- Department of Ocean and Earth Sciences, Old Dominion University, Norfolk, VA, United States
Photorespiration, commonly viewed as a loss in photosynthetic productivity of C3 plants, is expected to decline with increasing atmospheric CO2, even though photorespiration plays an important role in the oxidative stress responses. This study aimed to quantify the role of photorespiration and alternative photoprotection mechanisms in Zostera marina L. (eelgrass), a carbon-limited marine C3 plant, in response to ocean acidification. Plants were grown in controlled outdoor aquaria at different [CO2]aq ranging from ~55 (ambient) to ~2121 μM for 13 months and compared for differences in leaf photochemistry by simultaneous measurements of O2 flux and variable fluorescence. At ambient [CO2], photosynthesis was carbon limited and the excess photon absorption was diverted both to photorespiration and non-photochemical quenching (NPQ). The dynamic range of NPQ regulation in ambient grown plants, in response to instantaneous changes in [CO2]aq, suggested considerable tolerance for fluctuating environmental conditions. However, 60 to 80% of maximum photosynthetic capacity of ambient plants was diverted to photorespiration resulting in limited carbon fixation. The photosynthesis to respiration ratio (PE : RD) of ambient grown plants increased 6-fold when measured under high CO2 because photorespiration was virtually suppressed. Plants acclimated to high CO2 maintained 4-fold higher PE : RD than ambient grown plants as a result of a 60% reduction in photorespiration. The O2 production efficiency per unit chlorophyll was not affected by the CO2 environment in which the plants were grown. Yet, CO2 enrichment decreased the light level to initiate NPQ activity and downregulated the biomass specific pigment content by 50% and area specific pigment content by 30%. Thus, phenotypic acclimation to ocean carbonation in eelgrass, indicating the coupling between the regulation of photosynthetic structure and metabolic carbon demands, involved the downregulation of light harvesting by the photosynthetic apparatus, a reduction in the role of photorespiration and an increase in the role of NPQ in photoprotection. The quasi-mechanistic model developed in this study permits integration of photosynthetic and morphological acclimation to ocean carbonation into seagrass productivity models, by adjusting the limits of the photosynthetic parameters based on substrate availability and physiological capacity.
Introduction
Photosynthesis and photorespiration are competing processes due to the bi-functionality of ribulose 1,5-biphosphate carboxylase/oxygenase (Rubisco) (Spreitzer and Salvucci, 2002). Since the oxygenation reaction of Rubisco decreases photosynthetic carbon gain, it has been viewed as an inefficient legacy of evolution that might be engineered out of terrestrial plants in a quest for increased productivity (Andrews and Lorimer, 1978; Somerville, 2001; Xin et al., 2015). Recent work, however, suggests that Rubisco’s CO2/O2 specificity in different species may approach optimal acclimation to their gaseous environment in which the plants are grown (Tcherkez et al., 2006; Bathellier et al., 2018). More importantly, especially for carbon-limited seagrasses, photorespiration may serve as an important metabolic “clutch” to protect the photochemical pathway at high irradiance (Heber and Krause, 1980; Osmond, 1981; Osmond et al., 1997; Igamberdiev et al., 2001). When the Calvin Benson cycle is limited by the availability of CO2, continuation of light reactions over-reduces the thylakoid electron transport chain and generates O2 and reactive oxygen species (ROS) that potentiates oxidative stress (Voss et al., 2013). Photorespiration helps to balance the redox state and minimize the accumulation of ROS by dissipating the excess reducing equivalents (NADPH) as well as energy (ATP) (Foyer et al., 2009). By recycling the photorespired CO2, photorespiration may also facilitate carbon assimilation in CO2 limited environments, thereby minimizing photosynthetic inefficiencies resulting from C-limitation (Busch et al., 2013; Xin et al., 2015).
Photorespiration is often considered to be of minor importance in aquatic systems as a result of carbon concentrating mechanisms (CCMs) that facilitate the transport of HCO3- and its dehydration by algal pyrenoids that effectively deliver CO2 to Rubisco (Frost-Christensen and Sand-Jensen, 1992; Madsen et al., 1993; Meyer et al., 2017). In today’s oceanic water (pH ~8.2), 89% of the DIC is in form of HCO3- and only 0.5% exists as dissolved CO2 (Zeebe, 2012). However, not all aquatic C3 plants have similar efficiencies to use both forms of DIC for photosynthesis (Raven and Beardall, 2003; Raven et al., 2011; Raven and Beardall, 2014). Additionally, CO2 acquisition by simple diffusion through the leaf surface is more difficult for submerged plants due to the 10,000-fold lower diffusion rates of gases in a liquid environment relative to air (Borum et al., 2006). Consequently, for aquatic C3 plants such as seagrasses that do not use CCMs effectively, carbon limitation likely increases the photorespiratory function of Rubisco (Tolbert and Osmond, 1976; Touchette and Burkholder, 2000).
Seagrasses are flowering marine plants that evolved from terrestrial monocots in the middle Cretaceous (Larkum et al., 2006b) when higher atmospheric and oceanic CO2 concentrations likely supported photosynthesis and minimized photorespiration (Kuypers et al., 1999; Zeebe, 2012). In colonizing the aquatic habitat, seagrass evolved adaptations to a submerged environment that produced important anatomical differences from their terrestrial ancestors (Zimmerman et al., 1997; Larkum et al., 2006a). Seagrass leaves have no stomatal openings as gas exchange occurs across both leaf surfaces by diffusion, which uncouples carbon uptake from water relations. Seagrasses also have a lacunal system with aerenchyma extending from the roots to the leaves that facilitates the transport of O2 to the roots buried in permanently flooded anoxic sediments, and allows transport of CO2 from the roots to leaves, providing an alternative carbon source (Madsen and Sand-Jensen, 1991). Like their terrestrial ancestors, however, seagrass chloroplasts lack pyrenoids that serve as an important CCM in most aquatic algae (Meyer et al., 2017) and seagrasses are typically less efficient in utilizing HCO3- than macroalgae (Beer et al., 1991). Although Rubisco activity in seagrasses is lower than the typical activities in freshwater emergent angiosperms and marine red algae, it is comparable to that observed in marine green and brown macroalgae (Beer et al., 1991). Simulations of nearshore seawater DIC distribution during the Cretaceous period have predicted that photosynthetic rates of seagrasses would have been similar to macroalgae (Beer and Koch, 1996). However, in today’s oceans, seagrass photosynthesis is generally considered to be carbon limited (Durako, 1993; Beer and Koch, 1996; Zimmerman et al., 1997; Invers et al., 2001).
Carbon limited photosynthesis also restricts seagrasses to shallow, high light environments, where low daytime CO2:O2 ratios in the water column may increase seagrass vulnerability to photorespiration (Buapet et al., 2013b). The photorespiratory pathway was confirmed in marine plants and macrophytes by showing that photosynthesis could be inhibited by increasing the O2 concentration, resulting in higher concentrations of glycolate pathway intermediates (Hough, 1974; Black et al., 1976; Burris et al., 1976; Downton et al., 1976; Hough and Wetzel, 1977; Andrews and Abel, 1979). The decreasing O2 evolution rates relative to electron transfer rates measured by PAM fluorometry at high irradiances in Zostera marina and Halophila stipulacea also suggested a role for photorespiration in these seagrass species (Beer et al., 1998). More recent studies demonstrated the influence of oxygen concentrations and temperature on photorespiration in seagrass that fluctuate in natural environment because of eutrophication, high community productivity and elevated ocean temperatures; and therefore, will play a role in predicting the health status of these plants in warmer climate scenarios (Buapet and Björk, 2016; Rasmusson et al., 2020). The plastochron interval, which defines leaf life span, leaf turnover and elongation rates, plays an important role in photoacclimation strategies that differ among species at the chloroplast, leaf and shoot levels (Schubert et al., 2018). However, we still do not understand how long-term acclimation to climate warming and ocean acidification/carbonation will affect photorespiration and photoprotection in seagrasses (Koch et al., 2013).
Several experiments simulating ocean acidification/carbonation impacts on time scales of hours to >1 year have quantified the positive impacts of CO2 availability on carbon balance, growth, survival and reproductive output in seagrasses (Zimmerman, 2021). During the most recent of these studies, the down-regulation of pigment content with increasing CO2 resembled the photoacclimation response to high light environment that pointed to the importance of metabolic acclimation regulating the redox state of the chloroplast in eelgrass (Zimmerman et al., 2017; Celebi-Ergin et al., 2021). Therefore, the objectives of this study were to estimate the importance of photorespiration in the marine angiosperm Zostera marina L. (eelgrass) under today’s oceanic carbon concentrations and explore the potential acclimation response to prolonged ocean acidification/carbonation by 1) quantifying the photochemical rates under different light and CO2 availability by using eelgrass grown in a high light low CO2 environment, i.e., representing the baseline photosynthetic capacity under today’s oceanic conditions; and 2) comparing the relative contribution of different photochemical pathways in eelgrass after 13 months of acclimation to different CO2 environments superimposed open daily and seasonal patterns of solar radiation, temperature and salinity.
Materials and methods
Table 1 provides a complete list of all abbreviations, acronyms, and symbols along with their units used throughout this paper.
The experimental facility and sampling from pH treatments
Eelgrass shoots used in this study were grown in an outdoor aquatic climate research facility at the Virginia Aquarium and Marine Science Center, VA, USA. The experimental design and control of manipulations for this-long term project were detailed in Zimmerman et al. (2017). Briefly, eelgrass plants, harvested in May 2013 from a subtidal population growing in South Bay, a coastal lagoon on the Virginia portion of the DelMarVa Peninsula, USA, were transplanted into 20 fiberglass open top aquaria (3 m3 each) plumbed with running seawater from Owl’s Creek, VA and exposed to natural sunlight. Temperature, pH, salinity, and irradiance were monitored continuously in all aquaria. Beverage-grade CO2 gas was used to enrich the experimental aquaria from June 2013 to October 2014 using a system of pH-controlled solenoid valves. pH treatment levels ranged from pH 6 ([CO2(aq)] ≅ 2121 µM) to ambient (no CO2 addition, pH ≅ 7.7, [CO2(aq)] ≅ 55 µM), with 0.5 pH intervals between the treatments (4 aquaria at each pH). The experimental CO2 manipulation produced consistently different levels of [CO2(aq)] and pH among the treatments day and night throughout the duration of the 13-month experiment. Plant performance was monitored monthly while environmental parameters, which varied daily and seasonally, were recorded at 10-minute intervals.
During July 2014, after 13 months of cultivation in the experimental aquaria, freshly collected 2nd youngest leaves from pH 6.1 (2121 µM CO2(aq)), pH 6.9 (371 µM CO2(aq)) and ambient pH 7.7 (55 µM CO2(aq)) treatments were harvested for laboratory measurements of photochemistry under fully controlled incubation conditions. Hereafter, the three treatments will be referred to as GpH6, GpH7 and GpH8, for simplicity. During these measurements, the daily seawater temperature in aquaria ranged from 25 to 28°C; allowing all the incubation measurements described here to be conducted at the optimal temperature of 25°C without inducing heat stress. The daily total surface irradiance ranged from 10 to 29 mol photons m-2 d-1; corresponding to more than 6 h of photosynthetically saturating irradiance (>200 µmol photons m-2 s-1) per day, under which conditions the leaves of all plants should have been acclimated to a high light environment (Cummings and Zimmerman, 2003).
Incubation measurements of leaf photochemistry
Photosynthesis and respiration rates were measured using polarographic O2 electrodes in temperature controlled, water-jacketed glass metabolic incubation chambers (Rank Bros., Cambridge, UK). Variable fluorescence was measured simultaneously on each leaf using a Pulse Amplitude Modulated (PAM) fluorometer (Mini PAM, Walz, Germany). Incubation seawater pH (a proxy for dissolved inorganic carbon (DIC) concentration) was measured using an epoxy mini-electrode and pH meter (Cole-Parmer) calibrated with NBS buffers. The lid of the incubation chamber was modified to hold the pH electrode in the incubation water and the miniature fiberoptic probe of the PAM device in close proximity to the leaf surface. The chamber was continuously mixed by a magnetic stirrer which homogenized the incubation medium and provided turbulent flow to reduce boundary layer limitation of gas exchange across the leaf surface. Continuous analog signals from the three sensors were recorded digitally using custom software written with LabView (2009 edition, National Instruments). Voltage data were post processed into metabolic rates using MATLAB R2014 (The MathWorks Inc.). A Kodak slide projector fitted with a halogen (ELH) bulb provided photosynthetically active radiation (PAR). The intensity of PAR was adjusted with neutral density filters and calibrated daily with a QSL 2100 scalar radiometer (Biospherical Instruments Inc.).
Concentrations of CO2(aq) in the aquaria and metabolic incubation chambers were determined from measured values of pH, alkalinity, salinity and temperature using CO2SYS (Ver. 2.1; Lewis and Wallace, 1998). Leaves, harvested from plants grown at pH/CO2 treatments GpH6 ([CO2(aq)] = 2121 µM), GpH7 ([CO2(aq)] = 371 µM) and GpH8 ([CO2(aq)] =55 µM), were used to measure the photosynthetic response at pH/CO2(aq) levels of 6 (MpH6), 7 (MpH7) and 8 (MpH8). Seawater [DIC] and pH in the incubation chambers were adjusted by bubbling with a gas mixture of CO2, O2 and N2 that maintained [O2] at air saturation (~215 µM). Seawater temperature was maintained at 25°C by a circulating water bath. Leaves were cleaned of epiphytes by gently scraping with a razor blade and kept in the dark for 20 minutes before the incubation measurements. A separate three cm long pieces of leaf tissue, cut approximately one cm above the meristem, was used during each ten min dark (i.e., dark respiration) and ten min light (i.e., net photosynthesis) measurement. The pigment content and optical properties of the leaf tissues (Table 1) were measured after each incubation as described by Celebi-Ergin et al. (2021).
The seawater used during all incubations was collected in April 2014 from Owl’s Creek, the tidal estuary used as source water for the experimental facility (Zimmerman et al., 2017). This seawater stock, with salinity of 24 (PSS-78, (Lewis, 1980) was filtered through 0.2 µm Nucleopore membrane filters and stored under refrigeration in dark bottles until used in these experiments. After incubations, alkalinity was determined on aliquots of seawater taken from the chamber using an automated potentiometric titrator (Metroohm). Table 2 summarizes measured parameters of seawater used in metabolic incubation chamber.
