- 1Institute of Food and Agricultural Technology-CIDSAV-XaRTA, University of Girona, Girona, Spain
- 2LIPPSO, Department of Chemistry, University of Girona, Girona, Spain
The hybrid peptide BP178 (KKLFKKILKYLAGPAGIGKFLHSAKKDEL-OH), derived from BP100 (KKLFKKILKYL) and magainin (1–10), and engineered for plant expression, had a strong bactericidal activity but not fungicidal. Moreover, the preventive spray of tomato plants with BP178 controlled infections by the plant pathogenic bacteria Pseudomonas syringae pv. tomato and Xanthomonas campestris pv. vesicatoria, as well as the fungus Botrytis cinerea. The treatment of tomato plants with BP178 induced the expression of several genes according to microarray and RT-qPCR analysis. Upregulated genes coded for several pathogenesis-related proteins, including PR1, PR2, PR3, PR4, PR5, PR6, PR7, PR9, PR10, and PR14, as well as transcription factors like ethylene transcription factors, WRKY, NAC and MYB, involved in the salicylic acid, jasmonic acid, and ethylene-signaling pathways. BP178 induced a similar gene expression pattern to flg15 according to RT-qPCR analysis, whereas the parent peptide BP100 did not trigger such as a strong plant defense response. It was concluded that BP178 was a bifunctional peptide protecting the plant against pathogen infection through a dual mechanism of action consisting of antimicrobial activity against bacterial pathogens and plant defense elicitation on plant host.
Introduction
Chemical control with conventional pesticides is an important part of the management of bacterial and fungal diseases of plant crops, but their extensive use has a negative environmental impact and often results in the emergence of resistance within the pathogen population (McManus et al., 2002; Brent and Hollomon, 2007; Sundin et al., 2016). Biological control appears to be an alternative or complement to the use of chemical pesticides, and several bacterial and fungal strains are commercialized as microbial biopesticides (Johnson and Temple, 2013; Montesinos and Bonaterra, 2017). Similarly, nonmicrobial biopesticides offer great possibilities for a sustainable disease management, and antimicrobial peptides (AMPs) have been proposed as novel pesticides to overcome problems due to fungal and bacterial plant pathogens (Montesinos et al., 2012; Zeitler et al., 2013; Datta et al., 2015; Badosa et al., 2017; Li et al., 2021). In addition, the conventional management of plant bacterial and fungal diseases has been based on targeting directly plant pathogens, but considerable efforts are oriented to identify compounds that activate the immune system of the plant (Tripathi and Dubey, 2004; Reignault and Walters, 2007; Thakur and Sohal, 2013; Abdul Malik et al., 2020). Thus, crop disease protection is currently oriented to a multitarget approach, consisting of pathogen inactivation and plant defense stimulation.
Plants have evolved several defense strategies to protect themselves from biotic and abiotic stresses (Montesano et al., 2003; Nejat and Mantri, 2017; Lamers et al., 2020). These responses include a set of induced mechanisms at the tissular level, like the rapid and localized cell death, termed hypersensitive response, and the production and accumulation of near 17 families of pathogenesis-related (PR) proteins (van Loon et al., 1994; Christensen et al., 2002; Jiang et al., 2015). PR expression is known to be regulated by defense or stress-signaling molecules and by abiotic agents (Jiang et al., 2015). In addition, plants have the ability to recognize microbe-associated molecular patterns (MAMPs) or pathogen-associated molecular patterns (PAMPs) that trigger a cascade of reactions conferring disease resistance (Albert, 2013; Beunouaret et al., 2014). Some examples of MAMPs/PAMPs include bacterial flagellin, peptidoglycans, lipopolysaccharides, cell wall glucans, fungal chitin, and sterols, among several compounds (Mishra et al., 2012; Gao et al., 2015). These MAMPs are recognized by pattern recognition receptors (PRRs) and elicit basal resistance referred to as PAMP/MAMP-triggered immunity (PTI-MTI) (Ausubel, 2005; Newman et al., 2013; Gao et al., 2015; Saijo et al., 2018). Apart from microbial elicitors, plants sense damage-associated molecular patterns (DAMPs), a plant-derived type of molecules like systemin (Boller and Felix, 2009; Albert, 2013). Besides the induction of locally restricted responses, plants have the ability to induce systemic defense responses, the so-called systemic acquired resistance (SAR) and induced systemic resistance (ISR), generally termed as induced resistance (IR). IR involves three main signaling transduction pathways, mediated by the phytohormones salicylic acid (SA), jasmonic acid (JA), and ethylene (Park et al., 2007; Rivas-San and Plasencia, 2011; Pieterse et al., 2014; Dhar et al., 2020). The application of chemical or biological elicitors to plants (e.g., harpins, acibenzolar-S-methyl, and fosetyl-Al) has been reported to protect plants from biotic stresses (Bektas and Eulgem, 2015; Badosa et al., 2017).
In the past years, there has been intensive research to identify plant defense elicitors from natural origin, and several functional peptides have been reported. This is the case of bacterial flagellin, which has been shown to act as a plant defense elicitor (Meindl et al., 2000), because the perception of bacterial flagellin by plant cells leads to the induction of defense-related genes followed by an oxidative burst, callose deposition, and ethylene production (Gómez-Gómez and Boller, 2002). Interestingly, analogs of flagellin (flg22 or flg15) and several natural or synthetic peptides were reported to trigger innate immunity in plants (Meindl et al., 2000; Brotman et al., 2009; Wei et al., 2017; Czékus et al., 2021). In this context, flg15 induced ROS production and the expression of several genes involved in salicylic acid, jasmonic acid, and ethylene-signaling pathways in tomato plants (Robatzek et al., 2007; Caravaca-Fuentes et al., 2021).
Our group has developed several families of peptides derived from natural compounds or de novo designed. Our goal was to find short sequences with high antimicrobial activity, low toxicity, and high stability to protease degradation (Montesinos et al., 2012). In particular, we designed and synthesized a library of linear undecapeptides (CECMEL11) (Ferré et al., 2006; Badosa et al., 2007), from which we identified sequences with an excellent biological activity profile that have been used successfully to control diseases caused by fungal and bacterial plant pathogens of economic importance (Badosa et al., 2007, 2009; Baró et al., 2020). Several peptide conjugates from members of the CECMEL11 library, like BP358 (containing flg15 and BP16), showed antimicrobial and plant defense elicitation activities in the Erwinia amylovora/pear pathosystem (Caravaca-Fuentes et al., 2021).
In addition, we designed a family of hybrid peptides to be produced in plant systems. Among them, BP178 (KKLFKKILKYL-AGPA-GIGKFLHSAK-KDEL-OH), incorporating BP100 (KKLFKKILKYL), magainin (1–10), an AGPA hinge for connecting both, and a KDEL endoplasmic reticulum retention signal, exhibited a strong bactericidal effect against several plant pathogenic bacteria and a very slight toxicity, but gave an HR-type reaction in tobacco leaves (Badosa et al., 2013). The peptide was expressed in the transgenic rice seed endosperm and protected seedlings from bacterial infection, but the protective effect was not completely explained by its antimicrobial properties (Montesinos et al., 2017).
In the present study, we planned to elucidate the mechanism of action of BP178 and whether it is able to trigger plant defense responses in tomato as a model plant. Specifically, the aim of this work was to determine if the topical application of the peptide to plants (1) protects against bacterial and fungal infection and (2) induces defense and stress-related gene expression. The effect of BP178 was compared to the plant defense elicitor peptide flg15, which has no antimicrobial activity, and to the parent bactericidal undecapeptide BP100 with bactericidal but no defense elicitor activity.
Materials and Methods
Bacterial and Fungal Strains and Growth Conditions
The bacterial pathogens Xanthomonas campestris pv. vesicatoria Xcv206 (Xcv) (D. F. Ritchie, Department of Plant Pathology, North Carolina State University) and Pseudomonas syringae pv. tomato DC3000 (Pto) (J. Murillo, Plant Pathology, Public University of Navarra, Spain), and the necrotrophic fungus Botrytis cinerea (Bc) (CECT 20518) were used. Bacterial strains were cultured in LB agar for 24 h at 28°C and scrapped from the surface to prepare suspensions adjusted to 108 CFU/ml. Bc was grown on potato dextrose agar (PDA) for 10 days at 23°C. Spores were collected by spreading sterile distilled water containing 0.01% (v/v) tween-20 onto the surface of the plate. The spore suspension was filtered through three layers of sterile cheesecloth and adjusted to 5 × 105 spores/ml.
Synthesis of Peptides
Peptides BP178 (KKLFKKILKYLAGPAGIGKFLHSAKKDEL-OH), flg15 (RINSAKDDAAGLQIA-OH), and BP100 (KKLFKKILKYL-NH2) were synthesized using the solid phase procedure as previously described (Badosa et al., 2007, 2013; Caravaca-Fuentes et al., 2021) (Supplementary Figure 1). An Fmoc-Rink-MBHA resin (0.55 mmol/g) was used for the synthesis of BP100, and a PAC-ChemMatrix resin (0.66 mmol/g) for the synthesis of flg15 and BP178. Once the peptidyl sequences were completed, the resulting resins were treated with trifluoroacetic acid (TFA)/H2O/triisopropylsilane (TIS) (95:2.5:2.5) for 2 h at room temperature. Following TFA evaporation and diethyl ether extraction, the crude peptides were dissolved in H2O, lyophilized, analyzed by HPLC, and characterized by mass spectrometry. BP178 tR = 6.50 min (90% purity); MS (MALDI-TOF) m/z: 3,242.7 [M + H]+. flg15 tR = 5.80 min (>99% purity); MS (ESI) m/z: 1,542.8 [M + H]+. BP100 tR = 5.02 min (>99% purity); MS (ESI) m/z: 1,421 [M + H]+. Lyophilized peptides (acetate salts) were solubilized in double-distilled water to a final concentration of 1 mM and filter sterilized through a 0.2 μm pore Whatman filter. Dilutions of the peptides were made in double-distilled water to obtain the desired final concentrations.
