- 1The State Key Laboratory of Crop Genetics and Germplasm Enhancement, Nanjing Agricultural University, Nanjing, China
- 2Plant Biology Section, School of Integrated Plant Science, Cornell University, Ithaca, NY, United States
- 3Department of Electrical and Electronic Engineering, Guilin University of Technology, Nanning, China
The dehydration-responsive element binding protein 1 (DREB1)/C-repeat-binding factor (CBF) genes are key regulators of cold acclimation and freezing tolerance in the chilling tolerant Arabidopsis thaliana. Here, we investigated the function of three members of the 10 rice DREB1 genes, OsDREB1C, E, and G, in the chilling sensitive rice plants. Their loss of function (LOF) mutants were each more chilling susceptible compared to the wild type, and the LOF mutants of all three genes, dreb1ceg, were more chilling susceptible than any of the single mutants. Strikingly, these mutants were capable of cold acclimation, indicating that these rice DREB1 genes are important for basal chilling tolerance but not cold acclimation. Transcriptome and physiology analyses suggest that the OsDREB1C/E/G genes are involved in reactive oxygen species (ROS) scavenging and cell death regulation under chilling. Furthermore, these three rice DREB1 genes are found to promote tolerance to other abiotic stresses: the OsDREB1C/E/G genes are positive regulators of heat tolerance, OsDREB1C and OsDREB1G are positive regulators of salt tolerance, and OsDREB1G is a positive regulator of drought tolerance. These findings expand our knowledge of the roles of DREB1 proteins in plants, enhance our mechanistic understanding of abiotic stress tolerance and will facilitate the generation of stress-tolerant crop plants.
Introduction
Cold stresses, both freezing and chilling (above freezing), are a major threat to crop production worldwide (Pearce, 2001; Zhang et al., 2019). Rice (Oryza sativa), with a tropical and temperate origin, is susceptible to cold stress at all developmental stages (Zhang et al., 2014). A temperature lower than 15°C causes drastic physiological changes and inhibits the growth and development of rice (Jacobs and Pearson, 1999; Aghaee et al., 2011). Chilling stress results in poor germination, stunted seedlings, yellowing or withering of leaves, and reduced tillering in rice (Yadav, 2010). Chilling induces accumulation of reactive oxygen species (ROS) which serves as both a signaling molecule of cold response and a damaging molecule to the cell (Hasanuzzaman et al., 2013; Devireddy et al., 2021). Excess ROS is harmful to the stability of cell membrane and proteins and leads to growth inhibition or cell death (Mittler, 2002; Mhamdi and Van Breusegem, 2018). ROS-scavenging has an important role in protecting plants against chilling stress, and a correlation of ROS scavenging and chilling tolerance has been reported (Fang et al., 2020; Ge et al., 2020).
Plants have multiple mechanisms to promote tolerance to cold stresses. The most extensively studied process is cold acclimation, where freezing tolerance is enhanced by a prior exposure to low non-freezing temperatures (Thomashow, 1999). The dehydration-responsive element binding factor 1 (DREB1)/C-repeat binding factor (CBF) genes in the ERF transcription factor family play a critical role in this cold acclimation in Arabidopsis thaliana. Their transcripts are rapidly induced by chilling stress (Gilmour et al., 1998), and the CBF/DREB1 proteins recognize and bind to the C-repeat/dehydration-responsive (CRT/DRE) cis-elements in the promoters of cold-responsive (COR) genes to activate COR genes expression for freezing tolerance (Stockinger et al., 1997; Liu et al., 1998; Sakuma et al., 2002). Arabidopsis has six DREB1 genes, namely DREB1A/CBF3, DREB1B/CBF1, DREB1C/CBF2, DREB1D/CBF4, DREB1E/DDF2, and DREB1F/DDF1 (Sakuma et al., 2002). Three of them, CBF1, CBF2, and CBF3 genes, are shown to be required for freezing tolerance and cold acclimation by the study of knockout mutant combinations generated by genome editing (Hua, 2016). The cbf1 cbf2 cbf3 triple mutants were more susceptible to freezing than the wild type (WT) with prior low temperature treatment (Jia et al., 2016; Zhao et al., 2016). Without prior treatment, the cbf1 cbf2 cbf3 mutants did not exhibit more susceptible to freezing (Jia et al., 2016) or was only slightly more susceptible under one out of eight freezing temperatures tested (Zhao et al., 2016). The three Arabidopsis CBF/DREB1 proteins are predicted to positively regulate expression of a total of 346 and 112 COR genes in two independent studies (Jia et al., 2016; Zhao et al., 2016). Recently, 146 Arabidopsis genes are defined as direct CBF/DREB1 targets by chromatin immunoprecipitation (ChIP) combined with a transcriptional analysis, and these genes are involved in abiotic stress responses, hormone signaling, and environmental signaling (Song et al., 2021).
CBF/DREB1 genes are also implicated in tolerance to other abiotic stresses. Compared to the WT, the Arabidopsis cbf1 cbf2 cbf3 triple mutant is more susceptible to salt (Zhao et al., 2016), and cbf4 mutant is more susceptible to drought (Vonapartis et al., 2022). Overexpression of the Arabidopsis DREB1A, DREB1B, and DREB1C enhanced tolerance to freezing, salt, and drought in Arabidopsis (Liu et al., 1998; Kasuga et al., 1999; Gilmour et al., 2004). Overexpression of the Arabidopsis DREB1D enhanced drought tolerance in soybean (Guttikonda et al., 2014), while overexpression of the Arabidopsis DREB1F/DDF1 enhanced tolerance to freezing, drought, and heat in Arabidopsis (Kang et al., 2011). The function of DREB1 genes in other plants have also been investigated. Overexpression of DREB1 genes from cotton, soybean, and zoysia grass was shown to enhance tolerances to chilling, drought, heat, and salt (Gao et al., 2005; Shan et al., 2007; Kidokoro et al., 2015; Feng et al., 2019; Zhou et al., 2020). These results suggest that DREB1 proteins could regulate tolerance to multiple abiotic stresses.
The roles of rice DREB1 genes have been investigated mostly by overexpression studies. Overexpression of four rice DREB1 genes is shown to enhance stress tolerance: OsDREB1A for freezing and high salt tolerances in Arabidopsis (Dubouzet et al., 2003), OsDREB1D for tolerances to freezing and high-salt in Arabidopsis (Zhang et al., 2009), OsDREB1F for tolerances to salt, drought, and cold in both Arabidopsis and rice (Wang et al., 2008), and OsDREB1G for chilling tolerance in rice (Moon et al., 2019). The only study of DREB1 function by its loss of function (LOF) mutant is on OsDREB1A which was shown to be a positive regulator of cold-induced calcium influx and chilling tolerance in rice (Wang et al., 2021).
In this study, we investigated the roles of three rice DREB1 genes (OsDREB1C, E, and G) which are the closest homologs of the three Arabidopsis DREB1 genes (CBF1, CBF2, and CBF3) by generating and analyzing their LOF mutants. These genes are found to be positive regulators of chilling tolerance but do not affect cold acclimation. They are also found to promote tolerance to other abiotic stresses including heat, salt and drought in rice. Transcriptome analysis suggests that these OsDREB1 genes affect ROS scavenging and cell death. Thus, our study uncovers function of OsDREB1C/E/G in multiple abiotic stress tolerance in rice and reveals potential similar and distinct functions of these genes in rice and Arabidopsis.
Materials and Methods
Phylogenetic and Expression Analyses
Protein sequences of DREB1 genes from Arabidopsis and rice were aligned using ClustalX. A neighbor-joining tree with a bootstrap setting of 1,000 was generated using MEGA7.0.14 (Kumar et al., 2016). Expression data of OsDREB1 genes in response to abiotic stresses were obtained from GENEVESTIGATOR.1
Mutant Generation and Identification
All rice plants in this study are Oryza sativa cv. Nipponbare (NIP). Mutants were generated in NIP using CRISPR/Cas9 system (Xing et al., 2014). Specific gRNA targets were designed using CRISPR-PLANT website.2 The gRNA targets were cloned into the pHUE411 vector (Xing et al., 2014), and the resulting constructs were transformed into NIP by Agrobacterium-mediated transformation (Nishimura et al., 2006). Genomic fragments around the target sites in the mutants were amplified by PCR, and the edited mutations were identified by sequencing the PCR products. The homozygous mutants were selected by PCR based genotyping. All the primers used in this study are listed in Supplementary Table 1.
Plant Growth Conditions and Abiotic Stress Treatments
All plants were grown in growth chamber with a photoperiod of 16h-light/8h-dark and at 28°C unless specified (such as for stress treatment). Seeds were sterilized with 5% bleach for 10 min followed by three washes with water. The sterilized seeds were soaked in water at 28°C for 3 days for germination. For hydroponic growth, seeds at a similar germination stage were transferred to a 96-well plate with the bottom cut out. Seedlings were grown hydroponically till the third leaf just emerged, and then cultured in Yoshida nutrient solution (Yoshida et al., 1971). For soil growth, the germinated seeds were transferred from water to soil (nutrient soil:vermiculite = 3:1) and grown in growth chamber.
All stress treatments were carried out in growth chambers. For chilling treatment, the hydroponically grown seedlings at three-leaf stage (when the third leaf was fully expanded) were subject to 6°C treatment for 2.5–4 days and then returned to 28°C for 7 days for recovery growth. For suboptimal low temperature treatment, germinated seeds were transferred to soil and grown under 16°C. For cold acclimation treatment, seedlings at three-leaf stage (grown hydroponically at 28°C) were subject to 1 day of 12°C growth (cold acclimation, CA) or kept at 28°C for 1 day (no acclimation, NA). They were then subject to a 6°C treatment for 3.5–4 days before a 7-day recovery growth at 28°C. For heat treatment, the soil grown seedlings at three-leaf stage were treated at 48°C for 2 days and then returned to 28°C for 7-day recovery growth. For salt treatment, the soil grown seedlings of 3-day-old were treated with 200 mM NaCl for 21–23 days. For drought treatment, the soil-grown seedlings at three-leaf stage were withheld of watering for 10 days and then re-watered for 7 days for recovery growth. For survival analysis, 24 hydroponically grown seedlings or 21 soil growth seedlings were used in a biological repeat. At least three biological replicates were performed for each analysis.
DAB and Trypan Blue Staining
Accumulations of H2O2 and cell death were detected by 3,3′-diaminobenzidine (DAB) staining and trypan blue staining, respectively, as previously described (Bach-Pages and Preston, 2018). The second leaves of the soil grown seedlings at three-leaf stage were used.
