- 1Department of Molecular Genetics and Biotechnology, Yuriy Fedkovych Chernivtsi National University, Chernivtsi, Ukraine
- 2Center of Plant Molecular Biology (ZMBP), Eberhard Karls University of Tübingen, Tübingen, Germany
The Solanum genus, being one of the largest among high plants, is distributed worldwide and comprises about 1,200 species. The genus includes numerous agronomically important species such as Solanum tuberosum (potato), Solanum lycopersicum (tomato), and Solanum melongena (eggplant) as well as medical and ornamental plants. The huge Solanum genus is a convenient model for research in the field of molecular evolution and structural and functional genomics. Clear knowledge of evolutionary relationships in the Solanum genus is required to increase the effectiveness of breeding programs, but the phylogeny of the genus is still not fully understood. The rapidly evolving intergenic spacer region (IGS) of 5S rDNA has been successfully used for inferring interspecific relationships in several groups of angiosperms. Here, combining cloning and sequencing with bioinformatic analysis of genomic data available in the SRA database, we evaluate the molecular organization and diversity of IGS for 184 accessions, representing 137 species of the Solanum genus. It was found that the main mechanisms of IGS molecular evolution was step-wise accumulation of single base substitution or short indels, and that long indels and multiple base substitutions, which arose repeatedly during evolution, were mostly not conserved and eliminated. The reason for this negative selection seems to be association between indels/multiple base substitutions and pseudogenization of 5S rDNA. Comparison of IGS sequences allowed us to reconstruct the phylogeny of the Solanum genus. The obtained dendrograms are mainly congruent with published data: same major and minor clades were found. However, relationships between these clades and position of some species (S. cochoae, S. clivorum, S. macrocarpon, and S. spirale) were different from those of previous results and require further clarification. Our results show that 5S IGS represents a convenient molecular marker for phylogenetic studies on the Solanum genus. In particular, the simultaneous presence of several structural variants of rDNA in the genome enables the detection of reticular evolution, especially in the largest and economically most important sect. Petota. The origin of several polyploid species should be reconsidered.
Introduction
Regions coding for 5S rRNA (rDNA) are present in genomes of all cellular organisms. In eukaryotes, 5S rDNA belongs to the class of moderately repeated sequences and is represented by hundreds or thousands of copies of tandemly arranged repeated units (repeats). 5S rDNA clusters are mostly located in one or two chromosomes, although multiple loci are also found (Volkov et al., 2004; Hasterok et al., 2006; Garcia et al., 2012; Bustos et al., 2020; Vozárová et al., 2021). In contrast to majority of repeated sequences whose functions largely remain uncertain, the activity of 5S rDNA is vital for cells, providing rRNA indispensable for assembly of functional ribosomes. The copy number of rDNA repeats is higher than what is required for rRNA synthesis, and redundant copies of rDNA are transcriptionally silenced (Volkov et al., 2004; Tucker et al., 2010; Layat et al., 2012; Matyasek et al., 2016).
Each 5S rDNA repeat consists of an evolutionarily conserved region encoding 5S rRNA (coding sequence, CDS) and a rapidly evolving intergenic spacer (IGS) (Volkov et al., 2001; Ishchenko et al., 2018; Simon et al., 2018; Qin et al., 2019). The high evolutionary stability of the CDS is the result of purifying selection to maintain the function of 5S rRNA as a component of a large ribosome subunit (Vizoso et al., 2011; Mahelka et al., 2013).
Transcription of 5S rDNA is provided by RNA polymerase III (Pol III) and corresponding transcription factors (TFs). The Pol III promoter consists of internal and external elements. The internal elements of the promoter, A-Box, IE, and C-Box, are located in the CDS and represent targets for TFs, which are necessary for the recruitment of Pol III to the transcription initiation complex (Douet and Tourmente, 2007; Layat et al., 2012). Respectively, mutations in internal elements of the promoter should not only disturb the structure of 5S rRNA and ribosome but also affect binding of TFs to the promoter and, thus, the expression of 5S rDNA.
In contrast to the CDS, the main part of the IGS is not transcribed and probably does not have any function. Accordingly, it is believed that any mutation in the IGS is selectively neutral; therefore, this region evolves with a high rate. However, it was found that the IGS of Arabidopsis thaliana contains short-sequence motifs involved in initiation (external elements of the promoter) and termination (terminator) of 5S rDNA transcription (Douet and Tourmente, 2007; de Souza et al., 2020). Thus, one would expect that these regions are to be relatively conservative, as has been demonstrated in several taxonomic groups (Falistocco et al., 2007; Tynkevich and Volkov, 2014; Tynkevich et al., 2015; Mlinarec et al., 2016; Ishchenko et al., 2018; Alexandrov et al., 2021). However, the existing knowledge of the organization of external promoter elements and their molecular evolution is still incomplete.
In many diploid species, numerous copies of rDNA repeats in the same genome tend to be nearly identical because of sequence homogenization (Volkov et al., 1999b,2001, 2003), i.e., individual copies of repeated elements do not evolve independently but in a concerted manner (Arnheim et al., 1980; Coen et al., 1982). To explain the high intragenomic similarity of 5S rDNA repeats, the “concerted evolution” and “birth and death” hypotheses were proposed. The mechanisms and intensity of homogenization may differ in different taxa and for different groups of repeated sequences, e.g., for 5S and 35S rDNA (Pinhal et al., 2011; Vizoso et al., 2011; Song et al., 2012; Mahelka et al., 2013; Galián et al., 2014; Barman et al., 2016; Volkov et al., 2007, 2017; Chen et al., 2021).
Additive inheritance of both parent variants of 5S and 35S rDNA is usually observed in the first generation of interspecific hybrids/allopolyploids. However, in ancient allopolyploids, 35S rDNA can be the subject of interlocus sequence conversion (Volkov et al., 1999a,b, 2007; Vizoso et al., 2011), while different variants of 5S rDNA can coexist in a plant genome for a long time without being homogenized (Fulnecek et al., 2002; Song et al., 2012; Mahelka et al., 2013; Mlinarec et al., 2016; Volkov et al., 2017; Ishchenko et al., 2018). Especially, a significant sequence divergence was found for spatially distant 5S rDNA variants that are located in different loci of the same chromosome set (Cronn et al., 1996; Vozárová et al., 2021) and for different parent loci in genomes of hybrid origin (Fulnecek et al., 2002; Matyasek et al., 2002), while repeats from the same locus appeared to be highly homogenized. Accordingly, 5S rDNA became an attractive focus for investigation of molecular evolution of repeated sequences, identification of hybrids, and phylogenetic studies on angiosperms (Blöch et al., 2009; Baum et al., 2012; Simeone et al., 2018; Tynkevich and Volkov, 2019; Ishchenko et al., 2020, 2021; Cardoni et al., 2021; Vozárová et al., 2021). However, 5S rDNA is still poorly characterized in many important plant groups such as the Solanum L. genus.
The Solanum (nightshade) genus is an attractive model for comparative genomics and investigation of molecular evolution of repeated sequences. With around 1,200 species, it belongs to the so-called “giant genera” and is the fifth largest genus of flowering plants (Frodin, 2004; Echeverría-Londoño et al., 2020). Solanum species are distributed worldwide from tropical to temperate areas and grow under diverse ecological conditions. Most Solanum species inhabit the New World, although secondary centers of diversity have been found in Africa, Asia, and Australia (D’Arcy, 1991). Overall, the Solanum genus is an example of unusual hyperdiversity in life forms, morphological features, and ecological preferences, representing a unique system for studying the diversification of plants (Knapp et al., 2004; Echeverría-Londoño et al., 2020). The genus includes important crops such as S. tuberosum L. (potato), S. lycopersicum L. (tomato), and S. melongena L. (brinjal eggplant, aubergine), about 20 cultivated species of local significance like S. aethiopicum L. (Ethiopian eggplant), S. betaceum Cav. (tamarillo), S. muricatum Aiton (pepino), and S. quitoense Lam. (lulo), as well as several medicinal and ornamental plants (S. marginatum L.f., S. aviculare G. Forst., S. mammosum L., and S. pseudocapsicum L.).
In the Solanaceae family, the Solanum genus belongs to the strongly supported large “x = 12” clade (Olmstead et al., 2008). The most common chromosome number in Solanum is x = 12, which occurs in 97% of species examined, such as diploids (77%), tetraploids (14%), hexaploids (4%), triploids (2%), and octoploids (0.2%). Application of in situ hybridization showed that 55 out of 64 (85.9%) diploid species possess only one 5S locus per chromosome set (Chiarini et al., 2018). Up to now, the molecular organization and evolution of the 5S rDNA in the genus Solanum have only been analyzed in about 35 species and breeding lines (Volkov et al., 2001; Davidjuk et al., 2010; Sun et al., 2014). In this study, combining cloning and sequencing with analysis of available genomic data in the Sequence Read Archive (SRA) public database, we evaluate the molecular organization, diversity, and evolution of the IGS for 184 plant accessions, representing 137 species across the Solanum genus. Especially, our results shed a new light on the phylogeny of the genus and reticulate evolution of the largest and economically most important sect. Petota.
Materials and Methods
Plant Material and DNA Extraction
A plant material of the Solanum species was obtained from several collections (see Tables 1, 2). A plant material of out-group species, Lycianthes lycioides (L.) Hassl. and Physalis peruviana L. (acc. no. NK-03), was obtained from Orto Botanico di Padova (Italy) and National Botanical Garden of National Academy of Sciences of Ukraine (Kyiv, Ukraine), respectively.
Total genomic DNA was isolated from herbarium specimens according to the CTAB method of DNA extraction (Porebski et al., 1997). In addition, DNA was treated with Proteinase K (Sigma-Aldrich, United States).
Amplification, Cloning, and Sequencing of 5S rDNA Repeats
Repeated units of 5S rDNA were amplified using the primers Pr5S-L and Pr5S-R, complementary to the 5S rRNA CDS. These primers provide amplification of complete 5S IGS and flanking regions of the CDS (Volkov et al., 2001). PCR amplification was performed as described previously (Tynkevich and Volkov, 2019). PCR products were ligated into plasmid vector pJET 1.2/blunt using CloneJET PCR Cloning Kit (Thermo Fisher Scientific, United States). Screening of recombinant clones and size selection of inserts were performed by colony PCR with pJET 1.2 forward and reverse primers. Two to eight clones per plant accession were Sanger-sequenced by LGC Genomics (Germany). Primary processing of nucleotide sequences and calculation of sequence similarity levels were performed using the Chromas software and the DNASTAR software package. The obtained sequences were deposited in the GenBank database under the accession numbers listed in Table 3.
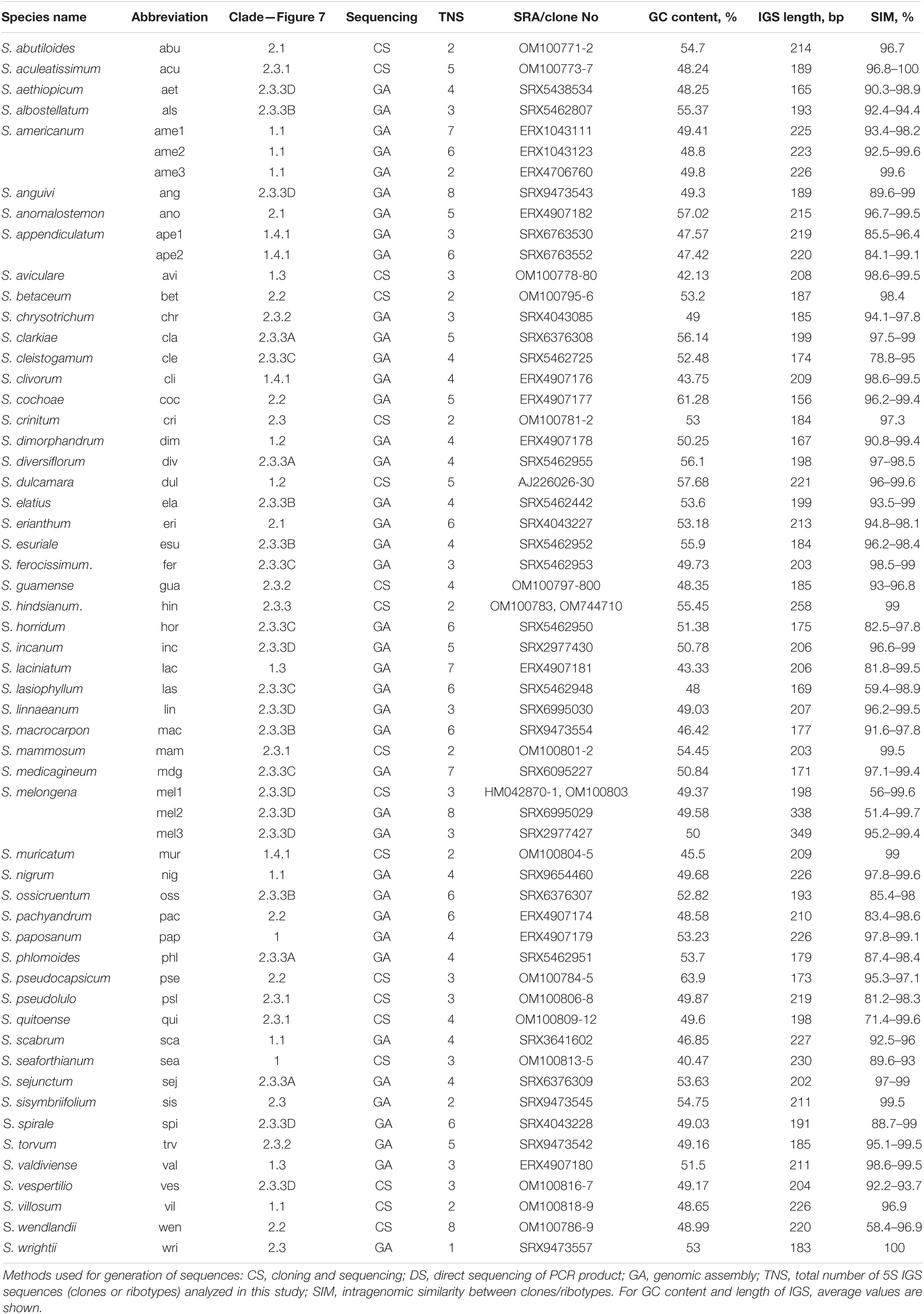
Table 3. Characteristics of the 5S intergenic spacer region (IGS) of Solanum species analyzed (excluding sect. Petota).
Assembly of 5S rDNA Repeats From Illumina Short Reads
De novo assembly of 5S rDNA repeats was performed using libraries of pre-filtered paired or single Illumina reads from raw data of Solanum species genomes available in SRA (Tables 3, 4). Read filtering was carried out using the built-in tool on the sequence download page: https://trace.ncbi.nlm.nih.gov/Traces/sra/sra.cgi?view=search_seq_name. To filter reads containing 5S rDNA fragments, 20-bp long fragments of CDS were used for matching. De novo assembly was conducted using SeqMan NGen 14 (DNASTAR Lasergene suite). Libraries of filtered reads were automatically trimmed for quality, and the following assembly parameters were used: mer size 31, minimum match percentage 100%, and coverage threshold 100 reads. In the obtained contigs with highest coverage from 2 to 12, 5S rDNA repeats that contain one full IGS flanked by two fragments of CDS were identified and collected.
Prediction of 5S rRNA Secondary Structure
Hypothetical secondary structures of potential 5S rRNA transcripts were predicted using the Fold online tool in the RNAstructure server (Reuter and Mathews, 2010).1 Lowest free energy structures were calculated using the following default parameters: temperature (in K) 310.15; maximum loop size 30; minimum helix length 3.
Median-Joining Network and Phylogenetic Analysis
Relationships among IGS sequences of the Solanum species were analyzed applying the median-joining network approach implemented in SplitsTree 5 (Huson and Bryant, 2006). Alignments of the IGS sequences were performed in the MAFFT server using the G-INS-I method, which is most suitable for sequences with global homology (Katoh et al., 2019).
For alignment of the IGS sequences of Solanum species belonging to different taxonomic groups in the genus, we applied the E-INS-I method implemented in the MAFFT server (Katoh et al., 2019). The generated alignment was checked and adjusted manually with the UGENE software.