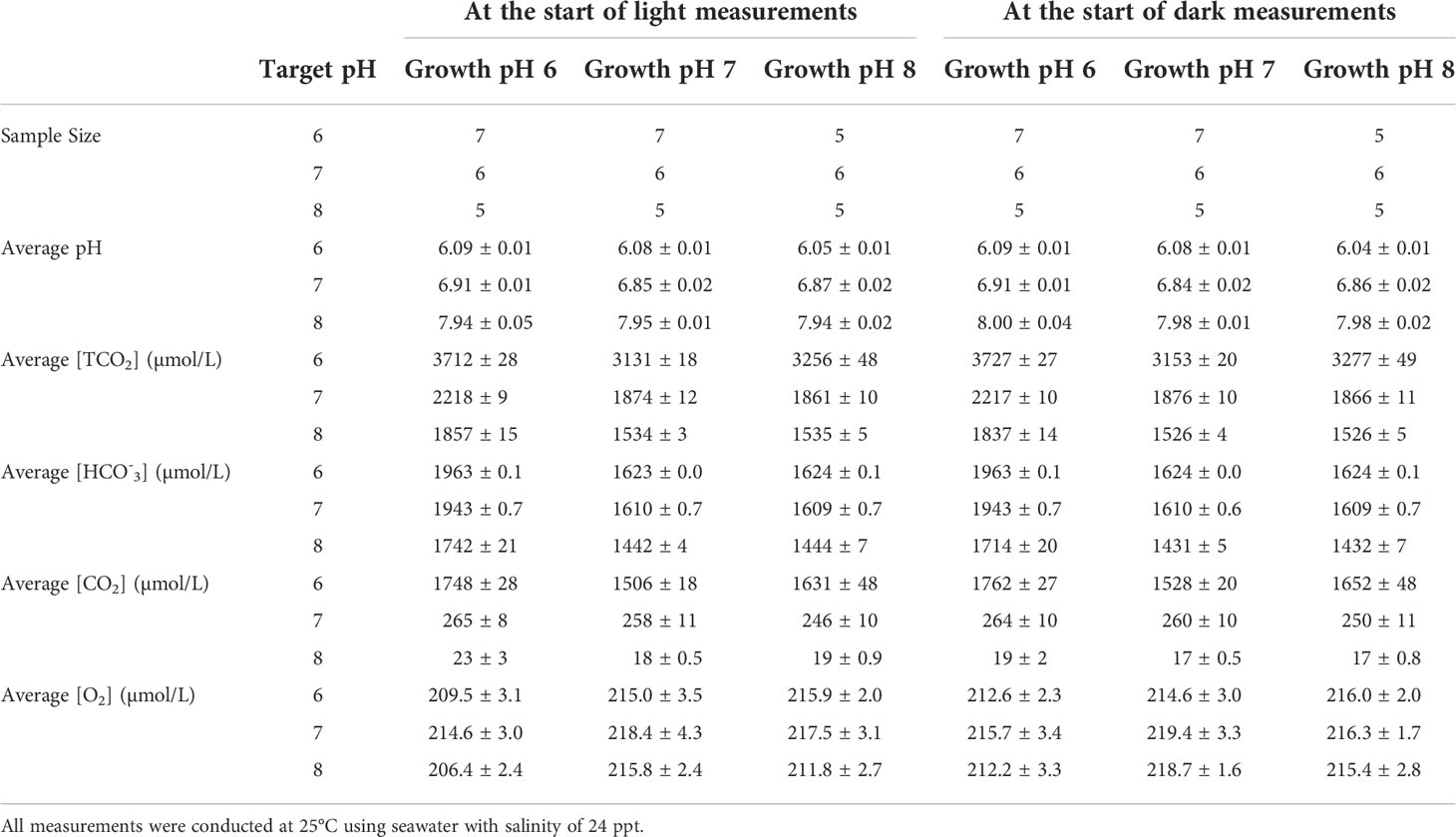
Table 2 Distribution of dissolved inorganic carbon and dissolved oxygen concentrations in seawater during the incubation measurements of net photosynthesis at different light levels, including dark respiration measurements.
Determination of photochemical rates
Oxygen evolution rates of each tissue were separately normalized to fresh weight, leaf area and total pigment concentration to explore the effects of phenotypic differences resulting from acclimation to different growth conditions. Parameters of photosynthesis (P) vs Irradiance (E) curves were estimated by fitting the data to a cumulative one-hit Poisson model pioneered for photosynthesis by Webb et al. (1974):
where Pnet was the measured rate of net photosynthesis and RD was the measured rate of dark respiration, from which the gross photosynthesis (Pg) was calculated according to Equation (1). Pg was defined as a function of light, where PE represented the light-saturated rate of gross photosynthesis that varied with [CO2] and [HCO3-] (sensu McPherson et al. (2015)). Ek was the irradiance threshold for photosynthetic saturation. E was separately defined as photosynthetically available radiation () and as photosynthetically utilized radiation , where A(λ) was the spectral leaf absorptance that integrated the variability of light capture efficiency due to changes in leaf optical properties and pigment content/composition. The quantum yield of oxygen evolution (ΦO2) at different irradiances (in units of mol O2 mol-1 absorbed photon) was calculated by ΦO2 = Pg/PUR. Maximum quantum yield was calculated as Φmax = PE/Ek (PUR).
Although Equation (1) represents the typical method for determining gross photosynthesis from measured values of Pnet and RD, the model does not separately account for O2 consumed by photorespiration in the chloroplast. It also assumes that the Mehler Ascorbate Peroxidase pathway does not affect net O2 exchange even though it may facilitate ATP generation and electron flow, which might be detected by fluorescence measurements (Larkum et al., 2006a). Following the principle explained by Raghavendra (2000) gross photosynthesis (Pg) can be detailed as the difference between true photosynthesis (PT) and photorespiration (PR):
Under CO2-saturation (i.e., at low pH that increases CO2:O2 ratio in seawater, Table 2), PR should approach a minimum (~ 0), so that Pg will be an approximate estimate of true photosynthetic O2 production (PT). In this study, O2 production rates measured at pH 6 were assumed to approximate the true photosynthesis (PT) for each growth condition. Therefore, photorespiration was calculated by subtracting the carbon limited Pg measured at pH > 6 from Pg measured at pH 6:
Thus, Pg approached PE when saturated by light and flow, and it approached the true physiological capacity (Pm) when saturated by CO2, light and flow. In this formulation, the limit of Pm is set by availability of cellular components such as enzyme and pigment concentrations that may change under different growth conditions.
Pulsed Amplitude Modulation (PAM) fluorescence measurements were analyzed following the calculations outlined in Baker (2008). The maximum (Fm) and minimum (F0) fluorescence emissions were measured in the dark after at least 10 min of acclimation while simultaneously measuring respiration. The maximum variable fluorescence yield (Fv = Fm - F0) was used to quantify the maximum quantum yield of fluorescence (Fv/Fm), which is a measure of maximum efficiency at which absorbed light by photosystem II (PSII) can be used for photochemistry. The maximum (F’m) and minimum fluorescence (Ft) emissions induced by the short saturating pulse of PAM were measured again in the light while simultaneously measuring Pnet. Based on these emissions under the presence of the actinic background light, the effective quantum yield of PSII (ΦPSII), also known as photochemical quenching, was determined as:
ΦPSII provides an estimate of the quantum yield of linear electron flow through PSII at a given irradiance. The other non-radiative energy loss that quenches fluorescence, called Non-Photochemical Quenching (NPQ), results from the dissipation of excess excitation energy as heat via the Xanthophyll cycle. NPQ was estimated as:
For comparisons among the treatments and incubations, NPQ and ΦPSII at different light levels were fitted to a four-parameter sigmoid curve, which is commonly used for dose response analysis (Motulsky and Christopoulos, 2004), with the following formula:
where the exponent H was Hill slope that controlled the steepness of the dose-response curve. EC50 was the PUR level required to provoke a response halfway between the baseline and maximum responses. The threshold for NPQmax was constrained to 10 based on literature values (Kalaji et al., 2014).
The electron transport rate (ETR) was estimated from ΦPSII as:
where FII was the fraction of PUR captured by PSII and its light harvesting complexes (LHC). The typical value of FII for Chlorophyta and seagrasses is about 0.5 (Figueroa et al., 2003; Larkum et al., 2006a). Photosynthetic parameters of ETR curves (i.e., ETRmax, αETR and Ek -ETR) were calculated by modifying the model of O2 based P vs E. curves (Equation 2):
Linear electron flow through PSII is directly related to photosynthetic oxygen production, therefore the gross photosynthesis based on fluorescence measurements (Pg-ETR) were estimated from ETR as:
where τ was the ratio of oxygen evolved per electron generated at PSII. Since four stable charge separations are necessary to generate one mole of O2 at PSII, τ is equal to 0.25.
Statistical analysis
Effects of growth [CO2] on pigment content and optical properties of leaves were analyzed by one-way Analysis of Variance (ANOVA) followed by the Tukey multiple comparison method when significant overall effects were identified. Effects of growth [CO2] and measurement [CO2] on dark respiration rates, measured with the O2 evolution method, were analyzed by Analysis of Covariance (ANCOVA).
O2 evolution and fluorescence models were implemented by using the non-linear curve fitting tools in SigmaPlot (Systat Software Inc., Version 13.0). This tool provided the mean estimates of the model parameters with their error estimates and significances using computational procedures described by Draper and Smith (1981) and Zimmerman et al. (1987). Additionally, analysis of variance for the regression models were presented to account for the goodness of fit of the P vs E curves for each experimental condition (Supplementary Tables 1–3). Significant effects of measurement [CO2] and growth [CO2] on model parameters obtained by non-linear regression were analyzed by ANCOVA, with growth pH as the primary (categorial) factor and measurement pH as the continuous covariate.
Results
Photoacclimation to growth CO2
Pigment content and optical properties varied significantly among the leaves grown in different [CO2] treatments (Table 3). Both total chlorophyll and carotenoid content decreased with increasing growth [CO2], while the molar ratio of Total Car : Total Chl remained constant across CO2 treatments at about 0.27. The decrease in total chlorophyll resulted in an increased optical cross section (aL*(λ)) with growth [CO2], thereby reducing the package effect that results in Chlorophyll self-shading. Growth [CO2] increased the thickness of the unpigmented mesophyll, thereby increasing the leaf biomass per unit of surface area. These phenotypic responses, consistent with the long term acclimation responses described by Celebi-Ergin et al. (2021), had important consequences for the comparison of photosynthetic efficiencies when metabolic rates were normalized to different leaf properties.
Light response curves of oxygen flux
Rates of dark respiration, whether normalized to biomass (RD (FW)) or leaf area (RD (LA)), were not affected by growth [CO2] or instantaneous variations of [CO2] within the metabolic incubation chambers (Table 4). Therefore, the average rate of dark respiration for all samples combined was 5.96 ± 0.31 µmol O2 hr-1 g-1 FW or 0.50 ± 0.03 µmol O2 m-2 s-1. Dark respiration rates were also independent of pH within the range examined here, indicating no negative impact of changing ionic composition on respiration.
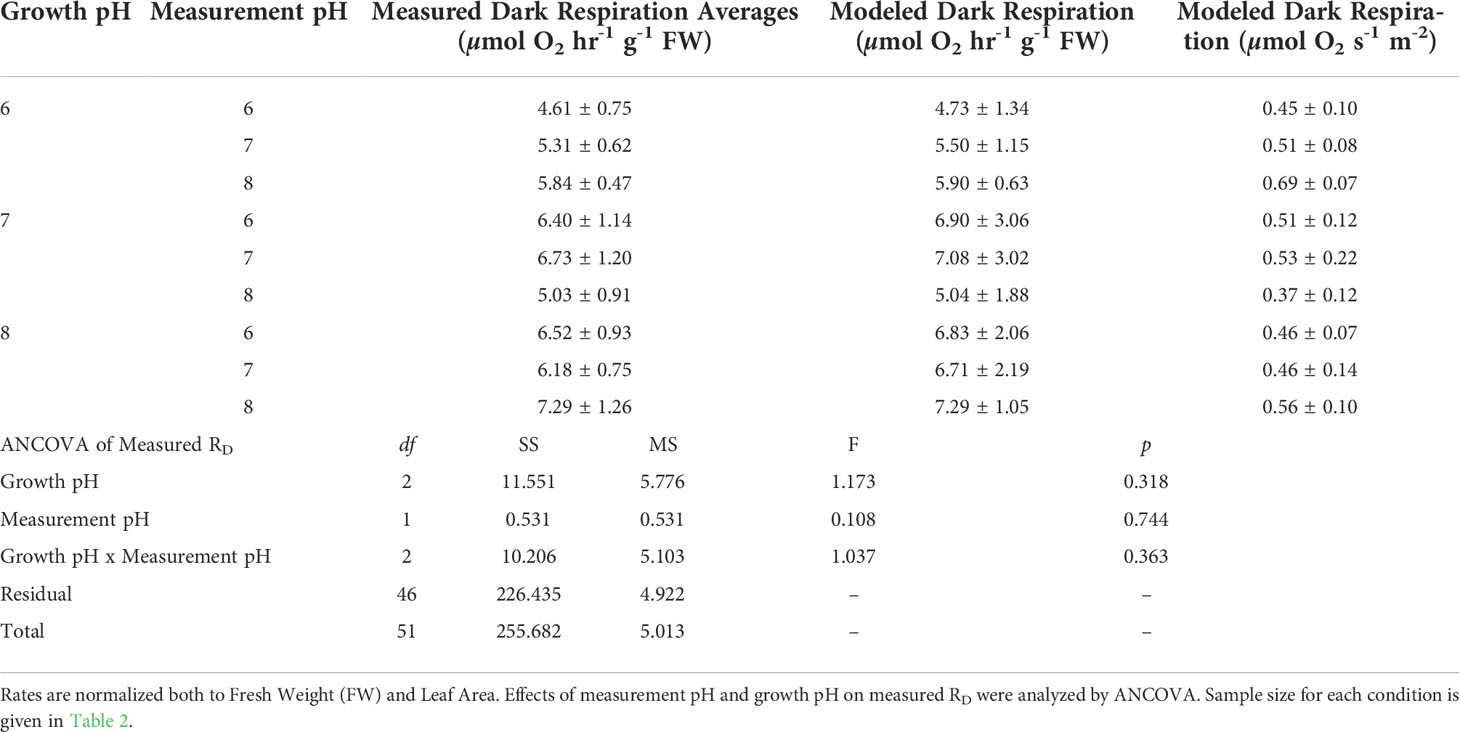
Table 4 Dark respiration (RD) rates measured with O2 evolution method and estimated by non-linear model fit to P vs E curves.
