- Institute of Plant Biochemistry and Photosynthesis, Consejo Superior de Investigaciones Científicas-University of Sevilla, Sevilla, Spain
The elongation of the linear chains of starch is undertaken by starch synthases. class 3 of starch synthase (SS3) has a specific feature: a long N-terminal region containing starch binding domains (SBDs). In this work, we analyze in vivo the contribution of these domains to the localization pattern of the enzyme. For this purpose, we divided the N-terminal region of Arabidopsis SS3 in three domains: D1, D2, and D3 (each of which contains an SBD and a coiled-coil site). Our analyses indicate that the N-terminal region is sufficient to determine the same localization pattern observed with the full-length protein. D2 binds tightly the polypeptide to the polymer and it is necessary the contribution of D1 and D3 to avoid the polypeptide to be trapped in the growing polymer. The localization pattern of Arabidopsis SS3 appears to be the result of the counterbalanced action of the different domains present in its N-terminal region.
Introduction
Most plants store any excess energy and carbon produced by photosynthesis in the form of starch. Starch granules are semicrystalline structures composed of two different α-glucan polymers: amylose and amylopectin. Both are composed of linear chains of α-1,4-linked glucose units branched through α-1,6 bonds (Buléon et al., 1998). Amylose, the minor component, has an amorphous structure and few branches, while the major partner, amylopectin, is moderately branched and gives rise to the semicrystalline structure of the starch granule (Buléon et al., 1998). The synthesis of starch requires the concerted action of different activities: the elongation of the chains of the glucan polymer by starch synthases (SSs, ADP-Glc: α-1,4 glucan α-4-glucosyltransferases, EC 2.4.1.21), the synthesis of branches by starch branching enzyme (SBE, α-1,4 glucan branching enzyme, EC 2.4.1.18) and the removal of excess branches (to allow the crystallization of amylopectin branches grouped in clusters) by starch debranching enzyme (DBE or Isoamylase, EC 3.2.1.68). All these enzymes have different isoforms. Thus, Arabidopsis contains six classes of SSs, GBSS (granule-bound starch synthase), SS1, SS2, SS3, SS4, and SS5. In some cases, such as cereals, maize or rice, there are more than one isoform for each class (Jeon et al., 2010). GBSS is responsible for the synthesis of amylose (Kuipers et al., 1994; Denyer et al., 1995; Nakamura et al., 1995), SS4 and SS5 are involved in the process of initiation of the starch granule (Roldán et al., 2007; Abt et al., 2020), and the remaining SSs elongate the different chains of the amylopectin molecule (Szydlowski et al., 2011).
SS3 was first identified in potato (Edwards et al., 1995; Abel et al., 1996) and is present in all starch-accumulating organisms (Ball and Morell, 2003). The suppression of SS3 activity in Chlamydomonas (Fontaine et al., 1993) and potato (Edwards et al., 1999) has a major impact on the synthesis of amylopectin, resulting in a polymer with a modified chain-length distribution and the reduced synthesis of starch. Arabidopsis mutants lacking SS3 (AtSS3 mutants) activity showed significant increases in the frequency of linear chains of amylopectin with a degree of polymerization >60. Furthermore, the total SS activity is increased in Atss3 mutants, with plants showing greater starch content in the leaves during the light period, suggesting that SS3 has a negative regulatory function on the biosynthesis of transitory starch in Arabidopsis (Zhang et al., 2005). Our group has also shown that SS3 can partially substitute the function of SS4 in the initiation of the starch granule, and therefore most of the chloroplasts of the ss3-ss4 double mutant are devoid of starch (Szydlowski et al., 2009). Other authors report that SS3, together with other enzymes involved in starch metabolism (SS2, SBE2a and SBE2b), form a multiprotein complex in maize amyloplasts, indicating that they may modulate each other's activities (Hennen-Bierwagen et al., 2009).
SS3 contains different functional domains that enable it to perform the functions described above. Like other SSs, SS3 is a GT-B-fold glycosyltransferase, classified within family GT5 in the CAZy database (Coutinho et al., 2003). The C-terminal region is homologous to other SSs and contains the catalytic (GT5) and the glycosyltransferase (GT1) domains. SS3, however, has a long N-terminal region, exclusive to its class with three Starch Binding Domains (SBDs) belonging to the family 53 of Carbohydrate Binding Modules (CBM) (Palopoli et al., 2006) (http://www.cazy.org). These SBDs have been comprehensively analyzed in vitro both in Arabidopsis (Valdez et al., 2008; Wayllace et al., 2010) and the picoalgae Ostreococcus tauri (Barchiesi et al., 2015, 2018). These studies indicate that they modulate the catalytic activity of the C-terminal region, and highlight the importance of SBDs 2 and 3 in binding to starch and in the regulation and catalysis of SS3 (Wayllace et al., 2010). Computational analysis of the N-terminal region of maize SS3 has identified two coiled-coil (CC) domains, which typically function to mediate protein-protein interactions (Rose and Meier, 2004). The two CC domains flank the central SBD, which Wayllace et al. (2010) indicate to be the most significant domain with respect to substrate affinity. These analyses suggest that the N-terminal region of SS3 may contain regions that undertake protein-protein interactions and non-catalytic glucan binding (Hennen-Bierwagen et al., 2009).
