- 1Key Laboratory of Biology and Genetic Improvement of Oil Crops, Ministry of Agriculture and Rural Affairs, Oil Crops Research Institute, Chinese Academy of Agricultural Sciences, Wuhan, China
- 2National Key Laboratory of Crop Genetic Improvement, College of Plant Science and Technology, Huazhong Agricultural University, Wuhan, China
- 3Collaborative Innovation Center of Henan Grain Crops, College of Agronomy, Henan Agricultural University, Zhengzhou, China
Leaf-chewing insects are important pests that cause yield loss and reduce seed quality in soybeans (Glycine max). Breeding soybean varieties that are resistant to leaf-chewing insects can minimize the need for insecticide use and reduce yield loss. The marker gene for QTL-M, Glyma.07g110300 (LOC100775351) that encodes a UDP-glycosyltransferase (UGT) is the major determinant of resistance against leaf-chewing insects in soybean; it exhibits a loss of function in insect-resistant soybean germplasms. In this study, Agrobacterium-mediated transformation introduced the CRISPR/Cas9 expression vector into the soybean cultivar Tianlong No. 1 to generate Glyma.07g110300-gene mutants. We obtained two novel types of mutations, a 33-bp deletion and a single-bp insertion in the GmUGT coding region, which resulted in an enhanced resistance to Helicoverpa armigera and Spodoptera litura. Additionally, overexpressing GmUGT produced soybean varieties that were more sensitive to H. armigera and S. litura. Both mutant and overexpressing lines exhibited no obvious phenotypic changes. The difference in metabolites and gene expression suggested that GmUGT is involved in imparting resistance to leaf-chewing insects by altering the flavonoid content and expression patterns of genes related to flavonoid biosynthesis and defense. Furthermore, ectopic expression of the GmUGT gene in the ugt72b1 mutant of Arabidopsis substantially rescued the phenotype of H. armigera resistance in the atugt72b1 mutant. Our study presents a strategy for increasing resistance against leaf-chewing insects in soybean through CRISPR/Cas9-mediated targeted mutagenesis of the UGT genes.
Introduction
Soybean (Glycine max) is an important industrial crop that provides edible oil, vegetable protein, and active compounds, such as flavonoids (Arifin et al., 2021). Leaf-chewing insects, such as Helicoverpa zea, Helicoverpa armigera Hübner, and Spodoptera litura Fabricius, are important insect pests that cause a loss in yield and a decline in the quality of soybeans (Otuka et al., 2020; Haile et al., 2021). Farmers mainly depend on chemical pesticides to control this threat. However, the long-term use of chemical pesticides leads to the development of drug resistance in the target insects, contaminates land and water resources, and increases costs of environmental management (Elba et al., 2014; Bondori et al., 2018). Breeding soybean varieties that are resistant to leaf-chewing insects can minimize the need for insecticides, improve soybean yields, and reduce concerns stemming from pesticide use (Ortega et al., 2016).
Transgenic breeding offers key breakthroughs in plant breeding, and the cultivation of transgenic crops has appreciably increased world agricultural productivity in the past two decades (Raman, 2017). Genetically modified cultivars expressing insecticidal proteins have become vital to the effective management of leaf-chewing insect pests. Major insecticidal proteins include the crystal protein (CRY), the vegetative insecticidal protein (VIP), and the protease inhibitor (PI), whose genes have been used to develop insect-resistant genetically modified cultivars (Saikhedkar et al., 2019; Kumar et al., 2020). Many insect-resistant transgenic crops now exist; there are six commercialized soybean cultivars with various insect-resistance (IR) genes (Ichim, 2019; Kumar et al., 2020). This genetic trait provides an effective control strategy for defoliators, including H. armigera. However, transgenic crops that have foreign DNA randomly integrated into their genomes have always been controversial, owing to the durability of resistance, environmental safety, and potential adverse health effects on consumers, limiting such transgenic breeding (Domingo and Bordonaba, 2011; Raman, 2017; Schiemann et al., 2019; Pozebon et al., 2020; Gao, 2021).
In addition to the deployment of genetically modified soybean varieties that overexpress one or more insecticidal protein genes, other soybean varieties have been screened for native resistance to leaf-chewing insects, such as H. armigera (Haile et al., 2021). Zhu et al. (2006) fine-mapped a major quantitative trait locus (QTL-M) for insect resistance in PI 229358 and developed Benning near-isogenic lines (NILs) resistant to H. zea. Similarly, several studies have reported insect-resistance QTLs against S. litura (Kim et al., 2013, 2015; Liu et al., 2016). Ghione et al. (2021) found four markers associated with resistance genes against stink bugs using the association mapping strategy. To date, several insect-resistance QTLs, specific for leaf-chewing insects, have been identified in the soybean germplasm (Rector et al., 2000; Zhu et al., 2008; Wang et al., 2015; Ortega et al., 2016; Sabljic et al., 2020; Ghione et al., 2021; Lucini et al., 2021). Interestingly, Ortega et al. (2017) reported that the Glyma.07g110300 marker (encoding a UDP-glycosyltransferase) in QTL-M was a functional single-nucleotide polymorphism (SNP) that could be used for the marker-assisted selection of insect-resistance QTLs and Glyma.07g110300 exhibits a loss of function in insect-resistant soybean germplasms. Native resistance traits have been reported against H. zea, but information about the GmUGT gene and its roles in resistance to H. armigera remains unclear.
The CRISPR/Cas9 (clustered regularly interspaced short palindromic repeat-associated endonucleases) is an efficient tool for genetic manipulation; it uses simple RNA-guided DNA recognition and binding for sequence-specific nucleic acid cleavage. The CRISPR/Cas9-based genome-editing can help produce crop varieties, similar to non-transgenic crops, without adding any foreign DNA to the genome, and thus, has become a powerful tool to advance crop breeding (Knott and Jennifer, 2018; Chen et al., 2019; Schiemann et al., 2019; Gao, 2021). This technology has been widely applied in soybeans (Bao et al., 2019; Cheng et al., 2019; Han et al., 2019; Cai et al., 2020). Therefore, CRISPR/Cas9-mediated construction of insect-resistant germplasms to reduce the dependence on pesticides has the potential to become a simple and feasible breeding method for soybeans in the future.
In this study, we employed the CRISPR/Cas9 system to create GmUGT-gene knockout mutants and analyzed the change in resistance of the mutant to leaf-chewing insects. The H. armigera and S. litura larval feeding assay demonstrated that the mutagenesis of GmUGT-improved soybean resistance against leaf-chewing insects by altering the biosynthesis pathway of flavonoids in soybeans. Furthermore, the ectopic expression of the GmUGT gene substantially rescued the phenotype of resistance against H. armigera in the atugt72b1 mutant. Findings from this study present a strategy to increase the resistance to leaf-chewing insects in soybean and other crops through CRISPR/Cas9-mediated targeted mutagenesis of UGT genes.
Materials and Methods
Plant Materials and Growth Conditions
Soybean cultivar Tianlong No. 1 (WT), as well as transgenic varieties generated by the CRISPR/Cas9-mediated mutagenesis of GmUGT gene and GmUGT-overexpression, were grown in pots (height × top diameter = 18.5 cm × 18.5 cm). Three plants per pot were grown in an artificial climate chamber under 14-h light/10-h dark photoperiod conditions at 26°C. Arabidopsis thaliana Columbia-0 (Col-0) and the T-DNA insertion mutant, atugt72b1, were obtained from the Arabidopsis Biological Resource Center (ABRC). The Col-0, atugt72b1, and transgenic Arabidopsis lines were grown in pots (height × top diameter = 10 × 8 cm) with four plants per pot in an artificial climate chamber under 8-h light/16-h dark photoperiod conditions at 22°C.
Vector Constructs and Plants Transformation
To edit the GmUGT gene, the pSC1-CRISPR/Cas9PGmUbi3-BK plasmids were constructed, following the method of Du et al. (2016). Briefly, the target adaptor was designed using the CRISPR-P1 web tool; it was then integrated into the single guide RNA (sgRNA) expression cassettes driven by the GmU6 promoter, which was subsequently built into the pSC1-CRISPR/Cas9PGmUbi3-BK vector. The full-length coding sequence (CDS) of GmUGT was cloned from the Tianlong No. 1 complementary DNA (cDNA) library. The GmUGT CDS was subcloned into the pB2GW7.0 binary vector (Shen et al., 2018) through the LR recombinase reaction (Invitrogen, Carlsbad, CA, United States). The constructs, P35S-GmUGT-pB2GW7.0 and PGmU6-sgRNA-pSC1-CRISPR/Cas9PGmUbi3-BK, were introduced into Agrobacterium tumefaciens EHA105 by electroporation and then transformed into the WT as previously described (Chen et al., 2021).
For Arabidopsis transformation, the GmUGT gene was subcloned into pFGC5941 (Lei et al., 2021) driven by the ATUGT72B1 promoter using the ClonExpress II One Step Cloning Kit (Vazyme, Nanjing, China); the clone, thus, prepared was introduced into the Arabidopsis atugt72b1 mutant with EHA105 by the floral dip method (Clough and Bent, 1998).
Identification of Transformants and Mutations
For PCR, genomic DNA was extracted from the leaves of the WT plants and the transgenic varieties generated by the CRISPR/Cas9-mediated mutagenesis of GmUGT and GmUGT-overexpression. Transgenic plants were tested with a Transgenic Plant Bar Gene Rapid Detection Kit (Institute of Oil Crops, Chinese Academy of Agricultural Sciences, Wuhan, China).
The PCR products were separated by electrophoresis on 1% agarose in 1 × Tris-acetate-EDTA (TAE) buffer to screen the GmUGT mutants. The purified DNA fragments with Bar gene detection primers were sequenced and analyzed. To identify mutations in the regenerated plants, amplified DNA surrounding the target regions of the sgRNA was sequenced and analyzed. The successfully edited types were identified by sequence alignment with the WT DNA sequence. The PCR identified GmUGT-overexpressing transgenic soybean plants with vector detection primers. The real-time quantitative PCR (qRT-PCR) was used to determine the expression levels of GmUGT in overexpressing transgenic lines. The GmSKIP16 gene was used as the internal control in qRT-PCR analysis. Rescued lines of Arabidopsis were selected on the soil by spraying Basta at 1:1000 concentrations (Bayer CropScience, Monheim, Germany). The qRT-PCR was used to determine the expression levels of GmUGT in transgenic Arabidopsis lines. The AtACTIN2 gene was used as the internal control in qRT-PCR analysis.
Detection of Off-Target Sites
To consider the off-target effects, the potential off-target sites were predicted by the website CCtop2. The overlapping sequence between the similar position of the off-target site gene (Glyma.09G245300) and the sgRNA was 16-bp long. This gene was checked by PCR amplification and sequencing. Off-target site detection primers are listed in Supplementary Table 1.
Analysis of Insect Resistance in Mutant and Transgenic Lines
For the larval feeding assay, soybean plants were grown until they had five fully unfolded leaves. Arabidopsis plants were grown until they had 10 fully unfolded leaves. The H. armigera and S. litura larvae obtained from Huazhong Agricultural University were hatched at 28°C from a single egg mass. To analyze insect resistance in the WT plant and the transgenic varieties from CRISPR/Cas9-mediated mutagenesis of the GmUGT gene and GmUGT overexpression, leaves were collected from the same positions. Four small rounded blades (each with a diameter of 4 cm) were collected from each leaf with a hole puncher. The leaves were placed in a Petri dish (90 × 15 mm) containing two pieces of soaked filter paper. A second instar larva was placed on each leaf. Three days after the treatment, representative photos were taken, and the leaf area of soybean varieties was measured using a leaf area meter. After feeding for 7 days, the weight per larvae was recorded. Two knockout mutants (ko-3 and ko-5), three overexpressing transgenic lines (OX-1, OX-2, and OX-44), and the WT plants underwent fifty biological replicates. For the analysis of insect resistance in Arabidopsis, two whole plants were used as one sample. Plants were wrapped in moistened absorbent cotton and placed in 500 mL round disposable plastic boxes with 100 freshly hatched larvae. Three days after treatment, representative photos were taken. After feeding for 7 days, the weight per larvae and the number of surviving larvae were recorded. Three transgenic lines of soybean (RE-1, RE-2, and RE-3), atugt72b1, and Col-0 were tested in three biological replicates.