In vitro Antimicrobial Activity of Peptides
Antimicrobial activities were determined using a growth inhibition assay, as described previously (Badosa et al., 2007, 2009). Briefly, 20 μl of each peptide concentration were mixed in a microtiter plate with 20 μl of the suspension of the plant pathogenic bacteria (at final concentration of 107 CFU/ml) and added to 160 μl trypticase soy broth (TBS) (Biòmereux, France). For Bc, 80 μl spore suspension (104 conidia/ml) was mixed with 20 μl of each peptide dilution and 100 μl of double-concentrated PDB to a total volume of 200 μl PDB. Three replicates for peptide and concentration were used. Positive controls containing water instead of peptide and negative controls containing peptide without bacterial/fungal suspension were included. Microplates were incubated at 25°C for 48 h (Pto and Xcv) or 20°C for 6 days (Bc). Microbial growth was determined automatically every hour by optical density measurement at 600 nm (Bioscreen C; Labsystems, Helsinki, Finland) after shaking. The minimal inhibitory concentration (MIC) value was taken as the lowest peptide concentration with no growth at the end of the experiment.
In vitro Bactericidal and Fungicidal Activity of Peptides
Bactericidal activity of the antimicrobial peptides was determined by a contact test or killing assay, consisting of the exposure of the target microorganism to an antimicrobial compound for a given time and determining the surviving cells (Lambert, 2004). Twenty μl of the corresponding peptide concentration were mixed in a microtiter plate with 180 μl of bacterial or fungal suspension (at final concentration of 107 CFU/ml for bacteria and 104 CFU/ml for Bc) to a total volume of 200 μl. Three replicates for each concentration, peptide, and pathogen were used. Controls containing water instead of peptide or containing peptide without bacterial/fungal suspension were included. Microplates were incubated at 25°C (Pto and Xcv) or 20°C (Bc) for 1 h. Then, bactericidal activity was assessed through quantification of culturable cells by plate counting and the cell activity was determined using the resazurin method (alamarBlue® cell proliferation and viability reagent, Invitrogen, Thermo Fisher Scientific, Waltham, MA, USA). For bactericidal activity, aliquots of each peptide and concentration were taken and submitted to decimal dilutions, and 20 μl plated onto the surface of LB agar plates. Then, colony forming units (CFU) were quantified at 24–48 h after the incubation at 28°C. Fungicidal activity was determined similarly by spreading 100 μl onto the surface of PDA plates, and CFU were quantified after 7 days of incubation at 23°C. For cell viability measurements, 10 μl of alamarBlue® reagent were mixed with 90 μl of the corresponding microtiter cell suspension at the end of the experiment and transferred to a new microtiter. Incubation was performed for 4 h at 25°C in an automatic spectral scanning multimode reader (Varioskan, Ascent FL; Labsystems, Finland), and fluorescence emission measured at 590 nm as relative fluorescence units (RFUs) (excitation at 560 nm).
Effect of Peptide Treatment on Bacterial and Fungal Infections in Tomato Plants
The efficacy of peptides in controlling infections by the bacterial and fungal plant pathogens was evaluated in potted tomato plants under greenhouse conditions. Tomato plants cv. Rio Grande were grown in 500 ml plastic pots in the greenhouse and were fertilized one time every week with 200 ppm of water-soluble NPK (20:10:20). Disease was determined in leaves of plants that have been sprayed with aqueous solutions of BP178, flg15, or BP100 at 125 μM. Streptomycin (0.10 mg/ml) was used as a reference control product, and water-sprayed plants were used as non-treated controls. Treatments were applied 24 h before pathogen inoculation. Pathogens were applied by spraying the corresponding suspensions until drop-off, and plants were incubated in the controlled environment greenhouse at 23 ± 2°C and a photoperiod of 16 h of light and 8 h dark and 60% relative humidity. The experimental design consisted of three biological replicates of three plants per each treatment and pathogen. The experiment was conducted two times.
After incubation, disease symptoms were allowed to develop, and the intensity of the infections was scored 10 days after pathogen inoculation, using a severity index ranging from 0 to a maximum of 4 (0, no symptoms; 1, necrosis/lesions up to 25% of the leaf surface; 2: necrosis/lesions on 25-50% of the leaf surface; 3, severe necrosis/lesions on 50–75% of the leaf surface; and 4, severe necrosis/lesions on >75% of the leaf surface). In every plant, each of the seven leaves (each with 4–5 leaflet) was rated according to the index, and it was used to calculate a disease severity index per plant according to the formula:
where S is the severity of the infections per plant, Ii is the severity index for each leaf, n is the number of leaves measured, which is multiplied by the maximum severity index (i.e., 4). Then, the mean of the three plants for each biological replicate was used for the statistical analysis. Data set were subjected to analysis of variance (one-way ANOVA) to determine if there were significant differences between treatments in bacterial- and fungal-disease control. Efficacy of each treatment was calculated based on the severity of the treatment in relation to severity observed in the plants NTC group according to the formula:
Plant Materials, Treatments, and RNA Extraction for Gene Expression Analysis
Seeds of tomato plants cv. Rio Grande were sown in hydroponic seed plugs (rockwool), germinated and grown under controlled greenhouse conditions (25 ± 2°C, 16-h light/15 ± 2°C, 8-h dark, and 60% RH). Two-week-old seedlings (two cotyledons) were transplanted into Rockwool plugs (7.5 × 7.5 × 6.5 cm, Grodan Ibérica). The experimental design consisted of three biological replicates of 10 plants per replicate (30 plants per treatment) and treatments with BP178, BP100, flg15, and SA, JA, and ethylene that were included as positive controls of defense-signaling pathways.
After 2 weeks from transplanting, plants were sprayed with aqueous solutions of BP178, BP100 or flg15 at 125 μM, SA, and JA at 2.5 mM (Sigma-Aldrich, St. Louis, MO, USA) to the run-off point. For the ethylene treatment, plants were enclosed in a sealed chamber and exposed to ethylene obtained by reacting ethephon (1 mM) (Nufarm España, Spain) with a disodium hydrogen phosphate buffer (2.5 mM) (Zhang and Wen, 2010). The concentrations of the peptides BP100 and BP178 were chosen on the basis of the concentrations that were found effective against infections by plant pathogens observed in planta assays that were previously reported (Badosa et al., 2017; Caravaca-Fuentes et al., 2021). In the case of SA, JA, and ethylene, the concentrations were selected because they were used in other reports on topical application of defense elicitors in plants (Reignault and Walters, 2007; Rivas-San and Plasencia, 2011; Zhang et al., 2011).
Control plants were treated with distilled water. About 24 h after product application, leaf samples were collected, immediately frozen in liquid nitrogen, and stored at −80°C.
For total RNA extraction, the plant material was ground to a fine powder in liquid nitrogen with the Tissuelyzer II system (Qiagen, Hilden, Germany). Total RNA was extracted from leaves using TriZol® (Invitrogen, Life Technologies) according to the manual of the manufacturer. Following the extraction protocol, RNA samples were routinely subjected to DNAse treatment (Ambion® Turbo DNA-free™, Life Technologies, Thermo Fisher Scientific) to remove any contaminant DNA. In each step, RNA was quantified at 260 nm using a Nanodrop N-2000 spectrophotometer (Nanodrop Technologies LLC, Wilmington, DE, USA), and its integrity and quality verified by denaturing agarose gel electrophoresis and OD 260/280-nm absorption ratios, respectively. RNA samples of 10 plants were pooled in the same Eppendorf tube, and three biological replicates per treatment were analyzed (30 plants/treatment). This RNA was used as starting material to analyze the expression profiles of treated plants.
Microarray Analyses
The GeneChip™ Tomato Gene 1.0 ST Array (Affymetrix, Thermo Fisher Scientific) was used for comparing transcriptomes from plants treated with BP178 and flg15. In addition, plants treated with the reference products SA, JA, and ethylene, as well as non-treated control plants were included in the analyses. The tomato GeneChip contains 37,815 probe sets to analyze 715,135 transcripts (20–25 probes per gene). Three GeneChips were used to analyze three biological replicates per treatment (three replicates x 10 plants). About 1 μg of DNAse-treated RNA was sent to the Unit of Genomics at the Complutense University of Madrid for cDNA synthesis, labeling, hybridization to whole transcriptome array, washing, scanning, and data collection. High-quality RNA was subjected to the GeneChip® WT Plus Reagent Kit (Affymetrix) that is used to prepare RNA samples for whole transcriptome expression analysis. Briefly, the integrity of the RNA samples was tested in the Agilent Bioanalyser (Agilent Technologies Inc., Sta. Clara, CA, USA) and used to synthesize double-stranded cDNA. After in vitro transcription (IVT) reaction in the presence of biotinylated UTP and CTP, a biotin-labeled cRNA was generated from the double-stranded cDNA. The cRNA is cleaned and fragmented into sequence of about 100 nucleotides, labeled using TdT, and hybridized to the Tomato Gene 1.0 ST Arrays. Subsequently, chips were washed and fluorescence stained with phycoerythrin using the antibody amplification step described in the GeneChip™Fluidics Station 450 (Thermo Fisher Scientific), and fluorescence was quantified. After sample scanning, data were extracted, background-adjusted and normalized intensities of all probes were summarized into gene expression by the GeneChip Expression Console Software (Affymetrix, Thermo Fisher Scientific), using the Robust Multichip Average (RMA) algorithm (Irizarry et al., 2003). Preprocessed data were analyzed by the web-based Babelomics (Medina et al., 2010) for gene expression analysis as the ratio of normalized fluorescence value between two compared treatments. This ratio was then scaled using base 2 logarithm to obtain the log2 ratio, which, in absolute terms, is known as fold-change. Sequences showing expression changes higher than 2-fold change (fold change, FC), and with FDR-adjusted p value below 0.05, were considered to be differentially expressed.
Overexpressed genes were functionally annotated using the gene function analysis tools included in the PANTHER classification system (v. 14.0) and/or in the SOL Genomics Network.
Quantitative Real-Time PCR Analyses
To validate the expression patterns detected by microarray analyses, we analyzed a total of 14 Solanum lycopersicum genes encoding proteins involved in plant defense mechanisms (Table 1). These genes showed different fold change patterns, including upregulation and no significance changes after BP178 treatment. Oligonucleotide primers were designed according to the nucleotide sequence available at the Sol Genomics Network (ITAG release 2.40) using Primer Designing Tool included in the NCBI database. The reference gene actin was used as an internal control. Primers and the tomato genes implicated in plant defense response are listed in Supplementary Table 1.