We have previously shown that AtSS3 surrounds the surface of the starch granule and, after disrupting the chloroplasts, it is found in the soluble, stromal fraction; it is not detected in the membrane or starch fractions (Gámez-Arjona et al., 2014). The present work shows that the N-terminal region of AtSS3 is responsible for the localization pattern of the enzyme. This region contains three predicted CC domains and three SBDs. We show that the region containing the CC and SBD 1 and 2 are necessary, and sufficient, for correct localization (AtSS3 localization) to be achieved. Those containing the CC and SBD 2 and 3, in the absence of CC1 and SBD1, change the localization pattern, with the polypeptide appearing tightly bound to the starch granule.
Materials and Methods
Plant Materials and Growth Conditions
Nicotiana benthamiana plants were grown in soil (in pots) in a greenhouse under a 16-h light/8-h dark cycle (light intensity 250 μmoles m−2s−1) at 22°C.
Plasmids Construction
cDNAs required for the transient expression of truncated versions of AtSS3 in N. benthamiana were cloned using the Gateway system (ThermoFisher Scientific). The different fragments were amplified using the oligonucleotides shown in Supplementary Table 1. The sequenced fragments were cloned into the pDONR207 plasmid (ThermoFisher Scientific) via BP clonase reaction and subsequently transferred to pEarleyGate103 (Earley et al., 2006) via a LR clonase reaction to allow the C-terminus translational fusion of the fragments to GFP. An overlapping PCR strategy was employed to clone fragments D13, D23, D2, and D3 and to fuse the AtSS3 chloroplast transit peptide (CTP) with the sequence of interest.
Transient Expression in N. benthamiana
Corresponding transgene vectors were electroporated into Agrobacterium tumefaciens strain C58 (Wood et al., 2001). The agroinfiltration of N. benthamiana leaves was assessed as previously described (Gámez-Arjona et al., 2014).
Confocal Microscopy
A DM6000 confocal laser-scanning microscope (Leica Microsystems) equipped with a ×63 oil immersion objective and a ×20 objective was used to detect the localization of the GFP fusion proteins. GFP and chlorophyll autofluorescence imaging was performed by exciting the cells with an argon laser at 488 nm and detecting fluorescence emissions at 500–525 nm and 630–690 nm, respectively.
AtSS3 Fragments Localization Analysis by Immunoblotting
The fragments D123, D12, D2, and D23, cloned into pEarlyGate 103 vector, were transiently expressed in N. benthamiana leaves by agroinfiltration as described above. Leaf material (500 mg) was harvested 2 days after infiltration at the same time of the day/night cycle to avoid differences of starch accumulation between samples analyzed. Samples were disrupted in liquid nitrogen with a mortar and pestle. Extracts were resuspended in 500 μl PD buffer (20 mM HEPES-KOH, pH 7.6, 80 mM NaCl, 1 mM MgCl2, and 1 mM DTT) supplemented with 2 μl protease inhibitor cocktail (SIGMA) and filtered through Miracloth (Merck). The extract was centrifuged at 12,000 × g at 4°C for 20 min over 1 ml Percoll 90% diluted in PD buffer. The pellet (starch granules) was washed with 500 μl PD buffer supplemented with 0.2% Triton X-100 and pelleted again by centrifugation at 12,000 × g at 4°C for 20 min. The pellet was resuspended in 20 μl preloading buffer (50 mM Tris-HCl, pH 6.8, 2% SDS) and boiled for 10 min. Samples were then centrifuged a 12,000 × g at 4°C for 10 min to eliminate debris. Protein in the supernatant fractions was determined according to the Lowry method (Lowry et al., 1951) and 2 μg of each sample were analyzed by SDS-PAGE (Laemmli, 1970) (15% total acrylamide) in a Mini-Protean Tetra cell (BIO-RAD), transferred to nitrocellulose membrane by electroblotting in a Trans-Blot Turbo transfer cell (BIO-RAD) and immunoblotted using anti-His5 horseradish peroxidase conjugate antibody (QIAGEN) at 1:2,000 dilution. The chemiluminescence was visualized using a Chemidoc Imaging System (BIO-RAD) running Quantity One software (BIO-RAD).
