- 1Collaborative Innovation Center of Modern Biological Breeding, Henan Institute of Science and Technology, Xinxiang, China
- 2State Key Laboratory of Cotton Biology, Institute of Cotton Research of CAAS, Anyang, China
The KNOX (KNOTTED1-like homeobox) transcription factors play an important role in leaf, shoot apical meristem and seed development and respond to biotic and abiotic stresses. In this study, we analyzed the diversity and evolutionary history of the KNOX gene family in the genome of tetraploid cotton (Gossypium hirsutum). Forty-four putative KNOX genes were identified. All KNOX genes from seven higher plant species were classified into KNOXI, KNOXII, and KNATM clades based on a phylogenetic analysis. Chromosomal localization and collinearity analysis suggested that whole-genome duplication and a polyploidization event contributed to the expansion of the cotton KNOX gene family. Analyses of expression profiles revealed that the GhKNOX genes likely responded to diverse stresses and were involved in cotton growth developmental processes. Silencing of GhKNOX2 enhanced the salt tolerance of cotton seedlings, whereas silencing of GhKNOX10 and GhKNOX14 reduced seedling tolerance to salt stress. Silencing of GhSTM3 influenced the cotton flowering time and plant development. These findings clarify the evolution of the cotton KNOX gene family and provide a foundation for future functional studies of KNOX proteins in cotton growth and development and response to abiotic stresses.
Introduction
Cotton (Gossypium spp.) is the most important natural fiber source worldwide. Gossypium hirsutum, known as allotetraploid cotton, is among the most widely cultivated species and accounts for more than 90% of the global textile fiber production. The genome of G. hirsutum comprises the diploid A genome (Gossypium arboreum) and diploid D genome (Gossypium raimondii) derived from ancestral allopolyploidization (Zhang et al., 2015; Huang et al., 2020). The assembly of complete genome sequences for Gossypium species has provided substantial raw data, as well as a novel perspective of evolutionary conservation, divergence, and innovation in gene function in cotton.
Transcription factors are essential for the control of gene expression in plant developmental processes, such as leaf and floral development (McGarry et al., 2016; Zhang et al., 2016; Cheng et al., 2021), fiber elongation (Pei, 2015; Sun et al., 2019), biotic and abiotic stress responses (Shah et al., 2013; Yang et al., 2017), and hormone regulation (Li et al., 2014; Fiene et al., 2017). KNOTTED1-like homeobox (KNOX) genes belong to the three-amino-acid-loop-extension (TALE) superfamily and encode KNOX proteins with KNOXI, KNOXII, ELK, and homeobox KN binding domains (Bhatt et al., 2004). The first KNOTTED1-like homeobox gene to be identified, Knotted 1 (Kn1), was isolated from a maize mutant (Smith et al., 1992). Additional KNOX homolog genes were identified from Arabidopsis and other plant species (Hareven et al., 1996; Long et al., 1996; Nadakuduti et al., 2014; Di Giacomo et al., 2017). On the basis of the similarities in the homodomain structure, Arabidopsis KNOX family genes can be divided into KNOXI, KNOXII, and KNATM clades. In Arabidopsis, KNOXI clade genes comprise SHOOT MERISTEMLESS (STM), KNAT1, KNAT2, and KNAT6. STM plays an important role in maintenance of apical meristem differentiation and floral development (Takano et al., 2010; Spinelli et al., 2011), broadened plant cell organwide growth and increased leaf complexity (Kierzkowski et al., 2019), and also regulates multiple floral fate genes (Roth et al., 2018). Arabidopsis fiber development is promoted by the plant hormone gibberellin and KNAT1, which is repressed by DELLA proteins (Felipo-Benavent et al., 2018). KNAT2 plays an important role in carpel development (Pautot et al., 2001). The knat6 mutation enhances the phenotype of the stm-2 mutant, and reveals that KNAT6 is involved in SAM maintenance and boundary establishment and modulates lateral root formation through the STM/CUC pathway (Dean et al., 2004; Belles-Boix et al., 2006). Expression of KNAT2 and KNAT6 may be restricted by the BP and PNY proteins to promote correct inflorescence development (Ragni et al., 2008). KNOXII clade genes of Arabidopsis comprise KNAT3, KNAT4, KNAT5, and KNAT7, which show diverse expression patterns in different organs, including roots, leaves, stems, and flowers (Truernit et al., 2006). KNAT3 regulates seed germination and seedling growth through the abscisic acid signaling pathway (Kim et al., 2013a). KNAT7 interacts with OVATE FAMILY PROTEINS to influence secondary cell wall formation (Li et al., 2011, 2012), and orthologs of KNAT7 expressed to varying degrees in fibrous wood species may explain differences in wood development (Reyes-Rivera et al., 2017). In cotton, the KNAT7 homolog GhKNL1 may partially rescue the phenotype of the Arabidopsis knat7 mutant. GhKNL1 encodes a protein that interacts with GhBEL1-like proteins to form heterodimers to regulate fiber development (Gong et al., 2014; Ma et al., 2019). Arabidopsis KNATM, which lacks the homeobox KN domain, is expressed in proximal-lateral domains of organ primordia and at the boundary of mature organs, and functions in leaf proximal-distal patterning (Magnani and Hake, 2008).
Additional research has revealed that KNOX genes are involved in abiotic stress responses. In soybean, most KNOXII genes exhibit higher expression levels in response to saline stress and dehydration (Wang et al., 2021). GmSBH1, a member of the KNOXI clade, is involved in the response to high temperature and humidity stress in soybean seed development (Shu et al., 2015). In Populus, the KNOX homolog gene PagKNAT2/6b alters plant architecture and improves drought resistance (Song et al., 2021). Wheat LRD, a KNAT3 homolog gene, affects lateral root growth and grain size under water limitation (Placido et al., 2020). Although KNOX genes have been partly elucidated in plant development, and KNOX genes have been previously studied in cotton (Gong et al., 2014; He et al., 2021), our understanding of KNOX family members in cotton remains limited. In this study, we conducted a genome-wide analysis to identify 44 putative KNOX family members in G. hirsutum. In addition, gene expression patterns in specific tissues and in response to stress treatment were analyzed. A virus-induced gene silencing (VIGS) assay was used to study the function of GhKNOX genes. These results provide a basis for future investigations of the roles of KNOX proteins in plant development of cotton.
Materials and Methods
Sequence Identification
The complete G. hirsutum genome sequence data were obtained from COTTONGEN1 (Yu et al., 2014). The protein sequences of five additional plant species comprising Physcomitrella patens, Selaginella moellendorffii, Oryza sativa, Theobroma cacao, and Populus trichocarpa were retrieved from the JGI Phytozome database2 and Genbank database3. The amino acid sequences of KNOX proteins from Arabidopsis thaliana, which were used as query sequences to search for cotton KNOX ortholog proteins in local BLAST with BlastP (with an threshold value of E ≤ 1e-5), were accessed from TAIR 104. Then, the collected KNOX-like candidate proteins were subjected to SMART for further selection based on their conserved domain5.
Conserved Sequence and Phylogenetic Analysis
Multiple sequence alignment was performed with ClustalW6. The conserved KNOXI, KNOXII, and ELK domain sequences of cotton and Arabidopsis KNOX proteins were aligned. A phylogenetic tree was constructed from full-length KNOX amino acid sequences of seven plant species, using the neighbor-joining method combined with a bootstrap analysis and the Jones–Taylor–Thornton substitution model as implemented in MEGA7.0. Branch support was estimated by performing a bootstrap analysis with 1000 replicates (Tamura et al., 2007).
Chromosome Location and Gene Structural Features
Chromosome size and gene location information for GhKNOX genes were extracted from the gene annotations (gff3) file accessible from the Gossypium hirsutum genome. MapChart 2.2 software was used to determine the distribution of the genes on the G. hirsutum chromosomes. The exon and intron structure was displayed using the GSDS 2.0 online server7. The collinearity of gene pairs in the GhKNOX family were mapped to generate a collinearity map using Circos software.
Plant Growth and Stress Treatment
All upland cotton plants were grown in the field at the Henan Institute of Science and Technology. Different tissues were sampled from plants of the cultivar ‘TM-1.’ For stress treatments, seeds were sown in plastic pots under a 14 h light/10 h dark photoperiod at 28°C until the seedlings attained the second leaf expanded stage and were then treated with 20% polyethylene glycol 6000 (PEG) or 200 mM NaCl. Leaves were harvested at 0, 1, 3, 6, 12, and 24 h, immediately frozen in liquid nitrogen, and stored at –80°C for total RNA extraction. Shoot meristems were harvested from plants of the early maturing cultivar ‘Zao1’ and the late-maturing cultivar ‘CCRI50’ for RNA-sequencing (RNA-seq) from the fourth leaf expanded to the seventh leaf expanded stages. The raw reads were processed to retain only clean reads by removing the adaptor sequences, low-quality sequence reads (Q < 20), and poly-N stretches (>10%). The clean reads were mapped to the upland cotton reference genome to obtain unigenes using the Tophat2 software (Kim et al., 2013b). Expression of KNOX genes in different tissues and the cold, heat, salt and drought stress treatments was analyzed using raw RNA-seq data. The raw RNA-seq data were downloaded from the NCBI Sequence Read Archive8. The RNA-seq expression analysis was conducted using TopHat and Cufflinks. Gene expression was expressed as fragments per kilobase of transcripts per million mapped reads (FPKM). A heatmap was generated using TBtools (Chen et al., 2020).
RNA Isolation and Quantitative Real-Time PCR
Total RNA was isolated from samples using a plant RNA purification kit (Tiangen). The first-strand cDNA was synthesized using the PrimeScript™ 1st Strand cDNA Synthesis Kit for RT-PCR (TaKaRa). Transcript levels were determined by quantitative real-time PCR (qRT-PCR) analysis using the Q6 Real-Time PCR System (Applied Biosystems) and SYBR Premix Ex Taq (2×) (TaKaRa). To normalize these samples, GhACTIN was as an endogenous control. Determination of reaction specificities and data processing were performed as described in previous study (Schmittgen and Livak, 2008). Gene-specific primers used for the PCR are listed in Supplementary Table 1. Three biological replicates were analyzed. The significance of differences between means was determined using analysis of variance implemented in SAS software (∗P < 0.05, ∗∗P < 0.01). The data were graphed using GraphPad Prism 5.
Analysis of Genetic Variation and Artificial Selection of Gossypium hirsutum KNOX Genes
The basic information for 82 early and 67 modern cultivars from a core collection of upland cotton and the relative genomic variants were downloaded from the Hebei Agricultural University website9. Single-nucleotide polymorphisms (SNPs) in the KNOX genes were detected, based on the genomic location of the genes, and the number of SNPs per gene was scored using Excel 2010. The fixation statistic (Fst) was calculated with Genepop 4.0 software (Rousset, 2008). The genes Fst > 0.45 were identified as putative sites under selection during improvement (Li et al., 2018).
