- 1Peking University Institute of Advanced Agricultural Sciences, Weifang, China
- 2Department of Plant Sciences, University of California, Davis, Davis, CA, United States
- 3College of Plant Protection, Shenyang Agricultural University, Shenyang, China
- 4US Department of Agriculture-Agricultural Research Service, Cereal Disease Laboratory and Department of Plant Pathology, University of Minnesota, St. Paul, MN, United States
- 5Howard Hughes Medical Institute, Chevy Chase, MD, United States
- 6State Key Laboratory of North China Crop Improvement and Regulation, College of Plant Protection, Hebei Agricultural University, Baoding, China
Wheat stem (or black) rust is one of the most devastating fungal diseases, threatening global wheat production. Identification, mapping, and deployment of effective resistance genes are critical to addressing this challenge. In this study, we mapped and characterized one stem rust resistance (Sr) gene from the tetraploid durum wheat variety Kronos (temporary designation SrKN). This gene was mapped on the long arm of chromosome 2B and confers resistance to multiple virulent Pgt races, such as TRTTF and BCCBC. Using a large mapping population (3,366 gametes), we mapped SrKN within a 0.29 cM region flanked by the sequenced-based markers pku4856F2R2 and pku4917F3R3, which corresponds to 5.6- and 7.2-Mb regions in the Svevo and Chinese Spring reference genomes, respectively. Both regions include a cluster of nucleotide binding leucine-repeat (NLR) genes that likely includes the candidate gene. An allelism test failed to detect recombination between SrKN and the previously mapped Sr9e gene. This result, together with the similar seedling resistance responses and resistance profiles, suggested that SrKN and Sr9e may represent the same gene. We introgressed SrKN into common wheat and developed completely linked markers to accelerate its deployment in the wheat breeding programs. SrKN can be a valuable component of transgenic cassettes or gene pyramids that includes multiple resistance genes to control this devastating disease.
Introduction
The total world human population is expected to increase 35% by 2050, which will require an increase of current food production levels by 70–100% (Godfray et al., 2010). Wheat, Triticum aestivum L. (2n = 6x = 42, AABBDD) and Triticum turgidum subsp. durum (Desf.) Husn. (2n = 4x = 28, AABB), provide roughly 20% of calories consumed by the human population and play a major role in global food security. To achieve further increases in wheat production, it is critical to reduce yield losses caused by the fungal pathogens. Puccinia graminis f. sp. tritici (Pgt), the causal agent of wheat stem (or black) rust, is one of the most yield-limiting diseases throughout the wheat-growing regions worldwide (Leonard, 2001). For the past several decades, stem rust has been effectively controlled by the use of genetic resistance and eliminating the alternate host barberry (Berberis vulgaris L.) (Peterson et al., 2005; Singh et al., 2015).
Unfortunately, this disease reemerged as a serious threat with the detection of a highly virulent isolate TTKSK (also known as Ug99) in Uganda in 1998. Ug99 is virulent to most of the deployed stem rust resistance genes, such as the widely deployed Sr31 gene (Pretorius et al., 2000; Jin et al., 2007). Currently, 13 variants in the Ug99 lineage have been detected in the 13 countries extending from Africa to Asia (Nazari et al., 2009; Bhardwaj et al., 2019). Additional challenges are emerging from the appearance of other virulent races unrelated to the Ug99 race group, such as TRTTF, JRCQC, TKTTF, and TTRTF (Olivera et al., 2012, 2015; Tesfaye et al., 2020).
The non-Ug99 race TRTTF, which was first discovered in Yemen and subsequently in East Africa, defeated the resistance conferred by genes SrTmp, Sr36, and Sr1RSAmigo that are effective against Ug99 (Olivera et al., 2012). The races TRTTF and JRCQC overcame the resistance provided by genes Sr9e and Sr13 (Olivera et al., 2012), which are important sources of stem rust resistance in many commercial durum wheat cultivars (Periyannan et al., 2014; Singh et al., 2015). Virulent race TKTTF was responsible for a severe stem rust epidemic in the south of Ethiopia and caused nearly 100% yield losses on the Ug99 resistant wheat variety “Digalu” (Olivera et al., 2015). Another race of concern is TTRTF, which was first identified in Georgia in 2014 (Olivera et al., 2019), and subsequently spread to more countries, such as Hungary, Egypt, and Ethiopia (Tesfaye et al., 2020). Since Pgt has already demonstrated its ability for rapid spread and evolution, additional sources of resistance are needed to diversify the combinations of deployed Sr genes, including those from the primary wheat gene pool.
Triticum turgidum ssp. durum, which is part of the wheat primary gene pool, is grown in about 18 million ha worldwide with an annual production of approximately 35 million tons (Cakmak et al., 2010). Tetraploid wheat (T. turgidum ssp.) has contributed several stem rust resistance genes, including Sr9d/Sr9e/Sr9g, Sr11, Sr12, Sr13a/Sr13b, Sr14, and Sr17 (Bariana, 2008; Singh et al., 2011, 2015; Zhang et al., 2017). The recent development of next-generation sequencing (NGS) and genome-wide high throughput genotyping platforms, such as the Illumina iSelect single nucleotide polymorphism (SNP) array (Illumina Inc., CA, USA) (Wang et al., 2014) and the wheat exome capture (Krasileva et al., 2017), have accelerated the identification of new stem rust resistance genes (Letta et al., 2014; Nirmala et al., 2017; Miedaner et al., 2019; Megerssa et al., 2020).
The durum wheat variety “Kronos” (PI 576168) developed by Arizona Plant Breeders Inc. (AZ, USA) was previously postulated to carry Sr13 and a second TRTTF resistance gene, temporarily designated as SrKN (Zhang et al., 2017). Sr13 has been cloned and encodes a typical coiled-coil nucleotide-binding leucine-rich repeat protein (Zhang et al., 2017). The objectives of this study were to: (1) characterize and genetically map SrKN; (2) identify the corresponding regions in the different sequenced wheat genomes; and (3) introgress the chromosome segment carrying SrKN into hexaploid wheat.
Materials and Methods
Plant Materials and Mapping Population
To map the TRTTF resistance gene, the Kronos sr13 mutant line T4-3102, carrying a premature stop codon in the LRR domain, was crossed with the susceptible durum line Rusty (Klindworth et al., 2006). For the initial map, we evaluated a subset of 90 F2 plants with Pgt race TRTTF (isolate 06YEM34-1) and a separate subset of 145 F2 plants from the same population with BCCBC (isolate 09CA115-2). We tested the observed segregation ratios using χ2 tests.
For the construction of the high-resolution genetic map, we selected four F2 plants (plants 17, 31, 47, and 87) heterozygous for the SrKN candidate region using molecular markers and produced 1,468 F3 plants. These plants were genotyped with SrKN flanking markers to identify recombination events in the candidate gene region. The plants carrying these recombination events and their F4 progenies were challenged with Pgt races BCCBC and 34MKGQM.
To evaluate the resistance profile of SrKN to multiple Pgt races, we developed a pair of F5 sister lines homozygous for the presence (Td31-5R) or absence (Td31-7S) of SrKN using molecular markers and their levels of resistance to race BCCBC. This additional criterion was used to eliminate a minor Sr resistance gene present in T4-3102 that confers a mild resistance to BCCBC but not to TRTTF (as shown in the Results section). Td31-7S was F4 plant number 7 from F3 family 31, which was very susceptible to BCCBC. Td31-5R was F4 plant number 5 from the same segregating family, which carried the SrKN based on the flanking markers, but that showed an intermediate resistance reaction to BCCBC (we assumed that the very resistant parental line T4-3102 carries both genes).
Finally, we used a collection of 23 accessions of T. turgidum ssp. durum and 16 of T. aestivum to determine the value of the closely linked markers identified in this study for marker-assisted selection.
Stem Rust Assays
The infection types (IT) of mutant line T4-3102 and Rusty to Pgt races TTKSK (isolate 04KEN156/04), TRTTF (06YEM34-1), TKTTF (13ETH18-1), and JRCQC (09ETH08-3) were reported in the previous study (Zhang et al., 2017). In this study, the parental lines T4-3102 and Rusty, and their segregating populations were re-evaluated with races TRTTF (06YEM34-1) and BCCBC (09CA115-2) at the United States Department of Agriculture-Agricultural Research Service (USDA-ARS) and Cereal Disease Laboratory and the University of California, Davis (UCD), respectively. Evaluations with four Chinese Pgt races 21C3CTTTM (20GH13), 34MKGQM (20IAL06), 34MTGSM (20GSA1), and 34C3RTGQM (20IAL32) were performed at Peking University Institute of Advanced Agricultural Sciences, Weifang, Shandong, China.
The avirulence/virulence formulae of the Pgt races used in this study are presented in Supplementary Table S1. The procedures for inoculation were as described previously (Rouse et al., 2011) and ITs were scored using a 0–4 scale also described before (Stakman et al., 1962; Rouse et al., 2011; Chen et al., 2015). The additional symbols “+” or “–” were used to indicate larger or smaller pustules within the same IT (Roelfs and Martens, 1988).
