- 1School of Natural Sciences, Bangor University, Bangor, United Kingdom
- 2School of Agricultural Engineering, CEIGRAM, Universidad Politecnica de Madrid, Madrid, Spain
- 3School of Agriculture and Environment, The University of Western Australia, Perth, WA, Australia
- 4Centre for Microscopy, Characterisation and Analysis, The University of Western Australia, Perth, WA, Australia
- 5Centre for Sustainable Farming Systems, Food Futures Institute, Murdoch University, Murdoch, WA, Australia
- 6Department of Geography, Scott Polar Research Institute, Cambridge, United Kingdom
- 7School of Geography, Politics and Sociology, Newcastle University, Newcastle, United Kingdom
- 8Servicio de Microscopıa Electronica, Instituto Ciencias Agrarias CSIC, Madrid, Spain
- 9Biosciences, University of Exeter, Exeter, United Kingdom
- 10Lancaster Environment Centre, Lancaster University, Lancaster, United Kingdom
- 11Advanced Microscopy and Bioimaging, Institute of Biological, Environmental and Rural Sciences, Aberystwyth University, Aberystwyth, United Kingdom
- 12School of Biological Sciences, The University of Western Australia, Perth, WA, Australia
- 13Department of Forest Ecosystems & Society, College of Forestry, Oregon State University, Corvallis, OR, United States
Introduction: Biological N2 fixation in feather-mosses is one of the largest inputs of new nitrogen (N) to boreal forest ecosystems; however, revealing the fate of newly fixed N within the bryosphere (i.e. bryophytes and their associated organisms) remains uncertain.
Methods: Herein, we combined 15N tracers, high resolution secondary ion mass-spectrometry (NanoSIMS) and a molecular survey of bacterial, fungal and diazotrophic communities, to determine the origin and transfer pathways of newly fixed N2 within feather-moss (Pleurozium schreberi) and its associated microbiome.
Results: NanoSIMS images reveal that newly fixed N2, derived from cyanobacteria, is incorporated into moss tissues and associated bacteria, fungi and micro-algae.
Discussion: These images demonstrate that previous assumptions that newly fixed N2 is sequestered into moss tissue and only released by decomposition are not correct. We provide the first empirical evidence of new pathways for N2 fixed in feather-mosses to enter the boreal forest ecosystem (i.e. through its microbiome) and discuss the implications for wider ecosystem function.
Introduction
Cyanobacterial N2 fixation within feather-moss communities is a primary source of new nitrogen (N) in boreal forest ecosystems (DeLuca et al., 2002a, DeLuca et al., 2002b; Zackrisson et al., 2004; DeLuca et al., 2008; Renaudin et al., 2022a, Renaudin et al., 2022b); however, understanding of how this N source influences key ecosystem processes (e.g. productivity, biodiversity and carbon [C] cycling) remains limited with the precise cellular mechanisms yet to be defined. This is exemplified by the lack of knowledge about the fate of fixed N and its contribution to N nutrition of coexisting organisms (Jones and Wilson, 1978; Lindo et al., 2013; Kardol et al., 2016; DeLuca et al., 2022). Previous studies have demonstrated that N2 fixed within the moss-cyanobacteria system is highly conserved, with little direct transfer to higher plants and soil (Hyodo et al., 2013; Rousk et al., 2014; DeLuca et al., 2022). The lack of specialized symbiotic structures to facilitate transfer of N between cyanobacteria and its host moss (Solheim and Zielke, 2002) makes it difficult to assess how, and to what extent, nutrient exchange occurs between them. For example, direct uptake of fixed N has been reported in moss species such as Sphagnum (Berg et al., 2013), Hymenostylium recurvirostrum (Hedw.) Dixon (Jones and Wilson, 1978) and Pleurozium schreberi (Brid.) Mitt. (Bay et al., 2013). However, other studies have shown that not all the fixed N is directly transferred to P. schreberi (Kardol et al., 2016) or have failed to detect any direct transfer to moss tissue (Hyodo et al., 2013).
Even less is known about the relationship between the cyanobacteria and other components of the moss microbiome. All organisms within the boreal bryosphere require N for their maintenance, growth and reproduction, so competition between the moss and its associated-microbiome to access newly fixed N is likely. Further, competition can be expected to differ spatially based on vertical differentiation of both moss traits, e.g. physiological decline with ageing (Bates, 1979), and bryosphere microbial communities (Solheim and Zielke, 2002; Lindo and Gonzalez, 2010; Osono and Trofymow, 2012; Davey et al., 2013; Xiang et al., 2014). However, our understanding of fixed N dynamics within the bryosphere has traditionally only considered the moss and more specifically the presence and activity of cyanobacteria. More recently, some studies have demonstrated that mosses host a broad diversity of other putative N2-fixing bacterial lineages, for examples studies on Sphagnum mosses (Bragina et al., 2012; Bragina et al., 2013; Ho and Bodelier, 2015) and boreal mosses (Holland-Moritz et al., 2018). Consequently, uncertainty exists regarding both the pathways followed by cyanobacterial-fixed N within the moss-cyanobacteria system (Lindo et al., 2013; Ho and Bodelier, 2015; Kardol et al., 2016) and the possible role of other N2-fixers as N source in the boreal bryosphere.
Here we aim to describe the microbial and diazotrophic community associated with P. schreberi and trace the accumulation of newly fixed 15N2 in the moss and its associated microbiome to: 1) Identify the primary N2 fixing organisms in P. schreberi, a dominant moss species in the boreal forest; 2) Determine whether microbes living in association with the moss can access the fixed N; 3) Investigate if there is spatial differentiation for fixed N accumulation by moss cells related to tissue age (i.e., between cells in the young tip of the moss stem and the old bottom segments); 4) Evaluate the potential implications of the results in the context of boreal ecosystem functioning.
The boreal forest is the largest terrestrial biome on earth and its ability to deliver a range of globally-important ecosystem services is critically dependent on the availability of N (DeLuca and Boisvenue, 2012). Our study offers evidence of rapid pathways of N fixed in feather-mosses into the boreal forest soil (i.e., through the moss microbiome rather than moss tissue decomposition), with implications for wider ecosystem function (i.e., a rapid route for N2 fixation to influence ecosystem functions compared with N release by decomposition of moss tissues).
Material and methods
Site description and 15N2 incubation experiment
This study was conducted in the boreal forest of Northern Sweden (65°46′-65°56′N, 18°20′E-19°6′E) where feather-moss carpets dominate the forest floor. The characteristics of the landscape in this area have been described in detail elsewhere (Zackrisson et al., 1996; DeLuca et al., 2002a; Zackrisson et al., 2004; DeLuca et al., 2022). We selected two forest sites with different canopy structure and nitrogenase activity (i.e., proxy of N2 fixation): Njällatjirelg, an open canopy forest with high forest floor moss N2 fixation and Reivo, a variably dense canopy forest with moderately high N2 fixation in the moss layer (Supplementary Figure 1). In September 2013, we conducted a 15N-labeled tracer addition experiment using 15N2 gas (Supplementary Figure 2). Three moss sample cores (including living and dead segments of the moss shoot, litter layer and humus soil) were collected at each forest site (Njällatjirelg and Reivo) using stainless steel cores (20 cm × 7 cm dia) and subsequently placed into acrylic tubes (20 cm × 7 cm dia) ensuring all cores had a headspace of 200 ml (extra humus soil was used to fill the bottom empty space when needed). Moss cores were hydrated by spraying distilled water to ensure adequate moisture for optimum physiological activation for the moss and its associated microbiome. Within 24 h of collection each tube was then hermetically sealed at the top and bottom. The lid was fitted with a rubber septum to facilitate injection of the 15N2 gas. A total of 200 ml of headspace was removed from each incubation vessel and was replaced by 200 ml of 15N2 gas (98 atom % 15N enriched, Sigma-Aldrich, UK). 80% of the cylinder was filled with the moss core (living and dead segments of the moss shoot, litter layer and humus soil) that contained trapped air allowing oxic conditions during the incubation. All incubation vessels were placed together into holes in the moss cushions directly in the field in a forest located closed to our laboratory base at Silvermuseet (Arjeplog, Sweden; 65°57’43”N, 18°17’57”E). Continuous 15N2 incubation took place for one week, the upper and lower caps were then removed, opened tubes with moss samples were then placed back into the holes and six moss shoot samples were collected immediately after the incubation ceased (0 wk: one week 15N2 exposure) and one and two weeks after the incubation ceased (1wk: 1 week 15N2 exposures plus 1 week with open tube; 2wk: 1 week 15N2 exposures plus 2 weeks with open tube). The 0 wk exposure served as a control for 15N exposure. The one week exposure provided ample time for diazatrophs to fix 15N2 through nitrogenase activity. The one week and two week field incubations allowed for potential transfer of 15N assimilated by diazatrophs to moss tissue or other organisms within the moss microbiome. Three control samples (6 moss shoots each) for each forest site were collected before the injection of 15N2 to determine the natural abundance of 15N within the cores.
