- National Engineering Laboratory for Resource Development of Endangered Crude Drugs in Northwest of China, Key Laboratory of the Ministry of Education for Medicinal Resources and Natural Pharmaceutical Chemistry, College of Life Sciences, Shaanxi Normal University, Xi’an, China
Salvia miltiorrhiza Bunge (S. miltiorrhiza), a traditional Chinese medicinal herb, contains numerous bioactive components with broad range of pharmacological properties. By increasing the levels of endogenous jasmonate (JA) in plants or treating them with methyl jasmonate (MeJA), the level of tanshinones and salvianolic acids can be greatly enhanced. The jasmonate ZIM (JAZ) proteins belong to the TIFY family, and act as repressors, releasing targeted transcriptional factors in the JA signaling pathway. Herein, we identified and characterized 15 TIFY proteins present in S. miltiorrhiza. Quantitative reverse transcription PCR analysis indicated that the JAZ genes were all constitutively expressed in different tissues and were induced by MeJA treatments. SmJAZ3, which negatively regulates the tanshinones biosynthesis pathway in S. miltiorrhiza and the detailed molecular mechanism is poorly understood. SmJAZ3 acts as a bait protein to capture and identify a WD-repeat containing the protein SmWD40-170. Further molecular and genetic analysis revealed that SmWD40-170 is a positive regulator, promoting the accumulation of secondary metabolites in S. miltiorrhiza. Our study systematically analyzed the TIFY family and speculated a module of the JAZ-WD40 complex provides new insights into the mechanisms regulating the biosynthesis of secondary metabolites in S. miltiorrhiza.
Introduction
The plant-specific TIFY family is characterized by a highly conserved motif (TIF[F/Y]XG) positioned within a TIFY domain of approximately 28-amino acids (aa) (Vanholme et al., 2007; Bai et al., 2011). According to phylogenetic and structural analyses, genes in that family can be assigned to four subgroups: TIFY, JAZ, PEAPOD (PPD), and ZIM-like (ZML). The TIFY subfamily proteins contain only a TIFY domain, whereas the ZML subfamily, including the ZIM and ZML proteins, contain a C2C2-GATA zinc-finger domain and a CCT domain (CONSTANS, CO-like, TOC1) (Staswick, 2008; Chung et al., 2009). In addition to the TIFY domain, the JAZ subfamily proteins are characterized by a conserved Jas motif of approximately 27 aa, near the C-terminal. These Jas sequences possess the characteristic SLX2FX2KRX2RX5PY motif and are similar to the N-terminal portion of the CCT domain (Staswick, 2008; Chung et al., 2009). In contrast, the PPD subfamily proteins possess a characteristic N-terminal PPD domain, and a modified Jas motif, that lacks the conserved PY (proline tyrosine) in the C-terminal region (Chung et al., 2009). Genes in the TIFY family have previously been systematically analyzed in several plant species, including 36 in Brassica rapa, 21 in Brachypodium distachyon, 34 in Glycine soja, 19 in grape (Vitis vinifera), 20 in rice (Oryza sativa), and 18 in Arabidopsis (Vanholme et al., 2007; Ye et al., 2009; Bai et al., 2011; Zhang et al., 2012, 2015; Zhu et al., 2013; Saha et al., 2016). The members of this family are involved in regulating diverse aspects of plant development, responses to abiotic stresses, and phytohormone treatments. For example, the PvTIFY gene plays a vital role in the adaptation of Phaseolus vulgaris to phosphorus (P) starvation by mediating JA signaling (Aparicio-Fabre et al., 2013). Certain VvTIFY genes in grapes can be induced by osmotic, low temperature, or drought, salinity conditions, as well as jasmonic acid (JA), or abscisic acid (ABA) treatments (Zhang et al., 2012). In rice, most OsTIFY genes are responsive to at least one type of abiotic stress, such as drought, salinity, or low temperatures (Ye et al., 2009). Perhaps the best-characterized members are the JAZ genes, which play a key role in the JA pathway (Chini et al., 2007; Thines et al., 2007; Yan et al., 2007; Garrido-Bigotes et al., 2019). Jasmonoyl-isoleucine (JA-Ile), the bioactive JA, is an important plant hormone that regulates various biological processes, including plant development, defense processes, and secondary metabolism (Creelman and Mullet, 1997; Liechti and Farmer, 2002; Farmer et al., 2003; De Geyter et al., 2012; Wasternack and Hause, 2013). Proteomic analysis of the JAZ interacting proteins under MeJA treatments in Eleusine coracana, illustrated that EcJAZ acts as a signaling hub for JA and other phytohormone signaling pathways (Sen et al., 2016). Moreover, JAZ expression, which regulates and fine-tunes the expression of downstream JA-responsive genes, is differential in various pathways and with certain stress responses (Demianski et al., 2011).
Salvia miltiorrhiza (S. miltiorrhiza), a model medicinal plant, is a well-known traditional Chinese herb (Ma et al., 2012; Xu et al., 2015). Its dried roots have been used to treat cardiovascular and cerebrovascular disorders, such as coronary heart disease, hyperlipidemia, and acute ischemic strokes (Su et al., 2015; Liu et al., 2016). S. miltiorrhiza has two primary active compounds namely water-soluble phenolic acids, including caffeic acid, rosmarinic acid, and salvianolic acid B (Liu et al., 2006), and lipid-soluble tanshinones containing dihydrotanshinone, cryptotanshinone, tanshinone I, and tanshinone IIA (Kai et al., 2011). These natural products can accumulate at low levels in plants over a long period (Ma et al., 2015). Both biotic and abiotic elicitors can induce the accumulation of secondary metabolites in S. miltiorrhiza (Zhao et al., 2010). Treatment with MeJA can lead to marked increase in tanshinones and salvianolic acids levels, as well as the expression of genes involved in their biosynthesis (Gu et al., 2012; Luo et al., 2015). However, it remains unclear how JA modulates the synthesis of these secondary metabolites in S. miltiorrhiza. The process of JA signal transduction occurs in three main stages: (1) The bioactive JA, JA-Ile is recognized by coronatine-insensitive 1 (COI1) protein forming Skp1/Cullin1/F-box protein COI1 (SCFCOI1) complexes. (2) JAZ proteins are ubiquitinated by SCFCOI1-type E3 ubiquitin ligase and degraded by the 26S proteasome. (3) MYC TFs are released thereby inducing the expression of downstream genes (Farmer, 2007). The JAs, SCFCOI1 receptor complex, JAZ repressors, and TFs are all involved in JA signal transduction (Xie et al., 1998; Xu et al., 2002; Boter et al., 2004; Thines et al., 2007; Chini et al., 2010). JAZ is an important juncture that represses responses to JA by interacting with bHLH-TFs (MYC2, MYC3, MYC4, MYC5, GL3, EGL3, and TT8) and R2R3-MYB TFs (PAP, GL1, MYB21, and MYB24) (Fernández-Calvo et al., 2011; Song et al., 2011, 2013; Fonseca et al., 2014; Qi et al., 2014; Garrido-Bigotes et al., 2020). Certain JAZ proteins and their interaction partners have been identified in S. miltiorrhiza. SmMYC2a and SmMYC2b may interact with SmJAZ1 and SmJAZ2 to positively regulate tanshinones and salvianolic acid B production (Zhou et al., 2016; Yang et al., 2017). SmJAZ8 is a repressor involved in JA-induced biosynthesis of salvianolic acids and tanshinones via interactions with SmMYC2a (Ge et al., 2015; Pei et al., 2018). SmJAZ3 and SmJAZ9 negatively regulate tanshinones biosynthesis and JA signaling pathway in S. miltiorrhiza (Shi et al., 2016). SmJAZ9 can interact with AtMYC2, whereas SmJAZ3 cannot. In our study, we investigated the interaction partners of SmJAZ3 protein and molecular mechanism underlaying SmJAZ3 role in JA signaling pathway to regulate secondary metabolism.
WD40 repeat (WDR)-containing proteins feature a conserved sequence of approximately 40 amino acids, identified as the WD40 motif, which begins with glycine-histidine (Gly-His) and ends with tryptophan-aspartate (Trp-Asp) (Neer et al., 1994; Smith et al., 1999). Without a DNA-binding site, WD40 functions as a rigid scaffold for protein–protein and protein–DNA interactions, rather than directly regulating gene expression (Ramsay and Glover, 2005). Among the WD40 families, TTG1 has been the most studied regarding secondary metabolism. AtTTG1 and ortholog TTG1 genes from Zea mays, Medicago truncatula, Vitis vinifera, and Malus domestica have been reported to be involved in the biosynthesis of anthocyanins or flavonoids (Walker et al., 1999; Carey et al., 2004; Pang et al., 2009; Matus et al., 2010; An et al., 2012). We have previously reported that SmTTG1 increased salvianolic acid B accumulations by forming the SmTTG1-SmMYB111-SmbHLH51 ternary transcription complex (Li et al., 2018). We also identified 225 SmWD40 genes and analyzed the evolutionary relationship, gene structure, and conserved protein motif, which provided prelamination data for studying the function of SmWD40 in secondary metabolism (Liu et al., 2020).
In this study, we aimed to identify and characterize TIFY proteins present in S. miltiorrhiza. Phylogenetic trees, gene structures, and conserved motif analyses were conducted on the SmTIFY proteins. Further, a Y2H screening assay was used to elucidate the molecular mechanisms of SmJAZ3 in mediating JA signaling and secondary metabolism. The functions of its interaction partner, SmWD40-170, were then also identified and analyzed. We hypothesize that the molecular mechanism underlaying JAZ3-mediated regulation of secondary metabolism is via the JAZ-WD40 regulatory module in S. miltiorrhiza.
Materials and Methods
Plant Material
Salvia miltiorrhiza plants were acquired from Shangluo County, Shaanxi Province, China, and maintained in our laboratory at Shaanxi Normal University, Xi’an, China. Root, stem, leaf, and flower samples were collected from uniformly grown 2-year-old plants and immediately frozen in liquid nitrogen.
Tissue culture-derived plants of S. miltiorrhiza were used for the JA treatment experiments. After 30 days of culturing under normal laboratory conditions, the plants were assigned to two groups: (1) mock control, in which the leaves were sprayed with 10% ethanol; or (2) JA treatment, in which the leaves were sprayed with a solution of 100 μM MeJA plus 10% ethanol (Gu et al., 2012). Each experiment was performed three times (n = 3 per group) and whole plants were harvested from each group after 0.5 h, 1 h, 2 h, 4 h, 8 h, and 12 h. All samples were immediately frozen in liquid nitrogen and stored at −80°C until RNA extraction.
Identification and Characterization of TIFY Family Genes and Phylogenetic Analyses
To identify all of the putative SmTIFY family members, we used the sequences for 18 Arabidopsis TIFY family genes, as well as 20 from rice, and 19 from grape, that were obtained from the TAIR databases1, TIGR databases2, and the Grape Genome Database3, respectively. Local BLASTs were conducted with the S. miltiorrhiza genome (Xu et al., 2016). The protein sequences database for Arabidopsis, rice, and grape TIFY proteins were used as queries for BioEdit. The SmTIFYs were determined by screening for the conserved TIFY domains using an NCBI conserved domain search4, Pfam5, HM-MER6, InterPro7, and SMART8 online tools. MEGA 6 software9 was used to investigate the phylogenetic relationships among TIFY proteins in the four species, based on the neighbor-joining algorithm and the bootstrap method (1000 replicates).
Sequence Analysis of the SmTIFY Proteins
Relative molecular weights and isoelectric points (pI) of the TIFY family members were analyzed using ExPASy10. Subcellular localization of the proteins was determined according to WoLF PSORT11. The DNA and CDS sequences of the SmTIFY genes were submitted to the GSDS online tool12 to analyze their gene structures. The SmTIFY protein sequences were submitted to the MEME web server13 for analysis of the protein motifs. To explore potential cis-elements in the promoter sequences, 2000 bp of the SmTIFY genomic DNA upstream of the initiation codon (ATG) were downloaded, using the S. miltiorrhiza genome database. The promoter sequences were submitted to the Plant CARE database14 to predict the cis-acting elements.
Expression Analysis of SmTIFY Genes in Different Tissues and Under MeJA Treatment
Total RNA was extracted from the S. miltiorrhiza root, stem, leaf, and flower samples. First-strand cDNA was reversed from the total mRNA, according to the instructions for the Prime Script® RT Master Mix (Takara). RT-qPCR primer sequences were designed using Primer Premier 5.0, and a housekeeping gene (β-Actin) was used as an internal control (Supplementary Table 1). Several key genes encoding enzymes such as phenylalanine ammonialyase (PAL), cinnamate 4-hydroxylase (C4H), hydroxycinnamate-CoA ligase (4CL), tyrosine aminotransferase (TAT), hydroxyl phenylpyruvate reductase (HPPR), rosmarinic acid synthase (RAS), 1-deoxy-D-xylulose-5-phosphate synthase (DXS), 3-hydroxy-3-methylglutaryl-CoA reductase (HMGR), farnesyl diphosphate synthase (FPPS), geranylgeranyl diphosphate synthase (GGPPS), copalyl diphosphate synthase (CPS), and kaurene synthase-like synthase (KSL) were investigated using RT-qPCR (Supplementary Table 1). RT-qPCR analysis was conducted with SYBR Green (Takara Biotechnology) and a Roche LightCycler® 96 real-time PCR machine. All experiments were performed with three biological replicates. Relative expression levels were calculated based on the 2–△△Ct method (Vandesompele et al., 2002). Statistical significance was assessed using the Student’s t-test.
