- 1Rice Research Institute, Sichuan Agricultural University, Chengdu, China
- 2College of Agriculture, Forestry and Health, The Open University of Sichuan, Chengdu, China
- 3Liaoning Rice Research Institute, Liaoning Academy of Agricultural Sciences, Shenyang, China
- 4State Key Laboratory of Crop Gene Exploration and Utilization in Southwest China, Sichuan Agricultural University, Chengdu, China
Rice grain yield consists of several key components, including tiller number, grain number per panicle (GNP), and grain weight. Among them, GNP is mainly determined by panicle branches and spikelet formation. In this study, we identified a gene affecting GNP and grain yield, OsSPL9, which encodes SQUAMOSA-PROMOTER BINDING PROTEIN-LIKE (SPL) family proteins. The mutation of OsSPL9 significantly reduced secondary branches and GNP. OsSPL9 was highly expressed in the early developing young panicles, consistent with its function of regulating panicle development. By combining expression analysis and dual-luciferase assays, we further confirmed that OsSPL9 directly activates the expression of RCN1 (rice TERMINAL FLOWER 1/CENTRORADIALIS homolog) in the early developing young panicle to regulate the panicle branches and GNP. Haplotype analysis showed that Hap3 and Hap4 of OsSPL9 might be favorable haplotypes contributing to high GNP in rice. These results provide new insights on high grain number breeding in rice.
Introduction
Rice is a staple food and provides energy for more than half of the population in the world. Grain number per panicle (GNP) is an important factor determining grain yield and is associated with panicle branches and spikelet formation in rice. To date, several genes for GNP have been cloned in rice. IPA1/OsSPL14 (Ideal Plant Architecture 1) and DEP1 (DENSE AND ERECT PANICLE 1) are crucial regulators of inflorescence branches (Huang et al., 2009; Jiao et al., 2010; Miura et al., 2010). Gn1a (Grain number 1a), encoding a cytokinin oxidase, negatively regulates cytokinin accumulation in inflorescence meristems and the number of reproductive organs (Ashikari et al., 2005). TAWAWA1 encodes a nuclear protein that regulates inflorescence development by promoting inflorescence meristem activity and suppressing the phase change to spikelet meristem identity (Yoshida et al., 2013). Lax panicle genes, LAX1 and LAX2, are involved in the process of axillary meristems formation (Komatsu et al., 2003; Tabuchi et al., 2011). Rice TERMINAL FLOWER 1/CENTRORADIALIS homologs, RCN1 and RCN2, play important roles in controlling the timing of phase transition. Overexpression of these two genes delays transition from the branch shoot to the floral meristem state and causes the formation of higher-order branches (Nakagawa et al., 2002). FZP (FRIZZLE PANICLE) controls the transition from panicle branches to spikelet formation by negatively regulating APO2 (RFL/ABERRANT PANICLE ORGANIZATION 2) and positively regulating the related OsMADS-box genes (Bai et al., 2016). FON4 (Floral organ number 4) genetically interacts with floral homeotic genes and is responsible for regulating meristem size and determinacy of flora. Loss of function of FON4 caused multi-floret spikelets in rice (Xu et al., 2017; Ren et al., 2019). MULTI-FLORET SPIKELET1(MFS1) and MFS2 are involved in determining the fate of spikelet meristem in rice (Ren et al., 2013; Li et al., 2020). These studies showed that the genetic and molecular mechanisms underlying the panicle branches and spikelet formation are involved in a complex regulatory network. Hence, identification and characterization of diverse mutants related to grain numbers are necessary for further understanding of this process in rice.
SQUAMOSA-PROMOTER BINDING PROTEIN-LIKE (SPL) family proteins are plant-specific transcription factors with a conserved SBP domain consisting of 76–80 amino acid residues (Birkenbihl et al., 2005). There are 19 putative SPL genes in rice (Xie et al., 2006). To date, 14 genes have been identified to be involved in different regulatory pathways. For example, several SPL genes, such as OsSPL2, OsSPL4, OsSPL7, OsSPL13, OsSPL14, OsSPL16, OsSPL17, and OsSPL18, directly regulate yield-related traits, including tiller, panicle branches, grain size, and grain shape (Jiao et al., 2010; Luo et al., 2012; Wang et al., 2012, 2015; Si et al., 2016; Yue et al., 2017; Zhang et al., 2017; Dai et al., 2018; Yuan et al., 2019; Hu et al., 2020). In addition, OsSPL3 and OsSPL12 regulate crown root development in rice (Shao et al., 2019). Seed-specific overexpression of OsSPL12 enhances seed dormancy and inhibits pre-harvest sprouting (Qin et al., 2020). OsSPL8 is involved in the development of ligule, auricle, and panicle branch angle (Lee et al., 2007). OsSPL10 negatively controls salt tolerance but positively controls trichome formation in rice (Lan et al., 2019). OsSPL6 controls panicle cell death by repressing the transcriptional activation of the ER stress sensor, IRE1 (Wang et al., 2018). Overexpression of OsSPL9 caused Cu accumulation in the shoot of rice seedlings and in the grain after maturation (Tang et al., 2016); moreover, OsSPL9 directly binds the miR528 promoter to regulate antiviral defense and promote flowering under long-day conditions (Yang et al., 2019; Yao et al., 2019). However, it is unclear whether OsSPL9 is involved in the regulation of yield-related traits in rice.
In this study, we identified a less grain number 5 (lgn5) mutant, through MutMap analysis and a transgenic experiment, and confirmed that the lgn5 phenotype was controlled by an SPL family transcription factor, OsSPL9. Furthermore, RCN1, a positive regulator of panicle branches and GNP (Nakagawa et al., 2002; Wang et al., 2015) was identified as a plausible downstream target of OsSPL9. The results will enrich the genetic network regulating grain number and the available genetic resources for breeding improvement of grain number.
Materials and Methods
Plant Materials and Growth Conditions
The lgn5 mutant was identified from an ethyl methanesulfonate (EMS) mutant library of indica rice variety, Shuhui498 (R498). A segregation population derived from the cross between lgn5 and R498 was used for genetic analysis and gene mapping. All plants including the transgenic lines were grown in an experimental field plot of Sichuan Agricultural University (Chengdu, China) during the normal growing seasons.
Microscopic Observation
Tissues were collected and immediately fixed in a 2.5% (v/v) glutaraldehyde solution overnight and then dehydrated in an alcohol gradient. Scanning electron microscopy (SEM) observations were processed using a JSM-7500F field emission SEM (JEOL, Japan), as previously described in the study by Li et al. (2014).
Gene Mapping
MutMap was used for gene mapping (Abe et al., 2012). Briefly, plants with the lgn5 phenotype were selected from the F2 population of the cross between lgn5 and R498, which were identified as recessive individuals. The DNA of 25 F2 plants with lgn5 was extracted and mixed in an equal proportion, and the mixed DNA was subjected to whole-genome sequencing. The DNA of R498 was re-sequenced as a control. Then, these short reads obtained from mutant-type plants and R498 were aligned to the reference genome sequence (Nipponbare).
