- 1Department of Biochemistry and Molecular Biology of Plant Products, Instituto de la Grasa (IG-CSIC), Campus Universidad Pablo de Olavide, Seville, Spain
- 2BioLabs, Institute of Life Sciences, Scuola Superiore Sant’Anna, Pisa, Italy
Three different cDNA sequences, designated OepPDAT1-1, OepPDAT1-2, and OepPDAT2, encoding three phospholipid:diacylglycerol acyltransferases (PDAT) have been isolated from olive (Olea europaea cv. Picual). Sequence analysis showed the distinctive features typical of the PDAT family and together with phylogenetic analysis indicated that they encode PDAT. Gene expression analysis in different olive tissues showed that transcript levels of these three PDAT genes are spatially and temporally regulated and suggested that, in addition to acyl-CoA:diacylglycerol acyltransferase, OePDAT1-1 may contribute to the biosynthesis of triacylglycerols in the seed, whereas OePDAT1-2 could be involved in the triacylglycerols content in the mesocarp and, therefore, in the olive oil. The relative contribution of PDAT and acyl-CoA:diacylglycerol acyltransferase enzymes to the triacylglycerols content in olive appears to be tissue-dependent. Furthermore, water regime, temperature, light, and wounding regulate PDAT genes at transcriptional level in the olive fruit mesocarp, indicating that PDAT could be involved in the response to abiotic stresses. Altogether, this study represents an advance in our knowledge on the regulation of oil accumulation in oil fruit.
Introduction
Plant oils are mainly composed of triacylglycerols (TAG), which consist of a glycerol backbone esterified by three fatty acids. TAG constitutes a key storage lipid compound that represents a highly reduced form of carbon to be used as an energy reserve during seed germination and early seedling development (Xu et al., 2018). In addition, TAG are involved in many essential physiological processes such as stress response and pollen germination, since they supply the precursors for lipid signaling and membrane biosynthesis (Yang and Benning, 2018). Plant oils are mainly used for edible applications (food and feed), although there is an increasing interest in their use as renewable raw materials for the production of biofuels, biolubricants, and other bioproducts. For that reason, the global demand for plant oils is rapidly growing (Weselake et al., 2009). To meet this demand, one of the possible strategies is to improve the oil yield in the main oilseed and oil fruit crops (Carlsson et al., 2011). In the oil palm mesocarp, the oil content is about 80% and, therefore, it could be very difficult to achieve an additional increment because a minimum of structural components is necessary to confine this oil within the cell. In seeds, this high oil content could not be reached because they need storage proteins for germination and other cellular components for desiccation. However, in the case of olive mesocarp, which is characterized by an oil content of 40–50%, there is still room for an increase.
Olive is the second most important oil fruit crop cultivated worldwide, with olive oil ranking ninth in the global production of vegetable oils (Rallo et al., 2018). Virgin olive oil is a natural fruit juice increasingly demanded in recent decades due to its exceptional organoleptic properties and potential health benefits (Covas, 2008). Thus, one of the main objectives of the olive breeding programs is the generation of new cultivars, which possess a higher oil content in the olive fruit (Baldoni and Belaj, 2009). However, although new olive cultivars have been generated in the last years, none of them was selected for a higher oil yield despite the first Quantitative Trait Loci (QTLs) controlling oil accumulation in the olive fruit have been reported (Atienza et al., 2014).
In higher plants, TAG are synthesized in the endoplasmic reticulum (ER) by the sequential incorporation of fatty acids in the form of acyl-CoAs into the glycerol backbone (Chapman and Ohlrogge, 2012; Bates, 2016). The final rate-limiting step of this acyl-CoA-dependent pathway, commonly known as the Kennedy pathway, is catalyzed by the acyl-CoA:diacylglycerol acyltransferase (DGAT), which is responsible for the final acylation at the sn-3 position of 1,2-diacylglycerol (DAG) to be converted to TAG, using an acyl-CoA as substrate (Lung and Weselake, 2006). DGAT1 enzymes have been mainly related to the accumulation of TAG in oilseeds, whereas DGAT2 shows no sequence homology to DGAT1, and has been involved in the incorporation of unusual fatty acids (Xu et al., 2018).
In the year 2000, a new enzyme called phospholipid:diacylglycerol acyltransferase (PDAT) was discovered, which catalyzes the acyl-CoA-independent synthesis of TAG by transferring an acyl group from the sn-2 position of a phospholipid, mainly phosphatidylcholine (PC), to the sn-3 position of DAG, yielding TAG and a lysophospholipid (Dahlqvist et al., 2000). The PDAT activity was first characterized in microsomal preparations of the yeast Saccharomyces cerevisiae and developing oilseeds (Dahlqvist et al., 2000), although later investigations have confirmed its presence in algae (Yoon et al., 2012; Liu et al., 2016) but not in mammals. In the same study, the first PDAT gene was identified from S. cerevisiae. Subsequently, two homologs, AtPDAT1 and AtPDAT2, have been identified and characterized from Arabidopsis (Dahlqvist et al., 2000; Ståhl et al., 2004). Later studies indicate that PDAT is encoded by gene families in most plant species (Pan et al., 2015; Falarz et al., 2020).
Concerning its physiological role, PDAT could not be a major determinant of TAG content and composition in developing seeds, since low transcript levels of AtPDAT1 were observed in Arabidopsis seeds (Ståhl et al., 2004). In addition, there were no significant changes in seed oil content or fatty acid composition in Arabidopsis plants overexpressing AtPDAT1 (Ståhl et al., 2004) or in an Arabidopsis pdat1 knockout mutant (Mhaske et al., 2005). However, when AtPDAT1 was suppressed using RNAi in a dgat1 mutant background, the seed oil content was reduced by 70–80%, while neither silencing of AtPDAT2 nor AtDGAT2 showed a decrease in the oil content compared with the dgat1 control (Zhang et al., 2009). Accordingly, the expression of AtPDAT1 is greatly upregulated in seeds of the Arabidopsis dgat1 mutant, while the AtPDAT2 and AtDGAT2 transcript levels were not significantly altered (Xu et al., 2012). Additionally, normal seed and pollen development in the Arabidopsis pdat1 dgat1 double mutant was disrupted (Zhang et al., 2009). In a more recent study, it has been reported that the MYB96 transcription factor regulates TAG accumulation by activating DGAT1 and PDAT1 expression in Arabidopsis seeds (Lee et al., 2018). All these data strongly indicate overlapping roles of PDAT1 and DGAT1 in Arabidopsis seed oil accumulation, which allow PDAT1 to partially compensate for a loss of DGAT1 activity.
In other oilseeds such as Camelina sativa, silencing of PDAT1 using the CRISPR/Cas system (Aznar-Moreno and Durrett, 2017) or microRNA-mediated downregulation (Marmon et al., 2017) brings about a decrease in the linoleic acid content of seed oil, although a reduction in the total oil content was only observed in the first study. These results confirm that DGAT and PDAT enzymes cooperate in the TAG synthesis in oilseeds. Interestingly, the relative in vitro activities of both enzymes considerably differed between plant species, and stages of seed development (Banás et al., 2013). Therefore, the relative contribution of PDAT and DGAT enzymes to TAG synthesis is still unclear and needs to be further explored in other oil-bearing and vegetative plant tissues and species (Chapman and Ohlrogge, 2012; Chen et al., 2015).
In some plant species, PDAT exhibits high activity and unique specificity for PC containing unusual fatty acids and channeling them from PC to TAG, to be removed from membrane lipids and sequestered into TAG. For example, Crepis palaestina PDAT catalyzes the incorporation of vernoloyl groups into TAG (Dahlqvist et al., 2000), while PDAT1-2 is responsible for the specific transfer of ricinoleoyl groups in castor bean (Kim et al., 2011). In the same way, a specialized PDAT1 has been identified in flax that selectively incorporates α-linolenic acid into TAG (Pan et al., 2013). These PDAT with unique substrate selectivity are mostly expressed in developing seeds and are grouped into a single clade, which is distinct from those of PDAT1 and PDAT2 (Pan et al., 2015).
As opposed to Arabidopsis seeds, overexpression of AtPDAT1 in Arabidopsis leaves resulted in significant changes in oil content and fatty acid composition, indicating that PDAT1 is a key enzyme for TAG synthesis in this tissue (Fan et al., 2013a). Further studies also revealed a critical physiological role for AtPDAT1-mediated TAG synthesis in the protection against fatty acid-induced cell death in growing tissues (Fan et al., 2013b), and in the process of diverting fatty acids from membrane lipids toward β-oxidation, thereby maintaining membrane lipid homeostasis in Arabidopsis leaves (Fan et al., 2014). In the same tissue, it has also been reported that AtPDAT1 could be involved in stress responses (Fan et al., 2017; Mueller et al., 2017; Demski et al., 2020), although the role of PDAT in plant responses to different stresses remains to be completely elucidated.
Contrary to AtPDAT1, AtPDAT2 seems to be not involved in TAG biosynthesis in Arabidopsis (Zhang et al., 2009), although its transcript level in developing seeds is higher than that of AtPDAT1 (Ståhl et al., 2004). Similarly, PDAT2 homologs from castor bean (RcPDAT2) and flax (LuPDAT6) did not show a substantial role in TAG biosynthesis (Kim et al., 2011; Pan et al., 2013).
Regarding biotechnological applications, castor bean PDAT has been employed to obtain Arabidopsis transgenic plants with increased amounts (up to 25%) of ricinoleic acid in the seed oil by co-expression with castor bean fatty acid hydroxylase (Kim et al., 2011; van Erp et al., 2011, 2015) or lysophosphatidic acid acyltransferase (Lunn et al., 2020). In addition, the α-linolenic acid content of Arabidopsis seed oil was increased up to 10% by seed-specific expression of a flax PDAT (Pan et al., 2013). Furthermore, PDAT overexpression enhanced TAG content of vegetative tissues like in Arabidopsis leaves by up to 7% of the dry weight without affecting membrane lipid composition and plant growth (Fan et al., 2013b, 2019).
Unlike oilseeds, information about the relative contribution of DGAT and PDAT enzymes to TAG biosynthesis in oil fruit is very scarce. From a biotechnological point of view, oil fruit mesocarp possesses the advantage, compared to oilseeds, of altering TAG content and composition without affecting germination rates. In addition, olive fruit constitutes an interesting system to investigate TAG synthesis, because oil accumulation takes place in two distinct regions: the seed, which is enclosed in a woody endocarp, and the mesocarp, with a major impact on the final composition of the olive oil. In the mesocarp, TAG are accumulated to attract animals to aid seed dissemination, while TAG are synthesized in the seed as storage lipids to nourish the embryo during the early steps of germination (Sánchez, 1994). In olive, two DGAT genes (OeDGAT1 and OeDGAT2) have been isolated and characterized, which show overlapping but distinct expression patterns during olive mesocarp growth (Giannoulia et al., 2000; Banilas et al., 2011). In contrast, no PDAT genes have been characterized in olive to date, although two genes (OePDAT1-1 and OePDAT1-2) have been identified in the olive pollen (Hernández et al., 2020a).
In the present work, the isolation and characterization of three PDAT genes in olive are reported. Transcriptional analysis in olive fruit during development and ripening from two distinct olive cultivars was carried out to investigate the physiological role of each PDAT gene. In particular, their specific contribution to the oil content in different tissues and their potential involvement in the response to different abiotic stresses in the olive mesocarp were examined.
Materials and Methods
Plant Material and Stress Treatments
For tissues and developmental studies, olive (Olea europaea L. cv. Picual and Arbequina) trees were grown in the experimental orchard of Instituto de la Grasa, Seville (Spain), with drip irrigation and fertirrigation from the time of full bloom to fruit maturation. Young drupes, developing seeds, and mesocarp tissue were harvested from at least three different olive trees at different weeks after flowering (WAF) corresponding to different developmental stages of the olive fruit: green (9, 12, 16, and 19 WAF); yellowish (23 WAF); turning or veraison (28 and 31 WAF); and mature or fully ripe (35 WAF). Immediately after harvesting, olive tissues were frozen in liquid nitrogen and stored at −80°C. Young leaves were similarly collected.
The study of water deficit was conducted at the Sanabria orchard, a commercial super high-density olive (cv. Arbequina) orchard near Seville (Spain). The full irrigation (FI) and two regulated deficit irrigation (RDI) treatments (60RDI and 30RDI) were applied as defined by Fernández et al. (2013). Olive mesocarp tissue was sampled at different WAF as described by Hernández et al. (2018).
Stress treatments were carried out according to Hernández et al. (2019). Olive branches from Picual and Arbequina cultivars with about 100 olive fruit at turning stage (28 WAF) were collected from different olive trees and incubated in a growth chamber at 25°C with a 12 h light/12 h dark cycle, and a light intensity of 300 μmol m–2 s–1. These incubation parameters attempted to mimic the physiological conditions of the tree and were considered the standard conditions. For stress treatments, standard conditions were modified depending on the effect studied. For low and high temperature experiments, the branches containing the olive fruit were incubated at 15 or 35°C, respectively, at the standard light intensity. To assess the effect of the darkness, the light was turned off and the standard temperature was maintained. To study the effect of wounding, the whole surface of the olive fruit was mechanically damaged affecting mesocarp tissue, with pressure at zero time using forceps with serrated tips. The zero time of each experiment was selected 2 h after the beginning of the light period to maintain the natural photoperiod day/night of the olive fruit. When indicated, olive mesocarp tissues were sampled, frozen in liquid nitrogen, and stored at −80°C.