Yeast Two-Hybrid (Y2H) Screening and Assay
The CDS and partial Jas domain of the SmJAZ3 were amplified with specific primers (Supplementary Table 2) and cloned into the pGBKT7 vector as bait, to search for interacting proteins. The autoactivation test of the SmJAZ3 in the yeast was conducted as previously described (Li et al., 2018). Based on the previously described protocols (Pan et al., 2017), the S. miltiorrhiza cDNA library expressed in the Y187 yeast cells was mated with the AH109 yeast cells expressing SmJAZ3, and then screened for the interacting partners of SmJAZ3.
The Y2H assay was conducted to confirm the interaction between SmJAZ3 and SmWD40-170. The CDS of SmWD40-170 was cloned into pGADT7 to fuse with the activation domain as the prey. According to the manufacturer’s protocol for the Matchmaker Gold Yeast Two-Hybrid System (Clontech), BD-SmJAZ3 and AD-SmWD40-170 fusion constructs were co-transformed into yeast strain AH109, using the lithium acetate method (Gietz and Schiestl, 2007), and yeast cells were grown on SD/-Leu/-Trp medium. Positive clones were then selected on SD/-Ade/-His/-Leu/-Trp medium with X-α-gal. The sequences for the primers used are listed in Supplementary Table 2.
Bimolecular Fluorescence Complementation (BiFC) Assay
Based on the protocol from the Gateway technology manufacturer (Invitrogen, United States), the ORFs (without the termination codon) of SmJAZ3 (SmWD40-170) were amplified from pMD19T-SmJAZ3 (pMD19T-SmWD40-170) with adaptor primers (Supplementary Table 2) and then cloned into the pDONR207 vector using a BP recombination reaction. For the BiFC assay, pDONR207-SmJAZ3 and pDONR207-WD40-170 were cloned into pEarleyGate202-YC and pEarleyGate201-YN, respectively, using the LR recombination reaction (Earley et al., 2010). Equal concentrations of the YN and YC recombinant plasmids were mixed before co-transformation. Co-transformed YC-SmJAZ3/YN and YN-SmWD40-170/YC served as negative controls. Then, the mixed plasmids were bombarded into onion epidermal cells, using particle bombardment with the Gene Gun PDS-1000, and then incubated at 28°C for 24 h. The fluorescence signals were detected using a Leica DM6000B microscope (Leica, Germany) at an excitation wavelength of 475 nm.
Determination of Salvianolic Acids and Tanshinones Concentrations by Liquid Chromatography/Mass Spectrometry (LC/MS) Analysis
Transgenic lines were obtained by Agrobacterium-mediated transformation method (Yan and Wang, 2007). Three OE lines (OE-3, OE-7, and OE-8) and three RNAi lines (i-11, i-14, and i-15) were acquired from our laboratory and used for further studies (Liu et al., 2020). Two-month-old culture seedlings were first cultivated in hydroponic cultures for 7 days and then transplanted into the soil medium (perlite: vermiculite: grass ash = 1:1:3). After 2 months of cultivation, the roots were removed, washed, dried in an oven at 30°C, and then ground to a powder. The salvianolic acids and tanshinones compounds were extracted, as previously described (Wang et al., 2018).
Separation of the lipophilic tanshinone and hydrophilic salvianolic acid was performed using a conventional Welch Ultimate XB-C18 column with two ion monitoring modes (2.1 × 150 mm, 3 μm, Agilent Corporation, MA, United States) and the following conditions: mobile phase A, acetonitrile; mobile phase B, 0.1% formic acid; injection volume, 5 μL; flow rate, 0.4 mL/min; gradient elution conditions, 0 min (25% A) → 5 min (10% B); ion source, AJS (Agilent jet) and ESI (electrospray ionization); quantitative detection, multiple reaction monitoring (MRM) mode. The negative ion mode detection of salvianolic acid B and rosmarinic acid had the following properties: salvianolic acid B detection range (m/z), 717 519; rosmarinic acid detection range (m/z), 359 → 161; fragment voltage, 130 V; collision energy, 20 eV. The ion pattern test for the tanshinone IIA had the following properties: tanshinone IIA detection range (m/z), 295.1 → 277.1, fragment voltage of 140 V, and collision energy of 32 eV. The ion pattern test for cryptotanshinone had the following properties: cryptotanshinone detection range (m/z), 297.1 → 254.1, fragment voltage of 140 V, and collision energy of 26 eV. The standards and samples were tested according to the above conditions, and standard curves were constructed. The peak area measured with the standard solution was the ordinate, while the standard concentration was the abscissa. Regression equations and linear coefficients were then calculated.
Results
Identification and Characterization of the TIFY Family Genes in the S. miltiorrhiza Genome
In additions to the four SmJAZ genes that we have previously reported, a total of 15 SmTIFY genes (1 TIFY, 1 PPD, 3 ZMLs, and 10 JAZs) were identified in the S. miltiorrhiza genome (Table 1) (Ge et al., 2015; Xu et al., 2016). These were named according to the existing numbering system used for the phylogenetic tree of Arabidopsis (Figure 1). The lengths of the SmTIFY amino acids ranged from 122 to 455, which is higher than that of other species. Subcellular localization analysis indicated that 10 of the SmTIFY proteins were nuclear, while SmJAZ7, SmJAZ8, and SmJAZ10 were located in the chloroplasts, and SmJAZ1 and SmJAZ6 in the cytoplasm and mitochondria, respectively.
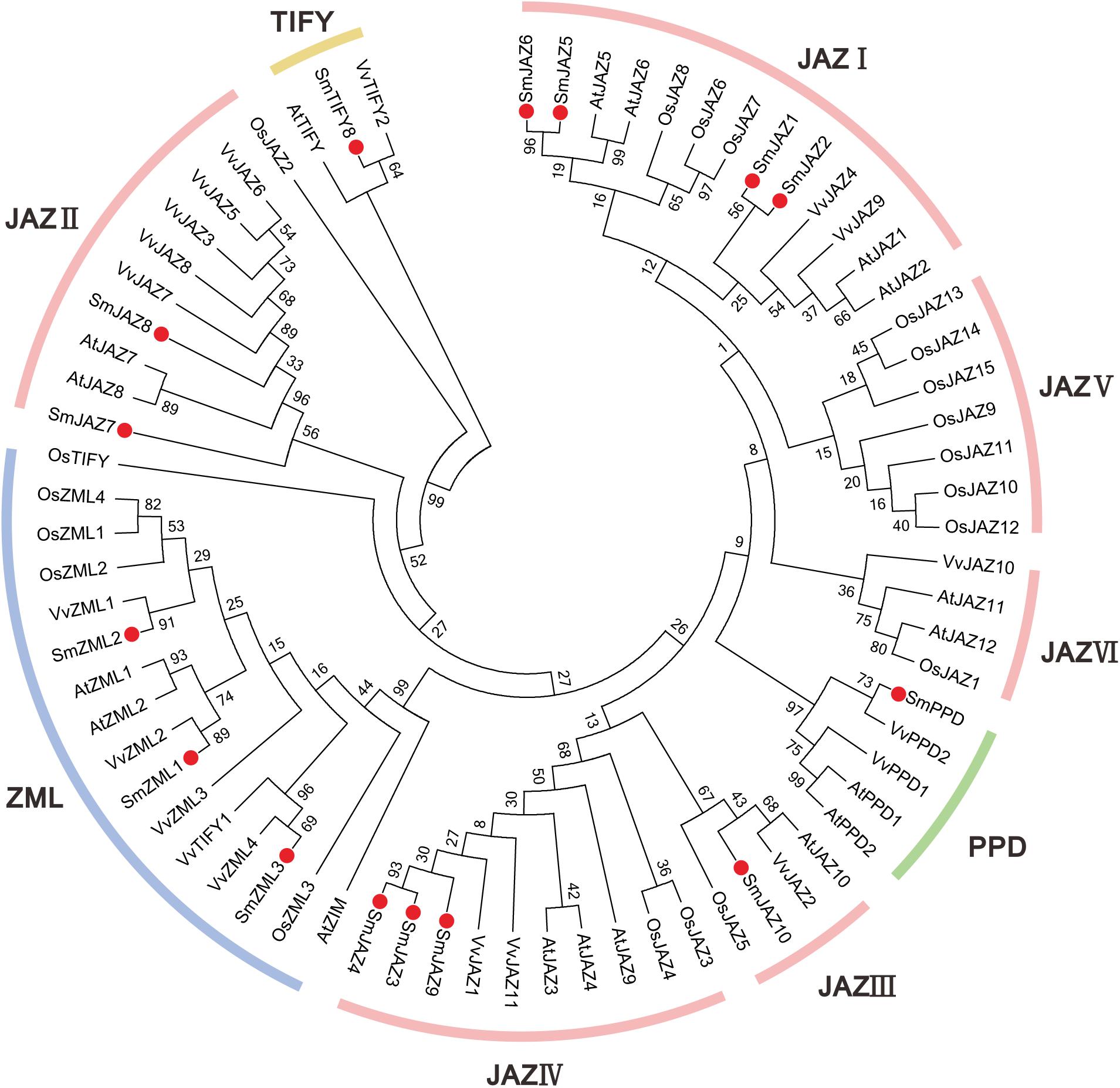
Figure 1. Neighbor-joining phylogenetic tree of TIFY proteins from Salvia miltiorrhiza, Arabidopsis, Vitis vinifera, and Oryza sativa. The tree was developed using MEGA 6 software and the bootstrap method (1000 replicates).
Phylogenetic Analysis of the TIFY Family Members
To investigate the evolutionary patterns and phylogenic relationships among the TIFYs in S. miltiorrhiza (15 proteins), grape (19 proteins), rice (20 proteins), and Arabidopsis (18 proteins), a neighbor-joining phylogenetic tree was constructed (Figure 1). All proteins fell into four major groups: TIFY, PPD, ZML, and JAZ. Among them, the ZML/ZIM proteins from all four species were clustered together. The TIFY proteins, which contain only a TIFY domain, were clustered into a single branch, except for OsTIFY. The PPD subfamily members comprised only AtPPD, VvPPD, and SmPPD. As expected, the JAZ subfamily members accounted for most proteins, including all the AtJAZ, VvJAZ, SmJAZ, and some OsTIFY (putative OsJAZ) proteins. SmJAZ proteins were clustered into four layers (JAZ I, JAZ II, JAZ III, and JAZ IV). SmJAZ1, SmJAZ2, SmJAZ5, and SmJAZ6 were assigned to JAZ I; SmJAZ7 and SmJAZ8, to JAZ II; and SmJAZ3, SmJAZ4, and SmJAZ9, to JAZ IV; JAZ III contained only one of these proteins, SmJAZ10.
Sequence Analysis of the SmTIFY Family
The full lengths of the CDSs with their corresponding genomic DNA sequences were compared to determine the number and positions of the exons and introns. Each SmTIFY gene has three to nine exons (Figure 2B). The structures in this family are diverse, especially within the SmJAZ subfamily. To identify the distribution of the conserved domains and the multiple sequence alignments among the SmTIFY proteins, we examined their sequences. The results from our MEME analysis showed that members of this family have six putative conserved domains, namely the TIFY domain, Jas domain, GATA zinc finger, CCT domain, PPD domain, and EAR-like motif (Figure 2A). In general, the TIFY domain contains 31 amino acids, with a highly conserved pattern of TIFYXG, T [L/I] SFXG, and SLSFQG (Figure 2C). All SmTIFY proteins include a TIFY domain, while all SmJAZ proteins have a Jas domain, with the conserved motif SLX2FX2KRX2RX5PY (Figure 2D). In addition, the N-terminals of SmJAZ1, SmJAZ2, SmJAZ5, and SmJAZ6 have EAR-like motifs. Three of the SmZML proteins (SmZML1, SmZML2, and SmZML3) contain a TIFY domain, Jas domain, CCT domain, and GATA zinc finger. The N-terminal of the SmPPD also has a PPD domain. In contrast to all other members, SmTIFY8 only has a TIFY domain.