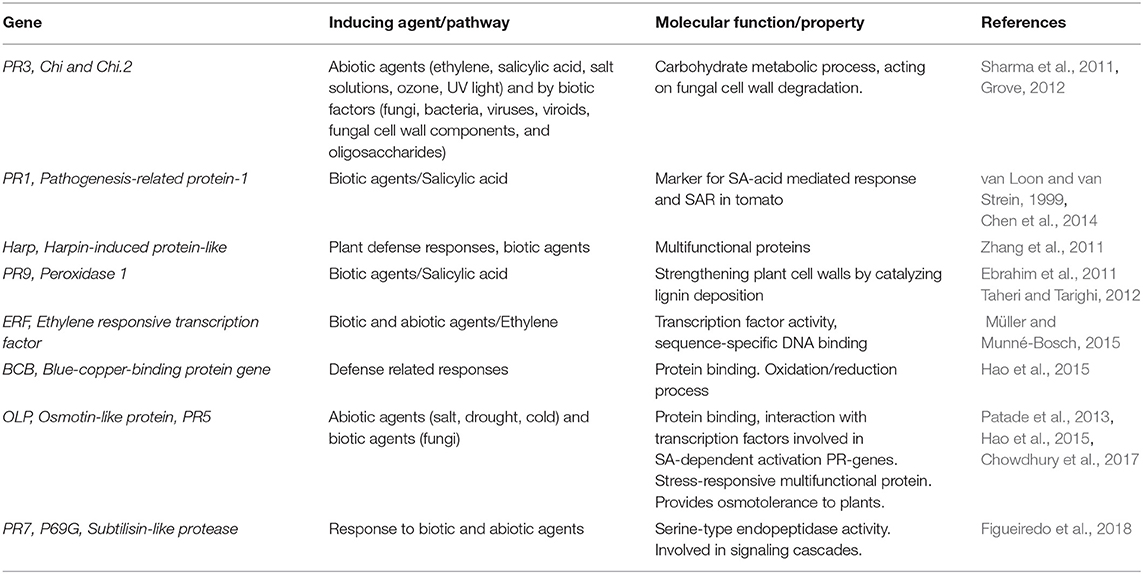
Table 1. Associated functions to overexpressed defense related genes, according to RT-qPCR, in tomato plants in response to BP178 treatment.
For each gene system, the concentration of the primer pair was optimized to prevent nonspecific reactions or artifacts that could hide the real result. Melting (dissociation) curve analysis was performed after each amplification to confirm the specificity of the amplified product/to prevent the detection of artifacts (as described in Badosa et al., 2017).
Gene expression analysis was performed by Quantitative Real-Time PCR (RT-qPCR). First-strand of complementary DNA (cDNA) was generated from leave RNA using reverse transcriptase (High Capacity cDNA Reverse Transcription Kit, Invitrogen) according to the manual of the manufacturer. This cDNA product was generated from each sample and was assayed for quantification of the expression levels of each of 25 tomato genes.
Quantitative Real Time-PCR was carried out in a fluorometric thermal cycler (7300 Real-Time PCR System, Applied Biosystems®, Waltham, MA, USA) using the Mix SYBR® Green PCR Master Mix (Applied Biosystems) as described in Badosa et al., 2017. The total reaction volume was 20 μl containing 1x Sybr Green Master Mix (Applied Biosystems), the optimized concentration of primers (final concentration of 300 mM for LePPO-f/LePPO-r, LeGLUA-f/LeGLUA-r, and LeAct-f/LeAct-r primer pair; 100 mM for the rest of primers used in this study) and 2 μL of RT reaction (cDNA). qPCR conditions were as follows: (1) an initial denaturation step (10 min at 95°C); (2) amplification and quantification (50 cycles of 15 s at 95°C and 1 min at 60°C); and a melting curve program (60-95°C with a heating rate of 0.5°C/s) as described in Badosa et al. (2017). Reactions were carried out in duplicate in 96-well plates. Controls from no cDNA template were included as negative controls. The relative quantification of each individual gene expression was performed using the 2−ΔΔCt method (Livak and Schmittgen, 2001). Relative expression values of each plant defense were calculated normalizing against the tomato actin gene as an internal control. Statistical significance was determined using the REST2009 Software (Pfaffl et al., 2002).
Results
Antimicrobial Activity
Antibacterial and antifungal activity of BP178, flg15, and BP100 are shown in Table 2. BP178 and BP100 exhibited strong activity against Pto and Xcv. Specifically, BP178 showed a minimal inhibitory concentration (MIC) < 1 μM against Xcv and between 1 and 10 μM against Pto. The parent peptide BP100 showed MIC values, ranging from 1 to 10 μM against both bacterial pathogens. In contrast, the antifungal activity of BP178 and BP100 against Bc was very low, with MIC values ranging between 25 and 100 μM. Peptide flg15 was neither antibacterial nor antifungal at the maximum dose tested (100 μM).
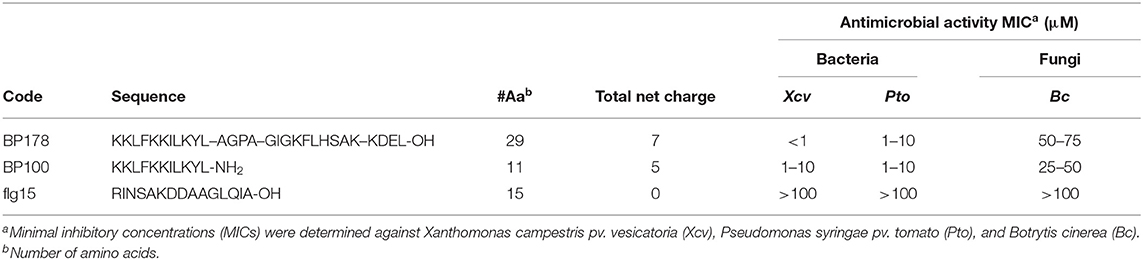
Table 2. Sequence, number of amino acids, charge, and antimicrobial activity of the peptides used in this study.
The bactericidal and fungicidal activities as determined by the contact and resazurin tests (cell survival and cell viability, respectively) are shown in Figure 1. BP178 led to a decrease in the survival of Xcv and Pto of 2.29 log reduction (N0/N) at 0.5 μM, which increased to 5.5 at 1.6 μM. For BP100, a maximum Pto and Xcv survival reduction of 5.4 and 5.7 log was observed after incubation at 3.2 and 12.5 μM, respectively. BP178 and BP100 practically showed a very slight fungicidal activity against Bc. As expected, flg15 did not reduce bacterial or fungal survival. The resazurin test confirmed the findings on cells survival, because survival was inversely related to resazurin cell viability (y = – 0.2401x + 2.4557, R2 0.892) (Supplementary Figure 2).
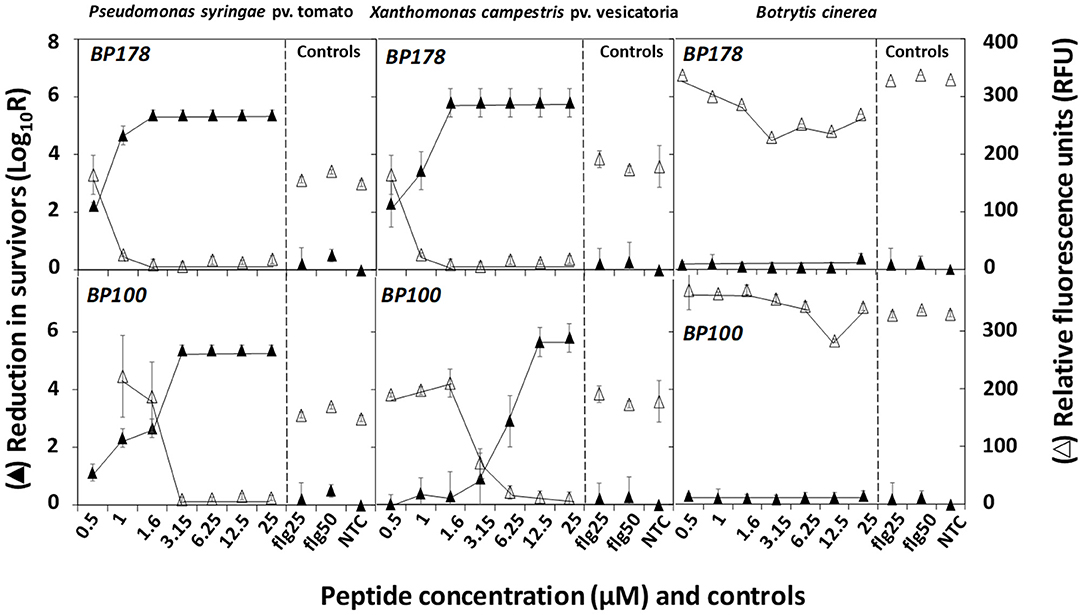
Figure 1. Effect of peptides BP178 and BP100 in cell survival (black triangles) and resazurin cell viability (white triangles) of Pseudomonas syringae pv. tomato, Xanthomonas campestris pv. vesicatoria, and Botrytis cinerea after exposure to the peptides for 60 min. Controls of flg15 at 25 (flg25) or 50 μM (flg50) and non-treated (NTC) were included. Values are the means of three replicates, and error bars represent standard deviation of the mean.
Effect of Peptides Treatment of Tomato Plants on Bacterial and Fungal Infections
The results of the effect of treatments were consistent but slightly different between the two experiments performed. The preventive spray of peptide BP178 on tomato plants inhibited infections caused by Xcv, Pto, and Bc (Figure 2). More in detail, after treatment, disease severity in bacterial speck (Pto) was 21.3 and 27.9% for the two experiments performed (52.1 and 64.9% efficacy), and, in bacterial spot (Xcv), it was of 14.2 and 15.5 (around 70% efficacy), compared with non-treated controls (58.2% in experiment 1 and 60.8% in Pto in experiment 2, and 47.5% in experiment 1 and 51.9% in the second experiment in Xcv). The effect of BP100 and flg15 was similar to BP178 against Pto and Xcv infections.
