- 1Shandong Provincial Key Laboratory of Plant Stress, College of Life Sciences, Shandong Normal University, Jinan, China
- 2State Key Laboratory of Crop Biology, College of Agronomic Sciences, Shandong Agricultural University, Tai’an, China
- 3Linyi Center for Disease Control and Prevention, Linyi, China
Salt stress is an important environmental factor limiting plant growth and crop production. Plant adaptation to salt stress can be improved by chemical pretreatment. This study aims to identify whether hydrogen peroxide (H2O2) pretreatment of seedlings affects the stress tolerance of Arabidopsis thaliana seedlings. The results show that pretreatment with H2O2 at appropriate concentrations enhances the salt tolerance ability of Arabidopsis seedlings, as revealed by lower Na+ levels, greater K+ levels, and improved K+/Na+ ratios in leaves. Furthermore, H2O2 pretreatment improves the membrane properties by reducing the relative membrane permeability (RMP) and malonaldehyde (MDA) content in addition to improving the activities of antioxidant enzymes, including superoxide dismutase, and glutathione peroxidase. Our transcription data show that exogenous H2O2 pretreatment leads to the induced expression of cell cycle, redox regulation, and cell wall organization-related genes in Arabidopsis, which may accelerate cell proliferation, enhance tolerance to osmotic stress, maintain the redox balance, and remodel the cell walls of plants in subsequent high-salt environments.
Introduction
Salt stress seriously influences plant growth, development, and crop yield (Deinlein et al., 2014; Gong et al., 2020; Zhao et al., 2020). High salinity can cause hyperosmotic stress, ion toxicity, nutrient deficiency, and subsequent oxidative damage due to the overproduction of reactive oxygen species (ROS) in plants, ultimately leading to plant cell dysfunction, growth inhibition, leaf senescence, and even plant death (Munns and Tester, 2008; Van Zelm et al., 2020). In order to adapt to salt stress, plants have developed a series of sophisticated physiological mechanisms, such as the adjustment of membrane systems, reconstruction of ionic and osmotic homeostasis, modification of cell wall structure, and maintenance of redox balance (Cramer et al., 2011; Van Zelm et al., 2020). In addition to these physiological mechanisms, there exist measures in production practice to increase salt tolerance, such as gene engineering, chemical pretreatment, and abiotic stress acclimation (Shen et al., 2014; Tian et al., 2018). Among the various strategies, chemical pretreatment, especially hydrogen peroxide (H2O2) pretreatment, is a very simple, low-cost, and effective approach to enhance plant tolerance to environmental stresses (Ashraf and Foolad, 2005; Beckers et al., 2009; Wahid and Shabbir, 2015).
H2O2 is the most stable component of ROS and has generally been considered to be a toxic cellular metabolite (Anjum et al., 2015). On the other hand, it can function as a signaling molecule in both animal and plant cells, adjusting their tolerance to adverse environments (Cerny et al., 2018). Several previous studies have reported that H2O2 may play a dual role in plants (Neill et al., 2002). At high concentrations, H2O2 can cause lipid peroxidation, protein disfunction, and programmed cell death. By contrast, at low concentrations, H2O2 acts as a messenger molecule that may directly regulate the expression of numerous genes and trigger the responses of plants to abiotic stresses (Vandenabeele et al., 2003; Petrov and Van Breusegem, 2012). Hence, H2O2 signaling is of potential significance in improving crop tolerance to environmental stresses.
Several studies have shown that the pretreatment of plants with exogenous H2O2 can significantly increase abiotic stress tolerance. For example, pretreatment of H2O2 protected Arabidopsis thaliana leaves against excess light damage (Karpinski et al., 1999), induced the adaptation of rice seedlings to salt stress and high temperature (Uchida et al., 2002), improved the salt resistances of barley (Fedina et al., 2009), maize (Gondim et al., 2012) and sunflower (Silva et al., 2020), enhanced the chilling tolerance of the two Zoysia cultivars Manila grass (Zoysia matrella) and Mascarene grass (Zoysia tenuifolia) (Wang et al., 2010), induced salt stress acclimation in maize plants (De Azevedo Neto et al., 2005), and alleviates drought stress in soybean plants (Ishibashi et al., 2011). The pretreatment of wheat seeds with H2O2 also enhanced the subsequent drought (He et al., 2009) and salt (Wahid et al., 2007) resistances of the seedlings. Additionally, H2O2 pretreatment protected tobacco from oxidative stresses generated by high light intensities or the catalase inhibitor aminotriazole through induction of a set of antioxidant enzymes (Gechev et al., 2002). Therefore, the accumulation of H2O2 in specific tissues and at appropriate levels could enhance the activities of antioxidant enzymes and, therefore, aid plants in adaptation to different unfavorable environmental cues (Bowler and Fluhr, 2000).
Although H2O2 pretreatment is important for improving plant salt tolerance, little is known about the mechanism of salt tolerance improvement by H2O2 pretreatment during the growth and development of plants.
In this study, 4-week-old Arabidopsis leaves were sprayed with H2O2 before being subjected to NaCl stress. We found that H2O2 pretreatment resulted in improvements to some physiological and biochemical responses of the Arabidopsis seedling to salt stress. To obtain insights into the molecular mechanisms of H2O2-induced salt tolerance, we then performed transcriptome profiling of the Arabidopsis seedlings under H2O2 pretreatment followed by salt stress. This work aims to understand the mechanisms of salt stress acclimation in Arabidopsis induced by H2O2.
Materials and Methods
Plant Materials and Growth Conditions
Arabidopsis thaliana ecotype Columbia (Col-0) was used in this study. Arabidopsis seeds were sterilized with 75% (v/v) ethanol and then washed with sterile distilled water. The seeds were then sown on half-strength Murashige and Skoog (MS) medium. After stratification for 3 days in a 4°C refrigerator, the plates were transferred to growth chambers. Eight days after seed germination, Arabidopsis seedlings were transferred into 9 cm diameter pots containing soil, perlite, and vermiculite (2:1:1), with irrigation of half-strength Hoagland’s nutrient solution. The growth condition in the growth chambers was a 16 h light/8 h dark photoperiod with a day/night thermoperiod of 22°C/18°C, a relative humidity of 70%, and irradiance of 110 μmol m–2 s–1.
H2O2 Foliar Spraying of Arabidopsis Seedling Followed by NaCl Stress
Four-week-old seedlings were randomly divided into four groups, and the seedlings in each group were subjected to treatment as follows: pretreatment–stressed (pretreated with H2O2 and salt-stressed, HN); non-pretreatment–stressed (pretreated with water and salt-stressed, WN); pretreatment–non-stressed (pretreated with H2O2 and not salt-stressed, HW); and non-pretreatment–non-stressed (i.e., control; pretreated with water and not salt-stressed, WW). For pretreatment–stressed and pretreatment–non-stressed plants, leaves were sprayed with 20 μM of H2O2 solution four times at 4-h intervals, while non-pretreatment–stressed and non-pretreatment–non-stressed plants were sprayed with water. Twenty-four hours after foliar spraying, Arabidopsis seedlings from pretreatment–stressed and non-pretreatment–stressed groups were subsequently watered with 150 mM NaCl every day, whereas pretreatment–non-stressed and control plants were treated with water. Twelve hours after treatment with 150 mM NaCl, Arabidopsis seedlings were collected for transcriptome profiling analysis. Four days after treatment with 150 mM NaCl, Arabidopsis seedlings were collected for the determination of various physiological parameters.
Measurement of Dry and Fresh Weight of Seedlings
The fresh weight of the shoots from each treatment was determined immediately after harvesting, and samples were dried in an oven at 70°C for 24 h to obtain dry weights. Twenty individual plants were collected for each replicate and triplicates were analyzed in parallel.
Determination of Relative Membrane Permeability
The relative membrane permeability (RMP) of the seedlings was determined following the method of Yang et al. (1996). Excised fresh leaves (0.5 g) were immediately put into test tubes containing 10 mL of deionized distilled water and briefly vortexed. The solution was used to measure initial electrical conductivity (EC0). The test tubes containing leaves in distilled water were kept at 4°C for 24 h and EC1 was determined. The test tubes were then placed in a boiling water bath for 10 min, cooled to room temperature, and the boiled leachate was filtered and measured for EC2. RMP was computed using the following formula: RMP (%) = [(EC1 − EC0)/(EC2 − EC0)] × 100.
Measurement of Malonaldehyde
The level of lipid peroxidation in the leaf tissue was measured in terms of MDA (a product of lipid peroxidation) content, detected by the thiobarbituric acid reaction using the method of Dhindsa et al. (1981). Fresh leaf samples (0.4 g) were homogenized in 5 mL of 0.1% trichloroacetic acid, vortexed, and then 4 mL of 0.5% thiobarbituric acid was added. The mixture was heated to 95°C for 30 min and was quickly cooled in an ice bath. Afterward, the mixture was centrifuged at 3000 rpm for 10 min. The supernatant fraction was collected, and the absorbance of the supernatant at 532 and 600 nm was read. The value for the non-specific absorption at 600 nm was subtracted from that at 532 nm (Zhang and Kirkham, 1996). The concentration of MDA was calculated using the extinction coefficient of MDA (155 mM–1 cm–1) (Heath and Packer, 1968) and expressed as nmol MDA g–1 fresh weight. Each treatment was carried out in triplicate.
Determination of Na+ and K+ Content
Fifteen dry plants were pooled together and ground into fine powder; 0.02 g of dry powder was ashed in a muffle furnace at 300°C for 2 h, then 550°C for 10 h. The ash was resolved into small amounts of concentrated nitric acid and adjusted to a final volume of 10 mL. The ion content of samples was determined with a flame photometer (2655-00 Digital Flame Analyzer, Cole-Parmer Instrument Company, Chicago, IL, United States). Three independent determinations were performed for each treatment (Song et al., 2005).
Transcriptome Profiling Analysis
Twelve hours after treatment with 150 mM NaCl, Arabidopsis seedlings from the four experimental groups (WW, WN, HW, and HN) were collected to extract total RNA using Biozol reagent (Bio Flux, Beijing, China) according to the manufacturer’s instructions. The integrity and quality of the isolated RNA were monitored by agarose gel electrophoresis. RNA concentration was quantified by a Nanodrop ND-1000 spectrophotometer (Thermo Scientific, Massachusetts, United States). The qualified RNA samples were sent to the Annoroad Gene Technology Corporation (Beijing, China), and the libraries were sequenced on an Illumina platform and 150 bp paired-end reads were generated. RNA-Seq data of the four experimental samples were obtained from three biological replicates, respectively. RNA-Seq data were deposited into the NCBI’s Sequence Read Archive (the accession number is PRJNA612654).
Gene Ontology and Kyoto Encyclopedia of Genes and Genomes Enrichment Analysis
Low-quality reads were trimmed using Trimmomatic (Bolger et al., 2014) (v 0.36) with the settings “LEADING:3 TRAILING:3 SLIDINGWINDOW:4:15 MINLEN:36”. Clean reads were mapped to the Arabidopsis TAIR10 release obtained from TAIR1 using TopHat (v 2.1.1) with settings “-N 1 –num-threads 6”. Count data were generated by Cufflinks (v 2.2.1) and FPKM (fragments per kilobase per million mapped reads) was used to estimate the expression levels of individual genes.
Differentially expressed genes (DEGs) were identified by DESeq2 Moderated estimation of fold change and dispersion (Love et al., 2014) using the Bioconductor software2, based on a comparison across all samples under control or different experimental conditions with false discovery rate (FDR) less than 0.05. The Goatools (v 0.8.9) python package was used for GO term enrichment (Klopfenstein et al., 2018) with the Arabidopsis association files downloaded from TAIR10. Kyoto Encyclopedia of Genes and Genomes (KEGG) pathway enrichment analysis of genes was performed using clusterProfiler package (v 4.2.2, Wu et al., 2021).
Reverse Transcription and Quantitative Real-Time PCR Analysis
Transcriptome profiling results were validated and verified by quantitative real-time PCR experiments, in which 2 μg of total RNA was used for reverse transcription to obtain the cDNA using FastQuant RT Kit (with gDNase, TIANGEN, Beijing, China). The SuperReal PreMix Plus Kit (SYBR Green, TIANGEN) was used along with the cDNA for quantitative real-time PCR experiments using a real-time fluorescence quantitative PCR instrument (LightCycler® 96, Roche, Basel, Switzerland). All reactions were assayed using three replicates. Actin2 was used as an endogenous control. The relative expression levels are presented as values relative to that of the corresponding control sample at the indicated time after normalization to actin transcript levels. Primer sequences are shown in the Supplementary Table 1.
Measurement of Enzyme Activity
Extract Preparation
Frozen leaves (0.2 g) were crushed into a fine powder with a mortar and pestle in liquid N2. Soluble proteins were extracted by homogenizing the powder in 1 mL of 100 mM potassium phosphate buffer (pH 7.5) containing 2 mM EDTA, 1% (w/v) PVP-40, 10 mM DTT, and 1 mM PMSF. The homogenate was centrifuged at 12,000 rpm for 15 min and the supernatant fraction was used as a crude extract for enzyme activity. All operations were carried out at 4°C. The protein concentration was determined using the Bradford method (Bradford, 1976).
Enzyme Activity Assays
Superoxide Dismutase
Total superoxide dismutase (SOD) activity was determined by measuring its ability to inhibit the photochemical reduction of nitro blue tetrazolium chloride (NBT), as described by Giannopolitis and Ries (1977). The reaction mixture (3 mL) contained 50 mM phosphate buffer (pH 7.8), 0.1 mM EDTA, 13 mM methionine, 75 μM NBT, 2 μM riboflavin, 0.05 M sodium carbonate (pH 10.2), and 100 μL enzyme extract. Riboflavin was added last and the tubes were shaken under fluorescent lamps at 110 μmol m–2 s–1. This reaction was allowed to proceed for 15 min, after which the lights were switched off and the tubes were covered with a black cloth. The absorbance of the reaction mixture was read at 560 nm. One unit of SOD activity (U) was defined as the amount of enzyme required to cause 50% inhibition of the NBT photoreduction rate. Results are expressed as units mg–1 protein per minute.
Glutathione Peroxidase
Total glutathione peroxidase (GPX) activity was determined as described by Drotar et al. (1985), with a reaction mixture (4 mL) containing 50 mM phosphate buffer (pH 7.0), 2.0 mM EDTA, 2.0 mM GSH, 0.1 mM NADPH, 2.5 units of glutathione reductase, and 100 μL enzyme extract; 0.09 mM H2O2 was added last to mark the beginning of the reaction. The reaction rate was measured by following the loss of NADPH spectrophotometrically at 340 nm. One unit of GPX activity was defined as the amount of enzyme that would cause the oxidation of 1.0 nmol of NADPH to NADP+ per minute at 25°C.
Statistical Analysis
All the above experiments involved three biological replicates, and each experiment (except RNA-Seq) was carried out twice at different times. All data are expressed as means ± standard deviation and the significance of differences between datasets was evaluated by one-way ANOVA following SPSS. P-values of <0.05 were considered to be significantly different.
Results
Effect of H2O2 Foliar Spraying on Physiological Indices of Arabidopsis Seedlings
In order to evaluate the effects of H2O2 pretreatment on Arabidopsis growth under salinity, we sprayed the leaves of Arabidopsis seedling with 20 μM H2O2 and subsequently exposed them to 150 mM NaCl. We then determined some physiological indices as shown below.
Dry and Fresh Weight
Data of shoot fresh weight and dry mass are shown in Figures 1A,B, respectively. Compared with controls, the pretreatment of seedlings with H2O2 significantly increased the aerial dry and fresh weight, regardless of the stress conditions. Although the salt-stressed plants had reduced shoot dry mass and fresh weight compared to unstressed plants, the growth inhibition caused by the salt stress decreased when the seedlings were sprayed with H2O2. Compared with WN plants, the HN plants increased shoot fresh weight and dry weight by 48.4 and 181.25%, respectively.
