- Division of Molecular Biology, Department of Biology, Faculty of Science, University of Zagreb, Zagreb, Croatia
During plant embryogenesis, regardless of whether it begins with a fertilized egg cell (zygotic embryogenesis) or an induced somatic cell (somatic embryogenesis), significant epigenetic reprogramming occurs with the purpose of parental or vegetative transcript silencing and establishment of a next-generation epigenetic patterning. To ensure genome stability of a developing embryo, large-scale transposon silencing occurs by an RNA-directed DNA methylation (RdDM) pathway, which introduces methylation patterns de novo and as such potentially serves as a global mechanism of transcription control during developmental transitions. RdDM is controlled by a two-armed mechanism based around the activity of two RNA polymerases. While PolIV produces siRNAs accompanied by protein complexes comprising the methylation machinery, PolV produces lncRNA which guides the methylation machinery toward specific genomic locations. Recently, RdDM has been proposed as a dominant methylation mechanism during gamete formation and early embryo development in Arabidopsis thaliana, overshadowing all other methylation mechanisms. Here, we bring an overview of current knowledge about different roles of DNA methylation with emphasis on RdDM during plant zygotic and somatic embryogenesis. Based on published chromatin immunoprecipitation data on PolV binding sites within the A. thaliana genome, we uncover groups of auxin metabolism, reproductive development and embryogenesis-related genes, and discuss possible roles of RdDM at the onset of early embryonic development via targeted methylation at sites involved in different embryogenesis-related developmental mechanisms.
Introduction
In vascular plants, embryogenesis begins by establishing cell embryogenic competence, which is followed by formation of distinct embryonic stages. Besides the dominant form of embryogenesis which involves a fertilized egg cell or a zygote (zygotic embryogenesis, ZE), flowering plants have evolved alternative fertilization-independent mechanisms of embryo formation (classified under the umbrella term asexual embryogenesis; AE). The general characteristic of AE mechanisms is the variability of cells that can develop competency for embryogenesis. Common forms of AE that occur in vivo are parthenogenesis, where a reduced egg cell develops the embryo, gametic embryogenesis, where an unreduced egg cell or sperm cell develops the embryo and adventitious embryony, where embryo is formed from cells of nucellus or integument (Hand et al., 2016). The rarest naturally occurring AE process is somatic embryogenesis (SE), characterized by the possibility of embryo formation from virtually any somatic cell. This process is independent not only of fertilization but also of existence of gametes, gametophyte, ovules or reproductive tissues, and is the strongest evidence of plant cell totipotency. It is thought that a plant cell in any developmental stage or form has the potential to, under suitable environmental conditions, initiate regulatory mechanisms which will lead to cell dedifferentiation to a state of competency followed by re-differentiation and consequently embryonic development (Fehér, 2005).
Although ZE and SE differ in the initiation stage of embryogenesis, evidence shows overall similarity between the two processes on the level of both morphology and genetics. For instance, a somatic cell undergoing embryogenesis mimics the zygotic pattern of cell division – in other words, just like its zygotic counterpart, it divides asymmetrically (Dodeman et al., 1997; Vasilenko et al., 2000) and forms a suspensor-like structure and a somatic embryo (Leljak-Levanić et al., 2015). Furthermore, similar to zygotic embryogenesis which is marked by existence of embryo and non-embryonic endosperm, different cell types were found in SE cultures, such as embryonic and non-embryonic cell clusters identified in maize microspore cultures (Massonneau et al., 2005). Analyses of cellular types and secreted molecules of in vitro cultures suggest endosperm-like functions of these non-embryonic cell clusters, which are thought to communicate with embryonic cells via signaling molecules to direct embryo development, much like the mutually dependent development of embryo and endosperm within the female gametophyte (reviewed in Matthys-Rochon, 2005). In Arabidopsis and other dicots, cultured somatic embryos go through all the major stages of development described for zygotic embryos, namely the globular, heart, torpedo and cotyledonary stage (Kurczyńska et al., 2007). Additionally, similar sets of transcription factors are active during SE and ZE, indicating similar transcriptional regulatory mechanisms between the two processes (Gliwicka et al., 2013; Jin et al., 2014; Leljak-Levanić et al., 2015). With this in mind, a recent RNAseq study of an Arabidopsis embryonic culture reveals surprising results – remarkably, the SE transcriptome has more similarities with transcriptome of germinating seeds than early zygotic embryos (Hofmann et al., 2019). Contrary to previous indications, this finding suggests there might be no general regulatory mechanisms mediating ZE and SE, but does not exclude a subset of specific mechanisms common for both ZE and SE. Identification of these specific yet common mechanisms presents both a challenge and an opportunity for implementing novel approaches to DNA methylation research. Comparative analysis of ZE and SE transcriptome during the initiation stage still holds potential for identification of a specific set of common regulators and regulatory mechanisms between the two types of embryogenesis. If we consider the vast array of possibilities that might lead to SE (different cell types, different environmental conditions etc.), it seems even more likely that some of the mechanisms and molecules involved in SE will overlap with initiation of ZE.
Embryogenesis implies a state of intensive developmental transitions. The role of epigenetic mechanisms during the initiation and maturation stages of embryogenesis was shown in analyses of mostly SE in species such as barley, soybean, common bean, cotton, Norway spruce (for a review, see Nic-Can and De la Peña, 2014), Arabidopsis (Grzybkowska et al., 2018), carrot (LoSchiavo et al., 1989; Yamamoto et al., 2005), pumpkin (Leljak-Levanić et al., 2004) and others. In Arabidopsis, DNA methylation mechanisms have been shown to underlie both ZE (Xiao et al., 2006; Pillot et al., 2010; Ingouff et al., 2017; Forgione et al., 2019) and SE (Grzybkowska et al., 2018; Osorio-Montalvo et al., 2018). Here, we review recent findings on DNA methylation during plant ZE and SE, and propose a central role of RdDM in gene expression regulation during these processes. Assuming that RdDM activity is determined by PolV targeting, we analyze recently published chromatin-immunoprecipitation data based on the Arabidopsis genome (Liu et al., 2018) and list genes related to reproductive development, embryogenesis and auxin dynamics as possible targets of RdDM.
Epigenetic Reprogramming and DNA Methylation in Early Plant Development
DNA methylation is an epigenetic mechanism commonly found in mammals, plants, filamentous fungi, fish and insect species, among others (Martienssen and Colot, 2001; Li, 2002; Chan et al., 2005; Zhong, 2016; Bewick et al., 2017; Anastasiadi et al., 2018). While many aspects of DNA methylation show striking levels of evolutionary conservation, different organisms have also evolved unique mechanisms. For instance, despite a high structural similarity between mammal and plant methyltransferases, the exact mechanisms by which they establish DNA methylation and the regulatory factors they associate with during this process are often different (Zhong, 2016). In contrast to mammals that primarily methylate CG dinucleotides, plants methylate their DNA in all sequence contexts: symmetric CG, CHG, and asymmetric CHH (H = A, C, or T) by different classes of DNA methyltransferases (Elhamamsy, 2016).
Pioneer work in the field has associated DNA methylation with a range of cellular functions, including transposable element silencing, maintenance of genome integrity, genomic imprinting and X-chromosome inactivation (for a review, see Zhang et al., 2018). In recent years, the focus of attention has become the elucidation of DNA methylation mechanisms in regulation of gene expression, which has also been implicated during plant growth and development (Finnegan et al., 1996; Jacobsen et al., 2000; Xiao et al., 2006; Bartels et al., 2018; Zhang et al., 2018).
In plants, global methylation levels are dynamic and variable throughout development. On the one hand, DNA methylation can be conservatively inherited through cell divisions, ensuring epigenetic memory of their cellular predecessors and can be heritable across generations (Schmitz et al., 2013; Iwasaki and Paszkowski, 2014). On the other hand, differences in methylation profiles can be found even between cells of the same origin separated by only a few divisions, such as different cells of a plant gametophyte, as shown for Arabidopsis and rice (Ibarra et al., 2012; Park et al., 2016; Han et al., 2019; Borg et al., 2021). Perhaps the most dramatic feature of epigenetics is ‘epigenetic reprogramming,’ a term used to describe a process in which epigenetic marks of a previous developmental stage or cellular form are erased and a novel epigenetic pattern is established de novo. Plants are remarkable in this aspect because they seem to possess a dual ability to both stably inherit epialleles across generations, and to undergo significant epigenetic reprogramming during male and female gametophyte development and embryogenesis (reviewed in Kawashima and Berger, 2014; Gehring, 2019). In recent years, several papers demonstrated the occurrence of epigenetic reprogramming during developmental transitions in different species of the plant kingdom. In the liverwort Marchantia polymorpha, a species with a dominant gametophyte generation, epigenetic reprogramming occurs at least twice, once in the gametophytic and once in the sporophytic generation (Schmid et al., 2018). Because the morphology and transcriptional profiles of flowering plants markedly shift between the haploid gametophyte and diploid sporophyte, it is safe to assume that epigenetic reprogramming occurs here as well, once at the diploid-to-haploid transition and a second time during haploid-to-diplod transition. In Arabidopsis, the loss of histone H3 methylation (H3K9me2) and DNA demethylation of transposon-associated cis-regulatory elements guides the diploid-to-haploid transition, which later in the vegetative nucleus of pollen grain unlocks genes involved in sperm cell transport and delivery. Conversely, the loss of another methylation mark (H3K27me3) underlies the haploid-to-diploid transition in sperm cells, unlocking the set of developmental genes required to initiate development of the new generation upon fertilization (Borg et al., 2020, 2021). Similar epigenetic reprogramming might regulate egg and central cell fates and transitions between haploid and diploid generations in the female gametophyte. Furthermore, it seems plausible that embryonic epigenetic reprogramming is involved in control of post-embryogenic development, as specifically shown for a seed-specific transcription factor in Arabidopsis (Tao et al., 2017), and that epigenetically based communication pathways exist between distinct embryonic stages to finely tune development of a new organism.
DNA Demethylases in Plants
In plants, as in mammals, the loss of DNA methylation marks can be achieved passively during cell division when DNA methyltransferases are inactive, but it can also be an active, site-specific process (Furner and Matzke, 2011; Elhamamsy, 2016). In mammals, active demethylation occurs by oxidation or deamination. First, ten–eleven translocation enzymes (TET) hydroxylate 5-methylcytosine to 5-hydroxymethylcytosine. Further oxidation by TET produces 5-formylcytosine, which can be either further oxidized or cleaved by thymine-DNA glycosylase (TDG) (Elhamamsy, 2016). In plants, DEMETER DNA GLYCOSYLASES (DME) and REPRESSOR OF SILENCING 1 (ROS1) are multifunctional enzymes that function as DNA gylcosylases that specifically excise 5-methylcytosine through cleavage of the N-glycosylic bond (Penterman et al., 2007).
ROS1 is the dominant DNA demethylase in vegetative tissues (Gong et al., 2002), where it presumably targets specific TEs and prevents spreading of their methylation patterns onto nearby protein-coding genes (Tang et al., 2016). In reproductive tissues, DME is the major DNA demethylase specifically expressed in the central cell of the female gametophyte, i.e., the future endosperm (Choi et al., 2002) and the vegetative cell of the bicellular male gametophyte (Schoft et al., 2011). In the endosperm, DME is involved in establishing gene imprinting, or the preferential expression of either the maternal or paternal allele of the same gene. For instance, DME demethylates Polycomb-group protein genes MEDEA (MEA) and FERTILIZATION INDEPENDENT SEED 2 (FIS2) (Gehring et al., 2006; Jullien et al., 2012) and a transcription factor gene FLOWERING WAGENINGEN (FWA) (Kinoshita et al., 2004), all of which are maternally expressed. The exact mechanism of gene imprinting regulation is still unclear, with indications of several additional factors other than DME affecting endosperm imprinting, such as the antagonistic effect of DNA methylation (Xiao et al., 2003), histone methylation (Gehring et al., 2006), and parental genome dosage imbalance (Jullien and Berger, 2010). Nevertheless, the importance of DME during Arabidopsis reproductive development is illustrated by evidence that DME accounts for all demethylation in the central cell (Choi et al., 2002), and that central cell demethylation also reinforces transposon methylation in the egg cell (Park et al., 2020). The same scenario occurs in the male gametophyte, all of which probably contributes to stable silencing of transposable elements across generations (Ibarra et al., 2012). Functionally related to DME and ROS1 demethylases, proteins known as Effector of transcription (ET) were recently proposed as epigenetic regulators during reproductive development. Lack of ETs expression is manifested during gametophyte and endosperm development (Tedeschi et al., 2019), suggesting them as novel plant-specific regulators of DNA methylation during reproduction.