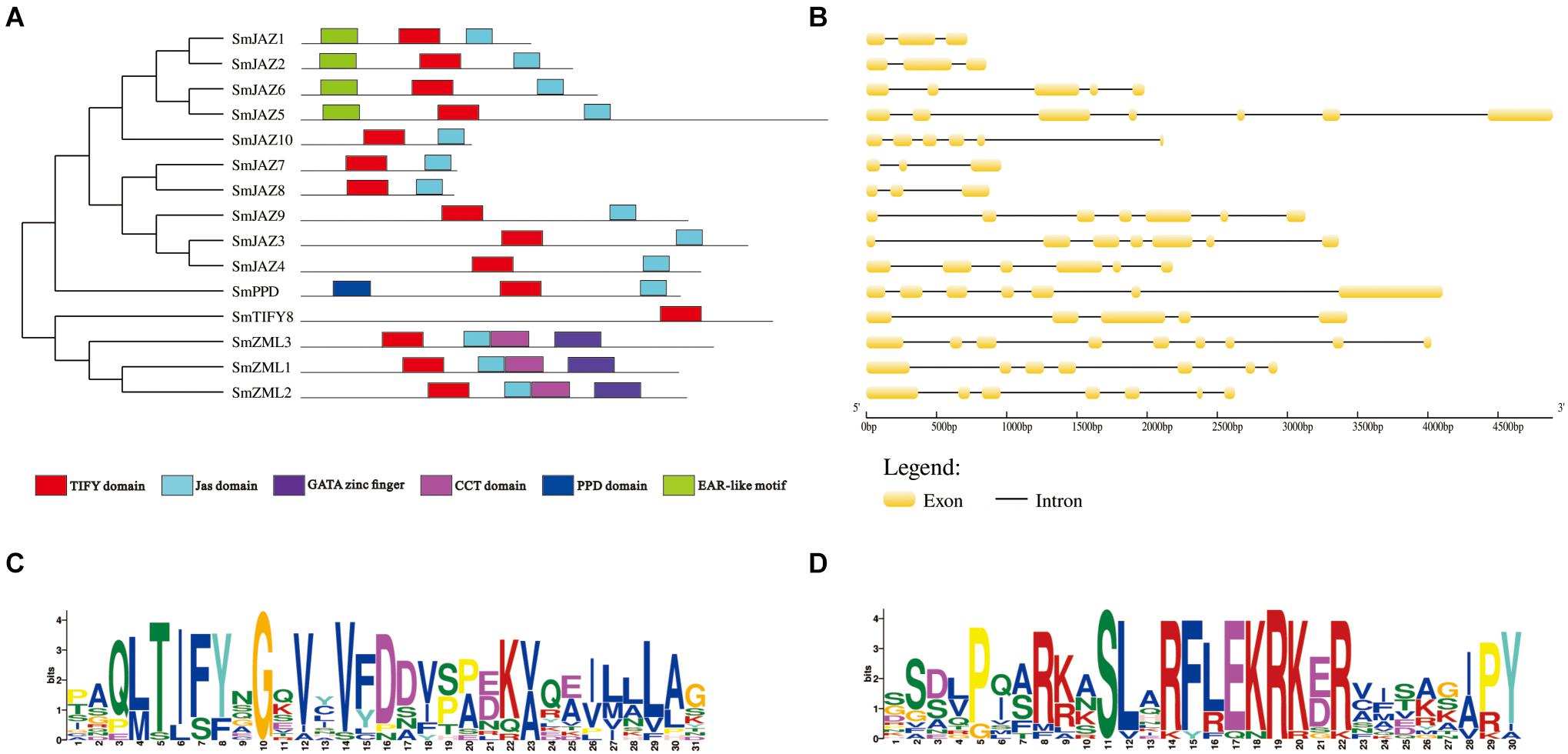
Figure 2. (A) Distribution of conserved domains within SmTIFY, SmJAZ, SmPPD, and SmZML proteins. Relative positions of domains within each protein are shown in different colors. (B) Gene structures of SmTIFY gene family. Exon, yellow-filled boxes; intron, black single lines. (C) Sequence logos of TIFY domains from SmTIFY proteins. (D) Sequence logos of Jas domains from SmTIFY proteins.
Analysis of the Cis-Elements in the SmTIFY Family
A search of the PlantCARE database showed the promoter sequences of the SmTIFYs. Among them, were a series of cis-elements that are involved in responses to light, biotic and abiotic stresses, phytohormones (ABA, MeJA, auxin, SA, gibberellin, and ethylene), circadian rhythms, and fungal elicitors (Supplementary Table 3). We also identified TF binding sites, such as an MBS and G-box. Almost all members, except SmPPD and SmZML1, contained TC-rich repeats that are involved in plant defenses and stress responsiveness. All these genes, except SmJAZ1, SmTIFY8, and SmZML2, also have TCA-elements that are responsive to SA. SmJAZ1, SmJAZ2, SmJAZ5, SmJAZ6, SmJAZ7, and SmZML2 have cis-elements involved in responses to MeJA. Except for SmJAZ1 and SmZML2, all genes contain a HSE and MBS in their promoter sequences, and, except for SmJAZ3, have a G-box, which is a possible MYC2-binding motif (Yu et al., 2016). However, only SmJAZ10 has C-repeat/DRE and WUN-motif elements that are involved in responses to cold, dehydration, and wounding. MBSI, an MBS that helps regulate genes for flavonoid biosynthesis, was only found in the SmPPD promoter sequence. All these cis-elements have essential roles in modulating gene expression, by controlling promoter efficiency. Therefore, these results provide vital information for further research into the functions of SmTIFY genes.
Expression Analysis of the SmTIFY Genes
To determine the function of the SmTIFY genes, we monitored the expression of these genes in four tissue types sampled from S. miltiorrhiza (Figure 3). The most highly expressed genes were as follows: SmJAZ1, SmJAZ6, SmJAZ8, and SmJAZ9 in the leaf; SmJAZ4, SmJAZ5, SmJAZ7, SmPPD, SmZML2 and SmZML3, in the flower; and SmJAZ3 was predominantly expressed in the root. Expressions of SmJAZ2, SmJAZ6, SmJAZ10, SmTIFY8, and SmZML1 were lower in the flower tissues, while the expressions of SmJAZ4 and SmJAZ7 were lower in the leaf tissues. Interestingly, the expression patterns of SmJAZ1 and SmJAZ8 were similar in different tissues. However, none of the genes in the same subfamily showed similar expression patterns, which indicates that each member plays an irreplaceable role. To investigate the role of the SmTIFY family genes in the JA signaling pathway, we monitored their expression in response to exogenous MeJA (Figure 4). Most genes were induced within 2 h of treatment and their expression continued to increase over time. In particular, the peak in expression of SmJAZ3, SmJAZ4, and SmJAZ9 was delayed compared with that of the other SmJAZ genes. In contrast, MeJA inhibited the expression of SmTIFY8, SmPPD, and SmZML1 at 2 h, but delayed the response of SmZML2 and SmZML3.
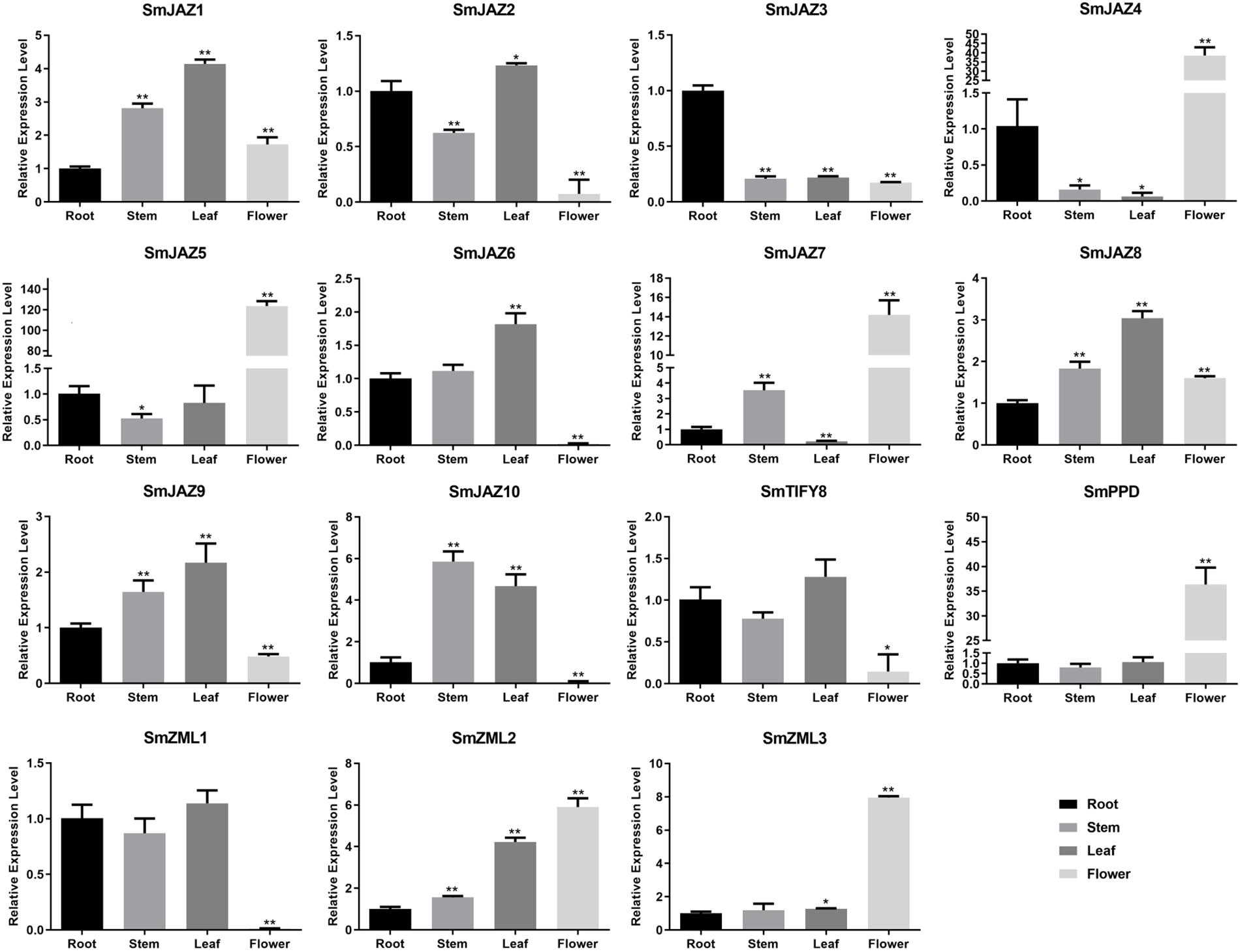
Figure 3. Relative expression of SmTIFY genes in root, stem, leave, and flower. All data represent averages of three biological replicates, error bars indicate SD. Statistical significance was determined using the Student’s t-test (*p < 0.05, **p < 0.01) between root, stem, leaf, and flower.
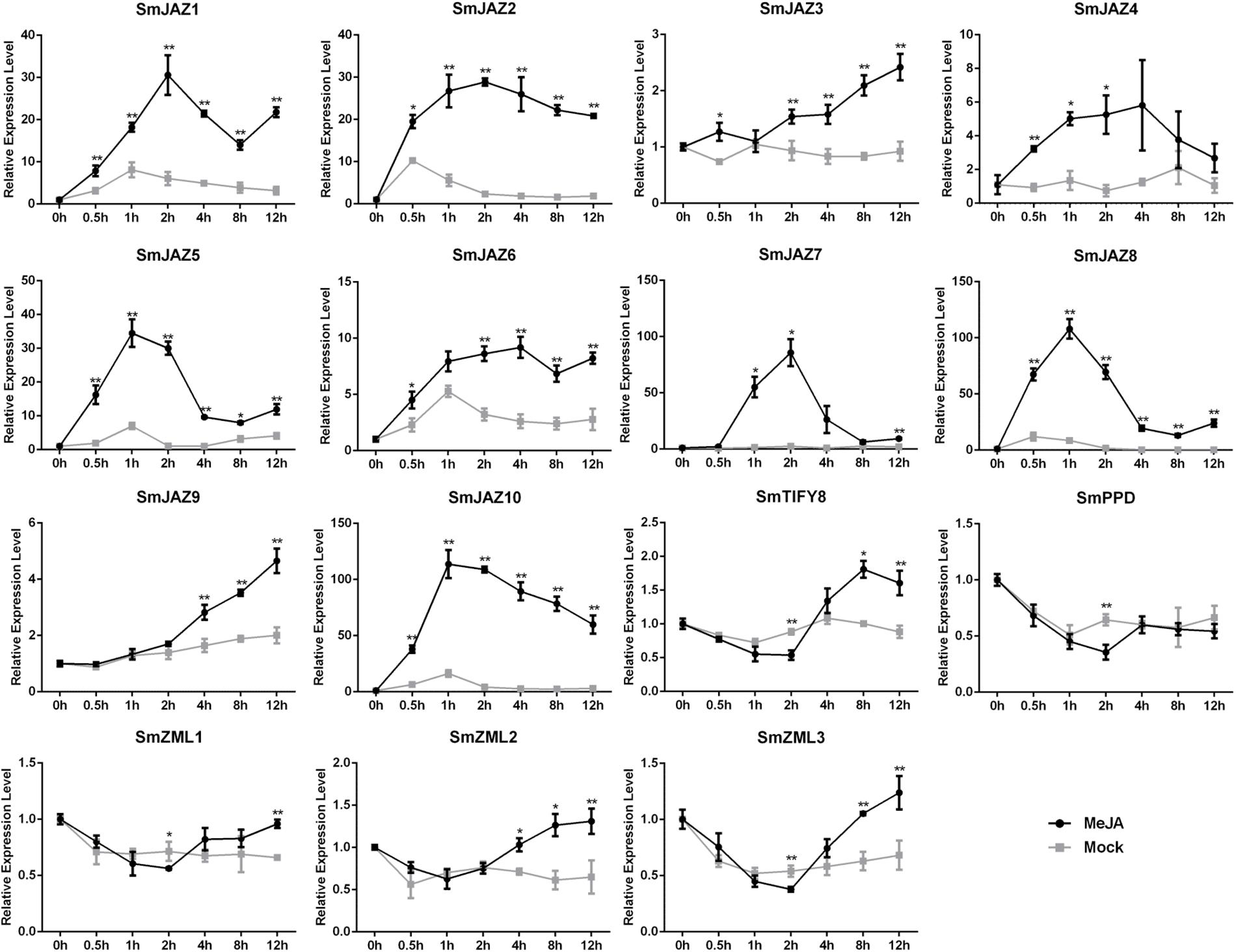
Figure 4. Relative expression level of SmTIFY genes in S. miltiorrhiza plants treated with mock control and 100 μM MeJA. All data represent averages of three biological replicates, error bars indicate SD. Statistical significance was determined by the Student’s t-test (*p < 0.05, **p < 0.01).