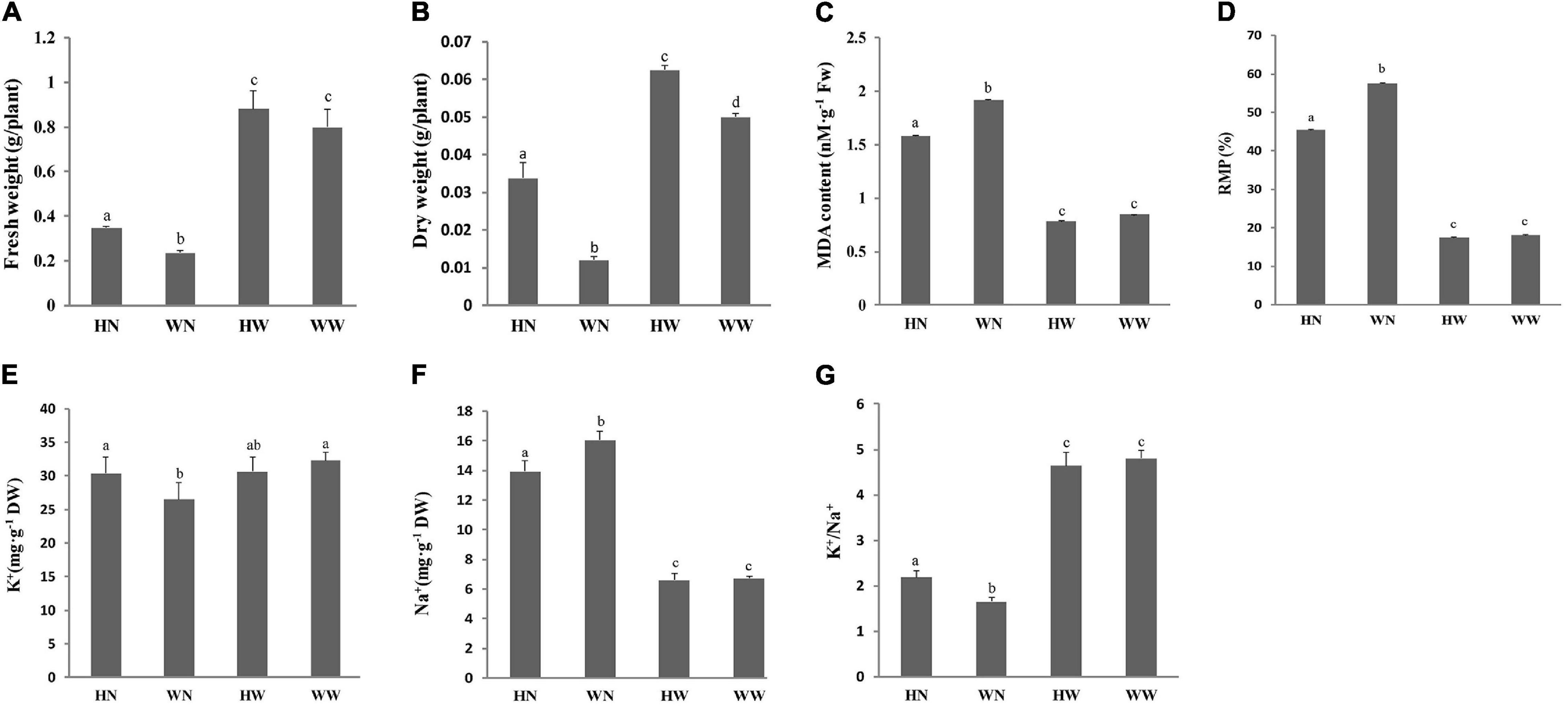
Figure 1. Physiological response of Arabidopsis seedlings to salt stress after H2O2 pretreatment. The influence of H2O2 pretreatment on the aerial fresh weight (A) and dry weight (B) of Arabidopsis seedlings under different treatments; the impact of H2O2 pretreatment on MDA content (C) and RMP (D) of Arabidopsis leaves under different treatments; and the impact of H2O2 pretreatment on K+ (E) and Na+ (F) content and K+/Na+ (G) in leaves of Arabidopsis seedlings under different treatments. Data are represented as means ± SD. Three biological replicates per experiment. Means for each treatment that do not share a common letter are significantly different at P < 0.05, estimated with one-way ANOVA following SPSS. WW, pretreated with water and not salt-stressed; WN, pretreated with water and salt-stressed; HW, pretreated with H2O2 and not salt-stressed; HN, pretreated with H2O2 and salt-stressed.
Effect of H2O2 Pretreatment on Malonaldehyde Content and the Relative Membrane Permeability of the Leaves
There were multiple significant differences in MDA content between the HN and WN groups under salt stress conditions. Compared to the WW group (control), the HW group had a slightly lower MDA content, however, the difference was not significant (Figure 1C). These results indicate that H2O2 pretreatment can reduce membrane lipid peroxidation of plant cells and, therefore, maintain the stability of the membrane.
Relative membrane permeability was greatly increased due to salinity, while the pretreatment of seedlings with 20 μM H2O2 reduced the RMP of corresponding seedlings under salt stress. Thus, the RMP of the WN group was higher than that of the HN group (Figure 1D).
Impact of H2O2 Pretreatment on Na+, K+ Content and K+/Na+ Ratio of Arabidopsis Shoots
Compared with the WN group, the seedlings in the HN group contained higher K+ (Figure 1E) and lower Na+ (Figure 1F) levels under the same salinity, which indicates that H2O2 pretreatment improved K+ uptake and K+/Na+ (Figure 1G) of Arabidopsis under salt stress conditions, thereby reducing the harm caused by Na+ to the plant.
Transcriptome Profiling Analysis
In order to analyze the molecular mechanisms of salt tolerance improvement induced by H2O2 pretreatment, we collected the leaves of 4-week-old Arabidopsis seedlings treated with HN, WN, HW, and WW (with WW being the control) and the total RNA was extracted for genome-wide transcriptome analysis. RNA-Seq data were analyzed from a total of twelve samples comprising three biological replicates for each treatment.
In total, 19,391 genes were detected in the leaves of Arabidopsis. We further obtained 1493 DEGs in HW, compared to WW, with at least twofold change of gene expression at P-value < 0.05. Among these, 993 genes were up-regulated and 500 genes were down-regulated (Figure 2A and Supplementary Table 2). Similarly, of the 2467 DEGs specifically responding to HN treatment in comparison to WW, 1212 genes were up-regulated whereas 1255 genes were down-regulated (Figure 2A and Supplementary Table 3). Among the 1533 DEGs in WN compared to WW, 922 genes were up-regulated while 604 genes were down-regulated (Figure 2A and Supplementary Table 4). We performed a preliminary analysis of up-regulated (Figure 2B) and down-regulated (Figure 2C) genes through Venn diagrams of HN vs. WW, HW vs. WW, and WN vs. WW. We found 602 unique DEGs in HW vs. WW (Supplementary Table 5), 455 unique DEGs in HN vs. WW (Supplementary Table 6), and 364 unique DEGs in WN vs. WW (Supplementary Table 7). There were an additional 361 DEGs that were common to both HW vs. WW and HN vs. WW (Supplementary Table 8), 169 DEGs common to both HW vs. WW and WN vs. WW (Supplementary Table 9), and 535 DEGs common to both HN vs. WW and WN vs. WW (Supplementary Table 10). Relevant data on down-regulated genes are also presented (Figure 2C and Supplementary Tables S11–16).
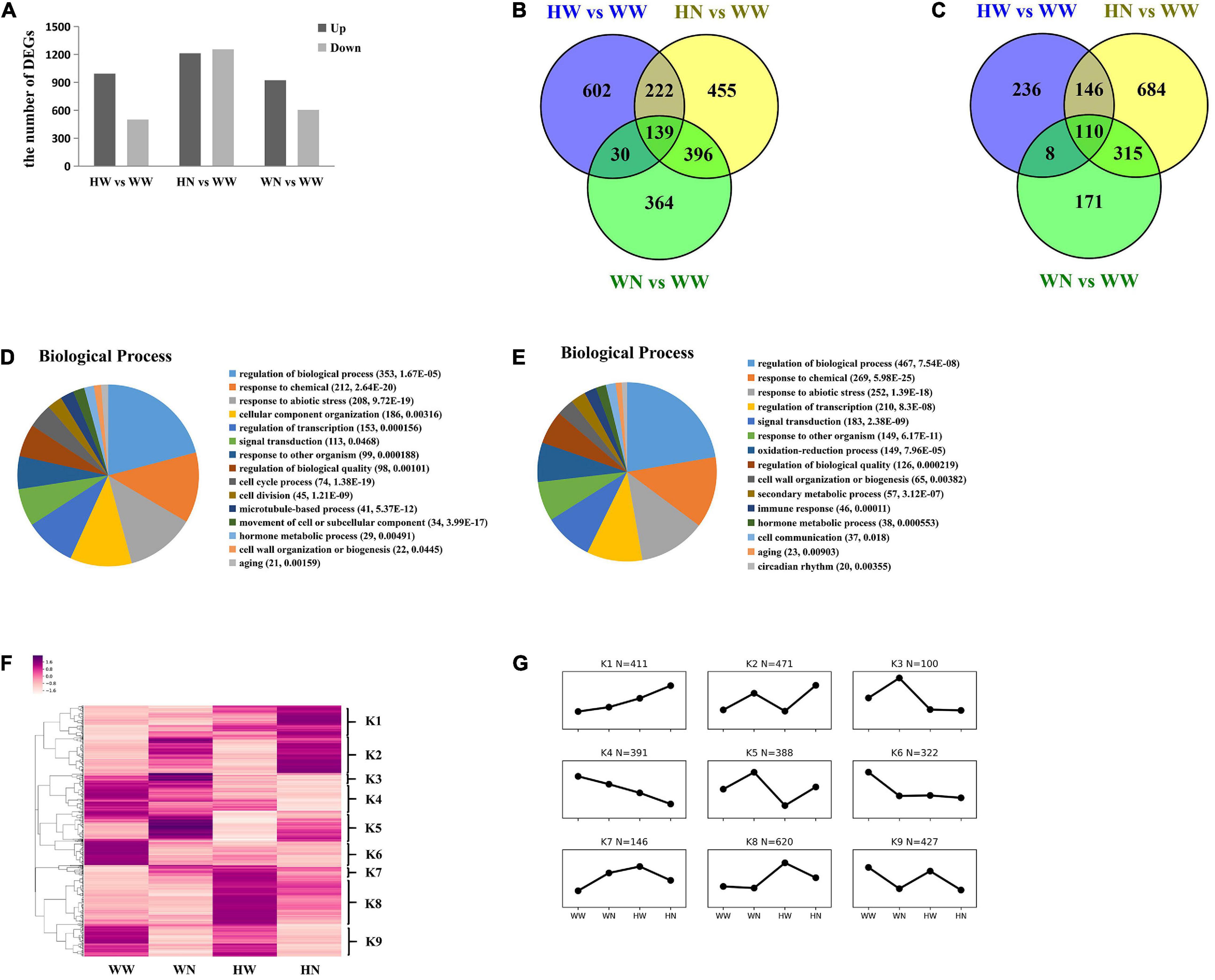
Figure 2. Transcriptome analysis of Arabidopsis leaves under WW, WN, HW, and HN treatments. (A) In HW vs. WW, 993 DEGs were up-regulated, whereas 500 DEGs were down-regulated. In HN vs. WW, 1212 DEGs were up-regulated, whereas 1255 DEGs were down-regulated. In WN vs. WW, 922 DEGs were up-regulated, whereas 604 DEGs were down-regulated. (B) Venn diagram of DEGs up-regulated in WN vs. WW, HW vs. WW, and HN vs. WW. There were 602 unique DEGs in HW vs. WW, 455 unique DEGs in HN vs. WW, and 364 unique DEGs in WN vs. WW. There were 361 DEGs common to both HW and HN, 169 DEGs common to both HW and WN, and 535 DEGs common to both HN and WN. (C) Venn diagram of DEGs down-regulated in WN vs. WW, HW vs. WW, and HN vs. WW. (D) Biological process in HW vs. WW; (E) biological process in HN vs. WW. DEGs were identified by DESeq2 using Bioconductor (http://www.bioconductor. org/) based on a comparison across all samples under control or HW and control or HN conditions with FDR less than 0.05. The Goatools (v0.8.9) python package was used for GO terms enrichment with the Arabidopsis association files downloaded from TAIR10. (F) 1493 DEGs in HW vs. WW and 1766 DEGs in HN vs. WW clustered by heat-mapping under WW, WN, HW, and HN treatments (p ≤ 0.05). (G) Nine groups of genes were identified based on the heatmap dendrogram. Averaged values for each condition in every group were used to generate the line chart, which represents the comprehensive expression patterns of each group. WW, pretreated with water and not salt-stressed; WN, pretreated with water and salt-stressed; HW, pretreated with H2O2 and not salt-stressed; HN, pretreated with H2O2 and salt-stressed.
In order to rule out that some DEGs may have only been influenced by salt stress in HN vs. WW, we removed the DEGs from HN vs. WW that did not have a large difference in abundance with WN vs. WW; that is, those genes whose ratio of log2FC (HN vs. WW)/log2FC (WN vs. WW) was between 0.67–1.5. Thus, there were still 1766 DEGs mainly affected by both H2O2 and NaCl in HN vs. WW at this time, where 780 DEGs were up-regulated and 986 DEGs were down-regulated. All subsequent data analysis on HN vs. WW was mainly carried out for these 1766 DEGs (Supplementary Table 17).
Gene ontology (GO) enrichment analysis was performed on 1493 and 1766 DEGs according to the biological processes in HW vs. WW (Figure 2D) and HN vs. WW (Figure 2E), respectively. GO terms both in HW vs. WW and HN vs. WW mainly included “regulation of biological processes” (HW vs. WW, P = 1.67 × 10–5; HN vs. WW, P = 7.54 × 10–8), “response to chemicals” (HW vs. WW, P = 2.64 × 10–20; HN vs. WW, P = 5.98 × 10–25), “response to abiotic stresses” (HW vs. WW, P = 9.72 × 10–19; HN vs. WW, P = 1.39 × 10–18), and “regulation of transcription” (HW vs. WW, P = 1.56 × 10–4; HN vs. WW, P = 8.3 × 10–8). This implies that many genes up-regulated by individual H2O2 pretreatment alone or HN, or both, may be related to the enhanced salt tolerance of plants through their function in the abovementioned process. More interestingly, we found that the GO terms related to “cell cycle process” (P = 1.38 × 10–19) and “cell division” (P = 1.21 × 10–9) were specially enriched in HW vs. WW; therefore, we speculate that the up-regulation of these genes may affect plant growth to cope with the subsequent stresses.
To assess the major transcriptional dynamics associated with the responses to H2O2 pretreatment and/or both H2O2 and NaCl, we further clustered these DEGs from HW vs. WW and HN vs. WW into nine groups according to their expression trends under the four different combinations of treatments (Figures 2F,G). Of these clusters, we mainly focus on five clusters on the basis of their functional annotations and the expression profiles which were up-regulated either in HW vs. WW, HN vs. WW, or both. The K1 group clusters those genes which may be primed by H2O2 pretreatment and have up-regulated expression with subsequent salt stress. These genes are enriched in response to abiotic stimuli, illustrating their positive regulatory roles in increased plant salt tolerance. The genes in K2 were significantly up-regulated in HN vs. WW without obviously different expression in HW vs. WW and, so, they are enriched in oxidation–reduction processes, response to abiotic stimuli, and cell wall organization or biogenesis, which may mean that the expression of these genes is initiated during H2O2 pretreatment and mainly functions in subsequent salt stress. The K5 group comprises genes which mitigated the degree of up-regulation due to H2O2 pretreatment followed by NaCl, as compared with NaCl alone, which is characterized by an abundance of genes related to stress responses, especially osmotic stress and ABA, response to chemicals, and response to abiotic stimuli. The K7 group clusters those genes that were successively up-regulated by individual H2O2 and H2O2 plus NaCl, but the magnitude of the increase in the latter was less than in NaCl alone. Based on K5 and K7, we speculate that pretreatment with H2O2 can alleviate the oscillation of plant intracellular environment caused by subsequent NaCl exposure. The K8 group includes genes which were mainly up-regulated by H2O2 pretreatment, while only a few of these were up-regulated by combined H2O2 and NaCl treatment. Moreover, these genes are concentrated in cell cycle processes and cell division, implicating that after H2O2-induced expression, these genes may promote cell proliferation, resulting in plant growth under subsequent high-salt stress.