Selection of moss samples for NanoSIMS analysis
To establish the optimal samples for the NanoSIMS measurements, bulk-levels of 15N enrichment of bryosphere samples from each forest site (i.e., Njällatjirelg and Reivo) were analyzed along the incubation period using IRMS (Methods S1). We selected the highest bulk (IRMS) 15N enriched samples for high resolution NanoSIMS analysis. Bulk 15N natural abundance level was determined from control samples collected before 15N2 addition.
Resin embedding and sectioning
In parallel to IRMS sampling, twenty-four moss shoots (one shoot per sample core and time-point) were fixed for resin embedding and sectioning. Immediately after each collection, fully hydrated moss shoots were fixed in 3% (v/v) glutaraldehyde in 0.1 M phosphate buffer, pH 7.4 for 4 h. After the primary fixation, samples were rinsed three times with phosphate buffer and one individual shoot carefully stored in 15 ml buffer. Samples were shipped to the Instituto Ciencias Agrarias (CSIC, Spain) for osmium tetroxide post-fixation, resin embedding, and sectioning (Methods S2). Shoots from the highest bulk (IRMS) 15N enriched samples were selected for further fixation. Four branches per selected shoot (two from the green and two from the brown parts, Supplementary Figure 4) were embedded for sectioning. Three sections were prepared from each embedded sample for optical (0.35 µm thick), transmission electron microscopy (TEM; 80 nm thick) and dual TEM - NanoSIMS (150 nm thick) observations. Three moss shoots (two from Njällatjirelg and one Reivo) from the unlabeled samples were used as controls for NanoSIMS analysis.
NanoSIMS analysis and image processing
In situ isotopic mapping was performed at The University of Western Australia using a NanoSIMS 50 (Cameca, Gennevilliers, France), with a 16 keV Cs+ primary ion beam as described in Methods S3. Statistics from each region of interest (ROI: discrete groups of pixels that define a particular feature) were calculated. Following Berry et al. (2013), individual ROIs were considered significantly enriched in 15N if the mean value atom % 15N was above the 95th percent confidence interval of unlabeled control ROIs from each particular component of the bryosphere and if the measurement error (2σ, Poisson) was smaller than the difference between the atom % of the labeled sample and the mean atom % of unlabeled control samples. To be confident that results were representative, 540 ROIs were analyzed in thirteen rastered sections across the green portion of the stem (n = 7 individual branches, Supplementary Figure 4 and Supplementary Figure 5), 352 ROIs in ten sections across the brown portion (n = 5 individual branches, Supplementary Figure 4 and Supplementary Figure 5) and 86 ROIs in five rastered sections for unlabeled samples from the green portion of the stem (n = 3 individual branches). Samples from the brown segments of P. schreberi from Njällatjirelg were removed from data analysis after NanoSIMS measurements since we could not detect 15N enrichment despite the presence of cyanobacteria.
Microbial molecular analysis
In parallel with NanoSIMS sampling, six P. schreberi stems from Njällatjirelg (open canopy and high cyanobacteria colonization) and Reivo (variably dense canopy and moderately high cyanobacteria colonization) were collected from twelve sampling locations from each forest site. Stems were divided into light green (new growth tissue), dark green (mature tissue) and brown (senescent tissue) segments. These were pooled according to site and sampling location. For each site, stem segments of the same type from locations 1-6 and 7-12 were pooled, resulting in two pooled samples per site, per stem segment (twelve samples in total). DNA was extracted from pooled stem samples with the MoBio PowerSoil Kit according to the manufacturer’s instructions, quantified with the Qubit dsDNA HS Assay Kit on a Qubit 1.0 fluorometer (Life Technologies Ltd), and diluted to 0.5 ng/μL in 10 mM Tris pH 8.5.
Three targets were amplified for paired-end 300 bp sequencing on the Illumina MiSeq platform: Bacterial/archaeal 16S rRNA V4 with primers 515fB/806rB, fungal ITS1 with primers ITS1Fngs/ITS2 and nifH with primers IGK3/DVV. Primer sequences are listed in Supplementary Table 1. Library preparation and multiplexing were carried out using a 2-step PCR approach with the Nextera XT Index Kit (Methods S4). Bioinformatic pipelines for the three amplicon data sets are described in Methods S5. 16S rRNA amplicon data were processed with mothur 1.38 (Schloss et al., 2009; Kozich et al., 2013), and ITS1 amplicon data were processed with mothur and ITSx (Bengtsson-Palme et al., 2013). The nifH amplicon pipeline included steps in mothur, in the RDP functional gene pipeline (Fish et al., 2013), and in ARB (Ludwig et al., 2004), as well as incorporating the classification and regression trees model (Frank et al., 2016) for assigning nifH sequences to clusters and identifying paralogues. Fungal OTUs were also assigned to putative trophic mode using the FunGuild tool (Nguyen et al., 2016).
Results
Accumulation of newly fixed 15N2 in the moss and its associated microbiome
To trace the fate of fixed 15N2 at a cellular level, we correlated high-resolution morphology images (transmission electron microscopy and 12C14N NanoSIMS images) to sub-cellular scale isotope enrichment (> 0.37 atom % 15N) 12C15N NanoSIMS images (Supplementary Figure 5), to allow the identification of cell types by specific ultrastructure and the level of 15N enrichment accumulated. We detected 15N enriched cyanobacteria cells (i.e. metabolically active cells) associated with both the younger dark green segments of P. schreberi shoots (atom % 15N data = 0.47 and 0.53 [median] from Njällatjirelg and Reivo, respectively Figures 1A, B), and older brown segments (atom % 15N data = 0.85 [median] from Reivo, Figure 1C). In addition to cyanobacterial cells, 15N enrichment was observed in moss cells but the level of enrichment differed based on the age (proxy for metabolic activity; Bates, 1979) of moss tissues. In this sense, younger dark green moss segments showed higher 15N enrichment (moss atom % 15N data = 0.43 and 0.41 [median] from Njällatjirelg and Reivo respectively; range for both forests = 0.40 – 0.44, Figures 1A, B) than older brown moss segments (moss atom % 15N data = 0.39 [median] from Reivo; range = 0.39 – 0.40, Figure 1C). The percentage of 15N enriched regions of interest (ROIs, red dots in Figure 1), from the total analyzed, was also higher in the younger dark green moss segments (29%, n = 205) than in the older brown moss segments (18% n = 140). Specifically, 15N enrichment was located in the cell walls and cytoplasm of younger dark green segments of moss shoots (Figures 2E, F, from Reivo and 2h,i from Njällatjirelg: see red arrows) whereas in older brown moss segments, only discrete hotspots of 15N enrichment were observed in moss cell cytoplasm (Figures 3B, C, E, F from Reivo: see red arrows). Importantly, there was an extracellular 15N enrichment (probably extracellular polysaccharide; EPS) on older moss cell (Figure 3E, see two white arrows) that was accessible to epiphytic microbes (blue green color), whereas moss cell walls were not 15N enriched (≤ 0.37 atom % 15N, dark blue color).
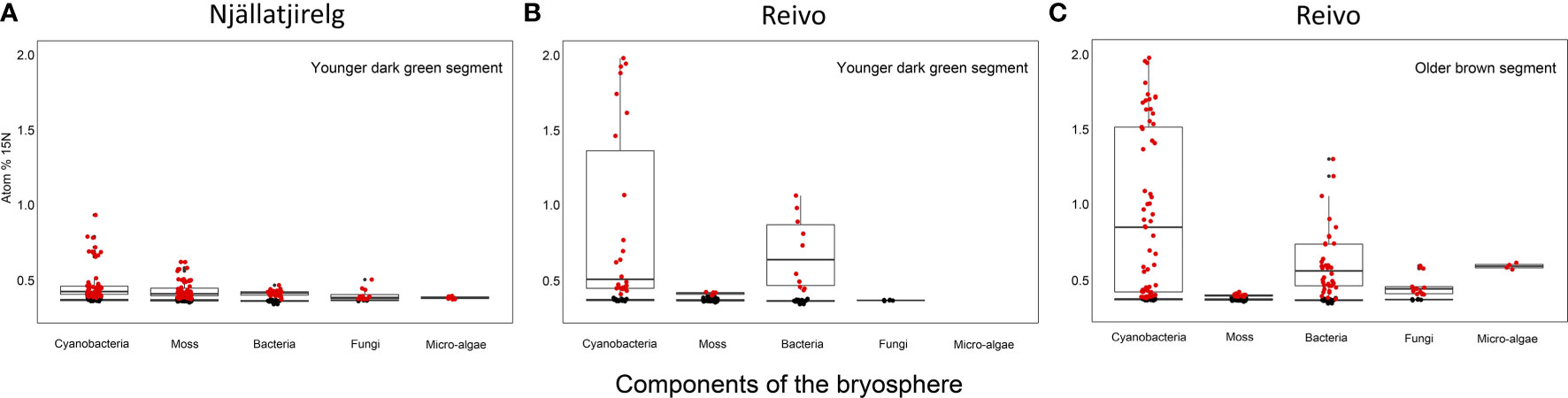
Figure 1 15N accumulation (atom% 15N) of metabolically active cell components of the bryosphere of two northern Sweden boreal forest after 15N2 incubation. (A) Upper younger dark green segment of Pleurozium schreberi shoot from Njällatjirelg, an open canopy forest with high moss N2 fixation, and (B) from Reivo, a variably dense canopy forest with moderately high N2 fixation in the moss layer, and (C) lower older brown segment of P. schreberi shoot from Reivo. Data from unlabeled replicates from each site used to generate 15N natural abundance values of each component - shown as black dots. Only data from the active (i.e. 15N enriched, red dots) cells is presented, > 95% confidence intervals of unlabeled controls and error (2σ, Poisson) smaller than the difference between the atom % of the labeled sample and the mean atom % of unlabeled control samples. Box plots summarize the quartiles of the target components of the bryosphere where boxes and whiskers encompass 25–75% and 5–95% quantiles of the data, respectively, with the median indicated by a dark horizontal line. Samples from the brown segments of P. schreberi from Njällatjirelg were removed from data analysis after NanoSIMS measurements since we could not detect 15N enrichment despite the presence of cyanobacteria. Branch location along the moss shoot selected for NanoSIMS analysis and raw images of rastered sections used for data analysis can be found in Figure S4 and Figure S6 respectively.