Y2H Screening
Since the JA-responsive gene SmJAZ3 negatively regulates tanshinones biosynthesis in S. miltiorrhiza (Shi et al., 2016), we selected SmJAZ3 as a candidate gene, to explore the molecular mechanisms underlaying the regulation of JA-mediated secondary metabolism of S. miltiorrhiza. To determine whether SmJAZ3 affects potential target proteins or TFs, we screened for interacting proteins using a Y2H system. Initially, no autoactivation of the SmJAZ3 baits was detected (Supplementary Figure 1C). Since the results were not satisfactory when the full-length SmJAZ3 was used as the bait protein for the library screening, we used the Jas domain as the bait. The screening for the cDNA library in S. miltiorrhiza, resulted in 36 candidate proteins being identified, that interacted with SmJAZ3-Jas (Supplementary Figure 1F and Supplementary Table 4). Function annotation suggested that these candidate interaction proteins including JA signal member MYC2, as well as some enzymes are associated with biosynthesis process, metabolism process, and stress resistance (Supplementary Table 4). Interestingly, a WD40 protein, SmWD40-170 (Gene ID: ATA66299), was among them. The full-length CDS of the SmWD40-170 was 972 bp, and it encoded a protein of 323 amino acids. It was located in the nucleus and the cytoplasm and is predicted to respond to MeJA-responsive elements (Liu et al., 2020). In addition, the SmWD40-170 responded to drought stress and regulated ABA- and H2O2-induced stomatal movements in the S. miltiorrhiza (Liu et al., 2020).
Interactions Between the SmJAZ3 and SmWD40-170 Proteins
We analyzed the interactions between the SmJAZ3 and SmWD40-170 proteins. For the Y2H, except for the full-length sequence, the SmJAZ3 was divided into three parts: N-terminal fragment (amino acids 1 to 275), Jas motif fragment (amino acids 276 to 304), and C-terminal fragment (amino acids 305 to 336), to examine whether other domains of the SmJAZ3 were responsible for the interaction with the SmWD40-170 protein (Figure 5A). The results showed that the full-length and Jas motif of the SmJAZ3 interacted with the SmWD40-170 in yeast (Figure 5B). A BiFC assay was then used to examine the Y2H results. SmWD40-170 and SmJAZ3 were fused with the N-terminal and C-terminal, respectively, of the YFP. As expected, a strong fluorescent signal was detected in the nucleus when SmWD40-170 and SmJAZ3 were co-transformed into onion epidermal cells, whereas no fluorescent signal was observed in the control groups (Figure 5C). Taken together, our results suggest that SmJAZ3 could interact with SmWD40-170.
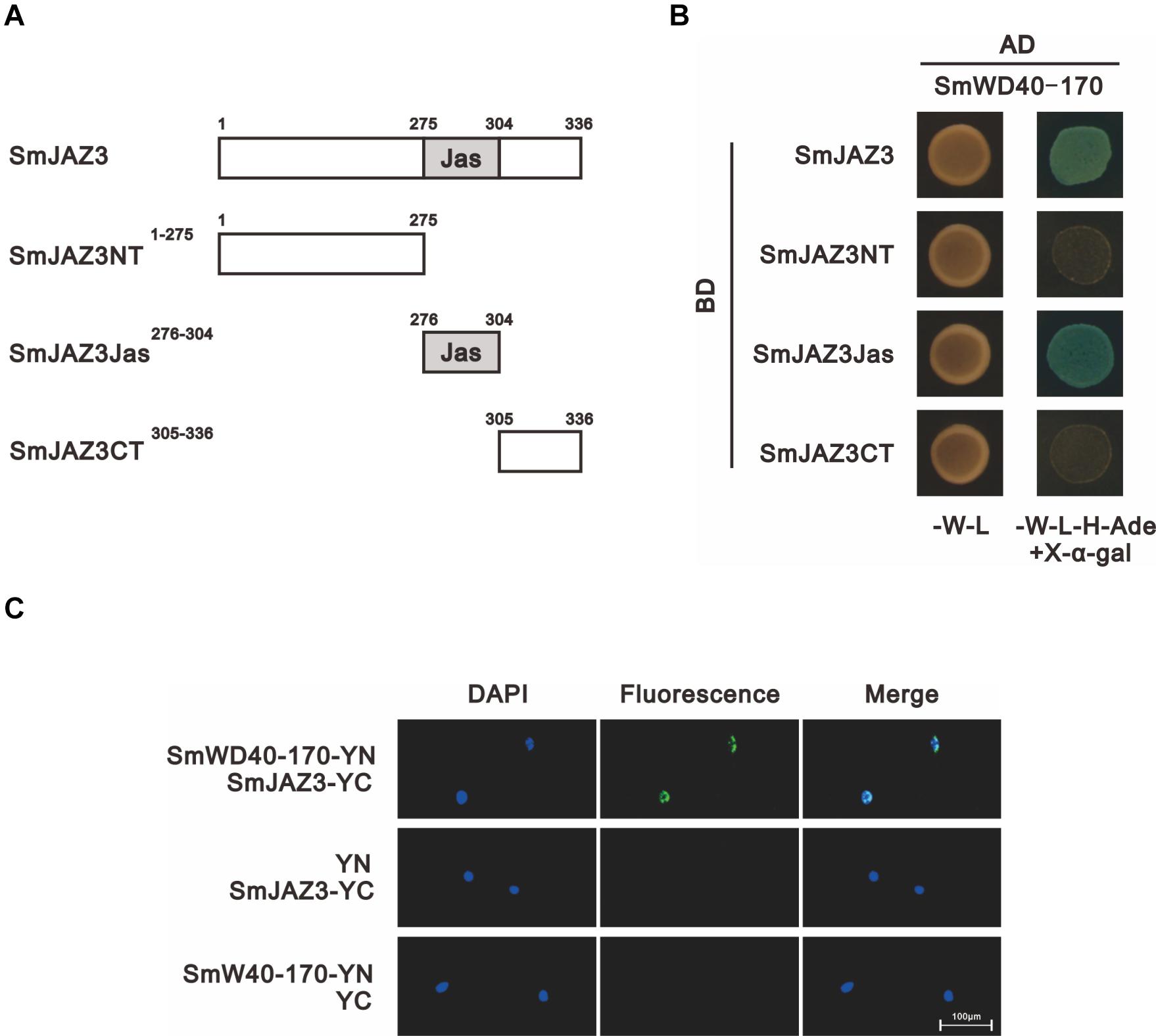
Figure 5. SmJAZ3 interacts with SmWD40-170. (A) Schematic diagrams show domain constructs of SmJAZ3. (B) Y2H assays was used to test the interactions of SmWD40-170 with different domains of SmJAZ3. (C) BiFC assays was used to detect the interaction between SmJAZ3 and SmWD40-170.
SmWD40-170 Affects the Biosynthesis and Accumulation of Secondary Metabolites in S. miltiorrhiza
The levels of the total phenolic acids and total flavonoids in the OE-3, OE-7, and OE-8 lines, as well as in the i-11, i-14, and i-15 RNAi lines, were determined using the Folin–Ciocalteu method and the sodium nitrite-aluminum chloride colorimetric method (Dewanto et al., 2002; Chew et al., 2009) with 2-month-old roots of S. miltiorrhiza. Compared to the CK, the total phenolic acids and total flavonoids contents increased by more than 2 and 1.5 times, respectively, in the three OE lines. In the three RNAi lines, especially i-14, the contents of both total phenolic acids and total flavonoids were significantly decreased by 59.47% and 50.63%, respectively (Figure 6).
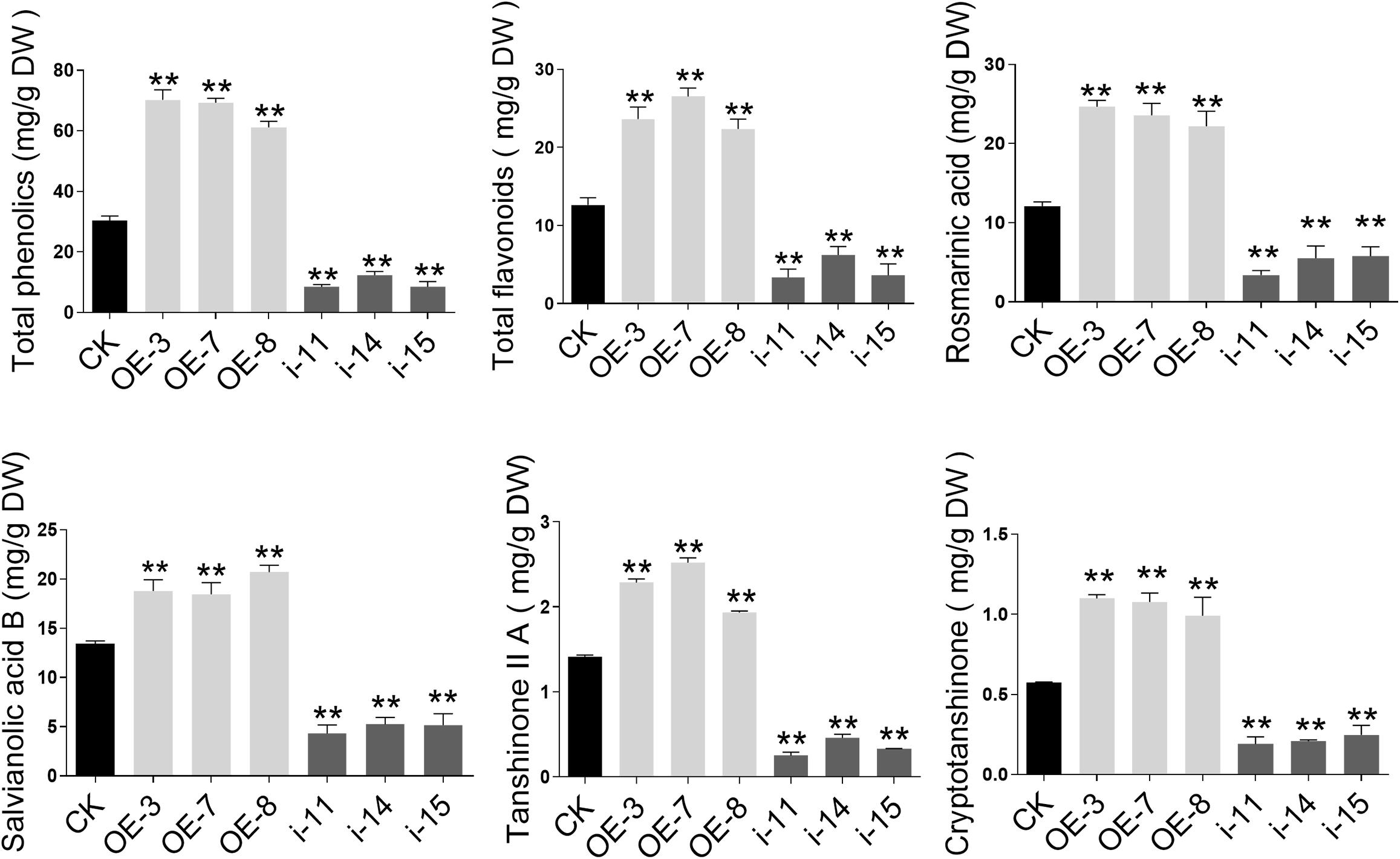
Figure 6. Secondary metabolites contents in control and SmWD40-170 transgenic roots of S. miltiorrhiza. Comparisons of total phenolic acid, total flavonoids, rosmarinic acid, salvianolic acid B, tanshinone IIA, and cryptotanshinone concentration among transgenic and control lines. All data represent averages of three biological replicates, error bars indicate SD. Statistical significance was determined using the Student’s t-test (**p < 0.01).