In contrast, net O2 production rates increased with light and incubation [CO2] for all plants, regardless of the CO2 environment in which they were grown (Figure 1). The biomass specific rate of light-saturated photosynthesis (PE (FW)) averaged 14.1 µmol O2 hr-1 g-1 FW at low incubation [CO2] for all plants and increased as a function of incubation [CO2] (Figure 1). However, PE (FW) of the plants grown under ambient conditions (GpH8) was twice as sensitive to increasing incubation [CO2] as plants grown under the highest CO2 enrichment (GpH6) (Table 5, 86.8 vs 33.5 µmol O2 hr-1 g-1 FW at MpH 6 respectively). This difference was associated with 2-fold higher biomass specific pigment content of the plants grown under ambient [CO2] (Table 3). Thus, low rates of oxygen evolution by ambient plants in their natural low CO2 environment resulted mainly from photorespiration and not the lack of photosynthetic capacity characterized by light harvesting, electron transport and carbon fixation.
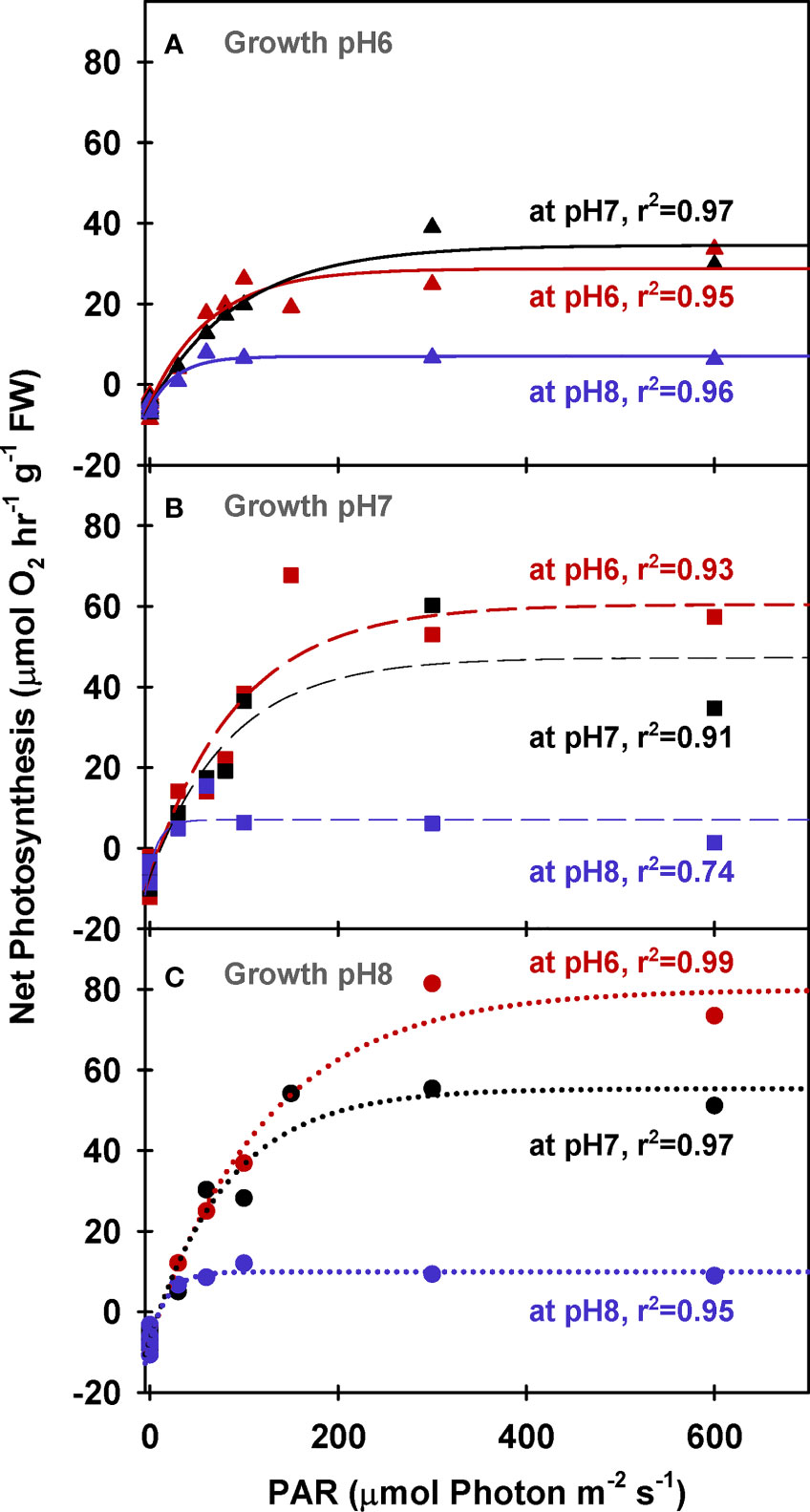
Figure 1 Net photosynthesis of eelgrass leaves (biomass normalized) as a function of irradiance. O2 production rates were measured at different pH levels (red: pH6, black: pH7 and blue: pH8) using leaves grown at pH6 (2121 µM CO2(aq)) (A), pH7 (371 µM CO2(aq)) (B) and ambient pH8 (55 µM CO2(aq)) (C). Curves were fit using Equation (2).
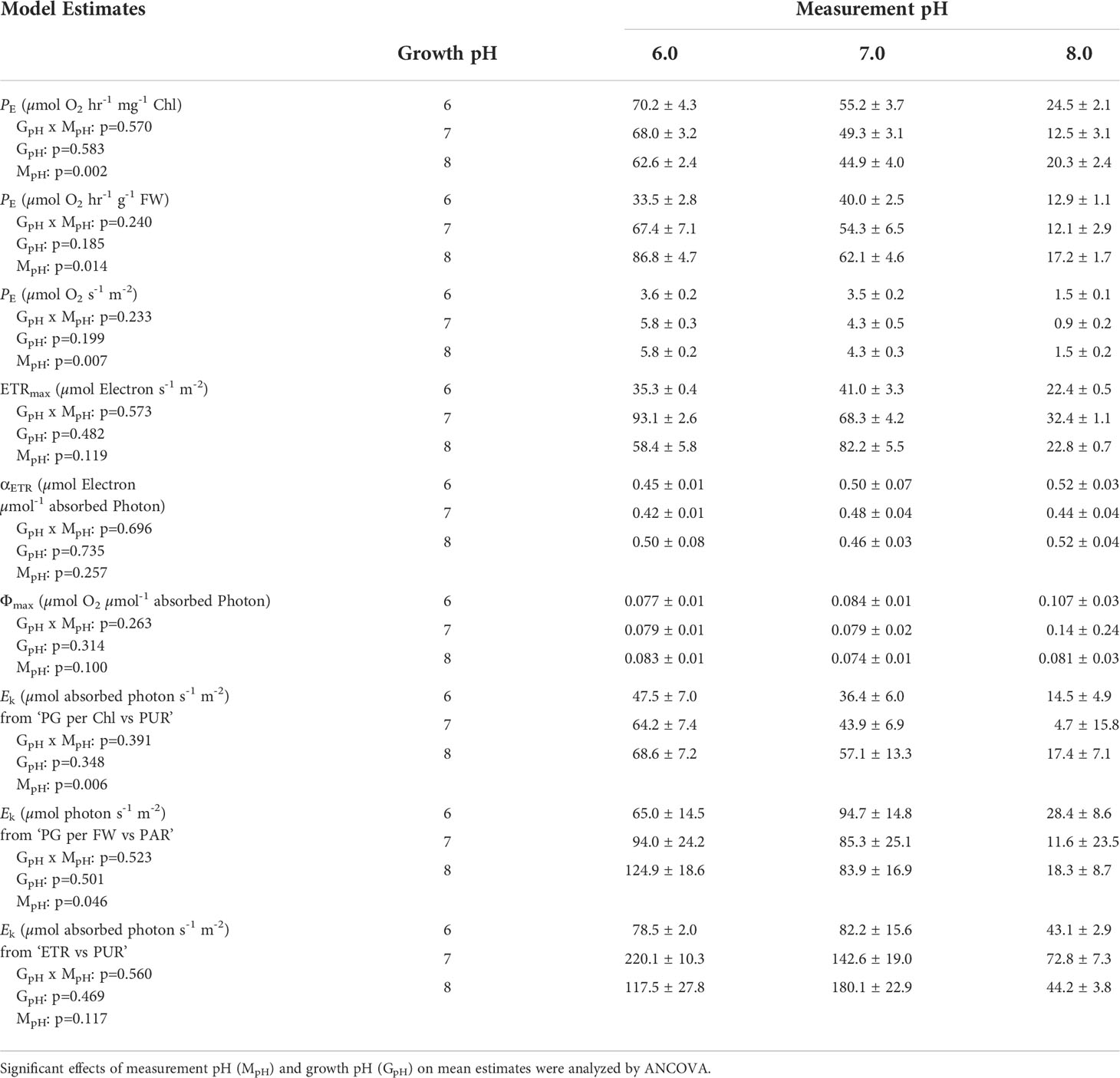
Table 5 Model estimates (mean ± 1 SE) of photosynthesis parameters generated by non-linear regression fit to the experimental data using Equation (2) (N.S. stands for non-significant parameter estimate).
For all plants, increased incubation [CO2] also increased the irradiance required to saturate photosynthetic oxygen production (Ek (PAR) and Ek (PUR)); rather than changing the photosynthetic efficiency (α) within the light limited region of P versus E response curves (Table 5 and Supplementary Table 1). Overall, photoacclimation of eelgrass leaves to ocean carbonation increased Ek (PUR) values from 17 to 44 and 48 µmol absorbed photon s-1 m-2 for pH 8 (55 µM CO2(aq)), pH 7 (371 µM CO2(aq)) and pH 6 (2121 µM CO2(aq)) respectively.
Chlorophyll specific rates of light-saturated photosynthesis (PE (Chl)) were the same for all plants grown at different CO2 environments and produced an identical response to incubation [CO2] (Figure 2). Consequently, the O2 production efficiency per unit chlorophyll was not affected by the CO2 environment in which the plants were grown (Table 5 and Supplementary Table 2) and the stimulatory effect of [CO2] on O2 evolution was instantaneous (Figure 3A). The most likely explanation for this instantaneous response would be a reversible and light dependent O2 consuming process involving the chloroplast, such as photorespiration (PR), that is competitively inhibited by increasing [CO2]. Therefore, for all plants grown under all treatments, PE (Chl) rates at high incubation [CO2] (i.e., at MpH6) were assumed to be the true physiological photosynthetic capacity (Pm) under light, carbon and flow saturation. Based on this assumption, photorespiration rates were quantified by solving the Equation 4 with the chlorophyll specific gross photosynthesis models (Figure 3B). Normalizing the models to pigment, rather than biomass or area, eliminated the effect of morphological differences among the plants on net oxygen metabolism.
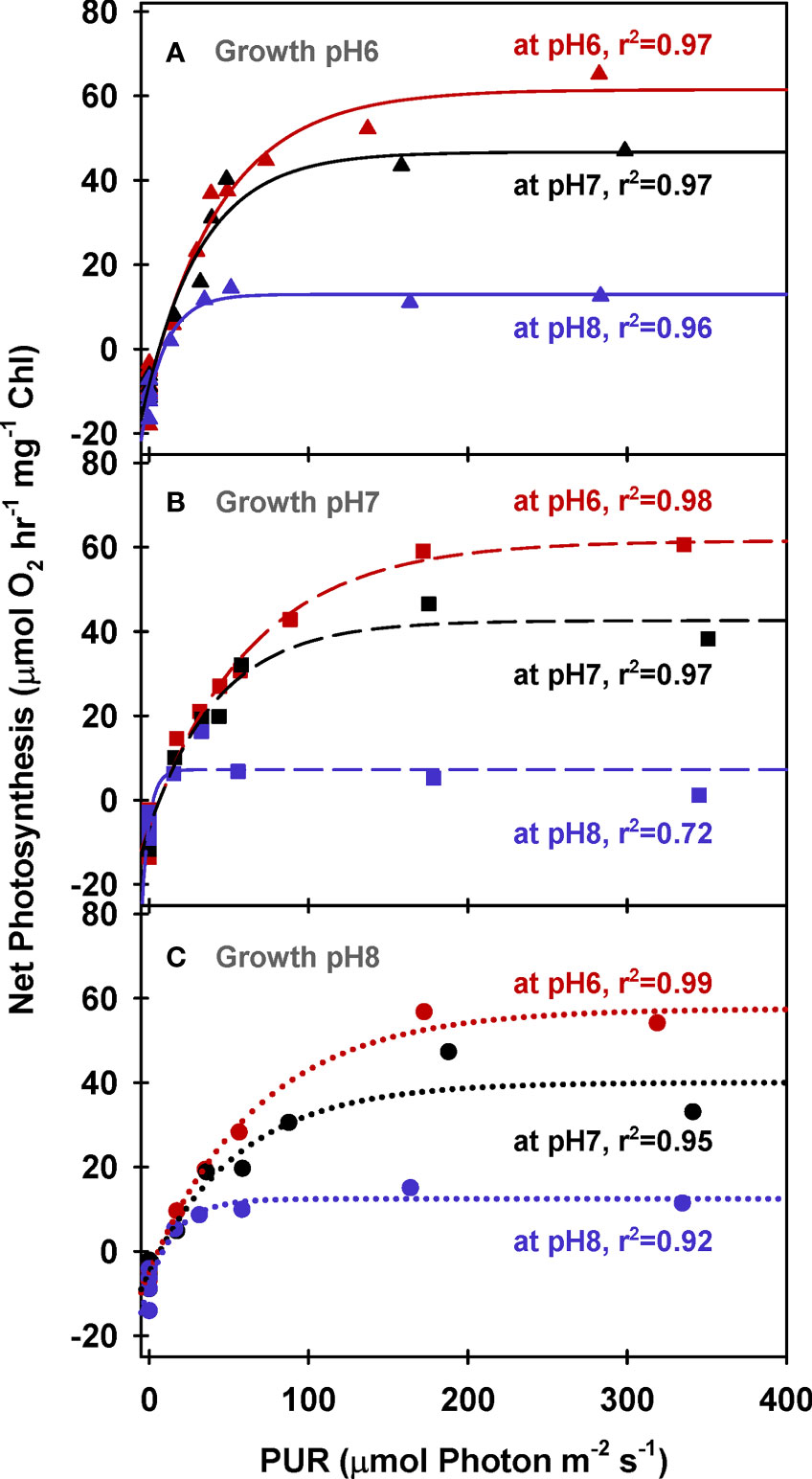
Figure 2 Net photosynthesis of eelgrass leaves (Chlorophyll normalized) as a function of absorbed irradiance. O2 production rates were measured at different pH levels (red: pH6, black: pH7 and blue: pH8) using leaves grown at pH6 (2121 µM CO2(aq)) (A), pH7 (371 µM CO2(aq)) (B) and ambient pH8 (55 µM CO2(aq)) (C). Curves were fit using Equation (2).
