- 1School of Pharmaceutical Sciences, South-Central University for Nationalities, Wuhan, China
- 2Institute of Ethnomedicine, South-Central University for Nationalities, Wuhan, China
Entada phaseoloides stem is known for its high medicinal benefits and ornamental value. Flavonoids are one of the main active constituents in E. phaseoloides stem. However, the regulatory mechanism of flavonoids accumulation in E. phaseoloides is lacking. Here, phytochemical compounds and transcripts from stems at different developmental stages in E. phaseoloides were investigated by metabolome and transcriptome analysis. The metabolite profiling of the oldest stem was obviously different from young and older stem tissues. A total of 198 flavonoids were detected, and flavones, flavonols, anthocyanins, isoflavones, and flavanones were the main subclasses. The metabolome data showed that the content of acacetin was significantly higher in the young stem and older stem than the oldest stem. Rutin and myricitrin showed significantly higher levels in the oldest stem. A total of 143 MYBs and 143 bHLHs were identified and classified in the RNA-seq data. Meanwhile, 34 flavonoid biosynthesis structural genes were identified. Based on the expression pattern of structural genes involved in flavonoid biosynthesis, it indicated that flavonol, anthocyanin, and proanthocyanin biosynthesis were first active during the development of E. phaseoloides stem, and the anthocyanin or proanthocyanin biosynthesis branch was dominant; the flavone biosynthesis branch was active at the late developmental stage of the stem. Through the correlation analysis of transcriptome and metabolome data, the potential candidate genes related to regulating flavonoid synthesis and transport were identified. Among them, the MYBs, bHLH, and TTG1 are coregulated biosynthesis of flavonols and structural genes, bHLH and transporter genes are coregulated biosynthesis of anthocyanins. In addition, the WDR gene TTG1-like (AN11) may regulate dihydrochalcones and flavonol biosynthesis in specific combinations with IIIb bHLH and R2R3-MYB proteins. Furthermore, the transport gene protein TRANSPARENT TESTA 12-like gene is positively regulated the accumulation of rutin, and the homolog of ABC transporter B family member gene is positively correlated with the content of flavone acacetin. This study offered candidate genes involved in flavonoid biosynthesis, information of flavonoid composition and characteristics of flavonoids accumulation, improved our understanding of the MYBs and bHLHs-related regulation networks of flavonoid biosynthesis in E. phaseoloides stem, and provided references for the metabolic engineering of flavonoid biosynthesis in E. phaseoloides stem.
Introduction
Entada phaseoloides (L.) is a medical and ornamental legume that belongs to the genus Entada and grows in the southern of China, other tropical, and sub-tropical regions worldwide. E. phaseoloides is notable for its large woody vines (stems) and seed pods. E. phaseoloides is a perennial, grows in a hollow of the hills or hillside mixed forest, and usually climbs trees using it as support. The genus Entada includes approximately thirty species, belonging to the subfamily Mimosoideae (Ohashi et al., 2010). Entada species are rich in saponins and flavonoids (Dong et al., 2012; Roheem et al., 2019; Xiong et al., 2021). The stems of Entada species have been widely used as a traditional medicine owing to its pharmacological activities, such as antioxidant activity (Diallo et al., 2001; Njayou et al., 2013; Njayou et al., 2015; Roheem et al., 2019), anti-microbial activity (Teke et al., 2011; Wan et al., 2020), and anti-inflammatory activity (Ayissi et al., 2013; Xiong et al., 2021). The ethyl acetate extracts from the stems of E. phaseoloides showed significant antioxidant activity by radical scavenging activity, β-carotene bleaching, reducing power, and superoxide radical scavenging effects in vitro experiments, and most of the compounds isolated from ethyl acetate fraction belonged to flavonoids and displayed antioxidant ability by above-mentioned tests (Teke et al., 2011; Zhao et al., 2011; Dong et al., 2012). Thus, flavonoids are considered as one of the important bio-activities in the stems of E. phaseoloides. However, the biosynthetic pathway of flavonoids has not been reported in Entada species.
Flavonoids are one of the most important polyphenolic compounds, consisting of over 8,000 metabolites widely distributed in plants (Wen et al., 2020). Flavonoids have been reported to have various pharmaceutical activities. They often act as antioxidants due to their effectively scavenging free radical ability (Bors et al., 1990; Grigalius and Petrikaite, 2017), in the rat brain mitochondria in vitro experiment showed that flavonoids inhibited the non-enzymatic lipid peroxidation (Ratty and Das, 1998). Flavonoids also exhibited anti-cancer (Kopustinskiene et al., 2020), anti-inflammation (Mower et al., 1984), antimicrobial activity (Cushnie and Lamb, 2005), neuroprotective activities (Spagnuolo et al., 2018), and antidiabetic effects (Sarkhail et al., 2007; Bansal et al., 2012; Yang et al., 2015) in many in vitro and animal models. In addition, flavonoids also possess multiple ecological roles, including regulation of plant–microbe and plant–plant interactions (Leoni et al., 2021), facilitating pollination (Saigo et al., 2020), improving resistance to biotic and abiotic stresses (Casas et al., 2016; Barcelo et al., 2017; Tohge et al., 2018) and UV-light protection (Tohge et al., 2016; Peng et al., 2017). Thus, flavonoids are widely used for the pharmaceutical, cosmetic, and food industries and have gained increasing attention (Soto-Vaca et al., 2012; Grigalius and Petrikaite, 2017; Khan et al., 2021).
Flavonoids are characterized by a common diphenylpropane (C6-C3-C6) backbone in which two aromatic rings are connected by a three-carbon chain (Stobiecki and Kachlicki, 2006; Wen et al., 2020). Based on the oxidation pattern of the heterocyclic C ring, flavonoids are divided into several categories, including flavones, flavanones, flavonols, flavanols, isoflavones, and anthocyanidins (Wen et al., 2020). The flavonoid synthesis pathway begins with the catalytic action of phenylalanine ammonia-lyase (PAL) on the precursor phenylalanine and then trans-cinnamate 4-monooxygenase (C4H), leading to the production of chalcone (Koukol and Conn, 1961; Russell and Conn, 1967; Ververidis et al., 2007; Jia et al., 2008; Hassani et al., 2020). The first committed step of flavonoid synthesis is mediated through chalcone synthase (CHS), which catalyzes malonyl-CoA and p-coumaroyl-CoA to generate naringenin chalcone (Kreuzaler and Hahlbrock, 1975; Kreuzaler et al., 1983). Then, naringenin chalcone undergoes isomerization to produce flavanone naringenin through chalcone isomerase (CHI) (Moustafa and Wong, 1967; Jez et al., 2000). Next, the flavanones act as substrates for a series of other enzymes, giving rise to different subclasses of flavonoids, such as flavanones, dihydroflavonols, and anthocyanins; these enzymes include flavonoid 3-hydroxylase (F3H), flavonoid 3′-hydroxylase (F3′H), flavonoid 3′5′-hydroxylase (F3′5′H), flavanone 3b-hydroxylase (FHT), flavonol synthase (FLS), dihydroflavonol 4-reductase (DFR), anthocyanidin synthase (ANS), and anthocyanin reductase (ANR) (Holton and Cornish, 1995; Winkel, 2006; Gebhardt et al., 2007; Tohge et al., 2017; Jun et al., 2018). Heterologously overexpression of Ginkgo biloba L. GbF3′5′H1 can increase the contents of 4′,5-dihydroxy-7-glucosyloxyflavanon, epicatechin, and gallocatechin in transgenic Populus (Wu et al., 2020). High expression of the CHS gene enhances the accumulation of flavonoids in the leaves of Perilla frutescens based on the metabolomic and transcriptomic data (Jiang et al., 2020). Moreover, some transcription factors (TFs) have been proved to participate in regulating flavonoid synthesis. Overexpression of Poplar MYB117 promotes anthocyanin synthesis in all tissues and enhances flavonoid B-ring hydroxylation by upregulating the F3′5′H gene (Ma et al., 2021). Overexpressed FtMYB31 increases the rutin content of Fagopyrum tataricum Gaertn (Hou et al., 2021). Wang et al. (2018) prove that overexpression of MdWRKY11 could promote the expression of F3H, FLS, DFR, ANS, and UFGT, thereby increasing the accumulation of flavonoids and anthocyanin in apple calli. In Petunia hybrida, an R2R3-MYB TF AN4 promotes anthocyanin biosynthesis by enhancing the expression of anthocyanin biosynthesis genes, such as CHS, CHI, F3H, and DFR (Zhang et al., 2021). Cucumis sativus CsMYB60 also can promote flavonol and proanthocyanidin biosynthesis by inducing the expression of CsFLS and CsLAR (Li et al., 2020). Among the TFs, R2R3-MYB, WD-repeat proteins (WDR), and basic helix-loop-helix proteins (bHLH) have been received more attention (Koes et al., 2005; Mehrtens et al., 2005; Jaakola, 2013; Xu et al., 2015; Karppinen et al., 2021).
In this study, untargeted metabolite analysis was performed to determine global metabolic profiles and analyze the characteristic of flavonoid accumulation in three developmental stages of stems from E. phaseoloides. Global gene expression profiling was explored during the development of the stem, and the structural genes of flavonoid biosynthesis and some TFs were identified and classified by transcriptome data. Meanwhile, the coexpression networks and candidate genes regulating the biosynthesis of flavonoids in E. phaseoloides were revealed by an integrated analysis of metabolome and transcriptome. This study not only clarified the accumulation of flavonoids in E. phaseoloides stem at different development stages but improved the current understanding of the MYBs and bHLHs-related molecular network of flavonoid synthesis in E. phaseoloides and provided gene sequences information of flavonoid biosynthesis and research clues for the future studies in other medicinal plants and legume plants.
Materials and Methods
Plant Materials
Entada phaseoloides stem tissue used for this study was obtained from the mixed forest of Botanical Garden of Xishuangbanna South Medicine at an altitude of about 553 m, Xishuangbanna, Yunnan Province, China. The Mucuna sempervirens Hemsl is next to E. Phaseoloides. The stems were collected at 15, 45, and 75 days at fruit developmental stages, corresponding to the stem_young (S1), stem_older (S2), and stem_oldest (S3), respectively. The temperature on the day of harvest was 27°C. Samples were collected on ice and frozen immediately in liquid nitrogen and stored at −80°C until further use. Additionally, all samples were divided into two parts for metabolites determination and extraction of RNA for transcriptome sequencing. To ensure the consistency of subsequent correlation analysis, the metabolites and transcripts were assessed from the same samples.
Extraction and Separation of Metabolites
Each sample had six independent biological replicates. A total of 18 samples were collected as metabolite studies. Metabolite extraction for non-targeted metabolite profiling was conducted as previously described (Tohge and Fernie, 2010). The tissues (25 mg) were weighed and put into a 1.5-ml EP tube. A total of 800 μl pre-cooled precipitant (methanol: acetonitrile: water = 2:2:1) were added into the tube. The tissues were crushed with steel beads using a grinder for 4 min at 60 Hz. After ultrasonic extraction for 10 min (power 80 HZ) in an ice bath, the mixture was frozen for 120 min and centrifugated at 25,000 g for 15 min at 4°C. The supernatants were collected and repeated one time and then dried by a freeze dryer. Redissovle by adding 600 μl of 10% methanol solution, sonicate for 10 min (power 80 Hz) in an ice bath, and centrifuge for 15 min at 4°C to take the supernatant; about 50 μl of each sample was taken and mixed into a QC sample.
High-resolution mass spectrometry (MS) was performed on a Xevo G2-XS QTOF mass spectrometer (Waters, United Kingdom) following chromatographic separation on a Waters 2777C ultra-performance liquid chromatography (UPLC) system (Waters, United Kingdom) using a Waters ACQUITY UPLC HSS T3 column (100 mm * 2.1 mm, 1.8 μm, Waters, United Kingdom), with column temperature control at 50°C. The elution gradient at 0.4 ml/min using solvent A (water + 0.1% formic acid) and solvent B (acetonitrile + 0.1% formic acid) was applied/set as follows: 0–2 min with 100% phase (A), followed by a 2–11 min gradient from 0 to 100% (B), 11–13 min with 100% (B), 13–15 min gradient from 0 to 100% (A), the injection volume for each sample was 5 μl. The QTOF mass spectrometer was operated in both positive and negative ion modes. The MS data were acquired in Centroid MSE mode. The TOF mass range was from 50 to 1,200 Da, and the scan time was 0.2 s. For the MS/MS detection, all the precursors were fragmented using 20–40 eV, and the scan time was 0.2 s.
Pre-identification and Analysis of Metabolites
Mass spectrometry raw data were preprocessed and analyze using Progenesis QI (v2.2, Waters, Milford, MA, United States) and metaX (Wen et al., 2017). The ions meeting a relative standard deviation (RSD) ≤ 30% threshold were selected for downstream statistical analysis. Metabolites were identified by a comparison of the measured molecular mass-to-charge ratio (m/z) or molecular weight with information available in the KEGG database. The information of adducts and isotopes is utilized to assist in metabolite identification if it is present. The mass deviation was 10 ppm. The pre-identifications are Level II following the standardization of metabolomics data after the International Metabolomics Society. Rutin, myricitrin, and acacetin were verified based on the authentic standard. The related information (MS/MS spectrum) was added to Supplementary File 1.