The best-fit nucleotide substitution model was estimated with the lowest value of Bayesian Information Criterion (BIC) using the Find Best-Fit Substitution Model tool in Mega X (Kumar et al., 2018). A maximum likelihood (ML) phylogenetic tree was generated with the PhyML plugin for Geneious Prime 2021.0.3.2 The IGS sequences of L. lycioides and Ph. peruviana produced in this study (acc. nos. OM100793-4 and OM744711-3) as well as those of four Capsicum species available in GenBank (C. baccatum L.: AF217951, C. frutescens L.: AF217952, C. chinense Jacq.: AF217953, and C. pubescens Ruiz and Pav.: AF217954) were used as outgroups. Branch support was calculated by approximate likelihood ratio tests, aLRT-Chi2 (Anisimova and Gascuel, 2006), and bootstrap analysis with 1,000 resampling replicates. Phylogenetic analysis was also performed by Bayesian inference using the MrBayes 2.2.4 plugin for Geneious Prime 2021.0.3. Four independent Monte Carlo Markov Chains (MCMCs) of 1,000,000 iterations each were run to generate phylogenetic trees with Bayesian posterior probabilities. Trees were sampled every 500 generations. The resulting trees were exported in Newick format and annotated using “Interactive tree of life” (iTOL v6).
Results
Cloning of 5S rDNA Repeats
5S rDNA repeats of 17 Solanum species representing different taxonomic groups were amplified by PCR using primers complementary to the coding region, cloned, and sequenced (Table 3 and Supplementary Material). Analysis of the obtained sequences showed that majority of the clones contained IGS flanked on both sides by fragments of the coding region including the primers used for PCR. Besides, we obtained 5S rDNA clones of S. vespertilio and S. pseudocapsicum that contain rDNA dimers, i.e., two adjacent copies of IGS, and the whole sequence of the CDS between them. Also, two clones containing 5S rDNA dimers and one clone containing a trimer were sequenced for S. wendlandii.
Intragenomic Diversity of Intergenic Spacer: In-Depth Analysis of Sequence Read Archive Data
In order to assess the intragenomic variability of 5S rDNA, we evaluated how many different types/variants of repeated units (ribotypes) are present in genomes of the Solanum species. Genomes of three diploid species, S. lycopersicum-3 (SRX5538725), S. stenotomum-2 (SRX4645231) of sect. Petota, and S. melongena-2 (SRX6995029) of sect. Melongena, were selected for detailed analysis. For these genomes, we assembled de novo 5S rDNA repeats composed of complete IGS and two flanking fragments of CDS. If the CDS contained indels or several SNP, the repeat was considered a pseudogene and excluded from further analysis. Variants of IGS that differed in at least one SNP were considered as distinct ribotypes. The total number of IGS ribotypes was 45, 177, and 31 in S. lycopersicum-3, S. stenotomum-2, and S. melongena-2, respectively. In order to visualize the intragenomic diversity of the ribotypes found in the three species, median-joining networks were constructed (Figure 1).
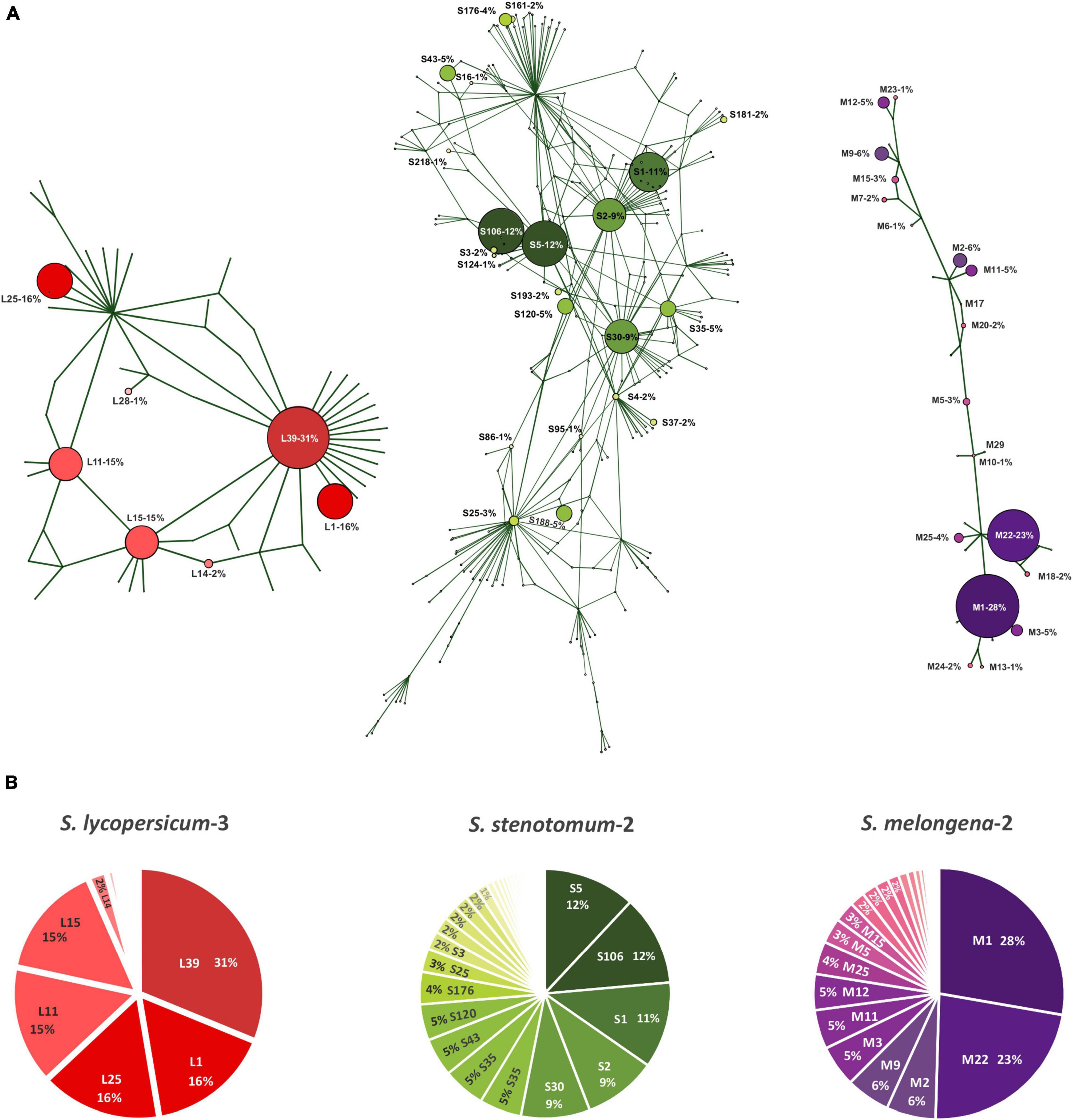
Figure 1. Analysis of 5S rDNA intragenomic diversity in diploid Solanum species. (A) Median joining networks for IGS types/variants (ribotypes) of S. lycopersicum-3, S. stenotomum-2, and S. melongena-2. Ribotypes are designated by the first letter of corresponding species name with index numbers. The size of the circles is proportional to the relative content (in %) of each ribotype in the genome. (B) Relative content (in %) of ribotypes.
After that, we mapped the reads of complete genomic libraries to the reference sequences of all collected ribotypes in order to estimate their relative content in the genomes. The obtained results showed that the IGS ribotypes differ significantly in this parameter. Accordingly, we classified the ribotypes as major (≥10% of all IGS copies present in the genome), minor (<10 but ≥5%), or rare (<5%). The number of major, minor, and rare ribotypes is 5, 0, and 40 in S. lycopersicum-3: 3, 5 and 169 in S. stenotomum-2; 2, 5, and 24 in S. melongena-2 (Figure 1). Altogether, the major and minor ribotypes represent 93, 68, and 78% of all rDNA repeats present in the genomes of these three species. Based on the results obtained, in the further analysis of 5S rDNA in other species, we considered only major and minor ribotypes. The variability of IGS sequences in each examined sample is given in Supplementary Material.
Length and GC Content of the 5S rDNA Repeated Units
Using sequences of clones and major + minor ribotypes, we determined GC content in the IGS of the Solanum species (Tables 3, 4) and found that this value ranges from 40.5% in S. seaforthianum to 63.9% in S. pseudocapsicum. In 90% of the species, intragenomic difference in GC content between individual ribotypes and clones was less than 4%. A greater difference was observed in repeats that were subjected to deletions, particularly in the AT-rich region of the IGS. No significant changes in GC content were found for taxonomic groups in the Solanum genus, suggesting that this parameter remained relatively constant during evolution.
The typical length of IGS in members of the Solanum genus is about 190–220 bp (Tables 3, 4). The shortest IGS were found in S. cochoae, 155–158 bp, and in S. aethiopicum, 162–175 bp. In S. lasiophyllum, however, one ribotype (las-C2R1) is even shorter, 115 bp, although five other ribotypes in this species are 180 bp in length. The longest IGSs were found in S. melongena, 344–360 bp, and in S. lycopersicum, 234–235 bp. The extremely long IGS length in S. melongena is associated with large duplication of the spacer sequence. There is no significant difference in IGS length among the taxonomic groups in the Solanum genus. In general, our data show that the length remained largely unchanged during the evolution of the Solanum genus.
Long Duplication in the Intergenic Spacer of S. melongena
Two structural variants of IGS, long (∼350 bp) and short (∼200 bp) were identified in S. melongena-2 (mel2). The long variant was found in three accessions, mel1 (analyzed by cloning and sequencing) and in mel2 and mel3 (extracted from SRA), while the short variant was only detected in mel1 and mel2. In mel2, all major and minor as well as majority of rare ribotypes belong to the long variant, while the short variant is only represented by two rare ribotypes, M17 and M29 (Figure 1B), whose relative content in the genome is below 1%.
Numerous single nucleotide polymorphisms (SNPs) and two oligonucleotide indels are present in the M29 sequence, so this ribotype appears to be a pseudogene. The ribotype M17 (mel2-C17R1, see Supplementary Material) also contains several SNPs compared to other ribotypes of S. melongena. Interestingly, this ribotype is identical to the most common ribotype of a closely related species, S. linnaeanum.
A detailed sequence analysis showed that the long variant contains a 146-bp-long tandem duplication in the central part of the IGS (Figure 2). The duplicated region consists of a 32-bp-long 3′-fragment of the coding region and an adjacent 114-bp fragment of the IGS. Two copies of the duplicated segment differ by 6 SNPs and one 8-bp-long indel. All mutations are localized in the fragment of the IGS, not in the coding region.
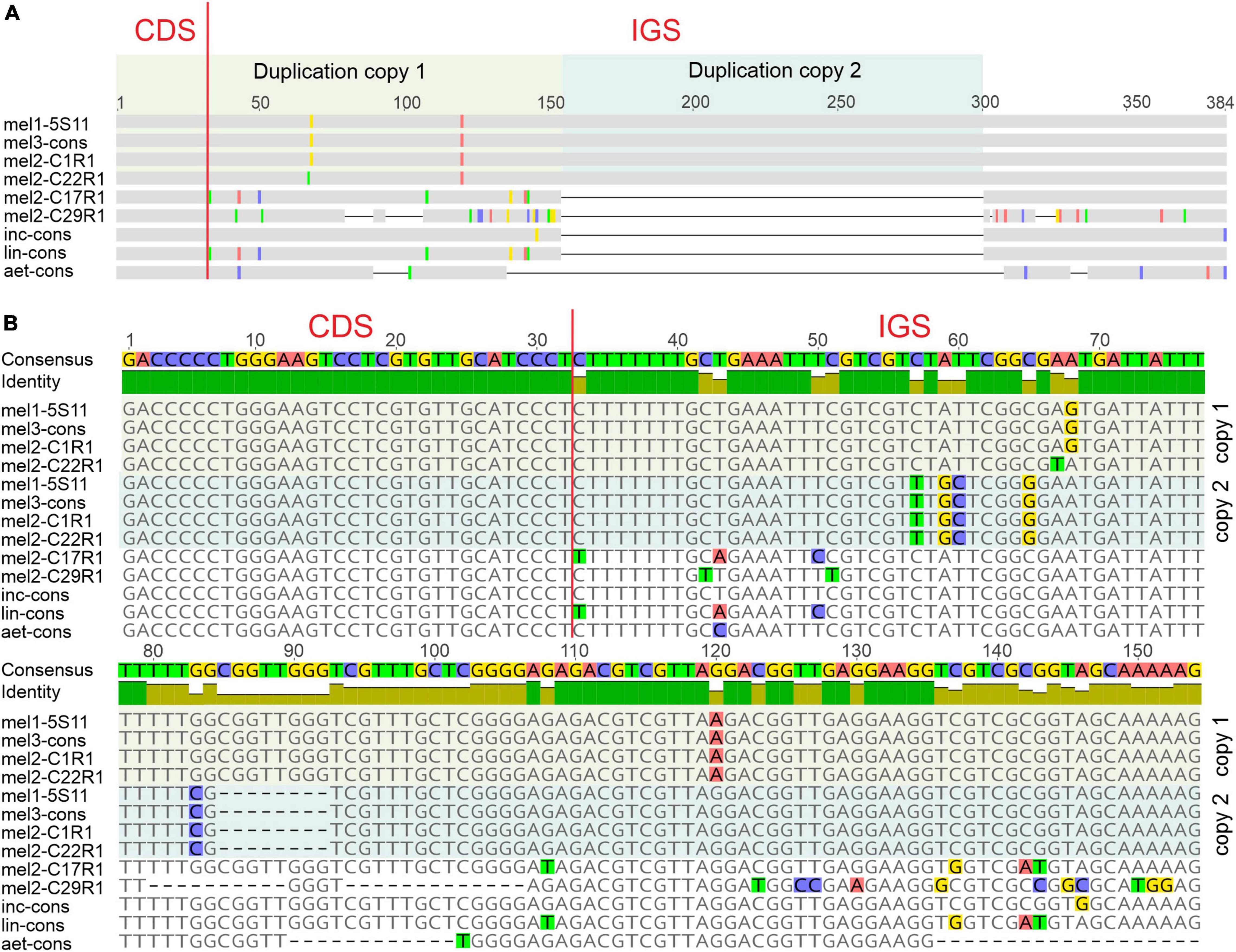
Figure 2. (A) Schematic representation of the molecular organization and (B) sequence alignment of the 3′ fragment of the coding sequence (CDS) and the complete intergenic spacer region (IGS) the of 5S rDNA of S. melongena (mel) and closely related species S. aethiopicum (aet), S. incanum (inc), S. linnaeanum (lin). Two copies of duplication are shown separately and highlighted in different colors. Ribotype and clone names are shown after abbreviations of species names; cons, consensus sequence.
High Diversity of 5S rDNA in S. wendlandii
For the 5S rDNA of S. wendlandii, we sequenced four clones, pSowen-3,-13,-14, and-18, which bore inserts of different lengths, 732, 912, 319, and 657 bp, respectively. Sequence analysis showed that the shortest insert contains one copy of IGS flanked by CDS fragments. The longer inserts represent two dimers and a trimer composed of adjacent copies of 5S rDNA repeats (Figure 3A).