Results
The N-Terminal Region of SS3 Determines the Localization of the Enzyme
AtSS3 has two well-differentiated regions, the C-terminal region, containing the catalytic and substrate binding sites, and the N-terminal region, which contains three SBDs (SBD1 from amino acid 205 to 290, SBD2 from 380 to 476, and SBD3 from 547 to 637, according to TAIR – http://www.arabidopsis.org-) plus CC domains of imprecise localization. The DeepCoil tool of the web service for protein bioinformatic analysis MPI Bioinformatics Toolkit (https://toolkit.tuebingen.mpg.de) (Zimmermann et al., 2018) was used to predict the position of these CC. Three were predicted: CC1 from amino acid 161 to 177, CC2 from 301 to 349, and CC3 from 493 to 517. Figure 1 shows the different regions and domains of AtSS3.
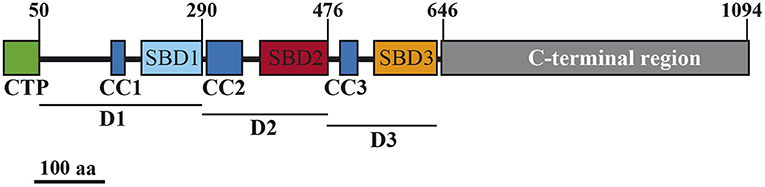
Figure 1. Schematic representation of the AtSS3 protein. Numbers at the top indicate amino acid positions. SBD, starch-binding domain; CC, coiled-coils sites; CTP, chloroplast transit peptide. D1, D2, and D3 are the domains employed in this study.
We have previously shown that each Arabidopsis SS displays a specific pattern of localization in the chloroplast. The localization of AtSS3 has been indicated as restricted to the region surrounding the starch granules in the chloroplast (Gámez-Arjona et al., 2014). Using the same technical approach as reported in this latter paper, analyses were made to determine whether the N-terminal region of AtSS3 is sufficient to determine the localization pattern observed for the full-length protein. Figure 2 shows that the N-terminal region of AtSS3 (denoted D123) displayed the same localization pattern than the complete protein. Figures 2G–I show magnified images of the localization of D123-GFP, with the polypeptide surrounding the starch granule. Starch granules appear as black areas lacking chlorophyll autofluorescence (Figure 2H).
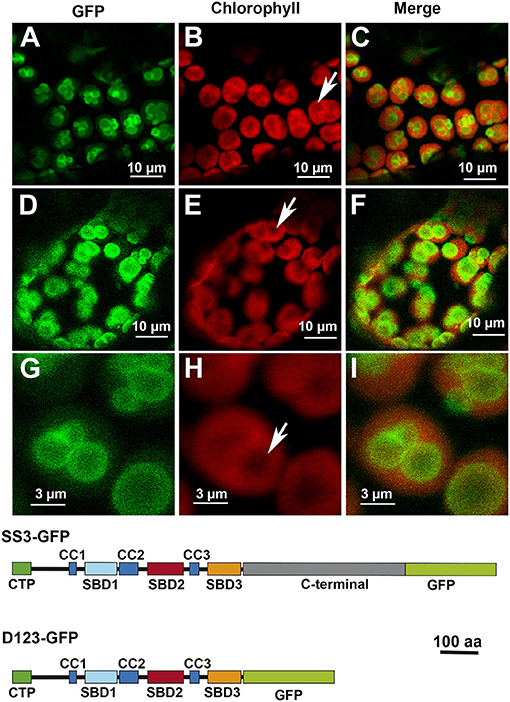
Figure 2. Localization of full-length AtSS3 (A–C) and the N-terminal region of AtSS3 (D–I) fused to GFP in Nicotiana benthamiana chloroplasts. GFP column: fluorescence of the GFP marker. Chlorophyll column: autofluorescence of the chlorophyll. Merge column: merged images of previous columns. Bottom: structures of the constructs fused to GFP. SS3-GFP: full-length AtSS3 fused to GFP. D123-GFP, N-terminal region of AtSS3 fused to GFP. The white arrows indicate the presence of a starch granule.
Contribution of Each Domain to the Localization Pattern of AtSS3
To analyze the contribution of the different domains in the AtSS3 N-terminal region to the localization pattern of the full protein, the region was divided into three domains: D1, containing CC1 and SBD1, D2, containing CC2 and SBD2, and D3, containing CC3 and SBD3 (see Figure 1). The effect of eliminating one or two domains on the pattern of localization was then tested.
