- 1State Key Laboratory of Tea Plant Biology and Utilization, Anhui Agricultural University, Hefei, China
- 2School of Life Sciences, Anhui Agricultural University, Hefei, China
The ubiquitin/26S proteasome pathway is a critical protein-degradation pathway in plant growth and development as well as in nearly all biological and abiotic stress processes. Although as a member of the ubiquitin/26S proteasome pathway, the E3 ubiquitin ligase family has been shown to be essential for the selective degradation of downstream target proteins, it has been rarely reported in tea plants (Camellia sinensis). In this study, through database searches and extensive manual deduplication, 335 RING finger family proteins were selected from the Tea Plant Information Archive. These proteins were divided into six categories by the difference of RING finger domain: RING-H2, RING-HCa, RING-HCb, RING-C2, RING-v, and RING-G. Stress-induced differential gene expression analysis showed that 53 proteins in RING finger family can respond to selected exogenous stress. In vitro ubiquitination assays indicated that TEA031033, which was named CsMIEL1, exhibited the activity of E3 ubiquitin ligases. CsMIEL1-overexpressing transgenic Arabidopsis thaliana seedlings were resistant to some exogenous abiotic stresses, such as salt and drought stress but sensitive to exogenous methyl jasmonate treatment. Furthermore, CsMIEL1 reduced the accumulation of anthocyanin in transgenic plants in response to low temperature treatment. The results of this article provide basic date for studying the role of ubiquitin/26S proteasome pathway in tea plants response to stresses.
Introduction
Tea plant [Camellia sinensis (L.) O. Kuntze], a perennial evergreen woody plant, encounters various abiotic (drought, heat, cold, and salt) and biological stresses (pests, viruses, and herbivore foraging). Under these stresses, plants undergo various degrees of damage. For example, under high salt stress, an imbalance of Na+/K+ inside and outside the cell restricts plant growth and accumulates excessive reactive oxygen species, which causes osmotic and even oxidative stresses (Munns and Tester, 2008). Through long-term natural selection, plants have evolved various physiological and biochemical defense mechanisms to deal with stresses (Hou et al., 2009), such as antioxidant defense mechanisms (Dai and Mumper, 2010), apoptosis (Jones, 2001), and autophagy (Avin-Wittenberg, 2019). Plants can tolerate a harsh environment and maintain normal growth, in recent years, more and more researches have begun to pay attention to how the ubiquitination process of plants works under various stresses.
The 26s-proteasome-mediated ubiquitination degradation pathway (ubiquitin/26s proteasome) is central to apoptosis (Kurepa et al., 2012). The ubiquitination of substrate proteins requires the participation of E1-ubiquitin-activating, E2-ubiquitin-conjugating enzymes, and E3-ubiquitin ligases. First, the cysteine residue (Cys) on the active site of E1 is covalently bound to the terminal glycine of the ubiquitin molecule through a high-energy thioester bond, and Adenosine 5′-triphosphate (ATP) is consumed to activate the ubiquitin (Stone, 2014). Second, the ubiquitin is transferred to the Cys residue of E2 to form an E2-Ub thioester complex. Finally, E3 transfers the ubiquitin from E2 to the substrate protein (Downes et al., 2003). After that, the 26S proteasome degrades the ubiquitinated-modified substrate protein, and the deubiquitinating enzyme hydrolyzes and releases the ubiquitin, which triggers the ubiquitination modification process.
As a key of the ubiquitin-proteasome pathway, E3 ligases are critical in the recognition of target substrates. Because of the importance of E3 ligase, the genes encoding E3 are the most numerous in the same plant genome. For example, in the Arabidopsis thaliana genome, approximately 6% of the genes are related to the ubiquitin/26s proteasome pathway (Fu et al., 1999; Yan et al., 2000; Bachmair et al., 2001; Gagne et al., 2002; Kosarev et al., 2002). Two genes encode E1 and are the least numerous. At least 37 genes encode E2 and E2-like proteins, but more than 1,400 genes encode E3 (Smalle and Vierstra, 2004; Mazzucotelli et al., 2006; Vierstra, 2009). E3 ubiquitin ligases can be divided into HECT, Ubox, and RING types, but RING-type ligases can be additionally divided into complex and simple types (Vierstra, 2003). The Cys-rich RING protein was originally named the “Really Interesting New Gene” based on its unique domain (Freemont, 1993), the structure of the RING finger domain that binds a pair of zinc atoms is conserved (Yang et al., 2019), and eight conserved docking sites in the domain exist for binding the ubiquitin-E2 intermediate during ubiquitin transfer (Barlow et al., 1994; Borden et al., 1995; Zheng et al., 2000). The RING finger domain is similar to the well-known Zinc-finger domain in structure; however, the Zinc-finger domain functions in the form of DNA-protein binding, whereas the RING-finger domain functions in the form of a protein–protein interaction (Nardelli et al., 1991).
In previous studies, it has been shown that more than 70% of RING-containing proteins in Arabidopsis thaliana act as an E3 ubiquitin ligase (Stone et al., 2005). For example, AtATL78 is a RING-type E3 ubiquitin ligase located in the plasma membrane, which promotes ABA-dependent plant stomata closure, and also can participate in cold and drought stress responses. In a study of cold stress response, it was found that compared with the wild-type plants, the transcription levels of cold-stress-induced genes RD29A, RD29B, RD20, and P5CS1 were significantly upregulated in atl78 mutants, and lower H2O2 accumulation was detected, which indicated that AtATL78 was a negative factor in cold stress regulation (Suh et al., 2016). Research has also investigated the RING-H2 type protein MYB30-INTERACTING E3 LIGASE 1 (MIEL1). In Arabidopsis, MIEL1 mediates the degradation of the transcription factor MYB30, which acts as a positive regulator of the hypersensitive cell death program in plants, and it can interact with AtMIEL1 (Marino et al., 2019), the interaction between MIEL1 and MYB30 affected the formation of the plant’s waxy layer, weakens the plant’s defense ability (Lee et al., 2017), and MIEL1 also negatively regulates ABA signaling by promoting MYB96 turnover (Lee and Seo, 2016). In apples, MdMYB308L is a known target gene identified to interact with MIEL1, it positively regulates cold tolerance and anthocyanin accumulation by interacting with MdbHLH33 and enhancing its binding to MdCBF2 and MdDFR promoters. When MIEL1 interacts with MYB308L, MdMIEL1 directly degrades MdMYB308L through the 26S-proteasome-mediated ubiquitination degradation pathway, thereby reducing the cold tolerance and anthocyanin accumulation promoted by MdMYB308L (An et al., 2017).
Although numerous studies have concluded that RING domain–containing proteins have multiple regulatory roles in plant growth and development, few studies have investigated the function of RING finger family proteins in tea plants. In this study, RING-containing proteins in tea plants were selected from the Tea Plant Information Archive (TPIA), and the predicted responses of RING domain proteins to abiotic and biotic stresses were explored. One of them was proven to have a ubiquitination function and likely contributing to the resistance of A. thaliana to abiotic stress.
Materials and Methods
Identification of RING Domain-Containing Proteins in the Tea Plant
We downloaded the sequence of 477 RING-containing proteins that have been reported to be present in Arabidopsis (Stone et al., 2005) from The Arabidopsis Information Resource (TAIR)1 and constructed their domains in Pfam2. Six different Hidden Markov model (HMM) motifs were collected. HMM-Blast was applied to the protein sequences in the database downloaded from TPIA3 with HMMer4 (Mistry et al., 2013). For the prediction and analysis of the domains of these proteins, we used InterPro5 for the initial appraisal. Pfam (see text footnote 2) and SMART6 for a secondary verification and determination of redundant domains.
Expression Analysis of RING Finger Family Genes in the Tea Plant
We downloaded the expression levels of these RING family genes under different stresses from TPIA (Supplementary Table 1). The gradient of cold treatment was CA1-6h (10°C for 6 h), CA1-7d (4–10°C for 7 days), CA2-7d (0–4°C for 7 days), and DA-7d [recovery afterward at room temperature (20–25°C) for 7 days] (Wang et al., 2013). Salt and drought treatments were conducted for 24, 48, and 72 h (Zhang et al., 2017). MeJA treatment was applied in gradients of 12, 24, and 48 h (Shi et al., 2015). We calculated the relative ratio of these expression levels to the control, which was normalized by log2 (Supplementary Figure 1). When the expression of H2-type genes under stress at any time is upregulated or down-regulated twice as much as the control (Supplementary Figure 2), we selected and collected it for Venn diagram analysis (Figure 2).