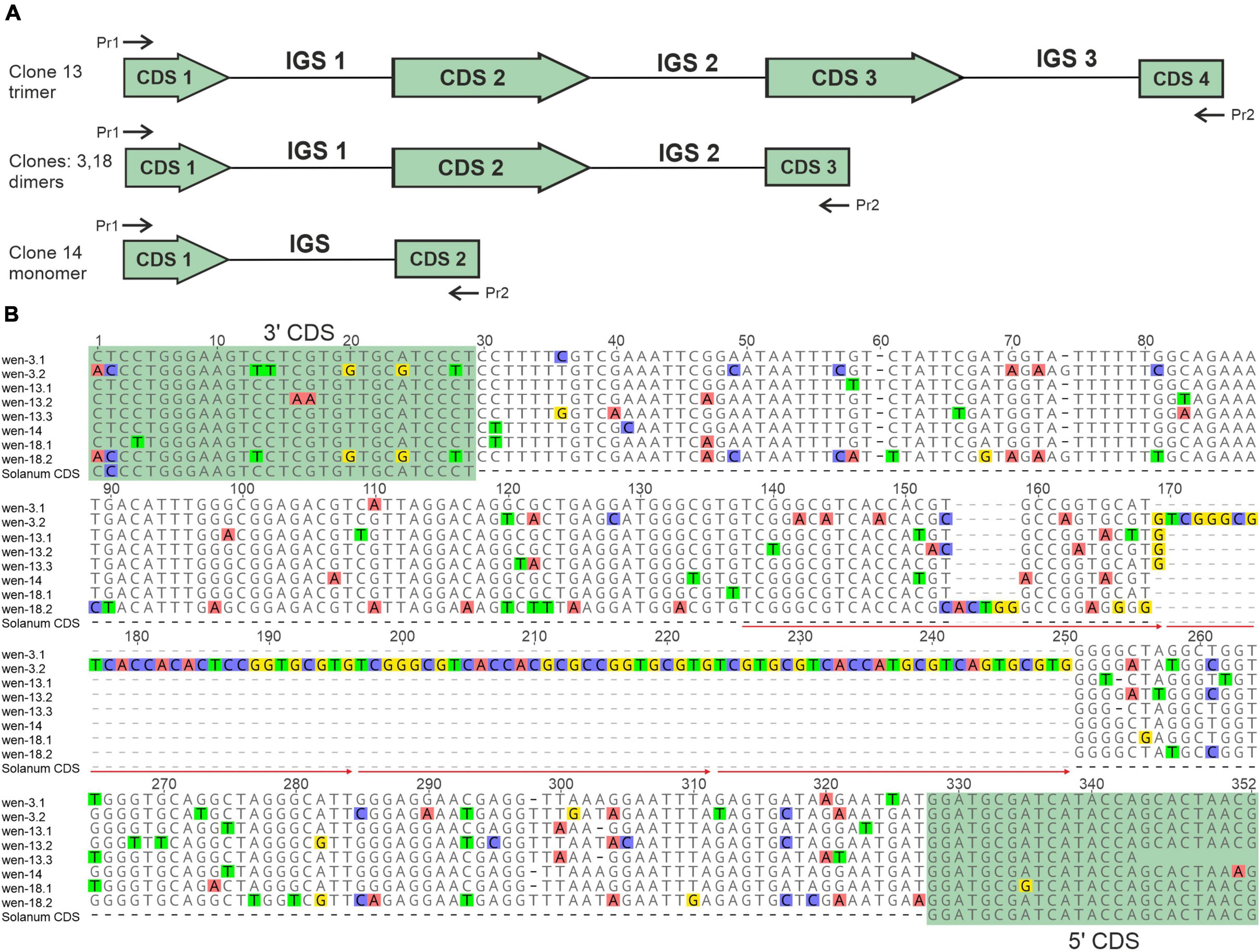
Figure 3. Molecular organization of 5S rDNA repeats in S. wendlandii (wen). (A) General organization of 5S rDNA clones. Pr1 and Pr2, position of primers Pr5S-L and Pr5S-R used for PCR/cloning. (B) Sequences alignment of IGS and flanking fragments of CDS. The consensus sequence of CDS of the genus Solanum (Solanum CDS) is shown for comparison. The arrows indicate the location of repeated motifs.
The sequence alignment revealed an obvious difference among IGS sequences of the adjacent 5S rDNA copies (Figure 3B), which is due to numerous nucleotide substitutions and insertions of different lengths of 1–82 bp. The 82-bp-long insertion harbors three tandem copies of the adjacent sequence, which is normally present once in the IGS. The level of sequence similarity among the compared IGS copies ranges from 58.4 to 96.9%, which indicates high intragenomic heterogeneity of the IGS in S. wendlandii.
Comparison of the 5S rRNA CDS of S. wendlandii and several Solanum species representing different intrageneric clades revealed that the CDS is, as expected, highly conserved in the genus. Analysis of the 5S rDNA clones/ribotypes of several Solanum species showed that a single CDS usually contains no more than two mutations compared to the respective consensus sequence (data not shown), which agrees well with the observation on other plant taxa (Park et al., 2000; Mahelka et al., 2013). In contrast, the complete CDS sequences of S. wendlandii each contain 5–16 base substitutions (Figure 4A).
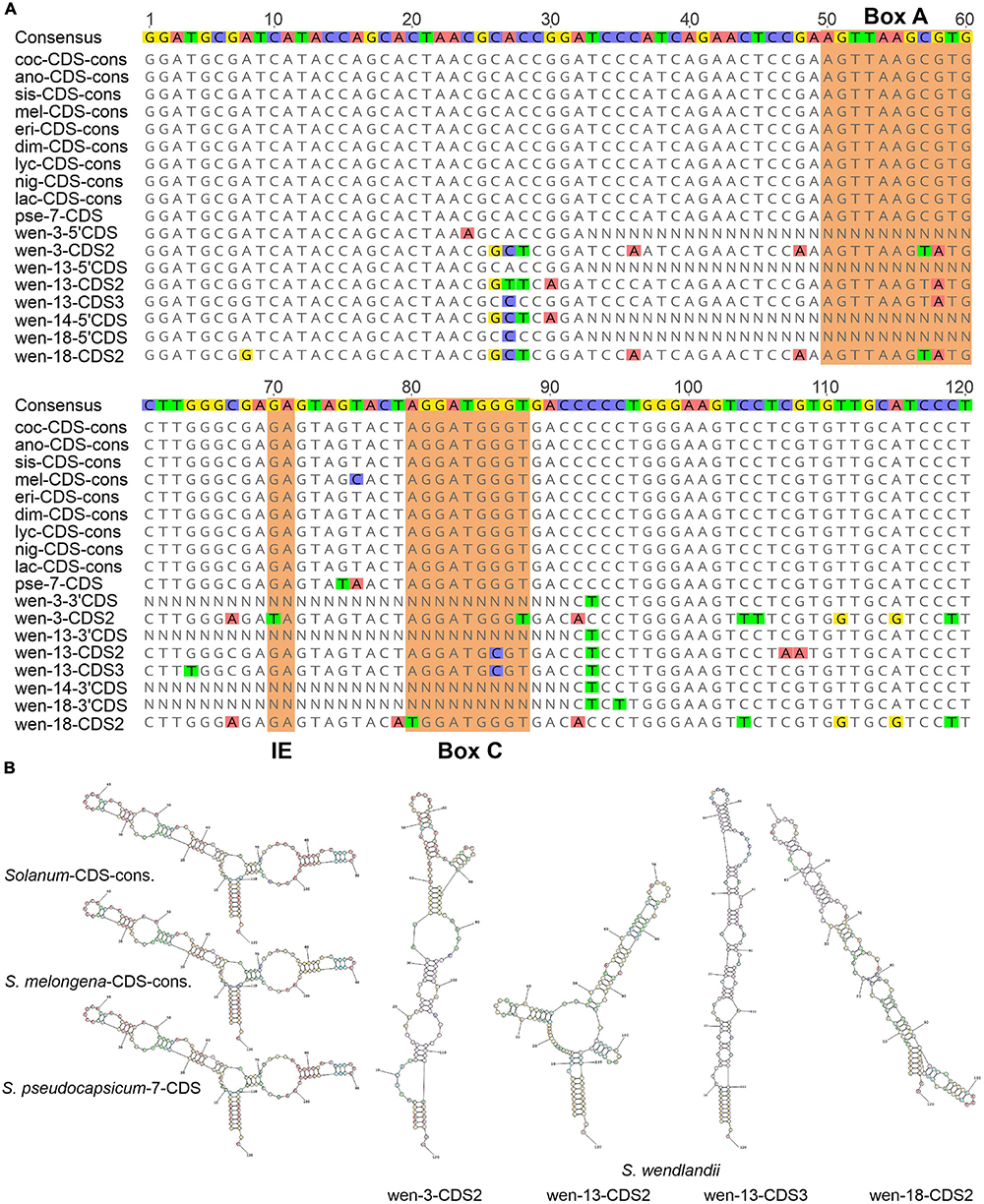
Figure 4. Comparison of Solanum 5S rRNA CDS. (A) Alignment of the CDS of distantly related Solanum species and 5S rDNA clones of S. wendlandii. (B) Predicted secondary structures of 5S rRNA transcripts. Abbreviations of species names are given in Table 1.
The presence of numerous mutations in the CDS suggests its transformation into a pseudogene. To test this possibility, we calculated the secondary structure for transcripts of the complete CDS from the clones pSowen-3,-13, and-18. For comparison, the secondary structure was also calculated for (i) the total consensus CDS of the Solanum genus, (ii) consensus CDS of S. melongena, which differs from the total consensus by one base substitution, and (iii) CDS of S. pseudocapsicum (dimer clone pSpse-5S7), which contains two base substitutions (Figure 4B). The sequences examined formed a secondary structure typical for 5S rRNA (Sun and Caetano-Anollés, 2009), with the exception of the CDS of S. wendlandii, which appeared to be significantly changed, suggesting that the transcripts cannot fulfill their function in the ribosome.
Hence, the 5S rDNA of S. wendlandii appears to be very heterogeneous in both the IGS and CDS regions and likely contains numerous pseudogenes. Unfortunately, the complete genome sequence of S. wendlandii is currently not presented in the GeneBank and cannot, therefore, be used to further elucidate the unusual organization of 5S rDNA in this species.
Intergenic Spacer Organization in Distantly Related Solanum Species
To reveal the molecular organization and evolution of IGS in Solanum, we compared the IGS sequences of 37 species representing distantly related groups (D’Arcy, 1991; Nee, 1999; Bohs, 2005; Särkinen et al., 2013) of the genus (Figure 5). The total length of the alignment obtained is 287 bp. Only 9 identical nucleotides were found in the compared sequences, and average pairwise identity value was 55.1%, indicating significant divergence of the IGS in the genus. Multiple base substitutions and indels of various lengths are scattered along the entire IGS in the species studied compared to the consensus sequence. The largest 31-bp-long indel is located in the central part of the IGS between the positions 144 and 174 bp. Despite numerous species-specific mutations, the sequence of the central indel shows an obvious sequence similarity in the species compared. Analysis of the phylogenetic dendrogram obtained by comparing IGS sequences (Figure 6, see also below) revealed that the central indel is present in the species belonging to major clade 1 (with the exception of S. muricatum) but is partially or completely absent in members of clade 2 (with the exception of S. anomalostemon). Hence, the central indel was present in the common ancestor of the Solanum genus and was later lost in some species during the course of evolution.
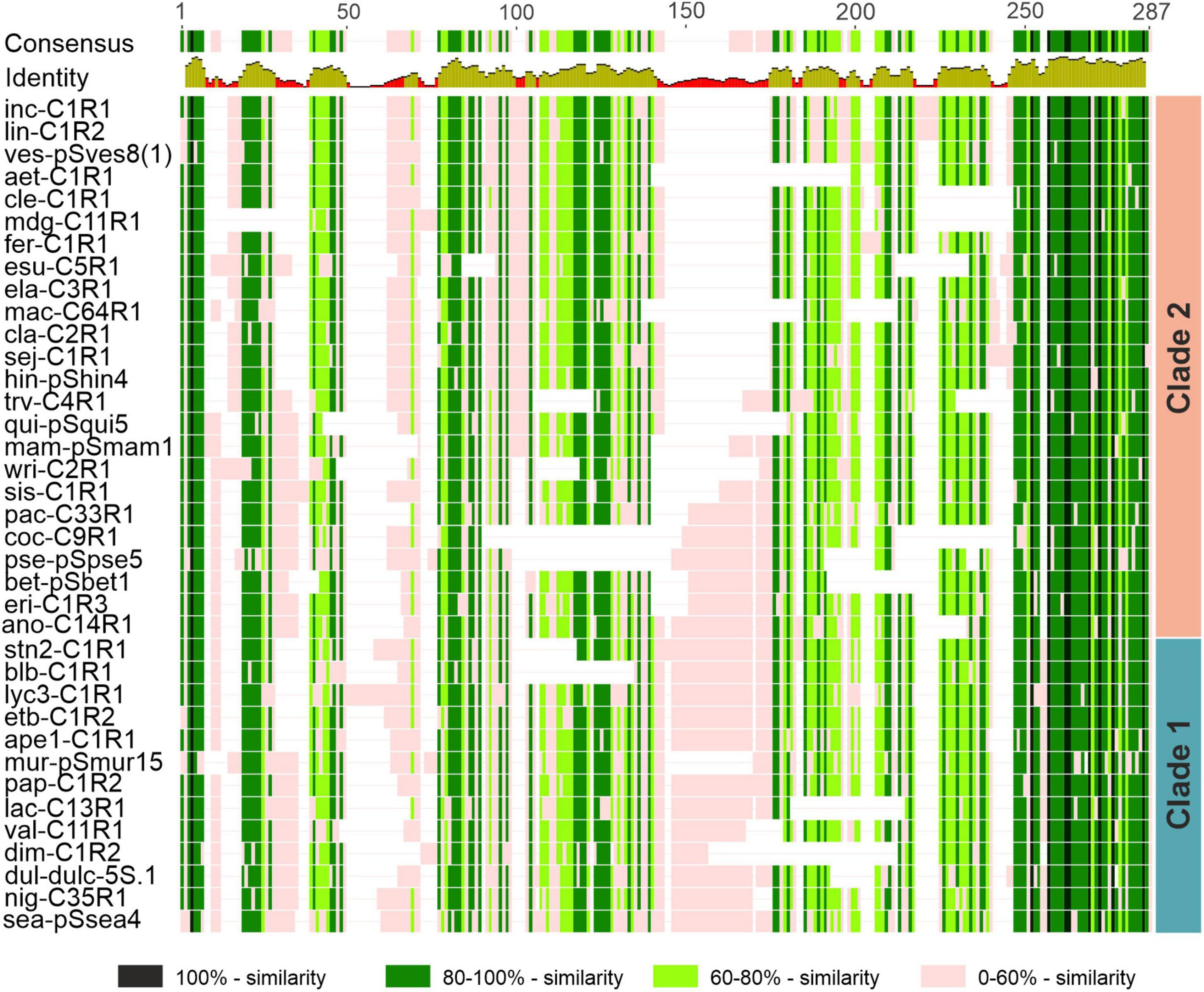
Figure 5. Schematic representation of the sequence alignment of the 5S rDNA IGS of distantly related Solanum species. The level of sequence similarity is shown in different colors. Abbreviations of species names are given in Table 1. Taxonomic assignment of species to Clades 1 and 2 in the maximum likelihood (ML) dendrogram (see Figure 7) is shown.
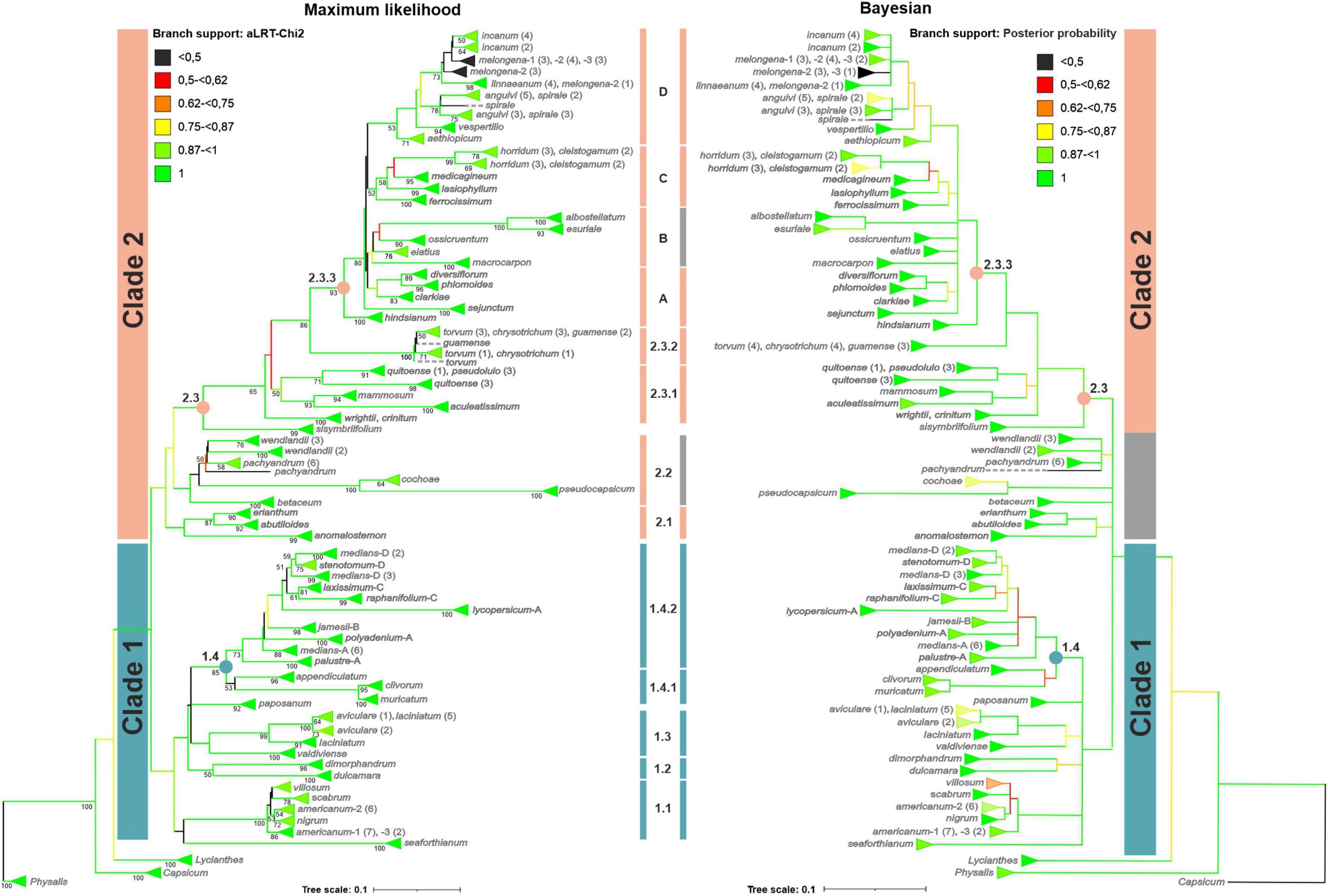
Figure 6. Phylogenetic ML and Bayesian dendrograms constructed by comparison of 5S IGS sequences of Solanum species. The aLRT support and posterior probabilities are represented by different branch colors. Numbers in the ML dendrogram represent bootstrap support values. Terminal clades are collapsed to species level. Numbers in brackets near the collapsed nodes indicate the number of ribotypes used. IGS structural variants A-D are given for members of sect. Petota (Clade 1.4.2). ML clades not confirmed by Bayesian analysis are highlighted in gray color.