Maintenance and de novo Methyltransferases in Plants
DNA methylation can be either maintained or established de novo. In plants, two DNA methyltransferases work to maintain DNA methylation, DNA METHYLTRANSFERASE 1 (MET1), an ortholog of mammalian DNMT1 which maintains CG methylation, and the plant-specific CHROMOMETHYLASE 3 (CMT3) which maintains CHG methylation (H = A, C, or T) (Finnegan and Kovac, 2000; Chan et al., 2005). A related methyltrasferase, CMT2, maintains CHG and CHH methylation in a process guided by methylation of histone H3 (Stroud et al., 2014). A different pathway, RNA-directed DNA methylation (RdDM) is responsible for de novo DNA methylation in all three sequence contexts and is mediated by activity of two methyltransferases, DOMAINS REARRANGED METHYLTRANSFERASE 1 and 2 (DRM1 and DRM2) (Cao and Jacobsen, 2002; Zhang and Jacobsen, 2006). RdDM is controlled by a two-armed mechanism based around the activity of two RNA polymerases. PolIV transcribes siRNA precursors (P4-RNAs), which are processed in two steps: first, RNA-DEPENDENT RNA POLYMERASE 2 (RDR2) transcribes them into double-stranded RNAs (Haag et al., 2012) and then the DICER-LIKE 3 (DCL3) protein cleaves them into 24 nt-long siRNAs (Qi et al., 2005). The ARGONAUTE 4 (AGO4) protein binds the siRNAs, forming AGO4-siRNA complexes (Qi et al., 2006; Kuo et al., 2017). The second polymerase, PolV, produces long non-coding RNAs (lncRNAs) using specific genomic loci as templates (Wierzbicki et al., 2008; Böhmdorfer et al., 2016). Genomic positioning of PolV is reinforced through binding of previously methylated DNA sites by the SU(VAR)3–9 homolog proteins SUVH2 and SUVH9 (Liu et al., 2014) and interaction with the DDR complex consisting of DEFECTIVE IN MERISTEM SILENCING 3 (DMS3), DEFECTIVE IN RNA-DIRECTED DNA METHYLATION 1 (DRD1), and RNA-DIRECTED DNA METHYLATION 1 (RDM1) (Zhong et al., 2012). It is thought that PolV-produced lncRNAs act as scaffolds for base-pairing with siRNA and associated AGO4 (Wierzbicki et al., 2009) which brings the main components of the two arms of RdDM – one led by PolIV and the other by PolV – into contact with the DRM2-mediated methylation machinery, recruiting it onto specific sites on the genome (Zhong et al., 2014). The mechanism described is the so-called canonical RdDM pathway and according to its current model, the genomic position destined for methylation is determined primarily by the activity of PolV and its suite of supporting proteins (Zhong et al., 2012; Böhmdorfer et al., 2016). Novel findings constantly challenge the current model of RdDM. For instance, although the model assumes that the slicing activity of AGO4 is not required for siRNA biogenesis, recent evidence shows that a subset of 24 nt-siRNAs is indeed sliced by AGO4, which possibly occurs in a self-reinforced loop dependent on PolV and DRM2 (Wang and Axtell, 2017). Not only that, AGO4 can also slice PolV nascent transcripts, suggesting a dual mechanism by which AGO4 recruits DRM2 through both protein-protein interaction (current model) and Pol V transcript slicing (Liu et al., 2018). The importance of AGO4 and related AGO6 and AGO9 was highlighted in a study by Gallego-Bartolomé et al. (2019) who analyzed the order of action within the RdDM pathway and the ability of different components to induce methylation when others are mutated. Their results show an essential role of AGO proteins in methylation targeting and, to make matters even more complex, show that an AGO protein can successfully bridge PolV and DRM2 to induce de novo DNA methylation even in the absence of siRNAs produced by PolIV (Gallego-Bartolomé et al., 2019). There is still a long way to go in understanding the mechanisms and roles of RdDM in plants. Indeed, canonical RdDM further extends into several non-canonical pathways which, like canonical RdDM, utilize siRNA-AGO-PolV complexes, but in which siRNAs are produced by Pol II. Non-canonical RdDM pathways are largely unexplored, possibly due to their minor role in transcription silencing. They are limited in their dependence on Pol II production of mRNA and are mostly targeting the same loci as canonical RdDM, seemingly acting as a means to produce alternatively sourced siRNAs to feed into the more predominant canonical form (for a review, see Cuerda-Gil and Slotkin, 2016). Canonical or not, there seems to be a consensus about the crucial role of PolV in determining the genomic site to be methylated via RdDM. This is particularly interesting in the context of land plant evolution – unlike the PolIV arm of RdDM, which is commonly found in land plant species, the PolV arm has reached its most complex level in flowering plants, involving several plant-specific members, and characterized by rapid evolution of its main polymerase (for a review, see Matzke et al., 2015).
In the following chapters, we discuss the role of DNA methylation during plant reproductive development and embryogenesis. Figure 1 illustrates the changes in activity of maintenance methyltransferases (CMT3 and MET1), the RdDM pathway and demethylase DME during specific developmental stages of zygotic and somatic embryogenesis in Arabidopsis thaliana, providing an overview of latest findings and a comparison of the two processes.
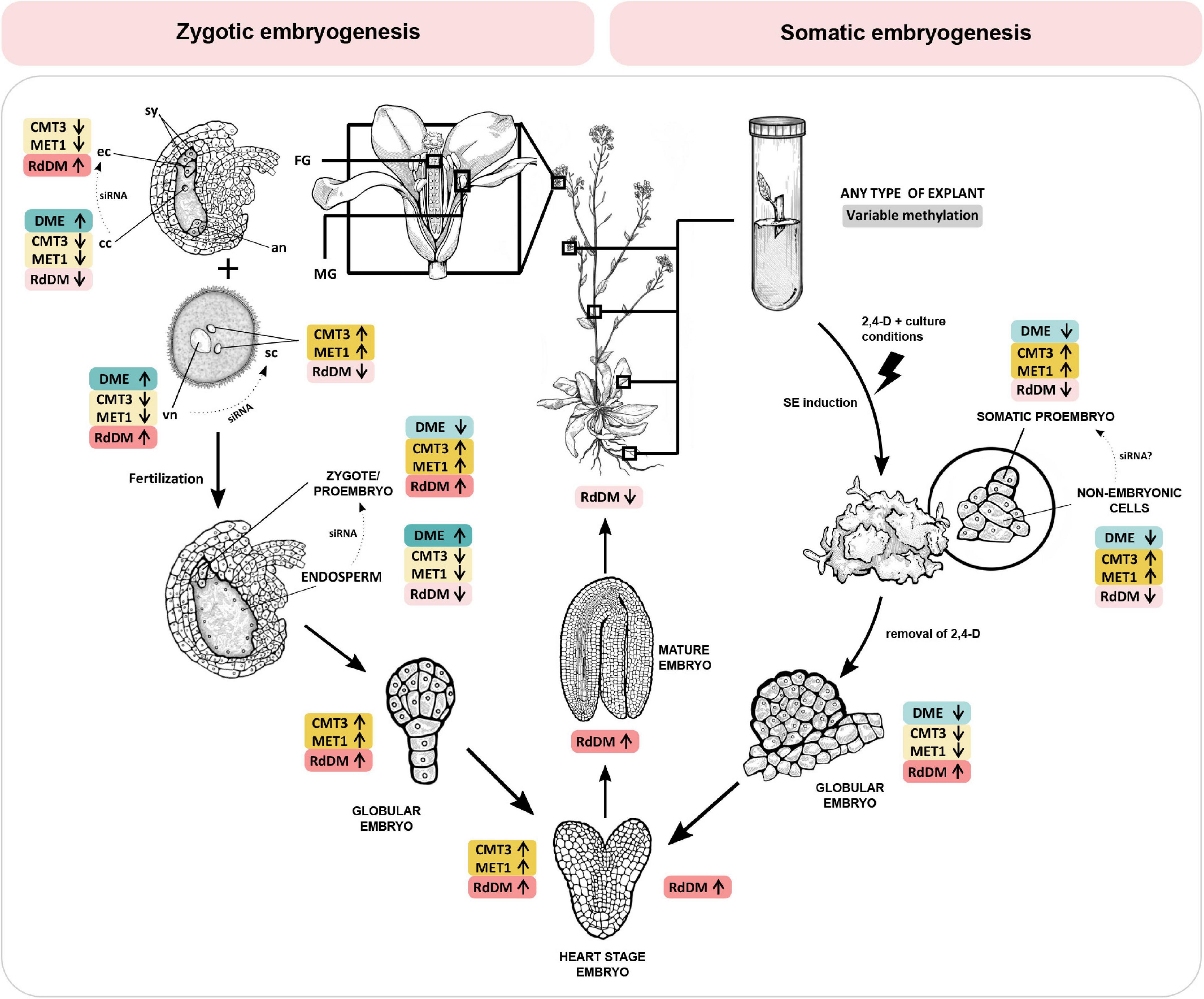
Figure 1. DNA methylation mechanisms change activity during specific stages of zygotic and somatic embryogenesis in Arabidopsis thaliana. CMT3 and MET1 (yellow) maintain DNA methylation in CHG and CG context, respectively. The RdDM pathway (red) methylates DNA de novo in all contexts, and DME (blue) actively demethylates DNA regardless of context. Zygotic embryogenesis (left). Before fertilization, RdDM is the dominant DNA methylation mechanism in the egg cell. Conversely, in the central cell, CMT3, MET1 and RdDM activity is low and DME activity is high, resulting in DNA hypomethylation. In the two spermal cells, CMT3 and MET1 are the dominant methyltransferases. Conversely, in the vegetative cell CMT3 and MET1 activity is low and DME activity is high, resulting in DNA hypomethylation. RdDM activity in the vegetative nucleus progressively increases. Embryogenesis begins when two spermal cells fertilize the egg and central cell of the female gametophyte, respectively. After fertilization, DNA methylation in the zygote and proembryo increases due to inherited activity of RdDM and increased expression of MET1 and CMT3. In the endosperm, all three methylation mechanisms reduce their activity, and DME remains active, resulting in DNA hypomethylation. RdDM activity progressively increases during embryo maturation, and drops after germination. Somatic embryogenesis (right). Upon induction of somatic embryogenesis by 2,4-D and specific culture conditions, CMT3 and MET1 become the dominant methyltransferases in both the somatic embryo and the surrounding non-embryonic cell clusters. DME activity is low. RdDM activity is initially low but progressively increases during embryo maturation, following a course similar to zygotic embryogenesis. The DNA of the central cell, vegetative nucleus and the endosperm is hypomethylated, resulting in expression of transposons and eventually biogenesis of siRNA which are transferred into the egg cell, spermal cells and the embryo, respectively (dotted arrows), to ensure genome stability. As of yet, there is no evidence of siRNA-mediated communication between somatic proembryo and non-embryonic cells in culture. Information presented in the figure is based on findings published in Jullien et al. (2012); Grzybkowska et al. (2018), and Gehring (2019). 2,4-D, 2,4-dichlorophenoxyacetic acid; an, antipodal cells; cc, central cell; CMT3, CHROMOMETHYLASE 3; DME, DEMETER DNA GLYCOSYLASE; ec, egg cell; FG, female gametophyte; MET1, DNA METHYLTRANSFERASE 1; MG, male gametophyte; RdDM, RNA-directed DNA methylation; sc, sperm cell; siRNA, small interfering RNA; sy, synergide; vn, vegetative nucleus. Enzyme activity is indicated by arrows and color intensity (up/dark – high activity, down/bright – low activity).
DNA Methylation at the Onset of Zygotic Embryogenesis
Zygotic embryogenesis in flowering plants begins with the process of double fertilization. Of the two identical sperm cells, one fuses with the egg cell and the other with the central cell, which leads to simultaneous formation of the embryo and the endosperm, respectively. In other words, within the female gametophyte, in the mutually close proximity begins the rise of two distinct kinds of progeny, the embryo as the progenitor of the next generation and the triploid endosperm with a temporary and supporting role. The majority of findings on the subject of angiosperm embryogenesis was built on evidence gained from A. thaliana, a species with highly predictable patterns of cell division and cell fate determination during embryogenesis (Mayer et al., 1993; Möller and Weijers, 2009).
Arabidopsis embryogenesis begins with a two-fold to three-fold elongation of the zygote, followed by the first asymmetric cell division which gives rise to a two-celled proembryo. The apical cell gives rise to most of the embryo, while the basal cell forms the extraembryonic suspensor which gradually disintegrates through programmed cell death. Only the topmost cell of the suspensor, the hypophysis, comprises the embryo and later forms a root meristem (Willemsen and Scheres, 2004). From the very onset of embryogenesis, asymmetricity plays the lead role, as eventually evident by establishment of the apical-basal axis which will guide the development of shoot and root tissues later on. Elongation and asymmetric division of the zygote is coordinated by two leading factors: a paternally activated MAPKK Kinase YODA (YDA) and a patterning gene WOX8 (Lukowitz et al., 2004; Ueda et al., 2011). The YDA signaling pathway regulates zygote elongation and induces phosphorylation of transcription factor WRKY2, which then directly activates WOX8 and leads to a polarized positioning of organelles and eventually an asymmetric zygote division (Ueda et al., 2011, 2017). The YDA-WRKY2-WOX8 signaling cascade is the first major regulatory point at which DNA methylation could affect early embryo development, and there has been indication that MET1 might play a role in this process (Figure 1). Namely, mutations of the MET1 gene significantly impact DNA methylation, YDA, WOX2 and WOX8 gene expression, and embryo development (Xiao et al., 2006; Table 1).