Wheat 90K iSelect Assay
Genomic DNA of the parents and F2 plants was extracted using the cetyltrimethylammonium bromide (CTAB) method (Murray and Thompson, 1980). The quality and quantity of DNA were measured using a NanoDrop Spectrophotometer (Thermo Fisher Scientific, MA, USA) and normalized to 50 ng/μl. We genotyped the parental lines and 46 F2 plants at the USDA-ARS Small Grain Genotyping Lab at Fargo (ND, USA) with the wheat 90K SNP iSelect Illumina platform (Wang et al., 2014). The SNP genotype calling was processed using Illumina GenomeStudio v.2011.1 (Illumina Inc., CA, USA). The polymorphic SNP markers with more than 20% missing values were removed.
Marker Development
Once the linked SNPs were identified using the 90K SNP array, their flanking sequences were used to perform BLASTN (Basic Local Alignment Search Tool for nucleotide sequence) searches in the reference genomes of hexaploid wheat Chinese Spring (CS) (The International Wheat Genome Sequencing Consortium, 2018) and tetraploid wheat Svevo (Maccaferri et al., 2019) to define the SrKN candidate region in these two genomes. To accelerate the development of markers in the candidate region, we performed exome-capture for the susceptible parent Rusty (accession number PRJNA751176), since the sequence of Kronos assembly (Walkowiak et al., 2020) was already available. Genomic library preparation, exome capture, sequencing, and data analysis were conducted using the same methods as described before (Krasileva et al., 2017; Mo et al., 2018). We aligned the Rusty and Kronos sequences of the genes in the candidate region, identified the polymorphic sites, and generated sequence-based markers spaced throughout the candidate gene region.
DNA amplification was carried out in a Veriti 96-Well Fast Thermal Cycler with the following thermal cycling profile: an initial denaturation step of 94°C for 3 min, followed by 35 cycles consisting of 94°C for 30 s, annealing at 50–65°C for 30 s, and extension at 72°C for 60 s, ending with a final step at 72°C for 10 min. After the PCR amplification, 10 μl PCR products were subjected to agarose gel electrophoresis (~1.5% agarose), and the gels were stained with ethidium bromide.
Allelism Test
The tetraploid durum wheat variety Vernal was originally hypothesized to have both Sr9e and Sr13 (Saini et al., 2018). However, using a published diagnostic marker for Sr13 (Zhang et al., 2017), we found that Vernal carries the Sr13 susceptible haplotype (S7). To obtain the Sr9e monogenic line, Vernal was crossed with susceptible line Rusty, and the resulting F1 was backcrossed two times with Rusty. The presence of the Vernal allele in the Sr9e region was monitored during backcrossing using the cleaved amplified polymorphic sequence (CAPS) markers pku4861F7R7 and pku4922F1R2. The Sr9e monogenic line (referred hereafter as Vernal-BF9e) was selected from the BC2F2 plants. An allelism test between SrKN and Sr9e was carried out using 470 F2 plants derived from the cross between the monogenic lines Td31-5R (SrKN) × Vernal-BF9e (Sr9e) inoculated with Pgt race 34MKGQM.
Transferring of T. durum Segment Carrying SrKN Into Hexaploid Wheat
Triticum turgidum subsp. durum wheat variety Kronos was crossed with the T. monococcum wheat accession PI 306540 (AmAm) as described before (Chen et al., 2020). The resulting F1 triploid plants were completely male sterile and were crossed with common wheat variety Clear White (PVP 2004-00244). Next, the F1 plants were backcrossed to the hexaploid wheat line Fielder. Flanking and completely linked PCR markers (Table 2) were used to validate the presence of Kronos segment, including SrKN during backcrossing. One BC2F2 plant heterozygous for the SrKN candidate chromosome region and without other Sr resistance genes was self-pollinated. The selected BC2F3 plants were divided into two groups and inoculated with Pgt races 34MKGQM and 34C3RTGQM, respectively. After phenotyping, the BC2F3 plants homozygous for SrKN were transplanted and then, self-pollinated to generate the BC2F4 seeds.
Statistical Analyses
We mapped the Rusty reads from the exome capture on the Kronos assembly and called SNPs using SAMtools. We generated the pileup files and used BCFtools for variant calling (http://samtools.sourceforge.net/). The variants with a sequencing depth of ≤ 5 and mapping quality of ≤ 50 were removed for subsequent analysis. The polymorphic markers and the stem rust resistance phenotypes were used to construct the genetic linkage maps using the software JoinMap 4.0 and MapChart 2.2 (Kyazma BV, Wageningen, Netherlands; https://www.wur.nl/en/show/Mapchart.htm) (Stam, 1993; Voorrips, 2002; Van Ooijen, 2006). The BLASTN searches against the hexaploid wheat CS (https://wheat-urgi.versailles.inra.fr/Seq-Repository/BLAST), and the tetraploid wheat Svevo and Kronos (https://wheat.pw.usda.gov/blast/) were used to assist the marker development.
Results
Characterization of Stem Rust Resistance in Durum Wheat Line T4-3102
In the seedling tests, the durum wheat line T4-3102 displayed resistant ITs (ITs = 1; to 1+) to Pgt race TRTTF (isolate 06YEM34-1), whereas Rusty exhibited ITs of “3+” to “4” (Figure 1A). In a subset of 90 F2 plants from the cross, T4-3102 × Rusty evaluated with TRTTF, the plants with ITs ranging between “1;” and “1+” (similar to T4-3102) were classified as resistant and those with ITs from “3+” to “4” (similar to Rusty) were recorded as susceptible (Figure 1A). Among them, 69 plants were resistant and 21 were susceptible, which fits well the 3:1 (resistant:susceptible) segregation ratio expected for a single genetic locus (χ2 = 0.13, P = 0.72).
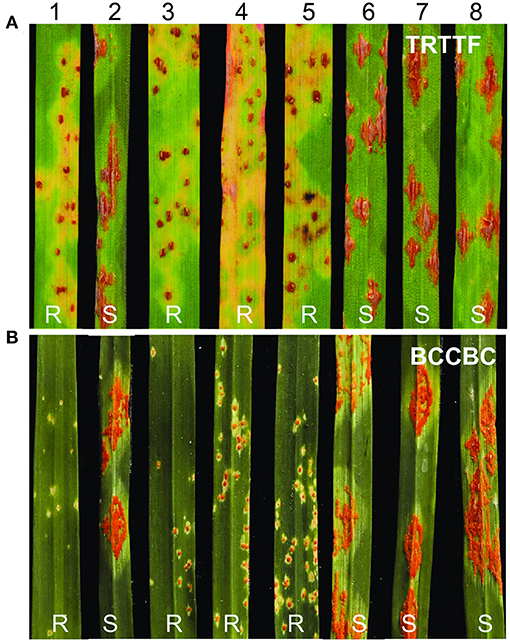
Figure 1. Reactions to Pgt races TRTTF and BCCBC in segregating population. (A) Inoculated with race TRTTF. (B) Inoculated with race BCCBC. 1, T4-3102 (SrKN); 2, Rusty; 3–5, resistant plants; 6–8, susceptible plants. R, resistant; S, susceptible.
In seedling of the two parental lines inoculated with race BCCBC (09CA115-2), T4-3102 exhibited high levels of resistance (ITs = 0; to 1–), whereas Rusty was fully susceptible (ITs = 3+ to 4; Figure 1B). We evaluated another subset of 145 F2 individuals from the same population with race BCCBC and found some F2 plants with intermediate reactions (Supplementary Figure S1), likely due to additional minor Sr gene(s) in T4-3102 resistant to this race. We converted the Pgt reactions into two genotypic classes for the mapping purposes: ITs ranging from “0” to “2–” were considered as resistant and ITs from “3+” to “4” as susceptible [20 plants with intermediate reactions (ITs = “2+” to “3”) were discarded in the classification]. Among the 125 F2 plants showing clear phenotypic segregation, we observed 97 resistant plants and 28 susceptible ones, which did not deviate from the expected 3:1 segregation ratio for a single dominant gene (χ2 = 0.45, P = 0.50).
Mapping of a Stem Rust Resistance Gene on Chromosome Arm 2BL
For the initial mapping, we genotyped the parental lines and the more susceptible and resistant lines from the two sub-populations evaluated with TRTTF and BCCBC using the 90K SNP iSelect Illumina assay. For the 90 F2 plants inoculated with race TRTTF, we genotyped 10 resistant and 10 susceptible plants, whereas, for the 125 F2 plants challenged with race BCCBC, we genotyped 13 resistant and 13 susceptible phenotypes. We identified 4,652 polymorphic SNPs with <20% missing data between T4-3102 and Rusty. Of those, we detected 19 SNPs (Table 1) on the long arm of chromosome 2B that were significantly correlated with both TRTTF and BCCBC resistance phenotypes, suggesting that the same Sr gene was conferring resistance to both races. These SNPs were distributed from 106.5 to 119.6 cM (Table 1) in the 90K consensus map of chromosome 2B (https://triticeaetoolbox.org/wheat/). Based on a preliminary linkage map constructed using the 46 genotyped plants (Figure 2A), the TRTTF- and BCCBC-resistance gene SrKN was mapped to a 3.2 cM region between the SNPs IWB73343 and IWB35200.