Transcriptome Analysis
The three-leaf stage seedlings that were soil grown at 28°C were treated at 6°C for 0, 4, and 24 h before all above ground tissue of three biological replicates were collected. Total RNA was extracted from tissues using an RNA extraction kit (RP1002, Bioteke Corporation). RNA sequencing (RNA-seq) was carried out at Berry Genomics.3 Approximately 6.0 GB of raw reads were generated from each sample. The clean reads were mapped to rice genome,4 and expression level for each gene was determined by its relative abundance or value of fragments per kilobase of exon model per million mapped (FPKM). Gene expression values in FPKM was used for principal component analysis.5 Differentially expressed genes (DEGs) were identified using edgeR package (3.32.1) by R Language (R Core Team, 2021). DEGs between two samples were defined by fold change ≥ 2 and false discovery rate (FDR) ≤ 0.01. Heat maps were plotted via TBtools or using pheatmap (1.0.12) and ggplot2 (3.3.3) packages by R language (Chen et al., 2020; R Core Team, 2021). Venn diagrams were plotted using Venn Diagram package (1.6.20) by R language (R Core Team, 2021). Gene ontology (GO) enrichment analysis was performed by PANTHER.6 GO annotation was downloaded from RiceXPro.7 The promoter analysis was performed by a manual search for the DRE/CRT motif (G/ACCGAC) in the 1.5 kb fragment 5′ to the translation start codon of the candidate gene. The RNA-seq data were submitted to National Center for Biotechnology Information (accession number: PRJNA797855).8
Results
Phylogenetic and Expression Analyses of the OsDREB1 Genes in Rice
The DREB1 family has six members in Arabidopsis and 10 members (named OsDREB1A to OsDREB1J) in rice.9 Analysis of genes from 38 organisms revealed that this gene family diverged before the separation of Arabidopsis and rice, and the DREB1 genes have independently amplified in two species (Supplementary Figure 1). This suggests that the three CBF genes in Arabidopsis do not have a one-to-one orthologous DREB1 genes in rice. Protein sequence homology analysis of the six Arabidopsis and 10 rice DREB1 genes, however, revealed that OsDREB1C, OsDREB1E, OsDREB1F, and OsDREB1G are likely closer to the six Arabidopsis DREB1 genes in sequence identity (Figure 1A).
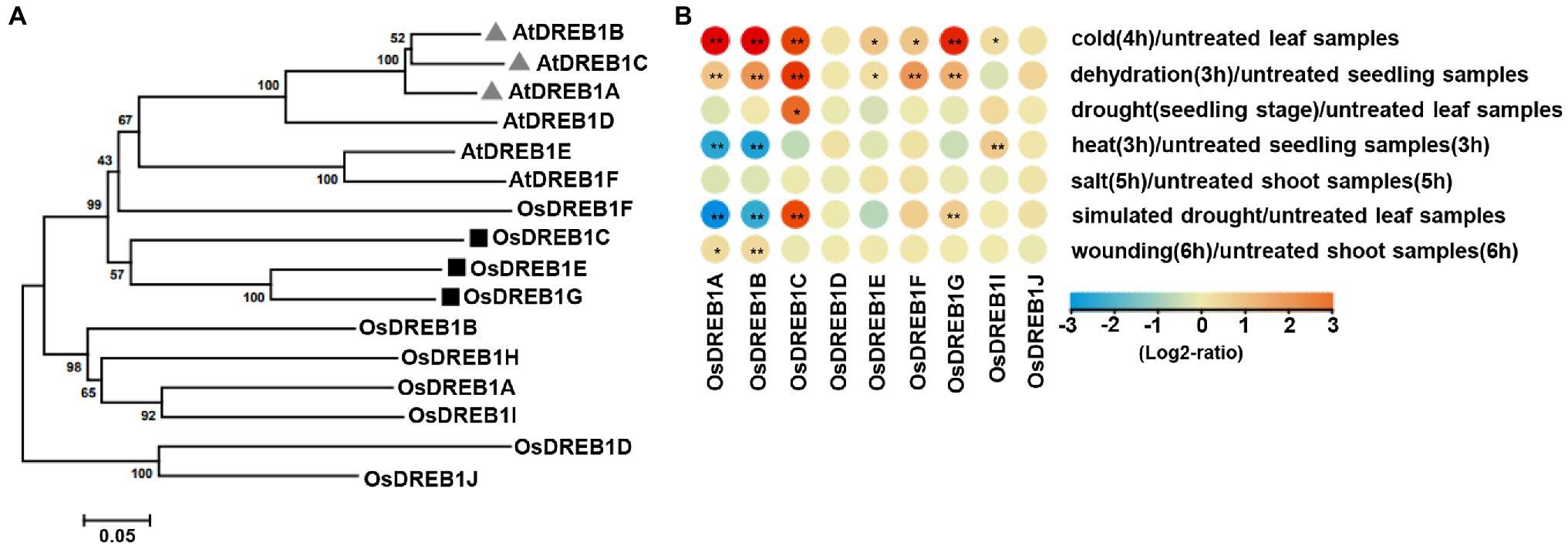
Figure 1. Expression patterns and phylogenetic analyses of OsDREB1 genes in rice. (A) Phylogenetic tree of the OsDREB1 and AtDREB1 proteins. The protein sequences were aligned using ClustatW method, and the neighbor-joining tree with a bootstrap setting of 1,000 was generated using MEGA7.0.14 software. The genes marked with black targets are discussed in this study. The AtDREB1 and OsDREB1 protein sequences were available by TAIR (https://www.arabidopsis.org/) and RiceXPro (https://ricexpro.dna.affrc.go.jp/), respectively. (B) Expression patterns of OsDREB1 genes in response to abiotic stresses. Heat map was plotted via Tbtools. Expression levels are given as log2 ratio values. Expression values are obtained from GENEVESTIGATOR (https://genevestigator.com/). * and ** indicate significant differences compared to no treatment at p < 0.05 and p < 0.01, respectively, by Student’s t-test.
We further examined the expression patterns of these 10 OsDREB1 genes (except for OsDREB1H which is absent in the database) using the curated high-quality transcriptome data at GENEVESTIGATOR (see Footnote1). All these nine OsDREB1 genes (1A, 1B, 1C, 1D, 1E, 1F, 1G, 1I, and 1J) have altered expression in response to one or multiple abiotic stresses. Chilling treatment (4°C for 4 h) increased the expression of 1A, 1B, 1C, 1E, 1F, 1G and 1I. Dehydration treatment (on 3 mm Whatman paper for 3 h) increased the expression of 1A, 1B, 1C, 1E, 1F, and 1G. Drought treatment (no watering till leaves were completely rolled) increased the expression of 1C. Heat treatment (42°C for 3 h) increased expression of 1I and repressed the expression 1A and 1B. Salt treatment (300 mM NaCl for 5 h) had no significant effect on the expression of any of these nine OsDREB1 genes. Simulated drought treatment (25% polyethylene glycol6000 for 1 h) increased the expression of 1C and 1G and repressed the expression 1A and 1B. Wounding treatment (needle puncturing 15 times in 6 h) increased expression of 1A and 1B (Figure 1B). Therefore, most of the OsDREB1 genes had increased expression in response to chilling and dehydration stresses.
Generation of dreb1 Mutants in Rice
We selected three OsDREB1 genes (OsDREB1C, OsDREB1E, and OsDREB1G) for further functional analysis because they are more related to each other and are more closely related to the Arabidopsis DREB1 genes than six other rice genes (Figure 1A). LOF mutants of these three genes were generated in the NIP background by using CRISPR/Cas9 gene editing system (Xing et al., 2014). Two gRNA targets were used to generate single mutants of dreb1c (via targets C1, C2), dreb1e (via targets E1, E3), and dreb1g (via targets G2, G3). A total of four dreb1c mutant alleles (dreb1c-1, dreb1c-2, dreb1c-3, and dreb1c-4), two dreb1e alleles (dreb1e-1 and dreb1e-2), and one dreb1g mutant (dreb1g-1) were obtained (Table 1). A gRNA target (C2) specific for OsDREB1C and gRNA targets (E2, G1) for both OsDREB1E and OsDREB1G was used to generate the dreb1ceg triple mutant (Table 1).
All these mutants were LOF as predicted by the mutation types. The dreb1c-1 mutant has an insertion of “C” at position 12 bp (relative to the translation initiation site) and a deletion at 13–279 bp, which are predicted to cause a reading frame shift. The dreb1c-2 mutant has a deletion of 35–37 bp and an insertion of “A” at 275 bp, leading to a reading frame shift. The dreb1c-3 mutant has an insertion of “T” and “A” at 38 and 275 bp, respectively, leading to coding termination. The dreb1c-4 mutant has a deletion at 32–67 bp and an insertion of “A” at 275 bp leading to a reading frame shift. The dreb1e-1 mutant has an insertion of “TGG” and “T” at 21 and 337 bp, respectively, leading to a reading frame shift. The dreb1e-2 mutant has an insertion of “G” at 21 bp and a deletion of “G” at 336 bp, leading to protein sequence changes starting from amino acid (aa) positions 8 and 112, respectively. The dreb1g-1 mutant has an insertion of “A” at 164 bp, leading to coding termination. The dreb1ceg mutant has a deletion of “G” at 274 bp in OsDREB1C, and an insertion of “A” and “C” at 50 bp in OsDREB1E and OsDREB1G, respectively, leading to a reading frame shift of OsDREB1E and coding terminations of OsDREB1C and OsDREB1G (Table 1). Homozygous single or triple mutants of these three genes were used for further analyses.
OsDREB1C/E/G Genes Promote Basal Chilling Tolerance in Rice at Seedling Stage
The role of OsDREB1C/E/G genes in chilling tolerance was assessed by chilling treatment of the single and triple mutants of dreb1c, dreb1e, and dreb1g. Three-leaf-stage seedlings were subjected to 2.5–4 days of chilling treatment at 6°C and allowed to recover for 7 days. All dreb1 single mutants had significantly lower survival rates than NIP, although the survival rate of the WT differed among different sets of experiments likely due to a slight variation of temperature and duration of chilling treatment (Figures 2A–F; Supplementary Figure 2). The dreb1c-1 and dreb1c-2 mutants had survival rates of 19% and 27%, respectively, lower than the rate of 42% for NIP (Figure 2A). The dreb1c-3 and dreb1c-4 mutants had survival rates of 55% and 49%, respectively, lower than the rate of 92% for NIP (Supplementary Figures 2A–C). The dreb1e-1 mutant had a survival rate of 36%, lower than the rate of 55% for NIP (Figure 2B). The dreb1e-2 mutant had survival rates of 53%, lower than the rate of 72% for NIP (Supplementary Figures 2D,E). The dreb1g-1 mutant had a survival rate of 29%, lower than the rate of 56% for NIP (Figure 2C). The dreb1ceg triple mutant also had lower survival rate than the wild type NIP (27% versus 57%; Figure 2D). Chilling tolerance levels among dreb1 single mutants and the triple mutants were also compared. The survival rates of dreb1e-1 and dreb1g-1 were 50% and 59%, respectively, higher than the rate of 23% for dreb1c-3 (Figure 2E). The survival rate of dreb1c-3 was 63%, higher than the rate of 30% for dreb1ceg (Figure 2F). These results indicate that all three rice genes, OsDREB1C/E/G, positively regulate chilling tolerance in rice at seedling stage.