Expression Pattern Analysis of GmUGT
The tissues of the WT plants were tested at different stages to analyze the expression pattern of GmUGT in soybean. Cotyledons, roots, simple leaves, trifoliate leaves, and stems were harvested from the WT at the V2 stage, flowers were harvested at the R2 stage, and pods at the R5 stage for RNA purification. The harvested samples were stored at −80°C until total RNA extraction was performed for gene expression analyses.
For the induced expression pattern analysis, 10 H. armigera larvae were placed on the first and second trifoliate leaves of the WT at the V4 stage. The leaves of the control and treated plants were excised at nine sampling times (0, 1, 2, 3, 6, 12, 24, 36, 48, or 72 h) after being attacked by H. armigera to identify the induced resistance. For mechanical damage treatment, the damage was simulated using a needle with 48 spines per leaf. A mix of damaged and undamaged leaves was collected. The H. armigera attack and mechanical damage treatments were performed in biological triplicates.
RNA Isolation and Real-Time Quantitative PCR Analysis
Total RNA was extracted from plant tissues using TRIzol reagent (Invitrogen, Carlsbad, CA, United States). The RNA concentration was measured using NanoDrop One (Thermo Scientific, Waltham, MA, United States) for qRT-PCR and RNA sequence (RNA-seq) assays. One microgram of total RNA per sample was used to synthesize the first-strand of cDNA using the TransScript® First-Strain cDNA Synthesis SuperMix according to the manufacturer’s instructions (TransGen Biotech, Beijing, China). A total volume of 20 μL was set up for the qRT-PCR that contained 0.5 μM forward and reverse primers, 10 μL of the SYBR premix, and 20 ng of the cDNA. The assay was performed in a QuantStudio™ 5 Real-Time PCR Instrument (Thermo Scientific, Waltham, MA, United States) under the following cycle conditions: 95°C for 30 s, 40 cycles of denaturation at 95°C for 5 s, annealing at 60°C for 30 s, and extension at 72°C for 30 s with simultaneous fluorescence measurement and a melting curve at 55–95°C (0.5°C increments with each cycle). Three biological replicates were assayed. The GmSKIP16 (Glyma.12g05510), AtACTIN2 (At3G18780), and HaActin (GU182917) were used as endogenous controls for experiments involving nucleic acids from Arabidopsis, soybean, and H. armigera, respectively. The expression levels of the target genes were quantified using the 2–ΔΔCt method (Livak and Schmittgen, 2001). Unless otherwise indicated, the wild-type untreated samples were set to a relative value of 1. All the primers used for qRT-PCR are listed in Supplementary Table 1.
Subcellular Localization of GmUGT-YFP Fusion Proteins
The full-length CDS of GmUGT was amplified with two primers, GmUGT–CDS-F/R (Supplementary Table 1). The PCR product was subcloned into the pEarlyGate 101 vector (Deguchi et al., 2020) under the control of the CaMV35S promoter using the ClonExpress II One Step Cloning Kit (Vazyme, Nanjing, China). This yielded a pEarlyGate101-GmUGT-YFP construct, which was transformed into Agrobacterium strain GV31011 by electroporation. The Nicotiana benthamiana plants were grown in a plant growth chamber at 26°C under a 16 h/8 h (light/dark) regimen until they reached heights of approximately 10–20 cm. The plantlets were then infiltrated with Agrobacterium strain GV3101 containing pEarlyGate101-GmUGT-YFP. Infiltration was performed as described by Bai et al. (2013). The YFP alone was used as the control, and FIBRILLARIN2-mCherry (FIB2-mCherry) was used as a nucleolar marker (Missbach et al., 2013). The agroinfiltrated leaves were photographed 72 h after infiltration. The fluorescing fusion protein was visualized by confocal microscopy with excitation at 520 nm for YFP and 570–620 nm for FIB2-mCherry.
Metabolomics Analysis
For the metabolomics analysis, samples were isolated from the WT and the ko-3 leaves; these leaves included unattacked and attacked samples by H. armigera for 36 h and were taken from the same place on the plants. Nine fully expanded leaves from nine independent plants were pooled as one composite biological replicate. Three biological replicates were performed. Biological samples were extracted, and the sample extracts were analyzed using a ultra-performance liquid chromatography with electrospray ionization tandem mass spectrometry (UPLC–ESI–MS/MS) system as described previously (Chen et al., 2013). Significantly regulated metabolites between groups were determined by variable importance in projection (VIP) ≥ 1 and absolute log2 fold change (log2FC) ≥ 1. The VIP values were extracted from the Orthogonal Partial Least Squares Discrimination Analysis (OPLS-DA) results, which contained scores and permutation plots generated using the R package, MetaboAnalystR 3.03. The data were log-transformed (log2) and mean-centered before OPLS-DA. Identified metabolites were annotated using the KEGG Compound database4, and annotated metabolites were then mapped to the Kyoto Encyclopedia of Genes and Genomes (KEGG) Pathway database5. Pathways with significantly regulated metabolites were mapped and then fed into metabolite set enrichment analysis (MSEA). Their significance was determined by the p values of the hypergeometric tests (Thevenot et al., 2015; Zhang X. L. et al., 2017).
RNA-Seq and Bioinformatics Analysis
Six fully expanded leaves from three independent WT and ko-3 plants after H. armigera larval attack and unattack were pooled as a single biological replicate. The three biological replicates were used for total RNA extraction followed by RNA-seq analysis. The RNA-seq experiment and preliminary data analysis were carried out by the Beijing Biomarker Technology Corporation (Biomarker, Beijing, China). The total RNA was extracted, and sequencing libraries were generated as previously described (Kou et al., 2021). Clean reads were mapped to the soybean reference genome (G. max Wm82.a2.v1) downloaded from Phytozome using SOAP aligner/SOAP2. Differentially expressed genes (DEGs) relative to control samples were identified with the absolute value of | log2 FC| ≥ 1 and false discovery rate (FDR) <0.5 as the threshold. The KEGG pathway enrichment of the DEGs was generated using R using enrichment factors, Q-values, and the number of enriched genes in this pathway (Chen et al., 2020).
Statistical Analysis
All data were collected and analyzed using Microsoft Office Excel 2010. All data analyses were performed by Student’s t-test to compare the means of two samples (or treatments) to determine significant differences. Unless otherwise specified, experiments were conducted in triplicates. The values are given as mean ± SD. The consistency between RNA-seq and qRT-PCR analyses was evaluated by the Pearson correlation method based on log2FC. Pearson correlation analyses were performed using GraphPad Prism 8.0 software (GraphPad Software, San Diego, CA, United States).
Results
CRISPR/Cas9-Mediated Mutagenesis of GmUGT in Soybean Enhanced Resistance to Helicoverpa armigera and Spodoptera litura
To better understand the GmUGT gene and its function in soybean resistance against leaf-chewing insects, Agrobacterium-mediated transformation was used to introduce the CRISPR/Cas9 expression vector into the soybean cultivar Tianlong No. 1 (wild type, WT). This generated mutants of the Glyma.07g110300 gene with a guide RNA targeting GmUGT (Figures 1A,B). We obtained four T0 transgenic lines with insertions of the Bar gene (Supplementary Figures 1A,B). Several T1 generations were sequenced to identify the CRISPR/Cas9-induced mutant lines, and five edited mutants were identified (Figures 1C–G and Supplementary Figure 1C). The ko-1, ko-2, ko-3, and ko-4 mutants had 4-bp, 6-bp, 33-bp, and 65-bp deletions, respectively, while ko-5 had a single-bp insertion in the target site, resulting in altered amino acid sequence or frameshift mutations in the protein encoded by GmUGT (Figures 1C–G and Supplementary Figure 2A). Due to the possibility of off-targeting, potential off-target sites predicted by the website CCtop6 were also sequenced to detect all cleavages in the mutants (Supplementary Figure 2B). We did not observe a significant difference in plant growth and development between the five homozygous mutants and the WT plants (Supplementary Figure 1C). The ‘transgene-clean’ homozygous ko-3 and ko-5 mutants were used for further analysis (Supplementary Figures 1D–F).
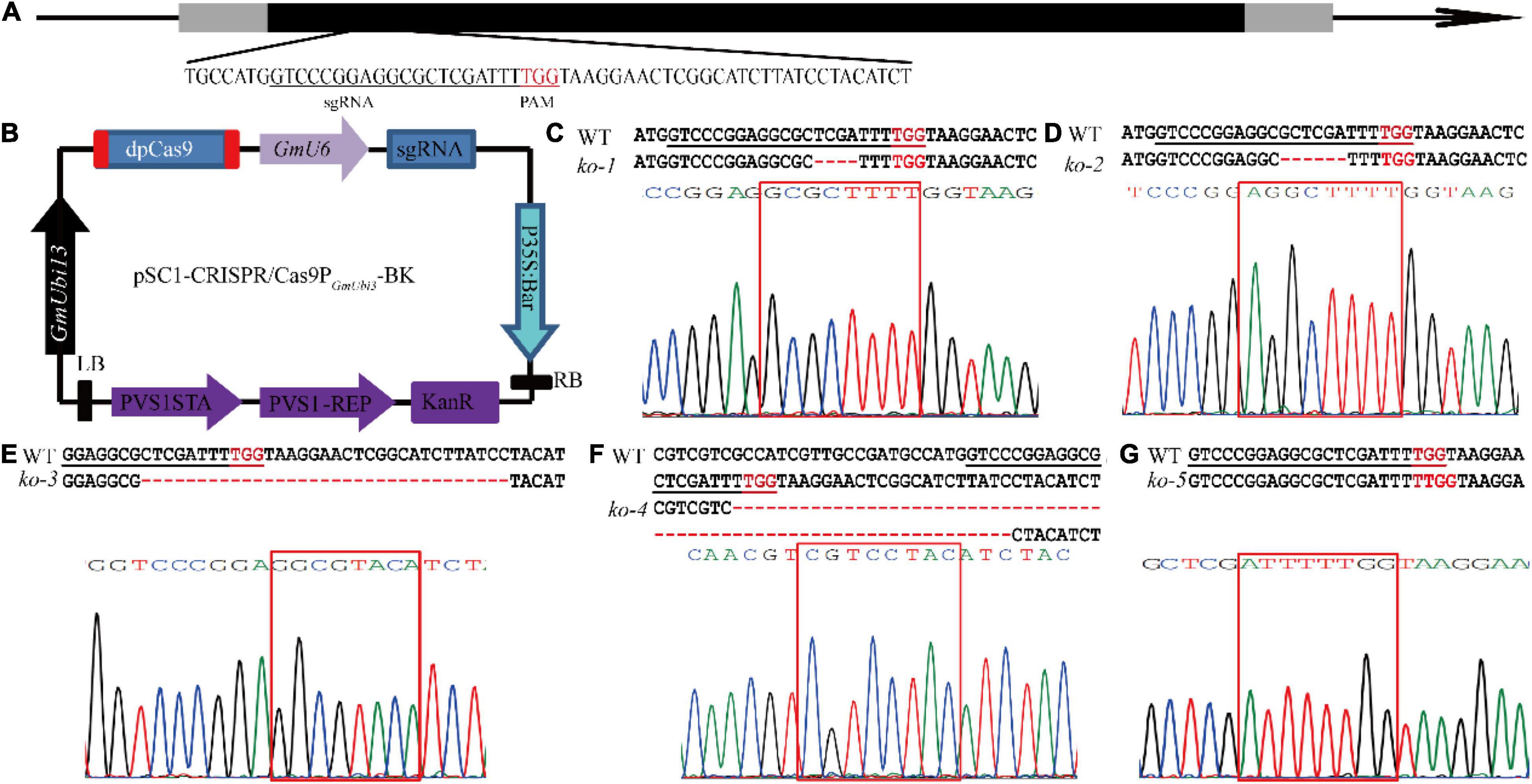
Figure 1. Target site in the GmUGT gene and results obtained from the mutagenesis of GmUGT through CRISPR/Cas9 technology. (A) Schematic figure of the target site in GmUGT. Nucleotides in red represent the protospacer adjacent motif (PAM). Nucleotides underlined indicate the target site. (B) Schematic figure of the binary vector designed for mutagenesis of the GmUGT gene using CRISPR/Cas9 technology. The pSC1-CRISPR/Cas9-PGmUbi3-BK was derived from VK005-04-soU6-2-GmUbi3. The sgRNA with a targeting site was directed by the GmU6 promoter. (C–G) Detailed sequence of the target site in T2 generations of the separated lines. ‘–’ signs indicate the number of deleted nucleotides. The WT represents Tianlong No. 1 wild type sequence.