Intergenic Spacer Organization in Sect. Petota
Analysis of IGS molecular organization in the species-rich sect. Petota was performed separately. By sequence comparison, numerous base substitutions and indels were detected, which appear to be randomly distributed along the IGS (Figure 7), except for the presumptive external promoter region just upstream of the CDS (see section “Discussion”).
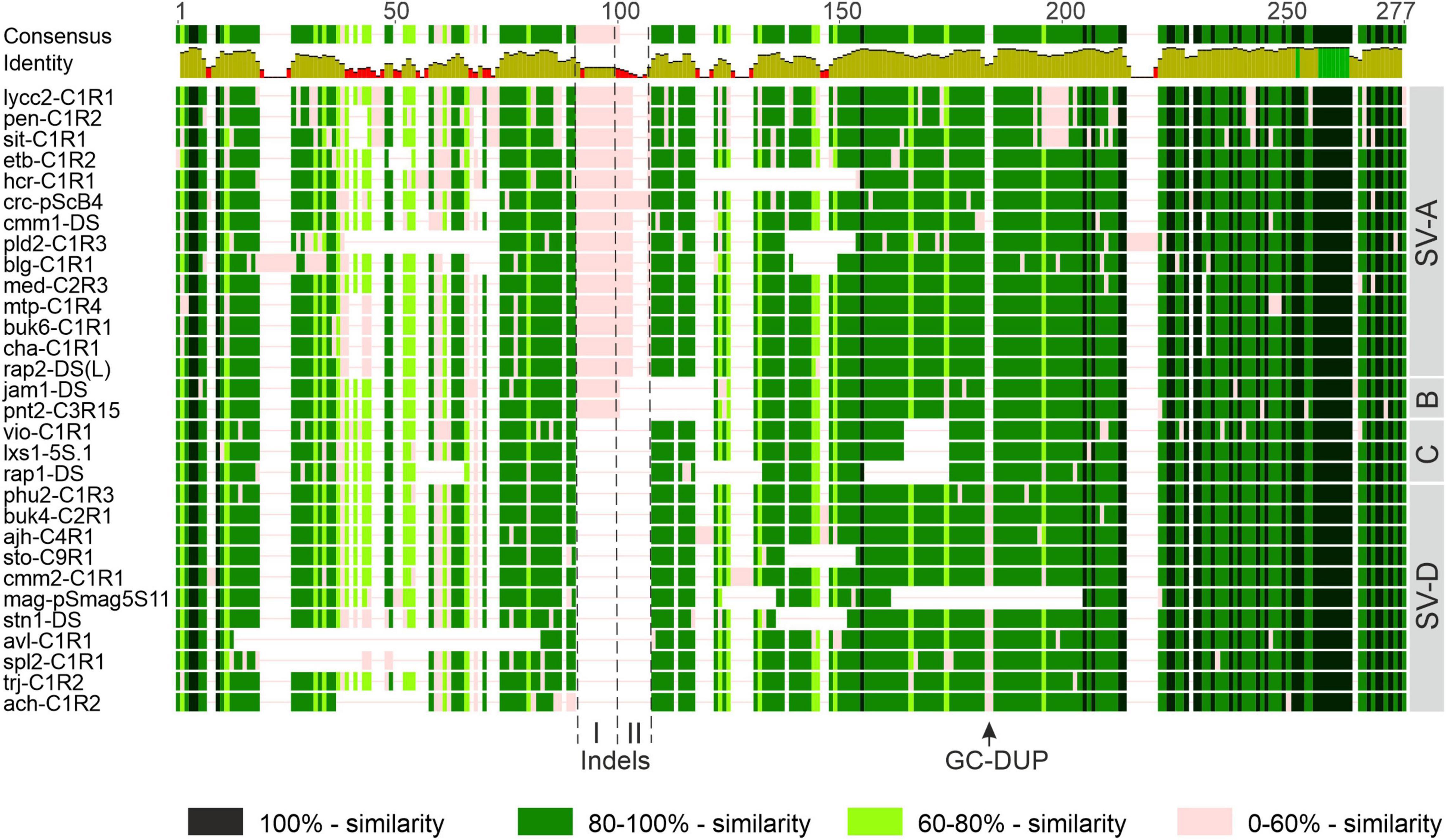
Figure 7. Schematic representation of sequence alignment of the 5S IGS of sect. Petota members. The level of sequence similarity is shown in different colors. Positions of group-specific indels I and II and GC-duplication (GC-DUP) are indicated. Abbreviations of species names are given in Table 2. Structural features of presented IGS sequences are indicated as structural variants (SVs) A-D.
The alignment of the sequences revealed that in the central part of the IGS there are two group-specific indels, I and II. Also, a lot of species contain a GC-duplication (GC-DUP) in the IGS (Figure 7). It is likely that these structural rearrangements occurred in different stages during the evolution of sect. Petota. With regard to the presence/absence of these molecular features, four structural variants (SVs) of the IGS can be distinguished. The evolutionary ancestral SV-A contains both specific indels, while independent deletions of indels II and I resulted in the formation of the derived SV-B and –C/D, respectively. SV-D additionally contains a GC-DUP.
Phylogenetic Analysis
The IGS sequences obtained by cloning as well as major and minor ribotypes extracted from SRA were used to reconstruct the phylogenetic relationships among Solanum species representing different taxonomic groups of the genus. For sect. Petota, seven species were selected whose IGS sequences belong to different structural classes (see section “Discussion”).
Multiple sequence alignment for the whole genus Solanum phylogeny was generated with the Mafft E-INS-I method and then manually corrected. The final 609-bp length alignment presented only one identical site, with an average pairwise identity of 54.7%. The best-fit phylogenetic model was estimated using Mega X to be general time-reversible (GTR) + gamma (G) (Kumar et al., 2018). The obtained maximum likelihood (ML) phylogenetic tree has 302 leaves, which correspond to the IGS sequences of 65 Solanum species (Figure 6). Calculating the statistical support applying the aLRT-Chi2 method and boot-strap analysis showed that majority of the tree’s nodes have a high or moderate support. The ML tree mostly matched the dendrogram generated by Bayesian inference.
On the dendrograms, all investigated species of the Solanum genus form a well-supported monophyletic group with L. lycioides as sister taxon. In the ML-tree, the Solanum species are divided into two major clades, 1 and 2, with high statistical support. In the clades, several well-supported minor clades were found. The monophyly of the Solanum genus and clade 1 is also confirmed by Bayesian inference. In contrast, clade 2 is represented by polytomy in the Bayesian dendrogram.
Discussion
Phylogeny of the Solanum Genus
Since the nineteenth century, the Solanum genus has been traditionally divided into two main groups, the so-called spiny and non-spiny solanums (Dunal, 1852; Seithe, 1962), which were further subdivided into sections, subsections, and series using morphological characters (D’Arcy, 1991; Nee, 1999). However, application of molecular methods shed a new light on the phylogeny of Solanum, demonstrating that these groups are mainly not monophyletic, and that the genus can be divided into 13 clades (Bohs, 2005; Weese and Bohs, 2007; Särkinen et al., 2013). Some of these clades have high statistical support, while the taxonomic placement and composition of the others are uncertain.
Analysis of several chloroplast genes and nuclear regions (e.g., ITS1/2 and waxy) is often performed in molecular phylogenetics. However, incongruence of results obtained by application of different markers is a well-known problem. Respectively, other genomic regions, particularly the 5S rDNA IGS, can additionally be used to clarify the phylogeny of lower-ranking taxa (Blöch et al., 2009; Tynkevich and Volkov, 2019; Cardoni et al., 2021; Ishchenko et al., 2021), including sect. Petota of the Solanum genus (Volkov et al., 2001). To evaluate the possibility of using this region to reconstruct phylogenetic relationships in the Solanum genus, we constructed an ML dendrogram that embraces 68 accessions from 63 species.
The ML dendrogram includes two major clades (Figure 6). Similar to our data, two clades in the Solanum genus were found by comparing sequences of plastid, nuclear ribosomal ITS and low-copy nuclear (waxy) genes (Särkinen et al., 2013, 2015). Particularly, four species, S. abutiloides, S. erianthum, S. cochoa, and S. pseudocapsicum, are included in Clade 2 of our dendrogram, which is in agreement with recent molecular data (Särkinen et al., 2013, 2015) but in contrast to the previous taxonomy of Nee (1999), who placed the species in the sections Brevantherum, Basarthrum, and Holophylla (see Table 1).
Clade 1 is composed of four smaller clades. Clade 1.1 contains four species of the Morelloid clade, S. americanum, S. nigrum, S. scabrum, and S. villosum (Särkinen et al., 2013, 2015). Two other species, S. anomalostemon and S. valdiviense previously associated with Morelloids are placed outside Clade 1.1, further supporting the phylogeny of the group proposed by Särkinen et al. (2015).
Clades 1.2–1.4 are combined in a polytomy. S. dimorphandrum of the Thelopodium clade (Bohs, 2005) and S. dulcamara of the Dulcamaroid clade (Bohs, 2005; Särkinen et al., 2013) belong to Clade 1.2, while another member of the Dulcamaroid clade, S. seaforthianum, occupies a basal position in Clade 1. S. valdiviense is included in Clade 1.3, which also comprises two species of sect. Archaesolanum, S. aviculare and S. laciniatum. The taxonomic position of S. valdiviense found in our analysis is fully consistent with previous data (Särkinen et al., 2015). S. aviculare and S. laciniatum are closely related (Figure 6): There are several ribotypes in the genome of S. aviculare that are very similar and even identical to those of S. laciniatum. These data indicate incomplete lineage sorting during speciation or subsequent hybridization among these species. The close relationship between S. aviculare and S. laciniatum confirms the taxonomy derived from sequencing of three chloroplast and two nuclear regions in which these two species represent sister taxa (Poczai et al., 2011; Särkinen et al., 2015).
Clade 1.4.1 comprises Central American S. appendiculatum and South American S. clivorum, which were previously assigned, respectively, to sect. Anarrhichomenum and Holophylla (Nee, 1999) as well as S. muricatum of sect. Basarthrum (Nee, 1999; Särkinen et al., 2013), while Clade 1.4.2 embraces numerous species of sect. Petota (including tomato) (Hawkes, 1990; Nee, 1999; Komarova et al., 2008). According to a recent analysis (Särkinen et al., 2015; Gagnon et al., 2021), S. appendiculatum and S. muricatum, similar to our results, belong to the potato clade, in contrast to S. clivorum, which was placed outside clade I.
Clade 1 also includes the South American species S. paposanum, which represents the Regmandra clade (Bohs, 2005; Särkinen et al., 2013). It was found that this clade was resolved in different positions in three data sets used for comparison (Särkinen et al., 2015; Gagnon et al., 2021).
Clade 2 consists of three smaller clades, 2.1–2.3. Clade 2.1 comprises two closely related species, S. abutiloides and S. erianthum, which were assigned by Nee (1999) to sect. Brevantherum of the Solanum subgenus. Later, sect. Brevantherum was transferred to clade II consisting of predominantly spiny and shrubby species (Särkinen et al., 2013, 2015; Gagnon et al., 2021). Similarly, the third member of Clade 2.1, S. anomalostemon, was assigned to the Morelloid clade (Bohs, 2005) but later transferred to clade II (Särkinen et al., 2015; Gagnon et al., 2021). Accordingly, the inclusion of S. abutiloides, S. erianthum, and S. anomalostemon in clade II is further supported by our results.
Clade 2.2 contains five species, which were previously assigned to different taxonomic groups. According to Nee (1999), two Central/South American species, S. wendlandii and S. pachyandrum, are members of sect. Herposolanum. Later, it was shown that S. wendlandii belongs to clade Wendlandii/Allophyllum, while the position of S. pachyandrum appeared unclear (Bohs, 2005; Särkinen et al., 2013, 2015). Thereafter, both species were assigned to sect. Aculeigerum (Clark et al., 2015). Our data also confirm the phylogenetic affinity of S. wendlandii and S. pachyandrum.
The next two species, South American S. cochoae and S. pseudocapsicum, have been previously assigned to different sections, Basarthrum and Holophylla (Anderson and Bernardello, 1991; Nee, 1999). In contrast, S. cochoae and S. pseudocapsicum are combined in a well-supported clade in our ML dendrogram.
Originally, S. cochoae was included in sect. Basarthrum on the basis of morphological analyses and crossing experiments, although all crosses with related wild species were unsuccessful. Surprisingly, the only species crossed with S. cochoae was cultivated S. muricatum, despite large differences in karyotypes of these two species (Anderson and Bernardello, 1991). However, the possibility of obtaining hybrids cannot be seen as a decisive argument for the close relationship between these two species, as it is sometimes possible to successfully cross distant Solanum species (Daunay et al., 2019). The close relationship between S. cochoae and S. muricatum is also supported by recent molecular data (Gagnon et al., 2021). In our dendrogram, however, S. cochoae does not appear to be related to S. muricatum but to S. pseudocapsicum, a member of the Geminata clade (Gagnon et al., 2021).
It should be noted that the common feature of the 5S rDNA repeats of S. cochoae and S. pseudocapsicum is short length due to deletion in the central part of the IGS. In addition, each species possesses specific deletions in other IGS regions (Figure 5). Altogether, these structural features can affect the position of the species in the dendrogram. Accordingly, we believe that the taxonomic position of S. cochoae close to S. pseudocapsicum should be interpreted with appropriate reservation in this stage, and that further studies should be carried out in order to finally clarify the question.
The last member of Clade 2.2 is S. betaceum, which has been previously treated as a member of separate genus Cyphomandra (D’Arcy, 1991) and then later placed to Solanum (Bohs, 1995) and assigned to sect. Pachyphylla of the Bassovia subgenus (Nee, 1999) or clade Cyphomandra in clade II (Särkinen et al., 2013, 2015; Gagnon et al., 2021). In our dendrogram, S. betaceum is a sister taxon for the other members of Clade 2.2.
Clade 2.3 includes three clades of lower ranks, 2.3.1–2.3.3. Clade 2.3.1 comprises two pairs of species, the South American S. aculeatissimum and S. mammosum of the section/clade Acanthophora as well as Andean cultivated species S. quitoense (naranjilla or lulo) and its wild relative S. pseudolulo of clade Lasiocarpa (Nee, 1999; Bohs, 2005; Levin et al., 2006; Särkinen et al., 2013). According to a molecular analysis, the clades Acanthophora and Lasiocarpa represent sister taxa (Särkinen et al., 2013; Gagnon et al., 2021). In the genome of S. quitoense, a ribotype similar to that of S. pseudolulo was detected, which could be due to hybridization between these species (Fory Sánchez et al., 2010).