The variable importance in projection (VIP) values of the first two principal components in the multivariate PLS-DA model, combined with fold-change (FC) and q-value of univariate analysis, were used to choose differential metabolites. The filtering rules are VIP ≥ 1; fold-change ≥ 1.2 or ≤0.8333; and q-value < 0.05. Metabolic pathway analysis is based on the KEGG database.
Transcriptome Sequencing (RNA-Seq) and Analysis
The stem samples of three developmental stages used for RNA-seq were the same as metabolome analysis samples. Each sample had three independent biological replicates. The total RNA and mRNA from E. phaseoloides stems were obtained as previously described (Fu et al., 2021). The RNA-seq libraries were constructed according to Illumina instruction and then sequenced using an Illumina HiSeq X Ten platform. Low-quality raw read sequences were removed using SOAPnuke with default parameters (-l 15 -q 0.2 -n 0.05) (v1.5.21). The clean reads were mapped to E. phaseoloides reference genome sequence using HISAT with default parameters (--phred64 --sensitive --no-discordant --no-mixed -I 1 -X 1000) (v2-2.0.42) and then assembled by StringTie with default parameters (-f 0.3 -j 3 -c 5 -g 100 -s 10000 -p 8) (v1.3.3b3). The fragments per kilobase per million mapped fragments (FPKM) values and transcripts per million (TPM) values were then calculated for each gene in all samples. Differentially expressed genes (DEGs) were identified by DESeq2 (v1.34.0, fold-change ≥ 2 and adjusted p-value ≤ 0.05) (Anders and Huber, 2010; Love et al., 2014) and annotated to Nr, GO, and KEGG database.
Identification of Enzyme-Coding Genes and Transcription Factors
To identify the candidate structure genes involved in the biosynthesis of flavonoids, the genes that are annotated to these pathways (ko00940, ko00941, ko00942, ko00943, and ko00944) using KEGG (E-value ≤ 1e--10) were retrieved. Subsequently, the identified candidate genes involved in flavonoid biosynthesis were confirmed using the knowledge-based identification of pathway enzymes (KIPEs4) (Pucker et al., 2020a).
The identification and classification of MYB and bHLH were based on the signature domain and phylogenetic tree as described in the previous studies (Pucker, 2021; Song et al., 2021). The MYB domain sequences of published multiple plant species were merged to generate a bait sequence collection. MYB candidates are checked for conserved domains and assigned to orthologs in other plant species (Pucker, 2021).
Protein sequences of Arabidopsis from the TAIR database were established as references. The pipeline for automatic identification and annotation in a bait sequence data set was used to get E. phaseoloides MYB candidates. Non-redundant MYB genes were obtained by mapping identified gene models of the previous step to unique loci in the genome. For the bHLH, the most conserved sequence in the bHLH region (Toledo-Ortiz et al., 2003) was used for tBLASTn searches. Protein sequences of Arabidopsis from the TAIR database were established as references. The intact bHLH domain was analyzed by the HMMER (v3.0). Other steps were similar to MYB processing. Multiple sequence alignments of the predicted genes and Arabidopsis were performed using muscle (v3.8.31) with default parameters. Phylogenetic trees were constructed by FastTree (v2.1) using the maximum likelihood (ML) method. E. phaseoloides genes homologs were classified according to their relationships with corresponding Arabidopsis. The resulting genes were named following the position of the chromosome.
Integrated Analysis Between Metabolite Profiling and Transcriptome
To identify the potential key genes and networks involved in flavonoid biosynthesis in E. phaseoloides, correlation analysis was performed by calculating the Pearson correlation coefficient (PCC) values in the R software between each set of variables (DEGs and differentially accumulated metabolites) across the profiles. The | PCC| ≥ 0.80 and p-value ≤ 0.05 between DEGs with metabolites were selected as significant correlations. The correlation network was visualized by Cytoscape software (v 3.6.1). The sequences of genes involved in the network are presented in the Supplementary Files 2, 3.
Results
Metabolite Profiling of Stem Samples
Untargeted metabolite analysis was performed to determine the global metabolic profiles of E. phaseoloides stem tissues. Among all the detected phytochemical compounds, terpenoids, flavonoids, and alkaloids are the main metabolites (Supplementary Figure 1). Partial least squares discriminant analysis (PLS-DA) was conducted to obtain an overview of metabolic changes and plot comparison of differences and similarities. The PLS-DA of the metabolites showed a clear separation in the first principal component for the composition of stems metabolites in both positive and negative modes, explaining 32.06 and 35.63% of the variation in the data, respectively; the metabolite profiling of stem_oldest (S3) was obviously different from stem_young (S1) and stem_older (S2) tissues (Supplementary Figure 2). Flavonoids are one of the main important active ingredients in E. phaseoloides stems (Teke et al., 2011; Dong et al., 2012). A total of 198 putative flavonoids were detected, and flavones (31), flavonols (25), anthocyanins (19), isoflavones (18), flavanones (12), pterocarpans (12), biflavonoids, and polyflavonoids (11), chalcones (7), proanthocyanidins (8), and dihydrochalcones (7) were the top 10 most abundant classes (Figure 1 and Supplementary Table 1). This is the first time that so many types of flavonoid compounds were isolated in E. phaseoloides, including myricitrin and acacetin. Combing VIP in the PLS-DA model variable, FC, and q-value, 366 differentially accumulated metabolites (DAMs) were identified among all the compared samples. KEGG pathway enrichment analysis indicated that these DAMs mainly enriched in pathways of flavonoid biosynthesis, flavone and flavonol biosynthesis, anthocyanin biosynthesis, and isoflavonoid biosynthesis (Supplementary Figure 3). Taken together, the flavonoids were analyzed in detail in this study.
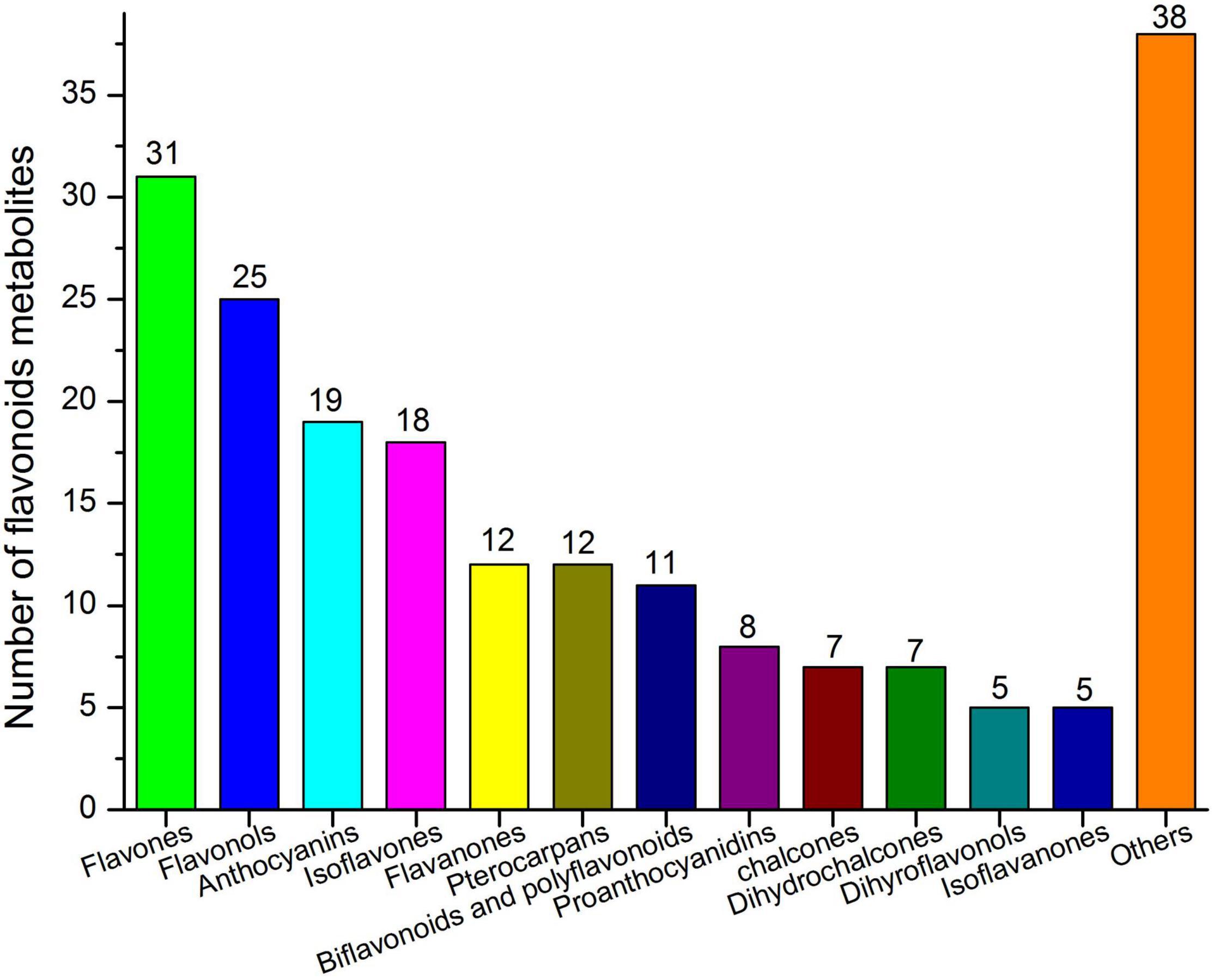
Figure 1. Classification and statistical analysis of all detected flavonoids in all samples. The x-axis represents the different categories, and the y-axis represents the number of each category.
Flavonoids Differences Among Different Stem Samples
A total of 97 differentially accumulated flavonoids (DAFs) were detected among all comparison groups. Flavones (17), flavonols (17), and anthocyanins (10) are the three major categories in DAFs (Supplementary Table 2). In detail, there were 0, 72, and 84 significantly DAFs, respectively, in the 3 comparison groups, including S1 versus S2, S2 versus S3, and S1 versus S3; there were 61 DAFs changed in common between S2 versus S3 and S1 versus S3. Pairwise comparison of three groups revealed that the difference of content of flavonoids between the S1 and S2 was small, whereas large between S2 versus S3 and S1 versus S3 (Supplementary Table 2). Based on the VIP values of the PLS-DA, the 20 dominant components responsible for the separation among different stems in DAFs were identified and shown in Figure 2. In the comparison between S2 and S3, these 20 flavonoids included five flavones, three flavonols, two isoflavones, two dihyroflavonols, one anthocyanin, one biflavonoid, and polyflavonoid, one flavan 3-ol, one flavan, one flavonoid glycoside, one isoflavan, one o-methylated flavonoid, and one pterocarpan (Figure 2A and Supplementary Table 3); whereas for the S1 and S3 comparison groups, there were five flavonols, four flavones, three anthocyanins, two isoflavones, one chalcone, one 3-arylcoumarin, one biflavonoid and polyflavonoid, one flavan 3-ol, one flavan, and one O-methylated flavonoid (Figure 2B and Supplementary Table 3). Among them, rutin, myricitrin, 6-methoxyluteolin 7-rhamnoside, 3-methoxyapigenin, cinnamtannin A1, and (-)-epiafzelechin showed significantly higher levels in S3 than both S1 and S2, whereas the content of acacetin, pinobanksin 3-O-acetate, 8-hydroxykaempferol, phellamurin, quercitrin, pelargonidin 3-O-(6-caffeoyl-beta-D-glucoside), desmethylxanthohumol, dasytrichone, delphinidin 3-(6-p-coumaroyl) glucoside, and eriodictyol chalcone was significantly lower in S3 then both S1 and S2 (Supplementary Table 3).