To test the resistance of the GmUGT mutants to leaf-chewing insects, we fed the larvae of H. armigera and S. litura detached leaves of the ko-3 and ko-5 mutants and the WT plants. Figure 2 shows that both the ko-3 and ko-5 mutants exhibited resistance to H. armigera and S. litura. The leaf area loss in the ko-3 and ko-5 mutants attacked by H. armigera was significantly less than that in the WT plants (Figures 2A,B). The average weight of H. armigera fed with the leaves of ko-3 and ko-5 mutants was also significantly less than that fed with leaves of the WT plants (Figures 2C,D). Similar results were observed when the larvae of S. litura were fed detached leaves of the ko-3 and ko-5 mutants and the WT plants (Figures 2E–H). These results demonstrated that the CRISPR/Cas9-mediated targeted mutagenesis of GmUGT increased resistance against H. armigera and S. litura in soybeans.
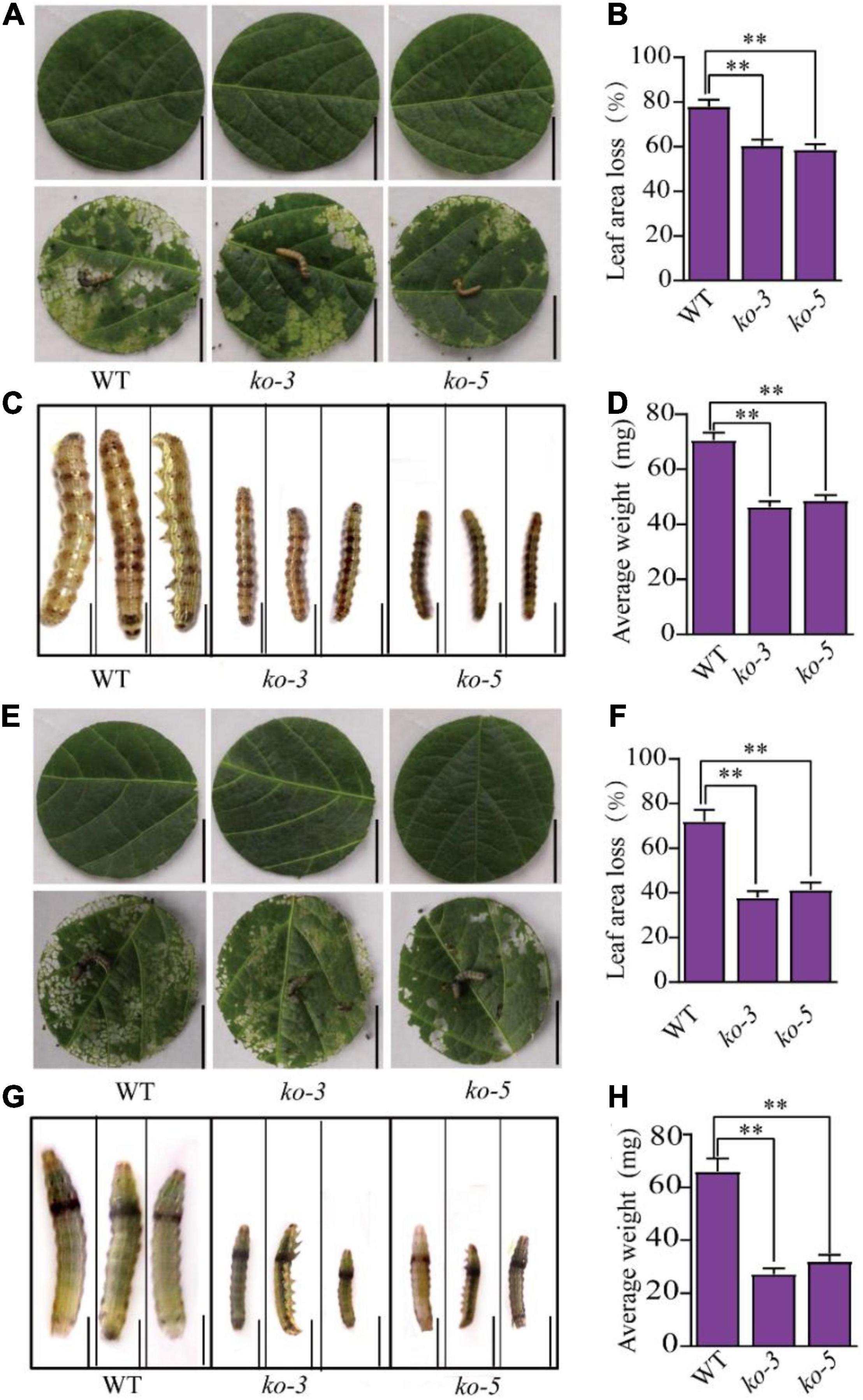
Figure 2. The CRISPR/Cas9-mediated mutagenesis of GmUGT enhanced resistance to H. armigera and S. litura in soybean. (A) The phenotype of detached leaves of ko-3, ko-5, and WT plants attacked by H. armigera for 3 days (bar = 2 cm). (B) Percentage of leaf area loss in ko-3, ko-5, and WT plants attacked by H. armigera for 3 days (n = 50 biological repeats). (C) The phenotype of H. armigera larvae fed detached leaves of ko-3, ko-5, and WT plants for 7 days (bar = 0.2 cm). (D) The average weight of H. armigera larvae that were fed detached leaves of ko-3, ko-5, and WT plants for 7 days (n = 50 larvae). (E) The phenotype of detached leaves of ko-3, ko-5, and WT that was attacked by S. litura for 3 days (bar = 2 cm). (F) Percentage of leaf area loss in ko-3, ko-5, and WT plants attacked by S. litura for 3 days (n = 50 biological repeats). (G) The phenotype of S. litura larvae that were fed detached leaves of ko-3, ko-5, and WT plants for 7 days (bar = 0.2 cm). (H) The average weight of S. litura larvae that were fed detached leaves of ko-3, ko-5, and WT for 7 days (n = 50 larvae). Data shown are means and standard deviations. Statistically significant differences are marked with asterisks (**p < 0.01; Student’s t-test).
Soybean Varieties Overexpressing GmUGT Were More Sensitive to H. armigera and S. litura
Because the GmUGT mutants (ko-3 and ko-5) showed enhanced resistance to leaf-chewing insects, we wondered whether the overexpression of GmUGT would be more sensitive to H. armigera and S. litura. Four transgenic lines that overexpressed the GmUGT gene were generated (OX-1, OX-2, OX32, and OX-44) (Supplementary Figure 3); three of these (OX-1, OX-2, and OX-44) were used to evaluate resistance against H. armigera and S. litura. As expected, all three GmUGT-overexpressing lines were more sensitive to H. armigera and S. litura in soybean (Figure 3). The loss in leaf area in GmUGT-overexpressing lines attacked by H. armigera and S. litura was more significant than in the WT plants. Furthermore, the average weights of larvae fed GmUGT-overexpressing leaves were higher than those that were fed WT leaves (Figure 3). These results suggest that the GmUGT gene compromises the resistance of soybeans to leaf-chewing insects.
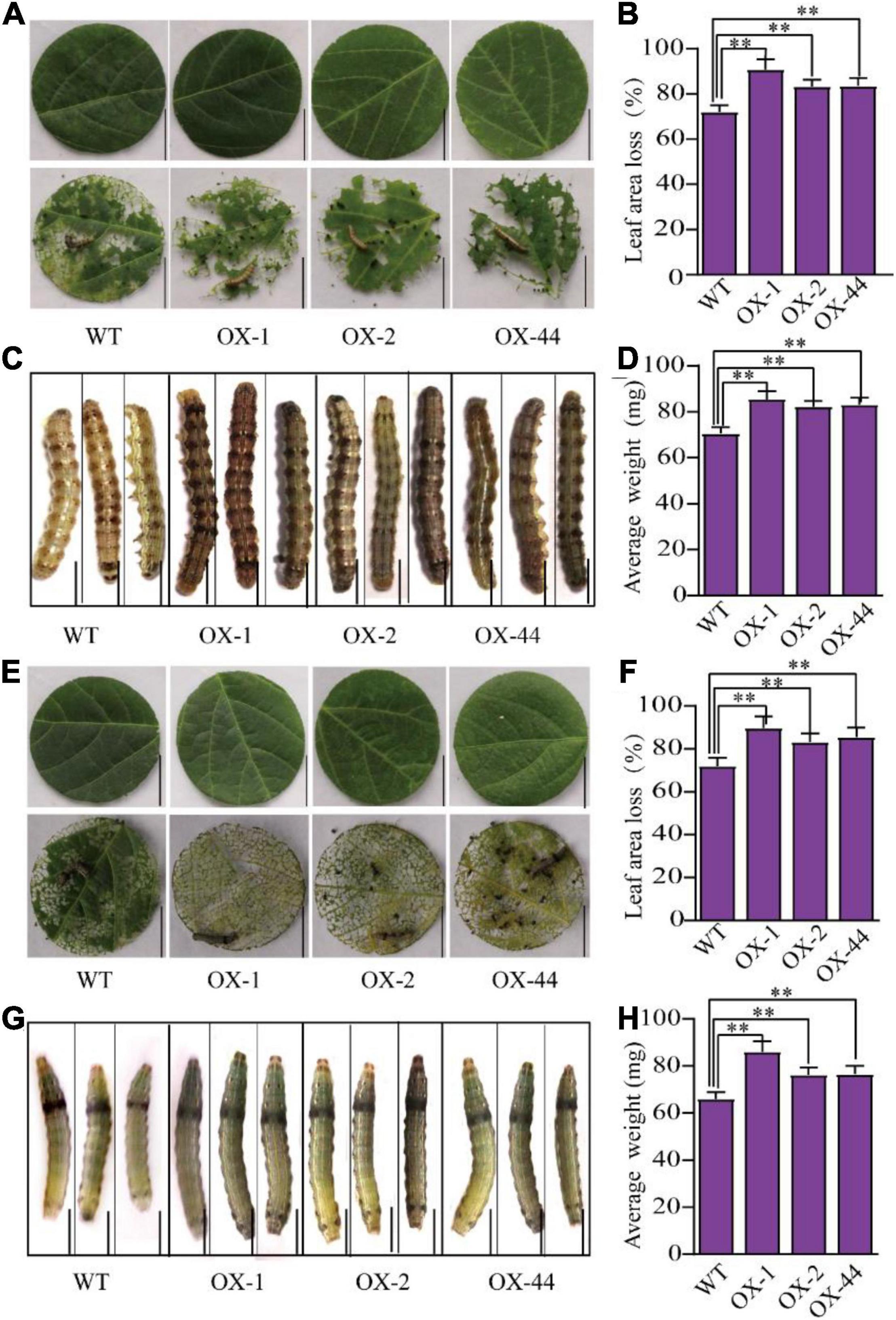
Figure 3. GmUGT-OX transgenic soybean plants were susceptible to H. armigera and S. litura. (A) The phenotype of detached leaves of three GmUGT-OX transgenic plants (OX-1, OX-2, and OX-44) and WT plants attacked by H. armigera for 3 days (bar = 2 cm). (B) Percentage of leaf area loss in OX-1, OX-2, OX-44, and WT plants attacked by H. armigera for 3 days (n = 50 biological repeats). (C) The phenotype of H. armigera larvae that were fed detached leaves of OX-1, OX-2, OX-44, and WT plants for 7 days (bar = 0.2 cm). (D) The average weight of H. armigera larvae that were fed detached leaves of OX-1, OX-2, OX-44, and WT plants for 7 days (n = 50 larvae). (E) The phenotype of detached leaves of OX-1, OX-2, OX-44, and WT plants attacked by S. litura for 3 days (bar = 2 cm). (F) Percentage of leaf area loss in OX-1, OX-2, OX-44, and WT plants attacked by S. litura for 3 days (n = 50 biological repeats). (G) The phenotype of S. litura larvae that were fed detached leaves of OX-1, OX-2, OX-44, and WT plants for 7 days (bar = 0.2 cm). (H) The average weight of S. litura larvae that were fed detached leaves of OX-1, OX-2, OX-44, and WT plants for 7 days (n = 50 larvae). Data shown are means and standard deviations (n = 50). Statistically significant differences are marked with asterisks (**p < 0.01; Student’s t-test).