To identify the metabolic pathways in which the DEGs were involved and enriched, KEGG analysis was also performed. The results revealed that in HW vs. WW, 993 up-regulated genes were enriched in six pathways, including ribosome biogenesis in eukaryotes (ath03008, P = 3.46 × 10–10), DNA replication (ath03030, P = 0.00011), flavonoid biosynthesis (ath00941, P = 0.00030), homologous recombination (ath03440, P = 0.00082), cutin, suberine and wax biosynthesis (ath00073, P = 0.00275), and mismatch repair (ath03430, P = 0.00019); 500 down-regulated genes were assigned to plant hormone signal transduction (ath04075, P = 0.01571) and alpha-linolenic acid metabolism (ath00592, P = 0.000588). Likewise, in HN vs. WW, 780 up-regulated genes were enriched in starch and sucrose metabolism (ath00500, P = 0.00496), glucosinolate biosynthesis (ath00966, P = 0.00496), and cutin, suberine and wax biosynthesis (ath00073, P = 0.02513); while 986 down-regulated genes mainly participated in plant hormone signal transduction pathway (ath04075, P = 2.68 × 10–18) (Figure 3).
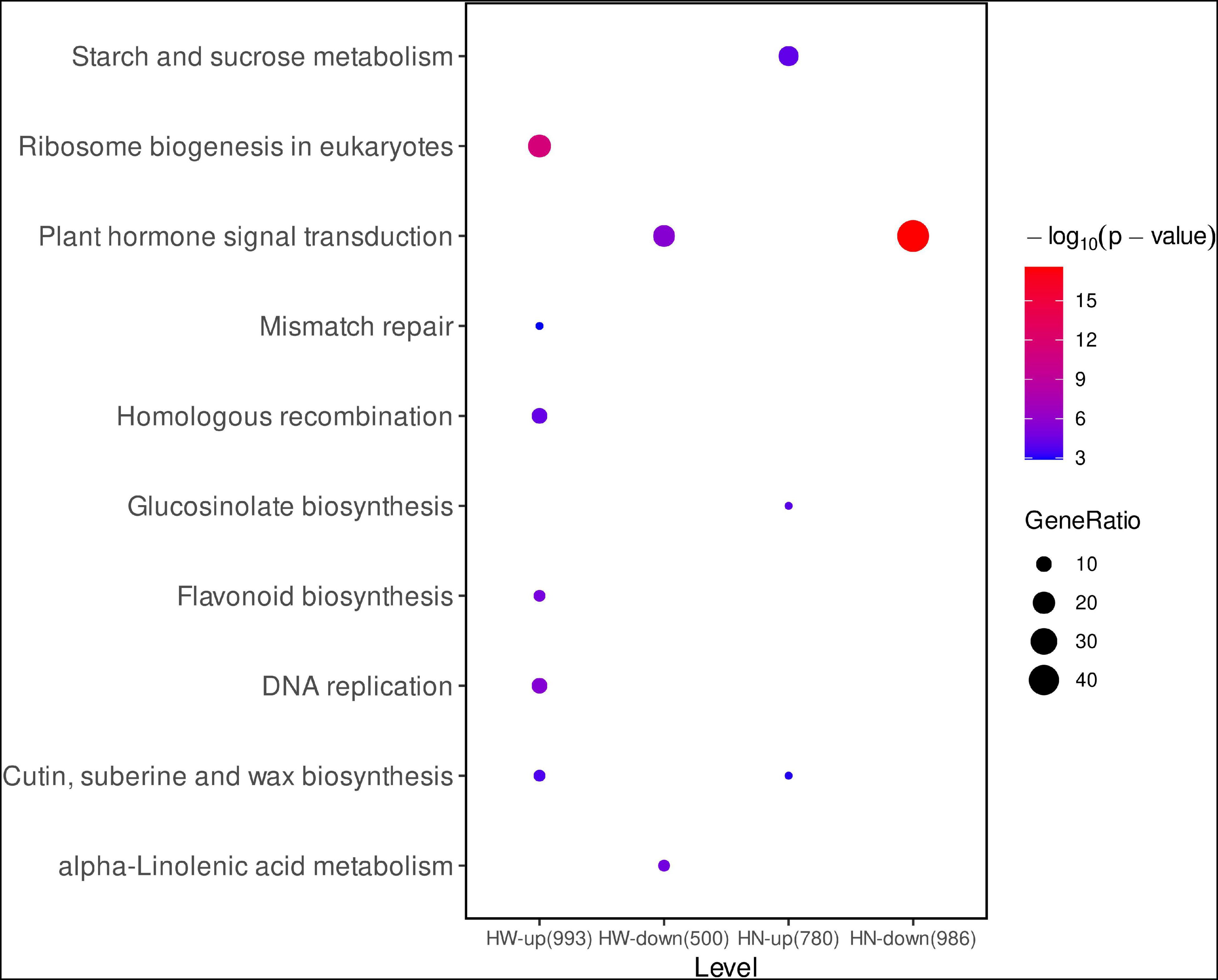
Figure 3. The kyoto encyclopedia of genes and genomes (KEGG) pathway of DEGs. The pathway names are provided on the vertical axis. The color of the dot represents p value and the size of dot represents gene ratio in each functional category. The rich level in the horizontal axis is the size of the point, which represents the number of DEGs, and the color of the dot represents the q value. HW-up (993), 993 up-regulated DEGs in HW vs. WW; HW-down (500), 500 down-regulated DEGs in HW vs. WW; HN-up (780), 780 up-regulated DEGs in HN vs. WW; HN-down (980), 980 down-regulated DEGs in HN vs. WW.
H2O2-Pretreatment Activates Cell Cycle Process and Cell Division
Further mining the transcriptome data, we found that after low-concentration H2O2 pretreatment of seedlings, a large proportion of genes related to the cell cycle and cell division were up-regulated, and most were significantly induced only under HW vs. WW (Figure 4A, Table 1, and Supplementary Table 18). Even though the expression levels of a few genes were increased under HW vs. WW and HN vs. WW, the extent of increase in the former was higher than in the latter (Figure 4A, Tables 1, 2, and Supplementary Tables 18, 20). Among these are sixteen core cell cycle genes, including two A-type cyclins (CYCA1;1, CYCA2;4), seven B-type cyclins (CYCB1;1, CYCB1;2, CYCB1;3, CYCB1;4, CYCB2;2, CYCB2;3, CYCB2;4), two plant-specific B-type CDKs (CDKB1;2; CDKB2;1) and upstream regulator DEL1 and its target CDT1A, and minichromosome maintenance genes (MCM2, MCM3, MCM6). B-type CDKs are plant-specific and are divided into two subtypes: CDKB1 and CDKB2. CDKB1 is activated by A2-type and all B-type cyclins and functions in the late S-to-M phase, while B2-type CDKs exclusively associate with B1-type cyclins and have transcript levels peaking late in the M phase (Van Leene et al., 2010). In our data, the increased transcript levels of both CDKB1;2, CDKB2;1 and their corresponding partners CYCB2;4, CYCB1;1 implied that the CDKB1;2/CYCB2;4 and CDKB2;1/CYCB1;1 complex may promote cell cycle progression through late S-to-M or M phases (Van Leene et al., 2010).
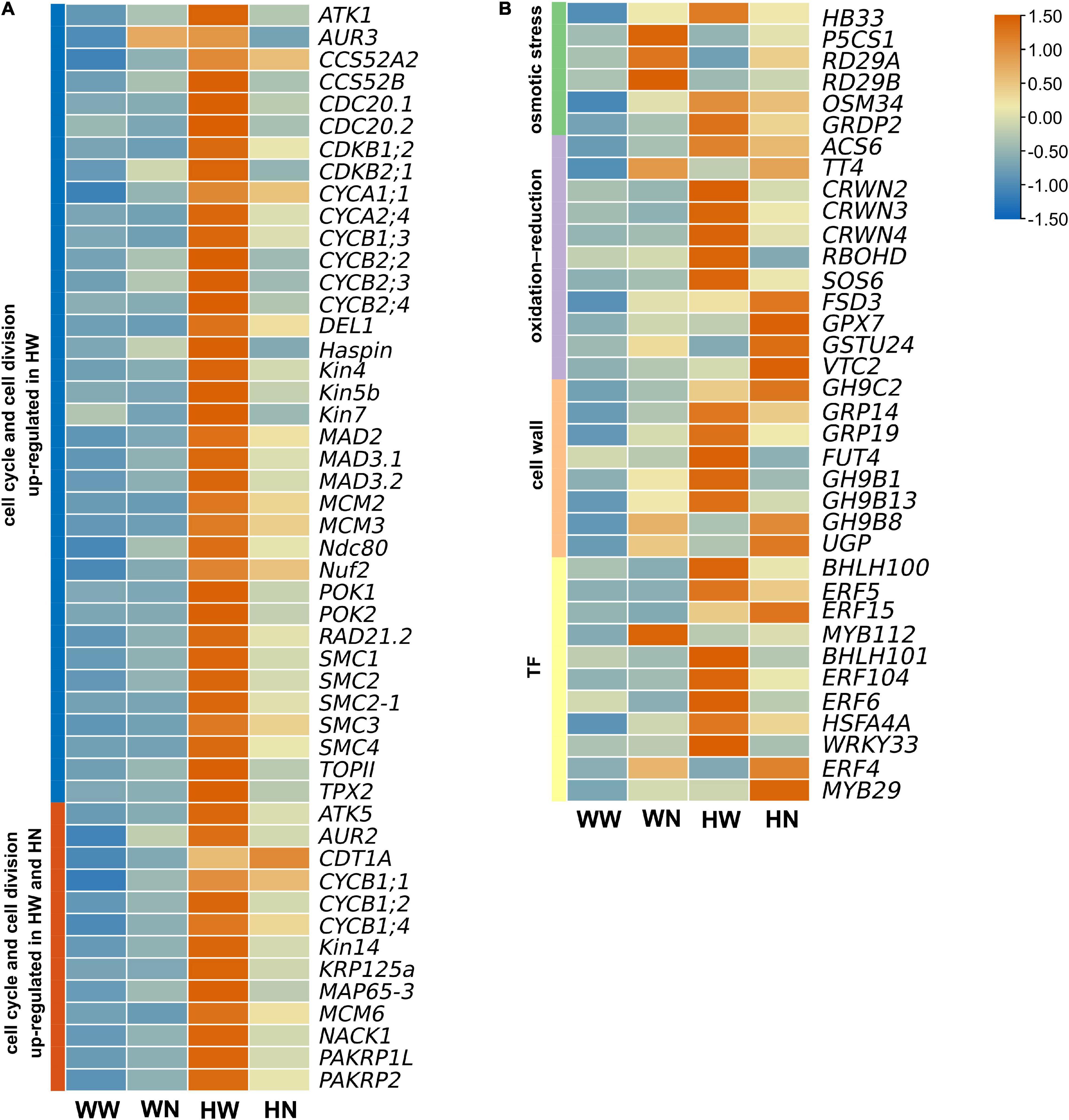
Figure 4. The expression patterns of selected differentially expressed genes (DEGs) represented as a heatmap. (A) Up-regulated DEGs related to the cell cycle and cell division only in HW vs. WW, or both in HW vs. WW and HN vs. WW. (B) Up-regulated DEGs related to salt stress only or both in HW vs. WW and HN vs. WW. Among osmotic stress responsive genes, HB33 was up-regulated in HW vs. WW; P5CS1, RD29A, and RD29B were up-regulated in HN vs. WW. BGLU6 and GRDP2 were significantly up-regulated in HW vs. WW and HN vs. WW; Of DEGs involved in oxidation–reduction processes, ACS6 and TT4 were significantly up-regulated in HW vs. WW and HN vs. WW; CRWN2, CRWN3, CRWN4, RBOHD, and SOS6 were up-regulated in HW vs. WW; FSD3, GSTU24, and VTC2 were up-regulated in HN vs. WW; Among genes related to cell wall organization, GH9C3 and GRP14 were significantly up-regulated in HW vs. WW and HN vs. WW; GRP19, FUT4, GH9B1, and GH9B13 were up-regulated in HW vs. WW; and GH9B8 and UGP1 were up-regulated in HN vs. WW; Of genes related to the transcription factors, BHLH100, ERF5, ERF15, and WRKY38 were significantly up-regulated in HW vs. WW and HN vs. WW; BHLH101, ERF104, ERF6, HSFA4A, and WRKY33 were up-regulated in HW vs. WW; and ERF4 and MYB29 were up-regulated in HN vs. WW. WW, pretreated with water and not salt-stressed; WN, pretreated with water and salt-stressed; HW, pretreated with H2O2 and not salt-stressed; HN, pretreated with H2O2 and salt-stressed. Heat map diagram of the log2FC, the red and blue colors specify up-and down-regulated expressions.
Besides CDKs and their cyclin partners, E2F transcription factors also belong to the core cell cycle machinery. Upon H2O2 pretreatment, the atypical E2F DP-E2F-like 1 (DEL1) was up-regulated, indicating that DEL1 may enhance cell proliferation by repressing the transcription of CCS52A2, which is required for endocycle onset (Lammens et al., 2008). DEL1 can also restrain the stress-induced switch from mitosis to the endocycle in dividing cells exposed to osmotic stress (Cookson et al., 2006). Contradictory with DEL1 inhibiting CCS52A2, CCS52A2 was also markedly up-regulated only under HW; this inconsistency may have been due to using transcriptomic data from whole shoots of Arabidopsis instead of defined cells. Besides CCS52A2, other anaphase-promoting complex/cyclosome (APC/C) coactivators such as CCS52B and CDC20 (CDC20.1, CDC20.2) were significantly elevated in transcripts under HW compared to WW, and this may be responsible for facilitating the switch from mitosis to endoreduplication through targeting of mitotic cyclins for destruction, thus inactivating cyclin-dependent kinase (CDK) (Kevei et al., 2011; Yang et al., 2017). CDKs/cyclins and their regulators DEL1, CCS52A2, CCS52B, CDC20.1, and CDC20.2 coordinate to balance cell proliferation and cell differentiation/expansion and, thus, balance plant growth and development.
Furthermore, H2O2 pretreatment also induced the expression of CDT1A and minichromosome maintenance genes (MCM2, MCM3, MCM6), where only CDT1A and MCM6 were differentially expressed under HW and HN, while only MCM2 and MCM3 were differentially expressed under HW (Figure 4A). CDT1A, as a DNA replication licensing factor, can recruit the MCM complex to form the components of the pre-replicative complex at the G1 phase (Nishitani et al., 2001). Therefore, high transcript levels of these genes facilitate activation of the replication origin, which can ensure that genomic DNA is replicated completely and accurately only once during the S phase in a single cell cycle (Tuteja et al., 2011). Meanwhile, according to previous research results, the high H2O2-induced expression of MCM6 can presumably confer plant salt tolerance by preserving normal DNA replication under salinity stress conditions (Dang et al., 2011).