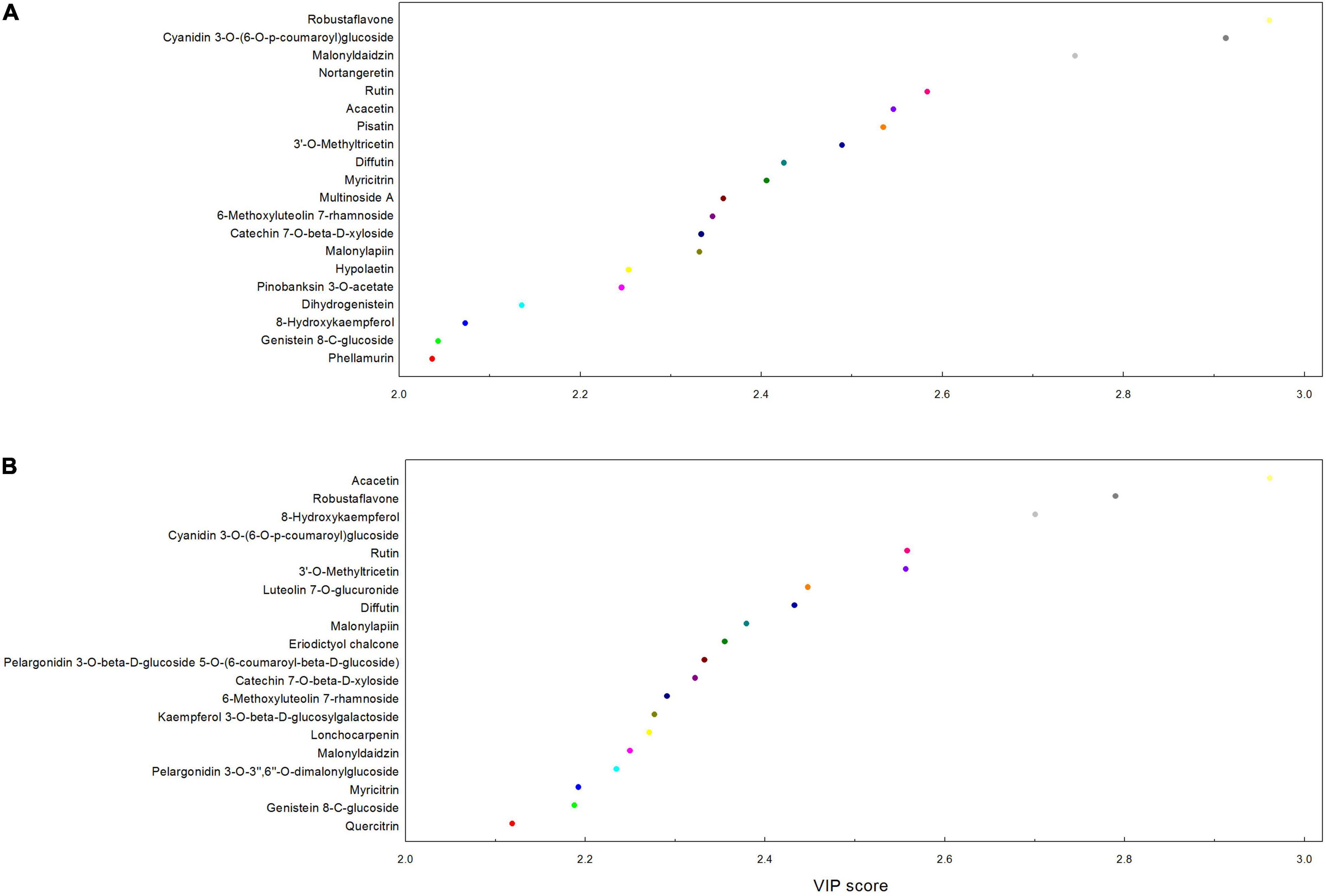
Figure 2. Variable importance in projection (VIP) score of the top 20 flavonoids in each compared group. Results were from the PLS-DA. (A) S2 versus S3 and (B) S1 versus S3. The VIP scores displayed the relative contribution of these flavonoids to the separation among different stems. The higher the VIP score, the larger the contributions. Stem_young, stem_older, and stem_oldest were renamed as S1, S2, and S3, respectively.
Transcriptome Analysis of Stem Samples
To gain the global gene expression in three different types of stem samples, a total of 57.85 Gb of clean data were obtained, and the clean reads ranged from 42.07 to 43.44 Mb in each library, with an average of 96.85% of bases scoring Q30. All the clean reads were mapped to the reference genome sequence with mapping ratios in the range of 86.23–91.81% (Supplementary Table 4). These data demonstrated that the transcriptome sequencing was high quality and could be used for further analysis. Totally, 24,240 genes were detected for further analysis, and their gene expression levels were estimated by FPKM and TPM values. Among these genes, 21,462 were expressed in all three different stems (S1, S2, and S3), and 316, 456, and 581 specific expressed genes were found in S1, S2, and S3, respectively (Supplementary Figure 4).
A total of 143 MYBs and143 bHLHs were identified in E. phaseoloides (Supplementary Table 5). To unravel the evolutionary relationship of these genes, the phylogenetic trees were constructed. The Arabidopsis MYB family (131 members, including 124 R2R3-MYBs, five MYB3R, one CDC5, and one MYB4R) and bHLH family (116 members) were used, respectively. A set of 120 R2R3-MYBs proteins, 13 R1-MYB proteins, 8 R1R2R3-MYB (MYB3R) proteins, and 2 unclassified MYB proteins (pseudo) were identified (Supplementary Table 5 and Figure 3). R2R3-MYBs account for the largest subfamily size in E. phaseoloides. E. phaseoloides bHLHs were classified into 23 subfamilies, including subgroup Ia, XII, IVa, Ib, VIIIb+c, IIId+e, Vb, III b, VIIa+b, IX, XIII, IIIc, IVb, IVc, VIIIa, XI, II, IIIf, VI, IIIa, IVd, Va, and X based on Arabidopsis bHLH subgroups. Among them, Ia (16 members), XII (16 members), IVa (13 members), Ib (12 members), and VIIIb+c (14 members) were the largest top five subgroups of EpbHLHs, while subgroups IIIa, IVd, Va, and X were the smallest, each with only one member (Supplementary Table 5 and Figure 4). The number of subgroup XII was similar to Arabidopsis and Ficus carica L., but the number of subgroup X was significantly less than these two species.
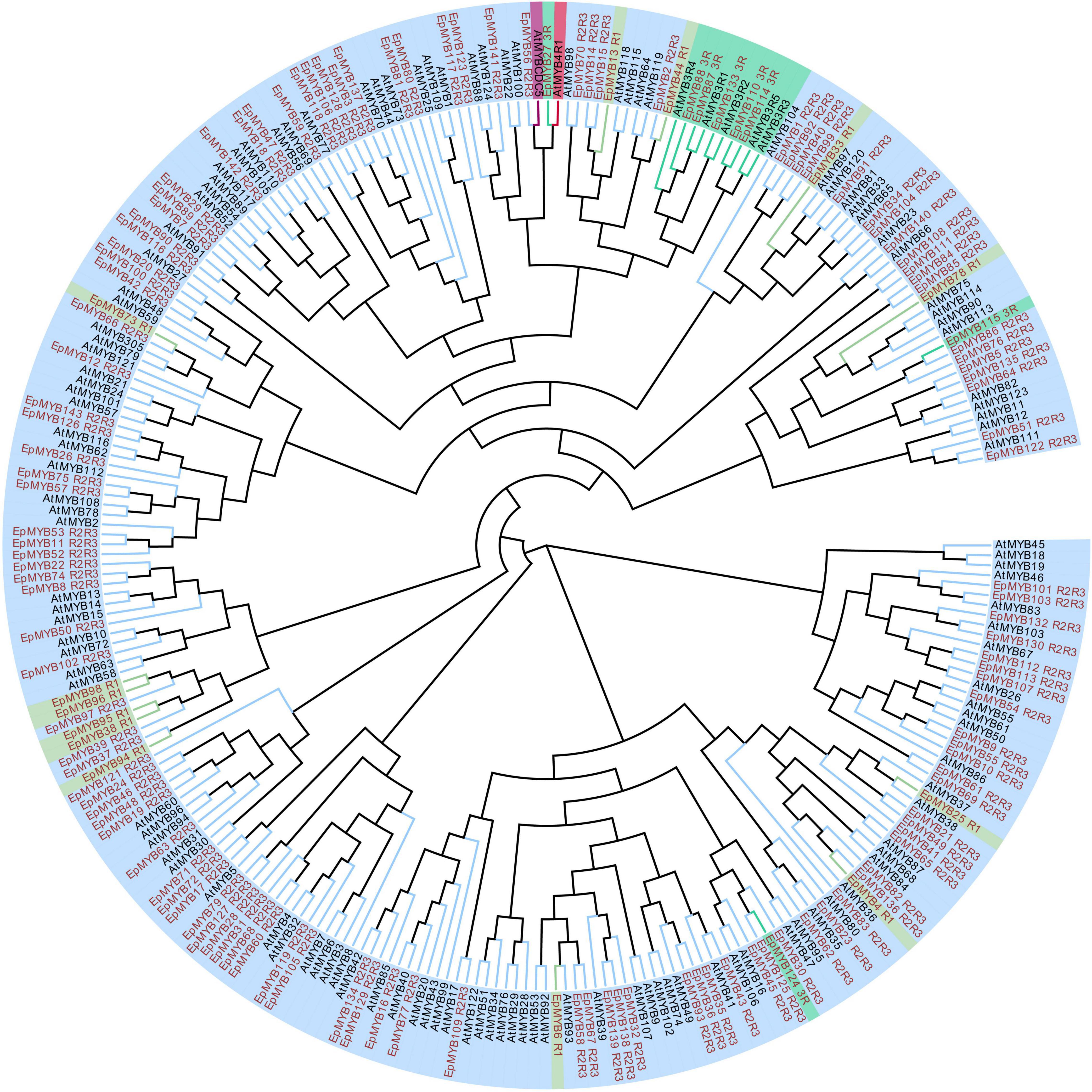
Figure 3. Phylogenetic tree of EpMYBs. The EpMYBs are marked in red, AtMYBs are marked in black. The subgroups are marked in different colors on the periphery of the circle.
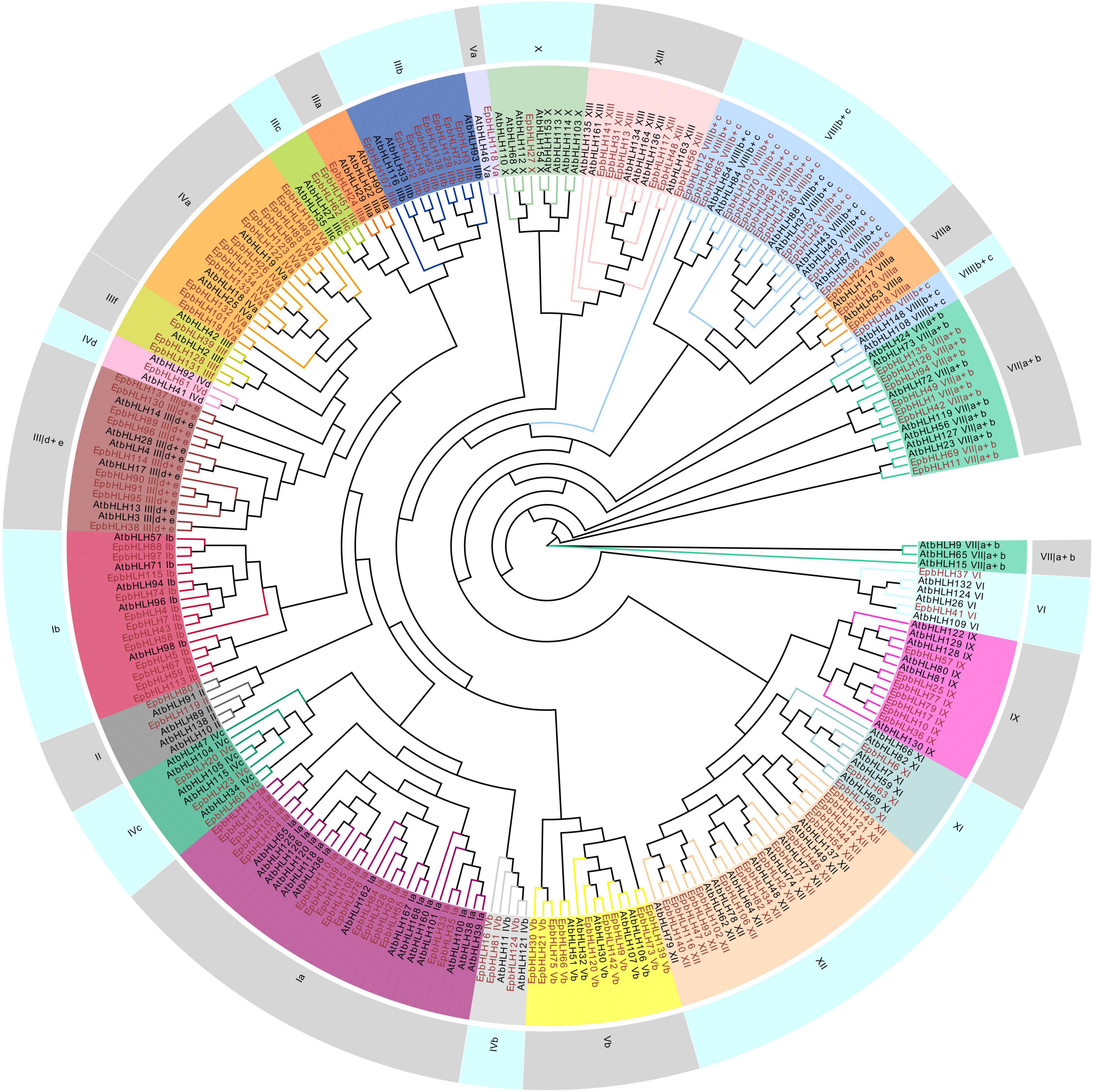
Figure 4. Phylogenetic tree of EpbHLHs. The EpbHLHs are marked in red, AtbHLHs are marked in black. The subgroups are marked in different colors on the periphery of the circle.
