- Cell and Developmental Biology Department, John Innes Centre, Norwich Research Park, Norwich, United Kingdom
The plant vasculature plays essential roles in the transport of water and nutrients and is composed of xylem and phloem, both of which originate from undifferentiated cells found in the cambium. Development of the different vascular tissues is coordinated by hormonal and peptide signals and culminates in extensive cell wall modifications. Pectins are key cell wall components that are modified during cell growth and differentiation, and pectin fragments function as signals in defence and cell wall integrity pathways, although their role as developmental signals remains tentative. Here, we show that the pectin lyase-like gene PLL12 is required for growth of the vascular bundles in the Arabidopsis inflorescence stem. Although PLL12 was expressed primarily in the phloem, it also affected cambium and xylem growth. Surprisingly, PLL12 overexpression induced ectopic cambium and xylem differentiation in the inflorescence apex and inhibited development of the leaf vasculature. Our results raise the possibility that a cell wall-derived signal produced by PLL12 in the phloem regulates cambium and xylem development.
Introduction
The vasculature of land plants is critical for growth, as it distributes water and nutrients throughout the plant and provides mechanical support (Ruonala et al., 2017). Each vascular bundle contains three types of tissues: xylem, including water-transporting xylem elements, the mechanically reinforced xylem fibres and parenchyma; phloem, containing the sugar-transporting sieve elements, the companion cells that regulate movement of molecules in and out of the phloem, phloem fibres and parenchyma; and cambium, a layer of undifferentiated cells that produces new xylem cells toward the plant’s main axis and new phloem cells away from it (Nieminen et al., 2015).
During the early stages of vascular development, the position of xylem, phloem and the procambium (precursor of the cambium) is defined by intercellular signals mediated by the auxin and cytokinin hormones, in combination with mobile transcription factors and microRNAs (Carlsbecker et al., 2010; Miyashima et al., 2019). The subsequent production of new vascular cells by the cambium is regulated by the peptide signals CLAVATA3/EMBRYO SURROUNDING REGION (CLE) CLE41/CLE44, which activate the Phloem Intercalated With Xylem (PXY) receptor to regulate the balance between the production of new phloem and xylem cells (Ito et al., 2006; Fisher and Turner, 2007; Hirakawa et al., 2008). The ensuing differentiation of these tissues is guided by cell type-specific transcription factors, such as VASCULAR-RELATED NAC DOMAIN 6 (VND6) and VND7, which specify xylem cell types (Kubo et al., 2005), or Altered Phloem Development (APL) (Bonke et al., 2003) and NAC DOMAIN-CONTAINING PROTEIN 45 (NAC45) (Furuta et al., 2014), which control phloem differentiation.
Among the processes regulated during vascular differentiation, modifications of the cell wall feature prominently, for example in the deposition of lignified hoops that mechanically reinforce xylem elements (Mitsuda et al., 2007; Sugiyama et al., 2017), or in the development of perforated plates that connect adjacent sieve elements (Kalmbach and Helariutta, 2019). Pectic polysaccharides, of which the most abundant form is homogalacturonan, are important components of the plant cell wall that are modified during cell growth and differentiation. Pectins are secreted in a methyl-esterified form and modified by pectin methylesterase (PME) to control the degree of methylesterification. Pectins with low levels of methylesterification bind Ca2+ within the cell wall to form a cross-linked gel that surrounds cellulose and is believed to decrease cell wall extensibility (Wolf et al., 2012; Yang et al., 2018). Developmentally controlled pectin methylesterification modulates not only wall extensibility, but also access to further cell wall-modifying enzymes, such as those involved in the deposition of lignin during xylem differentiation (Wolf et al., 2012).
One group of cell wall modifiers that target demethylesterified pectin are the PECTATE LYASE-LIKE (PLL) proteins, which are found throughout the plant kingdom and are encoded by large gene families (Uluisik and Seymour, 2020). PLLs have an N-terminal signal peptide that targets them for secretion into the cell wall, where they cut homogalacturonan at β(1–4) linkages to generate oligogalacturonides (OGs) of different sizes (Sénéchal et al., 2014; Yang et al., 2018). Pectin cleavage occurs during cell expansion or remodelling, and in the middle lamella during cell separation (Yang et al., 2018). Accordingly, PLLs have been implicated in cell expansion processes, such as elongation of cotton fibres (Sun et al., 2020), in secondary wall formation in the xylem, and in cell separation during fruit ripening (Uluisik and Seymour, 2020). Additionally, the OGs produced during pectin cleavage have a signalling role, studied mostly in relation to pathogen attack and as part of the cell wall integrity pathway (Brutus et al., 2010; Ferrari et al., 2013). Externally applied OGs also have developmental consequences, for example by inhibiting the effect of auxin on pea stem elongation (Branca et al., 1988), although a role for OGs as endogenous developmental signals still remains to be established (Ferrari et al., 2013).
Here, we characterise the function of PLL12 in Arabidopsis vascular development. We selected this gene based on ChIP-seq data for the BEL1-like homeodomain transcription factor REPLUMLESS (RPL) (also known as PENNYWISE, PNY and BELLRINGER, BLR) (Byrne et al., 2003; Roeder et al., 2003; Smith and Hake, 2003), which is required for correct vascular patterning (Smith and Hake, 2003; Etchells et al., 2012) and directly interacts with multiple genes that regulate vascular development, such as PXY, CLE41 and NAC45 (Bencivenga et al., 2016). Furthermore, RPL has been implicated in the expression of cell wall enzymes involved in vascular differentiation (Peaucelle et al., 2011; Etchells et al., 2012). We reasoned that less well-characterised RPL target genes, such as PLL12, might reveal novel aspects of vascular development. Our functional analysis showed that PLL12 is expressed in the phloem but has unexpected cell non-autonomous effects on cambium and xylem development.
Materials and Methods
Plant Material
Plants were grown on JIC Arabidopsis Soil Mix (Levington F2 compost plus Intercept and 4 mm grit at a 6:1 ratio) at 16°C under continuous light (100 mE) or in a Sanyo cabinet at 18°C under 16 h light/8 h dark cycles (100 mE). Arabidopsis thaliana Columbia (Col) and Landsberg-erecta (L-er) were used as wild-types; pll12 (AT5g04310; SAIL 1149_C06) was obtained from The Nottingham Arabidopsis Stock Centre and genotyped using primers 1–3 Table 1. For the construction of RPS5A:LhGR:opPLL12, the PLL12 CDS was amplified from Col cDNA (primers 12–13 Table 1), cloned into pGEMT-EASY, sequenced, moved into pOWL49 by SalI/KpnI restriction cloning and transformed into RPS5A:LhGR in L-er background. Transformants were selected on gentamycin/kanamycin Murashige and Skoog medium (M&S) with agar, transferred to soil and treated at appropriate developmental stages with either 10 μM dexamethasone (from a 10 mM stock in ethanol) or the equivalent volume of ethanol (mock-treatment) diluted in aqueous 0.015% Silwet L-77 (49). Some seedlings were germinated and grown on M&S agar and then transferred to M&S agar supplemented with either 10 μM dexamethasone or ethanol (mock).
To complement the pll12 phenotype, a full length pPLL12:PLL12 construct was generated as follows. Using Columbia genomic DNA and Phusion polymerase (New England Biolabs), the promoter and 5′ utr (1,884 bp) were amplified as two fragments, the gene (3,997 bp) as four fragments and the 702 bp 3′ utr as a single fragment using primers 14–27 in Table 1. The seven fragments were assembled into plasmid G45 by Golden Gate cloning (Engler et al., 2014). The assembly was verified by sequencing and inserted into plasmid G800 by Gateway cloning. For construction of pPLL12:PLL:GUS, PLL12 was amplified from Col-0 genomic DNA and fused in-frame with GUS and cloned into pPZP222 (Hajdukiewicz et al., 1994) using Golden Gate cloning as above, with primers listed in Table 1. The final constructs were transformed into Col-0 by the floral dip method (Clough and Bent, 1998) and transformants were selected on Murashige and Skoog medium supplemented with gentamycin 100 μg/mL.
Measurements of Stem Growth
Arabidopsis Col and pll12 plants were grown until the first flower reached stage 17 (Smyth et al., 1990), ink marks were placed on the stem at 2 mm intervals and photographed next to a ruler using a Nikon D3100 DSLR camera and 18–55 mm VR lens. Plants were returned to the growth cabinet for 4 d and then re-photographed. Manual land marking of the stem ink marks and calibration points on the ruler was performed using the Point Picker plugin of Fiji (Schindelin et al., 2012). Digitalised coordinates were measured, graphs were plotted, and Mann-Whitney U tests and Student’s t tests were performed using standard functions in matplotlib,1 Python 2.7, and Scientific Python.2
Microscopy and Staining
Expression of GUS reporters in stem tissue was performed as described (Sieburth et al., 1998), stained tissue was observed with a Leica S8APO stereozoom or Zeiss Axio Imager Z2. Modified pseudo-Schiff propidium iodide (mPS-PI) staining was performed before imaging samples with a Zeiss LSM780 confocal microscope as described (Serrano-Mislata et al., 2015). Staining with 0.04% Calcofluor was performed on Technovit sections for 5 min at room temperature.