Isolation of Phospholipid:Diacylglycerol Acyltransferase Full-Length cDNA Clones
Candidate olive PDAT sequences were found in the olive transcriptome (Muñoz-Mérida et al., 2013) and the olive (var. sylvestris) genome (Unver et al., 2017) using the tblastn algorithm together with the amino acid sequences of Arabidopsis PDAT1 and PDAT2 genes (Ståhl et al., 2004). Based on these three new sequences, specific pairs of primers for each gene were designed and utilized for PCR amplification with ACCUZYMETM DNA polymerase (Bioline, Spain), which has proofreading activity. An aliquot of an olive Uni-ZAP XR cDNA library constructed with mRNA isolated from 13 WAF olive fruit of cultivar Picual (Haralampidis et al., 1998) was used as DNA template. One fragment with the expected size was generated in each reaction, subcloned into the vector pSpark® I (Canvax, Spain), and sequenced in both directions.
DNA sequencing was performed by GATC (Biotech, Germany). The DNA sequence data were compiled and analyzed with the LASERGENE software package (DNAStar, Madison, WI). The multiple sequence alignments of olive PDAT amino acid sequences were calculated using the ClustalX program and displayed with GeneDoc. Phylogenetic tree analysis was performed using the neighbor-joining method implemented in the Phylip package using Kimura’s correction for multiple substitutions and a 1000 bootstrap data set. TreeView was used to display the tree. The conserved domains in the deduced amino acid sequences were analyzed using the NCBI Conserved Domain Search1 and Pfam software.2 Prediction on putative N-glycosylation sites was performed using the software NetNGlyc 1.0.3 TMHMM analysis was carried out4 and subcellular localization was predicted using two different programs: ProtComp 9.05 and TargetP-2.0.6
Total RNA Isolation and cDNA Synthesis
Total RNA isolation was performed as described by Hernández et al. (2005) using 1.5 g of frozen olive tissue. RNA quality verification, removal of contaminating DNA, and cDNA synthesis were carried out according to Hernández et al. (2009).
Expression Analysis of Phospholipid:Diacylglycerol Acyltransferase Genes
The expression levels of the olive PDAT genes were determined by quantitative real-time PCR (qRT-PCR) using a CFX Connect real-time PCR System and iTaq Universal SYBR Green Supermix (BioRad, California, United States) as previously described (Hernández et al., 2019). Primers for gene-specific amplification for OePDAT1-1, OePDAT1-2, and OePDAT2 were designed using the Primer3 program7 and the Gene Runner software (Supplementary Table 1). The housekeeping olive ubiquitin2 gene (OeUBQ2, AF429430) was used as an endogenous reference to normalize (Hernández et al., 2009). For tissues and developmental studies, the relative expression level of each gene was calculated using the equation 2–ΔCt where ΔCt = (CtGOI − CtUBQ2) (Livak and Schmittgen, 2001; Pfaffl, 2004). This method has the advantage of making comparisons at the level of gene expression across developmental stages, cultivars, and genes. Regarding irrigation studies and stress treatments, the qRT-PCR data were calibrated relative to the corresponding gene expression level at 13 WAF from FI treatment and zero time for each stress treatment and cultivar, respectively, as calibrator. In these cases, the 2–ΔΔCt method for relative quantification was followed (Livak and Schmittgen, 2001). The data are presented as means ± SD of three biological replicates, each having two technical replicates per 96 well plate.
Oil Content Determination
Lipids were extracted as described by Hara and Radin (1978). Fatty acid methyl esters were produced by acid-catalyzed transmethylation (Garcés and Mancha, 1993) and analyzed by gas-liquid chromatography (Román et al., 2015). Heptadecanoic acid was used as an internal standard. Oil content (μg/mg DW) was calculated as the sum of the different fatty acids. Results are presented as means ± SD of three biological replicates, each having three technical replicates.
Results and Discussion
cDNA Isolation and Sequence Analysis of Three Olive Phospholipid:Diacylglycerol Acyltransferase Genes
Three sequences were selected from the olive transcriptome (Muñoz-Mérida et al., 2013), which exhibited a high degree of similarity to the Arabidopsis PDAT1 and PDAT2 genes (Ståhl et al., 2004). These sequences match with the loci LOC111401505, LOC111386227, LOC111374273, and LOC111401783 of the olive (var. sylvestris) genome (Unver et al., 2017). Based on these sequences, pairs of specific primers were designed and used for PCR amplification, together with an aliquot of a 13 WAF olive fruit cDNA library (cv. Picual). Three full-length cDNA clones were obtained that were designated as OepPDAT1-1, OepPDAT1-2, and OepPDAT2, with sizes of 2,445, 2,247, and 2,171 bp, respectively. They contained ORFs of 2,007, 2,070, and 2,046 bp, encoding predicted proteins of 669, 690, and 682 amino acid residues, which correspond to calculated molecular masses of 74.7, 76.2, and 76.1 kDa, and pI values of 6.1, 7.4, and 8.8, respectively. Alignment of the three olive PDAT deduced amino acid sequences (Figure 1) showed that OepPDAT1-1 displayed 74 and 57% identity with respect to OepPDAT1-2 and OepPDAT2, respectively, while OepPDAT1-2 shared 59% identity with OepPDAT2.
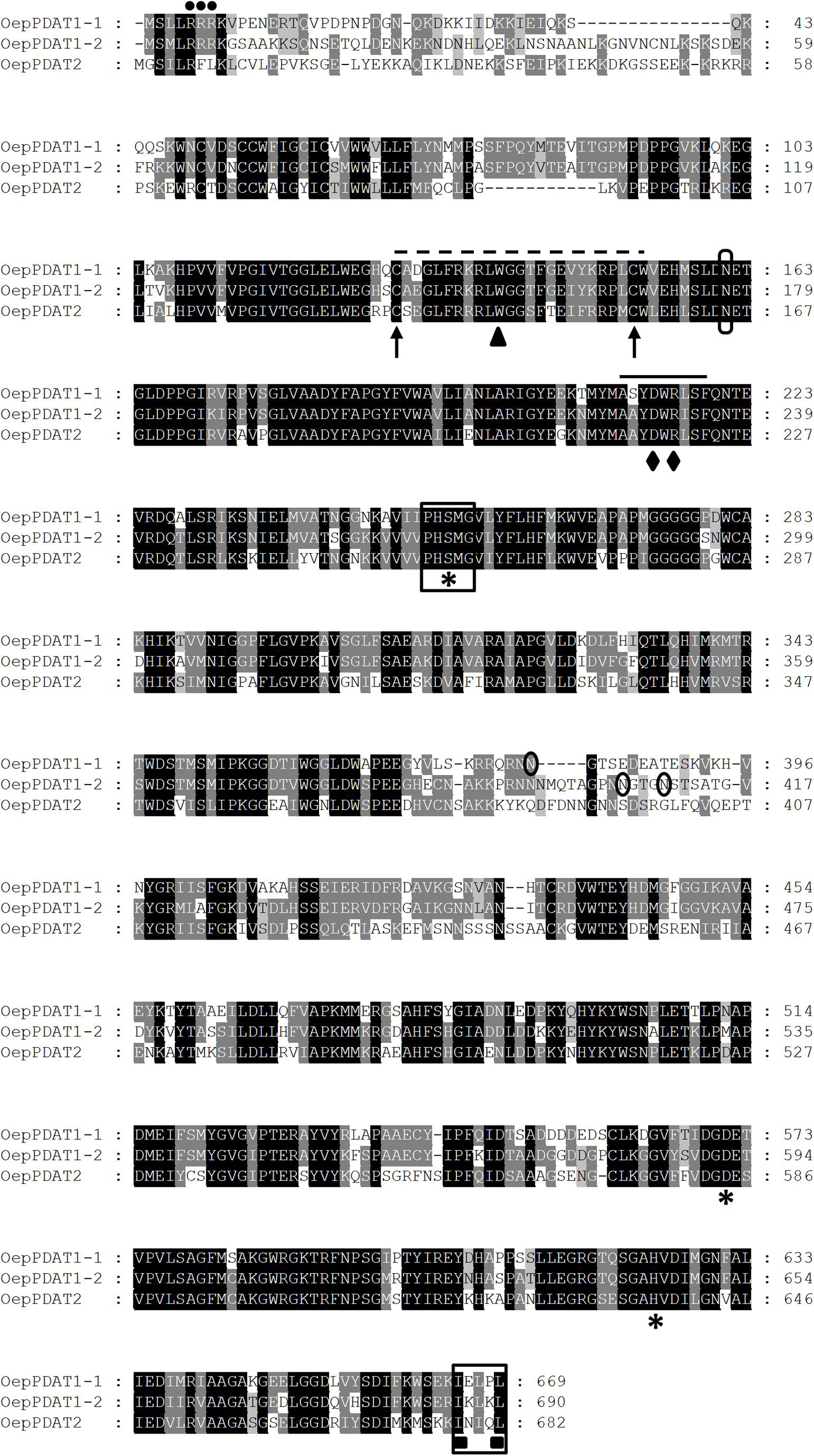
Figure 1. Comparison of the deduced amino acid sequences of olive PDAT genes. The sequences were aligned using the ClustalX program and displayed with GeneDoc. Identical and similar residues are shown on a background of black and gray, respectively. The position of the three consecutive Arg residues corresponding to the cluster is marked by dots. The lid domain is identified by a dashed line, flanked by the two conserved Cys residues denoted by arrows and including the conserved Trp residue indicated by a triangle. The conserved domain, which contains a salt bridge between Asp and Arg residues denoted by rhombus, is indicated by a continuous line. The catalytic triad (Ser-Asp-His) is labeled with asterisks and the lipase motif is boxed. The putative N-glycosylation sites are circled. The predicted ER-retrieval motif at the C-terminus of OepPDAT proteins is framed and the two hydrophobic residues are identified with squares. The cDNA sequences corresponding to OepPDAT1-1, OepPDAT1-2, and OepPDAT2 have been deposited in the GenBank/EMBL/DDBJ database with accession numbers MZ614942, MZ614943, and MZ614944, respectively.
The Conserved Domain search indicated that the three proteins belong to the PLNO2517 superfamily, with phosphatidylcholine-sterol O-acyltransferase activity. Further characterization of the three olive PDAT using Pfam analysis revealed the presence of a lecithin:cholesterol acyltransferase (LCAT) domain between amino acids 133–630, 149–651, 137–644 for OepPDAT1-1, OepPDAT1-2, and OepPDAT2, respectively, suggesting that they belong to the LCAT superfamily (Pfam:02450).
Several characteristic conserved amino acids and domains were detected in the alignment of the olive PDAT deduced amino acid sequences (Figure 1). A catalytic triad (Ser-Asp-His), which is conserved in all LCAT-like proteins including PDAT (Ståhl et al., 2004). This triad is part of the catalytic domain of LCAT enzymes, in which a fatty acid is transesterified from the sn-2 position of phosphatidylcholine to a free hydroxyl group of cholesterol to yield a cholesterol ester (Peelman et al., 1998). Recently, site-directed mutagenesis studies in Arabidopsis PDAT1 have shown that the first residue downstream the Ser of the catalytic triad, a conserved Met, is critical for maintain enzyme activity (Falarz et al., 2020). This Ser residue is also part of a motif analogous to the conserved lipase motif with the consensus sequence Gly-X-Ser-X-Gly (Schrag and Cygler, 1997). In plant PDAT, including the three from olive, the first Gly is replaced with a Pro resulting in the absence of the two β-sheets that may be essential for lipase activity (Yoon et al., 2012). Olive PDAT also contain a so-called lid domain, which is characteristic of lipases and LCAT and is closed by a disulphide bridge. The lid structure occurs between two conserved neighbor Cys residues. This 20–25 (24 for olive PDAT) amino acid long, highly mobile element covers the hydrophobic active site of these enzymes. This lid domain is possibly involved in destabilizing the lipid bilayer, thus facilitating the binding of the hydrophobic substrate and its diffusion into the active site of the enzyme (Peelman et al., 1999; Ståhl et al., 2004). A conserved Trp residue present in this lid domain was predicted to bind the cleaved fatty acid in the active site of these enzymes (Martinelle et al., 1996). In addition, a highly conserved domain that is present in all PDAT sequences contains a salt bridge between Asp and Arg, which may be involved in phospholipid recognition (Yoon et al., 2012), and includes some critical amino acids responsible for its substrate specificity and binding (Peelman et al., 1998). On the other hand, the three olive PDAT deduced amino acid sequences exhibit several potential N-glycosylation sites. Experimental evidence has been recently shown which demonstrates that four Asn residues of the S. cerevisiae PDAT protein are glycosylated as a result of a post-translational modification (Feng et al., 2019). However, none of them is conserved in the plant PDAT sequences.