Four Exogenous Hormone Treatments on Branches of the Tea Plant
Tea plants (Camellia sinensis var. Sinensis cv. Shuchazao) were collected from the Tea Plant Cultivar and Germplasm Resource Garden in Guohe Town, Anhui Agricultural University. Tea branches having the same growth conditions and divided into four groups, after which they were placed in erlenmeyer flasks containing the same amount of water. After 24 h of adaptation, the water was replaced with 200 mM NaCl solution for Group 1 and with 25% PEG4000 solution for Group 2. Group 3 was treated by smearing 0.25% MeJA on each leaf. Group 4 was treated at 10°C, respectively. The 2nd leaves tissues were collected at seven time points: 0, 6, 12, 24, 36, 42, and 60 h for Group 1; 0, 4, 8, 16, 24, and 36 h for Group 2; at 0, 6, 12, 24, and 36 h for Group 3; 0, 6, 12, 24, 36, 72, and 96 h for Group 4. At each time point, three repeats were performed. Samples were immediately frozen in liquid nitrogen and kept at −80°C for RNA extraction.
Quantitative Real-Time RT-PCR Analysis
Total RNA was extracted using the FastPure Plant Total RNA Isolation Kit (polysaccharides and polyphenolics-Rich) (Vazyme Cat. RC401-01) according to the manufacturer recommendations. The cDNA were synthesized using PrimeScript RT Reagent Kit Perfect Real Time (TaKaRa, Dalian, China; Code: DRR037A) and stored at −20°C for later use. Verifying PCR product-amplification specificity was determined using the fusion curve (55–95°C). The housekeeping gene was glyceraldehyde-3-phosphate dehydrogenase. The RT-qPCR mixture consisted of 10 μL of CHAMQ SYBR qPCR mixture (Vazyme), 7.4 μL of ddH2O, 0.8 μL of upstream and downstream primers, and 1 μl of cDNA. RT-qPCR reacted in the 96-well optical reaction plates at 95°C for 30 s, followed by 40 cycles at 95°C for 5 s and reaction at 60°C for 30 s PCR product amplification specificity was determined using the melting curve (55–95°C). Three biological replicates and three experimental replicates were applied for each sample. The relative expression values were calculated using the 2–ΔΔCt method. In each group, untreated branches were used as control. All primers used for RT-qPCR analysis were recorded in Supplementary Table 4.
Subcellular Location Analysis
The steps of subcellular localization analysis were referred to Yoo et al. (2007), and some modifications were made. CsMIEL1 was constructed on pUC19-GFP vector and transformed into Arabidopsis protoplasts of leaves.
In vitro Ubiquitination Assays
The entire CsMIEL and CsMIEL1-C192S open reading frame was cloned into the pEGX vector and expressed in Escherichia coli, the primers are displayed in Supplementary Table 5. The in vitro ubiquitination assays were performed as described elsewhere (Zhao et al., 2012). For the E3 ubiquitin ligase activity assay, recombinant wheat (Triticum aestivum) E1 (GI: 136632), human E2 (UBCH5B; 100 ng), and purified Arabidopsis ubiquitin with HIS-tag (UBQ14, AT4G02890; 500 ng) were mixed and used for the assay. The mixture was incubated at 30°C for 2 h, boiled at 100°C for 5 min, and frozen at −20°C until the SDS-PAGE analysis. After Western blotting, the reactants isolated by SDS-PAGE were incubated with GST antibody (1:10,000) and observed using ECL chromogenic solution.
Sequence Alignment and Phylogenetic Analysis
Multiple sequence alignments of the full-length RING proteins were performed by DNAMAN (Version 6.0; Lynnon Corporation, Quebec City, QC, Canada) with default parameters. The phylogenetic tree was constructed with molecular evolutionary genetics analysis (MEGA) software (Version 6.0)7 (Tamura et al., 2013), using the neighbor-joining (NJ), minimal evolution (ME), and maximum parsimony (MP) methods and the bootstrap test carried out with 1,000 iterations to test the significance of the nodes.
Generation of the Overexpressing Transgenic Arabidopsis thaliana
Arabidopsis ecotype Columbia (Col-0) plants were grown in Murashige and Skoog (MS) media at 22°C in long day conditions (16 h of light and 8 h of darkness) and used as wild types and for genetic transformation and other analyses. The Agrobacterium tumefaciens GV3101 strain was grown in LB media supplemented with 50 μg/mL kanamycin and 20 μg/mL rifampicin. The CsMIEL1-MYC and CsMIEL1-C192S-MYC construct consisted of the coding sequence under the control of a 35S promoter. Transgenic Arabidopsis thaliana organisms were generated using floral-dip transformation (Clough and Bent, 1998). All T0 generation seeds were sown on MS medium containing 15 μg/mL glyphosate for selection. RNA was extracted and reverse transcribed into cDNA for semi-quantitative PCR, and finally four CsMIEL1-MYC transgenic lines and CsMIEL1-C192S-MYC transgenic lines were obtained. Among them, Line 2, 4, and 6 of CsMIEL1 have high expression level, and Line 8 is low. Line 2, 3, and 4 of C192S are high, and Line 1 has low expression level (Supplementary Figure 3). Through the glyphosate selecting of each generation until the T3 generation, PCR verification assays have confirmed that the overexpressing line is homozygous.
Stress Induction in Arabidopsis thaliana Seedlings
Wild-type, CsMIEL1-MYC, and CsMIEL1-C192S-MYC overexpressing Arabidopsis thaliana were sown simultaneously in Petri dishes containing the same volume of MS medium under common growth conditions. After 10 days of pre-culture, the seedling of the same size was selected and treated on the MS medium with 100 mM NaCl (Zhang et al., 2008; Kuki et al., 2020), 200 mM mannitol (Malefo et al., 2020), 100 μm of MeJA (Leon-Reyes et al., 2010), and 10°C (Zhang et al., 2008) for another 10 days. Plants were grown for 10 days under normal conditions after pre-culture as controls (Mock). In all biological replicates, at least 50 plants were used for each treatment, and the error bars represent the standard deviation of replicates.
Extraction of Anthocyanin Content
Extraction of anthocyanin was extracted according to Jiang et al. (2017) with some modifications. In total, 0.2 g of plant tissue was quick-frozen in liquid nitrogen for preparation for subsequent experiments. During extraction, plant tissues were fully ground with a ball mill and extracted four times through the addition of a 500 μL extraction buffer. The ratio of the buffer was 80% methanol, 1% hydrochloric acid, and 19% water. After each extraction, the homogenate was centrifuged at 12,000 rpm for 10 min and combined. The absorbance values were measured at 530 nm, and each genotype was repeated at least three times.
Measurement of Plants Growth Index
The plants were stripped from the MS medium, and various growth indicators were measured manually. We recorded the data of all biological replicates for calculating the average and standard deviation. The measurement was all done by one person to control the error.
Statistical Analysis
For statistical analyses, IBM SPSS Statistics version 19 were use. The Tukey’s and least significant difference (LSD) multiple comparison tests were used to analyze significant differences between pairs. Differences were considered statistically significant when ∗P < 0.05 and ∗∗P < 0.01. All the results were based on the average of three parallel experiments.
Results
Classification of RING Protein in Camellia sinensis
We downloaded the sequence of the RING family in Arabidopsis for analysis of this huge family in the tea plant. After deduplication and comparison, 335 RING-contain proteins were identified. On the basis of the differences in amino acid sequences at these binding sites and the number of amino acids between each site, these 335 domain in tea plant were divided into six categories: RING-H2, RING-HCa, RING-HCb, RING-C2, RING-v, and RING-G. In all types, the amino acid residues at positions 1, 2, 3, 6, 7, and 8 were conserved and consisted of Cys, whereas the amino acid residues 4 and 5 were not conserved. According to the aforementioned classifications, 64% (214) of the RING domain in tea plant were distributed into the RING-H2 group. The metal-ligand positions 4 and 5 are two His residues, which accounted for the name of the RING-H2 group. According to the characteristics of the amino acid residues of metal ligands 4 and 5, the remaining RING types were classified into RING-HCa, HCb, C2, V, and G groups. The differences between HCa and HCb groups corresponded to the number of amino acids between positions 7 and 8. Between metal ligands 7 and 8, the HCa group comprised two and four amino acids, respectively. Few members belonged to the HCb and G groups (only three members and one member, respectively). Similar to Arabidopsis, most proteins were the H2 type followed by the HCa type. In contrast to Arabidopsis thaliana, among the 477 RING proteins in Arabidopsis thaliana, 41 (8.6%) HCb-type proteins were present, whereas only 3 HCbs were present in the tea plants. We downloaded data from the Grape Genome Browser8 database and performed the same manual search and identification of the RING domain in grapes to compare the RING-containing protein classifications of different species (Supplementary Table 2). Apple’s data in the table comes from previous reports (Zhang et al., 2008). Among the four species, the distribution of RING domains was consistent; the H2-type domains were the most numerous followed by the HCa-type domains. The number of V-type and C2-type domains in each species was nearly identical, but the number of HCb-type domains differed substantially. Research has speculated that the RING-D domain may only exists in Arabidopsis thaliana (Stone et al., 2005). Accordingly, in our search of tea plants and grapes, we did not identify this type of RING domain. But in Apple, a D-type RING domain was found (Zhang et al., 2008). However, no C2-type grape RING domains were found in this selection. Perhaps due to the problem of genome assembly and annotation, the identification in tea plant did not involve S/T-Type, D-type, mH2-type and mHC-type, and the structure of these types was not explained in detail (Figure 1).
