- 1Instituto Andaluz de Investigación y Formación Agraria (IFAPA) Centro de Málaga, Málaga, Spain
- 2Universidad de Málaga, Málaga, Spain
- 3Instituto Andaluz de Investigación y Formación Agraria (IFAPA) Centro La Mojonera, Almería, Spain
The recurrent emergence of viral diseases in intensive horticultural crops requires alternative control strategies. The topical application of double-stranded RNA (dsRNA) molecules homologous to pathogens has been proposed as a tool for virus control in plants. These dsRNAs induce the silencing mechanism, the RNA interference (RNAi), that degrades homologous dsRNAs. Cucumber green mottle mosaic virus (CGMMV) represents a serious threat to cucurbit crops. Since genetic resistance to the virus is not yet available in commercial varieties, we aimed to control this virus by RNAi. For this purpose, we obtained constructions both for expressing dsRNA in bacteria to treat cucumber plants by topical application and for agroinoculation in experiments done in the growth chamber. Besides, greenhouse tests were performed in spring and in summer when plants were challenged with the virus, and differences in several parameters were investigated, including the severity of symptoms, dry weight, total height, virus accumulation, and virus-derived small interfering RNAs (vsiRNAs). Spraying of plants with dsRNA reduced significatively CGMMV symptoms in the plants in growth chamber tests. Agroinfiltration experiments done under identical conditions were also effective in limiting the progress of CGMMV disease. In the greenhouse assay performed in spring, symptoms were significantly reduced in dsRNA-sprayed plants, and the development of the plants improved with respect to non-treated plants. Virus titers and vsiRNAs were clearly reduced in dsRNA-treated plants. The effect of protection of the dsRNA was less evident in the greenhouse assay carried out in the summer. Besides, we investigated the mobility of long (ds)RNA derived from spraying or agroinfiltrated dsRNA and found that it could be detected in local, close distal, and far distal points from the site of application. VsiRNAs were also detected in local and distal points and the differences in accumulation were compared. In parallel, we investigated the capacity of dsRNAs derived from genes of tomato leaf curl New Delhi virus (ToLCNDV), another economically important virus in cucurbits, to limit the disease in zucchini, both by agroinfiltration or by direct spraying, but found no protective effect. In view of the results, the topical application of dsRNAs is postulated as a promising strategy for CGMMV control in the cucumber.
Introduction
Food production faces a dual challenge: to increase by 60% in order to feed the estimated global human population of 10 billion people expected by 2050, while reducing the overall loss of food due to pests and pathogens which now range between 17 and 30% depending on the crop species [Food and Agriculture Organization of the United Nations (FAO), 2019; Savary et al., 2019]. Current approaches to the management of these pathogens and diseases are based on the use of chemicals, insecticides, and fungicides or on the development of genetic resistance to diseases and/or on the generation of transgenic cultivars. Legislation and consumer demands urge the use of sustainable pest and disease control, and the development of alternatives to improve crop yields as the pressure on global nutrition grows every year (Cagliari et al., 2019). In particular, the increasing number of emerging viral diseases require alternative control strategies (Velasco et al., 2020). As one of such recently developed plant protection tools, the exogenous application of dsRNA to induce RNA interference (RNAi) is one key step-change that can impact the way we will protect crops from pathogen attack in the future (Tenllado et al., 2003, 2004; Mitter et al., 2017b; Niehl et al., 2018; Fletcher et al., 2020). RNAi refers to a natural regulatory mechanism in gene expression. This mechanism was discovered in Caenorhabditis elegans in 1998 and since then great advances have been made in its potential applications for the control of plant pathogens (Fire et al., 1998; Pugsley et al., 2021). It is mediated mainly by two types of molecules, small-interfering RNAs (siRNAs) and microRNAs (miRNAs) (Sanan-Mishra et al., 2021). Whereas, the former is endogenously-derived and involved in the regulation of gene expression, small RNAs can be of exogenous origin from viruses or artificial supply (Matranga and Zamore, 2007), endogenous derived from transposons (Golden et al., 2008), from overlapping transcripts, such as natural antisense siRNAs (nat-siRNAs), or secondary siRNAs-phased siRNAs (phasiRNAs), and transacting siRNAs (tasiRNAs) (Ivanova et al., 2022). The presence of exogenous double-stranded RNA (dsRNA) generates the activation of DICER and RISC complexes that cleave dsRNA and use the siRNAs as a template for the degradation of complementary RNAs (Vargason et al., 2003; Pantaleo et al., 2007). This mechanism is known as post-transcriptional gene silencing (PTGS) in plants and is the way for plants to cope with viral genomes, either by inducing immunity or recovery from infection, depending on whether PTGS occurs before or after viral infection (Vaucheret et al., 2001; Pooggin et al., 2003). The major challenge of RNAi application in plants is to cross the cell wall and reach the cell interior. Topical application of free dsRNA/siRNA for virus control has been shown effective in several pathosystems, but prior to reaching the market, several issues require to be addressed, including improving the formulations (Cagliari et al., 2019; Ricci et al., 2021). Hence, some authors have proposed the use of nanoparticles (NPs) to improve the delivery conditions of dsRNA to the cellular interior, release dsRNA in a controlled manner, and achieve protection against the virus for 20 days (Mitter et al., 2017a).
Cucumber green mottle mosaic virus (CGMMV) belongs to the genus Tobamovirus and the family Virgaviridae (Adams et al., 2012). It was described as the first-known tobamovirus that infects cucurbits, by Ainsworth (1935), as a severe threat to cucumbers. It causes severe symptoms including leaf mottle mosaic, leaf blistering, stunted growth, and distortion in fruits and leaves in cucumber but remains symptomless in zucchini (Cucurbita pepo L.) (Crespo et al., 2017, 2019). The CGMMV has a 6.4 kb single-stranded, positive-sense RNA genome encapsidated within ~2,000 molecules of a single species of capsid protein particles, forming rigid rods ca 300 × 18 nm with a helical structure (Hollings et al., 1975). The genomic RNA contains four open reading frames (ORFs) that encode four defined proteins. Two polypeptides are necessary for its replication complex. First, a 129 kDa polypeptide containing methyltransferase and helicase motifs is required for RNA replication. Second, a long 186-kDa polypeptide formed by the suppression of a UAG termination codon encodes an RNA-dependent RNA polymerase at its carboxyl-terminal domain (Crespo et al., 2017). Two additional proteins are translated from subgenomic mRNAs corresponding to ORFs in the 3′ half of the genomic RNA (Dombrovsky et al., 2017). It is a seed mechanically transmitted and is characterized by its stability and ability to persist for long periods without a host. The CGMMV is responsible for extensive damage to cucurbit crops, including Spain, which is one of the leading producers in Europe (Crespo et al., 2017). CGMMV causes systemic symptoms, such as mottling, mosaic, blistering, leaf stunting, and dwarfing (Mandal et al., 2008) in cucumber. No effective genetic resistance to CGMMV is yet available in commercial cucurbits, and the control of the virus consists in limiting the spread in the field through management practices, such as removing the infected material and solarization of the soil and the use of healthy plant material and seeds.
