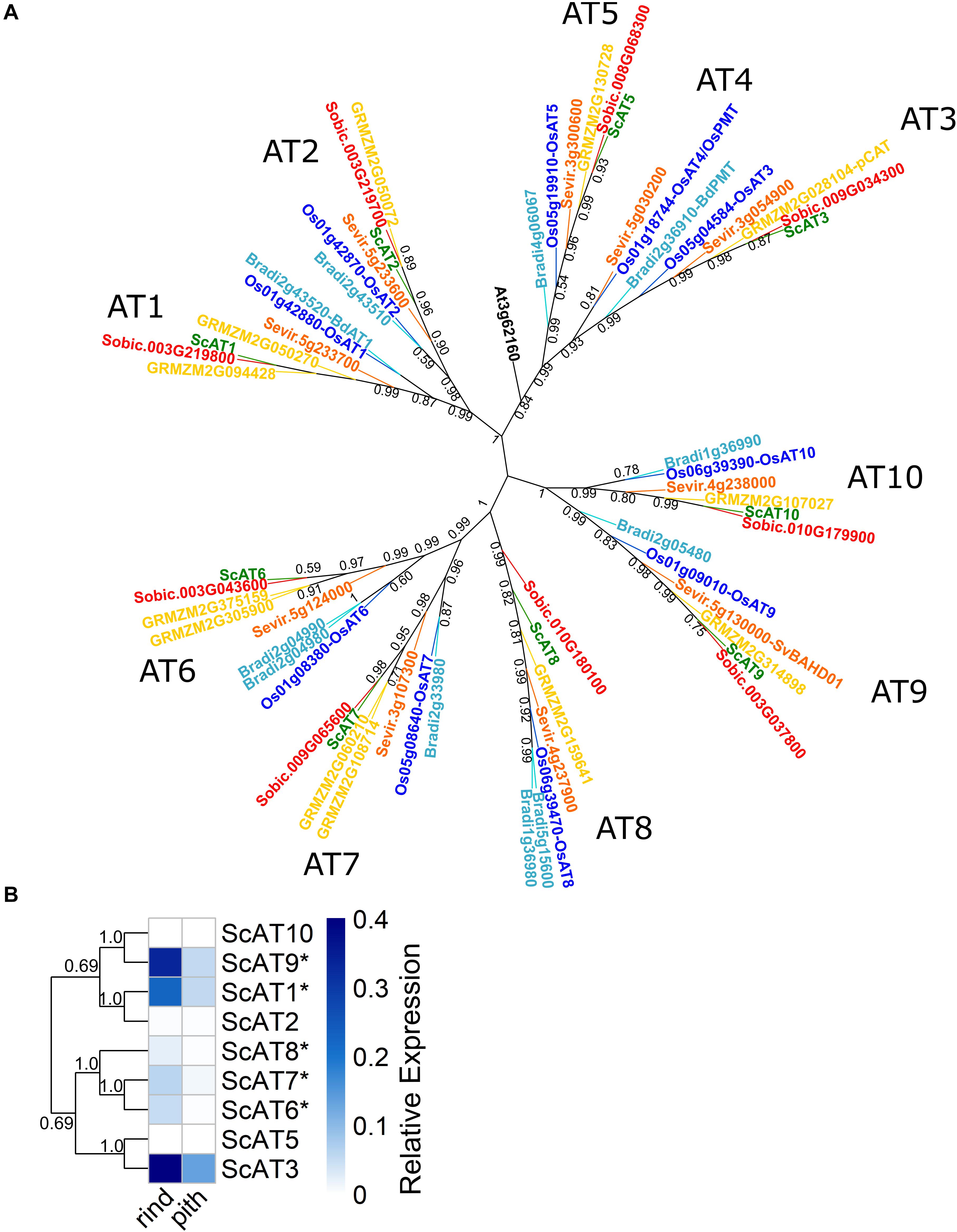
Figure 1. Evolutionary relationship and expression profile of sugarcane Mitchell clade A genes. (A) Unrooted maximum likelihood phylogeny of sugarcane, related grasses, and Arabidopsis. Topology support shown was obtained with the aLRT method, and values below 0.4 were not represented. Species and branches are colored in dark green (Saccharum spp.), red (Sorghum bicolor), yellow (Zea mays), orange (Setaria viridis), light blue (Brachypodium distachyon), dark blue (Oryza sativa), and black (Arabidopsis thaliana). (B) Heatmap of RT-qPCR relative expression levels of Mitchell clade A genes in sugarcane rind and pith sections of a young internode. Dendrogram represents a neighbor-joining phylogenetic analysis of the sugarcane genes, with topology support shown as the ratio of bootstrap runs. Relative expression was normalized with the reference gene ScGAPDH (2–ΔCt) (Pfaffl, 2001). Values with * were significantly different between tissues by Student’s t-test, p < 0.05.
Expression Analysis of Sugarcane Genes From the Mitchell Clade A
To investigate the expression profile of sugarcane genes identified in Mitchell clade A, we analyzed EST data from distinct libraries of SUCEST (Vettore et al., 2003; Vicentini et al., 2012; Supplementary Figure 5). All nine genes were expressed, indicating that they are functional in at least one tissue at a stage of sugarcane development. In the internode tissues, ScAT3, ScAT8, ScAT9, and ScAT1 had the highest levels of expression. All these genes were also expressed in a reproductive (inflorescence) stage. On the other hand, ScAT5 and ScAT10 showed low and restricted expression in root and inflorescence tissues, respectively.
Considering the importance of sugarcane bagasse as a feedstock for biorefineries, RT-qPCR analysis was conducted in culm tissues. We selected the young internode one (below the uppermost node) from a hemicellulose-rich sugarcane hybrid H321 (Masarin et al., 2011), which was separated into the rind (outermost part) and pith (inner part) regions. It has been shown that the sugarcane rind and pith have distinct chemical compositions, with the rind having more lignin and hemicellulose than the pith (Costa et al., 2013). Overall, five genes (ScAT1, ScAT6, ScAT7, ScAT8, and ScAT9) had significantly higher expression levels (p < 0.05) in the rind than in the pith (Figure 1B). The predominant cell types in the rind are vessels and sclerenchyma fibers, whereas in the pith, parenchyma cells are more abundant (Costa et al., 2013; Ferraz et al., 2014). The vessels and fibers present a higher content of hydroxycinnamates and lignin (Siqueira et al., 2011). Therefore, a higher expression in the rind corroborates the hypothesis that the enzymes encoded by these genes are related to FA or pCA incorporation into secondary cell walls.
Both ScAT10 and ScAT5 expressions, on the other hand, were not detected in the internode (Figure 1B), as found for EST data. However, they were expressed in young sugarcane leaves at low levels (Supplementary Figure 6). This expression pattern of ScAT10 supports a potential role of this gene in pCA incorporation into hemicelluloses, considering that pCA-Ara was detected at low levels in cell walls from grasses and in a higher proportion in leaves than in internodes (Mueller-Harvey et al., 1986; Lapierre et al., 2018).
Protein Identity and In silico Expression Pattern of AT10 Orthologous Genes
The sugarcane ScAT10 full-length amino acid sequence shares a high percentage of identity with the AT10 amino acid sequences of Brachypodium (79%), rice (80%), Setaria (87%), maize (86%), and sorghum (93%) (Supplementary Table 4A). In the transferase domain region, which contains motifs associated with BAHD acyltransferase function, the degree of identity is even higher, having more than 81% identity with proteins in the C3 photosynthetic pathway species (rice and Brachypodium) and more than 90% for C4 species (sorghum, maize, and Setaria) (Supplementary Table 4B and Supplementary Figure 7). This suggests that AT10 enzymes may have a similar role in a variety of C3 and C4 plant species.
We analyzed the AT10 orthologs’ expression patterns among different organs and tissues in silico using available data with the eFP browser tool (see text Footnote 4) (Winter et al., 2007) for rice, Brachypodium, and maize (Supplementary Figure 8). For rice, OsAT10 expression levels were higher in the inflorescence (Supplementary Figure 8A; Jain et al., 2007), similar to sugarcane EST data (Supplementary Figure 5). In Brachypodium, expression was higher in young leaf tissue, root, and young internode (Winter et al., 2007; Sibout et al., 2017; Supplementary Figure 8B). For maize, ZmAT10 showed higher expression in one specific immature leaf stage, followed by meiotic tassel and seeds in different stages (Hoopes et al., 2019; Supplementary Figure 8C). Similarly to ScAT10 (Figure 1B and Supplementary Figure 5), the expression pattern of OsAT10 and ZmAT10 consisted of relatively high expression levels in less lignified tissues, such as reproductive organs and immature leaves (Jung and Casler, 2006; Rancour et al., 2012; Bottcher et al., 2013), and lower or no expression in analyzed internodes (Supplementary Figures 8A,C). This profile provided further evidence that AT10 enzymes may share a conserved role, at least in rice, maize, and sugarcane. This expression pattern is also consistent with this AT10’s role in pCA levels of hemicellulose, and not lignin, as already shown for OsAT10.