Virus-Induced Gene Silencing Assay and Stress Treatment
Based on previously described VIGS assay method (Gao et al., 2011), the genes GhKNOX2-A, GhKNOX10-A, GhKNOX14-A, GhSTM2-A, and GhSTM3-A/D were amplified from the ‘CCRI50’ cDNA library and inserted in the pCLCrVA vector. Gene-specific primers used for the VIGS assay are listed in Supplementary Table 1. The recombinant vectors were transformed separately into Agrobacterium strain GV3101. The GV3101 cells harboring recombinant plasmid were mixed with cells carrying pCLCrVB (1:1 ratio). The GV3101 cells were cultured then were injected into 10-day-old cotton cotyledons. The cotton plants were analyzed with regard to their gene expression profiles and phenotypes under salt stress. The inoculated cotton plants were grown in a greenhouse at 22°C under a 16 h light/8 h dark photoperiod. The content of malondialdehyde (MDA) and activity of peroxidase (POD) were assessed using a MDA assay kit and POD Assay Kit (Nanjing Jiancheng). The analysis was repeated three times, and each data type was analyzed from a sample of at least five plants in each independent biological experiment. The significance of differences between means was determined using Student’s t-test (∗P < 0.05, ∗∗P < 0.01).
Results
Identification of KNOX Genes in Gossypium hirsutum
We identified putative KNOX genes in the reference genome of G. hirsutum. Forty-four GhKNOX genes were identified. The GhKNOX genes were named on the basis of the similarity of the encoded amino acid sequence with that of Arabidopsis orthologs; ‘A’ and ‘D’ indicated derivation in the A and D subgenomes, and ‘a’ and ‘b’ were used to distinguish the corresponding paralogs of the same Arabidopsis ortholog. Thus, the 44 putative KNOX family genes were named GhKNOX1 to GhKNOX7 and GhSTM1 to GhSTM3, and GhKNL1 was identified in a previous study (Gong et al., 2014). The other genes identified had no highly orthologous counterparts in Arabidopsis and were named GhKNOX8 to GhKNOX14. The cotton KNOX family genes encoded a peptide ranging in length from 102 to 865 amino acids, the molecular weight ranged between 11.31 and 98.44 KDa, and the isoelectric point value ranged from 4.08 to 8.81 (Table 1).
Phylogenetic and Structural Analysis of GhKNOX Proteins
To explore the evolutionary relationships of KNOX proteins among cotton and six other plant species, a neighbor-joining tree was constructed based on a multiple alignment of KNOX amino acid sequences. The KNOX proteins were divided into KNOXI, KNOXII, and KNATM clades (Figure 1). The KNOXI clade comprised the STM, KNAT1, KNAT2, and KNAT6 homologs derived from ferns, lycophytes, and angiosperms, and 24 GhKNOX proteins were clustered in this clade. The KNOXII clade comprised KNAT3, KNAT4, KNAT5, and KNAT7 homolog proteins. GhKNOX1-A/D were clustered in the KNATM clade. Most cotton KNOX proteins showed higher similarity with proteins from cacao and poplar; these genes were consistently clustered closely on one branch in the phylogenetic tree. Based on the classification of Arabidopsis KNOX proteins, subclades I and II belonged to the class KNOXI, and clades III and IV belonged to KNOXII and KNATM, respectively (Figure 2A).
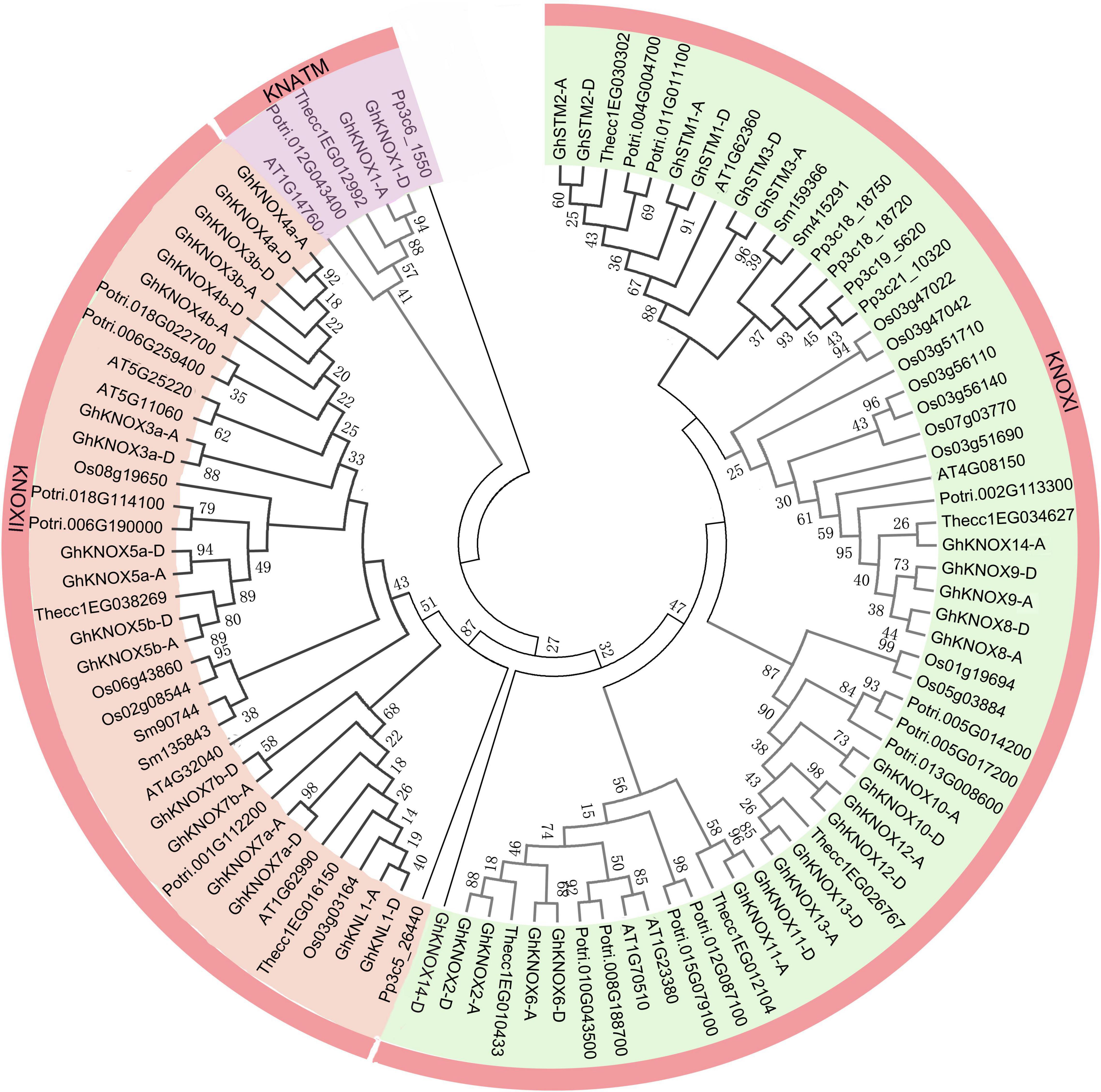
Figure 1. Phylogenetic relationship of KNOX family proteins of seven species. The phylogenetic tree was constructed from KNOX amino acid sequences using the neighbor-joining method with 1000 bootstrap replicates. The inner circle is marked in purple, orange, and green representing the KNOXI, KNOXII, and KNATM clades, respectively.
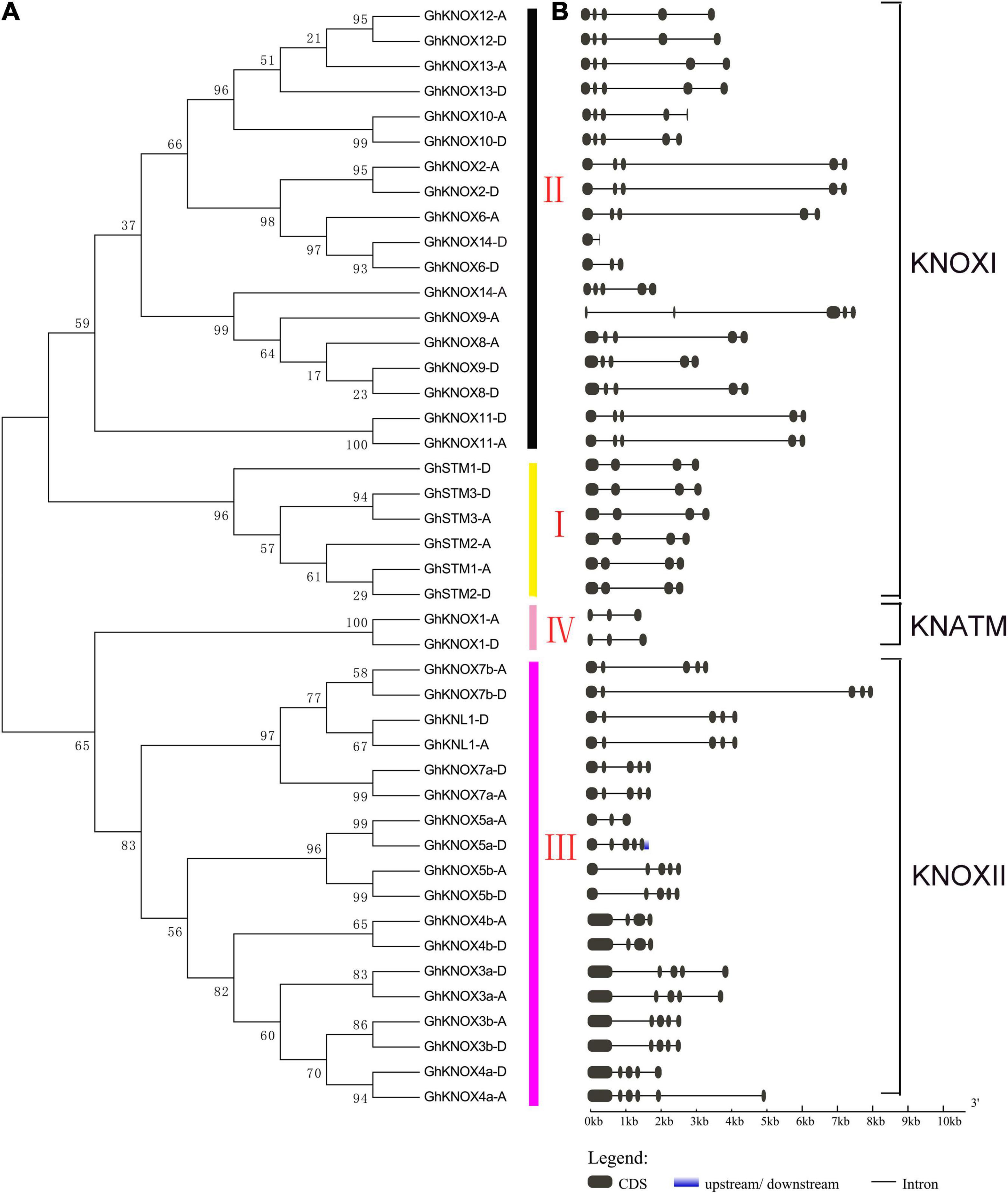
Figure 2. Phylogenetic relationships and genomic structure of GhKNOX genes. (A) Neighbor-joining tree of GhKNOXs. The GhKNOX genes were classified into four subclades (I, II, III, and IV). The subclades I and II were clustered in the KNOXI clade. The subclades III and IV belonged to the KNOXII and KNATM clades, respectively. (B) Exon–intron structural features of GhKNOX genes. Black boxes and lines indicate exons and introns, respectively.
Most (30 of 44) of the G. hirsutum KNOX genes contained four introns and five exons, and eight KNOX genes contained only three introns and four exons (Figure 2B). Only GhKNOX14-D incorporated one intron and two exons, and four genes (GhKNOX1-A/D, GhKNOX5a-A, and GhKNOX6-D) included two introns and three exons. The most highly similar exon and intron structures were observed in cotton genes within the same phylogenetic clade, thus supporting the reliability of the phylogenetic analysis. A multiple alignment of protein sequences was generated to detect the KNOX domain motifs in Arabidopsis and G. hirsutum (Supplementary Figures 1, 2). Four G. hirsutum proteins (GhKNOX1-A/D, GhKNOX5a-A, and GhKNOX6-D) contained only the KNOXI and KNOXII domains and lacked the ELK domain and homeobox KN binding domain. The GhKNOX10-A protein lacked the DNA-binding domain.
