- 1Laboratory of Cotton Genetics, Genomics and Breeding/Joint Laboratory for International Cooperation in Crop Molecular Breeding, Ministry of Education/Beijing Key Laboratory of Crop Genetic Improvement, College of Agronomy and Biotechnology, China Agricultural University, Beijing, China
- 2Department of Ecology, Evolution and Organismal Biology, Iowa State University, Ames, IA, United States
Wild cotton species can contribute to a valuable gene pool for genetic improvement, such as genes related to salt tolerance. However, reproductive isolation of different species poses an obstacle to produce hybrids through conventional breeding. Protoplast fusion technology for somatic cell hybridization provides an opportunity for genetic manipulation and targeting of agronomic traits. Transcriptome sequencing analysis of callus under salt stress is conducive to study salt tolerance genes. In this study, calli were induced to provide materials for extracting protoplasts and also for screening salt tolerance genes. Calli were successfully induced from leaves of Gossypium sturtianum (C1 genome) and hypocotyls of G. raimondii (D5 genome), and embryogenic calli of G. sturtianum and G. raimondii were induced on a differentiation medium with different concentrations of 2, 4-D, KT, and IBA, respectively. In addition, embryogenic calli were also induced successfully from G. raimondii through suspension cultivation. Transcriptome sequencing analysis was performed on the calli of G. raimondii and G. sturtianum, which were treated with 200 mM NaCl at 0, 6, 12, 24, and 48 h, and a total of 12,524 genes were detected with different expression patterns under salt stress. Functional analysis showed that 3,482 genes, which were differentially expressed in calli of G. raimondii and G. sturtianum, were associated with biological processes of nucleic acid binding, plant hormone (such as ABA) biosynthesis, and signal transduction. We demonstrated that DEGs or TFs which related to ABA metabolism were involved in the response to salt stress, including xanthoxin dehydrogenase genes (ABA2), sucrose non-fermenting 1-related protein kinases (SnRK2), NAM, ATAT1/2, and CUC2 transcription factors (NAC), and WRKY class of zinc-finger proteins (WRKY). This research has successfully induced calli from two diploid cotton species and revealed new genes responding to salt stress in callus tissue, which will lay the foundation for protoplast fusion for further understanding of salt stress responses in cotton.
Background
Cotton (genus Gossypium) is an agronomically important crop plant consisting of four independently domesticated species (i.e., G. hirsutum, G. barbadense, G. herbaceum, and G. arboreum) that collectively dominate the natural fiber market. In addition to these important crop species, the genus include over 50 additional species, most of which produce some degree of fiber (Wang et al., 2018). While these species may represent a collective source of fiber improvement genes, wild species also comprise a natural source of resistance to biotic and abiotic stresses, male sterility, and improvement of fiber strength (Fryxell et al., 1992; Grover et al., 2012, 2015; Li et al., 2014; Huang et al., 2017; Wang et al., 2018). Introgression between wild and domesticated cotton is not always straightforward, however, as mating barriers exist among numerous interspecific crosses (Rebeca et al., 2018).
Somatic embryogenesis (SE) and protoplast hybridization are biotechniques used in agriculture to facilitate the exchange of elite and/or desired alleles among species (Li et al., 1991; Przetakiewicz et al., 2003; Navrátilová, 2004; Sakhanokho and Rajasekaran, 2016), including cotton (Wang et al., 2008). In general, protoplasts can be isolated from different explants, such as leaves, hypocotyls, young roots, embryogenic callus, etc. Among them, callus culture is not restricted by geographical and environmental conditions in wild cotton species, and callus can be proliferated continuous and obtained numerous materials for protoplast extraction. Somatic embryogenesis is a process by which plants are regenerated from non-germline tissue, either directly (clonal) or indirectly through the formation of a callus. Plant calluses (or calli) are growing masses of cells that may be derived from various explants, including leaves, hypocotyls, young roots, etc. Embryogenic callus induction (subsequent to the protoplast formation/fusion) is a complex multifactorial system that can be affected by both the endogenous hormones and the exogenous plant growth regulators (PGRs) used to facilitate the callus formation (Nascimento-Gavioli et al., 2017). Therefore, our ability to induce and to successfully develop embryonic calluses for a diversity of species comprises key steps in generating interspecific hybrids through protoplast fusion/plant regeneration (Sun et al., 2005).
Cotton species are known to be difficult for regenerating plantlets, due to the length of the procedure and the variety of limitations encountered (Xu et al., 2013). Although SE and plant regeneration in cultivated G. hirsutum and G. barbadense have been successful (Xu et al., 2013; Yang et al., 2014; Zheng et al., 2014; Liu et al., 2017; Pandeya et al., 2017; Wei et al., 2019), the conditions necessary to culture and regenerate wild cotton have been explored for few species, i.e., G. davidsonii, G. klotzschianum, G. stocksii, G. nelsonii, G. gossypioides, and G. tomentosum (Tan and Qian, 1988; Sun et al., 2003, 2006; Yan et al., 2010; Zhang et al., 2010; Sun and Hua, 2020).
Previous reports have shown that the success of cotton embryogenesis callus induction may be determined by genotype (Yang and Zhang, 2010), status of explants (Trolinder and Goodin, 1987; Trolinder and Xhixian, 1989), and media constituents (Trolinder and Goodin, 1988), including plant growth regulators (PGRs) (Sun et al., 2003; Sakhanokho et al., 2004; Ree and Guerra, 2015), nitrogen sources (Cangahuala-Inocente et al., 2014), and inorganic ions (Kumar et al., 2015; Cheng et al., 2016). In general, glucose was the best carbon source used to induce embryogenesis and callus formation, vastly outperforming sucrose (Babbar and Gupta, 1986). Although 2,4-dichlorophenoxyacetic acid (2,4-D, a synthetic auxin) and kinetin (KT) could induce tissue cultures, the application of little to no 2,4-D was better for embryogenic culture production (Sun et al., 2003).
Salt stress is one of the most serious abiotic stresses, capable of causing ion toxicity, oxidative damage, and water deficits through the destruction of ion and osmotic homeostasis, which further affect photosynthesis, metabolic processes, and cellular structures in plants (Gill and Tuteja, 2010; Juknys et al., 2012). Although cotton is known for salt tolerance, increasing salt stress due to climate change and agricultural practices inhibits cotton growth and decreases the yield. The completion of genome sequencing in G. raimondii (Paterson et al., 2012; Wang et al., 2012, 2020; Udall et al., 2019), G. arboreum (Li et al., 2014; Huang et al., 2020), G. hirsutum (Li et al., 2015; Zhang et al., 2015; Hu et al., 2019; Wang et al., 2019; Huang et al., 2020), and G. barbadense (Liu et al., 2015; Yuan et al., 2015; Hu et al., 2019; Wang et al., 2019) offers new resources to explore salt stress responses in cotton. Recent research has revealed that transcription factors, such as WRKY, NAC, ERF, and DREB, are critical to salt tolerance. These transcription factors are thought to regulate the expression patterns of downstream genes and further influence the level of salt tolerance in plants. In addition, dynamic changes under salt stress of hormone signaling, such as abscisic acid (ABA), jasmonate (JA), gibberellic acid (GA), ethylene, and brassinosteroid (BR), also regulate the transcriptional level of stress response genes in plants (Deinlein et al., 2014). Overexpression or knockdown of these genes, such as CBL-interacting protein kinase gene (CIPK6) and SnRK2.6a, could improve salt tolerance in transgenic cotton (He et al., 2013; Su et al., 2017b, 2020; Guo et al., 2019).
Wild cotton species contain many excellent agronomic traits, including their response to salt stress (Wei et al., 2017; Guo et al., 2019). Phenotypic analyses of salt-stressed plants, for example, have identified wild diploid cotton species with better salt tolerance than cultivated accessions (Dong et al., 2020), which makes introgression of that trait into cultivated cotton desirable; however, natural barriers to hybridization reduce our ability to introgress traits such as salt tolerance. Methods such as protoplast fusion and somatic embryogenesis are suitable options for breeding programs involving wild cotton species, although these are reliant on the establishing methods for somatic embryogenesis for each cotton species.
Here, we describe the first set of successful methods for inducing embryogenic calli in G. sturtianum and G. raimondii. Calli were obtained from the leaves of G. sturtianum and hypocotyls of G. raimondii on MSB5 medium (Murashige and Skoog + B5 vitamins) supplied with 0.10 mg·L−1 2, 4-D (synthetic auxin), 0.10 mg·L−1 KT (kinetin), and 0.10 mg·L−1 IAA (auxin) or 0.60 mg·L−1 2, 4-D and 0.25 mg·L−1 KT, respectively. In addition, we performed transcriptomic analysis of calli over 48 h (0, 6, 12, 24, and 48) in response to 200 mM NaCl stress to evaluate whether salt stress responses in calli were similar to the patterns reported in whole plants, thereby bypassing the need to grow the entire plant prior to abiotic stress screening. In general, we found that, as previously reported for cotton, salt stress resulted in differential gene expression of many transcription factors and ABA-related genes for both G. sturtianum and G. raimondii. Our findings lay the foundation for establishing an efficient protocol to prepare materials for protoplast fusion and somatic hybridization and provide valuable information for cotton salt tolerance and abiotic stress research using calli that may be useful in germplasm improvement and cotton breeding.
Materials and Methods
Plant Materials, Callus Induction, and Proliferation
The plant materials in this study were two diploid wild cotton species, G. sturtianum (C1) and G. raimondii (D5, Paterson et al., 2012; Wang et al., 2012; Udall et al., 2019). Seeds were delinted and surface-sterilized with 70% (w/v) alcohol for 30 sec, then immersed in 0.1% (w/v) HgCl2 for 10 min followed by five rinses with sterile ddH2O. The sterilized cotton seeds were germinated and grown on 1/2 MS (Murashige and Skoog, pH 5.9) with 7.5 g·L−1 agar and 20 g·L−1 sucrose in the dark for 7 days at 28 ± 2°C. Hypocotyls and leaves of the seedlings were cut into sections (0.5–1.0 cm each) or small pieces (1 cm2) as explants for callus induction.
The callus induction medium for both species was MSB5, i.e., MS medium plus B5 vitamins (Lommen et al., 2017), supplemented with additional plant hormones and growth regulators. For G. sturtianum, the MSB5 media were supplemented with 30 g/L glucose, 2.7 g·L−1 phytagel, 0.1 mg·L−1 2,4-D, 0.1 mg·L−1 KT, and 0.10 mg·L−1 IAA. For G. raimondii, the hormone concentrations suitable for initiating the callus formation were 0.60 mg·L−1 2,4-D and 0.25 mg·L−1 KT. In both species, calli were cultured at 28 ± 2°C with a 16-h photoperiod. After 4 weeks of growth in the induction media, fresh, loose, and yellow-green non-embryogenic calli were transferred into MSB5 medium supplemented with different ratios of 2,4-D and KT.
Embryogenic Callus Induction and Maintenance
The calli of G. sturtianum and G. raimondii were transferred to media containing 3% (w/v) sugar (glucose or sucrose) for three to four subcultures (one per month). Different PGRs and nitrogen sources were added to the medium to induce embryogenic callus formation in G. sturtianum (Table 1, Supplementary Table 1) and G. raimondii (Supplementary Table 2). Potential embryogenic calli of G. sturtianum and G. raimondii were selected and transferred into liquid media, which was identical to the solid MSB5 media for each species, but without the phytagel and with the addition of 1.00 g·L−1 glutamine and 0.50 g·L−1 asparagine (Supplementary Table 3). For each species, about 1–2 g of friable non-embryogenic calli was selected for liquid culture (flasks with 40 ml liquid media), which were shaken at 120 rpm under a 16/8-h light/dark cycle at 28°C. The larger cell masses were removed every 1–2 days, and the culture media were replaced every 7 days.