Regarding the membrane topology, transmembrane predictions based on a hidden Markov model (TMHMM) analysis of the olive PDAT proteins were generated (Supplementary Figure 1). Olive PDAT1-1 and PDAT1-2 showed a short hydrophilic N-terminal tail, followed by a putative transmembrane domain (TMD) of 22 amino acids (53–75 and 69–91 for OepPDAT1-1 and OepPDAT1-2, respectively), and the rest of the protein localized in the membrane. This result is in agreement with the topology described for plant PDAT1 proteins (Pan et al., 2015). The hydrophilic N-terminal region preceding the TMD has been reported as the most divergent region, which exhibits a cluster of consecutive Arg residues, as shown in Figure 1 for olive PDAT1-1 and PDAT1-2. The function of these conserved Arg residues remains unknown, although it has been suggested that they are possibly an ER localization signal (Liu et al., 2012). Furthermore, even though the deletion of the TMD together with the N-terminal end of S. cerevisiae PDAT does not affect its enzymatic activity and substrate selectivity (Ghosal et al., 2007), the N-terminus of plant PDAT1 could be involved in the sorting of the protein to the ER (Pelham, 2000). Unlike both olive PDAT1 proteins, PDAT2 does not exhibit a TMD (Supplementary Figure 1). Interestingly, except for Arabidopsis PDAT2 (Ståhl et al., 2004), none of the other PDAT2 characterized to date showed a TMD, as it is the case of PDAT2 from Ricinus communis (Kim et al., 2011), Linum usitatissimum (Pan et al., 2013), Camelina sativa (Yuan et al., 2017), and Sesamum indicum (Chellamuthu et al., 2019).
Concerning the subcellular localization, the three olive PDAT sequences presented a C-terminal motif (Figure 1), which is both necessary and sufficient for maintaining localization of the enzymes in the ER (McCartney et al., 2004). Similar results have been previously described for Arabidopsis (Ståhl et al., 2004) and Camelina sativa (Yuan et al., 2017) PDAT1 and PDAT2. Since the ER is the main site for TAG biosynthesis in plants (Xu et al., 2018), it is assumed that plant PDAT are inserted into the ER and interpret the topology data based on the ER structure. As shown in Supplementary Figure 1, both olive PDAT1 have a short N-terminal end facing the cytosolic side, a single TMD region, and the rest of the protein residing on the lumen side of the ER. This result is consistent with the topology reported for yeast and Arabidopsis PDAT (Ståhl et al., 2004), and with the results reported by Dahlqvist et al. (2000), who detected PDAT activity in yeast and plant microsomal preparations, suggesting a putative localization of the PDAT enzyme in the ER. It is also important to point out that all the conserved motifs and amino acid residues mentioned above are located at the C-terminus of the TMD, indicating that the active and/or binding sites of plant PDAT possibly face the luminal side of the ER (Pan et al., 2015). On the other hand, analysis of the three deduced olive PDAT protein sequences with subcellular localization prediction software such as ProtComp suggests that both olive PDAT1 proteins could be located in the ER, whereas PDAT2 could be localized in the plasma membrane. In line with this observation, Ricinus communis PDAT1-1 and PDAT1-2 are located in the ER of epidermal cells of tobacco leaves, while PDAT2 is localized in the plasma membrane (Kim et al., 2011). Moreover, no putative transit peptide was detected in any of the three olive PDAT protein sequences using TargetP software, as previously reported for other plant PDAT (Pan et al., 2015).
A phylogenetic tree based on deduced amino acid sequences of all known and characterized algal and plant phospholipid:diacylglycerol acyltransferase was constructed to elucidate the phylogenetic relationship of olive phospholipid:diacylglycerol acyltransferases (Figure 2). In agreement with previous findings, plant PDAT could be classified into three different subfamilies: PDAT1, PDAT1-like, and PDAT2, which correspond to clades VI, V, and VII, respectively, according to Pan et al. (2015). OepPDAT1-1 was positioned close to OepPDAT1-2, in the group of PDAT1 enzymes. On the other hand, OepPDAT2 was situated in the branch together with other plant PDAT2, which show preferential expression in the seed. Interestingly, none of the olive PDAT were placed in clade V, which comprise PDAT enzymes majorly expressed in seeds and with substrate selectivity for unusual or highly unsaturated fatty acids. This is in agreement with the fact that olive oil does not contain unusual fatty acids and exhibits a very low amount of α-linolenic acid.
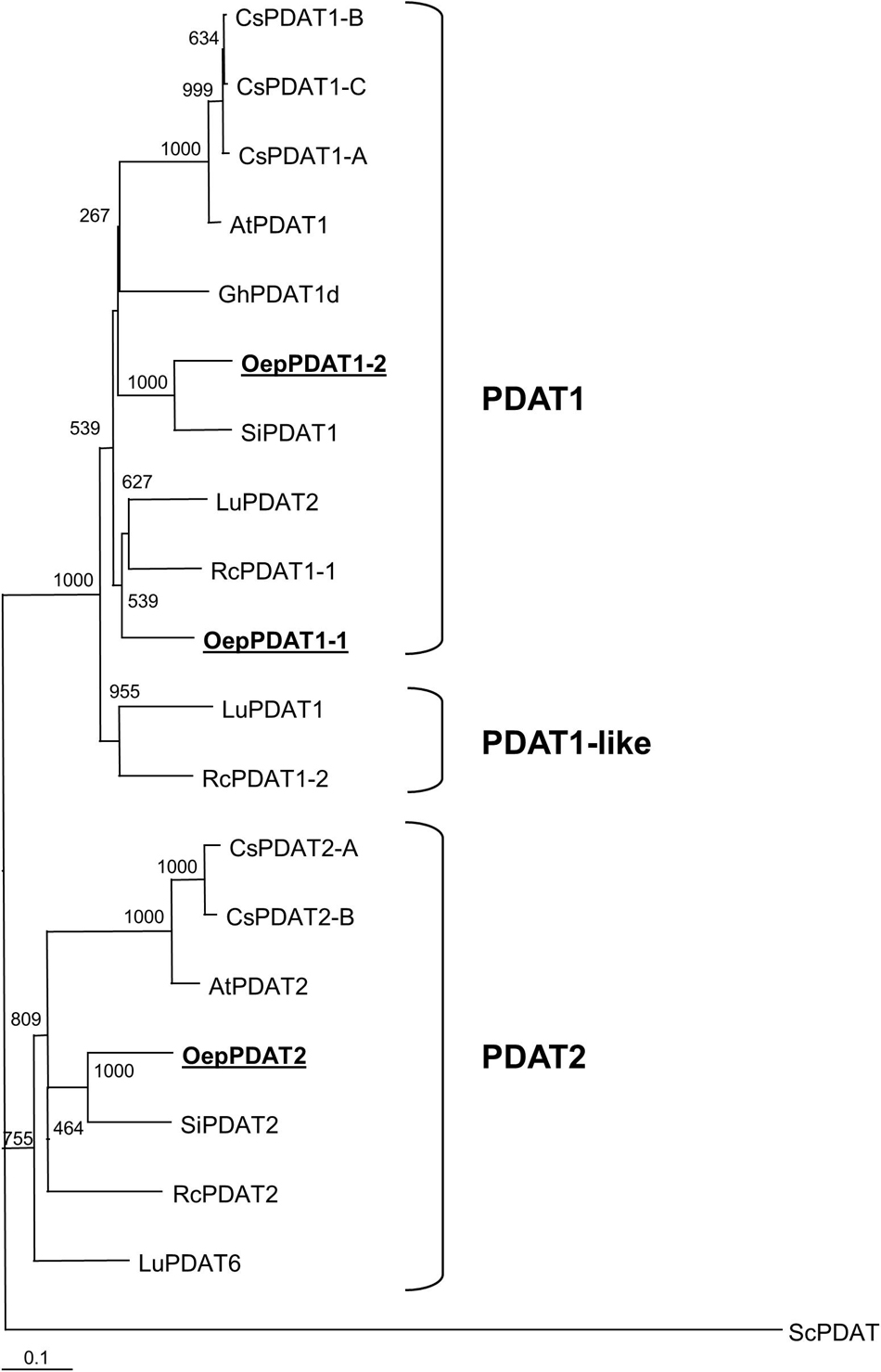
Figure 2. Phylogenetic tree analysis of plant phospholipid:diacylglycerol acyltransferases. Alignments were calculated with ClustalX and the analysis was performed using the neighbor-joining method implemented in the Phylip package using Kimura’s correction for multiple substitutions, and a 1000 bootstrap data set. The Saccharomyces cerevisiae PDAT sequence was defined as outgroup. TreeView was used to display the tree. Positions of the three olive PDAT are in bold and underlined. Accession numbers of the different PDAT included in this analysis apart from olive: Arabidopsis thaliana (AtPDAT1, AT5G13640; AtPDAT2, AT3G44830); Camelina sativa (CsPDAT1-A, XP_010453452; CsPDAT1-B, XP_010419957; CsPDAT1-C, XP_010492131; CsPDAT2-A, XP_010503132; CsPDAT2-B, XP_010514811); Gossypium hirsutum (GhPDAT1d, Gh_D09G0766), Linum usitatissimum (LuPDAT1, KC437085; LuPDAT2, KC437086; LuPDAT6, KC437087); Ricinus communis (RcPDAT1-1, AEJ32005; RcPDAT1-2, AEJ32006; RcPDAT2, AEJ32007); Sesamum indicum (SiPDAT1, XP_020553631; SiPDAT2, XP_011088820), and Saccharomyces cerevisiae (ScPDAT, NM_001183185).
Collectively, sequence analysis of the three olive PDAT showed the distinctive features typical of the PDAT family and together with phylogenetic analysis indicated that they code for phospholipid:diacylglycerol acyltransferase enzymes.
Tissue Specificity of Olive Phospholipid:Diacylglycerol Acyltransferase Genes
To investigate the distinct physiological functions of the three olive PDAT genes (OePDAT1-1, OePDAT1-2, and OePDAT2), their expression levels were analyzed in olive organs and tissues from the cultivars Picual and Arbequina that exhibit a very active lipid biosynthesis. Specifically, it has been examined young drupes before pit hardening (10–12 WAF), when the seed and mesocarp cells still have not been differentiated and no oil deposition is observed; mesocarp tissue, characterized by the presence of active chloroplasts as well as a strong TAG accumulation; developing seeds, with a high rate of storage lipid biosynthesis; and finally young leaves, where membrane lipid biosynthesis for the photosynthetic machinery is very significant.
As shown in Figure 3, the OePDAT1-1 gene was highly expressed in young drupes, leaves, and, particularly, in seeds at late stages of development (31 WAF), while OePDAT1-2 exhibited the highest transcript levels in mesocarp tissue. Regarding OePDAT2, expression levels were almost undetectable except in the case of developing seeds, as it has been reported for other plant PDAT2 genes (Ståhl et al., 2004; Pan et al., 2013). All these data indicate a spatial regulation of PDAT genes in olive since they were differentially expressed in all organs and tissues studied. Besides Arabidopsis, the presence of several PDAT isoforms have been described in numerous plant species, each one showing different expression patterns in distinct tissues. Three different PDAT genes have been described in castor bean, with RcPDAT1-1 showing high expression in vegetative tissues, and RcPDAT1-2 (PDAT1-like) and RcPDAT2 strongly and predominantly expressed in developing seeds (Kim et al., 2011). Pan et al. (2013) detected six different PDAT genes in flax. LuPDAT2 and LuPDAT4 (PDAT1 type) were strongly expressed in leaves and other vegetative tissues, whereas LuPDAT1 and LuPDAT5 (PDAT1-like), and LuPDAT3 and LuPDAT6 (PDAT2 type) were highly and majorly expressed in developing seeds. In Camelina sativa five genes have been reported (Yuan et al., 2017). CsPDAT1-A and CsPDAT1-C exhibited high transcript levels in developing seeds and leaves, respectively, while CsPDAT2-A and CsPDAT2-B showed low expression in both cases. By contrast, CsPDAT1-B was poorly expressed in all organs and tissues studied. In the case of PDAT1 and PDAT2 genes from sesame, both displayed high transcript levels in developing seeds and low in leaves and other vegetative organs (Chellamuthu et al., 2019).