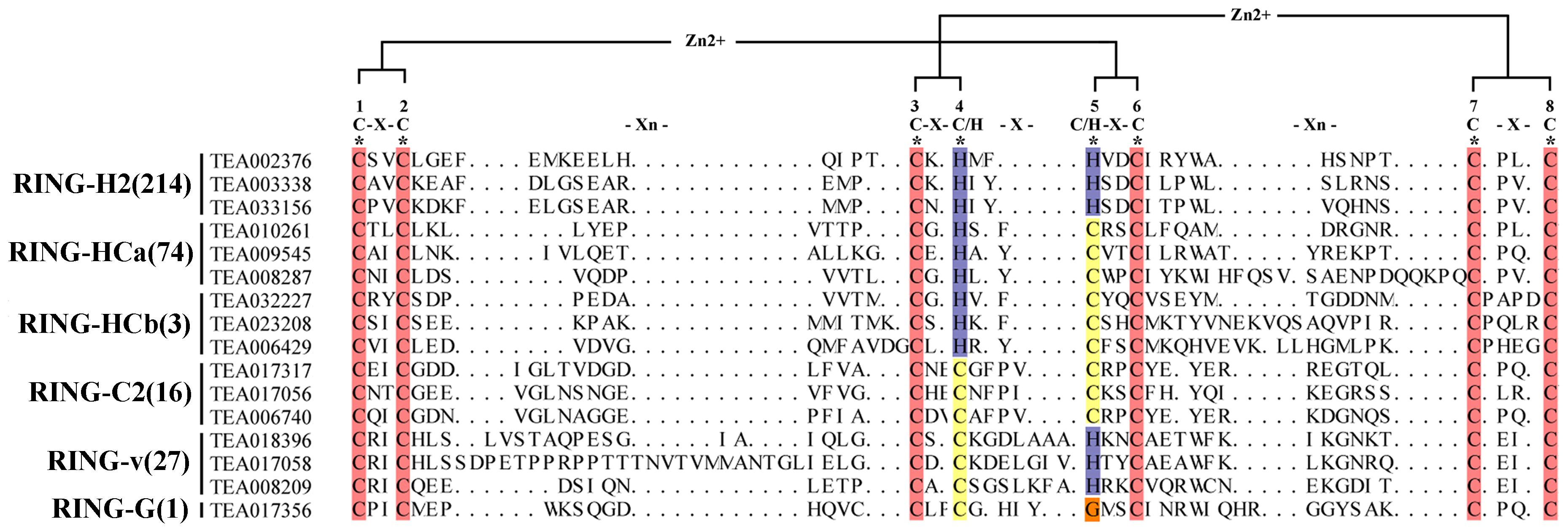
Figure 1. Structural analysis of RING finger domains. Bracketed numbers represent the amount in each RING domain. *Eight conserved docking sites. Red represents amino acid conserved sites, other colors represent different residues.
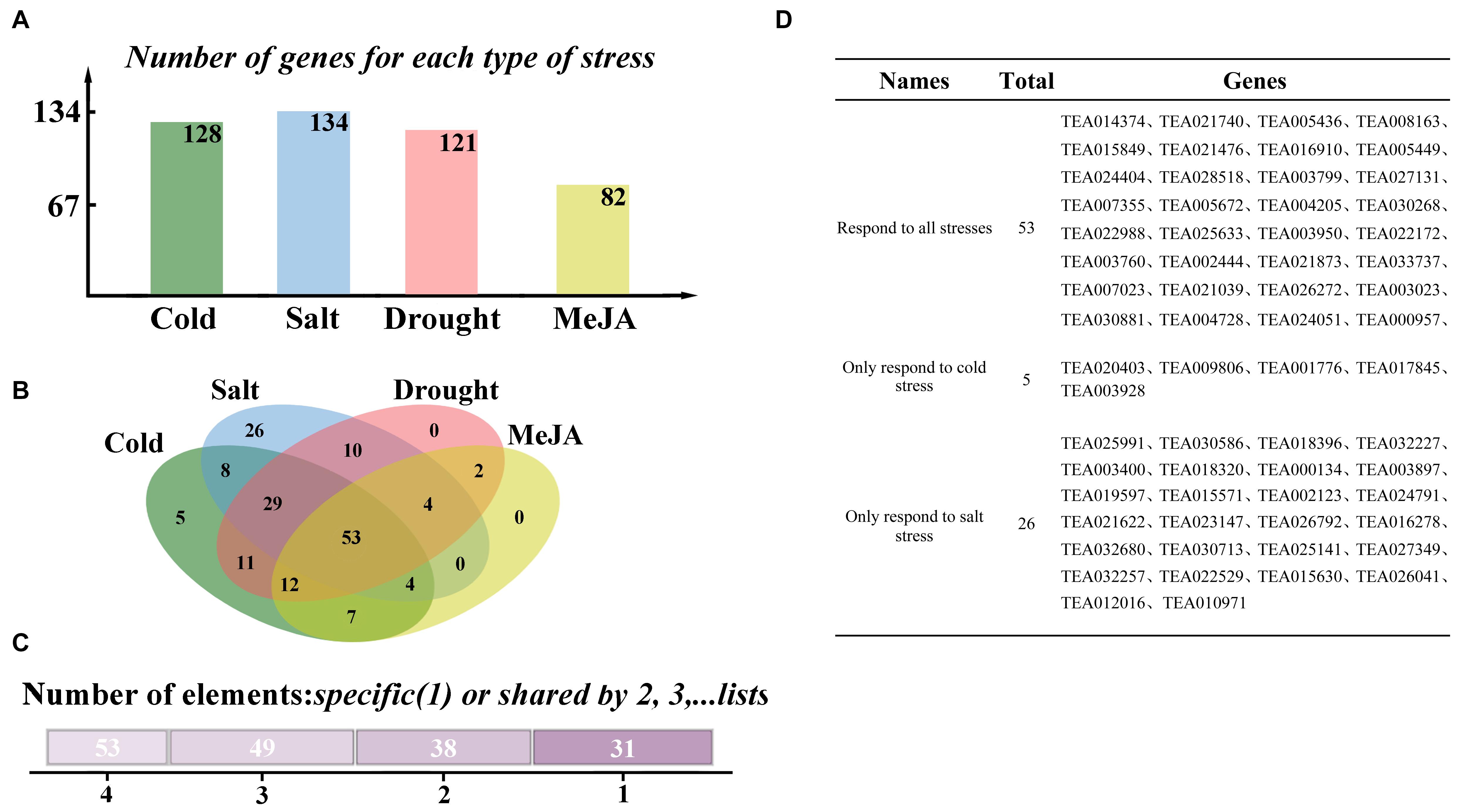
Figure 2. Selection of RING-containing proteins in response to stresses in tea plants. (A) Number of genes responding to various types of stresses. (B) Venn diagram of genes responding to different stresses. (C) The number of genes responding to stresses at the same time. (D) List of genes responding to different stresses.
We predict other motifs of RING finger proteins in tea plants. The results indicated that 235 members exhibited no other motifs and contained only the RING finger domain. An additional 17 domains were identified in the remaining RING-containing proteins, such as the WD40 domain, IBR domain, and other motifs with unknown functions (Supplementary Table 3). The WD40 domain is cited as a binding module for pSer/Thr. Additionally, when present in ubiquitin ligase, the WD40 domain may also be related to the phosphorylation of the relevant substrate (Deshaies, 1999; Koepp et al., 1999; Nakayama et al., 2000). The IBR domain is a common Cys-rich region located between two RING domains, but its function is unknown (van der Reijden et al., 1999). The identification of numerous domains suggests that the RING-containing proteins in tea plants may have multiple regulation or regulated mechanisms and perform diverse functions.
Selection of RING-Containing Proteins Involved in Stress Resistance
To figure out the RING finger genes related to stress responses in tea plants, we analyzed the induced expression difference data of 335 genes provided by TPIA under different stresses. Abiotic stresses included cold, salt, and drought treatments. Responses to biological stress were simulated by detecting the response of plants to exogenous methyl jasmonate (MeJA). Differentially expressed H2-type RING genes were individually compared and analyzed, and the results are presents in Supplementary Figure 2. Figure 2A reports the number of genes responding to various stresses. In total, 128, 134, 121, and 82 genes responded to cold stress, salt stress, drought stress, and exogenous MeJA treatment, respectively. A Venn diagram indicates that 53 genes responded to all stresses (cold stress, drought stress, salt stress, and MeJA treatment), and 31 genes respond to only one form of stress. A total of 26 genes responded only to salt stress, and five responded only to cold stress. However, no gene responded only to drought stress or MeJA treatment (Figures 2B,C). These findings are reported in Figure 2D.