Salt Stress Treatments, Sampling, and RNA Sequencing
The resulting embryogenic calluses of G. raimondii and G. sturtianum were treated with 200 mM NaCl after they were grown in culture for 21 days. Three biological replicates were harvested for each sample at various timepoints (0, 6, 12, 24, and 48 h) after exposure to salt stress. Total RNA of each sample was isolated using a modified CTAB method according to the instruction manual (Zhao et al., 2012), and 1 μg RNA per sample was used as input material for cDNA synthesis. The first-strand cDNA synthesis was accomplished using random hexamer primers and M-MuLV Reverse Transcriptase (RNase H−); then the second-strand synthesis was accomplished using DNA polymerase I and RNase H to generate the final cDNAs. These cDNAs were subjected to end-repair/dA-tail (DNA A-Tailing Kit, TaKaRa) and adaptor ligation, and the resulting libraries were sequenced on the Illumina Novaseq platform as 150-bp paired-end reads with 6 × depth.
Quality Control, Mapping, and Quantification of Gene Expression Levels
A total of 1,631 million raw reads (accession number: PRJNA736855) were first processed through in-house Perl scripts (Supplementary Table 4), which removed adapter sequences, poly-N reads, and low-quality reads from raw data. The resulting clean reads were mapped to the cotton genome (G. raimondii, Paterson et al., 2012) using Tophat2.2.0 (Kim et al., 2013), resulting in ~97% and 78–83% of the clean reads per sample successfully mapping to the G. raimondii reference genome from the G. raimondii and G. sturtianum samples, respectively, 94% and 76–80% of which were uniquely mapping (Supplementary Table 5). FPKM (fragments per kilobase of exon per million fragments mapped) was calculated for each gene using Cufflinks (Kim et al., 2013). Correlation coefficient and principal component analysis (PCA) of the gene expression showed that three biological replicates of each sample have good reproducibility and high reliability, indicating that these sequencing data could be used for differential gene expression (Supplementary Figures 1A,B).
Gene Differential Expression and Functional Analysis
Differential expression analysis of two groups (three biological replicates per condition) was performed using the DESeq2 R package (1.20.0). Only genes with an absolute value of log2 ratio ≥ 1 and FDR significance score <0.01 were considered differentially expression genes (DEGs). Gene ontology (GO) annotation of DEGs was obtained via the Blast2GO program (Götz et al., 2008), and subsequent visualization, comparisons, and plotting of GO annotations were performed using OmicShare tools, a free online platform for data analysis (http://www.omicshare.com/tools). The output of enrichment was limited to FDR < 0.05. KEGG pathway analysis was performed through alignment against KEGG (https://www.genome.jp/kegg/).
Quantitative Real-Time PCR
Quantitative real-time PCR was performed using SYBR Green PCR Master mix according to the manufacturer's instructions. The constitutively expressed gene GhUBQ7 was used for the normalization of gene expression (Nie et al., 2018). All reactions were run three times, and the average number of threshold cycle (Ct) values was produced automatically by the qRT-PCR amplifier. The relative gene expression of each transcript was normalized against the reference gene GhUBQ7 using the 2−ΔΔCt method (Livak and Schmittgen, 2001).
Results
Embryogenic Callus Induction and Proliferation
Calli of leaves in G. sturtianum were induced on MS basal medium supplemented with 0.10 mg·L−1 2, 4-D, 0.10 mg·L−1 KT, and 0.10 mg·L−1 IAA, whereas G. raimondii had slightly different induction conditions (i.e., MS basal medium with 0.60 mg·L−1 2, 4-D, 0.25 mg·L−1 KT). Notably, the colors and textures of the calli from each species were different. Hypocotyls were used for G. raimondii, which produced a greater number and more friable calli than the cotyledons used for G. sturtianum. The phenotypic characteristics of these calli are shown in Figures 1A,B. While the original calli for both G. sturtianum and G. raimondii were loose, slight color differences were observable (i.e., yellow-green vs. light yellow, for G. sturtianum and G. raimondii, respectively).
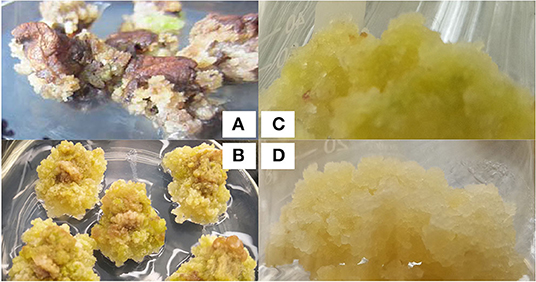
Figure 1. Embryogenic callus induction in two diploid wild cotton species. (A) Callus induced from G. sturtianum, (B) callus induced from G. raimondii. (C) embryogenic callus induced from G. sturtianum, (D) embryogenic callus induced from G. raimondii.
Embryogenic calli induction also required different conditions for each species. Callus proliferation progressed rapidly during continuous subculture, becoming friable after 3–4 months and with a tendency to differentiate embryogenic callus. Calli from G. sturtianum were light yellow and loose under N or C starvation conditions, and these were selected for subculture on media lacking either carbon or nitrogen sources to induce embryogenic callus formation. Although G. sturtianum calli were gray under nitrogen deficiency, they grew rapidly, particularly in N1 and N3 media (Supplementary Table 1); however, when grown in media lacking a carbon source, calli were green and slow to proliferate, indicating that embryogenic callus induction was more successful under nitrogen deficiency. These calli were subsequently transferred to either N4, N5, N6, or N7 medium (Supplementary Table 1), all of which typically produced light-yellow embryogenic calli (Figure 1C). In contrast, G. raimondii embryogenic calli were most successfully induced under a double concentration of KNO3, with a 2–3 ratio of IBA/KT as most appropriate (Figure 1D). As expected, sucrose was better than glucose at promoting the formation of embryogenic calli for G. raimondii (see Supplementary Table 2 for the effects of each media combination on the induction of embryogenic callus).
Successful retrieval of EC was different for each species. Throughout a month-long suspension culture, the calli and liquid media of G. raimondii remained relatively bright, with smaller cell masses having uniform size and synchronous growth (Supplementary Figure 2A). Subsequent transfer to a solid form of the same media allowed the suspension culture cell masses to gradually develop into brightly colored embryogenic calli with a loose texture (Supplementary Figure 2B). Conversely, the liquid media for G. sturtianum calli induction tended to oxidize, resulting in quick browning, with most calli dying after transfer to solid medium (Supplementary Figures 2C,D), suggesting further modifications to the G. sturtianum EC induction media were necessary.
Critical Factors Affecting Proliferation and Differentiation of G. sturtianum Embryogenic Calli
Because different plant growth regulator (PGR) combinations are important for the proliferation and differentiation of embryogenic calli (Sakhanokho et al., 2004; Ge et al., 2017), optimization is often required for EC induction and proliferation. In the initial EC induction, we found that G. sturtianum did not require a nitrogen source (here, NH4NO3; Table 1), but that the addition of IBA was conducive to the proliferation of the embryonic callus at an optimal IBA/KT ratio of 3 (on MSB5 media; Figure 2I). These results suggest that uncoordinated proportions of hormones can easily lead to the reversal of EC into non-embryonic calli.
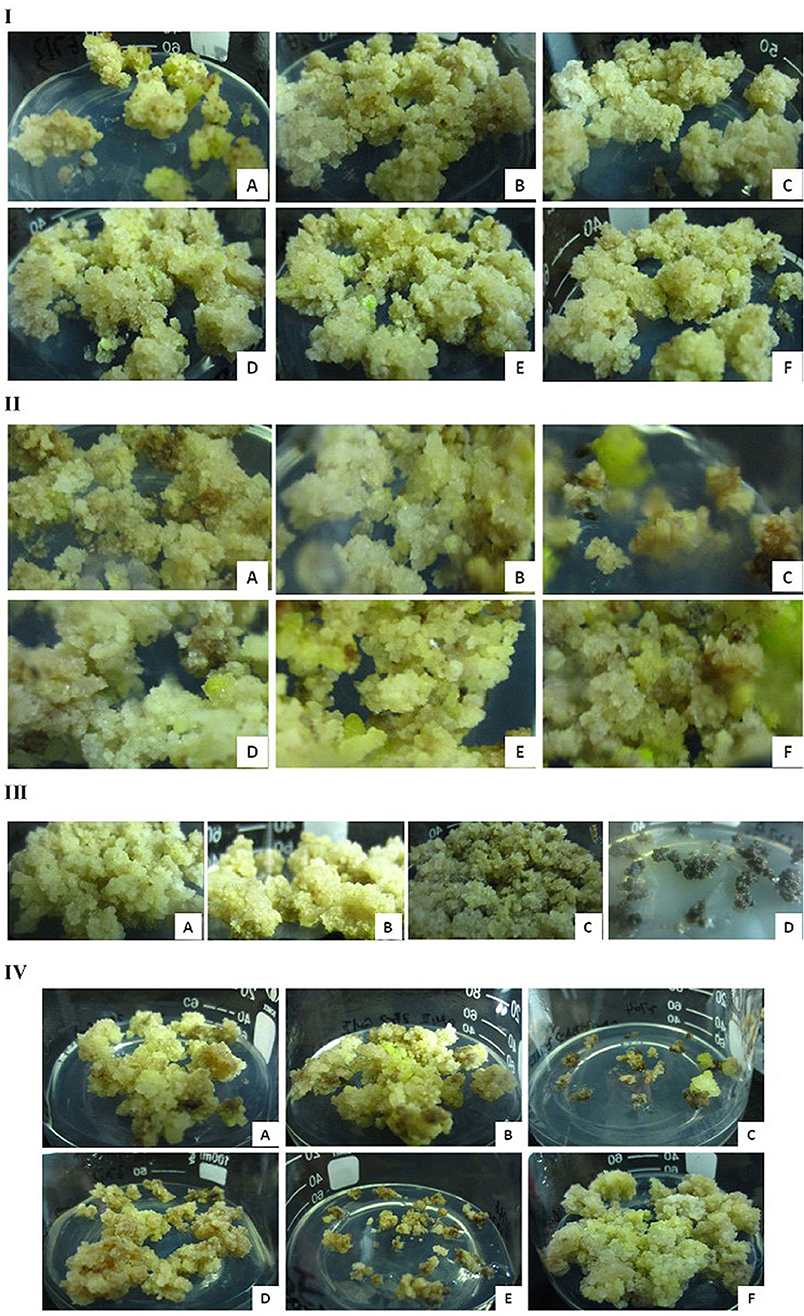
Figure 2. Embryogenic callus initiation and proliferation of G. sturtianum. (I) IBA and KT, (A–F) EI1-EI6 corresponding to Table 1; (II) nitrogen source, (A–C) C1, C2, C3 corresponding to Supplementary Table 6 (D–F) EI7, E1, EI9 corresponding to Table 1 and Supplementary Table 6; (III) FeSO4, (A–D) C1, E4, E6, E7 corresponding to Supplementary Table 7; (IV) CuSO4, (A,B) C1 corresponding to Supplementary Table 7; (C–F) E7–E10 corresponding to Supplementary Table 7.
To further refine our understanding of the nutrient and hormone requirements for G. sturtianum embryonic calli induction, we evaluated EC induction on the basic media containing different concentration gradients of KNO3 and supplemented with different PGRs combinations (Supplementary Table 6, Figure 2II). High concentrations of KNO3 combined with low concentrations of PGRs resulted in embryogenic calli that subsequently turned brown and died (Figure 2II). When the PGR concentrations were doubled, the inhibition associated with high concentrations of KNO3 was eliminated; however, the resulting calli exhibited no embryogenicity. Only those calli that were grown in media containing 7.60 g·L−1 KNO3 with lower concentrations of KT and with the addition of IBA resulted in embryogenic calli, suggesting that IBA may facilitate EC proliferation.