CRISPR/Cas9 Vector Construction and Rice Transformation
To knock out OsSPL9, we designed the target site at the third exon of OsSPL9 (Xie et al., 2017), and the target sequence was cloned into a sgRNA expression cassette driven by the OsU6a promoter. The sgRNA cassette was recombined into the pYLCRISPR/Cas9Pubi-H vector as previously described by Shan et al. (2013). The final CRISPR/Cas9 construct was introduced into japonica variety, Zhonghua11 (ZH11), by Agrobacterium tumefaciens-mediated transformation (Jeon et al., 2000). For mutation detection, DNA of T0 transgenic plants was extracted and the target region was amplified by PCR for sequencing. The primers for vector construction and detection are listed in Supplementary Table 1.
RNA Extraction and Reverse Transcription Quantitative PCR Analysis
Total RNA was extracted from different rice tissues (root, stem, leaf blade, leaf sheath, young panicle, hull, and endosperm) using plant RNA Kit I (OMEGA Bio-Tek, Norcross, United States). cDNA was synthesized from 500 ng of total RNA using a reverse transcription kit (TaKaRa, Dalian, China). RT-qPCR analysis was performed using the SYBR Green Real-Time PCR Mix (KAPA, Boston, United States) on a CFX96™ Real-Time PCR system (Bio-Rad, CA, United States). The ACTIN gene (LOC_Os03g50885) was used as an internal control. The primer sequences used for RT-qPCR analysis are listed in Supplementary Table 1.
GUS Staining
A 2.5 Kb region upstream of OsSPL9 was amplified and cloned into the vector DX2181 to generate the proOsSPL9::GUS construct. The construct was transformed into ZH11 by A. tumefaciens-mediated transformation (Jeon et al., 2000). Various rice tissues were collected and submerged into the GUS staining solution [50 mM Na3PO4, pH 7.2, 1 mM K4Fe(CN)6, 1 mM K3Fe(CN)6, 1 mM 5-bromo-4-chloro-3-indolyl-β-d-glucuronic acid, and 0.1% (v/v) Triton X-100] and then incubated at 37°C for 12–24 h. The stained tissues were transferred to 70% (v/v) ethanol for decolorization. Photographs were taken using a a MICROTEK ScanMaker i800 plus scanner (Shanghai, China).
Dual-Luciferase Assay
The transcriptional activation of RCN1 by OsSPL9 was performed using the dual-luciferase assay. The full-length CDS of OsSPL9 was amplified and inserted into the pGreen II 62-SK driven by a CaMV 35S promoter for the effector construct. A fragment size of 2,000 bp of RCN1 promoter was inserted into the pGreen II 0800-LUC (LUC) vector to generate the reporter construct. Renilla luciferase (REN) driven by the CaMV 35S promoter in the same construct was used as an internal control. The effector and reporter constructs were transiently co-expressed in rice protoplasts. LUC and REN activities were measured using a Dual-Luciferase Reporter Assay System (Promega, United States) according to the instructions of the manufacturer. The primer sequences used for vector construction are listed in Supplementary Table 1.
Haplotype Analysis
The single nucleotide polymorphism (SNP) and insertion/deletion (InDel) information of the OsSPL9 genome in rice germplasms and the grain number of Hap1–Hap4 haplotypes were obtained from RiceVarMap v2.0.1 Haplotype analysis was performed based on the non-synonymous variations.
Results
Characterization of the lgn5 Mutant
The lgn5 mutant was isolated from an EMS mutant library of indica rice variety, Shuhui498 (R498), which is an excellent restorer of hybrid rice. Compared with R498, the plant height of lgn5 was reduced by 7.6% (Figures 1A,I), but there was no significant difference in the tiller number and panicle length of lgn5 (Figures 1B,J,K). Similarly, there was no significant difference in the number of primary branches; however, the number of secondary branches was significantly reduced by 41.7%, leading to a 54.7% reduction in GNP (Figures 1C,D,L–N). The grain length had no significant change, but the grain width decreased by 4.7%, leading to a 5.8% reduction in 1,000-grain weight (Figures 1O–Q). Finally, the grain yield per plant was reduced by 59% (Figure 1R).
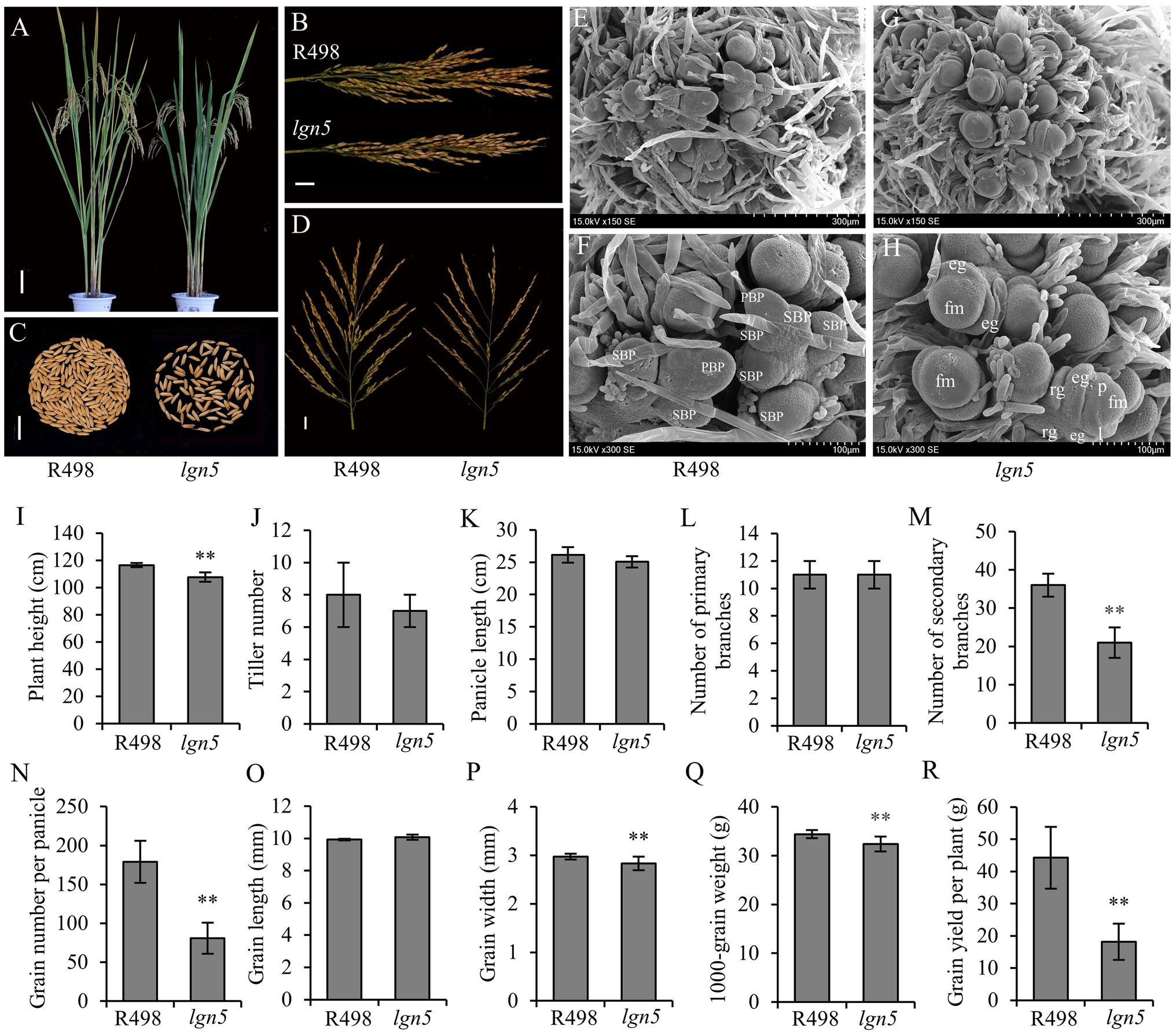
Figure 1. Phenotype of less grain number 5 (lgn5) mutant. (A) Plant morphology of R498 and lgn5 during grain filling. Scale bar: 10 cm. (B) Panicles of R498 and lgn5. Scale bar: 2 cm. (C) Comparison of grain number per panicle (GNP) between R498 and lgn5. Scale bar: 2 cm. (D) Panicle morphology of R498 and lgn5. Scale bar: 2 cm. (E–H) Scanning electron microscopy (SEM) observation of the young panicle in the early developmental stage between R498 and lgn5. (E,G) Young panicle of 0.5–1 mm. (F,H) Enlarged views of (E,G), respectively. PBP, primary branch primordia; SBP, secondary branch primordia; fm, floral meristem; p, palea; l, lemma; eg, empty glume; rg, rudimentary glume. Scale bar: 300 μm (E,G) and 100 μm (F,H). (I–R) Statistical analysis of major agronomic traits in R498 and lgn5. Data are given as means ± SD of five biological replicates. ** indicates p < 0.01 by Student’s t-test.