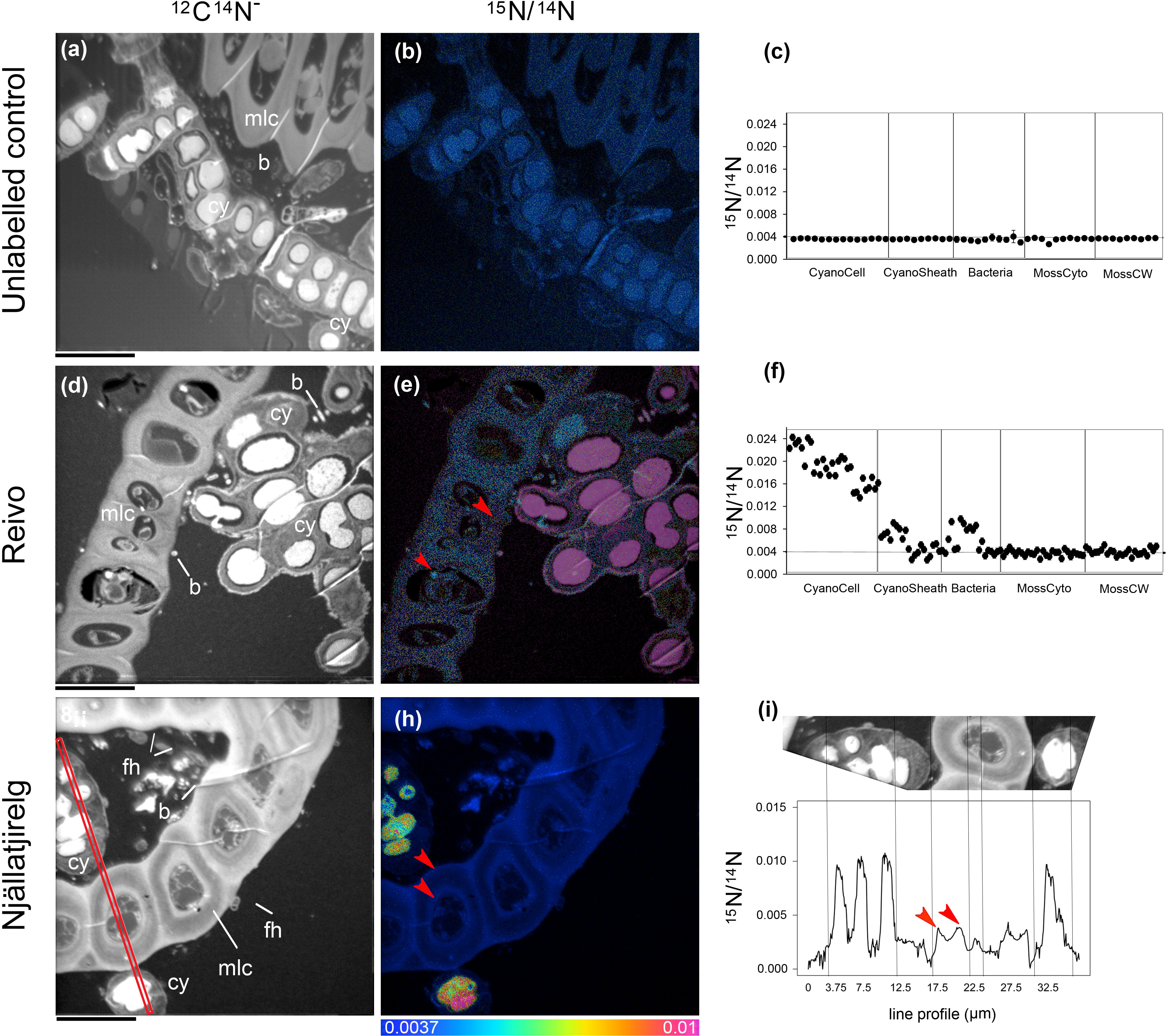
Figure 2 Spatial distribution of 15N accumulation in younger dark green segments of Pleurozium schreberi shoot and associated microbiome.15N concentration (15N/14N) of representative samples of an unlabeled shoot (A–C) and shoot labeled with 15N2 and incubated for one week (Reivo forest site d-f; Njällatjirelg forest site g-i). 15N enrichment can be distinguished in cyanobacteria cells (CyanoCell) and in the moss cell wall (MossCW) and cytoplasm (MossCyto) in both forest sites (f and red arrow in e, h and i for moss cells). Epiphytic bacterial cells in direct contact with cyanobacteria can be seen enriched in 15N (D–F). Fungal hyphae attached to the moss leaf close to the cyanobacteria cells can be distinguished (G). Cellular structures are visible in the greyscale 12C14N images (A, D, G), with corresponding 15N/14N (B, E, H) images reflecting levels of 15N enrichment. The HSI colour scale (0.0037–0.01; 15N/14N natural abundance = 0.0037) applies to all HSI images. Dots and line scans show numerical levels of 15N enrichment (15N/14N) across the subcellular regions (C, F, I), with data acquired from the line (512 × 512 pixels) (I) indicated on the respective 12C14N image (G). Structural features: cyanobacteria (cy), bacteria (b), fungal hyphae (fh), and moss leaf cell (mlc). Bars, 10 µm (for all images).