Differential Gene Expression Analysis
Based on the fold-change of gene expression and a false discovery rate (FDR), a total of 1,183 (908 up- and 275 downregulated), 3,289 (1,150 up- and 2,139 downregulated), and 3,112 (1,509 up- and 1,603 downregulated) DEGs were found in the S1 versus S2, S2 versus S3, and S1 versus S3, respectively (Figure 5 and Supplementary Table 6). The results demonstrated that the expression profile of S1 and S2 is closer, whereas the gene expressions between S2 and S3, S1, and S3 were obviously different, which is similar to the results obtained based on the metabolome analysis. This implied that the DAMs in these samples have certain correlations with regulation by DEGs.
To explore the potential biological significance of these DEGs, GO, and KEGG function annotation and enrichment analysis were performed. GO functional classification analysis showed that all the DEGs could be grouped into 48 functional groups, including 23 biological process, 15 cellular component, and 10 molecular function categories (Supplementary Table 7). Within the biological processes, cellular process, metabolic process, and biological regulation were the most enriched terms, in the cellular component category, the top three represented terms were membrane, membrane part, and cell, and under the molecular function category, the most abundant terms were catalytic activity, binding, and transporter activity. To further illustrate the alterations of metabolic pathways during stem development, the KEGG analysis of all the DEGs was conducted. A total of 976 (S1 versus S2), 2,665 (S2 versus S3), and 2,554 (S1 versus S3) DEGs were grouped to 117, 130, and 133 KEGG pathways, respectively, and five pathways that are related to the biosynthesis of flavonoids were enriched in all comparison groups, including phenylpropanoid biosynthesis, flavonoid biosynthesis, flavone and flavonol biosynthesis, anthocyanin biosynthesis, and isoflavonoid biosynthesis (Supplementary Table 8). KEGG significantly enrichment analysis showed a marked difference. Starch and sucrose metabolism and flavonoid biosynthesis were significantly enriched in the S1 versus S2 comparison group, and the number of upregulated DEGs were significantly more than downregulated DEGs in these pathways. Starch and sucrose metabolism, plant hormone signal transduction, phenylpropanoid biosynthesis, other glycan degradation, and MAPK signaling pathway were significantly enriched in the comparison group of S2 versus S3. Other glycan degradation, flavone and flavonol biosynthesis, and MAPK signaling pathway were significantly enriched in the S1 versus S3 comparison group (Supplementary Table 8). The genes involved in these significantly enriched flavonoid biosynthesis-related pathways may contribute to the changes in flavonoid content. It further illustrated a close relationship between flavonoids and DEGs.
Patterns of Expression of Flavonoid Biosynthetic Structural Genes
To further explore the molecular mechanism of accumulating flavonoids in E. phaseoloides stems, the genes related to flavonoids biosynthetic pathways were identified, and then the expression patterns of these genes were analyzed. A total of 34 structural genes of flavonoid biosynthesis were identified based on KEGG pathway annotations and KIPEs in this work, including PAL (3), C4H (3), 4CL (2), CHS (5), CHII (1), CHIIII (1), F3H (1), F3′H (4), F3′5′H (1), FHT (1), FNSII (1), FLS (3), DFR (2), ANS (2), LAR (2), and ANR (2) (Table 1). In addition, functionally relevant amino acid residues of most identified genes showed highly conservative, and no F3′5′H, FHT, ANR, and LAR candidates with conservation of all functionally relevant amino acids were detected (Supplementary Table 9). Most candidates showed high transcriptional activity at least in one stem developmental stage in our transcriptome data had a high gene expression level (TPM > 10). However, there were some isoforms of candidates that displayed very low transcript abundance at all the stages. It indicated that other isoforms may perform functions during the process of flavonoid biosynthesis in E. phaseoloides stems (Table 1).
Among them, there were 20 structural genes differentially expressed, including three C4H genes, one 4CL gene, four CHS genes, one CHII gene, one F3H, one F3′5′H, one FHT, one FNSII, one DFR, two LAR genes, two ANS gene, and two ANR gene (Supplementary Table 10 and Figure 6). Among these genes, two C4H (C4H_1 and C4H_3) genes, two CHS genes (CHS_2 and CHS_3), one CHII gene, one F3H gene, one F3′5′H gene, one DFR (DFR_1) gene, two ANS gene, two ANR gene, and one LAR (LAR_2) were obviously upregulated in S1 versus S2, and one CHS (CHS_4) was remarkably downregulated during all the stem developmental stage. However, in the comparison group of S2 and S3, most of the differentially expressed structural genes were significantly downregulated, including one 4CL (4CL_1), two C4H (C4H_2 and C4H_3), two CHS genes (CHS_3 and CHS_4), one CHII, one F3H, one F3′5′H, one DFR (DFR_1), two ANS, and two ANR. Only one FHT and two LAR were upregulated in S1 versus S3. FNSII gene was significantly upregulated during the late stem developmental stages. Most of the differentially expressed structural genes were significantly downregulated from S2 to S3 stage (Supplementary Table 10).
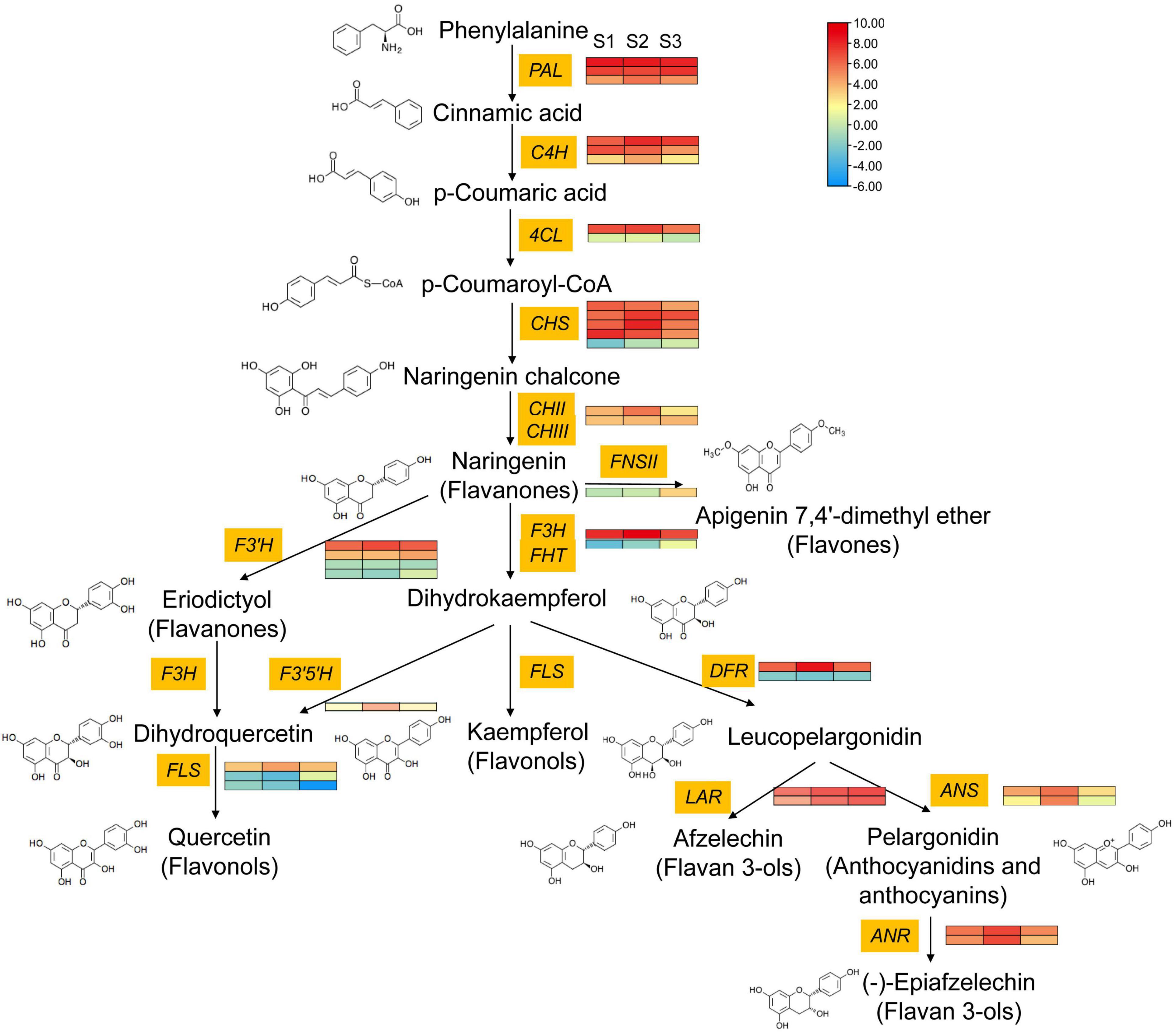
Figure 6. Comparative transcriptomic analysis of structural genes involved in the flavonoid biosynthesis pathway at different stems. The three columns for each gene represent the expression level at S1, S2, and S3 stage, respectively. Red represents the high expression level; blue represents the low expression level. Stem_young, stem_older, and stem_oldest were re-named as S1, S2, and S3, respectively.
Correlation Analysis Between Transcripts and Flavonoids
Many studies have already been performed to investigate the synthesis of flavonoids, and many structural and regulatory genes were identified in other plants (Falcone Ferreyra et al., 2012; Liu et al., 2020); it is still not clear which genes control the production of which flavonoids in E. phaseoloides. To reveal the regulatory network and candidate genes of flavonoid biosynthesis in E. phaseoloides, correlation analysis between the DAFs content and the expression of DEGs was performed. All detected correlations between DAFs and DEGs are shown in Supplementary Table 11. There were 34 flavonoids that showed higher correlation coefficient values (r > 0.8, p-value < 0.05) with 17 differentially expressed flavonoid biosynthesis structural genes (Supplementary Table 12), and their interaction networks are organized in Figure 7A. The 17 structural genes contained one 4CL, three C4H, three CHS, one CHII, one F3H, one F3’5’H, one FHT, one FNSII, one DFR, one DFR, two ANS, and one ANR, which are the major structural genes in the flavonoid biosynthesis pathway. The 34 flavonoids include seven flavones, six anthocyanins, four biflavonoids and polyflavonoids, three flavanones, three pterocarpans, two chalcones, two flavonols, one dihyroflavonol, one flavan 3,4-diol, one dihydrochalcone, one isoflavan, one isoflavone, one flavonoid glycoside, and one proanthocyanidin (Supplementary Table 12).
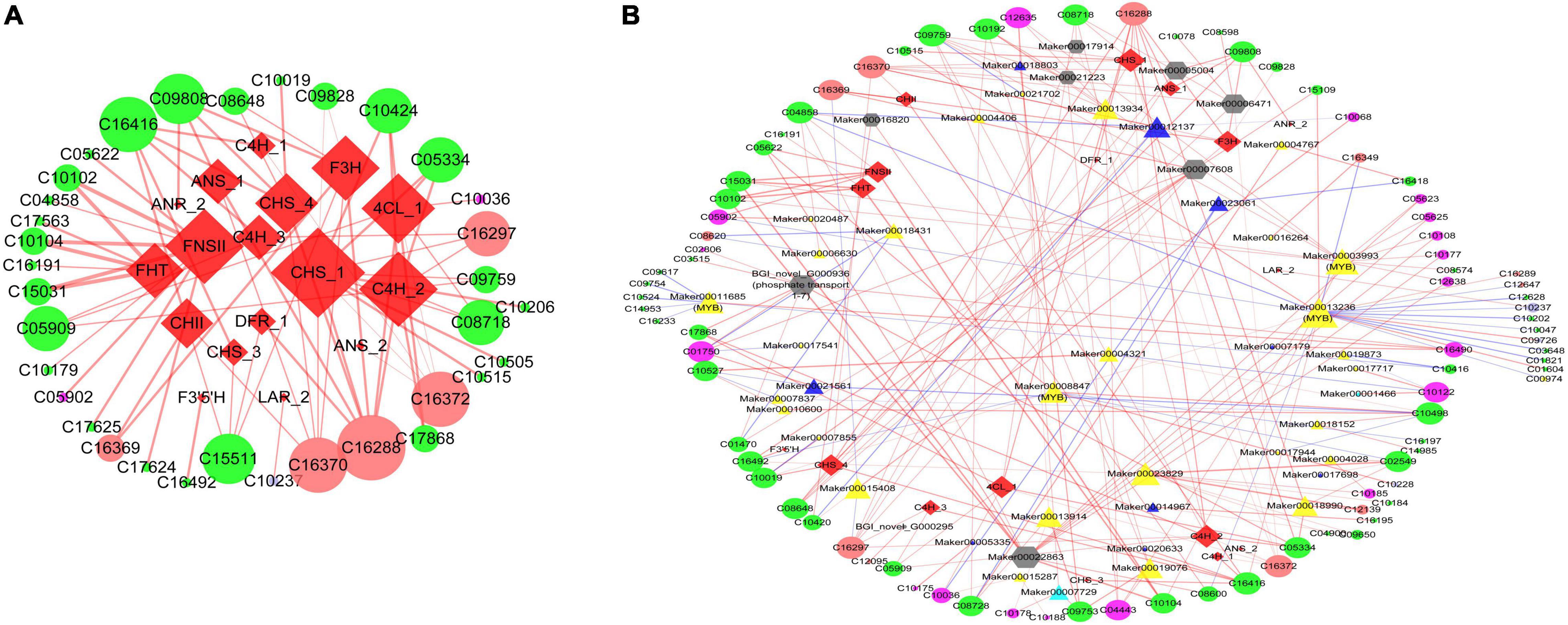
Figure 7. Connection network between flavonoid biosynthesis related genes and flavonoids. (A) Connection network between flavonoid biosynthesis structural genes and flavonoids; (B) connection network between flavonoids and MYBs, bHLHs, WDRs, transport genes, and structural genes. The magenta circle represents flavonols; the Indian red circle represents anthocyanins; the gray violet circle represents proanthocyanidins; the green circle represents other flavonoids; the red diamond represents the structural genes; the triangle represents TFs, the yellow triangle represents MYB families, the blue triangle represents bHLH families, the Caribbean green triangle represents WDR families; the gray hexagon represents transport genes. The size of the shapes in the graph shows the number of connections of the input genes and flavonoids in the network. The red line indicates a positive correlation, and the blue line indicates a negative correlation.