To understand the cytological mechanism of panicle development in lgn5, we performed SEM observation. When the length of young panicles was 0.1–0.5 mm, R498 plants had a large number of secondary branch primordia, while lgn5 plants were already in the stage of spikelet primordia differentiation (Figures 1E–H). This result suggested that the panicle development of lgn5 was earlier than that of R498. Taken together, these results indicated that the decrease of secondary branches in lgn5 might be caused by the shortened differentiation period of secondary branch primordia and the early formation of spikelets.
OsSPL9 Is Responsible for the lgn5 Phenotype
To identify the gene responsible for the lgn5 phenotype, lgn5 was backcrossed with the parental line R498. Approximately, one-quarter of the F2 plants (χ2c = 0.260 < χ20.05,1 = 3.84) had fewer grains (Supplementary Figure S1A), indicating that a single recessive allele was responsible for the lgn5 phenotype. Then, we performed gene mapping using the MutMap strategy and identified a linkage region on chromosome 5 (Supplementary Figures S1B,C). Three linked SNPs with the SNP index of 1 on chromosome 5 were identified in LOC_Os05g31480, LOC_Os05g31830, and LOC_Os05g33810. Among these SNPs, SNP1 in LOC_Os05g31480 was located in an intron; SNP2 in LOC_Os05g31830 led to a synonymous mutation; and SNP3 in LOC_Os05g33810 caused a non-synonymous mutation (Glu561Lys) in an exon (Supplementary Figure S1D). Therefore, LOC_Os05g33810, which encodes the SPL family transcription factor, OsSPL9, was the likely candidate gene for the lgn5 phenotype. Phylogenetic analysis of OsSPL9 in different plant species revealed that OsSPL9 was conserved in Poacea (Supplementary Figure S2). In addition, amino acid substitution in the lgn5 mutant was highly conserved among different plant species (Supplementary Figure S3), indicating that this amino acid may be an essential component for OsSPL9 function.
To further confirm that the lgn5 phenotype was caused by the mutation of OsSPL9, we obtained knockout (KO) mutant plants of OsSPL9 in the Zhonghua11 (ZH11) background using the CRISPR/Cas9 genome editing system (Shan et al., 2013). Two independent homozygous KO mutants with different mutation types were generated, and both mutations caused premature stop codons (Supplementary Figure S4). Compared with wild-type ZH11 plants, the plant height and the panicle length of KO mutants were significantly decreased (Figures 2A,C,F,H). The number of primary branches did not differ, but the number of secondary branches reduced by 51.2% on average, and the GNP decreased by 41.7% (Figures 2B,D,I–K). The grain length was not significantly different, but the grain width and 1,000-grain weight were reduced by 6.8 and 10.6% on average, respectively (Figures 2L–N). The reduction in both grain number and grain weight caused a 47% reduction in grain yield per plant in knockout mutants of OsSPL9 (Figure 2O). Taken together, knockout mutants of OsSPL9 showed similar phenotype variations as the lgn5 mutant, indicating that the SNP3 in OsSPL9 is responsible for the lgn5 mutant phenotype.