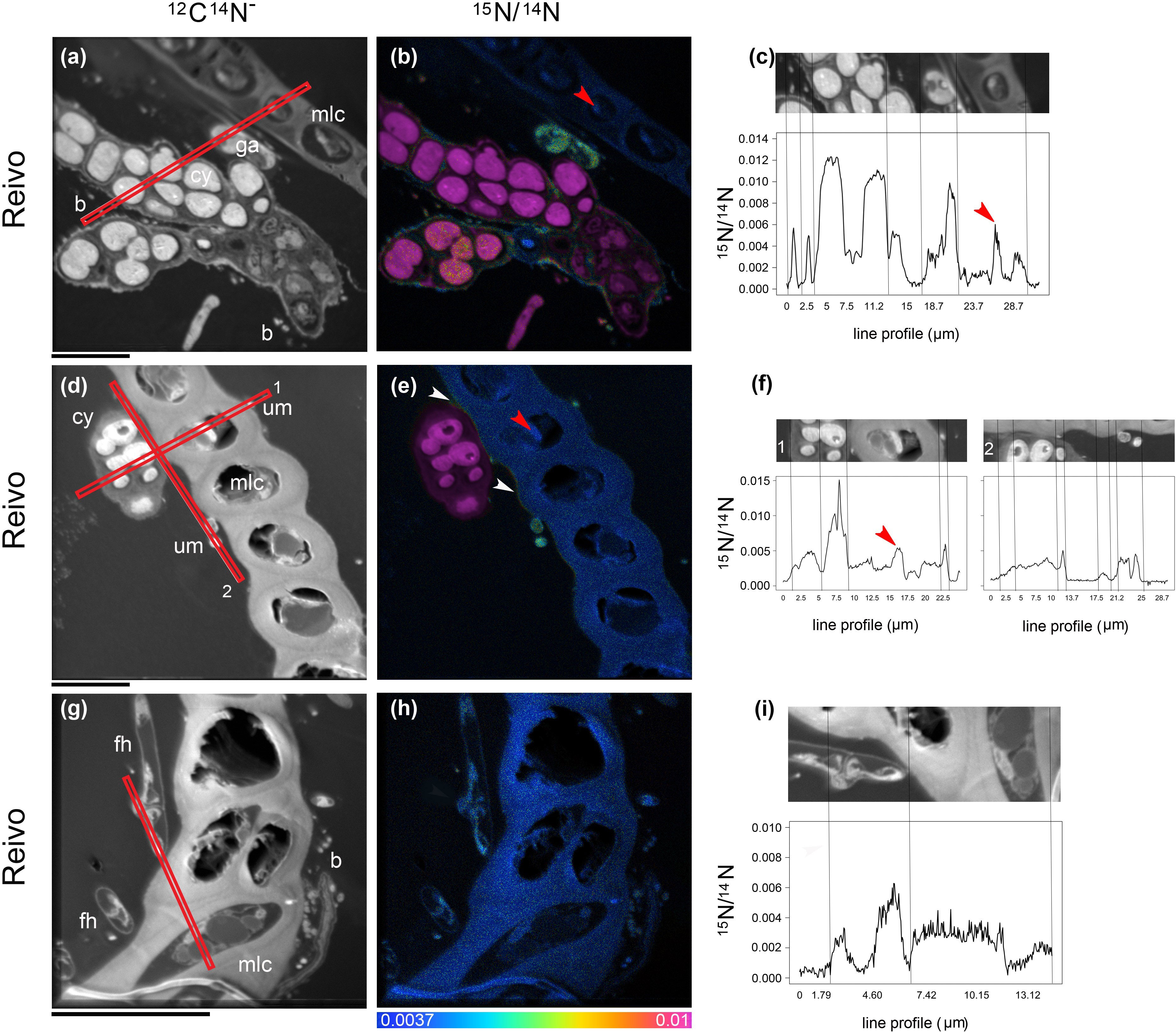
Figure 3 Spatial distribution of 15N accumulation in older brown segments of Pleurozium schreberi shoot and associated microbiome. Enrichment of both moss cells and moss-associated microbiome can be distinguished after one week of continuous exposure to 15N2 (a-i). Low 15N enrichment within the moss cell can be detected in some organelles (red arrow in B, C and E, F) whereas the cell wall and lipid bodies are not enriched (< 0.0037 15N/14N, f). Epiphytic bacterial cells in direct contact with cyanobacteria can be seen enriched in 15N (A–C); the uptake of 15N fixed by cyanobacteria cells into green algae (A–C) and a hypha from a Basidiomycete (distinguished by a clamp connection at the hyphal septa, white arrows in G–I) were also detected. In (D–F) an extracellular gradient of 15N enrichment is visible over the moss cell (indicated by two white arrows). Epiphytic microbes seated on the moss leaf have access to the fixed 15N and were enriched (E, F), whereas the moss cell walls are not enriched (< 0.0037 15N/14N). Cellular structures are visible in the greyscale 12C14N images (A, D, G), with corresponding 15N/14N (B, E, H) images reflecting levels of 15N enrichment. The HSI color scale (0.0037–0.01; 15N/14N natural abundance = 0.0037) applies to all HIS images. Line scans (512 x 512 pixels) show numerical levels of 15N enrichment (15N/14N) across the subcellular regions (C, F, I), with data acquired from the lines indicated on each of the respective 12C14N images. Structural features: cyanobacteria (cy), bacteria (b), fungal hyphae (fh), unicellular green algae (ga), unidentified microbes (um) and moss leaf cell (mlc). Bars, 10 µm (for all images).
The non-cyanobacterial microbiome (i.e. other bacteria, fungi, and micro-algae) associated with P. schreberi was enriched in 15N compared with unlabeled controls (Figure 1). The enrichment (> 0.37 atom % 15N) in heterotrophic bacterial cells was higher in Reivo (0.43 - 1.06 atom % 15N in younger green segments and 0.37 - 1.30 atom % 15N in older brown moss segments, Figures 1B, C) than in Njällatjirelg (range 0.37 – 0.47 atom % 15N in green segments, Figure 1A). Fungal cells associated with younger dark green segments in Njällatjirelg had an enrichment above natural abundance of 0.38 atom % 15N (median) with a maximum of 0.50 atom % (Figure 1A); in Reivo fungal cells associated with older brown moss segments had an enrichment of 0.44 atom % 15N (median) with a maximum of 0.59 atom % (Figure 1C). The 15N enrichment of micro-algae was between 0.38 and 0.59 atom % 15N (median) from Njällatjirelg and Reivo respectively; the highest 15N enrichment measured in an individual micro-algae cell was 0.40 atom % in Njällatjirelg and 0.61 atom % in Reivo (Figures 1A, C). NanoSIMS images also revealed that the highest 15N enrichment in bacteria cells were observed in close proximity to the cyanobacteria (Figures 2D–F vs. 2g-i and Figures 3A–C vs. 3g-i, Supplementary Figure 6 for all rastered sections). NanoSIMS images also revealed uptake of 15N fixed by cyanobacteria cells into green algae (Figures 3A–C) and the hyphae of a Basidiomycete, distinguished by a clamp connection at the hyphal septa (Figures 3G–I: white arrow).
Bacterial diversity and N fixation capacity of the moss microbiome
We estimated that ∼24% (n = 100) of the total moss-associated heterotrophic bacteria in Njällatjirelg and ~53% (n = 86) in Reivo were enriched in 15N (Figure 1, red dots), indicating active uptake of 15N2. To help elucidate whether these heterotrophic bacteria were capable of direct N2 fixation, we profiled both the total bacterial community (targeting the 16S rRNA gene) and the diazotrophic (N2 fixing) community (targeting the nifH gene that encodes nitrogenase subunits). The total bacterial communities across both forest sites were dominated by four phyla, namely Proteobacteria (classes Alpha-, Beta- and Gammaproteobacteria), Acidobacteria, Actinobacteria, and Cyanobacteria (Figure 4 and Supplementary Table 2), with large relative abundances of potential N2 fixing proteobacterial taxa such as Burkholderia spp., Pseudomonas spp (Bragina et al., 2012; Bragina et al., 2013), and Alphaproteobacteria (Holland-Moritz et al., 2018).
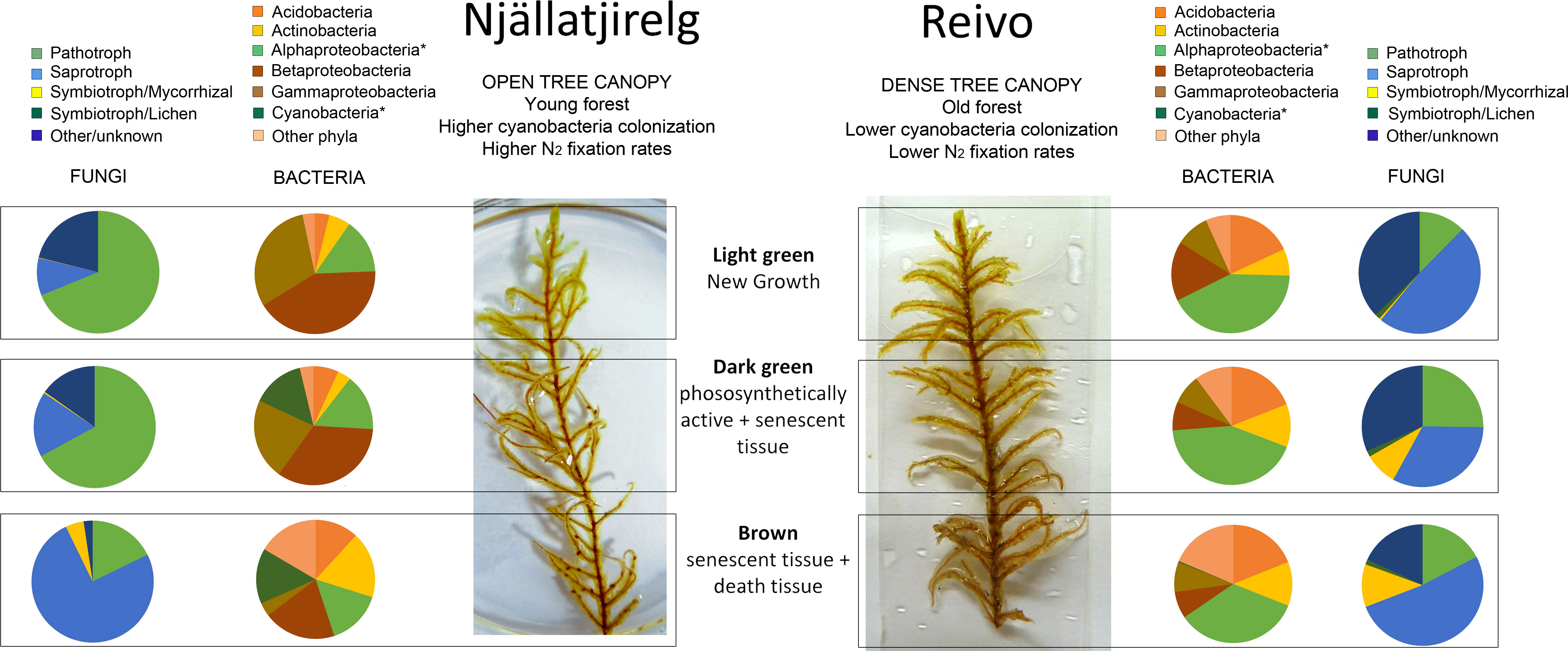
Figure 4 Bacterial and fungal community shifts along the Pleurozium schreberi shoot profile revealed by 16S and ITS analysis from two northern Sweden boreal forests. Pie charts represent relative abundance of the major bacterial taxa (brownish pie charts) and fungal trophic mode (blueish pie charts) associated with the light green (new growth tissue), dark green (mature tissue) and brown (senescent tissues) segments of the moss P. schreberi. Samples collected in Njällatjirelg, open canopy forest with high nitrogenase activity, and Reivo, variably dense canopy and moderately nitrogenase activity. Results are based on the number of sequence reads from taxa that could be assigned to genus level or lower (detailed results on Supplementary Table 2 and Supplementary Table 3). Bacterial taxa marked with asterisks were present in the nifH data set (Cyanobacteria 99.90 % and Alphaproteobacteria 0.10 % of the total number of nifH sequences).