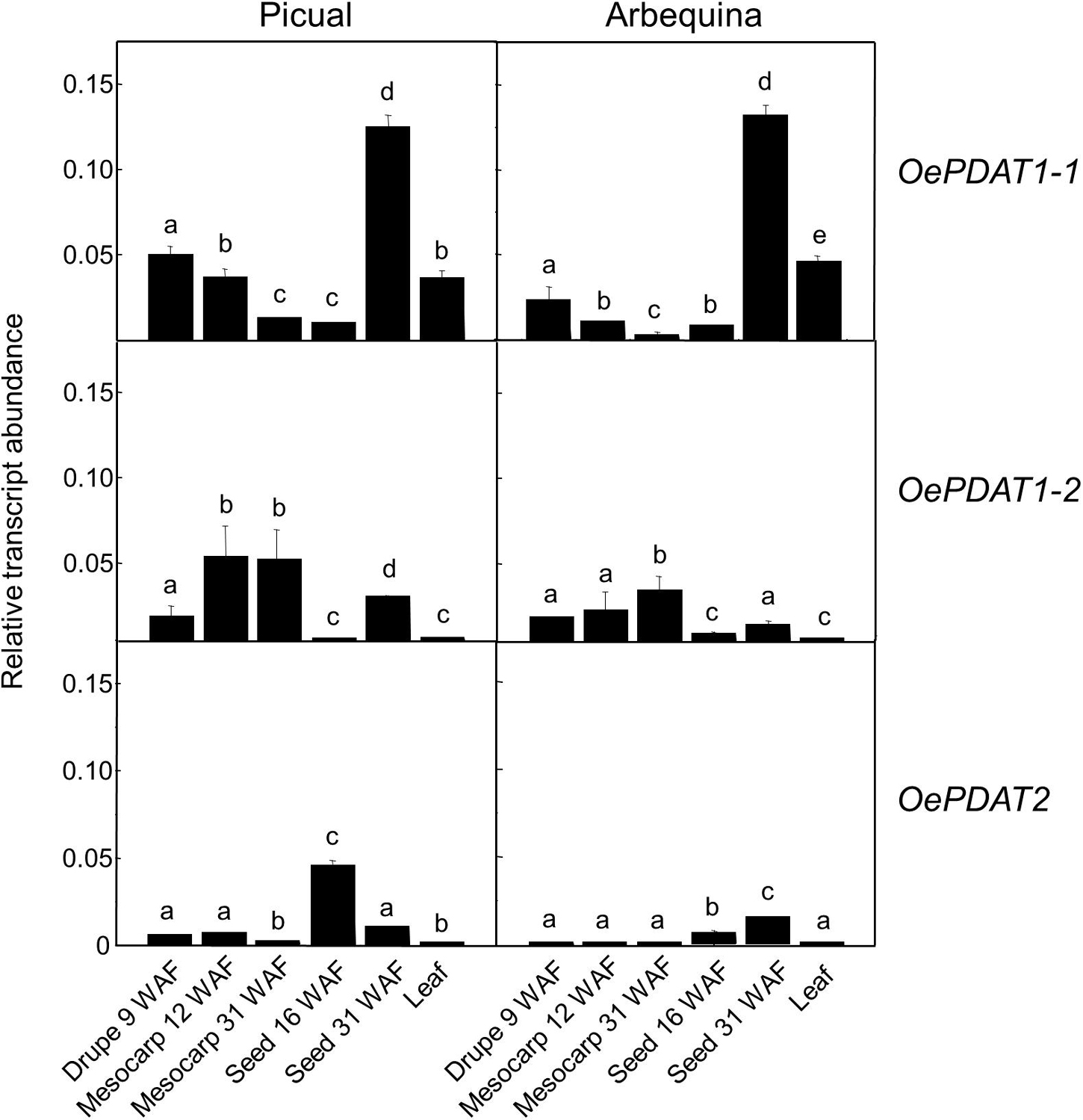
Figure 3. Relative transcript abundance of olive PDAT genes in different tissues of Picual and Arbequina cultivars. The relative transcript abundance was determined by qRT-PCR in the indicated tissues as described under section “Materials and Methods.” Data are presented as means ± SD of three biological replicates. Different letters denote significant differences (P < 0.05) for each gene and cultivar by one-way ANOVA followed by Tukey’s post-test for multiple comparisons.
In young drupes and leaves, majorly OePDAT1-1 transcripts were detected. Low levels of expression have been reported for OeDGAT1 in young drupes, while OeDGAT2 transcripts were not observed (Banilas et al., 2011). According to our results, OePDAT1-1 could be also involved together with OeDGAT1 in the synthesis of the very low amount of TAG present in this organ. In the case of leaves, relatively high transcription levels of both OeDGAT genes were observed (Banilas et al., 2011). Therefore, our expression data suggest that OePDAT1-1 could participate together with OeDGAT1 and OeDGAT2 in TAG synthesis in olive leaves. In Arabidopsis leaves, contradictory conclusions have been obtained in different studies regarding the relative contribution of DGAT1 and PDAT1 to TAG synthesis. Comparison of leaf TAG content in the wild type with that of dgat1 and pdat1 mutants indicated that in Arabidopsis leaves PDAT1 plays a more essential role than DGAT1 in TAG synthesis (Fan et al., 2013a). On the contrary, in vivo labeling experiments showed that [14C]12:0 was incorporated into TAG by leaves of the dgat1 mutant at a much lower rate than that of the pdat1 mutant, suggesting that DGAT1 is the preponderant enzyme in Arabidopsis leaves responsible for TAG synthesis (Tjellström et al., 2015). Nevertheless, medium chain fatty acids are poorly incorporated into the sn-2 position of PC, the acyl donor substrate of PDAT. Thus, it is probable that both DGAT and PDAT are involved in TAG synthesis in leaves, with the relative participation of each enzyme depending on the substrate and conditions of acyl flux (Bates, 2016).
Developmental Expression of Phospholipid:Diacylglycerol Acyltransferase Genes in the Olive Fruit in Relation to the Oil Content
Next, the transcript levels of olive PDAT genes in developing seeds and mesocarp tissue as well as the oil content in the course of fruit development and ripening from the cultivars Picual and Arbequina were examined in more depth, to investigate their specific potential contribution to TAG synthesis in these two oil-accumulating regions from the olive fruit.
Concerning developing seeds, the oil content rapidly increased from the initial stages, reaching a plateau at around 24 WAF in Picual and Arbequina cultivars (Figure 4A). Gene expression analysis revealed that OePDAT1-2 and OePDAT2 exhibited in both cultivars low and constant transcript levels during the whole period of olive fruit development and ripening (Figure 4B). Therefore, none of these two genes seems to be involved in the oil accumulation in olive developing seeds. On the contrary, OePDAT1-1 showed a significant increase in its expression levels at the onset of ripening of Picual and Arbequina cultivars (Figure 4B), suggesting that this gene could contribute to the oil seed content. However, it is important to point out that the observed increase in OePDAT1-1 transcript levels occurred when the oil content is constant at later stages of seed development, questioning the possible participation of this PDAT gene in TAG synthesis in olive developing seeds.
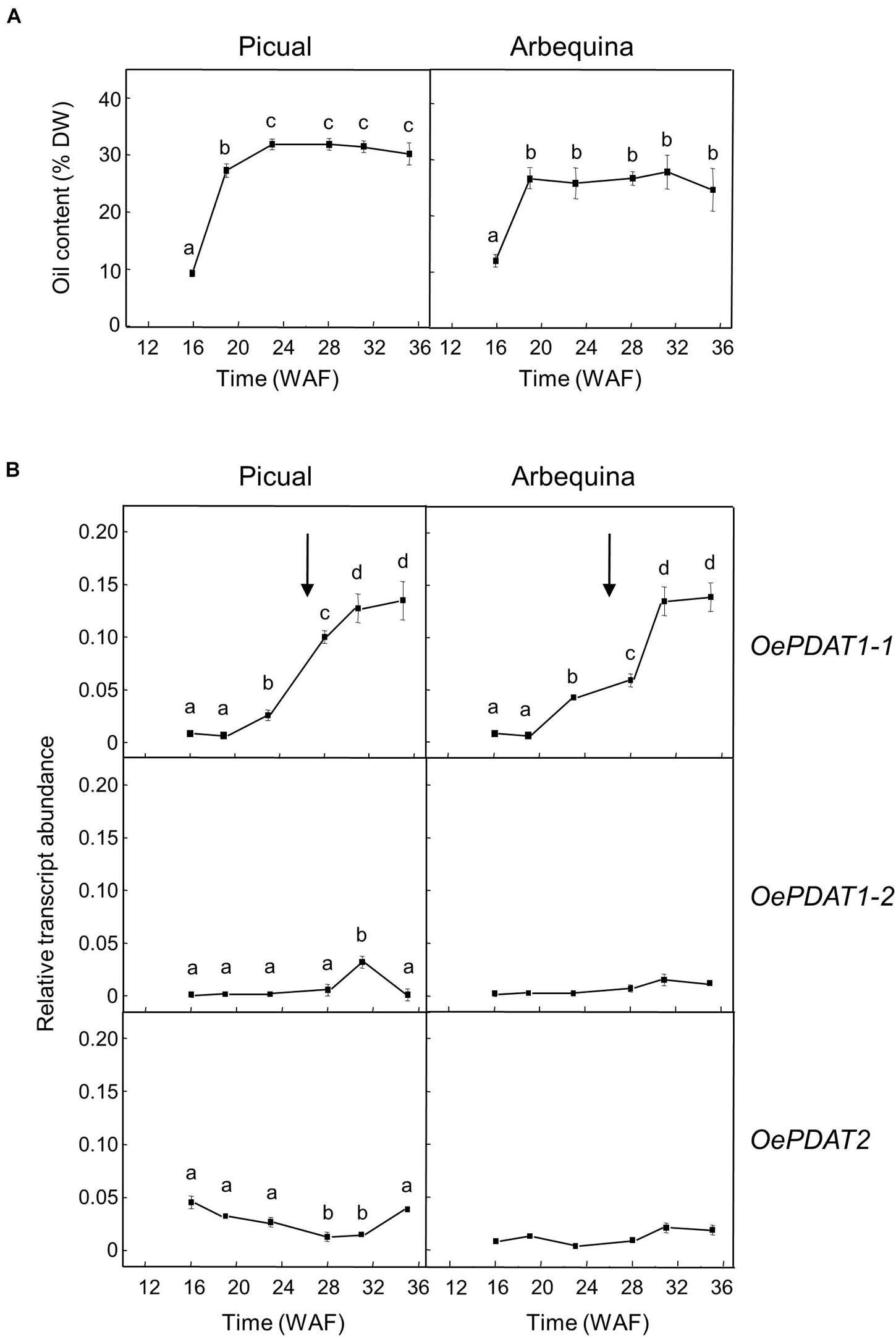
Figure 4. Time-course during olive fruit development and ripening of the oil content (A) and the relative transcript abundance of olive PDAT genes (B) in the seed tissue from Picual and Arbequina cultivars. The beginning of fruit ripening, corresponding to the appearance of pink-purple color, is denoted by an arrow. The oil content and the relative transcript abundance were determined at the indicated stages of fruit development as described under section “Materials and Methods.” Data are presented as means ± SD of three biological replicates. Different letters denote significant differences (P < 0.05) for each gene and cultivar by one-way ANOVA followed by Tukey’s post-test for multiple comparisons.
In oilseeds such as flax, the highest expression of PDAT genes in the seed was reported at the early stages of development during the phase of active oil accumulation (Pan et al., 2013). However, the Camelina sativa CsPDAT1-A and CsPDAT1-C genes augmented their transcript levels in the early and late stages of seed development, respectively, demonstrating that both genes can differently contribute to TAG synthesis (Yuan et al., 2017). In the case of the sesame PDAT genes, they showed a continuous increment of expression levels during seed development (Chellamuthu et al., 2019).
In olive developing seeds, the OeDGAT1 gene has been reported to show a bell-shaped expression pattern, with a peak at 19 WAF and a substantial reduction at 22 WAF, which is similar to that reported for the oleosin gene (Giannoulia et al., 2007). In contrast, OeDGAT2 expression levels were weak throughout the complete development period (Banilas et al., 2011). Therefore, OeDGAT1 seems to be responsible for the early and fast oil accumulation observed in olive developing seeds, with a putative contribution of OePDAT1-1 to TAG synthesis in the final stages of seed development.
A similar study was conducted in the olive mesocarp (Figure 5). Unlike olive seed, the oil accumulation in the mesocarp of both cultivars gradually increased from the beginning of the fruit development and continued during the ripening period until reach a higher oil content than the seed (Figure 5A). The expression analysis of PDAT genes in the mesocarp of Picual and Arbequina cultivars showed low transcript levels and a gradual decrease during the developmental phase for OePDAT1-1, while OePDAT2 expression was almost undetectable (Figure 5B). Conversely, a bell-shaped pattern was detected for OePDAT1-2 expression levels in both cultivars, showing a maximum at 28 WAF (Figure 5B), which can be associated with the mentioned increment in mesocarp oil content observed during the ripening phase (Figure 5A). These data suggest that the olive PDAT1-2 gene could contribute to TAG synthesis in the mesocarp when the fruit is in the course of the ripening period. Interestingly, this increase of OePDAT1-2 transcript levels detected at the onset of ripening is parallel to that reported for the linoleic acid content in the same period for both cultivars (Hernández et al., 2009). These data indicate that the OePDAT1-2 gene could be involved in the transfer of the linoleic acid synthesized de novo in the sn-2 position of PC by the microsomal oleate desaturase (FAD2) to the sn-3 position of DAG to yield TAG during the ripening period. However, fatty acid analysis of lipid classes during olive mesocarp development and ripening suggested that the incorporation of linoleic acid into TAG might preferentially occur via the Kennedy pathway, with a minor contribution of PDAT activity (Hernández et al., 2020b).