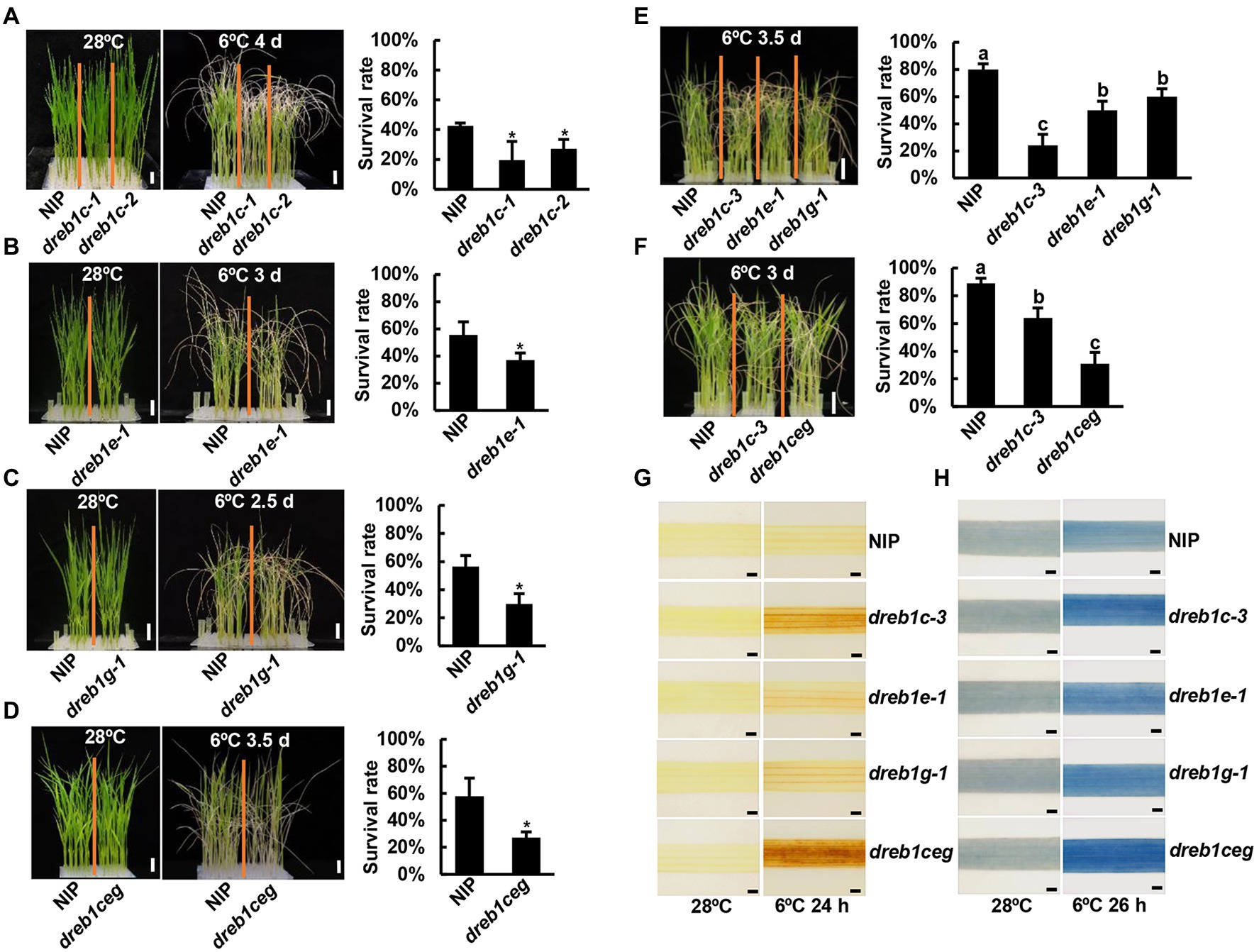
Figure 2. The dreb1 mutants are more susceptible to chilling at seedling stage. (A–F) Growth phenotypes and survival rates of dreb1c and WT (A), dreb1e and WT (B), dreb1g and WT (C), dreb1ceg and WT (D), dreb1c, dreb1e, dreb1g, and WT (E) as well as dreb1c, dreb1ceg, and WT (F) after chilling treatment and recovery. Seedlings were hydroponically grown at 28°C for 3 weeks, incubated at 6°C (for 2.5–4 days as indicated) and then transferred to normal conditions for 7 days for recovery. Left panels show plant morphology and bars = 2 cm. Right panels show mean values of survival rates from three biological replicates ±SD (standard deviation). Each biological replicate has more than 24 seedlings. Asterisks indicate significant differences compared to NIP (*p < 0.05, Student’s t-test). Lowercase letters above the bars indicate significant differences among samples at p < 0.05, by SSR-Test. (G,H) DAB (G) and trypan blue (H) staining of the second leaves of dreb1c-3, dreb1e-1, dreb1g-1, dreb1ceg, and NIP plants before and after chilling treatment at 6°C for 24 h (DAB) or 26 h (trypan blue). Bars = 1 mm.
We further analyzed H2O2 accumulation by DAB staining as ROS accumulation is highly related to chilling tolerance. All dreb1 mutants had more H2O2 accumulation in leaves than NIP after 6°C treatment for 24 h. The dreb1c-3 mutant had more H2O2 accumulation than dreb1e-1 and dreb1g-1, and dreb1ceg had the highest H2O2 accumulation than all single mutants (Figure 2G). Cell death caused by chilling was subsequently analyzed by trypan blue staining. All dreb1 mutants had more cell death than NIP after chilling treatment. The dreb1c-3 mutant had more cell death than dreb1e-1 and dreb1g-1, and dreb1ceg had the highest cell death than all single mutants (Figure 2H). Therefore, H2O2 accumulation and cell death are each associated with chilling susceptibility in the rice dreb1 mutants.
OsDREB1C/E/G Genes Promote Rice Growth Under Suboptimal Low Temperature
We analyzed the growth phenotypes of the dreb1 mutants at non-chilling temperatures. Under normal growth temperature (28°C), no growth difference was observed between the dreb1 mutants and NIP at seedling, tillering, or mature stages (Supplementary Figure 3). Under a suboptimal low temperature (16°C), none of the single dreb1 mutants showed detectable growth difference compared with NIP at the seedling stage (Figures 3A–C). In contrast, the dreb1ceg triple mutant was significantly shorter than the WT NIP at 16°C, with a height of 7.9 cm compared to 11.5 cm of the WT (Figure 3D). These results indicate that the OsDREB1C/E/G genes have an overlapping function in promoting rice growth at suboptimal low temperature.
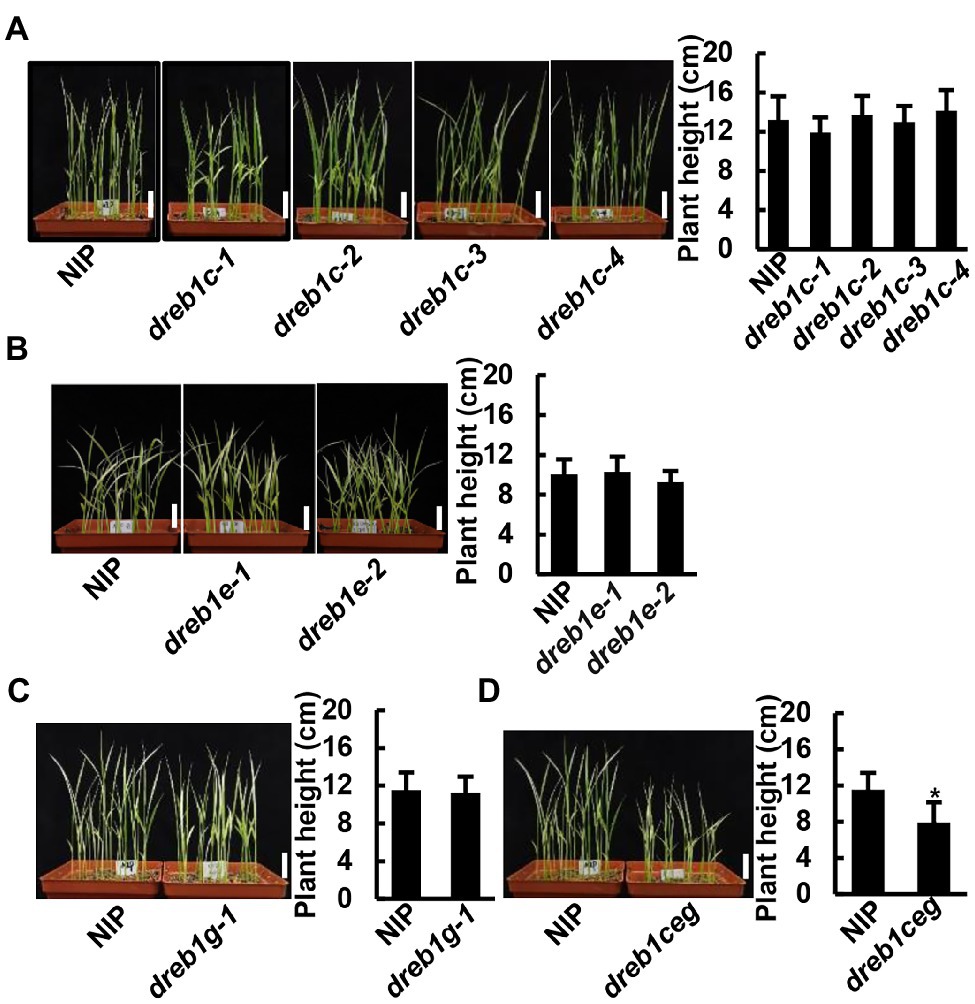
Figure 3. The dreb1ceg mutant had reduced growth at seedling stage under suboptimal low temperature. Growth phenotypes and quantification of plant heights of dreb1c (A), dreb1e (B), dreb1g (C) and dreb1ceg (D) grown at 16°C for 32 days. Seeds were germinated at 28°C for 3 days, and then transferred to 16°C for 32 days. Shown is the mean value ±SD of 24 seedlings. Similar results were obtained from two additional biological repeats. Each replicate has 24 seedlings. Asterisks indicate significant differences compared to NIP (*p < 0.05, Student’s t-test). Bars in plant images represent 2 cm.