The previous research showed that many MBW complexes are involved in regulating flavonoid synthesis (Ramsay and Glover, 2005; Xu et al., 2015; Xu et al., 2021). In this study, the expression of 47 genes encoding MYB, bHLH, and TRANSPARENT TESTA GLABRA 1 (TTG1) homologs was highly correlated with the amount of 81 flavonoids, including 32 MYBs, 13 bHLHs, and 2 TTG1 homologs (Supplementary Table 13). Furthermore, the expression level of 86 transporters was also showed highly associated with quantitative changes of 76 flavonoids (Supplementary Table 14). The solute carrier family, glutathione S-transferase, ABC transporter B family, and multidrug resistance protein were the most members in the 86 genes. It was in accordance with the genes involved in flavonoid transport observed in pepper fruit (Liu et al., 2020). The previous studies found that H+-ATPase are associated with flavonoid uptake efficiency in the vacuoles and cytoplasm (Kitamura, 2006). Mutations of P-type H+-ATPase (AHA10) lead to vacuolar morphology defects and reduced proanthocyanidin content in Arabidopsis seeds (Baxter et al., 2005). Maker00021223 and Maker00005004, a member of the H+-transporting ATPase, are closely positively related to one flavonol (3-O-methylquercetin) and one flavone (tricetin), two anthocyanidins (pelargonidin 3-O-[6-caffeoyl-beta-D-glucoside] and delphinidin 3-[6-p-coumaroyl] glucoside), one chalcone (isobavachalcone), one dihydrochalcone (isouvaretin), and one pterocarpan (glyceollin III), respectively.
In addition, the phosphate transporters 1–7 (BGI_novel_G000936 and BGI_novel_G000295) were significantly positively related to one flavanone (isosakuranetin), three flavanones (acacetin, luteolin 7-O-glucuronide, and morusinol), three flavonols (quercitrin, 8-hydroxykaempferol, and kaempferol 3-O-beta-D-glucosylgalactoside), two flavones (acacetin and morusinol), and one anthocyanidin (cyanidin 3-O-[6-O-p-coumaroyl] glucoside), respectively. The TRANSPARENT TESTA 12-like gene (Maker00022863) is obviously positively associated with five flavones (nortangeretin, 6-methoxyluteolin 7-rhamnoside, lucenin-2, malonylapiin, and apiin), four flavonols (quercetin 3-O-glucoside, robinetin, rutin, quercetin 3-O-[6-O-malonyl-beta-D-glucoside], and myricitrin), and one flavanone (silandrin). The previous studies have shown that TRANSPARENT TESTA genes are associated with flavonoid accumulation (Appelhagen et al., 2011; Appelhagen et al., 2015). The results indicated that the transporter genes are conserved in plants.
The correlation regulatory network of some significant DAFs and structural gene, MYBs, bHLH, TTG1 homologs, and key transporter genes in each comparison group is displayed in Figure 7B (Supplementary Table 13). In this coexpression network, many hub genes were identified, including structural genes, MYBs (Maker00011685, Maker00013236, Maker00003993, and Maker00008847), bHLHs, TTG1 homologs, and transporter genes, which correlated with multiple different flavonoids. FHT (Maker00004594) and FNSII (Maker00012388) are positively correlated with 6-methoxyluteolin 7-rhamnoside, lucenin-2, malonylapiin, nortangeretin, and 3-methoxyapigenin. CHS_4 gene (BGI_novel_G002275) is positively correlated with pelargonidin 3-O-3′′,6′′-O-dimalonylglucoside, delphinidin, 3-(6-p-coumaroyl) glucoside, isobavachalcone, phellamurin, apigenin 7,4′-dimethyl ether, and desmethylxanthohumol. EpMYB41 (Maker00003993) is positively correlated with seven flavonols, four flavones, one anthocyanidin, and one dihydrochalcone. Maker00007729 is belonged to WDR protein and was the homolog of AtTTG1-like (AtAN11). The amino acid identity between them was 92.8%. Maker00007729 is positively correlated with one dihyroflavonol and two flavonols. Some MYBs genes clustered in the subgroup of confirmed flavonoid biosynthesis-regulating Arabidopsis MYB family (Figure 3 and Supplementary Table 13), which also significantly correlated with flavonoids in this study. EpMYB84 (Maker00010600, AtMYB4) and EpMYB76 (Maker00016264, AtMYB123 [AtTT2]) are positively correlated with one anthocyanin (pelargonidin 3-O-beta-D-glucoside 5-O-[6-coumaroyl-beta-D-glucoside]) and two isoflavones (luteone, sayanedine), respectively (Figure 7B and Supplementary Table 13). EpMYB135 (Maker00004321, AtMYB5) is positively correlated with two isoflavones (luteone, sayanedine) and negatively correlated with one flavonol (quercetagetin). EpMYB64 (Maker00021702, AtMYB82) is negatively correlated with one dihydrochalcone (isouvaretin). EpMYB51 (Maker00018152, AtMYB12 [PFG1]) is positively correlated with one flavone (6-hydroxy-4′,5,7-trimethoxyflavone).
Furthermore, there were also exist one metabolite associated with multiple genes (Figure 7B and Supplementary Table 13). The expression level of Maker00007729 (AtTTG1-like [AtAN11]), EpMYB41 (Maker00003993, AtMYB36), EpMYB119 (Maker00013914, AtMYB4), EpMYB118 (Maker00013934, AtMYB44), and EpbHLH53 (Maker00018803, IIIb, AtbHLH116) was showed highly associated with the content of kaempferol 3-sophorotrioside (flavonols). Meanwhile, the accumulation of glepidotin B (dihyroflavonol) was also positively corrected with the expression of Maker00007729 (AtTTG1-like [AtAN11]), EpMYB119 (Maker00013914, AtMYB4), and EpbHLH53 (Maker00018803, IIIb, AtbHLH116). The structure genes [CHS_1 (Maker00016317), CHS_4 (BGI_novel_G002275), CHII (Maker00010747), F3H (Maker00011936), ANS_1 (Maker00011665)], EpbHLH128 (Maker00012137, IIIf, AtbHLH2), and transport genes [ABC transporter B family (Maker00006471), H+-transporting ATPase (Maker00005004)] are positively correlated with delphinidin 3-(6-p-coumaroyl) glucoside (anthocyanin). It suggested these genes may coregulated the biosynthesis of flavonols and anthocyanins.
Discussion
Analysis of Flavonoids in E. phaseoloides Stem
The specialized metabolism of legumes mainly includes terpenoids, flavonoids, and alkaloids (Wink, 2013; de Souza et al., 2019). As a member of the Leguminous family, many previous studies on E. phaseoloides metabolites have focused on terpenoids (Iwamoto et al., 2012; Xiong et al., 2013; Xiong et al., 2014). In our study, it was shown that flavonoids are also the main metabolites in E. phaseoloides stem by untargeted metabolite analysis (Supplementary Figure 1). The number of detected flavonoids type from E. phaseoloides stems was far more than previously reported (Zhao et al., 2011; Dong et al., 2012). It suggested that untargeted metabolite analysis can be applied for the comprehensively detected phytochemical compounds in the absence of knowledge of chemical components. In addition, although the types of flavonoids in different developmental stages of stem were the same, the content of compounds showed a markedly different, particularly between the young stem and the old stem. It suggested that flavonoids exhibited quantitative pattern between different developmental stages in E. phaseoloides stem. Thus, the metabolic profiling of flavonoids can provide a theoretical basis for selecting the appropriate development stage of stem to collect samples, to obtain more accumulation of active ingredients from E. phaseoloides. However, accurate characterization and quantification of flavonoids in E. phaseoloides require targeted metabolomics and more standards in future.
Analysis of Structural Genes, Transcription Factors in E. phaseoloides Stem
Flavonoids are synthesized by many pathways (including phenylpropanoid biosynthesis, flavonoid biosynthesis, anthocyanin biosynthesis, isoflavonoid biosynthesis, and flavone and flavonol biosynthesis) and are regulated by many structural genes (Koukol and Conn, 1961; Russell and Conn, 1967; Jez et al., 2000; Jun et al., 2018; Wu et al., 2020). Herein, thirty-four structural genes involved in flavonoid biosynthesis were identified (Table 1). Functionally relevant amino acid residues of most identified genes showed highly conservative. Hydroxylation of the 5′ position by F3′5′H is a particularly important step, which determines the B-ring tri-hydroxyl flavonoid end-product (epigallocatechin-3-gallate or delphinidin) synthesized in plants (Bailey et al., 2003). The F3′5′H candidate showed 60.36% similarity to previously characterized Glycine max F3′5′H sequences, but lacking functionally relevant amino acids P33, P34, P36, and P40, and one substitution of an amino acid residue in R/T389. The lacked functionally relevant amino acids may be due to the obtained transcript sequence is incomplete. Thus, it needs additional confirmation.
Dihydroflavonol is the common precursor for flavonol, anthocyanin, and proanthocyanin. Flavonol biosynthesis is competing with anthocyanin or proanthocyanidin biosynthesis. The anthocyanin or proanthocyanidin biosynthesis rate-limiting genes were significantly upregulated in the S2 stage: (DFR_1 (log2Ratio[S2/S1]: 2.23), ANS_1 (log2Ratio[S2/S1]: 1.35), ANS_2 (log2Ratio[S2/S1]: 3.03), LAR_2 (log2Ratio[S2/S1]: 1.58), ANR_1 (log2Ratio[S2/S1]: 1.37), ANR_2 (log2Ratio[S2/S1]: 1.86)). Although flavonol biosynthesis key gene FLS_1 was upregulated, the p-value is greater than 0.05 and the expression level was obviously lower than anthocyanin or proanthocyanidin biosynthesis key genes (Table 1 and Supplementary Table 10). Thus, it speculated that the anthocyanin or proanthocyanidin biosynthesis particularly anthocyanin biosynthesis was more active at the S2 stage. In addition, FNSII was significantly upregulated in the S3 stage (log2Ratio[S3/S2): 2.88) (Table 1 and Supplementary Table 10), which indicated the flavones biosynthesis was active at this stage. In brief, with the development of E. phaseoloides stem, flavonol, anthocyanin, and proanthocyanin biosynthesis were first active, and the anthocyanin or proanthocyanin biosynthesis branch was dominant. Subsequently, the flavones biosynthesis branch was active at the late developmental stage of the stem.
In addition to structural genes, TFs also play important roles in flavonoid biosynthesis (Baudry et al., 2004; Davies et al., 2012; Lloyd et al., 2017; Liu et al., 2021). As two types of the most reported TFs regulating flavonoid synthesis, the MYBs and bHLHs were identified and classified. The number and type of MYB genes identified in E. phaseoloides were in the same range as previously reported in other plant species, but no MYB4R proteins (Stracke et al., 2001; Pucker et al., 2020b). As showed in MYB phylogenetic tree (Figure 3), a total of 11 R2R3-MYBs, one R1-MYB, and one 3R-MYB were closely clustered into the clades of flavonoid biosynthesis-related Arabidopsis MYBs. Among them, eight MYBs (EpMYB108, EpMYB111, EpMYB84, EpMYB85, EpMYB78, EpMYB115, EpMYB86, and EpMYB76) were in the same clade of AtMYB75/AtMYB90/AtMYB113/AtMYB114, which have been confirmed to be related to anthocyanin biosynthesis (Borevitz et al., 2000; Teng et al., 2005; Gonzalez et al., 2008), and three MYBs (EpMYB5, EpMYB135, EpMYB64) and two MYBs (EpMYB51 and EpMYB122) were assigned into a big clade of AtMYB123 and AtMYB11/AtMYB12/AtMYB111, which have been confirmed to regulate the biosynthesis proanthocyanidin (Nesi et al., 2001; Hancock et al., 2012) and flavonol (Stracke et al., 2007; Stracke et al., 2010), respectively. The results indicate that these MYBs may have the potential ability to regulate the biosynthesis of flavonoids.