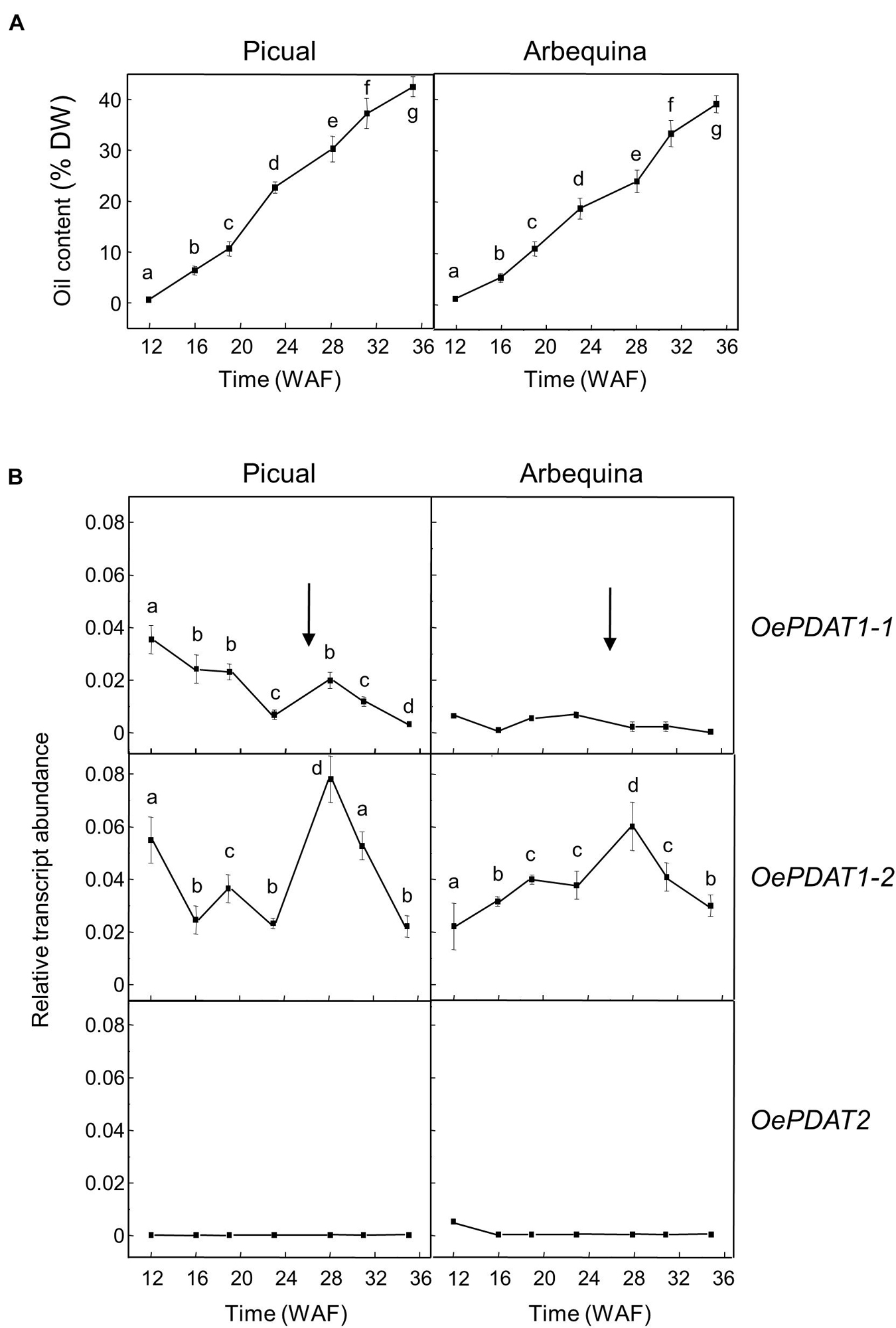
Figure 5. Time-course during olive fruit development and ripening of the oil content (A) and the relative transcript abundance of olive PDAT genes (B) in the mesocarp tissue from Picual and Arbequina cultivars. The beginning of fruit ripening, corresponding to the appearance of pink-purple color, is denoted by an arrow. The oil content and the relative transcript abundance were determined at the indicated stages of fruit development as described under section “Materials and Methods.” Data are presented as means ± SD of three biological replicates. Different letters denote significant differences (P < 0.05) for each gene and cultivar by one-way ANOVA followed by Tukey’s post-test for multiple comparisons.
In the olive mesocarp, OeDGAT1 and OeDGAT2 genes have been reported to show distinct expression patterns (Banilas et al., 2011). OeDGAT1 exhibited high transcript levels at the later stages of fruit development (19–25 WAF), with OeDGAT2 increasing its expression levels at the onset of fruit ripening. Thus, including our results, OeDGAT1 could be involved in TAG synthesis in the course of the developmental period with a minor contribution of OePDAT1-1, whereas OeDGAT2 and OePDAT1-2 may have overlapping roles for oil accumulation during the ripening phase.
All of these data also indicate a temporal regulation of the expression of PDAT genes in the olive fruit. Moreover, the fact that both, seed and mesocarp, have at least two genes involved in TAG synthesis could be to guarantee oil deposition throughout the long period of development and ripening of the olive fruit, which takes about 35–40 weeks (Sánchez, 1994). Furthermore, since the TAG content of the mesocarp, with a minor contribution of the seed, determines the total TAG content of the olive fruit, OeDGAT1, OeDGAT2, and OePDAT1-2 seem to be the genes mainly responsible for the olive oil content of the fruit.
In summary, all these results suggest that the relative contribution of PDAT and DGAT enzymes to TAG synthesis in olive seems to be tissue-dependent. Supporting this hypothesis, previous studies from our group indicated that PDAT activity may also participate in the TAG biosynthesis in olive callus culture (Hernández et al., 2008), while during pollen germination and pollen tube growth the contribution of DGAT1 but not DGAT2, PDAT1-1 and PDAT1-2 to de novo TAG synthesis has been reported (Hernández et al., 2020a).
Effect of Regulated Deficit Irrigation on Phospholipid:Diacylglycerol Acyltransferase Gene Expression in the Olive Fruit Mesocarp
Several studies have evaluated the effect of different water regimes on olive oil yield and composition (Fernández, 2014; Gonçalves et al., 2020). One of the most intriguing aspects when applying RDI strategies in olive is that a significant reduction of irrigation is not usually proportionally reflected in the reduction of oil yield (Iniesta et al., 2009; Hernandez-Santana et al., 2017). The tolerance of olive fruit to water stress has been explained by the lower sensitivity of the oil synthesis processes to water deficit than other processes like vegetative growth (Hernandez-Santana et al., 2017). Previous work from our group studied the effect of three different RDI treatments on the Arbequina mesocarp oil content, fatty acid composition, and fatty acid desaturase gene expression levels (Hernández et al., 2018). 60RDI and 30RDI treatments, which produced substantial levels of water stress, did not cause significant differences in mesocarp oil content between RDI and FI treatments, except a small decrease detected at the initial stages of mesocarp development. In line with those observations, in the present work, no significant alterations were found in the transcript levels of any of the olive PDAT genes when comparing RDI and FI treatments, apart from a slightly higher expression level for OePDAT1-1 and OePDAT2 in the case of the 60RDI and 30RDI treatments at the onset of the ripening period (Figure 6). On the contrary, a strong increase and decrease in the expression levels of CsPDAT2-A and CsPDAT2-B genes, respectively, have been reported in Camelina sativa seedlings in response to drought treatment (Yuan et al., 2017). These contrasting results could suggest the existence of species-dependent transcriptional mechanisms affecting PDAT genes in the water stress response.
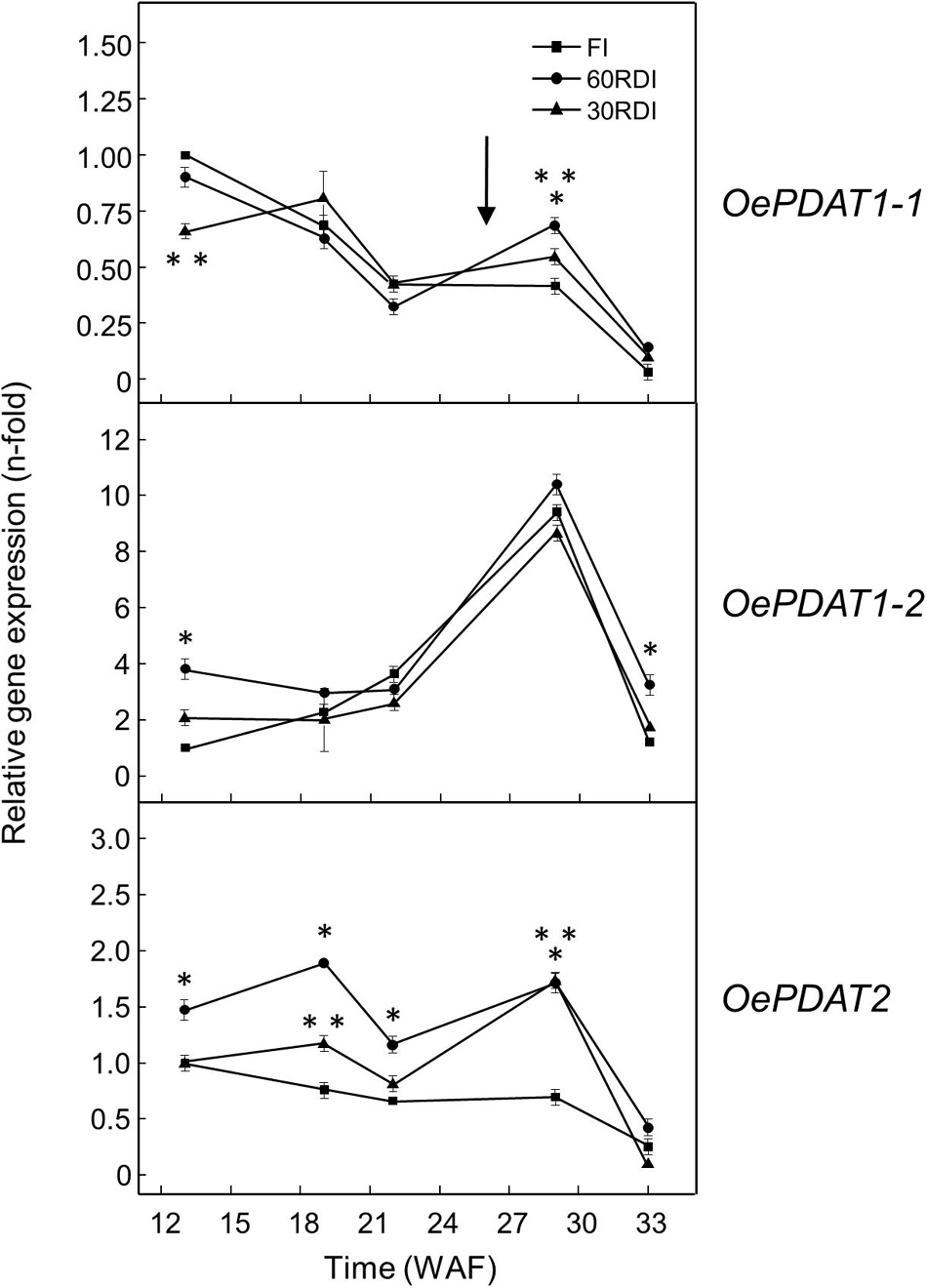
Figure 6. Effect of regulated deficit irrigation treatments on the relative expression levels of olive PDAT genes in the mesocarp tissue from cultivar Arbequina during olive fruit development and ripening. The relative expression levels were determined by qRT-PCR at the indicated stages of fruit development as described in “Materials and Methods,” using the expression level of the corresponding gene at 13 WAF from FI treatment as calibrator. Data are presented as means ± SD of three biological replicates. ∗Indicates that 60 RDI is significantly different (P < 0.05) to FI by two-way ANOVA with a Bonferroni post-test. ∗∗Indicates that 30 RDI is significantly different (P < 0.05) to FI by two-way ANOVA with a Bonferroni post-test.
Transcriptional Regulation of Phospholipid:Diacylglycerol Acyltransferase Genes in the Olive Fruit Mesocarp in Response to Abiotic Stresses
To analyze the effect of distinct abiotic stresses on the transcript levels of the olive PDAT genes in Picual and Arbequina mesocarp tissue, olive tree branches with olive fruit at turning stage (28 WAF) were incubated for 24 h altering the standard conditions (25°C with 12 h light/12 h dark cycle) dependent on the effect to be examined. No significant changes in the oil content were observed in the fruit mesocarp during the stress treatments, very likely because the timescale involved (24 h) may be too short to observe that effect (Supplementary Figures 2, 3). In addition, no substantial variations in the expression levels of olive PDAT genes were detected in the mesocarp tissue when olive fruit were incubated under the above-mentioned standard conditions (Supplementary Figure 4).
The incubation at low temperature (15°C) of olive fruit only caused a strong increment of 12-fold in OePDAT1-1 of cultivar Picual transcript levels during the first 6 h of treatment, keeping high values the rest of the time of the experiment (Figure 7A). These results indicate a cultivar-dependent differential transcriptional response to cold of the olive PDAT1-1 gene, as it has been reported for the olive FAD2 genes (Hernández et al., 2020b). A similar up-regulation has been described for CsPDAT1-A and CsPDAT1-C genes in Camelina sativa seedlings under cold stress (Yuan et al., 2017). Regarding high temperature, when olive fruit was incubated at 35°C the transcript levels of the three olive PDAT genes remained almost unaffected (Figure 7B). Interestingly, it has been recently reported that heat stress stimulates AtPDAT1 expression in rosettes of Arabidopsis seedlings (Demski et al., 2020). Furthermore, AtPDAT1-mediated TAG accumulation in Arabidopsis seedlings has been found to increase heat resistance and augments basal thermotolerance (Mueller et al., 2017). All these data indicate that PDAT plays a role in the response and adaptation of plants to temperature alterations.