Except for the abovementioned cell cycle components, low levels of H2O2 also increased transcription of a large number of genes encoding spindle assembly factors. These include genes for mitosis kinases (AUR2, AUR3, and AtHaspin); microtubule-associated proteins (MAPs), including TPX2, MAP65-3, and members of the kinesin superfamily (Kin4/chromokinesin, Kin5, Kin7, Kin12 and Kin14 families); chromosome organization proteins (SMC1, SMC2, SMC3, SMC4, RAD21.2, and TOPII); kinetochore complex (Ndc80 and Nuf2); and spindle assembly checkpoint complex (Mad2, Mad3.1, and Mad3.2). Among these, AtHaspin can activate AUR3 and promote its centromeric localization on chromosomes by phosphorylating histone H3 at Thr3. Then, AtHaspin and AUR3 together regulate proper chromosome alignment in the spindle during prometaphase/metaphase and chromosome segregation (Kozgunova et al., 2016). In this process, the cohesin complex, containing SMC1, SMC3, and RAD21, can contribute to chromosome alignment, while TOPII can release these cohesins from chromosomes to allow for chromosome segregation (Higgins, 2010; Kamenz and Hauf, 2017). In addition, the condensin complex, comprising SMC2 and SMC4, also ensures chromosome condensation and proper segregation (Wang H. et al., 2019). Ndc80 and Nuf2, as components of the kinetochore complex, are localized at the outer kinetochore, connecting spindle fibers to the kinetochore as well as mediating chromosome segregation during cell division (Shin et al., 2018). In mitosis, the SAC core proteins Mad2 and Mad3.2 are recruited to the kinetochore that is unattached to the spindle; then, Mad2 and Mad3.2 together with Mad3.1 may bind CDC20 to form the mitotic checkpoint complex (MCC) to inhibit the activity of APC/C (Komaki and Schnittger, 2017). Until the kinetochore is correctly attached to the spindle, CDC20 is released, which then activates APC/C for the removal of cohesin, thus promoting entry into anaphase (Singh et al., 2014). Moreover, AUR3 is present at kinetochores and is involved in kinetochore assembly during mitosis (Lermontova et al., 2015). Therefore, during exposure to H2O2 or combined H2O2 and NaCl treatment, all these up-regulated genes may coordinate to control proper condensation and segregation of chromosomes for successful cell division.
AUR2 is another member of the Arabidopsis Aurora kinase family, which is associated with spindle assembly, phragmoplast organization, and cell plate orientation during mitotic division (Demidov et al., 2014). In this process, AUR2 activity may be controlled by its upstream regulators AtHaspin (Kozgunova et al., 2016) and TPX2 (Petrovska et al., 2012). TPX2 is a MAP with multiple functions in microtubule organization, and can activate and phosphorylate AUR2. The TPX2–AUR2 complex can colocalize on spindle microtubules during mitosis and thereby control cell division (Petrovska et al., 2012).
Besides TPX2, many other MAPs regulate microtubule dynamics for the proper formation of different MT arrays during the cell cycle. AtMAP65-3 begins to accumulate at the narrow midline of the spindle at metaphase and is involved in antiparallel MT bundling at the phragmoplast midline at telophase. Similar to AtMAP65-3, kinesin-5 interdigitates microtubules at both spindle and phragmoplast midline (Bannigan et al., 2007). The kinesin-7 family member NACK1 participates in phragmoplast organization by recruiting MAPKKK (ANP) to the phragmoplast midline and activating the MAP kinase cascade during the late mitosis phase, which is critical for cell plate formation (Sasabe et al., 2015). The kinesin-12 family members POK1 and POK2 are important for PPB function (Rasmussen et al., 2011), and PAKRP1 and PAKRP1L are involved in MT interdigitation at the phragmoplast midline (Lee et al., 2007). ATK1 and ATK5 are two minus-end-directed kinesin-14s which are essential in spindle assembly and function (Ambrose and Cyr, 2007). Therefore, the H2O2-induced expression of all these MAP genes contributes to the assembly of microtubule arrays and the progression of cell division.
The abovementioned cell cycle genes are associated with mitotic cell cycle, chromatin dynamics, and microtubule-related processes in promoting cell proliferation and maintaining the normal structure of chromosomes. As cell proliferation and cell expansion are the main driving forces in leaf growth, the up-regulation of these genes may maintain plant growth in cope with subsequent stresses; however, the mechanisms by which low levels of H2O2 promote plant cell cycle progression and growth remain unclear.
Differentially Expressed Genes Associated With Osmotic Stress
Plants first adopt a series of molecular mechanisms in response to osmotic stress when exposed to high salinity, such as regulating the expression of many genes involved in stomatal closure and synthesizing osmotically protective substances (Feng et al., 2016).
In HW vs. WW, some of the identified DEGs were osmotic stress-responsive (Table 1 and Supplementary Tables 18, 19), but relatively few of these types of genes were up-regulated. Of these, HB33 was induced mainly by H2O2, while the transcript increases under HN and WN were not distinctly different from WW (Figure 4B). Some studies have shown that HB33 is a positive regulator in ABA, mediating plant growth and development as well as response to different abiotic stresses, such as osmotic stress (Wang et al., 2011). In HN vs. WW, more osmotic stress-responsive DEGs were up-regulated (Table 2 and Supplementary Tables 20, 21). RD29A, RD29B, and P5CS1 are typical representatives, but the magnitude of their expression increases were less than in WN vs. WW (Figure 4B). We speculate that pretreatment with H2O2 perhaps mitigates the osmotic stress caused by subsequent salt stress. RD29A and RD29B are osmotic stress-related marker genes, and their encoding proteins RD29A and RD29B act as protective molecules in response to osmotic stress. P5CS1 encodes a key enzyme in proline biosynthesis and promotes proline accumulation to confer plant osmotic stress resistance (Feng et al., 2016). Comparing the DEGs in HW vs. WW with those in HN vs. WW, we found that OSM34 and GRDP2 were significantly up-regulated under both conditions (Tables 1, 2); however, their expression patterns were different. The expression level of the former in HN was higher than that in HW, while for the latter, the converse was observed (Figure 4B). Osmotin34 (OSM34) encodes osmotin to combat osmotic stress (Sharma et al., 2013), and AtGRDP2 encodes a short glycine-rich domain protein which may improve the growth of plants under osmotic stress (Ortega-Amaro et al., 2014).
Differentially Expressed Genes Associated With Oxidation-Reduction Process
To investigate which genes or biological processes are involved in the H2O2-primed oxidative stress tolerance of plants, we performed GO analysis on all DEGs in HW vs. WW and HN vs. WW, and found that some DEGs which may protect plants from damage during subsequent salt stress that were activated by H2O2 (Supplementary Tables 18–21).
Comparing the DEGs in HW vs. WW with those in HN vs. WW, we found that ACS6 and TT4 were jointly up-regulated under both conditions (Tables 1, 2); however, their expression patterns were distinct, with ACS6 expression higher in HW than in HN, whereas TT4 expression increased progressively with HW and HN (Figure 4B). ACS6 is one of the most important genes in ethylene biosynthesis, controlling the level of ethylene. Datta et al. (2015) reported that ACS6 was significantly up-regulated during the glutathione–ethylene interaction in response to salt stress. Our transcriptomic data implies that H2O2 pretreatment may elevate ethylene production by ACS6 transcription increase to activate the ROS-detoxifying system in defending against subsequent salt stress. The expression pattern of ACS6 further confirmed that ethylene may participate in H2O2-primed redox balance reconstruction under salt stress. TT4 encodes chalcone synthase (CHS), a key enzyme involved in the biosynthesis of flavonoids. It has been reported that the up-regulation of TT4 led to an increase in anthocyanin synthesis. Anthocyanins can function as antioxidants, helping plants to scavenge ROS and maintaining redox homeostasis during salt stress. The DEGs only up-regulated in HW vs. WW, such as CRWN2, CRWN3, CRWN4, SOS6, and RBOHD, were all clustered to K8 (Figures 2F, 4B and Table 1). CRWNs constitute a small gene family containing only CRWN1–4 members, three of which were detected in our transcription data. CRWN proteins have been reported to maintain the size and morphology of the nucleus in order to promote the normal growth of plants (Wang et al., 2013). We speculate that H2O2 induces the expression of CRWN2, CRWN3, and CRWN4, which may be positive regulators of oxidative stress tolerance, inhibiting ROS production and DNA damage during subsequent salt stress (Wang Q. et al., 2019). RBOHD is a key member of the RBOHs family, where RBOH-mediated spatiotemporal control of ROS production is required for appropriate cell elongation. We consider that H2O2 pretreatment promoted moderate expression of RBOHD, thereby promoting the growth and development of plants and improving salt tolerance (Marino et al., 2012; Suzuki et al., 2012). SOS6 has an important role in osmotic stress tolerance and may be involved in the regulation of ROS levels under oxidative stress (Yang et al., 2016). The DEGs only in HN vs. WW include VTC2, FSD3, GPX7, and GSTUs, all of which were up-regulated (Figure 4B and Table 2). VTC2 encodes GDP-L-galactose phosphorylase, catalyzing the conversion of GDP-L-Gal into L-Gal, which is considered to be a committed step in ascorbate biosynthesis (Koffler et al., 2014). Ascorbate, as a relatively abundant small-molecule antioxidant in plants, can detoxify ROS throughout the cell. FeSOD is one of the three major classes of SOD. Overexpression of FSD3 results in great tolerance to oxidative stress through scavenging of ROS (Myouga et al., 2008). GPXs are important ROS scavengers due to their broad substrate specificity and high affinity for H2O2. The up-regulated expression of GPX7 has been shown to be important for maintenance of redox balance in the cell (Chang et al., 2009). Glutathione S-transferases (GSTs) protect plants from oxidative damage and enhance the antioxidant capacity of plants. We also found that several GSTUs were up-regulated, such as GSTU24.
Differentially Expressed Genes Associated With Cell Wall Organizations
The plant cell wall is the first defense against external environmental stresses. To check whether H2O2 pretreatment invoked the expression of genes encoding for cell wall components, we further analyzed the transcription data and found that, based on GO analysis, some genes could be primed by H2O2 pretreatment (Supplementary Tables 18, 19), whereas some genes were regulated by subsequent salt stress (Supplementary Tables 20, 21). These genes are involved in the regulation of the synthesis of various components of the cell wall, causing the cell wall to harden. The formation of a physical barrier protects plant cells from further dehydration and death under salt stress, thereby resisting salt stress.
The identical DEGs in HW vs. WW and HN vs. WW, such as AtGH9C2 and GRP14, were up-regulated (Figure 4B and Tables 1, 2). AtGH9C2 is a class C endo-1, 4-β-glucanase (cellulase). Some studies have found that such endoglucanases affect cell wall development by promoting cell wall crystallization processes (Glass et al., 2015). GRP14 is an important structural protein which is widely found in plant cell walls, and the expression of GRP14 helps in cell wall remodeling when plants are exposed to salt stress (Le Gall et al., 2015). The DEGs up-regulated only in HW vs. WW, such as AtGH9B1, AtGH9B13, GRP19, and FUT4, were clustered to K7 (Figures 2F, 4B and Table 1). AtGH9B1 and AtGH9B13 are both class B endoglucanases that play an important role in cell wall relaxation during cell growth and expansion (Tsabary et al., 2003; Urbanowicz et al., 2007). GRP19 is a glycine-rich protein with similar function to GRP14 (Le Gall et al., 2015). FUT4 is an arabinogalactan (AG)-specific fructosyltransferase (FUT), which is responsible for the fructosylation of proteins glycosylated with arabinogalactan (AGPs) in leaves, which maintains proper cell expansion and root growth under salt stress conditions (Tryfona et al., 2014).
The DEGs up-regulated only in HN vs. WW, such as AtGH9B8 and UGP, were the representatives of cluster K2 (Figures 2F, 4B and Table 2). Like AtGH9B1 and AtGH9B13, AtGH9B8 also belongs to class B endoglucanases and is involved in cell wall relaxation during cell growth and expansion (Mele et al., 2003; Urbanowicz et al., 2007; Tryfona et al., 2014). The up-regulated expression of UGP1 can promote the biosynthesis of the cell wall, maintaining plant growth under salt stress and, therefore, increasing the salt tolerance of plants, after H2O2 pretreatment.
Differentially Expressed Genes Associated With Transcription Factors
Following H2O2 pretreatment, some transcription factors were accumulated in plants to defend against subsequent high-salt stress. After GO analysis, we identified some transcription factors encoding genes in HW vs. WW (Supplementary Tables 18, 19) and HN vs. WW (Supplementary Tables 20, 21), respectively. The gene number in the former accounts for a large proportion of the DEGs, with significantly more than the latter. These transcription factors can be divided into five categories, belonging to the ERF, MYB, WRKY, HSFA, and bHLH families.
The identical DEGs up-regulated in HW vs. WW and HN vs. WW included MYB112, ERF5, ERF15, and bHLH100 (Tables 1, 2); however, the expression levels of bHLH100 and ERF5 were higher in HW than in HN, while the converse was the case for ERF15 and MYB112 (Figure 4B). MYB112 is a member of the R2R3 MYBs. The up-regulated expression of MYB112 promotes the accumulation of anthocyanins, which can respond to different abiotic stresses, including oxidative stress, osmotic stress, and high-salt stress (Lotkowska et al., 2015). The DEGs up-regulated only in HW vs. WW, including WRKY33, ERF6, ERF104, bHLH101, and HSFA4A, clustered to K8 (Figures 2F, 4B and Table 1). Some studies have shown that WRKY33, ERF6, and ERF104 can regulate the expression of salt-tolerant genes in different signaling pathways, and that the overexpression of these genes can increase salt tolerance in plants (Jiang and Deyholos, 2009; Vogel et al., 2014; Van den Broeck et al., 2017). bHLH101 can increase the oxidative stress tolerance of plants (Noshi et al., 2018). HSFA4A encodes a member of heat stress transcription factors (Hsfs), certain members of which have been shown to function as ROS-dependent redox sensors, controlling gene expression during oxidative stress. Although we do not know whether HSFA4A is such a redox sensor, there has been evidence showing that it plays key roles in a variety of stress signaling pathways, and its overexpression enhances a variety of stress tolerances, including salt stress, osmotic stress, oxidative stress, and heavy metal stress (Perez-Salamo et al., 2014; Lin et al., 2018).
The DEGs up-regulated only in HN vs. WW include ERF4 and MYB29 (Figure 4B and Table 2). ERF4 are important molecules in the signaling pathways of ethylene and jasmonic acid, regulating the expression of a large number of genes involved in many plant defense mechanisms. Overexpression of ERF4 has been shown to increase salt and drought stress tolerance in plants (Seo et al., 2010).
Confirmation of RNA-Seq Data by RT-qPCR
To further validate whether the expression of DEGs was induced by only H2O2 pretreatment or both H2O2 pretreatment and subsequent salt stress, we selected 15 genes involved in signal transduction (Figure 5A), response to osmotic stress (Figure 5B), response to oxidation–reduction process (Figure 5C), cell wall organization (Figure 5D), and transcription factors (Figure 5E) for RT-qPCR. It was verified that the expression trends of these genes tested by RT-qPCR were highly consistent with the transcriptome data; therefore, the conclusions obtained from the transcriptome analysis are reliable. Primer information is presented in Supplementary Table 1.