To determine if xylem bundles in pll12 stems were continuous and intact, 1 cm stem apices were stained with propidium iodide (Banasiak et al., 2019) and examined using a Zeiss Axiozoom V16 microscope.
Tissues for light microscopy were fixed in either 1% glutaraldehyde in 0.05 M cacodylate buffer pH 7.2 or formalin:acetic acid:ethanol (4:5:50), dehydrated in an ethanol series and embedded in Technovit following the manufacturer’s instruction. Sections were cut 5 μm thick with glass knives and a Leica ultramicrotome UC7, stained with toluidine blue and examined with a Zeiss Axio Imager Z2 microscope.
Stems for vibratome sectioning were prepared following the protocol of Wang et al. (2014), sections were cut 25–30 μm thick using a Leica VT1000S vibratome, stained with phloroglucinol-HCl (Mitra and Loqué, 2014) and mounted on glass slides following Speer’s modification (Speer, 1987).
RNA in situ Hybridisation Experiments
Stem apices of RPS5A:LhGR:opPLL12 plants were treated with or without dexamethasone: all stem apices were fixed and subsequently processed according to Rebocho et al. (2017). Serial sections from control (mock-treated) and experimental (dex-treated) samples were collected onto the same Poly-L-Lysine coated slide. Duplicate slides were sandwiched together between plastic spacers and loaded with either sense or antisense probes. To generate specific digoxigenin-labelled riboprobes probes, 550 bp of PXY coding sequence was cloned using primers 28-29 (Table 1) and BamH1/Sal1 ligated into pBluescript II KS9 (±). Antisense probes were PCR amplified using combinations of M13R primer and the specific gene F primer, whilst sense probes were amplified similarly using M13F and specific gene R primers. Purified antisense and sense probes were obtained by RNA transcription using the T3 and T7 promoters respectively following the protocol of Rebocho et al. (2017).
Rhodamine B Uptake
Col and pll12 plants grown to the same developmental stage (3–5 flowers/siliques) were removed from their pots and very gently washed under running tap water to remove soil from the root balls before blotting dry with absorbent paper towels. Roots were then threaded through holes cut into a disc of polystyrene foam and the plants floated in a glass beaker containing 50 ml of 0.25% rhodamine B (w/v) so that the roots were immersed in the solution. Batches of 3–4 Col and pll12 plants were incubated simultaneously under a 6 h light-8 h dark-6 h light regime at 16°C in a Sanyo cabinet. The following day, excess dye was rinsed off the roots which were then blotted dry and the plants photographed and stem samples collected. All but the smallest flower buds were removed before the apical 1 cm and basal 1 cm of stem above the rosette were isolated, frozen in liquid nitrogen and stored at −70°C. Individual stem samples were ground up in 400 μl protein extraction buffer (100 mM HEPES pH 7.5, 5% v/v glycerol, 50 mM KCl, 5 mM EDTA, 0.1% v/v Triton X-100, 1 mM DTT and one Complete EDTA-free protease inhibitor cocktail tablet/50 ml buffer) and rotated at 4°C for 1 h to extract soluble proteins and the rhodamine B dye. After a 10 min centrifugation at 12,000 g to pellet insoluble material, 100 μl aliquots of the extracts were collected into wells of a 96-well microtitre plate and assayed spectrophotometrically at A550nm; dye concentrations were calculated against a rhodamine B standard curve. Soluble proteins were isolated from the extracts by methanol-chloroform precipitation and the rhodamine B removed by methanol washes, protein pellets were then resuspended in 100 μl of protein extraction buffer and protein concentrations calculated by Bradford reactions.
Quantitative Reverse Transcription-Polymerase Chain Reaction
mRNA levels were measured by quantitative reverse transcription-polymerase chain reaction (qRT-PCR) as published (Schiessl et al., 2012), with primers 8–11 listed in Table 1.
Results
Genes annotated as belonging to the pectin lyase superfamily are enriched among candidate targets of RPL (Bencivenga et al., 2016; Supplementary Table 1); among these, PLL12 (AT5G04310) showed clear binding to RPL in three ChIP-seq biological replicates, but in none of the negative controls (Supplementary Figure 1). Furthermore, PLL12 expression was located specifically in vascular cell types in a root transcriptomic atlas (Brady et al., 2007) and was associated with vascular differentiation in vitro (Kondo et al., 2016). Based on these data, we hypothesised that PLL12 might have a role in cell wall modification during vascular development, in addition to the previously described function in stomatal guard cells (Chen et al., 2021).
To confirm the PLL12 expression during vascular development, we used the GUS reporter fused to the complete PLL12 gene. Previous reporter genes using GUS fused to 2 kb of the putative PLL12 promoter gave weak and variable expression (Sun and Van Nocker, 2010) or suggested widespread expression (Chen et al., 2021). Our ChIP-seq data, however, indicated RPL binding to intron 3 of PLL12 (Supplementary Figure 1), raising the possibility that regulatory sequences have been missed in previous constructs. To include all potential regulatory sequences, we used a genomic fragment that fully complemented the pll12 mutation (see below), with GUS fused in frame after the last exon of PLL12. In nine independent lines, this pPLL12:PLL12-GUS reporter was expressed in stomata and throughout the vasculature of seedlings (Figure 1). In both the root and in the inflorescence, expression initiated close to the apical meristem, indicating that PLL12 begins to function during early stages of vascular development. Cross-sections of the stem showed that within the vascular strands, PLL12 was expressed primarily in the phloem. This localised expression pattern was in agreement with the reported role of PLL12 in stomatal guard cells (Chen et al., 2021) and with reports of phloem-specific PLL12 expression in the root (Brady et al., 2007; Kalmbach et al., 2022) and during in vitro differentiation (Kondo et al., 2016).
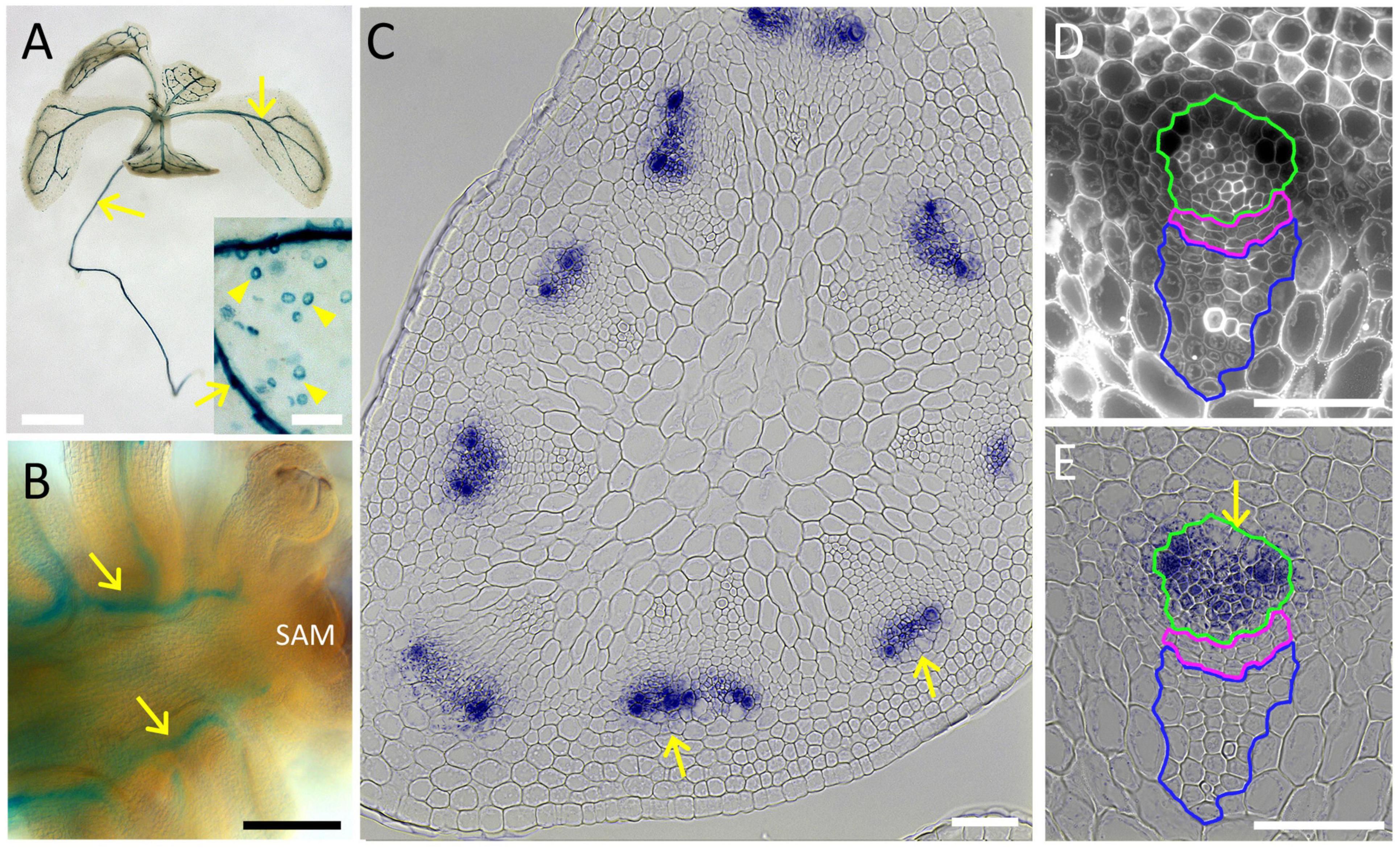
Figure 1. PLL12 is expressed in the stem phloem. (A,B): pPLL12:PLL12:GUS seedling (A) and inflorescence apex (B) stained for GUS and cleared with chloral hydrate, showing GUS activity in the vasculature (arrows); in panel (A), note also the dotted signal on cotyledons due to expression in stomatal guard cells. Bars: 1 cm (A), 500 μm (B). (C–E): Cross-section of the inflorescence stem showing pPLL12:PLL12:GUS expression in phloem cells; (C): overview of a stem section, with GUS staining in part of the vascular bundles (arrows); (D): calcofluor-stained section through a single vascular bundle, with the phloem, cambium and xylem regions enclosed in green, magenta, and blue lines, respectively; (E): same section as in panel (D), showing GUS staining specifically in the phloem (arrow); bars: 50 μm.