OsDREB1C/E/G Genes Do Not Participate in Cold Acclimation in Rice
We further determined whether OsDREB1C/E/G genes regulate cold acclimation. The WT NIP plants exhibited a higher survival rate at 6°C with a prior 12°C treatment of 1 day compared to no prior treatment (Figure 4), indicating that rice has cold acclimation for chilling tolerance. All the dreb1 mutants also exhibited cold acclimation. In one set of experiment, NIP, dreb1c-1, and dreb1c-2 had survival rates of 63%, 42%, and 53%, respectively, with prior cold treatment (cold acclimation, CA), higher than the rates of 37%, 0%, and 0% observed with no prior cold treatment (no acclimation, NA; Figure 4A). NIP and dreb1e-1 had survival rates of 57% and 34%, respectively, with CA, higher than the rates of 28% and 9% with NA (Figure 4B). NIP and dreb1g-1 had survival rates of 75% and 52%, respectively, with CA, higher than the rates of 54% and 25% with NA (Figure 4C). NIP and dreb1ceg had survival rates of 86% and 72%, respectively, with CA, higher than the rates of 53% and 29% with NA (Figure 4D). With CA, all mutants including dreb1c-1, dreb1e-1, dreb1g-1, and dreb1ceg plants showed more chilling susceptibility compared with WT NIP (Figures 4A–D). The only exception was dreb1c-2 which had a lower survival rate compared with NIP, but the difference was not significant (Figure 4A). This indicates that OsDREB1C/E/G genes do not affect cold acclimation in rice. We further analyzed the genotype by environment (G x E) interaction between each genotype pair (WT versus the dreb1 mutants) and cold acclimation (NA versus CA) on survival rates. No significant interaction was found for any of the OsDREB1 genotype and CA, indicating that the dreb1 mutants have a cold acclimation-independent chilling susceptibility (Figure 4). These results indicate that OsDREB1C/E/G are not important for cold acclimation in rice, or they have overlapping functions with other OsDREB1 genes in cold acclimation.
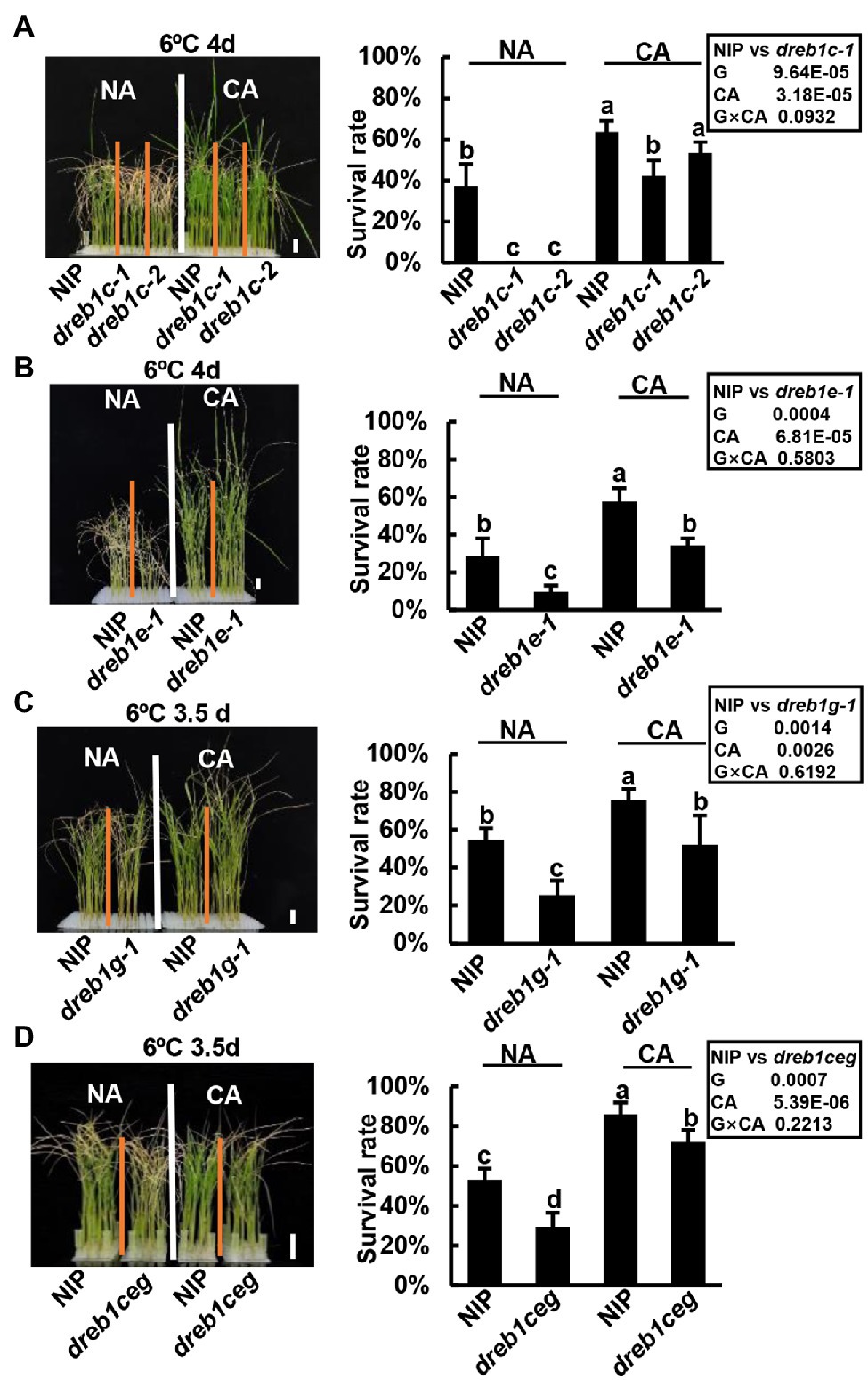
Figure 4. OsDREB1C/E/G genes do not affect cold acclimation in rice. Growth phenotypes and survival rates of dreb1c (A), dreb1e (B), dreb1g (C), and dreb1ceg (D) after chilling treatment and recovery growth with cold acclimation (CA) and without cold acclimation (NA). Seedlings were hydroponically grown at 28°C for 3 weeks till three-leaf stage, treated 1 day at 12°C (CA) or 1 day at 28°C (NA), before treated at 6°C for 3.5 or 4 days followed by a recovery growth at 28°C for 7 days. Left panels show plant morphology after recovery. Bars in plant images represent 2 cm. Right panels show survival rates expressed as mean ± SD from three biological replicates each with 24 seedlings. Lowercase letters above the bars indicate significant differences among samples at p < 0.05, by SSR test. Insert shows multiway-ANOVA analysis of genotype (NIP versus dreb1 mutants) and cold acclimation (NA versus CA).
Transcriptome Analysis of the dreb1 Mutants
To further understand how OsDREB1 regulate chilling tolerance in rice, we carried out RNA-seq analysis on the WT, dreb1c-2, dreb1g-1, and dreb1ceg plants at 3-leaf-stage before and after a chilling treatment of 6°C. Principal component analysis (PCA) revealed that the WT and the dreb1 mutants were similar to each other at any time points in the first two principal components, and chilling treatment was the most important factor in differentiating the samples (Supplementary Figure 4).
This PCA showed that chilling treatment induced a similar global transcriptional change in WT and dreb1 mutants. A total of 747 chilling up-regulated and 406 chilling down-regulated DEGs were shared among the WT, dreb1c-2, dreb1g-1, and dreb1ceg at 4 h of 6°C treatment, accounting for 69%, 56%, 56%, and 70% of up-regulated DEGs as well as 52%, 45%, 42%, and 52% of down-regulated DEGs in the WT, dreb1c, dreb1g, and dreb1ceg, respectively (Supplementary Figure 5). Moreover, 2,774 up-regulated and 2,148 down-regulated DEGs were shared among the WT and three dreb1 mutants at 24 h, accounting for 77%, 84%, 71%, and 71% of up-regulated DEGs as well as 64%, 65%, 59%, and 57% of down-regulated DEGs in the WT, dreb1c, dreb1g, and dreb1ceg, respectively (Supplementary Figure 5). These genes represent the majority of the DEGs in WT or dreb1 mutants, indicating that the dreb1 mutations do not drastically alter chilling-induced or chilling-repressed DEGs. At 4 h, 895 up-regulated and 565 down-regulated DEGs were identified in both dreb1g and dreb1ceg, and 874 up-regulated and 506 down-regulated DEGs were identified in both dreb1c and dreb1ceg (Supplementary Figure 5). At 24 h, 3,419 up-regulated and 2,960 down-regulated DEGs were identified in both dreb1g and dreb1ceg, and 2,962 up-regulated and 2,570 down-regulated DEGs were identified in both dreb1c and dreb1ceg (Supplementary Figure 5). The dreb1g mutant shared more DEGs with dreb1ceg than dreb1c, suggesting that OsDREB1G has a larger functional contribution than OsDREB1C to gene expression changes under chilling.
Considering that OsDREB1 genes are likely transcriptional activators, we examined genes that had a reduced expression in the dreb1ceg mutant under chilling because they are potentially regulatory target genes of OsDREB1C/E/G. These include chilling up-regulated DEGs in WT but not in the triple mutant as well as chilling down-regulated DEGs in dreb1ceg but not in WT at 4 h and/or 24 h. These DEGs were analyzed for enrichment of Gene Ontology (GO) terms defined by FDR < 0.05. No enriched GO terms were identified for the 428 up-regulated DEGs that appeared in WT but not in dreb1ceg (Supplementary Figure 6A). The 1,092 down-regulated DEGs that appeared in dreb1ceg but not in WT were enriched for the GO terms “plant-type hypersensitive response”, “protein maturation”, and “peptidyl-amino acid modification”, with “plant-type hypersensitive response” having the highest fold enrichment of 24 (Supplementary Figures 6B,C). Under the GO term “plant-type hypersensitive response” were four genes that had a lower expression at 24 h in dreb1ceg compared to the WT (Supplementary Figure 6D). These four genes code for a transporter, a ubiquitin-protein ligase, and two MACPF domain-containing protein. One MACPF domain containing protein CAD1 is a negative regulator of the cell death in Arabidopsis, and its mutant exhibited a hypersensitive response-like cell death (Morita-Yamamuro et al., 2005). The ubiquitin-protein ligase OsNLA1 modulates phosphate accumulation in rice, and the loss of its function leads to lethality (Zhong et al., 2017). This suggests that the LOF of OsDREB1C/E/G leads to reduced expression of potential negative regulators of cell death, which might contribute to the increased cell death in the dreb1ceg mutant under chilling.
Chilling downregulated 3,568, 3,534, 4,006, and 4,006 genes in WT, dreb1c, dreb1g, and dreb1ceg, respectively (Supplementary Figure 7A). GO analysis on downregulated DEGs in WT and the three dreb1 mutants was performed to identify differential GO terms between WT and the mutants. One GO term “hydrogen peroxide catabolic process” was present in all dreb1 mutants but not in WT (Supplementary Figure 7B). Under this enriched GO term were 18 chilling downregulated DEGs (1 peroxiredoxin-2E-1, 17 peroxidase) which had a lower expression in both WT and triple mutant at 24 h compared to 0 h. Under this GO term were also 10 chilling downregulated genes (one peroxiredoxin-2E-1, nine peroxidase) that had a lower expression in dreb1ceg compared to the WT at 24 h (Supplementary Figure 7C). Therefore, more genes that are involved in ROS scavenging have a lower expression in the dreb1 mutant compared to the wild type at 24 h. Plant peroxidase scavenges ROS and protects host cells from damages made by excessive ROS (Liu et al., 2021a). These results suggest that OsDREB1C/E/G may promote ROS scavenging under chilling.