The removal of D3 did not seem to affect the localization pattern: the D12-GFP polypeptide displayed the same localization than D123-GFP (Figures 3A–C). The elimination of D2, however, modified the localization pattern, with the resulting D13-GFP polypeptide localizing preferentially in certain patches without apparent relation to any starch granules (Figures 3D–F). Finally, the elimination of D1 led to the resulting polypeptide, D23-GFP, localizing throughout the chloroplast but mainly in areas coincident with the starch granules (Figures 3G–I). D1 and D3 alone drove a similar pattern, with the resulting D1-GFP and D3-GFP polypeptides found all over the chloroplast (Figures 4A–C,G–I, respectively). D2-GFP showed a similar localization pattern to D23-GFP, with signals across the entire chloroplast, but especially concentrated in the regions coincident with the starch granules (Figures 4D–F).
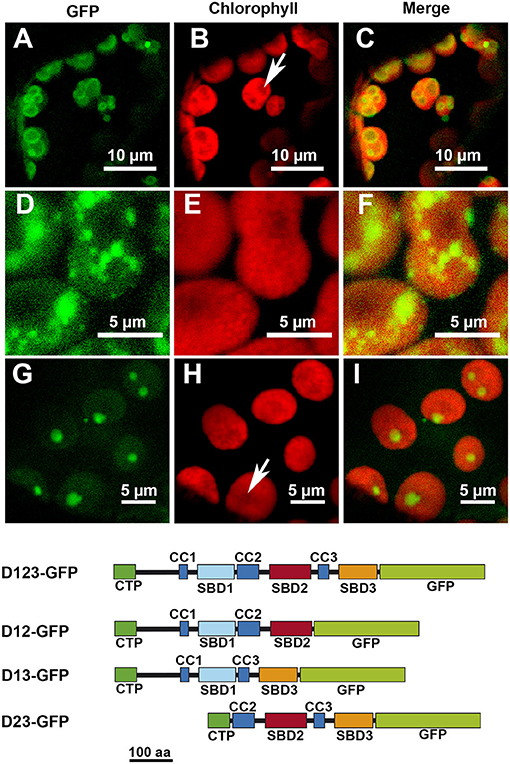
Figure 3. Localization of different truncated versions of AtSS3 fused to GFP in N. benthamiana chloroplasts. D12-GFP: (A–C). D13-GFP: (D–F). D23-GFP: (G–I). GFP column: fluorescence of the GFP marker. Chlorophyll column: autofluorescence of the chlorophyll. Merge column: merged images of previous columns. Bottom: structures of the constructs fused to GFP. D12-GFP: N-terminal region of AtSS3 without D3 fused to GFP. D13-GFP: N-terminal region of AtSS3 without D2 fused to GFP. D23-GFP: N-terminal region of AtSS3 without D1 fused to GFP. The white arrows indicate the presence of a starch granule.
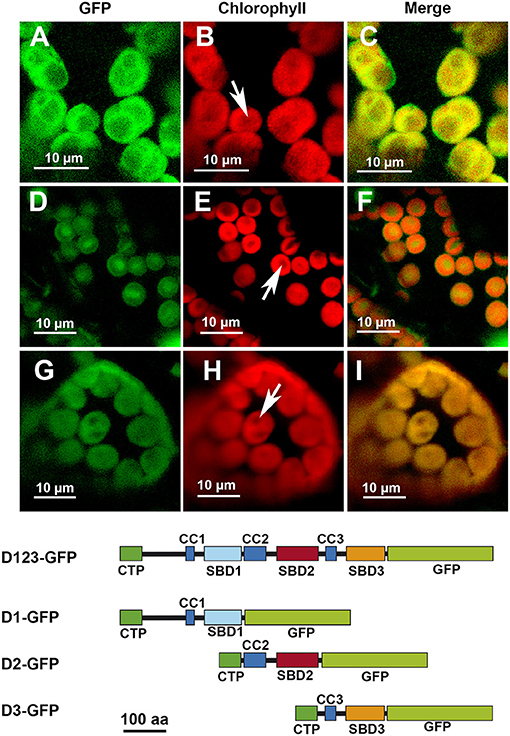
Figure 4. Localization of different truncated versions of AtSS3 fused to GFP in N. benthamiana chloroplasts. D1-GFP: (A–C). D2-GFP: (D–F). D3-GFP: (G–I). GFP column: fluorescence of the GFP marker. Chlorophyll column: autofluorescence of the chlorophyll. Merge column: merged images of previous columns. Bottom: structures of the constructs fused to GFP. D1-GFP: N-terminal region of AtSS3 without D2 and D3 domains fused to GFP. D2-GFP: N-terminal region of AtSS3 without D1 and D2 domains fused to GFP. D2-GFP: N-terminal region of AtSS3 without D1 and D2 domains fused to GFP. The white arrows indicate the presence of a starch granule.