ScAT10 is, therefore, a strong candidate for studies aiming at functional characterization of genes encoding enzymes involved with cell wall p-coumaroylation. Taken together, the undetected ZmAT10 expression in internodes, maize’s close evolutionary relationship with sugarcane, and its relative ease of transformation (Joyce et al., 2010), all make maize a suitable plant for ScAT10 functional characterization.
Overexpression of ScAT10 in Maize Lines
To conduct the functional characterization of ScAT10, maize HiII lines were transformed with the sugarcane ScAT10 full-length cDNA under control of the maize ubiquitin promoter (Supplementary Figure 2), generating 10 independent lines, 8 confirmed as positives for the presence of the transgene (named with prefix Ubi:ScAT10 followed by -2, -4, -6, -7, -11, -13, -14, and -15) and 2 negatives for the presence of the transgene (named escape-2 and escape-3 empty vectors), which were used together with wild-type B73 as controls in further analyses (Supplementary Figure 9). RT-qPCR in the first leaf blade above the ear of maize plants showed a high expression level of ScAT10 in all positive transgenic lines, especially Ubi:ScAT10-14, whereas the controls wild-type and escape lines did not show expression of ScAT10 (Figure 2A). We also analyzed the expression levels of the endogenous ZmAT10 in both transgenic and control lines, and no expression was detected, confirming data from eFP analysis (Supplementary Figure 8C).
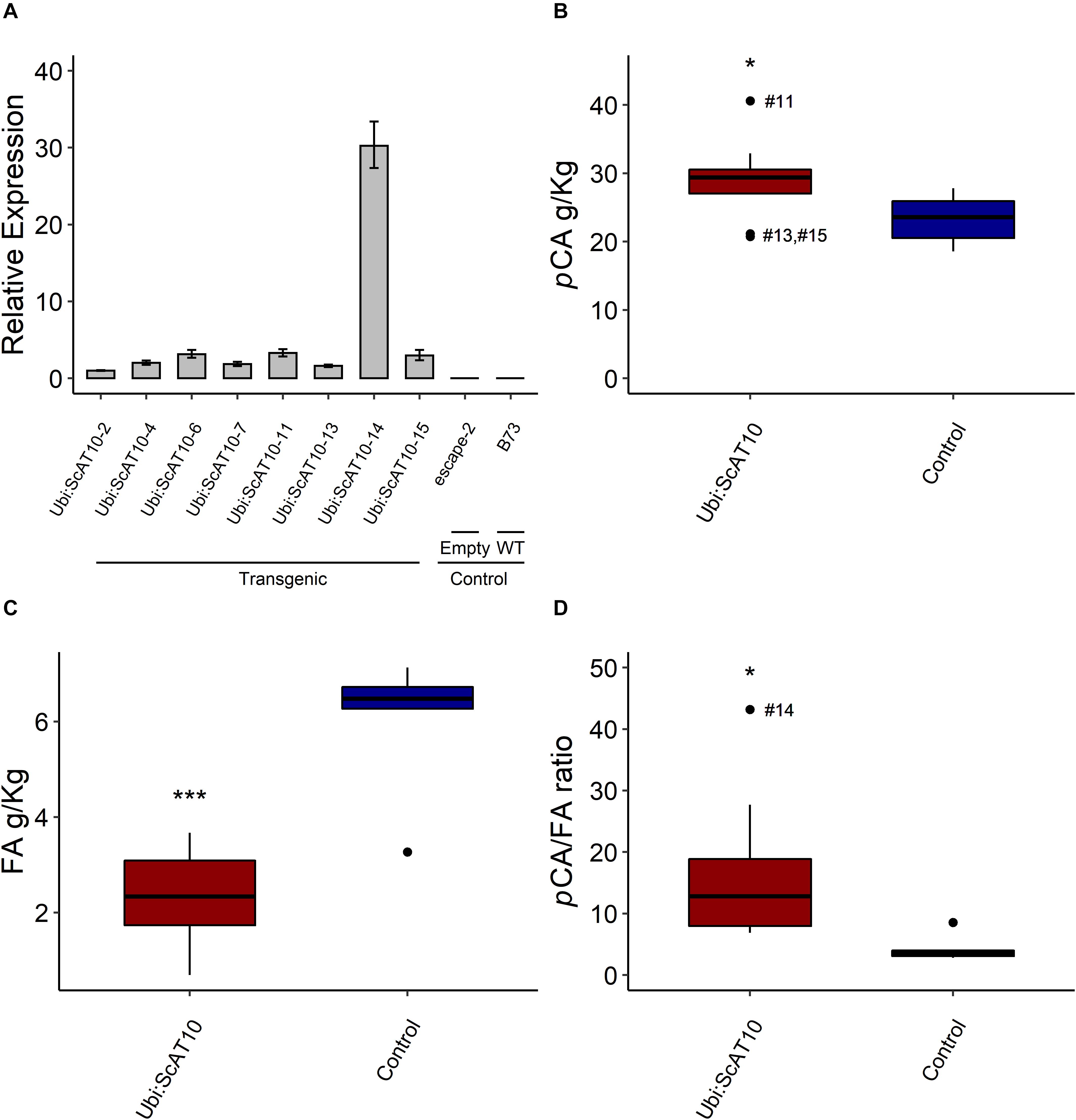
Figure 2. Impact of overexpressing ScAT10 in maize lines. (A) Relative expression levels of ScAT10 gene in maize independent transgenic lines (Ubi:ScAT10), empty vector lines escape-2 and escape-3, and wild-type (WT) B73. Expression levels calculated by the ΔΔCt method (Pfaffl, 2001; Vandesompele et al., 2002) using ZmMEP and ZmLUG as reference genes and escape-2 as the reference sample. Columns represent the means, and error bars represent the superior and inferior limits determined by standard deviation (n = 3 technical replicates). (B) p-Coumaric acid content (pCA), (C) Ferulic acid (FA) content, and (D) Ratio of pCA/FA content from culms, released by mild alkaline treatment. Red boxes represent the distribution of values for transgenic lines (Ubi:ScAT10-2, -4, -6, -7, -11, -13, -14, and -15, total n = 8 plants). Blue boxes represent the distribution of values for control lines (empty vector escape-2 and escape-3 and wild-type (WT) B73, total n = 6 plants, one for each empty vector and four B73). Each box delimits values within the first and third quartile ranges. The horizontal black lines in the boxes represent the median values. Whiskers represent the data range. Outliers were represented by black dots, and the #numbers identify the number of the line for Ubi:ScAT10 lines. * represents the significant difference at p < 0.05, and *** represents the significant difference at p < 0.001 between the mean of transgenics and the mean of controls using Welch’s t-test. See Supplementary Table 5 for actual values for each line.
Impact of ScAT10 Overexpression on Maize Cell Wall Hydroxycinnamate Content
To assess the impact of the overexpression of ScAT10 in maize cell walls from mature stems, we first measured ester-linked pCA and FA released by mild alkaline treatment (Figure 2 and Supplementary Table 5). The comparison of the mean values of Ubi:ScAT10 transgenic lines (29.13 g/kg) with the mean of control lines (23.30 g/kg) using a Welch’s t-test showed a significant increase in pCA content (p = 0.027), corresponding to an increase of 75% for Ubi:ScAT10-11 (Figure 2B). Transgenic lines also showed a significant decrease in FA (p = 0.0002), reaching 88% decrease for line Ubi-ScAT10-14 (Figure 2C). The effect of the overexpression of ScAT10 on both pCA and FA contents can be better visualized by examining the pCA/FA ratio, which had a significant increase (p = 0.013) when comparing the means of transgenic with control lines (Figure 2D). Independent lines Ubi-ScAT10-2, Ubi:ScAT10-6, Ubi:ScAT10-11, and Ubi:ScAT10-14, which had the greatest pCA/FA ratios, were selected for further analysis.