Most bHLHs involved in regulating anthocyanin biosynthesis are belonged to subgroup III, which is functionally conserved and has been demonstrated to participate in regulating plant development and defense response (Ludwig et al., 1989; Bailey et al., 2003; Heim et al., 2003; Song et al., 2021). A total of 23 subgroup III bHLHs were noted in this study, including nine subgroup IIId+e, eight subgroup IIIb, three subgroup IIIf, two subgroup IIIc, and one subgroup IIIa. It suggested that similar bHLHs regulatory for regulation of flavonoid synthesis may exist in E. phaseoloides. These predicted genes and related information will facilitate research on further investigations of the potential function of these genes in E. phaseoloides and other plants.
Analysis of Key Candidate Genes Involved in Flavonoids Accumulation in E. phaseoloides Stem
At present, transcriptome and metabolome combined analysis has been widely used to explore the relationships between the expression of genes and metabolites related to biosynthetic pathways, so as to obtain metabolites-related functional genes and regulator networks (Liu et al., 2020; Du et al., 2021; Fu et al., 2021; Hou et al., 2021; Zou et al., 2021; Wu et al., 2022). The transcriptome and metabolome association analysis results showed that some transporter genes were also obviously positively associated with various types of flavonoids. For example, the TRANSPARENT TESTA protein [Maker00022863] was the homolog of TRANSPARENT TESTA 12-like (TT12-like), encoding a multidrug and toxin extrusion (MATE) transporter, which is involved in proanthocyanidin biosynthesis in Arabidopsis seed coat and Medicago truncatula hairy roots (Debeaujon et al., 2001; Marinova et al., 2007; Zhao and Dixon, 2009). It is positively correlated with five flavonols, five flavones, and one flavanone accumulation in this study. It indicated that the TT12-like gene may be involved in other flavonoids transport, such as flavonols, flavones, and flavanones.
As shown in the phylogenetic tree results (Figure 3), EpMYB84 (Maker00010600, AtMYB4) and EpMYB76 (Maker00016264, AtMYB123 [AtTT2]), EpMYB135 (Maker00004321, AtMYB5), EpMYB64 (Maker00021702, AtMYB82), and EpMYB51 (Maker00018152, AtMYB12 [PFG1]) were closely clustered into the clade of anthocyanin and proanthocyanidin or flavanols biosynthesis-related Arabidopsis MYBs, respectively. EpMYB84 and EpMYB76 are positively correlated with one anthocyanin and two isoflavones, respectively. However, EpMYB135 is positively correlated with two isoflavones and negatively correlated with one flavonol. EpMYB64 is negatively correlated with one dihydrochalcone. EpMYB51 is positively correlated with one flavone. It is speculated that these genes may regulate different types of flavonoids with different additional regulators.
Moreover, there was also existed one metabolite associated with multiple genes. A previous study of TTG1-like (also known as AtAN11, Light-regulated WD1, or LWD1) indicated that it acts as a clock protein, contributing to the regulation of circadian period length and photoperiodic flowering (Wu et al., 2016). AtMYB4 has been shown to act as a transcriptional repressor for the expression of early phenylpropanoid genes C4H (Jin et al., 2000). Our correlation results showed that the Maker00007729 (AtTTG1-like [AtAN11]) is positively regulated glepidotin B (dihyroflavonol) and kaempferol 3-sophorotrioside (flavonol) biosynthesis by interaction with bHLH proteins such as EpbHLH53 (Maker00018803, IIIb, AtbHLH116) and EpMYB119 (Maker00013914, AtMYB4). These findings suggested that the WDR gene TTG1-like (AN11) regulated dihydrochalcones and flavonol biosynthesis in specific combinations with IIIb bHLH and R2R3MYBs in E. phaseoloides stem.
This was the first report for flavonoid synthesis in the genus Entada. Our data suggested that some genes might be regulated together as a regulator network involved in flavonoid biosynthesis, particularly flavonols and anthocyanins in E. phaseoloides stems. Meanwhile, it also indicated that some genes regulate not only one type of flavonoid but several distinct subclasses of flavonoid biosynthesis. Taken together, these results indicated that the process of flavonoids metabolism keeps notably activated during the development of stems in E. phaseoloides, and it is a complicated process coregulated by multiple genes. However, these results were based on the bioinformatic analysis data. Experimental verification such as transgenic studies will be needed to confirm the roles of these genes in flavonoid biosynthesis in E. phaseoloides in the future.
In spite of this, this study offered candidate genes involved in flavonoid biosynthesis and characteristics of flavonoids accumulation, improved our understanding of the MYBs and bHLHs-related regulation networks of flavonoid biosynthesis in E. phaseoloides stem, and also provided references for the metabolic engineering of flavonoid biosynthesis in E. phaseoloides stem.
Data Availability Statement
The sequencing raw data have been deposited to China National GeneBank (CNGB: https://db.cngb.org) with project accession ID: CNP0002249 (S1: CNR0454679, CNR0454680, and CNR0454681; S2: CNR0454682, CNR0454683, and CNR0454684) and CNP0001900 (S3: CNR0390213, CNR0390214, and CNR0390215).
Author Contributions
ZM designed the project. ML accomplished the experiments. ZZ helped with sample treatment and data analyzing. ML wrote and revised the manuscript. All authors read and agreed to the final manuscript.
Funding
This work was funded by grants from the National Major Scientific and Technological Special Project for “Significant New Drugs Development” of China (no. 2017ZX09301060) and National Key Research and Development Program of China (no. 2018YFC1708004).
Conflict of Interest
The authors declare that the research was conducted in the absence of any commercial or financial relationships that could be construed as a potential conflict of interest.
Publisher’s Note
All claims expressed in this article are solely those of the authors and do not necessarily represent those of their affiliated organizations, or those of the publisher, the editors and the reviewers. Any product that may be evaluated in this article, or claim that may be made by its manufacturer, is not guaranteed or endorsed by the publisher.
Acknowledgments
We are grateful to Yinghong Zhao for helping with sampling. We also thank friends Jianbo Jian and Chunhai Chen from BGI-Shenzhen for sample sequencing, some analysis, and discussions.
Supplementary Material
The Supplementary Material for this article can be found online at: https://www.frontiersin.org/articles/10.3389/fpls.2022.792674/full#supplementary-material
Footnotes
- ^ https://github.com/BGI-flexlab/SOAPnuke/
- ^ http://daehwankimlab.github.io/hisat2/
- ^ http://ccb.jhu.edu/software/stringtie/
- ^ https://github.com/bpucker/KIPEs
References
Anders, S., and Huber, W. (2010). Differential expression analysis for sequence count data. Genome Biol. 11:R106. doi: 10.1186/gb-2010-11-10-r106
Appelhagen, I., Lu, G. H., Huep, G., Schmelzer, E., and Sagasser, M. (2011). TRANSPARENT TESTA1 interacts with R2R3-MYB factors and affects early and late steps of flavonoid biosynthesis in the endothelium of Arabidopsis thaliana seeds. Plant J. 67, 406–419. doi: 10.1111/j.1365-313X.2011.04603.x
Appelhagen, I., Nordholt, N., Seidel, T., Spelt, K., Koes, R., Quattrochio, F., et al. (2015). TRANSPARENT TESTA 13 is a tonoplast P3A -ATPase required for vacuolar deposition of proanthocyanidins in Arabidopsis thaliana seeds. Plant J. 82, 840–848. doi: 10.1111/tpj.12854
Ayissi, O. B., Njayou, N. F., Laufer, S., Moundipa, P. F., and Schluesener, H. J. (2013). A fraction of stem bark extract of Entada africana suppresses lipopolysaccharide-induced inflammation in RAW 264.7 cells. J. Ethnopharmacol. 149, 162–168. doi: 10.1016/j.jep.2013.06.016
Bailey, P. C., Martin, C., Toledo-Ortiz, G., Quail, P. H., Huq, E., Heim, M. A., et al. (2003). Update on the basic helix-loop-helix transcription factor gene family in Arabidopsis thaliana. Plant Cell 15, 2497–2502. doi: 10.1105/tpc.151140
Bansal, P., Paul, P., Mudgal, J., Nayak, P. G., Pannakal, S. T., Priyadarsini, K. I., et al. (2012). Antidiabetic, antihyperlipidemic and antioxidant effects of the flavonoid rich fraction of Pilea microphylla (L.) in high fat diet/streptozotocin-induced diabetes in mice. Exp. Toxicol. Pathol. 64, 651–658. doi: 10.1016/j.etp.2010.12.009
Barcelo, S., Peralta, M., Calise, M., Finck, S., Ortega, G., Diez, R. A., et al. (2017). Interactions of a prenylated flavonoid from Dalea elegans with fluconazole against azole-resistant Candida albicans. Phytomedicine 32, 24–29. doi: 10.1016/j.phymed.2017.05.001
Baudry, A., Heim, M. A., Dubreucq, B., Caboche, M., Weisshaar, B., and Lepiniec, L. (2004). TT2, TT8, and TTG1 synergistically specify the expression of BANYULS and proanthocyanidin biosynthesis in Arabidopsis thaliana. Plant J. 39, 366–380. doi: 10.1111/j.1365-313X.2004.02138.x
Baxter, I. R., Young, J. C., Armstrong, G., Foster, N., Bogenschutz, N., Cordova, T., et al. (2005). A plasma membrane H+-ATPase is required for the formation of proanthocyanidins in the seed coat endothelium of Arabidopsis thaliana. Proc. Natl. Acad. Sci. U.S.A. 102, 2649–2654. doi: 10.1073/pnas.0406377102
Borevitz, J. O., Xia, Y., Blount, J., Dixon, R. A., and Lamb, C. (2000). Activation tagging identifies a conserved MYB regulator of phenylpropanoid biosynthesis. Plant Cell 12, 2383–2394. doi: 10.1105/tpc.12.12.2383
Bors, W., Heller, W., Michel, C., and Saran, M. (1990). Flavonoids as antioxidants: determination of radical-scavenging efficiencies. Methods Enzymol. 186, 343–355. doi: 10.1016/0076-6879(90)86128-i
Casas, M. I., Lorena Falcone-Ferreyra, M., Jiang, N., Mejia-Guerra, M. K., Rodriguez, E., Wilson, T., et al. (2016). Identification and characterization of maize salmon silks genes involved in insecticidal maysin biosynthesis. Plant Cell 28, 1297–1309. doi: 10.1105/tpc.16.00003
Cushnie, T., and Lamb, A. (2005). Antimicrobial activity of flavonoids. Int. J. Antimicrob. Agent 26, 343–356. doi: 10.1016/j.ijantimicag.2005.09.002
Davies, K. M., Albert, N. W., and Schwinn, K. E. (2012). From landing lights to mimicry: the molecular regulation of flower colouration and mechanisms for pigmentation patterning. Funct. Plant Biol. 39, 619–638. doi: 10.1071/FP12195
de Souza, L. P., Scossa, F., Proost, S., Bitocchi, E., Papa, R., Tohge, T., et al. (2019). Multi-tissue integration of transcriptomic and specialized metabolite profiling provides tools for assessing the common bean (Phaseolus vulgaris) metabolome. Plant J. 97, 1132–1153. doi: 10.1111/tpj.14178