Characterization of the GmUGT Gene in Soybeans
The tissue expression pattern showed that the GmUGT gene was ubiquitously expressed in all tissues. The expression levels in the flower, simple leaf, trifoliate leaves, and pod were higher than those in other tissues (Figure 4A). Leaves are the favorite food of H. armigera and S. litura larvae. Additionally, Aljbory and Chen (2018) reported that insect attacks can stimulate plant resistance by mechanical damage and/or the application of an elicitor. Thus, we examined the expression patterns of the GmUGT gene in the leaves of WT plants after exposure to H. armigera attacks and mechanical damage at different times. The transcription of GmUGT was induced by H. armigera 1 h after the attack, followed by a gradual increase for 36 h and eventually a decrease after 48 h; contrarily, the expression levels of GmUGT remained unchanged after mechanical damage (Figure 4B). These results indicate that GmUGT is expressed in response to the attack of leaf-chewing insects rather than mechanical damage alone in soybean. Phylogenetic analysis showed that the GmUGT protein shared high sequence homology with ATUGT72B1 in Arabidopsis (Figure 4C), which catalyzes the glucose conjugation of monolignols (Lin et al., 2016). Previous works have reported that genes belonging to the UGT family function as glycosyltransferase in the cytoplasm (Bowles et al., 2005; Sun et al., 2013; Smehilova et al., 2016). To assess the subcellular localization of GmUGT protein, a plasmid carrying GmUGT fused with a yellow fluorescent protein (YFP) gene driven by the CaMV 35S promoter was injected into tobacco leaves. The diffused fluorescence of the fusion protein was observed in the cytosol, accumulating along the cell membrane and in the nuclei (Figure 4D), proving GmUGT to be a cytosolic enzyme.
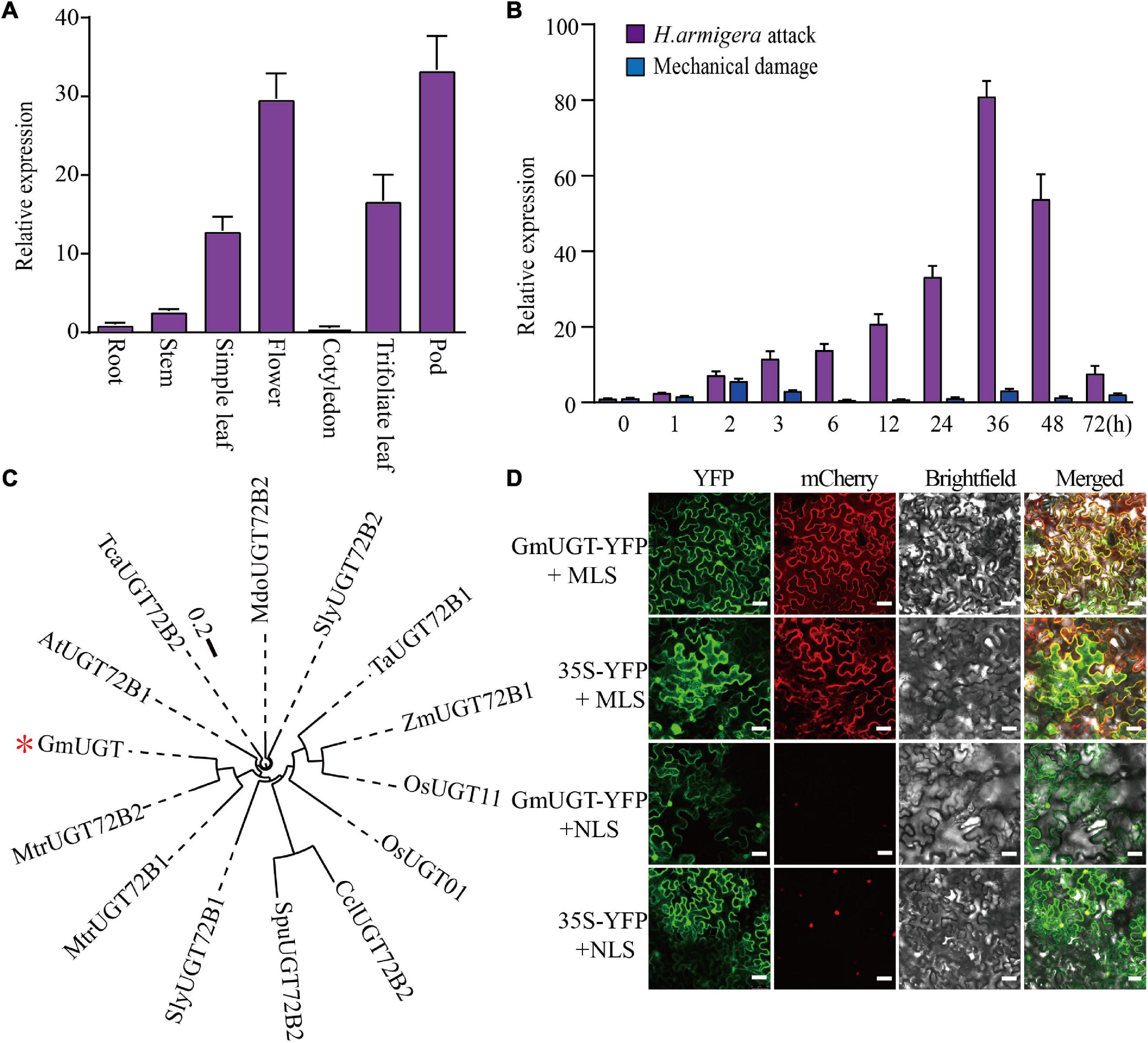
Figure 4. Gene expression analysis, phylogenetic relationship, and localization assay of the GmUGT gene. (A) Expression of GmUGT in roots, stems, simple leaves, flowers, cotyledons, trifoliate leaves, and pods (n = 3 biological repeats). (B) Expression patterns of GmUGT in leaves after attack by H. armigera and mechanical damage (n = 3 biological repeats). (C) Phylogenetic analysis of GmUGT and other UGT proteins from diverse species. The scale bar indicates 0.2 substitutions per site. The accession numbers of these proteins in the GenBank database are as follows: GmUGT (Glyma.07g110300); OsUGT01 (LOC_Os01g43280); OsUGT11 (LOC_Os11g38650); AtATUGT72B1 (AT4G01070); MdoUGT72B2 (MDP0000875654); SlyUGT72B2 (Solyc01g095620.2); SlyATUGT72B1 (Solyc04g080010.2); MtrUGT72B1 (Medtr8g006260); MtrUGT72B2 (Medtr1g019510); ZmATUGT72B1 (Zm00008a014945_T01); TaATUGT72B1 (Traes_6AS_43AF9291); TcaUGT72B2 (Thecc1EG005182t1); SpuUGT72B2 (SapurV1A.00130600); and CclUGT72B2 (Ciclev1000172B2). (D) Subcellular localization of YFP-GmUGT fusion proteins in the tabacum leaf cells. The fluorescence patterns of GmUGT-YFP and YFP (negative control) were visualized with a high-resolution laser confocal microscope. The extreme left panel shows YFP fluorescence, the middle panel shows mCherry and the bright field, and the right panel represents an overlay of the two images, bar = 75 μm. mCherry, FIBRILLARIN2-mCherry nucleolar marker; MLS, membrane localization signal; NLS, nuclear localization signal.
GmUGT Negatively Regulates Flavonoids Content
Because the Arabidopsis ugt72b1 mutant previously displayed an aggravated cell wall lignification and an enhanced flavonoid content (Lin et al., 2016), we investigated whether GmUGT, the homolog of ATUGT72B1, plays a similar role in soybeans. First, we evaluated the lignin in the cell walls of the leaves of the ko-3 and WT plants to find out if they had similar cell wall lignin (Supplementary Figure 4A). To determine the flavonoid content, metabolomics analysis was performed on the ko-3 and WT plants 36 h after attack or unattack by H. armigera; this duration was chosen considering that GmUGT gene expression reached its peak after 36 h of attack by H. armigera (Figure 4B). Differential metabolite analysis showed that 70 metabolites in unattacked ko-3 plants mapped to 14 KEGG pathways, while 56 metabolites in the attacked ko-3 mapped to 10 KEGG pathways (Supplementary Tables 2, 3 and Figures 5A,B). The GmUGT encodes a UDP-7-O-glucosyltransferase, which catalyzes the final step of flavonoid biosynthesis to form their glycoside derivatives. Loss of function of GmUGT resulted in increased daidzein and formononetin contents, while genistein content was reduced due to the redirection of metabolic flux to other products (Supplementary Figures 4B–D). Among these KEGG pathways, isoflavonoid and flavonoid biosynthesis were significantly enriched in the ko-3 plants attacked by H. armigera after 36 h (Figures 5A,B). Different flavonoid metabolites were upregulated in the ko-3 mutants 36 h after attack by H. armigera (Figure 5C). These were related to insect resistance, including 7,4-dihydroxyflavone, eupatilin, isoformononetin, ononin, and daidzein (Figure 5D). Furthermore, we tested the expression levels of 12 immunity-related genes in H. armigera larvae that were fed ko-3 plant leaves for 7 days to observe that the expression of most of these genes was significantly decreased (Supplementary Figures 4E–O). These results indicate that GmUGT mutagenesis affects the flavonoid content in soybean attacked by H. armigera.