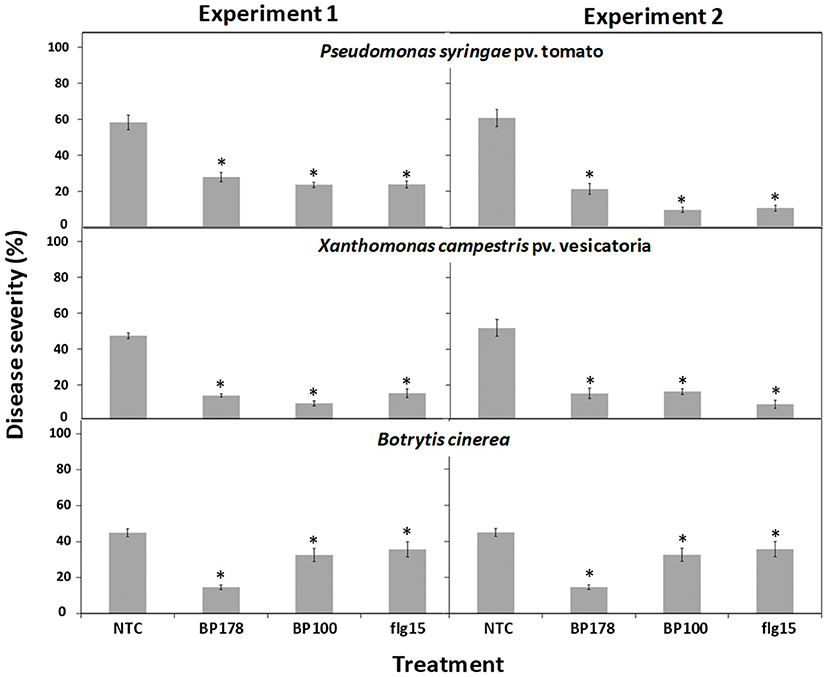
Figure 2. Protection of tomato plants against bacterial and fungal infection after topical treatment with BP178 in comparison with the parent peptide BP100 and flg15. Two independent assays were performed, and peptides were applied at 125 μM by spraying plants 24 h before pathogen inoculation. Disease severity was evaluated on tomato plants 10 days after pathogen inoculation (107 ufc/ml for bacterial pathogens; 2.5 × 10 5 conidia/ml for B. cinerea). Values correspond to the mean disease severity of three replicates of three plants per each treatment. Standard errors are indicated on bars. The asterisk denotes statistically significant differences with non-treated control plants (NTC) (Tukey's test, p > 0.05).
In the case of Bc, disease severity due to the BP178 treatment was 14.6 and 29.4% for the two experiments (67.4 and 38% efficacy), compared to non-treated controls (47.5% in experiment 1 and 44.9% in experiment 2). Interestingly, there was a slight effect, but significant, of BP100 and flg15. This result greatly contrasts with the slight antifungal activity of BP178, BP100, and flg15 in vitro.
Effect of Peptide Treatments on the Expression of Defense-Related Genes in Tomato
Microarray Analysis
The analysis revealed that of the 37,815 genes in the tomato microarray, the treatments modified the expression of several genes, following different patterns. According to the criteria for upregulation (fold change (FC ≥ 2) and downregulation (FC ≤ 0.5), the expression was modified in: 112 genes in BP178 (100 upregulated, 12 downregulated), 191 genes in flg15 (160 upregulated, 31 downregulated), 2,974 genes in SA (1,534 upregulated, 1,440 downregulated), 2,236 genes in JA (1,122 upregulated, 1,114 downregulated) and 1,280 in ethylene (826 upregulated, 454 downregulated). A detailed list of the differentially expressed genes for BP178, flg15, SA, JA, and ethylene treatments is given in Supplementary Table 2.
After the BP178 treatment, a total of 100 genes were upregulated (more than 2-fold) in comparison to the non-treated control. A set of 90 genes was functionally annotated, while the remaining 10 transcripts had unknown function or had no available hit. From the annotated genes, 74.4% of transcripts were identified as defense-related genes (67 out of 90 mapped ID), sharing homology with transcription factors (WRKY, MYB, and NAC), signal transduction genes (ethylene responsive transcription factor (ERF), serine/threonine protein-kinase), hormone-related genes, lipoxygenases, harpins, acetyltransferases, cytochrome P450, and several well-known pathogeneses-related genes (Table 3). PR-genes overexpressed after BP178 treatment, coded for antifungal/antimicrobial proteins (PR1), β-1,3-glucanases (PR2), chitinases (PR3, PR4), thaumatin-like proteins (PR5), endopeptidases inhibitor (PR6), subtilisin-like proteins (PR7), peroxidases (PR9), ribonuclease-like proteins (PR10), and lipid-transfer protein (PR14). The number of highly overexpressed genes (FC > 4) was 22, where the maximum FC values were reported in lipoxygenases (FC 14.01), endochitinases (FC 7.36), and lipid-transfer proteins (FC 7.18).
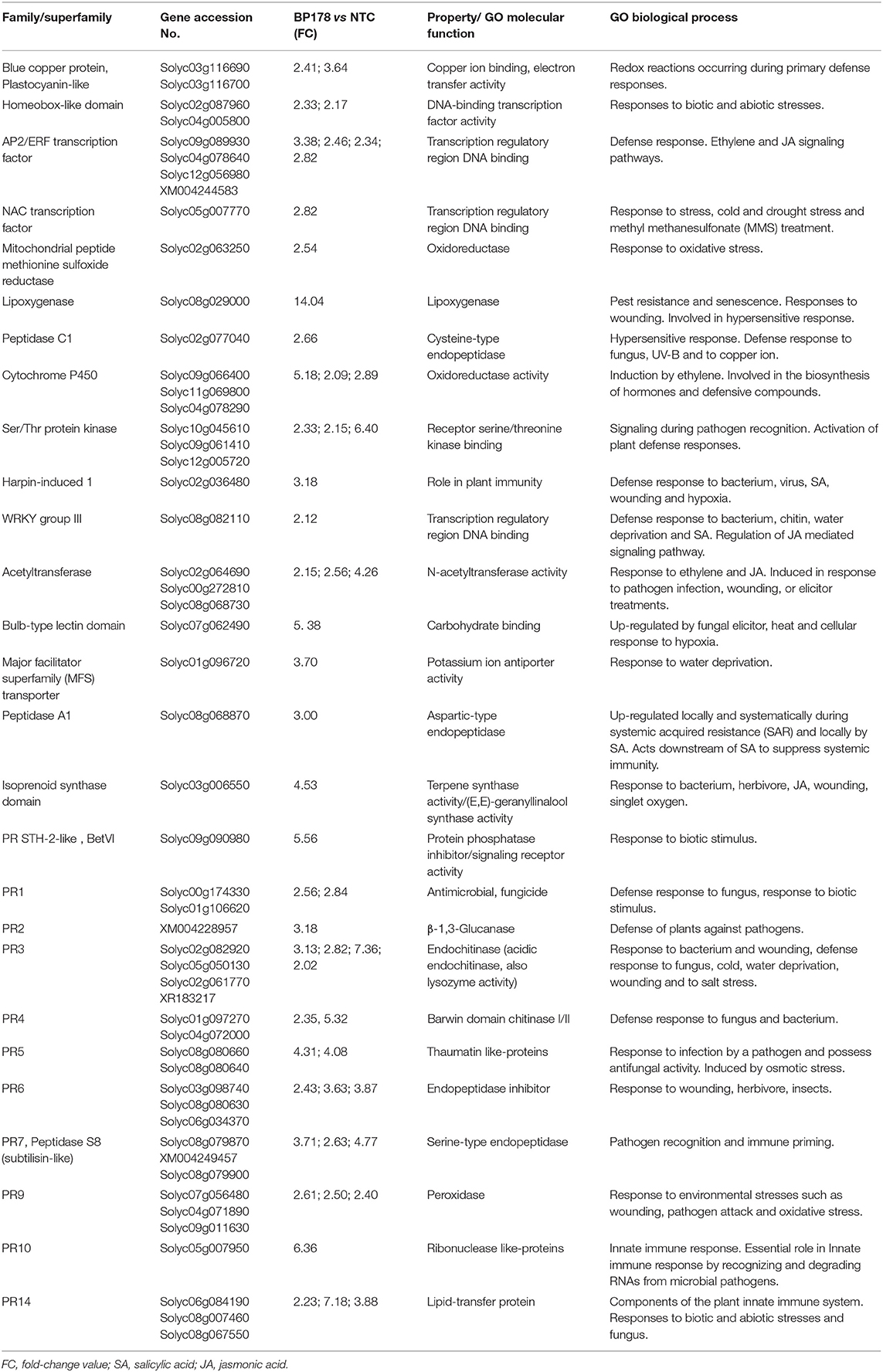
Table 3. Relevant upregulated (2-fold or higher; FDR< 0.05) transcripts after BP178 treatment (125 μM), identified in this study, associated with plant-defense response (GO term GO:0006952).
A Venn diagram (Bardou et al., 2014), to overlap differentially overexpressed genes after the treatments and to compare gene expression between response to BP178 and the other treatments, is shown in Figure 3. Among the BP178-upregulated genes, five genes were also induced after flg15, SA, JA, and ethylene treatment. Specifically, these transcripts corresponded to chitinase (PR4; FC 5.32), endochitinase (PR3; FC 3.16), a glycoprotein involved in signaling mechanisms (FC 5.38), acetyltransferase (FC 4.26), and hydrolase (FC 3.39). Except the hydrolase, all the other genes code for proteins directly involved in plant-defense responses. Ten genes were transcriptionally induced exclusively by the BP178 treatment, and seven of them can be mapped and identified as pathogenesis-related protein-1, glycosidase, a member of ABC transporter family, ser/thr protein kinase, cold shock protein (chaperone), pre-mRNA-splicing factor CLF1, and CXE carboxylesterase.
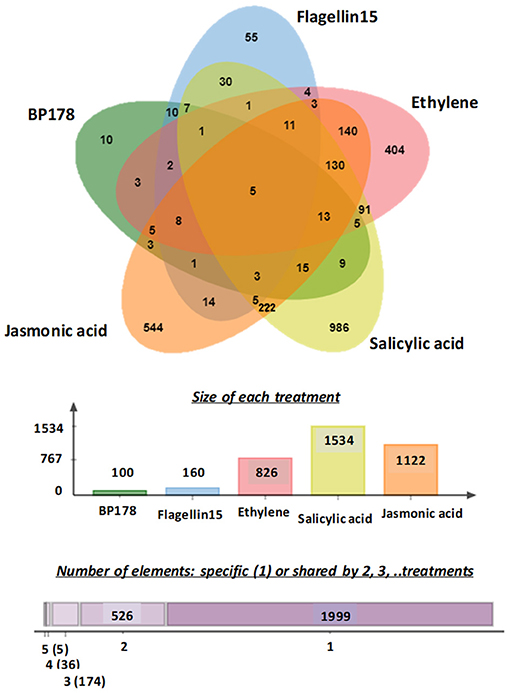
Figure 3. A Venn diagram of overexpressed genes in tomato plants after BP178, flagelin15, ethylene, salicylic acid, and jasmonic acid treatment. Overlapping regions of the circles indicate genes that are overexpressed in more than one treatment. Genes with fold-change above two were included in the analysis. The numbers in the graphic indicate the total numbers of overexpressed genes in each treatment. In the second chart, 1,999 overexpressed genes are specific of one list; 526 overexpressed genes are shared by two lists. Numbers in brackets represent the number of overexpressed genes shared by three, four, and five lists.