To verify the response of these genes to stress, we treated the branches of tea plants with a low degree of lignification with NaCl, PEG4000, a low-temperature condition, and exogenous MeJA to simulate the four selected stresses. After the treatments were completed, RNA was extracted from the second leaf and reverse transcribed into cDNA for Quantitative real-time RT–PCR analysis (RT-qPCR) (Figure 3A). In the process of designing and RT-qPCR primers, we found that for many genes, the target fragments could not be cloned, or the amplified products were not specific, and most genes could not design primers with an efficiency of 90–110%. This may be caused by genome assembly and annotation issues. Therefore, we selected five genes that met the requirements of amplification efficiency for RT-qPCR: TEA031033, TEA023239, TEA28518, TEA030031, and TEA018024. The results of the RT-qPCR analysis indicated that gene responses to salt and MeJA were relatively low (Figures 3B,E), but responses to drought and cold were more substantial (Figures 3C,D). Among the selected genes, TEA031033 had the strongest response when treated with NaCl for 12 h: the response was 3.28 times that of the control (Figure 3B). After 24 h of MeJA treatment, the expression level of TEA031033 was approximately three times that of the control (Figure 3E), which also represented the most considerable gene response to exogenous MeJA. TEA030031 and TEA018024 responded significantly to cold stress; their strongest response to cold stress were 9.6 and 6.6 times that of the control, respectively (Figure 3C), but TEA030031 had a weak response to salt, drought, and MeJA treatment. Under drought stress, the strongest response values of TEA28518, TEA031033, and TEA018024 were 8.23, 7.55, and 4.8 times that of the control, respectively. These five genes exhibited different levels of response to biotic and abiotic stresses, but TEA031033 had a relatively high level of response to all four types of stress. Therefore, we conducted an in-depth investigation on this gene to identify its specific function in tea plants.
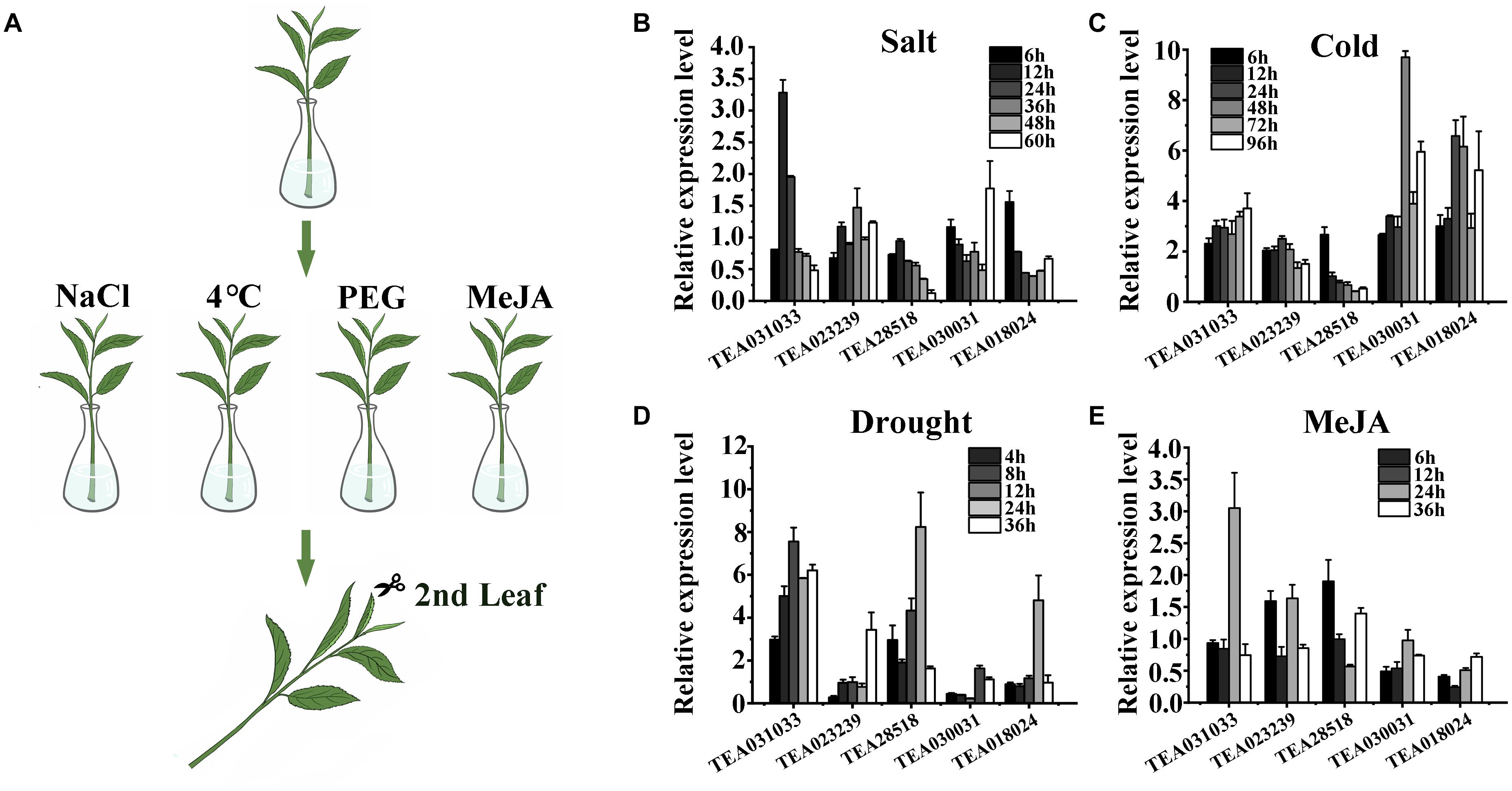
Figure 3. Validation of transcriptomic data by quantitative real-time analysis. (A) Schematic of four stress treatments on branches of the tea plant. (B–E) Quantitative real-time analysis data of five selected genes subjected to four stresses.
CsMIEL1 as an E3 Ubiquitin Ligase
We cloned TEA031033 and compared its sequence with that of other species. Our results indicated that TEA031033 has a RING finger domain at the C-terminus and a Zinc-finger domain at the N-terminus (Figure 4A). The phylogenetic analysis results indicated that AtMIEL1 had the highest homology with TEA031033 (Figure 4B). Consequently, TEA031033 was named CsMIEL1. Subcellular localization results show that CsMIEL1 is localized in the nucleus (Supplementary Figure 4).
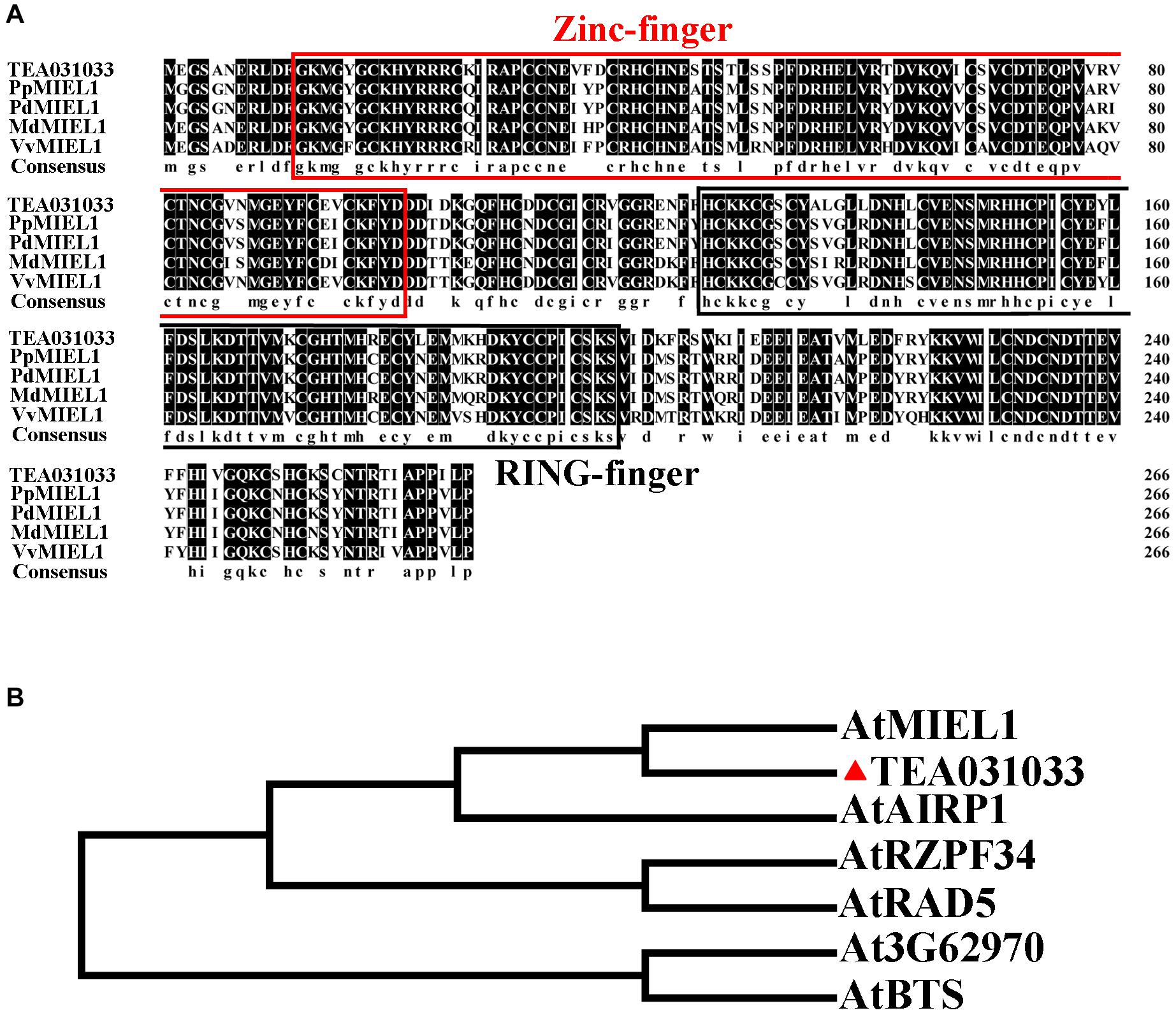
Figure 4. Bioinformatics analysis of CsMIEL1. (A) Amino acid sequence alignment of MIEL1 in different species. (B) Phylogenetic analysis of TEA031033 and RING-containing proteins in Arabidopsis.