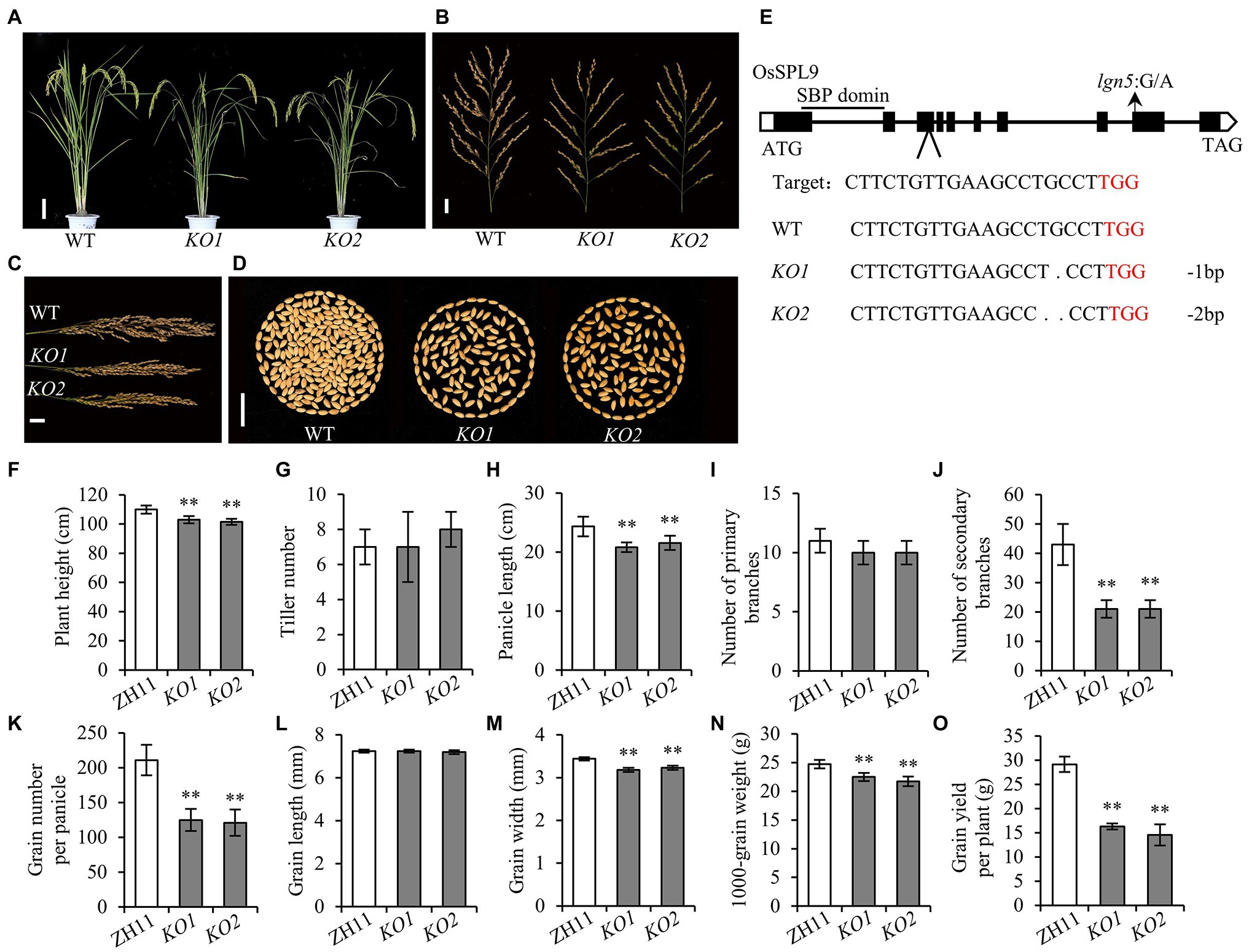
Figure 2. Phenotypic comparison of wild type and CRISPR mutants. (A) Plant morphology of wild type and CRISPR mutants. Scale bar: 10 cm. (B) Panicle morphology of wild type and CRISPR mutants. Scale bar: 2 cm. (C) Panicles of wild type and CRISPR mutants. Scale bar: 2 cm. (D) Comparison of GNP between wild type and CRISPR mutants. Scale bar: 2 cm. (E) Schematic diagram of the target site in OsSPL9 and the target sequence alignment of wild type and CRISPR mutants. The number of altered bases is indicated on the right; “−” indicates deleted bases. ATG, start codon; TAG, stop codon; the arrow shows the mutant site in lgn5. (F–O) Agronomic traits in wild type and CRISPR mutants. Data are given as means ± SD of five biological replicates. ** indicates p < 0.01 by the Student’s t-test.
Expression Pattern of OsSPL9
We analyzed the spatial expression of OsSPL9 by RT-qPCR. OsSPL9 was expressed in all of the investigated organs and was highly expressed in leaf blades and developing young panicles (Figure 3A). GUS staining was further performed in the ProOsSPL9::GUS transgenic plants. GUS activity was detected predominantly in the root, leaf sheath, stem, and developing young panicles (Figure 3B). These results indicate that OsSPL9 is constitutively expressed, and the high expression level in young panicles supported its role in the regulation of panicle development and grain number.
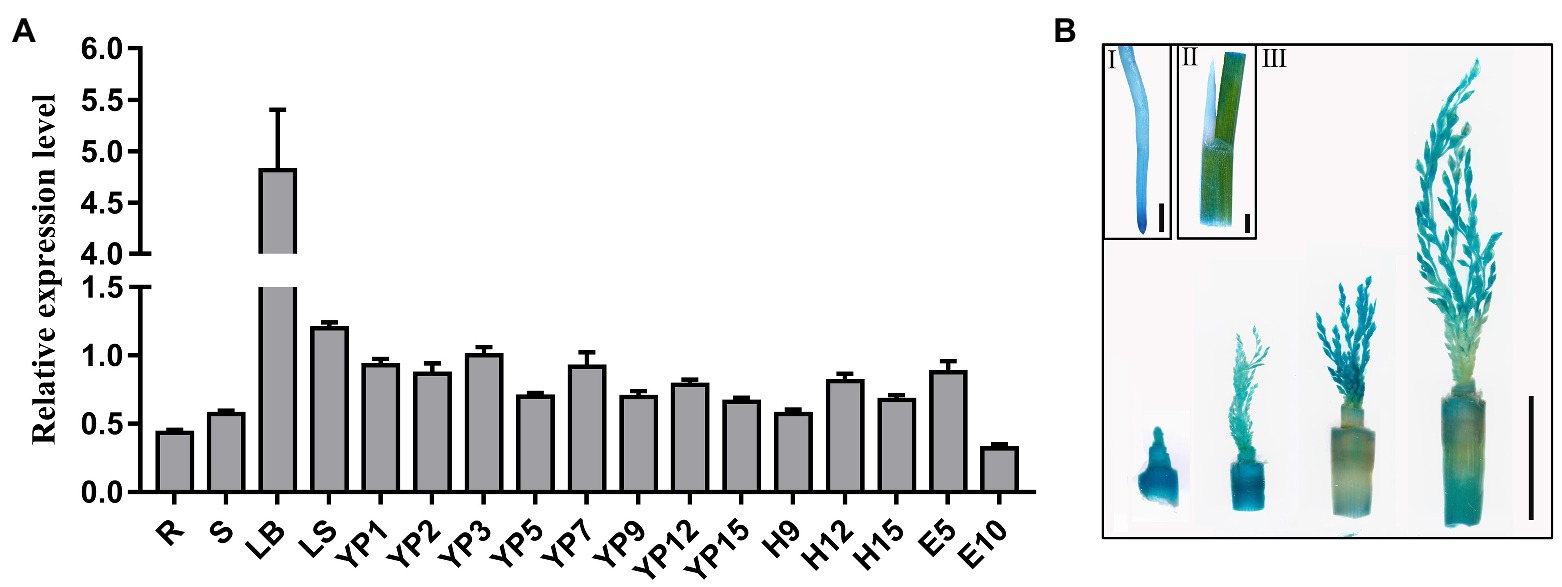
Figure 3. Expression pattern of OsSPL9. (A) Transcript levels of OsSPL9 in various organs by RT-qPCR. R, young root; S, stem; LB, leaf blade in the tillering stage; LS, leaf sheath; YP1–YP15, young panicles of different lengths (1–15 cm). H9–H15, hulls from panicles with different lengths (9–15 cm). E5–E10, endosperm at 5 and 10 days after fertilization. Data are given as means ± SD of three biological replicates. (B) GUS staining in the ProOsSPL9::GUS transgenic plants. Root (I); leaf sheath (II); and early developing young panicle and stem (III). Scale bars: 2 mm (I,II) and 1 cm (III).