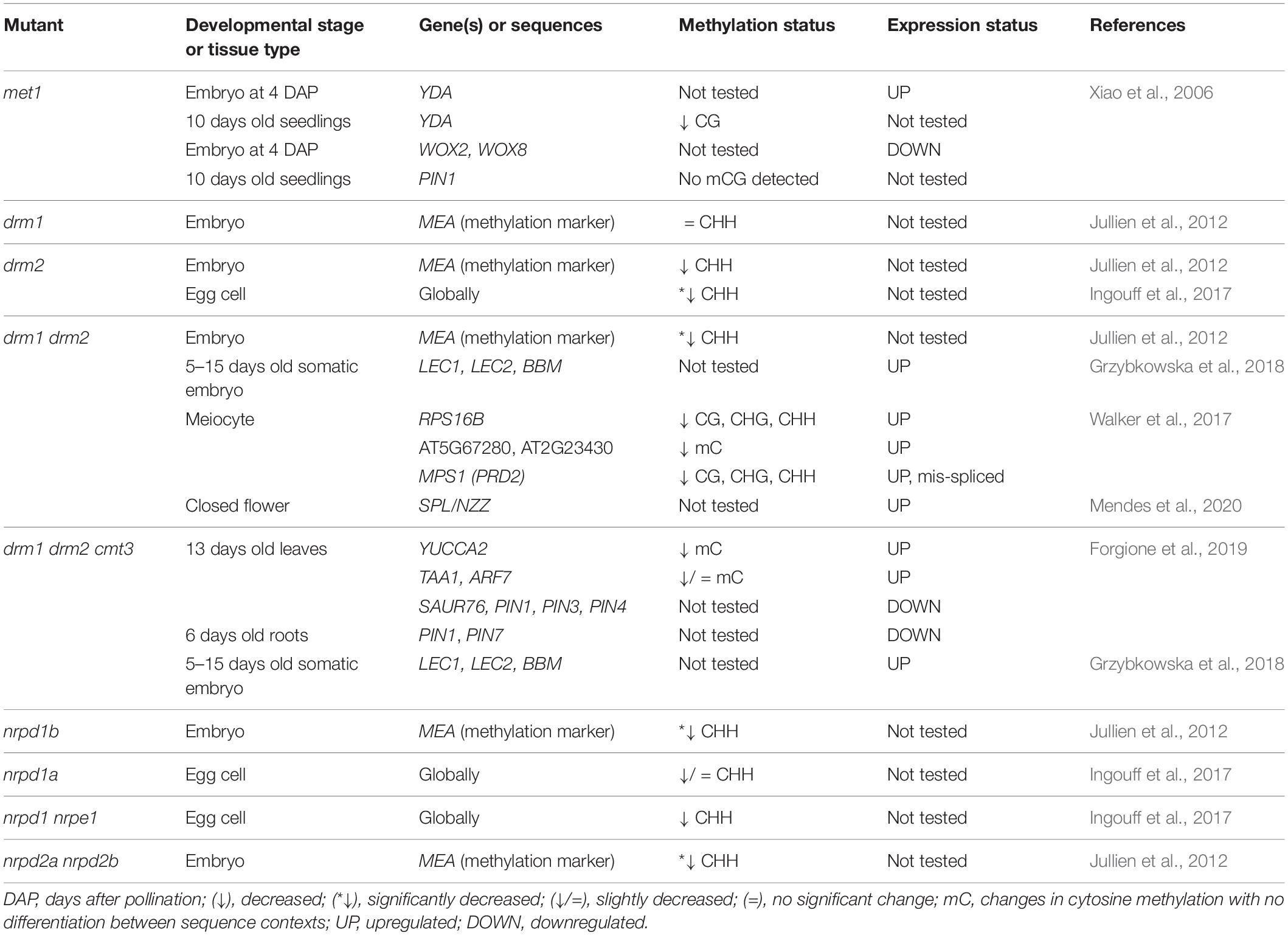
Table 1. Changes in DNA methylation and/or gene expression detected in embryos and young seedlings of Arabidopsis mutants with non-functional DNA methylation mechanisms.
The plant hormone auxin is the second major component guiding the establishment of the apical-basal axis. Specifically, what drives axis development is the sum effect of auxin biosynthesis, canalization and global distribution. In Arabidopsis, the bulk of indole-3-acetic acid, a predominant form of auxin, is synthesized from tryptophan in two steps. The first step is catalyzed by TRYPTOPHANE AMINOTRANSFERASE OF ARABIDOPSIS 1 (TAA1) and the TAA1-related enzymes TAR1/TAR2, and the second step is under control of YUCCA monooxygenases (YUC1–11). Expression of these genes has been interpreted as a proxy for auxin production (Zhao, 2012.) Interestingly, transcription of YUCCA was also shown to be methylation-dependent (Forgione et al., 2019). During embryogenesis, auxin is distributed into developmentally relevant auxin maximums via activity of embryogenic efflux carriers of the PINFORMED (PIN) family (Friml et al., 2003). Their expression is also regulated by methylation, which can be induced by different classes of methyltransferases (Xiao et al., 2006; Forgione et al., 2019). The first PIN protein expressed in the early embryo is PIN7, whose activity is limited to the basal cell after the first division of the zygote, and later the suspensor. The protein localizes in the apical domain of the plasma membrane, which results in a bottom-to-top efflux of auxin and creates an auxin maximum in the apical cell. Lack of PIN7-derived auxin maximum causes an abnormal division of the apical cell, which highlights the importance of directed auxin efflux at the 2-celled proembryo stage. In pin7 mutant embryos, the auxin maximum shifts basally into the suspensor (Friml et al., 2003; Robert et al., 2013). A similar pattern emerges in the triple methylation mutant drm1 drm2 cmt3, also termed ddc (Forgione et al., 2019).
Different Methyltransferases Are Dominant Before and After Fertilization
To clarify the role of DNA methylation during embryogenesis, Jullien et al. (2012) analyzed the activity of specific DNA methyltransferases in different embryonic stages of Arabidopsis thaliana. This study shows a dramatic shift in availability of methyltransferases between the egg cell and the zygote (Figure 1). In the egg cell, DNA methylation relies predominantly on de novo DNA methyltransferases DRM1 and DRM2. Expression of all three methyltranferases of the DRM class (DRM1, DRM2, and DRM3) is high, while expression of methylation-maintaining enzymes MET1 and CMT3 is low. Genes encoding other components of the RdDM pathway (AGOs, PolIV, PolV, DMS3) are also highly expressed, pointing toward an important role of RdDM during this reproductive stage (Jullien et al., 2012). Following fertilization and the first division of the zygote, DRM1 expression dramatically decreases and DRM2 becomes the main de novo methyltransferase during embryogenesis (Jullien et al., 2012). This could be the cause of a significant increase in CHH methylation during embryogenesis in Arabidopsis (Bouyer et al., 2017), an effect which was also shown in soybean (Lin et al., 2017), chickpea (Rajkumar et al., 2020), and Brassica rapa (Chakraborty et al., 2021). Additionally, all three major DNA methyltransferases (MET1, CMT3, and DRM2) become strongly expressed in both the embryo proper and the suspensor (Jullien et al., 2012; Figure 1). The authors suggest that the fertilization event is the trigger which leads to a rise in methyltransferase activity to levels higher than those in vegetative tissues. If so, the same trend of methylation changes would be expected in both fertilized gametes, the egg and the central cell, regardless of the different levels of methylation established in them before fertilization (Gehring et al., 2009). However, fertilization of the central cell does not lead to a similar rise in DNA methyltransferase activity but actually leads to a wholly different effect – a decrease in global methylation and quantity of methyltranferases (Ibarra et al., 2012; Park et al., 2016, 2020), despite both spermal cells possessing identical regulatory potential (Ingouff et al., 2017). Therefore, strong activation of DNA methyltranferases could occur independently of fertilization and a similar rise in activity might be occurring during both ZE and SE, or any other type of asexual embryogenesis. This implies that a set of signals beyond the fertilization event marks the beginning of embryogenesis and thus shapes the methylation patterns of the early embryo, regardless of its origin.
DNA Methylation at the Onset of Somatic Embryogenesis
Somatic embryogenesis is a process during which somatic cells gain embryogenic competence to develop morphologically distinct embryonic stages which will give rise to a new plant organism. Virtually any plant cell at any given moment has the capacity to acquire developmental characteristics of a fertilized egg cell, which is followed by intensive developmental reprogramming (Nishiwaki et al., 2000; Fehér et al., 2003; Fehér, 2005). Although SE can occur naturally, as found in the genus Kalanchoë, it is much more common in plant in vitro culture, where it can be induced in numerous plant species and from different types of explants if granted adequate conditions (Loyola-Vargas and Ochoa-Alejo, 2016). Acquiring embryogenic competence relies on morphological, genetic and most likely epigenetic plasticity. The first effect is dedifferentiation to a state of totipotency which can then lead to a broad spectrum of possible redifferentiation outcomes, including embryogenesis (Verdeil et al., 2007). Specific plant growth regulators or application of stressful conditions can be used to stimulate embryogenic competence in somatic cells. Auxins, and especially synthetic auxin 2,4-dichlorophenoxyacetic acid (2,4-D), are the most effective inductors of SE, while their removal from growth medium stimulates embryo maturation. Exogenous auxin helps establish the auxin gradient within the explant. The auxin maximum builds at the site of contact between medium and tissue and, following auxin uptake by the tissue, the auxin level progressively decreases depending on the direction of auxin transport within the explant. At specific sites, the optimal auxin level and hormone balance is reached, which ensures favorable conditions for acquiring embryogenic competence (Fehér, 2005). In Arabidopsis SE, much like in ZE, PIN-mediated polar transport of auxin is essential for establishing auxin gradients and subsequent induction of embryogenesis (Su et al., 2009). Similar auxin dynamics in ZE and SE are backed by similar transcription patterns of genes involved in auxin distribution and transport, as well as genes involved in regulation of specific auxin responses, such as genes encoding AUXIN RESPONSE FACTORS (ARFs) and AUXIN/IAA inhibitors (Aux/IAAs) (Gliwicka et al., 2013). In general, there are many similarities between ZE and SE at the level of gene expression. In cotton, the processes of ZE and SE share more than 50% of highly expressed genes involved in methylation, stress response, hormone response, embryonic fate regulation, polarity and pattern formation (Jin et al., 2014). A similar overlap exists in Arabidopsis, where most abundant transcription factors during SE are those involved in developmental processes, phytohormone and stress responses (Gliwicka et al., 2013) and many of these genes were also found during ZE (Leljak-Levanić et al., 2015). However, a recent global transcriptome analysis in Arabidopsis revealed a higher level of similarity between transcriptomes of SE and germinating seeds, rather than ZE, indicating more complex dynamics than suggested by previous research (Hofmann et al., 2019).
Auxin Treatment Regulates DNA Methyltransferase Activity and Expression of Somatic Embryogenesis-Marker Genes
Reports on Daucus carota and Arabidopsis indicate that auxin-related conditions which promote embryogenesis are associated with DNA hypermethylation (LoSchiavo et al., 1989; Yamamoto et al., 2005; Jiang et al., 2015). Exogenous auxin increases cytosine methylation during somatic embryo induction in carrot, while auxin removal rapidly decreases it (LoSchiavo et al., 1989). This is probably a consequence of auxin-mediated increase of DNA methyltransferase gene expression and downregulation of demethylases (Figure 1), as described for Arabidopsis (Grzybkowska et al., 2018). Leljak-Levanić et al. (2004) show that in pumpkin (Cucurbita pepo) not only auxin treatment but other SE-inducing stress treatments, like nitrogen-starvation, cause hypermethylation of DNA during SE induction. However, in the majority of reports an inverse relationship between embryogenic competence and DNA methylation was observed. In Eleutherococcus senticosus (Chakrabarty et al., 2003), Pinus nigra (Noceda et al., 2009), and Picea abies (Ausin et al., 2016) DNA hypomethylation seems to be associated with early stages and embryo induction. Moreover, DNA hypomethylation provoked by demethylation agents 5-azacitide has been recommended for improving the embryogenic capacity of poorly responding plant species or for aged cultures of Theobroma cacao (Pila Quinga et al., 2017). Due to the diversity of results, it is clear that the global level of DNA methylation is not specifically related to the embryogenesis process but more likely reflects the epigenetic status of explants caused by tissue culture conditions.