Presence of the AtSS3 Truncated Versions in the Starch
The localization pattern of D23-GFP and D2-GFP seemed to indicate that these polypeptides co-localized with the starch granules. To determine whether these truncated polypeptides were bound to the polymer, their presence was sought on purified starch granules. Purified starch granules from N. benthamiana leaves agroinfiltrated with the constructs D12-, D123-, D2-, and D23-GFP were washed to eliminate proteins weakly bound to the polymer and then boiled to extract any proteins tightly bound to the polysaccharide. These proteins were analyzed by immunoblot using anti-His × 5 antibody (pEarleyGate 103 allows the C-terminus translational fusion of the fragment to GFP and to the 5 × His tail). This confirmed D2-GFP and D23-GFP to be tightly bound to the starch (Figure 5B). A faint band was also observed in the starch purified from D12-GFP infiltrated leaves, suggesting that its binding is weaker than that shown by D2-, and D23-GFP polypeptides. No signal was detected in the starch purified from leaves infiltrated with the D123-GFP polypeptide.
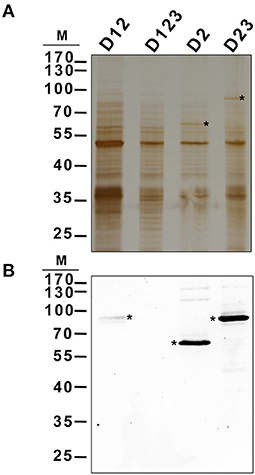
Figure 5. Localization of different AtSS3 truncated versions in starch of agroinfiltrated N. benthamiana leaves. The constructs D12-GFP, D123-GFP, D2-GFP and D23-GFP were transiently expressed in N. benthamiana leaves by agroinfiltration. Infiltrated leaves were harvested and starch was purified, washed and boiled to extract proteins localized in the polymer. 2 μg of protein were electrophoresed in SDS-PAGE and silver stained (A) or immunoblotted using anti-His5x antibody (B). Asterisks indicate corresponding polypeptides. M, kDa marker.
Discussion
Most of the proteins involved in the starch metabolism are found in the soluble fraction of chloroplasts, although they must interact with the surface of the polymer, where its synthesis and degradation take place. Some of these proteins interact with the starch granule through SBDs (non-catalytic CBMs) whereas others, such as GBSS, require interaction with other polypeptides that contain a CBM. Indeed, the localization of GBSS in the starch granule requires an interaction with Protein Targeting To Starch 1, a polypeptide with a CC domain through which interacts with GBSS and a SBD domain belonging to the CBM48 family required for binding the protein to starch (Seung et al., 2015). Analysis of the interaction of glucan water dikinase and α-amylase 3 (both examples of starch metabolism proteins with SBDs belonging to the CBM45 family) with starch indicates that they bind with an affinity about two orders of magnitude lower than that of classical SBDs in microbial extracellular amylolytic enzymes. This suggests that low-affinity SBDs are a feature in plastidial starch metabolism, which allows binding to be reversible and facilitates the regulation of enzyme activities (Glaring et al., 2011).