To further understand the effects of SmWD40-170 on the biosynthesis and accumulation of secondary metabolites in S. miltiorrhiza, the active components of salvianolic acids and tanshinones were more accurately determined and analyzed using LC/MS (Supplementary Figure 2). In comparison to CK, the concentrations of salvianolic acids and tanshinones were significantly increased in the OE-3, OE-7, and OE-8 lines (Figure 6). The rosmarinic acid content increased by 2.04 times, at most, and the salvianolic acid B content increased by approximately 1.54 times in the three OE lines, while the two tanshinones compounds increased by 1.37–1.78 times for tanshinone IIA and 1.72–1.91 times for cryptotanshinone, respectively. Compared to the CK, the levels of the three interference lines were the opposite, as was expected, and the contents of the four detected secondary metabolites were reduced by more than half. In particular, in the i-11 interference line, rosmarinic acid, salvianolic acid B, tanshinone II A, and cryptotanshinone were reduced by 71.93%, 67.95%, 82.23%, and 66.83%, respectively.
To determine whether metabolite accumulation was caused by changes in enzyme gene expression in the metabolic pathway, RT-qPCR was used to detect the expression of key enzyme genes involved in the salvianolic acids and tanshinones biosynthesis pathways in different lines. In the salvianolic acid biosynthesis pathway, the expression levels of SmTAT, SmHPPR, SmPAL, SmC4H, Sm4CL, SmRAS, and SmCYP98A14 in the OE lines were significantly upregulated compared to the CK, but the activities of all the enzymes were repressed in the three interference lines (Figures 7A,C). In the tanshinones biosynthesis pathway, several enzyme genes, such as SmDXS, SmHMGR, SmFPPS, SmGGPPS, SmCPS, SmKSL, and SmCYP76AH1 were markedly induced in the OE lines. In contrast, the expression levels of these genes were decreased in the interference lines compared to those in the CK (Figures 7B,D).
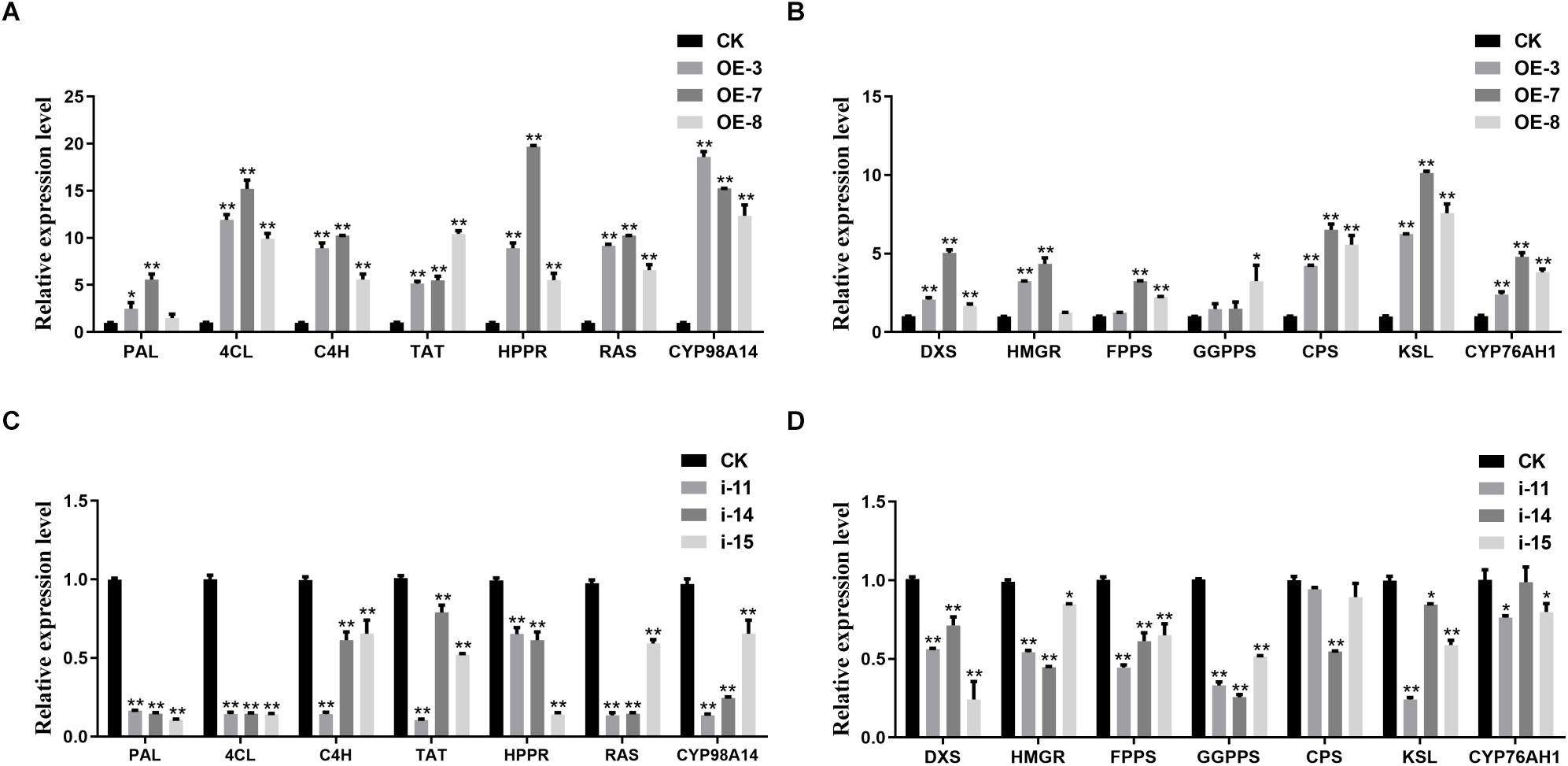
Figure 7. Expression analysis of salvianolic acids and tanshinones biosynthesis genes. RT-qPCR analyses of the key enzyme genes of salvianolic acids biosynthetic pathway in OE (A) and RNAi (C) lines. RT-qPCR analyses of the key enzyme genes of tanshinones biosynthetic pathway in OE (B) and RNAi (D) lines. All data represent averages of three biological replicates, error bars indicate SD. Statistical significance was determined using the Student’s t-test (*p < 0.05, **p < 0.01). PAL, phenylalanine ammonialyase; C4H, cinnamate 4-hydroxylase; 4CL, hydroxycinnamate-CoA ligase; TAT, tyrosine aminotransferase; HPPR, hydroxyl phenylpyruvate reductase; RAS, rosmarinic acid synthase; DXS, 1-deoxy-D-xylulose-5-phosphate synthase; HMGR, 3-hydroxy-3-methylglutaryl-CoA reductase; FPPS, farnesyl diphosphate synthase; GGPPS, geranylgeranyl diphosphate synthase; CPS, copalyl diphosphate synthase; KSL, kaurene synthase-like synthase.
Morphological Differences Between Transgenic Lines of SmWD40-170 in S. miltiorrhiza
After obtaining the OE and interference lines of S. miltiorrhiza, the plantlet seedlings were cultured for 60 days, transplanted into soil media as part of a hydroponic cultivation system, and then cultivated for another 60 days. There were obvious morphological differences in the OE and interference SmWD40-170 transgenic lines. The results showed that the growth state of OE-3 line was better than the CK line and had higher biomass accumulation in both leaves and roots. In contrast, the RNAi line was shorter, and the growth state was poorer (Figures 8A–F). Statistical data was consistent with the phenotype. Compared with the CK, the OE lines had the advantages of root length. The feature was more obvious compared to those in the RNAi lines (Figures 8G–I,M). In addition, morphological changes were observed in the leaves. There was no significant difference in leaf size between OE with CK lines, however, the RNAi lines had smaller leaves with curled leaf edges (Figures 8J–L,N). These results indicate that the SmWD40-170 protein is necessary for the growth and development of S. miltiorrhiza.
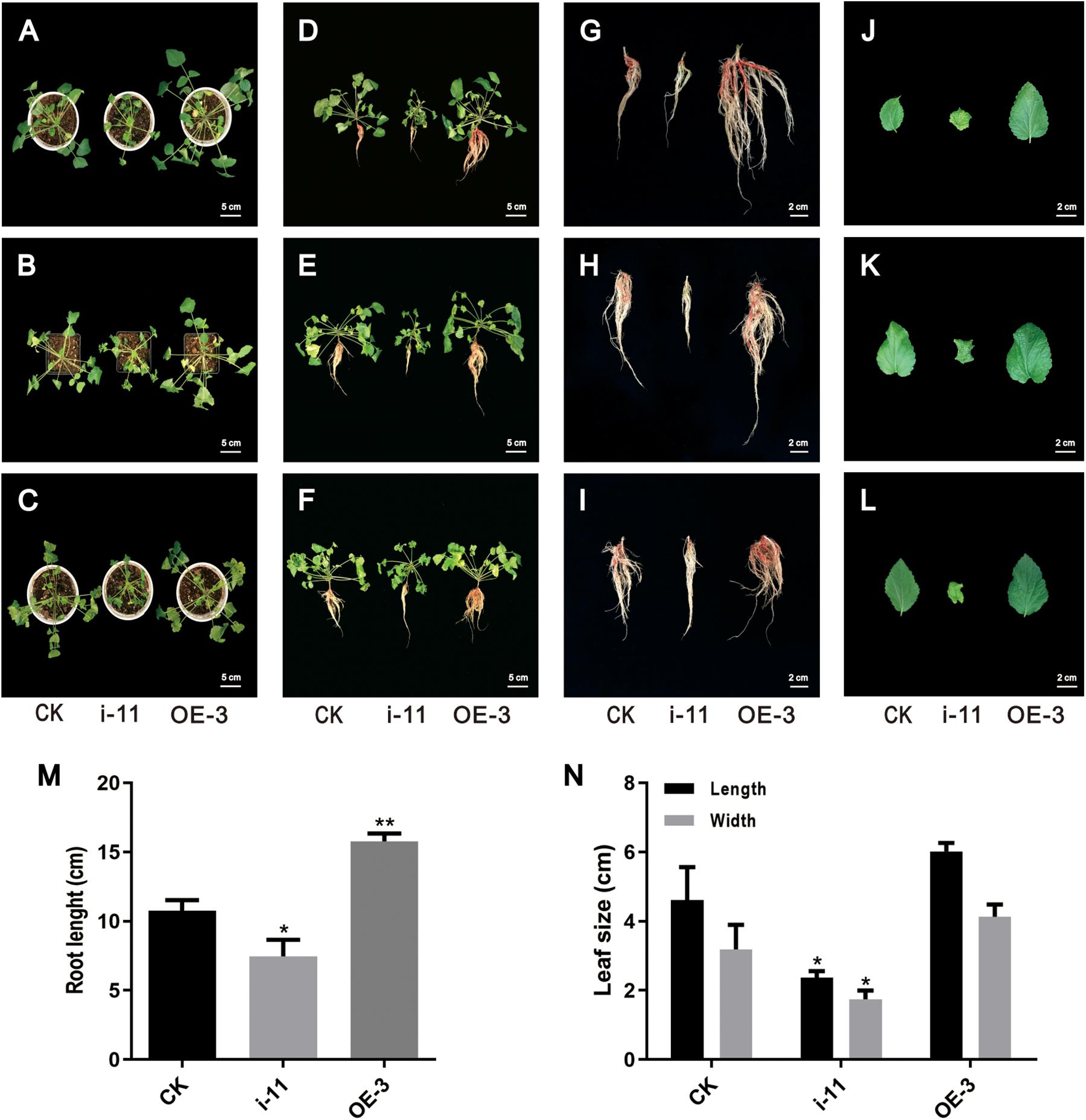
Figure 8. Morphological differences in the control and SmWD40-170 transgenic lines. (A/B/C,D/E/F,G/H/I,J/K/L) represent three independent repeats. (A–F) Control and transgenic seedlings cultured in soil for 2 months. (G–I) Control and transgenic roots. (J–L) Control and transgenic leaves. (M) Comparison of the root length in control and transgenic lines. (N) Comparison of the leaf size in control and transgenic lines. All data represent averages of three biological replicates, error bars indicate SD. Statistical significance was determined using the Student’s t-test (*p < 0.05, **p < 0.01).