In addition, the Venn diagram revealed the commonly overexpressed transcripts in the five datasets (treatments). Within the 90 overexpressed and mapped genes after BP178 treatment, 37 were also overexpressed by flg15, 42 by ethylene, 58 by SA, and 53 by JA treatments (Figure 3).
The raw data of the microarray study are deposited in the National Center for Biotechnology Information (NCBI) repository, as metadata (experimental procedures for the transcriptomics analysis and experiment design) and the matrix data results for the different treatments. The code number at GEO webpage for the accession is GSE183707.
Quantitative Real-Time PCR Analyses
RT-qPCR was performed with 14 selected defense genes in order to validate the gene expression profile revealed by microarrays analysis in response to BP178 treatment. These candidate genes were chosen among genes showing significant induction profiles in the previous microarray analysis of Solanum lycopersicum, which encode proteins involved in plant-defense mechanisms (Supplementary Table 1) or with no significant changes in expression after the treatments.
A significant correlation was observed between the RT-qPCR and microarray data (Chi-square Pearson correlation coefficient of 0.789, p < 0.001, n = 70) (Supplementary Figure 3). Specifically, BP178 treatment induced overexpression of harpin, PR9, PR3, ERF, PR2, BCB, PR5, and PR7, similarly to the flg15 treatment that, apart from these genes, also overexpressed a polyphenol oxidase and the transcription factor WRKY3 (Figure 4). Contrarily, the treatment with the bactericidal peptide BP100 caused a slight overexpression of only one out of 14 genes (e.g., polyphenol oxidase).
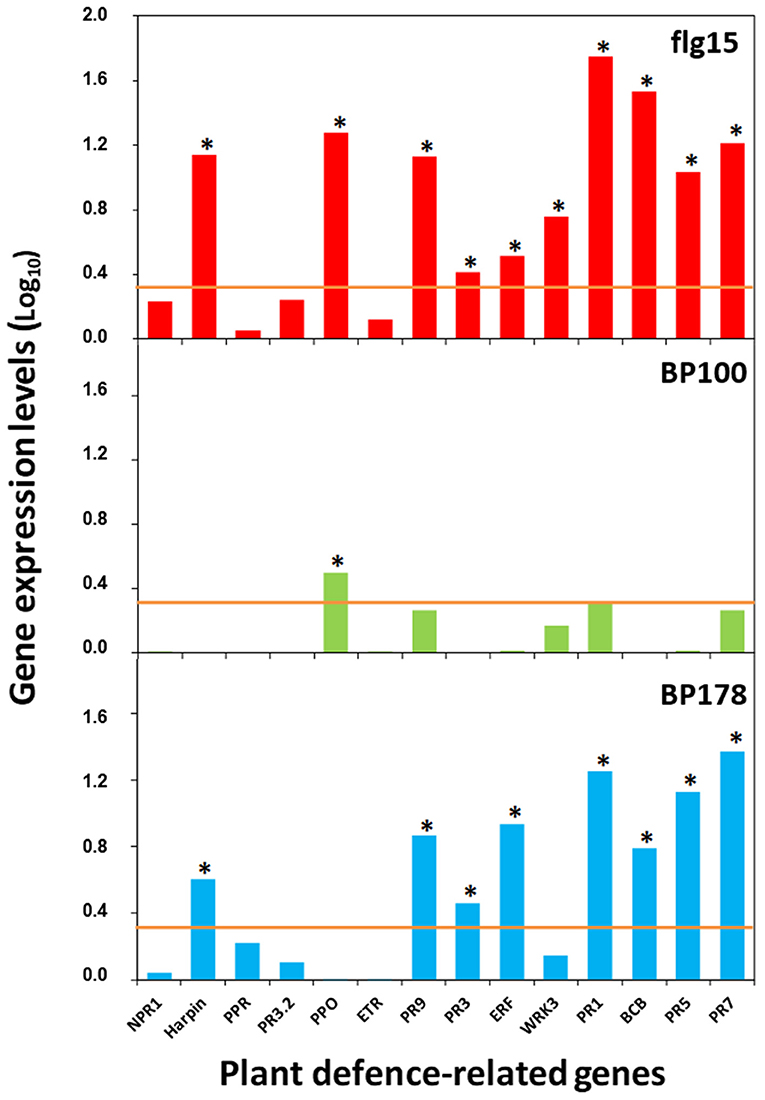
Figure 4. Relative expression levels (log10) of selected tomato plant-defense genes verified by qPCR analysis after treatment with the peptides. Orange line, cut-off values for gene induction are considered fold changes above 2 (log10, 0.3) (relative quantification using the ΔΔCt method). Asterisk, significant values of fold change. Gene expression data for BP100 and flg15 in the case of PR1 gene have been previously published (Badosa et al., 2017).
Discussion
Biostimulant application in agriculture represents a powerful strategy to improve both plant yield and tolerance to abiotic and biotic stresses (Rouphael and Colla, 2020). These products interact with plant-signaling cascades that triggered the expression of stress-responsive genes. Rapid responses to plant pathogens could trigger systemic signaling pathways and lead to plant resistance against pathogen attack (Moore et al., 2011; Wu et al., 2014). In the present study, we investigated the antimicrobial activity of peptide BP178 (Badosa et al., 2013; Montesinos et al., 2017) and its potential use as biostimulant to improve resistance to biotic and abiotic stresses in tomato, one of the major crops cultivated worldwide. In addition, the activity of BP178 was compared to the antibacterial peptide BP100 that does not have plant defense elicitation activity and to the plant-defense elicitor peptide flg15.
BP178 showed potent bactericidal activity against Xanthomonas campestris pv. vesicatoria and Pseudomonas syringae pv. tomato. In addition, we have shown here that BP178 applied by spraying to tomato plants was effective against infection by Pto, Xcv, and also Bc. These results agree with previous reports, indicating the effect against other plant pathogenic bacteria like X. arboricola pv. pruni, Erwinia amylovora, and Xylella fastidiosa (Badosa et al., 2013; Baró et al., 2020). However, the control of Bc infections in tomato was not expected due to the low in vitro antifungal activity exhibited by BP178. Therefore, we hypothesized a possible role of BP178 as a plant-defense elicitor. This possibility was previously pointed out because tobacco leaf infiltration with BP178 showed an HR-type response in tobacco plants, similarly to other hybrid peptides, incorporating BP100 (Badosa et al., 2013).
The treatment of tomato plants with BP178 and the subsequent analysis of microarray data revealed that 100 genes showed differential expression, compared to the non-treated control. Ninety of these genes were functionally annotated, and 74.4% were identified as defense-related genes. Furthermore, when the gene expression profile of tomato plants challenged with BP178 was compared to that of SA, JA, ethylene, and flg15 profile, several upregulated genes were found to be shared with these pathways. Flg15, as has been previously reported in pear plants (Badosa et al., 2017), triggered plant-defense responses, but has no antibacterial activity, whereas, contrarily, BP100 was strongly antibacterial, but had no significant gene induction activity according to the genes that were analyzed by RT-qPCR. Unfortunately, in the present work, the gene expression analysis of BP100 treatment was not included in the microarray, because we had previous evidence by RT-qPCR (Badosa et al., 2017; Oliveras et al., 2018) that, among 16 genes studied, only PinII and PPO were slightly overexpressed. Then, we cannot exclude that BP100 would induce the expression of genes other than the ones tested by RT-qPCR.
The present results are also in agreement with other reports involving flagellin (Zipfel et al., 2004; Pastor-Fernández et al., 2020). In addition, and as expected, we have found that tomato plants sprayed with SA, JA, or ethylene increased expression of a wide range of defense-related genes, but 10 genes were unique to BP178 challenged plants. Seven of these genes were mapped and identified as pathogenesis related protein-1, glycosidase, a member of the ABC transporter family, ser/thr protein kinase, cold shock protein, pre-mRNA-splicing factor CLF1, and CXE carboxylesterase.
Several pathways seem to be involved in BP178-triggered plant immunity, although pathways related to biotic stress were predominant. For instance, we found upregulation of genes coding for pathogenesis-related proteins like PR1, PR2, PR3, PR4, PR5, PR6, PR7, PR9, PR10, and PR14. This finding can be related to the decrease in severity of bacterial and fungal infections in tomato plants treated with BP178. The overexpression of PR genes was also reported as the reason to enhanced resistance in a variety of plants (i.e., potato, rice, grapevine, and tobacco) against a wide range of pathogens (Ali et al., 2018). Interestingly, it has been reported that the SA mediated activation, triggered after biotrophic/hemibiotrophic and necrothrophic pathogen attack, leads to expression of PR1, PR2, and PR5 genes (Ali et al., 2018). In fact, the increased expression of PR1 and PR2 genes has been used as a molecular marker of the SAR pathway (Ceasar and Ignacimuthu, 2012), and the expression of PR3, PR4, and PR12 genes is considered a signature of the JA pathway (Ali et al., 2018). Although both pathways follow different signaling systems, they can interact (Narváez et al., 2020), as we observed in BP178-challenged tomato plants.
The overexpression of the antifungal proteins PR2, PR3, PR4, and PR5 by BP178 treatment is particularly relevant since the plants are able to control infections caused by Bc, although this peptide has no significant in vitro antifungal activity. Interestingly, upregulation of PR3 and PR4 genes (chitinases) was reported in a Fusarium-resistant banana cultivar (Niu et al., 2018). Besides playing a key role against fungal pathogens, PR3 and PR4 also increase by other biotic factors, such as bacteria, viruses, viroids, or insects, and abiotic stresses, including osmotic, salt, cold, or wounding stresses, and salicylic acid and ethylene (Sharma et al., 2011; Grove, 2012). As mentioned above, the treatment with BP178 resulted also in the induction of PR2, PR3, and PR5 genes involved in the ethylene-signaling pathway, in agreement with several studies reporting that ethylene perception and signaling are key factors in plant resistance to fungal and bacterial pathogens in many horticultural crops (Ravanbakhsh et al., 2018).