SS3 contains three SBDs belonging to the CBM53 family (Palopoli et al., 2006). The functionality of the SBDs domains of Arabidopsis and Ostreococcus tauri SS3 have been comprehensively studied in vitro (Palopoli et al., 2006; Valdez et al., 2008; Wayllace et al., 2010; Barchiesi et al., 2015, 2018) and the results indicate that the N-terminal region shows an increased capacity to bind starch depending on the number of SBDs modules present. Moreover, the region containing SBD2 and 3 appear to makes the greatest contribution to binding (Valdez et al., 2008). The N-terminal SBDs also seem to have a regulatory role modulating the catalytic properties of SS3 (Valdez et al., 2008; Wayllace et al., 2010). We have previously shown that AtSS3 is localized to the area surrounding the starch granules, and that there is no strong interaction with the polysaccharide since the polypeptide is not found in the starch fraction after disrupting the chloroplasts (Gámez-Arjona et al., 2014). In the present work we show that the N-terminal region of AtSS3, which contains three SBDs, is sufficient to determine the same localization pattern shown by the full-length protein (Figure 1). In agreement with results of Valdez et al. (2008), SBD2 binds tightly to the starch granule, and the constructs D2-GFP and D23-GFP are detected in the purified starch granules of infiltrated Nicotiana leaves (Figure 5), likely because the tight binding of these polypeptides to the starch granule determine that they become trapped and buried in the growing polymer. The presence of D1 appears to weaken this interaction with the polymer, allowing the construct D12-GFP to show a localization pattern similar to that of the complete AtSS3. D1 seems to be more efficient than D3 at weakening the interaction of D2 with the starch granule (see the different localization pattern of D12-GFP and D23-GFP in Figure 3), but the presence of D12-GFP in the purified starch and the absence of D123-GFP (Figure 5) indicates that D3 is also necessary to prevent excessive binding. SBDs 1 and 3 seem unable to drive the localization of the polypeptide to the starch granule (see Figures 3D–F, 4A–C,G–I), supporting the idea that SBD2 is necessary for this to occur. Nevertheless, each domain contains a SBD and also a CC site, and it cannot be ruled out that some of the effect observed for the different construct are the consequence of the presence or elimination of these CC sites. It has been shown that SS3 form multiprotein complexes with other proteins of the starch metabolism in developing maize endosperm, and these interactions likely take place via the CC sites of the enzyme (Hennen-Bierwagen et al., 2008). Thus, the weakening effect of the SBD2-mediated interaction with starch observed for the D1 and D3 modules may be the consequence of interactions with other proteins exerted through their CC domains.
In summary, the in planta localization pattern of AtSS3 would appear to be the result of a balance between the different activities of the domains present in the N-terminal region of the enzyme.
Data Availability Statement
The raw data supporting the conclusions of this article will be made available by the authors, without undue reservation.
Author Contributions
FG-A conducted the experimental work. ÁM designed the work and wrote the manuscript. All authors contributed to the article and approved the submitted version.
Funding
This work was funded by Grants BIO2012-35403 and PGC2018-096851-B-C22 from the Spanish Ministry of Science and Innovation (MICINN) and the European Fund for Regional Development.
Conflict of Interest
The authors declare that the research was conducted in the absence of any commercial or financial relationships that could be construed as a potential conflict of interest.
Publisher's Note
All claims expressed in this article are solely those of the authors and do not necessarily represent those of their affiliated organizations, or those of the publisher, the editors and the reviewers. Any product that may be evaluated in this article, or claim that may be made by its manufacturer, is not guaranteed or endorsed by the publisher.
Acknowledgments
We thank Alicia Orea for technical assistant with confocal microscopy.
Supplementary Material
The Supplementary Material for this article can be found online at: https://www.frontiersin.org/articles/10.3389/fpls.2021.704161/full#supplementary-material
References