OsSPL9 Positively Regulates the Expression of RCN1
As the mutants of OsSPL9 (lgn5 and KO lines) showed a significant decrease in grain number, we detected the expression level of the genes related to grain number in the early developing young panicles of the KO1 line. The results showed that the expression level of most tested genes (including DST, FZP, OsGRF6, GNP1, Gn1a, DEP1, DEP2, DEP3, OsCLV1, LAX1, and LAX2) had no significant difference between wild type and KO1; however, RCN1, a positive regulator of panicle branches in rice (Nakagawa et al., 2002; Wang et al., 2015), was significantly downregulated in both KO and lgn5 mutants (Figure 4A; Supplementary Figure S5). Overexpression of RCN1 promotes the production of higher-order branches and spikelets (Nakagawa et al., 2002; Wang et al., 2015); hence, OsSPL9 might regulate panicle branches and grain number through RCN1. Consistent with this hypothesis, sequence analysis showed five GATC motifs in the promoter region (from −2000 to −1 bp) of RCN1 (Supplementary Figure S6), which was the recognition sequence of SPL protein for DNA binding (Birkenbihl et al., 2005). Therefore, we performed dual-luciferase assays in rice protoplasts to test whether OsSPL9 could directly bind to the promoter of RCN1. The results showed that OsSPL9 significantly enhanced the expression of the LUC reporter gene (Figure 4B). Thus, the results indicated that OsSPL9 regulates panicle branches and grain numbers by upregulating RCN1 expression in rice.
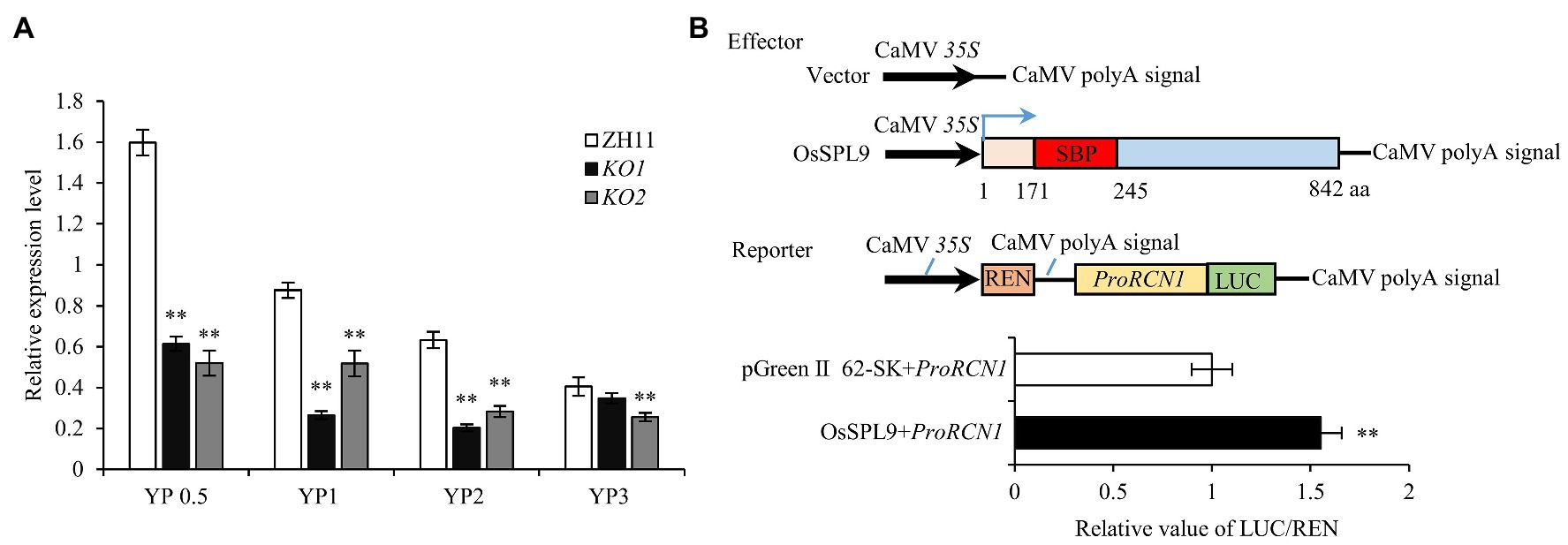
Figure 4. OsSPL9 activates the transcription of rice TERMINAL FLOWER 1/CENTRORADIALIS homolog (RCN1). (A) The transcriptional levels of RCN1 were downregulated in young panicles in KO mutants. YP0.5, YP1, YP2, and YP3, young panicles of 0.5, 1, 2, and 3 cm in length, respectively. Data are given as means ± SD of three biological replicates. ** indicates p < 0.01 by Student’s t-test. (B) Luciferase transient transcriptional activity assay in rice protoplasts to show that OsSPL9 activates RCN1 promoter. The pGreen II 0800-LUC/Renilla luciferase (LUC/REN) ratio of empty vector and proRCN1::LUC was normalized to 1. Data are given as means ± SD of three biological replicates. ** indicates p < 0.01 by Student’s t-test.
Haplotype Analysis of OsSPL9
We analyzed the genome variation of OsSPL9 in 4,726 rice accessions using Rice Variation Map v2.0, (see footnote 1) and 12 polymorphisms were detected in the exons of OsSPL9; however, only seven polymorphisms were non-synonymous (Supplementary Table 2). We used these seven non-synonymous polymorphisms for haplotype analysis in 375 cultivars with different GNP and found that five of the polymorphisms (sites in 395, 598, 2,855, 7,214, and 8,616) were identical in these cultivars, and four haplotypes (Hap1–Hap4) were identified based on two polymorphisms (sites in 11 and 8,502; Figure 5A). Phylogenetic analysis showed that the four haplotypes were divided into two clades: the clade I containing Hap1 and Hap3 was mainly found in japonica cultivars, and the clade II containing Hap2 and Hap4 was mainly present in indica cultivars (Figures 5A,B), indicating the divergence of OsSPL9 between japonica and indica cultivars. Furthermore, we compared the GNP between these haplotypes and found that Hap3 and Hap4 had the highest GNP, and Hap1 had the lowest GNP (Figures 5B,C). To exclude the influence of the genetic background of each haplotype, we also compared the GNP of different haplotypes in the same subspecies. For example, in the same japonica background, Hap3 had 50.5% higher GNP than Hap1 on average; and in the same indica background, Hap4 had 34.4% higher GNP than Hap2 on average (Figure 5D). Taken together, these results indicated that Hap3 and Hap4 of OsSPL9 might be favorable haplotypes.