Tomato leaf curl New Delhi virus (ToLCNDV) is an ssDNA virus with a bipartite genome that belongs to the genus Begomovirus. It has two genome components, named DNA-A and DNA-B (Padidam et al., 1996). DNA-A contains the AV1 (encoding a coat protein) and AV2 genes in the virion sense orientation, and AC1 (coding a virus replication-associated protein), AC2 (encoding a replication enhancer protein), AC3 (coding a transcriptional activator protein), and AC4 in the complementary sense orientation (Padidam et al., 1996). ToLCNDV DNA-B contains the BV1 gene (coding a nuclear shuttle protein) in the virion sense orientation, and the BC1 gene (coding a movement protein) in the complementary sense orientation (Fondong, 2013). The virus is transmitted by the whitefly Bemisia tabaci and affects crops from the Solanaceae and Cucurbitaceae families, causing severe economic losses worldwide. This virus is mainly present in Asia, but recently, a variant has spread to the Mediterranean basin, affecting mainly zucchini, cucumber, and melon, and has tomato as a reservoir host (Fortes et al., 2016; Janssen et al., 2022). In Spain, ToLCNDV infection produces severe symptoms in cucurbits, such as curling and distortion of leaves, green and yellow mosaics, and other deformations as well as stunting of the plant. Control of the virus has been achieved by controlling the vector and progress is being made in search for resistance sources for breeding commercial cultivars (Sáez et al., 2016; Tellez et al., 2017). In this study, we evaluated the effect of the exogenous application of homologous dsRNA derived from CGMMV and ToLCNDV in cucumber and zucchini, respectively, to elicit natural RNAi response and eventually resistance to these diseases.
Materials and Methods
Nucleic Acid Extraction and the Obtention of Constructions for the Generation of dsRNAs
Total RNA was extracted from young cucumber leaves infected with the Asian isolate of CGMMV (CGSPCu16) detected in Spain (Crespo et al., 2017), using the Spectrum Plant Total RNA Kit (Sigma, Spain) and following the manufacturer's instructions. Synthesis of cDNA by reverse transcription was performed in 20 μl reactions with 200 ng of total RNA with the high-capacity cDNA reverse transcription kit (Applied Biosystems, USA). For the subsequent PCRs, primer pairs that included attB adaptors were designed based on the cp (coat protein gene) and mp (movement protein gene) sequences of CGMMV CGSPCu16 (Supplementary Table 1; Delgado-Martín and Velasco, 2021). PCR reactions were carried out with the AmpliTools master mix (Biotools, Spain) using 50 pmol each of forward and reverse primers in the following conditions: an initial denaturing cycle of 2 min at 94°C, then 40 cycles of 30 s at 95°C, 30 s at 55°C, and 40 s at 72°C, and a final extension step of 5 min at 72°C. The amplicons were cloned into vector pDONR221 using the BP clonase (Thermo Scientific, Spain) and Escherichia coli. Top10 cells were transformed and selected in Luria broth (LB) supplemented with kanamycin. The plasmids obtained, pENT-CP and pENT-MP, were sequenced in order to check the correctness of the insertions. Next, the CP or MP fragments were cloned into vector L4440gtwy that includes attR sites flanked by T7 promoters (G. Caldwell, Addgene plasmid # 11,344) using LR clonase (Thermo Scientific, Spain). The obtained plasmids, pL4440-CP and pL4440-MP, were used to transform E. coli HT115(DE3) cells that were selected in LB supplemented with ampicillin. E. coli HT115(DE3) contains the T7 RNA polymerase gene in its chromosome under the lac promoter and lacks RNAse III activity. For the constructions derived from ToLCNDV, we used a plant infected with the infective clone of ToLCNDV-ES (Ruiz et al., 2017). Total DNA was extracted from the plant with the GeneJet Plant Genomic DNA purification kit (Thermo Scientific, Spain) and used to produce amplicons from the genes, AV1 and BC1, flanked by attB sites (Supplementary Table 1). The amplicons were used to obtain plasmids, L4440-AV1 and L4440-BC1, following a similar strategy to that described above. These plasmids were used to transform E. coli HT115 for the synthesis of the AV1- and BC1-dsRNAs.
In vivo Production of dsRNAs in E. coli HT115
Escherichia coli HT115(DE3) cells that contained plasmids pL4440-CP, -MP, -AV1, or -BC1 were grown in LB supplemented with ampicillin, and IPTG was used as an inducer of the expression of the T7 RNA polymerase gene. For dsRNA production, 4 ml of the bacteria were grown overnight at 37°C in LB plus ampicillin. One milligram of the culture was used to inoculate 250 ml flasks that contained 100 ml of LB, ampicillin (100 μg/mL), and IPTG (1 mM). The cells were grown for about 6 h at 37°C, when optical density (OD) determined the steady-state of the growth. The dsRNA was isolated from E. coli cells using phenolic extractions. Briefly, E. coli cells were collected after centrifugation in Falcon tubes at 8,000 × g, then resuspended using the vortex in 4 ml of Trisure (Bioline Iberia, Spain), and the lysis was performed after incubation for 5 min at room temperature. Next, 0.2 ml of chloroform was added and mixed up and for phase separation, samples were centrifuged in cold at 12,000 × g for 15 min. The upper phase was collected in new tubes and the nucleic acids were precipitated with cold isopropanol. Afterward, the nucleic acids were resuspended in MilliQ water and examined in 1% agarose gels stained with RedSafe (iNtRON Biotechnology, South-Korea) under UV light.