The concentrations of FeSO4 and CuSO4 also greatly affected the growth rate and yield of embryonic calli in G. sturtianum. Most of the calli in media containing 1-2x concentrations of FeSO4 (E4) resulted in EC that were light-yellow and soft with fast proliferation (Figure 2III), suggesting that these FeSO4 concentrations (optimally 1.5–2.0x) promote embryonic callus proliferation and differentiation (Supplementary Table 7). Notably, low concentrations of FeSO4 resulted in callus death. Conversely, both high and zero concentrations of CuSO4 performed non-optimally. Calli grown on media with high CuSO4 (both with and without 2,4-D; Supplementary Table 7) either rapidly died or were brown and slow to proliferate, remaining non-embryogenic and ultimately becoming dying (Figure 2IV). Calli grown in the absence of CuSO4 were equally unusable, either rapidly dying (if 2,4-D was excluded from the media) or proliferating well but remaining non-embryogenic (in the presence of 2,4 D; Supplementary Table 7). Together, these results suggest that a slight elevation of FeSO4 and a standard concentration of CuSO4 are suitable for EC generation.
Characterization of EC DEGs in Response to Salt Stress
Generation of EC is often promoted to protoplast fusion to generate hybrids between species/accessions that have natural reproductive barriers, which is particularly useful in agricultural breeding programs. Accordingly, it may be useful to understand whether responses to stress can be characterized in the calli themselves. Here, we explore the gene expression patterns of calli in G. sturtianum and G. raimondii under salt stress to determine if the abiotic stress response in calli is similar to that previously described for cotton plants. Toward this end, transcriptome sequencing was performed on calli treated with 200 mM NaCl and sampled at 0, 6, 12, 24, and 48 h, respectively; the direction of regulation (i.e., upregulated or downregulated) here refers to the comparison with 0-h salt treatment. Differential gene expression was detected in 5,522 genes in G. raimondii and 10,484 genes in G. sturtianum, with the majority of DEG exhibiting expression changes during the first two timepoints. The number of DEG peaked at 12 h post-exposure (3,552 in G. raimondii and 6,917 in G. sturtianum), subsequently returning to the lower levels. These observations suggest that the initial timepoints after salt stress are critical for the calli to respond to stress. This changing trend is similar to the DEGs in roots and leaves in cotton under salt stress (Su et al., 2020). It is also worth noting that the number of downregulated DEGs was greater than that of the upregulated DEGs in each salt stress period for both species, indicating that most genes are negatively regulated in response to salt stress (Figure 3A; Supplementary Figure 1C).
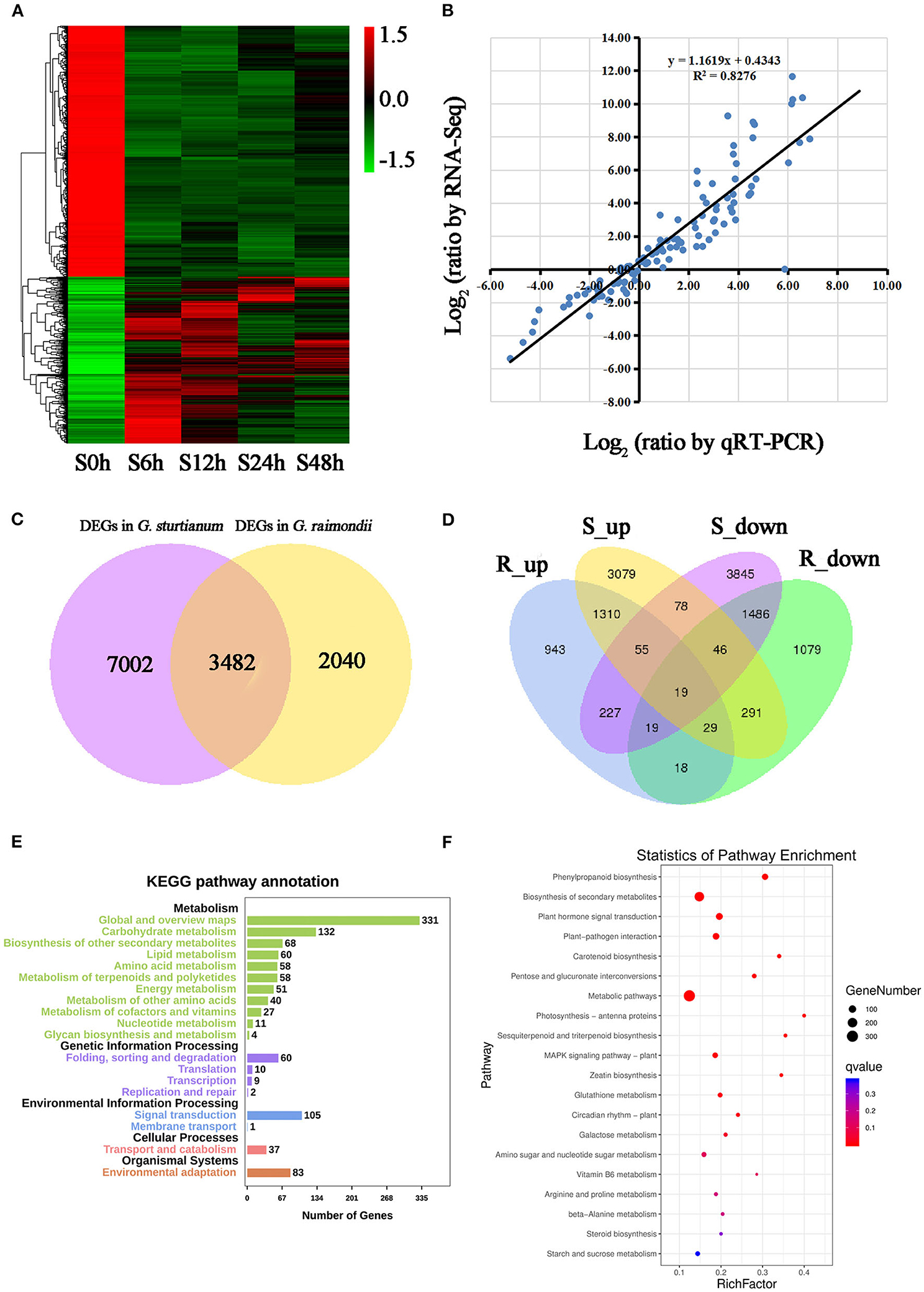
Figure 3. Identification and functional analysis of differently expressed genes during different stages under salt stress in G. raimondii and G. sturtianum; (A) Heatmap of differential expression during diverse phases under salt stress in G. sturtianum; (B) Correlation analysis of gene expression profiles between RNA-Seq and qRT-PCR; (C) Venn diagrams of all DEGs of G. raimondii and G. sturtianum; (D) Venn diagrams of upregulated and downregulated DEGs of G. raimondii and G. sturtianum; (E,F) KEGG enrichment analysis of common DEGs in G. raimondii and G. sturtianum. S0h, Callus of G. sturtianum under salt stress for 0 h; S6h, Callus of G. sturtianum under salt stress for 6 h; S12h, Callus of G. sturtianum under salt stress for 12 h; S24h, Callus of G. sturtianum under salt stress for 24 h; S48h, Callus of G. sturtianum under salt stress for 48 h (both here and below). R_up, Upregulated genes under salt stress in G. raimondii; R_down, Downregulated genes under salt stress in G. raimondii; S_up, Upregulated genes under salt stress in G. sturtianum; S_down, Downregulated genes under salt stress in G. sturtianum.
Among the 5,522 DEGs of G. raimondii, 850 were differentially expressed during all four periods after salt stress (Supplementary Figure 3A), and there were 508, 531, 418, and 420 genes that were specifically differently expressed at the stage of salt stress 6, 12, 24, and 48 h, respectively. Similarly, among the 10,484 DEGs of G. sturtianum, 1,207, 694, 646, and 716 genes were specifically differentially expressed at the 6, 12, 24, and 48 h after salt stress, respectively, with 2,583 common DEGs in each stage (Figure 3A; Supplementary Figure 1D). In order to validate the reliability of RNA sequencing, qRT-PCR was carried out to detect the expression patterns of DEGs in different samples. Fourteen genes were randomly selected (Supplementary Table 8), whose abundance confirms the general agreement between quantitative RT-PCR measurements and estimates of abundance from transcriptome sequencing (R2 = 0.8276; Figure 3B; Supplementary Figure 3B).
In total, 12,524 DEGs were collectively detected in G. sturtianum and G. raimondii during salt stress. From this pool of DEGs, 3,482 were identified as differentially expressed in both species (Figure 3C), with 1,310 upregulated in both and 1,486 downregulated in both (Figure 3D). Functional annotation of these shared DEGs (based on GO ontology) identified GO categories for 2,552 genes that mainly included the categories Biological Process, Molecular Function, and Cellular Component and included 54 secondary GO functional classifications (Supplementary Figure 3C). We also employed the KEGG pathway analysis to predict important metabolic processes and networks operating during salt stress in cotton calli. Fewer shared DEGs (1,147) were annotated by KEGG, which were mainly divided into five categories: metabolism, genetic information processing, environmental information processing, cellular processes, and organismal systems (Figure 3E). Most (73.23%) of the genes were involved in metabolic pathways, and they were mainly enriched in the subcategories metabolic pathways (310), biosynthesis of secondary metabolites (201), plant–pathogen interaction (69), and glutathione metabolism (65). In addition, the biosynthetic pathways of some hormones, such as carotenoid biosynthesis pathways related to abscisic acid synthesis (16), cysteine and methionine metabolic pathways related to ethylene synthesis (cysteine and methionine metabolism, 20), zeatin biosynthesis related to cytokinin synthesis (zeatin biosynthesis, 10), and one pathway for “plant hormone signal transduction” (78) (Figure 3F) were also prominent salt stress response DEGs. The combined results of GO and KEGG enrichment analysis suggest that genes related to hormone signal transduction and nucleic acid binding were significantly affected under salt stress in cotton callus. These results were consistent with previous research, which identified salt tolerance genes in cotton roots and leaves (Rodriguez-Uribe et al., 2011; Shi et al., 2015).
Abscisic Acid Metabolic Pathway Is Very Important to Respond to Salt Stress in Cotton Callus
Abscisic acid is a critical regulatory factor in response to abiotic stress in plants; large numbers of studies have proved that ABA metabolism participates in response to salt stress in plants, such as in roots and leaves (Gong et al., 2020; Su et al., 2020). In our study, lots of common DEGs shared by G. sturtianum and G. raimondii is significantly enriched in the ABA synthesis and signal transduction pathway. There were 12 DEGs enriched in the carotenoid synthesis pathway, which related to abscisic acid synthesis (Table 2), including eight xanthoxin dehydrogenase genes (ABA2), two 9-cis-epoxycarotenoid dioxygenase genes (NCED), and two abscisic acid 8′-hydroxylase genes (CYP707A). Most of the ABA2 genes showed similar expression patterns in the two cotton species, with a trend of first up and then down after salt stress; these genes include Gorai.002G236800, Gorai.002G237000, Gorai.002G237200, Gorai.002G237300, Gorai.002G237400, Gorai.006G151500, and Gorai.006G151900 (Figures 4A,B). Two NCED genes (Gorai.002G038100 and Gorai.004G270800) were upregulated under salt stress and the abundance grew to the peak at 6 h and 12 h after salt stress, then gradually decreased (Figures 4A,B). Interesting, the expression abundance of two CYP707A genes (Gorai.004G177200 and Gorai.008G218900), which are mainly involved in the ABA decomposition process, was downregulated after salt stress (Figures 4A,B).