We next analysed the function of PLL12, focussing especially on vascular development. For this, we used two independent T-DNA mutant alleles, pll12-1 (SAIL 1207_A07) (Chen et al., 2021) and pll12-2 (SAIL 1149_C06). As reported, pll12-1 had undetectable PLL12 mRNA (Chen et al., 2021), and the same was seen for pll12-2 (Supplementary Figure 2). Both alleles caused similar phenotypes: the mutants were dwarf and late flowering, with slow stem growth (Figure 2). Complementation with full genomic fragments (from 1,884 bp upstream of the start codon to 702 bp downstream of the stop codon) confirmed that the pll12 mutations caused these phenotypes, and that the selected genomic region contained all regulatory and coding regions required for function (Figure 2). Because the mutants had comparable and fully recessive phenotypes, from this point on we used the previously characterised pll12-1 mutant.
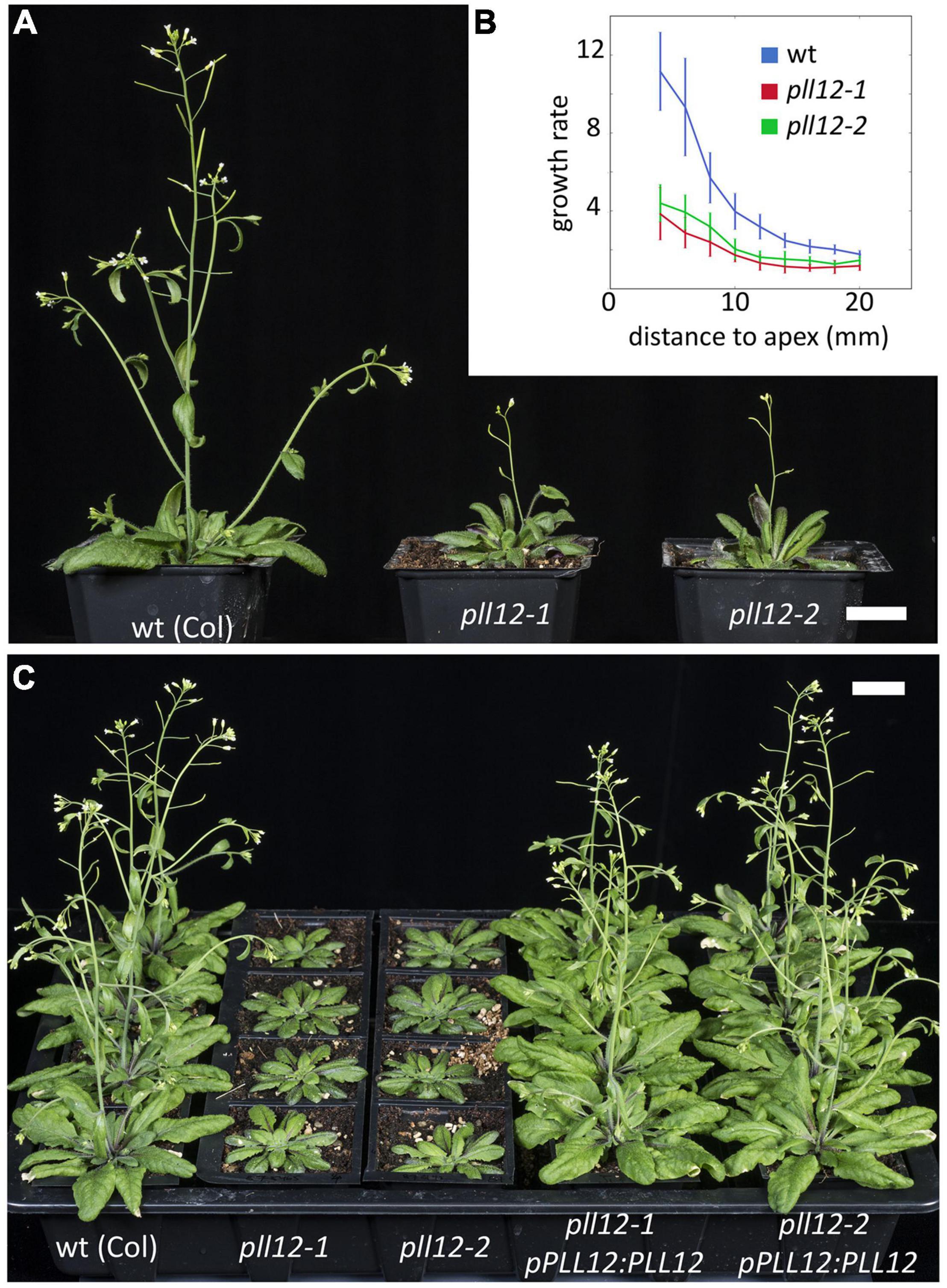
Figure 2. pll12 mutants have reduced growth and slow stem elongation. (A): Adult wild type (Col-0) and two different pll12 mutant alleles grown side by side for 40 days. Both mutant lines bolted late and produced slow growing stems with few lateral organs. Scale bar: 2 cm. (B): Relative growth rate (fold increase in length over 4 days) in Col-0 and pll12 mutants measured at different starting positions along the stem. The stem elongates faster nearer to the apex and in the mutants this differential growth was significantly reduced. (C): A genomic pPLL12:PLL12 construct transformed into both mutant lines rescued the wild type phenotype. Scale bar: 2 cm.
The vascular pattern of pll12-1 appeared normal and was uninterrupted in leaves and in the inflorescence apex (Supplementary Figure 3). The vascular bundles contained all the expected cell types in their correct positions, but appeared smaller, especially in the mature region of the inflorescence stem (Figure 3). To detect growth defects, we measured the size and cell numbers in different regions of the stem vasculature. To compensate for differences in the growth rate between the mutants and the wild type, stems were collected when the fifth flower had matured (i.e., after a longer period of growth in pll12-1). Within stems at this stage, we collected sections at two positions: at the base of the fifth flower, corresponding to the immature region of the stem, which is still elongating (less than 1 cm of the apex, see Figure 2), and near the first silique, where stem elongation is complete and the vascular bundles have started to deposit secondary cell walls (more than 2 cm from the apex, Figure 2). In pll12-1, the size of immature vascular strands was not different from the wild type (number of cells per cross-section, mean ± SD: 101.4 ± 19.9 in wt, 98.7 ± 15.9 in pll12-1, Supplementary Table 2). However, as the stem matured, differences became clearer (213.9 ± 27.7 in wt, 142.4 ± 33.5 in pll12-1, Supplementary Table 2) and, contrary to the expectation based on the expression pattern, were not specific to the phloem. Instead, all three regions of the vascular strand (phloem, cambium and xylem) had reduced growth, measured either by cross-sectional area or cell numbers (Figures 3D,F–H). The region most affected was the cambium, which already had significantly fewer cells in the mutant before differences were detectable in the phloem and xylem (Figures 3G,H). Furthermore, the cambium was the only region with no detectable growth (measured as either cross-sectional area or by number of cells in cross-section), in contrast to the phloem and xylem, which grew at about half the wild-type rate. These results showed that PLL12 is not essential for the initial vascular patterning or differentiation but is required for subsequent growth of all regions of the vascular strand, particularly the cambium.