Discussion
The plant-specific TIFY family plays vital roles in the growth, development, and secondary metabolism of plants (Vanholme et al., 2007; Ye et al., 2009; Bai et al., 2011; Zhang et al., 2012, 2015; Zhu et al., 2013; Saha et al., 2016). Significant progress has been made toward identifying and characterizing the TIFY genes in various species. In the present study, we identified and analyzed the TIFY families in S. miltiorrhiza. This species contains 10 SmJAZs, 3 SmZMLs, 1 SmTIFY, and 1 SmPPD genes (Table 1). Similar to other species, the SmJAZ proteins have two typical domains, N-terminal TIFY and C-terminal Jas. The TIFY domain participates in homomeric and heteromeric interactions, or in the interactions between JAZ proteins and MYC TFs (Bai et al., 2011). The Jas motif interacts with COI1, bHLH, or R2R3-MYB members (Chico et al., 2008; Browse, 2009). The SmZML proteins not only have these two domains, but also contain a CCT domain, similar to the Jas domain, and a GATA zinc finger. In contrast, SmPPD also has a PPD domain at its N-terminal (Figure 2A). SmJAZ1, SmJAZ2, SmJAZ5, and SmJAZ6 each carry an EAR-like motif (Figure 2A), which also exists in plant AUX/IAA proteins and functions as a binding motif for the regulator repressor TOPLESS. This suggests that these four proteins have different functions that are more critical than those of the other SmTIFY proteins (Szemenyei et al., 2008). Although all SmJAZ proteins could be clustered into the four groups previously described for other species (Figure 1), the high degree of variability among the sequences within this subfamily, suggests that these proteins have had a possible divergence in functions.
Members of the SmTIFY family are diverse in their exons and introns (Figure 2B), implying an important evolutionary role for their gene structure (Bai et al., 2011). Sequence alignments and phylogenetic analysis of the SmTIFY proteins indicated that almost all could be classified into the same four major groups with TIFY proteins from other species with the exception is for the rice members, from which OsPPD is missing. Screening the TIFY proteins from the different species has demonstrated that they only occur among Embryophyta (land plants) and that both group I (TIFY proteins with a C2C2-GATA domain) and group II (TIFY proteins without C2C2-GATA domain) of TIFY proteins are present in the liverworts (Marchantiophyta), which are considered primitive land plants (Vanholme et al., 2007). This suggests that the TIFY family was essential for the emergence of land plants during a series of evolutionary adaptations that increased the complexity of plant structure. This probably also enhanced the ability of those plants to respond to adverse environmental conditions. In contrast to many plant-specific gene families, obvious diversification has occurred between monocot and dicot species (Bai et al., 2011). Therefore, the plant TIFY genes may be derived from common ancestors that existed before the divergence of monocot and dicot species.
SmTTG1, a member of the WD40 protein family, has been shown to promote the accumulation of salvianolic acids in S. miltiorrhiza (Li et al., 2018). In the present study, we screened a WD40 protein, which participates in both salvianolic acids and tanshinones biosynthesis, except in drought stress responses (Liu et al., 2020). In the OE lines, the contents of the salvianolic acids and tanshinones were significantly increased, by approximately two fold, and the RNAi lines were expectedly decreased by at least 50%, which is consistent with the changes in the biosynthesis of the enzyme genes in the transgenic lines (Figures 6, 7). Notably, all 14 key genes selected were induced by SmWD40-170. However, the WD40 protein is unable to directly regulate enzyme genes, and probably functions together with MYB and bHLH proteins to generate the MBW complex. In future research, we will focus on investigating the interaction partners of the SmWD40-170 protein. In addition, some obvious phenotypic changes were observed in the transgenic lines. Compared with the CK line, the RNAi lines presented underdeveloped roots, smaller leaves, and curled leaf edges, but the OE lines showed the opposite traits, with well-developed roots (Figure 8). In particular, the roots of OE lines were redder than those of the CK and RNAi lines. These results are consistent with the content determination of tanshinones in the transgenic and CK lines (Figure 6), and in accordance with the fact that more tanshinones were gathered in the redder roots of S. miltiorrhiza (Wang et al., 2014). It is great significance when aiming to improve the quality of S. miltiorrhiza. SmWD40-170 not only regulates secondary metabolism, but also affects growth and development, however, links between growth and secondary metabolite accumulation warrant further investigation.
Jasmonic acid has been shown to enhance the accumulation of secondary metabolites and promote growth and development in S. miltiorrhiza (Xiao et al., 2010; Gu et al., 2012; Ge et al., 2015; Shi et al., 2020), where SmCOI1 also plays a critical role (Chen et al., 2018). JAZ proteins are the targets of the SCFCOI1 complex and function as key components of the JA signaling pathway (Shi et al., 2016; Pei et al., 2018), however, the mechanisms underlying JAZ-regulated repression events remain unclear for S. miltiorrhiza. Since JAZ proteins contain no DNA-binding domain, JAZs might affect gene expression and metabolite accumulation through their interactions with target genes (Cheng et al., 2011). It has been reported that a Jas motif of the JAZ proteins, participates in the protein–protein interactions with the MYB, bHLH, and other TFs (Withers et al., 2012). In the present study, we found that a WD40 protein interacts with the Jas motif of SmJAZ3 using Y2H screening, and determine the interaction relationship between the SmJAZ3 and SmWD40-170. SmJAZ3 has been reported to act as a repressive transcriptional regulator in tanshinones biosynthesis regulation (Shi et al., 2016), while SmWD40-170 responds to drought stress by regulating ABA- and H2O2- induced stomal movement in S. miltiorrhiza (Liu et al., 2020). In the present study, we speculated that SmWD40-170 regulates the accumulation of secondary metabolites by interacting with SmJAZ3 in S. miltiorrhiza, which brings insights into the molecular mechanism of SmJAZ3 regulates tanshinone biosynthesis. We propose that SmJAZ3 protein interacts with SmWD40-170 in the JA signaling pathway and plays a vital role in the accumulation of secondary metabolites (Figure 9), which provides essential information for further exploring the mechanisms by which JA regulates the biosynthesis and accumulation of secondary metabolites in S. miltiorrhiza.
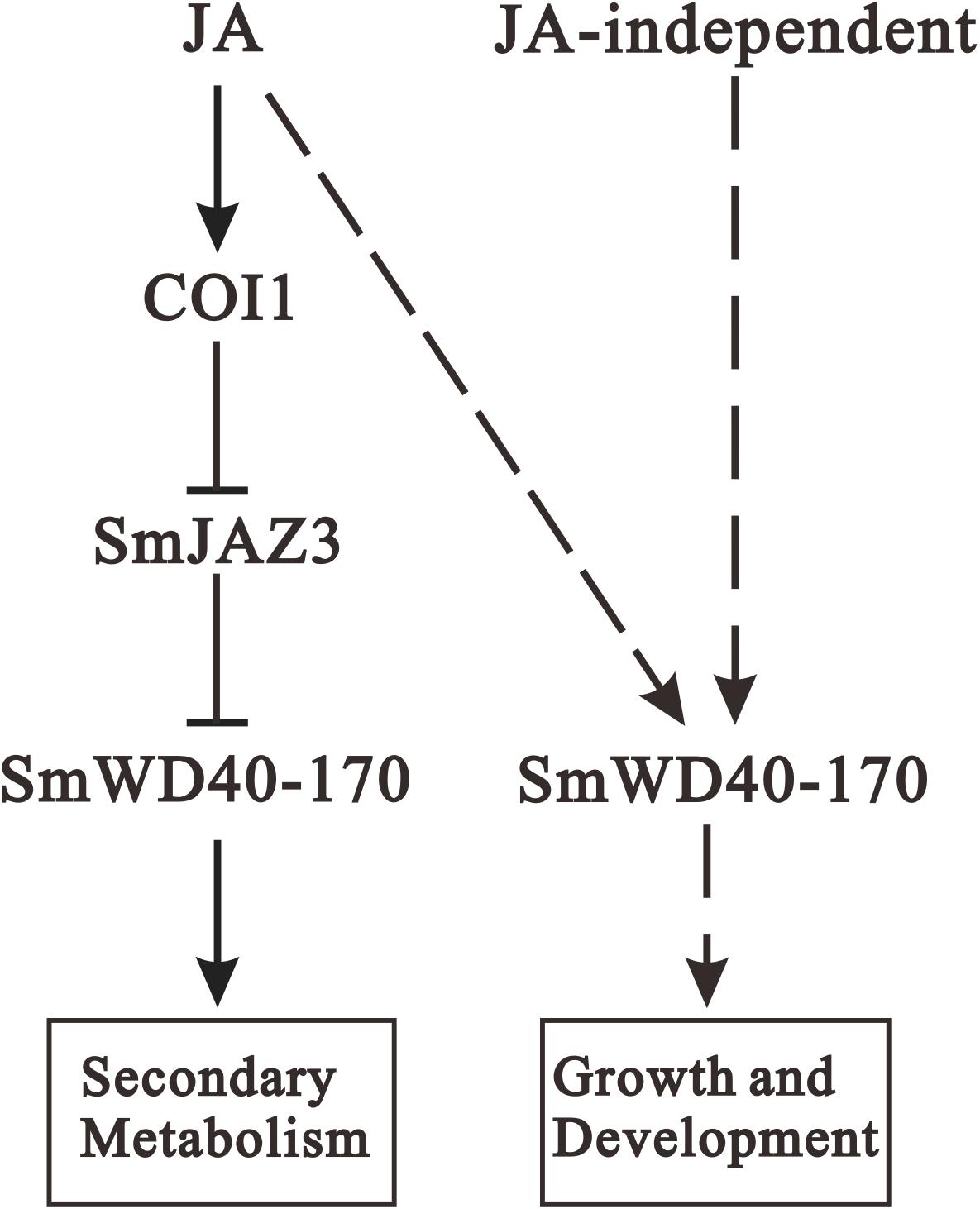
Figure 9. Proposed module of the roles of JA in regulating secondary metabolites biosynthesis as well as growth and development. When treating with exogenous JA, the complex formation between the JA-Ile and COI1 promotes SmJAZ3 degradation via the 26S proteasome, and release the positive regulators such as WD40, then enhances the activities of enzymes to promote secondary metabolites biosynthesis. In addition, SmWD40-170 protein may regulate growth and development through JA-dependent or JA-independent pathway. Arrows, positive regulation; blunt ends, negative regulation; dotted line, uncertified process.
Conclusion
This study identified and analyzed 15 SmTIFY family members. Phylogenetic analysis suggested that the SmTIFY proteins could be clustered into four groups. RT-qPCR results showed that most of the SmTIFY genes responded to the MeJA treatments. Our analysis illustrated diversity in the cis-elements among the SmTIFY members, indicating that these genes have important roles in several hormone signaling pathways and stress responses, and may thus be applied to increase the production of valuable plant compounds. Furthermore, a novel interaction partner of SmJAZ3 was screened and physical interactions between SmJAZ3 and SmWD40-170 was also demonstrated in this study, which suggests a potential regulation mechanism for the SmJAZ involved in the JA signaling pathway. Subsequently, genetic assays showed that SmWD40-170 positively induced the accumulation of salvianolic acids and tanshinones, and promoted plant growth and development. Collectively, our research lays a foundation for future investigations into the mechanism of the hormone signal regulation network among the SmTIFY family members.
Data Availability Statement
The original contributions presented in the study are included in the article/Supplementary Material, further inquiries can be directed to the corresponding authors.
Author Contributions
LL, XC, and ZW designed the experiments. LL, YH, and YL performed the experiments. YL, WM, and DW contributed analytical tools and provided technical support. LL and YH wrote the manuscript. BL, XC, and ZW promoted the manuscript. All authors revised and approved the manuscript.
Funding
This research was supported by the National Natural Science Foundation of China (31670299, 31870276, 31900254, and 31800259), and the Project of the National Key Technologies R&D Program for Modernization of Traditional Chinese Medicine (2017YFC1701300 and 2019YFC1712602), and Fundamental Research Funds for the Central Universities (GK202003056).
Conflict of Interest
The authors declare that the research was conducted in the absence of any commercial or financial relationships that could be construed as a potential conflict of interest.
Supplementary Material
The Supplementary Material for this article can be found online at: https://www.frontiersin.org/articles/10.3389/fpls.2021.630424/full#supplementary-material
Supplementary Figure 1 | Screening of cDNA library using SmJAZ3-jas as bait. SmJAZ3 (A) and SmJAZ3-jas (B) bait vectors construction for screening yeast two-hybrid library. (C) Transcriptional activity analysis of BD-SmJAZ3 in yeast. (D) Bait bacteria with library bacteria forming yeast conjugates. (E) Part of the positive clones of yeast two-hybrid screening. (F) Agarose gel electrophoresis examine the PCR products for part of the yeast plaque.
Supplementary Figure 2 | MRM maps of rosmarinic acid, salvianolic acid B, tanshinone IIA, and cryptotanshinone standard and sample.