First, to verify the specific function of CsMIEL1 in tea plants, we investigated the potential presence of E3 ubiquitin ligase activity, an in vitro ubiquitination assay was conducted. We cloned the open reading frame of the CsMIEL1 and C192S site-directed mutagenesis, the 192th residue of C192S was changed from Cys to Ser (Figure 5A). Polyubiquitinated CsMIEL1 were found in the presence of ATP, ubiquitin, E1, E2, and CsMIEL1-GST fused proteins using an anti-GST antibody, an absence of any factor had cause CsMIEL1 to not be ubiquitinated. However, even in the presence of all the auxiliary factors, no polyubiquitin CsMIEL1-C192S was detected in assays (Figure 5B). As a result, we concluded that the RING finger family protein CsMIEL1 exhibited E3 ubiquitin ligase activity and that this ubiquitination activity was lost after mutation at position 192. Moreover, we concluded that the complete RING domain is necessary to preserve the E3 ubiquitin ligase activity of the RING finger protein.
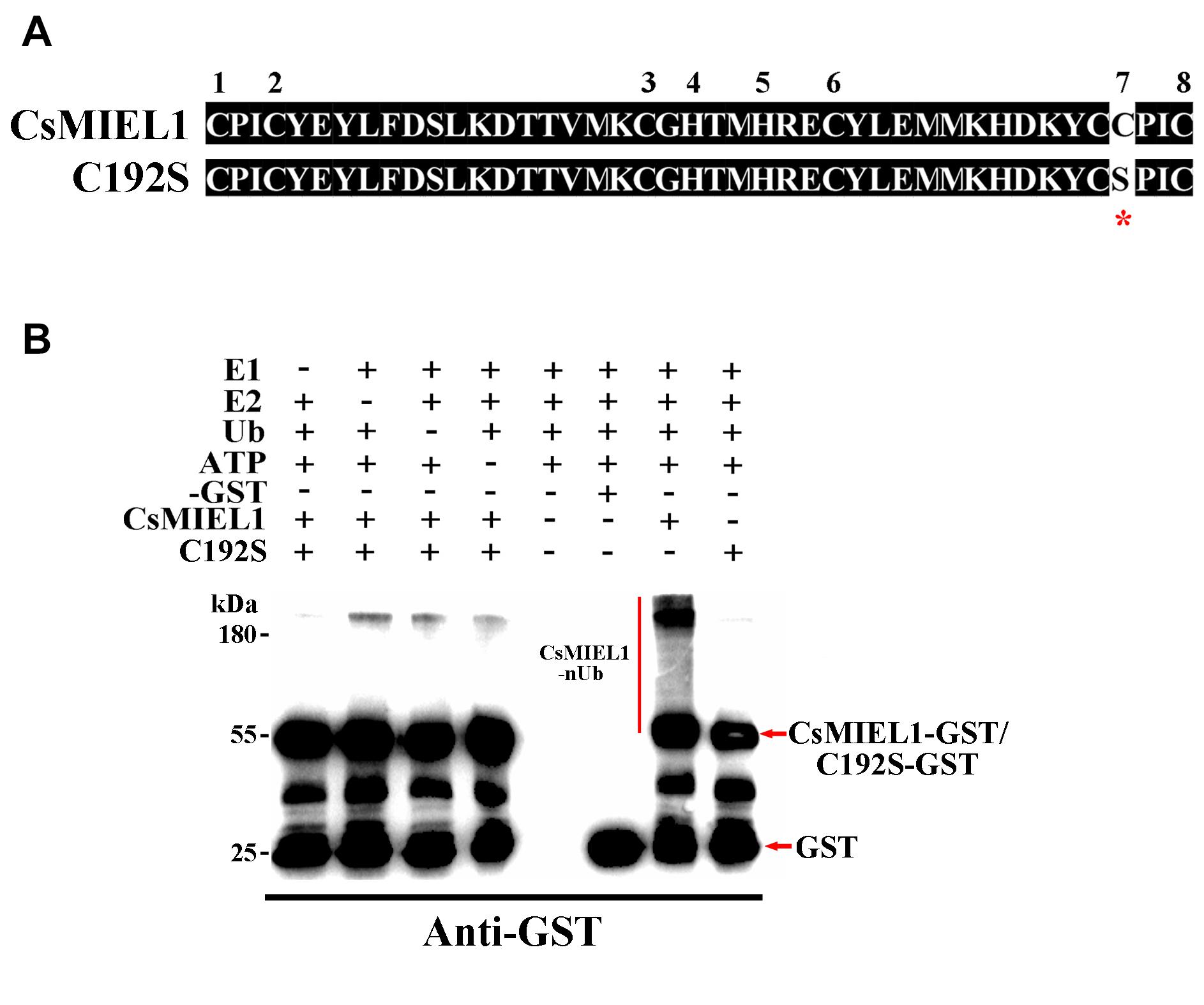
Figure 5. Verification of the activity of CsMIEL1. (A) Comparison of the amino acid sequence of CsMIEL1 and CsMIEL1-C192S. (B) In vitro ubiquitination analysis of CsMIEL1 and CsMIEL1-C192S. *Sited-directed mutagenesis position.
Overexpression of CsMIEL1 Alters Root Development Under Various Stresses
To investigate the functions of CsMIEL1, we obtained 35S: CsMIEL1-MYC and 35S: CsMIEL1-C192S-MYC overexpressing Arabidopsis plants. The four selected stress treatments were performed on the wild-type, CsMIEL1-MYC, and CsMIEL1-C192S-MYC, respectively (Figure 6A). For the transgenic plant seedlings and wild-type seedlings grown at room temperature for 10 days without any exogenous hormones, no significant differences existed across the growth indicators, including leaf length (LL) and width (WL), hypocotyl length (LH), and root number and length (Figure 6B).