Chromosomal Location and Synteny Analysis of GhKNOX Genes
Among the 44 G. hirsutum KNOX genes, 41 members were located on 20 of the 26 chromosomes assembled in the G. hirsutum genome v1.1, and the remaining three genes were located on three unmapped scaffolds (scaffold4079, scaffold503, and scaffold1256) (Figure 3). The number of KNOX genes per chromosome ranged from zero to five. Chromosomes A05 and D05 carried five genes, whereas no KNOX gene was detected on chromosomes A01/D01, A04/D04, and A09/D09. The KNOX genes located on homoeologous A and D chromosomes was conserved identical except for A02/D02, A03/D03, and A06/D06. The circos software was used to analyze GhKNOX gene duplication events in the upland cotton genome (Figure 4). The GhKNOX genes were unevenly distributed in A and D subgenomes, and specific duplications also occurred in the two subgeomes. More than ten GhKNOX genes were located in the A and D subgenome regions, respectively. Chromosomes A01/D01, A04/D04, A07, and A09/D09 did not contain any duplicated genes, whereas chromosomes A05/D05 and A06/D06 harbored the highest number of duplications. Chromosomes A03/D03 had three genes, but only one of them was paralog gene. Chromosomes D07 had one gene, while chromosomes A07 had no paralog gene. The collinearity analysis indicated that GhKNOX genes diverged from a common ancestor, but these genes were not conserved in the A and D subgenomes.
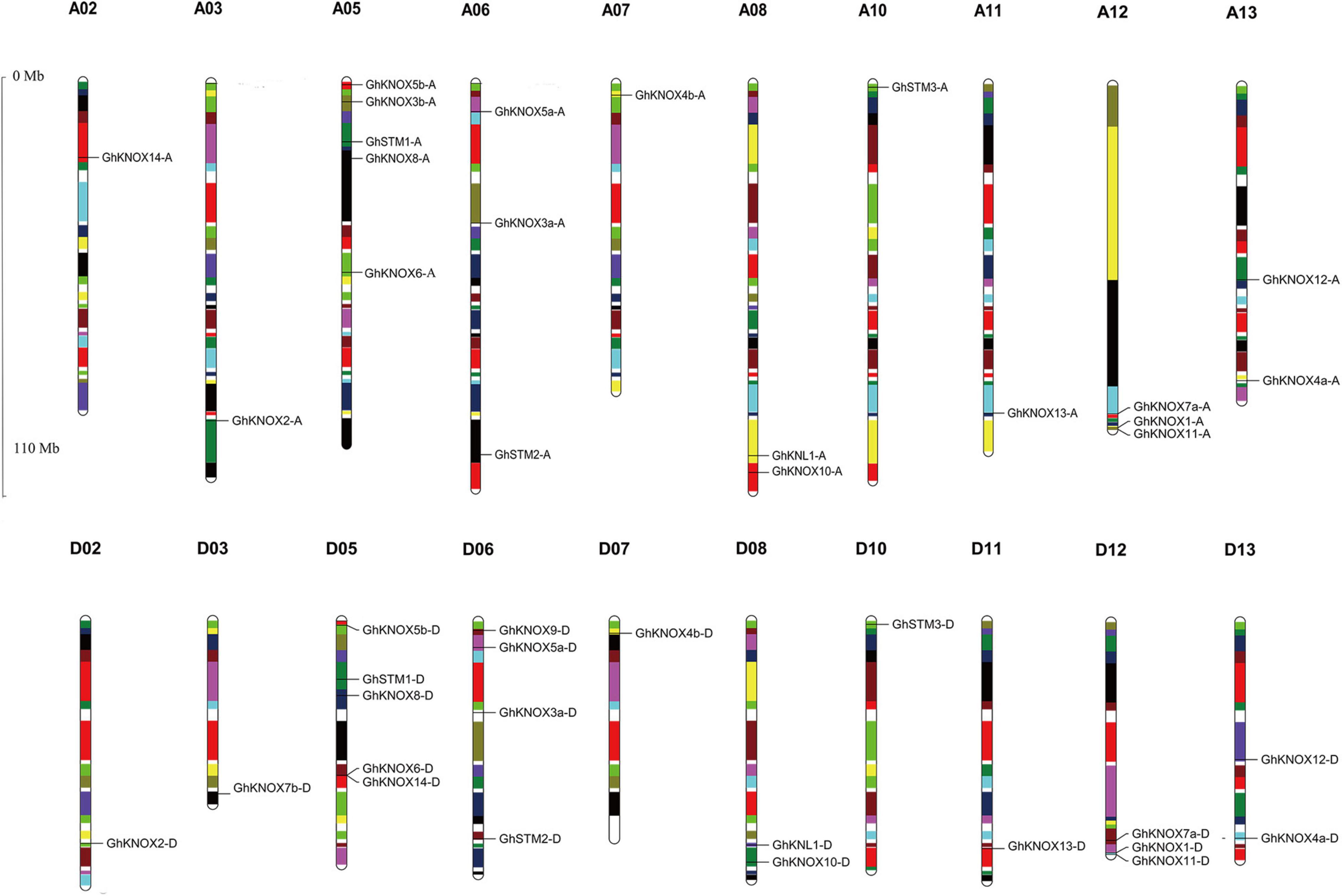
Figure 3. Physical locations of KNOX genes on G. hirsutum chromosomes. The upper and lower panels represent A subgenome chromosomes and D subgenome chromosomes, respectively. The scale is provided in megabases.
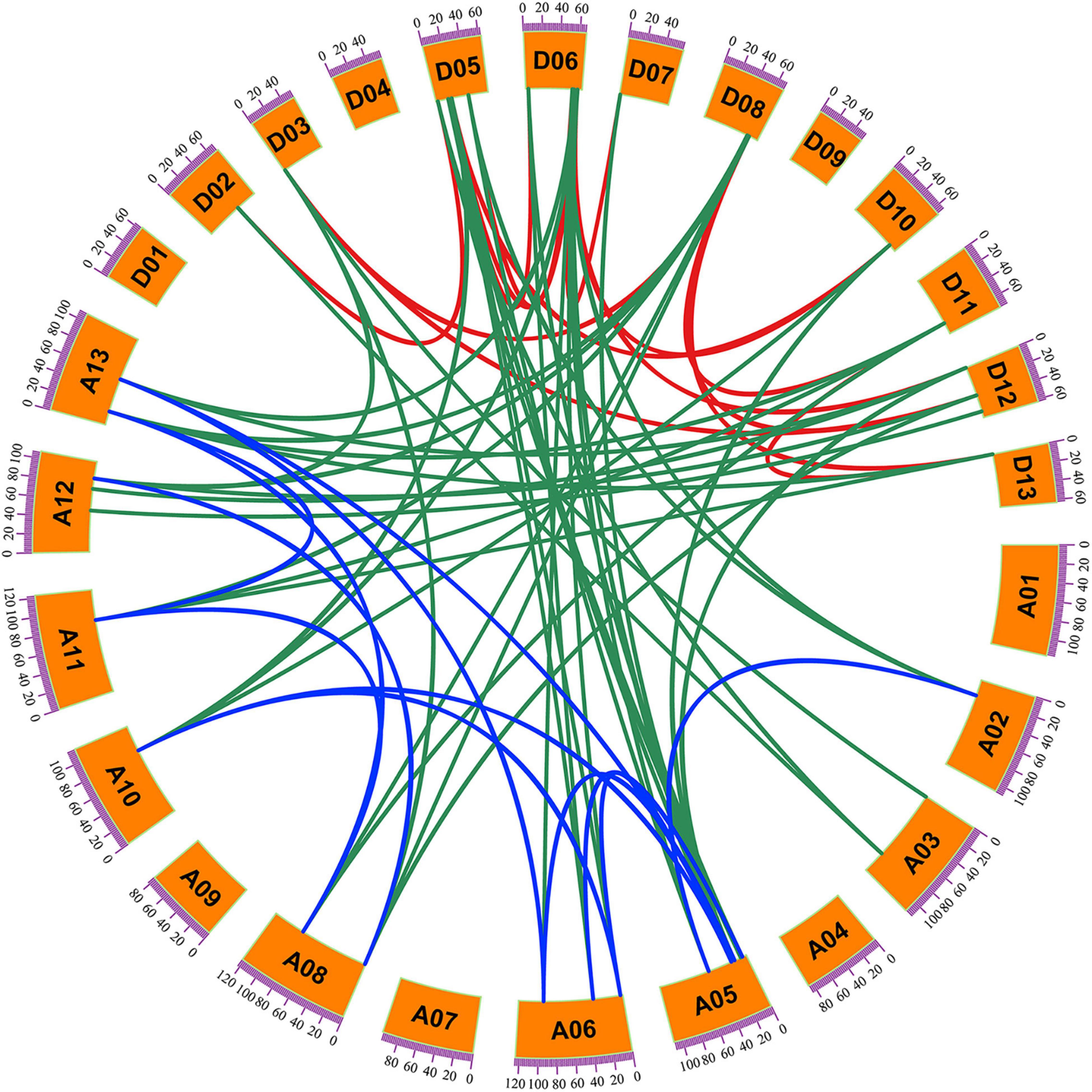
Figure 4. Collinearity analysis of KNOX genes on G. hirsutum chromosomes. Green lines link two homoeologous genes located in the A and D subgenome chromosomes. Red and blue lines link the two homologs formed by segmental duplication within the D subgenome and A subgenome, respectively. A01–A13 indicate the chromosomes in the A subgenome and D01–D13 indicate the chromosomes in the D subgenome.
Expression Pattern of GhKNOX Genes in Different Tissues and Shoot Meristem Developmental Stages
Gene expression in different tissues may be associated with diversity in biological functions. The expression patterns of GhKNOX genes in ten organs (root, stem, leaf, torus, sepal, bract, anther, filament, fiber, and ovule) were analyzed (Figure 5). Among these genes, six genes (GhKNOX1-A, GhKNL1-D, GhKNOX3a-A/D, GhKNOX3b-D, and GhKNOX5b-A) in class a showed higher expression levels at different stages of fiber and ovule development. The class b genes GhKNOX1-D, GhKNOX3b-A, GhKNOX6-A, GhKNOX10-A, and GhKNOX11-D showed higher expression in floral organs, such as the torus, sepal, and bract. Most class c genes showed higher expression levels in tissues except fibers. Among these genes, GhKNOX4b-D, GhKNOX12-D, and GhSTM2-D were more highly expressed in the root, whereas GhKNL1-A, GhKNOX4b-A, GhKNOX8-D, GhKNOX9-D, GhKNOX10-D, GhKNOX14-A/D, and GhSTM2-A were predominantly expressed in ovules. The class d genes showed diverse expression patterns, which were focused on the root, sepal, anther, and filament. These results indicated that GhKNOX genes may have diverse biological functions in different tissues.