Hydroxycinnamate Content Specifically Attached to GAX or Lignin
As pCA and FA are attached to both GAX and lignin in grass cell walls (Ralph, 2010; Wilkerson et al., 2014; Karlen et al., 2016; Hatfield et al., 2017), we used two orthogonal methods available to diagnose the hydroxycinnamates on each. To access hydroxycinnamate content specifically from the hemicellulosic component, we used a mild acidolysis procedure (HCl/dioxane) (Lapierre et al., 2018; Figure 3 and Supplementary Table 6). This method cleaves GAX arabinosyl glycosidic bonds while leaving most esters intact, allowing the determination of pCA-Ara and FA-Ara contents. Transgenic lines showed a very large (around 160-fold) and significant (p = 0.004) increase in pCA-Ara (Figure 3A). Overexpression of ScAT10 resulted in modified cell wall composition, promoting pCA incorporation into the xylan, a characteristic that was nearly non-existent in the cell wall from the culms of the wild-type maize. The FA-Ara levels in those same tissues were significantly (p = 0.012) reduced in transgenic lines (Figure 3B), which had 75% less FA than controls on average. To determine the fraction of pCA specifically attached to lignin, we used the derivatization followed by the DFRC method, which cleaves lignin β-aryl ether bonds and preserves ester bonds, releasing monolignol ester conjugates, such as S-pCA (sinapyl p-coumarate, from syringyl units) and G-pCA (coniferyl p-coumarate, from guaiacyl units) (Lu and Ralph, 1999; Regner et al., 2018; Figure 4 and Supplementary Table 7). No significant difference was detected in the means of S-pCA levels of transgenic lines and controls (p = 0.164), showing that the overexpression of ScAT10 had no effect on pCA content in the lignin. G-pCA levels were also not significantly different (p = 0.483) and very low in transgenic and control lines, corroborating that pCA is mostly attached to S units in grasses as has been noted previously (Ralph et al., 1994a; Hatfield et al., 2009; Ralph, 2010). DFRC data also reveal no significant difference for non-acylated H (p = 0.417), G (p = 0.453), and S (p = 0.176) unit contents, suggesting that ScAT10 overexpression had no impact on the proportion of non-acylated lignin units. In summary, the perturbation of pCA levels on the arabinoxylans had insignificant effect on the lignin component.
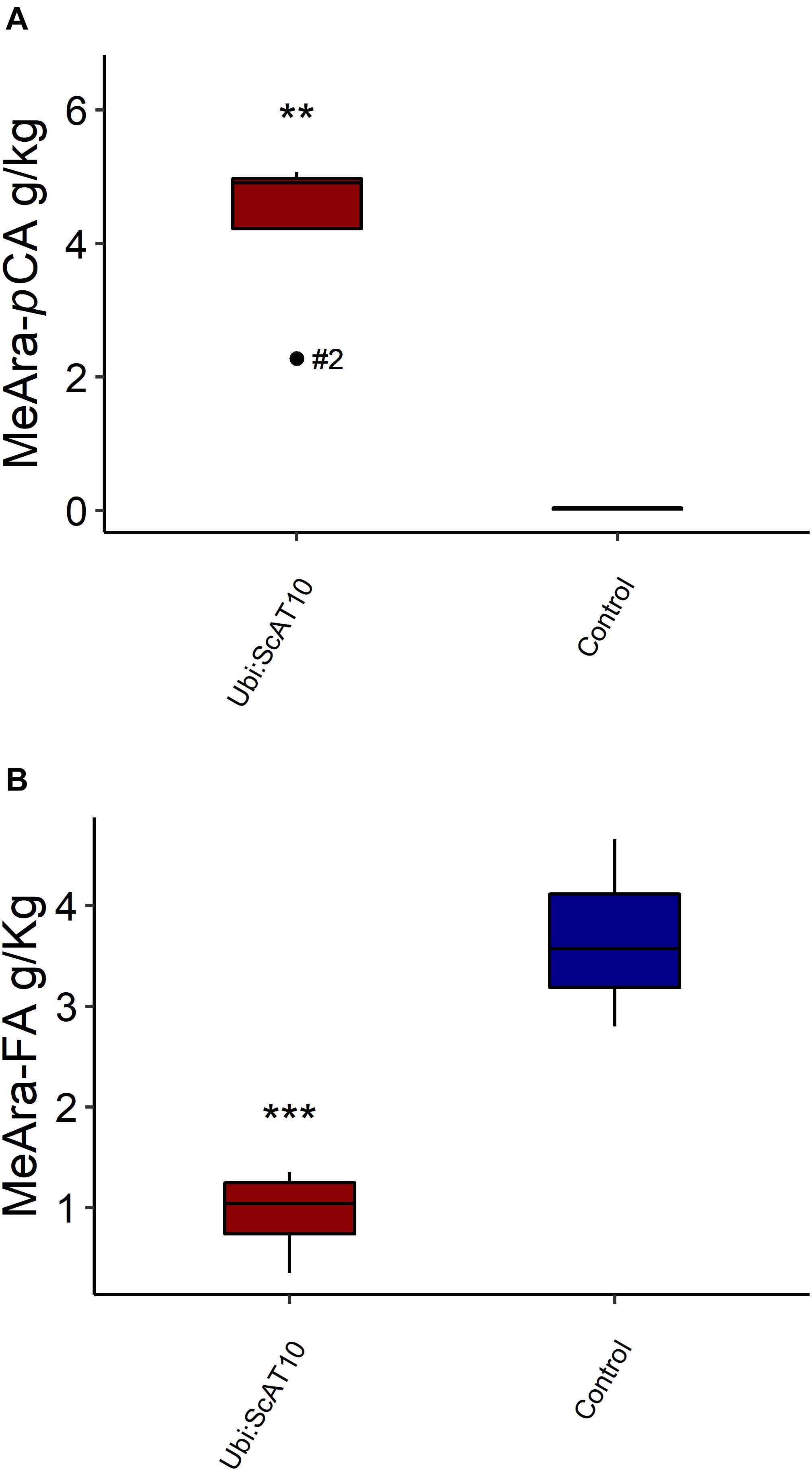
Figure 3. Hydroxycinnamates specifically attached to GAX in culms cell walls, released by mild acidolysis, from transgenic Ubi:ScAT10 and control lines. (A) p-Coumaric acid linked to arabinose (pCA-Ara). (B) Ferulic acid linked to arabinose (FA-Ara). Red boxes represent the distribution of values for transgenic lines (Ubi:ScAT10-2, -6, -11, and -14, total n = 4 plants). Blue boxes represent the distribution of values for control lines (empty vector escape-2 and escape-3 and wild-type (WT) B73, total n = 3 plants). Each box delimits values within the first and third quartile ranges. The horizontal black lines in the boxes represent the median values. Whiskers represent the data range. Outliers were represented by black dots, and the numbers identify the number of the line for Ubi:ScAT10 lines. ** represents the significant difference at p < 0.01, and *** represents the significant difference at p < 0.001 between the mean of transgenics and the mean of controls using Welch’s t-test. See Supplementary Table 6 for actual values for each line.
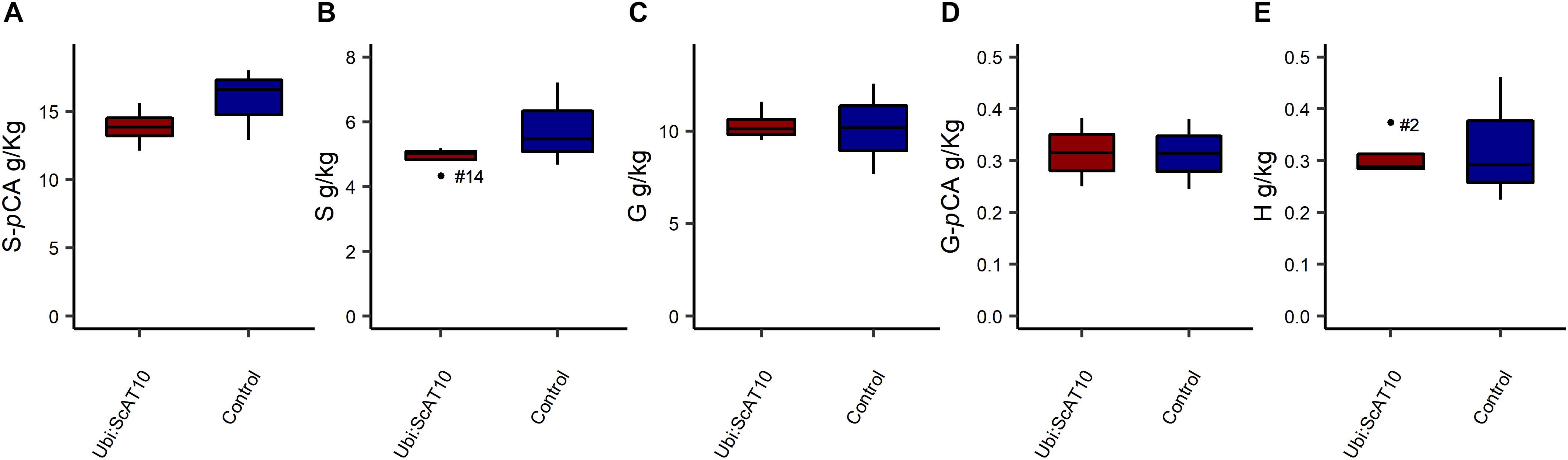
Figure 4. (A) S-pCA units, (B) S non-acylated units, (C) G non-acylated units, (D) G-pCA units, and (E) H units amounts in lignin from transgenic Ubi:ScAT10 and control lines as determined by DFRC in culms cell walls. Red boxes represent the distribution of values for transgenic lines (Ubi:ScAT10-2, -6, -11, and -14, total n = 4 plants). Blue boxes represent the distribution of values for control lines (empty vector escape-2 and escape-3 and wild-type (WT) B73, total n = 3 plants). Each box delimits values within the first and third quartile ranges. The horizontal black lines in the boxes represent the median values. Whiskers represent the data range. Outliers were represented by black dots, and the numbers identify the number of the line for Ubi:ScAT10 lines. See Supplementary Table 7 for actual values for each line.