A recent gene expression analysis of four major methyltranferases during SE in Arabidopsis shows that MET1 and CMT3 transcripts highly accumulate during early SE and that expression of DRM1 and DRM2 decreases, but is followed by a striking increase in DRM2 expression in later stages (Grzybkowska et al., 2018). Similarly, addition of 2,4-D to carrot culture positively correlates with expression of MET1 during induction of SE and before the formation of embryonic cell clumps (Yamamoto et al., 2005). It appears that MET1 and CMT3 are the dominant methyltransferases during induction of SE (Figure 1), and in Arabidopsis this interplay is nicely illustrated by the presence of an Auxin Response Element (AuxRE) in the CMT3 promoter, signifying a mode through which auxin can directly control CMT3 activity (Grzybkowska et al., 2018). It is interesting to note that during Arabidopsis SE, an increase in methyltransferase gene expression is combined with a decrease in expression of demethylase genes but that overall, surprisingly, global methylation level decreases (Grzybkowska et al., 2018). When it comes to global methylation, it remains difficult to clarify the highly complex regulation of DNA methylation mechanisms during SE. However, the authors show that in SE cultures of a mutant with non-functional DRMs (drm1 drm2) and a triple mutant with non-functional DRMs and CMT3 (drm1 drm2 cmt3) SE-related genes of the LEAFY COTYLEDON (LEC) transcription factor family, LEC1, LEC2 (Lotan et al., 1998; Harada, 2001; Gaj et al., 2005; Stone et al., 2008; Wójcikowska et al., 2013) and BABYBOOM (BBM; Boutilier et al., 2002; Casson et al., 2005) are significantly upregulated (Grzybkowska et al., 2018), which indicates that these same genes could be differentially methylated genes during SE, a hypothesis which remains to be tested in the future. In embryogenic culture of Daucus carota, promoters of LEC1 and WUSCHEL (WUS) are hypomethylated (Shibukawa et al., 2009). Similarly, promoters of SOMATIC EMBRYOGENESIS RECEPTOR KINASE (SERK; Schmidt et al., 1997; Hecht et al., 2001), LEC2, and WUS are hypomethylated in embryogenic tissue of Boesenbergia rotunda (Karim et al., 2018). In addition, a recent epigenome-wide study of nine different developmental stages of SE in soybean revealed an early wave of hypermethylation, especially in the CHH context. This was linked to auxin treatment and increased RdDM activity during induction and early SE (Ji et al., 2019).
Defects in Reproductive Development of DNA Methylation Mutants
DNA methylation mutants of Arabidopsis thaliana have been invaluable for exploration of mechanisms which underlie the activity of specific DNA methylation pathways during ZE and SE. First, DNA methylation mechanisms involve numerous proteins and different combinations of their mutations lead to different phenotypic characteristics, from those evident during haploid reproductive stages to those which manifest during embryogenesis. For a comprehensive list of mutations and the associated phenotypes, see Supplementary Table 1. Abolition of different DNA methylation mechanisms by loss-of-function mutations causes temporally specific phenotypes, affecting different stages of reproductive development. For instance, the loss of function of both RdDM methyltransferases, DRM1 and DRM2 (drm1 drm2), causes an aberrant female gametophyte, while loss of function of MET1 and CMT3 results in aberrant embryos (Jullien et al., 2012).
Phenotypic changes related to premeiotic development can be observed during cell fate specification of the megaspore mother cell (MMC). In wild type Arabidopsis, one cell of the hypodermal ovule layer is specified as the MMC. In the double drm1 drm2 mutant, multiple cells become specified as the MMC, resulting in multiple precursors of the female gametophyte (Mendes et al., 2020; Figure 2). Here, loss of DRM function causes upregulation of the SPOROCYTELESS/NOZZLE (SPL/NZZ) transcript encoding a protein involved in balancing the reproductive cell fate establishment in the premeiotic ovule (Mendes et al., 2020). A similar phenotype develops in mutants of the AGO4, AGO6, AGO8 and AGO9 genes (shown for AGO4 in Figure 2), and depending on the mutated gene, the number of MMCs varies, from two to four (Hernández-Lagana et al., 2016). Multiple MMC-like cells are also caused by loss-of-function mutations of genes encoding proteins involved in the PolIV arm of RdDM, such as the aforementioned polymerase RDR2 (rdr2) which produces double-stranded siRNAs, its ortholog RDR6 (rdr6) which acts in non-canonical RdDM, an RNA-binding protein called SUPPRESSOR OF GENE SILENCING 3 (sgs3) and the siRNA-processing protein DCL3 (dcl3) (Olmedo-Monfil et al., 2010). A similar phenotype is also found in a double mutant in which both NRPD1a and NRPD1b (also known as NRPE1), the respective largest subunits of PolIV and PolV are mutated (nrpd1a nrpd1b) and both polymerases are non-functional (Olmedo-Monfil et al., 2010). It should be noted here that loss of function of newly discovered ET demethylases decreases the SPL/NZZ expression (Tedeschi et al., 2019) suggesting the possible balancing effects of RdDM methylation and ET-specific demethylation during plant reproduction.
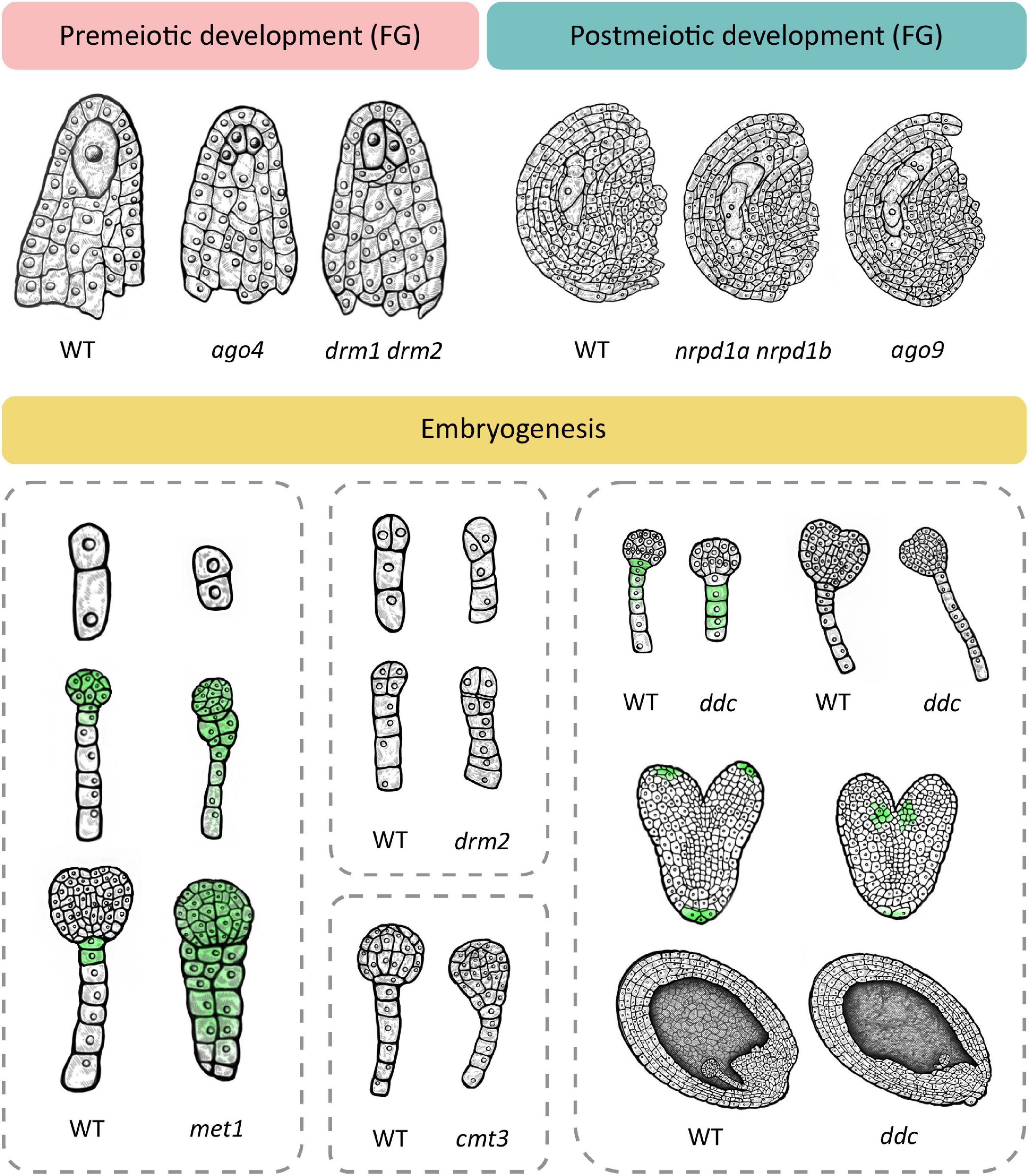
Figure 2. Loss of function of different methylation mechanisms leads to several dominant phenotypes at the premeiotic and postmeiotic stage and during embryogenesis. Premeiotic development. In wild type, one cell of the ovule is specified as the megaspore mother cell (MMC) which divides meiotically to give rise to the female gametophyte (FG). Several mutants with non-functional RdDM develop multiple MMC-like cells in premeiotic ovules, exemplified here in ago4 and drm1 drm2. Postmeiotic development. The wild type megaspore divides mitotically to produce a female gametophyte. RdDM mutants such as ago9 and the double nrpd1 nrpd2 mutant exhibit two female gametophytes in postmeiotic ovules. Embryogenesis. Wild type embryogenesis begins with zygote elongation, asymmetrical division and subsequent formation of embryo and suspensor. In the met1 mutant with non-functional MET1, the zygote remains short and divides symmetrically (top) and longitudinal divisions in the suspensor lead to unclear demarcation of the embryo-suspensor border (middle and bottom). Additionally, auxin transport is disturbed which leads to even distribution of auxin throughout the embryo (green). The cmt3 mutant with non-functional CMT3 also shows unclear demarcation of the embryo-suspensor border in early globular stage. Loss of function of RdDM leads to similar aberrations. The drm2 mutant shows disturbed patterns of cell divisions in the early embryo in both the suspensor (top and bottom) and the embryo proper (bottom). The triple drm1 drm2 cmt3 mutant (ddc) exhibits a reduced number of suspensor cells at the globular stage with a hypophysis devoid of auxin signal (top left) and a longer suspensor at early heart stage (top right). At the heart stage, auxin maximums appear basally from cotyledons (middle). Wild type embryos are positioned in parallel with the top–bottom axis of the ovule. In a portion of ddc mutant plants, embryos are positioned perpendicular to the axis, and the endosperm is histologically disorganized (bottom). This schematic image was created based on phenotypes described in relevant scientific articles. For details and references, see Supplementary Table 1.
Besides exhibiting a premeiotic phenotype, ago9, rdr2, dcl3 and the nrpd1a nrpd1b double mutant are additionally affected in postmeiotic development, with noted formation of multiple female gametophytes (shown for nrpd1a nrpd1b and ago9 in Figure 2). In some cases, two developing gametophytes are separated by several somatic cells, indicating that they originated from non-sister cells, of which one had to be of somatic origin (Olmedo-Monfil et al., 2010). This phenomenon could serve as an illustration of the potency of epigenetic mechanisms in regulating development and even establishing a novel trajectory of development from unlikely origins, as described for SE. In the aforementioned mutants with non-functional RdDM, methyltransferase MET1 is functional but it does not compensate for the lack of RdDM, possibly due to low expression of MET1 (Jullien et al., 2012) or the functional limitations of MET1 activity, i.e., its dependence on previous methylation and specificity for the CG context.
Deficiencies in RdDM and other DNA methylation mechanisms also cause aberrations during embryonic development. Interestingly, the loss-of-function MET1 mutant (met1), displays a wide array of successive phenotypes (Xiao et al., 2006; Figure 2) which first manifest during the elongation and asymmetric division of the zygote and continue later with abnormalities in numbers and planes of cell division throughout embryogenesis as well as delays in embryo development. According to Xiao et al. (2006), loss of MET1 directly or indirectly affects transcription of genes that regulate cell identity during early embryogenesis. Specifically, it causes downregulation of WOX2 and WOX8, upregulation of YDA and altered expression pattern of PIN1, which becomes evenly distributed throughout the entire embryo, in stark contrast to its usual accumulation in the apical cell-derived regions. Concurrently, auxin becomes evenly distributed in both the apical and basal cell-derived regions, which hinders the establishment of the auxin maximum, possibly accounting for the lack of demarcation between embryo and suspensor (Figure 2). The authors postulate that hypomethylation is the most probable cause of phenotypic defects in the met1 mutant. They also suggest the possibility of compensation for loss of CG-specific MET1 through induced activation of other methylation mechanisms which could then cause ectopic hypermethylation on specific positions and result in further developmental aberrations (Xiao et al., 2006).
Loss of function of the non-CG-specific methyltransferase CMT3 (cmt3) leads to aberrations in later stages of embryogenesis, with a lack of clear demarcation between the embryo and suspensor due to longitudinal cell divisions in the suspensor (Figure 2). The double met1 cmt3 mutant embryos display similar aberrations but with more dramatic effects on embryo development, seed viability and plant development (Xiao et al., 2006).