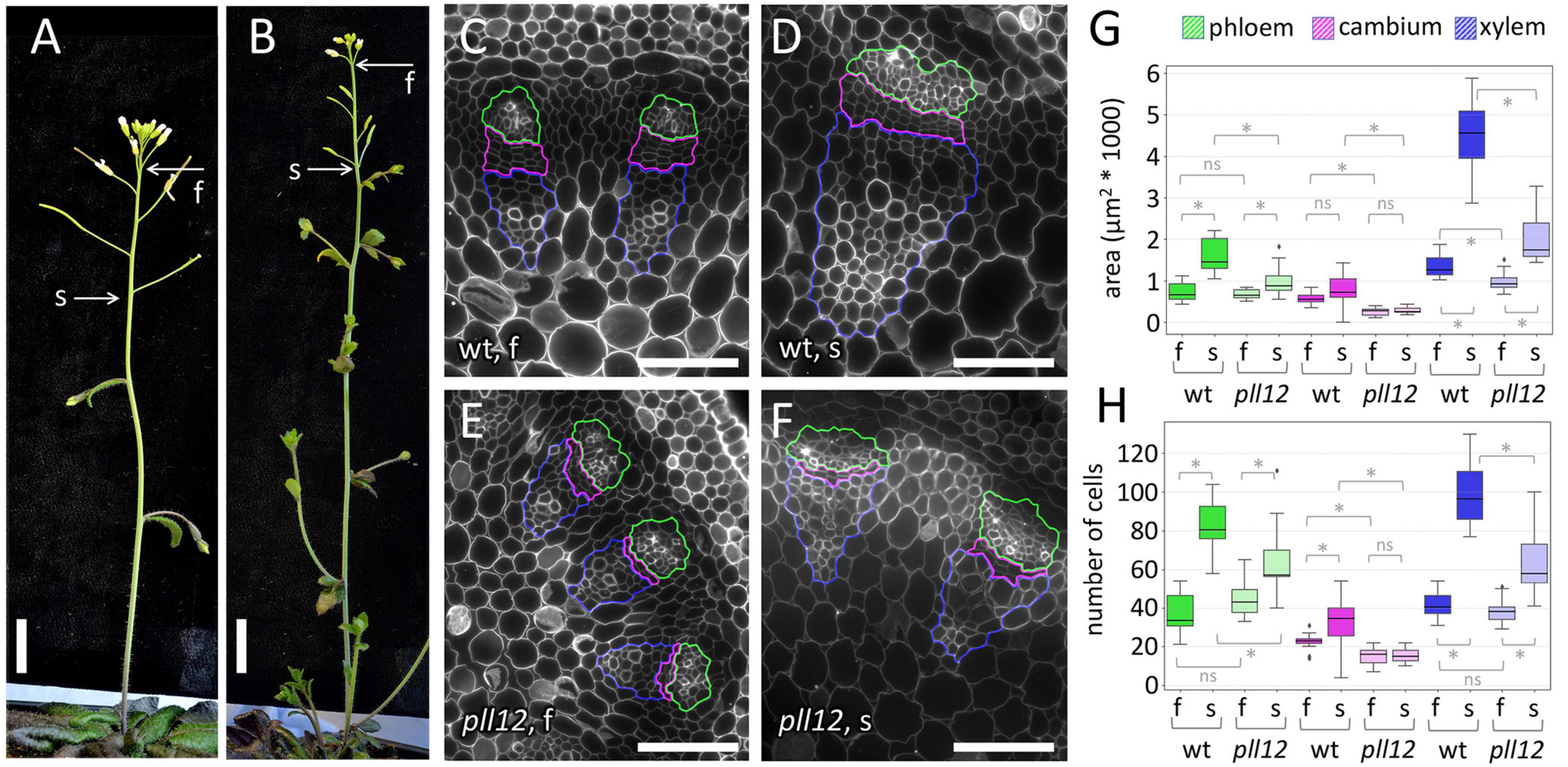
Figure 3. PLL12 is required for growth of the cambium and vascular bundles in the stem. (A,B): overview of wt (A) and pll12-1 (B) inflorescence stems, with the position of the most mature flower before organ abscission (f) and of the oldest silique (s) indicated; scale bars: 1 cm. (C–F): calcofluor-stained cross-sections of vascular bundles of wt (C,D) and pll12-1 (E,F) stems, at the position of the most mature flower (C,E) or of the oldest silique (D,F); the phloem, cambium and xylem regions are enclosed in green, magenta, and blue lines, respectively; scale bars: 50 μm. (G,H): boxplots of the area (G) and cell number (H) within phloem, cambium and xylem regions selected as in (C–F), comparing vascular bundles at positions f and s in wt and pll12-1 stems; * and ns indicate significant and non-significant differences in pairwise comparisons (p < 0.05, Mann-Whitney test); Supplementary Table 2 contains a full statistical analysis, including numbers of replicates, p-values and power analysis.
To reveal physiological consequences of the histological defects described above, we investigated whether pll12-1 affected phloem and xylem function. Using carboxyfluorescein diacetate (CFDA) as a tracer, reduced phloem transport from hypocotyls to roots was shown in pll12 seedlings (Kalmbach et al., 2022). Although we were not able to adapt this method to measure phloem transport in the inflorescence stem, it would be reasonable to assume that a similar defect would occur in the stem vasculature. As an indirect method to detect defects in phloem function in the stem, we assayed for high starch levels after a dark period, which are typically seen in plants with compromised sugar transport (Barratt et al., 2010; Bezrutczyk et al., 2018). Consistent with a defect in sugar export, staining with Lugol’s Iodine reagent revealed a strong accumulation of starch in the cortex of the inflorescence stem in pll12-1 compared to the wild type (Figure 4). To assess xylem function, we measured transport of rhodamine B dye from roots to the inflorescence apex. Both visually (Figures 4E,F) and based on the dye concentration in the apex (normalised to protein concentration, Figure 4G), the mutant showed a clear reduction in transport through the xylem. We conclude that the reduced growth of vascular bundles in pll12 was accompanied by a decrease in both sugar and water transport, which might be the cause of the general inhibition of growth in the mutant. Similarly, slow growth of the cambium and xylem in the mutant might be an indirect consequence of a reduced sugar supply, caused by a primary defect in the phloem. Alternatively, PLL12 might be required to produce a phloem-derived signal that promotes development of the cambium and xylem.
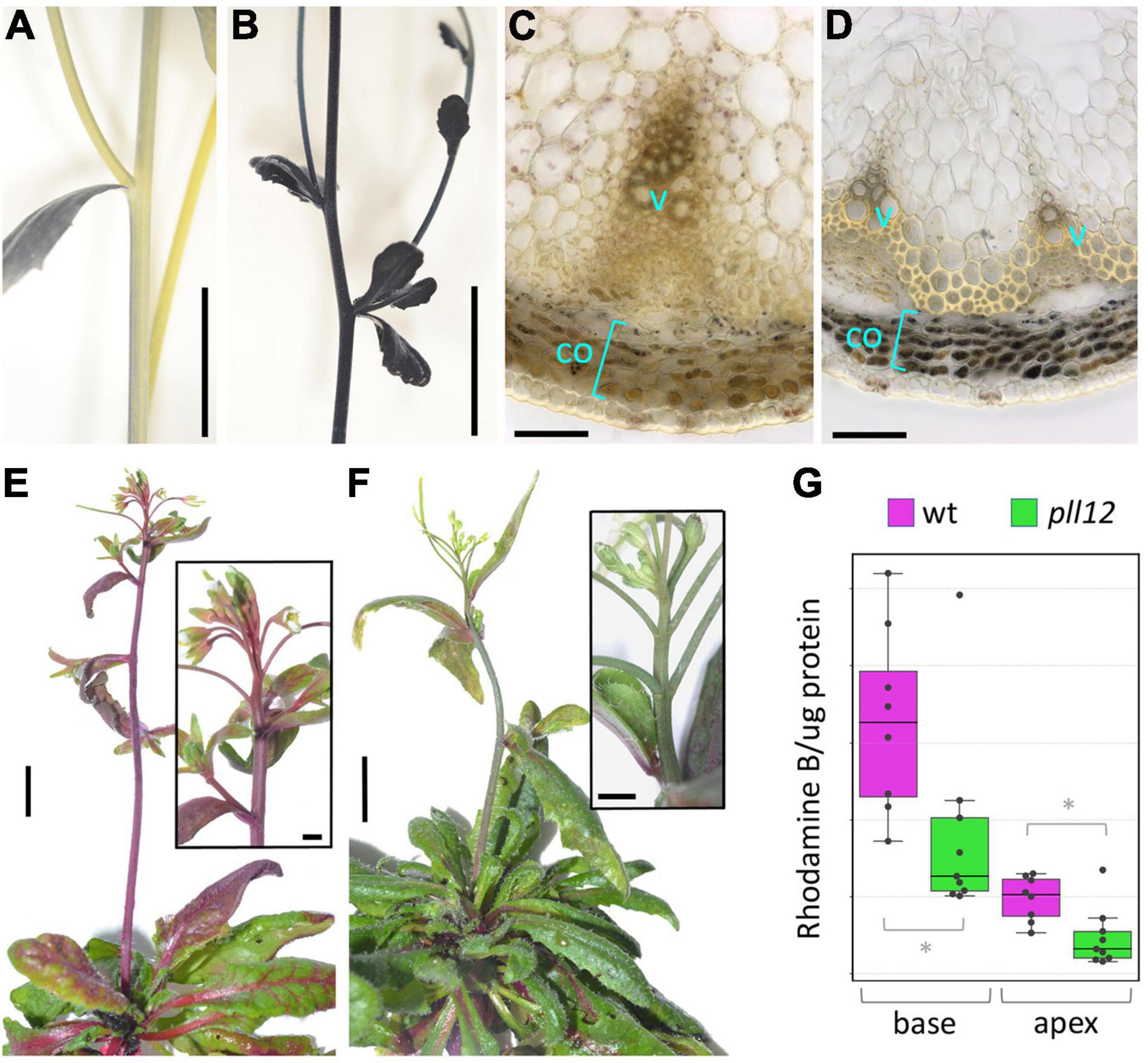
Figure 4. PLL12 is required for both phloem and xylem function. (A,B): inflorescence stems of wt (A) and pll12-1 (B) stained with Lugol’s solution, showing accumulation of starch in the mutant; scale bars: 1 cm. (C,D): transversal sections of wt (C) and pll12 (D) stems stained with Lugol’s solution, showing accumulation of starch grains in cortex cells (co) in the mutant; scale bars: 50 μm. (E,F): wt (E) and pll12-1 (F) plants grown for 20 h with their roots immersed in rhodamine B solution; the dye was transported efficiently throughout the wt inflorescence, whilst the mutant stems transported far less dye to the apex; insets show the inflorescence apices at a higher magnification; scale bars: 1 cm (main panels), 250 μm (insets). (G): Boxplots showing Rhodamine content (normalised to protein concentration to correct for differences in tissue mass) in the apical and basal 1 cm segments of wt (magenta) and pll12-1 (green) stems; * indicate significant differences (p < 0.05, Mann-Whitney test; full statistical analysis in Supplementary Table 2).