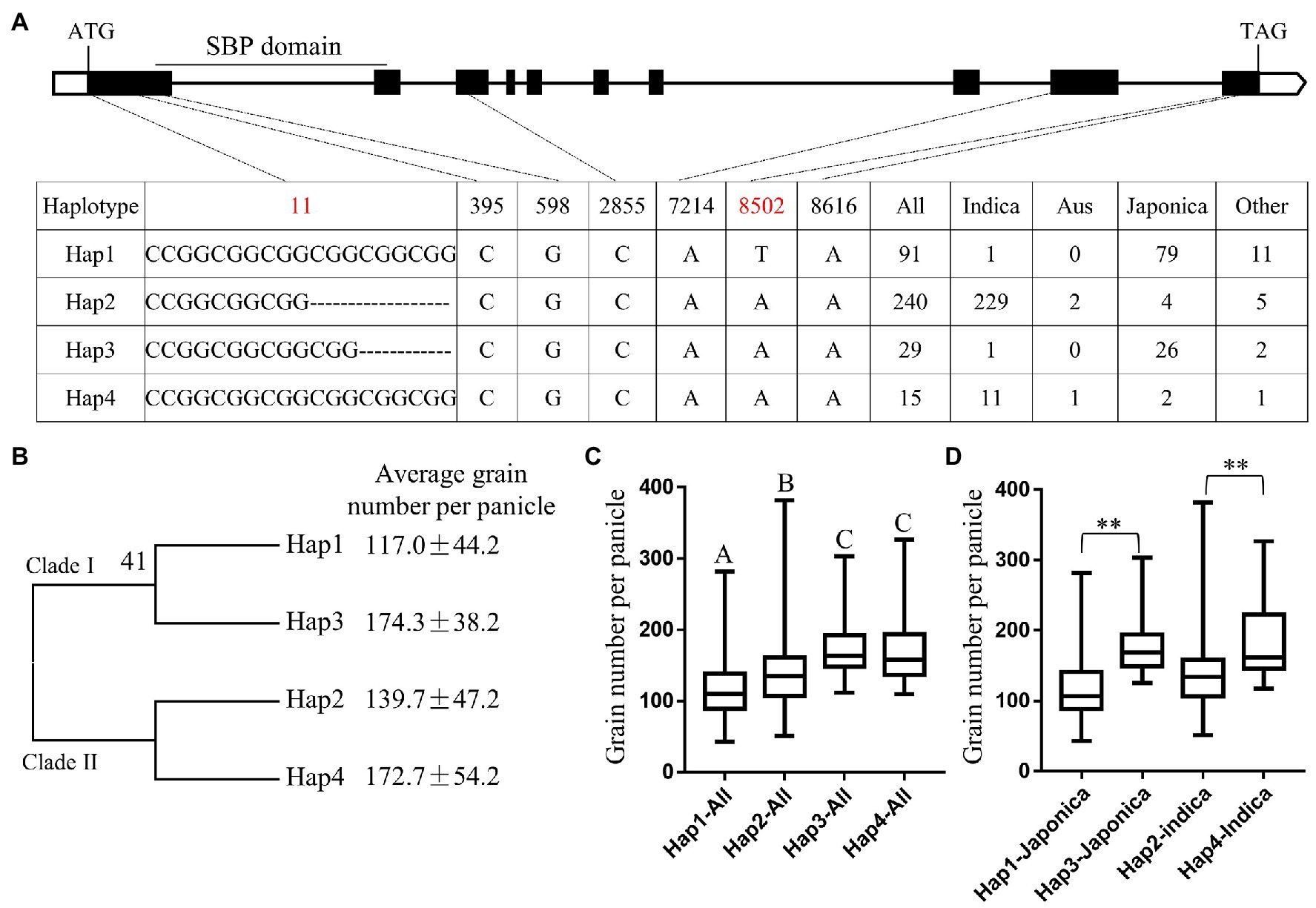
Figure 5. Haplotype analysis of OsSPL9 in rice accessions. (A) Single nucleotide polymorphism (SNP) divergence in the coding region of OsSPL9. (B) Phylogenetic analysis of OsSPL9 coding region using the neighbor-joining method. (C) The average GNP of OsSPL9 haplotypes in cultivars. Data are means ± SD. n = 91, 240, 29, and 15. The letters above the error bars indicate significant differences at p < 0.01 using multiple comparisons. (D) Comparison of the average GNP of OsSPL9 haplotypes in the same subspecies. Data indicate means ± SD. n = 79, 26, 229, and 11. Significant differences are determined using Student’s t-test. ** indicates p < 0.01.
Discussion
OsSPL9 Plays an Important Role in the Regulation of Grain Number
There are 19 putative SPL genes in rice (Xie et al., 2006), and 14 genes have been identified to be involved in various biological processes during rice growth and development, such as regulating tiller, panicle branches, grain size, the development of crown root, ligule, auricle and trichome, and stress response (Lee et al., 2007; Jiao et al., 2010; Luo et al., 2012; Wang et al., 2012, 2015; Si et al., 2016; Yue et al., 2017; Zhang et al., 2017; Dai et al., 2018; Lan et al., 2019; Shao et al., 2019; Yuan et al., 2019; Hu et al., 2020). OsSPL9 was proven to regulate Cu uptake and distribution by directly activating the expression of Cu transporter genes in rice (Tang et al., 2016), and an OsSPL9-miR528 pathway was involved in the regulation of plant resistance to rice stripe virus and rice flowering under long-day conditions (Yang et al., 2019; Yao et al., 2019). However, there has not been a comprehensive investigation on the function of the OsSPL9 on yield-related traits. In this study, we investigated the genetic effects of OsSPL9 on agronomic traits in the indica and japonica backgrounds and found that the number of secondary branches and GNP were significantly reduced in the mutants of OsSPL9 in both indica (R498) and japonica (ZH11) backgrounds (Figures 1, 2), indicating that OsSPL9 plays an important role in the regulation of grain number. Consistent with its function, OsSPL9 was expressed in the early developing young panicles (Figure 3B).
Moreover, haplotype analysis showed that Hap3 and Hap4 of OsSPL9 might be favorable haplotypes, and considering that both Hap3 and Hap4 accounted only for a relatively lower proportion (7.7 and 4.0%, respectively) of these cultivars (Figure 5), they might have a great potential for higher grain number breeding in rice. In future, it will be of great significance to reveal the functional differences of different haplotypes at the biochemical, molecular, and genetic levels. Especially, the polymorphisms located at 11 and 8,502, which, respectively, led to amino acid in-frame deletion and substitution (Supplementary Table 2), might be responsible for biological functional differences.
The C-Terminal Region of OsSPL9 Is Essential for Its Biological Function
SQUAMOSA-PROMOTER BINDING PROTEIN-LIKE family proteins are plant-specific transcription factors. They all contain the highly conserved SBP-domain, which is necessary for DNA binding and nuclear localization (Birkenbihl et al., 2005). In addition, the N-terminus or C-terminus of SPL proteins exhibits transcriptional activation activity. For example, the activation domain of OsSPL16/OsSPL9 is located at the N-terminus (Wang et al., 2012; Tang et al., 2016), while the activation domain of OsSPL14 is located in the C-terminus region (Lu et al., 2013). Both N-terminus and C-terminus of OsSPL18 have transcriptional activation activity (Yuan et al., 2019). In this study, although the amino acid substitution in lgn5 mutant and the premature protein of KO mutants of OsSPL9 were located at the C-terminus without disrupting its N-terminal activation domain and SBP-domain (Figure 2E; Supplementary Figure S4), both lgn5 and KO mutants of OsSPL9 exhibited significantly decreased GNP and grain yield (Figures 1, 2). Thus, the results genetically demonstrated that the C-terminal region of OsSPL9 is essential for its biological function.