Prediction of Potential Target Genes of OsDREB1C/E/G
As transcriptional factors, OsDREB1 proteins are expected to bind to specific cis-element motifs in the promoter region of their target genes and affect their gene expression. Because AtDREB1s mainly function as transcriptional activators (Song et al., 2021), we searched among genes with reduced expression in the dreb1ceg mutant compared to the WT for potential direct regulatory targets of OsDREB1C/E/G by the criterion of having DRE/CRT element(s) in their promoter region. We first examined the genes under GO terms uniquely enriched in the mutant but not the WT for chilling down regulated DEGs. Among the four genes under the GO term “plant-type hypersensitive response”, one gene (LOC_Os07g07194, MACPF domain-containing protein) contained a DRE/CRT (G/ACCGAC) element in its promoter region. Among the 18 genes under the term “hydrogen peroxide catabolic process”, three genes (LOC_Os03g22020, LOC_Os06g42000, and LOC_Os11g43980) contained one DRE/CRT element in their promoter regions. Therefore, these four genes are potential direct targets of OsDREB1C/E/G.
In addition, we examined genes with reduced expression in dreb1ceg compared to the WT at each of the three time points (0, 4, and 24 h) of chilling treatment. With 21, 38, and 145 downregulated DEGs (mutant versus WT) at 0, 4, and 24 h, respectively, the dreb1ceg mutant had a total of 168 downregulated DEGs compared to the WT with three time points combined (Figure 5A). These genes were defined as potential OsDREB1C/E/G target genes.
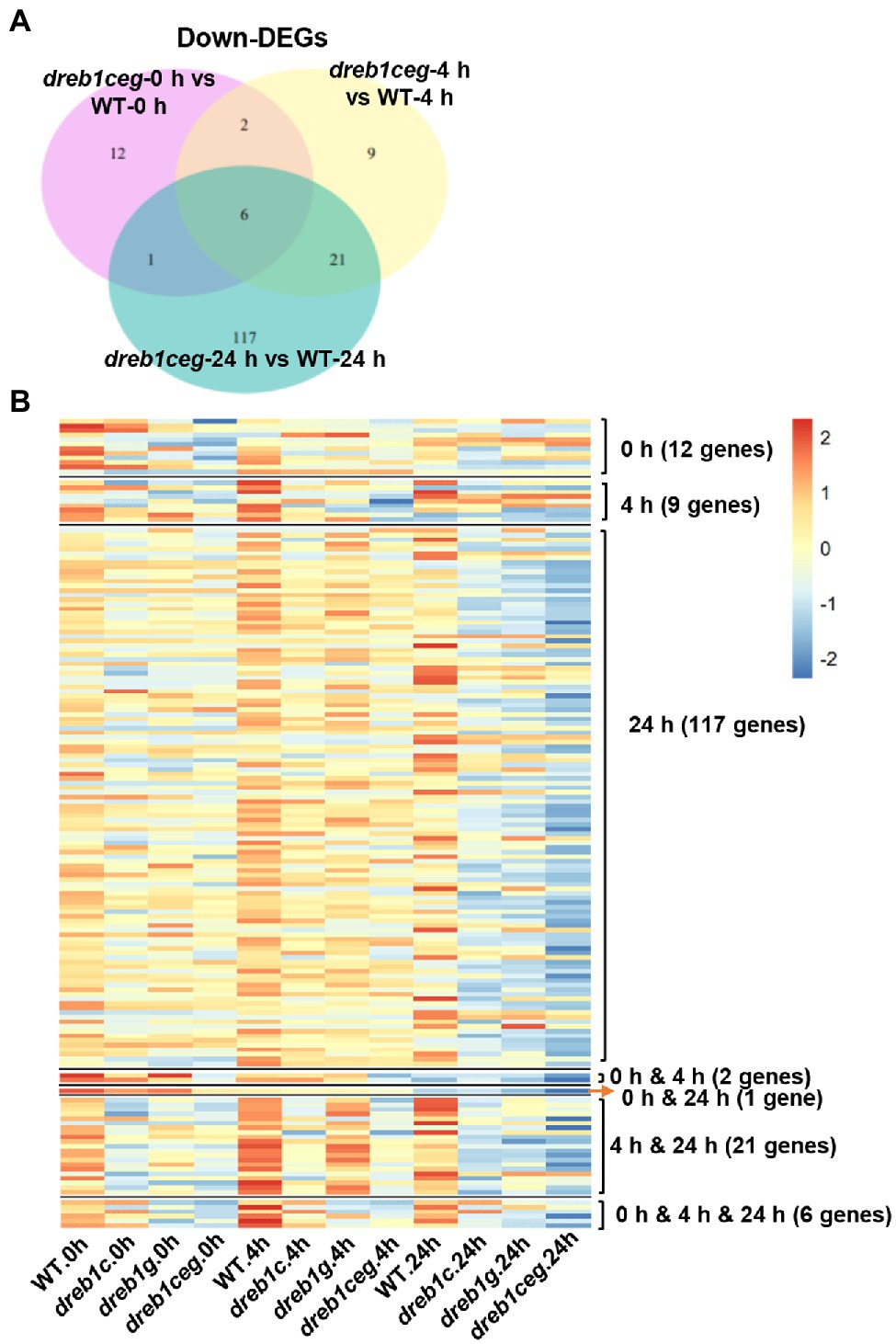
Figure 5. Transcriptome analysis of the dreb1 mutants. (A) Venn diagram indicates the number of downregulated DEGs (fold change ≥ 2, FDR ≤ 0.01) of dreb1ceg versus the WT at 0, 4, and 24 h after chilling treatment at 6°C. (B) Heat map of expression levels of 168 OsDREB1C/E/G-activated genes in the WT and the dreb1 mutants at 0, 4, and 24 h after chilling treatment. The OsDREB1C/E/G-activated genes are defined by a reduced expression in dreb1ceg compared to the WT (fold reduction ≥ 2, FDR ≤ 0.01) at any of the three time points. Heat map is plotted using pheatmap package (1.0.12) and ggplot2 (3.3.3) by R language. Venn diagram is plotted using VennDiagram package (1.6.20) by R language.
The potential function of these 168 potential OsDREB1C/E/G target genes was subject to GO analysis. Among the 95 genes that had a GO annotation in the biological process, no enrichment of GO terms was identified for these genes. However, many of these genes have shared GO terms. Seventy genes had the GO term “metabolic process”, including carbohydrate metabolism, protein metabolism, lipid metabolism, photosynthesis, organic acid metabolism, protein modification process and nucleobase, nucleoside, nucleotide, and nucleic acid metabolic process (Supplementary Figure 8). A total of 33, 16, 37, 7, and 14 genes had the GO terms of “response to stimulus”, “transport”, “cellular process”, “signal transduction”, and “developmental process”, respectively (Supplementary Figure 8). Sixteen and 26 genes had the GO terms of “response to abiotic stimulus”, and “response to stress”, respectively. Two genes were under the term “cell death,” which is a related term of “plant-type hypersensitive response”.
We further examined the expression pattern of these 168 potential OsDREB1C/E/G target genes to determine whether their regulation by OsDREB1C/E/G genes is time-point specific. Heat map analysis revealed that these genes, although identified from a specific time point, mostly had a reduced expression at all three time points (Figure 5B). For the 12 DEGs of dreb1ceg that are unique at 0 h, almost all also had a lower expression in dreb1c (11 genes) and dreb1g (12 genes) than in WT. Ten of these genes had a lower expression in the dreb1c, dreb1g, and dreb1ceg mutants compared to WT at 4 h, and most of them (nine for dreb1c, seven for dreb1g, and five for dreb1ceg) had a lower expression at 24 h. A similar low specificity for time points was also observed for DEGs at 4 and 24 h where they had lower expression at the other two time points in the mutants compared to the WT. In sum, the majority of these genes had decreased expression in the dreb1ceg mutants at all three time points although the fold change is less than 2 and were therefore were not identified as DEGs at all time points.
Next, we search for the presence of sequence motif G/ACCGAC, the DRE/CRT element, in the promoter region of the potential OsDREB1C/E/G target genes. Because some genes do not have defined transcription initiation sites, we used the 1.5 kb fragment 5′ to the translation start codon of these genes as a proxy for promoter fragment. Among the 168 genes, 53 genes contained at least one DRE/CRT element in their promoter region, with 15 genes containing more than one element (Supplementary Table 2). Eight genes have only one element within the 100 bp region 5′ to the translation start codon (Supplementary Table 2), and whether those are in the 5’ UTR region needs further investigation. Function of ten out of these 53 genes have been previously studied. Four of them, OsHAN1, OsDREB1F, OsNAP, and OsGNA1, have been shown to be involved in chilling tolerance or growth. The OsHAN1 gene codes for an oxidase, and it LOF mutant had enhanced chilling tolerance (Mao et al., 2019). OsDREB1F is member of DREB1 family, and its overexpression has been shown to enhance chilling tolerance in rice (Wang et al., 2008). It is interesting that OsDREB1F is a potential target of the OsDREB1C/E/G proteins in the same family. OsNAP encodes a NAC transcription factor, and its overexpression also enhances chilling tolerance rice (Chen et al., 2014). OsGNA1, encoding a glucosamine-6-phosphate acetyltransferase, and its LOF mutant has a temperature-sensitive defect in root elongation (Jiang et al., 2005). In addition, OsGRX2 encodes glutaredoxin that is involved in detoxification processes (Jeong et al., 2018) and could contribute to stress tolerance. Five other genes are involved in growth and developmental processes. OsDof18 is involved in growth and ammonium transport regulation (Wu et al., 2017), OsDjA7/8 is essential for chloroplast development (Zhu et al., 2015), OsNIA2, encoding a nitrate reductase, increases nitrogen uptake capacity (Sun et al., 2015), OsMYB103L is involved in GA-mediated regulation of secondary wall biosynthesis (Ye et al., 2015), and OsSPW1 controls floral organ identity (Nagasawa et al., 2003). Thus, these potential target genes may contribute to chilling tolerance and chilling growth mediated by OsDREB1C/E/G.
OsDREB1C/E/G Genes Promote Tolerance to Heat, Salt and Drought at Seedling Stage
To determine whether these OsDREB1C/E/G genes also play a role in tolerance to other abiotic stresses in rice, the dreb1 mutants were subjected to heat, salt, and drought treatments. The survival rates for the WT NIP and the mutant dreb1 plants grown in the same pot were compared in the same pot to control variability of stress treatment.
For heat stress, 10-day-old seedlings grown on soil were treated at 48°C for 2 days, followed by a recovery growth. The NIP had a survival rate of 36%, while the dreb1ceg mutant plants all died (Figures 6A,B). These data indicate that OsDREB1C/E/G genes are positive regulators of tolerance to heat in rice.