In contrast, the nifH data were dominated by cyanobacterial sequences (Supplementary Figure 7), with heterotrophic bacterial nifH sequences, clustering with the Alphaproteobacteria, accounting for only 0.1% of the total (18 out of 17768 nifH sequences, Supplementary Figure 7A). The cyanobacterial nifH sequences fell into three clusters (Supplementary Figure 7B): i) a Nostoc cluster (0.60% total nifH reads), ii) a Stigonema cluster (0.15% total nifH reads) that has been described previously (Ininbergs et al., 2011) and contains nifH sequences of cyanobacteria found in association with P. schreberi and Hylocomium splendens (i.e. bands D, J, 6, 17 and clones A11, C11, E11) (Ininbergs et al., 2011), and iii) a third cluster, here provisionally named Stigonema cluster II, that included some sequences from cyanobacteria associated with boreal moss species (i.e. clones H10, G10, 6, 29 and 3-17) (Ininbergs et al., 2011; Leppanen et al., 2013), as well as numerous Stigonema species. Stigonema cluster II contained the majority of the nifH sequences generated in this study (99.15%), suggesting that this cluster is the main contributor of the newly fixed N input among N-fixer of the diazotrophic community studied here. The identification of these key community members was further confirmed by BLAST analyses of representative sequences from the 16S rRNA cyanobacterial OTUs, which showed that 97.2% of cyanobacterial 16S rRNA sequences could be placed in the genus Stigonema, with the remaining 2.8% of cyanobacterial sequences belonging to the genus Nostoc.
The 16S rRNA data showed distinct distribution profiles of bacterial OTUs between forest sites and tissue type (Supplementary Figure 8), with clear differences in relative abundance emerging at the phylum level (Figure 4). In particular, the distribution of cyanobacteria was not uniform, but rather was localized to the dark green and brown segments of P. schreberi from Njällatjirelg (∼14% both, Figure 4), with lower abundances detected in Reivo (∼0.34% brown segments, Figure 4), the lower N2 fixation site (Supplementary Figure 1). Betaproteobacteria and Gammaproteobacteria were more abundant in Njällatjirelg and on younger segments (light and dark green segments), notably Burkholderia spp. (21%), other Burkholderiales (15%) and Pseudomonas spp. (24%) (Supplementary Table 1). In Reivo, Alphaproteobacteria were more abundant (38%) - notably taxa from the order Rhizobiales (10%) and the Acetobacteraceae (16%) - and were evenly distributed along the moss shoot. Acidobacteria (18%), notably Granulicella spp., were more abundant on P. schreberi growing in Reivo and evenly distributed along the moss shoot (Figure 4). The relative abundance of Actinobacteria was similar between forest sites and increased with depth along the moss shoot (i.e., higher abundance on old, brown segments at the base of stems) (Figure 4).
Fungal diversity of the moss microbiome
Fungal community composition was strongly influenced by forest site and, to a lesser degree, by moss tissue type (Supplementary Figure 8). The bryosphere was dominated by fungi from the Ascomycota (74%); and Basidiomycota (21%) (Supplementary Table 3). Fungal community structure and composition varied between the forest sites. In Njällatjirelg, the fungal community was characterized by the saprotrophic fungi Penicillium sp., Hypocrea sp., Cantharellula umbonata, Cystofilobasidium capitatum, Mortierella sp., the parasitic fungi Cystodendron sp., Phacidium lacerum, the yeast Cryptococcus victoriae and the ericoid mycorrhizal fungi Oidodendron sp. At Reivo, the P. schreberi-associated fungal community was characterized by the parasitic fungi Cadophora sp., Hyaloscypha sp, Venturia sp., the saprotrophic fungi Cladophialophora sp. and Rhodotorula sp. and the ectomycorrhizal fungi Clavulina sp. Most taxa (161 genera, 52.4% of the total recovered sequences) could be confidently assigned a unique trophic mode (Supplementary Table 4). Plant pathogens were more abundant (∼ 68% of total assigned reads) in Njällatjirelg and associated with younger segments (light and dark green), while saprotrophs were more abundant in Reivo across the moss shoot and in particular associated with older brown segments (senescent tissues ∼50%, Figure 4). Symbiotrophic fungi were dominated by mycorrhizal fungi (Figure 4). Mycorrhizal fungi were mainly recovered from Reivo and associated with senescent tissue (dark green and brown segments, Figure 4, Supplementary Table 5).
Discussion
Our results confirm that N newly fixed in feather-mosses can be readily transferred to moss cells and co-existing microbes. The idea that N2 fixed by cyanobacteria associated with mosses could be incorporated into other bryobiota was first suggested by Jones and Wilson (1978) four decades ago; however, since then, it has been raised by others, but never empirically addressed (Solheim et al., 2002; Solheim et al., 2004; Lindo et al., 2013; Kardol et al., 2016). Our results, obtained from two distinctive locations in the northern Swedish boreal forest, provide compelling evidence that not all of the N2 fixed in P. schreberi is transferred to the moss and at least some is consumed by organisms within the moss microbiome, which has implications for wider ecosystem function as discussed below. This new knowledge of fixed-N pathways is crucial to understanding the functional significance of moss-cyanobacteria associations in boreal forest ecosystems where N is limited.
Fixed-N accumulation in multiple microbial groups within the boreal bryosphere
NanoSIMS images revealed the presence of 15N2 fixed within cyanobacteria cells and its presence adjacent moss cells and the moss microbiome, including heterotrophic bacteria, fungi and micro-algae. We propose three possible pathways for the transfer of fixed N, based on previous studies of Sphagnum-methanotroph systems (Ho and Bodelier, 2015) and bryophyte-cyanobacteria systems (Jones and Wilson, 1978; Lindo et al., 2013): 1) Fixed N may end up in the moss tissue and microbiome via an indirect pathway of cyanobacterial cell death and lysis; 2) Bacterivores grazing P. schreberi may ingest and subsequently release part of the N2 fixed by cyanobacteria prey cells; 3) A direct pathway involving an exchange of N-compounds between the cyanobacteria, moss tissue and moss microbiome. The close proximity of cyanobacteria, moss tissue and other microbial cells (e.g., bacteria, micro-algae and fungi) coupled with the level of 15N enrichment observed on younger photosynthetically active moss cells (maximum 0.44 atom % 15N) and moss-microbiome (maximum 1.30, 0.59, 0.61 atom % 15N in heterotrophic bacterial, fungal and micro-algae cells) suggest a direct exchange of N; however, the first two pathways may also occur. On the other hand, the high level of 15N enrichment observed in some bacterial cells could also suggest the presence of other N2-fixers associated with the moss (e.g., N2 fixing proteobacteria) as discussed below.