Clade 2.3.2 comprises two Central/South American species, S. chrysotrichum and S. torvum of the section/clade Torva, as well as S. guamense, an endangered endemic species in Northern Mariana Islands (Stone, 1970) whose taxonomic status remains unclear (Nee, 1999; Bohs, 2005; Särkinen et al., 2013; Aubriot et al., 2016). Our analysis revealed that the three species share common ribotypes and are, therefore, unresolved in the dendrogram. The high genetic affinity of S. chrysotrichum and S. torvum agrees well with their morphological similarity. S. guamense also appeared to be closely related to these species.
Clade 2.3.3 includes S. hindsianum (clade Elaeagnifolium, Bohs, 2005; Särkinen et al., 2013), an endemic to the Sonoran Desert region of southern Arizona and northern Mexico (Knapp et al., 2017), and a well-supported monophyletic clade of 21 species, most of which have been assigned to sect. Melongena (Nee, 1999) or the Old World clade (Levin et al., 2006; Särkinen et al., 2013; Aubriot et al., 2016) of the Leptostemonum subgenus. However, the taxonomic position of five species (S. albostellatum, S. elatius, S. lasiophyllum, S. medicagineum, and S. spirale) has not yet been clarified, especially with molecular methods. In the Old World clade, there are four groups, A–D, of closely related species.
Clade 2.3.3A comprises members of “Dioicum Complex,” a set of several dioecious species (Whalen, 1984; Bean, 2004) from tropical Australia. Our data show that S. diversiflorum and S. phlomoides are closely related, and that S. clarkiae is a more distant species. S. sejunctum is placed outside clade 2.3.3A. This result agrees with the phylogeny based on the analysis of trnK–matK and ITS data sets (Martine et al., 2006, 2009).
Four other Australian species, S. albostellatum, S. esuriale, S. elatius, S. ossicruentum, as well as S. macrocarpon (African eggplant), belong to the next clade, 2.3.3B, although with a moderate statistical support. The West African species S. macrocarpon was previously assigned to Anguivi Grade (Aubriot et al., 2016, 2018; Gagnon et al., 2021), a group of Old World Leptostemonum species closely related to S. melongena (see our clade 2.3.3D below). Respectively, the phylogenetic affinity of S. macrocarpon to the Australian species seems somewhat unexpected and can be explained by the presumptive hybrid origin of S. macrocarpon (Daunay et al., 2019).
According to our data, S. albostellatum and S. esuriale from Western Australia show the closest relationship in Clade 2.3.3B, which is in good agreement with the high morphological similarity of these species (Davis and Hurter, 2012). S. elatius is also a member of the S. esuriale group (Bean, 2013).
S. ossicruentum represents a functionally dioecious bush tomato from northwestern Australia. Earlier, it was recognized as a variant of S. dioicum, a member of “Dioicum Complex.” However, later molecular analysis shows that S. ossicruentum is either a sister taxon to the rest of this group or represents an independent dioecious lineage (Martine et al., 2016). Our data further supported the second opinion and indicate a phylogenetic affinity between S. ossicruentum and members of the S. esuriale group.
Clade 2.3.3C comprises five Australian species. Two species, S. cleistogamum and S. horridum, contain very similar sets of ribotypes in their genomes and appear unresolved in the dendrogram. A sister taxon to them is S. medicagineum, while S. lasiophyllum and S. ferocissimum are more distantly related species. A close relationship among S. cleistogamum, S. horridum, and S. medicagineum has been shown earlier (Bean, 2004, 2012; Levin et al., 2006).
Hence, the Australian Solanum species studied here belong to three clades, 2.3.3A, B, and C. Similarly, monophyly of the Australian species was not supported by the analysis of seven nuclear genes (Martine et al., 2019).
Clade 2.3.3D comprises seven species naturally distributed in Africa and Asia. In particular, this clade includes two very morphologically and genetically similar domesticated plants, S. aethiopicum (bitter tomato, Ethiopian eggplant) and S. melongena as well as their presumptive wild ancestors, S. anguivi and S. incanum. The second species is very similar and can even be confused with S. linnaeanum (Daunay et al., 2001; Doganlar et al., 2002; Prohens et al., 2012). S. vespertilio, a species endemic to the Canary Islands, appears to be closely related to the other members of clade 2.3.3D.
Previously, the phylogeny of Old World “spiny solanums” was clarified using plastid and nuclear markers (Aubriot et al., 2016, 2018; Vorontsova and Knapp, 2016; Knapp et al., 2019; Gagnon et al., 2021). It was demonstrated that S. incanum, S. linnaeanum, and S. melongena are closely related and belong to the Eggplant clade, and that S. aethiopicum, S. anguivi, S. vespertilio (and S. macrocarpon, which is placed to clade 2.3.3B in our dendrogram) are included in Anguivi Grade outside the Eggplant clade. Hence, our novel data mainly confirm these results.
Surprisingly, S. anguivi and morphologically different S. spirale, a tetraploid (Randell and Symon, 1976) species from East Asia, are not resolved in the dendrogram (see Figure 6). A possible explanation for this result could be the allopolyploid origin of S. spirale. In this case, the 5S rDNA inherited from the parent related to S. anguivi could be retained in the genome, while the DNA of the other parent was lost. The uniparental inheritance of 5S rDNA in allopolyploids, both young and old, has been reported for several taxonomic groups including Solanaceae (Pontes et al., 2004; Volkov et al., 2017).
Clade 2.3 also comprises three species that do not belong to clades 2.3.1–2.3.3 presented above. Two species, S. crinitum and S. wrightii, represent the clade Androceras/Crinitum, while S. sisymbriifolium belongs to the clade Sisymbriifolium (Levin et al., 2006; Särkinen et al., 2013; Gagnon et al., 2021). The taxonomic position of these species in our dendrogram agrees well with previous results of molecular phylogenetics studies.
Majority of the clades identified in the ML tree was also recognized in the Bayesian dendrogram (Figure 6). However, the monophyly of Clade 2 was not confirmed by Bayesian inference: Clades 2.1, 2.2, and 2.3 are not combined with each other but belong to a basal polytomy in the Solanum genus.
Thus, in this study, we present the phylogeny of the Solanum genus derived from the analysis of 5S IGS sequences. Six species (S. albostellatum, S. elatius, S. guamense, S. lasiophyllum, S. medicagineum, and S. spirale) were characterized here for the first time using molecular taxonomy methods. The obtained dendrograms are mainly congruent with the published data for other regions of nuclear and plastid genomes: same major and minor clades were found for the species examined. However, taxonomic relationships between these clades and position of some species (e.g., S. cochoae, S. clivorum, S. macrocarpon, S. spirale) differ from previous results and require further clarification. Taken together, our results show that the 5S IGS represents a convenient molecular marker for phylogenetic studies on the Solanum genus. In particular, the simultaneous presence of several variants of rDNA in the genome enables the detection of cases of reticular evolution such as incomplete lineage sorting and interspecific hybridization.
Molecular Evolution and IGS Diversity in Sect. Petota
One of the species-rich groups in genus Solanum is sect. Petota, which has about 250 members (Hawkes, 1990; Nee, 1999). In our ML dendrogram (Figure 6), sect. Petota belongs to Clade 1.4.2.
To analyze the molecular evolution of 5S rDNA in this section and in more details, we assembled IGS ribotypes for 125 accessions representing 83 species (Table 4) and compared the results with our previous data, obtained by cloning and sequencing of 5S rDNA of 32 wild species and breeding lines of sect. Petota (Volkov et al., 2001).
Analysis of the IGS sequences revealed that they differ in base substitutions and indels (Figure 7). Same indels mostly occur in a single or some closely related species and, therefore, represent convenient molecular markers for their identification. For example, non-tuber-bearing species S. etuberosum and S. palustre (series Etuberosa; Hawkes, 1990) possess a common specific deletion at the beginning of the IGS, or S. laxissimum and S. violaceimarmoratum (series Conicibaccata) have a deletion in the central part of it (Figure 7). Several species-specific indels in the IGS of the Solanum species have already been described (Volkov et al., 2001), and our actual analysis additionally identifies new ones for the novel species. This finding further confirms our earlier assumption that indels are a characteristic feature of IGS evolution in sect. Petota. We have also argued that because of the high frequency of indels compared to base substitutions, IGS cannot be used for phylogenetic reconstruction of this section applying standard algorithms. However, the indels represent unique evolutionary events that should be considered in taxonomic studies.
Considering the location of group-specific indels I and II as well as GC-duplication (Figure 7), four major structural variants of the IGS were identified. Accordingly, members of sect. Petota can be divided into four groups, A–D.
Group A comprises species that belong to the subsection Estolonifera including the tomato group, and to the series Pinnatisecta, Polyadenia, Commersoniana, Circaeifolia, Megistacroloba, Conicibaccata, and Piurana of the subsection Potatoe (Hawkes, 1990; Nee, 1999), or to clades 1+2 and 3 (Spooner et al., 2014; Huang et al., 2019). Also, SV-A was found in four species (S. acroscopicum, S. andreanum, S. abancayense, S. multiinterruptum) that were assigned to ser. Tuberosa (Hawkes, 1990; Nee, 1999) or clade 4 (Spooner et al., 2014; Huang et al., 2019). Group B includes only two species, S. jamesii and S. pinnatisectum of ser. Pinnatisecta (Hawkes, 1990; Nee, 1999) or clade 1+2 (Huang et al., 2019). This means that the SR-B arose relatively recently during speciation in clade 1+2, just before the divergence of S. jamesii and S. pinnatisectum but after their separation from the sister taxon, which was similar to S. stenophyllidium.
Group C includes accessions of three species, S. raphanifolium, S. laxissimum, and S. violaceimarmoratum, which belong to the series Megistacroloba and Conicibaccata (Hawkes, 1990) or clade 4 (Spooner et al., 2014; Huang et al., 2019). Group D embraces numerous species that belong to the series Yungasensa, Megistacroloba, Cuneoalata, Maglia, and Tuberosa (Hawkes, 1990) or clade 4 (Spooner et al., 2014; Huang et al., 2019). SV-C and D were not found outside of clade 4, which, however, also includes four species possessing SV-A. Therefore, SV-C and D arose from SV-A after separation of clades 3 and 4.
Interestingly, in some cases, rDNA repeats representing different structural variants were found in the same plant accession (see below).
We have also found that the central part of the IGS is completely deleted in S. bulbocastanum of the series Bulbocastana (Hawkes, 1990) or clade 1+2 (Huang et al., 2019). Accordingly, the structural organization of ITS characteristic of this species cannot be assessed and used for phylogenetic reconstruction.
Analysis of our data showed that the most common IGS variants are SV-A and-D, and SV-B and-C were found only in four and three species, respectively. In order to assess the molecular diversity of SV-A and-D, we constructed median-joining networks for these two IGS variants using 204 and 353 sequences (Figure 8). In the median-joining networks, the sequences of SV-A and-D are distributed between the six and ten main clusters according to their similarity. In the vast majority of cases, each node corresponds to only one sequence, with the exception of one node in median-joining network A and seven nodes in median-joining network D. These nodes include two to nine ribotypes that mainly represent genomes of different species. Therefore, identical IGS sequence variants can be present in genomes of different species or plant accessions, suggesting their common origin.
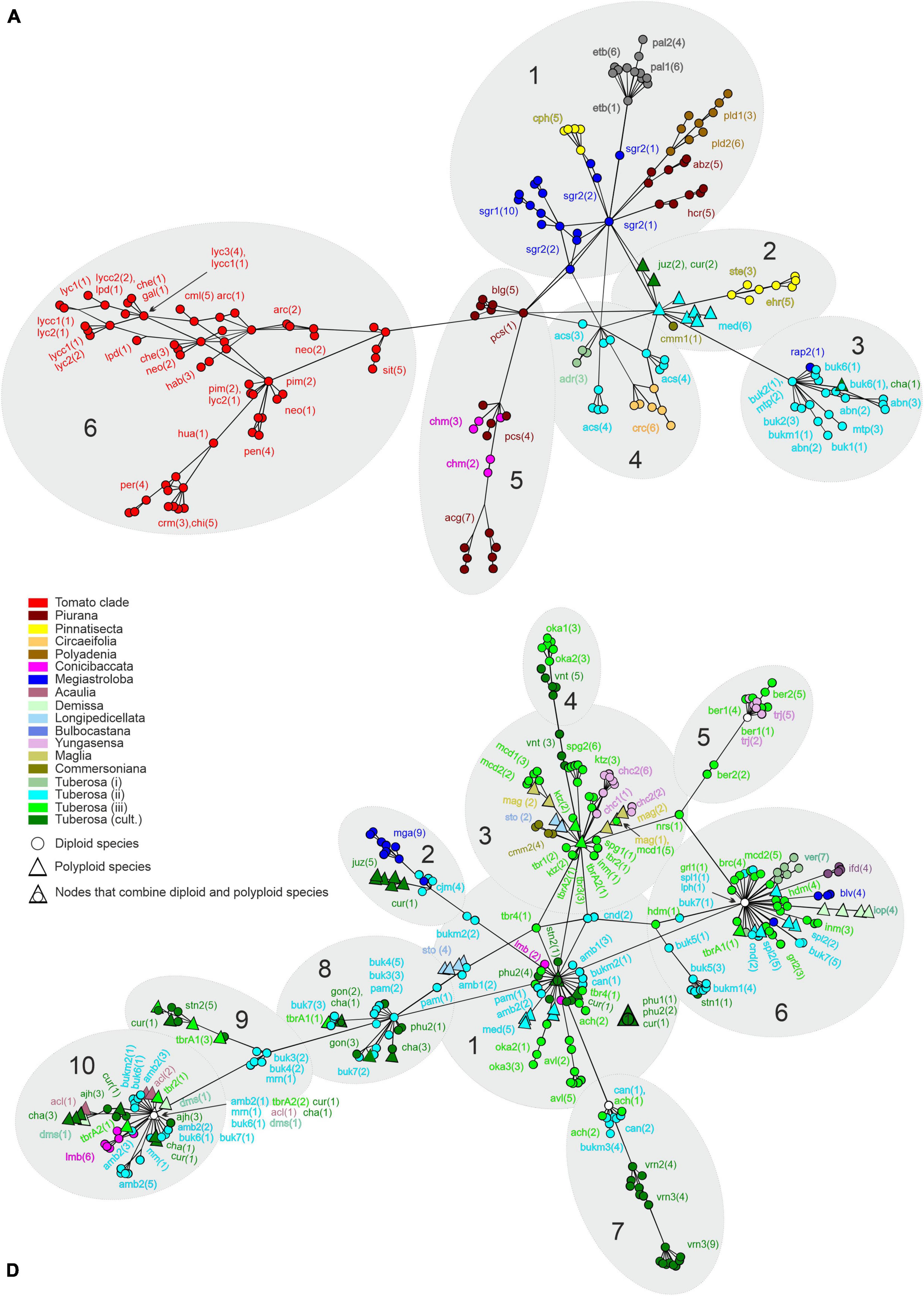
Figure 8. Median-joining networks depicting relationships of 5S IGS structural variants A and D of the species of sect. Petota. Names of main clusters are given. The affiliation of the species to the series proposed by Hawkes (1990) is indicated by different colors. Numbers in brackets indicate the number of ribotypes used. Abbreviations of species names are given in Table 2.
SV-D sequences are distributed among ten clusters, D1-D10 (Figure 8). The largest clusters, D1, D3, D6, and D10, contain 50, 56, 68, and 51 sequences, respectively. The sequences included in cluster D1 are nearly identical to SV-D consensus sequence and, therefore, represent evolutionary ancestral ribotypes, while the other clusters comprise derived sequences containing specific base substitution and indels. Starting from cluster D1, five evolutionary lineages can be distinguished.
Taken together, our data agree well with modern taxonomy, which is based on the application of molecular methods (Spooner et al., 2014; Huang et al., 2019) but are less consistent with the traditional classification of Hawkes (1990). In particular, the sections proposed by Hawkes (1990) are not confirmed, because species from different sections are mixed up and belong to different clusters in the median-joining network. In contrast, our results agree well with the molecular data, since clades 4 North and 4 South (Spooner et al., 2014; Huang et al., 2019) can also be recognized in our median-joining network: members of the North and South clades belong to clusters D1, D7-D10, and D2-D6.