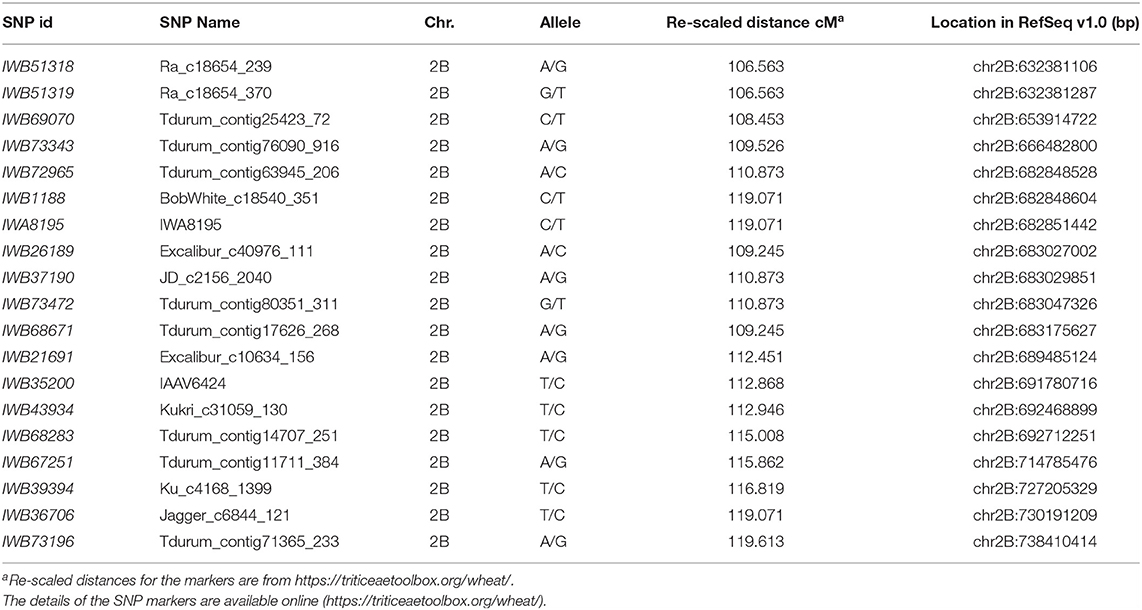
Table 1. The single nucleotide polymorphisms (SNPs) linked with SrKN and their locations in the Chinese Spring (CS) reference genome RefSeq v1.0 coordinates.
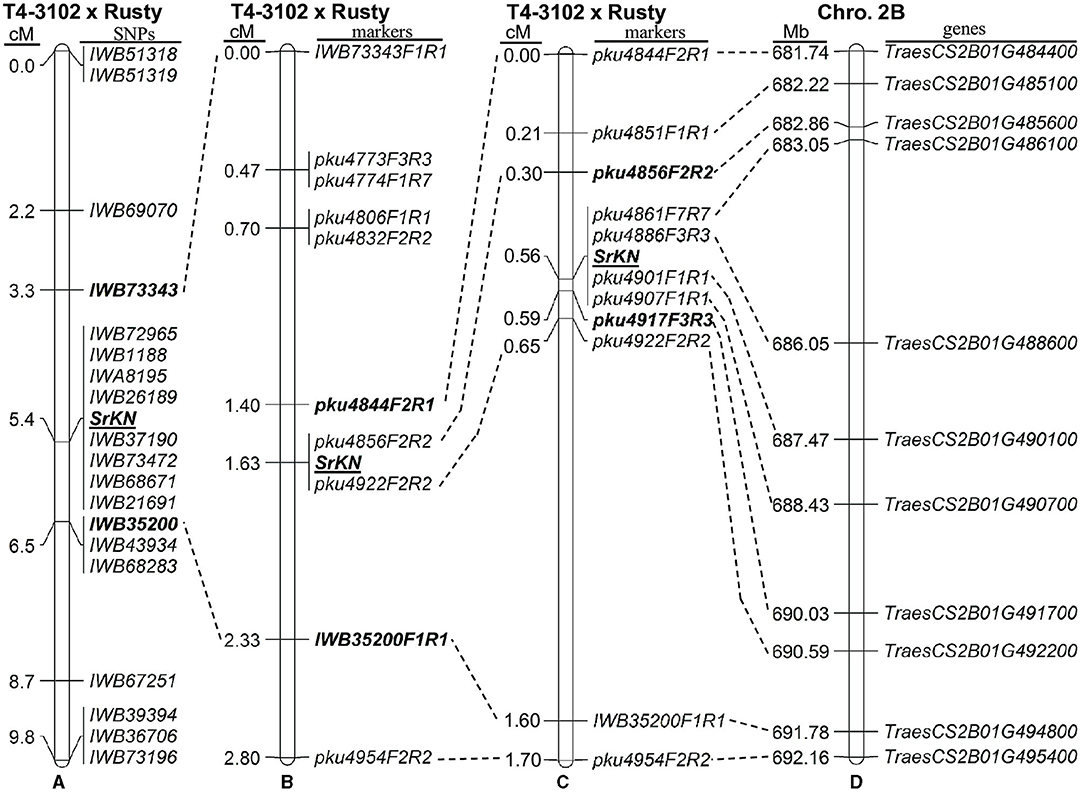
Figure 2. Genetic maps of SrKN on chromosome arm 2BL. (A) Initial map based on 46 F2 plants and wheat 90K single nucleotide polymorphism (SNP) iSelect array; (B) Genetic map based on 215 F2 plants and 10 molecular markers; (C) High-density map based on 1,683 F2 plants and 11 molecular markers; (D) Colinear region in the sequenced Chinese Spring (CS) reference genome (RefSeqv1.0).
Using the sequences flanking the target SNPs, we performed BLASTN searches against the reference genome of hexaploid wheat CS (RefSeqv1.0). This defined a candidate gene region on the long arm of chromosome 2B extending from 666.5 to 691.8 Mb (Table 1). Since flanking SNP markers IWB73343 and IWB35200 were located within the wheat genes TraesCS2B01G470100 and TraesCS2B01G494800, we developed B-genome specific PCR markers IWB73343F1R1 and IWB35200F1R1 (Table 2) using these two genes sequences. Using these markers, we genotyped the 215 F2 plants previously phenotyped with races TRTTF (90 plants) and BCCBC (125 plants), which provided a better estimate of the genetic length of the candidate region (2.3 cM). Based on this new data, SrKN was mapped 1.6 cM distal to IWB73343F1R1 and 0.7 cM proximal to IWB35200F1R1 (Figure 2B). We then developed molecular markers for seven additional genes within the candidate gene region (Figure 2B; Table 2) and mapped SrKN between CAPS markers pku4844F2R1 and IWB35200F1R1, and completely linked to markers pku4856F2R2 and pku4922F2R2 (Figure 2B).
To define the position of SrKN more precisely, we screened another 1,468 plants from four selected segregating F3 families with the new flanking markers pku4844F2R1 and IWB35200F1R1. The distance between these two flanking markers was estimated to be 1.6 cM based on the 50 plants with recombination events identified in this screen and the four recombinants identified between these same markers in the previous 215 plants. For these 54 informative F3 families, we performed progeny tests (25 plants per family) with races BCCBC and 34MKGQM in growth chambers. Using these new recombination events and six new markers developed in this region (Table 2; Supplementary Figure S2), we further delimited the SrKN candidate region to a 0.29-cM interval (7.2-Mb, CS RefSeq v1.0 coordinates) flanked on the proximal side by marker pku4856F2R2 (0.26 cM) and on the distal side by pku4917F3R3 (0.03 cM) (Figure 2C).
Candidate Genes for SrKN Within the Colinear Regions of Tetraploid and Hexaploid Wheat Genomes
The 0.29 cM candidate region between the markers pku4856F2R2 and pku4917F3R3 defines a 5.6-Mb region in T. turgidum ssp. durum cv. Svevo (672.6–678.2 Mb, Supplementary Table S2) and a 7.2-Mb region in T. aestivum cv. CS (682.8–690.0 Mb, Figure 2D; Supplementary Table S3). These candidate gene regions include 52 annotated high-confidence genes in Svevo (TRITD2Bv1G223060–TRITD2Bv1G224370) and 59 in Chinese Spring (TraesCS2B02G485600–TraesCS2B02G491700) (Figure 2D). These genes included nine typical NBS-LRR (NLR) in Svevo and six in CS, which is of particular interest for this project because NLRs are the most frequent gene class associated with disease resistance in the plants.
Among the 52 genes annotated in the candidate gene region in the Svevo genome, we found that 35 of them were expressed in Kronos, based on BLASTN searches in the published Kronos transcriptome database (Krasileva et al., 2013) (https://dubcovskylab.ucdavis.edu/wheat_blast). The expressed genes include seven of the nine annotated NLR genes (TRITD2Bv1G223210, TRITD2Bv1G223370, TRITD2Bv1G223450, TRITD2Bv1G223460, TRITD2Bv1G223490, TRITD2Bv1G223550, and TRITD2Bv1G223640). We designed two to four pairs of primers for each of the seven expressed NLR genes and all of them amplified the expected bands in Kronos genomic DNA (Supplementary Table S4). By contrast, only two of the 22 primers pairs (TRI2B223210F7R7 and TRI2B223490F3R3) amplified products in Rusty, suggesting that these NLRs may be absent in Rusty (or partially deleted). To rule out the possibility that the lack of amplification in Rusty was caused by degraded DNA, the same genomic DNAs were tested with the primers pku4856F2R2, pku4861F7R7, pku4886F3R3, pku4907F1R1, and pku4917F3R3 (Table 2) and the expected bands were obtained in Rusty (Supplementary Table S4). We cannot rule out the possibility that some of the primers that failed to amplify the Rusty genomic DNA were caused by polymorphisms in the primer regions rather than by the absence of the genes.