Constructs for Agroinoculation
Plasmid pHellsgate 8 (Helliwell and Waterhouse, 2003) was the backbone vector for cloning the virus segments in direct and reverse directions separated by a hairpin. The plasmid was digested with XhoI and XbaI (New England Biolabs, UK). Next, amplicons were obtained using the cDNA from CGMMV and ToLCNDV and the primers described in Supplementary Table 2. The amplicons, derived from the cp and mp genes of CGMMV and the AV1 and BC1 genes from ToLCNDV were used for Gibson assembly to pHellsgate 8. For each construction, four segments were assembled following the manufacturer's recommendations (New England Biolabs, UK), including the virus gene(s) with direct and reverse positions and the corresponding segments for the XbaI-XhoI digestions of pHellsgate8 vector that included the hairpin. The resulting plasmids, pGHE-CP, pGHE-MP, pGHE-AV1, and pGHE-BC1 were used to transform Top10 E. coli cells that were grown in LB plates supplemented with streptomycin (100 μg/ml). Plasmids were extracted from the cells and checked by restriction fragment analysis and Sanger sequencing. The plasmids were then transferred to Agrobacterium tumefaciens LBA4404 by electroporation and selected with the same antibiotic.
Agroinoculation Procedures
For agroinoculation with the Agrobacterium tumefaciens LBA4404 strain carrying either pGHE-CP/pGHE-MP in cucumber or pGHE-AV1/pGHE-BC1 in zucchini, one colony of each clone was collected in 5 mL of liquid LB and streptomycin and grown overnight at 30°C with constant shaking. Next, the cells were harvested by centrifugation at 3,000 × g for 10 min at 4°C and resuspended in an infiltration medium (10 mM MES pH 5.8, 200 μM acetosyringone) adjusting the OD600 to 0.5. The agroinoculation in the abaxial side of fully expanded leaves and/or cotyledons of the plants was performed using a needleless 2 ml syringe. Virus infection and symptom inspection were performed as described above.
Plant Material, Inoculum Source, and Data Analyses
Cucumis sativus cv, “Bellpuig” (Fitó, Spain) was used in all CGMMV experiments. In growth chamber experiments (phytotron), the plants were treated with the CP/MP-dsRNAs using several different approaches at two fully expanded leaves. In two separate greenhouse experiments, one performed in the month of April, and a second in July of 2021, plants were treated with the CP-dsRNA at three fully expanded leaves. The dsRNA was resuspended in water and applied either by rubbing on two contiguous leaves using 60 μg of total RNA/leaf mixed with carborundum powder (400 mesh, Aldrich) or alternatively by spraying the same amount of total RNA, without the abrasive, using an artist airbrush at 2.5 bar pressure. After the dsRNA treatments, the virus was mechanically inoculated in the cotyledons as described elsewhere (Crespo et al., 2018). For the virus challenge, 1 g of leaf material from a Cochliobolus sativus plant infected with the Asian isolate of CGMMV (CGSPCu16) was used as an inoculum source (Crespo et al., 2017). The plant tissue was crushed in A cold buffer with a pestle and mortar in phosphate buffer (5 mM, pH 7) and activated charcoal. Prior to inoculation, carborundum powder was applied to the cotyledons. At 18–25 days post-inoculation (dpi), symptoms (Supplementary Figure 1) and the total length of the plants were recorded and rated. The dry weight of the complete plants was registered for comparison. Samples were taken for RNA extraction and virus and virus-derived small interfering RNAs (vsiRNAs) quantitation. In the greenhouse experiments, three groups of fifteen plants were used in each experimental condition: mock-treated plants, non-inoculated plants, and dsRNA-treated plants. The effect of virus-species-specific dsRNAs on plants infected with ToLCNDV was studied in groups of 10 zucchini (Cucurbita pepo cv. “Victoria,” Clause, Spain) plants. Treatments consisted of rubbing with 120 μg each of the AV1- and BC1-dsRNA. Infection was done using viruliferous whiteflies reared on plants previously infected with ToLCNDV-ES. Another group of 10 plants was inoculated but not treated and used as a control. Plants were kept in the growth chamber and symptoms were inspected at 18 dpi. The statistical analyses of the data were conducted by ANOVA followed by mean separation using the post-hoc Tukey's (equal variances) test as available in JAMOVI v.2.2.3 statistical suite1.
Small RNA Sequencing and Analysis
RNA extracts of CP-dsRNA-treated and mock (non-treated) plants from the greenhouse assay performed in spring, were pooled in two independent samples (DS and MO, respectively). RNA samples (NI) were additionally obtained from dsRNA-treated leaves from a pool of six non-infected plants. The small RNA fractions were excised from gels and the cDNA libraries were prepared for high-throughput sequencing (HTS) as single reads with the Illumina platform using the services provided by Sistemas Genómicos (Valencia, Spain). The Illumina sequencing adapter was trimmed off from the raw sequences, and reads between 18 and 24 nt in length were used in the subsequent analyses. The small RNA populations were aligned to the indexed CGMMV CGSPCu16 genomic sequence using the Bowtie2 module (Langmead and Salzberg, 2012) as available in Geneious (Biomatters). The BAM alignment files produced were processed using MISIS-2 (Seguin et al., 2016) and the tables generated were graphically represented in Veusz2.
CGMMV Quantitation by RT-qPCR
A hole punch was used to obtain ~100 mg of leaf tissue from each sample (15 biological replicates per condition), and the total RNAs were extracted using the Spectrum Plant RNA kit (Merck, Spain). RNAs were quantified using the NanoDrop ND-1000 (Thermo Fisher Scientific, Waltham, MA, USA). Subsequently, cDNA was produced using 2 μg of the total RNA extract from each plant and the High-Capacity cDNA Reverse Transcription in 20 μl reaction volume following the manufacturers' instructions. Each qPCR reaction (20 μl final volume), in triplicate, contained 1 μl of the cDNA, 10 μl of KAPA SYBR Green qPCR mix (KAPA Biosystems, MA, USA), and 500 nM of each CGMMV cp or mp primers (Supplementary Table 2). In separate reactions, we included the primers for the C. sativus 18S rRNA gene as a reference. The specificity of the amplicons obtained was checked with the Bio-Rad Optical System Software v.2.1 by means of melting-curve analyses (60 s at 95°C and 60 s at 55°C), followed by fluorescence measurements (from 55 to 95°C, with increments by 0.5°C). The geometric mean of their expression ratios was used as the normalization factor in all samples for measuring the quantification cycle (Cq). The relative expressions of the CGMMV amounts were calculated based on the comparative Cq (2−ΔΔCq) method as described by Livak and Schmittgen (2001).