The pathogenesis-related gene Osmotin/OLP (coding a osmotin PR5 family) was highly induced in tomato plants in response to BP178 treatment. Osmotin overproduction has an effect against infection by several fungal plant pathogens, such as Bc (Monteiro et al., 2003), Fusarium solani, and Colletotrichum gloeosporoides (de Freitas et al., 2011), in agreement with our results of Bc infection control in tomato plants. In addition, it has been reported that the osmotin accumulated in plant cells in response to biotic or abiotic stresses (Chowdhury et al., 2017) provided osmotolerance, as well as induced cryoprotective functions (Barthakur et al., 2001; Goel et al., 2010). Moreover, the overexpression of the osmotin gene in transgenic plants results in enhanced tolerance to abiotic stresses, such as cold, salt, and drought (Patade et al., 2013).
Various PR7 genes (subtilisin-like proteases, subtilases) were also overexpressed by the treatment of tomato plants with BP178. It is known that several PR7 proteins are specifically activated under different situations like after pathogen infection (Figueiredo et al., 2014) in tomato plants infected with citrus exocortis viroid (Granell et al., 1987), infection by Pseudomonas syringae or Phytpohtora infestans, and by SA treatment (Tornero et al., 1996; Jordá et al., 1999; Tian et al., 2005). In addition, subtilases are linked to immune priming in plants, and the DAMP systemin has been identified as one of the substrates of a subtilase (Schaller and Ryan, 1994, Kavroulakis et al., 2006). PR7s are also reported to be involved in abiotic stresses, such as drought and salt resistance mechanisms (Figueiredo et al., 2018).
Furthermore, plants challenged to BP178 overexpressed genes-coding PR10 proteins (ribonuclease-like proteins), which are known to confer activity against Pseudomonas syringae and Agrobacterium tumefaciens, among several pathogens (Ali et al., 2018). This finding is in agreement with the control of infections by Pto in tomato plants treated with BP178. Similarly, PR14 genes that were overexpressed in BP178 plants code for lipid-transfer proteins that exhibit both antibacterial and antifungal activities (Patkar and Chattoo, 2006).
In addition to the expression of several pathogenesis-related genes, BP178 induced several transcription factors, including ERF, WRKY, NAC and MYB, and enzymes implicated in cell wall and oxidative stress. ERFs are induced by SA, JA, and ethylene by integrating transcription factors and signaling pathways (Zheng et al., 2019). Our transcriptomic analysis with the microarray confirmed the overexpression of four ERF genes, and the RT-qPCR confirmed that BP178 almost triples the elicitor effect produced by flg15 on the ERF gene. ERFs are key regulators, integrating ethylene, abscisic acid, jasmonate, and the redox-signaling pathway in plant-defense response against abiotic stresses (Mizoi et al., 2012; Müller and Munné-Bosch, 2015). Moreover, BP178 challenged in tomato induced genes implicated in the synthesis of cytochrome P450, which is involved in plant steroid hormone biosynthesis (Farmer and Goossens, 2019).
Finally, the present study provides evidence that BP178 is a bifunctional peptide with bactericidal and defense-elicitor properties, protecting tomato from bacterial and fungal infections. This protection is partially due to the priming effect, similarly to flg15 that is conferred through very complex signaling pathways like the SA, JA, and ethylene. Interestingly, BP178 (C-terminal end) and flg15 (in the middle moiety) present a similar amino acid sequence [flg15: SAK-DDA (4-9 aa); BP178: SAKKDEL (23-29 aa)].
The singular properties of BP178, its biological performance, and the possibility to be produced using plants as biofactories (Montesinos et al., 2017), and, eventually, microorganisms, open great expectations for its future exploitation as a biopesticide for plant disease protection.
Data Availability Statement
The datasets presented in this study can be found in online repositories. The names of the repository/repositories and accession number(s) can be found below: www.ncbi.nlm.nih.gov/, GSE183707.
Author Contributions
EM, EB, MP, and LF obtained the financial support. LM, BG, LR, EB, and EM designed the research, analyzed the data, and wrote the paper. MP and LF provided the AMPs. LM, BG, and LR conducted and performed the experiments. All authors read, reviewed, and approved the final manuscript.
Funding
This work was supported by the Spanish Ministerio de Economia y Competitividad (MINECO) Grant Nos. AGL2012-39880-C02-02 and AGL2015-69876-C2-1-R.
Conflict of Interest
The authors declare that the research was conducted in the absence of any commercial or financial relationships that could be construed as a potential conflict of interest.
Publisher's Note
All claims expressed in this article are solely those of the authors and do not necessarily represent those of their affiliated organizations, or those of the publisher, the editors and the reviewers. Any product that may be evaluated in this article, or claim that may be made by its manufacturer, is not guaranteed or endorsed by the publisher.
Acknowledgments
We thank the Government of the Generalitat de Catalunya (Xarxa de Referencia en Biotecnología, and Xarxa de Referència en Tecnologia dels Aliments, SGR09626, 2008SGR812, and 2014SGR697) for support. The research group is accredited by SGR 2014-697 and TECNIO net from Catalonia. We also thank the University of Girona for a grant for research improvement 2015–2018.
Supplementary Material
The Supplementary Material for this article can be found online at: https://www.frontiersin.org/articles/10.3389/fpls.2021.756357/full#supplementary-material
References
Abdul Malik, N. A., Kumar, I. S., and Nadarajah, K. (2020). Elicitor and receptor molecules: orchestrators of plant defense and immunity. Int. J. Mol. Sci. 21:963. doi: 10.3390/ijms21030963
Albert, M. (2013). Peptides as triggers of plant defense. J Exp Bot. 64, 5269–5279. doi: 10.1093/jxb/ert275
Ali, S., Ganai, B. A., Kamili, A. N., Bhat, A. A., Mir, Z. A., Bhat, et al. (2018). Pathogenesis-related proteins and peptides as promising tools for engineering plants with multiple stress tolerance. Microbiol Res. 212–13, 29–37. doi: 10.1016/j.micres.2018.04.008
Ausubel, F. (2005). Are innate immune signalling pathways in plants and animals conserved? Nat. Immunol. 6, 973–979. doi: 10.1038/ni1253
Badosa, E., Ferré, R., Francés, J., Bardají, E., Feliu, L., Planas, M., et al. (2009). Sporicidal activity of synthetic antifungal undecapeptides and control of Penicillium rot of apples. Appl. Environ. Microbiol. 75, 5563–5569. doi: 10.1128/AEM.00711-09
Badosa, E., Ferré, R., Planas, M., Feliu, L., Besalú, E., Cabrefiga, J., et al. (2007). A library of linear undecapeptides with bactericidal activity against phytopathogenic bacteria. Peptides 28, 2276–2285. doi: 10.1016/j.peptides.2007.09.010
Badosa, E., Moiset, G., Montesinos, L., Talleda, M., Bardají, E., Feliu, L., et al. (2013). Derivatives of the antimicrobial peptide BP100 for expression in plant systems. PloS One 8, e85515. doi: 10.1371/journal.pone.0085515
Badosa, E., Montesinos, L., Cam,ó, C., Ruz, L., Cabrefiga, J., Francés, J., et al. (2017). Control of fire blight infections with synthetic peptides that elicit plant defense responses. J. Plant Pathol. 99, 65–73. doi: 10.4454/jpp.v99i0.3915
Bardou, P., Mariette, J., Escudié, F., Djemiel, C., and Klopp, C. (2014). Jvenn: an interactive Venn diagram viewer. BMC Bioinformatics. 15:293. doi: 10.1186/1471-2105-15-293
Baró, A., Badosa, E., Montesinos, L., Feliu, L., Planas, M., Montesinos, E., et al. (2020). Screening and identification of BP100 peptide conjugates active against Xylella fastidiosa using a viability-qPCR method. BMC Microbiol. 20:229. doi: 10.1186/s12866-020-01915-3
Barthakur, S., Babu, V., and Bansal, K. C. (2001). Over-expression of osmotin induce proline accumulation and confers tolerance to osmotic stress in transgenic tobacco. J Plant. Biochem. Biotech. 10, 31–37. doi: 10.1007/BF03263103
Bektas, Y., and Eulgem, T. (2015). Synthetic plant defense elicitors. Front. PlantSci. 5:804. doi: 10.3389/fpls.2014.00804
Beunouaret, R., Goujon, E., and Goupil, P. (2014). Grape marc extract causes early perception events, defense reactions and hypersensitive response in cultured tobacco cells. Plant Physiol. Biochem. 77, 84–89. doi: 10.1016/j.plaphy.2014.01.021
Boller, T., and Felix, G. (2009). A renaissance of elicitors: perception of microbe-associated molecular patterns and danger signals y pattern-recognition receptors. Annu. Rev. Plant Biol. 60, 379–406. doi: 10.1146/annurev.arplant.57.032905.105346
Brent, K. J., and Hollomon, D. W. (2007). Fungicide resistance in crop pathogens: How can it be managed. Brussels: CropLife International.