Debeaujon, I., Peeters, A. J., Léon-Kloosterziel, K. M., and Koornneef, M. (2001). The TRANSPARENT TESTA12 gene of Arabidopsis encodes a multidrug secondary transporter-like protein required for flavonoid sequestration in vacuoles of the seed coat endothelium. Plant Cell 13, 853–871. doi: 10.1105/tpc.13.4.853
Diallo, D., Marston, A., Terreaux, C., Touré, Y., Paulsen, B. S., and Hostettmann, K. (2001). Screening of Malian medicinal plants for antifungal, larvicidal, molluscicidal, antioxidant and radical scavenging activities. Phytother. Res. 15, 401–406. doi: 10.1002/ptr.738
Dong, Y., Shi, H., Yang, H., Peng, Y., Wang, M., and Li, X. (2012). Antioxidant phenolic compounds from the stems of Entada phaseoloides. Chem. Biodivers. 9, 68–79. doi: 10.1002/cbdv.201100002
Du, Z., Lin, W., Yu, B., Zhu, J., and Li, J. (2021). Integrated metabolomic and transcriptomic analysis of the flavonoid accumulation in the leaves of cyclocarya paliurus at different altitudes. Front. Plant Sci. 12:794137. doi: 10.3389/fpls.2021.794137
Falcone Ferreyra, M. L., Rius, S. P., and Casati, P. (2012). Flavonoids: biosynthesis, biological functions, and biotechnological applications. Front. Plant Sci. 3:222. doi: 10.3389/fpls.2012.00222
Fu, M., Yang, X., Zheng, J., Wang, L., Yang, X., Tu, Y., et al. (2021). Unraveling the regulatory mechanism of color diversity in Camellia japonica petals by integrative transcriptome and metabolome analysis. Front. Plant Sci. 12:685136. doi: 10.3389/fpls.2021.685136
Gebhardt, Y. H., Witte, S., Steuber, H., Matern, U., and Martens, S. (2007). Evolution of flavone synthase I from parsley flavanone 3beta-hydroxylase by site-directed mutagenesis. Plant Physiol. 144, 1442–1454. doi: 10.1104/pp.107.098392
Gonzalez, A., Zhao, M., Leavitt, J. M., and Lloyd, A. M. (2008). Regulation of the anthocyanin biosynthetic pathway by the TTG1/bHLH/Myb transcriptional complex in Arabidopsis seedlings. Plant J. 53, 814–827. doi: 10.1111/j.1365-313X.2007.03373.x
Grigalius, I., and Petrikaite, V. (2017). Relationship between antioxidant and anticancer activity of trihydroxyflavones. Molecules 22:2169. doi: 10.3390/molecules22122169
Hancock, K. R., Collette, V., Fraser, K., Greig, M., Xue, H., Richardson, K., et al. (2012). Expression of the R2R3-MYB Transcription factor TaMYB14 from Trifolium arvense activates proanthocyanidin biosynthesis in the legumes Trifolium repens and Medicago sativa. Plant Physiol. 159, 1204–1220. doi: 10.1104/pp.112.195420
Hassani, D., Fu, X., Shen, Q., Khalid, M., Rose, J., and Tang, K. (2020). Parallel transcriptional regulation of artemisinin and flavonoid biosynthesis. Trends Plant Sci. 25, 466–476. doi: 10.1016/j.tplants.2020.01.001
Heim, M. A., Jakoby, M., Werber, M., Martin, C., Weisshaar, B., and Bailey, P. C. (2003). The basic helix-loop-helix transcription factor family in plants: a genome-wide study of protein structure and functional diversity. Mol. Biol. Evol. 20, 735–747. doi: 10.1093/molbev/msg088
Holton, T. A., and Cornish, E. C. (1995). Genetics and biochemistry of anthocyanin biosynthesis. Plant Cell 7, 1071–1083. doi: 10.1105/tpc.7.7.1071
Hou, S., Du, W., Hao, Y., Han, Y., Li, H., Liu, L., et al. (2021). Elucidation of the regulatory network of flavonoid biosynthesis by profiling the metabolome and transcriptome in tartary buckwheat. J. Agric. Food Chem. 69, 7218–7229. doi: 10.1021/acs.jafc.1c00190
Iwamoto, Y., Sugimoto, S., Harinantenaina, L., Matsunami, K., and Otsuka, H. (2012). Entadosides A-D, triterpene saponins and a glucoside of the sulphur-containing amide from the kernel nuts of Entada phaseoloides (L.) Merrill. J. Nat. Med. Tokyo 66, 321–328. doi: 10.1007/s11418-011-0591-1
Jaakola, L. (2013). New insights into the regulation of anthocyanin biosynthesis in fruits. Trends Plant Sci. 18, 477–483. doi: 10.1016/j.tplants.2013.06.003
Jez, J. M., Bowman, M. E., Dixon, R. A., and Noel, J. P. (2000). Structure and mechanism of the evolutionarily unique plant enzyme chalcone isomerase. Nat. Struct. Biol. 7, 786–791. doi: 10.1038/79025
Jia, N., Shu, Q., Wang, L., Du, H., Xu, Y., and Liu, Z. (2008). Analysis of petal anthocyanins to investigate coloration mechanism in herbaceous peony cultivars. Sci. Hortic. Amsterdam 117, 167–173. doi: 10.1016/j.scienta.2008.03.016
Jiang, T., Guo, K., Liu, L., Tian, W., Xie, X., Wen, S., et al. (2020). Integrated transcriptomic and metabolomic data reveal the flavonoid biosynthesis metabolic pathway in Perilla frutescens (L.) leaves. Sci Rep 10:16207. doi: 10.1038/s41598-020-73274-y
Jin, H., Cominelli, E., Bailey, P., Parr, A., Mehrtens, F., Jones, J., et al. (2000). Transcriptional repression by AtMYB4 controls production of UV-protecting sunscreens in Arabidopsis. EMBO J. 19, 6150–6161. doi: 10.1093/emboj/19.22.6150
Jun, J. H., Xiao, X., Rao, X., and Dixon, R. A. (2018). Proanthocyanidin subunit composition determined by functionally diverged dioxygenases. Nat. Plants 4, 1034–1043. doi: 10.1038/s41477-018-0292-9
Karppinen, K., Lafferty, D. J., Albert, N. W., Mikkola, N., McGhie, T., Allan, A. C., et al. (2021). MYBA and MYBPA transcription factors co-regulate anthocyanin biosynthesis in blue-coloured berries. New Phytol. 232, 1350–1367. doi: 10.1111/nph.17669
Khan, J., Deb, P. K., Priya, S., Medina, K. D., Devi, R., Walode, S. G., et al. (2021). Dietary flavonoids: cardioprotective potential with antioxidant effects and their pharmacokinetic, toxicological and therapeutic concerns. Molecules 26:4021. doi: 10.3390/molecules26134021
Kitamura, S. (2006). “Transport of flavonoids: from cytosolic synthesis to vacuolar accumulation,” in The Science of Flavonoids, ed. E. Grotewold (New York, NY: Springer), 123–146. doi: 10.1007/978-0-387-28822-2_5
Koes, R., Verweij, W., and Quattrocchio, F. (2005). Flavonoids: a colorful model for the regulation and evolution of biochemical pathways. Trends Plant Sci. 10, 236–242. doi: 10.1016/j.tplants.2005.03.002
Kopustinskiene, D. M., Jakstas, V., Savickas, A., and Bernatoniene, J. (2020). Flavonoids as anticancer agents. Nutrients 12:457. doi: 10.3390/nu12020457
Koukol, J., and Conn, E. E. (1961). The metabolism of aromatic compounds in higher plants. IV. Purification and properties of the phenylalanine deaminase of Hordeum vulgare. J. Biol. Chem. 236, 2692–2698. doi: 10.1016/S0021-9258(19)61721-7
Kreuzaler, F., Ragg, H., Fautz, E., Kuhn, D. N., and Hahlbrock, K. (1983). UV-induction of chalcone synthase mRNA in cell suspension cultures of Petroselinum hortense. Proc. Natl. Acad. Sci. U.S.A. 80, 2591–2593. doi: 10.1073/pnas.80.9.2591
Kreuzaler, F., and Hahlbrock, K. (1975). Enzymic synthesis of an aromatic ring from acetate units. Partial purification and some properties of flavanone synthase from cell-suspension cultures of Petroselinum hortense. Eur. J. Biochem. 56, 205–213. doi: 10.1111/j.1432-1033.1975.tb02223.x
Leoni, F., Hazrati, H., Fomsgaard, I. S., Moonen, A. C., and Kudsk, P. (2021). Determination of the effect of co-cultivation on the production and root exudation of flavonoids in four legume species using LC-MS/MS analysis. J. Agric. Food Chem. 69, 9208–9219. doi: 10.1021/acs.jafc.1c02821
Li, J., Luan, Q., Han, J., Zhang, C., Liu, M., and Ren, Z. (2020). CsMYB60 directly and indirectly activates structural genes to promote the biosynthesis of flavonols and proanthocyanidins in cucumber. Hortic Res 7:103. doi: 10.1038/s41438-020-0327-z
Liu, Y., Lv, J., Liu, Z., Wang, J., Yang, B., Chen, W., et al. (2020). Integrative analysis of metabolome and transcriptome reveals the mechanism of color formation in pepper fruit (Capsicum annuum L.). Food Chem. 306:125629. doi: 10.1016/j.foodchem.2019.125629
Liu, Y., Ma, K., Qi, Y., Lv, G., Ren, X., Liu, Z., et al. (2021). Transcriptional regulation of anthocyanin synthesis by MYB-bHLH-WDR complexes in kiwifruit (Actinidia chinensis). J. Agric. Food Chem. 69, 3677–3691. doi: 10.1021/acs.jafc.0c07037
Lloyd, A., Brockman, A., Aguirre, L., Campbell, A., Bean, A., Cantero, A., et al. (2017). Advances in the MYB-bHLH-WD repeat (MBW) pigment regulatory model: addition of a WRKY factor and co-option of an anthocyanin MYB for betalain regulation. Plant Cell Physiol. 58, 1431–1441. doi: 10.1093/pcp/pcx075
Love, M. I., Huber, W., and Anders, S. (2014). Moderated estimation of fold change and dispersion for RNA-seq data with DESeq2. Genome Biol. 15:550. doi: 10.1186/s13059-014-0550-8
Ludwig, S. R., Habera, L. F., Dellaporta, S. L., and Wessler, S. R. (1989). Lc, a member of the maize R gene family responsible for tissue-specific anthocyanin production, encodes a protein similar to transcriptional activators and contains the myc-homology region. Proc. Natl. Acad. Sci. U.S.A. 86, 7092–7096. doi: 10.1073/pnas.86.18.7092
Ma, D., Tang, H., Reichelt, M., Piirtola, E., Salminen, J., Gershenzon, J., et al. (2021). Poplar MYB117 promotes anthocyanin synthesis and enhances flavonoid B-ring hydroxylation by up-regulating the flavonoid 3 ‘,5’-hydroxylase gene. J. Exp. Bot. 72, 3864–3880. doi: 10.1093/jxb/erab116
Marinova, K., Pourcel, L., Weder, B., Schwarz, M., Barron, D., Routaboul, J. M., et al. (2007). The Arabidopsis MATE transporter TT12 acts as a vacuolar flavonoid/H+ -antiporter active in proanthocyanidin-accumulating cells of the seed coat. Plant Cell 19, 2023–2038. doi: 10.1105/tpc.106.046029
Mehrtens, F., Kranz, H., Bednarek, P., and Weisshaar, B. (2005). The Arabidopsis transcription factor MYB12 is a flavonol-specific regulator of phenylpropanoid biosynthesis. Plant Physiol. 138, 1083–1096. doi: 10.1104/pp.104.058032
Moustafa, E., and Wong, E. (1967). Purification and properties of chalcone-flavanone isomerase from soya bean seed. Phytochemistry 6, 625–632. doi: 10.1016/S0031-9422(00)86001-X
Mower, R. L., Landolfi, R., and Steiner, M. (1984). Inhibition in vitro of platelet aggregation and arachidonic acid metabolism by flavone. Biochem. Pharmacol. 33, 357–363. doi: 10.1016/0006-2952(84)90226-0
Nesi, N., Jond, C., Debeaujon, I., Caboche, M., and Lepiniec, L. (2001). The Arabidopsis TT2 gene encodes an R2R3 MYB domain protein that acts as a key determinant for proanthocyanidin accumulation in developing seed. Plant Cell 13, 2099–2114. doi: 10.1105/tpc.13.9.2099
Njayou, F. N., Aboudi, E., Tandjang, M. K., Tchana, A. K., and Moundipa, P. F. (2013). Hepatoprotective and antioxidant activities of stem bark extract of Khaya grandifoliola (Welw) CDC and Entada africana Guill. Et Perr. J. Nat. Prod. 7, 73–80.