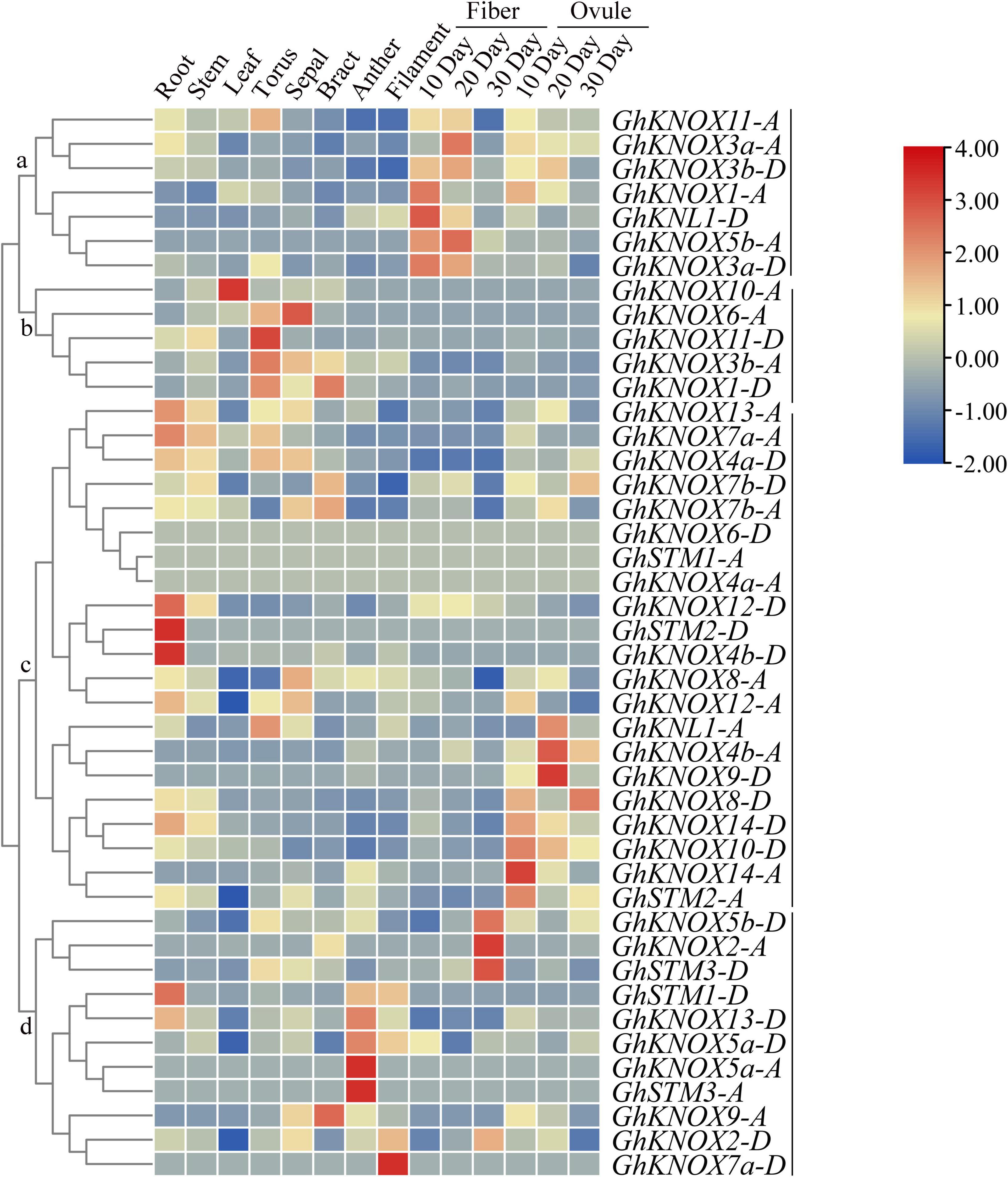
Figure 5. Expression patterns of GhKNOX genes in different tissues. The expression patterns were analyzed using hierarchical clustering. The FPKM values were calculated from RNA-seq data and are shown as a heatmap. The colored scale indicates the relative expression level.
The expression patterns of GhKNOX genes in the shoot meristem of the early maturing cultivar ‘Zao1’ and the late-maturing cultivar ‘CCRI50’ were analyzed from the fourth leaf expanded to the seventh leaf expanded stages (Supplementary Figure 3). Eight genes in class A showed decreased expression levels at the four shoot apical development stages of ‘Zao1’ compared with those of ‘CCRI50.’ The transcript level of class B genes was highest at the fourth leaf expanded stage of ‘Zao1’ and at the seventh leaf expanded stage of ‘CCRI50.’ Other GhKNOX genes in class C exhibited higher expression levels in ‘Zao1’ with a lower expression level detected at the fourth leaf expanded stage. In ‘CCRI50,’ the majority of GhKNOX genes showed the highest transcript level at the sixth and seventh leaf expanded stages except GhKNOX11-D. Six STM homolog genes showed higher expression levels in ‘Zao1’ than in ‘CCRI50.’ Thus, the functions of GhSTM genes in cotton growth and development require further verification.
Abiotic Stress Induced Expression Profiles of GhKNOX Genes
The expression pattern of the 44 GhKNOX genes in response to exposure to cold, heat, salt, and drought stress was analyzed at different time points. The expression of some KNOX genes was affected significantly, such as GhKNL1-D, GhKNOX2-D, GhKNOX3b-A, GhKNOX4b-A, GhKNOX6-A, GhKNOX10-A, and GhKNOX14-A. The expression level of GhKNOX2-D, GhKNOX4b-A, GhKNOX6-A, and GhKNOX14-A was increased in response to the four stresses. The genes GhKNOX2-A, GhKNOX14-D, and GhKNL1-A showed decreased expression under the four stress treatments. Expression of GhKNOX10-A was not influenced by heat, drought, and salt stress. GhKNOX5a-D and GhKNOX7b-D showed higher expression levels in response to cold stress only, whereas expression of GhKNOX5a-D, GhKNOX9-A, and GhSTM3-D increased at 1 h and thereafter decreased slightly. The present results indicated that GhKNOX genes from the A subgenome displayed superior adaptability to environmental stresses (Figure 6).
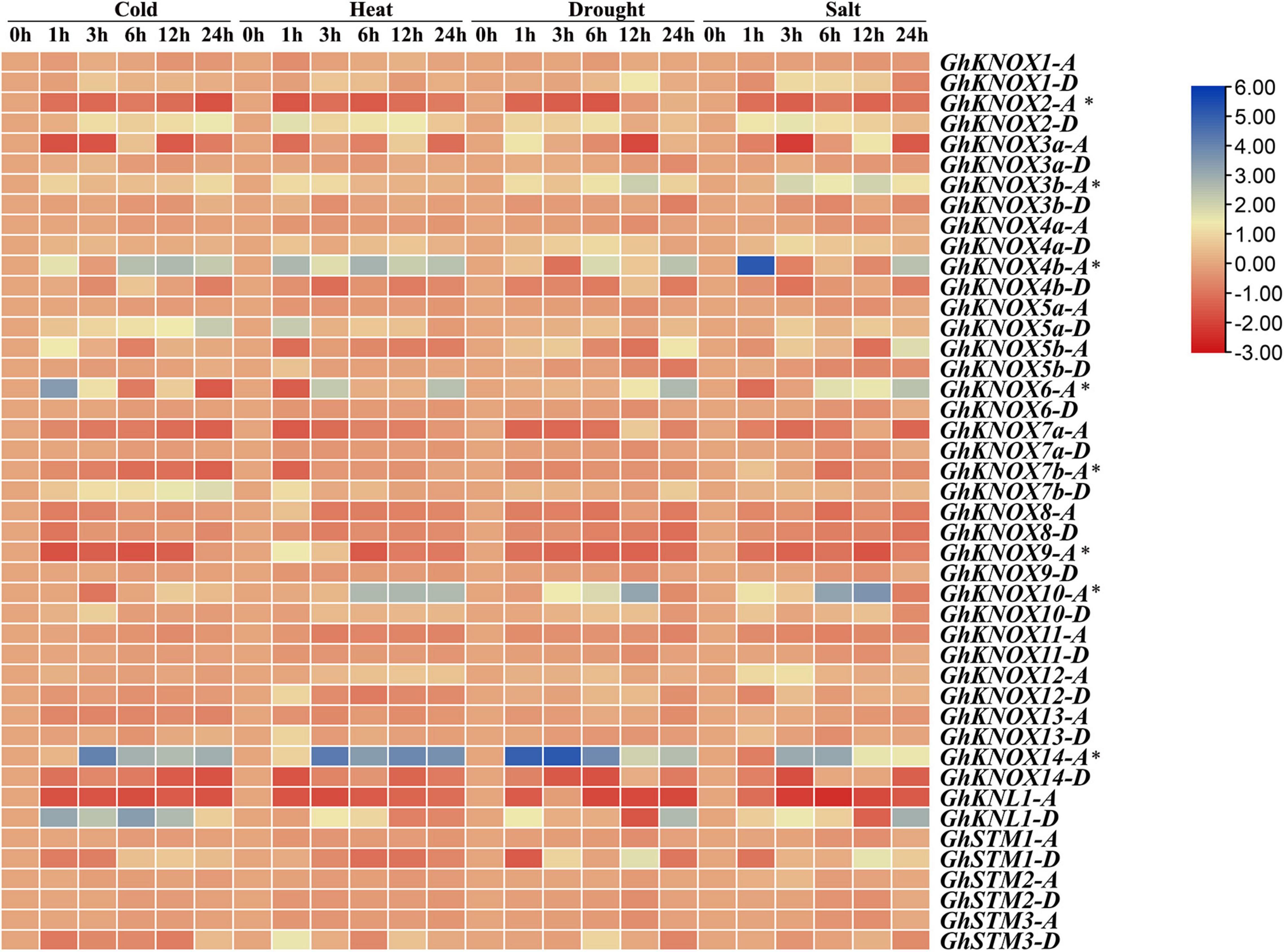
Figure 6. Expression profiles of GhKNOX genes in response to four abiotic stress treatments. The ratios of FPKM between the treatments (at 1, 3, 6, 12, and 24 h) and the control (at 0 h) were calculated from RNA-seq data and are shown as a heat map. The colored scale indicates the relative expression level. An asterisk indicates that expression of these genes requires verification by qRT-PCR.
To explore the expression of GhKNOX genes in response to abiotic stresses in greater detail, we selected eight GhKNOX genes for which expression was induced by drought and salt stress, and examined their expression following treatment with 20% PEG or 200 mM NaCl. The qRT-PCR results showed that GhKNOX4b-A/D, GhKNOX7b-A, GhKNOX10-A, and GhKNOX14-A were upregulated by PEG or NaCl treatment. Transcription of GhKNOX2-A and GhKNOX3b-A/D was upregulated by PEG treatment and downregulated by NaCl treatment. GhKNOX6-A, and GhKNOX9-A were downregulated by PEG or NaCl treatment (Figure 7). These results implied that GhKNOX family genes may show differential expression levels under different abiotic stresses.