Abel, G. J. W., Springer, F., Willmitzer, L., and Kossmann, J. (1996). Cloning and functional analysis of a cDNA encoding a novel 139 kDa starch synthase from potato (Solanum tuberosum L.). Plant J. 10, 981–991. doi: 10.1046/j.1365-313X.1996.10060981.x
Abt, M. R., Pfister, B., Sharma, M., Eicke, S., Bürgy, L., Neale, I., et al. (2020). STARCH SYNTHASE5, a noncanonical starch synthase-like protein, promotes starch granule initiation in arabidopsis[OPEN]. Plant Cell 32, 2543–2565. doi: 10.1105/tpc.19.00946
Ball, S. G., and Morell, M. K. (2003). From bacterial glycogen to starch: understanding the biogenesis of the plant starch granule. Annu. Rev. Plant Biol. 54, 207–233. doi: 10.1146/annurev.arplant.54.031902.134927
Barchiesi, J., Hedin, N., Gomez-Casati, D. F., Ballicora, M. A., and Busi, M. V. (2015). Functional demonstrations of starch binding domains present in Ostreococcus tauri starch synthases isoforms. BMC Res. Notes 8:613. doi: 10.1186/s13104-015-1598-6
Barchiesi, J., Velazquez, M. B., Palopoli, N., Iglesias, A. A., Gomez-Casati, D. F., Ballicora, M. A., et al. (2018). Starch synthesis in Ostreococcus tauri: the starch-binding domains of starch synthase III-B are essential for catalytic activity. Front. Plant Sci. 9:e01541. doi: 10.3389/fpls.2018.01541
Buléon, A., Colonna, P., Planchot, V., and Ball, S. (1998). Starch granules: structure and biosynthesis. Int. J. Biol. Macromol. 23, 85–112. doi: 10.1016/S0141-8130(98)00040-3
Coutinho, P. M., Deleury, E., Davies, G. J., and Henrissat, B. (2003). An evolving hierarchical family classification for glycosyltransferases. J. Mol. Biol. 328, 307–317. doi: 10.1016/S0022-2836(03)00307-3
Denyer, K., Barber, L. M., Burton, R., Hedley, C. L., Hylton, C. M., Johnson, S., et al. (1995). The isolation and characterization of novel low-amylose mutants of Pisum sativum L. Plant Cell Environ. 18, 1019–1026. doi: 10.1111/j.1365-3040.1995.tb00612.x
Earley, K. W., Haag, J. R., Pontes, O., Opper, K., Juehne, T., Song, K., et al. (2006). Gateway-compatible vectors for plant functional genomics and proteomics. Plant J. 45, 616–629. doi: 10.1111/j.1365-313X.2005.02617.x
Edwards, A., Fulton, D. C., Hylton, C. M., Jobling, S. A., Gidley, M., Rossner, U., et al. (1999). A combined reduction in activity of starch synthases II and III of potato has novel effects on the starch of tubers. Plant J. 17, 251–261. doi: 10.1046/j.1365-313X.1999.00371.x
Edwards, A., Marshall, J., Sidebottom, C., Visser, R. G., Smith, A. M., and Martin, C. (1995). Biochemical and molecular characterization of a novel starch synthase from potato tubers. Plant J. 8, 283–294. doi: 10.1046/j.1365-313X.1995.08020283.x
Fontaine, T., D'Hulst, C., Maddelein, M. L., Routier, F., Pepin, T. M., Decq, A., et al. (1993). Toward an understanding of the biogenesis of the starch granule. Evidence that Chlamydomonas soluble starch synthase II controls the synthesis of intermediate size glucans of amylopectin. J. Biol. Chem. 268, 16223–16230. doi: 10.1016/S0021-9258(19)85409-1
Gámez-Arjona, F. M., Raynaud, S., Ragel, P., and Mérida, Á. (2014). Starch synthase 4 is located in the thylakoid membrane and interacts with plastoglobule-associated proteins in Arabidopsis. Plant J. 80, 305–316. doi: 10.1111/tpj.12633
Glaring, M. A., Baumann, M. J., Hachem, M. A., Nakai, H., Nakai, N., Santelia, D., et al. (2011). Starch-binding domains in the CBM45 family - low-affinity domains from glucan, water dikinase and α-amylase involved in plastidial starch metabolism. FEBS J. 278, 1175–1185. doi: 10.1111/j.1742-4658.2011.08043.x
Hennen-Bierwagen, T. A., Lin, Q., Grimaud, F., Planchot, V., Keeling, P. L., James, M. G., et al. (2009). Proteins from multiple metabolic pathways associate with starch biosynthetic enzymes in high molecular weight complexes: a model for regulation of carbon allocation in maize amyloplasts. Plant Physiol. 149, 1541–1559. doi: 10.1104/pp.109.135293
Hennen-Bierwagen, T. A., Liu, F., Marsh, R. S., Kim, S., Gan, Q., Tetlow, I. J., et al. (2008). Starch biosynthetic enzymes from developing maize endosperm associate in multisubunit complexes. Plant Physiol. 146, 1892–1908. doi: 10.1104/pp.108.116285
Jeon, J.-S., Ryoo, N., Hahn, T. R., Walia, H., and Nakamura, Y. (2010). Starch biosynthesis in cereal endosperm. Plant Physiol. Biochem. 48, 383–392. doi: 10.1016/j.plaphy.2010.03.006
Kuipers, A. G. J., Jacobsen, E., and Visser, R. G. F. (1994). Formation and deposition of amylose in the potato tuber starch granule are affected by the reduction of granule-bound starch synthase gene expression. Plant Cell 6, 43–52. doi: 10.2307/3869673