The widespread incorporation of cyanobacteria-fixed 15N2 observed here has major ecological implications for N cycling and turnover within the broader boreal forest floor. Specifically, the capacity of the moss-cyanobacteria association to impact N cycling at ecosystem level depends on the fate of the newly fixed N within the bryosphere. Based on our observations, we confirm that fixed N can flow into the boreal ecosystem via a slow turnover pathway where N is incorporated into photosynthetically active moss tissues that are subsequently decomposed (Lang et al., 2009), and a fast pathway where N is incorporated into bacteria, fungal, and micro-algal biomass. In the former, the fixed N is highly conserved in the moss tissue (Turetsky, 2003; Gavazov et al., 2010; Rousk et al., 2014, Rousk et al., 2016), and slowly incorporated into the underlying litter and humus layer within a timescale of several years to decades (Lindo et al., 2013; DeLuca et al., 2022). In the latter, N2 fixed by cyanobacteria is rapidly available for transfer across trophic levels within the microbial and mesofaunal food web [e.g., bacterial food chain: bacteria, protozoa, rotifers, nematodes, arthropods, and the fungal food chain: saprophytic and mycorrhizal fungi, fungivorous arthropods, and nematodes (Lindo et al., 2013)]. These results are also consistent with the fast transfer of fixed 15N2 associated with mosses into other components of the biosphere (e.g. soil microbes, other plants) in a High Arctic, N-limited ecosystem (Rousk et al. (2017). These results support the view that cyanobacterial fixed-N can supplement the microbial food web associated with the mosses reinforcing the influence of this N source in key ecosystem processes in which bacterial- and fungal-based food webs are involved (i.e. organic matter decomposition, carbon sequestration, nutrient cycling; Moquin et al., 2012; Bragina et al., 2014; Jonsson et al., 2015; Kardol et al., 2016).
Cyanobacterial N2 fixation is far greater than heterotrophic N2 fixation in mosses
The potential for heterotrophic N2 fixation has been suggested in feather-mosses (Warshan et al., 2016; Holland-Moritz et al., 2018); however, its relative contribution to the total N2 fixation in boreal forest is not clear. We observed that although heterotrophic nifH sequences were present, their relative abundance was low (0.1%, see Supplementary Figure 7A). Similar to previous research (Ininbergs et al., 2011; Leppanen et al., 2015; Warshan et al., 2016), our results indicate that cyanobacteria are the most abundant diazotrophic group, where others may also occur as casual epiphytes on the feather-moss. We detected an anomalously low number of cyanobacterial nifH reads from the Reivo forest, the mature forest site, despite cyanobacteria being readily observed under NanoSIMS and microscopy analysis (Figures 2, 3). In particular, Warshan et al. (2016) showed variations of nifH gene abundance by sampling date, moss species and cyanobacterial cluster, which may partially explain differences in nifH abundance between both forest sites (i.e. this may reflect a primer bias due to cyanobacteria at Reivo being of a different strain or species from those at Njällatjirelg). In this light, we consider that even though the nifH gene has high taxonomic resolution among molecular markers used to study feather-moss associated cyanobacteria (as shown by Ininbergs et al., 2011), the actual number of cyanobacterial strains associated with boreal feathermosses may not necessarily be accurately estimated solely by using the nifH gene abundance (Gaby and Buckley, 2012; Warshan, 2017).
Given the caveats expressed above, the low contribution of heterotrophic N fixation was also apparent by linking taxonomic and functional patterns of Proteobacteria associated with P. schreberi. Despite the large relative abundances of putative N2 fixing proteobacterial taxa (Bragina et al., 2012; Bragina et al., 2013; Holland-Moritz et al., 2018) identified in our 16S rRNA assays in Reivo, we detected very few Proteobacterial nifH sequences (indicative of actual N2 fixation capacity) in both forest communities (only 10 sequences in Njällatjirelg samples and 8 sequences in Reivo: Supplementary Figure 7A). The high proportions of 15N-enriched bacteria found in both forest sites (24% in Njällatjirelg and 53% in Reivo) and, particularly, those found in close proximity to cyanobacteria (Figures 2D–F vs. 2g-i and Figures 3A–C vs. 3g-i, Supplementary Figure 5 for all rastered sections) suggest, therefore, that N released from the cyanobacteria is more likely consumed by co-occurring heterotrophic bacteria than derived from N2 fixation by heterotrophic bacteria. To date, few studies have described the bacterial community structure in bryophyte communities with notable exception of Sphagnum species (Estrada-De los Santos et al., 2001; Opelt et al., 2007; Moquin et al., 2012; Bragina et al., 2013; Jassey et al., 2013; Bragina et al., 2014) and boreal feather-mosses (Ininbergs et al., 2011; Leppanen et al., 2015; Warshan et al., 2016; Holland-Moritz et al., 2018). Unlike previous studies, however, we directly link the bacterial community structure to boreal ecosystem functioning by combining high resolution isotope imaging (Nano-SIMS) and high-throughput amplicon sequencing of several targets (16S rRNA, nifH). Our results show that, most likely, cyanobacteria-fixed N is assimilated by heterotrophic bacteria; this may alleviate N-limitation in bacterial decomposer communities and flow up to other trophic levels within the bacterial food chain. Overall, our results demonstrate that bacteria are key players in the transformation of the cyanobacterial fixed-N within the boreal bryosphere, warranting further and more specific inquiry (i.e. to determine their growth dynamics, N loss pathways etc.).
Fungal communities benefit from the newly fixed N2
The moss-associated fungal community detected here, represented a diverse assemblage of putative plant pathogens, saprotrophs and symbiotrophic fungi, which is consistent with previous reports of fungal community structure in boreal feather-mosses (Davey et al., 2017). NanoSIMS imaging results provide good evidence that fungi associated with P. schreberi do assimilate recently fixed N and into the fungal food chain consisting of saprophytic fungi, arbuscular- and ecto-mycorrhizal fungi, fungivorous arthropods, and nematodes (Lindo and Gonzalez, 2010). Remarkably, we found hyphae from Basidiomycetes enriched with 15N (Figures 3G–I white arrow) where mycorrhizae associated with the moss were more diverse and abundant (old segments of shoot samples from Reivo, Figure 4). Although these results do not prove a mycorrhizal pathway by which N fixed within the moss-cyanobacteria system is transferred to vascular plants (Carleton and Read, 1991; Lindo et al., 2013), it does provide new insight into the benefit obtained by the fungal community when growing in association within the bryosphere microbiome (Davey and Currah, 2006).
Conclusions
During the past four decades, scientists have argued about the possible pathways by which N2 fixed by cyanobacteria associated with mosses is incorporated into the wider boreal biome. Our results provide, for the first time under natural conditions, an explicit demonstration that N2 fixed by cyanobacteria associated with boreal feather-mosses can follow multiple transfer pathways including through the microbiome (Lindo and Gonzalez, 2010). By doing so, this study demonstrates that the assumption that only decomposition can release newly fixed N2 conserved in the moss tissue is not correct and provides compelling evidence that there are rapid transfer pathways through the microbial food webs associated with the feather-moss microbiome of P. schreberi. Overall, isotope imaging of 15N clearly exposed the complex and contrasting pathways by which newly fixed N2 enters and cycles through the boreal forest floor and highlights the need to better understand the ecological role of moss-cyanobacteria associations as part of a more complex boreal bryosphere.
Data availability statement
The original contributions presented in the study are publicly available. This data can be found here: NCBI, PRJNA376120, SRX2661814-SRX2661825 (ITS1), SRX2661826-SRX2661837 (16S rRNA) and SRX3747711-SRX3747722 (nifH). Representative nifH sequences used for phylogenetic analyses are available from GenBank, accession numbers MH019288- MH019294.
Author contributions
DM, MA-C, TD, PC, DJ, and JB designed the study. MA-C, TD, and JB performed the research. VS-E, MA-C, PC, and SW prepared moss samples for microscopy, TEM and NanoSIMS analyses and performed 80 nm TEM microscopy analyses. JB performed the NanoSIMS analysis. DC, NC, and MA-C carried out the molecular analysis. DC and NC carried out the bioinformatics analysis. MA-C and JB analyzed the NanoSIMS data. MA-C, JB, and DM wrote the initial draft of the paper. TD, NC, NO, DJ, DC, VS-E, and PC discussed the results and worked on manuscript revisions. All authors contributed to the article and approved the submitted version.
Acknowledgments
We thank Dr Karen Moore, Audrey Farbos and Dr Anja Nenninger for help during molecular analysis. We are grateful to Sarah Chesworth and Gwen Lancashire (Bangor University, UK) and Greger Hörnberg and Ingela Bergman from Silvermuseet (Arjeplog, Sweden) providing assistance with field and lab work. We thank Silvia Pressel and Jeffrey G. Duckett (Natural History Museum, London) for their useful advice on resin embedding protocol for Pleurozium schreberi shoots. Simon Oakley, Kelly Mason (UK CEH Lancaster, UK) and Lorna E. Street (Heriot-Watt University, UK) for their useful comments during workshops and group meetings. We acknowledge support from Swedish Research Council grant FORMAS 2003-618 (to TD); NERC grant NE/I027150/1 (to TD and DJ); Australian Research Council Future Fellowship FT110100246 (to DM). The authors acknowledge the use of the Australian Microscopy and Microanalysis Research Facility (CMCA, The University of Western Australia); the Advanced Microscopy and Bioimaging Facility (Aberystwyth University, UK); the Servicio de Microscopı́a Electrónica at the Instituto Ciencias Agrarias (CSIC, Spain); the Exeter Sequencing Service facility, supported by the Wellcome Trust Institutional Strategic Support Fund (WT097835MF), the Wellcome Trust Multi User Equipment Award (WT101650MA), Medical Research Council Clinical Infrastructure Funding (MR/M008924/1) and the BBSRC LOLA award (BB/K003240/1).