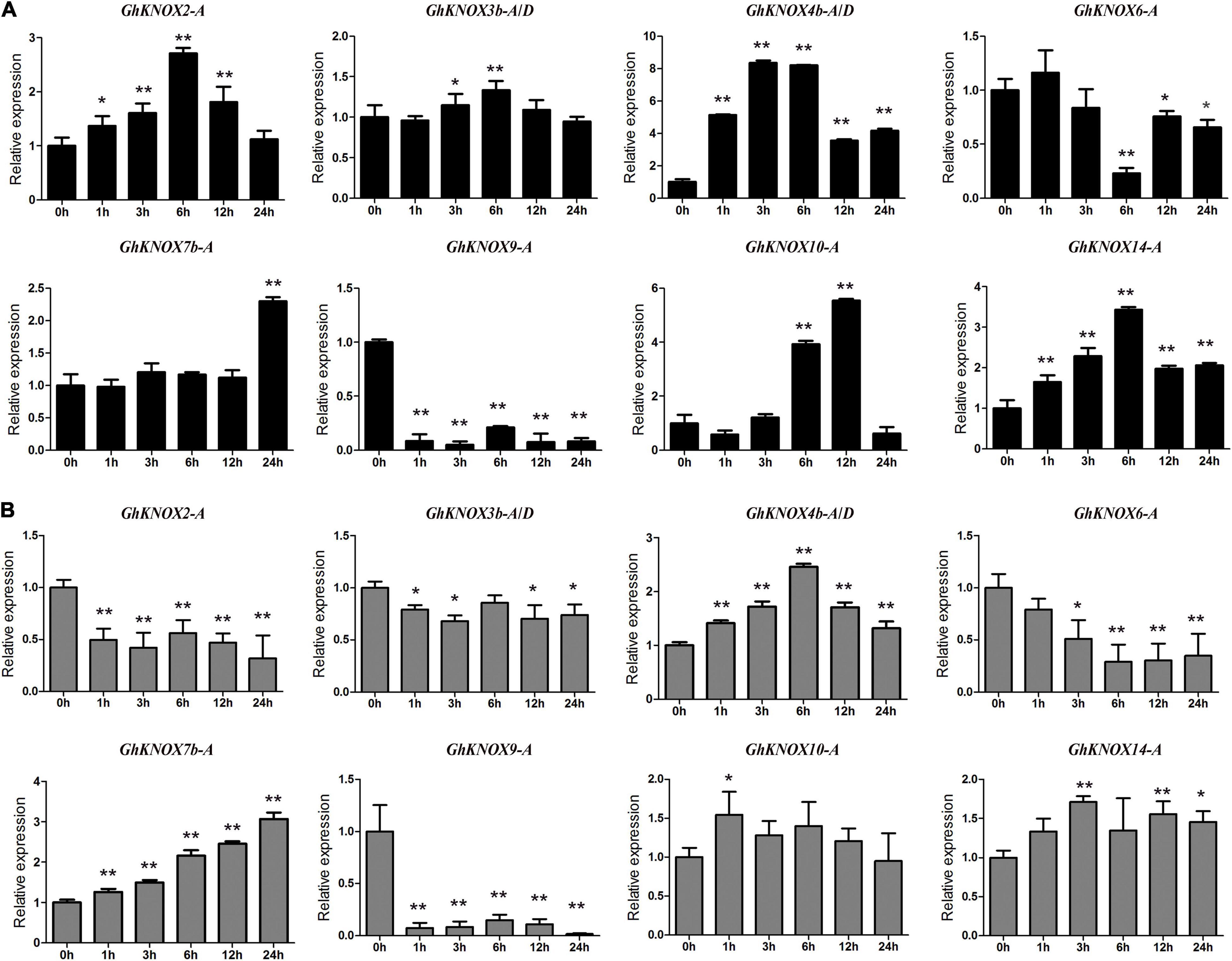
Figure 7. Expression patterns of selected GhKNOX genes in response to PEG or NaCl stress treatment. (A) Relative expression levels of GhKNOX genes between the control (0 h) and different time points (1, 3, 6, 12, and 24 h) under 20% PEG treatments. (B) Relative expression levels of GhKNOX genes between the control (0 h) and different time points (1, 3, 6, 12, and 24 h) under 200 mM NaCl treatment. GhACTIN (AY305733) was used as an internal control. Error bars indicate the standard deviation of three independent experiments. Relative expression was calculated using the 2–△△Ct method. The significance of differences between means was determined using analysis of variance (*P < 0.05, **P < 0.01).
Silencing of Five KNOX Homolog Genes in Cotton
GhKNOX2-A, GhKNOX10-A, GhKNOX14-A, GhSTM2-A, and GhSTM3-A/D belonged to the clade KNOXI, which includes the Arabidopsis homolog genes KNAT1, KNAT2, KNAT6, and STM. The expression patterns implied that GhKNOX2-A, GhKNOX10-A, and GhKNOX14-A are induced by salt stress. We used VIGS assays to investigate the functions of these five G. hirsutum genes. The appearance of white leaves indicated that VIGS was successful and qRT-PCR analysis confirmed that the expression levels of the five KNOX genes decreased significantly in the VIGS plants (Supplementary Figure 4). Silencing of GhKNOX2-A increased salt tolerance, therefore the silenced cotton seedlings grew better than the control seedlings in response to salt treatment (Figure 8A). The POD activity of the silenced plants was significantly higher than that for control seedlings (Figures 8G,H). Silencing of GhKNOX10-A and GhKNOX14-A decreased the salt tolerance (Figures 8B,C), therefore the silenced cotton seedlings showed inferior growth compared with the control seedlings in response to salt treatment. The MDA content in GhKNOX14-A VIGS plants was significantly higher than that in control seedlings, whereas the POD activity of silenced GhKNOX10-A plants was lower than that of control seedlings (Figures 8G,H). Compared with control plants, VIGS of GhSTM2-A and GhSTM3-A/D did not result in significant changes in MDA content after salt treatment, whereas the POD activity decreased compared with that of the control (Figures 8D–H). The flowering time was promoted in GhSTM3-A/D VIGS plants, and expression of GhFT and GhAP1 was upregulated with silencing of GhSTM3-A/D (Figures 8F,I). These results indicated that the five KNOX genes play an important role in salt stress tolerance and GhSTM3 might affect the floral transition of cotton.
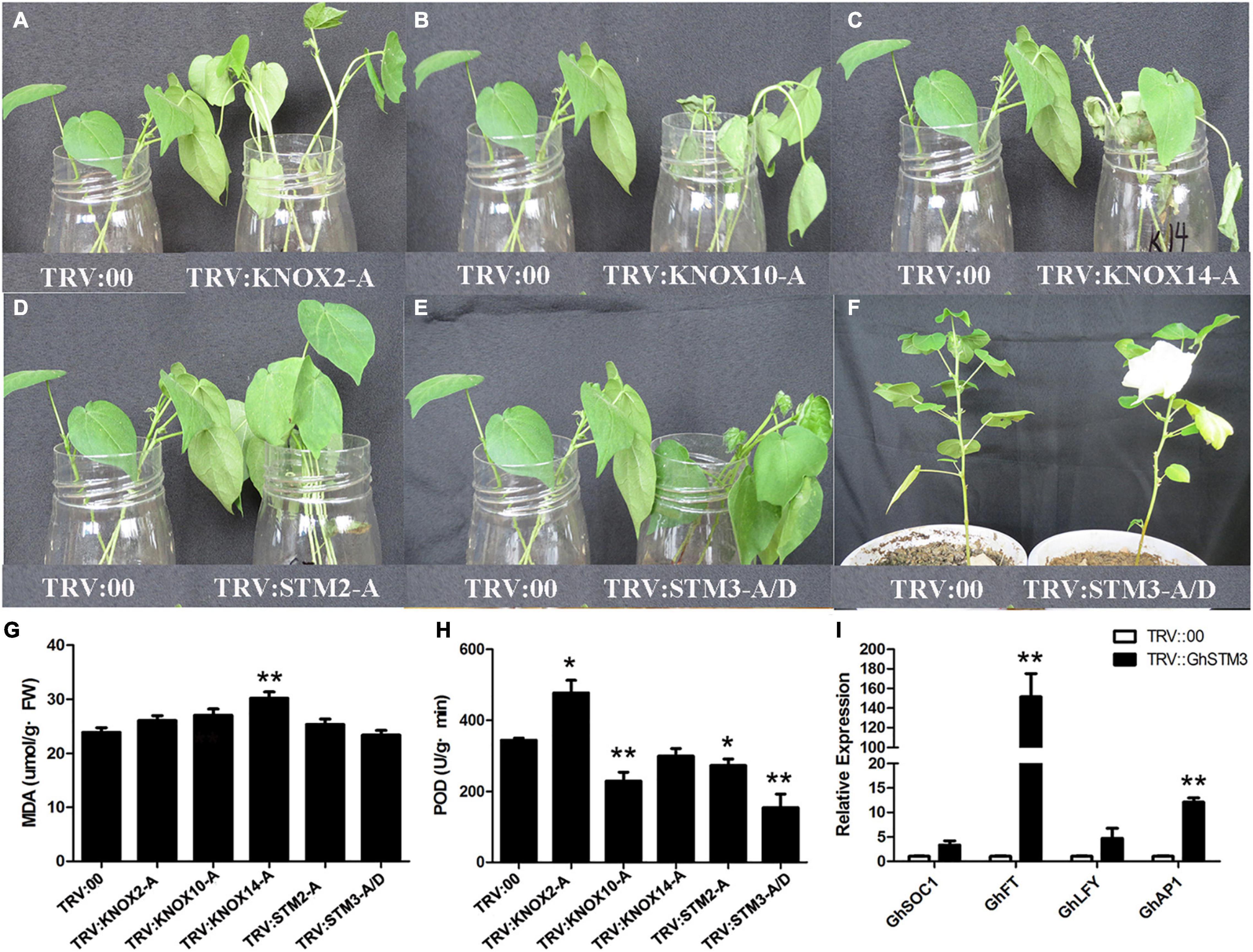
Figure 8. VIGS analysis of five G. hirsutum KNOX genes. (A–E) The left-hand plant is the control cotton transformed with the empty vector. In the right-hand plants, GhKNOX2-A, GhKNOX10-A, GhKNOX14-A, GhSTM2-A, and GhSTM3-A/D were silenced, respectively. (F) The control plant and GhSTM3-silenced plant of ‘CCRI50’ from left to right. (G) Malondialdehyde (MDA) content in TRV:00 and plants silenced for five G. hirsutum KNOX genes. (H) Peroxidase (POD) activity in TRV:00 and plants silenced for five G. hirsutum KNOX genes. (I) Relative expression levels of GhSOC1, GhFT, GhLFY, and GhAP1. The significance of differences was determined using Student’s t-test (*P < 0.05, **P < 0.01).
Genetic Variations and Artificial Selection of GhKNOX Genes During Breeding Improvement
The increase in availability of resequencing data for cultivated cotton species enabled assessment of genetic differences in KNOX genes over several decades of breeding. In this study, we estimated the genetic variation of 82 early and 67 modern cultivars that were sequenced and the data released from a core collection of upland cotton (Ma et al., 2018). The early cultivars included introductions and cultivars bred before 1976, and the modern cultivars comprised those bred during the period 1996–2008. To compare genetic variation among different KNOX family genes in cotton cultivars, we counted the number of SNPs per gene. A total of 64 SNPs were detected in 19 GhKNOX genes and the number SNPs per gene ranged from 1 to 11. The early cultivars contained 54 SNPs in 16 GhKNOX genes, whereas modern cultivars contained 57 SNPs in 18 GhKNOX genes. The SNPs density of modern cultivars was higher than that of early cultivars for the genes GhSTM1-A, GhSTM2-D, GhKNOX4b-D, GhKNOX8-A, and GhKNOX12-A, whereas the reverse result was observed for GhKNOX7b-D and GhSTM1-D. These results showed that the GhKNOX genes exhibited rich genetic variation among both early and modern cultivars (Figure 9A).