To test whether increased or ectopic PLL12 expression might be sufficient to promote cambium and xylem development, we generated plants in which PLL12 transcription could be induced ubiquitously. To do this, we used an pOp:PLL12 construct expressed in an RPS5A:LhGR background; when tissues are exposed to dexamethasone, this system induces PLL12 expression under the widely expressed RIBOSOMAL SUBUNIT 5A promoter (Supplementary Figure 4). After inflorescence tips were treated five times at 2-day intervals, dexamethasone-treated plants showed variable inhibition of growth, ranging from short internodes to death of the shoot apex (Figures 5A–C). These phenotypes were associated with different levels of lignin deposition in the cortex and vascular bundles within 1–2 mm below the shoot meristem (Figures 5D–F). In apices with a mild phenotype, it was possible to trace the earliest deposition of ectopic lignin to strands of cells leading basally away from the shoot apical meristem—this position is typically occupied by procambial strands in the wild type (Supplementary Figure 5), suggesting that cells at early stages of vascular differentiation were particularly sensitive to ectopic PLL12 expression.
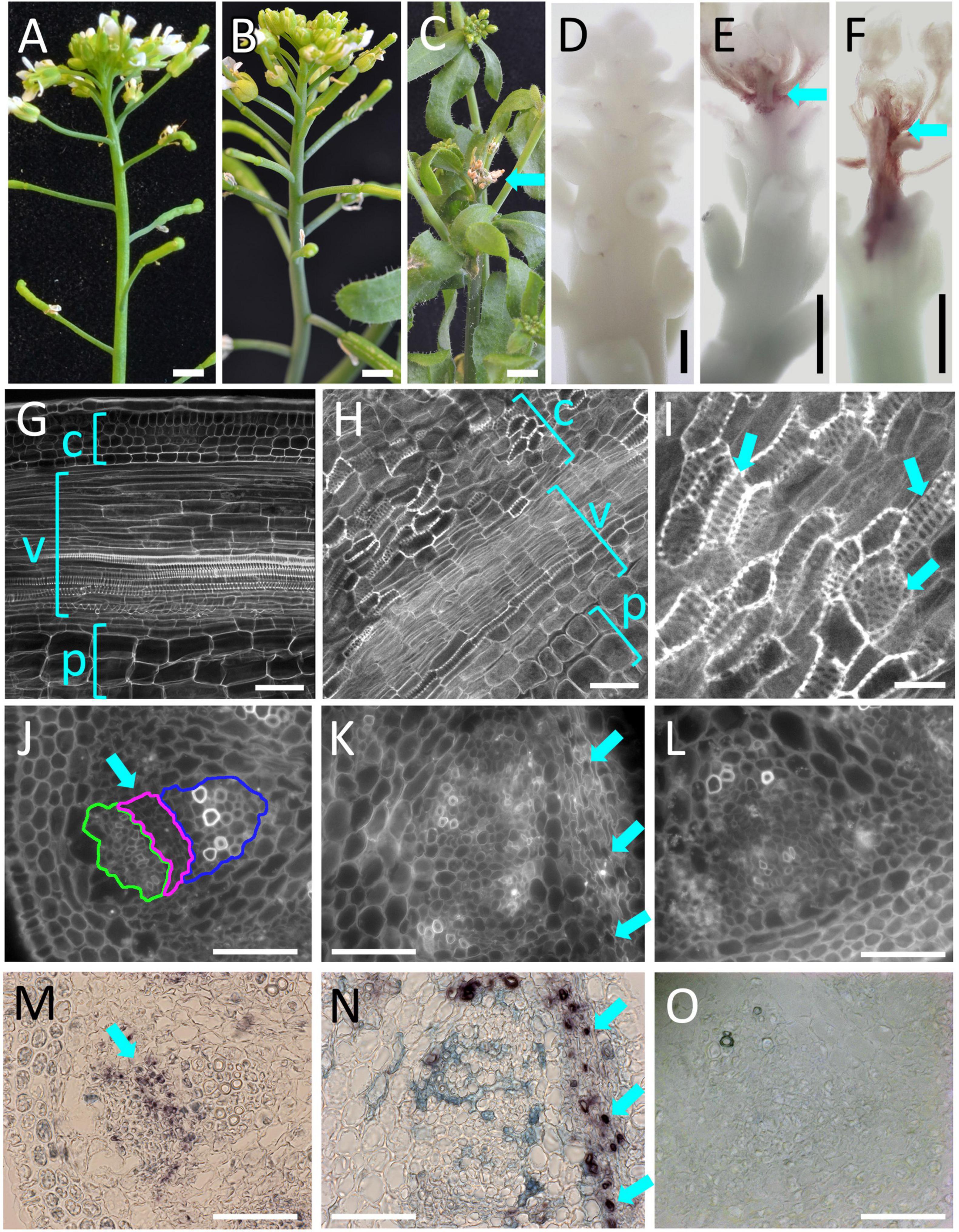
Figure 5. PLL12 over-expression induces ectopic xylem differentiation and expression of a cambium marker. (A–C): Mock-treated RPS5A:LhGR,pOp:PLL12 (A) looked normal, whilst induction of PLL12 expression with dexamethasone resulted in mild [(B), shortened internodes] to severe effects [(C), with death of the stem apex (arrow) and lateral organs]; scale bars: 2 mm. (D–F): Mock-treated (D) or dexamethasone-treated (E,F) inflorescence apices of RPS5A:LhGR, pOp:PLL12 plants stained with phloroglucinol, showing mild (E) to severe (F) lignification; scale bars: 400 μm. (G–I): Confocal images of RPS5A:LhGR:PLL12 stem apices stained with mPS-PI after mock-treatment (G) or dexamethasone treatment (H,I); c, v, and p indicate cortex, vasculature and pith; (I) higher magnification of stem section similar to panels (H), with arrows indicating cells with the pitted walls characteristic of xylem elements and the top left arrow marking an opening between adjacent pitted cells. Scale bars: 50 μm (G,H), 20 μm (I). (J–O): In situ hybridisation experiments showing PXY expression after mock (J,L,M,O) or dex-treatment (K,N) of RPS5A:LhGR:PLL12 stem apices. All sections were incubated with PXY anti-sense probe except (L,O) (sense probe controls). (J–L) calcofluor images of the corresponding images shown in panels (M–O) respectively; in panel (J), phloem, cambium and xylem regions are enclosed in green, magenta and blue lines, respectively. The normal PXY expression pattern in the cambial and early xylem precursor cells [arrows in panels (J,M)] is disrupted after dexamethasone treatment, which also induces strong PXY expression in a subset of cortical cells [arrows in panels (K,N)]. Bars: 50 μm.
Ectopic lignification is one of the responses elicited by cell wall fragments during pathogen attack (Ferrari et al., 2013), so lignification could have been a defence response activated by pectin fragments produced when PLL12 was overexpressed. However, closer examination of the dexamethasone-treated apices revealed cortex cells with lignification in a pitted pattern and openings between adjacent cells (Figure 5I), similar to root metaxylem and to ectopic metaxylem cells induced by the regulators of xylem differentiation, VND6 and SND5 (Figures 5G–I; Kubo et al., 2005; Zhong et al., 2021). Pitted cells were also seen within the vascular bundles of plants with a mild phenotype after PLL12 induction, but not in uninduced controls (Supplementary Figure 5). Further supporting the idea that ectopic PLL12 expression re-directed cortex cells to vascular development, ectopic expression of the regulator of cambium development, PXY, was detected by in situ hybridisation after PLL12 induction (Figure 5N). Conversely, the normal PXY expression in the cambium of the wild type was disrupted in the disorganised vascular bundles seen after dexamethasone treatment (Figures 5J–O). Together with the inhibition of cambium and xylem growth in the pll12-1 mutant (Figure 3), the results above supported a role for PLL12 in the regulation of cambium and xylem development, although the experiments could not distinguish whether PLL12 promoted cambium and xylem identity sequentially, or separately in different cells.