Brotman, Y., Makovitzki, A., Shai, Y., Chet, I., and Viterbo, A. (2009). Synthetic ultrashort cationic lipopeptides induce systemic plant defense responses against bacterial and fungal pathogens. Appl. Environ. Microbiol. 75, 5373–5379. doi: 10.1128/AEM.00724-09
Caravaca-Fuentes, P., Camó, C., Oliveras, À, Baró, A., Francés, J., Badosa, E., et al. (2021). A bifunctional peptide conjugate that controls infections of erwinia amylovora in pear plants. Molecules 26:3426. doi: 10.3390/molecules26113426
Ceasar, S. A., and Ignacimuthu, S. (2012). Genetic engineering of crop plants for fungal resistance: role of antifungal genes. Biotechnol. Lett. 34, 995–1002. doi: 10.1007/s10529-012-0871-1
Chen, Y. L., Lee, C. Y., Cheng, K. T., Chang, W. H., Huang, R. N., Nam, H. G., et al. (2014). Quantitative peptidomics study reveals that a wound-induced peptide from PR-1 regulates immune signaling in tomato. Plant Cell. 26, 4135–4148. doi: 10.1105/tpc.114.131185
Chowdhury, S., Basu, A., and Kundu, S. (2017). Overexpression of a new osmotin-like protein gene (sindolp) confers tolerance against biotic and abiotic stresses in sesame. Front. Plant Sci. 8:410. doi: 10.3389/fpls.2017.00410
Christensen, A. B., Cho, B. H., Næsby, M., Gregersen, P. L., Brandt, J., Madriz-Ordeñana, K., et al. (2002). The molecular characterization of two barley proteins establishes the novel PR-17 family of pathogenesis-related proteins. Mol. Plant Pathol. 3, 135–144. doi: 10.1046/j.1364-3703.2002.00105.x
Czékus, Z., Kukri, A., Hamow, K. Á., Szalai, G., Tari, I., Ördög, A., et al. (2021). Activation of local and systemic defense responses by Flg22 is dependent on daytime and ethylene in intact tomato plants. Int. J. Mol. Sci. 22:8354. doi: 10.3390/ijms22158354
Datta, A., Ghosh, A., Airoldi, C., Sperandeom, P., Mroue, K., Jiménez-Barbero, J., et al. (2015). Antimicrobial peptides: insights into membrane permeabilization, lipopolysaccharide fragmentation and application in plant disease control. Sci Rep. 5:11951. doi: 10.1038/srep11951
de Freitas, C. D., Nogueira, F. C., Vasconcelos, I. M., Oliveira, J. T., Domont, G. B., and Ramos, M. V. (2011). Osmotin purified from the latex of Calotropis procera: biochemical characterization, biological activity and role in plant defense. Plant Physiol. Biochem. 49, 738–743. doi: 10.1016/j.plaphy.2011.01.027
Dhar, N., Chen, J. Y., Subbarao, K. V., and Klosterman, S. J. (2020). Hormone signaling and its interplay with development and defense responses in verticillium-plant interactions. Front. Plant Sci. 11:584997. doi: 10.3389/fpls.2020.584997
Ebrahim, S., Usha, K., and Singh, B. (2011). “Pathogenesis Related (PR) Proteins in Plant Defense Mechanism”, in Science against microbial pathogens: communicating current research and technological advances, ed. Méndez-Vilas, A., (Microbiology Series)1043–1054.
Farmer, E. E., and Goossens, A. (2019). Jasmonates: what allene oxide synthase does for plants. J. Exp. Bot. 70, 3373–3378. doi: 10.1093/jxb/erz254
Ferré, R., Badosa, E., Feliu, L., Planas, M., Montesinos, E., and Bardají, E. (2006). Inhibition of plant-pathogenic bacteria by short synthetic cecropin A-melittin hybrid peptides. Appl. Environ. Microbiol. 72, 3302–3308. doi: 10.1128/AEM.72.5.3302-3308.2006
Figueiredo, A., Monteiro, F., and Sebastiana, M. (2014). Subtilisin-like proteases in plant–pathogen recognition and immune priming: a perspective. Front. Plant. Sci. 5:739. doi: 10.3389/fpls.2014.00739
Figueiredo, J., Sousa Silva, M., and Figueiredo, A. (2018). Subtilisin-like proteases in plant defense: the past, the present and beyond. Mol. Plant Pathol. 19, 1017–1028. doi: 10.1111/mpp.12567
Gao, Q.-M., Zhu, S., Kachroo, P., and Kachroo, A. (2015). Signal regulators of systemic acquired resistance. Front. Plant Sci. 6:228. doi: 10.3389/fpls.2015.00228
Goel, D., Singh, A. K., Yadav, V., Babbar, S. B., and Bansal, K. C. (2010). Overexpression of osmotin gene confers tolerance to salt and drought stresses in transgenic tomato (Solanum lycopersicum L.). Protoplasma 245, 133–141. doi: 10.1007/s00709-010-0158-0
Gómez-Gómez, L., and Boller, T. (2002). Flagellin perception: a paradigm for innate immunity. Trends Plant Sci. 7, 251–256. doi: 10.1016/S1360-1385(02)02261-6
Granell, A., Belles, J. M., and Conejero, V. (1987). Induction of pathogenesis-related proteins in tomato by citrus exocortis viroid, silver ion and ethephon. Physiol. Mol. Plant Pathol. 31, 83–90. doi: 10.1016/0885-5765(87)90008-7
Grove, A. (2012). Plant Chitinases: Genetic Diversity and Physiological Roles. CRC Crit. Rev. Plant Sci. 31, 57–73. doi: 10.1080/07352689.2011.616043
Hao, J., Wu, W., Wang, Y., Yang, Z., Liu, Y., Lv, Y., et al. (2015). Arabidopsis thaliana defense response to the ochratoxin A-producing strain (Aspergillus ochraceus 3.4412). Plant Cell Rep. 34, 705–719. doi: 10.1007/s00299-014-1731-3
Irizarry, R. A., Hobbs, B., Collin, F., Beazer-Barclay, Y. D., Antonellis, K. J, Scherf, U., et al. (2003). Exploration, normalization, and summaries of high density oligonucleotide array probe level data. Biostatistics. 4, 249–264. doi: 10.1093/biostatistics/4.2.249
Jiang, L., Wu, J., Fan, S., Li, W., Dong, L., Cheng, Q., et al. (2015). Isolation and characterization of a novel pathogenesis-related protein gene (GmPRP) with induced expression in soybean (Glycine max) during Infection with Phytophthora sojae. PLoS ONE. 10, e0129932. doi: 10.1371/journal.pone.0129932
Johnson, K. B., and Temple, T. N. (2013). Evaluation of strategies for fire blight control in organic pome fruit without antibiotics. Plant Disease 97, 402–409. doi: 10.1094/PDIS-07-12-0638-RE
Jordá, L., Coego, A., Conejero, V., and Vera, P. (1999). A genomic cluster containing four differentially regulated subtilisin-like processing protease genes is in tomato plants. J. Biol. Chem. 274, 2360–2365. doi: 10.1074/jbc.274.4.2360
Kavroulakis, N., Papadopoulou, K. K., Ntougias, S., Zervakis, G. I., and Ehaliotis, C. (2006). Cytological and other aspects of pathogenesis-related gene expression in tomato plants grown on a suppressive compost. Ann. Bot. 98, 555–564. doi: 10.1093/aob/mcl149
Lambert, R. J. W. (2004). “Evaluation of antimicrobial efficacy”, in Russell, Hugo & Ayliffe's principles and practice of disinfection, preservation and sterilization. Fraise AP, Lambert PA, Maillard JY, editors. (Oxford: Blackwell Publishing Ltd) 345–360. doi: 10.1002/9780470755884.ch11
Lamers, J, van der Meer, T., and Testerink, C. (2020). How plants sense and respond to stressful environments. Plant Physiol. 182, 1624–1635. doi: 10.1104/pp.19.01464
Li, J., Hu, S., Jian, W., Xie, C., and Yang, X. (2021). Plant antimicrobial peptides: structures, functions, and applications. Bot. Stud. 62:5. doi: 10.1186/s40529-021-00312-x
Livak, K. J., and Schmittgen, T. D. (2001). Analysis of relative gene expression data using real-time quantitative PCR and the 2[-Delta Delta C(T)] method. Methods 25, 402–408. doi: 10.1006/meth.2001.1262
McManus, P. S., Stockwell, V. O., Sundin, G. W., and Jones, A. L. (2002). Antibiotic use in plant agriculture. Annu. Rev. Phytopathol. 40, 443–465. doi: 10.1146/annurev.phyto.40.120301.093927
Medina, I., Carbonell, J., Pulido, L., Madeira, S. C., Goetz, S., Conesa, A., et al. (2010). Babelomics: an integrative platform for the analysis of transcriptomics, proteomics and genomic data with advanced functional profiling. Nucleic Acids Res. 38:W210–W213. doi: 10.1093/nar/gkq388
Meindl, T., Boller, T., and Felix, G. (2000). The bacterial elicitor flagellin activates its receptor in tomato cells according to the address-message concept. Plant Cell. 12, 1783–1794. doi: 10.1105/tpc.12.9.1783
Mishra, A. K., Sharma, K., and Misra, R. S. (2012). Elicitor recognition, signal transduction and induced resistance in plants. J. Plant Interact. 7, 95-120. doi: 10.1080/17429145.2011.597517
Mizoi, J., Shinozaki, K., and Yamaguchi-Shinozaki, K. (2012). AP2/ERF family transcription factors in plant abiotic stress responses. Biochim. Biophys. Acta. 1819, 86–96 doi: 10.1016/j.bbagrm.2011.08.004
Monteiro, S., Barakat, M., Piçarra-Pereira, M. A., Texeira, A. R., and Ferreira, R. B. (2003). Osmotin and thaumatin from grape: a putative general defense mechanism against pathogenic fungi. Phytopathology 93, 1505–1512. doi: 10.1094/PHYTO.2003.93.12.1505
Montesano, M., Brader, G., and Palva, E. T. (2003). Pathogen-derived elicitors: searching for receptor in plants. Mol. Plant Pathol. 4, 73–78. doi: 10.1046/j.1364-3703.2003.00150.x
Montesinos, E., Badosa, E., Cabrefiga, J., Planas, M., Feliu, L., and Bardají, E. (2012). “Antimicrobial peptides for plant disease control. From discovery to application”. In: Rajasekaran, K., Cary, J., Jaynes, J., and Montesinos, E, editors. Small Wonders: Peptides for Disease Control. Oxford: Blackwell's. 235–261. doi: 10.1021/bk-2012-1095.ch012
Montesinos, E., and Bonaterra, A. (2017). Pesticides, Microbial. In: Schaechter, M, editors. Encyclopedia of Microbiology. Elsevier. 110–120. doi: 10.1016/B978-012373944-5.00125-5
Montesinos, L., Bundó, M., Badosa, E., San Segundo, B., Coca, M., and Montesinos, E. (2017). Production of BP178, a derivative of the synthetic antibacterial peptide BP100, in the rice seed endosperm. BMC Plant Biol. 17:63. doi: 10.1186/s12870-017-1011-9