Quantitation of vsiRNAs
Cucumber green mottle mosaic virus vsiRNAs were quantified by RT-qPCR according to Shi and Chiang (2005) with some modifications: 2 μg of RNA extracts from the cucumber leaves were treated with DNaseI (Merck, Spain) and polyadenylated using the Poly(A) polymerase (New England Biolabs, UK) and ATP, following the manufacturer's recommendations. Next, the polyadenylated RNA was precipitated with ethanol, NaOAc 3M, pH 5.2, and glycogen (Merck, Spain) in cold, resuspended, and reverse transcribed with the High-Capacity cDNA Reverse Transcription Kit, and the 0.25 μM of the poly(T) adapter (Supplementary Table 1). The amplifications were carried out with specific primers, designed upon the vsiRNA hotspots for the CGMMV RdRp, mp, and cp genes, and the universal 3′-adapter reverse primer (URP). A primer based on the 5.8S rRNA was designed as a reference in the amplifications. Each reaction (20 μL final volume) contained 1 μl of the diluted cDNA, 10 μl of KAPA SYBR Green qPCR mix (KAPA Biosystems, USA), and 500 nM each of the specific and the URP primers. The cycling conditions consisted of an initial denaturation at 95°C for 10 min, followed by 45 cycles at 95°C for 15 s, 58°C for 20 s, and 60°C for 40 s. Five biological repetitions were included in each case. Each qPCR (biological sample), including those for the 5.8S as internal control, was repeated three times. The specificity of the amplicons obtained was checked as above. The relative expressions of the vsiRNAs were calculated based on the comparative Cq method as above.
Results
Generation of CGMMV and ToLCNDV-Derived dsRNA
By Gateway cloning of the viral amplicons, we obtained L4440-derived plasmids carrying partial segments of the AV1 and BC1 of ToLCNDV and the cp or mp gene of CGMMV flanked by two IPTG-inducible T7 promoters. These plasmids were subsequently introduced by transformation into the RNAse III-deficient strain E. coli HT115(DE3) for dsRNA expression. To analyze dsRNA synthesis from HT115 cells, we extracted total RNA by the Trisure method after 7 h of incubation with IPTG as inducer. The dsRNA of the expected sizes, 720, 700, 590, and 650 bp, were obtained in HT115 cells harboring plasmids, such as L4440-AV1, -BC1, -CP, or -MP, respectively, when induced with IPTG (Supplementary Figure 2). Yields were ~240 μg of total RNA extract per 5 ml of bacterial culture, which will allow us to treat a plant with 60 μg per leaf, the standard amount used in the assays described here.
Phenotypic Effects of dsRNA Applications on CGMMV-Inoculated Cucumber Plants
Preliminary experiments were performed in the phytotron in order to test the effect of CGMMV-derived dsRNAs, either in the form of rubbing, spraying, or agroinoculation. In the initial experiment, five cucumber plants at the cotyledon stage were used in each of the following treatments, both mock and CP/MP-dsRNA treatment by rubbing. The same day, the plants were inoculated (challenge) with CGSPCu16. At 15 dpi, 2 mock-inoculated plants initially showed virus symptoms that finally appeared in all the mock-inoculated plants at 18 dpi. In contrast, the dsRNA-treated plants remained symptomless in this period (Supplementary Figure 3). In a second experiment, performed in the phytotron, 10 plants in each condition were used. In this case, the virus challenge was done 3 days after the dsRNA application. At 25 dpi, symptoms in two dsRNA-treated plants were rated as severe (2), while seven remained asymptomatic, and one resulted dead. Among the mock-inoculated plants, four were dead and three showed very severe symptoms (rate = 3). However, three mock-treated CGMMV-inoculated plants remained asymptomatic in this period (Table 1). Other preliminary experiments showed that spraying with dsRNA was as effective as rubbing in limiting CGMMV disease severity (not shown).
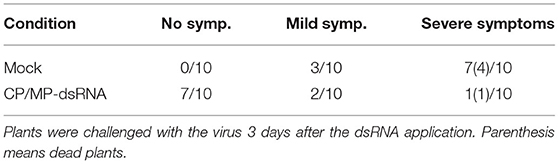
Table 1. Effects of CP/MP double-stranded RNA (dsRNA) treatments in plants inoculated with cucumber green mottle mosaic virus (CGMMV) Sp16 at 25 days post-inoculation (dpi) when grown in the phytotron (second assay).
An additional experiment was performed in the phytotron to trigger dsRNA synthesis in the plant by agroinoculation with the pGHE-CP and pGHE-MP constructions mediated by A. tumefaciens. A group of 10 plants was agroinfiltrated at 2–3 true leaves stage, while the second group of 10 plants was mock-treated. Both groups were challenged subsequently with the virus on the same day. The results showed that the agroinoculation limited the disease progress and protected the plants against CGMMV (Table 2). At 13 dpi, all the mock-treated plants displayed symptoms. Al 15 days post-treatment (dpt)/dpi, four of the agroinfiltrated plants showed symptoms while the rest remained asymptomatic. Finally, at 30 dpt/dpi, eight agroinfiltrated plants showed severe symptoms, one remained asymptomatic, and another one showed mild symptoms.
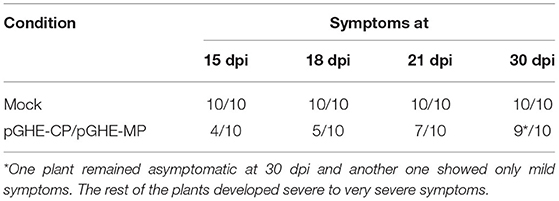
Table 2. Occurrence of symptoms with time (days post inoculation, dpi) in plants agroinoculated with pGHE-CP and pGHE-MP mediated by Agrobacterium tumefaciens LBA4404 compared with the mock control plants after challenging with CGMMV Sp16.
Once the experiments in the phytotron showed an effect of the dsRNAs on limiting CGMMV disease progress, we considered experimental assays under greenhouse conditions (Supplementary Figure 4). Two separate experiments were performed. The first experiment was started in mid-April 2021 (23.5°C average temperature in the greenhouse) when 15 plants were mock-treated, and 15 plants of another group were sprayed with CP-dsRNA at 2-−3 leaves stage. Subsequently, all the plants were inoculated with CGSPCu16. The third group of 15 plants was not inoculated as a reference. The treatment was performed by spraying with 60 μg of bacterial dsRNA extract in each of two true leaves per plant (120 μg/plant). At 3 dpt, the plants were challenged with the virus. At 18 dpi, the cucumber plants were harvested because almost all the mock-treated CGMMV inoculated plants showed severe or very severe symptoms. Then, the dry weight, total height, and level of symptom expression were determined (Figure 1). At this stage, samples were taken for RNA extraction and virus quantitation by RT-qPCR. Although we found only small differences between dry weight values, the non-inoculated control plants resulted significantly more robust than those inoculated with CGSPCu16 (P = 0.001) but not significantly different with respect to the dsRNA-treated plants (P = 0.378). The dsRNA-treated and mock-treated plants differed slightly, but significantly in dry weight (P = 0.036). As for the height, the control and the dsRNA-treated inoculated plants showed no significant differences (P = 0.954), in contrast with the dsRNA-untreated inoculated plants, which showed a significantly lower height with respect to the control (P = 0.005) and the dsRNA treated plants (P = 0.005) (Figure 1). The greatest differences were observed in the expression of disease symptoms. All the mock-treated virus-inoculated plants showed severe symptoms (average rating = 2.13) at 18 dpi. In contrast, four of the dsRNA-treated plants showed mild symptoms (rating = 1) and the rest remained asymptomatic. No symptoms appeared in the control plants.