Conserved Sequence Motifs in the Intergenic Spacer of Solanum Species
Comparative sequence analysis revealed that the most conservative regions of the IGS in Solanum species are the 7- and 40-bp-long fragments at the 5′ and 3′ ends (Figure 5). The evolutionary conservation of these regions has already been observed in other plants (Hemleben and Werts, 1988; Crisp et al., 1999; Tynkevich and Volkov, 2019; Ishchenko et al., 2020, 2021), and a possible reason for this seems to be their involvement in the transcription of 5S rDNA by RNA polymerase III (Pol III).
External elements of the Pol III promoter have been previously characterized in Arabidopsis thaliana (Douet and Tourmente, 2007; Vaillant et al., 2007; Layat et al., 2012; Simon et al., 2018). These signals include the TATATA motif (so-called TATA-box), GC-dinucleotide, and C nucleotide in positions-28,-13, and-1 bp, respectively. Similar sequences were also found in other plants (Tynkevich and Volkov, 2014; Tynkevich et al., 2015; Ishchenko et al., 2018, 2021). In representatives of the Solanum genus, as well as in Quercus (Tynkevich and Volkov, 2019) and Rosa (Tynkevich and Volkov, 2014), the TATA-box has a length of 7 bp and begins in position-30. Its sequence (TTTAATA) in Solanum is slightly different from that in other groups of plants.
Another external element of the Pol III promoter, the GC-dinucleotide (Douet and Tourmente, 2007) is duplicated in several Solanum species and is located, respectively, both in the typical position-12 and, additionally, in position-14. Similar to Solanum, duplication of this presumptive external promoter element was also found in the Quercus species (Tynkevich and Volkov, 2019).
The third conservative promoter element, cytosine, in position-1 (Douet and Tourmente, 2007; Simon et al., 2018), has been replaced by thymine in more than half of the Solanum species. In addition, we found that the dinucleotide GA in position-3 in the IGS is highly conserved, indicating its possible involvement in transcription initiation.
At the beginning of the IGS in Solanum, like in other genera, the oligo-T motif TTTTT was found, which probably represents a transcription termination site (Hemleben and Werts, 1988; Simon et al., 2018; de Souza et al., 2020).
The most variable central region of the IGS can be subdivided into (i) AT-rich and (ii) subrepeated regions. Previously, AT-rich regions were found in the IGS of Fabaceae (Hemleben and Werts, 1988) and Poaceae (Röser et al., 2001). AT-rich regions demonstrate a similarity to amplification-promoting sequences (Borisjuk et al., 2000), which may be involved in amplification of 5S rDNA repeats. Also, regions composed of subrepeats were described for the IGS of several plant taxa, e.g., Rosaceae (Tynkevich and Volkov, 2014) and Poaceae (Ishchenko et al., 2018, 2021). Previously, we have demonstrated that structural rearrangements of the variable central region of the IGS in Solanum species of sect. Petota as well as in distantly related S. melongena and S. betaceum are preferentially associated with four classes (A–D) of short direct subrepeats: the IGS evolved mainly by duplications of some sequence motifs, resulting in formation of several variants of subrepeats, which were independently amplified in different sections of the genus after radiation from a common ancestor (Volkov et al., 2001; Davidjuk et al., 2010, 2013).
Intragenomic Heterogeneity and Molecular Evolution of 5S rDNA
It is widely believed that 5S rDNA repeats present in the same genome (at least in diploid species) should be nearly identical because of concerted evolution (Coen et al., 1982; Tynkevich and Volkov, 2014; Barman et al., 2016). In our study, we performed a detailed analysis of 5S rDNA intragenomic sequence diversity and found several ribotypes in all the species studied. Comparative analysis of all available sequences showed that the IGS sequence similarity in Solanum species ranges from 51.4 to 100%. The highest levels were found in S. wrightii and four representatives of the tomato group, namely lycopersicum var. cerasiforme-2, S. galapagense, and S. huaylasense; each of which had only one ribotype detected. The high intragenomic homogeneity of IGS (over 95%) is also characteristic of other representatives of the tomato group with the exception of S. lycopersicum-2 and S. corneliomuelleri. The relatively low IGS similarity in S. corneliomuelleri (89.5%) is due to the presence of 10-bp deletion in one ribotype, while no further indels are found in any of the other members of the tomato group. Hence, deletions are very rare during the evolution of IGS in the tomato group, which is in obvious contrast to other Solanum groups, especially to closely related tuber-bearing species of Petota.
Our calculations indicated that the lowest level of intragenomic IGS sequence similarity is demonstrated by S. melongena-1 and -2 (56 and 51.4%), S. sogarandinum (54.9%), S. wendlandii (58.4%), and S. lasiophyllum (59.4%). In S. melongena-1 and -2, it is due to simultaneous existence of short and long (containing extra-long duplication, see Figure 2 and Supplementary Material) repeats in the genome, while in S. melongena-3, which possesses only long repeats, the similarity amounts to 95.2–99.4%. Similarly, in S. lasiophyllum, three adjacent deletions (65 bp in total) present in one of six ribotypes is the main reason for the low level of intragenomic similarity. In contrast, two mechanisms contribute to the low similarity of IGS in S. sogarandinum: (i) a long deletion in one ribotype and (ii) multiple base substitutions in another. In the second case, 33 of 210 bp in the same ribotype was changed compared to the consensus sequence. Notably, these mutations are present in the 3′ IGS region, which likely contains external promoter elements, suggesting putative pseudogenization of the ribotype. Putative 5S rDNA-related pseudogenes have already been described for members of Solanum and other genera of Solanaceae (Volkov et al., 2001, 2017).
In S. wendlandii, similar to S. sogarandinum, two mechanisms, an insertion and a large number of base substitutions, cause increased heterogeneity of IGS sequences (Figure 3). Accordingly, we excluded long (more than 5 bp) deletions and multiple base substitutions from our calculations and found that in this case the minimum level of intragenomic similarity of the IGS in Solanum species is around 85–90%.
In general, our results indicate that there are two mechanisms, long indels and multiple base substitutions, that significantly affect the heterogeneity of the IGS in Solanum species. Multiple base substitutions are rare events: out of about 900 analyzed sequences, only five ribotypes bearing multiple base substitutions were identified in four plant accessions (S. kurtzianum, S. pinnatisectum-2, S. sogarandinum-1, and S. vernei-3), while long indels are much more common.
The question, “what can be the source of the IGS intragenomic polymorphism?” arises. There are at least two possible options: (i) new variants emerge in the genome itself by accumulation of mutations and (ii) new variants appear in the genome as a result of introgression of genetic material due to interspecific hybridization. It is well known that in sect. Petota, especially in the S. brevicaule complex, interspecific hybridization is widespread at both the diploid and polyploid levels (Hawkes, 1990; Spooner et al., 2014). Among the 125 examined accessions representing sect. Petota, two or more structural variants of the IGS were found in 25 cases, and interspecific hybridization seems to be a plausible explanation for this polymorphism, especially when structurally different IGS variants (e.g., A and D) occur in the same genome. However, further research is needed to confirm this option.
Our data suggest that long indels and multiple base substitutions appeared repeatedly during the molecular evolution of IGS in the Solanum genus. However, it seems that they were mostly not conserved and eliminated. Accordingly, the length of IGS and contents of GC pairs did not change significantly during the course of speciation (see above). The likely reason for this negative selection could be the association between indels/multiple base substitutions and pseudogenization of 5S rDNA. Accordingly, it looks that the main road of the IGS molecular evolution seems to be step-wise accumulation of single base substitution or short indels.
Intraspecific 5S rDNA Heterogeneity
It could be anticipated that different accessions of same species possess identical/similar sets of ribotypes. To check this assumption, we examined two to three accessions for 25 diploid species (Tables 1, 2 and Supplementary Material), and in most cases, only one IGS variant was actually found. However, in seven species (S. ambosinum, S. commersonii, S. microdontum, S. okadae, S. phureja, S. raphanifolium, and S. stenotomum; Figure 8 and Supplementary Material), one or two structural variants were detected in different accessions, indicating presumptive interspecific hybridization.
Among the species studied, we examined the highest number of accessions in S. bukasovii, which was considered one of the ancestors of cultivated potato (Ugent, 1970; Hawkes, 1990; Hosaka, 1995; Spooner et al., 2005; Hardigan et al., 2015). This close relationship was also confirmed in our previous study by analyzing the 5′-external transcribed spacer (ETS) region of nuclear 35S rDNA (Volkov et al., 2003). According to the comparison of whole plastid genomes, the species belongs to clade 4 North (Huang et al., 2019). Respectively, based on its taxonomic position, it might be expected that S. bukasovii should possess the IGS variant D. However, SV-A3 was previously found in the buk1 accession (Volkov et al., 2001), which indicates incongruence among different phylogenetic markers. To further clarify the issue, we analyzed ten accessions of S. bukasovii in this study and found an extreme variability of the 5S IGS set. Two accessions (buk1 and buk2) contain only SV-A3 in the genome, while six others (buk3, buk4, buk5, buk7, bukm2, and bukm3) possess different D variants, SV-D1,-D4,-D7,-D8,-D9, and-D10 (see Table 4). Two remaining accessions (buk6 and bukm1) have both SV-A3 and –D4 or-D10. The D variants detected in accessions of S. bukasovii belong to different clusters in the median-joining network (Figure 8) and are identical or very similar to the D variants of several species (e.g., S. achacachense, S. ambosinum, S. canasense, S. marinasense, S. phureja, S. stenotomum, etc.) belonging to clade 4 North + cultivated (Huang et al., 2019). Similarly, the SV-A3 found in S. bukasovii is identical/similar to that of S. abancayense, S. multiinterruptum, S. raphanifolium, and S. chaucha. This unusual diversity of IGS might indicate a complex hybridogenic origin: i.e., some of the examined accessions could represent natural hybrids between S. bukosovii and different Petota species. The genetic heterogeneity of S. bukosovii accessions and the putative hybrid origin of some of them by crossing with S. sparsipilum or S. raphanifolium have recently been demonstrated using plastid and mitochondrial markers (Achakkagari et al., 2020, 2021; Bozan, 2021).
Origin of Polyploid Species
There are several polyploids among the studied species. Two of them, S. chaucha and S. juzepczukii, are triploids. It was originally postulated that S. chaucha arose as a result of hybridization between diploid S. phureja and tetraploid S. tuberosum ssp. andigena (Bukasov, 1939), but later, S. chaucha was recognized as the autotriploid of S. phureja (Bukasov, 1978). In contrast, Hawkes (1962, 1990) suggested that S. chaucha originated from hybridization between S. tuberosum ssp. andigena and diploid species S. stenotomum. Our analysis indicates an allotriploid origin of S. chaucha, as three structural variants of IGS, SV-A3,-D8, and-D10, are found in its genome (Table 4 and Figure 8). The molecular data confirm the close relationship among S. chaucha, S. phureja (accession phu2 but not phu1), S. tuberosum ssp. andigena, and a diploid species, S. stenotomum subsp. goniocalyx; all of which have SV-D8. However, the origin of S. chaucha appeared to be more complicated, because other structural variants of the IGS had to be inherited from species belonging to clusters A3 and D10.
It is widely believed that the triploid species S. juzepczukii originated from a natural cross between a cultivated diploid, S. stenotomum, and the wild tetraploid species S. acaule (maternal form), and pentaploid species S. curtilobum arose from a combination of non-reduced gamete of S. jusepczukii (3×, maternal form) with a reduced (2×) gamete of tetraploid S. tuberosum ssp. andigena (Bukasov, 1939; Hawkes, 1962, 1990; Schmiediche et al., 1980). The application of nuclear molecular markers confirmed this scenario (Rodríguez et al., 2010), but an alternative origin of S. curtilobum by hybridization of triploid species of the Andigenum group (non-reduced gamete, maternal form) and S. acaule was later proposed (Gavrilenko et al., 2013; Spooner et al., 2014). We found that the above-mentioned species contain the following IGS variants: S. acaule: SV-D10, S. jusepczukii: SV-A2 and-D2; S. tuberosum ssp. andigena: SV-D6,-D8, and-D9; S. curtilobum: SV-A2,-D1,-D2,-D9, and-D10. Three sets of IGS variants were identified for three accessions of S. stenotomum (stn1: SV-D1,-D9; stn2: SV-D4; gon: SV-D8). Remarkably, five IGS structural variants have been identified for pentaploid S. curtilobum that demonstrate the complex hybrid nature of this species. The analysis of the results showed that several common IGS structural variants are present in genomes of the examined species, which are correspondingly co-localized in the median-joining network (see Figure 8). However, no expected additivity of IGS structural variants from the presumptive parents in the derived alloploid progeny was found. It looks probable that the origin of S. jusepczukii and S. curtilobum may involve more parental diploids and requires further clarification using more plant accessions and additional molecular markers.
Two structural variants of IGS, SV-A2, and -D1, are present in the genome of S. medians, indicating its origin from a cross between potato species included in clusters A2 and D1. For S. medians, diploid and triploid populations were reported (Hijmans et al., 2007). Unfortunately, we could not find in SRA information about the ploidy level of the plant accession analyzed here.
According to a GISH analysis of meiotic preparations, S. stoloniferum appears to be an allotetrapolyploid species with a genomic constitution of AABB. It has been suggested that the species originated from S. verrucosum as the A genome donor and another North or Central American diploid species (e.g., S. cardiophyllum, S. ehrenbergii, or S. jamesii) as the B genome donor (Hijmans et al., 2007). In that case, regarding our data (Table 4 and Figure 8), it is expected that S. stoloniferum inherited SV-A1, -A2, or -B from S. cardiophyllum, S. ehrenbergii, or S. jamesii, respectively, as well as SV-D4 from S. verrucosum. However, S. stoloniferum possesses only D-variants of IGS, namely, SV-D1 and SV-D3, that could be inherited from S. ambosinum and from one of the species that belong to cluster D3.
Previously, we have discussed the presumptive origin of a tetraploid, S. acaule, and S. tuberosum as well as hexaploids S. demissum and S. iopetalum (Volkov et al., 2001). The new data confirm the close relationship between S. demissum and S. acaule (cluster D10) and more distant position of S. iopetalum (cluster D6), and provide new information on putative diploid ancestors of the polyploid species. Our novel data also show that the IGS variants of two accessions of S. tuberosum ssp. andigena belong to different clusters (tbrA1: clusters D4, D8, and D9, and tbrA2: clusters D3 and D10), indicating that these accessions have an independent hybrid origin from different parental diploids.
Data Availability Statement
The datasets presented in this study can be found in online repositories. The names of the repository/repositories and accession number(s) can be found in the article/Supplementary Material.
Author Contributions
RV, YT, and IP conceived and designed the study. YT, AS, and LK performed the experiments and collected the material. YT, RV, AS, IP, and VH analyzed the data. RV and VH wrote the manuscript. All authors contributed to the article and approved the submitted version.
Funding
This study was supported by the Ministry of Education and Science of Ukraine (Grant No. 0121U111109), Alexander v. Humboldt Foundation (Bonn, Germany) Fellowship for RV, and DAAD (German Academic Exchange Service) Research Fellowships for IP and YT.
Conflict of Interest
The authors declare that the research was conducted in the absence of any commercial or financial relationships that could be construed as a potential conflict of interest.
Publisher’s Note
All claims expressed in this article are solely those of the authors and do not necessarily represent those of their affiliated organizations, or those of the publisher, the editors and the reviewers. Any product that may be evaluated in this article, or claim that may be made by its manufacturer, is not guaranteed or endorsed by the publisher.
Acknowledgments
We are thankful to Frank van Caekenberghe (Meise Botanical Garden, Belgium), Tatiana Derevenko (Botanical Garden of the Chernivtsi National University, Ukraine), David Orr (Waimea Arboretum and Botanical Garden, Hawaii, United States), and Edith Stabentheiner (University of Graz, Austria) for providing the plant material. We are particularly grateful to the staff at Frontiers for exempting our article from paying the publication fee. We decided to donate this money to support the Ukrainian army and the needs of refugees from Russian aggression.