The OsSPL9-RCN1 Pathway Regulates Grain Number in Rice
Rice TERMINAL FLOWER 1/CENTRORADIALIS homolog was an important positive regulator of panicle branches (Nakagawa et al., 2002; Wang et al., 2015), and its expression level was significantly downregulated in the early stage of developing young panicles in the mutants of OsSPL9 (Figure 4A; Supplementary Figure S5), which was consistent with the decreased panicle branches and grain number in lgn5 and KO mutants (Figures 1, 2). Further, dual-luciferase assays confirmed that OsSPL9 could directly activate RCN1 expression (Figure 4B). In addition, the cytological analysis showed that OsSPL9 regulated panicle development by affecting the phase transition from branch primordia to the spikelet primordia state (Figures 1E–H), which is similar to that of RCN1 (Nakagawa et al., 2002). Therefore, the results revealed a plausible OsSPL9-RCN1 pathway regulating panicle branches and grain number in rice.
Data Availability Statement
The original contributions presented in the study are included in the article/Supplementary Material, further inquiries can be directed to the corresponding authors.
Author Contributions
LH and WC conceived the research, performed most of the experiments, and wrote the manuscript. WY, XL, and CZ were responsible for the identification and characterization of mutants. XZha, LZ, XZhu, and JY completed part of the work. PQ, YW, and BM provided the instructions about the experiments. SL, HY, and BT helped to review and revise the manuscript. All authors contributed to the article and approved the submitted version.
Funding
This research was supported by funds from the Rice Molecular Design Breeding (2016YFD0101801) and by breeding new varieties of disease-resistant transgenic rice by Ministry of Agriculture and Rural Affairs (2016ZX08001-002).
Conflict of Interest
The authors declare that the research was conducted in the absence of any commercial or financial relationships that could be construed as a potential conflict of interest.
Supplementary Material
The Supplementary Material for this article can be found online at: https://www.frontiersin.org/articles/10.3389/fpls.2021.682018/full#supplementary-material
Footnotes
References
Abe, A., Kosugi, S., Yoshida, K., Natsume, S., Takagi, H., Kanzaki, H., et al. (2012). Genome sequencing reveals agronomically important loci in rice using MutMap. Nat. Biotechnol. 30, 174–178. doi: 10.1038/nbt.2095
Ashikari, M., Sakakibara, H., Lin, S., Yamamoto, T., Takashi, T., Nishimura, A., et al. (2005). Cytokinin oxidase regulates rice grain production. Science 309, 741–745. doi: 10.1126/science.1113373
Bai, X., Huang, Y., Mao, D., Wen, M., Zhang, L., and Xing, Y. (2016). Regulatory role of FZP in the determination of panicle branching and spikelet formation in rice. Sci. Rep. 6:19022. doi: 10.1038/srep19022
Birkenbihl, R. P., Jach, G., Saedler, H., and Huijser, P. (2005). Functional dissection of the plant-specific SBP-domain: overlap of the DNA-binding and nuclear localization domains. J. Mol. Biol. 352, 585–596. doi: 10.1016/j.jmb.2005.07.013
Dai, Z., Wang, J., Yang, X., Lu, H., Miao, X., and Shi, Z. (2018). Modulation of plant architecture by the miR156f-OsSPL7-OsGH3.8 pathway in rice. J. Exp. Bot. 69, 5117–5130. doi: 10.1093/jxb/ery273
Hu, J., Zeng, T., Xia, Q., Huang, L., Zhang, Y., Zhang, C., et al. (2020). Identification of key genes for the ultrahigh yield of rice using dynamic cross-tissue network analysis. Genom. Proteom. Bioinform. 8, 256–270. doi: 10.1016/j.gpb.2019.11.007
Huang, X., Qian, Q., Liu, Z., Sun, H., He, S., Luo, D., et al. (2009). Natural variation at the DEP1 locus enhances grain yield in rice. Nat. Genet. 41, 494–497. doi: 10.1038/ng.352
Jeon, J. S., Lee, S., Jung, K. H., Jun, S. H., Jeong, D. H., Lee, J., et al. (2000). T-DNA insertional mutagenesis for functional genomics in rice. Plant J. 22, 561–570. doi: 10.1046/j.1365-313x.2000.00767.x
Jiao, Y., Wang, Y., Xue, D., Wang, J., Yan, M., Liu, G., et al. (2010). Regulation of OsSPL14 by OsmiR156 defines ideal plant architecture in rice. Nat. Genet. 42, 541–544. doi: 10.1038/ng.591
Komatsu, K., Maekawa, M., Ujiie, S., Satake, Y., Furutani, I., Okamoto, H., et al. (2003). LAX and SPA: major regulators of shoot branching in rice. Proc. Natl. Acad. Sci. U. S. A. 100, 11765–11770. doi: 10.1038/nbt.2095
Lan, T., Zheng, Y., Su, Z., Yu, S., Song, H., Zheng, X., et al. (2019). OsSPL10, a SBP-box gene, plays a dual role in salt tolerance and trichome formation in rice (Oryza sativa L.). G3 9, 4107–4114. doi: 10.1534/g3.119.400700
Lee, J., Park, J. J., Kim, S. L., Yim, J., and An, G. (2007). Mutations in the rice liguleless gene result in a complete loss of the auricle, ligule, and laminar joint. Plant Mol. Biol. 65, 487–499. doi: 10.1007/s11103-007-9196-1
Li, Y., Fan, C., Xing, Y., Yun, P., Luo, L., Yan, B., et al. (2014). Chalk5 encodes a vacuolar H(+)-translocating pyrophosphatase influencing grain chalkiness in rice. Nat. Genet. 46, 398–404. doi: 10.1038/ng.2923
Li, Y. F., Zeng, X. Q., Li, Y., Wang, L., Zhuang, H., Wang, Y., et al. (2020). MULTI-FLORET SPIKELET 2, a MYB transcription factor, determines spikelet meristem fate and floral organ identity in rice. Plant Physiol. 184, 988–1003. doi: 10.1104/pp.20.00743
Lu, Z., Yu, H., Xiong, G., Wang, J., Jiao, Y., Liu, G., et al. (2013). Genome-wide binding analysis of the transcription activator ideal plant architecture reveals a complex network regulating rice plant architecture. Plant Cell 25, 3743–3759. doi: 10.1105/tpc.113.113639
Luo, L., Li, W., Miura, K., Ashikari, M., and Kyozuka, J. (2012). Control of tiller growth of rice by OsSPL14 and strigolactones, which work in two independent pathways. Plant Cell Physiol. 53, 1793–1801. doi: 10.1093/pcp/pcs122