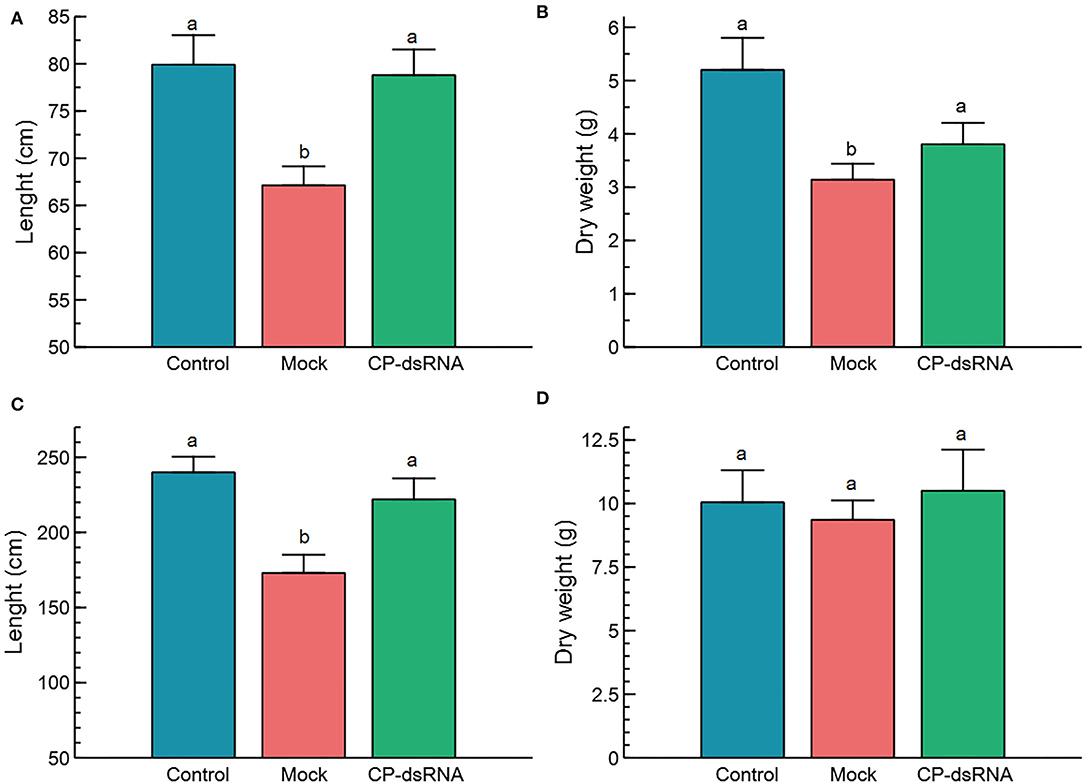
Figure 1. Phenotypical parameters in the plants following cucumber green mottle mosaic virus (CGMMV) inoculation and CP-dsRNA treatment. Length (A) and dry weight (B) of the plants in the spring assay; (C,D) represent, respectively, the values of the length and dry weight obtained in the summer assay. Plants were evaluated at 18 days post-inoculation (dpi), when the symptoms in all the mock-treated plants were rated severe (=2) or very severe (=3). Control refers to non-inoculated plants. Bars correspond to the standard error of the means. Different letters on the columns refer to significant differences (P < 0.05).
A similar greenhouse experimental approach was performed in summer, at higher temperatures (28°C average), starting at the beginning of July 2021. In this case, there were non-significant differences in dry weight among the treatments (P = 0.495), but significant differences appeared in length between the dsRNA treated and the non-treated plants (P = 0.018). With respect to the control non-inoculated plants, there were no differences with respect to the dsRNA-treated plants (P = 0.560). Regarding the differences in length between non-inoculated control plants and mock-treated plants, the result was significantly different (P = 0.001) (Figure 1). With respect to symptom expression, all the mock-treated plants showed symptoms rating 3 (very severe) at 18 dpi. The dsRNA-treated plants showed an average symptom rate of 1.6, with two plants showing very severe symptoms, one that remained asymptomatic, seven with mild symptoms, and the other five that showed severe symptoms. Plants that underwent an experiment in July were 70% higher than those grown in April and (dry) weighted 62% more. There was a significant correlation (R2) between the length and weight, 0.81 and 0.72 for the spring and summer experiments, respectively (Figure 2). Linear correlation analysis showed differences in the behavior of the treatments between both seasons that showed a greater effect of the dsRNA treatments in limiting the disease effects during April (Supplementary Table 3).