Unlike MET1 and CMT3, de novo methyltransferase DRM2 can induce DNA methylation in all three sequence contexts (Chan et al., 2005). Ingouff et al. (2017) show that the drm2 mutant suffers a global loss of maternally provided CHH methylome in the egg cell, causing abnormal patterning and division plane defects in the early embryo (Figure 2). Furthermore, the triple ddc mutant, in which DRM1, DRM2 and CMT3 are non-functional, shows various phenotypic aberrations during embryogenesis, which has been linked to an impaired auxin pathway (Forgione et al., 2019; Figure 2). In the early embryo stage, the ddc mutant exhibits a reduced number of suspensor cells and a delayed suspensor development. In the globular stage, suspensor cell proliferation is arrested, resulting in a shorter suspensor with a hypophysis devoid of auxin signal, while increased proliferation and a more elongated suspensor marks the young heart embryo stage. When the embryo reaches heart stage, auxin maximums appear basally from cotyledons, contrary to the usual accumulation of auxin in the apical regions of the cotyledons (Figure 2). Finally, aberrations in the embryo are combined with disordered histological organization of the endosperm (Figure 2). Interestingly, this aberration reminds of a phenotype described for the yda mutant, where embryos are positioned perpendicular to the top-bottom axis of the ovule, as if lying on their sides (Lukowitz et al., 2004). The leaf of the ddc mutant is marked by increased expression of genes involved in the auxin biosynthesis pathway, namely YUC2 and TAA1, and while TAA1 was not differentially methylated, the authors report significant demethylation in the promoter region of YUC2 (Forgione et al., 2019). Although gene expression and methylation levels of auxin-related genes have not been examined in ddc embryos (Forgione et al., 2019), the results obtained in leaf tissues combined with described auxin-related embryo aberrations serve as a novel link between de novo DNA methylation and the role of auxin pathways in embryonic development, which remains to be further explored in the future.
In mammals, loss-of-function mutation of DNA methyltransferase Dnmt1 causes an embryo lethal phenotype (Li et al., 1992), a dramatic effect which does not occur in plants, including Arabidopsis, when either of their three major methyltransferases is mutated. On the other hand, a number of methylation mutants of investigated plant species were shown to be either lethal at some point during development, hypomorphic, or depleted in multiple methylation contexts (Domb et al., 2020). To date, an Arabidopsis mutant with a complete loss of all DNA methylation has not been described, as zero-methylation state is most likely lethal. The existence of single mutants, however, suggests redundancy between mechanisms, additionally supported by the fact that mutations affecting more than one methylation mechanism lead to more pronounced developmental aberrations (Xiao et al., 2006). Interestingly, single-mechanism mutations lead to temporally specific phenotypes, indicating activity shaped by developmental needs. RdDM is particularly interesting in this aspect as it could naturally serve as a potent mechanism in not only substituting for missing methylation marks, but also in establishing novel methylation patterns in response to various internal and external cues. The RdDM pathway is comprised of numerous components, not all of which are indispensable for DNA methylation to occur. The highest level of functional promiscuity has been ascribed to DMS3, a protein which recruits PolV to the genome, and which seems to perform this role even when most other RdDM components have been mutated (Gallego-Bartolomé et al., 2019). The research of RdDM seems to be marked by exceptions, rather than rules, which could point to the pathway’s highly versatile roles, at least some of which could be linked to embryogenesis, including a specific role of auxin dynamics in regulating embryonic development. Clarification of the role of RdDM in these processes could be aided by identification of genes directly regulated by RdDM-mediated DNA methylation during embryogenesis. In the following section, we bring an overview of genomic regions which are potential targets of the PolV polymerase, a component of RdDM which determines the future methylation site, and analysis of loci specifically linked to auxin dynamics, reproductive development and embryogenesis.
Determination of Genomic Loci Targeted by RNA-Directed DNA Methylation
The chromatin association profile of NRPE1 (the largest subunit of PolV) in Arabidopsis thaliana Col-0 flowers is published by Liu et al. (2018). To determine specific genes potentially regulated by RdDM, read filtering, mapping, peak calling and peak annotation was performed to retain only the peaks associated with 1142 genes categorized into 79 gene ontologies (GO) related to auxin metabolism, reproductive development, and zygotic and somatic embryogenesis (for a complete list of GOs, refer to Supplementary Table 2). Finally, of the 441 remaining peaks, we retained peaks with fold change greater than 2.0, p-value less than 10^-12 and which were positioned up to 3000 bp upstream from the associated gene, resulting in 224 peaks in total (Supplementary Table 3). Following selection, most of the auxin metabolism genes with known roles in SE or ZE mentioned earlier were found as targets of PolV. Namely, the list contained genes involved in the biosynthesis of auxin (TAA1, TAR1, TAR2, YUC2, YUC5, YUC10, YUC1, LEC2), in the regulation of directed auxin transport (PIN3, PIN4, PIN7) and genes encoding auxin response factors (ARF1, ARF2, ARF8) and AUX/IAA inhibitors (IAA6, IAA8, IAA14, IAA18, IAA27). It was previously shown that YUC2 is hypomethylated in the dcc mutant, indicating a role of RdDM, possibly in combination with CMT3, while PIN1, PIN3, PIN4, and PIN7 have been suggested as potential targets due to their variable expression in the ddc mutant (Forgione et al., 2019). In addition, the WOX8 gene encoding a protein involved in establishment of apical-basal axis in the young embryo was also identified as a potential PolV target. Although it was previously shown that regulation of the WOX2/WOX8 pair depends on MET1 (Xiao et al., 2006), the connection with RdDM indicates the redundancy of this pathway in the WOX2/WOX8 gene expression regulation.
One of our additional criteria for gene selection was position of the peak up to 3000 bp upstream from the TSS of an associated gene. Zhong et al. (2012) show that PolV binds to promoters and that the loss of its largest subunit (NRPE1) leads to an increase in expression of genes located near the PolV binding site. Specifically, when PolV is non-functional, the effect of its loss on gene expression, i.e., upregulation, is higher for genes which have the PolV binding site closer to the TSS (Zhong et al., 2012). Therefore, we selected genes with up to 50 bp distance between the peak and the TSS to generate a list of genes most likely to be regulated by RdDM. This selection resulted in a list of 22 genes (Table 2), among which only SIR3 is functionally related to stress response. The remaining 21 genes are directly or indirectly related to reproductive development and their loss of function leads to aberrations in megaspore development, formation of supernumerary egg cells, zygotes or embryos and disturbances in auxin metabolism, transport or effects (for references see Table 2). Additionally, some of these genes affect embryogenesis through regulation of transcription, posttranscriptional regulation, proteasomal degradation, cell-to-cell signalization, t-RNA splicing, flavonoid biosynthesis, and biogenesis of multifunctional iron–sulfur clusters (for references see Table 2). Interestingly, out of 22 genes on the list, six belong to Early Culture Abundant 1 (ECA1) gametogenesis-related family, which is one of the three largest families encoding small cysteine-rich proteins, many of which are expressed during reproductive development (reviewed in Sprunck et al., 2014). Members of this family were first described in barley, where HvECA1 is responsible for stress-induced switch from gametophytic pathway to embryogenic route (Vrinten et al., 1999). Functional characterization of HvECA1 resulted in discovery of a significant number of similar CRPs in egg cell transcriptomes of different flowering plants. In Arabidopsis, there are 124 genes of ECA1 gametogenesis-related family (Sprunck et al., 2014). The best described protein candidate, EGG CELL 1 (EC1), is secreted from the egg cell and responsible for sperm cell activation to gain competence for gamete fusion, which indicates that it is essential for the reproductive phase of development (Sprunck et al., 2012). Besides egg cell-specific genes, a significant number of ECAs are expressed in synergids under control of the synergide-specific MYB98 transcription factor (Jones-Rhoades et al., 2007). Sprunck et al. (2014) argue that members of this family potentially partake in different processes related to reproductive development, including androgenesis, as occurs in barley (Sprunck et al., 2014). Our overview of PolV-bound genomic loci indicates ECA1 gametogenesis-related proteins as interesting targets for further research of RdDM roles in reproductive development. Interestingly, genes encoding ECA1 gametogenesis-related proteins have an unusual transposon-like pattern of methylation, in which RdDM mediates gene body methylation in CG, CHG and CHH contexts. This type of methylation is generally linked to expression downregulation in vegetative tissues and is usually low in synergids, in which many CRP genes are expressed (You et al., 2012). Therefore, ECA1 gametogenesis-related family could be additionally used to study the role of RdDM in transition between the reproductive and vegetative stage.
Concluding Remarks and Perspectives
There are still many aspects of plant embryogenesis that are not fully understood, especially at its onset. How is the reprogramming of the transcriptome and DNA methylome at the onset of embryogenesis controlled and what are the signals that direct or redirect the zygote or a somatic cell into a state of embryogenic competence? There is substantial evidence linking RdDM to gametophyte development and embryogenesis, but the exact mechanisms through which RdDM could regulate gene expression prior to and at the onset of plant embryogenesis remains to be elucidated. Here, we propose a list of genes presumably targeted by PolV, which could serve as a pool of gene candidates for future research of the roles of RdDM in reproductive development and embryogenesis, as well as the mechanisms by which auxin dynamic might shape these processes. Different components of the RdDM pathway certainly play their own distinct roles in this process. For instance, members of the AGO4 clade, consisting of AGO4, AGO6, and AGO9, all participate in the RdDM pathway but functionally diverge in terms of their ability to promote short RNA accumulation and DNA methylation, and this distinction is present even when different AGOs bind the same short RNAs (Havecker et al., 2010). At least in part, the difference in AGO function could be attributed to their distinct expression profiles (Havecker et al., 2010), with AGO9 primarily expressed in female gametes, where it has a role in TE silencing (Olmedo-Monfil et al., 2010). The specificity of individual components of RdDM for distinct tissues and even cell types could indicate the existence of specialized branches of RdDM, assembled according to different biological requirements and possibly consisting of undiscovered and highly specialized associated factors. In the future, it would be interesting to compare the siRNA profile of AGO9 with the PolV-bound genome sites, and potentially retrieve a set of genes presumably regulated by RdDM in a tissue-specific manner, with functions related to female gametophyte development. Clarification of the RdDM mechanism at the onset of embryogenesis is also of practical value, as it could open the door to an applicative function combining DNA methylation-based techniques with SE- mediated propagation. Treatment with epigenetic regulators that induce global demethylation, such as 5-azacytidine, was shown to be beneficial in plant breeding (Kondo et al., 2006), showing that loss of methylation can be a significant source of variation, with potentially favorable effects. On the other hand, the application of CRISPR/Cas technology to edit epigenetic marks at specific loci (McDonald et al., 2016; Papikian et al., 2019) and to consequently modulate gene expression, may lead to more precise and predictable breeding (Mercé et al., 2020), especially if we take into account that epigenetic marks are heritable through at least a few generations (Papikian et al., 2019).
Author Contributions
DLL developed the idea. LM carried out the bioinformatics and determination of RdDM genomic loci. MT and AŠ performed the mutant manuscript analysis and prepared the illustrations. DLL and AŠ drafted and wrote most of the manuscript while MT, NB, MJ, and TV participated in writing. All the authors contributed to the article and approved the submitted version.
Funding
This work was supported by grants from the Croatian Science Foundation (project PHYTOMETHDEV; IP 2016-06-6229 to DLL).
Conflict of Interest
The authors declare that the research was conducted in the absence of any commercial or financial relationships that could be construed as a potential conflict of interest.
Publisher’s Note
All claims expressed in this article are solely those of the authors and do not necessarily represent those of their affiliated organizations, or those of the publisher, the editors and the reviewers. Any product that may be evaluated in this article, or claim that may be made by its manufacturer, is not guaranteed or endorsed by the publisher.