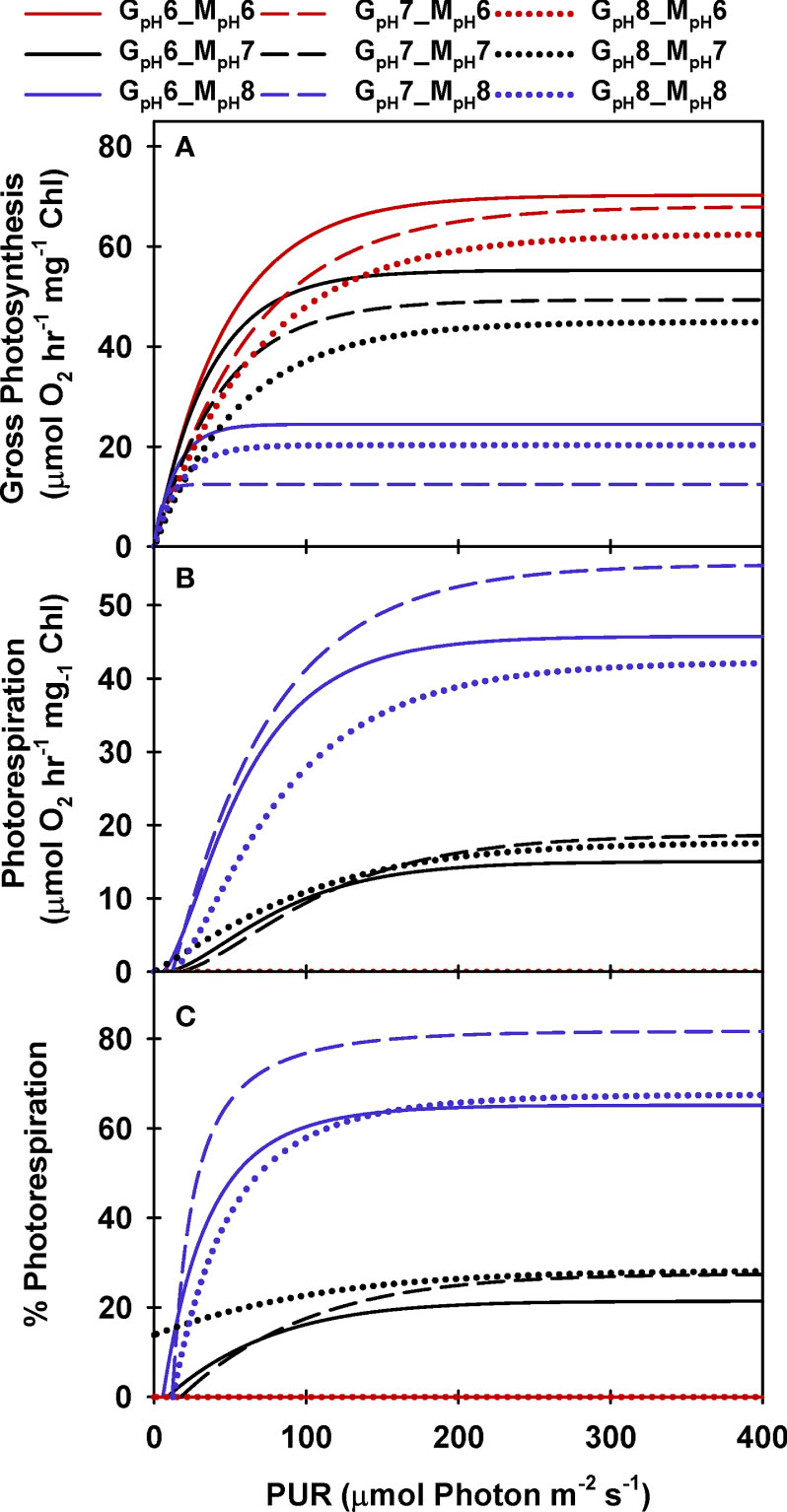
Figure 3 Modeled gross photosynthesis (A) and photorespiration (B, C) of eelgrass leaves as a function of absorbed irradiance. Colors represent different pH/CO2 levels at which the measurements (MpH) were performed; line styles represent the different pH/CO2 levels at which the plants were grown (GpH). Photorespiration at MpH6 were zero.
Like photosynthesis, photorespiration increased with light under constant [CO2], but decreased with increasing incubation [CO2], as carboxylation became increasingly favored over oxygenation (Figure 3B). Predicted PR rates increased rapidly with light to a maximum of 60 to 80% of Pm at low [CO2] (i.e., MpH8) (Figure 3C). When aqueous [CO2] was equal to aqueous [O2] (at MpH7, Table 2), maximum PR rates were only 20% of Pm, which is equivalent to the inherent carboxylation: oxygenation ratio of Rubisco.
All plants reached the lowest gross photosynthesis to dark respiration ratio (Pg : RD) of 2 at low incubation [CO2] when light saturated (Figure 4A). This ratio increased instantaneously when saturated with CO2 in the incubation medium, maximally up to 12 for ambient plants (GpH8). However, the PE : RD ratio of high CO2 grown plants peaked at 8 when saturated with CO2 in the incubation medium, illustrating the consequence of pigment acclimation on metabolic balance of plants grown in a high CO2 environment (Figure 4B, grey arrows). Having excess pigment content in a CO2-limited environment (as observed in ambient plants) did not improve the PE : RD under normal growth conditions even though it allowed the instantaneous 6-fold increase of PE : RD when incubation [CO2] increased. High CO2 acclimated plants, on the other hand, maintained a 4-fold higher PE : RD above ambient plants at their respective growth [CO2] even though pigment content of the high CO2 plants decreased by half.
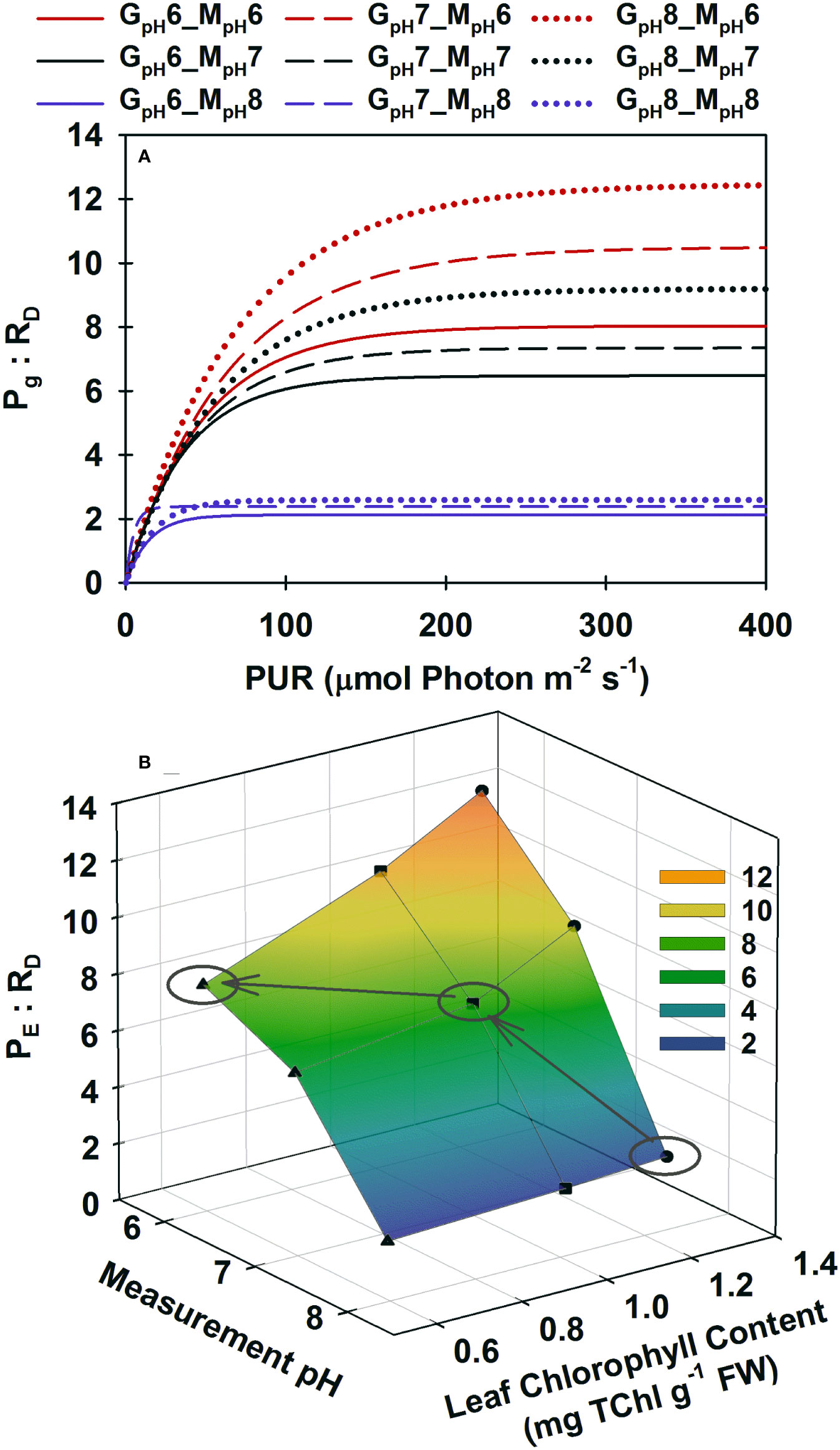
Figure 4 Modeled ratio of gross photosynthesis to dark respiration as a function of absorbed irradiance (A) and as a function of Chlorophyll content at saturating irradiances (B). Colors represent different pH/CO2 levels at which the measurements (MpH) were performed; line styles and symbols (▲, at pH6 ◼ at pH7, ● at ambient pH) represent the different pH/CO2 levels at which the plants were grown (GpH). (B) Ellipses highlight when plants from different treatments were incubated at their corresponding growth pH/CO2. Gray arrows show the trajectory of PE : RD as a result of phenotypic acclimation to the increasing CO2 environment.
Light response curves of variable fluorescence
Maximum quantum yields of fluorescence by dark-adapted leaves were above 0.7 regardless of incubation [CO2], indicating leaves from all growth treatments were healthy during the experiments (ΦPSII at PUR 0 µmol absorbed photon s-1 m-2, Figure 5). For all plants, effective quantum yields of fluorescence (ΦPSII) decreased faster with increasing light when the incubation [CO2] was low (MpH8). The decreased photochemical yield resulted from rapid induction of non-photochemical quenching (NPQ) when [CO2] was limited under light saturation (Figure 6). Increasing the growth [CO2], however, caused the light-dependent onset of NPQ to increase, as the NPQ pathway saturated more quickly due the decreased carotenoid content of leaves grown under high [CO2] (Table 3).
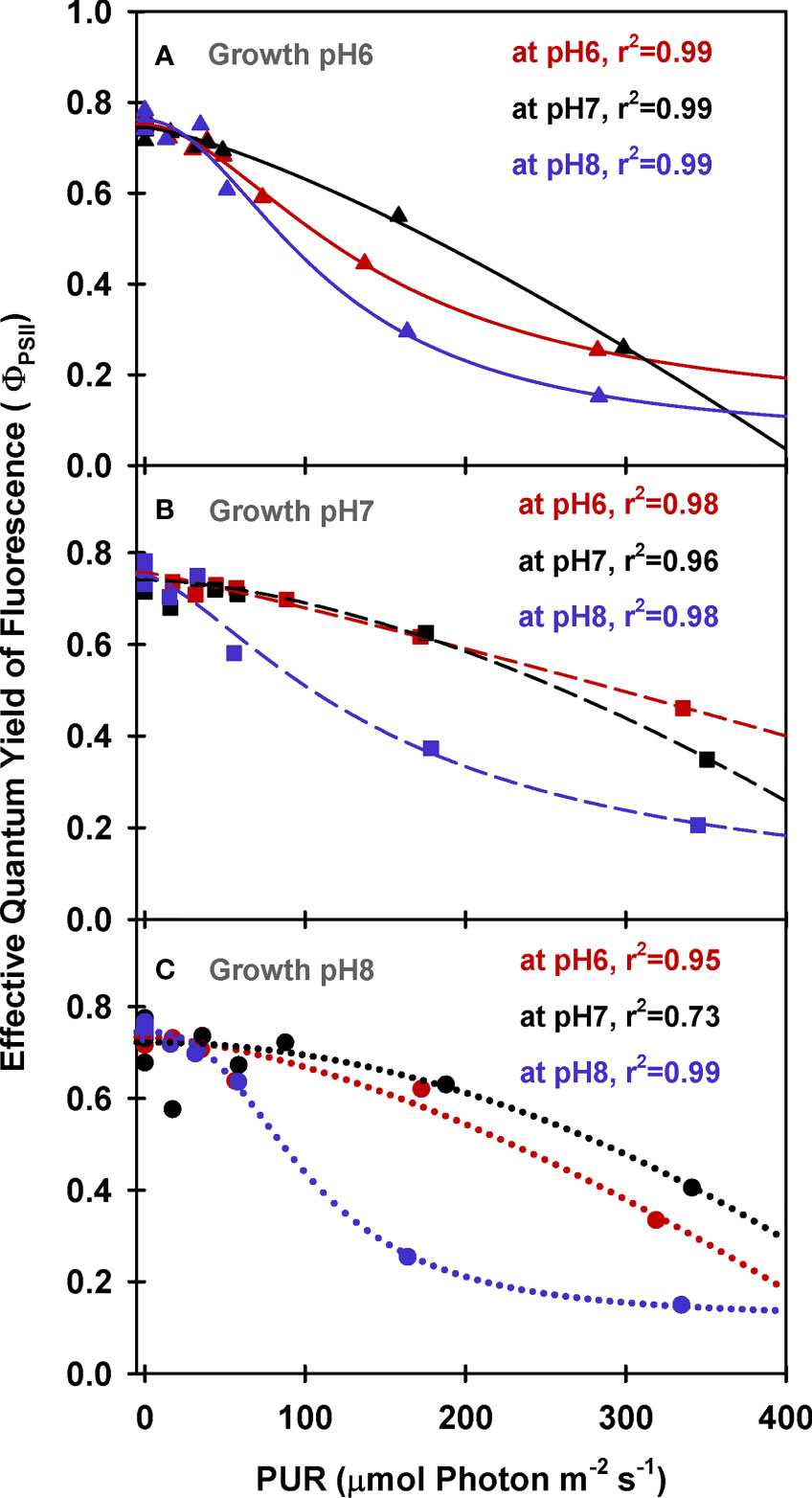
Figure 5 PAM fluorescence parameters of eelgrass leaves as a function of absorbed irradiance. PAM fluorescence measurements were performed at different pH/CO2 levels (red: pH6, black: pH7, blue: pH8) using leaves grown at pH6 (2121 µM CO2(aq)) (A), pH7 (371 µM CO2(aq)) (B) and ambient pH8 (55 µM CO2(aq)) (C). Curves were fit using Equation (9).