Supplementary Material
The Supplementary Material for this article can be found online at: https://www.frontiersin.org/articles/10.3389/fpls.2022.852406/full#supplementary-material
Footnotes
References
Achakkagari, S. R., Bozan, I., Anglin, N. L., Ellis, D., Tai, H. H., and Strömvik, M. V. (2021). Complete mitogenome assemblies from a panel of 13 diverse potato taxa. Mitochondrial DNA B Resour. 6, 894–897. doi: 10.1080/23802359.2021.1886016
Achakkagari, S. R., Kyriakidou, M., Tai, H. H., Anglin, N. L., Ellis, D., and Strömvik, M. V. (2020). Complete plastome assemblies from a panel of 13 diverse potato taxa. PLoS One 15, 894–897. doi: 10.1080/23802359.2021.1886016
Alexandrov, O. S., Razumova, O. V., and Karlov, G. I. (2021). A comparative study of 5S rDNA non-transcribed spacers in Elaeagnaceae species. Plants 10:4. doi: 10.3390/10010004
Anderson, G. J., and Bernardello, L. M. (1991). The relationships of Solanum cochoae (Solanaceae), a new species from Peru. Novon 1, 127–133. doi: 10.2307/3391369
Anisimova, M., and Gascuel, O. (2006). Approximate likelihood-ratio test for branches: a fast, accurate, and powerful alternative. Syst. Biol. 55, 539–552. doi: 10.1080/10635150600755453
Arnheim, N., Krystal, M., Schmickel, R., Wilson, G., Ryder, O., and Zimmer, E. (1980). Molecular evidence for genetic exchanges among ribosomal genes on non-homologous chromosomes in man and apes. Proc. Natl. Acad. Sci. U.S.A. 77, 7323–7327. doi: 10.1073/pnas.77.12.7323
Aubriot, X., Knapp, S., Syfert, M. M., Poczai, P., and Buerki, S. (2018). Shedding new light on the origin and spread of the brinjal eggplant (Solanum melongena L.) and its wild relatives. Am. J. Bot. 105, 1175–1187. doi: 10.1002/ajb2.1133
Aubriot, X., Singh, P., and Knapp, S. (2016). Tropical Asian species show the Old World clade of “spiny Solanums” (the Leptostemonum Clade: Solanaceae) is not monophyletic. Bot. J. Linn. Soc. 180, 1–27. doi: 10.1111/boj.12412
Barman, A. S., Singh, M., Singh, R. K., and Lal, K. K. (2016). Evidence of birth-and-death evolution of 5S rRNA gene in Channa species (Teleostei, Perciformes). Genetica 144, 723–732. doi: 10.1007/s10709-016-9938-6
Baum, B. R., Edwards, T., Mamuti, M., and Johnson, D. A. (2012). Phylogenetic relationships among the polyploid and diploid Aegilops species inferred from the nuclear 5S rDNA sequences (Poaceae: Triticeae). Genome 55, 177–193. doi: 10.1139/g2012-006
Bean, A. R. (2004). The taxonomy and ecology of Solanum subg. Leptostemonum (Dunal) Bitter (Solanaceae) in Queensland and far north-eastern New South Wales. Austrobaileya 6, 639–816.
Bean, A. R. (2012). A taxonomic revision of the Solanum echinatum group (Solanaceae). Phytotaxa 57, 33–50. doi: 10.11646/phytotaxa.57.1.6
Bean, A. R. (2013). A taxonomic review of the Solanum sturtianum subgroup of subgenus Leptostemonum (Solanaceae). Nuytsia 23, 129–161.
Blöch, C., Weiss-Schneeweiss, H., Schneeweiss, G. M., Barfuss, M. H., Rebernig, C. A., Villaseñor, J. L., et al. (2009). Molecular phylogenetic analyses of nuclear and plastid DNA sequences support dysploid and polyploid chromosome number changes and reticulate evolution in the diversification of Melampodium (Millerieae, Asteraceae). Mol. Phylogenet. Evol. 53, 220–233. doi: 10.1016/j.ympev.2009.02.021
Bohs, L. (1995). Transfer of Cyphomandra (Solanaceae) and its species to Solanum. Taxon 44, 583–587. doi: 10.2307/1223500
Bohs, L. (2005). Major clades in Solanum based on ndhF sequences. A festschrift for William G. D’Arcy: the legacy of a taxonomist. Monogr. Syst. Bot. Missouri Bot. Gard. 104, 24–49.
Borisjuk, N., Borisjuk, L., Komarnytsky, S., Timeva, S., Hemleben, V., Gleba, Y., et al. (2000). The tobacco ribosomal DNA spacer element stimulates amplification and expression of heterologous genes. Nat. Biotechnol. 18, 1303–1306. doi: 10.1038/82430
Bozan, I. (2021). Genome Analysis of the Diploid wild Potato Solanum bukasovii. Master thesis. Montreal: McGill University, 13–61.
Bustos, A., Figueroa, R. I., Sixto, M., Bravo, I., and Cuadrado, A. (2020). The 5S rRNA genes in Alexandrium: their use as a FISH chromosomal marker in studies of the diversity, cell cycle and sexuality of dinoflagellates. Harmful Algae 98:101903. doi: 10.1016/j.hal.2020.101903
Cardoni, S., Piredda, R., Denk, T., Grimm, G. W., Papageorgiou, A. C., Schulze, E. D., et al. (2021). High-throughput sequencing of 5S-IGS rDNA in Fagus L. (Fagaceae) reveals complex evolutionary patterns and hybrid origin of modern species. bioRxiv [Preprint] doi: 10.1101/2021.02.26.433057
Chen, G., Stepanenko, A., and Borisjuk, N. (2021). Mosaic arrangement of the 5S rDNA in the aquatic plant Landoltia punctate (Lemnaceae). Front. Plant Sci. 12:678689. doi: 10.3389/fpls.2021.678689
Chiarini, F., Sazatornil, F., and Bernardello, G. (2018). Data reassessment in a phylogenetic context gives insight into chromosome evolution in the giant genus Solanum (Solanaceae). Syst. Biodivers. 16, 397–416. doi: 10.1080/14772000.2018.1431320
Clark, J. L., Nee, M., Bohs, L., and Knapp, S. (2015). A revision of Solanum section Aculeigerum (the Solanum wendlandii group, Solanaceae). Syst. Bot. 40, 1102–1136. doi: 10.1600/036364415X690148
Coen, E. S., Thoday, J. M., and Dover, G. (1982). Rate of turnover of structural variants in the rDNA gene family of Drosophila melanogaster. Nature 295, 564–568. doi: 10.1038/295564a0
Crisp, M. D., Appels, R., Smith, F. M., and Keys, W. M. S. (1999). Phylogenetic evaluation of 5S ribosomal RNA gene and spacer in the Callistachys group (Fabaceae: Mirbelieae). Plant Syst. Evol. 218, 33–42. doi: 10.1007/BF01087032
Cronn, R. C., Zhao, X., Paterson, A. H., and Wendell, J. F. (1996). Polymorphism and concerted evolution in a tandemly repeated gene family: 5S ribosomal DNA in diploid and allopolyploid cottons. J. Mol. Evol. 42, 685–705. doi: 10.1007/BF02338802
D’Arcy, W. G. (1991). “The solanaceae since 1976, with a review of its biogeography,” in Solanaceae III: Taxonomy, Chemistry, Evolution, eds J. G. Hawkes, R. N. Lester, M. Nee, and N. Estrada (Kew: Royal Botanic Gardens), 75–137.
Daunay, M. C., Lester, R. N., and Ano, G. (2001). “Cultivated eggplants,” in Tropical Plant Breeding, eds A. Charrier, M. Jacquot, S. Hamon, and D. Nicolas (Oxford: Oxford University Press), 200–225.
Daunay, M. C., Salinier, J., and Aubriot, X. (2019). “Crossability and diversity of eggplants and their wild relatives,” in The Eggplant Genome, Compendium of Plant Genomes, ed. M. A. Chapman (Cham: Springer), doi: 10.1007/978-3-319-99208-2_11
Davidjuk, Y. M., Hemleben, V., and Volkov, R. A. (2010). Structural organization of 5S rDNA of eggplant, Solanum melongena L. Biol. Syst. 2, 3–6.
Davidjuk, Y. M., Moloda, O. O., and Volkov, R. A. (2013). Molecular organization of 5S rDNA of Solanum betaceum Cav. Bul. Vavilov Soc. Genet. Breed. Ukr. 11, 14–19.
Davis, R. W., and Hurter, P. J. H. (2012). Solanum albostellatum (Solanaceae), a new species from the Pilbara bioregion of Western Australia Nuytsia. Nuytsia 22, 329–334.
de Souza, T. B., Gaeta, M. L., Martins, C., and Vanzela, A. L. L. (2020). IGS sequences in Cestrum present AT-and GC-rich conserved domains, with strong regulatory potential for 5S rDNA. Mol. Biol. Rep. 47, 55–66. doi: 10.1007/s11033-019-05104-y
Doganlar, S., Frary, A., Daunay, M.-C., Lester, R. N., and Tanksley, S. D. (2002). A comparative genetic linkage map of eggplant (Solanum melongena) and its implications for genome evolution in the Solanaceae. Genetics 161, 1697–1711. doi: 10.1093/genetics/161.4.1697
Douet, J., and Tourmente, S. (2007). Transcription of the 5S rRNA heterochromatic genes is epigenetically controlled in Arabidopsis thaliana and Xenopus laevis. Heredity 99, 5–13. doi: 10.1038/sj.hdy.6800964
Dunal, M. F. (1852). “Solanaceae,” in Prodromus Systematis Naturalis Regni Vegetabilis, Vol. 13, ed. A. P. de Candolle (Paris: V. Masson), 1–690. doi: 10.2307/j.ctt16vj2hs.4
Echeverría-Londoño, S., Särkinen, T., Fenton, I. S., Purvis, A., and Knapp, S. (2020). Dynamism and context-dependency in diversification of the megadiverse plant genus Solanum (Solanaceae). J. Syst. Evol. 58, 767–782. doi: 10.1111/jse.12638
Falistocco, E., Passeri, V., and Marconi, G. (2007). Investigations of 5S rDNA of Vitis vinifera L.: sequence analysis and physical mapping. Genome 50, 927–938. doi: 10.1139/g07-070
Fory Sánchez, P. A., Sánchez Mosquera, I., Bohórquez Cháux, A., Ramírez, H., Medina Cano, C., and Lobo Arias, M. L. (2010). Genetic variability of the Colombian collection of Lulo (Solanum quitoense Lam.) and related species of section Lasiocarpa. Rev. Fac. Nac. Agron. Medellín 63, 5465–5476.
Frodin, D. G. (2004). History and concepts of big plant genera. Taxon 53, 753–776. doi: 10.2307/4135449
Fulnecek, J., Lim, K. Y., Leitch, A. R., Kovarik, A., and Matyasek, R. (2002). Evolution and structure of 5S rDNA loci in allotetraploid Nicotiana tabacum and its putative parental species. Heredity 88, 19–25. doi: 10.1038/sj.hdy.6800001
Gagnon, E., Hilgenhof, R., Orejuela, A., McDonnell, A., Sablok, G., Aubriot, X., et al. (2021). Phylogenomic data reveal hard polytomies across the backbone of the large genus Solanum (Solanaceae). bioRxiv [Preprint] doi: 10.1101/2021.03.25.436973
Galián, J. A., Rosato, M., and Rosselló, J. A. (2014). Partial sequence homogenization in the 5S multigene families may generate sequence chimeras and spurious results in phylogenetic reconstructions. Syst. Biol. 63, 219–230. doi: 10.1093/sysbio/syt101
Garcia, S., Garnatje, T., and Kovarik, A. (2012). Plant rDNA database: ribosomal DNA loci information goes online. Chromosoma 121, 389–394. doi: 10.1007/s00412-012-0368-7
Gavrilenko, T., Antonova, O., Shuvalova, A., Krylova, E., Alpatyeva, N., Spooner, D. M., et al. (2013). Genetic diversity and origin of cultivated potatoes based on plastid microsatellite polymorphism. Genet. Resour. Crop Evol. 60, 1997–2015. doi: 10.1007/s10722-013-9968-1
Hardigan, M. A., Bamberg, J., Buell, C. R., and Douches, D. S. (2015). Taxonomy and genetic differentiation among wild and cultivated germplasm of Solanum sect. Petota. Plant Genome 8:elantgenome2014.06.0025. doi: 10.3835/plantgenome2014.06.0025
Hasterok, R., Wolny, E., Hosiawa, M., Kowalczyk, M., Kulak-Ksiazczyk, S., Ksiazczyk, T., et al. (2006). Comparative analysis of rDNA distribution in chromosomes of various species of Brassicaceae. Ann. Bot. 97, 205–216. doi: 10.1093/aob/mcj031
Hawkes, J. G. (1962). The origin of Solanum juzepczukii Buk. and S. curtilobum Juz. et Buk. Z. Pflanzenzüchtung 47, 1–14.
Hawkes, J. G. (1990). The potato: evolution, biodiversity and genetic resources. Am. J. Pot. Res. 67, 733–735. doi: 10.1007/BF03044023
Hemleben, V., and Werts, D. (1988). Sequence organization and putative regulatory elements in the 5S rRNA genes of two higher plants (Vigna radiata and Matthiola incana). Gene 62, 165–169. doi: 10.1016/0378-1119(88)90591-4
Hijmans, R. J., Gavrilenko, T., Stephenson, S., Bamberg, J., Salas, A., and Spooner, D. M. (2007). Geographical and environmental range expansion through polyploidy in wild potatoes (Solanum section Petota). Glob. Ecol. Biogeogr. 16, 485–495. doi: 10.1111/j.1466-8238.2007.00308
Hosaka, K. (1995). Successive domestication and evolution of the Andean potatoes as revealed by chloroplast DNA restriction endonuclease analysis. Theor. Appl. Genet. 90, 356–363. doi: 10.1007/BF00221977
Huang, B., Ruess, H., Liang, Q., Colleoni, C., and Spooner, D. M. (2019). Analyses of 202 plastid genomes elucidate the phylogeny of Solanum section Petota. Sci. Rep. 9:4454. doi: 10.1038/s41598-019-40790-5
Huson, D. H., and Bryant, D. (2006). Application of phylogenetic networks in evolutionary studies. Mol. Biol. Evol. 23, 254–267. doi: 10.1093/molbev/msj030
Ishchenko, O. O., Bednarska, I. O., and Panchuk, I. I. (2021). Application of 5S ribosomal DNA for molecular taxonomy of subtribe Loliinae (Poaceae). Cytol. Genet. 55, 10–18. doi: 10.3103/S0095452721010096
Ishchenko, O. O., Mel’nyk, V. M., Parnikoza, I. Y., Budzhak, V. V., Panchuk, I. I., Kunakh, V. A., et al. (2020). Molecular organization of 5S ribosomal DNA and taxonomic status of Avenella flexuosa (L.) Drejer (Poaceae). Cytol. Genet. 54, 505–513. doi: 10.3103/S0095452720060055
Ishchenko, O. O., Panchuk, I. I., Andreev, I. O., Kunakh, V. A., and Volkov, R. A. (2018). Molecular organization of 5S ribosomal DNA of Deschampsia antarctica. Cytol. Genet. 52, 416–421. doi: 10.3103/S0095452718060105
Katoh, K., Rozewicki, J., and Yamada, K. D. (2019). MAFFT online service: multiple sequence alignment, interactive sequence choice and visualization. Brief. Bioinformatics 20, 1160–1166. doi: 10.1093/bib/bbx108
Knapp, S., Aubriot, X., and Prohens, J. (2019). “Eggplant (Solanum melongena L.): taxonomy and relationships,” in The Eggplant Genome. Compendium of Plant Genomes, ed. M. Chapman (Cham: Springer), 11–22. doi: 10.1007/978-3-319-99208-2_2
Knapp, S., Bohs, L., Nee, M., and Spooner, D. M. (2004). Solanaceae — a model for linking genomics with biodiversity. Comp. Funct. Genomics 5, 285–291. doi: 10.1002/cfg.393
Knapp, S., Sagona, E., Carbonell, A. K. Z., and Chiarini, F. (2017). A revision of the Solanum elaeagnifolium clade (Elaeagnifolium clade; subgenus Leptostemonum, Solanaceae). PhytoKeys 84, 1–104. doi: 10.3897/phytokeys.84.12695
Komarova, N. Y., Grimm, G. W., Hemleben, V., and Volkov, R. A. (2008). Molecular evolution of 35S rDNA and taxonomic status of Lycopersicon within Solanum sect. Petota. Plant Syst. Evol. 276, 59–71. doi: 10.1007/s00606-008-0091-2
Kumar, S., Stecher, G., Li, M., Knyaz, C., and Tamura, K. (2018). MEGA X: molecular evolutionary genetics analysis across computing platforms. Mol. Biol. Evol. 35, 1547–1549. doi: 10.1093/molbev/msy096
Layat, E., Sáez-Vásquez, J., and Tourmente, S. (2012). Regulation of Pol I-transcribed 45S rDNA and Pol III-transcribed 5S rDNA in Arabidopsis. Plant Cell Physiol. 53, 267–276. doi: 10.1093/pcp/pcr177
Levin, R. A., Myers, N. R., and Bohs, L. (2006). Phylogenetic relationships among the “spiny Solanums” (Solanum subgenus Leptostemonum, Solanaceae). Am. J. Bot. 93, 157–169. doi: 10.3732/ajb.93.1.157
Mahelka, V., Kopecký, D., and Baum, B. R. (2013). Contrasting patterns of evolution of 45S and 5S rDNA families uncover new aspects in the genome constitution of the agronomically important grass Thinopyrum intermedium (Triticeae). Mol. Biol. Evol. 30, 2065–2086. doi: 10.1093/molbev/mst106
Martine, C. T., Anderson, G. J., and Les, D. H. (2009). Gender-bending aubergines: molecular phylogenetics of cryptically dioecious Solanum in Australia. Aust. Syst. Bot. 22, 107–120. doi: 10.1071/SB07039
Martine, C. T., Cantley, J. T., Frawley, E. S., Butler, A. R., and Jordon-Thaden, I. E. (2016). New functionally dioecious bush tomato from Northwestern Australia, Solanum ossicruentum, may utilize “trample burr” dispersal. PhytoKeys 63, 19–29. doi: 10.3897/phytokeys.63.7743
Martine, C. T., Jordon-Thaden, I. E., McDonnell, A. J., Cantley, J. T., Hayes, D. S., Roche, M. D., et al. (2019). Phylogeny of the Australian Solanum dioicum group using seven nuclear genes, with consideration of Symon’s fruit and seed dispersal hypotheses. PLoS One 14:e0207564. doi: 10.1371/journal.pone.0207564
Martine, C. T., Vanderpool, D., Anderson, G. J., and Les, D. H. (2006). Phylogenetic relationships of andromonoecious and dioecious Australian species of Solanum subgenus Leptostemonum section Melongena: inferences from ITS sequence data. Syst. Bot. 31, 410–420. doi: 10.1600/036364406777585801
Matyasek, R., Dobesova, E., Huska, D., Ježková, I., Soltis, D. E., Soltis, P. S., et al. (2016). Interpopulation hybridization generates meiotically stable rDNA epigenetic variants in allotetraploid Tragopogon mirus. Plant J. 85, 362–377. doi: 10.1111/tpj.13110
Matyasek, R., Fulnecek, J., Lim, K. Y., Leitch, A. R., and Kovarik, A. (2002). Evolution of 5S rDNA unit arrays in the plant genus Nicotiana (Solanaceae). Genome 45, 556–562. doi: 10.1139/g02-017
Mlinarec, J., Franjević, D., Bočkor, L., and Besendorfer, V. (2016). Diverse evolutionary pathways shaped 5S rDNA of species of tribe Anemoneae (Ranunculaceae) and reveal phylogenetic signal. Bot. J. Linn. Soc. 182, 80–99. doi: 10.1111/boj.12452
Nee, M. (1999). “Synopsis of Solanum in the New World,” in Solanaceae IV: Advances in Biology and Utilization, eds M. Nee, D. E. Symon, R. N. Lester, and J. P. Jessop. (Kew: Royal Botanic Gardens), 285–333.