Supplementary Material
The Supplementary Material for this article can be found online at: https://www.frontiersin.org/articles/10.3389/fpls.2021.764999/full#supplementary-material
References
Anastasiadi, D., Esteve-Codina, A., and Piferrer, F. (2018). Consistent inverse correlation between DNA methylation of the first intron and gene expression across tissues and species. Epigenetics Chromatin 11:37. doi: 10.1186/s13072-018-0205-1
Ausin, I., Feng, S., Yu, C., Liu, W., Kuo, H. Y., Jacobsen, E. L., et al. (2016). DNA methylome of the 20-gigabase Norway spruce genome. Proc. Natl. Acad. Sci. U.S.A. 113, E8106–E8113. doi: 10.1073/pnas.1618019113
Bartels, A., Han, Q., Nair, P., Stacey, L., Gaynier, H., Mosley, M., et al. (2018). Dynamic DNA methylation in plant growth and development. Int. J. Mol. Sci. 19:2144. doi: 10.3390/ijms19072144
Bewick, A. J., Vogel, K. J., Moore, A. J., and Schmitz, R. J. (2017). Evolution of DNA methylation across insects. Mol. Biol. Evol. 34, 654–665. doi: 10.1093/molbev/msw264
Böhmdorfer, G., Sethuraman, S., Rowley, M. J., Krzyszton, M., Rothi, M. H., Bouzit, L., et al. (2016). Long non-coding RNA produced by RNA polymerase V determines boundaries of heterochromatin. Elife 5:e19092. doi: 10.7554/eLife.19092
Borg, M., Jacob, Y., Susaki, D., LeBlanc, C., Buendía, D., Axelsson, E., et al. (2020). Targeted reprogramming of H3K27me3 resets epigenetic memory in plant paternal chromatin. Nat. Cell Biol. 22, 621–629. doi: 10.1038/s41556-020-0515-y
Borg, M., Papareddy, R. K., Dombey, R., Axelsson, E., Nodine, M. D., Twell, D., et al. (2021). Epigenetic reprogramming rewires transcription during the alternation of generations in Arabidopsis. Elife 10:e61894. doi: 10.7554/eLife.61894
Boutilier, K., Offringa, R., Sharma, V. K., Kieft, H., Ouellet, T., Zhang, L., et al. (2002). Ectopic expression of BABY BOOM triggers a conversion from vegetative to embryonic growth. Plant Cell 14, 1737–1749. doi: 10.1105/tpc.001941
Bouyer, D., Kramdi, A., Kassam, M., Heese, M., Schnittger, A., Roudier, F., et al. (2017). DNA methylation dynamics during early plant life. Genome Biol. 18:179. doi: 10.1186/s13059-017-1313-0
Cao, X., and Jacobsen, S. E. (2002). Locus-specific control of asymmetric and CpNpG methylation by the DRM and CMT3 methyltransferase genes. Proc. Natl. Acad. Sci. U.S.A. 99(Suppl. 4), 16491–16498. doi: 10.1073/pnas.162371599
Casson, S., Spencer, M., Walker, K., and Lindsey, K. (2005). Laser capture microdissection for the analysis of gene expression during embryogenesis of Arabidopsis. Plant J. 42, 111–123. doi: 10.1111/j.1365-313X.2005.02355.x
Chakrabarty, D., Yu, K. W., and Paek, K. Y. (2003). Detection of DNA methylation changes during somatic embryogenesis of Siberian ginseng (Eleuterococcus senticosus). Plant Sci. 165, 61–68. doi: 10.1016/S0168-9452(03)00127-4
Chakraborty, T., Kendall, T., Grover, J. W., and Mosher, R. A. (2021). Embryo CHH hypermethylation is mediated by RdDM and is autonomously directed in Brassica rapa. Genome Biol. 22:140. doi: 10.1186/s13059-021-02358-3
Chan, S. W., Henderson, I. R., and Jacobsen, S. E. (2005). Gardening the genome: DNA methylation in Arabidopsis thaliana. Nat. Rev. Genet. 6, 351–360. doi: 10.1038/nrg1601
Choi, Y., Gehring, M., Johnson, L., Hannon, M., Harada, J. J., Goldberg, R. B., et al. (2002). DEMETER, a DNA glycosylase domain protein, is required for endosperm gene imprinting and seed viability in Arabidopsis. Cell 110, 33–42. doi: 10.1016/S0092-8674(02)00807-3
Colombo, M., Masiero, S., Vanzulli, S., Lardelli, P., Kater, M. M., and Colombo, L. (2008). AGL23, a type I MADS-box gene that controls female gametophyte and embryo development in Arabidopsis. Plant J. 54, 1037–1048. doi: 10.1111/j.1365-313X.2008.03485.x
Cuerda-Gil, D., and Slotkin, R. K. (2016). Non-canonical RNA-directed DNA methylation. Nat. Plants 2:16163. doi: 10.1038/nplants.2016.163
Dodeman, V. L., Ducreux, G., and Kreis, M. (1997). Zygotic embryogenesis versus somatic embryogenesis. J. Exp. Bot. 48, 1493–1509. doi: 10.1093/jxb/48.8.1493
Domb, K., Katz, A., Harris, K. D., Yaari, R., Kaisler, E., Nguyen, V. H., et al. (2020). DNA methylation mutants in Physcomitrella patens elucidate individual roles of CG and non-CG methylation in genome regulation. Proc. Natl. Acad. Sci. U.S.A. 117, 33700–33710. doi: 10.1073/pnas.2011361117
Elhamamsy, A. R. (2016). DNA methylation dynamics in plants and mammals: overview of regulation and dysregulation. Cell Biochem. Funct. 34, 289–298. doi: 10.1002/cbf.3183
Ezquer, I., Salameh, I., Colombo, L., and Kalaitzis, P. (2020). Plant cell walls tackling climate change: biotechnological strategies to improve crop adaptations and photosynthesis in response to global warming. Plants 9:212. doi: 10.3390/plants9020212
Fehér, A. (2005). Why somatic plant cells start to form embryos? Plant Cell Monogr. 2, 85–101. doi: 10.1007/7089_019
Fehér, A., Pasternak, T. P., and Dudits, D. (2003). Transition of somatic plant cells to an embryogenic state. Plant Cell Tissue Organ Cult. 74, 201–228. doi: 10.1023/A:1024033216561
Finnegan, E. J., and Kovac, K. A. (2000). Plant DNA methyltransferases. Plant Mol. Biol. 43, 189–201. doi: 10.1023/A:1006427226972
Finnegan, E. J., Peacock, W. J., and Dennis, E. S. (1996). Reduced DNA methylation in Arabidopsis thaliana results in abnormal plant development. Proc. Natl. Acad. Sci. U.S.A. 93, 8449–8454. doi: 10.1073/pnas.93.16.8449
Forgione, I., Wołoszyńska, M., Pacenza, M., Chiappetta, A., Greco, M., Araniti, F., et al. (2019). Hypomethylated drm1 drm2 cmt3 mutant phenotype of Arabidopsis thaliana is related to auxin pathway impairment. Plant Sci. 280, 383–396. doi: 10.1016/j.plantsci.2018.12.029
Friml, J., Benková, E., Blilou, I., Wisniewska, J., Hamann, T., Ljung, K., et al. (2002). AtPIN4 mediates sink-driven auxin gradients and root patterning in Arabidopsis. Cell 108, 661–673. doi: 10.1016/S0092-8674(02)00656-6
Friml, J., Vieten, A., Sauer, M., Weijers, D., Schwarz, H., Hamann, T., et al. (2003). Efflux-dependent auxin gradients establish the apical–basal axis of Arabidopsis. Nature 426, 147–153. doi: 10.1038/nature02085
Furner, I. J., and Matzke, M. (2011). Methylation and demethylation of the Arabidopsis genome. Curr. Opin. Plant Biol. 14, 137–141. doi: 10.1016/j.pbi.2010.11.004
Gaj, M. D., Zhang, S., Harada, J. J., and Lemaux, P. G. (2005). Leafy cotyledon genes are essential for induction of somatic embryogenesis of Arabidopsis. Planta 222, 977–988. doi: 10.1007/s00425-005-0041-y
Gallego-Bartolomé, J., Liu, W., Kuo, P. H., Feng, S., Ghoshal, B., Gardiner, J., et al. (2019). Co-targeting RNA polymerases IV and V promotes efficient de novo dna methylation in Arabidopsis. Cell 176, 1068.e–1082.e. doi: 10.1016/j.cell.2019.01.029
Gehring, M. (2019). Epigenetic dynamics during flowering plant reproduction: evidence for reprogramming? New Phytol. 1, 91–96. doi: 10.1111/nph.15856
Gehring, M., Bubb, K. L., and Henikoff, S. (2009). Extensive demethylation of repetitive elements during seed development underlies gene imprinting. Science 324, 1447–1451. doi: 10.1126/science.1171609
Gehring, M., Huh, J. H., Hsieh, T.-F., Penterman, J., Choi, Y., Harada, J. J., et al. (2006). DEMETER DNA glycosylase establishes MEDEA polycomb gene self-imprinting by allele-specific demethylation. Cell 124, 495–506. doi: 10.1016/j.cell.2005.12.034
Gliwicka, M., Nowak, K., Balazadeh, S., Mueller-Roeber, B., and Gaj, M. D. (2013). Extensive modulation of the transcription factor transcriptome during somatic embryogenesis in Arabidopsis thaliana. PLoS One 8:e69261. doi: 10.1371/journal.pone.0069261
Gong, Z., Morales-Ruiz, T., Ariza, R. R., Roldán-Arjona, T., David, L., and Zhu, J.-K. (2002). ROS1, a repressor of transcriptional gene silencing in Arabidopsis, encodes a DNA glycosylase/lyase. Cell 111, 803–814. doi: 10.1016/S0092-8674(02)01133-9
Groß-Hardt, R., Kägi, C., Baumann, N., Moore, J. M., Baskar, R., Gagliano, W. B., et al. (2007). LACHESIS restricts gametic cell fate in the female gametophyte of Arabidopsis. PLoS Biol. 5:e47. doi: 10.1371/journal.pbio.0050047
Grzybkowska, D., Morończyk, J., Wójcikowska, B., and Gaj, M. D. (2018). Azacitidine (5-AzaC)-treatment and mutations in DNA methylase genes affect embryogenic response and expression of the genes that are involved in somatic embryogenesis in Arabidopsis. Plant Growth Regul. 85, 243–256. doi: 10.1007/s10725-018-0389-1
Guillaumot, D., Lopez-Obando, M., Baudry, K., Avon, A., Rigaill, G., Falcon de Longevialle, A., et al. (2017). Two interacting PPR proteins are major Arabidopsis editing factors in plastid and mitochondria. Proc. Natl. Acad. Sci. U.S.A. 114, 8877–8882. doi: 10.1073/pnas.1705780114
Haag, J. R., Ream, T. S., Marasco, M., Nicora, C. D., Norbeck, A. D., Pasa-Tolic, L., et al. (2012). In vitro transcription activities of Pol IV. Pol V, and RDR2 reveal coupling of Pol IV and RDR2 for dsRNA synthesis in plant RNA silencing. Mol. Cell 48, 811–818. doi: 10.1016/j.molcel.2012.09.027
Han, Q., Bartels, A., Cheng, X., Meyer, A., An, Y. C., Hsieh, T.-F., et al. (2019). Epigenetics regulates reproductive development in plants. Plants 8:564. doi: 10.3390/plants8120564
Hand, M. L., de Vries, S., and Koltunow, A. M. (2016). A comparison of in vitro and in vivo asexual embryogenesis. Methods Mol. Biol. 1359, 3–23. doi: 10.1007/978-1-4939-3061-6_1
Harada, J. J. (2001). Role of Arabidopsis LEAFY COTYLEDON genes in seed development. J. Plant Physiol. 158, 405–409. doi: 10.1078/0176-1617-00351
Havecker, E. R., Wallbridge, L. M., Hardcastle, T. J., Bush, M. S., Kelly, K. A., Dunn, R. M., et al. (2010). The Arabidopsis RNA-Directed DNA methylation argonautes functionally diverge based on their expression and interaction with target loci. Plant Cell 22, 321–334. doi: 10.1105/tpc.109.072199
Hecht, V., Vielle-Calzada, J.-P., Hartog, M. V., Schmidt, E. D., Boutilier, K., Grossniklaus, U., et al. (2001). The Arabidopsis SOMATIC EMBRYOGENESIS RECEPTOR KINASE 1 gene is expressed in developing ovules and embryos and enhances embryogenic competence in culture. Plant Physiol. 127, 803–816. doi: 10.1104/pp.127.3.803
Hernández-Lagana, E., Rodríguez-Leal, D., Lúa, J., and Vielle-Calzada, J.-P. (2016). A multigenic network of ARGONAUTE4 clade members controls early megaspore formation in Arabidopsis. Genetics 204, 1045–1056. doi: 10.1534/genetics.116.188151
Hofmann, F., Schon, M. A., and Nodine, M. D. (2019). The embryonic transcriptome of Arabidopsis thaliana. Plant Reprod. 32, 77–91. doi: 10.1007/s00497-018-00357-2
Hua, X., Berkowitz, N. D., Willmann, M. R., Yu, X., Lyons, E., and Gregory, B. D. (2021). Global analysis of RNA-dependent RNA polymerase-dependent small RNAs reveals new substrates and functions for these proteins and SGS3 in Arabidopsis. Non Coding RNA 7:28. doi: 10.3390/ncrna7020028
Huh, S. U., Lee, S.-B., Kim, H. H., and Paek, K.-H. (2012). ATAF2, a NAC transcription factor, binds to the promoter and regulates NIT2 gene expression involved in auxin biosynthesis. Mol. Cells 34, 305–313. doi: 10.1007/s10059-012-0122-2
Ibarra, C. A., Feng, X., Schoft, V. K., Hsieh, T.-F., Uzawa, R., Rodrigues, J. A., et al. (2012). Active DNA demethylation in plant companion cells reinforces transposon methylation in gametes. Science 337, 1360–1364. doi: 10.1126/science.1224839