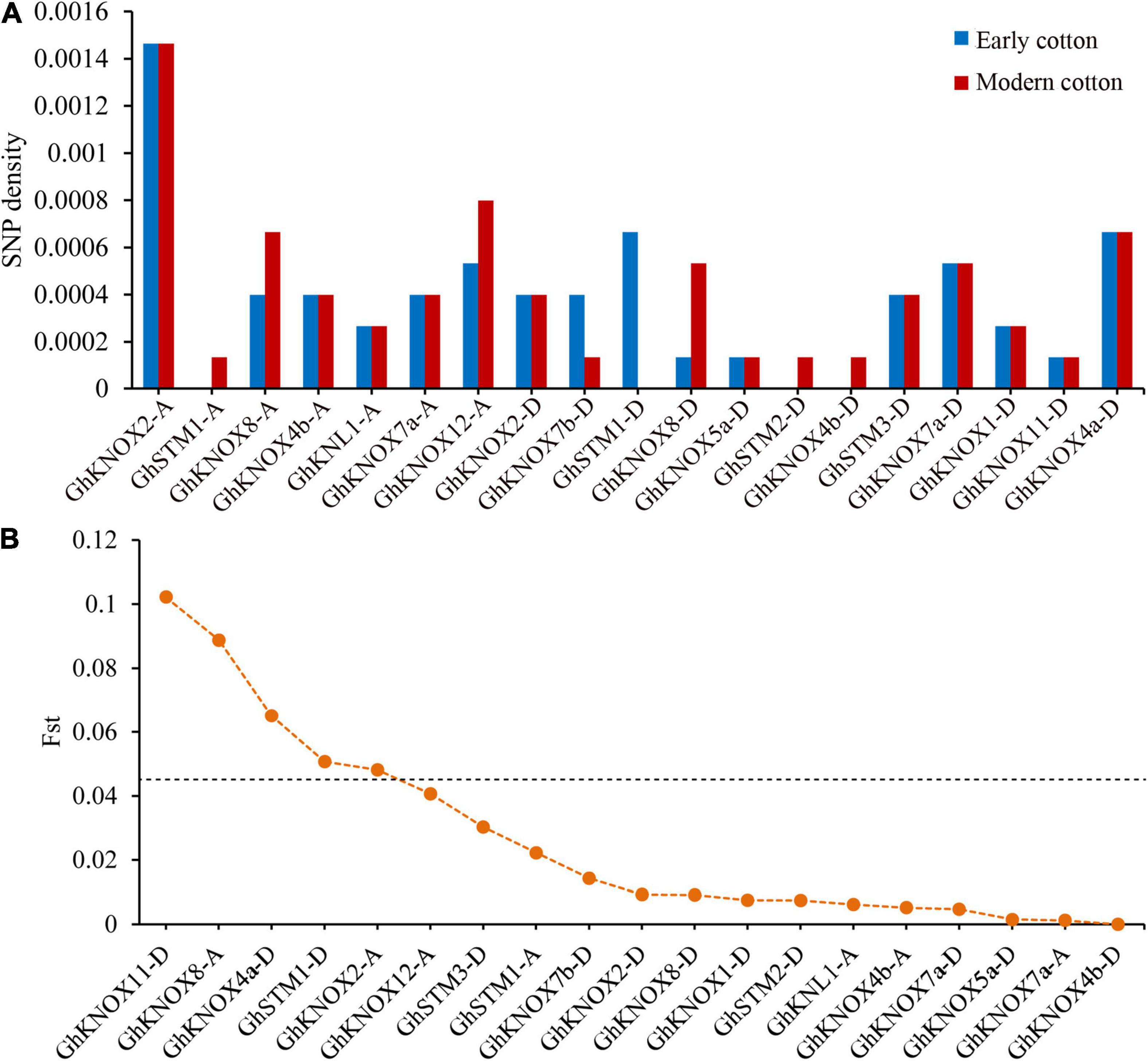
Figure 9. Genetic variation in G. hirsutum KNOX genes. (A) Density of SNPs in 19 GhKNOX genes. (B) The Fst values of the SNP loci in 19 GhKNOX genes. A Fst value for a SNP locus higher than 0.45 was considered to indicate putative sites under selection during domestication.
To clarify the selective pressure exerted during breeding, we estimated the genetic difference among the two groups of cultivars (Figure 9B). There were distinct selective signals for GhKNOX11-D (0.10), GhKNOX8-A (0.089), GhSTM1-D (0.65), GhKNOX2-A (0.51), and GhKNOX4a-D (0.48) during cotton improvement, showing that these genes were subjected to intensive artificial selection. In contrast, 11 genes (GhKNOX4b-A, GhKNL1-A, GhKNOX7a-A, GhKNOX2-D, GhKNOX7b-D, GhKNOX8-D, GhKNOX5a-D, GhSTM2-D, GhKNOX4b-D, GhKNOX7a-D, and GhKNOX1-D) showed few genetic differences and an average Fst of 0.006. These results indicated that the latter genes have not been subjected to breeding selection and are potential improvement targets for breeders in the future.
Discussion
Gossypium hirsutum is an allotetraploid species derived from hybridization between G. arboretum (A genome) and G. raimondii (D genome). A whole-genome duplication event occurred in the diploid species G. raimondii and G. arboretum (Huang et al., 2020). In the present study, we identified 44 GhKNOX genes in the cotton genome, which exceeds the nine KNOX genes identified in the Arabidopsis genome. Thus, the number of KNOX family genes has been expanded by approximately five-fold in cotton compared with that of Arabidopsis. The amino acid sequence alignment indicated that most GhKNOX proteins contained KNOXI, KNOXII, ELK, and homeobox KN binding domains except four proteins (GhKNOX1-A/D, GhKNOX5a-A, and GhKNOX6-D) that lacked the ELK and DNA binding domains, and GhKNOX10-A lacked the homeobox KN binding domain (Supplementary Figure 1). The ELK domain might be involved in transcriptional repression and function as a nuclear localization signal, and the homeobox KN binding domain located at the C-terminus is involved in DNA binding (Kerstetter et al., 1994; Nagasaki et al., 2001; Scofield and Murray, 2006). Thus, the five GhKNOX genes lacking these domains might have lost these respective functions.
Phylogenetic analysis revealed that KNOX genes were resolved into KNOXI, KNOXII, and KNATM clades. The KNOXI clade included the majority (58) of the KNOX genes, comprising two from Selaginella moellendorffii, four from Physcomitrella patens, four from Arabidopsis, ten from poplar, nine from rice, five from cacao, and 24 from cotton, whereas the KNOXII and KNATM clades consisted of 36 and six KNOX genes, respectively. Tandem and segmental duplications have been important for the expansion of gene families (Cannon et al., 2004). The expansion of gene number is important for adaptation to novel environments during plant evolution. GhKNOX family genes of cotton did not show tandem duplication, which is identical to Arabidopsis, Populus, and Glycine (Gao et al., 2015). Therefore, expansion of the GhKNOX gene family might have resulted from segmental duplication, and this reflects the adoption of novel functions in cotton. These differences suggest that the cotton KNOX gene family may have adapted to complex environmental conditions during evolution.
During plant evolution, KNOX genes have undergone major expansion from lycophytes to angiosperms, with not only increase in the large number of genes but also gene functional enrichment is apparent. The spatiotemporal expression patterns and functional analysis of KNOX genes have been studied in many species. The Arabidopsis STM gene is mainly expressed in the SAM and controls meristem formation and size (Aida et al., 1999; Spinelli et al., 2011). In the present study, STM homologs were strongly expressed in the SAM of the earlier-maturing cultivar ‘Zao1’ than that of the later-maturing ‘CCRI50.’ Also, GhSTM genes accumulated a number of SNP loci during evolution. Thus, we suggest that the function of these genes might be focused on plant growth and development. We used VIGS assays to investigate the functions of GhSTM2-A and GhSTM3-A/D. The results indicated that the flowering time was accelerated in GhSTM3-silenced cotton plants, and the expression levels of FT and AP1 homologs were upregulated significantly. Previous research revealed that repression of STM by AUXIN RESPONSE FACTOR (ARF) genes in Arabidopsis may promote flower initiation, which is mediated by histone deacetylation (Chung et al., 2019). Our results indicated that STM might have a negative function in the regulation of flowering time, which might be regulated by ‘florigen’ and floral development-related genes. KNOXI clade genes in Arabidopsis regulate inflorescence architecture, leaf shape, and internode development (Douglas et al., 2002; Smith and Hake, 2003; Chang et al., 2019). GhKNOX2-1 can interact with ARF16 to regulate leaf shape during the diversification of cotton species (He et al., 2021). PagKNAT2/6b, a class I KNOX gene in Populus, could improve drought resistance by inhibiting the synthesis of gibberellin (Song et al., 2021). However, the role of GhKNOX genes in response to stress is still limited in cotton. In the present study, the KNOXI homolog genes GhKNOX2-A, GhKNOX10-A, and GhKNOX14-A were highly expressed in the fiber and ovule, and were regulated by salt and drought stress. The VIGS assay for GhKNOX2-A increased the activity of POD and salt tolerance, whereas silencing of GhKNOX10-A and GhKNOX14-A decreased salt tolerance by reducing the activity of POD and increasing the MDA content, respectively. Previous study concluded that the root of plant can not absorb water under saline environment, and partial genes participate in plant stress signals by osmotic adjustment, osmoprotection, and protein accumulation (Buchanan et al., 2015; Li et al., 2020). Therefore, whether G. hirsutum KNOXI genes perform diverse functions that affect abiotic stress response and plant growth and development requires further study.
The KNOXII clade genes KNOX3/4/5/7 are involved in seed development and seed physical dormancy (Chai et al., 2016; Wang et al., 2020). The legume KNOX3 gene regulates nodule formation through cytokinin biosynthesis and activation (Azarakhsh et al., 2015). The tomato KNOX gene Tkn4 participates in pollen and pollen tube development and the regulation of plant growth through the gibberellin and auxin pathways (Yan et al., 2019). Rice KNOX7 integrates secondary wall and cell growth master regulators in internode and panicle development (Wang et al., 2019). Most soybean KNOX II genes exhibited higher expression levels during saline stress (Wang et al., 2021). Consistent with these results, the present expression analysis of GhKNOX3a, GhKNOX5b and GhKNL1 showed prior expression in the fiber, and GhKNL1 affected fiber development in the secondary cell wall biosynthesis pathway (Gong et al., 2014). The KNOXII clade genes GhKNOX4a, GhKNOX4b, and GhKNOX7b were highly expressed in the root. We also observed that KNOXII clade genes, such as GhKNOX3b-A, GhKNOX4b-A, GhKNOX5a-D, GhKNOX7b-D, and GhKNL1-D, showed distinct responses to abiotic stresses. These results implied that GhKNOX genes might play an active role in stress response induction. Although expression patterns have been illustrated, the functional roles of these KNOX family genes remain to be clarified. Thus, the comprehensive analysis of KNOX genes described could supply valuable information to elucidate the gene family in cotton.
In this study, we used available bioinformatic data and methods to explore the evolutionary relationships and functional roles of cotton KNOX genes. Phylogenetic analysis demonstrated that GhKNOX genes were divided into three clades and were expanded during genetic evolution. Analyses of expression profiles and gene function indicated that the GhKNOX genes likely responded to diverse stresses and were involved in plant development of cotton. These results provide useful information for future functional studies of KNOX family genes in cotton.
Data Availability Statement
The datasets presented in this study can be found in online repositories. The names of the repository/repositories and accession number(s) can be found in the article/Supplementary Material.
Author Contributions
QM and SF conceived and designed research. XZ and JZ conducted the experiments and wrote the manuscript. GH and XW revised the manuscript. All the authors read and approved the manuscript.