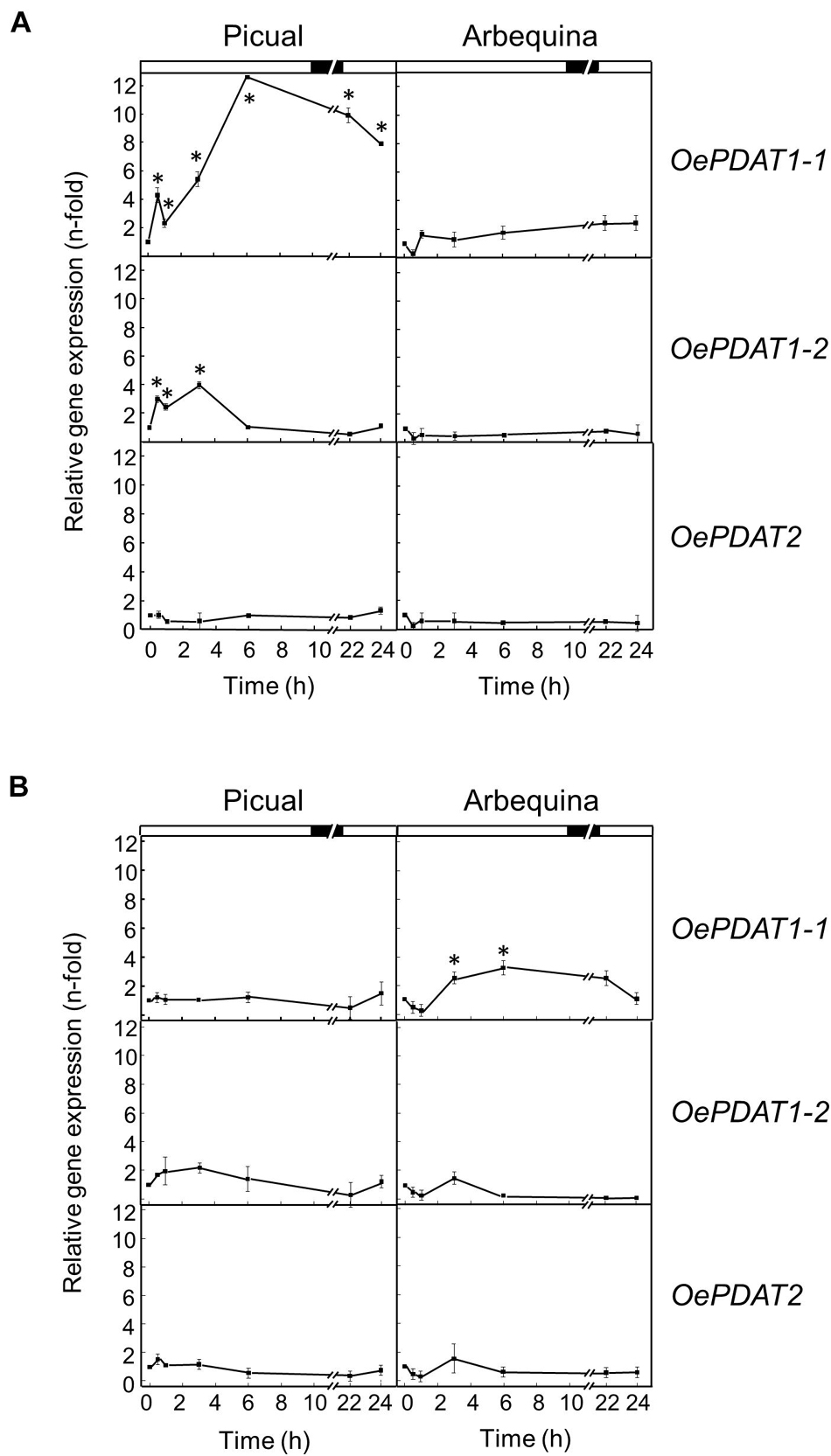
Figure 7. Effect of low temperature (A) and high temperature (B) on the relative expression levels of olive PDAT genes in the mesocarp tissue from Picual and Arbequina cultivars. Olive tree branches with about 100 olive fruit (28 WAF) were incubated using standard conditions except that the temperature was 15°C (A) or 35°C (B). At the indicated times, the relative expression levels were determined by qRT-PCR as described in “Materials and Methods,” using the expression level of the corresponding gene at zero time as calibrator. Data are presented as means ± SD of three biological replicates. ∗Indicates significantly different (P < 0.05) to time 0 h by two-way ANOVA with a Bonferroni post-test. Boxes in the upper part indicate light (open) or dark (closed) periods.
To examine whether darkness affects PDAT expression levels in the olive mesocarp from Picual and Arbequina cultivars, olive branches were incubated at 25°C for 24 h in the darkness. Expression analysis showed a fast and strong decline of OePDAT1-1, OePDAT1-2, and OePDAT2 transcript levels in both cultivars mostly during the first 3 h of treatment, remaining with small values the rest of the experiment (Figure 8A). Interestingly, the inactivation by darkness of the acetyl-CoA carboxylase enzyme, which catalyzed the first committed step of de novo fatty acid synthesis in plants, has been reported in Arabidopsis (Ye et al., 2020), which is in accordance with the observed down-regulation of olive PDAT genes. Additionally, in agreement with our results, Fan et al. (2017) reported that increased TAG accumulation in Arabidopsis leaves by overexpression of AtPDAT1 before prolonged dark treatment enhanced oxidative stress and dark-induced cell death. These data indicate that PDAT is not involved in plant survival under extended darkness.
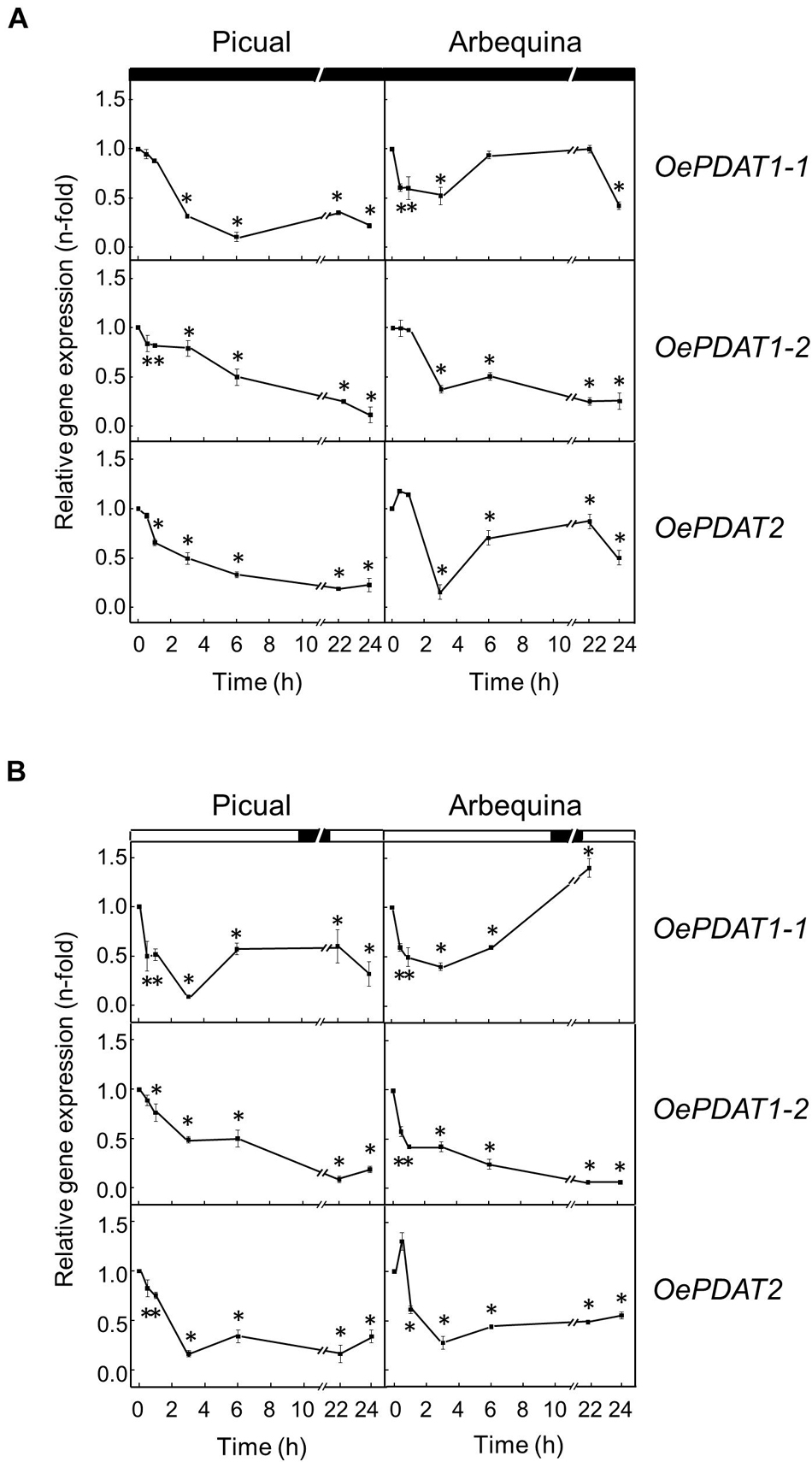
Figure 8. Effect of darkness (A) and wounding (B) on the relative expression levels of olive PDAT genes in the mesocarp tissue from Picual and Arbequina cultivars. Olive tree branches with about 100 olive fruit (28 WAF) were incubated using standard conditions except that the olive fruit were incubated under darkness (A) or were mechanically damaged (B). At the indicated times, the relative expression levels were determined by qRT-PCR as described in “Materials and Methods,” using the expression level of the corresponding gene at zero time as calibrator. Data are presented as means ± SD of three biological replicates. ∗Indicates significantly different (P < 0.05) to time 0 h by two-way ANOVA with a Bonferroni post-test. Boxes in the upper part indicate light (open) or dark (closed) periods.
Finally, the effect of wounding on the expression levels of olive PDAT genes was investigated in the olive mesocarp from Picual and Arbequina cultivars subjected to mechanical damage from branches incubated at standard conditions. First, it was confirmed that the wounding treatment of the mesocarp tissue was properly carried out because olive 13-lipoxygenase and 13-hydroperoxide lyase genes, which have been previously shown to be wound-inducible in plant tissues (Padilla et al., 2014), were transiently induced (Supplementary Figure 5 and Supplementary Table 1). In both cultivars, OePDAT1-1, OePDAT1-2, and OePDAT2 transcript levels rapidly and markedly decreased during the first 3 h after wounding, keeping at low levels the rest of the incubation (Figure 8B). The observed down-regulation of olive PDAT gene expression in response to wounding could be explained to avoid the channeling of linoleic and linolenic acids from PC to be accumulated in TAG since both polyunsaturated fatty acids can be transformed to lipid peroxides that may act as antimicrobial compounds (Wang et al., 1998), or they could act as precursors via the lipoxygenase pathway of oxylipins, which constitute signal molecules involved in plant defense (Wasternack and Feussner, 2018). Accordingly, the transient induction of several FAD2 genes has been described in the olive mesocarp in response to wounding (Hernández et al., 2011, 2020b).
Conclusion
The isolation and characterization of three olive PDAT genes have been carried out. Sequence analysis of these genes (OepPDAT1-1, OepPDAT1-2, and OepPDAT2) indicates that they code for PDAT enzymes. Transcript profiling shows a spatial and temporal regulation of the expression levels of the PDAT genes in the olive fruit during development and ripening and, together with the pattern of oil accumulation suggest that, in addition to DGAT genes, OePDAT1-1 could participate in the TAG synthesis in the seed, while OePDAT1-2 may contribute to the TAG content in the mesocarp and, therefore, in the olive oil. These data also indicate that the relative contribution of PDAT and DGAT enzymes to TAG synthesis in olive seems to be organ and tissue-dependent. Moreover, the expression of PDAT genes in the olive fruit is regulated by water regime, temperature, light, and wounding, indicating that PDAT is involved in the response to a range of relevant abiotic stresses. This research constitutes a significant step to elucidate the factors controlling the oil content and accumulation in oil fruit. With regard to olive, this information will help to design molecular markers for the marker-assisted selection of novel olive cultivars with increased oil content.
Data Availability Statement
The original contributions presented in the study are included in the article/Supplementary Material, further inquiries can be directed to the corresponding author/s.
Author Contributions
MLH managed and performed the harvest of plant material and the stress experiments, and carried out RNA isolation, cDNA synthesis, and oil content determination. SM, MDS, ÚG, and AP performed the gene cloning and qRT-PCR analysis. LS revised the study and the manuscript. JM-R conceived and designed the study and wrote the manuscript. All authors discussed, commented, and approved the final version of the manuscript.