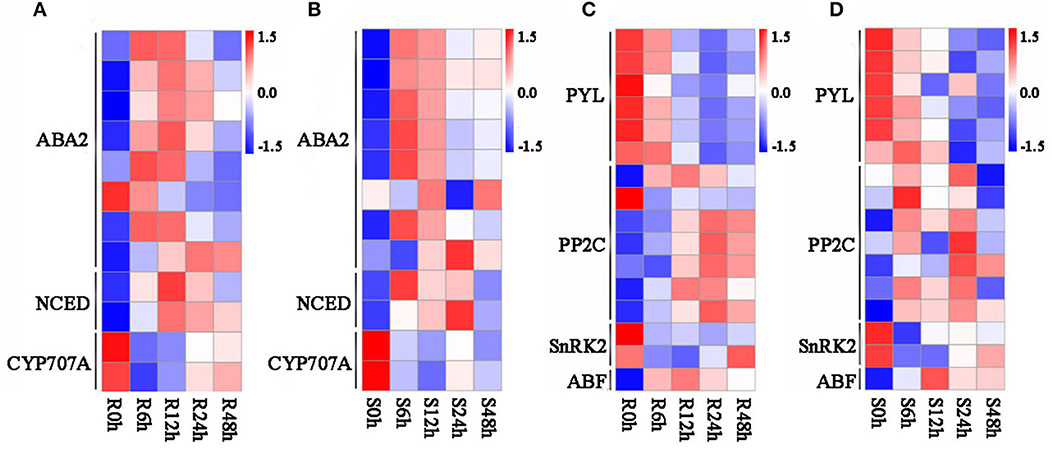
Figure 4. Expression pattern of genes related to ABA biosynthesis and signal transduction. (A) Expression pattern of genes related to ABA biosynthesis in G. raimondii; (B) expression pattern of genes related to ABA biosynthesis in G. sturtianum; (C) expression pattern of genes related to ABA signal transduction in G. raimondii; (D) expression pattern of genes related to ABA signal transduction in G. sturtianum. R0h, Callus of G. raimondii under salt stress for 0 h; R6h, Callus of G. raimondii under salt stress for 6 h; R12h, Callus of G. raimondii under salt stress for 12 h; R24h, Callus of G. raimondii under salt stress for 24 h; R48h, Callus of G. raimondii under salt stress for 48 h (both here and below).
Another 16 DEGs, including six abscisic acid receptor PYR/PYL family genes (PYL), seven protein phosphatase 2C genes (PP2C), two SnRK2 genes, and one ABA-responsive element binding factor (ABF), were participated in the ABA signaling pathway (Table 2). Among them, six PYL genes (Gorai.002G266100, Gorai.006G185000, Gorai.007G031800, Gorai.009G323000, Gorai.010G194800, and Gorai.011G290300) showed a continuous downward regulation after salt stress in G. raimondii (Figure 4C). Interestingly, except for Gorai.011G290300 with the upregulated expression at 6 h of salt stress in G. sturtianum, other five PYL genes present down-regulated trends after salt stress in G. sturtianum same as in G. raimondii (Figure 4D). The expression pattern of seven PP2C genes showed the same trends at different stages after salt stress in G. sturtianum, and all of them are upregulated in both 6- and 24-h periods and downregulated at 12 and 48 h. However, they displayed different expression patterns in two cotton species (Figure 4D). SnRK2 gene Gorai.011G121900 was downregulated first and then upregulated gradually after 12 h in both cotton species, while the expression abundance of another SnRK2 gene Gorai.002G006900 presented continuous downregulation after salt stress (Figures 4C,D).
The MAPK (mitogen-activated protein kinase) signal transduction pathway is conservative and important to stress response, and transgenic technology has proved that MAPK is critical to salt tolerance in many species (Kiegerl et al., 2000; Shi et al., 2009; Su et al., 2020). Among the 3,482 DEGs in callus, 39 ones are related to the MAPK signal pathway, including two MAPKK protein kinases, seven WRKY/ERF transcription factors, three ERF1 genes, and two WRKY genes (Supplementary Table 9). MKK2 gene Gorai.001G013600 was upregulated after salt stress, maybe positively correlated with a salt stress response. The MAPK signaling pathway interacts with the ABA signaling pathway, and ABA could activate the MAPK signaling pathway by acting as an upstream signaling molecule (Supplementary Figure 4). In this study, 15 DEGs were involved in the ABA-mediated MAPK signaling pathway, which were consistent with the genes involved in the ABA signaling pathway in the above mentioned. These results indicated that salt-tolerance genes related to MAPK signal pathway also participate in ABA signal transduction in cotton callus, this result has been confirmed in other tissues of the plants (Xing et al., 2007; Lin et al., 2017; Su et al., 2020).
Numerous TFs Are Responsive to Salt Stress in Cotton Callus
Many of the TF families were shown to be responsive to salt stress, and most of these might enhance salt tolerance through ABA signaling. Members of ERF, bHLH, MYB, bZIP, and C2H2 families are downregulated under salt tolerance in G. raimondii (Figure 5A). However, most of the differentially expressed TFs in G. sturtianum, such as ERF, bHLH, MYB, WRKY, and NAC, are upregulated after salt stress (Figure 5B). These results indicate that transcription factor responses to salt stress have species and spatiotemporal specificity.
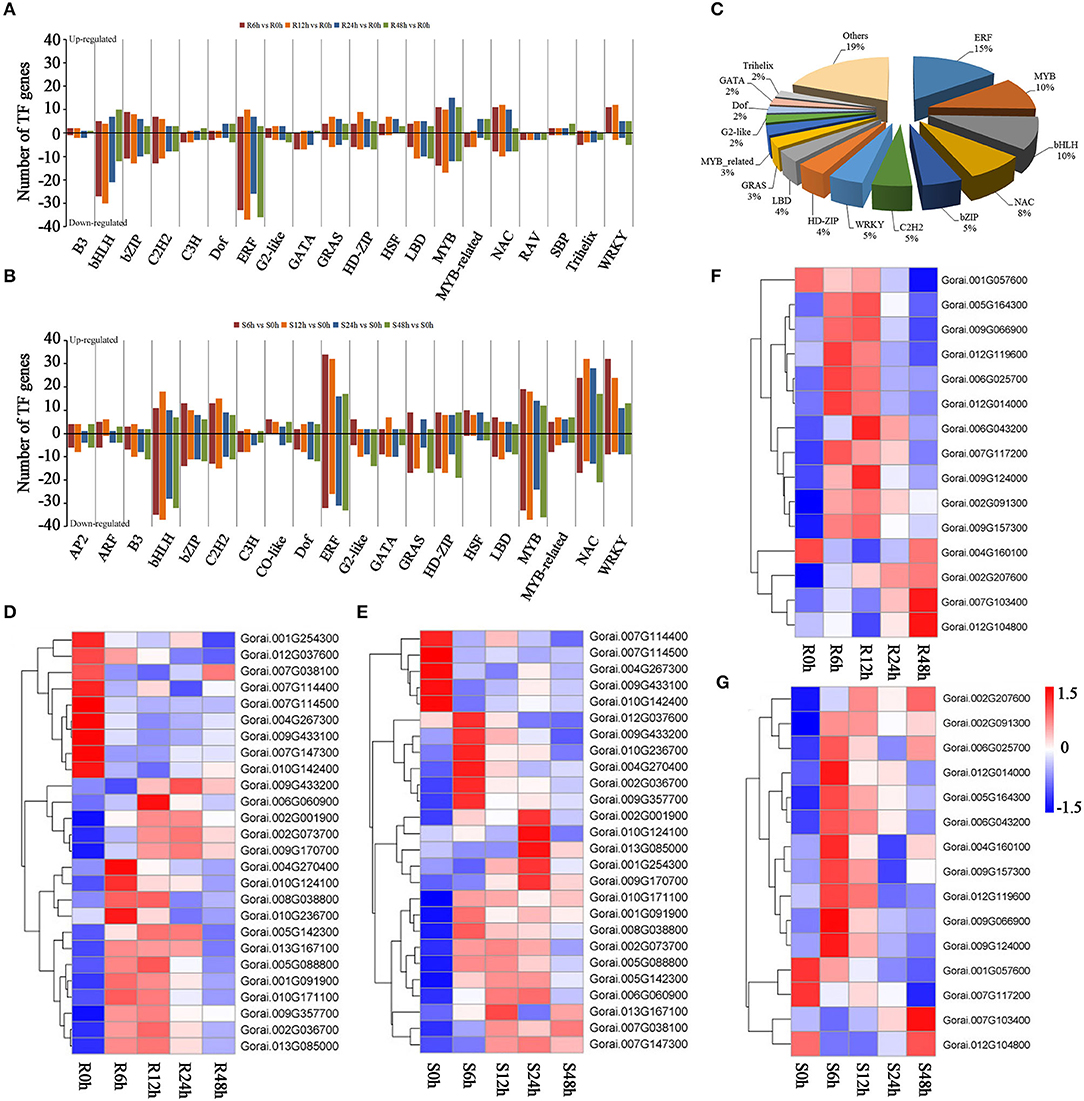
Figure 5. Transcription factors involved in salt tolerance. (A) Enrichment analysis of differentially expressed transcription factors in G. raimondii under salt stress; (B) enrichment analysis of differentially expressed transcription factors in G. sturtianum under salt stress; (C) statistics of common transcription factors in G. raimondii and G. sturtianum; (D) expression pattern of NAC TFs in G. raimondii; (E) expression pattern of NAC TFs in G. sturtianum; (F) expression pattern of WRKY TFs in G. raimondii; (G) expression pattern of WRKY TFs in G. sturtianum.
Of the 3,482 DEGs, 327 TFs were shared by G. raimondii and G. sturtianum, belonging to 40 TF families. Among these, ERF, MYB, bHLH, NAC, bZIP, C2H2, and WRKY families account for a large proportion (Figure 5C), suggesting that these transcription factors may play a role in salt stress response. In our study, 26 and 16 DEGs were annotated in the NAC and WRKY families, respectively, most upregulated after salt stress (Figures 5D–G). Previous research has shown that members of the NAC family are involved in ABA signaling (Chen et al., 2014). It is worth noting that there were 17 NAC family genes that were upregulated in G. raimondii (Figure 5D), and 21 genes were upregulated in G. sturtianum (Figure 5E). In addition, some NAC genes, such as Gorai.001G091900, Gorai.002G036700, Gorai.004G270400, Gorai.009G357700, Gorai.010G171100, and Gorai.009G170700, were both significantly upregulated in the two cotton varieties under salt stress. For the WRKY family, most genes were upregulated and expression was highest at 6 h or 12 h after salt stress in both G. raimondii and G. sturtianum (Figures 5F,G). The above results indicate that the members of the NAC and WRKY family are induced to be differentially expressed by salt stress, and their expression patterns are similar in the two cotton species, suggesting that they play a role in response to salt stress.
Discussion
Embryogenic Callus Induction Provides a Material Basis for Protoplast Fusion in Wild Cotton
There are many outstanding and valuable characteristics among wild species in cotton breeding programs; however, reproductive isolation among species is an obstacle to producing hybrid plants through conventional breeding. Therefore, it is essential to develop the resources for somatic cell regeneration of wild cotton species, which will lay the foundation for the creation of new germplasm resources through somatic hybridization of cultivated and wild cotton and subsequent regeneration. Protoplast fusion technology for somatic cell hybridization is an advantageous way to obtain interspecific hybrids, which operates through fused protoplasts derived from embryogenic calli and somatic embryos. A current limitation to this technology in cotton is that the growth and development of wild cotton species require specific environmental conditions, which make it difficult to breed these species and/or obtain a suitable number of materials for protoplast isolation. Therefore, our ability to cultivate and maintain calli from diverse wild cotton species will provide the materials necessary for protoplast separation and subsequent fusion. Presently, only a few reports related to embryogenesis callus and plant regeneration in wild cotton species are available (Sun et al., 2003, 2006; Yan et al., 2010), none of which are broadly applicable to other cotton species. Therefore, suitable induction systems for embryogenic calli must be individually established for different wild cotton species.