Laemmli, U. K. (1970). Cleavage of structural proteins during the assembly of the head of bacteriophage T4. Nature 227, 680–685. doi: 10.1038/227680a0
Lowry, O. H., Rosebrough, N. J., Farr, A. L., and Randall, R. J. (1951). Protein measurement with the Folin phenol reagent. J. Biol. Chem. 193, 265–275. doi: 10.1016/S0021-9258(19)52451-6
Nakamura, T., Yamamori, M., Hirano, H., Hidaka, S., and Nagamine, T. (1995). Production of waxy (amylose-free) wheats. Mol. Gen. Genet. 1, 253–259. doi: 10.1007/BF02191591
Palopoli, N., Busi, M. V., Fornasari, M. S., Gomez-Casati, D., Ugalde, R., and Parisi, G. (2006). Starch-synthase III family encodes a tandem of three starch-binding domains. Proteins-Struct. Funct. Bioinforma. 65, 27–31. doi: 10.1002/prot.21007
Roldán, I., Wattebled, F., Mercedes Lucas, M., Delvallé, D., Planchot, V., Jiménez, S., et al. (2007). The phenotype of soluble starch synthase IV defective mutants of Arabidopsis thaliana suggests a novel function of elongation enzymes in the control of starch granule formation. Plant J. 49, 492–504. doi: 10.1111/j.1365-313X.2006.02968.x
Rose, A., and Meier, I. (2004). Scaffolds, levers, rods and springs: diverse cellular functions of long coiled-coil proteins. Cell. Mol. Life Sci. 61, 1996–2009. doi: 10.1007/s00018-004-4039-6
Seung, D., Soyk, S., Coiro, M., Maier, B. A., Eicke, S., and Zeeman, S. C. (2015). Protein targeting to starch is required for localising granule-bound starch synthase to starch granules and for normal amylose synthesis in Arabidopsis. PLoS Biol. 13:e1002080. doi: 10.1371/journal.pbio.1002080
Szydlowski, N., Ragel, P., Hennen-Bierwagen, T. A., Planchot, V., Myers, A. M., Mérida, A., et al. (2011). Integrated functions among multiple starch synthases determine both amylopectin chain length and branch linkage location in Arabidopsis leaf starch. J. Exp. Bot. 62, 4547–4559. doi: 10.1093/jxb/err172
Szydlowski, N., Ragel, P., Raynaud, S., Lucas, M. M., Roldan, I., Montero, M., et al. (2009). Starch granule initiation in Arabidopsis requires the presence of either class IV or class III starch synthases. Plant Cell 21, 2443–2457. doi: 10.1105/tpc.109.066522
Valdez, H. A., Busi, M. V., Wayllace, N. Z., Parisi, G., Ugalde, R. A., and Gomez-Casati, D. F. (2008). Role of the N-Terminal Starch-Binding Domains in the Kinetic Properties of Starch Synthase III from Arabidopsis thaliana. Biochemistry 47, 3026–3032. doi: 10.1021/bi702418h
Wayllace, N. Z., Valdez, H. A., Ugalde, R. A., Busi, M. V., and Gomez-Casati, D. F. (2010). The starch-binding capacity of the noncatalytic SBD2 region and the interaction between the N- and C-terminal domains are involved in the modulation of the activity of starch synthase III from Arabidopsis thaliana: enzymes and catalysis. FEBS J. 277, 428–440. doi: 10.1111/j.1742-4658.2009.07495.x
Wood, D. W., Setubal, J. C., Kaul, R., Monks, D. E., Kitajima, J. P., Okura, V. K., et al. (2001). The genome of the natural geneticengineer Agrobacterium tumefaciens C58. Science 294, 2317–2323. doi: 10.1126/science.1066804
Zhang, X., Myers, A. M., and James, M. G. (2005). Mutations affecting starch synthase III in Arabidopsis alter leaf starch structure and increase the rate of starch synthesis. Plant Physiol. 138, 663–674. doi: 10.1104/pp.105.060319
Keywords: starch, starch binding domain, starch synthase 3, Arabidopsis, starch metabolism
Citation: Gámez-Arjona FM and Mérida Á (2021) Interplay Between the N-Terminal Domains of Arabidopsis Starch Synthase 3 Determines the Interaction of the Enzyme With the Starch Granule. Front. Plant Sci. 12:704161. doi: 10.3389/fpls.2021.704161
Received: 01 May 2021; Accepted: 27 August 2021;
Published: 23 September 2021.
Edited by:
Diego F. Gomez-Casati, National University of Rosario, ArgentinaReviewed by:
Alberto A. Iglesias, CONICET Coastline Agrobiotechnology Institute (IAL), ArgentinaTracie Hennen-Bierwagen, Iowa State University, United States
Copyright © 2021 Gámez-Arjona and Mérida. This is an open-access article distributed under the terms of the Creative Commons Attribution License (CC BY). The use, distribution or reproduction in other forums is permitted, provided the original author(s) and the copyright owner(s) are credited and that the original publication in this journal is cited, in accordance with accepted academic practice. No use, distribution or reproduction is permitted which does not comply with these terms.
*Correspondence: Ángel Mérida, YW5nZWxAaWJ2Zi5jc2ljLmVz
†Present address: Francisco M. Gámez-Arjona, Institute of Molecular Plant Biology, ETH, Zürich, Switzerland