Comparison of Mapping Positions and Resistance Profiles of SrKN, Sr9, and Sr28 Resistance Genes Located on Chromosome Arm 2BL
Comparison of Map Locations
Two wheat stem rust resistance genes, Sr9 and Sr28, were previously mapped close to SrKN on chromosome arm 2BL (Rouse et al., 2012, 2014; Yu et al., 2014). To compare their relative map positions, we used the simple sequence repeat (SSR) marker wmc332 that was previously shown to be linked to Sr9 and Sr28 (Rouse et al., 2012, 2014). We mapped wmc332 in the population of 215 F2 plants mentioned above and found that SrKN is located 13.7 cM proximal to this marker (Figure 3A), whereas Sr28 was mapped roughly 5.8 cM distal to the same marker (Figure 3B) (Rouse et al., 2012). These results suggest that SrKN and Sr28 are two different loci located about 20 cM apart (Figure 3).
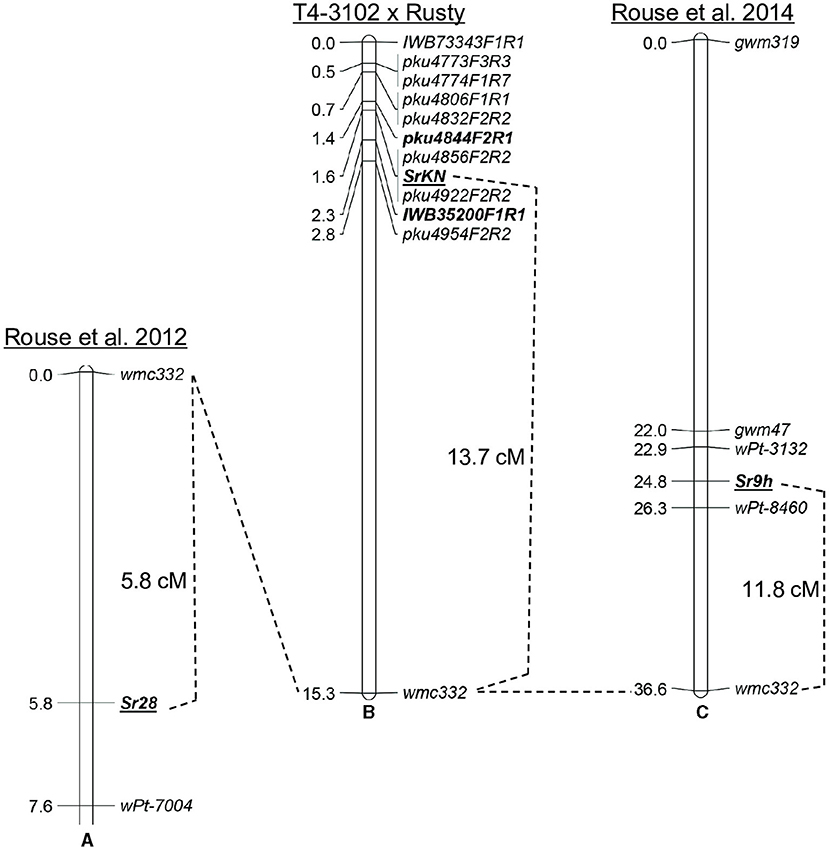
Figure 3. Relative map position of Sr28, SrKN, and Sr9h. (A) Genetic map of Sr28 derived from the population LMPG-6 × SD 1691 (Rouse et al., 2012); (B) Genetic map of SrKN from the population T4-3102 × Rusty in the present study; (C) Genetic map of Sr9h from the population Gabo 56 × CS (Rouse et al., 2014).
The Sr9 gene has multiple alleles that include Sr9a, Sr9b, Sr9d, Sr9e, Sr9f, Sr9g, and Sr9h (McIntosh et al., 2013; Rouse et al., 2014). The gene Sr9h was mapped 11.8 cM proximal to wmc332 (Rouse et al., 2014), indicating that SrKN and Sr9h loci can be close to each other or represent the same gene (Figures 3B,C). To test this hypothesis, we performed an allelism test using a BC2F2 monogenic line for Sr9e derived from the durum wheat variety Vernal (Vernal-BF9e) crossed by the monogenic SrKN line Td31-5R (as shown in Material and methods for the development of these lines). None of the 470 F2 plants generated from this cross inoculated with Pgt race 34MKGQM showed a susceptible reaction suggesting that Sr9 and SrKN are allelic.
Comparison of Resistance Profiles
Inoculation of the SrKN monogenic line Td31-5R and its sister line Td31-7S lacking SrKN with different Pgt races showed that this gene is ineffective against the evaluated races TTKSK, TKTTF, and JRCQC but confers resistance to the races BCCBC, TRTTF, 21C3CTTTM, 34MKGQM, 34MTGSM, and 34C3RTGQM (Supplementary Figure S3a; Table 3). Sr28 was evaluated against race TRTTF and another four races from China and was not effective against any of them (Li et al., 2016, 2018; Babiker et al., 2017) (Table 3), supporting the conclusion from the genetic data that Sr28 and SrKN are two different genes.
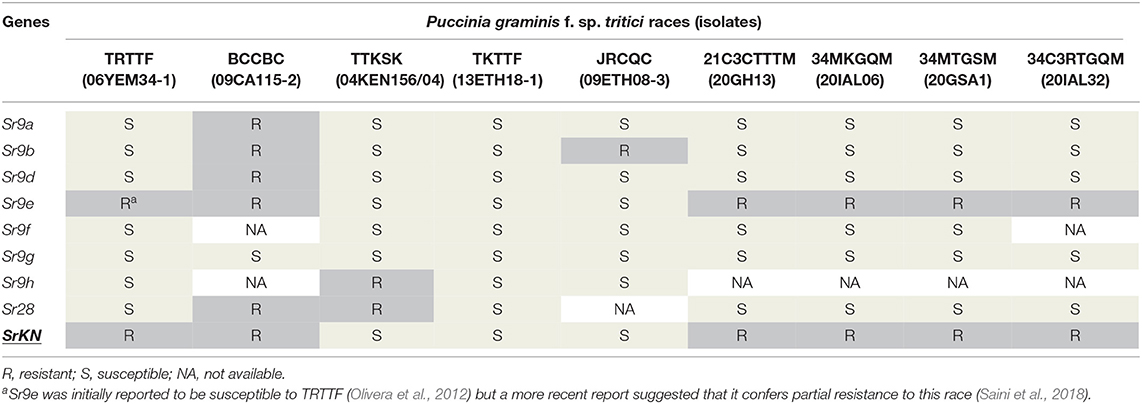
Table 3. The resistance profiles of Sr9 alleles, Sr28, and SrKN to multiple Puccinia graminis f. sp. tritici races.
Among the different Sr9 alleles, the most similar profile to SrKN was found for Sr9e. These two genes showed similar reactions for eight of the nine races tested, and differed only for race TRTTF for which SrKN was resistant and Sr9e was reported to be susceptible (Olivera et al., 2012) (Table 3). However, a more recent report suggested that Sr9e confers partial resistance to race TRTTF (Saini et al., 2018), which would result in identical profiles between SrKN and Sr9e. We also challenged the hexaploid line Vernstein, which is known to carry the Sr9e allele, with the Chinese race 34MKGQM and found a similar level of Pgt resistance to that conferred by lines Vernal-BF9e and Td31-5R (Supplementary Figure S3).
The alleles Sr9a, Sr9b, Sr9d, and Sr9g differ from SrKN by their susceptibility to races TRTTF, 34C3RTGQM, 34MKGQM, 34MTGSM, and 21C3CTTTM (Table 3). In addition, the Sr9f allele was shown to be ineffective to 21C3CTTTM, 34MKGQM, and 34MTGSM (Li et al., 2018) suggesting that Sr9 alleles Sr9a, Sr9b, Sr9d, Sr9f, and Sr9g have a very different resistance profile than SrKN. Finally, Sr9h was shown to be resistant to TTKSK (Rouse et al., 2014), whereas SrKN was ineffective against this race. In summary, based on the currently available information, the most similar Sr9 allele to SrKN is Sr9e.
Detection of SrKN and/or Sr9e Resistance Based on the Haplotype of Linked Markers
To determinate the value of the haplotype defined by the two flanking markers and three completely linked polymorphisms, we developed one Insertion/deletion (InDel) and four CAPS markers and used them to screen a panel of durum and bread wheat lines. The same lines were evaluated with Pgt race 34MKGQM (Supplementary Table S5). T4-3102 (SrKN) and Vernal (Sr9e) showed an identical haplotype indicating that these five markers are not sufficient to differentiate these genes/alleles. By contrast, Rusty differed from T4-3102 in all the five polymorphisms (Supplementary Table S5) indicating a very different haplotype.