Moore, J. W., Loake, G. J., and Spoel, S. H. (2011). Transcription Dynamics in Plant Immunity. The Plant Cell. 23, 2809–2820. doi: 10.1105/tpc.111.087346
Müller, M., and Munné-Bosch, S. (2015). Ethylene response factors: A key regulatory hub in hormone and stress signalling. Plant Physiol. 169, 32–41. doi: 10.1104/pp.15.00677
Narváez, I., Pliego Prieto, C., Palomo-Ríos, E., Fresta, L., Jiménez-Díaz, R. M., Trapero-Casas, J. L., et al. (2020). Heterologous Expression of the AtNPR1 Gene in Olive and Its Effects on Fungal Tolerance. Front. Plant Sci. 11:308. doi: 10.3389/fpls.2020.00308
Nejat, N., and Mantri, N. (2017). Plant immune system: crosstalk between responses to biotic and abiotic stresses the missing link in understanding plant defense. Curr. Issues Mol. Biol. 23, 1–16. doi: 10.21775/cimb.023.001
Newman, M.-A., Sundelin, T., Nielsen, J. T., and Erbs, G. (2013). MAMP (microbe-associated molecular pattern) triggered immunity in plants. Front. Plant. Sci. 4:139. doi: 10.3389/fpls.2013.00139
Niu, Y., Hu, B., Li, X., Chen, H., Takáč, T., S¨amaj, J., et al. (2018). Comparative digital gene expression analysis of tissue-cultured plantlets of highly resistant and susceptible banana cultivars in response to fusarium oxysporum. Int. J. Mol. Sci. 19:350. doi: 10.3390/ijms19020350
Oliveras, A., Baro, A., Montesinos, L., Badosa, E., Montesinos, E., Feliu, L., et al. (2018). Antimicrobial activity of linear lipopeptides derived from BP100 towards plant pathogens. PLoS One. doi: 10.1371/journal.pone.0201571
Park, S. W., Kaimoyo, E., Kumar, D., Mosher, S., and Klessig, D. F. (2007). Methyl salicylate is a critical mobile signal for plant systemic acquired resistance. Science. 318, 113–116. doi: 10.1126/science.1147113
Pastor-Fernández, J., Gamir, J., Pastor, V., Sanchez-Bel, P., Sanmartín, N., Cerezo, M., et al. (2020). Arabidopsis plants sense non-self peptides to promote resistance against Plectosphaerella cucumerina. Front. Plant Sci. 11:529. doi: 10.3389/fpls.2020.00529
Patade, V. Y., Khatri, D., Kumari, M., Grover, A., Mohan Gupta, S., and Ahmed, Z. (2013). Cold tolerance in Osmotin transgenic tomato (Solanum lycopersicum L.) is associated with modulation in transcript abundance of stress responsive genes. Springerplus. 2:117. doi: 10.1186/2193-1801-2-117
Patkar, R. N., and Chattoo, B. B. (2006). Transgenic indica rice expressing ns-LTP-like protein shows enhanced resistance to both fungal and bacterial pathogens. Mol. Breed. 17, 159-171. doi: 10.1007/s11032-005-4736-3
Pfaffl, M. W., Horgan, G. W., and Dempfle, L. (2002). Relative expression software tool (REST©) for group-wise comparison and statistical analysis of relative expression results in Real-Time PCR. Nucleic Acid Res. 30:e36. doi: 10.1093/nar/30.9.e36
Pieterse, C. M., Zamioudis, C., Berendsen, R. L., Weller, D. M., Van Wees, S. C., and Bakker, P. A. (2014). Induced systemic resistance by beneficial microbes. Annu. Rev. Phytopathol. 52, 347–375. doi: 10.1146/annurev-phyto-082712-102340
Ravanbakhsh, M., Sasidharan, R., Voesenek, L. A. C. J., Kowalchuk, G. A., and Jousset, A. (2018). Microbial modulation of plant ethylene signalling: ecological and evolutionary consequences. Microbiome. 6:52. doi: 10.1186/s40168-018-0436-1
Reignault, P., and Walters, D. (2007). “Topical application of inducers for disease control”. In: Walters, D., Newton, A., Lyon, G, editors. Induced Resistance for Plant Defense: A Sustainable Approach to Crop Protection. Ames, IA: Blackwell Publishing. 179–200. doi: 10.1002/9780470995983.ch10
Rivas-San, V. M., and Plasencia, J. (2011). Salicylic acid beyond defense: its role in plant growth and development. J. Exp. Bot. 62, 3321–3338. doi: 10.1093/jxb/err031
Robatzek, S., Bittel, P., Chinchilla, D., Köchner, P., Felix, G., Shiu, S. H., et al. (2007). Molecular identification and characterization of the tomato flagellin receptor LeFLS2, an orthologue of Arabidopsis FLS2 exhibiting characteristically different perception specificities. Plant Mol. Biol. 64, 539–547. doi: 10.1007/s11103-007-9173-8
Rouphael, Y., and Colla, G. (2020). Editorial: biostimulants in agriculture. Front. Plant Sci. 11:40. doi: 10.3389/fpls.2020.00040
Saijo, Y., Loo, E. P., and Yasuda, S. (2018). Pattern recognition receptors and signaling in plant-microbe interactions. Plant J. 93, 592–613. doi: 10.1111/tpj.13808
Schaller, A., and Ryan, C. A. (1994). Identification of a 50-kDa system-inbinding protein in tomato plasma membranes having Kex2p-like properties. Proc. Natl. Acad. Sci. 9, 11802–11806. doi: 10.1073/pnas.91.25.11802
Sharma, N., Sharma, K. P., Gaur, R. K., and Gupta, V. K. (2011). Role of chitinase in plant defense. Asian J. Biochem. 6, 29–37. doi: 10.3923/ajb.2011.29.37
Sundin, G. W., Castiblanco, L. F., Yuan, X., Zeng, Q., and Yang, C.-H. (2016). Bacterial disease management: challenges, experience, innovation and future prospects. Mol. Plant Pathol. 17, 1506–1518. doi: 10.1111/mpp.12436
Taheri, P., and Tarighi, S. (2012). The role of pathogenesis-related proteins in the tomato-Rhizoctonia solani interaction. J. Bot. doi: 10.1155/2012/137037
Thakur, M., and Sohal, S. S. (2013). Role of elicitors in inducing resistance in plants against pathogen infection. A review. ISRN Biochem. 2013:762412. doi: 10.1155/2013/762412
Tian, M., Benedetti, B., and Kamoun, S. (2005). A Second Kazal-like protease inhibitor from Phytophthora infestans inhibits and interacts with the apoplastic pathogenesis-related protease P69B of tomato. Plant Physiol. 138, 1785–1793. doi: 10.1104/pp.105.061226
Tornero, P., Conejero, V., and Vera, P. (1996). Primary structure and expression of a pathogen-induced protease (PR-P69) in tomato plants: Similarity of functional domains to subtilisin-like endoproteases. Proc. Nat. Acad. Sci. 93, 6332–6337. doi: 10.1073/pnas.93.13.6332
Tripathi, P., and Dubey, N. K. (2004). Exploitation of natural products as alternative strategy to control post-harvest fungal rotting of fruits and vegetables. Postharvest Biol. Technol. 32, 235–245. doi: 10.1016/j.postharvbio.2003.11.005
van Loon, L. C., Pierpoint, W. S., Boller, T., and Conejero, V. (1994). Recommendations for naming plant pathogenesis-related proteins. Plant Mol. Biol. Rep. 12, 245–264. doi: 10.1007/BF02668748
van Loon, L. C., and van Strein, E. A. (1999). The families of pathogenesis-related proteins, their activities, and comparative analysis of PR-1 type proteins. Physiol. Mol. Plant Pathol. 55, 85–97. doi: 10.1006/pmpp.1999.0213
Wei, Y., Caceres-Moreno, C., Jimenez-Gongora, T., Wang, K., Sang, Y., Lozano-Duran, R., et al. (2017). The Ralstonia solanacearum csp22 peptide, but not flagellin-derived peptides, is perceived by plants from the Solanaceae family. Plant Biotechnol. J. 16, 1349-1362. doi: 10.1111/pbi.12874
Wu, S., Shan, L., and He, P. (2014). Microbial signature-triggered plant defense responses and early signaling mechanisms. Plant Science. 228:118.126. doi: 10.1016/j.plantsci.2014.03.001
Zeitler, B., Herrera Diaz, A., Dangel, A., Thellmann, M., Meyer, H., Sattler, M., et al. (2013). De-novo design of antimicrobial peptides for plant protection. PloS ONE. 8:e71687. doi: 10.1371/journal.pone.0071687
Zhang, L., Xiao, S., Li, W., Feng, W., Li, J., Wu, Z., et al. (2011). Overexpression of a Harpin-encoding gene hrf1 in rice enhances drought tolerance. J. Exp. Bot. 62, 4229–4238. doi: 10.1093/jxb/err131
Zhang, W., and Wen, C. (2010). Preparation of ethylene gas and comparison of ethylene responses induced by ethylene, ACC, and ethephon. Plant Physiol. Biochem. 48:45–53. doi: 10.1016/j.plaphy.2009.10.002
Zheng, X., Xing, J., Zhang, K., Pang, X., Zhao, Y., Wang, G., et al. (2019). Ethylene Response Factor ERF11 Activates BT4 Transcription to Regulate Immunity to Pseudomonas syringae. Plant Physiol. 180, 1132–1151. doi: 10.1104/pp.18.01209
Keywords: bifunctional peptide, antimicrobial, plant defense elicitor, plant disease, tomato
Citation: Montesinos L, Gascón B, Ruz L, Badosa E, Planas M, Feliu L and Montesinos E (2021) A Bifunctional Synthetic Peptide With Antimicrobial and Plant Elicitation Properties That Protect Tomato Plants From Bacterial and Fungal Infections. Front. Plant Sci. 12:756357. doi: 10.3389/fpls.2021.756357
Received: 10 August 2021; Accepted: 13 September 2021;
Published: 18 October 2021.
Edited by:
Youfu “Frank” Zhao, University of Illinois at Urbana-Champaign, United StatesReviewed by:
Tiyakhon Chatnaparat, Kasetsart University, ThailandWei Wei, University of Illinois at Urbana-Champaign, United States
Copyright © 2021 Montesinos, Gascón, Ruz, Badosa, Planas, Feliu and Montesinos. This is an open-access article distributed under the terms of the Creative Commons Attribution License (CC BY). The use, distribution or reproduction in other forums is permitted, provided the original author(s) and the copyright owner(s) are credited and that the original publication in this journal is cited, in accordance with accepted academic practice. No use, distribution or reproduction is permitted which does not comply with these terms.
*Correspondence: Emilio Montesinos, emilio.montesinos@udg.edu