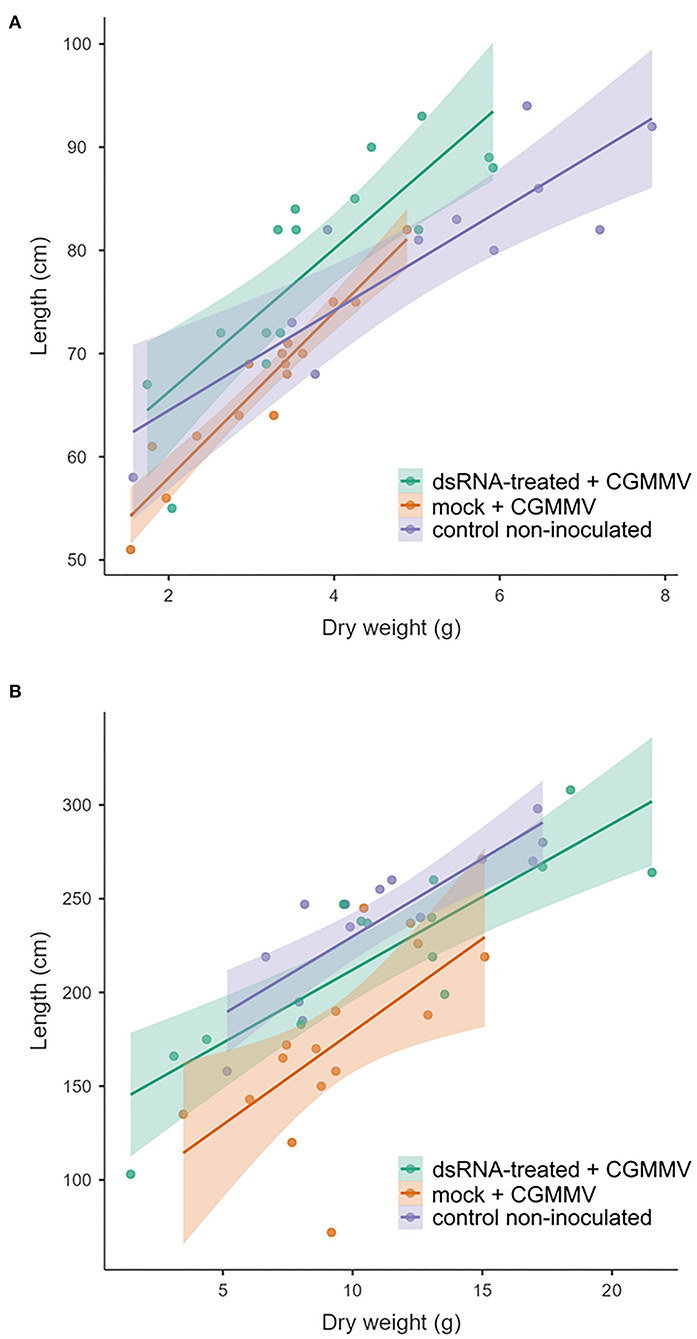
Figure 2. Scatter plot between length and dry weight of the plants of the experiments carried out in (A) spring and (B) summer. For the linear regression analysis, refer to Supplementary Table 3.
Quantitation of CGMMV Viral RNA in dsRNA-Treated and Untreated Plants
The determinations of relative viral accumulation were performed by comparing the differences in the expression of the CGMMV cp gene and the expression of the 18S rRNA gene of C. sativus in dsRNA-treated and untreated plants. In the spring assay, at 18 dpi, the average Cq for the cp gene in the untreated plants was 15.0 ± 1.8 and for the 18S rRNA, the resulting average Cq was 14.3 ± 1.9. In the dsRNA-treated plants, the average Cq was 18.7 ± 3.4 for the cp and 15.2 ± 2.6 for the 18S rRNA, respectively (Figure 3). Calculation of the ΔΔCCP−18S values resulted in a relative increase of 47.7-fold of virus accumulation in the untreated vs. the dsRNA-treated plants. Expression of the CGMMV cp gene was observed in all the inoculated plants, both in the untreated and in the dsRNA-treated ones, so that there were no escapes in virus inoculation. In the assay carried out in summer, the difference in ΔCqCP−18S of the untreated compared with the treated plants was less evident than in the spring assay. In this case, the reduction in CGMMV cp expression of the dsRNA-treated plants vs. the untreated plants was only 5.8-fold (Figure 3). All non-inoculated plants tested negative to the cp amplification in the RT-qPCRs. When the viral accumulation was compared between the seasons, we found a 30-fold higher amount than the untreated plants during summer with respect to spring. However, when we compared the amount of virus in the treated plants, the difference between summer and spring was 243-fold. Thus, the effect of the dsRNA in reducing viral accumulation was significantly higher in spring.
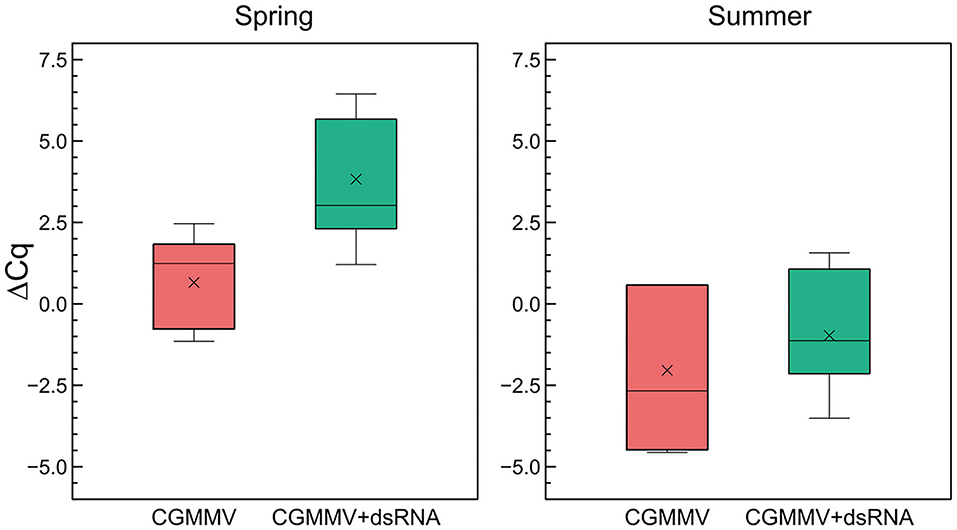
Figure 3. Quantitative differences in CGMMV accumulation between dsRNA-treated and untreated infected plants in two greenhouse assays carried out in spring and summer. The ΔCq refers to the differences between the averages of the Cq of the virus of the cp gene and the average Cq of the internal rRNA 18S control of cucumber. Lower ΔCqcp−18S values mean a higher dsRNA titer. Each boxplot shows the median (horizontal line), first, and third quartiles (lower and upper limits of boxes), and the minimum and maximum values (delimited by the external whiskers). Mean values are indicated by crosses.