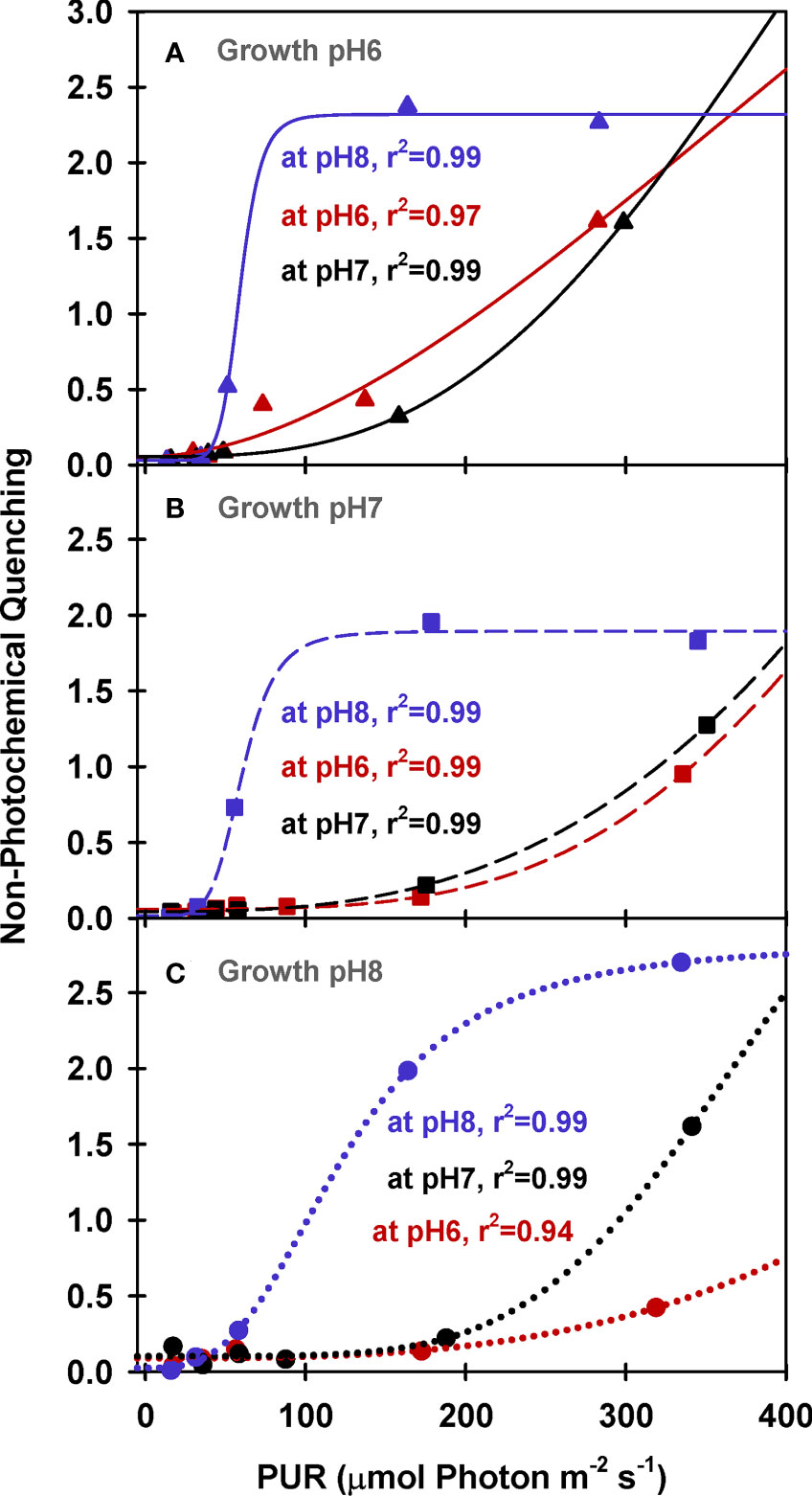
Figure 6 PAM fluorescence parameters of eelgrass leaves as a function of absorbed irradiance. PAM fluorescence measurements were performed at different pH/CO2 levels (red: pH6, black: pH7, blue: pH8) using leaves grown at pH6 (2121 µM CO2(aq)) (A), pH7 (371 µM CO2(aq)) (B) and ambient pH8 (55 µM CO2(aq)) (C). Curves were fit using Equation (9).
Under saturating irradiance (350 µmol absorbed photon s-1 m-2, Figure 6), NPQ values of ambient plants increased 5-fold as incubation [CO2] became increasingly limiting. In contrast, the plants grown under high [CO2] (GpH6) yielded the same light-saturated NPQ of 2.5 regardless of incubation [CO2]. The dynamic range of NPQ regulation in ambient grown plants in response to instantaneous changes in [CO2] suggests considerable tolerance for fluctuating environmental conditions (Figure 6C).
The relation between quantum yield of fluorescence (ΦPSII) and quantum yield of oxygen evolution (ΦO2) was nonlinear, and their ratios were closest to the theoretical value of 8 only at low light and high [CO2] conditions (Figure 7). For this ratio to be higher than 8, either less than half of the photons are directed to PSII (i.e., FII<0.5, Equation 10), and/or more than four electrons are processed to evolve one mole of oxygen (i.e., τ<0.25, Equation 12). Both outcomes highlight deviation from linear electron flow. For ambient plants, ΦO2 decreased faster than ΦPSII with increasing light resulting in a drastic increase in ΦPSII:ΦO2, especially at their growth CO2 (GpH8), suggesting that these plants were using an alternative pathway to maintain electron flow without the production or consumption of O2.
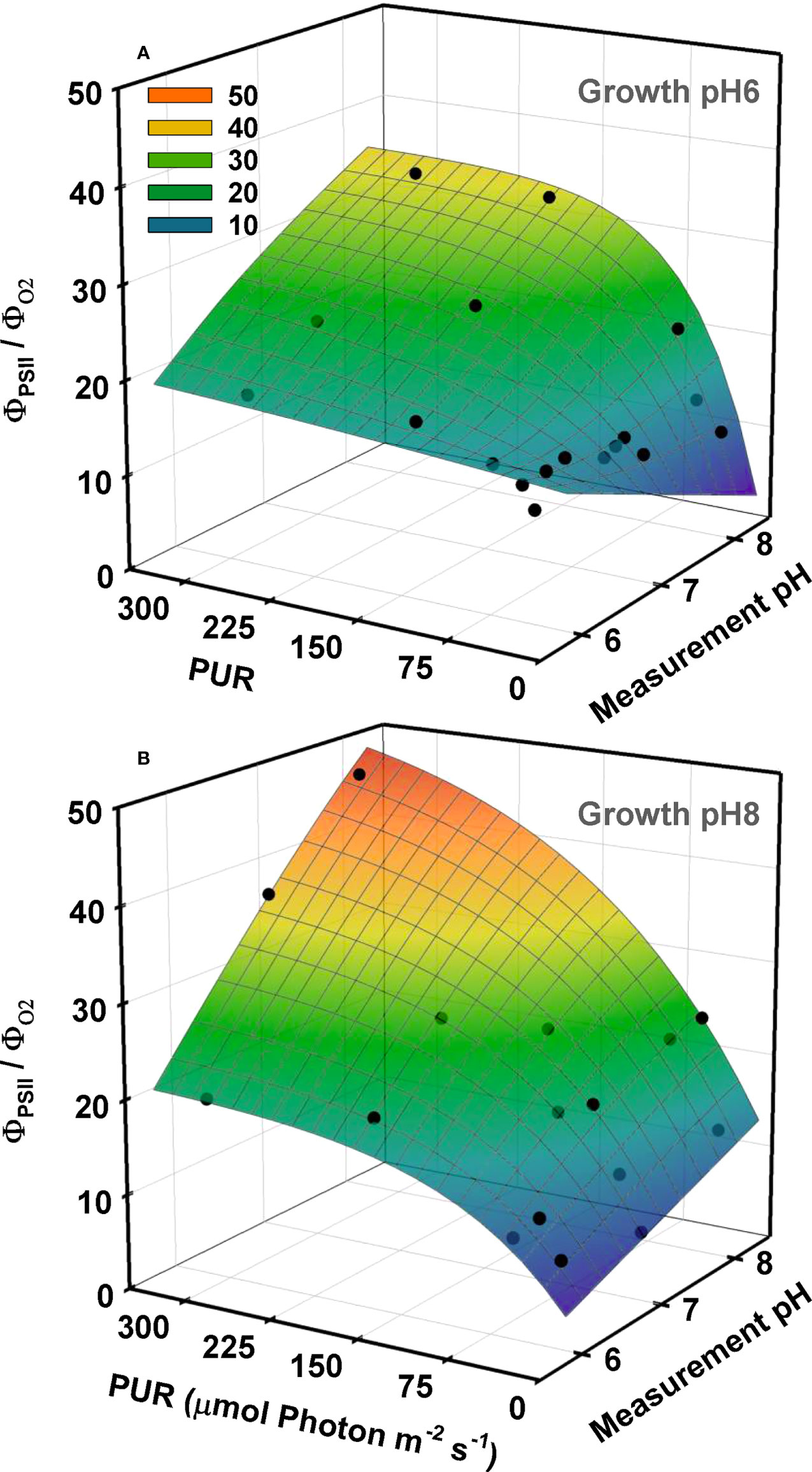
Figure 7 Ratio of the quantum yield of fluorescence (ФPSII) to the quantum yield of oxygen (ФO2) as a function of light and incubation pH/CO2. O2 production and fluorescence were measured simultaneously at different pH levels using eelgrass leaves grown at different CO2 treatments. Yields were calculated using PUR. Growth specific 3D relationships, at pH6 (2121 µM CO2(aq)) (A) and ambient pH8 (55 µM CO2(aq)) (B), were generated by the combination of non-linear and linear regression fits. First, the ФPSII/ФO2 ratio as a function of PUR were described by the exponential rise to maximum models separately for each incubation pH/CO2. Parameter estimates of these non-linear regression models were fitted as a function of incubation pH using linear regression.
Similar to net photosynthesis rates, electron transport rates (ETR) of all plants increased with light and were lowest at low incubation [CO2] (i.e., MpH8) (Figure 8 and Table 5). However, the increase of ETRmax with incubation CO2 was not consistent among the plants due to the non-monotonic response of ΦPSII with incubation [CO2] (Figure 5), in agreement with the findings by Celebi (2016). Only ETRmax of plants grown at GpH7 increased consistently with increasing incubation [CO2]. For all incubation experiments, PUR levels required to saturate ETR (Ek-ETR) were consistently higher than the Ek values required to saturate O2 production (Table 5 and Supplementary Table 3). For all plants, estimated gross photosynthesis based on ETR were also higher than the gross photosynthesis measured by the O2 evolution method (Figure 9). However, this overestimation was not consistent among plants grown at different CO2 environments. The PE (LA) to ETRmax ratio was around 0.1 for pH 6 and pH 8 plants when incubated at pH 6 and pH 8, instead of the theoretical value (τ) of 0.25 (Table 5).
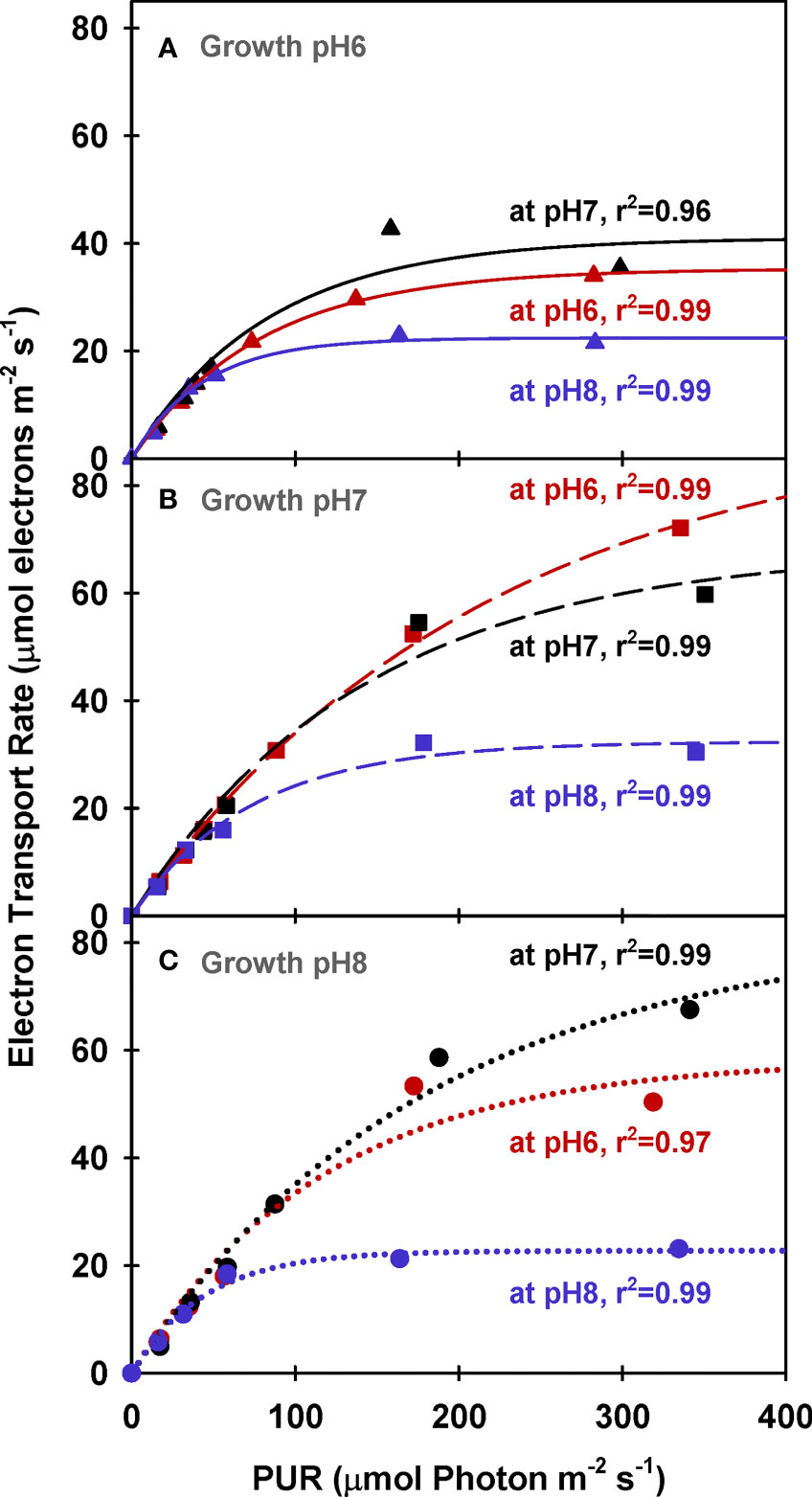
Figure 8 Electron transport rates of eelgrass leaves as a function of absorbed irradiance. PAM measurements were performed at different pH/CO2 levels (red: pH6, black: pH7 and blue: pH8) using leaves grown at pH6 (2121 µM CO2(aq)) (A), pH7 (371 µM CO2(aq)) (B) and ambient pH8 (55 µM CO2(aq)) (C). Curves were fit using Equation (11).