Among the 20 durum lines compared with T4-3102 (SrKN), Vernal (Sr9e), and Rusty, only Svevo and Langdon showed a haplotype identical to SrKN and Sr9e. These four lines also displayed a similar resistance response against race 34MKGQM, suggesting that Svevo and Langdon might carry SrKN or Sr9e. Eleven lines carried the Rusty haplotype and were susceptible to 34MKGQM (Supplementary Table S5), confirming the absence of SrKN in these lines. Among the other seven lines, four showed the same haplotype as Rusty but higher levels of resistance than T4-3102 suggesting the presence of other Sr genes. The last three lines showed different haplotypes from the three control lines and resistance levels higher than SrKN or Sr9e, also suggesting the presence of other Sr genes (Supplementary Table S5). Indeed, four of them were confirmed to carry the cloned gene Sr13 and the line PI 94701 was known to possess the resistance gene Srdp2 (Rondon et al., 1966) (Supplementary Table S5).
Among the 16 hexaploid wheat lines analyzed, we detected the SrKN/Sr9e haplotype in Vernstein (Sr9e), CnSr9g, and ISr9a-Ra, suggesting that these markers were not able to differentiate SrKN from Sr9g and Sr9a. All the tested hexaploid wheat lines were susceptible to race 34MKGQM except Vernstein (Supplementary Table S5). In summary, the five polymorphisms seem to be useful to predict the presence of the SrKN allele, but they cannot differentiate it from the more susceptible alleles Sr9g and Sr9a.
Transfer of Stem Rust Resistance to Hexaploid Wheat Background
To transfer the resistance gene SrKN to hexaploid wheat, we took advantage of the crosses previously used to transfer several T. monococcum resistance genes into hexaploid wheat (Figure 4). We first crossed Kronos with the T. monococcum accession PI 306540 (AmAm), which carries the additional stem rust resistance genes Sr21, Sr60, SrTm4, and SrTm5 (Briggs et al., 2015; Chen et al., 2018a,b; Chen et al., 2020). The resulting F1 triploid plants were crossed with common wheat variety Clear White (PVP 2004-00244) and then backcrossed two times to the hexaploid wheat line Fielder, which is susceptible to the Pgt races 34MKGQM and 34C3RTGQM. Four PCR markers pku4856F2R2, pku4861F7R7, pku4886F3R3, and pku4917F3R3 were used to confirm the presence of the Kronos segment in the final BC2F2 lines. Markers for the other T. monococcum genes identified one BC2F2 plant heterozygous for SrKN but lacking all the other parental Pgt resistance genes Sr21, Sr60, SrTm4, SrTm5 (from T. monococcum), and Sr13 (from Kronos).
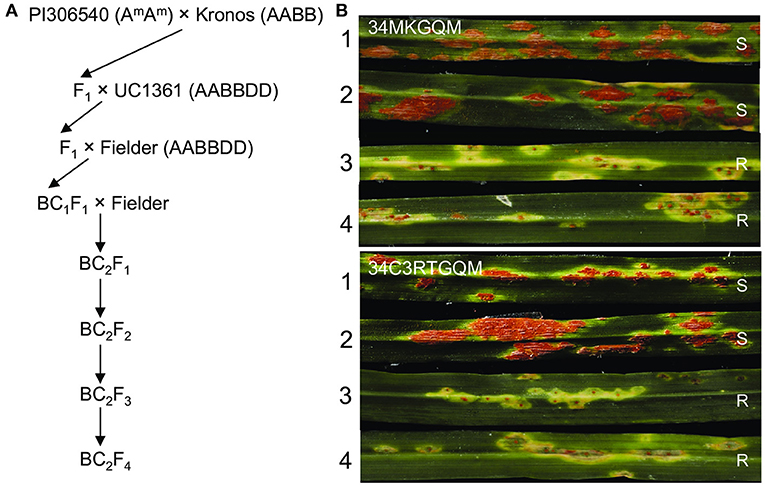
Figure 4. Introgression of SrKN into a hexaploid wheat background. (A) Procedure involved in the generation of the SrKN introgression into common wheat. Flanking markers pku4856F2R2 and pku4917F3R3 and completely linked markers pku4861F7R7 and pku4886F3R3 were used to confirm the presence of Kronos chromatin during crosses. (B) Infection types (ITs) from the BC2F3 plants were homozygous for the resistant SrKN allele (+SrKN) and the plants lacking SrKN (–SrKN). Two Pgt races 34MKGQM and 34C3RTGQM were used to evaluate. 1–2, BC2F3 plants lacking the resistant SrKN allele; 3–4, BC2F3 plants homozygous for the resistant SrKN allele. R, resistant; S, susceptible.
In the BC2F3 progeny derived from the selected BC2F2 plant, we identified eight plants homozygous for SrKN alone and six plants without any Sr genes (Supplementary Figure S4). Half of the plants from each genotype were inoculated with race 34MKGQM and the other half with 34C3RTGQM. The plants carrying SrKN exhibited good levels of resistance (IT = 1+) to both races, whereas plants lacking SrKN showed susceptible reactions (IT = 3+ to 4) to the same races (Figure 4). We are currently increasing the seeds from the plants carrying only SrKN to deposit them in the National Small Grain Collection in the United States and the Germplasm Bank of China.
Discussion
High-Density Mapping of SrKN and Delimitation of Its Candidate Gene Region
A previous study postulated that, in addition to Sr13, the durum wheat variety Kronos carries an undetermined stem rust resistance gene effective against Pgt race TRTTF (Zhang et al., 2017). In this study, we mapped this TRTTF-resistance gene SrKN within a 0.29 cM region on the distal region of chromosome arm 2BL using a high-density genetic map.
Using the published sequenced genomes of tetraploid and hexaploid wheat (The International Wheat Genome Sequencing Consortium, 2018; Maccaferri et al., 2019), we delimited the SrKN candidate gene region to a 5.6-Mb region in tetraploid wheat Svevo and a 7.2-Mb region in hexaploid wheat CS (Supplementary Tables S2, S3) including a cluster of NLR genes. Since NLR genes are the most frequent class associated with disease resistance in wheat and other plant species (Gassmann et al., 1999; Yuan et al., 2011; Saintenac et al., 2013; Zhang et al., 2014, 2017; Chen et al., 2018b; Li et al., 2019), we hypothesize that one of these genes could be a good candidate for SrKN. This hypothesis is supported by the complete linkage of this cluster to SrKN and by their likely absence in the susceptible parent Rusty (Supplementary Table S4). Similar to the SrKN candidate region, deletions, rearrangements, and duplications of NLR genes have been described for other cloned wheat NLR genes involved in resistance to Pgt, such as Sr21 and Sr13 (Zhang et al., 2017; Chen et al., 2018b). To determine if these NLR genes were required for resistance to TRTTF, we are currently testing truncation mutations for each gene from the published database of sequenced ethyl methane sulfonate (EMS) mutations in Kronos (Krasileva et al., 2017).
Since we do not have a contiguous sequence of the Kronos genome, we cannot rule out the possibility of additional NLR genes present in Kronos that are absent in the Svevo reference genome. However, this is unlikely because the sequences of all the genes in the candidate region (Supplementary Table S2, from start to stop codons) were 100% identical between Kronos and Svevo, suggesting that these two varieties have a very similar or identical haplotype in this region. In addition, Svevo has a similar resistance response against race 34MKGQM (Supplementary Figure S3; Supplementary Table S5). Taken together, these results suggest that Svevo may also carry SrKN or Sr9e. If this is confirmed, the availability of the Svevo genome can accelerate the identification of the causal gene.
Relationship Between SrKN and Other Sr Genes on Chromosome Arm 2BL
In addition to gene SrKN, previous studies have identified other four stem rust resistance loci on chromosome arm 2BL (Sr9, Sr16, Sr28, and Sr47) (McIntosh et al., 1995; Klindworth et al., 2012; Rouse et al., 2012). Among these genes, Sr47 confers resistance to race TTKSK and was transferred from Aegilops speltoides Tausch into polyploid wheat (Klindworth et al., 2012). Gene Sr16 is not effective against race TRTTF (Singh et al., 2015), and Sr28 showed a very different resistance profile to SrKN in this study (Table 3). The genetic analysis using a shared SSR marker indicates that Sr28 is located about 20 cM distal to SrKN (Figure 3). Gene Sr16 was placed approximately 34 cM distal to Sr28 by using monosomic analysis (~54 cM distal to SrKN) (McIntosh, 1978; Hiebert et al., 2010). Based on these data, we concluded that SrKN is different from genes Sr16, Sr28, and Sr47.
Conflictive or inconclusive results were reported regarding the mapping locations of Sr9. Gene Sr9e was initially mapped approximately 0.7 cM proximal to SSR marker gwm47 (685,759,255 bp, RefSeq v1.0 coordinates) (Bhavani et al., 2008). By contrast, another Sr9 allele, Sr9h, was mapped 2.8 cM distal to the same marker (Rouse et al., 2014). A recent study showed that the Sr9 locus is located within a region of chromosome 2B between 665.7 and 720.5 Mb in the reference genome of CS (RefSeq v1.0) (Aoun et al., 2019), which includes our proposed candidate region for SrKN (682.9–690.0 Mb). In addition, another recent study has mapped a TRTTF resistance quantitative trait locus (QTL) derived from tetraploid wheat accession Langdon on chromosome 2BL, which was designated as QSr.rwg-2B.2 and was hypothesized to be Sr9e (Sharma et al., 2021). Although the authors suggested that this QTL was mapped between the SNP markers IWB71742 (738.3 Mb, RefSeq v1.0) and IWB73196 (738.4 Mb), this QTL extends to a much larger region from IWB3657 (593.6 Mb) to IWB11280 (750.0 Mb) that includes our candidate gene region. Previous studies postulated that Langdon carries Sr9e (Luig, 1983; Singh et al., 1992), a conclusion supported by our analysis of the Langdon haplotype in the Sr9e region, which is identical to the one we found in Kronos (Supplementary Table S5).