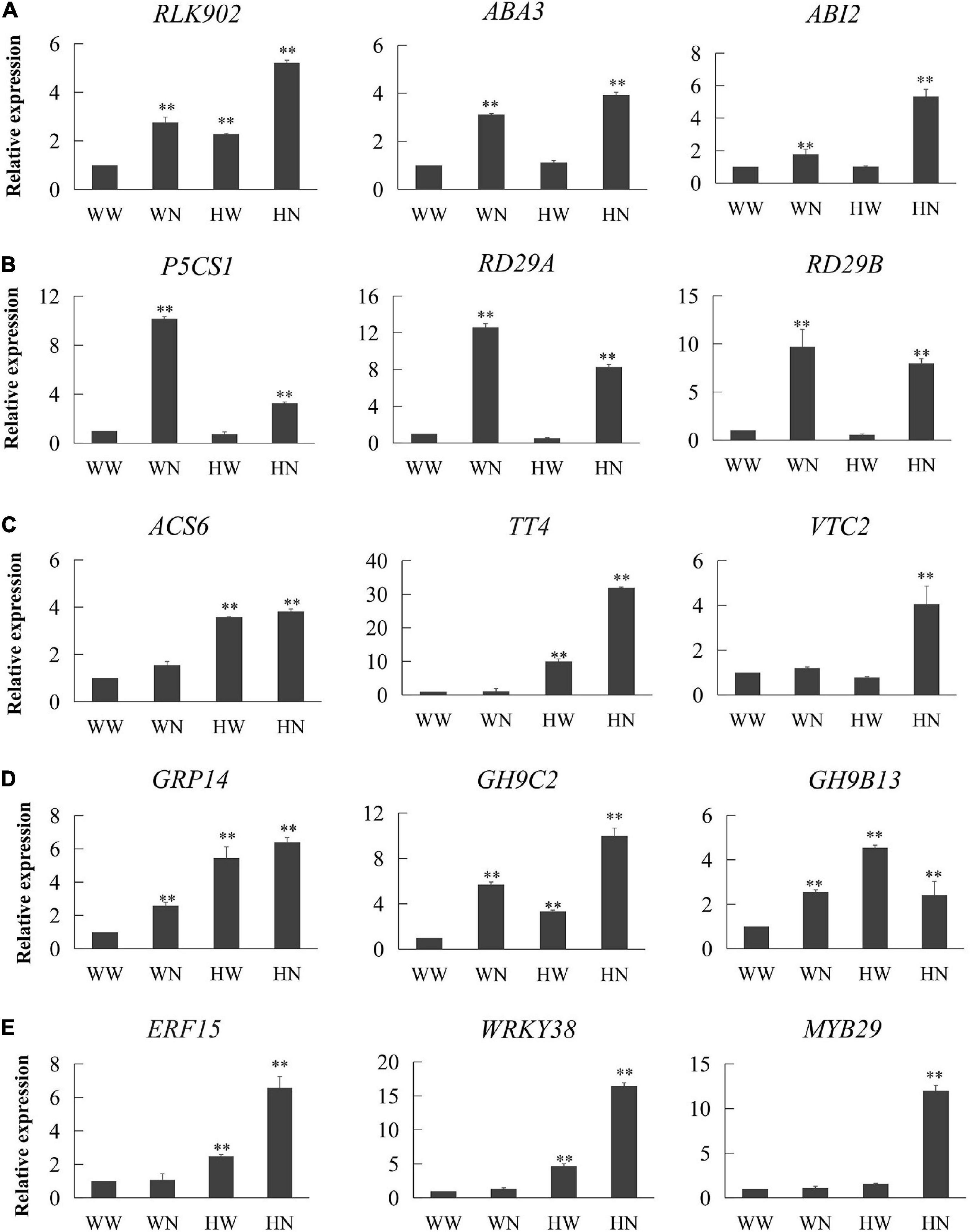
Figure 5. Confirmation of transcriptional changes by RT-qPCR. We selected 15 genes from the transcriptome to detect their expression trends: (A) genes involved in signal transduction, (B) genes involved in responding to osmotic stress, (C) genes involved in response to oxidation–reduction process, (D) genes involved in cell wall organization, and (E) genes related to transcription factors. The expression trends of these genes in the transcriptome are given in Tables 1, 2. RT-qPCR results are presented in the form of 2–△△CT. Three biological replicates per experiment. Data are represented as means ± SD. * and ** represented significantly and very significantly different at P < 0.05 and P < 0.01, respectively, estimated with one-way ANOVA following SPSS. WW, pretreated with water and not salt-stressed; WN, pretreated with water and salt-stressed; HW, pretreated with H2O2 and not salt-stressed; HN, pretreated with H2O2 and salt-stressed.
Influence of H2O2 Pretreatment on Antioxidative Enzyme Activities of Seedlings
Salinity-induced oxidative stress in plants is associated with ROS overproduction. Through transcriptome analysis, we found some genes involved in the scavenging of ROS, such as FSD3 and GPX7.
The total SOD, and GPX activities in leaves are shown in Figure 6. The results show that the SOD activities in the HN and WN groups were greatly higher than those in the HW and control (WW) groups, which exhibit consistency between SOD activity and FSD3 expression. Under stress treatment, SOD activity in the HN group increased greatly compared with that in the WN group (Figure 6A). GPX activities in the HN group were significantly higher than in the WN group and, similarly, markedly higher in the HW group than in the WW group (Figure 6B). These results for GPX activity coincided with GPX7 expression.
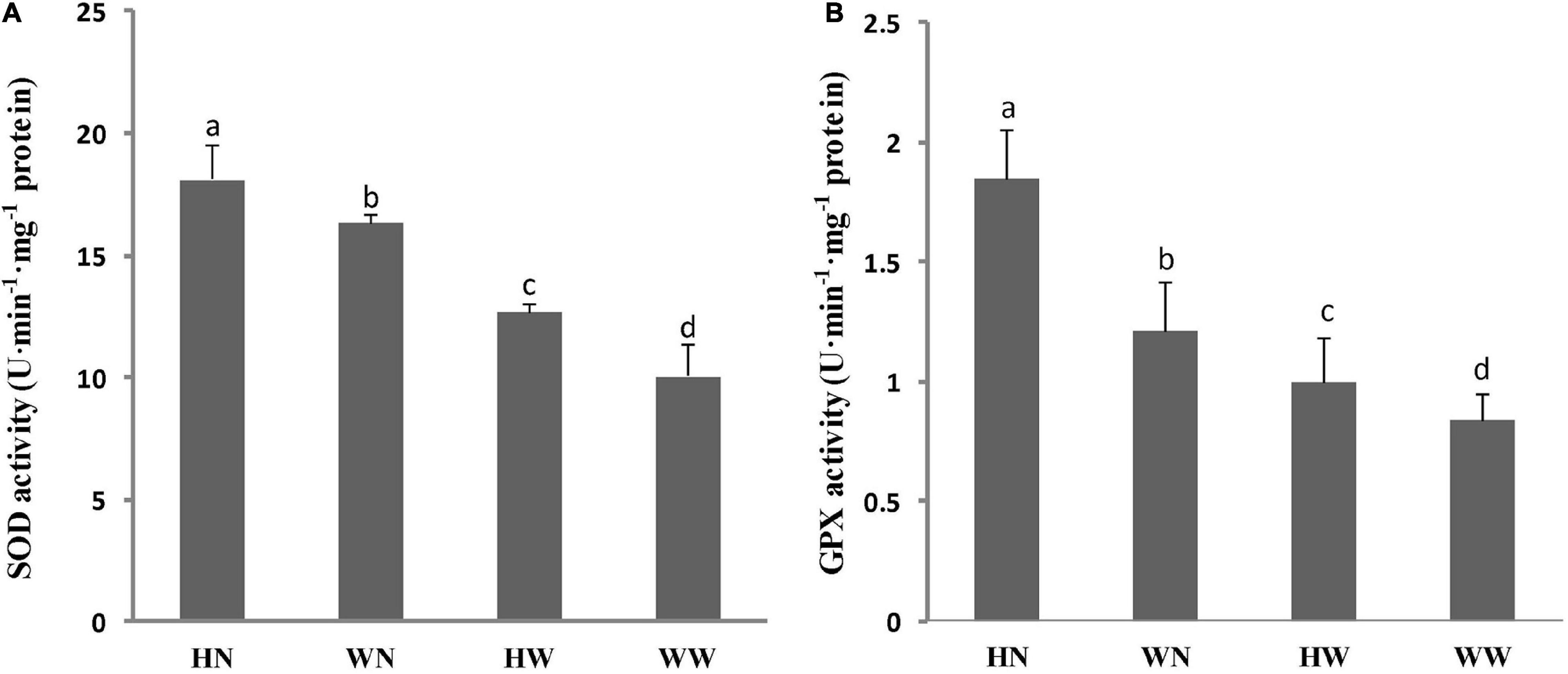
Figure 6. Influence of H2O2 pretreatment on SOD (A), and GPX (B) activities in Arabidopsis leaves under different treatments. Three biological replicates per experiment. Data are represented as means ± SD. Means for each treatment that do not share a common letter are significantly different at P < 0.05, estimated with one-way ANOVA following SPSS. WW, pretreated with water and not salt-stressed; WN, pretreated with water and salt-stressed; HW, pretreated with H2O2 and not salt-stressed; HN, pretreated with H2O2 and salt-stressed.
Discussion
Salt stress results in osmotic stress and oxidative stress, which limit plant growth and development and subsequently reduce crop yields. Plants can adapt to stressful environments through many physiological and molecular mechanisms, and plant resistance can be improved by many methods.
Seedling treatment with inorganic and organic agents greatly reduces the detrimental effects of stress and enhances essential nutrient content (Qiu et al., 2014; Cantabella et al., 2017; Ghassemi-Golezani and Farhangi-Abriz, 2018; Silva et al., 2020; Zahedi et al., 2021). In our present work, the pretreatment of seedlings with H2O2 increased the fresh and dry weights of salinity-treated seedlings.
H2O2 pretreatment helped seedlings to reduce the accumulation of Na+ and improve the K+ content and K+/Na+ ratio. Increased tissue K+ content and K+/Na+ ratio are important for retaining metabolic activities and, therefore, have been taken as valid physiological criteria for salt tolerance.
The stability of biological membranes has also been used as a screening tool to assess salt stress effects (Farooq and Azam, 2006). Seedling pretreatment with H2O2 reduced RMP in this study, although the change was not much different between the HW group and control. Lipid peroxidation of the plasma membrane is an important indicator of oxidative membrane damage induced by salt (Guo et al., 2017; Ma et al., 2017). Lipid peroxidation will eventually engender MDA, which can cause serious damage to cells. MDA has strong crosslinking properties and can bind with phosphatidyl ethanolamine, nucleic acid, and some amino acids, thereby producing lipofuscin-like pigments. Previous studies have found that the accumulation of MDA exhibited a positive correlation with an increase in plasma membrane permeability. Our experimental data showed that under salt stress conditions, the MDA content in H2O2-pretreated Arabidopsis seedlings was obviously lower than that in water-pretreated seedlings, indicating that H2O2 pretreatment can effectively alleviate the salt stress damage to the integrity and stability of the plant cell membrane. Therefore, the protective role of H2O2 involves improved tolerance of Arabidopsis seedlings to salt stress and maintenance of their growth during salt stress (De Azevedo Neto et al., 2005; Fedina et al., 2009; Wang et al., 2010; Gondim et al., 2012). Our research not only contributes to a better understanding of stress tolerance mechanisms in plants but is also of considerable value in developing effective methods for crop protection against environmental stresses during agricultural practice (Wahid et al., 2007).
To clarify the mechanisms implied in the physiological changes, we subsequently performed transcriptomic work to mine the gene expression patterns under different treatments (WW, WN, HW, and HN). Interestingly, a large number of genes involved in cell cycle control were up-regulated only or mainly in HW, including the core cell cycle genes (CYCs, CDKB1;2, CDKB2;1, their upstream regulator DEL1 and its target CDT1A, as well as MCM2, MCM3, and MCM6) and spindle assembly factor-encoding genes (mitosis kinases, MAPs, kinesin superfamily, chromosome organization proteins, and kinetochore complex). These cell cycle genes can promote cell proliferation and maintain the normal structure of chromosomes. Due to cell proliferation and cell expansion being the main driving forces for plant growth, we speculate that the up-regulation of these genes may enable maintenance of plant growth in coping with the subsequent stresses, which has been exemplified in many studies; for example, CYCB1;1 and CYCB2;2 overexpression in rice plants led to the accelerated growth of plants (Lee et al., 2003) and the potential contribution of the overexpression of MCM6 to the normal progression of DNA replication under salinity stress conditions and, thus, conferring salt tolerance in transgenic tobacco (Dang et al., 2011). Therefore, these findings provide strong supports for our conclusion based on our data: that H2O2 pretreatment enhanced Arabidopsis plant growth and salt tolerance. However, the mechanisms by which low levels of H2O2 promote plant cell cycle progression and growth remain unclear.
In various types of animal cells, it is already well-accepted that low levels of H2O2 can accelerate cell proliferation, perhaps by controlling the redox-dependent expression of D- and B-type cyclins (mainly D1 and B1 cyclins) and, thus, promoting G0/G1-to-S or S-to-G2 and -M cell cycle phase transitions (Burch and Heintz, 2005). In plants, D-type cyclins are also important regulators of G0/G1-to-S cell cycle phase transition, and ROS together with auxin may also play a role in the cell cycle activation of differentiated leaf cells by CDKA1 activation and acceleration of cell cycle re-entry (G0-to-G1) (Feher et al., 2008). Yu et al. (2016) reported that 25 μM H2O2 treatment increased the rate of cell division in the quiescent center of wild-type Arabidopsis root. In our work, H2O2 pretreatment enhanced the expression of many genes encoding B- rather than D-type cyclins. As a result, we propose that H2O2 can also expedite Arabidopsis cell proliferation mainly through promoting the S-to-M cell cycle phase transition. These cell cycle genes might contribute to the good performance of Arabidopsis plants under salt stress after H2O2 pretreatment. However, this speculation needs more in-depth research for confirmation.
Once a low concentration of H2O2 is applied to the blade surface, it can act as a signaling molecule, being sensed and delivered by certain proteins, including HSFA4A of the HSFA family (Miller and Mittler, 2006). The communication between cells and the extracellular environment is largely controlled by RLKs in plants (He and Wu, 2016). Our transcription data show that HSFA4A and RLK902 were significantly up-regulated in HW, which implies that HSFA4A and RLK902 may act as H2O2 sensors and transmit the H2O2 signal to activate transcription factors, including those in the WRKY, ERF, MYB, HSFA, and bHLH families. Then, these transcription factors regulate a series of downstream stress-responsive genes, ultimately improving plant growth and salt tolerance (Miller and Mittler, 2006; He and Wu, 2016).
As a key signaling molecule, H2O2 also connects the signaling pathways of multiple phytohormones; this connection was first found between H2O2 and ethylene. Besides ethylene, other key phytohormones such as abscisic acid, jasmonates (JAs), ethylene and salicylic acid are also closely related to H2O2. All of these phytohormones employ H2O2 in their signaling cascades, either upstream or downstream, to orchestrate plant growth, development, and stress responses (Saxena et al., 2016). In our work, we detected several up-regulated genes involved in hormone synthesis or related signaling pathways. Among them, ABA1 and ACS6 play a role in the first steps of ABA and ET biosynthesis, whereas ABA3, ABI2, ERF1, ERF4, ERF6, ERF106, MYB51, WRKY70, and VSP1 are involved in ABA, ET, or JA signal transduction. In addition, RBOHD, an important member of the RBOH family, was induced by H2O2 pretreatment. RBOHs have been recognized as important targets in the response of phytohormones and H2O2 to various environmental cues (Yao et al., 2017). Recently, H2O2 generated by RBOHs was found to be essential for the maintenance of acquired thermotolerance during recovery after acclimation (Sun et al., 2018). Accordingly, plants primed with H2O2 or with a higher basal level of H2O2 formation will exhibit enhanced resistance to stressors (Ellouzi et al., 2017).
Base on KEGG pathway analysis, more down-regulated genes were enriched in phytohormone signal transduction. Of them, many JA biosynthesis related genes, such as LOXs (LOX3 and LOX4), AOCs (AOC1 and AOC3), OPR3, ACX1; JA metabolism conversion related genes, including ILL6, JAOs (JAO2, JAO3, JAO4), CYP94B1, CYP94B3, ST2A, JMT; and JA signal transduction involved genes, like JAZs (JAZ2, JAZ3, JAZ5, JAZ7, JAZ8, JAZ9, JAZ10, JAZ13), were detected only or both in HW vs. WW and in HN vs. WW (Supplementary Figure 1 and Supplementary Tables 22, 23). These results implied that H2O2 pretreatment may decrease the levels of JA and its derivatives, but the JA signaling was still induced due to the decreased expression of many JAZs transcriptional repressors. Therefore, JA signaling plays an important role in the response of H2O2-pretreated Arabidopsis plants to subsequent salt stress. However, the action mechanisms of JA in plant salt stress tolerance remains largely elusive. Previous studies on different plants have given controversial conclusions (Qiu et al., 2014; Song et al., 2021), which means the roles of JA in plant salt stress tolerance are sophisticated. In this process, the combined action of JA with other plant hormones, such as ABA, ethylene, auxin, and salicylic acid, plus the regulation of hormonal homeostasis may jointly contribute to plant growth under salt stress (Raza et al., 2021; Zahedi et al., 2021; Zhao et al., 2021; Zhu et al., 2021).