Funding
This work was funded by the Spanish Ministry of Science, Innovation, and Universities through Research Grant AGL2017-87871-R (AEI/FEDER, UE). Agrobioscience Ph.D. program at Scuola Superiore Sant’Anna granted the scholarship to SM.
Conflict of Interest
The authors declare that the research was conducted in the absence of any commercial or financial relationships that could be construed as a potential conflict of interest.
Publisher’s Note
All claims expressed in this article are solely those of the authors and do not necessarily represent those of their affiliated organizations, or those of the publisher, the editors and the reviewers. Any product that may be evaluated in this article, or claim that may be made by its manufacturer, is not guaranteed or endorsed by the publisher.
Acknowledgments
We thank to Drs. José Enrique Fernández and Antonio Díaz-Espejo (IRNAS-CSIC, Seville, Spain) for the availability of the Sanabria olive orchard to harvest olive fruit samples with different irrigation treatments.
Supplementary Material
The Supplementary Material for this article can be found online at: https://www.frontiersin.org/articles/10.3389/fpls.2021.751959/full#supplementary-material
Footnotes
- ^ http://www.ncbi.nlm.nih.gov/structure/cdd/wrpsb.cgi
- ^ https://pfam.xfam.org/
- ^ http://www.cbs.dtu.dk/services/NetNGlyc/
- ^ http://www.cbs.dtu.dk/services/TMHMM/
- ^ http://www.softberry.com
- ^ http://www.cbs.dtu.dk/services/TargetP/
- ^ http://bioinfo.ut.ee/primer3/
References
Atienza, S. G., De la Rosa, R., León, L., Martín, A., and Belaj, A. (2014). Identification of QTL for agronomic traits of importance for olive breeding. Mol. Breed. 34, 725–737. doi: 10.1007/s11032-014-0070-y
Aznar-Moreno, J. A., and Durrett, T. P. (2017). Simultaneous targeting of multiple gene homeologs to alter seed oil production in Camelina sativa. Plant Cell Physiol. 58, 1260–1267. doi: 10.1093/pcp/pcx058
Baldoni, L., and Belaj, A. (2009). “Olive,” in Oils Crops, Handbook of Plant Breeding, eds J. Vollmann and I. Rajcan (New York: Springer), 397–421.
Banás, W., Sánchez García, A., Banás, A., and Stymne, S. (2013). Activities of acyl- CoA:diacylglycerol acyltransferase (DGAT) and phospholipid:diacylglycerol acyltransferase (PDAT) in microsomal preparations of developing sunflower and safflower seeds. Planta 237, 1627–1636. doi: 10.1007/s00425-013-1870-8
Banilas, G., Karampelias, M., Makariti, I., Kourti, A., and Hatzopoulos, P. (2011). The olive DGAT2 gene is developmentally regulated and shares overlapping but distinct expression patterns with DGAT1. J. Exp. Bot. 62, 521–532. doi: 10.1093/jxb/erq286
Bates, P. D. (2016). Understanding the control of acyl flux through the lipid metabolic network of plant oil biosynthesis. Biochim. Biophys. Acta 1861, 1214–1225. doi: 10.1016/j.bbalip.2016.03.021
Carlsson, A. S., Yilmaz, J. L., Green, A. G., Stymne, S., and Hofvander, P. (2011). Replacing fossil oil with fresh oil – with what and for what? Eur. J. Lipid Sci. Technol. 113, 812–831. doi: 10.1002/ejlt.201100032
Chapman, K. D., and Ohlrogge, J. B. (2012). Compartmentation of triacylglycerol accumulation in plants. J. Biol. Chem. 287, 2288–2294. doi: 10.1074/jbc.R111.290072
Chellamuthu, M., Kumaresan, K., Subramanian, S., and Muthumanickam, H. (2019). Functional analysis of sesame diacylglycerol acyltransferase and phospholipid:diacylglycerol acyltransferase genes using in silico and in vitro approaches. Plant Mol. Biol. Rep. 37, 146–156. doi: 10.1007/s11105-019-01144-7
Chen, G., Woodfield, H. K., Pan, X., Harwood, J. L., and Weselake, R. J. (2015). Acyl-trafficking during plant oil accumulation. Lipids 50, 1057–1068. doi: 10.1007/s11745-015-4069-x
Covas, M. I. (2008). Bioactive effects of olive oil phenolic compounds in humans: reduction of heart disease factors and oxidative damage. Inflammopharmacology 16, 216–218. doi: 10.1007/s10787-008-8019-6
Dahlqvist, A., Stahl, U., Lenman, M., Banas, A., Lee, M., Sandager, L., et al. (2000). Phospholipid:diacylglycerol acyltransferase: an enzyme that catalyzes the acyl-CoA-independent formation of triacylglycerol in yeast and plants. Proc. Natl. Acad. Sci. U.S.A. 97, 6487–6492. doi: 10.1073/pnas.120067297
Demski, K., Łosiewska, A., Jasieniecka-Gazarkiewicz, K., Klinska, S., and Banas, A. (2020). Phospholipid:diacylglycerol acyltransferase1 overexpression delays senescence and enhances post-heat and cold exposure fitness. Front. Plant Sci. 11:611897. doi: 10.3389/fpls.2020.611897
Falarz, L. J., Xu, Y., Caldo, K. M. P., Garroway, C. J., Singer, S. D., and Chen, G. (2020). Characterization of the diversification of phospholipid:diacylglycerol acyltransferases in the green lineage. Plant J. 103, 2025–2038. doi: 10.1111/tpj.14880
Fan, J., Yan, C., and Xu, C. (2013a). Phospholipid:diacylglycerol acyltransferase-mediated triacylglycerol biosynthesis is crucial for protection against fatty acid-induced cell death in growing tissues of Arabidopsis. Plant J. 76, 930–942. doi: 10.1111/tpj.12343
Fan, J., Yan, C., Zhang, X., and Xu, C. (2013b). Dual role for phospholipid: diacylglycerol acyltransferase: enhancing fatty acid synthesis and diverting fatty acids from membrane lipids to triacylglycerol in Arabidopsis leaves. Plant Cell 25, 3506–3518. doi: 10.1105/tpc.113.117358
Fan, J., Yan, C., Roston, R., Shanklin, J., and Xu, C. (2014). Arabidopsis lipins, PDAT1 acyltransferase, and SDP1 triacylglycerol lipase synergistically direct fatty acids toward β-oxidation, thereby maintaining membrane lipid homeostasis. Plant Cell 26, 4119–4134. doi: 10.1105/tpc.114.130377
Fan, J., Yu, L., and Xu, C. (2017). A central role for triacylglycerol in membrane lipid breakdown, fatty acid β-oxidation, and plant survival under extended darkness. Plant Physiol. 174, 1517–1530. doi: 10.1104/pp.17.00653
Fan, J., Zhou, C., Yu, L., Li, P., Shanklin, J., and Xu, C. (2019). Diversion of carbon flux from sugars to lipids improves the growth of an Arabidopsis starchless mutant. Plants 8:229. doi: 10.3390/plants8070229
Feng, Y., Zhang, Y., Ding, W., Wu, P., Cao, X., and Xue, S. (2019). Expanding of phospholipid:diacylglycerol acyltransferase (PDAT) from Saccharomyces cerevisiae as multifunctional biocatalyst with broad acyl donor/acceptor selectivity. Appl. Biochem. Biotechnol. 188, 824–835. doi: 10.1007/s12010-019-02954-x
Fernández, J. E. (2014). Understanding olive adaptation to abiotic stresses as a tool to increase crop performance. Environ. Exp. Bot. 103, 158–179. doi: 10.1016/j.envexpbot.2013.12.003
Fernández, J. E., Pérez-Martín, A., Torres-Ruiz, J. M., Cuevas, M. V., Rodríguez-Domínguez, C. M., Elsayed-Farag, S., et al. (2013). A regulated deficit irrigation strategy for hedgerow olive orchards with high plant density. Plant Soil 372, 279–295. doi: 10.1007/s11104-013-1704-2
Garcés, R., and Mancha, M. (1993). One-step lipid extraction and fatty acid methyl esters preparation from fresh plant tissues. Anal. Biochem. 211, 139–143. doi: 10.1006/abio.1993.1244
Ghosal, A., Banas, A., Stahl, U., Dahlqvist, A., Lindqvist, Y., and Stymne, S. (2007). Saccharomyces cerevisiae phospholipid:diacylglycerol acyl transferase (PDAT) devoid of its membrane anchor region is a soluble and active enzyme retaining its substrate specificities. Biochim. Biophys. Acta 1771, 1457–1463. doi: 10.1016/j.bbalip.2007.10.007
Giannoulia, K., Banilas, G., and Hatzopoulos, P. (2007). Oleosin gene expression in olive. J. Plant Physiol. 164, 104–107. doi: 10.1016/j.jplph.2006.03.016
Giannoulia, K., Haralampidis, K., Poghosyan, Z., Murphy, D. J., and Hatzopoulos, P. (2000). Differential expression of diacylglycerol acyltransferase (DGAT) genes in olive tissues. Biochem. Soc. Trans. 28, 695–697. doi: 10.1042/BST0280695
Gonçalves, A., Silva, E., Brito, C., Martins, S., Pinto, L., Dinis, L. T., et al. (2020). Olive tree physiology and chemical composition of fruits are modulated by different deficit irrigation strategies. J. Sci. Food Agric. 100, 682–694. doi: 10.1002/jsfa.10064
Hara, A., and Radin, N. S. (1978). Lipid extraction of tissues with a low-toxicity-solvent. Anal. Biochem. 90, 420–426. doi: 10.1016/0003-2697(78)90046-5
Haralampidis, K., Milioni, D., Sánchez, J., Baltrusch, M., Heinz, E., and Hatzopoulos, P. (1998). Temporal and transient expression of stearoyl-ACP carrier protein desaturase gene during olive fruit development. J. Exp. Bot. 49, 1661–1669. doi: 10.1093/jexbot/49.327.1661
Hernández, M. L., Guschina, I. A., Martínez-Rivas, J. M., Mancha, M., and Harwood, J. L. (2008). The utilization and desaturation of oleate and linoleate during glycerolipid biosynthesis in olive (Olea europaea L.) callus cultures. J. Exp. Bot. 59, 2425–2435. doi: 10.1093/jxb/ern121
Hernández, M. L., Lima-Cabello, E., Alché, J., de, D., Martínez-Rivas, J. M., and Castro, A. J. (2020a). Lipid composition and associated gene expression patterns during pollen germination and pollen tube growth in olive (Olea europaea L.). Plant Cell Physiol. 61, 1348–1364. doi: 10.1093/pcp/pcaa063
Hernández, M. L., Sicardo, M. D., Arjona, P. M., and Martínez-Rivas, J. M. (2020b). Specialized functions of olive FAD2 gene family members related to fruit development and the abiotic stress response. Plant Cell Physiol. 61, 427–441. doi: 10.1093/pcp/pcz208
Hernández, M. L., Mancha, M., and Martínez-Rivas, J. M. (2005). Molecular cloning and characterization of genes encoding two microsomal oleate desaturases (FAD2) from olive. Phytochemistry 66, 1417–1426. doi: 10.1016/j.phytochem.2005.04.004
Hernández, M. L., Padilla, M. N., Mancha, M., and Martínez-Rivas, J. M. (2009). Expression analysis identifies FAD2-2 as the olive oleate desaturase gene mainly responsible for the linoleic acid content in virgin olive oil. J. Agric. Food Chem. 57, 6199–6220. doi: 10.1021/jf900678z
Hernández, M. L., Padilla, M. N., Sicardo, M. D., Mancha, M., and Martínez-Rivas, J. M. (2011). Effect of different environmental stresses on the expression of oleate desaturase genes and fatty acid composition in olive fruit. Phytochemistry 72, 178–187. doi: 10.1016/j.phytochem.2010.11.026
Hernández, M. L., Sicardo, M. D., Alfonso, M., and Martínez-Rivas, J. M. (2019). Transcriptional regulation of stearoyl-acyl carrier protein desaturase genes in response to abiotic stresses leads to changes in the unsaturated fatty acids composition of olive mesocarp. Front. Plant Sci. 10:251. doi: 10.3389/fpls.2019.00251
Hernández, M. L., Velázquez-Palmero, D., Sicardo, M. D., Fernández, J. E., Diaz-Espejo, A., and Martínez-Rivas, J. M. (2018). Effect of a regulated deficit irrigation strategy in a hedgerow ‘Arbequina’ olive orchard on the mesocarp fatty acid composition and desaturase gene expression with respect to olive oil quality. Agric. Water Manag. 204, 100–106. doi: 10.1016/j.agwat.2018.04.002
Hernandez-Santana, V., Fernández, J. E., Cuevas, M. V., Pérez-Martín, A., and Diaz-Espejo, A. (2017). Photosynthetic limitations by water deficit: Effect on fruit and olive oil yield, leaf area and trunk diameter and its potential use to control vegetative growth of super-high density olive orchards. Agric. Water Manag. 184, 9–18. doi: 10.1016/j.agwat.2016.12.016