Funding
This work was supported by the State Key Laboratory of Cotton Biology (CB2018A08) and National Key Project of Science and Technology (2020ZX08009-12B).
Conflict of Interest
The authors declare that the research was conducted in the absence of any commercial or financial relationships that could be construed as a potential conflict of interest.
Publisher’s Note
All claims expressed in this article are solely those of the authors and do not necessarily represent those of their affiliated organizations, or those of the publisher, the editors and the reviewers. Any product that may be evaluated in this article, or claim that may be made by its manufacturer, is not guaranteed or endorsed by the publisher.
Supplementary Material
The Supplementary Material for this article can be found online at: https://www.frontiersin.org/articles/10.3389/fpls.2021.774161/full#supplementary-material
Supplementary Figure 1 | Distribution of G. hirsutum KNOX protein motifs. The different-colored boxes represent the conserved motifs containing KNOXI (green), KNOXII (purple), ELK (cyan-blue), and homeobox KN (red) binding domains.
Supplementary Figure 2 | Amino acid sequences showing the highly conserved KNOX domains in Arabidopsis (A) and G. hirsutum (B).
Supplementary Figure 3 | Expression profiles of GhKNOX genes at different stages of shoot meristem development in the G. hirsutum cultivars ‘Zao1’ and ‘CCRI50.’ Shoot apical buds were harvested at the fourth leaf expanded to the seventh leaf expanded stages. Colors from red to green represent expression levels ranging from high to low, respectively.
Supplementary Figure 4 | Expression analysis of five G. hirsutum KNOX genes in the VIGS assay. (A) Phenotype of the positive control plant transformed with the pCLCrVA-PDS vector. (B) Relative expression levels in empty control (TRV:00) and five GhKNOX genes VIGS plants. The five GhKNOX genes were GhKNOX2-A, GhKNOX10-A, GhKNOX14-A, GhSTM2-A, and GhSTM3-A/D, respectively.
Supplementary Table 1 | PCR primers used in the study.
Footnotes
- ^ http://www.cottongen.org
- ^ https://phytozome.jgi.doe.gov/pz/portal.html
- ^ https://www.ncbi.nlm.nih.gov/genbank/
- ^ http://www.arabidopsis.org
- ^ http://smart.embl-heidelberg.de/
- ^ http://www.ebi.ac.uk
- ^ http://gsds.gao-lab.org/
- ^ https://trace.ncbi.nlm.nih.gov/Traces/sra/
- ^ http://cotton.hebau.edu.cn/
References
Aida, M., Ishida, T., and Tasaka, M. (1999). Shoot apical meristem and cotyledon formation during Arabidopsis embryogenesis: interaction among the CUP-SHAPED COTYLEDON and SHOOT MERISTEMLESS genes. Development 126, 1563–1570.
Azarakhsh, M., Kirienko, A. N., Zhukov, V. A., Lebedeva, M. A., Dolgikh, E. A., and Lutova, L. A. (2015). KNOTTED1-LIKE HOMEOBOX 3: a new regulator of symbiotic nodule development. J. Exp. Bot. 66, 7181–7195. doi: 10.1093/jxb/erv414
Belles-Boix, E., Hamant, O., Witiak, S. M., Morin, H., Traas, J., and Pautot, V. (2006). KNAT6: an Arabidopsis homeobox gene involved in meristem activity and organ separation. Plant Cell 18, 1900–1907. doi: 10.1105/tpc.106.041988
Bhatt, A. M., Etchells, J. P., Canales, C., Lagodienko, A., and Dickinson, H. (2004). VAAMANA–a BEL1-like homeodomain protein, interacts with KNOX proteins BP and STM and regulates inflorescence stem growth in Arabidopsis. Gene 328, 103–111. doi: 10.1016/j.gene.2003.12.033
Buchanan, B. B., Gruissem, W., and Jones, R. L. (2015). Biochemistry & Molecular Biology of Plants, 2nd Edn. Hoboken: John Wiley & Sons Press.
Cannon, S. B., Mitra, A., Baumgarten, A., Young, N. D., and May, G. (2004). The roles of segmental and tandem gene duplication in the evolution of large gene families in Arabidopsis thaliana. BMC Plant Biol. 4:10. doi: 10.1186/1471-2229-4-10
Chai, M. F., Zhou, C. N., Molina, I., Fu, C. X., Nakashima, J., Li, G. F., et al. (2016). A class II KNOX gene, KNOX4, controls seed physical dormancy. Proc. Natl. Acad. Sci. U. S. A. 113, 6997–7002. doi: 10.1073/pnas.1601256113
Chang, L. J., Mei, G. F., Hu, Y., Deng, J. Q., and Zhang, T. Z. (2019). LMI1-like and KNOX1 genes coordinately regulate plant leaf development in dicotyledons. Plant Mol. Biol. 99, 449–460. doi: 10.1007/s11103-019-00829-7
Chen, C., Chen, H., Zhang, Y., Thomas, H. R., Frank, M. H., He, Y., et al. (2020). TBtools: an Integrative Toolkit Developed for Interactive Analyses of Big Biological Data. Mol. Plant 13, 1194–1202. doi: 10.1016/j.molp.2020.06.009
Cheng, S. S., Chen, P. Y., Su, Z. Z., Ma, L., Hao, P. B., Zhang, J. J., et al. (2021). High-resolution temporal dynamic transcriptome landscape reveals aGhCAL-mediated flowering regulatory pathway in cotton (Gossypium hirsutumL.). Plant Biotechnol. J. 19, 153–166. doi: 10.1111/pbi.13449
Chung, Y., Zhu, Y., Wu, M. F., Simonini, S., Kuhn, A., Armenta-Medina, A., et al. (2019). Auxin Response Factors promote organogenesis by chromatin-mediated repression of the pluripotency gene SHOOTMERISTEMLESS. Nat. Commun. 10:886. doi: 10.1038/s41467-019-08861-3
Dean, G., Casson, S., and Lindsey, K. (2004). KNAT6 gene of Arabidopsis is expressed in roots and is required for correct lateral root formation. Plant Mol. Biol. 54, 71–84. doi: 10.1023/B:PLAN.0000028772.22892.2d
Di Giacomo, E., Laffont, C., Sciarra, F., Iannelli, M. A., Frugier, F., and Frugis, G. (2017). KNAT3/4/5-like class 2 KNOX transcription factors are involved in Medicago truncatula symbiotic nodule organ development. New Phytol. 213, 822–837. doi: 10.1111/nph.14146
Douglas, S. J., Chuck, G., Dengler, R. E., Pelecanda, L., and Riggs, C. D. (2002). KNAT1 and ERECTA Regulate Inflorescence Architecture in Arabidopsis. Plant Cell 14, 547–558. doi: 10.1105/tpc.010391
Felipo-Benavent, A., Urbez, C., Blanco-Tourinan, N., Serrano-Mislata, A., Baumberger, N., Achard, P., et al. (2018). Regulation of xylem fiber differentiation by gibberellins through DELLA-KNAT1 interaction. Development 145:dev164962. doi: 10.1242/dev.164962
Fiene, J. G., Mallick, S., Mittal, A., Nansen, C., Kalns, L., Dever, J., et al. (2017). Characterization of transgenic cotton (Gossypium hirsutum L.) over-expressing Arabidopsis thaliana Related to ABA-insensitive3(ABI3)/Vivparous1 (AtRAV1) and AtABI5 transcription factors: improved water use efficiency through altered guard cell physiology. Plant Biotechnol. Rep. 11, 339–353. doi: 10.1007/s11816-017-0455-6
Gao, J., Yang, X., Zhao, W., Lang, T. G., and Samuelsson, T. (2015). Evolution, diversification, and expression of KNOX proteins in plants. Front. Plant Sci. 6:882. doi: 10.3389/Fpls.2015.00882
Gao, X., Britt, R. C. Jr., Shan, L., and He, P. (2011). Agrobacterium-mediated virus-induced gene silencing assay in cotton. J. Vis. Exp. 54:2938. doi: 10.3791/2938
Gong, S. Y., Huang, G. Q., Sun, X., Qin, L. X., Li, Y., Zhou, L., et al. (2014). Cotton KNL1, encoding a class II KNOX transcription factor, is involved in regulation of fibre development. J. Exp. Bot. 65, 4133–4147. doi: 10.1093/jxb/eru182
Hareven, D., Gutfinger, T., Parnis, A., Eshed, Y., and Lifschitz, E. (1996). The making of a compound leaf: genetic manipulation of leaf architecture in tomato. Cell 84, 735–744. doi: 10.1016/s0092-8674(00)81051-x
He, P., Zhang, Y., Li, H., Fu, X., Shang, H., Zou, C., et al. (2021). GhARF16-1 modulates leaf development by transcriptionally regulating the GhKNOX2-1 gene in cotton. Plant Biotechnol. J. 19, 548–562. doi: 10.1111/pbi.13484
Huang, G., Wu, Z. G., Percy, R. G., Bai, M. Z., Li, Y., Frelichowski, J. E., et al. (2020). Genome sequence of Gossypium herbaceum and genome updates of Gossypium arboreum and Gossypium hirsutum provide insights into cotton A-genome evolution. Nat. Genet. 52, 516–524. doi: 10.1038/s41588-020-0607-4
Kerstetter, R., Vollbrecht, E., Lowe, B., Veit, B., Yamaguchi, J., and Hake, S. (1994). Sequence analysis and expression patterns divide the maize knotted1-like homeobox genes into two classes. Plant Cell 6, 1877–1887. doi: 10.1105/tpc.6.12.1877
Kierzkowski, D., Runions, A., Vuolo, F., Strauss, S., Lymbouridou, R., Routier-Kierzkowska, A. L., et al. (2019). A Growth-Based Framework for Leaf Shape Development and Diversity. Cell 177, 1405–1418.e17. doi: 10.1016/j.cell.2019.05.011
Kim, D., Cho, Y. H., Ryu, H., Kim, Y., Kim, T. H., and Hwang, I. (2013a). BLH1 and KNAT3 modulate ABA responses during germination and early seedling development in Arabidopsis. Plant J. 75, 755–766. doi: 10.1111/tpj.12236
Kim, D., Pertea, G., Trapnell, C., Pimentel, H., Kelley, R., and Salzberg, S. L. (2013b). TopHat2: accurate alignment of transcriptomes in the presence of insertions, deletions and gene fusions. Genome Biol. 14:R36. doi: 10.1186/gb-2013-14-4-r36
Li, E., Wang, S., Liu, Y., Chen, J. G., and Douglas, C. J. (2011). OVATE FAMILY PROTEIN4 (OFP4) interaction with KNAT7 regulates secondary cell wall formation in Arabidopsis thaliana. Plant J. 67, 328–341. doi: 10.1111/j.1365-313X.2011.04595.x
Li, E. Y., Bhargava, A., Qiang, W. Y., Friedmann, M. C., Forneris, N., Savidge, R. A., et al. (2012). The Class II KNOX gene KNAT7 negatively regulates secondary wall formation in Arabidopsis and is functionally conserved in Populus. New Phytol. 194, 102–115. doi: 10.1111/j.1469-8137.2011.04016.x
Li, W., Huang, G. Q., Zhou, W., Xia, X. C., Li, D. D., and Li, X. B. (2014). A cotton (Gossypium hirsutum) gene encoding a NAC transcription factor is involved in negative regulation of plant xylem development. Plant Physiol. Biochem. 83, 134–141. doi: 10.1016/j.plaphy.2014.07.022
Li, W., Ren, Z., Wang, Z., Sun, K., Pei, X., Liu, Y., et al. (2018). Evolution and Stress Responses of Gossypium hirsutum SWEET Genes. Int. J. Mol. Sci. 19:769. doi: 10.3390/ijms19030769
Li, Z., Wang, X., Cui, Y., Qiao, K., Zhu, L., Fan, S., et al. (2020). Comprehensive Genome-Wide Analysis of Thaumatin-Like Gene Family in Four Cotton Species and Functional Identification of GhTLP19 Involved in Regulating Tolerance to Verticillium dahlia and Drought. Front. Plant Sci. 11:575015. doi: 10.3389/fpls.2020.575015
Long, J. A., Moan, E. I., Medford, J. I., and Barton, M. K. (1996). A member of the KNOTTED class of homeodomain proteins encoded by the STM gene of Arabidopsis. Nature 379, 66–69. doi: 10.1038/379066a0
Ma, Q., Wang, N. H., Hao, P. B., Sun, H. R., Wang, C. C., Ma, L., et al. (2019). Genome-wide identification and characterization of TALE superfamily genes in cotton reveals their functions in regulating secondary cell wall biosynthesis. BMC Plant Biol. 19:432. doi: 10.1186/S12870-019-2026-1
Ma, X., Wang, Z., Li, W., Zhang, Y., Zhou, X., Liu, Y., et al. (2018). Resequencing core accessions of a pedigree identifies derivation of genomic segments and key agronomic trait loci during cotton improvement. Plant Biotechnol. J. 17, 762–775. doi: 10.1111/pbi.13013
Magnani, E., and Hake, S. (2008). KNOX lost the OX: the Arabidopsis KNATM gene defines a novel class of KNOX transcriptional regulators missing the homeodomain. Plant Cell 20, 875–887. doi: 10.1105/tpc.108.058495
McGarry, R. C., Prewitt, S. F., Culpepper, S., Eshed, Y., Lifschitz, E., and Ayre, B. G. (2016). Monopodial and sympodial branching architecture in cotton is differentially regulated by the Gossypium hirsutum SINGLE FLOWER TRUSS and SELF-PRUNING orthologs. New Phytol. 212, 244–258. doi: 10.1111/nph.14037
Nadakuduti, S. S., Holdsworth, W. L., Klein, C. L., and Barry, C. S. (2014). KNOX genes influence a gradient of fruit chloroplast development through regulation of GOLDEN2-LIKE expression in tomato. Plant J. 78, 1022–1033. doi: 10.1111/tpj.12529
Nagasaki, H., Sakamoto, T., Sato, Y., and Matsuoka, M. (2001). Functional analysis of the conserved domains of a rice KNOX homeodomain protein, OSH15. Plant Cell 13, 2085–2098. doi: 10.1105/TPC.010113
Pautot, V., Dockx, J., Hamant, O., Kronenberger, J., Grandjean, O., Jublot, D., et al. (2001). KNAT2: evidence for a link between knotted-like genes and carpel development. Plant Cell 13, 1719–1734.