Besides the abovementioned genes, many DEGs involved in the redox balance were induced separately in HW, HN, or in both conditions. These genes include TT4, CRWN2, CRWN3, CRWN4, ACS6, SOS6, RBOHD, VTC2, FSD3, GPX7, and GSTU24, which are able to scavenge ROS to maintain the redox balance; moreover, their up-regulated expression enhances oxidative stress tolerance in plants. Then, we examined the enzyme activity of the antioxidative enzymes responsible for the scavenging of ROS. SOD is an important protective enzyme in the enzymatic defense system, which can eliminate superoxide radicals in the cell through a dismutation reaction, generating H2O2 and O2 (Noctor and Foyer, 1998). Our experiments showed that SOD activity increased in the leaves of both HN and HW seedlings, suggesting that H2O2 pretreatment might enhance the superoxide radical scavenging ability of plants. It has been shown that salt tolerance is directly related to an increase in SOD activity (Hernandez et al., 2000). It is also noteworthy that the enhancement of SOD activity in HN plant leaves was accompanied by increases in GPX activity. These results indicate that under stressed conditions, the ROS scavenging mechanism was more effective in acclimated than in unacclimated plants. Thus, our results suggest that SOD and GPX may play central protective roles in the O2– and H2O2 scavenging processes (Badawi et al., 2004; Gill and Tuteja, 2010), and that the active involvement of these enzymes is related, at least in part, to salt-induced oxidative stress tolerance in plants. In addition, we found that among the differentially up-regulated genes, the osmotic stress-responsive genes were mainly concentrated under HN conditions, while relatively few were associated with HW. RD29A and RD29B encode hydrophilic proteins which act as protective molecules in response to osmotic stress, and P5CS1 encodes a key enzyme in proline biosynthesis to promote the accumulation of proline (Feng et al., 2016).
Besides keeping osmotic balance, ion homeostasis maintenance is also an important mechanism for salinity tolerance in plants. From our RNA-Seq data, we also found some genes, such as NHX2, CAX3, and CIPK5 were upregulated under the condition of H2O2 pretreatment followed NaCl stress (Supplementary Table 23). NHX2, as a tonoplast-localized NHX isoform, contributes to both vacuolar pH and the uptake of K+ and Na+, therefore regulating intracellular ion homeostasis (Bassil et al., 2019). CAX3 is a vacuolar H+/Ca2+ antiporter, participating in vacuolar H+/Ca2+ transport during salt stress. Unlike CAX1, CAX3 expression is strongly induced by salt stress (Cheng et al., 2003) and therefore has a specific role in response to salt stress (Zhao et al., 2008). Ion homeostasis, especially K+ homeostasis, is critical for metabolism, cell expansion, plant growth and plant stress acclimation. In this work, many up-regulated DEGs in HN are enriched in starch and sucrose metabolism, may partly benefiting from this K+ homeostasis. Through these pathways plants can improve source/sink of carbon, and therefore maintain their growth and development under salt stress.
The plant cell wall is mainly composed of cellulose, hemicellulose, and pectin. In addition, it contains enzymes and structural proteins. Plant cell walls are essential for the normal growth and development of plants, having many functions such as determining cell shape, maintaining normal water balance and expansion pressure, regulating the spread of macromolecules, and resisting a variety of abiotic stresses. Under high-salt stress, the cell wall is destroyed and the cells lose water and die which, in turn, affects the normal growth and development of plants. Therefore, cell wall integrity is critical for plants growth and stress response (Zhao et al., 2021). Similar to our previous research results, a low level of H2O2 pretreatment and subsequent high salinity, individually or jointly, induced the expression of cell wall remodeling genes. AtGH9B1, AtGH9B8, and AtGH9B13 are class B endoglucanases, and AtGH9C2 is a class C endoglucanase, all of which regulate the synthesis of cellulose. FUT4 is responsible for the fucosylation of AGPs in leaves, and its up-regulation has been shown to thicken plant cell walls and enhance plant salt tolerance (Tryfona et al., 2014).
Based on our work, we suppose that H2O2 pretreatment activates multiple stress-responsive signal pathways which are integrated into a signal network that initiates the expression of many genes encoding transcription factors and protein kinases, hence transitioning plants into a primed state for combatting future salt stress (Hossain et al., 2015).
Conclusion
To summarize, under salt stress conditions, H2O2-pretreated plants displayed high-salt tolerance when compared to non-pretreated plants, as manifested in higher plant biomass, increased K+/Na+ ratio, declined MDA content and RMP decline, and elevated activities of antioxidative enzymes (including SOD, and GPX). These results were integrated with transcription data, and we propose a working model for H2O2 pretreatment-induced salt tolerance improvement of Arabidopsis plants. In brief, exogenous H2O2 may be perceived by certain sensors which transmit the H2O2 signal to transcription factors that, in turn, regulate the expression of downstream genes, thereby accelerating cell cycle progression and cell proliferation, enhancing osmotic stress tolerance, maintaining the redox balance, and remodeling the cell walls in plants under subsequent high-salt exposure. In accordance, pretreatment with H2O2 at an appropriate concentration can improve the growth and salt tolerance of Arabidopsis seedlings (Figure 7).
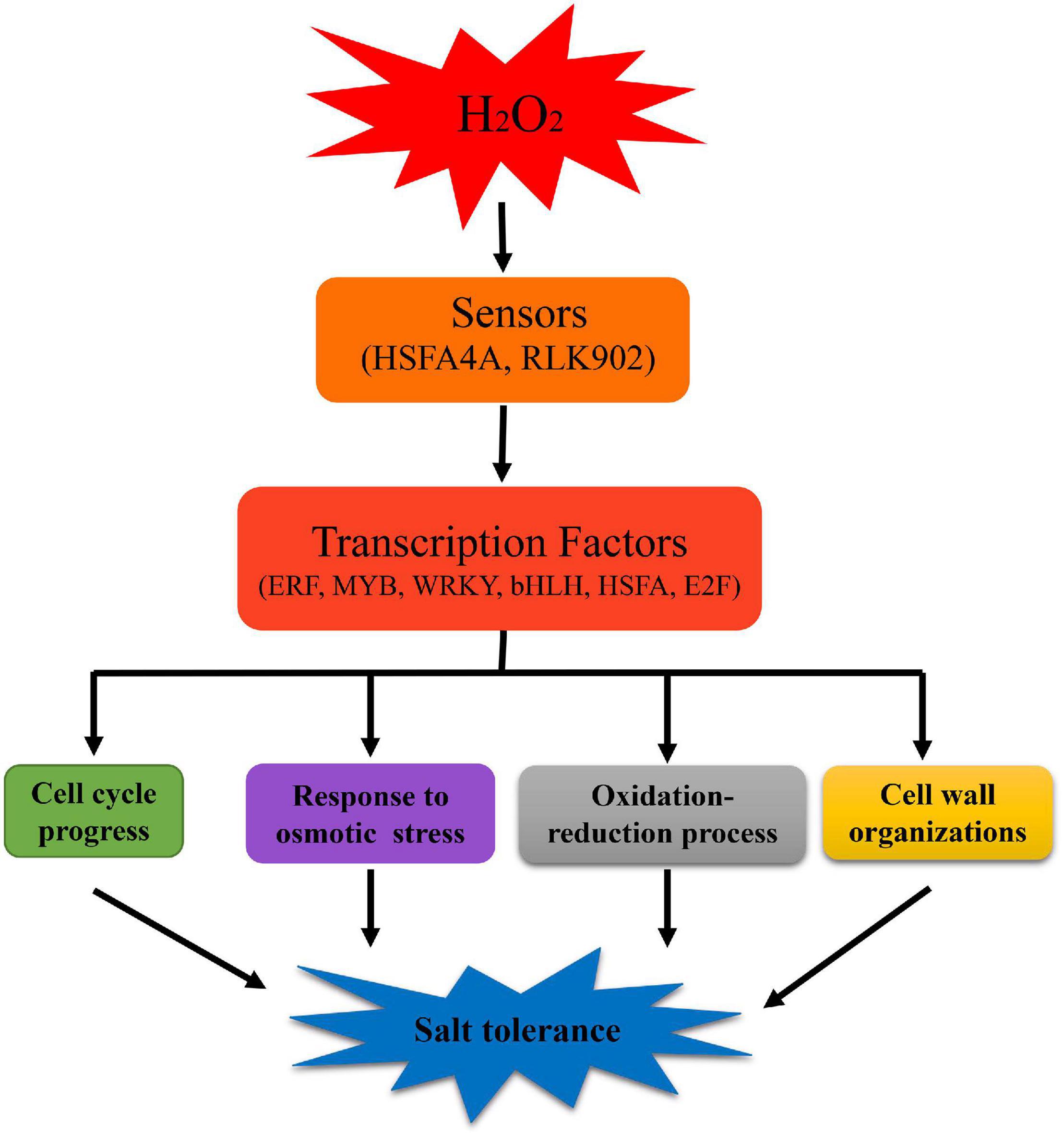
Figure 7. A working model of the mechanism underlying H2O2 pretreatment to improve salt tolerance in Arabidopsis. After H2O2 pretreatment, plants respond to salt stress via complex signal transduction pathways. We speculate that HSFA4A and RLK902 act as sensors in the H2O2 pretreatment process, transmitting the H2O2 signal to activate transcription factors, including those in WRKY, ERF, MYB, HSFA, bHLH, and E2F families. These transcription factors activate a series of genes with responses in cell cycle progress, osmotic stress, redox processes, and cell wall tissue, ultimately improving plant salt tolerance.
Data Availability Statement
The datasets presented in this study can be found in online repositories. The names of the repository/repositories and accession number(s) can be found in the article/Supplementary Material.
Author Contributions
QZ performed transcriptome analysis and wrote the manuscript. XD performed transcriptome analysis and made all heatmaps. HW and FW performed qRT-PCR experiments and modified the manuscript. DT performed plant material cultivation and RNA extraction. CJ and XZ measured physiological data. CM and HZ provided critical discussion. PL, YZ, and ZW proposed the idea of the manuscript, supervised the whole work, and wrote the final draft of the manuscript. All authors have read and agreed to the published version of the manuscript.
Funding
This work was supported in part by the National Natural Science Foundation of China (Grant No. 31970302), the Natural Science Foundation of Shandong Province, China (Grant No. ZR2017MC035), and by Science and Technology Development Plan of Shandong Province, China (Grant No. 2012GGB01136).
Conflict of Interest
The authors declare that the research was conducted in the absence of any commercial or financial relationships that could be construed as a potential conflict of interest.
Publisher’s Note
All claims expressed in this article are solely those of the authors and do not necessarily represent those of their affiliated organizations, or those of the publisher, the editors and the reviewers. Any product that may be evaluated in this article, or claim that may be made by its manufacturer, is not guaranteed or endorsed by the publisher.
Acknowledgments
The authors thank Annoroad Gene Technology Corporation (Beijing, China) for support with transcriptome sequencing.
Supplementary Material
The Supplementary Material for this article can be found online at: https://www.frontiersin.org/articles/10.3389/fpls.2022.866063/full#supplementary-material
Abbreviations
APX, ascorbate peroxidase; AsA, ascorbate; CAT, catalase; EDTA, ethylene diamine tetraacetic acid; ET, ethylene; GPX, glutathione peroxidase; GR, glutathione reductase; GSH, glutathione; H2O2, hydrogen peroxide; HW, pretreated with H2O2 and not salt-stressed; HN, pretreated with H2O2 and salt-stressed; MDA, malonaldehyde; NBT, nitro blue tetrazolium chloride; POD, peroxidase; RMP, relative membrane permeability; ROS, reactive oxygen species; SOD, superoxide dismutase; WW, pretreated with water and not salt-stressed; WN, pretreated with water and salt-stressed.