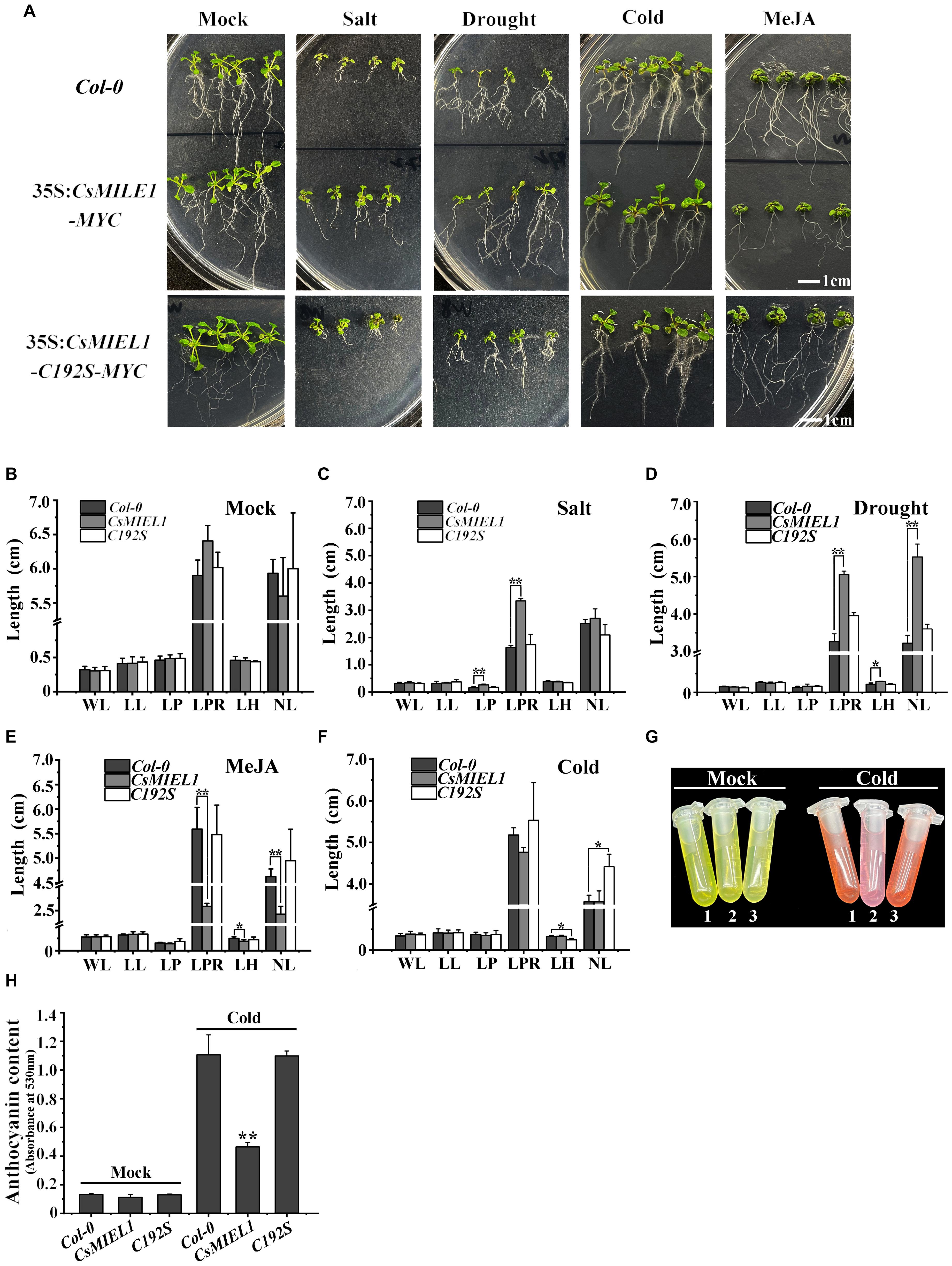
Figure 6. Resistance experiment of transgenic Arabidopsis plants to four stresses. (A) Four stress treatments for wild-type (Col-0), CsMIEL1, and CsMIEL1-C192S overexpressing Arabidopsis thaliana seedlings. (B–F) Data on each index of the three types of seedlings under the four stress treatments. Mock, Seedlings without stress treatment; WL, Width of leaf; LL, Length of leaf; LP, Length of petiole; LPR, Length of primary root; LH, Length of hypocotyl; NL, Number of lateral roots. (G,H) Anthocyanin concentration of three Arabidopsis genotype under cold stress and normal conditions. In all biological replicates, at least 50 plants were used for each treatment, and the error bars represent the standard deviation of replicates. The asterisks indicate the significance level (*P < 0.05, **P < 0.01) based on the LSD’s honestly significant difference test.
Under salt stress conditions, the growth of both transgenic plant seedlings and wild-type (WT) seedlings was inhibited. The growth of CsMIEL1-MYC transgenic plants was less pronounced than that of wild-type and CsMIEL1-C192S-MYC plants, which was reflected in the significantly longer length of the petiole (LP) and primary root (LPR) compared with the control (Figure 6C). Similarly, under drought stress, the growth of the transgenic plant and wild-type seedlings was also inhibited, but the length of the primary root (LPR) and hypocotyl (LH) and the number of lateral roots (NL) of CsMIEL1-MYC transgenic plants were significantly greater than those of the control and CsMIEL1-C192S-MYC (Figure 6D).
The effect of cold treatment on the growth of all seedlings was not particularly notable. The difference in growth indicators between CsMIEL1-MYC transgenic plants and WT did not reach a significant level, although its primary root length (LPT) was lower than these of wild-type and CsMIEL1-C192S-MYC transgenic plants (Figure 6F). Under low-temperature conditions, the anthocyanin levels of CsMIEL1-MYC transgenic plants were significantly lower than these in the control and CsMIEL1-C192S-MYC transgenic plants (Figures 6G,H).
The result in Figure 6E showed that CsMIEL1-overexpressing plants were hypersensitive to MeJA and that the growth of the root system of the CsMIEL1-MYC transgenic plant was significantly inhibited. Additionally, after these three genotypes were treated with exogenous MeJA, the primary root length (LPT), hypocotyl length (LH), and lateral root number (NL) of the CsMIEL1-overexpressing plants were shorter than those of the wild-type and CsMIEL1-C192S-MYC transgenic plants.
Discussion
RING finger proteins are vital in plant growth, development, and stress resistance (Deshaies and Joazeiro, 2009). Researchers have thoroughly investigated the RING finger family in Arabidopsis; at least 477 RING proteins have been identified (Stone et al., 2005; Yang et al., 2016), and many genes encoding RING domain proteins have been proven to be involved in cold stress (Dong et al., 2006; Suh et al., 2016), heat stress (Lim et al., 2013; Liu et al., 2016), drought stress (Lee et al., 2009; Ryu et al., 2010), salt stress (Qin et al., 2008; Fang et al., 2015), sugar treatment (Huang et al., 2010), and defense responses (Huang et al., 2010; Marino et al., 2019). Although RING proteins have been reported in many species, few studies have explored RING finger family proteins in tea plants. In this study, we could at least find 335 genes encoding RING proteins from tea plants genome. It can be seen that RING finger proteins is a superfamily in the plant kingdom.
In order to understand the role of RING finger proteins in the resistance of tea plants, we used the transcriptome sequencing data provided by the TPIA to select the RING finger genes involved in the resistance of tea plants to biotic and abiotic stresses. By comparing their gene expression differences, a total of 53 H2-type genes encoding RING proteins were found to respond to cold stress, drought stress, salt stress, and MeJA treatments. This also shows that the RING finger proteins family may be widely involved in the anti-stress effects of tea plants. Among them, TEA031033 was selected for function confirmation in this paper, due to its relatively high level of response to all four types of stress.
The protein cloned from TEA031033 is highly conserved with the MIEL proteins reported in other species (Lee and Seo, 2016; An et al., 2017), and named as CsMIEL1. CsMIEL1 contains a Zinc-finger domain at the N-terminus and a RING finger domain at the C-terminus, with 79.78% sequence identity with AtMIEL1 and 83% sequence identity with MdMIEL1. In vitro ubiquitination analysis has confirmed that CsMIEL1 has ubiquitin ligase activity, and its 192th amino acid Cys is closely related to its ubiquitination activity. Under exogenous hormones treatments, overexpressing CsMIEL1 transgenic plants showed a certain degree of resistance to salt stress and drought stress and were hypersensitive to exogenous MeJA. But C192S transgenic plants did not show the same response to these stresses as CsMIEL1, and most were no different from wild-type.
The lack of understanding of the targets of MIEL1 limits our understanding of the diversity of their responses to stress. E3 ubiquitin ligases often function by interacting with their target proteins. AtMYB30, acts as a positive regulator of the hypersensitive cell death program in plants, and it can interact with AtMIEL1 (Marino et al., 2019). Besides, AtMIEL1 can also change the response to ABA by interacting with AtMYB96 (Lee and Seo, 2016). MdMYB308L is a known target gene identified to interact with MIEL1. It positively regulates cold tolerance and anthocyanin accumulation by interacting with MdbHLH33 and enhancing its binding to MdCBF2 and MdDFR promoters, while apple RING E3 ubiquitin ligase MdMIEL1 decreases the cold tolerance and anthocyanin accumulation promoted by MdMYB308L and directly degrade MdMYB308L (An et al., 2017). The response of CsMIEL1 overexpressing plants to various stresses indicates the diversity of downstream target proteins. Consequently, the selection of CsMIEL1-interacting proteins is particularly critical for future research. In the previous studies, MIEL1 has a negative regulatory effect on both salt and drought stress, which is contrary to our results, while CsMIEL1’s regulation of cold stress was similar to MIEL1 in apples. Because E3s have a high selectivity for downstream proteins, we speculate that the downstream target protein of CsMIEL1 may not be homologous to the target protein reported in previous studies. We also need a variety of other methods (such as yeast library screening) to screen and identify the target protein.
Data Availability Statement
The datasets presented in this study can be found in online repositories. The names of the repository/repositories and accession number(s) can be found in the article/Supplementary Material.
Author Contributions
HR and GM were responsible for using the HMMer search sequence. TL was mainly responsible for the classification and deduplication of sequences. DX was responsible for the remaining work and writing of the manuscript. LG and TX conceived and designed the study. LG drafted the manuscript. All authors contributed to the article and approved the submitted version.
Funding
We thank the National Natural Science Foundation of China (31870677, 31870676, 32072621, and 31902069), National Key Research and Development Program of China (2018YFD1000601), and Natural Science Foundation of Anhui Province, China (1908085MC100).
Conflict of Interest
The authors declare that the research was conducted in the absence of any commercial or financial relationships that could be construed as a potential conflict of interest.
Publisher’s Note
All claims expressed in this article are solely those of the authors and do not necessarily represent those of their affiliated organizations, or those of the publisher, the editors and the reviewers. Any product that may be evaluated in this article, or claim that may be made by its manufacturer, is not guaranteed or endorsed by the publisher.