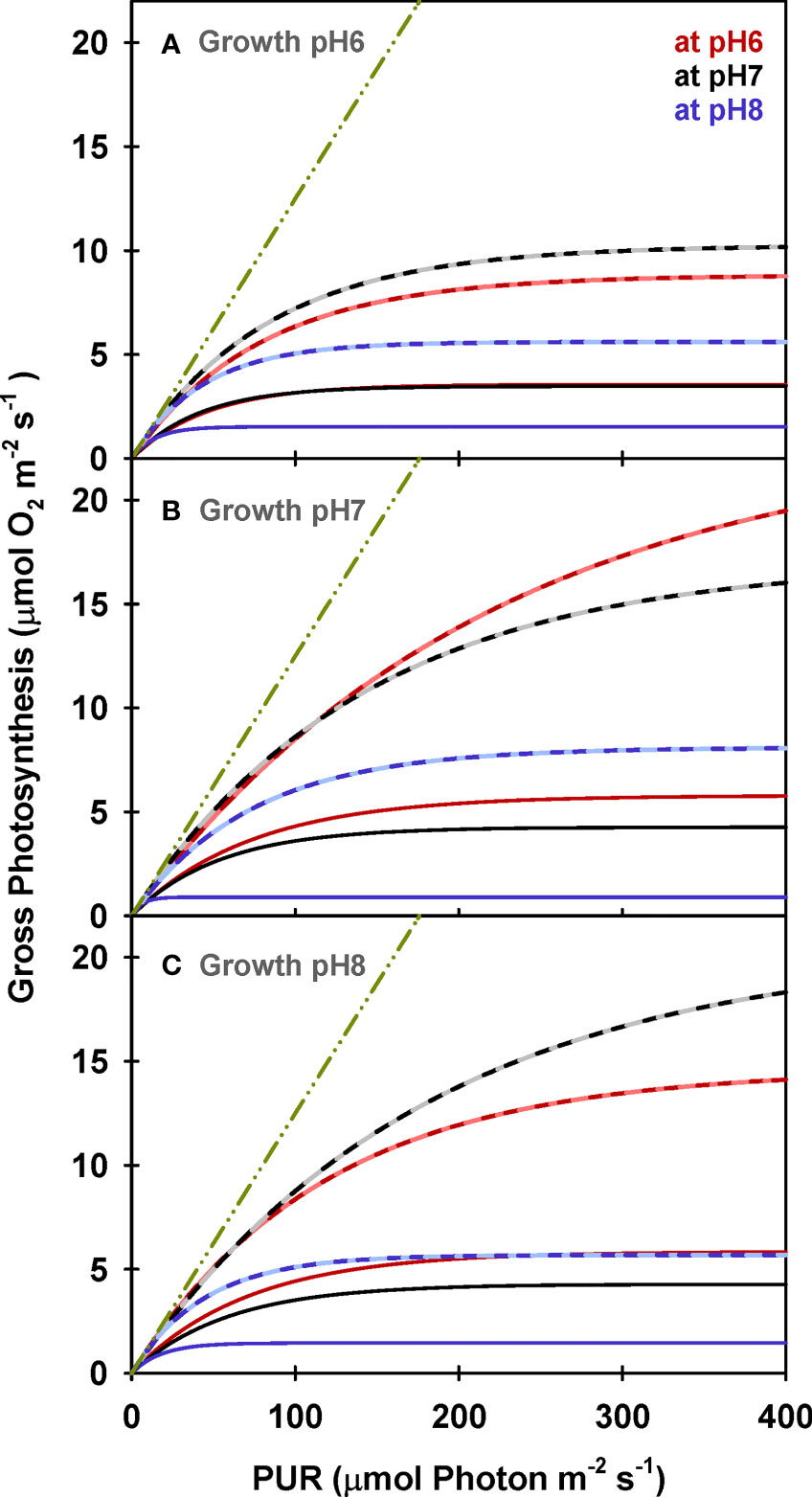
Figure 9 Modeled gross photosynthesis of eelgrass leaves as a function of absorbed irradiance for plants growing at pH6 (2121 μM CO2(aq)) (A), pH7 (371 μM CO2(aq)) (B) and ambient pH8 (55 μM CO2(aq)) (C). Solid lines are calculated from leaf area normalized O2 production rates (Equation 1) and dashed lines are estimated from ETR measurements (Equation 12). Colors represent incubation pH/CO2 levels. Green dot-dashed lines represent the theoretical O2 production per absorbed photon under non-limiting environmental conditions.
Discussion
Long-term growth under high [CO2] produced a remarkable combination of morphological and metabolic changes in eelgrass. Although pigment content decreased in plants grown at high CO2, leaf biomass increased as a direct result of the CO2-stimulated increase in photosynthetic carbon gain. The equivalent responses of chlorophyll normalized O2 production rates to increased incubation [CO2], independent of the growth CO2, allowed us to quantify the impact of [CO2] on photorespiration in eelgrass because the instantaneous difference in O2 production rates in CO2-saturated vs. CO2-limited incubation media corresponded to the amount of O2 consumed in the photorespiratory pathway. Thus, photosynthesis and photorespiration as a function of light for each growth condition were precisely predictable using the P versus E curves, although the responses to incubation CO2 differed between biomass and pigment normalization due to changes in leaf morphology. Presently, models of eelgrass performance do not consider these long-term morphological and metabolic acclimation responses (Zimmerman, 2003; Zimmerman, 2006; Zimmerman et al., 2015). Thus, the quasi-mechanistic model developed in this study permits integration of the photosynthetic and morphological acclimation due to ocean carbonation into seagrass productivity models, by adjusting the limits of the photosynthetic parameters based on substrate availability and physiological capacity.
Morphological acclimation and regulation of pigment content, Rubisco activity, light capture and carbon fixation as a function of CO2 availability have been previously observed in freshwater angiosperms (Madsen et al., 1996). Increasing Pg : RD due to the enhancing impact of [CO2] on PE was detected even in short term (2-6 weeks) studies using temperate and tropical seagrass species without any CO2 effect on pigment content (Zimmerman et al., 1997; Ow et al., 2015). Long term studies, moreover, reported significant increases in total shoot biomass, carbon allocation to roots and rhizomes (blue carbon), shoot survival and reproductive output by eelgrass in response to CO2 availability (Palacios and Zimmerman, 2007; Zimmerman et al., 2017). Despite the decreases in pigment content and leaf absorptance observed here, plants grown at high CO2 were able to maintain higher Pg : RD ratios than plants grown under ambient CO2; indicating a strong coupling between the regulation of photosynthetic structure and metabolic carbon demands. This coupling between photosynthetic regulation and growth might be poor for organisms that undergo photodamage because photosynthesis might accommodate the biochemical costs associated with protection and recovery rather than fueling the energy towards growth (Barra et al., 2014). On the other hand, the eelgrass used in these experiments show no sign of photodamage, either in the growth aquaria or in laboratory incubations even when photosynthesis was carbon limited but light saturated.
When measured at low [CO2], plants grown under ambient CO2 had the same photosynthetic O2 production as the plants grown at high [CO2]. These same photosynthetic rates highlighted the apparent lack of carbon concentrating mechanisms inducible by low CO2 availability in eelgrass, in contrast with marine algae and cyanobacteria that are capable of upregulating their carbon concentrating mechanisms via e.g., generation of pyrenoids, carboxysomes and periplasmic carbonic anhydrases when CO2 availability becomes limiting (Björk et al., 1993; Raghavendra, 2000; Falkowski and Raven, 2007; Meyer et al., 2017). This was also consistent with the limited sensitivity of eelgrass photosynthesis to the aqueous presence of acetazolamide, an inhibitor of periplasmic carbonic anhydrase (McPherson et al. (2015) and Celebi-Ergin - unpublished data). Seagrasses living in shallow estuarine environments, like the Chesapeake Bay eelgrass used in this study, are subject to highly variable CO2/pH levels daily and seasonally, which might explain the unresponsiveness of CCMs for ambient plants (Buapet et al., 2013a; Duarte et al., 2013; Ruesink et al., 2015; Zimmerman et al., 2017; Cyronak et al., 2018). Similarly, all plants had the same PE (Chl) when measured at saturating [CO2] due to minimized PR, indicating all plants approached the same physiological oxygen production capacity per available photosynthetic apparatus (i.e., Pm (Chl) was constant across all treatments). Therefore, the difference in PE : RD among growth [CO2] treatments when all were incubated at high [CO2] resulted from the downregulation of light harvesting components by plants grown in the high CO2 environment.
Despite phenotypic acclimation across the CO2 gradient, the maximum photosynthetic efficiency (Φmax) remained constant for all plants (~0.08 mol O2 mol-1 absorbed photon) but photosynthesis-saturating light levels (Ek) increased, as was predicted by the model of McPherson et al. (2015). Photosynthetic efficiency within and among seagrass species vary with efficiency of light absorption and the subsequent conversion of that energy into carbon assimilation (Ralph et al., 2007). Although the observed values of α in this study were in agreement with previous estimates for eelgrass (Frost-Christensen and Sand-Jensen, 1992), constant α across different CO2 regimes represents an interesting contrast to that observed in terrestrial C3 plants in which α increases with [CO2] availability (Raghavendra, 2000). This difference between responses of aquatic and terrestrial plants may result because CO2 responses are coupled to water stress in terrestrial plants but not in aquatic plants. The increased Ek and PE values for high CO2 acclimated plants will decrease the estimates of Hsat (i.e., average daily period of PE) required to maintain positive carbon balance for the whole plant. The Hsat requirement is a useful modeling tool in predicting the depth distribution of eelgrass in variable light environments (Dennison, 1987; Zimmerman et al., 1991; Zimmerman et al., 1994; Zimmerman et al., 1995).
A strong correlation between diurnal NPQ cycle (i.e., xanthophyll cycle) and high light exposure has been confirmed for eelgrass to avoid photodamage under fluctuating light environments (Ralph et al., 2002). High light acclimated eelgrass leaves have higher NPQ activity, and higher photosynthetic capacity, than low light acclimated leaves (Ralph and Gademann, 2005). Here, we demonstrated a similar effect of [CO2] availability on NPQ activity. Under ambient CO2 concentrations, the onset of photosynthesis CO2 limitation (Ek) occurred at lower irradiance, accelerating the diversion of excess photon absorption to NPQ, likely using the xanthophyll cycle as a photoprotective mechanism to prevent photoinhibition. The high CO2 incubations reduced this carbon limitation and increased the Ek, consequently reducing NPQ. Due to increased Ek, the same light environment became less damaging at high CO2, which may explain the reduction in both photosynthetic and photoprotective pigments observed in response to growth CO2. Thus, by reducing CO2 limitation of Rubisco, ocean carbonation should also reduce the vulnerability of eelgrass to excess reactive oxygen species (ROS) and therefore the need for photoprotection.
The simultaneous measurements of variable fluorescence, and O2 flux performed here yielded quantitative estimates of changes in photoprotective pathways of eelgrass acclimated to different CO2 environments. The difference between the theoretical O2 evolution (i.e., the linear increase of O2 with light) and the ETR estimates of gross photosynthesis (Pg-ETR) was most pronounced for plants grown at high CO2, accounting for the absorbed photons that did not contribute to the electron transport pathway (not exciting electrons at PSII), but explained by quenching pathways, such as fluorescence and NPQ. This trend was consistent with their lower area-specific O2 production rates at high CO2 incubations when compared to pH7 and ambient pH grown plants. These plants downregulated their pigment content but increased the light-dependent NPQ at lower irradiances even at high incubation [CO2]. This may indicate that phenotypic acclimation to ocean carbonation by downregulating the photosynthetic apparatus reduces the role of photorespiration but increases the role of NPQ in photoprotection.
On the other hand, as observed in all treatments, the difference between the ETR estimated gross photosynthesis (Pg-ETR) and the gross photosynthesis measured by oxygen production (Pg) may result from inaccurate assumptions of Fii and/or τ (Equation 12). In theory, 8 photons absorbed equivalently both by PSI and PSII (Fii= 0.5) excites a total of 4 electrons producing 1 mole of O2 (τ =0.25). This equilibrium of linear electron flow is valid when there is no limitation of resources such as CO2 and/or accumulation of byproducts such as reducing equivalents and ROS (Scheibe et al., 2005; Dietz and Pfannschmidt, 2011; Pfannschmidt and Yang, 2012). Under limiting conditions, this balance shifts towards pathways that ensure the optimal redox state of the chloroplast resulting in altered photon: electron: O2 ratios (Foyer et al., 2012).