Ingouff, M., Selles, B., Michaud, C., Vu, T. M., Berger, F., Schorn, A. J., et al. (2017). Live-cell analysis of DNA methylation during sexual reproduction in Arabidopsis reveals context and sex-specific dynamics controlled by noncanonical RdDM. Genes Dev. 31, 72–83. doi: 10.1101/gad.289397.116
Iwasaki, M., and Paszkowski, J. (2014). Epigenetic memory in plants. EMBO J. 33, 1987–1998. doi: 10.15252/embj.201488883
Jacobsen, S. E., Sakai, H., Finnegan, E. J., Cao, X., and Meyerowitz, E. M. (2000). Ectopic hypermethylation of flower-specific genes in Arabidopsis. Curr. Biol. 10, 179–186. doi: 10.1016/S0960-9822(00)00324-9
Ji, L., Mathioni, S. M., Johnson, S., Tucker, D., Bewick, A. J., Do Kim, K., et al. (2019). Genome-wide reinforcement of DNA methylation occurs during somatic embryogenesis in soybean. Plant Cell 31, 2315–2331. doi: 10.1105/tpc.19.00255
Jiang, F., Xu, X., Liu, H., and Zhu, J. (2015). DRM1 and DRM2 are involved in Arabidopsis callus formation. Plant Cell. Tissue Organ Cult. 123, 221–228. doi: 10.1007/s11240-015-0812-5
Jin, F., Hu, L., Yuan, D., Xu, J., Gao, W., He, L., et al. (2014). Comparative transcriptome analysis between somatic embryos (SEs) and zygotic embryos in cotton: evidence for stress response functions in SE development. Plant Biotechnol. J. 12, 161–173. doi: 10.1111/pbi.12123
Jones-Rhoades, M. W., Borevitz, J. O., and Preuss, D. (2007). Genome-wide expression profiling of the Arabidopsis female gametophyte identifies families of small, secreted proteins. PLoS Genet. 3, 1848–1861. doi: 10.1371/journal.pgen.0030171
Jullien, P. E., and Berger, F. (2010). Parental genome dosage imbalance deregulates imprinting in Arabidopsis. PLoS Genet. 6:e1000885. doi: 10.1371/journal.pgen.1000885
Jullien, P. E., Susaki, D., Yelagandula, R., Higashiyama, T., and Berger, F. (2012). DNA methylation dynamics during sexual reproduction in Arabidopsis thaliana. Curr. Biol. 22, 1825–1830. doi: 10.1016/j.cub.2012.07.061
Karim, R., Tan, Y. S., Singh, P., Khalid, N., and Harikrishna, J. A. (2018). Expression and DNA methylation of SERK. BBM, LEC2 and WUS genes in in vitro cultures of Boesenbergia rotunda (L.) Mansf. Physiol. Mol. Biol. Plants 24, 741–751. doi: 10.1007/s12298-018-0566-8
Kawashima, T., and Berger, F. (2014). Epigenetic reprogramming in plant sexual reproduction. Nat. Rev. Genet. 15, 613–624. doi: 10.1038/nrg3685
Kinoshita, T., Miura, A., Choi, Y., Kinoshita, Y., Cao, X., Jacobsen, S. E., et al. (2004). One-way control of FWA imprinting in Arabidopsis endosperm by DNA methylation. Science 303, 521–523. doi: 10.1126/science.1089835
Kondo, H., Ozaki, H., Itoh, K., Kato, A., and Takeno, K. (2006). Flowering induced by 5-azacytidine, a DNA demethylating reagent in a short-day plant, Perilla frutescens var. crispa. Physiol. Plant. 127, 130–137. doi: 10.1111/j.1399-3054.2005.00635.x
Kuo, H. Y., Jacobsen, E. L., Long, Y., Chen, X., and Zhai, J. (2017). Characteristics and processing of Pol IV-dependent transcripts in Arabidopsis. J. Genet. Genomics 44, 3–6. doi: 10.1016/j.jgg.2016.10.009
Kurczyńska, E. U., Gaj, M. D., Ujczak, A., and Mazur, E. (2007). Histological analysis of direct somatic embryogenesis in Arabidopsis thaliana (L.) Heynh. Planta 226, 619–628. doi: 10.1007/s00425-007-0510-6
Leljak-Levanić, D., Bauer, N., Mihaljević, S., and Jelaska, S. (2004). Somatic embryogenesis in pumpkin (Cucurbita pepo L.): control of somatic embryo development by nitrogen compounds. J. Plant Physiol. 161, 229–236. doi: 10.1078/0176-1617-01055
Leljak-Levanić, D., Mihaljević, S., and Bauer, N. (2015). Somatic and zygotic embryos share common developmental features at the onset of plant embryogenesis. Acta Physiol. Plant. 37, 1–14. doi: 10.1007/s11738-015-1875-y
Li, E. (2002). Chromatin modification and epigenetic reprogramming in mammalian development. Nat. Rev. Genet. 3, 662–673. doi: 10.1038/nrg887
Li, E., Bestor, T. H., and Jaenisch, R. (1992). Targeted mutation of the DNA methyltransferase gene results in embryonic lethality. Cell 69, 915–926. doi: 10.1016/0092-8674(92)90611-F
Lin, J.-Y., Le, B. H., Chen, M., Henry, K. F., Hur, J., Hsieh, T. F., et al. (2017). Similarity between soybean and and Arabidopsis seed methylomes and loss of non-CG methylation does not affect seed development. Proc. Natl. Acad. Sci 114, E9730–E9739. doi: 10.1073/pnas.1716758114
Liu, W., Duttke, S. H., Hetzel, J., Groth, M., Feng, S., Gallego-Bartolomé, J., et al. (2018). RNA-directed DNA methylation involves co-transcriptional small-RNA-guided slicing of polymerase V transcripts in Arabidopsis. Nat. Plants 4, 181–188. doi: 10.1038/s41477-017-0100-y
Liu, Z.-W., Shao, C.-R., Zhang, C.-J., Zhou, J.-X., Zhang, S.-W., Li, L., et al. (2014). The SET domain proteins SUVH2 and SUVH9 are required for Pol V occupancy at RNA-directed DNA methylation loci. PLoS Genet. 10:e1003948. doi: 10.1371/journal.pgen.1003948
LoSchiavo, F., Pitto, L., Giuliano, G., Torti, G., Nuti-Ronchi, V., Marazziti, D., et al. (1989). DNA methylation of embryogenic carrot cell cultures and its variations as caused by mutation, differentiation, hormones and hypomethylating drugs. Theor. Appl. Genet. 77, 325–331. doi: 10.1007/BF00305823
Lotan, T., Ohto, M., Yee, K. M., West, M. A., Lo, R., Kwong, R. W., et al. (1998). Arabidopsis LEAFY COTYLEDON1 is sufficient to induce embryo development in vegetative cells. Cell 93, 1195–1205. doi: 10.1016/S0092-8674(00)81463-4
Loyola-Vargas, V. M., and Ochoa-Alejo, N. (2016). “Somatic embryogenesis. An overview,” in Somatic Embryogenesis: Fundamental Aspects and Applications, eds V. Loyola-Vargas and N. Ochoa-Alejo (Cham: Springer), 1–8. doi: 10.1007/978-3-319-33705-0_1
Lukowitz, W., Roeder, A., Parmenter, D., and Somerville, C. (2004). A MAPKK kinase gene regulates extra-embryonic cell fate in Arabidopsis. Cell 116, 109–119. doi: 10.1016/S0092-8674(03)01067-5
Martienssen, R. A., and Colot, V. (2001). DNA methylation and epigenetic inheritance in plants and filamentous fungi. Science 293, 1070–1074. doi: 10.1126/science.293.5532.1070
Massonneau, A., Coronado, M.-J., Audran, A., Bagniewska, A., Mòl, R., Testillano, P. S., et al. (2005). Multicellular structures developing during maize microspore culture express endosperm and embryo-specific genes and show different embryogenic potentialities. Eur. J. Cell Biol. 84, 663–675. doi: 10.1016/j.ejcb.2005.02.002
Matthys-Rochon, E. (2005). Secreted molecules and their role in embryo formation in plants: a min-review. Acta Biol. Cracoviensia 47, 23–29.
Matzke, M. A., Kanno, T., and Matzke, A. J. (2015). RNA-Directed DNA methylation: the evolution of a complex epigenetic pathway in flowering plants. Annu. Rev. Plant Biol. 66, 243–267. doi: 10.1146/annurev-arplant-043014-114633
Mayer, U., Buttner, G., and Jurgens, G. (1993). Apical-basal pattern formation in the Arabidopsis embryo: studies on the role of the gnom gene. Development 117, 149–162. doi: 10.1242/dev.117.1.149
McDonald, J. I., Celik, H., Rois, L. E., Fishberger, G., Fowler, T., Rees, R., et al. (2016). Reprogrammable CRISPR/Cas9-based system for inducing site-specific DNA methylation. Biol. Open. 5, 866–874. doi: 10.1242/bio.019067
Meinke, D. W. (2019). Genome-wide identification of EMBRYO-DEFECTIVE (EMB) genes required for growth and development in Arabidopsis. New Phytol. 226, 306–325. doi: 10.1111/nph.16071
Mendes, M. A., Petrella, R., Cucinotta, M., Vignati, E., Gatti, S., Pinto, S. C., et al. (2020). The RNA-dependent DNA methylation pathway is required to restrict SPOROCYTELESS/NOZZLE expression to specify a single female germ cell precursor in Arabidopsis. Development 147:dev194274. doi: 10.1242/dev.194274
Mercé, C., Bayer, P. E., Tay Fernandez, C., Batley, J., and Edwards, D. (2020). Induced methylation in plants as a crop improvement tool: progress and perspectives. Agronomy 10:1484. doi: 10.3390/agronomy10101484
Möller, B., and Weijers, D. (2009). Auxin control of embryo patterning. Cold Spring Harb. Perspect. Biol. 1:a001545. doi: 10.1101/cshperspect.a001545
Muñoz-Nortes, T., Candela, H., and Micol, J. L. (2017). Suitability of two distinct approaches for the high-throughput study of the post-embryonic effects of embryo-lethal mutations in Arabidopsis. Sci. Rep. 7:17010. doi: 10.1038/s41598-017-17218-z
Nic-Can, G. I., and De la Peña, C. (2014). Epigenetic Advances on Somatic Embryogenesis of Agronomical and Important Crops. In: Epigenetics in Plants of Agronomic Importance: Fundamentals and Applications. Cham: Springer. doi: 10.1007/978-3-319-07971-4_6
Nishiwaki, M., Fujino, K., Koda, Y., Masuda, K., and Kikuta, Y. (2000). Somatic embryogenesis induced by the simple application of abscisic acid to carrot (Daucus carota L.) seedlings in culture. Planta 211, 756–759. doi: 10.1007/s004250000387
Noceda, C., Salaj, T., Pérez, M., Viejo, M., Cañal, M. J., Salaj, J., et al. (2009). DNA demethylation and decrease on free polyamines is associated with the embryogenic capacity of Pinus nigra Arn. cell culture. Trees 23, 1285–1293. doi: 10.1007/s00468-009-0370-8
Olmedo-Monfil, V., Durán-Figueroa, N., Arteaga-Vázquez, M., Demesa-Arévalo, E., Autran, D., Grimanelli, D., et al. (2010). Control of female gamete formation by a small RNA pathway in Arabidopsis. Nature 464, 628–632. doi: 10.1038/nature08828
Osorio-Montalvo, P., Sáenz-Carbonell, L., and De-la-Peña, C. (2018). 5-Azacytidine: a promoter of epigenetic changes in the quest to improve plant somatic embryogenesis. Int. J. Mol. Sci. 19:3182. doi: 10.3390/ijms19103182
Papikian, A., Liu, W., Gallego-Bartolomé, J., and Jacobsen, S. E. (2019). Site-specific manipulation of Arabidopsis loci using CRISPR-Cas9 SunTag systems. Nat. Commun. 10:729. doi: 10.1038/s41467-019-08736-7
Park, K., Kim, M. Y., Vickers, M., Park, J.-S., Hyun, Y., Okamoto, T., et al. (2016). DNA demethylation is initiated in the central cells of Arabidopsis and rice. Proc. Natl. Acad. Sci. U.S.A. 113, 15138–15143. doi: 10.1073/pnas.1619047114
Park, K., Lee, S., Yoo, H., and Choi, Y. (2020). DEMETER-mediated DNA demethylation in gamete companion cells and the endosperm, and its possible role in embryo development in Arabidopsis. J. Plant Biol. 63, 321–329. doi: 10.1007/s12374-020-09258-2
Peer, W. A., and Murphy, A. S. (2007). Flavonoids and auxin transport: modulators or regulators? Trends Plant Sci. 12, 556–563. doi: 10.1016/j.tplants.2007.10.003
Penterman, J., Zilberman, D., Huh, J. H., Ballinger, T., Henikoff, S., and Fischer, R. L. (2007). DNA demethylation in the Arabidopsis genome. Proc. Natl. Acad. Sci. U.S.A. 104, 6752–6757. doi: 10.1073/pnas.0701861104
Pila Quinga, L. A., Pacheco de Freitas Fraga, H., do Nascimento Vieira, L., and Guerra, M. P. (2017). DNA methylation and recovery of embryogenic potential. Plant Cell. Tissue Organ Cult. 131, 295–305. doi: 10.1007/s11240-017-1284-6
Pillot, M., Baroux, C., Vazquez, M. A., Autran, D., Leblanc, O., Vielle-Calzada, J. P., et al. (2010). Embryo and endosperm inherit distinct chromatin and transcriptional states from the female gametes in Arabidopsis. Plant Cell 22, 307–320. doi: 10.1105/tpc.109.071647
Qi, Y., Denli, A. M., and Hannon, G. J. (2005). Biochemical specialization within Arabidopsis RNA silencing pathways. Mol. Cell 19, 421–428.