Acknowledgments
Thanks to Yang (China Agricultural University, Beijing, China) for providing E1, E2, and ubiquitin fusion proteins for in vitro ubiquitination assay. Thanks to Zheng (Anhui Agricultural University, Hefei, China) for supporting my work and accompanying me in daily life.
Supplementary Material
The Supplementary Material for this article can be found online at: https://www.frontiersin.org/articles/10.3389/fpls.2021.733287/full#supplementary-material
Footnotes
- ^ https://www.arabidopsis.org
- ^ http://pfam.xfam.org
- ^ http://tpia.teaplant.org/
- ^ http://www.hmmer.org/
- ^ http://www.ebi.ac.uk/interpro/
- ^ http://smart.embl.de/
- ^ http://www.megasoftware.net/
- ^ https://www.genoscope.cns.fr/externe/GenomeBrowser/Vitis/
References
An, J. P., Liu, X., Li, H. H., You, C. X., Wang, X. F., and Hao, Y. J. (2017). Apple RING E3 ligase MdMIEL1 inhibits anthocyanin accumulation by ubiquitinating and degrading MdMYB1 protein. Plant Cell Physiol. 58, 1953–1962. doi: 10.1093/pcp/pcx129
Avin-Wittenberg, T. (2019). Autophagy and its role in plant abiotic stress management. Plant Cell Environ. 42, 1045–1053. doi: 10.1111/pce.13404
Bachmair, A., Novatchkova, M., Potuschak, T., and Eisenhaber, F. (2001). Ubiquitylation in plants: a post-genomic look at a post-translational modification. Trends Plant Sci. 6, 463–470. doi: 10.1016/S1360-1385(01)02080-5
Barlow, P. N., Luisi, B., Milner, A., Elliott, M., and Everett, R. (1994). Structure of the C3HC4 Domain by 1H-nuclear magnetic resonance spectroscopy: A new structural class of zinc-finger. J. Mol. Biol. 237, 201–211. doi: 10.1006/jmbi.1994.1222
Borden, K. L., Boddy, M. N., Lally, J., O’Reilly, N. J., Martin, S., Howe, K., et al. (1995). The solution structure of the RING finger domain from the acute promyelocytic leukaemia proto-oncoprotein PML. EMBO J. 14, 1532–1541. doi: 10.1002/j.1460-2075.1995.tb07139.x
Clough, S. J., and Bent, A. F. (1998). Floral dip a simplified method for Agrobacterium -mediated transformation of Arabidopsis thaliana.pdf. Plant J. 16, 735–743. doi: 10.1046/j.1365-313x.1998.00343.x
Dai, J., and Mumper, R. J. (2010). Plant phenolics: extraction, analysis and their antioxidant and anticancer properties. Molecules 15, 7313–7352. doi: 10.3390/molecules15107313
Deshaies, R. J. (1999). SCF and Cullin/Ring H2-based ubiquitin ligases. Annu. Rev. Cell. Dev. Biol. 15, 435–467. doi: 10.1146/annurev.cellbio.15.1.435
Deshaies, R. J., and Joazeiro, C. A. (2009). RING domain E3 ubiquitin ligases. Annu. Rev. Biochem. 78, 399–434. doi: 10.1146/annurev.biochem.78.101807.093809
Dong, C. H., Agarwa, M., Zhang, Y., Xie, Q., and Zhu, J. K. (2006). The negative regulator of plant cold responses, HOS1, is a RING E3 ligase that mediates the ubiquitination and degradation of ICE1. Proc. Natl. Acad. Sci. U S A 103, 8281–8286. doi: 10.1073/pnas.0602874103
Downes, B. P., Stupar, R. M., Gingerich, D. J., and Vierstra, R. D. (2003). The HECT ubiquitin-protein ligase (UPL) family in Arabidopsis: UPL3 has a specific role in trichome development. Plant J. 35, 729–742. doi: 10.1046/j.1365-313X.2003.01844.x
Fang, H., Meng, Q., Xu, J., Tang, H., Tang, S., Zhang, H., et al. (2015). Knock-down of stress inducible OsSRFP1 encoding an E3 ubiquitin ligase with transcriptional activation activity confers abiotic stress tolerance through enhancing antioxidant protection in rice. Plant Mol. Biol. 87, 441–458. doi: 10.1007/s11103-015-0294-1
Freemont, P. S. (1993). The RING finger. A novel protein sequence motif related to the zinc finger. Ann. N. Y. Acad. Sci. 684, 174–192. doi: 10.1111/j.1749-6632.1993.tb32280.x
Fu, H., Girod, P. A., Doelling, J. H., van Nocker, S., Hochstrasser, M., Finley, D., et al. (1999). Structure and functional analysis of the 26S proteasome subunits from plants. Mol. Biol. Rep. 26, 137–146. doi: 10.1023/A:1006926322501
Gagne, J. M., Downes, B. P., Shiu, S. H., Durski, A. M., and Vierstra, R. D. (2002). The F-box subunit of the SCF E3 complex is encoded by a diverse superfamily of genes in Arabidopsis. Proc. Natl. Acad. Sci. U S A 99, 11519–11524. doi: 10.1073/pnas.162339999
Hou, X., Xie, K., Yao, J., Qi, Z., and Xiong, L. (2009). A homolog of human ski-interacting protein in rice positively regulates cell viability and stress tolerance. Proc. Natl. Acad. Sci. U S A 106, 6410–6415. doi: 10.1073/pnas.0901940106
Huang, Y., Li, C. Y., Pattison, D. L., Gray, W. M., Park, S., and Gibson, S. I. (2010). SUGAR-INSENSITIVE3, a RING E3 ligase, is a new player in plant sugar response. Plant Physiol. 152, 1889–1900. doi: 10.1104/pp.109.150573
Jiang, X., Hou, H., Zhang, S., Liu, Y., Wang, H., Deng, W.-W., et al. (2017). Comparison of phenolic compound accumulation profiles in eight evergreen woody core eudicots indicating the diverse ecological adaptability of Camellia sinensis. Sci. Horticult. 219, 200–206. doi: 10.1016/j.scienta.2017.03.018
Jones, A. M. (2001). Programmed cell death in development and defense. Plant Physiol. 125, 94–97. doi: 10.1104/pp.125.1.94
Koepp, D. M., Harper, J. W., and Elledge, S. J. (1999). How the cyclin became a cyclin regulated proteolysis in the cell cycle. Cell 97, 431–434. doi: 10.1016/S0092-8674(00)80753-9
Kosarev, P., Mayer, K. F., and Hardtke, C. S. (2002). Evaluation and classification of RING-finger domains encoded by the Arabidopsis genome. Genome Biol. 3:RESEARCH0016. doi: 10.1186/gb-2002-3-4-research0016
Kuki, Y., Ohno, R., Yoshida, K., and Takumi, S. (2020). Heterologous expression of wheat WRKY transcription factor genes transcriptionally activated in hybrid necrosis strains alters abiotic and biotic stress tolerance in transgenic Arabidopsis. Plant Physiol. Biochem. 150, 71–79. doi: 10.1016/j.plaphy.2020.02.029
Kurepa, J., Wang, S., and Smalle, J. (2012). The role of 26S proteasome-dependent proteolysis in the formation and restructuring of microtubule networks. Plant Signal. Behav. 7, 1289–1295. doi: 10.4161/psb.21543
Lee, H. G., Kim, J., Suh, M. C., and Seo, P. J. (2017). The MIEL1 E3 ubiquitin ligase negatively regulates cuticular wax biosynthesis in arabidopsis stems. Plant Cell Physiol. 58, 1249–1259. doi: 10.1093/pcp/pcx065
Lee, H. G., and Seo, P. J. (2016). The Arabidopsis MIEL1 E3 ligase negatively regulates ABA signalling by promoting protein turnover of MYB96. Nat. Commun. 7:12525. doi: 10.1038/ncomms12525
Lee, H. K., Cho, S. K., Son, O., Xu, Z., Hwang, I., and Kim, W. T. (2009). Drought stress-induced Rma1H1, a RING membrane-anchor E3 ubiquitin ligase homolog, regulates aquaporin levels via ubiquitination in transgenic Arabidopsis plants. Plant Cell 21, 622–641. doi: 10.1105/tpc.108.061994
Leon-Reyes, A., Van der Does, D., De Lange, E. S., Delker, C., Wasternack, C., Van Wees, S. C., et al. (2010). Salicylate-mediated suppression of jasmonate-responsive gene expression in Arabidopsis is targeted downstream of the jasmonate biosynthesis pathway. Planta 232, 1423–1432. doi: 10.1007/s00425-010-1265-z
Lim, S. D., Cho, H. Y., Park, Y. C., Ham, D. J., Lee, J. K., and Jang, C. S. (2013). The rice RING finger E3 ligase, OsHCI1, drives nuclear export of multiple substrate proteins and its heterogeneous overexpression enhances acquired thermotolerance. J. Exp. Bot. 64, 2899–2914. doi: 10.1093/jxb/ert143