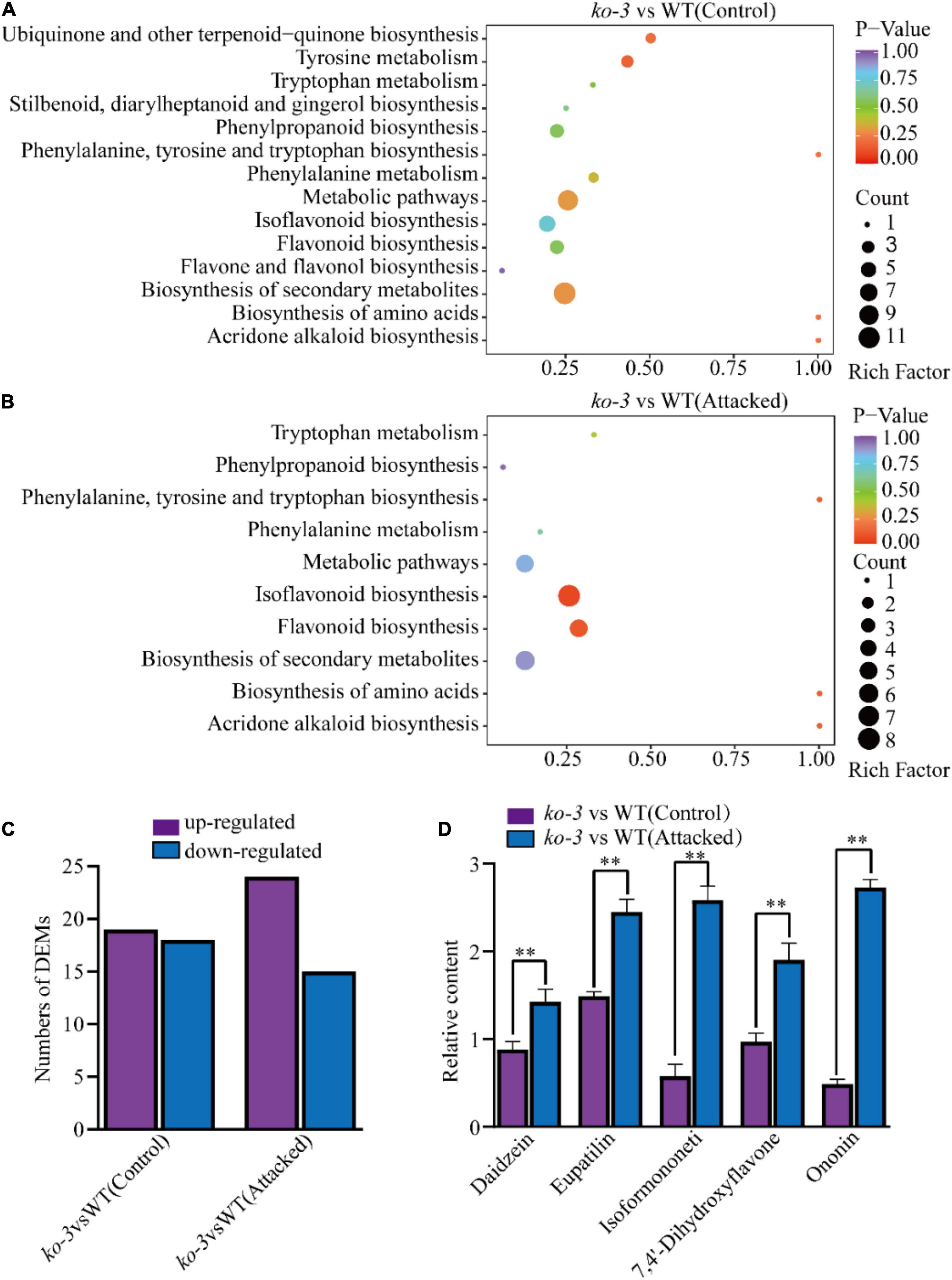
Figure 5. Analysis of different metabolites in ko-3 versus WT unattacked and attacked by H. armigera at 36 h. KEGG pathway enrichment of different metabolites in ko-3 versus WT plants unattacked by H. armigera. (A), and attacked by H. armigera at 36 h (B). Each dot represents a KEGG pathway. The Y-axis indicates the KEGG pathway, and the X-axis indicates the rich factor (indicating the degree of differential metabolite enrichment in each pathway; the larger the rich factor is, the greater the differential metabolite enrichment). The dot size and color indicate the number and p value of different metabolites in the pathway, respectively, and a larger dot means more different metabolites. (C) The number of different metabolites of flavonoids among ko-3 versus WT unattacked and attacked by H. armigera at 36 h. (D) Relative content of metabolites in ko-3 unattacked and attacked by H. armigera at 36 h. The data shown are the means and standard deviations (n = 3 biological repeats). Statistically significant differences are marked with asterisks (**p < 0.01; Student’s t-test).
Differently Expressed Genes in GmUGT Mutants and Wild Type Plants
A transcriptome assay was conducted on leaves from the WT, ko-3 plants that were attacked for 36 h by H. armigera, and an unattacked control to identify genes related to resistance against H. armigera. The most enriched DEGs in ko-3 according to the KEGG pathways were associated with isoflavonoid and flavonoid biosynthesis, defensive genes related to plant–pathogen interaction, and mitogen-activated protein kinase (MAPK) signaling pathway (Figures 6A,B, Supplementary Figure 5, and Supplementary Tables 4, 5). The qRT-PCR analysis was employed to investigate the expression of genes involved in flavonoid biosynthesis, including G. max CHALCONES SYNTHASE (GmCHS), G. max Chalcone reductase (GmCHR), and G. max Cytochrome P450 monooxygenase (GmCYP81E11). All three genes were upregulated in ko-3 as compared to in the WT plants attacked by H. armigera (Figures 6C–F). Additionally, genes associated with the defense response were assayed, including G. max MITOGEN-ACTIVATED PROTEIN KINASE (GmMAPK) and G. max PATHOGENESIS RELATED1 (PR1). These genes were also upregulated in ko-3 as compared to in the WT plant attacked by H. armigera (Figures 6G–K). The consistency between RNA-Seq and qRT-PCR analysis was evaluated by Pearson’s correlation (R) analysis based on log2FC revealing an R = 0.82 and p < 0.0001, which proved the reliability of the RNA-Seq data (Supplementary Figure 5C). These data indicated that the knockout of GmUGT enhanced the resistance of soybean against leaf-chewing insects by altering genes related to the flavonoid pathway and defense responses.
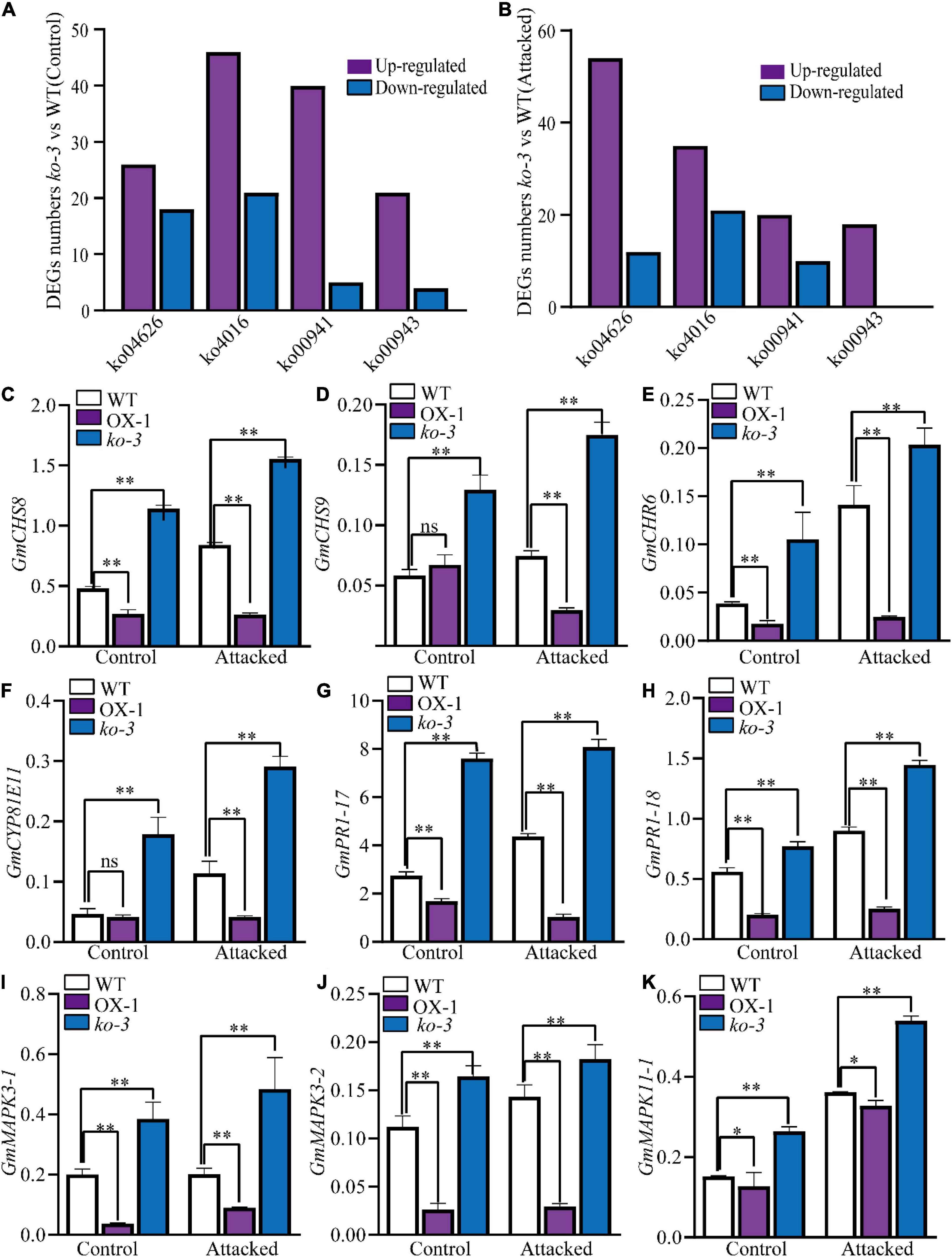
Figure 6. Analysis of differentially expressed genes (DEGs) in ko-3 versus WT plants unattacked and attacked by H. armigera at 36 h. The numbers of DEGs in ko-3 versus WT plants unattacked by H. armigera (A) and attacked by H. armigera at 36 h (B). (C–K) Validation of the expression levels of nine DEGs by qRT-PCR. Relative expression levels were normalized to the expression level of GmSKIP16. The data shown are the means and standard deviations (n = 3 biological repeats). Statistically significant differences are marked with asterisks (ns p > 0.05, 0.01< *p < 0.05, **p < 0.01; Student’s t-test; ns, non-significant).
Complementation of Resistance to Leaf-Chewing Insects of Arabidopsis ugt72b1 Mutant With GmUGT
Because the GmUGT protein shared high homology with the ATUGT72B1 protein (Figure 4C), we hypothesized that the loss-of-function in the atugt72b1 mutant would show increased resistance to leaf-chewing insects. The larval feeding assay on the atugt72b1 mutants and the WT plants was performed using the larvae of H. armigera. As expected, the atugt72b1 mutant showed higher resistance to H. armigera than the WT plants (Supplementary Figure 6). To further validate the role of GmUGT in imparting resistance to H. armigera, we introduced the GmUGT gene driven by the ATUGT72B1 promoter into the atugt72b1 mutant and obtained three transgenic lines (RE-1, RE-2, and RE-3) with elevated expression of GmUGT in Arabidopsis (Figure 7A). All three transgenic lines showed a substantial reduction in resistance to H. armigera (Figure 7), indicating that the UGT gene family is consistently involved in imparting resistance to H. armigera.