Qi, Y., He, X., Wang, X.-J., Kohany, O., Jurka, J., and Hannon, G. J. (2006). Distinct catalytic and non-catalytic roles of ARGONAUTE4 in RNA-directed DNA methylation. Nature 443, 1008–1012. doi: 10.1038/nature05198
Rajkumar, M. S., Gupta, K., Khemka, N. K., Garg, R., and Jain, M. (2020). DNA methylation reprogramming during seed development and its functional relevance in seed size/weight determination in chickpea. Commun. Biol. 3:340. doi: 10.1038/s42003-020-1059-1
Robert, H. S., Grones, P., Stepanova, A. N., Robles, L. M., Lokerse, A. S., Alonso, J. M., et al. (2013). Local auxin sources orient the apical-basal axis in Arabidopsis embryos. Curr. Biol. 23, 2506–2512. doi: 10.1016/j.cub.2013.09.039
Schmid, M. W., Giraldo-Fonseca, A., Rövekamp, M., Smetanin, D., Bowman, J. L., and Grossniklaus, U. (2018). Extensive epigenetic reprogramming during the life cycle of Marchantia polymorpha. Genome Biol. 19:9. doi: 10.1186/s13059-017-1383-z
Schmidt, E. D., Guzzo, F., Toonen, M. A., and de Vries, S. C. (1997). A leucine-rich repeat containing receptor-like kinase marks somatic plant cells competent to form embryos. Development 124, 2049–2062. doi: 10.1242/dev.124.10.2049
Schmitz, R. J., He, Y., Valdés-López, O., Khan, S. M., Joshi, T., Urich, M. A., et al. (2013). Epigenome-wide inheritance of cytosine methylation variants in a recombinant inbred population. Genome Res. 23, 1663–1674. doi: 10.1101/gr.152538.112
Schoft, V. K., Chumak, N., Choi, Y., Hannon, M., Garcia-Aguilar, M., Machlicova, A., et al. (2011). Function of the DEMETER DNA glycosylase in the Arabidopsis thaliana male gametophyte. Proc. Natl. Acad. Sci. U.S.A. 108, 8042–8047. doi: 10.1073/pnas.1105117108
Shibukawa, T., Yazawa, K., Kikuchi, A., and Kamada, H. (2009). Possible involvement of DNA methylation on expression regulation of carrot LEC1 gene in its 5’-upstream region. Gene 437, 22–31. doi: 10.1016/j.gene.2009.02.011
Sprunck, S., Hackenberg, T., Englhart, M., and Vogler, F. (2014). Same same but different: sperm-activating EC1 and ECA1 gametogenesis-related family proteins. Biochem. Soc. Trans. 42, 401–407. doi: 10.1042/BST20140039
Sprunck, S., Rademacher, S., Vogler, F., Gheyselinck, J., Grossniklaus, U., and Dresselhaus, T. (2012). Egg cell–secreted EC1 triggers sperm cell activation during double fertilization. Science 338, 1093–1097. doi: 10.1126/science.1223944
Stone, S. L., Braybrook, S. A., Paula, S. L., Kwong, L. W., Meuser, J., Pelletier, J., et al. (2008). Arabidopsis LEAFY COTYLEDON2 induces maturation traits and auxin activity: implications for somatic embryogenesis. Proc. Natl. Acad. Sci. U.S.A. 105, 3151–3156. doi: 10.1073/pnas.0712364105
Stroud, H., Do, T., Du, J., Zhong, X., Feng, S., Johnson, L., et al. (2014). Non-CG methylation patterns shape the epigenetic landscape in Arabidopsis. Nat. Struct. Mol. Biol. 21, 64–72. doi: 10.1038/nsmb.2735
Su, Y. H., Zhao, X. Y., Liu, Y. B., Zhang, C. L., O’Neill, S. D., and Zhang, X. S. (2009). Auxin-induced WUS expression is essential for embryonic stem cell renewal during somatic embryogenesis in Arabidopsis. Plant J. 59, 448–460. doi: 10.1111/j.1365-313X.2009.03880.x
Tang, K., Lang, Z., Zhang, H., and Zhu, J.-K. (2016). The DNA demethylase ROS1 targets genomic regions with distinct chromatin modifications. Nat. Plants 2:16169. doi: 10.1038/nplants.2016.169
Tao, Z., Shen, L., Gu, X., Wang, J., Yu, H., He, Y., et al. (2017). Embryonic epigenetic reprogramming by a pioneer transcription factor in plants. Nature 551, 124–128. doi: 10.1038/nature24300
Tedeschi, F., Rizzo, P., Huong, B. T. M., Czihal, A., Rutten, T., Altschmied, L., et al. (2019). EFFECTOR OF TRANSCRIPTION factors are novel plant-specific regulators associated with genomic DNA methylation in Arabidopsis. New Phytol 221, 261–278. doi: 10.1111/nph.15439
Ueda, M., Aichinger, E., Gong, W., Groot, E., Verstraeten, I., Vu, L. D., et al. (2017). Transcriptional integration of paternal and maternal factors in the Arabidopsis zygote. Genes Dev. 31, 617–627. doi: 10.1101/gad.292409.116
Ueda, M., Zhang, Z., and Laux, T. (2011). Transcriptional activation of Arabidopsis axis patterning genes WOX8/9 links zygote polarity to embryo development. Dev. Cell 20, 264–270. doi: 10.1016/j.devcel.2011.01.009
Vasilenko, A., McDaniel, J. K., and Conger, B. V. (2000). Ultrastructural analyses of somatic embryo initiation, development and polarity establishment from mesophyll cells of Dactylis glomerata. Vitr. Cell. Dev. Biol. Plant 36, 51–56. doi: 10.1007/s11627-000-0012-8
Verdeil, J.-L., Alemanno, L., Niemenak, N., and Tranbarger, T. J. (2007). Pluripotent versus totipotent plant stem cells: dependence versus autonomy? Trends Plant Sci. 12, 245–252. doi: 10.1016/j.tplants.2007.04.002
Vlachonasios, K. E., Thomashow, M. F., and Triezenberg, S. J. (2003). Disruption mutations of ADA2b and GCN5 transcriptional adaptor genes dramatically affect Arabidopsis growth, development, and gene expression. Plant Cell 15, 626–638. doi: 10.1105/tpc.007922
Vrinten, P. L., Nakamura, T., and Kasha, K. J. (1999). Characterization of cDNAs expressed in the early stages of microspore embryogenesis in barley (Hordeum vulgare) L. Plant Mol. Biol. 41, 455–463. doi: 10.1023/A:1006383724443
Walker, J., Gao, H., Zhang, J., Aldridge, B., Vickers, M., Higgins, J. D., et al. (2017). Sexual-lineage-specific DNA methylation regulates meiosis in Arabidopsis. Nat. Genet. 50, 130–137. doi: 10.1038/s41588-017-0008-5
Wang, F., and Axtell, M. J. (2017). AGO4 is specifically required for heterochromatic siRNA accumulation at Pol V-dependent loci in Arabidopsis thaliana. Plant J. 90, 37–47. doi: 10.1111/tpj.13463
Wierzbicki, A. T., Haag, J. R., and Pikaard, C. S. (2008). Noncoding transcription by RNA polymerase Pol IVb/Pol V mediates transcriptional silencing of overlapping and adjacent genes. Cell 135, 635–648. doi: 10.1016/j.cell.2008.09.035
Wierzbicki, A. T., Ream, T. S., Haag, J. R., and Pikaard, C. S. (2009). RNA polymerase V transcription guides ARGONAUTE4 to chromatin. Nat. Genet. 41, 630–634. doi: 10.1038/ng.365
Willemsen, V., and Scheres, B. (2004). Mechanisms of pattern formation in plant embryogenesis. Annu. Rev. Genet. 587–614. doi: 10.1146/annurev.genet.38.072902.092231
Wójcikowska, B., Jaskóła, K., Ga̧siorek, P., Meus, M., Nowak, K., and Gaj, M. D. (2013). LEAFY COTYLEDON2 (LEC2) promotes embryogenic induction in somatic tissues of Arabidopsis, via YUCCA-mediated auxin biosynthesis. Planta 238, 425–440. doi: 10.1007/s00425-013-1892-2
Wu, Y., Meeley, R. B., and Cosgrove, D. J. (2001). Analysis and expression of the α-expansin and β-expansin gene families in maize. Plant Physiol. 126, 222–232. doi: 10.1104/pp.126.1.222
Xiao, W., Custard, K. D., Brown, R. C., Lemmon, B. E., Harada, J. J., Goldberg, R. B., et al. (2006). DNA methylation is critical for Arabidopsis embryogenesis and seed viability. Plant Cell 18, 805–814. doi: 10.1105/tpc.105.038836
Xiao, W., Gehring, M., Choi, Y., Margossian, L., Pu, H., Harada, J. J., et al. (2003). Imprinting of the MEA Polycomb gene is controlled by antagonism between MET1 methyltransferase and DME glycosylase. Dev. Cell 5, 891–901. doi: 10.1016/S1534-5807(03)00361-7
Xiong, L., Ishitani, M., Lee, H., and Zhu, J.-K. (2001). The Arabidopsis LOS5/ABA3 locus encodes a molybdenum cofactor sulfurase and modulates cold stress– and osmotic stress–responsive gene expression. Plant Cell 13, 2063–2083. doi: 10.1105/TPC.010101
Xu, R., and Li, Q. Q. (2003). A RING-H2 zinc-finger protein gene RIE1 is essential for seed development in Arabidopsis. Plant Mol. Biol. 53, 37–50. doi: 10.1023/B:PLAN.0000009256.01620.a6
Xu, X. M., and Møller, S. G. (2011). Iron-sulfur clusters: biogenesis, molecular mechanisms, and their functional significance. Antioxid. Redox Signal. 15, 271–307. doi: 10.1089/ars.2010.3259
Yamamoto, N., Kobayashi, H., Togashi, T., Mori, Y., Kikuchi, K., Kuriyama, K., et al. (2005). Formation of embryogenic cell clumps from carrot epidermal cells is suppressed by 5-azacytidine, a DNA methylation inhibitor. J. Plant Physiol. 162, 47–54. doi: 10.1016/j.jplph.2004.05.013
Yang, K.-J., Guo, L., Hou, X.-L., Gong, H.-Q., and Liu, C.-M. (2017). ZYGOTE-ARREST 3 that encodes the tRNA ligase is essential for zygote division in Arabidopsis. J. Integr. Plant Biol. 59, 680–692. doi: 10.1111/jipb.12561
You, W., Tyczewska, A., Spencer, M., Daxinger, L., Schmid, M. W., Grossniklaus, U., et al. (2012). Atypical DNA methylation of genes encoding cysteine-rich peptides in Arabidopsis thaliana. BMC Plant Biol. 12:51. doi: 10.1186/1471-2229-12-51
Yu, T.-Y., Shi, D.-Q., Jia, P.-F., Tang, J., Li, H.-J., Liu, J., et al. (2016). The Arabidopsis receptor kinase ZAR1 is required for zygote asymmetric division and its daughter cell fate. PLoS Genet. 12:e1005933. doi: 10.1371/journal.pgen.1005933
Zhang, H., Lang, Z., and Zhu, J.-K. (2018). Dynamics and function of DNA methylation in plants. Nat. Rev. Mol. Cell Biol. 19, 489–506. doi: 10.1038/s41580-018-0016-z
Zhang, X., and Jacobsen, S. E. (2006). Genetic analyses of DNA methyltransferases in Arabidopsis thaliana. Cold Spring Harb. Symp. Quant. Biol. 71, 439–447. doi: 10.1101/sqb.2006.71.047
Zhao, Y. (2012). Auxin biosynthesis: a simple two-step pathway converts tryptophan to indole-3-acetic acid in plants. Mol. Plant 5, 334–338. doi: 10.1093/mp/ssr104
Zhong, X. (2016). Comparative epigenomics: a powerful tool to understand the evolution of DNA methylation. New Phytol. 210, 76–80. doi: 10.1111/nph.13540
Zhong, X., Du, J., Hale, C. J., Gallego-Bartolomé, J., Feng, S., Vashisht, A. A., et al. (2014). Molecular mechanism of action of plant DRM de novo DNA methyltransferases. Cell 157, 1050–1060. doi: 10.1016/j.cell.2014.03.056
Keywords: DNA methylation, RdDM, plant embryogenesis, zygotic embryogenesis, somatic embryogenesis, RNA polymerase V, Arabidopsis thaliana
Citation: Markulin L, Škiljaica A, Tokić M, Jagić M, Vuk T, Bauer N and Leljak Levanić D (2021) Taking the Wheel – de novo DNA Methylation as a Driving Force of Plant Embryonic Development. Front. Plant Sci. 12:764999. doi: 10.3389/fpls.2021.764999
Received: 26 August 2021; Accepted: 13 October 2021;
Published: 29 October 2021.
Edited by:
Paloma Moncaleán, Neiker-Tecnalia, SpainReviewed by:
Markus Kuhlmann, Leibniz Institute of Plant Genetics and Crop Plant Research (IPK), GermanyCélia M. Miguel, University of Lisbon, Portugal
Joseph Colasanti, University of Guelph, Canada
Copyright © 2021 Markulin, Škiljaica, Tokić, Jagić, Vuk, Bauer and Leljak Levanić. This is an open-access article distributed under the terms of the Creative Commons Attribution License (CC BY). The use, distribution or reproduction in other forums is permitted, provided the original author(s) and the copyright owner(s) are credited and that the original publication in this journal is cited, in accordance with accepted academic practice. No use, distribution or reproduction is permitted which does not comply with these terms.
*Correspondence: Dunja Leljak Levanić, ZHVuamFAemcuYmlvbC5wbWYuaHI=
†These authors have contributed equally to this work and share first authorship