Liu, J., Zhang, C., Wei, C., Liu, X., Wang, M., Yu, F., et al. (2016). The RING finger ubiquitin E3 Ligase OsHTAS enhances heat tolerance by promoting H2O2-induced stomatal closure in rice. Plant Physiol. 170, 429–443. doi: 10.1104/pp.15.00879
Malefo, M. B., Mathibela, E. O., Crampton, B. G., and Makgopa, M. E. (2020). Investigating the role of Bowman-Birk serine protease inhibitor in Arabidopsis plants under drought stress. Plant Physiol. Biochem. 149, 286–293. doi: 10.1016/j.plaphy.2020.02.007
Marino, D., Froidure, S., Canonne, J., Khaled, S. B., Khafif, M., Pouzet, C., et al. (2019). Addendum: Arabidopsis ubiquitin ligase MIEL1 mediates degradation of the transcription factor MYB30 weakening plant defence. Nat. Commun. 10:1475. doi: 10.1038/s41467-019-09341-4
Mazzucotelli, E., Belloni, S., Marone, D., De Leonardis, A. M., Guerra, D., Di Fonzo, N., et al. (2006). The E3 ubiquitin ligase gene family in plants_ regulation by degradation. Curr. Genom. 7, 509–522. doi: 10.2174/138920206779315728
Mistry, J., Finn, R. D., Eddy, S. R., Bateman, A., and Punta, M. (2013). Challenges in homology search: HMMER3 and convergent evolution of coiled-coil regions. Nucleic Acids Res. 41:e121. doi: 10.1093/nar/gkt263
Munns, R., and Tester, M. (2008). Mechanisms of salinity tolerance. Annu. Rev. Plant Biol. 59, 651–681. doi: 10.1146/annurev.arplant.59.032607.092911
Nakayama, K., Nagahama, H., Minamishima, Y. A., Matsumoto, M., Nakamichi, I., Kitagawa, K., et al. (2000). Targeted disruption of Skp2 results in accumulation of cyclin E and p27Kip1, polyploidy and centrosome overduplication. EMBO J. 19, 2069–2081. doi: 10.1093/emboj/19.9.2069
Nardelli, J., Gibson, T. J., Vesque, C., and Charnay, P. (1991). Base sequence discrimination by zinc-finger DNA-binding domains. Nature 349, 175–178. doi: 10.1038/349175a0
Qin, F., Sakuma, Y., Tran, L. S., Maruyama, K., Kidokoro, S., Fujita, Y., et al. (2008). Arabidopsis DREB2A-interacting proteins function as RING E3 ligases and negatively regulate plant drought stress-responsive gene expression. Plant Cell 20, 1693–1707. doi: 10.1105/tpc.107.057380
Ryu, M. Y., Cho, S. K., and Kim, W. T. (2010). The Arabidopsis C3H2C3-type RING E3 ubiquitin ligase AtAIRP1 is a positive regulator of an abscisic acid-dependent response to drought stress. Plant Physiol. 154, 1983–1997. doi: 10.1104/pp.110.164749
Shi, J., Ma, C., Qi, D., Lv, H., Yang, T., Peng, Q., et al. (2015). Transcriptional responses and flavor volatiles biosynthesis in methyl jasmonate-treated tea leaves. BMC Plant Biol. 15:233. doi: 10.1186/s12870-015-0609-z
Smalle, J., and Vierstra, R. D. (2004). The ubiquitin 26S proteasome proteolytic pathway. Annu. Rev. Plant Biol. 55, 555–590. doi: 10.1146/annurev.arplant.55.031903.141801
Stone, S. L. (2014). The role of ubiquitin and the 26S proteasome in plant abiotic stress signaling. Front. Plant Sci. 5:135. doi: 10.3389/fpls.2014.00135
Stone, S. L., Hauksdottir, H., Troy, A., Herschleb, J., Kraft, E., and Callis, J. (2005). Functional analysis of the RING-type ubiquitin ligase family of Arabidopsis. Plant Physiol. 137, 13–30. doi: 10.1104/pp.104.052423
Suh, J. Y., Kim, S. J., Oh, T. R., Cho, S. K., Yang, S. W., and Kim, W. T. (2016). Arabidopsis Toxicos en Levadura 78 (AtATL78) mediates ABA-dependent ROS signaling in response to drought stress. Biochem. Biophys. Res. Commun. 469, 8–14. doi: 10.1016/j.bbrc.2015.11.061
Tamura, K., Stecher, G., Peterson, D., Filipski, A., and Kumar, S. (2013). MEGA6: molecular evolutionary genetics analysis version 6.0. Mol. Biol. Evol. 30, 2725–2729. doi: 10.1093/molbev/mst197
van der Reijden, B. A., Erpelinck-Verschueren, C. A., Löwenberg, B., and Jansen, J. H. (1999). TRIADs: A new class of proteins with a novel cysteine-rich signature. Protein Sci. 8, 1557–1561. doi: 10.1110/ps.8.7.1557
Vierstra, R. (2003). The ubiquitin/26S proteasome pathway, the complex last chapter in the life of many plant proteins. Trends Plant Sci. 8, 135–142. doi: 10.1016/S1360-1385(03)00014-1
Vierstra, R. D. (2009). The ubiquitin-26S proteasome system at the nexus of plant biology. Nat. Rev. Mol. Cell. Biol. 10, 385–397. doi: 10.1038/nrm2688
Wang, X. C., Zhao, Q. Y., Ma, C. L., Zhang, Z. H., Cao, H. L., Kong, Y. M., et al. (2013). Global transcriptome profiles of Camellia sinensis during cold acclimation. BMC Genom. 14:415. doi: 10.1186/1471-2164-14-415
Yan, N., Yan, N., Doelling, J. H., Falbel, T. G., Durski, A. M., Vierstra, R. D., et al. (2000). The ubiquitin-specific protease family from arabidopsis.AtUBP1 and 2 are required for the resistance to the amino acid analog canavanine. Plant Physiol. 12, 1828–1843. doi: 10.1104/pp.124.4.1828
Yang, L., Liu, Q., Liu, Z., Yang, H., Wang, J., Li, X., et al. (2016). Arabidopsis C3HC4-RING finger E3 ubiquitin ligase AtAIRP4 positively regulates stress-responsive abscisic acid signaling. J. Integr. Plant Biol. 58, 67–80. doi: 10.1111/jipb.12364
Yang, L., Miao, M., Lyu, H., Cao, X., Li, J., Li, Y., et al. (2019). Genome-wide identification, evolution, and expression analysis of RING finger gene family in solanum lycopersicum. Int. J. Mol. Sci. 20:4864. doi: 10.3390/ijms20194864
Yoo, S. D., Cho, Y. H., and Sheen, J. (2007). Arabidopsis mesophyll protoplasts: a versatile cell system for transient gene expression analysis. Nat. Protoc. 2, 1565–1572. doi: 10.1038/nprot.2007.199
Zhang, Q., Cai, M., Yu, X., Wang, L., Guo, C., Ming, R., et al. (2017). Transcriptome dynamics of Camellia sinensis in response to continuous salinity and drought stress. Tree Genet. Genom. 13:78. doi: 10.1007/s11295-017-1161-9
Zhang, X., Liu, S., and Takano, T. (2008). Two cysteine proteinase inhibitors from Arabidopsis thaliana, AtCYSa and AtCYSb, increasing the salt, drought, oxidation and cold tolerance. Plant Mol. Biol. 68, 131–143. doi: 10.1007/s11103-008-9357-x
Zhao, Q., Liu, L., and Xie, Q. (2012). In vitro protein ubiquitination assay. Methods Mol. Biol. 876, 163–172. doi: 10.1007/978-1-61779-809-2_13
Keywords: Camellia sinensis, RING-containing proteins, E3 ubiquitin ligase, MIEL1, biological and abiotic stress
Citation: Xing D, Li T, Ma G, Ruan H, Gao L and Xia T (2021) Transcriptome-Wide Analysis and Functional Verification of RING-Type Ubiquitin Ligase Involved in Tea Plant Stress Resistance. Front. Plant Sci. 12:733287. doi: 10.3389/fpls.2021.733287
Received: 30 June 2021; Accepted: 29 September 2021;
Published: 21 October 2021.
Edited by:
Vagner A. Benedito, West Virginia University, United StatesReviewed by:
Dudy Bar-Zvi, Ben-Gurion University of the Negev, IsraelSandeep Chapagain, Louisiana State University, United States
Yuling Lin, Fujian Agriculture and Forestry University, China
Sung Chul Lee, Chung-Ang University, South Korea
Copyright © 2021 Xing, Li, Ma, Ruan, Gao and Xia. This is an open-access article distributed under the terms of the Creative Commons Attribution License (CC BY). The use, distribution or reproduction in other forums is permitted, provided the original author(s) and the copyright owner(s) are credited and that the original publication in this journal is cited, in accordance with accepted academic practice. No use, distribution or reproduction is permitted which does not comply with these terms.
*Correspondence: Liping Gao, gaolp62@126.com; Tao Xia, xiatao62@126.com