Following the linear assumption that 4 electrons produce 1 O2 (τ = 0.25) resulted in overestimation of the PGETR in all treatments. Since the molecular chemistry of water splitting at PSII is well-known, τ can only be reduced in an apparent sense. This apparent ratio can result from the excitation of four electrons (as detected with PAM) either without producing O2, indicating cyclic electron flow around PSII, or consumption of O2 in the chloroplast that would remain undetected by the gas exchange method (Foyer et al., 2009; Ananyev et al., 2017). Two possible pathways to explain a reduction in τ due to O2 consumption are (i) the Mehler reaction and (ii) photorespiration. The Mehler reaction increases the pH gradient that may induce NPQ (Demmig-Adams and Adams, 1996; Kanazawa and Kramer, 2002). However, in this study NPQ induction did not happen until ΦPSII values fell below 0.6 while O2 yield continuously decreased. Therefore, the observed nonlinearity between quantum yield of fluorescence and quantum yield of oxygen most likely resulted from O2 consumption via photorespiration, which probably represents the primary pathway to remove excess O2 buildup and use the ATP energy from light reactions for this purpose. NPQ was then triggered when photorespiration is incapable of consuming enough ATP to lower the pH gradient forming across lumen at very high irradiances.
Other pathways that keep the electron flow continuous without contributing to CO2 assimilation are the malate valve and the cyclic electron flow around PSI, which triggers NPQ by generating a pH gradient (Munekage et al., 2004; Johnson, 2005; Miyake, 2010). If PSI cyclic electron flow plays an important role, then the assumption of half of the absorbed photons going to PSII (e.g., Fii=0.5) would be inaccurate. Although PAM measurements are easily made under field conditions (including underwater) and provide non-intrusive information about the photoprotection of eelgrass through NPQ, the fluorescence measurements with PAM overestimate Pg-ETR and therefore are not equivalent to true carbon assimilation. Fluorescence measurements may account for the number of absorbed photons used in electron excitation but not necessarily towards the rates of oxygen production/consumption or carbon assimilation, especially when alternative electron sinks are available (Beer et al., 1998). Still, by having quantified the ratio of ΦPSII to ΦO2 as a function of light and carbon availability in response to acclimation to ocean carbonation, the alternative electron pathways can be accounted for in the future estimation of photosynthesis in eelgrass.
To conclude, photorespiration likely provides an important metabolic clutch to protect the photochemical pathway in CO2-limited eelgrass by maintaining electron flow to prevent the inhibitory damage to photosystems due to light saturation when carbon assimilation is limited by CO2 supply. In addition to providing a photoprotective role, photorespiration could serve multiple purposes by connecting different metabolic pathways that allow instantaneous energy and reductant modulation under fluctuating environmental conditions. Further, photorespiration may provide a carbon concentrating mechanism via recycling of photorespired CO2 and removing excess intracellular O2. Therefore, even though carbon limitation causes eelgrass photosynthesis to saturate at relatively low light levels in the modern ocean, longer daily periods of saturating irradiances might be required to keep the photosynthetic apparatus running to produce ATP to support photorespiration. Consequently, understanding photoprotection mechanisms employed by these remarkable plants that are permanently rooted in highly variable shallow-water environments, becomes important when high water column productivity causes [O2] to rise and [CO2] to fall just as daily irradiances begin to peak. More importantly, this study demonstrated that acclimation of photoprotective mechanisms in response to CO2 availability accounted for the previously reported physiological acclimations of enhanced growth and survival of this species under ocean acidification scenarios.
Data availability statement
The original contributions presented in the study are included in the article/Supplementary Material. Further inquiries can be directed to the corresponding author.
Author contributions
BC-E, RZ and VH conceived the project. BC-E, RZ and VH performed the research. BC-E and RZ analyzed the data. BC-E, RZ and VH wrote the article. All authors contributed to the article and approved the submitted version.
Funding
Financial support for this research was provided by the National Science Foundation (Award OCE-1061823 to RZ and VH), Virginia Sea Grant/NOAA (Award NA14OAR4170093 to RZ and BC-E) and the Department of Ocean, Earth & Atmospheric Sciences, Old Dominion University (to BC-E). This research was performed in partial completion of the requirements for the Ph.D. degree (Oceanography) at Old Dominion University.
Acknowledgments
Thanks to W. M. Swingle and the staff of the Virginia Aquarium & Marine Science Center for assistance with maintenance of the experimental facility, and to D. Ruble, M. Jinuntuya, C. Zayas-Santiago, T. Cedeno and M. Smith for assistance with experimental procedures and maintenance of the experimental plants.
Conflict of interest
The authors declare that the research was conducted in the absence of any commercial or financial relationships that could be construed as a potential conflict of interest.
Publisher’s note
All claims expressed in this article are solely those of the authors and do not necessarily represent those of their affiliated organizations, or those of the publisher, the editors and the reviewers. Any product that may be evaluated in this article, or claim that may be made by its manufacturer, is not guaranteed or endorsed by the publisher.
Supplementary material
The Supplementary Material for this article can be found online at: https://www.frontiersin.org/articles/10.3389/fpls.2022.1025416/full#supplementary-material
References
Ananyev, G., Gates, C., Kaplan, A., Dismukes, G. C. (2017). Photosystem II-cyclic electron flow powers exceptional photoprotection and record growth in the microalga chlorella ohadii. Biochim. Biophys. Acta (BBA) - Bioenergetics 1858 (11), 873–883. doi: 10.1016/j.bbabio.2017.07.001
Andrews, T. J., Abel, K. M. (1979). Photosynthetic carbon metabolism in seagrasses 14C-labeling evidence for the C3 pathway. Plant Physiol. 63 (4), 650–656. doi: 10.1104/pp.63.4.650
Andrews, T. J., Lorimer, G. H. (1978). Photorespiration – still unavoidable? FEBS Lett. 90 (1), 1–9. doi: 10.1016/0014-5793(78)80286-5
Baker, N. R. (2008). Chlorophyll fluorescence: A probe of photosynthesis In vivo. Annu. Rev. Plant Biol. 59 (1), 89–113. doi: 10.1146/annurev.arplant.59.032607.092759
Barra, L., Chandrasekaran, R., Corato, F., Brunet, C. (2014). The challenge of ecophysiological biodiversity for biotechnological applications of marine microalgae. Mar. Drugs 12 (3), 1641. doi: 10.3390/md12031641
Bathellier, C., Tcherkez, G., Lorimer, G. H., Farquhar, G. D. (2018). Rubisco is not really so bad. Plant Cell Environ. 41 (4), 705–716. doi: 10.1111/pce.13149
Beer, S., Koch, E. (1996). Photosynthesis of marine macroalgae and seagrasses in globally changing CO2 environments. Mar. Ecol. Prog. Ser. 141, 199–204. doi: 10.3354/meps141199
Beer, S., Sand-Jensen, K., Madsen, T. V., Nielsen, S. L. (1991). The carboxylase activity of rubisco and the photosynthetic performance in aquatic plants. Oecologia 87 (3), 429–434. doi: 10.1007/BF00634602
Beer, S., Vilenkin, B., Weil, A., Veste, M., Susel, L., Eshel, A. (1998). Measuring photosynthetic rates in seagrasses by pulse amplitude modulated (PAM) fluorometry. Mar. Ecology-Progress Ser. 174, 293–300. doi: 10.3354/meps174293
Björk, M., Haglund, K., Ramazanov, Z., Pedersén, M. (1993). Inducible mechanisms for HCO3- utilization and repression of photorespiration in protoplasts and thalli of three species of ulva (chlorophyta)1. J. Phycology 29 (2), 166–173. doi: 10.1111/j.0022-3646.1993.00166.x
Black, C., Burris, J., Everson, R. (1976). Influence of oxygen concentration on photosynthesis in marine plants. Funct. Plant Biol. 3 (1), 81–86. doi: 10.1071/PP9760081
Borum, J., Sand-Jensen, K., Binzer, T., Pedersen, O., Greve, T. (2006). “Oxygen movement in seagrasses,” in SEAGRASSES: BIOLOGY, ECOLOGYAND CONSERVATION (Springer, Dordrecht: Springer Netherlands), 255–270. doi: 10.1007/978-1-4020-2983-7_10
Buapet, P., Björk, M. (2016). The role of O2 as an electron acceptor alternative to CO2 in photosynthesis of the common marine angiosperm zostera marina l. Photosynthesis Res. 129 (1), 59–69. doi: 10.1007/s11120-016-0268-4
Buapet, P., Gullström, M., Björk, M. (2013a). Photosynthetic activity of seagrasses and macroalgae in temperate shallow waters can alter seawater pH and total inorganic carbon content at the scale of a coastal embayment. Mar. Freshw. Res. 64 (11), 1040–1048. doi: 10.1071/MF12124
Buapet, P., Rasmusson, L. M., Gullström, M., Björk, M. (2013b). Photorespiration and carbon limitation determine productivity in temperate seagrasses. PloS One 8 (12), e83804. doi: 10.1371/journal.pone.0083804
Burris, J., Holm-Hansen, O., Black, C. (1976). Glycine and serine production in marine plants as a measure of photorespiration. Funct. Plant Biol. 3 (1), 87–92. doi: 10.1071/PP9760087
Busch, F. A., Sage, T. L., Cousins, A. B., Sage, R. F. (2013). C3 plants enhance rates of photosynthesis by reassimilating photorespired and respired CO2. Plant Cell Environ. 36 (1), 200–212. doi: 10.1111/j.1365-3040.2012.02567.x
Celebi, B. (2016). Potential impacts of climate change on photochemistry of zostera Marina L. Doctor of Philosophy (PhD), Dissertation, Ocean & Earth Sciences, (Norfolk, VA: Old Dominion University). doi: 10.25777/8b9x-8303
Celebi-Ergin, B., Zimmerman, R. C., Hill, V. J. (2021). Impact of ocean carbonation on long-term regulation of light harvesting in eelgrass, Zostera marina l. Mar. Ecol. Prog. Ser. 671, 111–128. doi: 10.3354/meps13777
Cummings, M. E., Zimmerman, R. C. (2003). Light harvesting and the package effect in the seagrasses Thalassia testudinum banks ex könig and Zostera marina l.: optical constraints on photoacclimation. Aquat. Bot. 75 (3), 261–274. doi: 10.1016/s0304-3770(02)00180-8
Cyronak, T., Andersson, A. J., D’Angelo, S., Bresnahan, P., Davidson, C., Griffin, A., et al. (2018). Short-term spatial and temporal carbonate chemistry variability in two contrasting seagrass meadows: Implications for pH buffering capacities. Estuaries Coasts. 41, 1282–1296. doi: 10.1007/s12237-017-0356-5
Demmig-Adams, B., Adams, W. W., III (1996). The role of xanthophyll cycle carotenoids in the protection of photosynthesis. Trends Plant Sci. 1 (1), 21–26. doi: 10.1016/s1360-1385(96)80019-7
Dennison, W. C. (1987). Effects of light on seagrass photosynthesis, growth and depth distribution. Aquat. Bot. 27 (1), 15–26. doi: 10.1016/0304-3770(87)90083-0
Dietz, K.-J., Pfannschmidt, T. (2011). Novel regulators in photosynthetic redox control of plant metabolism and gene expression. Plant Physiol. 155 (4), 1477–1485. doi: 10.1104/pp.110.170043
Downton, W., Bishop, D., Larkum, A., Osmond, C. (1976). Oxygen inhibition of photosynthetic oxygen evolution in marine plants. Funct. Plant Biol. 3 (1), 73–79. doi: 10.1071/PP9760073
Draper, N. R., Smith, H. (1981). Applied regression analysis 2nd Edition (New York: John Wiley & Sons).
Duarte, C. M., Hendriks, I. E., Moore, T. S., Olsen, Y. S., Steckbauer, A., Ramajo, L., et al. (2013). Is ocean acidification an open-ocean syndrome? understanding anthropogenic impacts on seawater pH. Estuaries Coasts 36 (2), 221–236. doi: 10.1007/s12237-013-9594-3
Durako, M. J. (1993). Photosynthetic utilization of CO2(aq) and HCO3- in Thalassia testudinum (Hydrocharitaceae). Mar. Biol. 115 (3), 373–380. doi: 10.1007/bf00349834
Falkowski, P. G., Raven, J. A. (2007). Aquatic photosynthesis (Dordrecht: Princeton University Press).
Figueroa, F., Conde-Álvarez, R., Gómez, I. (2003). Relations between electron transport rates determined by pulse amplitude modulated chlorophyll fluorescence and oxygen evolution in macroalgae under different light conditions. Photosynthesis Res. 75 (3), 259–275. doi: 10.1023/A:1023936313544
Foyer, C. H., Bloom, A. J., Queval, G., Noctor, G. (2009). Photorespiratory metabolism: Genes, mutants, energetics, and redox signaling. Annu. Rev. Plant Biol. 60 (1), 455–484. doi: 10.1146/annurev.arplant.043008.091948
Foyer, C. H., Neukermans, J., Queval, G., Noctor, G., Harbinson, J. (2012). Photosynthetic control of electron transport and the regulation of gene expression. J. Exp. Bot. 63 (4), 1637–1661. doi: 10.1093/jxb/ers013
Frost-Christensen, H., Sand-Jensen, K. (1992). The quantum efficiency of photosynthesis in macroalgae and submerged angiosperms. Oecologia 91 (3), 377–384. doi: 10.1007/bf00317627
Heber, U., Krause, G. H. (1980). What is the physiological role of photorespiration? Trends Biochem. Sci. 5 (2), 32–34. doi: 10.1016/s0968-0004(80)80091-0
Hough, R. A. (1974). Photorespiration and productivity in submersed aquatic vascular plants. Limnology Oceanography 19 (6), 912–927. doi: 10.4319/lo.1974.19.6.0912
Hough, A. R., Wetzel, R. G. (1977). Photosynthetic pathways of some aquatic plants. Aquat. Bot. 3, 297–313. doi: 10.1016/0304-3770(77)90035-3
Igamberdiev, A. U., Bykova, N. V., Lea, P. J., Gardestrom, P. (2001). The role of photorespiration in redox and energy balance of photosynthetic plant cells: A study with a barley mutant deficient in glycine decarboxylase. Physiol. Plant 111 (4), 427–438. doi: 10.1034/j.1399-3054.2001.1110402.x
Invers, O., Zimmerman, R. C., Alberte, R. S., Pérez, M., Romero, J. (2001). Inorganic carbon sources for seagrass photosynthesis: an experimental evaluation of bicarbonate use in species inhabiting temperate waters. J. Exp. Mar. Biol. Ecol. 265 (2), 203–217. doi: 10.1016/s0022-0981(01)00332-x
Johnson, G. N. (2005). Cyclic electron transport in C3 plants: fact or artefact? J. Exp. Bot. 56 (411), 407–416. doi: 10.1093/jxb/eri106
Kalaji, H. M., Schansker, G., Ladle, R. J., Goltsev, V., Bosa, K., Allakhverdiev, S. I., et al. (2014). Frequently asked questions about in vivo chlorophyll fluorescence: practical issues. Photosynthesis Res. 122 (2), 121–158. doi: 10.1007/s11120-014-0024-6
Kanazawa, A., Kramer, D. M. (2002). In vivo modulation of nonphotochemical exciton quenching (NPQ) by regulation of the chloroplast ATP synthase. Proc. Natl. Acad. Sci. 99 (20), 12789–12794. doi: 10.1073/pnas.182427499
Koch, M., Bowes, G., Ross, C., Zhang, X.-H. (2013). Climate change and ocean acidification effects on seagrasses and marine macroalgae. Global Change Biol. 19 (1), 103–132. doi: 10.1111/j.1365-2486.2012.02791.x
Kuypers, M. M. M., Pancost, R. D., Damste, J. S. S. (1999). A large and abrupt fall in atmospheric CO2 concentration during Cretaceous times. Nature 399 (6734), 342–345. doi: 10.1038/20659
Larkum, A. D., Drew, E., Ralph, P. (2006a). “Photosynthesis and metabolism in seagrasses at the cellular level,” in Seagrasses: Biology, ecologyand conservation (Dordrecht: Springer Netherlands), 323–345. doi: 10.1007/978-1-4020-2983-7_14
Larkum, A. W. D., Orth, R. J., Duarte, C. M. (Eds.) (2006b). Seagrasses : biology, ecology, and conservation (Dordrecht: Springer).