HTS of vsiRNAs in CGMMV-Infected Plants
From the HTS sequencing of the small RNAs, a total of 12,967,977 raw reads were obtained from the inoculated and dsRNA-treated pool of samples (DS) and 12,819,530 reads from the non-treated inoculated plants (MO). After trimming the adapter and the low-quality reads, 3,162,386 and 6,355,112 reads between 18 and 24 nt were obtained from each sample, respectively. With respect to samples from dsRNA-treated uninfected (NI) plants, a total of 19,534,870 reads were obtained from which 5,685,355 reads between 18 and 24 nt were obtained after trimming. Profiling of the small RNA reads in the function of length was resulted when using the C. sativus reference in the sRNAtoolbox (Supplementary Figure 5) (Rueda et al., 2015). Read-length distributions resulted in the classification of the analyses according to the origin (Supplementary Figure 5). Alignment of the vsiRNAs to the CGMMV genome showed differences between the DS and MO samples. The 21–24 nt reads were aligned to the CGMMV genome (Genbank Acc. No. MH271441), resulting that 199,534 vsiRNAs matched to CGMMV in sample DS and 692,505 vsiRNAs in MO. CGMMV vsiRNAs represented 12.7% of the total 18–24 nt siRNAs in the MO sample and only 7.6% in the DS sample. Although in both samples, similar hotspots were observed in the mp and cp genes, a higher prevalence of vsiRNAs matching the replicase region (RdRp gene) was observed in the MO sample (Figure 4). From the 21–24 nt vsiRNAs aligning to CGMMV, 95% belong to the 21–22 nt class (Supplementary Figure 7). CGMMV vsiRNAs of 21-nt class clearly prevailed (55.06%), followed by the 22-nt class (41.09%) in the DS sample. Similarly, the vsiRNAs 21-nt (54.41%) prevailed over the 22-nt class (41.54%) in MO. About 55% of the 21 to 22-nt vsiRNAs aligning to the CGMMV genome were of negative polarity (69.7% in DS; 72.6 in MO), showing a bias toward antisense vsiRNAs. Adenine was the prevailing base at the 5′ end of the vsiRNAs (41.5 and 41.1% for DS and MO, respectively) and uracil at the 3′ end (45.6 and 43.2% for DS and MO, respectively) (Supplementary Figure 8). Reads from sample NI aligned only to the cp gene of CGMMV (11,332 vsiRNAs), being in this case, the sense sequences were clearly prevalent (85.9%) over the antisense (Figure 4C). The predominant vsiRNA class for CP-dsRNA processing was the 22-nt (44.9%) followed by 21-nt (21.0%), 24-nt (18.2%), and 23-nt classes (16.8%).
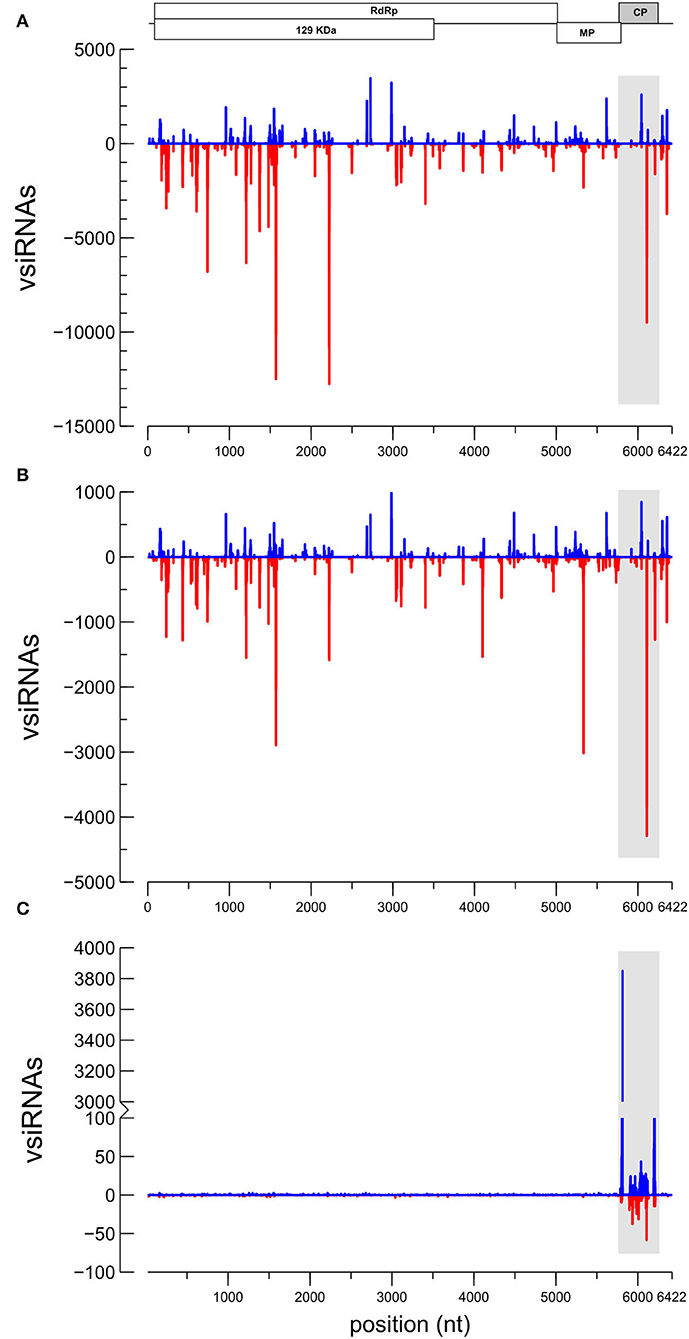
Figure 4. Profile distributions of the small RNAs of the 21–24 nt classes aligning to the genome of CGMMV: (A) Sample MO [CGMMV]; (B) sample DS [dsRNA+CGMMV]; and (C) sample NI [dsRNA]. Peaks above and below the x-axis stand for sense and antisense virus-derived small interfering RNAs (vsiRNA) orientations, respectively. A schematic view of the CGMMV genome is represented above for reference. The separation between marks in the x-axis represents 1 Kb. The shadowed area indicates the target for the sprayed dsRNA in the genome.
RT-qPCR of vsiRNAs in dsRNA-Treated and Untreated CGMMV Infected Plants
As the HTS allowed for the identification of several hotspots of vsiRNAs aligning to the CGMMV genome (Figure 4), we could design specific primers for vsiRNA detection and quantitation among plants and conditions (Supplementary Table 1). In the assay carried out in spring, CGMMV vsiRNAs were detected in dsRNA-treated and non-treated plants at 18 dpi (Figure 5). Non-inoculated control plants showed no specific amplification of the vsiRNAs as shown by the analysis of the melting curves (Supplementary Figure 9). DsRNA-treated plants showed a significant lower amount of specific vsiRNAs than the non-treated plants (Figure 5), being 7.4-fold lower for the 1,193-vsiRNA, located in the RdRp gene, 14-fold lesser for the 5,234-vsiRNA, matching the mp gene and 15.8-fold lower for the 6,125-vsiRNA, that corresponded to the cp gene of the virus. The 6,125-vsiRNAs was found to be the most abundant in both the dsRNA-treated and untreated plants.