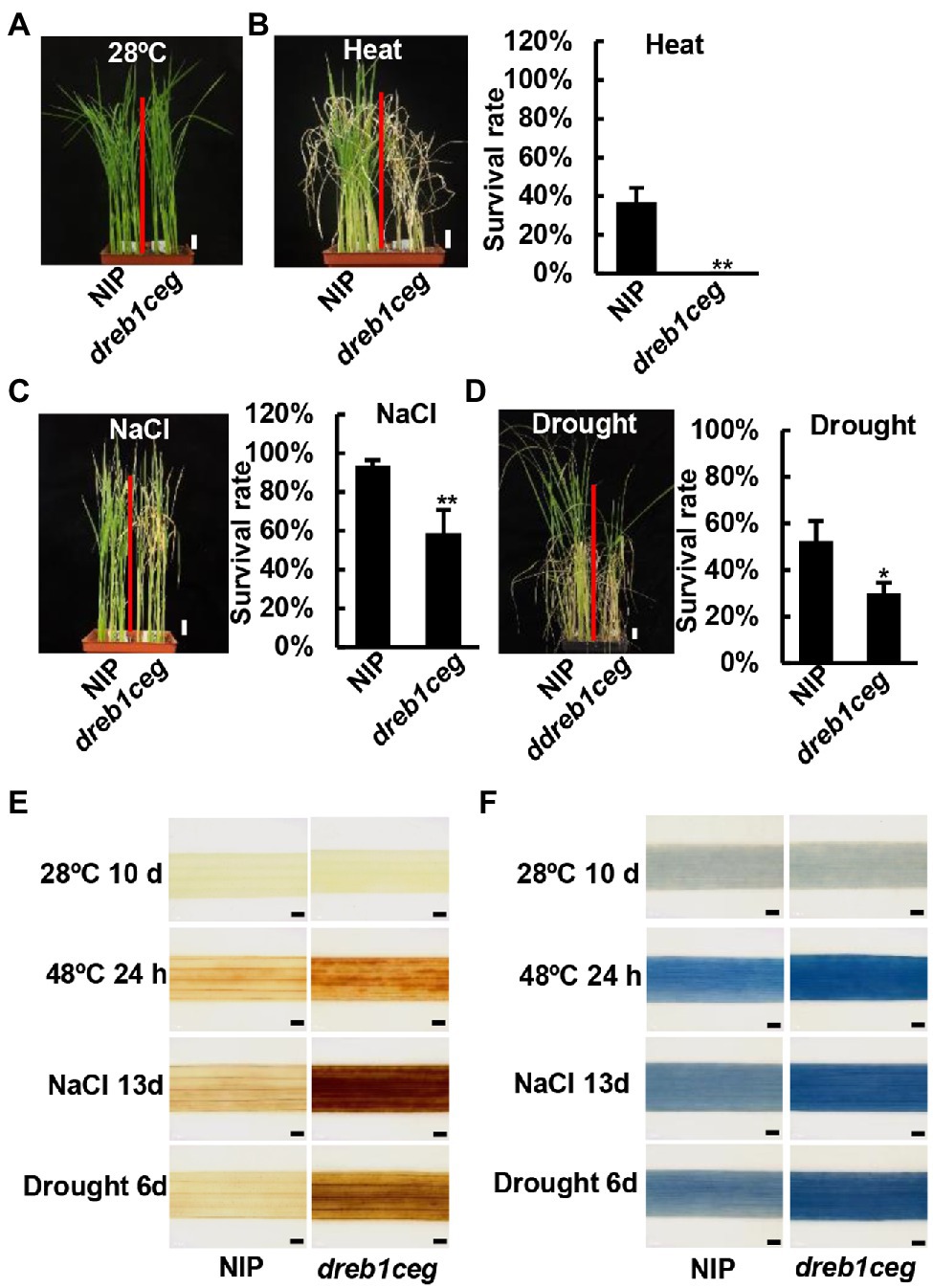
Figure 6. The dreb1ceg mutant is more susceptible to heat, salt and drought stress at seedling stage. (A) Growth phenotype of NIP and dreb1ceg under control condition of 28°C. (B) Growth phenotype and survival rates of NIP and dreb1ceg after heat treatment (48°C for 2 days) and 7-day recovery growth at 28°C. (C) Growth phenotype and survival rates of NIP and dreb1ceg after salt treatment (200 mM NaCl) for 23 days. (D) Growth phenotype and survival rates of NIP and dreb1ceg after drought treatment for 10 days followed by a 7-day recovery. (E,F) DAB (E) and trypan blue (F) staining of the second leaves of NIP and dreb1ceg under control condition (28°C), heat (48°C for 24 h), salt (200 mM for 13 days), and drought stress (6 days of water withheld). Bars in plant images represent 2 cm, and bars in staining images represent 1 mm. Survival rates in (B–D) are expressed as mean ± SD from three biological replicates. * and ** indicate significant differences at p < 0.05, and p < 0.01, respectively, by Student’s t-test.
For salt stress, 3-day-old seedlings were treated with 200 mM NaCl for 21 or 23 days. The survival rates for the four dreb1c mutants were 76%, 71%, 57%, and 61%, respectively, lower than the rate of 92% for NIP (Supplementary Figure 9A). No significant difference in salt tolerance was observed between dreb1e and NIP (Supplementary Figure 9B). The survival rate for dreb1g-1 was 74%, lower than the rate of 96% for NIP (Supplementary Figure 9C). The survival rate for dreb1ceg was 58%, lower than the rate of 93% for NIP (Figure 6C). We further compared salt tolerance of the single and triple mutants. The survival rates for dreb1c-1, dreb1g-1, and dreb1ceg were 42%, 41%, and 35%, respectively, all lower than the rate of 64% for NIP (Supplementary Figure 9D). However, no significant difference was observed between single and triple mutants (Supplementary Figure 9D). These results indicate that the OsDREB1C and OsDREB1G genes, but not the OsDREB1E gene, are positive regulators of salt tolerance in rice.
For drought stress, seedlings at three-leaf stage were withheld of water for 10 days and then re-watered for 7 days. No significant difference in survival rate was observed among dreb1c-2, dreb1e-1, and NIP (Supplementary Figures 10A,B). The survival rate for the dreb1g-1 mutant was 17%, lower than 53% for NIP (Supplementary Figure 10C). The survival rate for the dreb1ceg mutant was 29%, lower than 52% for NIP (Figure 6D). Compared drought tolerance between the dreb1g and dreb1ceg mutant, no significant difference was observed (Supplementary Figure 10D). These data indicate that the OsDREB1G gene but not OsDREB1C or OsDREB1E positively regulates drought tolerance in rice.
Accumulation of ROS and cell death was examined in the dreb1ceg mutant after each of the stress treatments: 24 h of 48°C, 13 days of 200 mM NaCl, and 6 days of water withheld. DAB staining revealed a higher H2O2 accumulation in leaves of the dreb1ceg mutant compared to that in NIP after all three stress treatments: heat, salt, and drought (Figure 6E). Similarly, leaves of the dreb1ceg mutant had more cell death than NIP after these stress treatments as indicated by trypan blue staining (Figure 6F). Therefore, OsDREB1C/E/G genes function to repress ROS overaccumulation and cell death under heat, salt, and drought stresses.
Discussion
DREB1 transcription factors are implicated in stress tolerance, especially cold tolerance. Three members in Arabidopsis, AtDREB1A, AtDREB1B, and AtDREB1C, are key regulators of cold acclimation for freezing tolerance, while AtDREB1D is a positive regulator of drought tolerance. The function of DREB1 proteins in other plant species are largely unexplored except for some overexpression studies in heterologous systems. Here, we report the function of three of the 10 DREB1 genes in rice, OsDREB1C/E/G, through mutant characterizations. These three genes are found to be critical for basal chilling tolerance and low temperature growth in rice. They are also important for stress tolerance to heat, salt and drought. These indicate that DREB1 genes have conserved function in stress tolerance in higher plants. These findings offer an explanation on previous observations that stress tolerance can be improved by overexpressing DREB1 genes in heterologous systems.
The rice OsDREB1C/E/G genes, similar to the Arabidopsis CBF1/2/3 genes, are positive regulators of cold tolerance. However, the Arabidopsis AtDREB1A/B/C genes are required for cold acclimation but not significantly for basal freezing tolerance (Jia et al., 2016). In contrast, the rice OsDREB1C/E/G genes are required for basal chilling tolerance but not for cold acclimation (Figure 4). This may suggest a species-specific function of DREB1 genes in basal cold tolerance and acclimation-induced cold tolerance. It could also result from an expansion of the DREB1 gene family in rice, and other OsDREB1 genes may function in cold acclimation or have overlapping function with OsDREB1C/E/G in cold acclimation. Further investigation on other OsDREB1 genes, especially OsDREB1F, could resolve this question. Alternatively, this differential function in cold acclimation may reflect a difference of cold tolerance between Arabidopsis and rice. Arabidopsis is chilling tolerant (surviving at 4°C), while rice is chilling susceptible (not surviving at 4°C). Cold acclimation for Arabidopsis happens at chilling temperatures such as 4°C to 10°C, while basal chilling tolerance in rice happens at chilling temperatures in the same range. Therefore, the DREB1 genes in Arabidopsis and rice genes may function under the same low temperature range.
Strikingly, we found that OsDREB1C/E/G genes are required for stress tolerance to chilling, salt, drought, and heat stress in rice. Although salt and drought tolerances have been reported for some OsDREB1 genes from overexpression studies, this is the first study reporting a regulation of heat tolerance by any OsDREB1 genes in rice. Not all three genes have a detectable function in all stress responses. While all three genes promote chilling tolerance, OsDREB1C and OsDREB1G promote salt tolerance, while OsDREB1G promotes drought tolerance. More detailed analysis is needed to reveal if these three genes have overlapping functions in each of the stress tolerance. It will also be interesting to determine the function of other seven OsDREB1 genes in stress tolerance.
Transcriptome analysis of the rice dreb1 mutants suggests that chilling tolerance promoted by the OsDREB1C/E/G genes is at least partially through scavenging ROS and reducing cell death. Genes with reduced expression in the dreb1ceg mutant compared to the WT were enriched in the GO terms “plant-type hypersensitive response” and “hydrogen peroxide catabolic process” (Supplementary Figures 5, 6). The dreb1 single mutants, and more so the triple mutant, have more H2O2 accumulation and cell death than the WT (Figures 2G,H). In addition, the dreb1ceg mutant has more H2O2 accumulation and cell death than the WT after treatment of other abiotic stresses (Figures 6E,F). Together, these results indicate that the OsDREB1C/E/G genes may regulate various abiotic stress responses through shared mechanisms such as ROS scavenging and cell death control. Early studies found a higher ROS accumulation and a more robust ROS response in the more chilling tolerant Japonica rice varieties compared to the less tolerant Indica rice varieties (Zhang et al., 2016). Here, a higher ROS in the dreb1 mutants compared to the WT (all in Japonica NIP background) is associated with more chilling susceptibility, suggesting that a controlled ROS accumulation is critical for chilling tolerance. This supports the dual role of ROS as signaling molecules and damaging molecules.