In general, differentiating embryogenic calli from non-embryogenic calli is challenging, and insufficient methods exist for most cotton species. The phenotypic characteristics of embryogenic calli from wild cotton species are similar to those found in upland cotton, i.e., they are friable, cream-colored, granular, and yellow-green (Sakhanokho et al., 2004; Zhang Y. et al., 2014). Here we used existing knowledge regarding EC induction in cotton and other plants to circumscribe a set of conditions with potential for EC induction in the wild cotton species G. sturtianum and G. raimondii. In general, we found that the required combinations of PGRs and nutrients were similar between G. sturtianum (0.10 mg·L−1 KT + 0.20 mg·L−1 2,4-D) and G. raimondii (0.60 mg·L−1 2, 4-D, 0.25 mg·L−1 KT), with deviations producing slower-growing, brown, or fatal results. It is clear that the concentration of 2,4-D plays a role in EC generation and that we need to gradually reduce the concentration of 2,4-D during subsequent subcultures. Explants were also found to influence the embryogenic calli production, with hypocotyls producing more friable calli than cotyledons, as previously noted (Chee et al., 1990). In addition, different concentrations of nitrogen sources supplemented in media also influence both viability and conversion from non-embryogenic to embryogenic calli (Loukanina and Thorpe, 2007).
Because alternating solid and liquid cultures can effectively shorten the time for embryogenic callus induction, as demonstrated in upland cotton (Rajasekaran, 1996), generating suitable growth conditions for this alternating procedure is advantageous for improving the pace of research and breeding. As with EC induction in general, however, it is difficult to induce embryogenic calli for diploid wild cotton in a solid-liquid alternate culture. Although we were able to improve the efficiency for embryogenic callus induction in G. raimondii based on suspension cultures, solid–liquid alternate cultures, and other methods, this strategy is not suitable for G. sturtianum embryogenic callus induction. In general, G. sturtianum EC induction was more challenging and further exploration is needed.
In Callus, DEGs Related to Salt Tolerance Also Played Key Roles in Whole Cotton Plants
At present, most studies about callus responses to salt stress focused on physiological indicator detection (Badawy et al., 2008), with few studies on the transcriptome of cotton callus in response to salt stress. Here, we obtained 5,522 and 10,484 genes that were differentially expressed by salt stress in G. raimondii and G. sturtianum, respectively. There were two reasons for much more DEGs in G. sturtianum than in G. raimondii; the first one is that the callus of G. sturtianum is more sensitive to salt stress than the callus in G. raimondii. Another reason is that the genome sequence of G. sturtianum (C1) has not been released; using the genome of G. raimondii (D5) as a reference for G. sturtianum RNA-Seq analysis will affect the analytical results. A total of 3,482 genes were differentially expressed under salt stress both in G. sturtianum and G. raimondii callus. These DEGs were enriched in the biological processes of cellular process, biosynthesis of secondary metabolites, plant hormone biosynthesis, signal transduction, and other metabolic pathways. These results imply the rapid response of secondary metabolites and plant hormones metabolism under salt stress, and a similar response mechanism also existed in cotton roots and leaves (Rodriguez-Uribe et al., 2011; Shi et al., 2015; Su et al., 2020). Furthermore, some transcription factor families such as NAC, WRKY, MYB, and a large number of genes that are implicated in ABA biosynthesis, ABA signaling transduction, and MAPK signaling pathway were also differentially expressed under salt stress in callus. These biological processes or TF families have been shown to play an important role in the response of whole cotton plants to salt stress (Golldack et al., 2011; Zhang et al., 2016; Wei et al., 2017; Su et al., 2020). G. aridum, G. davidsonii, and G. klotzschianum have strong salt tolerance, and the functional genes related to reactive oxygen species, plant hormones, and transcription factors were induced under salt stress in these species (Wei et al., 2017). Most of the differentially expressed genes isolated from G. aridum under salt stress were homologous to plant stress resistance genes, and 24% of them were related to metabolic processes. Overexpression of GarMSL and GarCYP in tobacco will increase the survival rate of transgenic plants under salt stress. GarWRKY5 participates in the salt stress response in G. aridum through a signaling pathway mediated by jasmonic acid or salicylic acid, and overexpressed GarWRKY5 in Arabidopsis enhances salt tolerance (Guo et al., 2019). It is worth noting that these genes or metabolism pathways were also identified in our research. The same salt stress response genes or metabolic pathways in callus suggest that calli might provide an effective screening tool that is relevant to salt stress in whole plants.
Most Genes Related to ABA Metabolism or TFs Are Important to Salt Tolerance Both in Cotton Plants and in Callus
Abscisic acid is a key regulatory factor in response to salt stress in plants (Moons, 1997; Gong et al., 2020). ABA2 and NCED are critical genes involved in the regulation of the abscisic acid biosynthesis pathway, and the CYP707A gene is a key enzyme for ABA catabolism. In maize plants, ZmABA2 was upregulated in leaves after 2 h of 250 mM NaCl treatment; overexpression of ZmABA2 in tobacco could increase ABA content and enhance the tolerance to salt stress (Ma et al., 2016). The expression abundance of OsNCED5 was significantly induced in roots and leaves when the rice is under high-salt conditions; overexpressed OsNCED5 in rice will increase the salt-tolerance (Huang et al., 2019). In addition, OsNCED3 and OsNCED4 had similar expression patterns with OsNCED5 under salt stress conditions (Huang et al., 2019). In our study, through treatment with 200 mM NaCl in cotton callus, we also identified 28 differentially expressed genes involved in the ABA synthesis pathway under salt stress, including seven ABA2 and two NCED genes upregulated under salt stress, and two CYP707A genes that were downregulated. These genes may respond to salt stress through the ABA pathway both in cotton plants and callus (Cheng et al., 2009; Barrero et al., 2010; Zheng et al., 2012).
When plants are subjected to salt stress, the content of ABA increases and binds to ABA receptor proteins (PYR/PYL/RCAR) to regulate the activity of PP2C. The activated PP2C genes directly interact with SnRK2 and transmit ABA signaling to the downstream AREB/ABF transcription factors, further mediating the expression patterns of salt stress-responsive genes (Wei et al., 2017). The results of transcriptome analysis of roots and leaves in G. klotzschianum showed that the PYL gene is significantly downregulated, and the PP2C and SnRK2 genes are significantly upregulated in salt stress (Wei et al., 2017), and similar results were demonstrated in our study in callus of cotton.
In our study, we identified only one MKK2 (MAPKK) gene and it was upregulated under salt stress in G. raimondii callus, but there were two MKK2, one MEKK1 (MAPKKK), and one MPK4 (MAPK) that were induced and upregulated under salt stress in G. sturtianum callus. MAPK is a mitogen-activated protein kinase that interacts with ABA2 and regulates ABA synthesis (Verma et al., 2020). In general, MAPK activates downstream elements such as transcription factors and causes related physiological and biochemical reactions in response to stress. Most of the MAPK genes could respond to salt stress in different tissues of plants. DSM1 (MAPKKK), OsMAPK44, and OsMAPK5 were upregulated under salt stress; overexpression of OsMAPK44 and OsMAPK5 in rice could increase the salt tolerance effectively (Xiong and Yang, 2003; Jeong et al., 2006; Ning et al., 2010). The transcriptional level of ZmMPK3 was significantly upregulated under high-salt conditions in maize seedlings (Wang et al., 2010). In addition, overexpression of ZmMPK5 in tobacco increased resistance to salt-alkali stress through scavenging excess reactive oxygen species (Zhang D. et al., 2014). GhMAPK, GhMPK2, and GhMPK4 play important roles in salt stress response in upland cotton (Wang et al., 2007, 2015; Zhang et al., 2011), and overexpression of GhMAP3K40 increases tolerance to salt stress during the germination period in cotton (Chen et al., 2015).
Transcription factors are critical to transfer stress signaling into the tolerance response genes. Multiple TFs such as bZIP, WRKY, ERF, MYB, bHLH, and NAC families are differentially expressed under salt stress (Golldack et al., 2011). Large numbers of transcription factors were annotated among the DEGs shared by two cotton species under salt stress in callus, including ERF, MYB, bHLH, NAC, bZIP, C2H2, and WRKY. Among them, members of the NAC and WRKY families occupy a large proportion, and most of these were upregulated under salt stress, indicating that these two TF families may positively regulate salt stress response in callus. Additional transcription factors may be also important in maintaining salt stress response. Overexpression of the GRAS transcription factor improves salt tolerance in Arabidopsis and yeast (Shi et al., 2015; Yuan et al., 2016; Zhang et al., 2020). OsDOF15 mediates ethylene biosynthesis in rice, and it is important in response to salt stress (Qin et al., 2019). Some GhDof genes are also differentially expressed in upland cotton under salt stress, and overexpression of GhDof1 in cotton increases salt tolerance compared to wild-type plants (Guo et al., 2015; Shi et al., 2015; Su et al., 2017a). WOX is an important transcriptional regulator involved in the development of plant embryos and is differentially expressed in abiotic stress conditions such as drought, salt, and cold. OsWOX3 and OsWOX5 are rapidly upregulated after treatment with 150 mM NaCl in rice (Cheng et al., 2014). We also identified a certain number of transcription factors such as Dof, GRAS, and WOX in callus under salt stress, suggesting that these TFs may also be important to salt tolerance in wild cotton not only in whole plants but also in callus.
Conclusion
Here, we demonstrate the successful induction of embryogenic callus from two wild diploid cotton species. We used these to perform the first comprehensive transcriptomic analysis of callus under salt stress, revealing numerous differentially expressed genes including TF families related to ABA biosynthesis and signal transduction, such as SnRK2, ABA2, NAC, and WRKY (Figure 6). Our study provides a foundation for plant regeneration through protoplast fusion and a useful resource for improving agronomically relevant salt resistance using beneficial genes from wild cotton species.
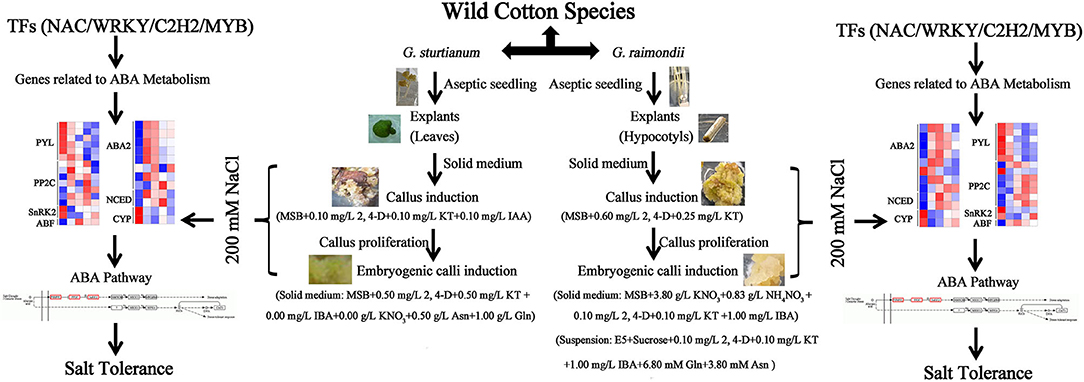
Figure 6. The mode of embryogenic callus induction and salt tolerance of two wild cotton species G. sturtianum and G. raimondii.