We initially thought SrKN and Sr9e were different genes because Sr9e was reported to be susceptible to race TRTTF (Olivera et al., 2012) and SrKN is not. However, more recent reports suggested that Sr9e conferred partial resistance to race TRTTF (Saini et al., 2018; Sharma et al., 2021). If this last result is confirmed to be correct, then the resistance profiles of SrKN and Sr9e would be identical. Taken together, the allelism test and the similar resistance profiles (Table 3) suggest (but do not demonstrate) that SrKN and Sr9e might be the same gene.
Introgression of SrKN Into Hexaploid Wheat and Its Utilization in Breeding
As durum and bread wheat have common A and B genomes, it is relatively easy to introgress important genes into bread wheat from T. durum. Several rust resistance genes have been identified and transferred from durum to hexaploid wheat, including the stripe rust resistance genes Yr5 (Zhang et al., 2009), Yr53 (Xu et al., 2013), Yr64, and Yr65 (Cheng et al., 2014); the leaf rust resistance genes Lr23 (McIntosh et al., 1995; Sibikeev et al., 2020), Lr61 (Herrera-Foessel et al., 2008), and Lr79 (Qureshi et al., 2018); and the stem rust resistance genes Sr12 (Sheen and Snyder, 1964), Sr13 (Simons et al., 2011; Zhang et al., 2017), and Sr8155B1 (Nirmala et al., 2017). Using the cross of Kronos (AABB) × PI 306540 (AmAm), we successfully introgressed SrKN into hexaploid wheat line Fielder. The same cross was also used to introgress the stem rust resistance gene Sr60 and SrTm5 from diploid wheat accession PI 306540 into the common wheat lines UC12014-36 and Fielder, respectively (Chen et al., 2018a, 2020).
Although the crosses between tetraploid and hexaploid wheat can generate viable pentaploid plants, some of these crosses result in hybrid necrosis limiting their use in commercial breeding programs. Therefore, the introgression of SrKN into a common wheat background will facilitate the utilization of this resistance gene in common wheat breeding programs. Since SrKN is not effective against several virulent Pgt races (Zhang et al., 2017), including the Ug99 race group and race TKTTF, it is important to deploy it in combination with other Sr genes. Some potentially useful combinations to expand the resistance spectrum include Sr21 (Chen et al., 2015), SrTm5 (Chen et al., 2018a), Sr36 (Singh et al., 2015), Sr1RSAmigo (Olivera et al., 2012), and SrTmp (Singh et al., 2015), which are susceptible to Pgt race TRTTF but confer resistance to TTKSK (Ug99).
In conclusion, the high-density map of SrKN, the closely linked molecular markers, and the introgression of the T. durum segment containing this gene into hexaploid wheat will accelerate its deployment and pyramiding with other Sr genes.
Data Availability Statement
Raw sequencing data of durum wheat Rusty has been deposited in NCBI's Sequence Read Archive (https://www.ncbi.nlm.nih.gov/sra, Bioproject PRJNA751176).
Author Contributions
HongnaL and LH performed most of the experimental work. MR performed the phenotyping experiments with race TRTTF. TL contributed part of the phenotyping experiments with Chinese Pgt races. SP, SB, TS, JL, HongyuL, and XW contributed the mapping and primers development. WZ created the mapping population and phenotyping with race BCCBC. SC analyzed the data and wrote the first version of the manuscript. SC and JD proposed and supervised the project, obtained the funding, and generated the final version of the paper. All authors revised the manuscript and provided suggestions.
Funding
Work at JD laboratory was supported by the Howard Hughes Medical Institute and by the Agriculture and Food Research Initiative Competitive Grant 2017-67007-25939 (WheatCAP) from the USDA National Institute of Food and Agriculture (NIFA). Work at SC laboratory was supported by the Provincial Technology Innovation Program of Shandong and by the State Key Laboratory of North China Crop Improvement and Regulation (NCCIR2020KF-4). Work at the USDA-ARS was supported by the USDA-ARS National Plant Disease Recovery System. Work at the XW laboratory was supported by the Provincial Natural Science Foundation of Hebei (C2021204008).
Conflict of Interest
The authors declare that the research was conducted in the absence of any commercial or financial relationships that could be construed as a potential conflict of interest.
Publisher's Note
All claims expressed in this article are solely those of the authors and do not necessarily represent those of their affiliated organizations, or those of the publisher, the editors and the reviewers. Any product that may be evaluated in this article, or claim that may be made by its manufacturer, is not guaranteed or endorsed by the publisher.
Acknowledgments
We thank Prof. Weining Song of Northwest Agriculture & Forestry University, Shanxi, China, for providing 17 accessions of T. durum.
Supplementary Material
The Supplementary Material for this article can be found online at: https://www.frontiersin.org/articles/10.3389/fpls.2021.751398/full#supplementary-material
References
Aoun, M., Kolmer, J. A., Rouse, M. N., Elias, E. M., Breiland, M., Bulbula, W. D., et al. (2019). Mapping of novel leaf rust and stem rust resistance genes in the Portuguese durum wheat landrace PI 192051. G3-Genes Genom. Genet. 9, 2535–2547. doi: 10.1534/g3.119.400292
Babiker, E., Gordon, T., Chao, S., Rouse, M., Wanyera, R., Acevedo, M., et al. (2017). Molecular mapping of stem rust resistance loci effective against the Ug99 race group of the stem rust pathogen and validation of a single nucleotide polymorphism marker linked to stem rust resistance gene Sr28. Phytopathology 107, 208–215. doi: 10.1094/PHYTO-08-16-0294-R
Bariana, H. (2008). “Stem rust resistance in wheat-the Australian experience,” in Proceeding of International Conference on Wheat Stem Rust Ug99-a Threat to Food Security (New Delhi: Indian Agricultural Research Institute).
Bhardwaj, S. C., Singh, G. P., Gangwar, O. P., Prasad, P., and Kumar, S. (2019). Status of wheat rust research and progress in rust management-Indian context. Agronomy 9:892. doi: 10.3390/agronomy9120892
Bhavani, S., Bansal, U. K., Hare, R. A., and Bariana, H. S. (2008). Genetic mapping of stem rust resistance in durum wheat cultivar ‘Arrivato'. Int. J. Plant Breed. 2, 23–26. Available online at: http://www.globalsciencejournals.info/Online/GSBOnline/images/0812/IJPB_2(1&2)/IJPB_2(1)23-26o.pdf (accessed September 25, 2021).