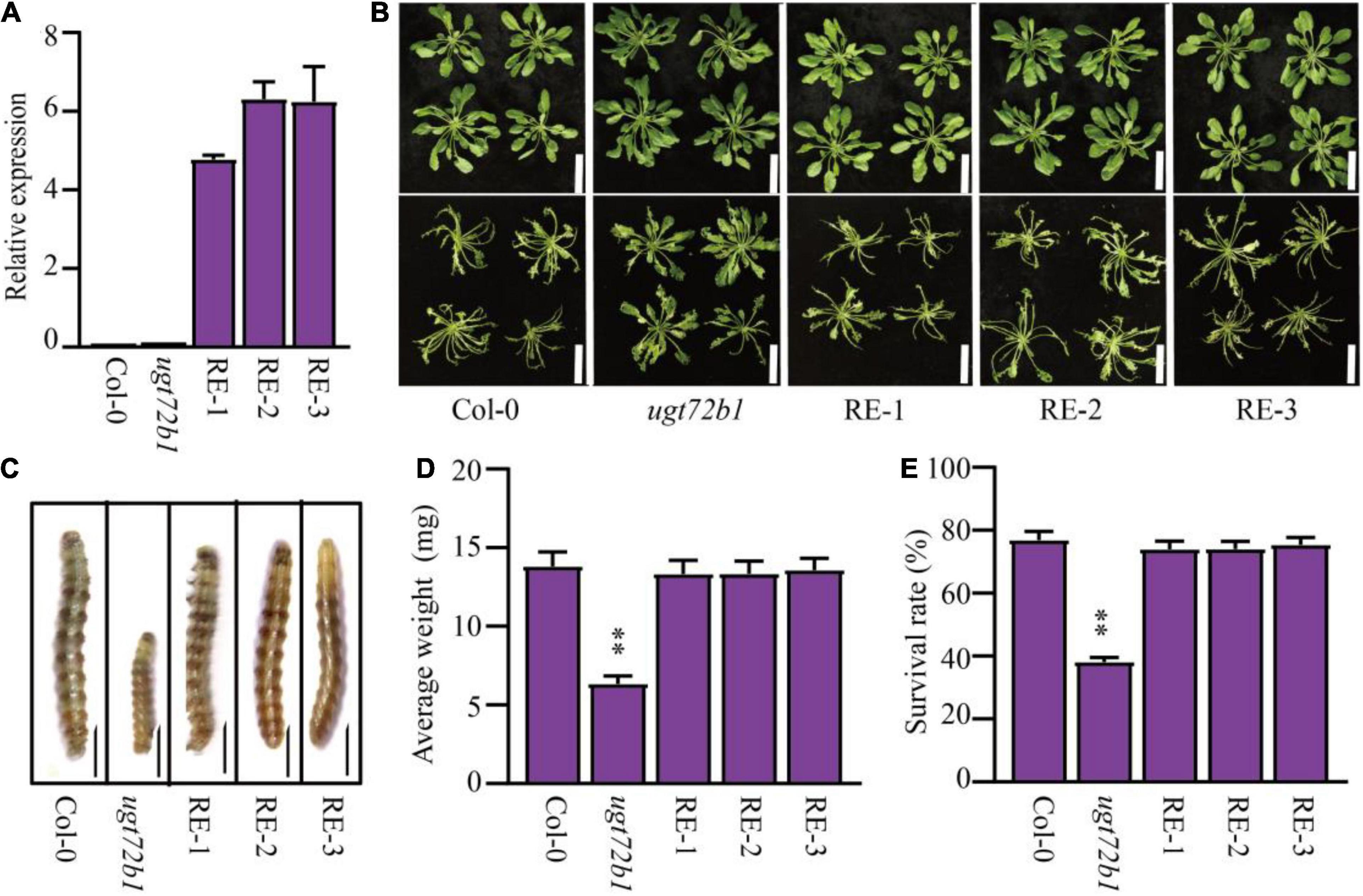
Figure 7. Complementation of resistance to H. armigera of Arabidopsis atugt72b1 mutant with GmUGT. (A) Confirmation of the expression of GmUGT in transgenic Arabidopsis atugt72b1 mutants. Gene expression levels were normalized with Col-0 (AtUGT72B1 expression level) as 1. AtACTIN2 was used as the internal reference control. The data shown are the means and standard deviations (n = 3 biological repeats). (B) Phenotype of Col-0, atugt72b1 and three transgenic lines (RE-1, RE-2 and RE-3) attacked by H. armigera for 3 days (bar = 2 cm). (C) Phenotype of H. armigera larvae fed Col-0, atugt72b1 and three transgenic lines for 7 days (bar = 0.1 cm). (D) Average weight of H. armigera larvae fed Col-0, atugt72b1 and three transgenic lines for 7 days. Data shown are the means and standard deviation (n = 60 larval). (E) The survival rate of H. armigera larvae fed Col-0, atugt72b1 and three transgenic lines for 7 days (n = 3 biological replicates, and 100 H. armigera larvae in each replicate). Statistically significant differences are marked with asterisks (ns p > 0.05, **p < 0.01; Student’s t-test; ns, non-significant).
Discussion
Defoliator insects affect soybean yield and quality, including H. zea, H. armigera, and S. litura (Otuka et al., 2020; Haile et al., 2021). Soybean varieties have been screened for native resistance to such defoliators (Kim et al., 2013, 2015; Liu et al., 2016; Haile et al., 2021). However, breeding such varieties can be labor-intensive and time-consuming (Ortega et al., 2016). Transgenic breeding is a crucial breakthrough in crops breeding for insect resistance. Nevertheless, only six commercialized soybean cultivars with various insect-resistance genes have been identified (Kumar et al., 2020). Among these soybean cultivars, genetically modified cultivars expressing one or more Bacillus thuringiensis (Bt) proteins have been widely deployed for pest management (Kumar et al., 2020). This trait provides effective control management for defoliators, including H. armigera. Similar to the transgenic crops randomly integrating foreign DNA into plant genomes; the durability, environmental safety, and potential adverse health effects of the Bt transgenic soybeans have always been controversial, limiting the transgenic breeding (Gao, 2021). Recently, CRISPR/Cas9-based genome editing has become popular as it allows the generation of crop varieties similar to non-transgenic crops without the addition of any foreign DNA to the genome (Bao et al., 2019). Here, we presented a strategy to increase the resistance against leaf-chewing insects in soybean through CRISPR/Cas9-mediated targeted mutagenesis of the UGT genes through the flavonoid biosynthesis pathway, which can help to accelerate the breeding process for insect resistance and minimize the great concern to human health.
Ortega et al. (2017) reported that GmUGT is a functional SNP marker that exhibits activity against H. zea, but information about the GmUGT gene and its role in imparting resistance to H. armigera remains limited. Here, we first characterized the GmUGT gene in soybean and observed that it could respond to defoliator attacks rather than mechanical damage (Figure 4B). We observed that the GmUGT gene possesses a conserved plant secondary product, the glycosyl-transferase-box (PSPG) motif in its C-terminus (Supplementary Figure 7). The PSPG motif is involved in the recognition and binding of sugar donors (Masada et al., 2007). Additionally, the UGT protein can transfer glycosyl moieties from UDP-sugars to flavonoids during flavonoid biosynthesis (Witte et al., 2009). In soybeans, Yin et al. (2017) identified 212 UGT genes and reported that several of them were potentially involved in flavonoid glycosylation. Here, we found that the CRISPR/Cas9-mediated mutagenesis of GmUGT led the transgenic plant to exhibit an enhanced resistance to H. armigera and S. litura (Figure 2). The Arabidopsis ugt72b1 mutant showed aggravated cell wall lignification and an enhanced flavonoid content (Lin et al., 2016). Both cell wall lignification and flavonoids contribute to resistance against leaf-chewing insects. Cell wall lignification is the first physical barrier against leaf-chewing insects (War et al., 2012). As a secondary metabolite, flavonoids protect plants against leaf-chewing insects by influencing insect behavior, growth, and development (Belete, 2018). Recently, Yang et al. (2021) reported that CRISPR/Cas9-mediated targeted mutagenesis of the OsUGT707A3 gene in rice (Oryza sativa) led to more accumulation of naringenin, lowered naringenin-7-O-b-D-glucoside and apigenin-7-O-b-D-glucoside, and increased the resistance to insects, such as S. litura. Thus, we investigated whether the enhanced resistance against leaf-chewing insects due to GmUGT mutations was attributable to aggravated lignification of cell walls and alteration of flavonoids. Our results indicated that the leaf cell wall lignification and GmUGT mutations were not significantly related (Supplementary Figure 4A).
Metabolomics analysis revealed that loss of function of GmUGT altered flavonoids in soybean leaves (Figure 5). The expression levels of immunity-related genes in H. armigera larvae, including pattern recognition receptors (PRRs) and antimicrobial peptides (AMPs) (Wang et al., 2010), decreased significantly in H. armigera larvae that were fed with ko-3 plant leaves for 7 days, indicating that long-term feeding with ko-3 plant leaves may destroy the immune systems of larvae (Supplementary Figures 4E–O). Therefore, the enhanced resistance of soybean due to GmUGT mutations against leaf-chewing insects may be dependent on altered flavonoids.
Transcriptome assay and qRT-PCR analysis showed that the genes involved in flavonoid biosynthesis were upregulated in ko-3 plants after the H. armigera attack. Previous studies have shown that the genes encoding the CHS, CHR, and CYP81E11 enzymes play a critical role in flavonoid biosynthesis (Dhaubhadel et al., 2007; Zhang X. B. et al., 2017; Mameda et al., 2018; Pi et al., 2019; Zuk et al., 2019). One of the earliest signaling events after leaf-chewing insect attack is the activation of mitogen-activated protein kinases (MAPKs), which are essential to activate the transcription of defense-related genes and accumulate associated metabolites in plants. For example, MAPKs can upregulate the defense gene, GmPR-1, when plants are attacked by insects (Hettenhausen et al., 2015; Giacometti et al., 2016; Breen et al., 2017; Fabricio and Venancio, 2021). Additionally, flavonoids that function as signal molecules may directly interfere with MAPK activities and profoundly affect signaling systems (Agati et al., 2013). Consistent with this, we found out that several genes that were involved in the MAPK pathway, along with the GmPR-1 genes, were upregulated in ko-3 compared with the WT plants upon attack by H. armigera (Figure 6). These results indicate that the GmUGT gene plays an essential role in controlling resistance to leaf-chewing insects through the flavonoid biosynthesis pathway in soybeans.
Interestingly, the Arabidopsis ugt72b1 mutant also exhibited increased resistance to H. armigera (Supplementary Figure 6), while GmUGT shared high homology with AtUGT72B1 (Figure 4C). Additionally, our results showed that the ectopic expression of the GmUGT gene in the Arabidopsis ugt72b1 mutant substantially lessened the resistance of Arabidopsis ugt72b1 mutant to H. armigera (Figure 7). Lin et al. (2016) reported that ugt72b1 mutant exhibited growth repression after bolting, while the ugt72b1 mutant phenotype was restored to the WT by expression of AtUGT72B1. However, we observed that the Arabidopsis ugt72b1 mutant was not restored to its WT by expressing GmUGT (Supplementary Figure 8). Furthermore, mutations and overexpression of GmUGT did not cause any noticeable phenotypic changes (Supplementary Figures 1C, 3A). These results indicate that the two homologous genes, AtUGT72B1 and GmUGT, are functionally differentiated, but they play a conserved role in imparting resistance to leaf-chewing insects.