Lewis, E. (1980). The practical salinity scale 1978 and its antecedents. IEEE J. Oceanic Eng. 5 (1), 3–8. doi: 10.1109/JOE.1980.1145448
Lewis, E., Wallace, D. W. R., Allison, L. J. (1998). Program developed for CO2 system calculations, carbon dioxide information analysis center. Oak Ridge, Tenn: Oak Ridge National Laboratory.
Madsen, T. V., Maberly, S. C., Bowes, G. (1996). Photosynthetic acclimation of submersed angiosperms to CO2 and HCO3-. Aquat. Bot. 53 (1-2), 15–30. doi: 10.1016/0304-3770(95)01009-2
Madsen, T. V., Sand-Jensen, K. (1991). Photosynthetic carbon assimilation in aquatic macrophytes. Aquat. Bot. 41 (1-3), 5–40. doi: 10.1016/0304-3770(91)90037-6
Madsen, T. V., Sand-Jensen, K., Beer, S. (1993). Comparison of photosynthetic performance and carboxylation capacity in a range of aquatic macrophytes of different growth forms. Aquat. Bot. 44 (4), 373–384. doi: 10.1016/0304-3770(93)90078-B
McPherson, M. L., Zimmerman, R. C., Hill, V. J. (2015). Predicting carbon isotope discrimination in eelgrass (Zostera marina l.) from the environmental parameters–light, flow, and [DIC]. Limnology Oceanography 60 (6), 1875–1889. doi: 10.1002/lno.10142
Meyer, M. T., Whittaker, C., Griffiths, H. (2017). The algal pyrenoid: key unanswered questions. J. Exp. Bot. 68 (14), 3739–3749. doi: 10.1093/jxb/erx178
Miyake, C. (2010). Alternative electron flows (Water–water cycle and cyclic electron flow around PSI) in photosynthesis: Molecular mechanisms and physiological functions. Plant Cell Physiol. 51 (12), 1951–1963. doi: 10.1093/pcp/pcq173
Motulsky, H., Christopoulos, A. (2004). Fitting models to biological data using linear and nonlinear regression : a practical guide to curve fitting (Oxford: Oxford University Press).
Munekage, Y., Hashimoto, M., Miyake, C., Tomizawa, K.-I., Endo, T., Tasaka, M., et al. (2004). Cyclic electron flow around photosystem I is essential for photosynthesis. Nature 429 (6991), 579–582. doi: 10.1038/nature02598
Osmond, C. B. (1981). Photorespiration and photoinhibition : Some implications for the energetics of photosynthesis. Biochim. Biophys. Acta (BBA) - Rev. Bioenergetics 639 (2), 77–98. doi: 10.1016/0304-4173(81)90006-9
Osmond, B., Badger, M., Maxwell, K., Björkman, O., Leegood, R. (1997). Too many photons: photorespiration, photoinhibition and photooxidation. Trends Plant Sci. 2 (4), 119–121. doi: 10.1016/s1360-1385(97)80981-8
Ow, Y. X., Collier, C. J., Uthicke, S. (2015). Responses of three tropical seagrass species to CO2 enrichment. Mar. Biol. 162 (5), 1005–1017. doi: 10.1007/s00227-015-2644-6
Palacios, S., Zimmerman, R. (2007). Response of eelgrass Zostera marina to CO2 enrichment: possible impacts of climate change and potential for remediation of coastal habitats. Mar. Ecol. Prog. Ser. 344, 1–13. doi: 10.3354/meps07084
Pfannschmidt, T., Yang, C. (2012). The hidden function of photosynthesis: a sensing system for environmental conditions that regulates plant acclimation responses. Protoplasma 249 (2), 125–136. doi: 10.1007/s00709-012-0398-2
Raghavendra, A. S. (2000). Photosynthesis: A comprehensive treatise (Cambridge, UK: Cambridge University Press).
Ralph, P. J., Durako, M. J., Enríquez, S., Collier, C. J., Doblin, M. A. (2007). Impact of light limitation on seagrasses. J. Exp. Mar. Biol. Ecol. 350 (1-2), 176–193. doi: 10.1016/j.jembe.2007.06.017
Ralph, P. J., Gademann, R. (2005). Rapid light curves: A powerful tool to assess photosynthetic activity. Aquat. Bot. 82 (3), 222–237. doi: 10.1016/j.aquabot.2005.02.006
Ralph, P. J., Polk, S. M., Moore, K. A., Orth, R. J., Smith, W. O. (2002). Operation of the xanthophyll cycle in the seagrass Zostera marina in response to variable irradiance. J. Exp. Mar. Biol. Ecol. 271 (2), 189–207. doi: 10.1016/s0022-0981(02)00047-3
Rasmusson, L., Buapet, P., George, R., Gullström, M., Gunnarsson, P., Björk, M. (2020). Effects of temperature and hypoxia on respiration, photorespiration, and photosynthesis of seagrass leaves from contrasting temperature regimes. ICES J. Mar. Science. 2056–2065. doi: 10.1093/icesjms/fsaa093
Raven, J., Beardall, J. (2003). “Carbon acquisition mechanisms of algae: Carbon dioxide diffusion and carbon dioxide concentrating mechanisms,” in Photosynthesis in algae. Eds. Larkum, A. D., Douglas, S., Raven, J. (Dordrecht: Springer Netherlands), 225–244. doi: 10.1007/978-94-007-1038-2_11
Raven, J. A., Beardall, J. (2014). CO2 concentrating mechanisms and environmental change. Aquat. Bot. 118 (0), 24–37. doi: 10.1016/j.aquabot.2014.05.008
Raven, J. A., Giordano, M., Beardall, J., Maberly, S. C. (2011). Algal and aquatic plant carbon concentrating mechanisms in relation to environmental change. Photosynth Res. 109 (1-3), 281–296. doi: 10.1007/s11120-011-9632-6
Ruesink, J. L., Yang, S., Trimble, A. C. (2015). Variability in carbon availability and eelgrass (Zostera marina) biometrics along an estuarine gradient in willapa bay, WA, USA. Estuaries Coasts 38 (6), 1908–1917. doi: 10.1007/s12237-014-9933-z
Scheibe, R., Backhausen, J. E., Emmerlich, V., Holtgrefe, S. (2005). Strategies to maintain redox homeostasis during photosynthesis under changing conditions. J. Exp. Bot. 56 (416), 1481–1489. doi: 10.1093/jxb/eri181
Schubert, N., Freitas, C., Silva, A., Costa, M. M., Barrote, I., Horta, P. A., et al. (2018). Photoacclimation strategies in northeastern Atlantic seagrasses: Integrating responses across plant organizational levels. Sci. Rep. 8 (1), 14825. doi: 10.1038/s41598-018-33259-4
Somerville, C. R. (2001). An early arabidopsis demonstration. resolving a few issues concerning photorespiration. Plant Physiol. 125 (1), 20–24. doi: 10.2307/4279600
Spreitzer, R. J., Salvucci, M. E. (2002). RUBISCO: Structure, regulatory interactions, and possibilities for a better enzyme. Annu. Rev. Plant Biol. 53 (1), 449–475. doi: 10.1146/annurev.arplant.53.100301.135233
Tcherkez, G. G. B., Farquhar, G. D., Andrews, T. J. (2006). Despite slow catalysis and confused substrate specificity, all ribulose bisphosphate carboxylases may be nearly perfectly optimized. Proc. Natl. Acad. Sci. 103 (19), 7246–7251. doi: 10.1073/pnas.0600605103
Touchette, B. W., Burkholder, J. M. (2000). Overview of the physiological ecology of carbon metabolism in seagrasses. J. Exp. Mar. Biol. Ecol. 250 (1-2), 169–205. doi: 10.1016/s0022-0981(00)00196-9
Voss, I., Sunil, B., Scheibe, R., Raghavendra, A. S. (2013). Emerging concept for the role of photorespiration as an important part of abiotic stress response. Plant Biol. 15 (4), 713–722. doi: 10.1111/j.1438-8677.2012.00710.x
Webb, W., Newton, M., Starr, D. (1974). Carbon dioxide exchange of Alnus rubra a mathematical model. Oecologia 17 (4), 281–291. doi: 10.1007/BF00345747
Xin, C.-P., Tholen, D., Devloo, V., Zhu, X.-G. (2015). The benefits of photorespiratory bypasses: How can they work? Plant Physiol. 167 (2), 574–585. doi: 10.1104/pp.114.248013
Zeebe, R. E. (2012). History of seawater carbonate chemistry, atmospheric CO2, and ocean acidification. Annu. Rev. Earth Planetary Sci. 40 (1), 141–165. doi: 10.1146/annurev-earth-042711-105521
Zimmerman, R. C. (2003). A biooptical model of irradiance distribution and photosynthesis in seagrass canopies. Limnology Oceanography 48 (1), 568–585. doi: 10.4319/lo.2003.48.1_part_2.0568
Zimmerman, R. C. (2006). “Light and photosynthesis in seagrass meadows," in seagrasses: Biology, ecology and conservation,”. Eds. Larkum, A. W. D., Orth, R. J., Duarte, C. M. (Dordrecht: Springer Netherlands), 303–321.
Zimmerman, R. C. (2021). Scaling up: Predicting the impacts of climate change on seagrass ecosystems. Estuaries Coasts 44 (2), 558–576. doi: 10.1007/s12237-020-00837-7
Zimmerman, R. C., Cabello-Pasini, A., Alberte, R. S. (1994). Modeling daily production of aquatic macrophytes from irradiance measurements: a comparative analysis. Mar. Ecol. Prog. Ser. 114, 185–196. doi: 10.3354/meps114185
Zimmerman, R. C., Hill, V. J., Gallegos, C. L. (2015). Predicting effects of ocean warming, acidification, and water quality on Chesapeake region eelgrass. Limnology Oceanography 60 (5), 1781–1804. doi: 10.1002/lno.10139
Zimmerman, R. C., Hill, V. J., Jinuntuya, M., Celebi, B., Ruble, D., Smith, M., et al. (2017). Experimental impacts of climate warming and ocean carbonation on eelgrass Zostera marina. Mar. Ecol. Prog. Ser. 566, 1–15. doi: 10.3354/meps12051
Zimmerman, R. C., Kohrs, D. G., Steller, D. L., Alberte, R. S. (1997). Impacts of CO2 enrichment on productivity and light requirements of eelgrass. Plant Physiol. 115 (2), 599–607. doi: 10.1104/pp.115.2.599
Zimmerman, R. C., Reguzzoni, J. L., Alberte, R. S. (1995). Eelgrass (Zostera marina l.) transplants in San Francisco bay: Role of light availability on metabolism, growth and survival. Aquat. Bot. 51 (1-2), 67–86. doi: 10.1016/0304-3770(95)00472-c
Zimmerman, R. C., Reguzzoni, J. L., Wyllie-Echeverria, S., Josselyn, M., Alberte, R. S. (1991). Assessment of environmental suitability for growth of Zostera marina l. (eelgrass) in San Francisco bay. Aquat. Bot. 39 (3-4), 353–366. doi: 10.1016/0304-3770(91)90009-t
Keywords: CO2, non-photochemical quenching, ocean acidification, photorespiration, photosynthesis, quantum yield, seagrass
Citation: Celebi-Ergin B, Zimmerman RC and Hill VJ (2022) Photorespiration in eelgrass (Zostera marina L.): A photoprotection mechanism for survival in a CO2-limited world. Front. Plant Sci. 13:1025416. doi: 10.3389/fpls.2022.1025416
Received: 22 August 2022; Accepted: 10 October 2022;
Published: 11 November 2022.
Edited by:
Benoit Schoefs, Le Mans Université, FranceReviewed by:
Milan Szabo, Eötvös Loránd Research Network (ELKH), HungaryAlexandrina Stirbet, University of Bucharest, Romania
Copyright © 2022 Celebi-Ergin, Zimmerman and Hill. This is an open-access article distributed under the terms of the Creative Commons Attribution License (CC BY). The use, distribution or reproduction in other forums is permitted, provided the original author(s) and the copyright owner(s) are credited and that the original publication in this journal is cited, in accordance with accepted academic practice. No use, distribution or reproduction is permitted which does not comply with these terms.
*Correspondence: Billur Celebi-Ergin, celebibillur@gmail.com
†Present address: Billur Celebi-Ergin, Department of Molecular Biology and Genetics, Koç University Rumelifeneri Yolu Sariyer, Istanbul, Turkey
‡ORCID: Billur Celebi-Ergin, https://orcid.org/0000-0002-9949-1617
Richard C. Zimmerman, orcid.org/0000-0002-9399-4264
Victoria J. Hill, orcid.org/0000-0001-8337-7441