Cell Wall Total Neutral Sugars Content
Modification in hydroxycinnamate levels attached to GAX was not accompanied by significant changes in cell wall neutral sugars composition. Xylose and arabinose, the main components of GAX, had similar levels in transgenic and control lines (p = 0.258 and p = 0.120, respectively). Furthermore, no significant effect was observed in glucose content (p = 0.253) (Figure 5 and Supplementary Table 8).
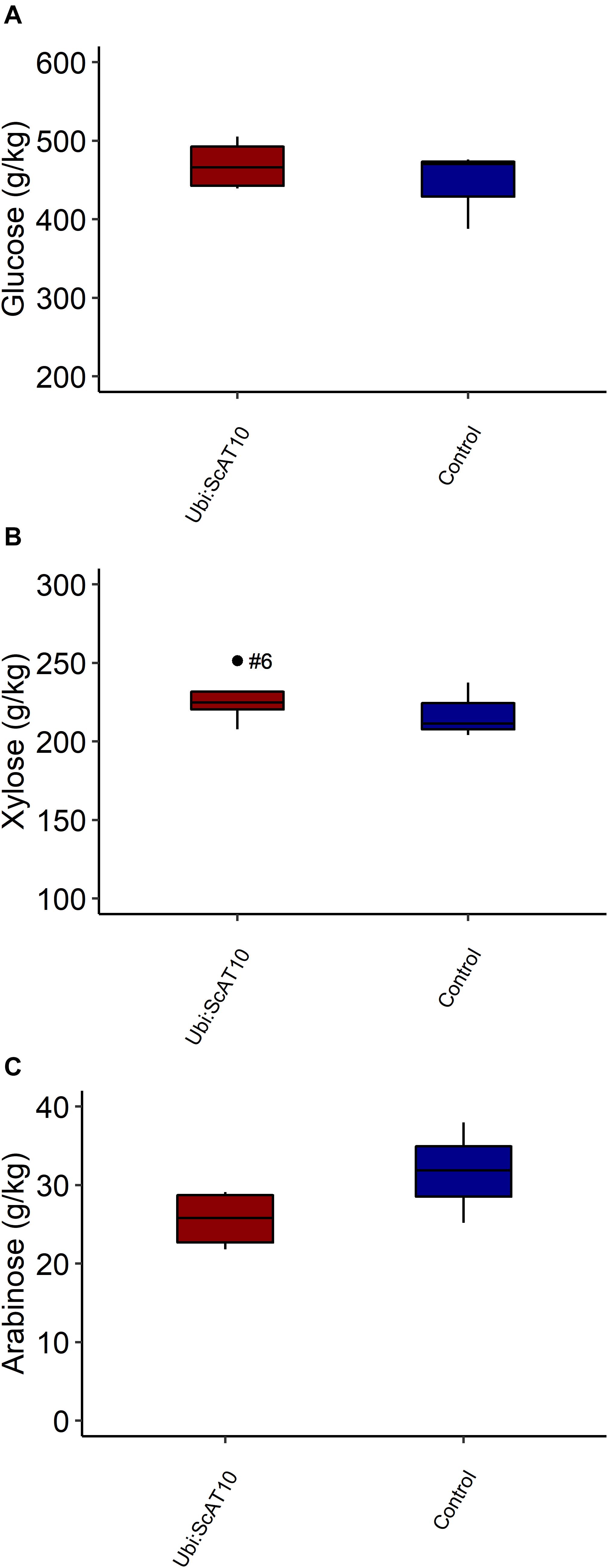
Figure 5. Total neutral sugars in culms cell walls from transgenic Ubi:ScAT10 and control lines. (A) Cell wall glucose content. (B) Cell wall xylose content. (C) Cell wall arabinose content. Red boxes represent the distribution of values for transgenic lines (Ubi:ScAT10-2, -6, -11, and -14, n = 4 plants). Blue boxes represent the distribution of values for control lines (empty vector escape-2 and escape-3 and wild-type (WT) B73, total n = 3 plants). Each box delimits values within the first and third quartile ranges. The horizontal black lines in the boxes represent the median values. Whiskers represent the data range. Outliers were represented by black dots, and the numbers identify the number of the line for Ubi:ScAT10 lines. See Supplementary Table 8 for actual values for each line.
Enzymatic Digestibility of Maize Lines Overexpressing ScAT10
Grass cell wall polymers present cross-linked ferulates and diferulates, forming structures, such as GAX-diFA-GAX and GAX-FA-Lignin (Jung et al., 1983; Ralph et al., 1994b, 1998; Grabber et al., 1998b, 2000). Several studies have shown that the content of FA can be inversely correlated with biomass digestibility (Lam et al., 2003; Bartley et al., 2013; de Souza et al., 2019). Considering the decrease of FA content in maize Ubi:ScAT10 lines (Figures 2C, 3B), the enzymatic digestibility of the isolated cell walls was investigated (Supplementary Figure 10 and Supplementary Table 9). In all evaluated samples, polysaccharide conversion ranged from 15 to 31%, which is a low digestibility associated with the direct enzymatic digestion of lignified cell walls without pretreatment (Bhutto et al., 2017). Direct in vitro digestibility of the transgenic lines did not differ significantly from control, suggesting that the levels of FA decrease observed in the transgenic lines were not enough to affect their digestibility without using sample pretreatment.
Discussion
In this study, the use of distinct sugarcane genomic and transcriptomic datasets associated with a robust bioinformatics and phylogenetic analysis allowed the identification of nine BAHD acyltransferase sugarcane unigenes that were identified in the Mitchell clade A (Figure 1A), including three (ScAT10, ScAT7, and ScAT5) genes not identified previously (de Souza et al., 2019). Overall, the expression profile of the identified Mitchell clade A sugarcane genes (Figure 1B and Supplementary Figure 5) supports the potential involvement of the enzymes encoded by them in hydroxycinnamate incorporation into cell walls. In both the EST and RT-qPCR data, the genes ScAT3, ScAT9, and ScAT1 were highly expressed in internode tissues, whereas ScAT5 and ScAT10 were not expressed. A previous study analyzing the expression profile of Mitchell clade A genes in stems of S. viridis and Brachypodium distachyon (de Souza et al., 2018) showed a similar pattern in these grasses, with AT9 and AT3 genes being highly expressed in both species, and very low expression levels found for AT5 and AT10. However, in contrast with the profile in sugarcane, they also found that SvAT7 had high expression levels in Setaria stems, second only to SvAT9. This data suggests a few differences among grasses in the expression pattern for some genes in this clade, which could indicate specific function.
In our profiling analysis, ScAT3 showed high expression in internode tissues, in which secondary cell wall deposition and lignification are high (He and Terashima, 1990; Collucci et al., 2019). These results support a potential role of this gene in pCA incorporation into lignin, as observed for its orthologous genes in Brachypodium (BdPMT), maize (ZmpCAT), and their closest homolog in rice (OsPMT) (Withers et al., 2012; Marita et al., 2014; Petrik et al., 2014). The gene ScAT1 was highly expressed in the internode as well as in other vegetative and reproductive tissues, similarly to ScAT9. The role of ScAT9 (SacBAHD01/AT9) in feruloylation of xylans has already been demonstrated (de Souza et al., 2019), and ScAT1 could have a similar role, which was suggested for its Brachypodium ortholog BdAT1 (Buanafina et al., 2016). Nonetheless, it has been recently shown that the ortholog in S. viridis, SvAT1, has a role in p-coumaroylation of GAX, similarly to rice OsAT10 and sugarcane ScAT10 (Bartley et al., 2013; Mota et al., 2020). The study also predicted that BdAT1 enzyme has higher affinity for FA, differently from SvAT1, which could accept both pCA and FA, suggesting that these enzymes are functionally distinct, despite their sequence similarity. Considering that in sugarcane and maize, pCA content is mainly associated with lignin, especially in the stems (Ralph, 2010; del Río et al., 2015), ScAT1 expression pattern suggests a similar role to BdAT1, more likely related to FA-Ara levels than to pCA-Ara levels, although further investigations are required.