Footnotes
References
Ambrose, J. C., and Cyr, R. (2007). The kinesin ATK5 functions in early spindle assembly in Arabidopsis. Plant Cell 19, 226–236. doi: 10.1105/tpc.106.047613
Anjum, N. A., Sofo, A., Scopa, A., Roychoudhury, A., Gill, S. S., Iqbal, M., et al. (2015). Lipids and proteins–major targets of oxidative modifications in abiotic stressed plants. Environ. Sci. Pollut. Res. Int. 22, 4099–4121. doi: 10.1007/s11356-014-3917-1
Ashraf, M., and Foolad, M. R. (2005). Pre-sowing seed treatment - A shotgun approach to improve germination, plant growth, and crop yield under saline and non-saline conditions. Adv. Agron. 88, 223–271. doi: 10.1016/S0065-2113(05)88006-X
Badawi, G. H., Yamauchi, Y., Shimada, E., Sasaki, R., Kawano, N., Tanaka, K., et al. (2004). Enhanced tolerance to salt stress and water deficit by overexpressing superoxide dismutase in tobacco (Nicotiana tabacum) chloroplasts. Plant Sci. 166, 919–928. doi: 10.1111/j.0031-9317.2004.00308.x
Bannigan, A., Scheible, W. R., Lukowitz, W., Fagerstrom, C., Wadsworth, P., Somerville, C., et al. (2007). A conserved role for kinesin-5 in plant mitosis. J. Cell Sci. 120, 2819–2827. doi: 10.1242/jcs.009506
Bassil, E., Zhang, S., Gong, H., Tajima, H., and Blumwald, E. (2019). Cation specificity of vacuolar NHX-Type Cation/H+ Antiporters. Plant Physiol. 179, 616–629. doi: 10.1104/pp.18.01103
Beckers, G. J., Jaskiewicz, M., Liu, Y., Underwood, W. R., He, S., Zhang, S., et al. (2009). Mitogen-activated protein kinases 3 and 6 are required for full priming of stress responses in Arabidopsis thaliana. Plant Cell 21, 944–953. doi: 10.1105/tpc.108.062158
Bolger, A. M., Lohse, M., and Usadel, B. (2014). Trimmomatic: a flexible trimmer for Illumina sequence data. Bioinformatics 30, 2114–2120. doi: 10.1093/bioinformatics/btu170
Bowler, C., and Fluhr, R. (2000). The role of calcium and activated oxygens as signals for controlling cross-tolerance. Trends Plant Sci. 5, 241–246. doi: 10.1016/s1360-1385(00)01628-9
Bradford, M. M. (1976). A rapid and sensitive method for the quantitation of microgram quantities of protein utilizing the principle of protein-dye binding. Anal. Biochem. 72, 248–254. doi: 10.1006/abio.1976.9999
Burch, P. M., and Heintz, N. H. (2005). Redox regulation of cell-cycle re-entry: cyclin D1 as a primary target for the mitogenic effects of reactive oxygen and nitrogen species. Antioxid. Redox Sign. 7, 741–751. doi: 10.1089/ars.2005.7.741
Cantabella, D., Piqueras, A., Acosta-Motos, J. R., Bernal-Vicente, A., Hernandez, J. A., and Diaz-Vivancos, P. (2017). Salt-tolerance mechanisms induced in Stevia rebaudiana bertoni: effects on mineral nutrition, antioxidative metabolism and steviol glycoside content. Plant Physiol. Biochem. 115, 484–496. doi: 10.1016/j.plaphy.2017.04.023
Cerny, M., Habanova, H., Berka, M., Luklova, M., and Brzobohaty, B. (2018). Hydrogen peroxide: its role in plant biology and crosstalk with signalling networks. Int. J. Mol. Sci. 19:2812. doi: 10.3390/ijms19092812
Chang, C., Slesak, I., Jorda, L., Sotnikov, A., Melzer, M., Miszalski, Z., et al. (2009). Arabidopsis chloroplastic glutathione peroxidases play a role in cross talk between photooxidative stress and immune responses. Plant Physiol. 150, 670–683. doi: 10.1104/pp.109.135566
Cheng, N. H., Pittman, J. K., Barkla, B. J., Shigaki, T., and Hirschi, K. D. (2003). The Arabidopsis cax1 mutant exhibits impaired ion homeostasis, development, and hormonal responses and reveals interplay among vacuolar transporters. Plant Cell 15, 347–364. doi: 10.1105/tpc.007385
Cookson, S. J., Radziejwoski, A., and Granier, C. (2006). Cell and leaf size plasticity in Arabidopsis: what is the role of endoreduplication? Plant Cell Environ. 29, 1273–1283. doi: 10.1111/j.1365-3040.2006.01506.x
Cramer, G. R., Urano, K., Delrot, S., Pezzotti, M., and Shinozaki, K. (2011). Effects of abiotic stress on plants: a systems biology perspective. BMC Plant Biol. 11:163. doi: 10.1186/1471-2229-11-163
Dang, H., Tran, N. Q., Gill, S. S., Tuteja, R., and Tuteja, N. (2011). A single subunit MCM6 from pea promotes salinity stress tolerance without affecting yield. Plant Mol. Biol. 76, 19–34. doi: 10.1007/s11103-011-9758-0
Datta, R., Kumar, D., Sultana, A., Hazra, S., Bhattacharyya, D., and Chattopadhyay, S. (2015). Glutathione regulates 1-aminocyclopropane-1-carboxylate synthase transcription via WRKY33 and 1-aminocyclopropane-1-carboxylate oxidase by modulating messenger RNA stability to induce ethylene synthesis during Stress. Plant Physiol. 169, 2963–2981. doi: 10.1104/pp.15.01543
De Azevedo Neto, A. D., Prisco, J. T., Eneas-Filho, J., Medeiros, J. V., and Gomes-Filho, E. (2005). Hydrogen peroxide pre-treatment induces salt-stress acclimation in maize plants. J. Plant Physiol. 162, 1114–1122. doi: 10.1016/j.jplph.2005.01.007
Deinlein, U., Stephan, A. B., Horie, T., Luo, W., Xu, G., and Schroeder, J. I. (2014). Plant salt-tolerance mechanisms. Trends Plant Sci. 19, 371–379. doi: 10.1016/j.tplants.2014.02.001
Demidov, D., Lermontova, I., Weiss, O., Fuchs, J., and Rutten, T. (2014). Altered expression of aurora kinases in Arabidopsis results in aneu- and polyploidization. Plant J. 80, 449–461. doi: 10.1111/tpj.12647
Dhindsa, R. S. Plumb-Dhinds, P., and Thorpe, T. A. (1981). Leaf senescence: correlated with increased levels of membrane permeability and lipid peroxidation, and decreased levels of superoxide dismutase and catalase. J. Exp. Bot. 32, 93–101. doi: 10.1093/jxb/32.1.93
Drotar, A., Phelps, P., and Fall, R. (1985). Evidence for glutathione peroxidase activities in cultured plant cells. Plant Sci. 42, 35–40. doi: 10.1016/0168-9452(85)90025-1
Ellouzi, H., Sghayar, S., and Abdelly, C. (2017). H2O2 seed priming improves tolerance to salinity, drought and their combined effect more than mannitol in Cakile maritima when compared to Eutrema salsugineum. J. Plant Physiol. 210, 38–50. doi: 10.1016/j.jplph.2016.11.014
Farooq, S., and Azam, F. (2006). The use of cell membrane stability (CMS) technique to screen for salt tolerant wheat varieties. J. Plant Physiol. 163, 629–637. doi: 10.1016/j.jplph.2005.06.006
Fedina, I. S., Nedeva, D., and Cicek, N. (2009). Pre-treatment with H2O2 induces salt tolerance in Barley seedlings. Biol. Plant. 53, 321–324. doi: 10.1007/s10535-009-0058-3
Feher, A., Otvos, K., Pasternak, T. P., and Szandtner, A. P. (2008). The involvement of reactive oxygen species (ROS) in the cell cycle activation (G(0)-to-G(1) transition) of plant cells. Plant Signal. Behav. 3, 823–826. doi: 10.4161/psb.3.10.5908
Feng, X., Li, J., Qi, S., Lin, Q., Jin, J., and Hua, X. (2016). Light affects salt stress-induced transcriptional memory of P5CS1 in Arabidopsis. Proc. Natl. Acad. Sci. U. S. A. 113, E8335–E8343. doi: 10.1073/pnas.1610670114
Gechev, T., Gadjev, I., Van Breusegem, F., Inze, D., Dukiandjiev, S., Toneva, V., et al. (2002). Hydrogen peroxide protects tobacco from oxidative stress by inducing a set of antioxidant enzymes. Cell Mol. Life Sci. 59, 708–714. doi: 10.1007/s00018-002-8459-x
Ghassemi-Golezani, K., and Farhangi-Abriz, S. (2018). Foliar sprays of salicylic acid and jasmonic acid stimulate H+-ATPase activity of tonoplast, nutrient uptake and salt tolerance of soybean. Ecotoxicol. Environ. Saf. 166, 18–25. doi: 10.1016/j.ecoenv.2018.09.059
Giannopolitis, C. N., and Ries, S. K. (1977). Superoxide dismutases: I. Occurrence in higher plants. Plant Physiol. 59, 309–314. doi: 10.1104/pp.59.2.309
Gill, S. S., and Tuteja, N. (2010). Reactive oxygen species and antioxidant machinery in abiotic stress tolerance in crop plants. Plant Physiol. Biochem. 48, 909–930. doi: 10.1016/j.plaphy.2010.08.016
Glass, M., Barkwill, S., Unda, F., and Mansfield, S. D. (2015). Endo-beta-1, 4-glucanases impact plant cell wall development by influencing cellulose crystallization. J. Integr. Plant Biol. 57, 396–410. doi: 10.1111/jipb.12353
Gondim, F. A., Gomes-Filho, E., Costa, J. H., Mendes Alencar, N. L., and Prisco, J. T. (2012). Catalase plays a key role in salt stress acclimation induced by hydrogen peroxide pretreatment in maize. Plant Physiol. Biochem. 56, 62–71. doi: 10.1016/j.plaphy.2012.04.012
Gong, Z., Xiong, L., Shi, H., Yang, S., Herrera-Estrella, L. R., Xu, G., et al. (2020). Plant abiotic stress response and nutrient use efficiency. Sci. China Life Sci. 63, 635–674. doi: 10.1007/s11427-020-1683-x
Guo, Z., Yang, N., Zhu, C., and Gan, L. (2017). Exogenously applied poly-gamma-glutamic acid alleviates salt stress in wheat seedlings by modulating ion balance and the antioxidant system. Environ. Sci. Pollut. Res. Int. 24, 6592–6598. doi: 10.1007/s11356-016-8295-4
He, K., and Wu, Y. (2016). Receptor-like kinases and regulation of plant innate immunity. Enzymes 40, 105–142. doi: 10.1016/bs.enz.2016.09.003
He, L., Gao, Z., and Li, R. (2009). Pretreatment of seed with H2O2 enhances drought tolerance of wheat (Triticum aestivum L.) seedlings. Afr. J. Biotechnol. 8, 6151–6157. doi: 10.5897/AJB09.490
Heath, R. L., and Packer, L. (1968). Photoperoxidation in isolated chloroplasts. I. Kinetics and stoichiometry of fatty acid peroxidation. Arch. Biochem. Biophys. 125, 189–198. doi: 10.1016/0003-9861(68)90654-1
Hernandez, J. A., Jimenez, A., Mullineaux, P., and Sevilla, F. (2000). Tolerance of pea (Pisum sativum L.) to long-term salt stress is associated with induction of antioxidant defences. Plant Cell Environ. 23, 853–862. doi: 10.1046/j.1365-3040.2000.00602.x
Higgins, J. M. (2010). Haspin: a newly discovered regulator of mitotic chromosome behavior. Chromosoma 119, 137–147. doi: 10.1007/s00412-009-0250-4
Hossain, M. A., Bhattacharjee, S., Armin, S. M., Qian, P., Xin, W., Li, H., et al. (2015). Hydrogen peroxide priming modulates abiotic oxidative stress tolerance: insights from ROS detoxification and scavenging. Front. Plant Sci. 6:420. doi: 10.3389/fpls.2015.00420
Ishibashi, Y., Yamaguchi, H., Yuasa, T., Iwaya-Inoue, M., Arima, S., and Zheng, S. H. (2011). Hydrogen peroxide spraying alleviates drought stress in soybean plants. J. Plant Physiol. 168, 1562–1567. doi: 10.1016/j.jplph.2011.02.003
Jiang, Y., and Deyholos, M. K. (2009). Functional characterization of Arabidopsis NaCl-inducible WRKY25 and WRKY33 transcription factors in abiotic stresses. Plant Mol. Biol. 69, 91–105. doi: 10.1007/s11103-008-9408-3
Kamenz, J., and Hauf, S. (2017). Time to split up: dynamics of chromosome separation. Trends Cell Biol. 27, 42–54. doi: 10.1016/j.tcb.2016.07.008
Karpinski, S., Reynolds, H., Karpinska, B., Wingsle, G., Creissen, G., and Mullineaux, P. (1999). Systemic signaling and acclimation in response to excess excitation energy in Arabidopsis. Science 284, 654–657. doi: 10.1126/science.284.5414.654
Kevei, Z., Baloban, M., Da Ines, O., Tiricz, H., Kroll, A., Regulski, K., et al. (2011). Conserved CDC20 cell cycle functions are carried out by two of the five isoforms in Arabidopsis thaliana. PLoS One 6:e20618. doi: 10.1371/journal.pone.0020618
Klopfenstein, D. V., Zhang, L., Pedersen, B. S., Ramirez, F., Warwick Vesztrocy, A., Naldi, A., et al. (2018). GOATOOLS: a python library for gene ontology analyses. Sci. Rep. 8:10872. doi: 10.1038/s41598-018-28948-z
Koffler, B. E., Luschin-Ebengreuth, N., Stabentheiner, E., Muller, M., and Zechmann, B. (2014). Compartment specific response of antioxidants to drought stress in Arabidopsis. Plant Sci. 227, 133–144. doi: 10.1016/j.plantsci.2014.08.002
Komaki, S., and Schnittger, A. (2017). The spindle assembly checkpoint in Arabidopsis is rapidly shut off during severe stress. Dev. Cell 43, 172–185.e5. doi: 10.1016/j.devcel.2017.09.017
Kozgunova, E., Suzuki, T., Ito, M., Higashiyama, T., and Kurihara, D. (2016). Haspin has multiple functions in the plant cell division regulatory network. Plant Cell Physiol. 57, 848–861. doi: 10.1093/pcp/pcw030
Lammens, T., Boudolf, V., Kheibarshekan, L., Zalmas, L. P., Gaamouche, T., Maes, S., et al. (2008). Atypical E2F activity restrains APC/CCCS52A2 function obligatory for endocycle onset. Proc. Natl. Acad. Sci. U. S. A. 105, 14721–14726. doi: 10.1073/pnas.0806510105
Le Gall, H., Philippe, F., Domon, J. M., Gillet, F., Pelloux, J., and Rayon, C. (2015). Cell wall metabolism in response to abiotic stress. Plants 4, 112–166. doi: 10.3390/plants4010112
Lee, J., Das, A., Yamaguchi, M., Hashimoto, J., Tsutsumi, N., Uchimiya, H., et al. (2003). Cell cycle function of a rice B2-type cyclin interacting with a B-type cyclin-dependent kinase. Plant J. 34, 417–425. doi: 10.1046/j.1365-313x.2003.01736.x
Lee, Y. R., Li, Y., and Liu, B. (2007). Two Arabidopsis phragmoplast-associated kinesins play a critical role in cytokinesis during male gametogenesis. Plant Cell 19, 2595–2605. doi: 10.1105/tpc.107.050716
Lermontova, I., Sandmann, M., Mascher, M., Schmit, A. C., and Chaboute, M. E. (2015). Centromeric chromatin and its dynamics in plants. Plant J. 83, 4–17. doi: 10.1111/tpj.12875
Lin, K., Tsai, M. Y., Lu, C., Wu, S., and Yeh, C. H. (2018). The roles of Arabidopsis HSFA2, HSFA4a, and HSFA7a in the heat shock response and cytosolic protein response. Bot. Stud. 59:15. doi: 10.1186/s40529-018-0231-0
Lotkowska, M. E., Tohge, T., Fernie, A. R., Xue, G., Balazadeh, S., and Mueller-Roeber, B. (2015). The Arabidopsis transcription factor mYB112 promotes anthocyanin formation during salinity and under high light stress. Plant Physiol. 169, 1862–1880. doi: 10.1104/pp.15.00605
Love, M. I., Huber, W., and Anders, S. (2014). Moderated estimation of fold change and dispersion for RNA-seq data with DESeq2. Genome Biol. 15:550. doi: 10.1186/s13059-014-0550-8
Ma, X., Zheng, J., Zhang, X., Hu, Q., and Qian, R. (2017). Salicylic acid alleviates the adverse effects of salt stress on dianthus superbus (Caryophyllaceae) by activating photosynthesis, protecting morphological structure, and enhancing the antioxidant system. Front. Plant Sci. 8:600. doi: 10.3389/fpls.2017.00600
Marino, D., Dunand, C., Puppo, A., and Pauly, N. (2012). A burst of plant NADPH oxidases. Trends Plant Sci. 17, 9–15. doi: 10.1016/j.tplants.2011.10.001
Mele, G., Ori, N., Sato, Y., and Hake, S. (2003). The knotted1-like homeobox gene brevipedicellus regulates cell differentiation by modulating metabolic pathways. Genes Dev. 17, 2088–2093. doi: 10.1101/gad.1120003
Miller, G., and Mittler, R. (2006). Could heat shock transcription factors function as hydrogen peroxide sensors in plants? Ann. Bot. 98, 279–288. doi: 10.1093/aob/mcl107