Miura, K., Ikeda, M., Matsubara, A., Song, X. J., Ito, M., Asano, K., et al. (2010). OsSPL14 promotes panicle branching and higher grain productivity in rice. Nat. Genet. 42, 545–549. doi: 10.1038/ng.592
Nakagawa, M., Shimamoto, K., and Kyozuka, J. (2002). Overexpression of RCN1 and RCN2, rice TERMINAL FLOWER 1/CENTRORADIALIS homologs, confers delay of phase transition and altered panicle morphology in rice. Plant J. 29, 743–750. doi: 10.1046/j.1365-313X.2002.01255.x
Qin, M., Zhang, Y., Yang, Y., Miao, C., and Liu, S. (2020). Seed-specific overexpression of SPL12 and IPA1 improves seed dormancy and grain size in rice. Front. Plant Sci. 11:532771. doi: 10.3389/fpls.2020.532771
Ren, D., Li, Y., Zhao, F., Sang, X., Shi, J., Wang, N., et al. (2013). MULTI-FLORET SPIKELET1, which encodes an AP2/ERF protein, determines SPIKELET meristem fate and sterile lemma identity in rice. Plant Physiol. 162, 872–884. doi: 10.1104/pp.113.216044
Ren, D., Xu, Q., Qiu, Z., Cui, Y., Zhou, T., Zeng, D., et al. (2019). FON4 prevents the multi-floret spikelet in rice. Plant Biotechnol. J. 17, 1007–1009. doi: 10.1111/pbi.13083
Shan, Q., Wang, Y., Li, J., Zhang, Y., Chen, K., Liang, Z., et al. (2013). Targeted genome modification of crop plants using a CRISPR-Cas system. Nat. Biotechnol. 31, 686–688. doi: 10.1038/nbt.2650
Shao, Y. L., Zhou, H. Z., Wu, Y. R., Zhang, H., Lin, J., Jiang, X. Y., et al. (2019). OsSPL3, a SBP-domain protein, regulates crown root development in rice. Plant Cell 31, 1257–1275. doi: 10.1105/tpc.19.00038
Si, L., Chen, J., Huang, X., Gong, H., Luo, J., Hou, Q., et al. (2016). OsSPL13 controls grain size in cultivated rice. Nat. Genet. 48, 447–456. doi: 10.1038/ng.3518
Tabuchi, H., Zhang, Y., Hattori, S., Omae, M., Shimizu-Sato, S., Oikawa, T., et al. (2011). LAX PANICLE2 of rice encodes a novel nuclear protein and regulates the formation of axillary meristems. Plant Cell 23, 3276–3287. doi: 10.1105/tpc.111.088765
Tang, M., Zhou, C., Meng, L., Mao, D., Peng, C., Zhu, Y., et al. (2016). Overexpression of OsSPL9 enhances accumulation of cu in rice grain and improves its digestibility and metabolism. J. Genet. Genom. 43, 673–676. doi: 10.1016/j.jgg.2016.09.004
Wang, Q. L., Sun, A. Z., Chen, S. T., Chen, L. S., and Guo, F. Q. (2018). SPL6 represses signalling outputs of ER stress in control of panicle cell death in rice. Nat. Plants 4, 280–288. doi: 10.1038/s41477-018-0131-z
Wang, L., Sun, S., Jin, J., Fu, D., Yang, X., Weng, X., et al. (2015). Coordinated regulation of vegetative and reproductive branching in rice. Proc. Natl. Acad. Sci. U. S. A. 112, 15504–15509. doi: 10.1073/pnas.1521949112
Wang, S., Wu, K., Yuan, Q., Liu, X., Liu, Z., Lin, X., et al. (2012). Control of grain size, shape and quality by OsSPL16 in rice. Nat. Genet. 44, 950–954. doi: 10.1038/ng.2327
Xie, X., Ma, X., Zhu, Q., Zeng, D., Li, G., and Liu, Y. G. (2017). CRISPR-GE: a convenient software toolkit for CRISPR-based genome editing. Mol. Plant 10, 1246–1249. doi: 10.1016/j.molp.2017.06.004
Xie, K., Wu, C., and Xiong, L. (2006). Genomic organization, differential expression, and interaction of SQUAMOSA promoter-binding-like transcription factors and micro RNA 156 in rice. Plant Physiol. 142, 280–293. doi: 10.1104/pp.106.084475
Xu, W., Tao, J., Chen, M., Dreni, L., Luo, Z., Hu, Y., et al. (2017). Interactions between FLORAL ORGAN NUMBER4 and floral homeotic genes in regulating rice flower development. J. Exp. Bot. 68, 483–498. doi: 10.1093/jxb/erw459
Yang, R., Li, P., Mei, H., Wang, D., Sun, J., Yang, C., et al. (2019). Fine-tuning of MiR528 accumulation modulates flowering time in rice. Mol. Plant 12, 1103–1113. doi: 10.1016/j.molp.2019.04.009
Yao, S., Yang, Z., Yang, R., Huang, Y., Guo, G., Kong, X., et al. (2019). Transcriptional regulation of miR528 by OsSPL9 orchestrates antiviral response in rice. Mol. Plant 12, 1114–1122. doi: 10.1016/j.molp.2019.04.010
Yoshida, A., Sasao, M., Yasuno, N., Takagi, K., Daimon, Y., Chen, R., et al. (2013). TAWAWA1, a regulator of rice inflorescence architecture, functions through the suppression of meristem phase transition. Proc. Natl. Acad. Sci. U. S. A. 110, 767–772. doi: 10.1073/pnas.1216151110
Yuan, H., Qin, P., Hu, L., Zhan, S., Wang, S., Gao, P., et al. (2019). OsSPL18 controls grain weight and grain number in rice. J. Genet. Genom. 46, 41–51. doi: 10.1016/j.jgg.2019.01.003
Yue, E., Li, C., Li, Y., Liu, Z., and Xu, J. H. (2017). MiR529a modulates panicle architecture through regulating SQUAMOSA PROMOTER BINDING-LIKE genes in rice (Oryza sativa). Plant Mol. Biol. 94, 469–480. doi: 10.1007/s11103-017-0618-4
Keywords: rice, panicle branches, grain number per panicle, grain yield, OsSPL9
Citation: Hu L, Chen W, Yang W, Li X, Zhang C, Zhang X, Zheng L, Zhu X, Yin J, Qin P, Wang Y, Ma B, Li S, Yuan H and Tu B (2021) OsSPL9 Regulates Grain Number and Grain Yield in Rice. Front. Plant Sci. 12:682018. doi: 10.3389/fpls.2021.682018
Edited by:
Hanwei Mei, Shanghai Agrobiological Gene Center, ChinaReviewed by:
Xiaoli Jin, Zhejiang University, ChinaJun Fang, Northeast Institute of Geography and Agroecology, Chinese Academy of Sciences (CAS), China
Copyright © 2021 Hu, Chen, Yang, Li, Zhang, Zhang, Zheng, Zhu, Yin, Qin, Wang, Ma, Li, Yuan and Tu. This is an open-access article distributed under the terms of the Creative Commons Attribution License (CC BY). The use, distribution or reproduction in other forums is permitted, provided the original author(s) and the copyright owner(s) are credited and that the original publication in this journal is cited, in accordance with accepted academic practice. No use, distribution or reproduction is permitted which does not comply with these terms.
*Correspondence: Bin Tu, dHViaW4xNDIxNkBzaWNhdS5lZHUuY24=; Hua Yuan, eXVhbmh1YTE0NTM2QHNpY2F1LmVkdS5jbg==
†These authors have contributed equally to this work and share first authorship