Data Availability Statement
The datasets presented in this study can be found in online repositories. The names of the repository/repositories and accession number(s) can be found below: NCBI SRA BioProject, accession no: PRJNA736855.
Author Contributions
HN, YW, and CW conducted the experiments and analyzed the data. HN prepared the manuscript. YS attended benchwork. CG and JW attended the discussion and revised the manuscript. JH conceived the experiments, provided the experimental platform, and revised the manuscript. All authors approved the final manuscript.
Funding
This research was financially supported by grants from the National Natural Science Foundation of China (31671741 and 31530053).
Conflict of Interest
The authors declare that the research was conducted in the absence of any commercial or financial relationships that could be construed as a potential conflict of interest.
Publisher's Note
All claims expressed in this article are solely those of the authors and do not necessarily represent those of their affiliated organizations, or those of the publisher, the editors and the reviewers. Any product that may be evaluated in this article, or claim that may be made by its manufacturer, is not guaranteed or endorsed by the publisher.
Acknowledgments
We would like to thank Dr. Zhengjie Liu, Ms. Yuan Zhang, and Ms. Haili Pei for their contributions. Thanks to Dr. Kunbo Wang and Dr. Fang Liu (National Wild Cotton Nursery, Sanya, China; Cotton Research Institute, Chinese Academy of Agricultural Sciences) for providing the seeds of wild cotton species.
Supplementary Material
The Supplementary Material for this article can be found online at: https://www.frontiersin.org/articles/10.3389/fpls.2021.715041/full#supplementary-material
Supplementary Figure 1. Correlation and different expression genes analysis of RNA-seq. (A) Statistical analysis of correlation coefficient between each sample; (B) principal component analysis of each sample; (C) statistics of the number of DEGs in G. raimondii and G. sturtianum; (D,E) Venn diagrams of DEGs in different salt stress stages of G. raimondii (D) and G. sturtianum (E).
Supplementary Figure 2. Callus suspension culture of G. raimondii and G. sturtianum. (A) Callus suspension culture of G. raimondii, (B) the callus of G. raimondii obtained from the suspension culture was inoculated to the solid medium, (C) callus suspension culture of G. sturtianum, (D) the callus of G. sturtianum obtained from the suspension culture was inoculated on solid medium.
Supplementary Figure 3. Identification and functional analysis of different expression genes during diverse stages under salt stress in G. raimondii and G. sturtianum; (A) The heatmap of co-genes which different expressed during 4 stages under salt stress in G. raimondii; (B) qRT-PCR results of 14 genes under salt stress in G. raimondii and G. sturtianum; (C) GO enrichment analysis of common DEGs in G. raimondii and G. sturtianum. GHUBQ7 gene as an internal control, and the error bars indicated the standard error of the means.
Supplementary Figure 4. MAPK signaling pathway.
Supplementary Table 1. The culture media for embryogenic callus induction of G. sturtianum.
Supplementary Table 2. Media used in embryogenic callus induction of G. raimondii.
Supplementary Table 3. The medium components for callus suspension culture of G. raimondii and G. sturtianum.
Supplementary Table 4. Summary of RNA-Seq data quality in different libraries.
Supplementary Table 5. Alignment result of sample sequencing data to the reference genome.
Supplementary Table 6. The comparison of nitrogen source used in embryonic callus of G. sturtianum.
Supplementary Table 7. The comparison of FeSO4, CuSO4 used in embryonic callus induction of G. sturtianum.
Supplementary Table 8. Primers of target genes for qRT-PCR.
Supplementary Table 9. List of genes related to MAPK signaling pathway.
Abbreviations
2, 4-D, 2, 4-Dichlorophenoxyacetic acid; ABA, Abscisic acid; ABA2, Xanthoxin dehydrogenase genes; BBM, Baby boom; CTAB, Cetyltrimethyl ammonium bromide; DEGs, Differentially expressed genes; FC, Fold change; FDR, False discovery rate; GO, Gene ontology; IAA, Indole-3-acetic acid; IBI, Indole-3-butyric acid; KT, Kinetin; KEGG, Kyoto Encyclopedia of Genes and Genomes; LEC, Leafy cotyledon; MSB5, MS salts and B5 vitamins; NAC, NAM (no apical meristem), ATAT1/2, and CUC2 (cup-shaped cotyledon); PCA, Principal component analysis; PGRs, Plant growth regulators; qRT-PCR, Quantitative real-time PCR; TFs, Transcription factors; SnRK, Sucrose non-fermenting 1-related protein kinases; SE, Somatic embryogenesis; WRKY, WRKY class of zinc-finger proteins.
References
Babbar, S. B., and Gupta, S. C. (1986). Effect of carbon source on datura metel microspore embryogenesis and the growth of callus raised from microspore-derived embryos. Biochemie Physiol. Pflanzen 181, 331–338. doi: 10.1016/S0015-3796(86)80006-3
Badawy, O. M., Nasr, M. I., and Alhendawi, R. A. (2008). Response of sugarcane (saccharum species hybrid) genotypes to embryogenic callus induction and in vitro salt stress. Sugar Tech. 10, 243–247. doi: 10.1007/s12355-008-0043-8
Barrero, J. M., Rodríguez, P. L., Quesada, V., Piqueras, P., and Micol, J. L. (2010). Both abscisic acid (ABA)-dependent and ABA-independent pathways govern the induction of NCED3, AAO3 and ABA1 in response to salt stress. Plant Cell Environ. 29, 2000–2008. doi: 10.1111/j.1365-3040.2006.01576.x
Cangahuala-Inocente, G. C., Silveira, V., Caprestano, C. A., Floh, E. I. S., and Guerra, M. P. (2014). Dynamics of physiological and biochemical changes during somatic embryogenesis of Acca sellowiana. In Vitro Cell Dev. Pl. 50, 166–117. doi: 10.1007/s11627-013-9563-3
Chee, R. P., Schultheis, J. R., and Cantliffe, D. J. (1990). Plant recovery from sweet potato somatic embryos. Hortscience 25, 795–797. doi: 10.21273/HORTSCI.25.7.795
Chen, X., Wang, Y. F., Lv, B., Li, J., Luo, L. Q., Lu, S. C., et al. (2014). The NAC family transcription factor OsNAP confers abiotic stress response through the ABA pathway. Plant Cell Physiol. 3, 604–620. doi: 10.1093/pcp/pct204
Chen, X. B., Wang, J., Zhu, M., Jia, H. H., Liu, D. D., Hao, L. L., et al. (2015). A cotton Raf-like MAP3K gene, GhMAP3K40, mediates reduced tolerance to biotic and abiotic stress in Nicotiana benthamiana by negatively regulating growth and development. Plant Sci. 240, 10–24. doi: 10.1016/j.plantsci.2015.08.012
Cheng, S. F., Huang, Y. L., Zhu, N., and Zhao, Y. (2014). The rice WUSCHEL-related homeobox genes are involved in reproductive organ development, hormone signaling and abiotic stress response. Gene 549, 266–274. doi: 10.1016/j.gene.2014.08.003
Cheng, W. H., Chiang, M. H., Hwang, S. G., and Lin, P. C. (2009). Antagonism between abscisic acid and ethylene in Arabidopsis acts in parallel with the reciprocal regulation of their metabolism and signaling pathways. Plant Mol. Biol. 71, 61–80. doi: 10.1007/s11103-009-9509-7
Cheng, W. H., Zhu, H. G., Tian, W. G., Zhu, S. H., Xiong, X. P., Sun, Y. Q., et al. (2016). De novo transcriptome analysis reveals insights into dynamic homeostasis regulation of somatic embryogenesis in upland cotton (G. hirsutum L.). Plant Mol. Biol. 92, 279–292. doi: 10.1007/s11103-016-0511-6
Deinlein, U., Stephan, A. B., Horie, T., Luo, W., Xu, G., and Schroeder, J. I. (2014). Plant salt tolerance mechanisms. Trends Plant Sci. 19, 6–15. doi: 10.1016/j.tplants.2014.02.001
Dong, Y., Hu, G., Yu, J., Thu, S. W., Grover, C. E., Zhu, S., et al. (2020). Salt-tolerance diversity in diploid and polyploid cotton (gossypium) species. Plant J. 101, 1135–1151. doi: 10.1111/tpj.14580
Fryxell, P., Craven, L., and Stewart, J. D. (1992). A revision of Gossypium sect. Grandicalyx (Malvaceae), including the description of six new species. Syst. Bot. 17, 91–114. doi: 10.2307/2419068
Ge, F., Hu, H., Huang, X., Zhang, Y., Wang, Y., Li, Z., et al. (2017). Metabolomic and proteomic analysis of maize embryonic callus induced from immature embryo. Sci. Rep UK 7, 1004–1020. doi: 10.1038/s41598-017-01280-8
Gill, S. S., and Tuteja, N. (2010). Reactive oxygen species and antioxidant machinery in abiotic stress tolerance in crop plants. Plant Physiol. Bioch. 48, 909–930. doi: 10.1016/j.plaphy.2010.08.016
Golldack, D., Luking, I., and Yang, O. (2011). Plant tolerance to drought and salinity: stress regulating transcription factors and their functional significance in the cellular transcriptional network. Plant Cell Rep. 30, 1383–1391. doi: 10.1007/s00299-011-1068-0
Gong, Z. Z., Xiong, L. M., Shi, H. Z., Yang, S. H., Herrera-Estrella, L. R., Xu, G. H., et al. (2020). Plant abiotic stress response and nutrient use efficiency. Sci. China Life Sci. 63, 635–674. doi: 10.1007/s11427-020-1683-x
Götz, S., García-Gómez, J. M., Terol, J., Williams, T. D., Nagaraj, S. H., José, M., et al. (2008). High-throughput functional annotation and data mining with the Blast2GO suite. Nucleic Acids Res. 36, 3420–3435. doi: 10.1093/nar/gkn176
Grover, C. E., Gallagher, J. P., Jareczek, J. J., Page, J. T., Udall, J. A., Gore, M. A., et al. (2015). Re-evaluating the phylogeny of allopolyploid Gossypium L. Mol. Phylogenet. Evol. 92, 45–52. doi: 10.1016/j.ympev.2015.05.023
Grover, C. E., Grupp, K. K., Wanzek, R. J., and Wendel, J. F. (2012). Assessing the monophyly of polyploid Gossypium species. Plant Syst. Evol. 298, 1177–1183. doi: 10.1007/s00606-012-0615-7
Guo, J. Y., Shi, G. Y., Guo, X. Y., Zhang, L. W., Xu, W. Y., Wang, Y. M., et al. (2015). Transcriptome analysis reveals that distinct metabolic pathways operate in salt-tolerant and salt-sensitive upland cotton varieties subjected to salinity stress. Plant Sci. 238, 33–45. doi: 10.1016/j.plantsci.2015.05.013
Guo, Q., Zhao, L., Fan, X., Xu, P., Xu, Z., Zhang, X., et al. (2019). Transcription factor GarWRKY5 is involved in salt stress response in diploid cotton species (Gossypium aridum L.). Int. J. Mol. Sci. 20, 5244–5257. doi: 10.3390/ijms20215244
He, L., Yang, X., Wang, L., Zhu, L., Zhou, T., Deng, J., et al. (2013). Molecular cloning and functional characterization of a novel cotton CBL-interacting protein kinase gene (GhCIPK6) reveals its involvement in multiple abiotic stress tolerance in transgenic plants. Biochem. Bioph. Res. Co. 435, 209–215. doi: 10.1016/j.bbrc.2013.04.080
Hu, Y., Chen, J., Fang, L., Zhang, Z., Ma, W., Niu, Y., et al. (2019). Gossypium barbadense and Gossypium hirsutum genomes provide insights into the origin and evolution of allotetraploid cotton. Nat. Genet. 51, 739–748. doi: 10.1038/s41588-019-0371-5
Huang, C., Nie, X. H., Shen, C., You, C. Y., Li, W., Zhao, W. X., et al. (2017). Population structure and genetic basis of the agronomic traits of upland cotton in China revealed by a genome-wide association study using high-density SNPs. Plant Biotechnol. J. 15, 1374–1386. doi: 10.1111/pbi.12722
Huang, G., Wu, Z., Percy, R. G., Bai, M. Z., and Zhu, Y. X. (2020). Genome sequence of Gossypium herbaceum and genome updates of Gossypium arboreum and Gossypium hirsutum provide insights into cotton A-genome evolution. Nat. Genet. 52, 516–524. doi: 10.1038/s41588-020-0607-4
Huang, Y., Jiao, Y., Xie, N. K., Guo, Y. M., Zhang, F., Xiang, Z. P., et al. (2019). OsNCED5, a 9-cis-epoxycarotenoid dioxygenase gene, regulates salt and water stress tolerance and leaf senescence in rice. Plant Sci. 287, 110188. doi: 10.1016/j.plantsci.2019.110188
Jeong, M. J., Lee, S. K., Kim, B. G., Kwon, T. R., Cho, W. S., et al. (2006). A rice (Oryza sativa L.) MAP kinase gene, OsMAPK44, is involved in response to abiotic stresses. Plant Cell Tiss Org. 85, 151–160. doi: 10.1007/s11240-005-9064-0
Juknys, R., Vitkauskait, G., Racaite, M., and Vencloviene, J. (2012). The impacts of heavy metals on oxidative stress and growth of spring barley. Cent. Eur. J. Biol. 7, 299–306. doi: 10.2478/s11535-012-0012-9
Kiegerl, S., Cardinale, F., Siligan, C., Gross, A., Baudouin, E., Liwosz, A., et al. (2000). Simkk, a mitogen-activated protein kinase (MAPK) kinase, is a specific activator of the salt stress-induced mapk, simk. Plant Cell 12, 2247–2258. doi: 10.1105/tpc.12.11.2247
Kim, D., Pertea, G., Trapnell, C., Pimentel, H., Kelley, R., and Salzberg, S. L. (2013). TopHat2: accurate alignment of transcriptomes in the presence of insertions, deletions and gene fusions. Genome Biol. 14:R36. doi: 10.1186/gb-2013-14-4-r36
Kumar, G. P., Subiramani, S., Govindarajan, S., Sadasivama, V., Manickama, V., Mogilicherlaa, K., et al. (2015). Evaluation of different carbon sources for high frequency callus culture with reduced phenolic secretion in cotton (Gossypium hirsutum L.) cv. SVPR-2. Biotechnol Rep. 33, 72–80. doi: 10.1016/j.btre.2015.05.005
Li, B. L., Zhu, S. J., Wang, H. M., and Zhang, B. J. (1991). Bred and studied of a new allotetraploid cotton germplasm with glandless seeds/glanded plant trait. Cotton Science 3, 29–32.