On the other hand, ScAT5 low and tissue-specific expression levels (Supplementary Figures 5, 6) are consistent with this gene likely encoding a feruloyl-CoA monolignol transferase that participates in FA incorporation into lignin as suggested for its rice ortholog (OsAT5), because monolignol ferulates were detected in low levels in some grass species (Karlen et al., 2016). These results open the possibility of further studies on functional characterization of the identified genes and ultimately their use to achieve cost-effective hydroxycinnamate production in biorefineries.
Considering that it has been shown that rice OsAT10 is involved with pCA levels in the cell wall (Bartley et al., 2013), we sought to investigate whether there is a conserved role of AT10 in other grasses and the potential of using sugarcane ScAT10 to generate C4 plants for biorefining purposes, with the goal of producing both bioenergy and pCA as a high-value co-product. The ScAT10 protein sequence similarity to that from other grasses (Supplementary Figure 7 and Supplementary Table 4A) and the general conserved expression pattern of AT10 orthologous genes observed among sugarcane, maize, and rice (Supplementary Figure 8) supported the contention that AT10 enzymes likely have a similar role, at least in these grasses.
We have shown that ScAT10 has a similar function to rice OsAT10 (Bartley et al., 2013), associated with the content of both pCA and FA specifically linked to GAX. The effect observed for the overexpression of ScAT10 in senescent tissues of maize (up to 75% pCA increase in senescent culms) (Figure 2) was similar to that observed for OsAT10 overexpression in rice straw (80% pCA increase) (Bartley et al., 2013). However, it contrasted with that observed for the overexpression of OsAT10 in senescent leaves of switchgrass, in which pCA released had a slight decrease of 15%, whereas the less lignified green leaves showed an increase in pCA (∼30%) (Li et al., 2018). Nonetheless, as pCA linked to GAX or lignin fractions was not distinguished in the switchgrass study (Li et al., 2018), it is possible that lignin pCA, not GAX, could have specifically decreased (possibly due to changes in the phenylpropanoid pathway), resulting in an overall slight decrease in the pCA levels of the more lignified senescent leaf.
Our data on neutral sugars measured in cell walls of transgenic lines indicate the absence of any compensatory effect in glucose and GAX sugar contents of the maize culms (Figure 5), similar to findings for the overexpression of OsAT10 in switchgrass (Li et al., 2018). In contrast, OsAT10 overexpression in rice resulted in a 20% increase in cell wall glucose (Bartley et al., 2013), suggesting that the effect observed in rice was specific for this species.
As previously discussed (Bartley et al., 2013), the observed decrease in FA could be a result of the competition of pCA and FA for arabinose, so that an increase in pCA-Ara resulted in fewer arabinose sites available for FA linkage. It is also possible that an increase in pCA incorporation into the cell wall could result in less feruloyl-CoA available for xylan modification, as pCA is a precursor in the phenylpropanoid pathway that leads to FA biosynthesis. Nevertheless, as for OsAT10, a characterization of ScAT10 enzyme activity is still needed. It is unknown if the pCA is transferred to monomeric arabinose (or an activated form, such as UDP-Ara) or directly into the arabinoxylan polymer. As most BAHD acyltransferases are cytoplasmatic and xylan synthesis occurs in the Golgi, ScAT10 could be transferring pCA to a cytoplasmatic precursor (Buanafina, 2009; Li et al., 2018).
Although there was a decrease in FA, we did not find significant increase in biomass digestibility of transgenic lines (Supplementary Figure 10), without using pretreatment. This suggests that even with less FA-Ara, other characteristics, such as high lignification of secondary cell walls, restrain direct polysaccharide hydrolysis of the samples. Pretreatment is required to release significant amounts of hemicellulose and/or lignin to allow proper enzymes access to cellulose (Himmel et al., 2007).
The impact of overexpressing ScAT10 in the pCA and FA contents can be properly explored in biorefineries producing both bioenergy and pCA as a high-value product. Biofuels could be produced by fermentation of the cell wall sugars. Although the digestibility of non-pretreated cell walls was not enhanced in the maize transgenic lines (Supplementary Figure 10), there is great potential for further evaluation of AT10 overexpressed plants under selected pretreatments. Decreased FA content can result in less cell wall cross-linked components, which could allow using milder than usual pretreatment conditions. Some studies have already described beneficial effects of genetically engineered lines with decreased FA contents associated with biological (Bartley et al., 2013) and chemical pretreatments (de Souza et al., 2019).
In the biorefinery context, pCA could be recovered from the pretreatment liquors (Timokhin et al., 2020). However, a techno-economic analysis showed that the production of hydroxycinnamic acids from biomass processed in alkaline conditions is currently not viable as purification yields are too low with current methods (Karlen et al., 2020). To achieve cost-effective hydroxycinnamate production, the authors suggested genetic engineering of the feedstock to produce high concentrations of a single type phenolic acid. Expression levels of several BAHD genes could be altered in order to manipulate the hydroxycinnamate content in biomass, and the best strategy would probably involve more than one gene in conjunction. Among BAHD genes, ScAT10 is one of the best candidates to that end, as its overexpression is related not only to increased pCA but also to a significant decrease in FA levels. Our results indicate that for maize lines overexpressing ScAT10, from the total mean content of pCA and FA in the cell wall, 93% corresponds to pCA, against 79% in control lines. This suggests high pCA/FA ratios in pretreatment liquors of autohydrolysis, acid catalysis, and alkaline processes (van der Pol et al., 2015), which opens the possibility for pCA recovery at high yields and purity. Therefore, we have generated a C4 plant that is promising for the achievement of cost-effective production of biofuels and pCA in a biorefinery.
Data Availability Statement
The original contributions presented in the study are included in the article/Supplementary Material, further inquiries can be directed to the corresponding author/s.
Author Contributions
AFa designed and performed the experiments, analyzed the data, and wrote the manuscript. DR designed and contributed with the destination vectors and assisted in the cloning process. MS performed the digestibility analysis and assisted in the experiments. SK and JR contributed with mild acid hydrolysis and DFRC experiments. DR-P provided the sugarcane genomics data. RV provided the sugarcane transcriptomic and its expression data. TS helped in the experiments, data analysis, and interpretation and revised the manuscript. AFe provided the samples, conceived the study, and revised the manuscript. RH provided the experimental tools, performed the digestibility analysis, and helped in the data analysis and interpretation. ER designed, conceived the strategy, directed, and supervised this study, helped in the experiments, and wrote and revised the manuscript. All authors read and approved the final manuscript.
Funding
ER thanks the Fundação de Amparo à Pesquisa do Estado de São Paulo (FAPESP; 2014/17486-6 and 2016/24391-7) under the FAPESP Bioenergy Research Program (BIOEN), grant 2021/02352-8 FAPESP, and Conselho Nacional de Desenvolvimento Científico e Tecnológico (CNPq; MCTIP/CNPq Universal 14/2014, process number 444912/2014-2) for grant support. ER thanks grant from Agência USP de Cooperação Acadêmica Nacional e Internacional (AUCANI) no. 527/2016 Novos Docentes, Novas Parcerias Internacionais for a visiting research fellowship. AFe and ER thank FAPESP (2014/06923-6) for grant support. TS thanks the FAPESP (2016/25785-9) for grant support. AFa thanks the CNPq (142474/2015-0) for a doctorate fellowship and Coordenação de Aperfeiçoamento de Pessoal de Nível Superior (CAPES) for the Programa de Doutorado-sanduíche no Exterior (PDSE; 88881.135309/2016-01) for financial support to conduct the Doctoral Sandwich abroad. This work is part of AFa’s Ph.D. thesis from the Departamento de Biotecnologia (EEL/USP), Brazil. SK and JR were funded by the DOE Great Lakes Bioenergy Research Center (DOE Office of Science BER DE-SC0018409).
Disclaimer
Mention of trade names or commercial products in this article is solely for the purpose of providing specific information and does not imply recommendation or endorsement by the United States Department of Agriculture.
Conflict of Interest
DR was employed by the company Lytic Solutions, LCC, Madison, WI, United States.
The remaining authors declare that the research was conducted in the absence of any commercial or financial relationships that could be construed as a potential conflict of interest.
Acknowledgments
The authors would like to thank J. M. Silva for his technical assistance.