The stronger effects of PLL12 overexpression close to the meristem and in cortex cells suggested that the response to a putative PLL12-produced signal depended on developmental stage and cell type. To study the effect of ectopic PLL12 in a different context, we also looked at seedlings. RPS5A:LhGR pOp:PLL12 plants were grown on medium without dexamethasone until the first pair of leaves emerged, then the seedlings were moved on to dexamethasone or mock treatment plates. Over the subsequent 7 days, a gradient of phenotypes became evident, ranging from small rosettes to stunted seedlings with severely deformed leaves (Figure 6), although root growth was initially unaffected (Supplementary Figure 6). In the most extreme cases, leaf blades failed to expand and became chlorotic (Figures 6D,E). Confocal imaging of these leaves stained with mPS-PI showed that the veins had irregular thickness, were discontinuous and showed cambium-like cell divisions along the leaf vasculature (Figures 6F–L). Thus, although ectopic PLL12 only appeared to induce extensive lignification in the inflorescence apex, it interfered with the differentiation of vascular cells both in the inflorescence and in seedlings.
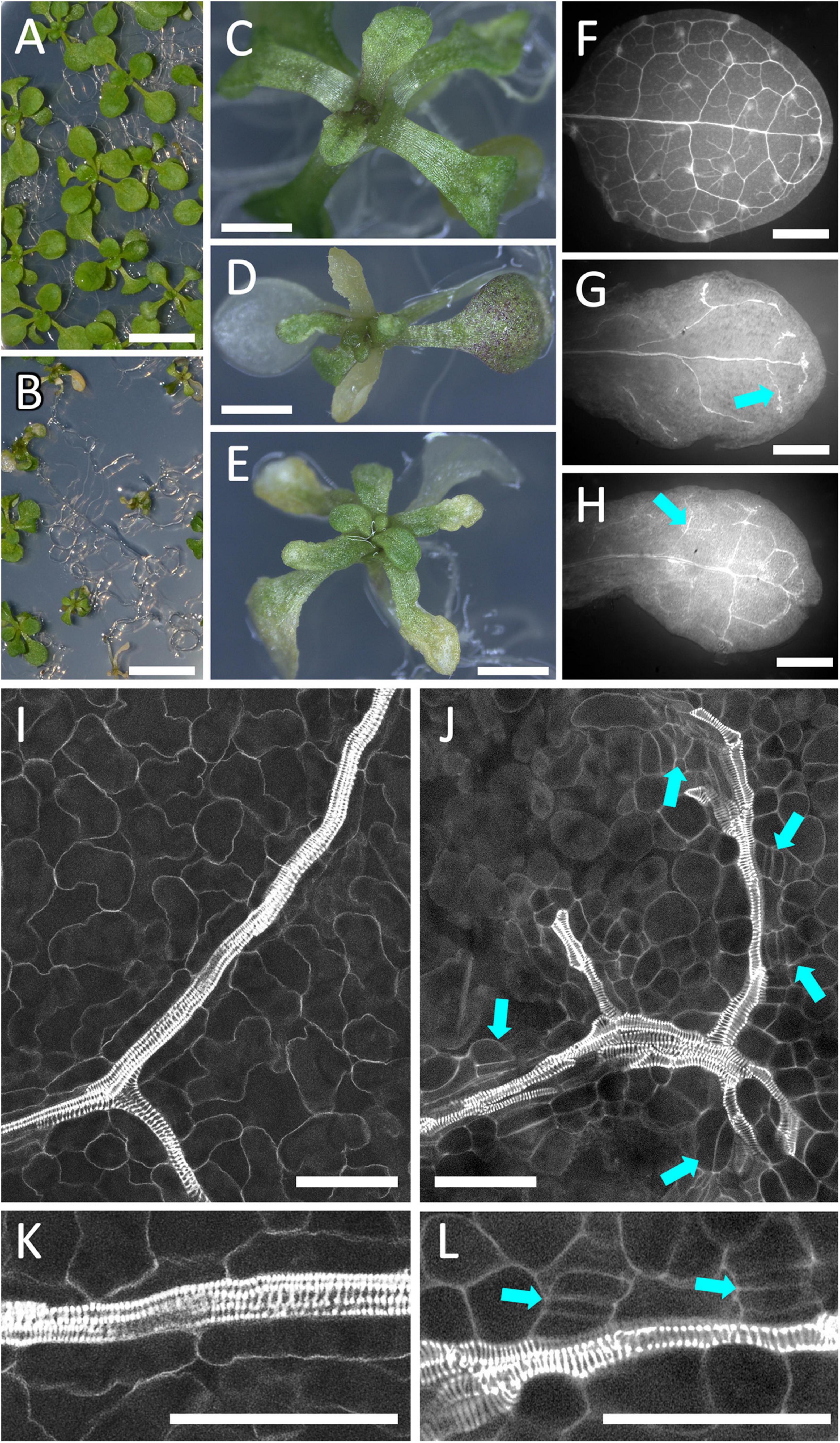
Figure 6. Over-expression of PLL12 disrupts the development of leaf vasculature. (A–E): pRPS5A:LhGR pOp:PLL12 plants growing for 4 days on medium containing dexamethasone (B,D,E) showed reduced growth and leaf chlorosis, in contrast to mock-treated plants (A,C); scale bars: 5 mm (A,B), 2 mm (C–E). (F–H): Low magnification images of mPS-PI-stained leaves from an uninduced control (F) and two seedlings grown for 4 days on dexamethasone-containing medium (G,H); arrows indicate interrupted, uneven vasculature seen after PLL2 induction; scale bars: 200 μm. (I–L): Confocal images of leaf veins from seedlings comparable to panels (A–H), grown without (I,K) or with dexamethasone (J,L); panels (K,L) are higher magnifications of panels (I,J); arrows indicate cell divisions oriented parallel to the veins, in a cambium-like pattern; scale bars: 50 μm.
Discussion
PLL12 has been shown to function in stomatal guard cells, where it affects cell wall properties and the speed of stomatal opening and closure (Chen et al., 2021). Here, we show that PLL12 is also required for the development and function of the stem vasculature. Loss of PLL12 function did not have obvious effects on patterning or cell differentiation, but inhibited growth of vascular bundles, with the earliest effects visible in the fascicular cambium, followed by reduced cell numbers in both phloem and xylem. The reduced cell numbers throughout the stem vascular bundles were accompanied by reduced transport of both water and sugar. The simplest interpretation of these phenotypes is that PLL12 function is limiting for the activity of the fascicular cambium, and consequently for the enlargement of vascular bundles during stem growth.
Despite the reduced growth across the vasculature (Figures 3G,H and Supplementary Table 2), PLL12 expression was specific to the phloem, in line with previous reports (Brady et al., 2007; Kalmbach et al., 2022). Thus, the effects on cambium and xylem growth were likely indirect consequences of a primary role of PLL12 in the phloem. In support of a phloem function for PLL12, a recent pre-print reports that pll12-1 has a defect in long-distance phloem transport, attributed to subtle changes in the formation of sieve plates between phloem elements (Kalmbach et al., 2022). However, defects in long-distance sugar transport would not readily account for the disproportionate effect of pll12-1 on the growth of established vascular bundles, in comparison to earlier stages. Moreover, a role exclusively in sieve plate formation would not easily explain why PLL12 overexpression induced ectopic cambium and xylem features and disrupted vascular differentiation in seedlings. To explain both loss- and gain-of-function phenotypes, and taking into account the phloem-specific expression, we propose that in addition to the reported role in sieve element development (Kalmbach et al., 2022), PLL12 activity influences an intercellular signal that coordinates development across vascular tissues.
PLL12 contains a canonical pectate lyase C domain, with conserved residues implicated in catalysis, Ca + + binding and exocytosis (Chen et al., 2021), so this protein is likely to cleave demethylesterified pectin within the cell wall. Accordingly, immunolocalisation showed increased levels of calcium-crosslinked pectin in pll12-1 stomatal walls (Chen et al., 2021) and mutation of the predicted pectate lyase catalytic site abolished the ability of PLL12 to complement the mutant (Kalmbach et al., 2022). Furthermore, PLL12 is homologous to a Zinnia PLL protein for which pectate lyase activity has been demonstrated in vitro (Domingo et al., 1998). Pectin cleavage would be expected to release oligogalacturonides (OGs), which bind to the WAK1 receptor and activate the cell wall integrity and pathogen defence signalling pathways, whose downstream responses include lignification (Caño-Delgado et al., 2003; Brutus et al., 2010; Ferrari et al., 2013). However, the induction of metaxylem-like pitted cell walls, combined with ectopic PXY expression, suggested that lignification after ectopic PLL12 expression was an aspect of vascular differentiation, rather than a generic defence or cell wall stress response.