Supplementary Table 1 | Primer sequences used for RT-qPCR.
Supplementary Table 2 | Primers for PCR and vectors construction.
Supplementary Table 3 | Part of cis-elements in SmTIFY family genes. A series of cis-elements involved in light response, the biotic and abiotic stress response, phytohormone (abscisic acid, auxin, salicylic acid, gibberellin, ethylene, MeJA) response, circadian control, MYB binding site, G-box, endosperm and meristem expression, and fungal elicitor response were identified.
Supplementary Table 4 | Yeast library screening with SmJAZ3-Jas-BD as bait protein, NCBI BlastX results.
Abbreviations
JA, jasmonate; MeJA, methyl jasmonate; JAZ, jasmonate ZIM; WD40 protein, WD40 repeat-containing protein; TF, transcription factor; CDS, coding sequence; RT-qPCR, quantitative reverse transcription PCR; LC/MS, liquid chromatography/mass spectrometry; CK, control check; OE, overexpression; RNAi, ribonucleic acid interference; YFP, yellow fluorescent protein; GFP, green fluorescent protein; Y2H, yeast two-hybrid; BiFC, bimolecular fluorescence complementation; EAR, ethylene-responsive element binding factor-associated amphiphilic repression; SA, salicylic acid; MBS, MYB binding site; HSE, heat shock element; DRE, dehydration responsive element; WUN, wound.
Footnotes
- ^ http://www.arabidopsis.org
- ^ http://rice.plantbiology.msu.edu
- ^ http://www.genoscope.cns.fr
- ^ https://www.ncbi.nlm.nih.gov/Structure/cdd/wrpsb.cgi
- ^ http://pfam.xfam.org/
- ^ http://www.ebi.ac.uk/Tools/hmmer/
- ^ http://www.ebi.ac.uk/interpro/search/sequence-search
- ^ http://smart.embl.de/
- ^ http://www.megasoftware.net/
- ^ http://web.expasy.org/protparam/
- ^ http://www.genscript.com/wolf-psort.html
- ^ http://gsds.gao-lab.org/
- ^ http://meme-suite.org/
- ^ http://bioinformatics.psb.ugent.be/webtools/plantcare/html/
References
An, X. H., Tian, Y., Chen, K. Q., Wang, X. F., and Hao, Y. J. (2012). The apple WD40 protein MdTTG1 interacts with bHLH but not MYB proteins to regulate anthocyanin accumulation. J. Plant Physiol. 169, 710–717. doi: 10.1016/j.jplph.2012.01.015
Aparicio-Fabre, R., Guillén, G., Loredo, M., Arellano, J., Valdés-López, O., Ramírez, M., et al. (2013). Common bean (Phaseolus vulgaris L.) PvTIFY orchestrates global changes in transcript profile response to jasmonate and phosphorus deficiency. BMC Plant Biol. 13:26. doi: 10.1186/1471-2229-13-26
Bai, Y. H., Meng, Y. J., Huang, D. L., Qi, Y. H., and Chen, M. (2011). Origin and evolutionary analysis of the plant-specific TIFY transcription factor family. Genomics 98, 128–136. doi: 10.1016/j.ygeno.2011.05.002
Boter, M., Ruiz-Rivero, O., Abdeen, A., and Prat, S. (2004). Conserved MYC transcription factors play a key role in jasmonate signaling both in tomato and Arabidopsis. Genes Dev. 18, 1577–1591. doi: 10.1101/gad.297704
Browse, J. (2009). Jasmonate passes muster: a receptor and targets for the defense hormone. Annu. Rev. Plant Biol. 60, 183–205.
Carey, C. C., Strahle, J. T., Selinger, D. A., and Chandler, V. L. (2004). Mutations in the pale aleurone color1 regulatory gene of the Zea mays anthocyanin pathway have distinct phenotypes relative to the functionally similar TRANSPARENT TESTA GLABRA1 gene in Arabidopsis thaliana. Plant Cell 16, 450–464. doi: 10.1105/tpc.018796
Chen, C., Cao, X. Y., Hua, W. P., Huang, Y., Lv, T. T., Zhang, Y., et al. (2018). Roles of SmCOI1 in pest resistance and secondary metabolism regulation based on Salvia miltiorrhiza Bunge genome. Sci. Sin. 48, 399–411. doi: 10.1360/N052017-00189
Cheng, Z. W., Sun, L., Qi, T. C., Zhang, B. S., Peng, W., Liu, Y. L., et al. (2011). The bHLH transcription factor MYC3 interacts with the jasmonate ZIM-domain proteins to mediate jasmonate response in Arabidopsis. Mol. Plant 4, 279–288. doi: 10.1093/mp/ssq073
Chew, Y. L., Goh, J. K., and Lim, Y. Y. (2009). Assessment of in vitro antioxidant capacity and polyphenolic composition of selected medicinal herbs from Leguminosae family in Peninsular Malaysia. Food Chem. 116, 13–18. doi: 10.1016/j.foodchem.2009.01.091
Chico, J. M., Chini, A., Fonseca, S., and Solano, R. (2008). JAZ repressors set the rhythm in jasmonate signaling. Curr. Opin. Plant Biol. 11, 486–494. doi: 10.1016/j.pbi.2008.06.003
Chini, A., Boter, M., and Solano, R. (2010). Plant oxylipins: COI1/JAZs/MYC2 as the core jasmonic acid-signalling module. FEBS J. 276, 4682–4692. doi: 10.1111/j.1742-4658.2009.07194.x
Chini, A., Fonseca, S., Fernandez, G., Adie, B., Chico, J. M., Lorenzo, O., et al. (2007). The JAZ family of repressors is the missing link in jasmonate signalling. Nature 448, 666–671. doi: 10.1038/nature06006
Chung, H. S., Niu, Y. J., Browse, J., and Howe, G. A. (2009). Top hits in contemporary JAZ: an update on jasmonate signaling. Phytochemistry 70, 1547–1559. doi: 10.1016/j.phytochem.2009.08.022
Creelman, R. A., and Mullet, J. E. (1997). Biosynthesis and action of jasmonates in plants. Ann. Rev. Plant Physiol. Plant Mol. Biol. 48, 355–381. doi: 10.1146/annurev.arplant.48.1.355
De Geyter, N., Gholami, A., Goormachtig, S., and Goossens, A. (2012). Transcriptional machineries in jasmonate-elicited plant secondary metabolism. Trends Plant Sci. 17, 349–359. doi: 10.1016/j.tplants.2012.03.001
Demianski, A. J., Chung, K. M., and Kunkel, B. N. (2011). Analysis of Arabidopsis JAZ gene expression during Pseudomonas syringae pathogenesis. Mol. Plant Pathol. 13, 46–57. doi: 10.1111/j.1364-3703.2011.00727.x
Dewanto, V., Wu, X. Z., Adom, K. K., and Liu, R. H. (2002). Thermal processing enhances the nutritional value of tomatoes by increasing total antioxidant activity. J. Agric. Food Chem. 50, 3010–3014. doi: 10.1021/jf0115589
Earley, K. W., Haag, J. R., Pontes, O., Opper, K., Juehne, T., Song, K., et al. (2010). Gateway-compatible vectors for plant functional genomics and proteomics. Plant J. 45, 616–629. doi: 10.1111/j.1365-313x.2005.02617.x
Farmer, E. E., Alméras, E., and Krishnamurthy, V. (2003). Jasmonates and related oxylipins in plant responses to pathogenesis and herbivory. Curr. Opin. Plant Biol. 6, 372–378. doi: 10.1016/S1369-5266(03)00045-1
Fernández-Calvo, P., Chini, A., Fernández-Barbero, G., Chico, J. M., Gimenez-Ibanez, S., Geerinck, J., et al. (2011). The Arabidopsis bHLH transcription factors MYC3 and MYC4 are targets of JAZ repressors and act additively with MYC2 in the activation of jasmonate responses. Plant Cell 23, 701–715. doi: 10.1105/tpc.110.080788
Fonseca, S., Fernández-Calvo, P., Fernández, G. M., Díez-Díaz, M., Gimenez-Ibanez, S., López-Vidriero, I., et al. (2014). bHLH003, bHLH013 and bHLH017 are new targets of JAZ repressors negatively regulating JA responses. PLoS One 9:e86182. doi: 10.1371/journal.pone.0086182
Garrido-Bigotes, A., Torrejón, M., Solano, R., and Figueroa, C. R. (2020). Interactions of JAZ repressors with anthocyanin biosynthesis-related transcription factors of Fragaria × ananassa. Agron. Basel 10:1586. doi: 10.3390/agronomy10101586
Garrido-Bigotes, A., Valenzuela-Riffo, F., and Figueroa, C. R. (2019). Evolutionary analysis of JAZ proteins in plants: an approach in search of the ancestral sequence. Intern. J. Mol. Sci. 20:5060. doi: 10.3390/ijms20205060
Ge, Q., Zhang, Y. C., Hua, W. P., Wu, Y. C., Jin, X. X., Song, S. H., et al. (2015). Combination of transcriptomic and metabolomic analyses reveals a JAZ repressor in the jasmonate signaling pathway of Salvia miltiorrhiza. Sci. Rep. 5, 2809–2823. doi: 10.1038/srep14048
Gietz, R. D., and Schiestl, R. H. (2007). High-efficiency yeast transformation using the LiAc/SS carrier DNA/PEG method. Nat. Protoc. 2, 31–34. doi: 10.1038/nprot.2007.15
Gu, X. C., Chen, J. F., Xiao, Y., Di, P., Xuan, H. J., Zhou, X., et al. (2012). Overexpression of allene oxide cyclase promoted tanshinone/phenolic acid production in Salvia miltiorrhiza. Plant Cell Rep. 31, 2247–2259. doi: 10.1007/s00299-012-1334-9
Kai, G. Y., Xu, H., Zhou, C. C., Liao, P., Xiao, J. B., Luo, X. Q., et al. (2011). Metabolic engineering tanshinone biosynthetic pathway in Salvia miltiorrhiza hairy root cultures. Metab. Eng. 13, 319–327. doi: 10.1016/j.ymben.2011.02.003
Li, S. S., Wu, Y. C., Kuang, J., Wang, H. Q., Du, T. Z., Huang, Y. Y., et al. (2018). SmMYB111 is a key factor to phenolic acid biosynthesis and interacts with both SmTTG1 and SmbHLH51 in Salvia miltiorrhiza. J. Agric. Food Chem. 66, 8069–8078. doi: 10.1021/acs.jafc.8b02548
Liechti, R., and Farmer, E. E. (2002). The jasmonate pathway. Science 296, 1649–1650. doi: 10.1126/science.1071547
Liu, A. H., Li, L., Xu, M., Lin, Y. H., Guo, H. Z., and Guo, D. A. (2006). Simultaneous quantification of six major phenolic acids in the roots of Salvia miltiorrhiza and four related traditional Chinese medicinal preparations by HPLC-DAD method. J. Pharm. Biomed. Analys. 41, 48–56. doi: 10.1016/j.jpba.2005.10.021
Liu, B. Y., Du, Y. H., Cong, L. X., Jia, X., and Yang, G. (2016). Danshen (Salvia miltiorrhiza) compounds improve the biochemical indices of the patients with coronary heart disease. Evid. Based Complement. Alternat. Med. 2016:9781715. doi: 10.1155/2016/9781715
Liu, Y. C., Ma, W., Niu, J. F., Li, B., Zhou, W., Liu, S., et al. (2020). Systematic analysis of SmWD40s, and responding of SmWD40-170 to drought stress by regulation of ABA- and H2O2- induced stomal movement in Salvia miltiorrhiza bunge. Plant Physiol. Biochem. 153, 131–140. doi: 10.1016/j.plaphy.2020.05.017
Luo, H. M., Zhu, Y. J., Song, J. Y., Xu, L. J., Sun, C., Zhang, X., et al. (2015). Transcriptional data mining of Salvia miltiorrhiza in response to methyl jasmonate to examine the mechanism of bioactive compound biosynthesis and regulation. Physiol. Plant. 152, 241–255. doi: 10.1111/ppl.12193
Ma, X. H., Ma, Y., Tang, J. F., He, Y. L., Liu, Y. C., Ma, X. J., et al. (2015). The biosynthetic pathways of tanshinones and phenolic acids in Salvia miltiorrhiza. Molecules 20, 16235–16254. doi: 10.3390/molecules200916235
Ma, Y. M., Yuan, L. C., Wu, B., Li, X. E., Chen, S. L., and Lu, S. F. (2012). Genome-wide identification and characterization of novel genes involved in terpenoid biosynthesis in Salvia miltiorrhiza. J. Exper. Bot. 63, 2809–2823. doi: 10.1093/jxb/err466
Matus, J. T., Poupin, M. J., Caón, P., Bordeu, E., Alcalde, J. A., and Arce-Johnson, P. (2010). Isolation of WDR and bHLH genes related to flavonoid synthesis in grapevine (Vitis vinifera L.). Plant Mol. Biol. 72, 607–620. doi: 10.1007/s11103-010-9597-4
Neer, E. J., Schmidt, C. J., Nambudripad, R., and Smith, T. F. (1994). The ancient regulatory-protein family of WD-repeat proteins. Nature 371, 297–300. doi: 10.1038/371812b0
Pan, M., Zhou, Y. Q., Wang, Y. F., Li, L. J., Song, Y. L., Hou, L., et al. (2017). Screening and identification of the host proteins interacting with Toxoplasma gondii rhoptry protein ROP16. Front. Microbiol. 8:2408. doi: 10.3389/fmicb.2017.02408
Pang, Y. Z., Wenger, J. P., Saathoff, K., Peel, G. J., Wen, J. Q., Huhman, D., et al. (2009). A WD40 repeat protein from Medicago truncatula is necessary for tissue-specific anthocyanin and proanthocyanidin biosynthesis but not for trichome development. Plant Physiol. 151, 1114–1129. doi: 10.1104/pp.109.144022
Pei, T. L., Ma, P. D., Ding, K., Liu, S. J., Jia, Y. Y., Ru, M., et al. (2018). SmJAZ8 acts as a core repressor regulating JA-induced biosynthesis of salvianolic acids and tanshinones in Salvia miltiorrhiza hairy roots. J. Exper. Bot. 69, 1663–1678. doi: 10.1093/jxb/erx484
Qi, T. C., Huang, H., Wu, D. W., Yan, J. B., Qi, Y. J., Song, S. S., et al. (2014). Arabidopsis DELLA and JAZ proteins bind the WD-repeat/bHLH/MYB complex to modulate gibberellin and jasmonate signaling synergy. Plant Cell 26, 1118–1133. doi: 10.1105/tpc.113.121731
Ramsay, N. A., and Glover, B. J. (2005). MYB-bHLH-WD40 protein complex and the evolution of cellular diversity. Trends Plant Sci. 10, 63–70. doi: 10.1016/j.tplants.2004.12.011
Saha, G., Park, J., Kayum, M., and Nou, S. (2016). A genome-wide analysis reveals stress and hormone responsive patterns of TIFY family genes in Brassica rapa. Front. Plant Sci. 7:936. doi: 10.3389/fpls.2016.00936
Sen, S., Kundu, S., and Dutta, S. (2016). Proteomic analysis of JAZ interacting proteins under methyl jasmonate treatment in finger millet. Plant Physiol. Biochem. 108, 79–89. doi: 10.1016/j.plaphy.2016.05.033
Shi, M., Liao, P., Nile, S. H., Georgiev, M. I., and Kai, G. Y. (2020). Biotechnological exploration of transformed root culture for value-added products. Trends Biotechnol. 39, 137–149.