Munns, R., and Tester, M. (2008). Mechanisms of salinity tolerance. Annu. Rev. Plant Boil. 59, 651–681. doi: 10.1146/annurev.arplant.59.032607.092911
Myouga, F., Hosoda, C., Umezawa, T., Iizumi, H., Kuromori, T., Motohashi, R., et al. (2008). A heterocomplex of iron superoxide dismutases defends chloroplast nucleoids against oxidative stress and is essential for chloroplast development in Arabidopsis. Plant Cell 20, 3148–3162. doi: 10.1105/tpc.108.061341
Neill, S. J., Desikan, R., Clarke, A., Hurst, R. D., and Hancock, J. T. (2002). Hydrogen peroxide and nitric oxide as signalling molecules in plants. J. Exp. Bot. 53, 1237–1247. doi: 10.1093/jexbot/53.372.1237
Nishitani, H., Taraviras, S., Lygerou, Z., and Nishimoto, T. (2001). The human licensing factor for DNA replication Cdt1 accumulates in G1 and is destabilized after initiation of S-phase. J. Biol. Chem. 276, 44905–44911. doi: 10.1074/jbc.M105406200
Noctor, G., and Foyer, C. H. (1998). Ascorbate and glutathione: keeping active oxygen under control. Annu. Rev. Plant Physiol. Plant Mol. Biol. 49, 249–279. doi: 10.1146/annurev.arplant.49.1.249
Noshi, M., Tanabe, N., Okamoto, Y., Mori, D., Ohme-Takagi, M., Tamoi, M., et al. (2018). Clade Ib basic helix-loop-helix transcription factor, bHLH101, acts as a regulatory component in photo-oxidative stress responses. Plant Sci. 274, 101–108. doi: 10.1016/j.plantsci.2018.05.012
Ortega-Amaro, M. A., Rodriguez-Hernandez, A. A., Rodriguez-Kessler, M., Hernandez-Lucero, E., Rosales-Mendoza, S., Ibanez-Salazar, A., et al. (2014). Overexpression of AtGRDP2, a novel glycine-rich domain protein, accelerates plant growth and improves stress tolerance. Front. Plant Sci. 5:782. doi: 10.3389/fpls.2014.00782
Perez-Salamo, I., Papdi, C., Rigo, G., Zsigmond, L., Vilela, B., Lumbreras, V., et al. (2014). The heat shock factor A4A confers salt tolerance and is regulated by oxidative stress and the mitogen-activated protein kinases MPK3 and MPK6. Plant Physiol. 165, 319–334. doi: 10.1104/pp.114.237891
Petrov, V. D., and Van Breusegem, F. (2012). Hydrogen peroxide-a central hub for information flow in plant cells. AoB Plants 2012:pls014. doi: 10.1093/aobpla/pls014
Petrovska, B., Cenklova, V., Pochylova, Z., Kourova, H., Doskocilova, A., Plihal, O., et al. (2012). Plant Aurora kinases play a role in maintenance of primary meristems and control of endoreduplication. New Phytol. 193, 590–604. doi: 10.1111/j.1469-8137.2011.03989.x
Qiu, Z., Guo, J., Zhu, A., Zhang, L., and Zhang, M. (2014). Exogenous jasmonic acid can enhance tolerance of wheat seedlings to salt stress. Ecotoxicol. Environ. Saf. 104, 202–208. doi: 10.1016/j.ecoenv.2014.03.014
Rasmussen, C. G., Sun, B., and Smith, L. G. (2011). Tangled localization at the cortical division site of plant cells occurs by several mechanisms. J. Cell Sci. 124, 270–279. doi: 10.1242/jcs.073676
Raza, A., Charagh, S., Zahid, Z., Mubarik, M. S., Javed, R., Siddiqui, M. H., et al. (2021). Jasmonic acid: a key frontier in conferring abiotic stress tolerance in plants. Plant Cell Rep. 40, 1513–1541. doi: 10.1007/s00299-020-02614-z
Sasabe, M., Ishibashi, N., Haruta, T., Minami, A., Kurihara, D., Higashiyama, T., et al. (2015). The carboxyl-terminal tail of the stalk of Arabidopsis NACK1/HINKEL kinesin is required for its localization to the cell plate formation site. J. Plant Res. 128, 327–336. doi: 10.1007/s10265-014-0687-2
Saxena, I., Srikanth, S., and Chen, Z. (2016). Cross talk between H2O2 and interacting signal molecules under plant stress response. Front. Plant Sci. 7:570. doi: 10.3389/fpls.2016.00570
Seo, Y. J., Park, J. B., Cho, Y. J., Jung, C., Seo, H. S., Park, S. K., et al. (2010). Overexpression of the ethylene-responsive factor gene BrERF4 from brassica rapa increases tolerance to salt and drought in Arabidopsis plants. Mol. Cells 30, 271–277. doi: 10.1007/s10059-010-0114-z
Sharma, S., Lin, W., Villamor, J. G., and Verslues, P. E. (2013). Divergent low water potential response in Arabidopsis thaliana accessions Landsberg erecta and Shahdara. Plant Cell Environ. 36, 994–1008. doi: 10.1111/pce.12032
Shen, X., Wang, Z., Song, X., Xu, J., Jiang, C., Zhao, Y., et al. (2014). Transcriptomic profiling revealed an important role of cell wall remodeling and ethylene signaling pathway during salt acclimation in Arabidopsis. Plant Mol. Biol. 86, 303–317. doi: 10.1007/s11103-014-0230-9
Shin, J., Jeong, G., Park, J. Y., Kim, H., and Lee, I. (2018). MUN (MERISTEM UNSTRUCTURED), encoding a SPC24 homolog of NDC80 kinetochore complex, affects development through cell division in Arabidopsis thaliana. Plant J. 93, 977–991. doi: 10.1111/tpj.13823
Silva, P. C. C., de Azevedo Neto, A. D., Gheyi, H. R., Ribas, R. F., Dos Reis Silva, C. R., and Cova, A. (2020). Salt-tolerance induced by leaf spraying with H2O2 in sunflower is related to the ion homeostasis balance and reduction of oxidative damage. Heliyon 6:e05008. doi: 10.1016/j.heliyon.2020.e05008
Singh, S. A., Winter, D., Kirchner, M., Chauhan, R., Ahmed, S., Ozlu, N., et al. (2014). Co-regulation proteomics reveals substrates and mechanisms of APC/C-dependent degradation. EMBO J. 33, 385–399. doi: 10.1002/embj.201385876
Song, J., Feng, G., Tian, C., and Zhang, F. (2005). Strategies for adaptation of Suaeda physophora, Haloxylon ammodendron and Haloxylon persicum to a saline environment during seed-germination stage. Ann. Bot. 96, 399–405. doi: 10.1093/aob/mci196
Song, R. F., Li, T. T., and Liu, W. C. (2021). Jasmonic acid impairs Arabidopsis seedling salt stress tolerance through MYC2-mediated repression of CAT2 expression. Front. Plant Sci. 12:730228. doi: 10.3389/fpls.2021.730228
Sun, M., Jiang, F., Cen, B., Wen, J., Zhou, Y., and Wu, Z. (2018). Respiratory burst oxidase homologue-dependent H2O2 and chloroplast H2O2 are essential for the maintenance of acquired thermotolerance during recovery after acclimation. Plant Cell Environ. 41, 2373–2389. doi: 10.1111/pce.13351
Suzuki, N., Koussevitzky, S., Mittler, R., and Miller, G. (2012). ROS and redox signalling in the response of plants to abiotic stress. Plant Cell Environ. 35, 259–270. doi: 10.1111/j.1365-3040.2011.02336.x
Tian, L., Zhang, Y., Kang, E., Ma, H., Zhao, H., Yuan, M., et al. (2018). Basic-leucine zipper 17 and Hmg-CoA reductase degradation 3A are involved in salt acclimation memory in Arabidopsis. J. Integr. Plant Biol. 61, 1062–1084. doi: 10.1111/jipb.12744
Tryfona, T., Theys, T. E., Wagner, T., Stott, K., Keegstra, K., and Dupree, P. (2014). Characterisation of FUT4 and FUT6 alpha-(1 -> 2)-fucosyltransferases reveals that absence of root arabinogalactan fucosylation increases Arabidopsis root growth salt sensitivity. PLoS One 9:e93291. doi: 10.1371/journal.pone.0093291
Tsabary, G., Shani, Z., Roiz, L., Levy, I., Riov, J., and Shoseyov, O. (2003). Abnormal ‘wrinkled’ cell walls and retarded development of transgenic Arabidopsis thaliana plants expressing endo-1, 4-beta-glucanase (cell) antisense. Plant Mol. Biol. 51, 213–224. doi: 10.1023/a:1021162321527
Tuteja, N., Tran, N. Q., Dang, H., and Tuteja, R. (2011). Plant MCM proteins: role in DNA replication and beyond. Plant Mol. Biol. 77, 537–545. doi: 10.1007/s11103-011-9836-3
Uchida, A., Jagendorf, A. T., Hibino, T., Takabe, T., and Takabe, T. (2002). Effects of hydrogen peroxide and nitric oxide on both salt and heat stress tolerance in rice. Plant Sci. 163, 515–523. doi: 10.1016/S0168-9452(02)00159-0
Urbanowicz, B. R., Bennett, A. B., Del Campillo, E., Catala, C., Hayashi, T., Henrissat, B., et al. (2007). Structural organization and a standardized nomenclature for plant endo-1, 4-beta-glucanases (cellulases) of glycosyl hydrolase family 9. Plant Physiol. 144, 1693–1696. doi: 10.1104/pp.107.102574
Van den Broeck, L., Dubois, M., Vermeersch, M., Storme, V., Matsui, M., and Inze, D. (2017). From network to phenotype: the dynamic wiring of an Arabidopsis transcriptional network induced by osmotic stress. Mol. Syst. Biol. 13:961. doi: 10.15252/msb.20177840
Van Leene, J., Hollunder, J., Eeckhout, D., Persiau, G., Van De Slijke, E., Stals, H., et al. (2010). Targeted interactomics reveals a complex core cell cycle machinery in Arabidopsis thaliana. Mol. Syst. Biol. 6:397. doi: 10.1038/msb.2010.53
Van Zelm, E., Zhang, Y., and Testerink, C. (2020). Salt tolerance mechanisms of plants. Annu. Rev. Plant Biol. 71, 403–433. doi: 10.1146/annurev-arplant-050718-100005
Vandenabeele, S., Van Der Kelen, K., Dat, J., Gadjev, I., Boonefaes, T., Morsa, S., et al. (2003). A comprehensive analysis of hydrogen peroxide-induced gene expression in tobacco. Proc. Natl. Acad. Sci. U. S. A. 100, 16113–16118. doi: 10.1073/pnas.2136610100
Vogel, M. O., Moore, M., Konig, K., Pecher, P., Alsharafa, K., Lee, J., et al. (2014). Fast retrograde signaling in response to high light involves metabolite export, MITOGEN-ACTIVATED PROTEIN KINASE6, and AP2/ERF transcription factors in Arabidopsis. Plant Cell 26, 1151–1165. doi: 10.1105/tpc.113.121061
Wahid, A., and Shabbir, A. (2015). Induction of heat stress tolerance in barley seedlings by pre-sowing seed treatment with glycinebetaine. Plant Growth Regul. 46, 133–141. doi: 10.1007/s10725-005-8379-5
Wahid, A., Perveen, M., Gelani, S., and Basra, S. M. (2007). Pretreatment of seed with H2O2 improves salt tolerance of wheat seedlings by alleviation of oxidative damage and expression of stress proteins. J. Plant Physiol. 164, 283–294. doi: 10.1016/j.jplph.2006.01.005
Wang, H., Dittmer, T. A., and Richards, E. J. (2013). Arabidopsis CROWDED NUCLEI (CRWN) proteins are required for nuclear size control and heterochromatin organization. BMC Plant Biol. 13:200. doi: 10.1186/1471-2229-13-200
Wang, H., Liu, Y., Yuan, J., Zhang, J., and Han, F. (2019). The condensin subunits SMC2 and SMC4 interact for correct condensation and segregation of mitotic maize chromosomes. Plant J. 102, 467–479. doi: 10.1111/tpj.14639
Wang, L., Hua, D., He, J., Duan, Y., Chen, Z., Hong, X., et al. (2011). Auxin response factor2 (ARF2) and its regulated homeodomain gene HB33 mediate abscisic acid response in Arabidopsis. PLoS Genet. 7:e1002172. doi: 10.1371/journal.pgen.1002172
Wang, Q., Liu, S., Lu, C., La, Y., Dai, J., Ma, H., et al. (2019). Roles of CRWN-family proteins in protecting genomic DNA against oxidative damage. J. Plant Physiol. 233, 20–30. doi: 10.1016/j.jplph.2018.12.005
Wang, Y., Li, J., Wang, J., and Li, Z. (2010). Exogenous H2O2 improves the chilling tolerance of manilagrass and mascarenegrass by activating the antioxidative system. Plant Growth Regul. 61, 195–204. doi: 10.1007/s10725-010-9470-0
Wu, T., Hu, E., Xu, S., Chen, M., Guo, P., Dai, Z., et al. (2021). clusterProfiler 4.0: a universal enrichment tool for interpreting omics data. Innovation 2:100141. doi: 10.1016/j.xinn.2021.100141
Yang, G., Rhodes, D., and Joly, R. J. (1996). Effect of high temperature on membrane stability and chlorophyll fluorescence in glycinebetaine-containing maize lines. Aust. J. Plant Physiol. 23, 431–443. doi: 10.1016/S0090-3019(01)00471-2
Yang, W., Schuster, C., Beahan, C. T., Charoensawan, V., Peaucelle, A., Bacic, A., et al. (2016). Regulation of meristem morphogenesis by cell wall synthases in Arabidopsis. Curr. Biol. 26, 1404–1415. doi: 10.1016/j.cub.2016.04.026
Yang, W., Wightman, R., and Meyerowitz, E. M. (2017). Cell cycle control by nuclear sequestration of CDC20 and CDH1 mRNA in plant stem cells. Mol. Cell 68, 1108–1119.e3. doi: 10.1016/j.molcel.2017.11.008
Yao, Y., He, R., Xie, Q., Zhao, X., Deng, X., He, J., et al. (2017). Ethylene response factor 74 (ERF74) plays an essential role in controlling a respiratory burst oxidase homolog D (RbohD)-dependent mechanism in response to different stresses in Arabidopsis. New Phytol. 213, 1667–1681. doi: 10.1111/nph.14278
Yu, Q., Tian, H., Yue, K., Liu, J., Zhang, B., Li, X., et al. (2016). A P-Loop NTPase regulates quiescent center cell division and distal stem cell identity through the regulation of ROS homeostasis in Arabidopsis root. PLoS Genet. 12:e1006175. doi: 10.1371/journal.pgen.1006175
Zahedi, S. M., Hosseini, M. S., Fahadi Hoveizeh, N., Gholami, R., Abdelrahman, M., and Tran, L. P. (2021). Exogenous melatonin mitigates salinity-induced damage in olive seedlings by modulating ion homeostasis, antioxidant defense, and phytohormone balance. Physiol. Plant. 173, 1682–1694. doi: 10.1111/ppl.13589
Zhang, J., and Kirkham, M. B. (1996). Antioxidant responses to drought in sunflower and sorghum seedlings. New Phytol. 132, 361–373.
Zhao, C., Jiang, W., Zayed, O., Liu, X., Tang, K., Nie, W., et al. (2021). The LRXs-RALFs-FER module controls plant growth and salt stress responses by modulating multiple plant hormones. Natl. Sci. Rev. 8:nwaa149.
Zhao, C., Zhang, H., Song, C., Zhu, J. K., and Shabala, S. (2020). Mechanisms of plant responses and adaptation to soil salinity. Innovation 1:100017. doi: 10.1016/j.xinn.2020.100017
Zhao, J., Barkla, B. J., Marshall, J., Pittman, J. K., and Hirschi, K. D. (2008). The Arabidopsis cax3 mutants display altered salt tolerance, pH sensitivity and reduced plasma membrane H+-ATPase activity. Planta 227, 659–669. doi: 10.1007/s00425-007-0648-2
Keywords: hydrogen peroxide, pretreatment, Arabidopsis thaliana, salt stress, transcriptome profiling
Citation: Zhang Q, Dai X, Wang H, Wang F, Tang D, Jiang C, Zhang X, Guo W, Lei Y, Ma C, Zhang H, Li P, Zhao Y and Wang Z (2022) Transcriptomic Profiling Provides Molecular Insights Into Hydrogen Peroxide-Enhanced Arabidopsis Growth and Its Salt Tolerance. Front. Plant Sci. 13:866063. doi: 10.3389/fpls.2022.866063
Received: 30 January 2022; Accepted: 28 February 2022;
Published: 06 April 2022.
Edited by:
Quan-Sheng Qiu, Lanzhou University, ChinaCopyright © 2022 Zhang, Dai, Wang, Wang, Tang, Jiang, Zhang, Guo, Lei, Ma, Zhang, Li, Zhao and Wang. This is an open-access article distributed under the terms of the Creative Commons Attribution License (CC BY). The use, distribution or reproduction in other forums is permitted, provided the original author(s) and the copyright owner(s) are credited and that the original publication in this journal is cited, in accordance with accepted academic practice. No use, distribution or reproduction is permitted which does not comply with these terms.
*Correspondence: Pinghua Li, cGluZ2h1YWxpQHNkYXUuZWR1LmNu; Yanxiu Zhao, emhhb3l4QHNkbnUuZWR1LmNu; Zenglan Wang, d2FuZ3psQHNkbnUuZWR1LmNu
†These authors have contributed equally to this work and share first authorship