Olmstead, R. G., Bohs, L., Migid, H. A., Santiago-Valentin, E., Garcia, V. F., and Collier, S. M. (2008). A molecular phylogeny of the solanaceae. Taxon 57, 1159–1181. doi: 10.1002/tax.574010
Park, Y. K., Park, K. C., Park, C. H., and Kim, N. S. (2000). Chromosomal localization and sequence variation of 5S rRNA gene in five Capsicum species. Mol. Cells 10, 18–24. doi: 10.1007/s10059-000-0018-4
Pinhal, D., Yoshimura, T. S., Araki, C. S., and Martins, C. (2011). The 5S rDNA family evolves through concerted and birth-and-death evolution in fish genomes: an example from freshwater stingrays. BMC Evol. Biol. 11:151. doi: 10.1186/1471-2148-11-151
Poczai, P., Hyvönen, J., and Symon, D. E. (2011). Phylogeny of kangaroo apples (Solanum subg. Archaesolanum, Solanaceae). Mol. Biol. Rep. 38, 5243–5259. doi: 10.1007/s11033-011-0675-8
Pontes, O., Neves, N., Silva, M., Lewis, M. S., Madlung, A., Comai, L., et al. (2004). Chromosomal locus rearrangements are a rapid response to formation of the allotetraploid Arabidopsis suecica genome. Proc. Natl. Acad. Sci. U.S.A. 101, 18240–18245. doi: 10.1073/pnas.0407258102
Porebski, S., Bailey, L. G., and Baum, B. R. (1997). Modification of a CTAB DNA extraction protocol for plants containing high polysaccharide and polyphenol components. Plant Mol. Biol. Rep. 15, 8–15. doi: 10.1007/BF02772108
Prohens, J., Plazas, M., Raigón, M. D., Seguí-Simarro, J. M., Stommel, J. R., and Vilanova, S. (2012). Characterization of interspecific hybrids and first backcross generations from crosses between two cultivated eggplants (Solanum melongena and S. aethiopicum Kumba group) and implications for eggplant breeding. Euphytica 186, 517–538. doi: 10.1007/s10681-012-0652-x
Qin, Q. B., Liu, Q. W., Wang, C. Q., Cao, L., Zhou, Y. W., Qin, H., et al. (2019). Molecular organization and chromosomal localization analysis of 5S rDNA clusters in autotetraploids derived from Carassius auratus Red Var. (♀) × Megalobrama amblycephala (♂). Front. Genet. 10:437. doi: 10.3389/fgene.2019.00437
Randell, B. R., and Symon, D. E. (1976). Chromosome numbers in Australian Solanum species. Aust. J. Bot. 24, 369–379. doi: 10.1071/BT9760369
Reuter, J. S., and Mathews, D. H. (2010). RNA structure: software for RNA secondary structure prediction and analysis. BMC Bioinformatics 11:129. doi: 10.1186/1471-2105-11-129
Rodríguez, F., Ghislain, M., Clausen, A. M., Jansky, S. H., and Spooner, D. M. (2010). Hybrid origins of cultivated potatoes. Theor. Appl. Genet. 121, 1187–1198. doi: 10.1007/s00122-010-1422-6
Röser, M., Winterfeld, G., Grebenstein, B., and Hemleben, V. (2001). Molecular diversity and physical mapping of 5S rDNA in wild and cultivated oat grasses (Poaceae: Aveneae). Mol. Phylogent. Evol. 21, 198–217. doi: 10.1006/mpev.2001.1003
Särkinen, T., Barboza, G. E., and Knapp, S. (2015). True black nightshades: phylogeny and delimitation of the morelloid clade of Solanum. Taxon 64, 945–958. doi: 10.12705/645.5
Särkinen, T., Bohs, L., Olmstead, R. G., and Knapp, S. (2013). A phylogenetic framework for evolutionary study of the nightshades (Solanaceae): a dated 1000-tip tree. BMC Evol. Biol. 13:214. doi: 10.1186/1471-2148-13-214
Schmiediche, P. E., Hawkes, J. G., and Ochoa, C. M. (1980). Breeding of the cultivated potato species Solanum x juzepczukii Buk. and Solanum x curtilobum Juz. et Buk. Euphytica 29, 685–704. doi: 10.1007/BF00023216
Seithe, A. (1962). Die Haararten der Gattung Solanum L. und ihre taxonomische Verwertung. Bot. Jahrb. Syst. Pflanzengesch. Pflanzengeogr. 81, 261–336.
Simeone, M. C., Cardoni, S., Piredda, R., Imperatori, F., Avishai, M., Grimm, G. W., et al. (2018). Comparative systematics and phylogeography of Quercus section Cerris in western Eurasia: inferences from plastid and nuclear DNA variation. PeerJ 6:e5793. doi: 10.7717/peerj.5793
Simon, L., Rabanal, F. A., Dubos, T., Oliver, C., Lauber, D., Poulet, A., et al. (2018). Genetic and epigenetic variation in 5S ribosomal RNA genes reveals genome dynamics in Arabidopsis thaliana. Nucleic Acids Res. 46, 3019–3033. doi: 10.1093/nar/gky163
Song, J., Shi, L., Li, D., Sun, Y., Niu, Y., Chen, Z., et al. (2012). Extensive pyrosequencing reveals frequent intra-genomic variations of internal transcribed spacer regions of nuclear ribosomal DNA. PLoS One 7:e43971. doi: 10.1371/journal.pone.0043971
Spooner, D. M., Ghislain, M., Simon, R., Jansky, S. H., and Gavrilenko, T. (2014). Systematics, diversity, genetics, and evolution of wild and cultivated potatoes. Bot. Rev. 80, 283–383. doi: 10.1007/s12229-014-9146-y
Spooner, D. M., McLean, K., Ramsay, G., Waugh, R., and Bryan, G. J. (2005). A single domestication for potato based on multilocus amplified fragment length polymorphism genotyping. Proc. Natl. Acad. Sci. U.S.A. 102, 14694–14699. doi: 10.1073/pnas.0507400102
Stone, B. C. (1970). The flora of Guam: a manual for the identification of the vascular plants of the island. Micronesica 6, 1–659.
Sun, F. J.Caetano-Anollés, G. (2009). The evolutionary history of the structure of 5S ribosomal RNA. J. Mol. Evol. 69, 430–443. doi: 10.1007/s00239-009-9264-z
Sun, Y. L., Kang, H. M., Kim, Y. S., Baek, J. P., Zheng, S. L., Xiang, J. J., et al. (2014). Tomato (Solanum lycopersicum) variety discrimination and hybridization analysis based on the 5S rRNA region. Biotechnol. Biotechnol. Equip. 28, 431–437. doi: 10.1080/13102818.2014.928499
Tucker, S., Vitins, A., and Pikaard, C. S. (2010). Nucleolar dominance and ribosomal RNA gene silencing. Curr. Opin. Cell Biol. 22, 351–356. doi: 10.1016/j.ceb.2010.03.009
Tynkevich, Y. O., Nevelska, A. O., Chorney, I. I., and Volkov, R. A. (2015). Organization and variability of the 5S rDNA intergenic spacer of Lathyrus venetus. Bull. Vavilov Soc. Genet. Breed. Ukr. 13, 81–87.
Tynkevich, Y. O., and Volkov, R. A. (2014). Structural organization of 5S ribosomal DNA in Rosa rugosa. Cytol. Genet. 48, 1–6. doi: 10.3103/S0095452714010095
Tynkevich, Y. O., and Volkov, R. A. (2019). 5S ribosomal DNA of distantly related Quercus species: molecular organization and taxonomic application. Cytol. Genet. 53, 459–466. doi: 10.3103/S0095452719060100
Vaillant, I., Tutois, S., Cuvillier, C., Schubert, I., and Tourmente, S. (2007). Regulation of Arabidopsis thaliana 5S rRNA genes. Plant Cell Physiol. 48, 745–752. doi: 10.1093/pcp/pcm043
Vizoso, M., Vierna, J., González-Tizón, A. M., and Martínez-Lage, A. (2011). The 5S rDNA gene family in mollusks: characterization of transcriptional regulatory regions, prediction of secondary structures, and long-term evolution, with special attention to mytilidae mussels. J. Heredity 102, 433–447. doi: 10.1093/jhered/esr046
Volkov, R. A., Borisjuk, N. V., Panchuk, I. I., Schweizer, D., and Hemleben, V. (1999b). Elimination and rearrangement of parental rDNA in the allotetraploid Nicotiana tabacum. Mol. Biol. Evol. 16, 311–320. doi: 10.1093/oxfordjournals.molbev.a026112
Volkov, R. A., Bachmair, A., Panchuk, I. I., Kostyshyn, S. S., and Schweizer, D. (1999a). 25S-18S rDNA intergenic spacer of Nicotiana sylvestris (Solanaceae): primary and secondary structure analysis. Plant Syst. Evol. 218, 89–97. doi: 10.1007/bf01087037
Volkov, R. A., Komarova, N. Y., and Hemleben, V. (2007). Ribosomal DNA in plant hybrids: inheritance, rearrangement, expression. Syst. Biodivers. 5, 261–276. doi: 10.1017/S1477200007002447
Volkov, R. A., Komarova, N. Y., Panchuk, I. I., and Hemleben, V. (2003). Molecular evolution of rDNA external transcribed spacer and phylogeny of sect. Petota (genus Solanum). Mol. Phylogenet. Evol. 29, 187–202. doi: 10.1016/s1055-7903(03)00092-7
Volkov, R. A., Medina, F. J., Zentgraf, U., and Hemleben, V. (2004). Molecular cell biology: organization and molecular evolution of rDNA, nucleolar dominance, and nucleolus structure. Prog. Bot. 65, 106–146. doi: 10.1007/978-3-642-18819-0_5
Volkov, R. A., Panchuk, I. I., Borisjuk, N. V., Hosiawa-Baranska, M., Maluszynska, J., and Hemleben, V. (2017). Evolutional dynamics of 45S and 5S ribosomal DNA in ancient allohexaploid Atropa belladonna. BMC Plant Biol. 17:21. doi: 10.1186/s12870-017-0978-6
Volkov, R. A., Zanke, C., Panchuk, I. I., and Hemleben, V. (2001). Molecular evolution of 5S rDNA of Solanum species (sect. Petota): application for molecular phylogeny and breeding. Theor. Appl. Genet. 103, 1273–1282. doi: 10.1007/s001220100670
Vorontsova, M. S., and Knapp, S. (2016). A revision of the “spiny Solanums”, Solanum subgenus Leptostemonum (Solanaceae) in Africa and Madagascar. Syst. Bot. Monogr. 99, 1–436. doi: 10.3897/phytokeys.145.48531
Vozárová, R., Herklotz, V., Kovaøík, A., Tynkevich, Y. O., Volkov, R. A., Ritz, C. M., et al. (2021). Ancient origin of two 5S rDNA families dominating in the genus Rosa and their behavior in the Canina-type meiosis. Front. Plant Sci. 12:643548. doi: 10.3389/fpls.2021.643548
Weese, T. L., and Bohs, L. (2007). A three-gene phylogeny of the genus Solanum (Solanaceae). Syst. Bot. 32, 445–463. doi: 10.1600/036364407781179671
Keywords: 5S rDNA, genomics, molecular evolution, hybridization, polyploidy, taxonomy, Solanum
Citation: Tynkevich YO, Shelyfist AY, Kozub LV, Hemleben V, Panchuk II and Volkov RA (2022) 5S Ribosomal DNA of Genus Solanum: Molecular Organization, Evolution, and Taxonomy. Front. Plant Sci. 13:852406. doi: 10.3389/fpls.2022.852406
Received: 11 January 2022; Accepted: 25 February 2022;
Published: 13 April 2022.
Edited by:
Peter Poczai, University of Helsinki, FinlandReviewed by:
Natalia Borowska-Zuchowska, University of Silesia in Katowice, PolandJosep A. Rossello, Jardí Botànic UV, Spain
Hannes Becher, University of Edinburgh, United Kingdom
Copyright © 2022 Tynkevich, Shelyfist, Kozub, Hemleben, Panchuk and Volkov. This is an open-access article distributed under the terms of the Creative Commons Attribution License (CC BY). The use, distribution or reproduction in other forums is permitted, provided the original author(s) and the copyright owner(s) are credited and that the original publication in this journal is cited, in accordance with accepted academic practice. No use, distribution or reproduction is permitted which does not comply with these terms.
*Correspondence: Roman A. Volkov, r.volkov@chnu.edu.ua
†ORCID: Yurij O. Tynkevich, orcid.org/0000-0002-0222-8098; Antonina Y. Shelyfist, orcid.org/0000-0001-5711-3362; Liudmyla V. Kozub, orcid.org/0000-0002-2675-5896; Vera Hemleben, orcid.org/0000-0002-5171-472X; Irina I. Panchuk, orcid.org/0000-0002-2837-4480; Roman A. Volkov, orcid.org/0000-0003-0673-2598