Li, F., Fan, G., Lu, C., Xiao, G., Zou, C., Kohel, R. J., et al. (2015). Genome sequence of cultivated Upland cotton (Gossypium hirsutum TM-1) provides insights into genome evolution. Nat. Biotechnol. 33, 524–530. doi: 10.1038/nbt.3208
Li, F., Fan, G., Wang, K., Sun, F., Yuan, Y., Song, G., et al. (2014). Genome sequence of the cultivated cotton Gossypium arboreum. Nat. Genet. 46, 567–572. doi: 10.1038/ng.2987
Lin, J. H., Dang, F. F., Chen, J. H., Bing-Jin, B. O., Qiu, A. L., Guan, D. Y., et al. (2017). Overexpression of CaMAPK9 significantly enhanced tolerance to salt stress in Arabidopsis thaliana. J. Agri. Biotechnol. 25, 1612–1621. doi: 10.3969/j.issn.1674-7968.2017.10.006
Liu, B. C., Mu, J. Q., Zhu, J. B., Liang, Z. Q., and Zhang, L. (2017). Saussurea involucrata Sidhn2 gene confers tolerance to drought stress in Upland cotton. Pak. J. Bot. 49, 465–473.
Liu, X., Zhao, B., Zheng, H. J., Hu, Y., Lu, G., Yang, C. Q., et al. (2015). Gossypium barbadense genome sequence provides insight into the evolution of extra-long staple fiber and specialized metabolites. Sci. Rep. UK 5, 14139–14153. doi: 10.1038/srep14139
Livak, K. J., and Schmittgen, T. D. (2001). Analysis of relative gene expression data using real-time quantitative PCR and the 2−ΔΔCT method. Methods 25, 402–408. doi: 10.1006/meth.2001.1262
Lommen, S. T. E., Ciappetta, S., Ghiani, A., Asero, R., Gentili, R., Schärer, H. M., et al. (2017). Defoliation of common ragweed by Ophraella communa beetle does not affect pollen allergenicity in controlled conditions. Plant Biosyst. 151, 1094–1100. doi: 10.1080/11263504.2016.1244122
Loukanina, N., and Thorpe, T. A. (2007). Arginine and ornithine decarboxylases in embryogenic and non-embryogenic carrot cell suspensions. In Vitro Cell Dev. Pl. 44, 59–64. doi: 10.1007/s11627-007-9080-3
Ma, F. F., Ni, L., Liu, L. B., Li, X., Zhang, H., Zhang, A., et al. (2016). ZmABA2, an interacting protein of ZmMPK5, is involved in abscisic acid biosynthesis and functions. Plant Biotechnol. J. 14, 771–782. doi: 10.1111/pbi.12427
Moons, A. (1997). Antagonistic effects of abscisic acid and jasmonates on salt stress-inducible transcripts in rice roots. Plant Cell 9, 2243–2259. doi: 10.1105/tpc.9.12.2243
Nascimento-Gavioli, M. C. A., Cangahuala-Inocente, G. C., Steinmacher, D., Ree, J. F., Steiner, N., and Guerra, M. P. (2017). Physiological and biochemica. features of embryogenic and non-embryogenic peach palm (Bactris gasipaes Kunth) cultures. In Vitro Cell Dev. Pl. 53, 33–40. doi: 10.1007/s11627-017-9805-x
Navrátilová, B. (2004). Protoplast cultures and protoplast fusion focused on brassicaceae- a review. Hortic Sci. 31, 140–157. doi: 10.17221/3809-HORTSCI
Nie, H., Wang, Y., Su, Y., and Hua, J. (2018). Exploration of miRNAs and target genes of cytoplasmic male sterility line in cotton during flower bud development. Funct. Integr. Genomic. 18, 457–476. doi: 10.1007/s10142-018-0606-z
Ning, J., Li, X. H., Hicks, L. M., and Xiong, L. Z. (2010). A Raf-like MAPKKK gene DSM1 mediates drought resistance through reactive oxygen species scavenging in rice. Plant Physiol. 152, 876–890. doi: 10.1104/pp.109.149856
Pandeya, D., Campbell, L. M., Nunes, E., Lopez-Arredondo, D. L., Janga, M. R., Herrera-Estrella, L., et al. (2017). ptxD gene in combination with phosphite serves as a highly effective selection system to generate transgenic cotton (Gossypium hirsutum L.). Plant Mol. Biol. 95, 567–577. doi: 10.1007/s11103-017-0670-0
Paterson, A. H., Wendel, J. F., Gundlach, H., Guo, H., Jenkins, J., Jin, D., et al. (2012). Repeated polyploidization of Gossypium genomes and the evolution of spinnable cotton fibres. Nature 492, 423–427. doi: 10.1038/nature11798
Przetakiewicz, A., Orczyk, W., and Nadolska-Orczyk, A. (2003). The effect of auxin on plant regeneration of wheat, barley and triticale. Plant Cell Tiss. Org. 73, 245–256. doi: 10.1023/A:1023030511800
Qin, H., Wang, J., Chen, X. B., Wang, F. F., Peng, P., Zhou, Y., et al. (2019). Rice OsDOF15 contributes to ethylene-inhibited primary root elongation under salt stress. New Phytol. 223, 798–813. doi: 10.1111/nph.15824
Rajasekaran, K. (1996). Regeneration of plants from cryopreserved embryogenic cell suspension and callus cultures of cotton (Gossypium hirsutum l.). Plant Cell. Rep. 15, 859–864. doi: 10.1007/BF00233157
Rebeca, V. L., Ana, W., Valeria, A., Javier, P. L., Valeria, V. B., Denise, A. L., et al. (2018). The mating system of the wild-to-domesticated complex of Gossypium hirsutum L. is mixed. Front. Plant Sci. 9, 574–588. doi: 10.3389/fpls.2018.00574
Ree, J. F., and Guerra, M. P. (2015). Palm (Arecaceae) somatic embryogenesis. In Vitro Cell Dev. Pl. 51, 589–602. doi: 10.1007/s11627-015-9722-9
Rodriguez-Uribe, L., Higbie, S. M., Stewart, J. M., Wilkins, T., Lindemann, W., Sengupta-Gopalan, C., et al. (2011). Identification of salt responsive genes using comparative microarray analysis in upland cotton (Gossypium hirsutum l.). Plant Sci. 180, 461–469. doi: 10.1016/j.plantsci.2010.10.009
Sakhanokho, H. F., and Rajasekaran, K. (2016). Cotton regeneration in vitro. Fiber Plants 13, 87–110. doi: 10.1007/978-3-319-44570-0_6
Sakhanokho, H. F., Zipf, A., and Rajasekaran, K. (2004). Somatic embryo initiation and germination in diploid cotton (Gossypium arboreum L.). In Vitro Cell Dev. Pl. 40, 177–181. doi: 10.1079/IVP2003497
Shi, G. Y., Guo, X. Y., Guo, J. Y., Liu, L. H., and Hua, J. P. (2015). Analyzing serial cDNA libraries revealed reactive oxygen species and gibberellins signaling pathways in the salt response of Upland cotton (Gossypium hirsutum L.). Plant Cell Rep. 34, 1005–1023. doi: 10.1007/s00299-015-1761-5
Shi, J., Xu, P. H., Fang, Y., Wang, M. M., Guo, X. Q., and Li, W. (2009). Overexpression of cotton mitogen-activated protein kinases (GhMAPK) gene improves salt tolerance in tobacco. Mol. Plant Breed. 7, 1113–1119. doi: 10.3969/mpb.007.001113
Su, Y., Guo, A., Huang, Y., Wang, Y. M., and Hua, J. P. (2020). GhCIPK6a increases salt tolerance in transgenic upland cotton by involving in ROS scavenging and MAPK signaling pathways. BMC Plant Biol. 20, 421–440. doi: 10.1186/s12870-020-02548-4
Su, Y., Liang, W., Liu, Z. J., Wang, Y. M., Zhao, Y. P., Ijaz, B., et al. (2017a). Overexpression of GhDof1 improved salt and cold tolerance and seed oil content in Gossypium hirsutum. J. Plant Physiol. 218, 222–234. doi: 10.1016/j.jplph.2017.07.017
Su, Y., Wang, Y. M., Zhen, J., Zhang, X., Chen, Z. W., Li, L., et al. (2017b). SnRK2 homologs in Gossypium and GhSnRK2.6 improved salt tolerance in transgenic upland cotton and Arabidopsis. Plant Mol. Biol. Rep. 35, 442–456. doi: 10.1007/s11105-017-1034-2
Sun, Y., and Hua, J. P. (2020). Research in tissue culture of diploid cotton species. Cotton Sci. 32, 158–169. doi: 10.11963/1002-7807.syhjp.20200305
Sun, Y. Q., Zhang, X. L., Huang, C., Nie, Y. C., and Guo, X. P. (2005). Plant regeneration via somatic embryogenesis from protoplasts of six explants in Coker 201 (Gossypium hirsutum). Plant Cell Tiss Org. 82:309–315. doi: 10.1007/s11240-005-1837-y
Sun, Y. Q., Zhang, X. L., and Huang, C. J. (2006). Somatic embryogenesis and plant regeneration from different wild diploid cotton (Gossypium) species. Plant Cell Rep. 25, 289–296. doi: 10.1007/s00299-005-0085-2
Sun, Y. Q., Zhang, X. L., and Jin, S. X. (2003). Somatic embryogenesis and plant regeneration in wild cotton (Gossypium klotzschianum). Plant Cell Tiss Org. 75, 247–253. doi: 10.1023/A:1025895631247
Tan, X. L., and Qian, Y. Q. (1988). The impact of different explants sources and culture conditions on plant regeneration of Gossypium gossypioide. Acta Genetica Sinica. 15, 81–85.