This study identified potential target genes that could mediate the function of OsDREB1C/E/G in chilling tolerance or general stress tolerance. A total of 53 potential OsDREB1C/E/G target genes based on the features that they contain the DRE/CRT element in the promoter region and that they have a lower expression in the dreb1ceg mutant compared to the WT at 0, 4, or 24 h of chilling treatment. This is likely a stringent criterion because some of the direct target genes of Arabidopsis DREB1 proteins do not have a DRE/CRT element in their promoters (Song et al., 2021). Therefore, more genes could be direct regulatory targets of OsDREB1C/E/G proteins. Nevertheless, some of the 53 candidate target genes could mediate the function of OsDREB1C/E/G in chilling tolerance. Among the 10 genes with functional information, some have been shown to promote chilling tolerance or growth. These genes include OsDREB1F, OsNAP, and OsGNA1, all of which have been shown to be positive regulators of chilling tolerance in rice (Jiang et al., 2005; Wang et al., 2008; Chen et al., 2014). Their lower expression in the dreb1ceg mutant after chilling compared to the WT may lead to reduced chilling tolerance. The nitrate reductase coding gene OsNIA2 could also mediate the function of OsDREB1C/E/G in chilling tolerance because nitrate reductase-regulated NO level is positively associated with freezing tolerance in Arabidopsis (Zhao et al., 2009). OsHAN1 is a negative regulator of chilling tolerance (Mao et al., 2019). Its lower expression at 0 h but higher expression in dreb1ceg at 24 h may contribute to chilling susceptibility. In addition, OsDREB1C/E/G proteins could regulate expression of genes involved in ROS and cell death control. One potential target gene OsGRX2 encodes glutaredoxin, and its lower expression in dreb1ceg could be one of the causes of high accumulation of ROS and cell death associated with more chilling susceptibility. Four other candidate target genes had GO terms of “plant-type hypersensitive response” and “hydrogen peroxide catabolic process,” suggesting that regulation on ROS and cell death could be one major mechanism for DREB1C/E/G to promote chilling tolerance. As regulation of ROS and cell death is also key to tolerance to other abiotic stresses, this could also be one mechanism for these proteins to promote tolerance to heat, drought and salt.
Conclusion
We revealed an important role of three out of 10 rice DREB1 genes (OsDREB1C/E/G) in stress tolerance to chilling, heat, salt, and drought in rice. In contrast to the Arabidopsis CBF1/2/3 genes, these rice genes are critical for basal chilling tolerance but not cold acclimation. This study also suggests that ROS scavenging and cell death control might be shared mechanisms for these DREB1 genes to promote tolerance to multiple stresses. This study enhances mechanistic understanding of general stress tolerance in plants and provides genetic materials for enhancing abiotic stress tolerance in crop plants.
Data Availability Statement
The datasets presented in this study can be found in online repositories. The names of the repository/repositories and accession number(s) can be found at: https://www.ncbi.nlm.nih.gov; PRJNA797855.
Author Contributions
JH, BZ, HW, and SL designed the experiments and wrote the manuscript. HW and XG performed the experimental work. HW, YJ, and BW processed and analyzed RNA-Seq data. All authors contributed to the article and approved the submitted version.
Funding
This work is supported by the National Natural Science Foundation of China (31700223 and 31971827), Open Funds of the State Key Laboratory of Plant Physiology and Biochemistry (SKLPPBKF2102), and Jiangsu Collaborative Innovation Center for Modern Crop Production and Cyrus Tang Innovation Center for Crop Seed Industry.
Conflict of Interest
The authors declare that the research was conducted in the absence of any commercial or financial relationships that could be construed as a potential conflict of interest.
Publisher’s Note
All claims expressed in this article are solely those of the authors and do not necessarily represent those of their affiliated organizations, or those of the publisher, the editors and the reviewers. Any product that may be evaluated in this article, or claim that may be made by its manufacturer, is not guaranteed or endorsed by the publisher.
Supplementary Material
The Supplementary Material for this article can be found online at: https://www.frontiersin.org/articles/10.3389/fpls.2022.851731/full#supplementary-material
Footnotes
1. ^https://www.genevestigator.com/
2. ^http://www.genome.arizona.edu/crispr/CRISPRsearch.html
3. ^http://www.berrygenomics.com/
4. ^http://plantgdb.org/OsGDB/
6. ^http://www.pantherdb.org/help/PANTHERhelp.jsp
7. ^https://ricexpro.dna.affrc.go.jp/
References
Aghaee, A., Moradi, F., Zare-Maivan, H., Zarinkamar, F., Irandoost, H. P., and Sharifi, P. (2011). Physiological responses of two rice (Oryza sativa L.) genotypes to chilling stress at seedling stage. Afr. J. Biotechnol. 10, 7617–7621. doi: 10.5897/AJB11.069
Bach-Pages, M., and Preston, G. M. (2018). Methods to quantify biotic-induced stress in plants. Methods Mol. Biol. 1734, 241–255. doi: 10.1007/978-1-4939-7604-1_19
Chen, C., Chen, H., Zhang, Y., Thomas, H. R., Frank, M. H., He, Y., et al. (2020). TBtools: an integrative toolkit developed for interactive analyses of big biological data. Mol. Plant 13, 1194–1202. doi: 10.1016/j.molp.2020.06.009
Chen, X., Wang, Y. F., Lv, B., Li, J., Luo, L. Q., Lu, S. C., et al. (2014). The NAC family transcription factor OsNAP confers abiotic stress response through the ABA pathway. Plant Cell Physiol. 55, 604–619. doi: 10.1093/pcp/pct204
Devireddy, A. R., Tschaplinski, T. J., Tuskan, G. A., Muchero, W., and Chen, J. G. (2021). Role of reactive oxygen species and hormones in plant responses to temperature changes. Int. J. Mol. Sci. 22:8843. doi: 10.3390/ijms22168843
Dubouzet, J. G., Sakuma, Y., Ito, Y., Kasuga, M., Dubouzet, E. G., Miura, S., et al. (2003). OsDREB genes in rice, Oryza sativa L., encode transcription activators that function in drought-, high-salt- and cold-responsive gene expression. Plant J. 33, 751–763. doi: 10.1046/j.1365-313X.2003.01661.x
Fang, C., Zhang, P., Li, L., Yang, L., Mu, D., Yan, X., et al. (2020). Serine hydroxymethyltransferase localised in the endoplasmic reticulum plays a role in scavenging H2O2 to enhance rice chilling tolerance. BMC Plant Biol. 20:236. doi: 10.1186/s12870-020-02446-9
Feng, W. Q., Li, J., Long, S. X., and Wei, S. J. (2019). A DREB1 gene from zoysiagrass enhances Arabidopsis tolerance to temperature stresses without growth inhibition. Plant Sci. 278, 20–31. doi: 10.1016/j.plantsci.2018.10.009
Gao, S. Q., Xu, H. J., Cheng, X. G., Chen, M., Xu, Z. S., Li, L. C., et al. (2005). Improvement of wheat drought and salt tolerance by expression of a stress-inducible transcription factor GmDREB of soybean (Glycine max). Chin. Sci. Bull. 50, 2714–2723. doi: 10.1360/982005-1234
Ge, Q., Zhang, Y. Y., Xu, Y. Y., Bai, M. Y., Luo, W., Wang, B., et al. (2020). Cyclophilin OsCYP20-2 with a novel variant integrates defense and cell elongation for chilling response in rice. New Phytol. 225, 2453–2467. doi: 10.1111/nph.16324
Gilmour, S. J., Fowler, S. G., and Thomashow, M. F. (2004). Arabidopsis transcriptional activators CBF1, CBF2, and CBF3 have matching functional activities. Plant Mol. Biol. 54, 767–781. doi: 10.1023/B:PLAN.0000040902.06881.d4
Gilmour, S. J., Zarka, D. G., Stockinger, E. J., Salazar, M. P., Houghton, J. M., and Thomashow, M. F. (1998). Low temperature regulation of the Arabidopsis CBF family of AP2 transcriptional activators as an early step in cold-induced COR gene expression. Plant J. 16, 433–442. doi: 10.1046/j.1365-313x.1998.00310.x
Guttikonda, S. K., Valliyodan, B., Neelakandan, A. K., Tran, L. S. P., Kumar, R., Quach, T. N., et al. (2014). Overexpression of AtDREB1D transcription factor improves drought tolerance in soybean. Mol. Biol. Rep. 41, 7995–8008. doi: 10.1007/s11033-014-3695-3
Hasanuzzaman, M., Nahar, K., and Fujita, M. (2013). “Extreme temperature responses, oxidative stress and antioxidant defense in plants,” in Abiotic Stress—Plant Responses and Applications in Agriculture. eds. K. Vahdati and C. Leslie (Rijeka: InTech), 169–205.