Funding
MA-C was also supported by the Comunidad de Madrid (Spain) and European Structural and Investment Funds (projects AGRISOST-CM S2013/ABI2717 and S2018/BAA4330).
Conflict of interest
The authors declare that the research was conducted in the absence of any commercial or financial relationships that could be construed as a potential conflict of interest.
Publisher’s note
All claims expressed in this article are solely those of the authors and do not necessarily represent those of their affiliated organizations, or those of the publisher, the editors and the reviewers. Any product that may be evaluated in this article, or claim that may be made by its manufacturer, is not guaranteed or endorsed by the publisher.
Supplementary material
The Supplementary Material for this article can be found online at: https://www.frontiersin.org/articles/10.3389/fpls.2022.1036258/full#supplementary-material
References
Bates, J. W. (1979). Relationship between physiological vitality and age in shoot segments of Pleurozium-schreberi (Brid) mitt. J. Bryology 10, 339–351. doi: 10.1179/jbr.1979.10.3.339
Bay, G., Nahar, N., Oubre, M., Whitehouse, M. J., Wardle, D. A., Zackrisson, O., et al. (2013). Boreal Feather mosses secrete chemical signals to gain nitrogen. New Phytol. 200, 54–60. doi: 10.1111/nph.12403
Bengtsson-Palme, J., Ryberg, M., Hartmann, M., Branco, S., Wang, Z., Godhe, A., et al. (2013). Improved software detection and extraction of ITS1 and ITS2 from ribosomal ITS sequences of fungi and other eukaryotes for analysis of environmental sequencing data. Methods Ecol. Evol. 4, 914–919. doi: 10.1111/2041-210X.12073
Berg, A., Danielsson, A., Svensson, B. H. (2013). Transfer of fixed-n from N2-fixing cyanobacteria associated with the moss Sphagnum riparium results in enhanced growth of the moss. Plant Soil 362, 271–278. doi: 10.1007/s11104-012-1278-4
Berry, D., Stecher, B., Schintlmeister, A., Reichert, J., Brugiroux, S., Wild, B., et al. (2013). Host-compound foraging by intestinal microbiota revealed by single-cell stable isotope probing. Proc. Natl. Acad. Sci. U.S.A. 110, 4720–4725. doi: 10.1073/pnas.1219247110
Bragina, A., Berg, C., Muller, H., Moser, D., Berg, G. (2013). Insights into functional bacterial diversity and its effects on alpine bog ecosystem functioning. Sci. Rep. 3, 1955. doi: 10.1038/srep01955
Bragina, A., Maier, S., Berg, C., Muller, H., Chobot, V., Hadacek, F., et al. (2012). Similar diversity of alphaproteobacteria and nitrogenase gene amplicons on two related sphagnum mosses. Front. Microbiol. 3, 10. doi: 10.3389/fmicb.2011.00275
Bragina, A., Oberauner-Wappis, L., Zachow, C., Halwachs, B., Thallinger, G. G., Muller, H., et al. (2014). The sphagnum microbiome supports bog ecosystem functioning under extreme conditions. Mol. Ecol. 23, 4498–4510. doi: 10.1111/mec.12885
Carleton, T. J., Read, D. J. (1991). Ectomycorrhizas and nutrient transfer in conifer feather moss ecosystems. Can. J. Bot. 69, 778–785. doi: 10.1139/b91-101
Davey, M. L., Currah, R. S. (2006). Interactions between mosses (Bryophyta) and fungi. Can. J. Bot. 84, 1509–1519. doi: 10.1139/b06-120
Davey, M. L., Heegaard, E., Halvorsen, R., Kauserud, H., Ohlson, M. (2013). Amplicon-pyrosequencing-based detection of compositional shifts in bryophyte-associated fungal communities along an elevation gradient. Mol. Ecol. 22, 368–383. doi: 10.1111/mec.12122
Davey, M. L., Skogen, M. J., Heegaard, E., Halvorsen, R., Kauserud, H., Ohlson, M. (2017). Host and tissue variations overshadow the response of boreal moss-associated fungal communities to increased nitrogen load. Mol. Ecol. 26, 571–588. doi: 10.1111/mec.13938
DeLuca, T. H., Boisvenue, C. (2012). Boreal Forest soil carbon: Distribution, function and modelling. Forestry 85, 161–184. doi: 10.1093/forestry/cps003
DeLuca, T. H., Nilsson, M. C., Zackrisson, O. (2002a). Nitrogen mineralization and phenol accumulation along a fire chronosequence in northern Sweden. Oecologia 133, 206–214. doi: 10.1007/s00442-002-1025-2
DeLuca, T. H., Zackrisson, O., Gundale, M. J., Nilsson, M. C. (2008). Ecosystem feedbacks and nitrogen fixation in boreal forests. Science 320, 1181–1181. doi: 10.1126/science.1154836
DeLuca, T. H., Zackrisson, O., Nilsson, M. C., Sellstedt, A. (2002b). Quantifying nitrogen-fixation in feather moss carpets of boreal forests. Nature 419, 917–920. doi: 10.1038/nature01051
DeLuca, T. H., Zackrisson, O., Nilsson, M. C., Sun, S. Q., Arroniz-Crespo, M. (2022). Long-term fate of nitrogen fixation in Pleurozium schreberi brid (Mitt.) moss carpets in boreal forests. Appl. Soil Ecol. 169, 104215. doi: 10.1016/j.apsoil.2021.104215
Estrada-De los Santos, P., Bustillos-Cristales, R., Caballero-Mellado, J. (2001). Burkholderia, a genus rich in plant-associated nitrogen fixers with wide environmental and geographic distribution. Appl. Environ. Microbiol. 67, 2790–2798. doi: 10.1128/AEM.67.6.2790-2798.2001
Fish, J. A., Chai, B. L., Wang, Q., Sun, Y. N., Brown, C. T., Tiedje, J. M., et al. (2013). FunGene: The functional gene pipeline and repository. Front. Microbiol. 4, 14. doi: 10.3389/fmicb.2013.00291
Frank, I. E., Turk-Kubo, K. A., Zehr, J. P. (2016). Rapid annotation of nifH gene sequences using classification and regression trees facilitates environmental functional gene analysis. Environ. Microbiol. Rep. 8, 905–916. doi: 10.1111/1758-2229.12455
Gaby, J. C., Buckley, D. H. (2012). A comprehensive evaluation of PCR primers to amplify the nifH gene of nitrogenase. PloS One 7, 12. doi: 10.1371/journal.pone.0042149
Gavazov, K. S., Soudzilovskaia, N. A., van Logtestijn, R. S. P., Braster, M., Cornelissen, J. H. C. (2010). Isotopic analysis of cyanobacterial nitrogen fixation associated with subarctic lichen and bryophyte species. Plant Soil 333, 507–517. doi: 10.1007/s11104-010-0374-6
Ho, A., Bodelier, P. L. E. (2015). Diazotrophic methanotrophs in peatlands: The missing link? Plant Soil 389, 419–423. doi: 10.1007/s11104-015-2393-9
Holland-Moritz, H., Stuart, J., Lewis, L., Miller, S., Mack, M., McDaniel, S., N. F. (2018). Novel bacterial lineages associated with boreal moss species. Environ. Microbiol. 20, 13. doi: 10.1111/1462-2920.14288
Hyodo, F., Kusaka, S., Wardle, D. A., Nilsson, M. C. (2013). Changes in stable nitrogen and carbon isotope ratios of plants and soil across a boreal forest fire chronosequence. Plant Soil 364, 315–323. doi: 10.1007/s11104-012-1339-8
Ininbergs, K., Bay, G., Rasmussen, U., Wardle, D. A., Nilsson, M. C. (2011). Composition and diversity of nifH genes of nitrogen-fixing cyanobacteria associated with boreal forest feather mosses. New Phytol. 192, 507–517. doi: 10.1111/j.1469-8137.2011.03809.x
Jassey, V. E. J., Chiapusio, G., Binet, P., Buttler, A., Laggoun-Defarge, F., Delarue, F., et al. (2013). Above- and belowground linkages in sphagnum peatland: Climate warming affects plant-microbial interactions. Global Change Biol. 19, 811–823. doi: 10.1111/gcb.12075
Jones, K., Wilson, R. E. (1978). The fate of nitrogen fixed by a free living blue-green alga. Ecol. Bulletins 26, 158–163.