Briggs, J., Chen, S., Zhang, W., Nelson, S., Dubcovsky, J., and Rouse, M. N. (2015). Mapping of SrTm4, a recessive stem rust resistance gene from diploid wheat effective to Ug99. Phytopathology 105, 1347–1354. doi: 10.1094/PHYTO-12-14-0382-R
Cakmak, I., Pfeiffer, W. H., and McClafferty, B. (2010). Biofortification of durum wheat with zinc and iron. Cereal Chem. 87, 10–20. doi: 10.1094/CCHEM-87-1-0010
Chen, S., Guo, Y., Briggs, J., Dubach, F., Chao, S., Zhang, W., et al. (2018a). Mapping and characterization of wheat stem rust resistance genes SrTm5 and Sr60 from Triticum monococcum. Theor. Appl. Genet. 131, 625–635. doi: 10.1007/s00122-017-3024-z
Chen, S., Rouse, M. N., Zhang, W., Jin, Y., Akhunov, E., Wei, Y., et al. (2015). Fine mapping and characterization of Sr21, a temperature-sensitive diploid wheat resistance gene effective against the Puccinia graminis f. sp. tritici Ug99 race group. Theor. Appl. Genet. 128, 645–656. doi: 10.1007/s00122-015-2460-x
Chen, S., Rouse, M. N., Zhang, W., Zhang, X., Guo, Y., Briggs, J., et al. (2020). Wheat gene Sr60 encodes a protein with two putative kinase domains that confers resistance to stem rust. New Phytol. 225, 948–959. doi: 10.1111/nph.16169
Chen, S., Zhang, W., Bolus, S., Rouse, M. N., and Dubcovsky, J. (2018b). Identification and characterization of wheat stem rust resistance gene Sr21 effective against the Ug99 race group at high temperature. PLoS Genet. 14:e1007287. doi: 10.1371/journal.pgen.1007287
Cheng, P., Xu, L. S., Wang, M. N., See, D. R., and Chen, X. M. (2014). Molecular mapping of genes Yr64 and Yr65 for stripe rust resistance in hexaploid derivatives of durum wheat accessions PI 331260 and PI 480016. Theor. Appl. Genet. 127, 2267–2277. doi: 10.1007/s00122-014-2378-8
Gassmann, W., Hinsch, M. E., and Staskawicz, B. J. (1999). The Arabidopsis RPS4 bacterial-resistance gene is a member of the TIR-NBS-LRR family of disease-resistance genes. Plant J. 20, 265–277. doi: 10.1046/j.1365-313X.1999.t01-1-00600.x
Godfray, H. C. J., Beddington, J. R., Crute, I. R., Haddad, L., Lawrence, D., Muir, J. F., et al. (2010). Food security: the challenge of feeding 9 billion people. Science 327, 812–818. doi: 10.1126/science.1185383
Herrera-Foessel, S., Singh, R., Huerta-Espino, J., William, H., Djurle, A., and Yuen, J. (2008). Molecular mapping of a leaf rust resistance gene on the short arm of chromosome 6B of durum wheat. Plant Dis. 92, 1650–1654. doi: 10.1094/PDIS-92-12-1650
Hiebert, C. W., Fetch, T. G., and Zegeye, T. (2010). Genetics and mapping of stem rust resistance to Ug99 in the wheat cultivar Webster. Theor. Appl. Genet. 121, 65–69. doi: 10.1007/s00122-010-1291-z
Jin, Y., Singh, R. P., Ward, R. W., Wanyera, R., Kinyua, M., Njau, P., et al. (2007). Characterization of seedling infection types and adult plant infection responses of monogenic Sr gene lines to race TTKS of Puccinia graminis f. sp tritici. Plant Dis. 91, 1096–1099. doi: 10.1094/PDIS-91-9-1096
Klindworth, D., Miller, J., and Xu, S. (2006). Registration of Rusty durum wheat. Crop Sci. 46, 1012–1014. doi: 10.2135/cropsci2005.05-0064
Klindworth, D. L., Niu, Z., Chao, S., Friesen, T. L., Jin, Y., Faris, J. D., et al. (2012). Introgression and characterization of a goatgrass gene for a high level of resistance to Ug99 stem rust in tetraploid wheat. G3-Genes Genom. Genet. 2, 665–673. doi: 10.1534/g3.112.002386
Krasileva, K. V., Buffalo, V., Bailey, P., Pearce, S., Ayling, S., Tabbita, F., et al. (2013). Separating homeologs by phasing in the tetraploid wheat transcriptome. Genome Biol. 14, 1–19. doi: 10.1186/gb-2013-14-6-r66
Krasileva, K. V., Vasquez-Gross, H., Howell, T., Bailey, P., Paraiso, F., Clissold, L., et al. (2017). Uncovering hidden variation in polyploid wheat. Proc. Natl. Acad. Sci. U.S.A. 114, E913–E921 doi: 10.1073/pnas.1619268114
Leonard, K. (2001). “Stem rust-future enemy?,” in Stem Rust of Wheat: From Ancient Enemy to Modern Foe, ed P. D. Peterson (St. Paul, MN: APS Press), 119–146.
Letta, T., Olivera, P., Maccaferri, M., Jin, Y., Ammar, K., Badebo, A., et al. (2014). Association mapping reveals novel stem rust resistance loci in durum wheat at the seedling stage. Plant Genome 7, 1–13. doi: 10.3835/plantgenome2013.08.0026
Li, T., Wu, X., Xu, X., Wang, W., and Cao, Y. (2016). Postulation of seedling stem rust resistance genes of Yunnan wheat cultivars in China. Plant Protect. Sci. 52, 242–249. doi: 10.17221/137/2015-PPS
Li, T. G., Wang, B. L., Yin, C. M., Zhang, D. D., Wang, D., Song, J., et al. (2019). The Gossypium hirsutum TIR-NBS-LRR gene GhDSC1 mediates resistance against Verticillium wilt. Mol. Plant Pathol. 20, 857–876. doi: 10.1111/mpp.12797
Li, T. Y., Ma, Y. C., Wu, X. X., Chen, S., Xu, X. F., Wang, H., et al. (2018). Race and virulence characterization of Puccinia graminis f. sp. tritici in China. PloS ONE 13:e0197579. doi: 10.1371/journal.pone.0197579
Luig, N. H. (1983). “A survey of virulence genes in wheat stem rust, Puccinia graminis f. sp. tritici,” in Advances in Plant Breeding (Berlin: Verlag Paul Parey). Available online at: https://agris.fao.org/agris-search/search.do?recordID=DE97B6241 (accessed September 25, 2021).
Maccaferri, M., Harris, N. S., Twardziok, S. O., Pasam, R. K., Gundlach, H., Spannagl, M., et al. (2019). Durum wheat genome highlights past domestication signatures and future improvement targets. Nat. Genet. 51, 885–895. doi: 10.1038/s41588-019-0381-3
McIntosh, R. (1978). Cytogenetical studies in wheat X. Monosomic analysis and linkage studies involving genes for resistance to Puccinia graminis f. sp. tritici in cultivar Kota. Heredity 41, 71–82. doi: 10.1038/hdy.1978.65
McIntosh, R., Yamazaki, Y., Dubcovsky, J., Rogers, W., Morris, C., Appels, R., et al. (2013). “Catalogue of gene symbols for wheat,” in: 12th International Wheat Genetics Symposium, ed R. A. McIntosh (Yokohama). Available online at: http://www.shigen.nig.ac.jp/wheat/komugi/genes/macgene/2013/GeneCatalogueIntroduction.pdf (accessed September 25, 2021).
McIntosh, R. A., Wellings, C. R., and Park, R. F. (1995). Wheat Rusts: An Atlas of Resistance Genes, ed K. Jean. Melbourne, VIC: CSIRO.
Megerssa, S. H., Ammar, K., Acevedo, M., Brown-Guedira, G., Ward, B., Degete, A. G., et al. (2020). Multiple-race stem rust resistance loci identified in durum wheat using genome-wide association mapping. Front. Plant Sci. 11:1934. doi: 10.3389/fpls.2020.598509
Miedaner, T., Rapp, M., Flath, K., Longin, C. F. H., and Würschum, T. (2019). Genetic architecture of yellow and stem rust resistance in a durum wheat diversity panel. Euphytica 215, 1–17. doi: 10.1007/s10681-019-2394-5
Mo, Y., Howell, T., Vasquez-Gross, H., De Haro, L. A., Dubcovsky, J., and Pearce, S. (2018). Mapping causal mutations by exome sequencing in a wheat TILLING population: a tall mutant case study. Mol. Genet. Genomics 293, 463–477. doi: 10.1007/s00438-017-1401-6
Murray, M., and Thompson, W. F. (1980). Rapid isolation of high molecular weight plant DNA. Nucleic Acids Res. 8, 4321–4326. doi: 10.1093/nar/8.19.4321
Nazari, K., Mafi, M., Yahyaoui, A., Singh, R., and Park, R. (2009). Detection of wheat stem rust (Puccinia graminis f. sp. tritici) race TTKSK (Ug99) in Iran. Plant Dis. 93:317. doi: 10.1094/PDIS-93-3-0317B
Nirmala, J., Saini, J., Newcomb, M., Olivera, P., Gale, S., Klindworth, D., et al. (2017). Discovery of a novel stem rust resistance allele in durum wheat that exhibits differential reactions to Ug99 isolates. G3-Genes Genom. Genet. 7, 3481–3490. doi: 10.1534/g3.117.300209
Olivera, P., Jin, Y., Rouse, M., Badebo, A., Fetch, T. Jr, Singh, R., et al. (2012). Races of Puccinia graminis f. sp. tritici with combined virulence to Sr13 and Sr9e in a field stem rust screening nursery in Ethiopia. Plant Dis. 96, 623–628. doi: 10.1094/PDIS-09-11-0793
Olivera, P., Newcomb, M., Szabo, L. J., Rouse, M., Johnson, J., Gale, S., et al. (2015). Phenotypic and genotypic characterization of race TKTTF of Puccinia graminis f. sp. tritici that caused a wheat stem rust epidemic in southern Ethiopia in 2013–14. Phytopathology 105, 917–928. doi: 10.1094/PHYTO-11-14-0302-FI
Olivera, P. D., Sikharulidze, Z., Dumbadze, R., Szabo, L. J., Newcomb, M., Natsarishvili, K., et al. (2019). Presence of a sexual population of Puccinia graminis f. sp. tritici in Georgia provides a hotspot for genotypic and phenotypic diversity. Phytopathology 109, 2152–2160. doi: 10.1094/PHYTO-06-19-0186-R