Supplementary Material
The Supplementary Material for this article can be found online at: https://www.frontiersin.org/articles/10.3389/fpls.2021.626168/full#supplementary-material
Footnotes
- ^ https://web.expasy.org/translate/
- ^ https://phytozome.jgi.doe.gov/pz/portal.html
- ^ https://itol.embl.de/
- ^ http://bar.utoronto.ca
- ^ http://www.biotech.iastate.edu/ptf/
- ^ https://www.r-project.org/
References
Altschul, S. F., Gish, W., Miller, W., Myers, E. W., and Lipman, D. J. (1990). Basic local alignment search tool. J. Mol. Biol. 215, 403–410. doi: 10.1016/S0022-2836(05)80360-2
Bartley, L. E., Peck, M. L., Kim, S.-R., Ebert, B., Manisseri, C., Chiniquy, D. M., et al. (2013). Overexpression of a BAHD acyltransferase, OsAt10, alters rice cell wall hydroxycinnamic acid content and saccharification. Plant Physiol. 161, 1615–1633. doi: 10.1104/pp.112.208694
Bhutto, A. W., Qureshi, K., Harijan, K., Abro, R., Abbas, T., Bazmi, A. A., et al. (2017). Insight into progress in pre-treatment of lignocellulosic biomass. Energy 122, 724–745. doi: 10.1016/j.energy.2017.01.005
Blakeney, A. B., Harris, P. J., Henry, R. J., and Stone, B. A. (1983). A simple and rapid preparation of alditol acetates for monosaccharide analysis. Carbohydr. Res. 113, 291–299. doi: 10.1016/0008-6215(83)88244-5
Bottcher, A., Cesarino, I., Brombini dos Santos, A., Vicentini, R., Mayer, J. L. S., Vanholme, R., et al. (2013). Lignification in sugarcane: biochemical characterization, gene discovery, and expression analysis in two genotypes contrasting for lignin content. Plant Physiol. 163, 1539–1557. doi: 10.1104/pp.113.225250
Buanafina, M. M. D. O., Fescemyer, H. W., Sharma, M., and Shearer, E. A. (2016). Functional testing of a PF02458 homologue of putative rice arabinoxylan feruloyl transferase genes in Brachypodium distachyon. Planta 243, 659–674. doi: 10.1007/s00425-015-2430-1
Buanafina, M. M. D. O. (2009). Feruloylation in grasses: current and future perspectives. Mol. Plant 2, 861–872. doi: 10.1093/mp/ssp067
Cardoso-Silva, C. B., Costa, E. A., Mancini, M. C., Balsalobre, T. W. A., Canesin, L. E. C., Pinto, L. R., et al. (2014). De Novo assembly and transcriptome analysis of contrasting sugarcane varieties. PLoS One 9:e88462. doi: 10.1371/journal.pone.0088462
Carpita, N. C., and McCann, M. C. (2008). Maize and Sorghum: genetic resources for bioenergy grasses. Trends Plant Sci. 13, 415–420. doi: 10.1016/j.tplants.2008.06.002
Cesarino, I., Simões, M. S., Brito, M., dos, S., Fanelli, A., Silva, T., et al. (2016). Building the wall: recent advances in understanding lignin metabolism in grasses. Acta Physiol. Plant. 38:269. doi: 10.1007/s11738-016-2293-5
Chandel, A. K., da Silva, S. S., Carvalho, W., and Singh, O. V. (2012). Sugarcane bagasse and leaves: foreseeable biomass of biofuel and bio-products. J. Chem. Technol. Biotechnol. 87, 11–20. doi: 10.1002/jctb.2742
Cheung, H. Y., Cheung, S. H., Law, M. L., and Lai, W. P. (2006). Simultaneous determination of key bioactive components in Hedyotis diffusa by capillary electrophoresis. J. Chromatogr. B Anal. Technol. Biomed. Life Sci. 834, 195–198. doi: 10.1016/j.jchromb.2006.02.007
Collucci, D., Bueno, R. C. A., Milagres, A. M. F., and Ferraz, A. (2019). Sucrose content, lignocellulose accumulation and in vitro digestibility of sugarcane internodes depicted in relation to internode maturation stage and Saccharum genotypes. Ind. Crops Prod. 139:111543. doi: 10.1016/j.indcrop.2019.111543
Costa, T. H. F., Masarin, F., Bonifácio, T. O., Milagres, A. M. F., and Ferraz, A. (2013). The enzymatic recalcitrance of internodes of sugar cane hybrids with contrasting lignin contents. Ind. Crops Prod. 51, 202–211. doi: 10.1016/j.indcrop.2013.08.078
de Souza, W. R., Martins, P. K., Freeman, J., Pellny, T. K., Michaelson, L. V., Sampaio, B. L., et al. (2018). Suppression of a single BAHD gene in Setaria viridis causes large, stable decreases in cell wall feruloylation and increases biomass digestibility. New Phytol. 218, 81–93. doi: 10.1111/nph.14970
de Souza, W. R., Pacheco, T. F., Duarte, K. E., Sampaio, B. L., de Oliveira Molinari, P. A., Martins, P. K., et al. (2019). Silencing of a BAHD acyltransferase in sugarcane increases biomass digestibility. Biotechnol. Biofuels 12, 1–14. doi: 10.1186/s13068-019-1450-7
del Río, J. C., Lino, A. G., Colodette, J. L., Lima, C. F., Gutiérrez, A., Martínez, ÁT., et al. (2015). Differences in the chemical structure of the lignins from sugarcane bagasse and straw. Biomass Bioenergy 81, 322–338. doi: 10.1016/j.biombioe.2015.07.006
DuBois, M., Gilles, K. A., Hamilton, J. K., Rebers, P. A., and Smith, F. (1956). Colorimetric method for determination of sugars and related substances. Anal. Chem. 28, 350–356. doi: 10.1021/ac60111a017
Eddy, S. R. (2011). Accelerated profile HMM searches. PLoS Comput. Biol. 7:e1002195. doi: 10.1371/journal.pcbi.1002195
Fanelli, A., Reinhardt, L., Matsuoka, S., Ferraz, A., da Franca Silva, T., Hatfield, R. D., et al. (2020). Biomass composition of two new energy cane cultivars compared with their ancestral Saccharum spontaneum during internode development. Biomass Bioenergy 141:105696. doi: 10.1016/j.biombioe.2020.105696
Ferraz, A., Costa, T. H. F., Siqueira, G., and Milagres, A. M. F. (2014). “Mapping of cell wall components in lignified biomass as a tool to understand recalcitrance,” in Biofuels in Brazil, eds S. S. da Silva and A. K. Chandel (Cham: Springer International Publishing), 173–202. doi: 10.1007/978-3-319-05020-1_9
Galbe, M., and Wallberg, O. (2019). Pretreatment for biorefineries: a review of common methods for efficient utilisation of lignocellulosic materials. Biotechnol. Biofuels 12, 1–26. doi: 10.1186/s13068-019-1634-1
Garsmeur, O., Droc, G., Antonise, R., Grimwood, J., Potier, B., Aitken, K., et al. (2018). A mosaic monoploid reference sequence for the highly complex genome of sugarcane. Nat. Commun. 9:2638. doi: 10.1038/s41467-018-05051-5
Gómez, L. D., Vanholme, R., Bird, S., Goeminne, G., Trindade, L. M., Polikarpov, I., et al. (2014). Side by side comparison of chemical compounds generated by aqueous pretreatments of maize stover, miscanthus and sugarcane bagasse. Bioenergy Res. 7, 1466–1480. doi: 10.1007/s12155-014-9480-2
Grabber, J. H., Hatfield, R. D., and Ralph, J. (1998a). Diferulate cross-links impede the enzymatic degradation of non-lignified maize walls. J. Sci. Food Agric. 77, 193–200. doi: 10.1002/(SICI)1097-0010(199806)77:2<193::AID-JSFA25<3.0.CO;2-A
Grabber, J. H., Hatfield, R. D., Ralph, J., Zoń, J., and Amrhein, N. (1995). Ferulate cross-linking in cell walls isolated from maize cell suspensions. Phytochemistry 40, 1077–1082. doi: 10.1016/0031-9422(95)00413-2
Grabber, J. H., Ralph, J., and Hatfield, R. D. (1998b). Ferulate cross-links limit the enzymatic degradation of synthetically lignified primary walls of maize. J. Agric. Food Chem. 46, 2609–2614. doi: 10.1021/jf9800099
Grabber, J. H., Ralph, J., and Hatfield, R. D. (2000). Cross-linking of maize walls by ferulate dimerization and incorporation into lignin. J. Agric. Food Chem. 48, 6106–6113. doi: 10.1021/jf0006978
Guindon, S., Dufayard, J. F., Lefort, V., Anisimova, M., Hordijk, W., and Gascuel, O. (2010). New algorithms and mehtods to estimate maximum-likelihood phylogenies: asessing the performance of PhyML 2.0. Syst. Biol. 59, 307–321.
Harris, P. J., and Hartley, R. D. (1980). Phenolic constituents of the cell walls of monocotyledons. Biochem. Syst. Ecol. 8, 153–160. doi: 10.1016/0305-1978(80)90008-3
Hartley, R. D. (1972). p-Coumaric and ferulic acid components of cell-walls of ryegrass and their relationship with lignin and digestibility. J. Sci. Food Agric. 23:1347.