Njayou, F. N., Amougou, A. M., Fouemene, T. R., Njikam, M. J., Rudraiah, S., Bradley, B., et al. (2015). Antioxidant fractions of Khaya grandifoliola C.DC. And Entada africana Guill. Et Perr. Induce nuclear translocation of Nrf2 in HC-04 cells. Cell Stress Chaperones 20, 991–1000. doi: 10.1007/s12192-015-0628-6
Ohashi, H., Huang, T. C., and Ohashi, K. (2010). Entada (Leguminosae subfam. Mimosoideae) of Taiwan. Taiwania 55, 43–53. doi: 10.1590/2175-7860201667319
Peng, M., Shahzad, R., Gul, A., Subthain, H., Shen, S., Lei, L., et al. (2017). Differentially evolved glucosyltransferases determine natural variation of rice flavone accumulation and UV-tolerance. Nat. Commun. 8:1975. doi: 10.1038/s41467-017-02168-x
Pucker, B. (2021). Automatic identification and annotation of MYB gene family members in plants. bioRxiv [Preprint] doi: 10.1101/2021.10.16.464636
Pucker, B., Reiher, F., and Schilbert, H. M. (2020a). Automatic identification of players in the flavonoid biosynthesis with application on the biomedicinal plant Croton tiglium. Plants (Basel) 9:1103. doi: 10.3390/plants9091103
Pucker, B., Pandey, A., Weisshaar, B., and Stracke, R. (2020b). The R2R3-MYB gene family in banana (Musa acuminata): genome-wide identification, classification and expression patterns. PLoS One 15:e239275. doi: 10.1371/journal.pone.0239275
Ramsay, N. A., and Glover, B. J. (2005). MYB-bHLH-WD40 protein complex and the evolution of cellular diversity. Trends Plant Sci. 10, 63–70. doi: 10.1016/j.tplants.2004.12.011
Ratty, A. K., and Das, N. P. (1988). Effects of flavonoids on nonenzymatic lipid peroxidation: structure-activity relationship. Biochem. Med. Metab. Biol. 39, 69–79. doi: 10.1016/0885-4505(88)90060-6
Roheem, F. O., Mat, S. S., Ahmed, Q. U., Ali, S. S., Latip, J., and Zakaria, Z. A. (2019). Evaluation of the enzyme inhibitory and antioxidant activities of entada spiralis stem bark and isolation of the active constituents. Molecules 24:1006. doi: 10.3390/molecules24061006
Russell, D. W., and Conn, E. E. (1967). The cinnamic acid 4-hydraxylase of pea seedlings. Arch. Biochem. Biophys. 122, 256–258. doi: 10.1016/0003-9861(67)90150-6
Saigo, T., Wang, T., Watanabe, M., and Tohge, T. (2020). Diversity of anthocyanin and proanthocyanin biosynthesis in land plants. Curr. Opin. Plant Biol. 55, 93–99. doi: 10.1016/j.pbi.2020.04.001
Sarkhail, P., Rahmanipour, S., Fadyevatan, S., Mohammadirad, A., Dehghan, G., Amin, G., et al. (2007). Antidiabetic effect of Phlomis anisodonta: effects on hepatic cells lipid peroxidation and antioxidant enzymes in experimental diabetes. Pharmacol. Res. 56, 261–266. doi: 10.1016/j.phrs.2007.07.003
Song, M., Wang, H., Wang, Z., Huang, H., Chen, S., and Ma, H. (2021). Genome-wide characterization and analysis of bHLH transcription factors related to anthocyanin biosynthesis in Fig (Ficus carica L.). Front. Plant Sci. 12:730692. doi: 10.3389/fpls.2021.730692
Soto-Vaca, A., Gutierrez, A., Losso, J. N., Xu, Z., and Finley, J. W. (2012). Evolution of phenolic compounds from color and flavor problems to health benefits. J. Agric. Food Chem. 60, 6658–6677. doi: 10.1021/jf300861c
Spagnuolo, C., Moccia, S., and Russo, G. L. (2018). Anti-inflammatory effects of flavonoids in neurodegenerative disorders. Eur. J. Med. Chem. 153, 105–115. doi: 10.1016/j.ejmech.2017.09.001
Stobiecki, M., and Kachlicki, P. (2006). “Isolation and identification of flavonoids,” in The Science of Flavonoidsed, ed. E. Grotewold (New York, NY: Springer), 47–69. doi: 10.1007/978-0-387-28822-2_2
Stracke, R., Ishihara, H., Huep, G., Barsch, A., Mehrtens, F., Niehaus, K., et al. (2007). Differential regulation of closely related R2R3-MYB transcription factors controls flavonol accumulation in different parts of the Arabidopsis thaliana seedling. Plant J. 50, 660–677. doi: 10.1111/j.1365-313X.2007.03078.x
Stracke, R., Jahns, O., Keck, M., Tohge, T., Niehaus, K., Fernie, A. R., et al. (2010). Analysis of PRODUCTION of FLAVONOL GLYCOSIDES-dependent flavonol glycoside accumulation in Arabidopsis thaliana plants reveals MYB11-, MYB12- and MYB111-independent flavonol glycoside accumulation. New Phytol. 188, 985–1000. doi: 10.1111/j.1469-8137.2010.03421.x
Stracke, R., Werber, M., and Weisshaar, B. (2001). The R2R3-MYB gene family in Arabidopsis thaliana. Curr. Opin. Plant Biol. 4, 447–456. doi: 10.1016/S1369-5266(00)00199-0
Teke, G. N., Lunga, P. K., Wabo, H. K., Kuiate, J. R., Vilarem, G., Giacinti, G., et al. (2011). Antimicrobial and antioxidant properties of methanol extract, fractions and compounds from the stem bark of Entada abyssinica Stend ex a. Satabie. BMC Complement. Altern. Med. 11:57. doi: 10.1186/1472-6882-11-57
Teng, S., Keurentjes, J., Bentsink, L., Koornneef, M., and Smeekens, S. (2005). Sucrose-specific induction of anthocyanin biosynthesis in Arabidopsis requires the MYB75/PAP1 gene. Plant Physiol. 139, 1840–1852. doi: 10.1104/pp.105.066688
Tohge, T., de Souza, L. P., and Fernie, A. R. (2017). Current understanding of the pathways of flavonoid biosynthesis in model and crop plants. J. Exp. Bot. 68, 4013–4028. doi: 10.1093/jxb/erx177
Tohge, T., Perez De Souza, L., and Fernie, A. R. (2018). On the natural diversity of phenylacylated-flavonoid and their in planta function under conditions of stress. Phytochem. Rev. 17, 279–290. doi: 10.1007/s11101-017-9531-3
Tohge, T., Wendenburg, R., Ishihara, H., Nakabayashi, R., Watanabe, M., Sulpice, R., et al. (2016). Characterization of a recently evolved flavonol-phenylacyltransferase gene provides signatures of natural light selection in Brassicaceae. Nat. Commun. 7:12399. doi: 10.1038/ncomms12399
Tohge, T., and Fernie, A. R. (2010). Combining genetic diversity, informatics and metabolomics to facilitate annotation of plant gene function. Nat. Protoc. 5, 1210–1227. doi: 10.1038/nprot.2010.82
Toledo-Ortiz, G., Huq, E., and Quail, P. H. (2003). The Arabidopsis basic/helix-loop-helix transcription factor family. Plant Cell 15, 1749–1770. doi: 10.1105/tpc.013839
Ververidis, F., Trantas, E., Douglas, C., Vollmer, G., Kretzschmar, G., and Panopoulos, N. (2007). Biotechnology of flavonoids and other phenylpropanoid-derived natural products. Part I: chemical diversity, impacts on plant biology and human health. Biotechnol. J. 2, 1214–1234. doi: 10.1002/biot.200700084
Wan, M. K. W., Shameli, K., Jazayeri, S. D., Othman, N. A., Che, J. N., and Hassan, N. M. (2020). Biosynthesized silver nanoparticles by aqueous stem extract of entada spiralis and screening of their biomedical activity. Front Chem 8:620. doi: 10.3389/fchem.2020.00620
Wang, N., Liu, W., Zhang, T., Jiang, S., Xu, H., Wang, Y., et al. (2018). Transcriptomic analysis of Red-Fleshed apples reveals the novel role of MdWRKY11 in flavonoid and anthocyanin biosynthesis. J. Agric. Food Chem. 66, 7076–7086. doi: 10.1021/acs.jafc.8b01273
Wen, B., Mei, Z., Zeng, C., and Liu, S. (2017). MetaX: a flexible and comprehensive software for processing metabolomics data. BMC Bioinformatics 18:183. doi: 10.1186/s12859-017-1579-y
Wen, W., Alseekh, S., and Fernie, A. R. (2020). Conservation and diversification of flavonoid metabolism in the plant kingdom. Curr. Opin. Plant Biol. 55, 100–108. doi: 10.1016/j.pbi.2020.04.004
Wink, M. (2013). Evolution of secondary metabolites in legumes (Fabaceae). S. Afr. J. Bot. 89, 164–175. doi: 10.1016/j.sajb.2013.06.006
Winkel, B. S. J. (2006). “The biosynthesis of flavonoids,” in The Science of Flavonoids, ed. E. Grotewold (New York, NY: Springer), 71–95. doi: 10.1007/978-0-387-28822-2_3
Wu, J. F., Tsai, H. L., Joanito, I., Wu, Y. C., Chang, C. W., Li, Y. H., et al. (2016). LWD-TCP complex activates the morning gene CCA1 in Arabidopsis. Nat. Commun. 7:13181. doi: 10.1038/ncomms13181
Wu, Y., Wang, T., Xin, Y., Wang, G., and Xu, L. (2020). Overexpression of GbF3’5’H1 provides a potential to improve the content of epicatechin and gallocatechin. Molecules 25:4836. doi: 10.3390/molecules25204836
Wu, Y., Zhang, C., Huang, Z., Lyu, L., Li, W., and Wu, W. (2022). Integrative analysis of the metabolome and transcriptome provides insights into the mechanisms of flavonoid biosynthesis in blackberry. Food Res. Int. 153:110948. doi: 10.1016/j.foodres.2022.110948
Xiong, H., Ding, X., Yang, X., Yang, G., and Mei, Z. (2014). Triterpene Saponins from the Stems of Entada phaseoloides. Planta Med. 80, 710–718. doi: 10.1055/s-0034-1368455
Xiong, H., Luo, M., Ju, Y., Zhao, Z., Zhang, M., Xu, R., et al. (2021). Triterpene saponins from Guo-gang-long attenuate collagen-induced arthritis via regulating A20 and inhibiting MAPK pathway. J. Ethnopharmacol. 269:113707. doi: 10.1016/j.jep.2020.113707
Xiong, H., Mei, Z., Yang, G., Mo, S., Yang, X., Zhang, P., et al. (2013). Triterpene Saponins from Entada phaseoloides. Helv. Chim. Acta 96, 1579–1589. doi: 10.1002/hlca.201200491
Xu, P., Wu, L., Cao, M., Ma, C., Xiao, K., Li, Y., et al. (2021). Identification of MBW complex components implicated in the biosynthesis of flavonoids in woodland strawberry. Front. Plant Sci. 12:774943. doi: 10.3389/fpls.2021.774943
Xu, W., Dubos, C., and Lepiniec, L. (2015). Transcriptional control of flavonoid biosynthesis by MYB-bHLH-WDR complexes. Trends Plant Sci. 20, 176–185. doi: 10.1016/j.tplants.2014.12.001
Yang, X., Yang, J., Xu, C., Huang, M., Zhou, Q., Lv, J., et al. (2015). Antidiabetic effects of flavonoids from Sophora flavescens EtOAc extract in type 2 diabetic KK-ay mice. J. Ethnopharmacol. 171, 161–170. doi: 10.1016/j.jep.2015.05.043
Zhang, B., Xu, X., Huang, R., Yang, S., Li, M., and Guo, Y. (2021). CRISPR/Cas9-mediated targeted mutation reveals a role for AN4 rather than DPL in regulating venation formation in the corolla tube of Petunia hybrida. Hortic. Res. 8:116. doi: 10.1038/s41438-021-00555-6
Zhao, J., and Dixon, R. A. (2009). MATE transporters facilitate vacuolar uptake of Epicatechin 3’-O-Glucoside for proanthocyanidin biosynthesis in Medicago truncatula and Arabidopsis. Plant Cell 21, 2323–2340. doi: 10.1105/tpc.109.067819
Zhao, Z. X., Jin, J., Lin, C. Z., Zhu, C. C., Liu, Y. M., Lin, A. H., et al. (2011). Two new chalcone glycosides from the stems of Entada phaseoloides. Fitoterapia 82, 1102–1105. doi: 10.1016/j.fitote.2011.07.005
Keywords: Entada phaseoloides, flavonoids, metabolome, transcriptome, structural genes, transcription factors, transport genes
Citation: Lin M, Zhou Z and Mei Z (2022) Integrative Analysis of Metabolome and Transcriptome Identifies Potential Genes Involved in the Flavonoid Biosynthesis in Entada phaseoloides Stem. Front. Plant Sci. 13:792674. doi: 10.3389/fpls.2022.792674
Received: 11 October 2021; Accepted: 21 March 2022;
Published: 10 May 2022.
Edited by:
Raimund Nagel, Leipzig University, GermanyReviewed by:
José Juan Ordaz-Ortiz, Centro de Investigación y de Estudios Avanzados del Instituto Politécnico Nacional (CINVESTAV), MexicoAnton R. Schäffner, Helmholtz Center München, Helmholtz Association of German Research Centres (HZ), Germany
Copyright © 2022 Lin, Zhou and Mei. This is an open-access article distributed under the terms of the Creative Commons Attribution License (CC BY). The use, distribution or reproduction in other forums is permitted, provided the original author(s) and the copyright owner(s) are credited and that the original publication in this journal is cited, in accordance with accepted academic practice. No use, distribution or reproduction is permitted which does not comply with these terms.
*Correspondence: Zhinan Mei, bWVpemhpbmFuQDE2My5jb20=