Conclusion
The CRISPR/Cas9-mediated mutagenesis of GmUGT increased soybean resistance to H. armigera and S. litura by altering flavonoid content and by changing the expression of genes related to flavonoid biosynthesis and defense response. The atugt72b1 mutant of Arabidopsis also exhibited an increased resistance to H. armiger, while the ectopic expression of the GmUGT gene in this mutant substantially reduced the resistance against H. armigera. Our findings highlight an effective strategy for increasing resistance to leaf-chewing insects in soybean through CRISPR/Cas9-mediated targeted mutagenesis of the UGT genes, which may also work for other crops.
Data Availability Statement
The datasets presented in this study can be found in online repositories. The names of the repository/repositories and accession number(s) can be found below: https://www.ncbi.nlm.nih.gov/, SAMN21448187, SAMN21448188, SAMN21448189, SAMN21448190, SAMN21448191, SAMN 21448192, SAMN21448193, SAMN21448194, SAMN21448195, SAMN21448196, and SAMN21448197.
Author Contributions
YZ, WG, DC, XL, and YJ designed the experiments. YZ, WG, LC, XS, HY, YF, WO, SM, HC, SC, QH, YH, DQ, ZS, ZY, SY, CZ, and XZ performed the experiments and analyzed the data. YZ, WG, DC, and XL wrote the manuscript. All authors contributed to the article and approved the submitted version.
Funding
The research was supported by The National Key Research and Development Program of China (2016YFD0100603); The National Natural Science Foundation of China (32072087); and The Science and Technology Innovation Project of Chinese Academy of Agricultural Sciences, Cultivation of New Science and Technology (2021-2060302-049-022).
Conflict of Interest
The authors declare that the research was conducted in the absence of any commercial or financial relationships that could be construed as a potential conflict of interest.
Publisher’s Note
All claims expressed in this article are solely those of the authors and do not necessarily represent those of their affiliated organizations, or those of the publisher, the editors and the reviewers. Any product that may be evaluated in this article, or claim that may be made by its manufacturer, is not guaranteed or endorsed by the publisher.
Supplementary Material
The Supplementary Material for this article can be found online at: https://www.frontiersin.org/articles/10.3389/fpls.2022.802716/full#supplementary-material
Footnotes
- ^ http://cbi.hzau.edu.cn/crispr/
- ^ https://cctop.cos.uni-heidelberg.de:8043
- ^ https://github.com/xia-lab/MetaboAnalystR
- ^ http://www.kegg.jp/kegg/compound/
- ^ http://www.kegg.jp/kegg/pathway.html
- ^ https://cctop.cos.uni-heidelberg.de:8043
References
Agati, G., Brunetti, C., Ferdinando, M. D., Ferrini, F., Pollastri, S., and Tattini, T. (2013). Functional roles of flavonoids in photoprotection: new evidence, lessons from the past. Plant. Physiol. Bioch. 72, 35–45. doi: 10.1016/j.plaphy.2013.03.014
Aljbory, Z., and Chen, M. S. (2018). Indirect plant defense against insect herbivores: a review. Insect. Sci. 25, 2–23. doi: 10.1111/1744-7917.12436
Arifin, H. A., Hashiguchi, T., Nagahama, K., Hashiguchi, M., Muguerza, M., Sakakibara, Y., et al. (2021). Varietal differences in flavonoid and antioxidant activity in Japanese soybean accessions. Biosci. Biotechnol. Biochem. 85, 916–922. doi: 10.1093/bbb/zbaa104
Bai, G., Yang, D. H., Zhao, Y., Ha, S., Yang, F., Ma, J., et al. (2013). Interactions between soybean ABA receptors and type 2C protein phosphatases. Plant. Mol. Biol. 83, 651–664. doi: 10.1007/s11103-013-0114-4
Bao, A., Chen, H. F., Chen, L. M., Chen, S. L., Hao, Q. N., Guo, W., et al. (2019). CRISPR/Cas9-mediated targeted mutagenesis of GmSPL9 genes alters plant architecture in soybean. BMC Plant. Biol. 19:131. doi: 10.1186/s12870-019-1746-6
Belete, T. (2018). Defense mechanisms of plants to insect pests: from morphological to biochemical approach. Trends. Tech. Sci. Res. 2, 30–38. doi: 10.1080/15548627.2020.1797280
Bondori, A., Bagheri, A., Damalas, C. A., and Allahyari, M. S. (2018). Use of personal protective equipment towards pesticide exposure: farmers’ attitudes and determinants of behavior. Sci. Total. Environ. 639, 1156–1163. doi: 10.1016/j.scitotenv.2018.05.203
Bowles, D., Isayenkova, J., Lim, E. K., and Poppenberger, B. (2005). Glycosyltransferases: managers of small molecules. Curr. Opin. Plant. Biol. 8, 254–263. doi: 10.1016/j.pbi.2005.03.007
Breen, S., Williams, S. J., Outram, M., Kobe, B., and Solomon, P. S. (2017). Emerging insights into the functions of pathogenesis-related protein 1. Trends. Plant. Sci. 22, 871–879. doi: 10.1016/j.tplants.2017.06.013
Cai, Y. P., Wang, L. W., Chen, L., Wu, T. T., Liu, L. P., Sun, S., et al. (2020). Mutagenesis of GmFT2a and GmFT5a mediated by CRISPR/Cas9 contributes for expanding the regional adaptability of soybean. Plant. Biotechnol. J. 18, 298–309. doi: 10.1111/pbi.13199
Chen, K., Wang, Y. W., Zhang, R., Zhang, H., and Gao, C. (2019). CRISPR/Cas genome editing and precision plant breeding inagriculture. Annu. Rev. Plant. Biol. 70, 667–697.
Chen, L., Yang, H., Fang, Y., Guo, W., Chen, H., Zhang, X., et al. (2021). Overexpression of GmMYB14 improves high-density yield and drought tolerance of soybean through regulating plant architecture mediated by the brassinosteroid pathway. Plant Biotechnol. J. 19, 702–716. doi: 10.1111/pbi.13496
Chen, W., Gong, L., Guo, Z. L., Wang, S. H., Zhang, H. Y., Liu, X. Q., et al. (2013). A novel integrated method for large-scale detection, identification, and quantification of widely targeted metabolites: application in the study of rice metabolomics. Mol. Plant. 6, 1769–1780. doi: 10.1093/mp/sst080
Chen, Y. K., Xu, X. P., Liu, Z. X., Zhang, Z. H., Han, X., Lin, Y. L., et al. (2020). Global scale transcriptome analysis reveals differently expressed genes involve in early somatic embryogenesis in Dimocarpus longan Lour. BMC Genomics. 21:4. doi: 10.1186/s12864-019-6393-7
Cheng, Q., Dong, L. D., Su, T., Li, T. Y., Gan, Z. R., Nan, H. Y., et al. (2019). CRISPR/Cas9-mediated targeted mutagenesis of GmLHY genes alters plant height and internode length in soybean. BMC Plant. Biol. 19:562. doi: 10.1186/s12870-019-2145-8
Clough, S. J., and Bent, A. F. (1998). Floral dip: a simplified method for Agrobacterium-mediated transformation of Arabidopsis thaliana. Plant. J. 16, 735–743.
Deguchi, M., Bogush, D., Weeden, H., Spuhler, Z., Potlakayala, S., Kondo, T., et al. (2020). Establishment and optimization of a hemp (Cannabis sativa L.) agroinfiltration system for gene expression and silencing studies. Sci. Rep. 10:3504. doi: 10.1038/s41598-020-60323-9
Dhaubhadel, S., Gijzen, M., Moy, P., and Farhangkhoee, M. (2007). Transcriptome analysis reveals a critical role of CHS7 and CHS8 genes for isoflavonoid synthesis in soybean seeds. Plant. Physiol. 143, 326–338. doi: 10.1104/pp.106.086306
Domingo, J. L., and Bordonaba, J. G. (2011). A literature review on the safety assessment of genetically modified plants. Environ. Int. 37, 734–742. doi: 10.1016/j.envint.2011.01.003
Du, H. Y., Zeng, X. R., Zhao, M., Cui, X. P., Wang, Q., Yang, H., et al. (2016). Efficient targeted mutagenesis in soybean by TALENs and CRISPR/Cas9. J. Biotechnol. 217, 90–97. doi: 10.1016/j.jbiotec.2015.11.005
Elba, D. L. C., Viria, B. D., Ramírez, F., and Castillo, L. E. (2014). Environmental hazards associated with pesticideimport into Costa Rica, 1977-2009. J. Environ. Biol. 35, 43–55.
Fabricio, A. S., and Venancio, T. M. (2021). Pathogenesis-related protein 1 (PR-1) genes in soybean: genome-wide identification, structural analysis and expression profiling under multiple biotic and abiotic stresses. Biorxiv [preprint] 5:23. doi: 10.1101/2021.03.27.437342
Gao, C. X. (2021). Genome engineering for crop improvement and future agriculture. Cell. 184, 1621–1635. doi: 10.1016/j.cell.2021.01.005
Ghione, C. E., Lombardo, L. A., Vicentin, I. G., and Heinz, R. A. (2021). Association mapping to identify molecular markers associated with resistance genes to stink bugs in soybean. Euphytica 217, 46–58.
Giacometti, R., Barneto, J., Barriga, L. G., Sardoy, P. M., Balestrasse, K., Andrade, A. M., et al. (2016). Early perception of stink bug damage in developing seeds of field-grown soybean induces chemical defences and reduces bug attack. Pest. Manag. Sci. 72, 1585–1594. doi: 10.1002/ps.4192
Haile, F., Nowatzki, T., Storer, N., and Davis, J. (2021). Overview of pest status, potential risk, and management considerations of Helicoverpa armigera (Lepidoptera: noctuidae) for U.S. soybean production. J. Integr. Pest. Manag. 12, 1–10.
Han, J. N., Guo, B. F., Guo, Y., Zhang, B., Wang, X. B., and Qiu, L. J. (2019). Creation of early flowering germplasm of soybean by CRISPR/Cas9 technology. Front. Plant. Sci. 10:1446. doi: 10.3389/fpls.2019.01446
Hettenhausen, C., Schuman, M. C., and Wu, J. Q. (2015). MAPK signaling: a key element in plant defense response to insects. Insect. Sci. 22, 157–164. doi: 10.1111/1744-7917.12128
Ichim, M. C. (2019). The Romanian experience and perspective on the commercial cultivation of genetically modified crops in Europe. Transgenic. Res. 28, 1–7. doi: 10.1007/s11248-018-0095-9
Kim, H., Xing, G. G., He, J. B., Zhao, T. J., Yang, S. P., Li, Y., et al. (2015). An environmental different association analysis of antibiosis to common cutworm in a Chinese soybean germplasm population and optimization of the cross design. Mol. Breeding. 35:76. doi: 10.1007/s11032-015-0267-8
Kim, H., Xing, G. G., Wang, Y. F., Zhao, T. J., Yu, D. Y., Yang, S. P., et al. (2013). Constitution of resistance to common cutworm in terms of antibiosis and antixenosis in soybean RIL populations. Euphytica 196, 137–154. doi: 10.1007/s10681-013-1021-0
Knott, G. J., and Jennifer, A. D. (2018). CRISPR-Cas guides the future of genetic engineering. Science. 361, 866–869. doi: 10.1126/science.aat5011
Kou, M. Z., Bastias, D. A., Christensen, M. J., Zhong, R., Nan, Z. B., and Zhang, X. X. (2021). The plant salicylic acid signalling pathway regulates the infection of a biotrophic pathogen in grasses associated with an Epichloe endophyte. J. Fungi. 7, 633–650. doi: 10.3390/jof7080633
Kumar, K., Gambhir, G., Dass, A., Tripathi, A. K., Singh, A., Jha, A. K., et al. (2020). Genetically modified crops: current status and future prospects. Planta. 251:91. doi: 10.1007/s00425-020-03372-8
Lei, X. J., Tan, B., Liu, Z. Y., Wu, J., Lv, J. X., and Gao, C. Q. (2021). ThCOL2 improves the salt stress tolerance of Tamarix hispida. Front. Plant. Sci. 12:653791. doi: 10.3389/fpls.2021.653791
Lin, J. S., Huang, X. X., Li, Q., Cao, Y., Bao, Y., Meng, X. F., et al. (2016). UDP-glycosyltransferase 72B1 catalyzes the glucose conjugation of monolignols and is essential for the normal cell wall lignification in Arabidopsis thaliana. Plant. J. 88, 26–42. doi: 10.1111/tpj.13229
Liu, H. J., Che, Z. J., Zeng, X. R., Zhang, G. Z., Wang, H., and Yu, D. Y. (2016). Identification of single nucleotide polymorphisms in soybean associated with resistance to common cutworm (Spodoptera litura Fabricius). Euphytica. 209, 49–62.