Hua, J. (2016). Defining roles of tandemly arrayed CBF genes in freezing tolerance with new genome editing tools. New Phytol. 212, 301–302. doi: 10.1111/nph.14183
Jacobs, B. C., and Pearson, C. J. (1999). Growth, development and yield of rice in response to cold temperature. J. Agron. Crop. Sci. 182, 79–88. doi: 10.1046/j.1439-037x.1999.00259.x
Jeong, K., Pantoja, O., Baten, A., Waters, D., Kretzschmar, T., Wissuwa, M., et al. (2018). Transcriptional response of rice flag leaves to restricted external phosphorus supply during grain filling in rice cv. IR64. PLoS One 13:e0203654. doi: 10.1371/journal.pone.0203654
Jia, Y., Ding, Y., Shi, Y., Zhang, X., Gong, Z., and Yang, S. (2016). The cbfs triple mutants reveal the essential functions of CBFs in cold acclimation and allow the definition of CBF regulons in Arabidopsis. New Phytol. 212, 345–353. doi: 10.1111/nph.14088
Jiang, H., Wang, S., Dang, L., Wang, S., Chen, H., Wu, Y., et al. (2005). A novel short-root gene encodes a glucosamine-6-phosphate acetyltransferase required for maintaining normal root cell shape in rice. Plant Physiol. 138, 232–242. doi: 10.1104/pp.104.058248
Kang, H. G., Kim, J., Kim, B., Jeong, H., Choi, S. H., Kim, E. K., et al. (2011). Overexpression of FTL1/DDF1, an AP2 transcription factor, enhances tolerance to cold, drought, and heat stresses in Arabidopsis thaliana. Plant Sci. 180, 634–641. doi: 10.1016/j.plantsci.2011.01.002
Kasuga, M., Liu, Q., Miura, S., Yamaguchi-Shinozaki, K., and Shinozaki, K. (1999). Improving plant drought, salt, and freezing tolerance by gene transfer of a single stress-inducible transcription factor. Nat. Biotechnol. 17, 287–291. doi: 10.1038/7036
Kidokoro, S., Watanabe, K., Ohori, T., Moriwaki, T., Maruyama, K., Mizoi, J., et al. (2015). Soybean DREB1/CBF-type transcription factors function in heat and drought as well as cold stress-responsive gene expression. Plant J. 81, 505–518. doi: 10.1111/tpj.12746
Kumar, S., Stecher, G., and Tamura, K. (2016). MEGA7: molecular evolutionary genetics analysis version 7.0 for bigger datasets. Mol. Biol. Evol. 33, 1870–1874. doi: 10.1093/molbev/msw054
Liu, H., Dong, S., Li, M., Gu, F., Yang, G., Guo, T., et al. (2021a). The class III peroxidase gene OsPrx30, transcriptionally modulated by the AT-hook protein OsATH1, mediates rice bacterial blight-induced ROS accumulation. J. Integr. Plant Biol. 63, 393–408. doi: 10.1111/jipb.13040
Liu, Q., Kasuga, M., Sakuma, Y., Abe, H., Miura, S., Yamaguchi-Shinozaki, K., et al. (1998). Two transcription factors, DREB1 and DREB2, with an EREBP/AP2 DNA binding domain separate two cellular signal transduction pathways in drought- and low-temperature-responsive gene expression, respectively, in Arabidopsis. Plant Cell 10, 1391–1406. doi: 10.1105/tpc.10.8.1391
Mao, D., Xin, Y., Tan, Y., Hu, X., Bai, J., Liu, Z. Y., et al. (2019). Natural variation in the HAN1 gene confers chilling tolerance in rice and allowed adaptation to a temperate climate. Proc. Natl. Acad. Sci. U. S. A. 116, 3494–3501. doi: 10.1073/pnas.1819769116
Mhamdi, A., and Van Breusegem, F. (2018). Reactive oxygen species in plant development. Development 145:dev164376. doi: 10.1242/dev.164376
Mittler, R. (2002). Oxidative stress, antioxidants and stress tolerance. Trends Plant Sci. 7, 405–410. doi: 10.1016/S1360-1385(02)02312-9
Moon, S. J., Min, M. K., Kim, J. A., Kim, D. Y., Yoon, I. S., Kwon, T. R., et al. (2019). Ectopic expression of OsDREB1G, a member of the OsDREB1 subfamily, confers cold stress tolerance in rice. Front. Plant Sci. 10:297. doi: 10.3389/fpls.2019.00297
Morita-Yamamuro, C., Tsutsui, T., Sato, M., Yoshioka, H., Tamaoki, M., Ogawa, D., et al. (2005). The Arabidopsis gene CAD1 controls programmed cell death in the plant immune system and encodes a protein containing a MACPF domain. Plant Cell Physiol. 46, 902–912. doi: 10.1093/pcp/pci095
Nagasawa, N., Miyoshi, M., Sano, Y., Satoh, H., Hirano, H., Sakai, H., et al. (2003). SUPERWOMAN1 and DROOPING LEAF genes control floral organ identity in rice. Development 130, 705–718. doi: 10.1242/dev.00294
Nishimura, A., Aichi, I., and Matsuoka, M. (2006). A protocol for agrobacterium-mediated transformation in rice. Nat. Protoc. 1, 2796–2802. doi: 10.1038/nprot.2006.469
R Core Team. (2021). R: A Language and Environment for Statistical Computing. R Foundation for Statistical Computing, Vienna, Austria.
Sakuma, Y., Liu, Q., Dubouzet, J. G., Abe, H., Shinozaki, K., and Yamaguchi-Shinozaki, K. (2002). DNA-binding specificity of the ERF/AP2 domain of Arabidopsis DREBs, transcription factors involved in dehydration- and cold-inducible gene expression. Biochem. Biophys. Res. Commun. 290, 998–1009. doi: 10.1006/bbrc.2001.6299
Shan, D. P., Huang, J. G., Yang, Y. T., Guo, Y. H., Wu, C. A., Yang, G. D., et al. (2007). Cotton GhDREB1 increases plant tolerance to low temperature and is negatively regulated by gibberellic acid. New Phytol. 176, 70–81. doi: 10.1111/j.1469-8137.2007.02160.x
Song, Y., Zhang, X., Li, M., Yang, H., Fu, D., Lv, J., et al. (2021). The direct targets of CBFs: in cold stress response and beyond. J. Integr. Plant Biol. 63, 1874–1887. doi: 10.1111/jipb.13161
Stockinger, E. J., Gilmour, S. J., and Thomashow, M. F. (1997). Arabidopsis thaliana CBF1 encodes an AP2 domain-containing transcriptional activator that binds to the C-repeat/DRE, a cis-acting DNA regulatory element that stimulates transcription in response to low temperature and water deficit. Proc. Natl. Acad. Sci. U. S. A. 94, 1035–1040. doi: 10.1073/pnas.94.3.1035
Sun, H., Li, J., Song, W., Tao, J., Huang, S., Chen, S., et al. (2015). Nitric oxide generated by nitrate reductase increases nitrogen uptake capacity by inducing lateral root formation and inorganic nitrogen uptake under partial nitrate nutrition in rice. J. Exp. Bot. 66, 2449–2459. doi: 10.1093/jxb/erv030
Thomashow, M. F. (1999). Plant cold acclimation: freezing tolerance genes and regulatory mechanisms. Annu. Rev. Plant. Physiol. Plant. Mol. Biol. 50, 571–599. doi: 10.1146/annurev.arplant.50.1.571
Vonapartis, E., Mohamed, D., Li, J., Pan, W., Wu, J., and Gazzarrini, S. (2022). CBF4/DREB1D represses XERICO to attenuate ABA, osmotic and drought stress responses in Arabidopsis. Plant J. doi: 10.1111/tpj.15713 [Epub ahead of print].
Wang, Q. Y., Guan, Y. C., Wu, Y. R., Chen, H. L., Chen, F., and Chu, C. C. (2008). Overexpression of a rice OsDREB1F gene increases salt, drought, and low temperature tolerance in both Arabidopsis and rice. Plant Mol. Biol. 67, 589–602. doi: 10.1007/s11103-008-9340-6
Wang, J., Ren, Y., Liu, X., Luo, S., Zhang, X., Liu, X., et al. (2021). Transcriptional activation and phosphorylation of OsCNGC9 confer enhanced chilling tolerance in rice. Mol. Plant 14, 315–329. doi: 10.1016/j.molp.2020.11.022
Wu, Y., Yang, W., Wei, J., Yoon, H., and An, G. (2017). Transcription factor OsDOF18 controls ammonium uptake by inducing ammonium transporters in rice roots. Mol. Cells 40, 178–185. doi: 10.14348/molcells.2017.2261
Xing, H. L., Dong, L., Wang, Z. P., Zhang, H. Y., Han, C. Y., Liu, B., et al. (2014). A CRISPR/Cas9 toolkit for multiplex genome editing in plants. BMC Plant Biol. 14:327. doi: 10.1186/s12870-014-0327-y
Yadav, S. K. (2010). Cold stress tolerance mechanisms in plants: a review. Agron. Sustain. Dev. 30, 515–527. doi: 10.1051/agro/2009050
Ye, Y., Liu, B., Zhao, M., Wu, K., Cheng, W., Chen, X., et al. (2015). CEF1/OsMYB103L is involved in GA-mediated regulation of secondary wall biosynthesis in rice. Plant Mol. Biol. 89, 385–401. doi: 10.1007/s11103-015-0376-0
Yoshida, S., Forno, D., and Cock, J. (1971). Laboratory Manual for Physiological Studies of Rice. Manila: The International Rice Research Institute. 83p.
Zhang, Y., Chen, C., Jin, X. F., Xiong, A. S., Peng, R. H., Hong, Y. H., et al. (2009). Expression of a rice DREB1 gene, OsDREB1D, enhances cold and high-salt tolerance in transgenic Arabidopsis. BMB Rep. 42, 486–492. doi: 10.5483/BMBRep.2009.42.8.486
Zhang, Q., Chen, Q., Wang, S., Hong, Y., and Wang, Z. (2014). Rice and cold stress: methods for its evaluation and summary of cold tolerance-related quantitative trait loci. Rice 7:24. doi: 10.1186/s12284-014-0024-3
Zhang, J. Y., Li, X. M., Lin, H. X., and Chong, K. (2019). Crop improvement through temperature resilience. Annu. Rev. Plant Biol. 70, 753–780. doi: 10.1146/annurev-arplant-050718-100016
Zhang, J., Luo, W., Zhao, Y., Xu, Y., Song, S., and Chong, K. (2016). Comparative metabolomic analysis reveals a reactive oxygen species-dominated dynamic model underlying chilling environment adaptation and tolerance in rice. New Phytol. 211, 1295–1310. doi: 10.1111/nph.14011
Zhao, M. G., Chen, L., Zhang, L. L., and Zhang, W. H. (2009). Nitric reductase-dependent nitric oxide production is involved in cold acclimation and freezing tolerance in Arabidopsis. Plant Physiol. 151, 755–767. doi: 10.1104/pp.109.140996
Zhao, C., Zhang, Z., Xie, S., Si, T., Li, Y., and Zhu, J. K. (2016). Mutational evidence for the critical role of CBF transcription factors in cold acclimation in Arabidopsis. Plant Physiol. 171, 2744–2759. doi: 10.1104/pp.16.00533
Zhong, S., Mahmood, K., Bi, Y. M., Rothstein, S. J., and Ranathunge, K. (2017). Altered expression of OsNLA1 modulates pi accumulation in rice (Oryza sativa L.) plants. Front. Plant Sci. 8:928. doi: 10.3389/fpls.2017.00928
Zhou, Y., Chen, M., Guo, J., Wang, Y., Min, D., Jiang, Q., et al. (2020). Overexpression of soybean DREB1 enhances drought stress tolerance of transgenic wheat in the field. J. Exp. Bot. 71, 1842–1857. doi: 10.1093/jxb/erz569
Keywords: OsDREB1, chilling tolerance, cold acclimation, abiotic stress, ROS, cell death, rice
Citation: Wang H, Lu S, Guan X, Jiang Y, Wang B, Hua J and Zou B (2022) Dehydration-Responsive Element Binding Protein 1C, 1E, and 1G Promote Stress Tolerance to Chilling, Heat, Drought, and Salt in Rice. Front. Plant Sci. 13:851731. doi: 10.3389/fpls.2022.851731
Edited by:
Yingfang Zhu, Henan University, ChinaReviewed by:
Tushar Suhas Khare, Savitribai Phule Pune University, IndiaWenjing Yao, Nanjing Forestry University, China
Copyright © 2022 Wang, Lu, Guan, Jiang, Wang, Hua and Zou. This is an open-access article distributed under the terms of the Creative Commons Attribution License (CC BY). The use, distribution or reproduction in other forums is permitted, provided the original author(s) and the copyright owner(s) are credited and that the original publication in this journal is cited, in accordance with accepted academic practice. No use, distribution or reproduction is permitted which does not comply with these terms.
*Correspondence: Baohong Zou, bhz@njau.edu.cn; Jian Hua, jh299@cornell.edu
†These authors have contributed equally to this work