Hatfield, R. D., Marita, J. M., Frost, K., Grabber, J. H., Ralph, J., Lu, F., et al. (2009). Grass lignin acylation: p-coumaroyl transferase activity and cell wall characteristics of C3 and C4 grasses. Planta 229, 1253–1267. doi: 10.1007/s00425-009-0900-z
Hatfield, R. D., Rancour, D. M., and Marita, J. M. (2017). Grass cell walls: a story of cross-linking. Front. Plant Sci. 7:2056. doi: 10.3389/fpls.2016.02056
He, L., and Terashima, N. (1990). Formation and structure of lignin in monocotyledons. III. Heterogeneity of sugarcane (Saccharum officinarum L.) lignin with respect to the composition of structural units in different morphological regions. J. Wood Chem. Technol. 10, 435–459. doi: 10.1080/02773819008050251
Himmel, M. E., Ding, S.-Y., Johnson, D. K., Adney, W. S., Nimlos, M. R., Brady, J. W., et al. (2007). Biomass recalcitrance: engineering plants and enzymes for biofuels production. Science 315, 804–807. doi: 10.1126/science.1137016
Hoang, N. V., Furtado, A., Botha, F. C., Simmons, B. A., and Henry, R. J. (2015). Potential for genetic improvement of sugarcane as a source of biomass for biofuels. Front. Bioeng. Biotechnol. 3:182. doi: 10.3389/fbioe.2015.00182
Hoopes, G. M., Hamilton, J. P., Wood, J. C., Esteban, E., Pasha, A., Vaillancourt, B., et al. (2019). An updated gene atlas for maize reveals organ-specific and stress-induced genes. Plant J. 97, 1154–1167. doi: 10.1111/tpj.14184
Houston, K., Learmonth, A., Hassan, A. S., Lahnstein, J., Looseley, M., Little, A., et al. (2020). The p-coumaroyl arabinoxylan transferase HvAT10 underlies natural variation in whole-grain cell wall phenolic acids in cultivated barley. bioRxiv [Preprint] doi: 10.1101/2020.12.21.423816
Jain, M., Nijhawan, A., Arora, R., Agarwal, P., Ray, S., Sharma, P., et al. (2007). F-Box proteins in rice. Genome-wide analysis, classification, temporal and spatial gene expression during panicle and seed development, and regulation by light and abiotic stress. Plant Physiol. 143, 1467–1483. doi: 10.1104/pp.106.091900
Jin, Q., Yang, L., Poe, N., and Huang, H. (2018). Integrated processing of plant-derived waste to produce value-added products based on the biorefinery concept. Trends Food Sci. Technol. 74, 119–131. doi: 10.1016/j.tifs.2018.02.014
Joyce, P., Kuwahata, M., Turner, N., and Lakshmanan, P. (2010). Selection system and co-cultivation medium are important determinants of Agrobacterium-mediated transformation of sugarcane. Plant Cell Rep. 29, 173–183. doi: 10.1007/s00299-009-0810-3
Jung, H. G., and Casler, M. D. (2006). Maize stem tissues: cell wall concentration and composition during development. Crop Sci. 46, 1793–1800. doi: 10.2135/cropsci2005.02-0085
Jung, H. J., Fahey, G. C. J., and Merchen, N. R. (1983). Effects of ruminant digestion and metabolism on phenolic monomers of forages. Br. J. Nutr. 50, 637–651. doi: 10.1079/bjn19830135
Karlen, S. D., Fasahati, P., Mazaheri, M., Serate, J., Smith, R. A., Sirobhushanam, S., et al. (2020). Assessing the viability of recovery of hydroxycinnamic acids from lignocellulosic biorefinery alkaline pretreatment waste streams. ChemSusChem 13, 2012–2024. doi: 10.1002/cssc.201903345
Karlen, S. D., Zhang, C., Peck, M. L., Smith, R. A., Padmakshan, D., Helmich, K. E., et al. (2016). Monolignol ferulate conjugates are naturally incorporated into plant lignins. Sci. Adv. 2:e1600393. doi: 10.1126/sciadv.1600393
Lam, T. B. T., Iiyama, K., and Stone, B. A. (2003). Hot alkali-labile linkages in the walls of the forage grass Phalaris aquatica and Lolium perenne and their relation to in vitro wall digestibility. Phytochemistry 64, 603–607. doi: 10.1016/S0031-9422(03)00301-7
Lapierre, C., Voxeur, A., Karlen, S. D., Helm, R. F., and Ralph, J. (2018). Evaluation of feruloylated and p-coumaroylated arabinosyl units in grass arabinoxylans by acidolysis in dioxane/methanol. J. Agric. Food Chem. 66, 5418–5424. doi: 10.1021/acs.jafc.8b01618
Lefort, V., Longueville, J. E., and Gascuel, O. (2017). SMS: smart model selection in PhyML. Mol. Biol. Evol. 34, 2422–2424. doi: 10.1093/molbev/msx149
Lerma-García, M. J., Herrero-Martínez, J. M., Simó-Alfonso, E. F., Mendonça, C. R. B., and Ramis-Ramos, G. (2009). Composition, industrial processing and applications of rice bran γ-oryzanol. Food Chem. 115, 389–404. doi: 10.1016/j.foodchem.2009.01.063
Li, G., Jones, K. C., Eudes, A., Pidatala, V. R., Sun, J., Xu, F., et al. (2018). Overexpression of a rice BAHD acyltransferase gene in switchgrass (Panicum virgatum L.) enhances saccharification. BMC Biotechnol. 18:54. doi: 10.1186/s12896-018-0464-8
Lu, F., and Ralph, J. (1999). Detection and determination of p-coumaroylated units in lignins. J. Agric. Food Chem. 47, 1988–1992. doi: 10.1021/jf981140j
Manoli, A., Sturaro, A., Trevisan, S., Quaggiotti, S., and Nonis, A. (2012). Evaluation of candidate reference genes for qPCR in maize. J. Plant Physiol. 169, 807–815. doi: 10.1016/j.jplph.2012.01.019
Marita, J. M., Hatfield, R. D., Rancour, D. M., and Frost, K. E. (2014). Identification and suppression of the p-coumaroyl CoA:Hydroxycinnamyl alcohol transferase in Zea mays L. Plant J. 78, 850–864. doi: 10.1111/tpj.12510
Masarin, F., Gurpilhares, D. B., Baffa, D. C. F., Barbosa, M. H. P., Carvalho, W., Ferraz, A., et al. (2011). Chemical composition and enzymatic digestibility of sugarcane clones selected for varied lignin content. Biotechnol. Biofuels 4:55. doi: 10.1186/1754-6834-4-55
McCormick, R. F., Truong, S. K., Sreedasyam, A., Jenkins, J., Shu, S., Sims, D., et al. (2018). The Sorghum bicolor reference genome: improved assembly, gene annotations, a transcriptome atlas, and signatures of genome organization. Plant J. 93, 338–354. doi: 10.1111/tpj.13781
Mitchell, R. A. C., Dupree, P., and Shewry, P. R. (2007). A novel bioinformatics approach identifies candidate genes for the synthesis and feruloylation of Arabinoxylan. Plant Physiol. 144, 43–53. doi: 10.1104/pp.106.094995
Molinari, H. B. C., Pellny, T. K., Freeman, J., Shewry, P. R., and Mitchell, R. A. C. (2013). Grass cell wall feruloylation: distribution of bound ferulate and candidate gene expression in Brachypodium distachyon. Front. Plant Sci. 4:50. doi: 10.3389/fpls.2013.00050
Mota, T. R., de Souza, W. R., Oliveira, D. M., Martins, P. K., Sampaio, B. L., Vinecky, F., et al. (2020). Suppression of a BAHD acyltransferase decreases p-coumaroyl on arabinoxylan and improves biomass digestibility in the model grass Setaria viridis. Plant J. 105, 136–150. doi: 10.1111/tpj.15046
Mueller-Harvey, I., Hartley, R. D., Harris, P. J., and Curzon, E. H. (1986). Linkage of p-coumaroyl and feruloyl groups to cell-wall polysaccharides of barley straw. Carbohydr. Res. 148, 71–85. doi: 10.1016/0008-6215(86)80038-6
Ou, S., and Kwok, K. C. (2004). Ferulic acid: pharmaceutical functions, preparation and applications in foods. J. Sci. Food Agric. 84, 1261–1269. doi: 10.1002/jsfa.1873
Pei, K., Ou, J., Huang, J., and Ou, S. (2016). p-Coumaric acid and its conjugates: dietary sources, pharmacokinetic properties and biological activities. J. Sci. Food Agric. 96, 2952–2962. doi: 10.1002/jsfa.7578
Petrik, D. L., Karlen, S. D., Cass, C. L., Padmakshan, D., Lu, F., Liu, S., et al. (2014). p-Coumaroyl-CoA: monolignol transferase (PMT) acts specifically in the lignin biosynthetic pathway in Brachypodium distachyon. Plant J. 77, 713–726. doi: 10.1111/tpj.12420
Pfaffl, M. W. (2001). A new mathematical model for relative quantification in real-time RT-PCR. Nucleic Acids Res. 29:e45.