Livak, K. J., and Schmittgen, T. D. (2001). Analysis of relative gene expression data using real-time quantitative PCR and the 2–Δ Δ CT method. Methods. 25, 402–408. doi: 10.1006/meth.2001.1262
Lucini, T. A., Panizzi, A. R., and Bueno, A. D. F. (2021). Evaluating resistance of the soybean block technology cultivars to the Neotropical brown stink bug, Euschistus heros (F.). J. Insect. Physiol. 131:104228. doi: 10.1016/j.jinsphys.2021.104228
Mameda, R. T., Waki, Y., Kawai, S., Takahashi, S., and Nakayama, T. (2018). Involvement of chalcone reductase in the soybean isoflavone metabolon: identification of GmCHR5, which interacts with 2-hydroxyisoflavanone synthase. Plant. J. 96, 56–74. doi: 10.1111/tpj.14014
Masada, S., Terasaka, K., and Mizukami, H. (2007). A single amino acid in the PSPG-box plays an important role in the catalytic function of CaUGT2 (Curcumin glucosyltransferase), a Group D Family 1 glucosyltransferase from Catharanthus roseus. FEBS. Letters. 581, 2605–2610. doi: 10.1016/j.febslet.2007.05.002
Missbach, S., Weis, B. L., Martin, R., Simm, S., Bohnsack, M. T., and Schleiff, E. (2013). 40S ribosome biogenesis co-factors are essential for gametophyte and embryo development. PLoS One 8:e54084. doi: 10.1371/journal.pone.0054084
Ortega, M. A., All, J. N., Boerma, H. R., and Parrott, W. A. (2016). Pyramids of QTLs enhance host-plant resistance and Bt-mediated resistance to leaf-chewing insects in soybean. Theor. Appl. Genet. 129, 703–715. doi: 10.1007/s00122-015-2658-y
Ortega, M. A., Lail, L. A., Wood, E. D., All, J. N., Li, Z., Boerma, H. R., et al. (2017). Registration of two soybean germplasm lines containing leaf-chewing insect resistance QTLs from PI 229358 and PI 227687 introgressed into ‘Benning’. J. Plant Regist. 11, 185–191. doi: 10.3198/jpr2016.04.0019crg
Otuka, A. M., Matsumura, M., and Tokuda, M. (2020). Dispersal of the common cutworm, spodoptera litura, monitored by searchlight trap and relationship with occurrence of soybean leaf damage. Insects 11:427. doi: 10.3390/insects11070427
Pi, E., Xu, J., Li, H. H., Fan, W., Zhu, C. M., Zhang, T. Y., et al. (2019). Enhanced salt tolerance of rhizobia-inoculated soybean correlates with decreased phosphorylation of the transcription factor GmMYB183 and altered flavonoid biosynthesis. Mol. Cell. Proteomics 18, 2225–2243. doi: 10.1074/mcp.RA119.001704
Pozebon, H., Marques, R. P., Padilha, G., Neal, M. O., Valmorbida, I., Bevilaqua, J. G., et al. (2020). Arthropodinvasions versus soybean production in Brazil: a review. J. Econ. Entomol. 113, 1591–1608. doi: 10.1093/jee/toaa108
Raman, R. (2017). The impact of genetically modified (GM) crops in modern agriculture: a review. GM Crops. Food 8, 195–208. doi: 10.1080/21645698.2017.1413522
Rector, B., All, J., Parrott, W., and Boerma, H. R. (2000). Quantitative trait loci for antibiosis resistance to corn earworm in soybean. Crop. Sci. 40, 233–238. doi: 10.2135/cropsci2000.401233x
Sabljic, I., Barneto, J. A., Balestrasse, K. B., Zavala, J. A., and Pagano, E. A. (2020). Role of reactive oxygen species and isoflavonoids in soybean resistance to the attack of the southern green stink bug. Peer. J. 8:e9956. doi: 10.7717/peerj.9956
Saikhedkar, N. S., Joshi, R. S., Yadav, A. K., Seal, S., Fernandes, M., and Giri, A. P. (2019). Phyto-inspired cyclic peptides derived from plant Pin-II type protease inhibitor reactive center loops for crop protection from insect pests. Biochim. Biophys. Acta. Gen. Subj. 1863, 1254–1262. doi: 10.1016/j.bbagen.2019.05.003
Schiemann, J., Dietz-Pfeilstetter, A., Hartung, F., Kohl, C., Romeis, J., and Sprink, T. (2019). Risk assessment and regulation of plants modified by modern biotechniques: current status and future challenges. Annu. Rev. Plant. Biol. 70, 699–726. doi: 10.1146/annurev-arplant-050718-100025
Shen, X. J., Wang, Y. Y., Zhang, Y. X., Guo, W., Jiao, Y. Q., and Zhou, X. A. (2018). Overexpression of the wild soybean R2R3-MYB transcription factor GsMYB15 enhances resistance to salt stress and Helicoverpa Armigera in transgenic Arabidopsis. Int. J. Mol. Sci. 19:3958. doi: 10.3390/ijms19123958
Smehilova, M., Dobruskova, J., Novak, Q., Takac, T., and Galuszka, P. (2016). Cytokinin-specific glycosyltransferases possess different roles in cytokinin homeostasismaintenance. Front. Plant. Sci. 7:1264. doi: 10.3389/fpls.2016.01264
Sun, Y. G., Wang, B., Jin, S. H., Qu, X. X., Li, Y. J., and Hou, B. K. (2013). Ectopic expression of Arabidopsis glycosyltransferase UGT85A5 enhances salt stress tolerance in tobacco. PLoS One 8:e59924. doi: 10.1371/journal.pone.0059924
Thevenot, E. A., Roux, A., Xu, Y., Ezan, E., and Junot, C. (2015). Analysis of the human adult urinary metabolome variations with age, body mass index, and gender by implementing a comprehensive workflow for univariate and OPLS statistical analyses. J. Proteome. Res. 14, 3322–3335. doi: 10.1021/acs.jproteome.5b00354
Wang, H., Yan, H. L., Du, H. P., Chao, M. N., Gao, Z. J., and Yu, D. Y. (2015). Mapping quantitative trait loci associated with soybean resistance to common cutworm and soybean compensatory growth after defoliation using SNP marker-based genome-wide association analysis. Mol. Breeding. 35:168. doi: 10.1016/j.cj.2014.08.004
Wang, Q., Liu, Y., He, H. J., Zhao, X. F., and Wang, J. X. (2010). Immune responses of Helicoverpa armigera to different kinds of pathogens. BMC Immunol. 11:9. doi: 10.1186/1471-2172-11-9
War, A. R., Paulraj, M. G., Ahmad, T., Buhroo, A. A., Hussain, B., Ignacimuthu, S., et al. (2012). Mechanisms of plant defense against insect herbivores. Plant. Signal. Behav. 7, 1306–1320. doi: 10.4161/psb.21663
Witte, S., Moco, S., Vervoort, J., Matern, U., and Martens, S. (2009). Recombinant expression and functional characterisation of regiospecific flavonoid glycosyltransferases from Hieracium pilosella L. Planta 229, 1135–1146. doi: 10.1007/s00425-009-0902-x
Yang, Z. Y., Li, N., Kitano, T., Li, P., Spindel, J. E., Wang, L. S., et al. (2021). Genetic mapping identifies a rice naringenin O-glucosyltransferase that influences insect resistance. Plant. J. 106, 1401–1413. doi: 10.1111/tpj.15244
Yin, Q. G., Shen, G. A., Di, S. K., Fan, C. Y., Chang, Z. Z., and Pang, Y. Z. (2017). Genome-wide identification and functional characterization of UDP-glucosyltransferase genes involved in flavonoid biosynthesis in Glycine max. Plant. Cell. Physiol. 58, 1558–1572. doi: 10.1093/pcp/pcx081
Zhang, X. B., Abrahan, C., Colquhoun, T. A., and Liu, C. J. (2017). A proteolytic regulator controlling chalcone synthase stability and flavonoid biosynthesis in Arabidopsis. Plant. Cell. 29, 1157–1174. doi: 10.1105/tpc.16.00855
Zhang, X. L., Zhou, Q. X., Zou, W., and Hu, X. G. (2017). Molecular mechanisms of developmental toxicity induced by graphene oxide at predicted environmental concentrations. Environ. Sci. Technol. 51, 7861–7871. doi: 10.1021/acs.est.7b01922
Zhu, S., Saski, C. A., Boerma, H. R., Tomkins, J. P., All, J. N., and Parrott, W. A. (2008). Construction of a BAC library for a defoliating insect-resistant soybean and identification of candidate clones using a novel approach. Plant Mol. Biol. Rep. 27, 229–235. doi: 10.1007/s11105-008-0077-9
Zhu, S., Walker, D. R., Boerma, H. R., All, J. N., and Parrott, W. A. (2006). Fine mapping of a major insect resistance QTL in soybean and its interaction with minor resistance QTLs. Crop. Sci. 46, 1094–1099.
Keywords: CRISPR/Cas9, soybean, UDP-glycosyltransferase, Helicoverpa armigera Hübner, Spodoptera litura Fabricius
Citation: Zhang Y, Guo W, Chen L, Shen X, Yang H, Fang Y, Ouyang W, Mai S, Chen H, Chen S, Hao Q, Yuan S, Zhang C, Huang Y, Shan Z, Yang Z, Qiu D, Zhou X, Cao D, Li X and Jiao Y (2022) CRISPR/Cas9-Mediated Targeted Mutagenesis of GmUGT Enhanced Soybean Resistance Against Leaf-Chewing Insects Through Flavonoids Biosynthesis. Front. Plant Sci. 13:802716. doi: 10.3389/fpls.2022.802716
Received: 27 October 2021; Accepted: 07 January 2022;
Published: 22 February 2022.
Edited by:
Deyue Yu, Nanjing Agricultural University, ChinaReviewed by:
Jia-He Wu, Institute of Microbiology, Chinese Academy of Sciences (CAS), ChinaXiangdong Yang, Jilin Academy of Agricultural Sciences (CAAS), China
Copyright © 2022 Zhang, Guo, Chen, Shen, Yang, Fang, Ouyang, Mai, Chen, Chen, Hao, Yuan, Zhang, Huang, Shan, Yang, Qiu, Zhou, Cao, Li and Jiao. This is an open-access article distributed under the terms of the Creative Commons Attribution License (CC BY). The use, distribution or reproduction in other forums is permitted, provided the original author(s) and the copyright owner(s) are credited and that the original publication in this journal is cited, in accordance with accepted academic practice. No use, distribution or reproduction is permitted which does not comply with these terms.
*Correspondence: Dong Cao, Y2FvZG9uZ0BjYWFzLmNu; Xia Li, eGxpQG1haWwuaHphdS5lZHUuY24=; Yongqing Jiao, eXFqaWFvQDEyNi5jb20=
†These authors have contributed equally to this work