Pei, Y. (2015). The homeodomain-containing transcription factor, GhHOX3, is a key regulator of cotton fiber elongation. Sci. China Life Sci. 58, 309–310. doi: 10.1007/s11427-015-4813-8
Placido, D. F., Sandhu, J., Sato, S. J., Nersesian, N., Quach, T., Clemente, T. E., et al. (2020). The LATERAL ROOT DENSITY gene regulates root growth during water stress in wheat. Plant Biotechnol. J. 18, 1955–1968. doi: 10.1111/pbi.13355
Ragni, L., Belles-Boix, E., Gunl, M., and Pautot, V. (2008). Interaction of KNAT6 and KNAT2 with BREVIPEDICELLUS and PENNYWISE in Arabidopsis inflorescences. Plant Cell 20, 888–900. doi: 10.1105/tpc.108.058230
Reyes-Rivera, J., Rodriguez-Alonso, G., Petrone, E., Vasco, A., Vergara-Silva, F., Shishkova, S., et al. (2017). Expression of the KNOTTED HOMEOBOX Genes in the Cactaceae Cambial Zone Suggests Their Involvement in Wood Development. Front. Plant Sci. 8:218. doi: 10.3389/Fpls.2017.00218
Roth, O., Alvarez, J. P., Levy, M., Bowman, J. L., Ori, N., and Shani, E. (2018). The KNOXI Transcription Factor SHOOT MERISTEMLESS Regulates Floral Fate in Arabidopsis. Plant Cell 30, 1309–1321. doi: 10.1105/tpc.18.00222
Rousset, F. (2008). genepop’007: a complete re-implementation of the genepop software for Windows and Linux. Mol. Ecol. Res. 8, 103–106. doi: 10.1111/j.1471-8286.2007.01931.x
Schmittgen, T. D., and Livak, K. J. (2008). Analyzing real-time PCR data by the comparative C(T) method. Nat. Protoc. 3, 1101–1108.
Scofield, S., and Murray, J. A. (2006). KNOX gene function in plant stem cell niches. Plant Mol. Biol. 60, 929–946. doi: 10.1007/s11103-005-4478-y
Shah, S. T., Pang, C. Y., Fan, S. L., Song, M. Z., Arain, S., and Yu, S. X. (2013). Isolation and expression profiling of GhNAC transcription factor genes in cotton (Gossypium hirsutum L.) during leaf senescence and in response to stresses. Gene 531, 220–234. doi: 10.1016/j.gene.2013.09.007
Shu, Y., Tao, Y., Wang, S., Huang, L., Yu, X., Wang, Z., et al. (2015). GmSBH1, a homeobox transcription factor gene, relates to growth and development and involves in response to high temperature and humidity stress in soybean. Plant Cell Rep. 34, 1927–1937. doi: 10.1007/s00299-015-1840-7
Smith, H. M. S., and Hake, S. (2003). The Interaction of Two Homeobox Genes, BREVIPEDICELLUS and PENNYWISE, Regulates Internode Patterning in the Arabidopsis Inflorescence. Plant Cell 15, 1717–1727. doi: 10.1105/tpc.012856
Smith, L. G., Greene, B., Veit, B., and Hake, S. (1992). A dominant mutation in the maize homeobox gene, Knotted-1, causes its ectopic expression in leaf cells with altered fates. Development 116, 21–30.
Song, X., Zhao, Y., Wang, J., and Lu, M. Z. (2021). The transcription factor KNAT2/6b mediates changes in plant architecture in response to drought via down-regulating GA20ox1 in Populus alba × P. glandulosa. J. Exp. Bot. 72, 5625–5637. doi: 10.1093/jxb/erab201
Spinelli, S. V., Martin, A. P., Viola, I. L., Gonzalez, D. H., and Palatnik, J. F. (2011). A mechanistic link between STM and CUC1 during Arabidopsis development. Plant Physiol. 156, 1894–1904. doi: 10.1104/pp.111.177709
Sun, W. J., Gao, Z. Y., Wang, J., Huang, Y. Q., Chen, Y., Li, J. F., et al. (2019). Cotton fiber elongation requires the transcription factor GhMYB212 to regulate sucrose transportation into expanding fibers. New Phytol. 222, 864–881. doi: 10.1111/nph.15620
Takano, S., Niihama, M., Smith, H. M., Tasaka, M., and Aida, M. (2010). gorgon, a novel missense mutation in the SHOOT MERISTEMLESS gene, impairs shoot meristem homeostasis in Arabidopsis. Plant Cell Physiol. 51, 621–634. doi: 10.1093/pcp/pcq028
Tamura, K., Dudley, J., Nei, M., and Kumar, S. (2007). MEGA4: molecular evolutionary genetics analysis (MEGA) software version 4.0. Mol. Biol. Evol. 24, 1596–1599. doi: 10.1093/molbev/msm092
Truernit, E., Siemering, K. R., Hodge, S., Grbic, V., and Haseloff, J. (2006). A map of KNAT gene expression in the Arabidopsis root. Plant Mol. Biol. 60, 1–20. doi: 10.1007/s11103-005-1673-9
Wang, L., Yang, X., Gao, Y., and Yang, S. (2021). Genome-Wide Identification and Characterization of TALE Superfamily Genes in Soybean (Glycine max L.). Int. J. Mol. Sci. 22:4117. doi: 10.3390/ijms22084117
Wang, S. G., Yang, H. L., Mei, J. S., Liu, X. L., Wen, Z., Zhang, L. J., et al. (2019). Rice Homeobox Protein KNAT7 Integrates the Pathways Regulating Cell Expansion and Wall Stiffness. Plant Physiol. 181, 669–682. doi: 10.1104/pp.19.00639
Wang, S. M., Yamaguchi, M., Grienenberger, E., Martone, P. T., Samuels, A. L., and Mansfield, S. D. (2020). The Class II KNOX genes KNAT3 and KNAT7 work cooperatively to influence deposition of secondary cell walls that provide mechanical support to Arabidopsis stems. Plant J. 101, 293–309. doi: 10.1111/tpj.14541
Yan, F., Deng, W., Pang, X. Q., Gao, Y. S., Chan, H. L., Zhang, Q., et al. (2019). Overexpression of the KNOX gene Tkn4 affects pollen development and confers sensitivity to gibberellin and auxin in tomato. Plant Sci. 281, 61–71. doi: 10.1016/j.plantsci.2018.12.024
Yang, Z., Gong, Q., Qin, W., Yang, Z., Cheng, Y., Lu, L., et al. (2017). Genome-wide analysis of WOX genes in upland cotton and their expression pattern under different stresses. BMC Plant Biol. 17:113. doi: 10.1186/s12870-017-1065-8
Yu, J., Jung, S., Cheng, C. H., Ficklin, S. P., Lee, T., Zheng, P., et al. (2014). CottonGen: a genomics, genetics and breeding database for cotton research. Nucleic Acids Res. 42, D1229–D1236. doi: 10.1093/nar/gkt1064
Zhang, T. Z., Hu, Y., Jiang, W. K., Fang, L., Guan, X. Y., Chen, J. D., et al. (2015). Sequencing of allotetraploid cotton (Gossypium hirsutum L. acc. TM-1) provides a resource for fiber improvement. Nat. Biotechnol. 33, 531–537. doi: 10.1038/nbt.3207
Keywords: evolutionary, cotton, KNOX, stress response, artificial selection, development
Citation: Zhang X, Zhao J, Wu X, Hu G, Fan S and Ma Q (2021) Evolutionary Relationships and Divergence of KNOTTED1-Like Family Genes Involved in Salt Tolerance and Development in Cotton (Gossypium hirsutum L.). Front. Plant Sci. 12:774161. doi: 10.3389/fpls.2021.774161
Received: 11 September 2021; Accepted: 25 November 2021;
Published: 14 December 2021.
Edited by:
Jia-He Wu, Institute of Microbiology, Chinese Academy of Sciences (CAS), ChinaReviewed by:
Chengzhen Liang, Chinese Academy of Agricultural Sciences (CAAS), ChinaSayaka Miura, Temple University, United States
Copyright © 2021 Zhang, Zhao, Wu, Hu, Fan and Ma. This is an open-access article distributed under the terms of the Creative Commons Attribution License (CC BY). The use, distribution or reproduction in other forums is permitted, provided the original author(s) and the copyright owner(s) are credited and that the original publication in this journal is cited, in accordance with accepted academic practice. No use, distribution or reproduction is permitted which does not comply with these terms.
*Correspondence: Shuli Fan, fsl427@126.com; Qifeng Ma, 13837240176@163.com
†These authors have contributed equally to this work