Ralph, J. (2010). Hydroxycinnamates in lignification. Phytochem. Rev. 9, 65–83. doi: 10.1007/s11101-009-9141-9
Ralph, J., Bunzel, M., Marita, J. M., Hatfield, R. D., Lu, F., Kim, H., et al. (2004). Peroxidase-dependent cross-linking reactions of p-hydroxycinnamates in plant cell walls. Phytochem. Rev. 3, 79–96. doi: 10.1023/B:PHYT.0000047811.13837.fb
Ralph, J., Hatfield, R. D., Grabber, J. H., Jung, H.-J. G., Quideau, S., and Helm, R. F. (1998). “Cell wall cross-linking in grasses by ferulates and diferulates,” in Lignin and Lignan Biosynthesis, eds N. G. Lewis and S. Sarkanen (Washington, DC: American Chemical Society), 209–236. doi: 10.1021/bk-1998-0697.ch016
Ralph, J., Hatfield, R. D., Quideau, S., Helm, R. F., Grabber, J. H., and Jung, H. J. G. (1994a). Pathway of p-coumaric acid incorporation into maize lignin as revealed by NMR. J. Am. Chem. Soc. 116, 9448–9456. doi: 10.1021/ja00100a006
Ralph, J., Quideau, S., Grabber, J. H., and Hatfield, R. D. (1994b). Identification and synthesis of new ferulic acid dehydrodimers present in grass cell walls. J. Chem. Soc. Perkin Trans. 1:3485. doi: 10.1039/p19940003485
Ramakers, C., Ruijter, J. M., Deprez, R. H. L., and Moorman, A. F. (2003). Assumption-free analysis of quantitative real-time polymerase chain reaction (PCR) data. Neurosci. Lett. 339, 62–66. doi: 10.1016/S0304-3940(02)01423-4
Rancour, D. M., Marita, J. M., and Hatfield, R. D. (2012). Cell wall composition throughout development for the model grass Brachypodium distachyon. Front. Plant Sci. 3:266. doi: 10.3389/fpls.2012.00266
Regner, M., Bartuce, A., Padmakshan, D., Ralph, J., and Karlen, S. D. (2018). Reductive cleavage method for quantitation of monolignols and low-abundance monolignol conjugates. ChemSusChem 11:1580. doi: 10.1002/cssc.201800958
Riaño-Pachón, D. M., and Mattiello, L. (2017). Draft genome sequencing of the sugarcane hybrid SP80-3280. F1000Res. 6, 1–7. doi: 10.12688/f1000research.11859.1
Ruijter, J. M., Ramakers, C., Hoogaars, W. M. H., Karlen, Y., Bakker, O., van den Hoff, M. J. B., et al. (2009). Amplification efficiency: linking baseline and bias in the analysis of quantitative PCR data. Nucleic Acids Res. 37:e45. doi: 10.1093/nar/gkp045
Saboe, P. O., Manker, L. P., Michener, W. E., Peterson, D. J., Brandner, D. G., Deutch, S. P., et al. (2018). In situ recovery of bio-based carboxylic acids. Green Chem. 20, 1791–1804. doi: 10.1039/c7gc03747c
Sibout, R., Proost, S., Hansen, B. O., Vaid, N., Giorgi, F. M., Ho-Yue-Kuang, S., et al. (2017). Expression atlas and comparative coexpression network analyses reveal important genes involved in the formation of lignified cell wall in Brachypodium distachyon. New Phytol. 215, 1009–1025. doi: 10.1111/nph.14635
Siqueira, G., Milagres, A. M., Carvalho, W., Koch, G., and Ferraz, A. (2011). Topochemical distribution of lignin and hydroxycinnamic acids in sugar-cane cell walls and its correlation with the enzymatic hydrolysis of polysaccharides. Biotechnol. Biofuels 4, 1–9. doi: 10.1186/1754-6834-4-7
Timokhin, V. I., Regner, M., Motagamwala, A. H., Sener, C., Karlen, S. D., Dumesic, J. A., et al. (2020). Production of p -coumaric acid from corn GVL-lignin. ACS Sustain. Chem. Eng. 8, 17427–17438. doi: 10.1021/acssuschemeng.0c05651
van der Pol, E., Bakker, R., van Zeeland, A., Sanchez Garcia, D., Punt, A., and Eggink, G. (2015). Analysis of by-product formation and sugar monomerization in sugarcane bagasse pretreated at pilot plant scale: differences between autohydrolysis, alkaline and acid pretreatment. Bioresour. Technol. 181, 114–123. doi: 10.1016/j.biortech.2015.01.033
van der Weijde, T., Alvim Kamei, C. L., Torres, A. F., Vermerris, W., Dolstra, O., Visser, R. G., et al. (2013). The potential of C4 grasses for cellulosic biofuel production. Front. Plant Sci. 4:107. doi: 10.3389/fpls.2013.00107
Vandesompele, J., De Preter, K., Pattyn, F., Poppe, B., Van Roy, N., De Paepe, A., et al. (2002). Accurate normalization of real-time quantitative RT-PCR data by geometric averaging of multiple internal control genes. Genome Biol. 3:research0034.1. doi: 10.1186/gb-2002-3-7-research0034
Vettore, A. L., da Silva, F. R., Kemper, E. L., Souza, G. M., da Silva, A. M., Ferro, M. I. T., et al. (2003). Analysis and functional annotation of an expressed sequence tag collection for tropical crop sugarcane. Genome Res. 13, 2725–2735.
Vicentini, R., Bottcher, A., Dos Santos Brito, M., Dos Santos, A. B., Creste, S., De Andrade Landell, M. G., et al. (2015). Large-scale transcriptome analysis of two sugarcane genotypes contrasting for lignin content. PLoS One 10:e0134909. doi: 10.1371/journal.pone.0134909
Vicentini, R., Del Bem, L. E. V., van Sluys, M. A., Nogueira, F. T. S., and Vincentz, M. (2012). Gene content analysis of sugarcane public ESTs reveals thousands of missing coding-genes and an unexpected pool of grasses conserved ncRNAs. Trop. Plant Biol. 5, 199–205. doi: 10.1007/s12042-012-9103-z
Wilkerson, C. G., Mansfield, S. D., Lu, F., Withers, S., Park, J.-Y., Karlen, S. D., et al. (2014). Monolignol ferulate transferase introduces chemically labile linkages into the lignin backbone. Science 344, 90–93. doi: 10.1126/science.1250161
Winter, D., Vinegar, B., Nahal, H., Ammar, R., Wilson, G. V., and Provart, N. J. (2007). An “electronic fluorescent pictograph” browser for exploring and analyzing large-scale biological data sets. PLoS One 2:e718. doi: 10.1371/journal.pone.0000718
Withers, S., Lu, F., Kim, H., Zhu, Y., Ralph, J., and Wilkerson, C. G. (2012). Identification of grass-specific enzyme that acylates monolignols with p-coumarate. J. Biol. Chem. 287, 8347–8355. doi: 10.1074/jbc.M111.284497
Keywords: BAHD acyltransferases, biomass engineering, biorefineries, ferulic acid (FA), p-coumaric acid (pCA)
Citation: Fanelli A, Rancour DM, Sullivan M, Karlen SD, Ralph J, Riaño-Pachón DM, Vicentini R, Silva TF, Ferraz A, Hatfield RD and Romanel E (2021) Overexpression of a Sugarcane BAHD Acyltransferase Alters Hydroxycinnamate Content in Maize Cell Wall. Front. Plant Sci. 12:626168. doi: 10.3389/fpls.2021.626168
Received: 05 November 2020; Accepted: 12 March 2021;
Published: 21 April 2021.
Edited by:
Berit Ebert, The University of Melbourne, AustraliaReviewed by:
Rowan Mitchell, Rothamsted Research, United KingdomLaura E. Bartley, Washington State University, United States
Copyright © 2021 Fanelli, Rancour, Sullivan, Karlen, Ralph, Riaño-Pachón, Vicentini, Silva, Ferraz, Hatfield and Romanel. This is an open-access article distributed under the terms of the Creative Commons Attribution License (CC BY). The use, distribution or reproduction in other forums is permitted, provided the original author(s) and the copyright owner(s) are credited and that the original publication in this journal is cited, in accordance with accepted academic practice. No use, distribution or reproduction is permitted which does not comply with these terms.
*Correspondence: Elisson Romanel, romanel@usp.br