Periyannan, S. K., Qamar, Z. U., Bansal, U. K., and Bariana, H. S. (2014). Development and validation of molecular markers linked with stem rust resistance gene Sr13 in durum wheat. Crop Pasture Sci. 65, 74–79. doi: 10.1071/CP13325
Peterson, P., Leonard, K., Roelfs, A., and Sutton, T. (2005). Effect of barberry eradication on changes in populations of Puccinia graminis in Minnesota. Plant Dis. 89, 935–940. doi: 10.1094/PD-89-0935
Pretorius, Z. A., Singh, R. P., Wagoire, W. W., and Payne, T. S. (2000). Detection of virulence to wheat stem rust resistance gene Sr31 in Puccinia graminis f. sp. tritici in Uganda. Plant Dis. 84:203. doi: 10.1094/PDIS.2000.84.2.203B
Qureshi, N., Bariana, H., Kumran, V. V., Muruga, S., Forrest, K. L., Hayden, M. J., et al. (2018). A new leaf rust resistance gene Lr79 mapped in chromosome 3BL from the durum wheat landrace Aus26582. Theor. Appl. Genet. 131, 1091–1098. doi: 10.1007/s00122-018-3060-3
Roelfs, A., and Martens, J. (1988). An international system of nomenclature for Puccinia graminis f. sp. tritici. Phytopathology 78, 526–533. doi: 10.1094/Phyto-78-526
Rondon, M., Gough, F., and Williams, N. D. (1966). Inheritance of stem rust resistance in Triticum aestivum ssp. vulgare ‘Reliance'and PI 94701 of Triticum durum 1. Crop Sci 6, 177–179. doi: 10.2135/cropsci1966.0011183X000600020020x
Rouse, M., Wanyera, R., Njau, P., and Jin, Y. (2011). Sources of resistance to stem rust race Ug99 in spring wheat germplasm. Plant Dis. 95, 762–766. doi: 10.1094/PDIS-12-10-0940
Rouse, M. N., Nava, I. C., Chao, S., Anderson, J. A., and Jin, Y. (2012). Identification of markers linked to the race Ug99 effective stem rust resistance gene Sr28 in wheat (Triticum aestivum L.). Theor. Appl. Genet. 125, 877–885. doi: 10.1007/s00122-012-1879-6
Rouse, M. N., Nirmala, J., Jin, Y., Chao, S. M., Fetch, T. G., Pretorius, Z. A., et al. (2014). Characterization of Sr9h, a wheat stem rust resistance allele effective to Ug99. Theor. Appl. Genet. 127, 1681–1688. doi: 10.1007/s00122-014-2330-y
Saini, J., Faris, J. D., Zhang, Q., Rouse, M. N., Jin, Y., Long, Y., et al. (2018). Identification, mapping, and marker development of stem rust resistance genes in durum wheat ‘Lebsock'. Mol. Breed. 38, 1–14. doi: 10.1007/s11032-018-0833-y
Saintenac, C., Zhang, W., Salcedo, A., Rousse, M., Trick, H., Akhunov, E., et al. (2013). Identification of wheat gene Sr35 that confers resistance to Ug99 stem rust race group. Science 341, 783–786. doi: 10.1126/science.1239022
Sharma, J. S., Overlander, M., Faris, J. D., Klindworth, D. L., Rouse, M. N., Kang, H., et al. (2021). Characterization of synthetic wheat line Largo for resistance to stem rust. G3-Genes Genom Genet 11:jkab193. doi: 10.1093/g3journal/jkab193
Sheen, S. J., and Snyder, L. A. (1964). Studies on the inheritance of resistance to six stem rust cultures using chromosome substitution lines of a Marquis wheat selection. Can. J. Genet. Cytol. 6, 74–82. doi: 10.1139/g64-010
Sibikeev, S., Druzhin, A., Gultyaeva, E., and Yankovskaya, A. (2020). Use of the durum wheat gene pool in breeding of spring bread wheat. Russ. Agric. Sci. 46, 432–436. doi: 10.3103/S1068367420050201
Simons, K., Abate, Z., Chao, S., Zhang, W., Rouse, M., Jin, Y., et al. (2011). Genetic mapping of stem rust resistance gene Sr13 in tetraploid wheat (Triticum turgidum ssp. durum L.). Theor. Appl. Genet. 122, 649–658. doi: 10.1007/s00122-010-1444-0
Singh, R., Bechere, E., and Abdalla, O. (1992). Genetic analysis of resistance to stem rust in ten durum wheats. Phytopathology 82:919–922. doi: 10.1094/Phyto-82-919
Singh, R. P., Hodson, D. P., Huerta-Espino, J., Jin, Y., Bhavani, S., Njau, P., et al. (2011). The emergence of Ug99 races of the stem rust fungus is a threat to world wheat production. Annu. Rev. Phytopathol. 49, 465–481. doi: 10.1146/annurev-phyto-072910-095423
Singh, R. P., Hodson, D. P., Jin, Y., Lagudah, E. S., Ayliffe, M. A., Bhavani, S., et al. (2015). Emergence and spread of new races of wheat stem rust fungus: continued threat to food security and prospects of genetic control. Phytopathology 105, 872–884. doi: 10.1094/PHYTO-01-15-0030-FI
Stakman, E. C., Stewart, D. M., and Loegering, W. Q. (1962). Identification of Physiologic Races of Puccinia graminis var. tritici. Washington, DC: United States Department of Agriculture Research Service E-617.
Stam, P. (1993). Construction of integrated genetic linkage maps by means of a new computer package: join map. Plant J. 3, 739–744. doi: 10.1111/j.1365-313X.1993.00739.x
Tesfaye, T., Chala, A., Shikur, E., Hodson, D., and Szabo, L. J. (2020). First report of TTRTF race of wheat stem rust, Puccinia graminis f. sp. tritici, in Ethiopia. Plant Dis. 104, 293–293. doi: 10.1094/PDIS-07-19-1390-PDN
The International Wheat Genome Sequencing Consortium (2018). Shifting the limits in wheat research and breeding using a fully annotated reference genome. Science 361:eaar7191. doi: 10.1126/science.aar7191
Van Ooijen, J. (2006). JoinMap® 4, Software for the Calculation of Genetic Linkage Maps in Experimental Populations. Wageningen: Kyazma BV.
Voorrips, R. (2002). MapChart: software for the graphical presentation of linkage maps and QTLs. J. Hered. 93, 77–78. doi: 10.1093/jhered/93.1.77
Walkowiak, S., Gao, L., Monat, C., Haberer, G., Kassa, M. T., Brinton, J., et al. (2020). Multiple wheat genomes reveal global variation in modern breeding. Nature 588, 277–283. doi: 10.1038/s41586-020-2961-x
Wang, S., Wong, D., Forrest, K., Allen, A., Chao, S., Huang, B. E., et al. (2014). Characterization of polyploid wheat genomic diversity using a high-density 90000 single nucleotide polymorphism array. Plant Biotechnol. J. 12, 787–796. doi: 10.1111/pbi.12183
Xu, L. S., Wang, M. N., Cheng, P., Kang, Z. S., Hulbert, S. H., and Chen, X. M. (2013). Molecular mapping of Yr53, a new gene for stripe rust resistance in durum wheat accession PI 480148 and its transfer to common wheat. Theor. Appl. Genet. 126, 523–533. doi: 10.1007/s00122-012-1998-0
Yu, L. X., Barbier, H., Rouse, M. N., Singh, S., Singh, R. P., Bhavani, S., et al. (2014). A consensus map for Ug99 stem rust resistance loci in wheat. Theor. Appl. Genet. 127, 1561–1581. doi: 10.1007/s00122-014-2326-7
Yuan, B., Zhai, C., Wang, W., Zeng, X., Xu, X., Hu, H., et al. (2011). The Pik-p resistance to Magnaporthe oryzae in rice is mediated by a pair of closely linked CC-NBS-LRR genes. Theor. Appl. Genet. 122, 1017–1028. doi: 10.1007/s00122-010-1506-3
Zhang, C., Liu, L., Wang, X., Vossen, J., Li, G., Li, T., et al. (2014). The Ph-3 gene from Solanum pimpinellifolium encodes CC-NBS-LRR protein conferring resistance to Phytophthora infestans. Theor. Appl. Genet. 127, 1353–1364. doi: 10.1007/s00122-014-2303-1
Zhang, P., McIntosh, R. A., Hoxha, S., and Dong, C. (2009). Wheat stripe rust resistance genes Yr5 and Yr7 are allelic. Theor. Appl. Genet. 120, 25–29. doi: 10.1007/s00122-009-1156-5
Keywords: durum wheat, stem rust, resistance gene, SrKN, introgression
Citation: Li H, Hua L, Rouse MN, Li T, Pang S, Bai S, Shen T, Luo J, Li H, Zhang W, Wang X, Dubcovsky J and Chen S (2021) Mapping and Characterization of a Wheat Stem Rust Resistance Gene in Durum Wheat “Kronos”. Front. Plant Sci. 12:751398. doi: 10.3389/fpls.2021.751398
Received: 01 August 2021; Accepted: 09 September 2021;
Published: 15 October 2021.
Edited by:
Feng Chen, Henan Agricultural University, ChinaReviewed by:
Caixia Lan, Huazhong Agricultural University, ChinaWang Xiue, Nanjing Agricultural University, China
Copyright © 2021 Li, Hua, Rouse, Li, Pang, Bai, Shen, Luo, Li, Zhang, Wang, Dubcovsky and Chen. This is an open-access article distributed under the terms of the Creative Commons Attribution License (CC BY). The use, distribution or reproduction in other forums is permitted, provided the original author(s) and the copyright owner(s) are credited and that the original publication in this journal is cited, in accordance with accepted academic practice. No use, distribution or reproduction is permitted which does not comply with these terms.
*Correspondence: Jorge Dubcovsky, amR1YmNvdnNreUB1Y2RhdmlzLmVkdQ==; Shisheng Chen, c2hpc2hlbmcuY2hlbkBwa3UtaWFhcy5lZHUuY24=
†ORCID: Matthew N. Rouse orcid.org/0000-0001-7763-8203
‡These authors have contributed equally to this work