Thus, the expected biochemical function of PLL12, together with its non-cell-autonomous effects and its ability to ectopically induce cambium and xylem identity, raise the possibility that a cell wall-derived signal participates in the regulation of vascular differentiation. The most straightforward candidate signal would be OGs, although their developmental roles remain speculative (Seifert and Blaukopf, 2010). Supporting a link to xylem differentiation, genes that respond early to OG treatment (Moscatiello et al., 2006) included SND5, which is part of the transcriptional network controlled by PXY (Smit et al., 2020) and encodes a NAC domain protein that regulates secondary wall deposition in the xylem (Zhong et al., 2021). However, indirect effects on other signalling pathways are also possible. External application of OGs has been shown to interfere with auxin signalling (Branca et al., 1988; Ferrari et al., 2013). However, the phenotypes caused by ectopic PLL12 activation were different from those seen in plants with an overall inhibition of auxin signalling; for example, there was little effect on roots, where auxin plays a central role (Israeli et al., 2020). The interrupted veins seen after ectopic PLL12 induction in cotyledons and leaves are also reminiscent of phenotypes seen after application or overexpression of the PXY ligand CLE41/44 (Hirakawa et al., 2008), or in mutants that affect phosphoinositide-regulated vesicle traffic, which may regulate auxin transport (Sieburth et al., 2006; Carland and Nelson, 2009). In the future, it will be interesting to explore how PLL12 function interacts with these signalling pathways.
Data Availability Statement
The original contributions presented in the study are included in the article/Supplementary Material, further inquiries can be directed to the corresponding author.
Author Contributions
RS: conceptualisation, software, funding acquisition, and supervision. MB and VS: investigation. MB and RS: writing—original draft. All authors: formal analysis, data curation, and writing—review and editing.
Funding
This work was supported by BBSRC grants BB/M003825/1, BB/S005714/1, and BBS/E/J/000PR9787.
Conflict of Interest
The authors declare that the research was conducted in the absence of any commercial or financial relationships that could be construed as a potential conflict of interest.
Publisher’s Note
All claims expressed in this article are solely those of the authors and do not necessarily represent those of their affiliated organizations, or those of the publisher, the editors and the reviewers. Any product that may be evaluated in this article, or claim that may be made by its manufacturer, is not guaranteed or endorsed by the publisher.
Acknowledgments
We thank the European Arabidopsis Stock Centre for seeds, G. Calder and E. Wegel for microscopy support, and members of the Sablowski laboratory past and present for helpful discussions.
Supplementary Material
The Supplementary Material for this article can be found online at: https://www.frontiersin.org/articles/10.3389/fpls.2022.888201/full#supplementary-material
Footnotes
References
Banasiak, A., Biedroń, M., Dolzblasz, A., and Berezowski, M. A. (2019). Ontogenetic changes in auxin biosynthesis and distribution determine the organogenic activity of the shoot apical meristem in pin1 mutants. Internat. J. Mole. Sci. 20:180. doi: 10.3390/ijms20010180
Barratt, D. H. P., Kölling, K., Graf, A., Pike, M., Calder, G., Findlay, K., et al. (2010). Callose Synthase GSL7 Is Necessary for Normal Phloem Transport and Inflorescence Growth in Arabidopsis. Plant Physiol. 155, 328–341. doi: 10.1104/pp.110.166330
Bencivenga, S., Serrano-Mislata, A., Bush, M., Fox, S., and Sablowski, R. (2016). Control of Oriented Tissue Growth through Repression of Organ Boundary Genes Promotes Stem Morphogenesis. Dev. Cell 39, 198–208. doi: 10.1016/j.devcel.2016.08.013
Bezrutczyk, M., Hartwig, T., Horschman, M., Char, S. N., Yang, J., Yang, B., et al. (2018). Impaired phloem loading in zmsweet13a, b, c sucrose transporter triple knock-out mutants in Zea mays. New Phytol. 218, 594–603. doi: 10.1111/nph.15021
Bonke, M., Thitamadee, S., Mähönen, A. P., Hauser, M.-T., and Helariutta, Y. (2003). APL regulates vascular tissue identity in Arabidopsis. Nature 426, 181–186. doi: 10.1038/nature02100
Brady, S. M., Orlando, D. A., Lee, J.-Y., Wang, J. Y., Koch, J., Dinneny, J. R., et al. (2007). A high-resolution root spatiotemporal map reveals dominant expression patterns. Science 318, 801–806. doi: 10.1126/science.1146265
Branca, C., Lorenzo, G. D., and Cervone, F. (1988). Competitive inhibition of the auxin-induced elongation by α-D-oligogalacturonides in pea stem segments. Physiol. Plant. 72, 499–504. doi: 10.1111/j.1399-3054.1988.tb09157.x
Brutus, A., Sicilia, F., Macone, A., Cervone, F., and De Lorenzo, G. (2010). A domain swap approach reveals a role of the plant wall-associated kinase 1 (WAK1) as a receptor of oligogalacturonides. Proc. Natl. Acad. Sci. 107, 9452–9457. doi: 10.1073/pnas.1000675107
Byrne, M. E., Groover, A. T., Fontana, J. R., and Martienssen, R. A. (2003). Phyllotactic pattern and stem cell fate are determined by the Arabidopsis homeobox gene BELLRINGER. Development 130, 3941–3950. doi: 10.1242/dev.00620
Caño-Delgado, A., Penfield, S., Smith, C., Catley, M., and Bevan, M. (2003). Reduced cellulose synthesis invokes lignification and defense responses in Arabidopsis thaliana. Plant J. 34, 351–362. doi: 10.1046/j.1365-313x.2003.01729.x
Carland, F., and Nelson, T. (2009). CVP2-and CVL1-mediated phosphoinositide signaling as a regulator of the ARF GAP SFC/VAN3 in establishment of foliar vein patterns. Plant J. 59, 895–907. doi: 10.1111/j.1365-313X.2009.03920.x
Carlsbecker, A., Lee, J.-Y., Roberts, C. J., Dettmer, J., Lehesranta, S., Zhou, J., et al. (2010). Cell signalling by microRNA165/6 directs gene dose-dependent root cell fate. Nature 465, 316–321. doi: 10.1038/nature08977
Chen, Y., Li, W., Turner, J. A., and Anderson, C. T. (2021). PECTATE LYASE LIKE12 patterns the guard cell wall to coordinate turgor pressure and wall mechanics for proper stomatal function in Arabidopsis. Plant Cell 33, 3134–3150. doi: 10.1093/plcell/koab161
Clough, S. J., and Bent, A. F. (1998). Floral dip: a simplified method for Agrobacterium-mediated transformation of Arabidopsis thaliana. Plant J. 16, 735–743. doi: 10.1046/j.1365-313x.1998.00343.x
Domingo, C., Roberts, K., Stacey, N. J., Connerton, I., Ruíz-Teran, F., and Mccann, M. C. (1998). A pectate lyase from Zinnia elegans is auxin inducible. Plant J. 13, 17–28. doi: 10.1046/j.1365-313x.1998.00002.x
Engler, C., Youles, M., Gruetzner, R., Ehnert, T.-M., Werner, S., Jones, J. D., et al. (2014). A golden gate modular cloning toolbox for plants. ACS Synthet. Biol. 3, 839–843. doi: 10.1021/sb4001504
Etchells, J. P., Moore, L., Jiang, W. Z., Prescott, H., Capper, R., Saunders, N. J., et al. (2012). A role for BELLRINGER in cell wall development is supported by loss-of-function phenotypes. BMC Plant Biol. 12:212–212. doi: 10.1186/1471-2229-12-212
Ferrari, S., Savatin, D., Sicilia, F., Gramegna, G., Cervone, F., and De Lorenzo, G. (2013). Oligogalacturonides: plant damage-associated molecular patterns and regulators of growth and development. Front. Plant Sci. 2013:4. doi: 10.3389/fpls.2013.00049
Fisher, K., and Turner, S. (2007). PXY, a receptor-like kinase essential for maintaining polarity during plant vascular-tissue development. Curr. Biol. 17, 1061–1066. doi: 10.1016/j.cub.2007.05.049
Furuta, K. M., Yadav, S. R., Lehesranta, S., Belevich, I., Miyashima, S., Heo, J.-O., et al. (2014). Arabidopsis NAC45/86 direct sieve element morphogenesis culminating in enucleation. Science 345, 933–937. doi: 10.1126/science.1253736
Hajdukiewicz, P., Svab, Z., and Maliga, P. (1994). The small, versatile pPZP family of Agrobacterium binary vectors for plant transformation. Plant Mole. Biol. 25, 989–994. doi: 10.1007/BF00014672
Hirakawa, Y., Shinohara, H., Kondo, Y., Inoue, A., Nakanomyo, I., Ogawa, M., et al. (2008). Non-cell-autonomous control of vascular stem cell fate by a CLE peptide/receptor system. Proc. Natl. Acad. Sci. 105:15208. doi: 10.1073/pnas.0808444105
Israeli, A., Reed, J. W., and Ori, N. (2020). Genetic dissection of the auxin response network. Nat. Plants 6, 1082–1090. doi: 10.1038/s41477-020-0739-7
Ito, Y., Nakanomyo, I., Motose, H., Iwamoto, K., Sawa, S., Dohmae, N., et al. (2006). Dodeca-CLE peptides as suppressors of plant stem cell differentiation. Science 313, 842–845. doi: 10.1126/science.1128436
Kalmbach, L., Bourdon, M., Heo, J.-O., Otero, S., Blob, B., and Helariutta, Y. (2022). Pectin cell wall remodeling through PLL12 and callose deposition through polar CALS7 are necessary for long-distance phloem transport. bioRxiv 2022:478312.