Jonsson, M., Kardol, P., Gundale, M. J., Bansal, S., Nilsson, M. C., Metcalfe, D. B., et al. (2015). Direct and indirect drivers of moss community structure, function, and associated microfauna across a successional gradient. Ecosystems 18, 154–169. doi: 10.1007/s10021-014-9819-8
Kardol, P., Spitzer, C. M., Gundale, M. J., Nilsson, M.-C., Wardle, D. A. (2016). Trophic cascades in the bryosphere: The impact of global change factors on top-down control of cyanobacterial N2-fixation. Ecol. Lett. 19, 967–976. doi: 10.1111/ele.12635
Kozich, J. J., Westcott, S. L., Baxter, N. T., Highlander, S. K., Schloss, P. D. (2013). Development of a dual-index sequencing strategy and curation pipeline for analyzing amplicon sequence data on the MiSeq illumina sequencing platform. Appl. Environ. Microbiol. 79, 5112–5120. doi: 10.1128/AEM.01043-13
Lang, S. I., Cornelissen, J. H. C., Klahn, T., van Logtestijn, R. S. P., Broekman, R., Schweikert, W., et al. (2009). An experimental comparison of chemical traits and litter decomposition rates in a diverse range of subarctic bryophyte, lichen and vascular plant species. J. Ecol. 97, 886–900. doi: 10.1111/j.1365-2745.2009.01538.x
Leppanen, S., Rissanen, A., Tiirola, M. (2015). Nitrogen fixation in sphagnum mosses is affected by moss species and water table level. Plant Soil 389, 185–196. doi: 10.1007/s11104-014-2356-6
Leppanen, S. M., Salemaa, M., Smolander, A., Makipaa, R., Tiirola, M. (2013). Nitrogen fixation and methanotrophy in forest mosses along a n deposition gradient. Environ. Exp. Bot. 90, 62–69. doi: 10.1016/j.envexpbot.2012.12.006
Lindo, Z., Gonzalez, A. (2010). The bryosphere: An integral and influential component of the earth's biosphere. Ecosystems 13, 612–627. doi: 10.1007/s10021-010-9336-3
Lindo, Z., Nilsson, M. C., Gundale, M. J. (2013). Bryophyte-cyanobacteria associations as regulators of the northern latitude carbon balance in response to global change. Global Change Biol. 19, 2022–2035. doi: 10.1111/gcb.12175
Ludwig, W., Strunk, O., Westram, R., Richter, L., Meier, H., Yadhukumar, A., et al. (2004). ARB: a software environment for sequence data. Nucleic Acids Res. 32, 1363–1371. doi: 10.1093/nar/gkh293
Moquin, S. A., Garcia, J. R., Brantley, S. L., Takacs-Vesbach, C. D., Shepherd, U. L. (2012). Bacterial diversity of bryophyte-dominant biological soil crusts and associated mites. J. Arid Environments 87, 110–117. doi: 10.1016/j.jaridenv.2012.05.004
Nguyen, N. H., Song, Z. W., Bates, S. T., Branco, S., Tedersoo, L., Menke, J., et al. (2016). FUNGuild: An open annotation tool for parsing fungal community datasets by ecological guild. Fungal Ecol. 20, 241–248. doi: 10.1016/j.funeco.2015.06.006
Opelt, K., Chobot, V., Hadacek, F., Schonmann, S., Eberl, L., Berg, G. (2007). Investigations of the structure and function of bacterial communities associated with sphagnum mosses. Environ. Microbiol. 9, 2795–2809. doi: 10.1111/j.1462-2920.2007.01391.x
Osono, T., Trofymow, J. A. (2012). Microfungal diversity associated with Kindbergia oregana in successional forests of British Columbia. Ecol. Res. 27, 35–41. doi: 10.1007/s11284-011-0866-8
Renaudin, M., Blasi, C., Bradley, R. L., Bellenger, J. P. (2022a). New insights into the drivers of moss-associated nitrogen fixation and cyanobacterial biomass in the eastern Canadian boreal forest. J. Ecol. 110, 1403–1418. doi: 10.1111/1365-2745.13881
Renaudin, M., Laforest-Lapointe, I., Bellenger, J. P. (2022b). Unraveling global and diazotrophic bacteriomes of boreal forest floor feather mosses and their environmental drivers at the ecosystem and at the plant scale in north America. Sci. Total Environ. 837, 155761. doi: 10.1016/j.scitotenv.2022.155761
Rousk, K., Jones, D. L., DeLuca, T. H. (2014). Moss-nitrogen input to boreal forest soils: Tracking 15N in a field experiment. Soil biology and. Biochemistry 72, 100–104. doi: 10.1016/j.soilbio.2014.01.031
Rousk, K., Sorensen, P. L., Michelsen, A. (2016). Nitrogen transfer from four nitrogen-fixer associations to plants and soils. Ecosystems 19, 1491–1504. doi: 10.1007/s10021-016-0018-7
Rousk, K., Sorensen, P. L., Michelsen, A. (2017). Nitrogen fixation in the high Arctic: A source of ‘new’ nitrogen? Biogeochemistry 136, 213–222. doi: 10.1007/s10533-017-0393-y
Schloss, P. D., Westcott, S. L., Ryabin, T., Hall, J. R., Hartmann, M., Hollister, E. B., et al. (2009). Introducing mothur: Open-source, platform-independent, community-supported software for describing and comparing microbial communities. Appl. Environ. Microbiol. 75, 7537–7541. doi: 10.1128/AEM.01541-09
Solheim, B., Johanson, U., Callaghan, T. V., Lee, J. A., Gwynn-Jones, D., Bjorn, L. O. (2002). The nitrogen fixation potential of arctic cryptogram species is influenced by enhanced UV-beta radiation. Oecologia 133, 90–93. doi: 10.1007/s00442-002-0963-z
Solheim, B., Wiggen, H., Roberg, S., Spaink, H. P. (2004). Associations between arctic cyanobacteria and mosses. Symbiosis 37, 169–187. doi: 10.1007/0-306-48005-0_15
Solheim, B., Zielke, M. (2002). “Associations between cyanobacteria and mosses,” in Cyanobacteria in symbiosis. Eds. Rai, A. N. B., Rasmussen, Ulla, B. (Dordrecht: Springer), 137–152.
Turetsky, M. R. (2003). The role of bryophytes in carbon and nitrogen cycling. Bryologist 106, 395–409. doi: 10.1639/05
Warshan, D. (2017). Cyanobacteria in symbiosis with boreal forest feathermosses (Stockholm, Sweden: Degree of Doctor of Philosophy in Plant Physiology Article, Stockholm University).
Warshan, D., Bay, G., Nahar, N., Wardle, D. A., Nilsson, M.-C., Rasmussen, U. (2016). Seasonal variation in nifH abundance and expression of cyanobacterial communities associated with boreal feather mosses. ISME J. 10, 2198–2208. doi: 10.1038/ismej.2016.17
Xiang, X., Wang, H. M., Gong, L. F., Liu, Q. (2014). Vertical variations and associated ecological function of bacterial communities from sphagnum to underlying sediments in dajiuhu peatland. Sci. China-Earth Sci. 57, 1013–1020. doi: 10.1007/s11430-013-4752-9
Zackrisson, O., DeLuca, T. H., Nilsson, M. C., Sellstedt, A., Berglund, L. M. (2004). Nitrogen fixation increases with successional age in boreal forests. Ecology 85, 3327–3334. doi: 10.1890/04-0461
Keywords: biological N2 fixation, boreal forest, moss-cyanobacteria associations, moss microbiome, NanoSIMS, nitrogen cycling, Pleurozium schreberi
Citation: Arróniz-Crespo M, Bougoure J, Murphy DV, Cutler NA, Souza-Egipsy V, Chaput DL, Jones DL, Ostle N, Wade SC, Clode PL and DeLuca TH (2022) Revealing the transfer pathways of cyanobacterial-fixed N into the boreal forest through the feather-moss microbiome. Front. Plant Sci. 13:1036258. doi: 10.3389/fpls.2022.1036258
Received: 04 September 2022; Accepted: 21 November 2022;
Published: 09 December 2022.
Edited by:
Luciano Kayser Vargas, State Secretariat of Agriculture, Livestock and Irrigation, BrazilReviewed by:
Danillo Oliveira Alvarenga, University of Copenhagen, DenmarkSigne Lett, University of Copenhagen, Denmark
Copyright © 2022 Arróniz-Crespo, Bougoure, Murphy, Cutler, Souza-Egipsy, Chaput, Jones, Ostle, Wade, Clode and DeLuca. This is an open-access article distributed under the terms of the Creative Commons Attribution License (CC BY). The use, distribution or reproduction in other forums is permitted, provided the original author(s) and the copyright owner(s) are credited and that the original publication in this journal is cited, in accordance with accepted academic practice. No use, distribution or reproduction is permitted which does not comply with these terms.
*Correspondence: Davey L. Jones, davey.jones@murdoch.edu.au