Trolinder, N. L., and Goodin, J. R. (1987). Somatic embryogenesis and plant regeneration in cotton (Gossypium hirsutum L.). Plant Cell Rep. 6, 231–234. doi: 10.1007/BF00268487
Trolinder, N. L., and Goodin, J. R. (1988). Somatic embryogenesis in cotton (Gossypium). I. Effects of source of explant and hormone regime. Plant Cell Tiss Org. 12, 31–42. doi: 10.1007/BF00043105
Trolinder, N. L., and Xhixian, C. (1989). Genotype specificity of the somatic embryogenesis response in cotton. Plant Cell Rep. 8, 133–136. doi: 10.1007/BF00716824
Udall, J. A., Long, E., Hanson, C., Yuan, D., Ramaraj, T., Conover, J. L., et al. (2019). De novo genome sequence assemblies of Gossypium raimondii and Gossypium turneri. Genes Genom Genet. 9, 3079–3085. doi: 10.1534/g3.119.400392
Verma, D., Bhagat, P. K., and Sinha, A. K. (2020). MKK3-MPK6-MYC2 module positively regulates aba biosynthesis and signalling in Arabidopsis. J Plant Biochem Biot. 29, 785–795. doi: 10.1007/s13562-020-00621-5
Wang, J., Sun, Y., Yan, S., and Zhu, S. (2008). High frequency plant regeneration from protoplasts in cotton via somatic embryogenesis. Biol. Plant. 52, 61–620. doi: 10.1007/s10535-008-0121-5
Wang, J. X., Ding, H. D., Zhang, A. Y., Ma, F. F., Cao, J. M., and Jiang, M. Y. (2010). A novel mitogen-activated protein kinase gene in maize (Zea mays), ZmMPK3, is involved in response to diverse environmental cues. J. Integr. Plant Biol. 52, 442–452. doi: 10.1111/j.1744-7909.2010.00906.x
Wang, K., Wang, Z., Li, F., Ye, W., Wang, J., Song, G., et al. (2012). The draft genome of a diploid cotton Gossypium raimondii. Nat. Genet. 44, 1098–1103. doi: 10.1038/ng.2371
Wang, K. B., Wendel, J. F., and Hua, J. P. (2018). Designations for individual genomes and chromosomes in Gossypium. J. Cotton Res. 1, 38–42. doi: 10.1186/s42397-018-0002-1
Wang, M., Li, J., Wang, P., Liu, Z., Liu, F., Zhao, G. N., et al. (2020). Comparative genome analyses highlight transposon-mediated genome expansion shapes the evolutionary architecture of 3D genomic folding in cotton. Mol. Biol. Evol. doi: 10.21203/rs.3.rs-93594/v1
Wang, M., Tu, L., Yuan, D., Shen, C., Li, J., Liu, F., et al. (2019). Reference genome sequences of two cultivated allotetraploid cottons, Gossypium hirsutum and Gossypium barbadense. Nat. Genet. 51, 224–229. doi: 10.1038/s41588-018-0282-x
Wang, M. M., Zhang, Y., Wang, J., Wu, X. L., and Guo, X. Q. (2007). A novel MAP kinase gene in cotton (Gossypium hirsutum L.), GhMAPK, is involved in response to diverse environmental stresses. J. Biochem. Mol. Biol. 40, 325–332. doi: 10.5483/BMBRep.2007.40.3.325
Wang, N., Zhao, L. L., Lu, R., Li, Y., and Li, X. (2015). Cotton mitogen-activated protein kinase4 (GhMPK4) confers the transgenic Arabidopsis hypersensitivity to salt and osmotic stresses. Plant Cell Tiss Org. 123, 619–632. doi: 10.1007/s11240-015-0865-5
Wei, X., Wang, Q. H., Ge, X. Y., Chen, Y. L., Ding, Y. P., Zhao, M. Z., et al. (2019). Effects of different red and blue ratios on the somatic embryogenesis and plant regeneration of cotton. Sci. Agricult. Sin. 52, 968–980. doi: 10.3864/j.issn.0578-1752.2019.06.002
Wei, Y., Xu, Y., Lu, P., Wang, X., Li, Z., Cai, X., et al. (2017). Salt stress responsiveness of a wild cotton species (Gossypium klotzschianum) based on transcriptomic analysis. PLoS ONE 12:e0178313. doi: 10.1371/journal.pone.0178313
Xing, Y., Jia, W., and Zhang, J. (2007). AtMEK1 mediates stress-induced gene expression of CAT1 catalase by triggering H2O2 production in Arabidopsis. J. Exp. Bot. 58, 2969–2981. doi: 10.1093/jxb/erm144
Xiong, L. Z., and Yang, Y. N. (2003). Disease resistance and abiotic stress tolerance in rice are inversely modulated by an abscisic acid-inducible mitogen-activated protein kinase. Plant Cell 15, 745–759. doi: 10.1105/tpc.008714
Xu, Z. Z., Zhang, C. J., and Zhang, X. Y. (2013). Transcriptome profiling reveals auxin and cytokinin regulating somatic embryogenesis in different sister lines of cotton cultivar CCRI24. J. Integr. Plant Biol. 55, 631–642. doi: 10.1111/jipb.12073
Yan, S. F., Zhang, Q., and Wang, J. E. (2010). Somatic embryogenesis and plant regeneration in two wild cotton species belong to G genome. In Vitro Cell Dev-Pl. 46, 298–305. doi: 10.1007/s11627-009-9270-2
Yang, X. Y., and Zhang, X. L. (2010). Regulation of somatic embryogenesis in higher plants. Crit. Rev. Plant Sci. 29, 36–57. doi: 10.1080/07352680903436291
Yang, Z. R., Li, C. F., and Wang, Y. (2014). GhAGL15s, preferentially expressed during somatic embryogenesis, promote embryogenic callus formation in cotton (Gossypium hirsutum L.). Mol. Genet. Genomics. 289, 873–883. doi: 10.1007/s00438-014-0856-y
Yuan, D., Tang, Z., Wang, M., Gao, W., Tu, L., Jin, X., et al. (2015). The genome sequence of Sea-Island cotton (Gossypium barbadense) provides insights into the allopolyploidization and development of superior spinnable fibres. Sci. Rep. UK 5:17662. doi: 10.1038/srep17662
Yuan, Y. Y., Fang, L. C., Karungo, S. K., Zhang, L. L., Gao, Y. Y., Li, S. H., et al. (2016). Overexpression of VaPAT1, a GRAS transcription factor from Vitis amurensis, confers abiotic stress tolerance in Arabidopsis. Plant Cell Rep. 35, 655–666. doi: 10.1007/s00299-015-1910-x
Zhang, D., Jiang, S., Pan, J., Kong, X., Zhou, Y., Liu, Y., et al. (2014). The overexpression of a maize mitogen-activated protein kinase gene (ZmMPK5) confers salt stress tolerance and induces defence responses in tobacco. Plant Biol. 16, 558–570. doi: 10.1111/plb.12084
Zhang, F., Zhu, G. Z., Du, L., Shang, X. G., Cheng, C. Z., Yang, B., et al. (2016). Genetic regulation of salt stress tolerance revealed by RNA-Seq in cotton diploid wild species, Gossypium davidsonii. Sci Rep-UK. 6, 20582–20596. doi: 10.1038/srep20582
Zhang, L., Xi, D. M., Li, S. W., Gao, Z., Zhao, S. L., Shi, J., et al. (2011). A cotton group C MAP kinase gene, GhMPK2, positively regulates salt and drought tolerance in tobacco. Plant Mol. Biol. 77, 17–31. doi: 10.1007/s11103-011-9788-7
Zhang, Q., Wang, J. E., and Ye, C. L. (2010). Somatic embryogenesis and plant regeneration in Gossypium tomentosum. Cotton Sci. 22, 217–223.
Zhang, S., Li, X. W., Fan, S. D., Zhou, L. J., and Wang, Y. (2020). Overexpression of HcSCL13, a Halostachys caspica GRAS transcription factor, enhances plant growth and salt stress tolerance in transgenic Arabidopsis. Plant Physiol. Bioch. 151, 243–254. doi: 10.1016/j.plaphy.2020.03.020
Zhang, T., Hu, Y., Jiang, W., Fang, L., Guan, X., Chen, J., et al. (2015). Sequencing of allotetraploid cotton (Gossypium hirsutum L. acc. TM-1) provides a resource for fiber improvement. Nat. Biotechnol. 33, 531–537. doi: 10.1038/nbt.3207
Zhang, Y., Yang, X., He, L., Li, L., Zhou, T., Jin, F., et al. (2014). Histological analysis of somatic embryogenesis and plant regeneration in Gossypium hirsutum Lumian 6. J. Huazhong Agricul. Univ. 33, 22–27. doi: 10.13300/j.cnki.hnlkxb.2014.02.016
Zhao, L., Ding, Q., Zeng, J., Wang, F., Zhang, J., Fan, S., et al. (2012). An improved CTAB-ammonium acetate method for total RNA isolation from cotton. Phytochem. Anal. 23, 647–650. doi: 10.1002/pca.2368
Zheng, W., Zhang, X. Y., and Yang, Z. R. (2014). AtWuschel promotes formation of the embryogenic callus in Gossypium hirsutum. PLoS ONE 1:e87502. doi: 10.1371/journal.pone.0087502
Keywords: embryogenic callus, salt stress, transcriptome analysis, abscisic acid, transcription factor, Gossypium raimondii, Gossypium sturtianum
Citation: Nie H, Wang Y, Wei C, Grover CE, Su Y, Wendel JF and Hua J (2021) Embryogenic Calli Induction and Salt Stress Response Revealed by RNA-Seq in Diploid Wild Species Gossypium sturtianum and Gossypium raimondii. Front. Plant Sci. 12:715041. doi: 10.3389/fpls.2021.715041
Received: 26 May 2021; Accepted: 26 July 2021;
Published: 25 August 2021.
Edited by:
Fanchang Zeng, Shandong Agricultural University, ChinaReviewed by:
Xiaoyang Ge, Cotton Institute of the Chinese Academy of Agricultural Sciences, ChinaWei Gao, Henan University, China
Copyright © 2021 Nie, Wang, Wei, Grover, Su, Wendel and Hua. This is an open-access article distributed under the terms of the Creative Commons Attribution License (CC BY). The use, distribution or reproduction in other forums is permitted, provided the original author(s) and the copyright owner(s) are credited and that the original publication in this journal is cited, in accordance with accepted academic practice. No use, distribution or reproduction is permitted which does not comply with these terms.
*Correspondence: Jinping Hua, amlucGluZ19odWFAY2F1LmVkdS5jbg==