Iniesta, F., Testi, L., Orgaz, F., and Villalobos, F. J. (2009). The effects of regulated and continuous deficit irrigation on the water use, growth and yield of olive trees. Eur. J. Agron. 30, 258–265. doi: 10.1016/j.eja.2008.12.004
Kim, H. U., Lee, K. R., Go, Y. S., Jung, J. H., Suh, M. C., and Kim, J. B. (2011). Endoplasmic reticulum-located PDAT1-2 from castor bean enhances hydroxy fatty acid accumulation in transgenic plants. Plant Cell Physiol. 52, 983–993. doi: 10.1093/pcp/pcr051
Lee, H. G., Kim, H., Suh, M. C., Kim, H. U., and Seo, P. J. (2018). The MYB96 transcription factor regulates triacylglycerol accumulation by activating DGAT1 and PDAT1 expression in Arabidopsis seeds. Plant Cell Physiol. 59, 1432–1442. doi: 10.1093/pcp/pcy073
Liu, Q., Siloto, R. M. P., Lehner, R., Stone, S. J., and Weselake, R. J. (2012). Acyl-CoA:diacylglycerol acyltransferase: molecular biology, biochemistry and biotechnology. Prog. Lipid Res. 51, 350–377. doi: 10.1016/j.plipres.2012.06.001
Liu, X. Y., Ouyang, L. L., and Zhou, Z. G. (2016). Phospholipid: diacylglycerol acyltransferase contributes to the conversion of membrane lipids into triacylglycerol in Myrmecia incisa during the nitrogen starvation stress. Sci. Rep. 6:26610. doi: 10.1038/srep26610
Livak, K. J., and Schmittgen, T. D. (2001). Analysis of relative gene expression data using real-time quantitative PCR and the 2ΔΔCT method. Methods 25, 402–408. doi: 10.1006/meth.2001
Lung, S. C., and Weselake, R. J. (2006). Diacylglycerol acyltransferase: a key mediator of plant triacylglycerol synthesis. Lipids 41, 1073–1088. doi: 10.1007/s11745-006-5057-y
Lunn, D., Le, A., Wallis, J. G., and Browse, J. (2020). Castor LPCAT and PDAT1A act in concert to promote transacylation of hydroxy-fatty acid onto triacylglycerol. Plant Physiol. 184, 709–719. doi: 10.1104/pp.20.00691
Marmon, S. K., Sturtevant, D., Herrfurth, C., Chapman, K. D., Stymne, S., and Feussner, I. (2017). Two acyltransferases contribute differently to linolenic acid levels in seed oil. Plant Physiol. 173, 2081–2095. doi: 10.1104/pp.16.01865
Martinelle, M., Holmquist, M., Clausen, I. G., Patkar, S., Svendsen, A., and Hult, K. (1996). The role of Glu87 and Trp89 in the lid of Humicola lanuginosa lipase. Protein Eng. 9, 519–524. doi: 10.1093/protein/9.6.519
McCartney, A. W., Dyer, J. M., Dhanoa, P. K., Kim, P. K., Andrews, D. W., McNew, J. A., et al. (2004). Membrane-bound fatty acid desaturases are inserted co-translationally into the ER and contain different ER retrieval motifs at their carboxy termini. Plant J. 37, 156–173. doi: 10.1111/j.1365-313X.2004.01949.x
Mhaske, V., Beldjilali, K., Ohlrogge, J., and Pollard, M. (2005). Isolation and characterization of an Arabidopsis thaliana knockout line for phospholipid: diacylglycerol transacylase gene (At5g13640). Plant Physiol. Biochem. 43, 413–417. doi: 10.1016/j.plaphy.2005.01.013
Mueller, S., Unger, M., Guender, L., Fekete, A., and Mueller, M. (2017). Phospholipid:diacylglycerol acyltransferase-mediated triacylglycerol synthesis augments basal thermotolerance. Plant Physiol. 175, 486–497. doi: 10.1104/pp.17.00861
Muñoz-Mérida, A., González-Plata, J. J., Cañada, A., Blanco, A. M., García-López, M. C., Rodríguez, J. M., et al. (2013). De novo assembly and functional annotation of the olive (Olea europaea) transcriptome. DNA Res. 20, 93–108. doi: 10.1093/dnares/dss036
Padilla, M. N., Hernández, M. L., Sanz, C., and Martínez-Rivas, J. M. (2014). Stress-dependent regulation of 13-lypoxygenases and 13-hydroperoxide lyase in olive fruit mesocarp. Phytochemistry 102, 80–88. doi: 10.1016/j.phytochem.2014.01.024
Pan, X., Peng, F. Y., and Weselake, R. J. (2015). Genome-wide analysis of phospholipid:diacylglycerol acyltransferase (PDAT) genes in plants reveals the eudicot-wide PDAT gene expansion and altered selective pressures acting on the core eudicot PDAT paralogs. Plant Physiol. 167, 87–90. doi: 10.1104/pp.114.253658
Pan, X., Siloto, R. M. P., Wickramarathna, A. D., Mietkiewska, E., and Weselake, R. J. (2013). Identification of a pair of phospholipid:diacylglycerol acyltransferases from developing flax (Linum usitatissimum L.) seed catalyzing the selective production of trilinolenin. J. Biol. Chem. 288, 24173–24188. doi: 10.1074/jbc.M113.475699
Peelman, F., Verschelde, J. L., Vanloo, B., Ampe, C., Labeur, C., Tavernier, J., et al. (1999). Effects of natural mutations in lecithin:cholesterol acyltransferase on the enzyme structure and activity. J. Lipid Res. 40, 59–69. doi: 10.1016/S0022-2275(20)33339-3
Peelman, F., Vinaimont, N., Verhee, A., Vanloo, B., Verschelde, J. L., Labeur, C., et al. (1998). A proposed architecture for lecithin cholesterol acyl transferase (LCAT): identification of the catalytic triad and molecular modeling. Protein Sci. 7, 587–599. doi: 10.1002/pro.5560070307
Pelham, H. R. B. (2000). Using sorting signals to retain proteins in endoplasmic reticulum. Methods Enzymol. 327, 279–283. doi: 10.1016/s0076-6879(00)27283-2
Pfaffl, M. W. (2004). “Quantification strategies in real-time PCR,” in A-Z of Quantitative PCR, ed. S. A. Bustin (La Jolla: International University Line), 87–112.
Rallo, L., Barranco, D., Díez, C. M., Rallo, P., Suárez, M. P., Trapero, C., et al. (2018). “Strategies for olive (Olea europaea L.) breeding: cultivated genetic resources and crossbreeding,” in Advances in Plant Breeding Strategies, eds J. M. Al-Khayri, S. M. Jain, and D. V. Johnson (Cham: Springer), 535–600.
Román, A., Hernández, M. L., Soria-García, A., López-Gomollón, S., Lagunas, B., Picorel, R., et al. (2015). Non-redundant contribution of the plastidial FAD8 ω-3 desaturase to glycerolipid unsaturation at different temperatures in arabidopsis. Mol. Plant 8, 1599–1611. doi: 10.1016/j.molp.2015.06.004
Sánchez, J. (1994). Lipid photosynthesis in olive fruit. Prog. Lipid Res. 33, 97–104. doi: 10.1016/0163-7827(94)90012-4
Schrag, J. D., and Cygler, M. (1997). Lipases and the α/β hydrolase fold. Methods Enzymol. 284, 85–107. doi: 10.1016/s0076-6879(97)84006-2
Ståhl, U., Carlsson, A. S., Lenman, M., Dahlqvist, A., Huang, B., Banas, W., et al. (2004). Cloning and functional characterization of a phospholipid:diacylglycerol acyltransferase from Arabidopsis. Plant Physiol. 135, 1324–1335. doi: 10.1104/pp.104.044354
Tjellström, H., Strawsine, M., and Ohlrogge, J. B. (2015). Tracking synthesis and turnover of triacylglycerol in leaves. J. Exp. Bot. 66, 1453–1461. doi: 10.1093/jxb/eru500
Unver, T., Wu, Z., Sterck, L., Turktas, M., Lohaus, R., Li, Z., et al. (2017). Genome of wild olive and the evolution of oil biosynthesis. Proc. Nat. Acad. Sci. U.S.A. 114, 9413–9422. doi: 10.1073/pnas.1708621114
van Erp, H., Bates, P. D., Burgal, J., Shockey, J., and Browse, J. (2011). Castor phospholipid:diacylglycerol acyltransferase facilitates efficient metabolism of hydroxy fatty acids in transgenic Arabidopsis. Plant Physiol. 155, 683–693. doi: 10.1104/pp.110.167239
van Erp, H., Shockey, J., Zhang, M., Adhikari, N. D., and Browse, J. (2015). Reducing isozyme competition increases target fatty acid accumulation in seed triacylglycerols of transgenic Arabidopsis. Plant Physiol. 168, 36–46. doi: 10.1104/pp.114.254110
Wang, C., Chin, C. K., and Chen, A. (1998). Expression of the yeast Δ-9 desaturase gene in tomato enhances its resistance to powdery mildew. Physiol. Mol. Plant Pathol. 52, 371–383. doi: 10.1006/pmpp.1998.0158
Wasternack, C., and Feussner, I. (2018). The oxylipin pathways: biochemistry and function. Annu. Rev. Plant Biol. 69, 363–386. doi: 10.1146/annurev-arplant-042817-040440
Weselake, R. J., Taylor, D. C., Rahman, M. H., Shah, S., Laroche, A., McVetty, P. B. E., et al. (2009). Increasing the flow of carbon into seed oil. Biotechnol. Adv. 27, 866–878. doi: 10.1016/j.biotechadv.2009.07.001
Xu, J., Carlsson, A. S., Francis, T., Zhang, M., Hoffman, T., Giblin, M. E., et al. (2012). Triacylglycerol synthesis by PDAT1 in the absence of DGAT1 activity is dependent on re-acylation of LPC by LPCAT2. BMC Plant Biol. 12:4. doi: 10.1186/1471-2229-12-4
Xu, Y., Caldo, K. M. P., Pal-Nath, D., Ozga, J., Lemieux, M. J., Weselake, R. J., et al. (2018). Properties and biotechnological applications of acyl-CoA: diacylglycerol acyltransferase and phospholipid: diacylglycerol acyltransferase from terrestrial plants and microalgae. Lipids 53, 663–688. doi: 10.1002/lipd.12081
Yang, Y., and Benning, C. (2018). Functions of triacylglycerols during plant stress and development. Curr. Opin. Biotechnol. 49, 191–198. doi: 10.1016/j.copbio.2017.09.003
Ye, Y., Fulcher, Y. G., Sliman, D. J., Day, M. T., Schroeder, M. J., Koppisetti, R. K., et al. (2020). The BADC and BCCP subunits of chloroplast acetyl-CoA carboxylase sense the pH changes of the light-dark cycle. J. Biol. Chem. 295, 9901–9916. doi: 10.1074/jbc.RA120.012877
Yoon, K., Han, D., Li, Y., Sommerfeld, M., and Hu, Q. (2012). Phospholipid: diacylglycerol acyltransferase is a multifunctional enzyme involved in membrane lipid turnover and degradation while synthesizing triacylglycerol in the unicellular green microalga Chlamydomonas reinhardtii. Plant Cell 24, 3708–3724. doi: 10.1105/tpc.112.100701
Yuan, L., Mao, X., Zhao, K., Ji, X., Ji, C., Xue, J., et al. (2017). Characterisation of phospholipid: diacylglycerol acyltransferases (PDATs) from Camelina sativa and their roles in stress responses. Biol. Open 6, 1024–1034. doi: 10.1242/bio.026534
Keywords: Olea europaea, olive fruit, oil content, PDAT, gene expression, triacylglycerol synthesis, abiotic stresses
Citation: Hernández ML, Moretti S, Sicardo MD, García Ú, Pérez A, Sebastiani L and Martínez-Rivas JM (2021) Distinct Physiological Roles of Three Phospholipid:Diacylglycerol Acyltransferase Genes in Olive Fruit with Respect to Oil Accumulation and the Response to Abiotic Stress. Front. Plant Sci. 12:751959. doi: 10.3389/fpls.2021.751959
Received: 02 August 2021; Accepted: 21 October 2021;
Published: 12 November 2021.
Edited by:
Eddo Rugini, University of Tuscia, ItalyReviewed by:
Samanta Zelasco, Council for Agricultural and Economics Research (CREA), ItalyIvano Forgione, University of Tuscia, Italy
Copyright © 2021 Hernández, Moretti, Sicardo, García, Pérez, Sebastiani and Martínez-Rivas. This is an open-access article distributed under the terms of the Creative Commons Attribution License (CC BY). The use, distribution or reproduction in other forums is permitted, provided the original author(s) and the copyright owner(s) are credited and that the original publication in this journal is cited, in accordance with accepted academic practice. No use, distribution or reproduction is permitted which does not comply with these terms.
*Correspondence: José M. Martínez-Rivas, mrivas@ig.csic.es
†Present address: M. Luisa Hernández, Departamento de Bioquímica Vegetal y Biología Molecular/Instituto de Bioquímica Vegetal y Fotosíntesis, Universidad de Sevilla-CSIC, Seville, Spain; Samuele Moretti, Laboratoire Vigne Biotechnologies et Environnement (LVBE, UR-3991), Université de Haute Alsace, Colmar, France