Kalmbach, L., and Helariutta, Y. (2019). Sieve plate pores in the phloem and the unknowns of their formation. Plants 8:25. doi: 10.3390/plants8020025
Kondo, Y., Nurani, A. M., Saito, C., Ichihashi, Y., Saito, M., Yamazaki, K., et al. (2016). Vascular Cell Induction Culture System Using Arabidopsis Leaves (VISUAL) Reveals the Sequential Differentiation of Sieve Element-Like Cells. Plant Cell 28, 1250–1262. doi: 10.1105/tpc.16.00027
Kubo, M., Udagawa, M., Nishikubo, N., Horiguchi, G., Yamaguchi, M., Ito, J., et al. (2005). Transcription switches for protoxylem and metaxylem vessel formation. Genes Dev. 19, 1855–1860. doi: 10.1101/gad.1331305
Mitra, P. P., and Loqué, D. (2014). Histochemical staining of Arabidopsis thaliana secondary cell wall elements. JoVE 2014, e51381. doi: 10.3791/51381
Mitsuda, N., Iwase, A., Yamamoto, H., Yoshida, M., Seki, M., Shinozaki, K., et al. (2007). NAC transcription factors, NST1 and NST3, are key regulators of the formation of secondary walls in woody tissues of Arabidopsis. Plant Cell 19, 270–280. doi: 10.1105/tpc.106.047043
Miyashima, S., Roszak, P., Sevilem, I., Toyokura, K., Blob, B., Heo, J.-O., et al. (2019). Mobile PEAR transcription factors integrate positional cues to prime cambial growth. Nature 565, 490–494. doi: 10.1038/s41586-018-0839-y
Moscatiello, R., Mariani, P., Sanders, D., and Maathuis, F. J. M. (2006). Transcriptional analysis of calcium-dependent and calcium-independent signalling pathways induced by oligogalacturonides. J. Exp. Bot. 57, 2847–2865. doi: 10.1093/jxb/erl043
Nieminen, K., Blomster, T., Helariutta, Y., and Mähönen, A. P. (2015). Vascular cambium development. Arabidop. Book/Am. Soc. Plant Biol. 2015:13.
Peaucelle, A., Louvet, R., Johansen, J. N., Salsac, F., Morin, H., Fournet, F., et al. (2011). The transcription factor BELLRINGER modulates phyllotaxis by regulating the expression of a pectin methylesterase in Arabidopsis. Development 138, 4733–4741. doi: 10.1242/dev.072496
Rebocho, A. B., Southam, P., Kennaway, J. R., Bangham, J. A., and Coen, E. (2017). Generation of shape complexity through tissue conflict resolution. Elife 6:e20156. doi: 10.7554/eLife.20156
Roeder, A. H. K., Ferrandiz, C., and Yanofsky, M. F. (2003). The role of the REPLUMLESS homeodomain protein in patterning the Arabidopsis fruit. Curr. Biol. 13, 1630–1635. doi: 10.1016/j.cub.2003.08.027
Ruonala, R., Ko, D., and Helariutta, Y. (2017). Genetic networks in plant vascular development. Annu. Rev. Genet. 51, 335–359. doi: 10.1146/annurev-genet-120116-024525
Schiessl, K., Kausika, S., Southam, P., Bush, M., and Sablowski, R. (2012). JAGGED controls growth anisotropy and coordination between cell size and cell cycle during plant organogenesis. Curr. Biol. 22, 1739–1746. doi: 10.1016/j.cub.2012.07.020
Schindelin, J., Arganda-Carreras, I., Frise, E., Kaynig, V., Longair, M., Pietzsch, T., et al. (2012). Fiji: an open-source platform for biological-image analysis. Nat. Methods 9, 676–682. doi: 10.1038/nmeth.2019
Seifert, G. J., and Blaukopf, C. (2010). Irritable Walls: the Plant Extracellular Matrix and Signaling. Plant Physiol. 153, 467–478. doi: 10.1104/pp.110.153940
Sénéchal, F., Wattier, C., Rustérucci, C., and Pelloux, J. (2014). Homogalacturonan-modifying enzymes: structure, expression, and roles in plants. J. Exp. Bot. 65, 5125–5160. doi: 10.1093/jxb/eru272
Serrano-Mislata, A., Schiessl, K., and Sablowski, R. (2015). Active control of cell size generates spatial detail during plant organogenesis. Curr. Biol. 25, 2991–2996. doi: 10.1016/j.cub.2015.10.008
Sieburth, L. E., Drews, G. N., and Meyerowitz, E. M. (1998). Non-autonomy of AGAMOUS function in flower development: use of a Cre/loxP method for mosaic analysis in Arabidopsis. Development 125, 4303–4312. doi: 10.1242/dev.125.21.4303
Sieburth, L. E., Muday, G. K., King, E. J., Benton, G., Kim, S., Metcalf, K. E., et al. (2006). SCARFACE Encodes an ARF-GAP That Is Required for Normal Auxin Efflux and Vein Patterning in Arabidopsis. Plant Cell 18, 1396–1411. doi: 10.1105/tpc.105.039008
Smit, M. E., Mcgregor, S. R., Sun, H., Gough, C., Bågman, A.-M., Soyars, C. L., et al. (2020). A PXY-mediated transcriptional network integrates signaling mechanisms to control vascular development in Arabidopsis. Plant Cell 32, 319–335. doi: 10.1105/tpc.19.00562
Smith, H. M. S., and Hake, S. (2003). The interaction of two homeobox genes, BREVIPEDICELLUS and PENNYWISE, regulates internode patterning in the Arabidopsis inflorescence. Plant Cell 15, 1717–1727. doi: 10.1105/tpc.012856
Smyth, D. R., Bowman, J. L., and Meyerowitz, E. M. (1990). Early flower development in Arabidopsis. Plant Cell 2, 755–767. doi: 10.2307/3869174
Speer, E. (1987). A method of retaining phloroglucinol proof of lignin. Stain Technol. 62, 279–280. doi: 10.3109/10520298709108008
Sugiyama, Y., Wakazaki, M., Toyooka, K., Fukuda, H., and Oda, Y. (2017). A Novel Plasma Membrane-Anchored Protein Regulates Xylem Cell-Wall Deposition through Microtubule-Dependent Lateral Inhibition of Rho GTPase Domains. Curr. Biol. 27, 2522.e–2528.e. doi: 10.1016/j.cub.2017.06.059
Sun, H., Hao, P., Gu, L., Cheng, S., Wang, H., Wu, A., et al. (2020). Pectate lyase-like Gene GhPEL76 regulates organ elongation in Arabidopsis and fiber elongation in cotton. Plant Sci. 293:110395. doi: 10.1016/j.plantsci.2019.110395
Sun, L., and Van Nocker, S. (2010). Analysis of promoter activity of members of the PECTATE LYASE-LIKE (PLL) gene family in cell separation in Arabidopsis. BMC Plant Biol. 10, 1–13. doi: 10.1186/1471-2229-10-152
Uluisik, S., and Seymour, G. B. (2020). Pectate lyases: their role in plants and importance in fruit ripening. Food Chem. 309:125559. doi: 10.1016/j.foodchem.2019.125559
Wang, Y., Wang, J., Shi, B., Yu, T., Qi, J., Meyerowitz, E. M., et al. (2014). The Stem Cell Niche in Leaf Axils Is Established by Auxin and Cytokinin in Arabidopsis. Plant Cell 26, 2055–2067. doi: 10.1105/tpc.114.123083
Wolf, S., Hématy, K., and Höfte, H. (2012). Growth control and cell wall signaling in plants. Annu. Plant Biol. 63, 381–407. doi: 10.1146/annurev-arplant-042811-105449
Yang, Y., Yu, Y., Liang, Y., Anderson, C. T., and Cao, J. (2018). A Profusion of Molecular Scissors for Pectins: classification, Expression, and Functions of Plant Polygalacturonases. Front. Plant Sci. 2018:9. doi: 10.3389/fpls.2018.01208
Keywords: Arabidopsis, shoot development, pectate lyase, phloem, cambium, xylem
Citation: Bush M, Sethi V and Sablowski R (2022) A Phloem-Expressed PECTATE LYASE-LIKE Gene Promotes Cambium and Xylem Development. Front. Plant Sci. 13:888201. doi: 10.3389/fpls.2022.888201
Received: 02 March 2022; Accepted: 17 March 2022;
Published: 26 April 2022.
Edited by:
Cristina Ferrandiz, Polytechnic University of Valencia, SpainReviewed by:
Peter Etchells, Durham University, United KingdomRosemary White, Commonwealth Scientific and Industrial Research Organisation (CSIRO), Australia
Copyright © 2022 Bush, Sethi and Sablowski. This is an open-access article distributed under the terms of the Creative Commons Attribution License (CC BY). The use, distribution or reproduction in other forums is permitted, provided the original author(s) and the copyright owner(s) are credited and that the original publication in this journal is cited, in accordance with accepted academic practice. No use, distribution or reproduction is permitted which does not comply with these terms.
*Correspondence: Robert Sablowski, cm9iZXJ0LnNhYmxvd3NraUBqaWMuYWMudWs=
†Present address: Vishmita Sethi, Indian Institute of Science Education and Research, Kolkata, India
‡These authors have contributed equally to this work