Shi, M., Zhou, W., Zhang, J. L., Huang, S. X., Wang, H. Z., and Kai, G. Y. (2016). Methyl jasmonate induction of tanshinone biosynthesis in Salvia miltiorrhiza hairy roots is mediated by JASMONATE ZIM-DOMAIN repressor proteins. Sci. Rep. 6:20919. doi: 10.1038/srep20919
Smith, T. F., Gaitatzes, C., Saxena, K., and Neer, E. J. (1999). The WD repeat: a common architecture for diverse functions. Trends Biochem. Sci. 24, 181–185. doi: 10.1016/S0968-0004(99)01384-5
Song, S. S., Qi, T. C., Fan, M., Zhang, X., Gao, H., Huang, H., et al. (2013). The bHLH subgroup IIId factors negatively regulate jasmonate-mediated plant defense and development. PLoS Genet. 9:e1003653. doi: 10.1371/journal.pgen.1003653
Song, S. S., Qi, T. C., Huang, H., Ren, Q. C., Wu, D. W., Chang, C. Q., et al. (2011). The jasmonate-ZIM domain proteins interact with the R2R3-MYB transcription factors MYB21 and MYB24 to affect jasmonate-regulated stamen development in Arabidopsis. Plant Cell 23, 1000–1013. doi: 10.1105/tpc.111.083089
Staswick, P. E. (2008). JAZing up jasmonate signaling. Trends Plant Sci. 13, 66–71. doi: 10.1016/j.tplants.2007.11.011
Su, C. Y., Ming, Q. L., Rahman, K., Han, T., and Qin, L. P. (2015). Salvia miltiorrhiza: traditional medicinal uses, chemistry, and pharmacology. Chin. J. Nat. Med. 13, 163–182. doi: 10.1016/S1875-5364(15)30002-9
Szemenyei, H., Hannon, M., and Long, J. A. (2008). TOPLESS mediates auxin-dependent transcriptional repression during Arabidopsis embryogenesis. Science 319, 1384–1386. doi: 10.1126/science.1151461
Thines, B., Katsir, L., Melotto, M., Niu, Y. J., Mandaokar, A., Liu, G. H., et al. (2007). JAZ repressor proteins are targets of the SCF(COI1) complex during jasmonate signalling. Nature 448, 661–665. doi: 10.1038/nature05960
Vandesompele, J., De Preter, K., Pattyn, F., Poppe, B., Van Roy, N., De Paepe, A., et al. (2002). Accurate normalization of real-time quantitative RT-PCR data by geometric averaging of multiple internal control genes. Genome Biol. 3, 467–470. doi: 10.1186/gb-2002-3-7-research0034
Vanholme, B., Grunewald, W., Bateman, A., Kohchi, T., and Gheysen, G. (2007). The tify family previously known as ZIM. Trends Plant Sci. 12, 239–244. doi: 10.1016/j.tplants.2007.04.004
Walker, A. R., Davison, P. A., Bolognesi-Winfield, A. C., James, C. M., Srinivasan, N., Blundell, T. L., et al. (1999). The TRANSPARENT TESTA GLABRA1 locus, which regulates trichome differentiation and anthocyanin biosynthesis in Arabidopsis, encodes a WD40 repeat protein. Plant Cell 11, 1337–1349. doi: 10.2307/3870753
Wang, B., Niu, J. F., Li, B., Huang, Y. Y., Han, L. M., Liu, Y. C., et al. (2018). Molecular characterization and overexpression of SmJMT increases the production of phenolic acids in Salvia miltiorrhiza. Intern. J. Mol. Sci. 19:3788. doi: 10.3390/ijms19123788
Wang, H., Yan, Z. Y., Shen, Y. X., He, D. M., Lan, Y., and Wan, D. G. (2014). Correlation of color feature with effective constituents of radix Salvia Miltiorrhiza. Tradit. Chin. Drug Res. Clin. Pharmacol. 3, 333–338.
Wasternack, C., and Hause, B. (2013). Jasmonates: biosynthesis, perception, signal transduction and action in plant stress response, growth and development. an update to the 2007 review in annals of botany. Ann. Bot. 111, 1021–1058. doi: 10.1093/aob/mct067
Withers, J., Yao, J., Mecey, C., Howe, G. A., Melotto, M., and He, S. Y. (2012). Transcription factor-dependent nuclear localization of a transcriptional repressor in jasmonate hormone signaling. Proc. Natl. Acad. Sci. U.S.A. 109, 20148–20153. doi: 10.1073/pnas.1210054109
Xiao, Y., Gao, S. H., Di, P., Chen, J. F., Chen, W. S., and Zhang, L. (2010). Methyl jasmonate dramatically enhances the accumulation of phenolic acids in Salvia miltiorrhiza hairy root cultures. Physiol. Plant. 137, 1–9. doi: 10.1111/j.1399-3054.2009.01257.x
Xie, D., Feys, B. F., James, S., Nieto-Rostro, M., and Turner, J. G. (1998). COI1: an Arabidopsis gene required for jasmonate-regulated defense and fertility. Science 280, 1091–1094. doi: 10.1126/science.280.5366.1091
Xu, H. B., Song, J. Y., Luo, H. M., Zhang, Y. J., Li, Q. S., Zhu, Y. J., et al. (2016). Analysis of the genome sequence of the medicinal plant Salvia miltiorrhiza. Mol. Plant 9, 949–952. doi: 10.1016/j.molp.2016.03.010
Xu, L., Liu, F., Lechner, E., Genschik, P., Crosby, W. L., Ma, H., et al. (2002). The SCF(COI1) ubiquitin-ligase complexes are required for jasmonate response in Arabidopsis. Plant Cell 14, 1919–1935. doi: 10.1105/tpc.003368
Xu, Z. C., Peters, R. J., Weirather, J., Luo, H. M., Liao, B. S., Zhang, X., et al. (2015). Full-length transcriptome sequences and splice variants obtained by a combination of sequencing platforms applied to different root tissues of Salvia miltiorrhiza and tanshinone biosynthesis. Plant J. 82, 951–961. doi: 10.1111/tpj.12865
Yan, Y., Stolz, S., Chetelat, A., Reymond, P., Pagni, M., Dubugnon, L., et al. (2007). A downstream mediator in the growth repression limb of the jasmonate pathway. Plant Cell 19, 2470–2483. doi: 10.1105/tpc.107.050708
Yan, Y. P., and Wang, Z. Z. (2007). Genetic transformation of the medicinal plant Salvia miltiorrhiza by Agrobacterium tumefaciens-mediated method. Plant Cell Tissue Organ. Culture 88, 175–184. doi: 10.1007/s11240-006-9187-y
Yang, N., Zhou, W. P., Su, J., Wang, X. F., Li, L., Wang, L. R., et al. (2017). Overexpression of SmMYC2 increases the production of phenolic acids in Salvia miltiorrhiza. Front. Plant Sci. 8:1804. doi: 10.3389/fpls.2017.01804
Ye, H. Y., Du, H., Tang, N., Li, X. H., and Xiong, L. Z. (2009). Identification and expression profiling analysis of TIFY family genes involved in stress and phytohormone responses in rice. Plant Mol. Biol. 71, 291–305. doi: 10.1007/s11103-009-9524-8
Yu, J., Zhang, Y. X., Di, C., Zhang, Q. L., Zhang, K., Wang, C. C., et al. (2016). JAZ7 negatively regulates dark-induced leaf senescence in Arabidopsis. J. Exper. Bot. 67, 751–762. doi: 10.1093/jxb/erv487
Zhang, L. H., You, J., and Chan, Z. L. (2015). Identification and characterization of TIFY family genes in Brachypodium distachyon. J. Plant Res. 128, 995–1005. doi: 10.1007/s10265-015-0755-2
Zhang, Y. C., Gao, M., Singer, S. D., Fei, Z. J., Wang, H., Wang, X. P., et al. (2012). Genome-wide identification and analysis of the TIFY gene family in grape. PLoS One 7:e44465. doi: 10.1371/journal.pone.0044465
Zhao, J. L., Zhou, L. G., and Wu, J. Y. (2010). Effects of biotic and abiotic elicitors on cell growth and tanshinone accumulation in Salvia miltiorrhiza cell cultures. Appl. Microbiol. Biotechnol. 87, 137–144. doi: 10.1007/s00253-010-2443-4
Zhou, Y. Y., Sun, W., Chen, J. F., Tan, H. X., Xiao, Y., Li, Q., et al. (2016). SmMYC2a and SmMYC2b played similar but irreplaceable roles in regulating the biosynthesis of tanshinones and phenolic acids in Salvia miltiorrhiza. Sci. Rep. 6, 1345–1359. doi: 10.1038/srep22852
Keywords: Salvia miltiorrhiza, jasmonate, TIFY proteins, SmJAZ3, SmWD40-170
Citation: Li L, Liu Y, Huang Y, Li B, Ma W, Wang D, Cao X and Wang Z (2021) Genome-Wide Identification of the TIFY Family in Salvia miltiorrhiza Reveals That SmJAZ3 Interacts With SmWD40-170, a Relevant Protein That Modulates Secondary Metabolism and Development. Front. Plant Sci. 12:630424. doi: 10.3389/fpls.2021.630424
Received: 17 November 2020; Accepted: 26 January 2021;
Published: 18 February 2021.
Edited by:
Jakob Franke, Leibniz University Hannover, GermanyReviewed by:
Aijia Ji, Guangzhou University of Chinese Medicine, ChinaZhichao Xu, Chinese Academy of Medical Sciences and Peking Union Medical College, China
Carlos R. Figueroa, University of Talca, Chile
Copyright © 2021 Li, Liu, Huang, Li, Ma, Wang, Cao and Wang. This is an open-access article distributed under the terms of the Creative Commons Attribution License (CC BY). The use, distribution or reproduction in other forums is permitted, provided the original author(s) and the copyright owner(s) are credited and that the original publication in this journal is cited, in accordance with accepted academic practice. No use, distribution or reproduction is permitted which does not comply with these terms.
*Correspondence: Xiaoyan Cao, Y2FveGlhb3lhbkBzbm51LmVkdS5jbg==; Zhezhi Wang, enp3YW5nQHNubnUuZWR1LmNu
†These authors have contributed equally to this work