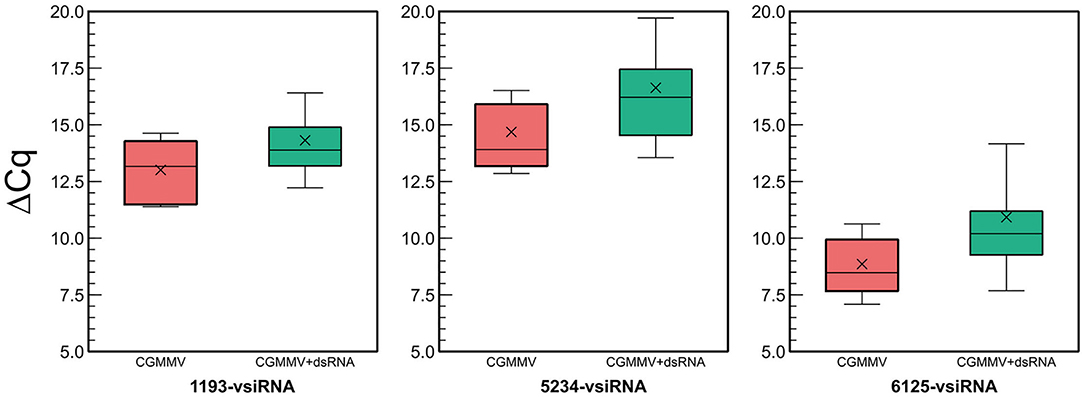
Figure 5. Quantitative differences in vsiRNA titters between untreated (red) and dsRNA-treated (green) CGMMV-infected plants in the experiment carried out in spring. The ΔCq refers to the differences between the Cq of the different vsiRNAs and the cucumber rRNA control (5.8-rRNA). Lower ΔCqvsiRNA−5.8SrRNA values mean higher vsiRNA titer.
Systemic Movements of Exogenous CGMMV (ds)RNAs and Derived vsiRNAs to Distal and Proximal Parts of the Plant
We investigated the systemic movements of long (ds)RNAs and derived siRNAs after the application of dsRNA. Here, we define (ds)RNAs as long RNA molecules that move in a cell-autonomous (apoplast, phloem) or non-cell autonomous ways (symplast), in single or double stranded forms. In a set of independent experiments performed in the growth chamber, we applied CP-dsRNA by spraying it on plant leaves of five plantlets (point 1 in Supplementary Figure 10). The leaves were washed thoroughly, and we analyzed the presence of (ds)RNAs and vsiRNAs at 3 dpt in distal leaves (point 3), that were foil-covered prior to spraying. In a parallel experiment, other five plants per condition were agroinfiltrated with pGHE-CP or pGHE-MP in point 1 (Supplementary Figure 11). Total RNA was then extracted from sampling point 3 and used for the quantitation of CP- or MP-(ds)RNA and the 6,126-vsiRNA or 5,324-vsiRNA. Analysis of the results showed that the (ds)RNAs and the vsiRNAs were detected in distal leaves (Figure 6), both in agroinoculated and in sprayed plants. Comparison of the ΔCq for agroinoculation of MP-dsRNA and CP-dsRNA of the long (ds)RNAs detected in distal leaves showed similar values, but lower than the ones obtained with sprayed dsRNA (Figure 6A). The calculated ΔΔCq values of leaves that were dsRNA-sprayed and agroinfiltrated differed 9-fold. On the other hand, a comparison of ΔΔCq values showed that the amounts of vsiRNAs derived from the CP- and MP-dsRNAs in agroinfiltrated plants were in the same range, while the 6,125-vsiRNAs derived from the CP-dsRNA in sprayed plants was 14-fold more abundant (Figure 6A). With respect to long RNAs, they were slightly more concentrated in distal leaves of plants that were sprayed when compared with those from plants that had been agroinoculated (Figure 6B).
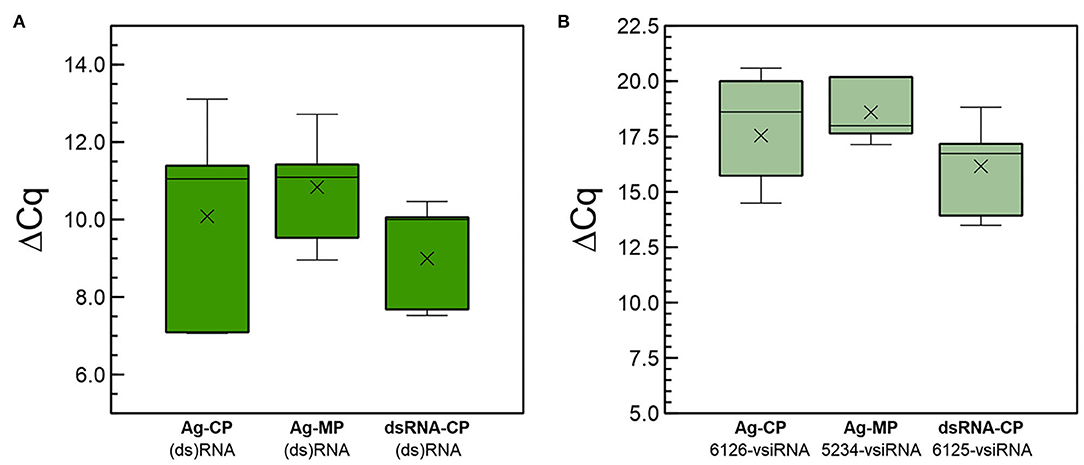
Figure 6. Quantitative differences at 3 dpt in (A) CP-ds(RNA) and (B) 5,234- or 6,125-vsiRNA amounts in distal (point 2) non-treated leaves among plants either directly sprayed with CP-dsRNA (dsRNA-CP) or agroinfiltrated with the CP-dsRNA (Ag-CP) or the MP-dsRNA (Ag-MP) expressing constructions pGHE-CP and pGHE-MP mediated by A. tumefaciens LBA4404.
In another experiment, we included six plants per condition and analyzed two additional points of sampling, including the site of application, to investigate the movement of the long RNAs and derived siRNAs that are distally close to the site of application of the dsRNA (point 2 in Supplementary Figure 10). Plants were prepared so that the dsRNAs were applied only on half of the leaves, keeping the other halves and the rest of the plant foil-covered to prevent them to be reached by the sprayed CP-dsRNA (Supplementary Figure 10). Three points were thus evaluated by RT-qPCR (Supplementary Figure 10B). Values of ΔCqCP−18S for the long (ds)RNAs are displayed in Figure 7A. Calculation of the ΔΔCqCP−18S enabled us to establish that at points 2 and 3, there was 3.8 × 104-fold and 1.55 × 105-fold less CP-(ds)RNA, respectively, with respect to the point of spraying the CP-dsRNA (point 1). Thus, (ds)RNA of about less than four orders of magnitude were moved from the point of application closer to the distal part of the leaf, and from there, long RNA molecules less than five orders of magnitude were moved to another leaf (far distal). When we compared the differences in CP-(ds)RNA amounts between the close distal (point 2) and the far distal point 3, the difference was only 50-fold of the former with respect to the latter.
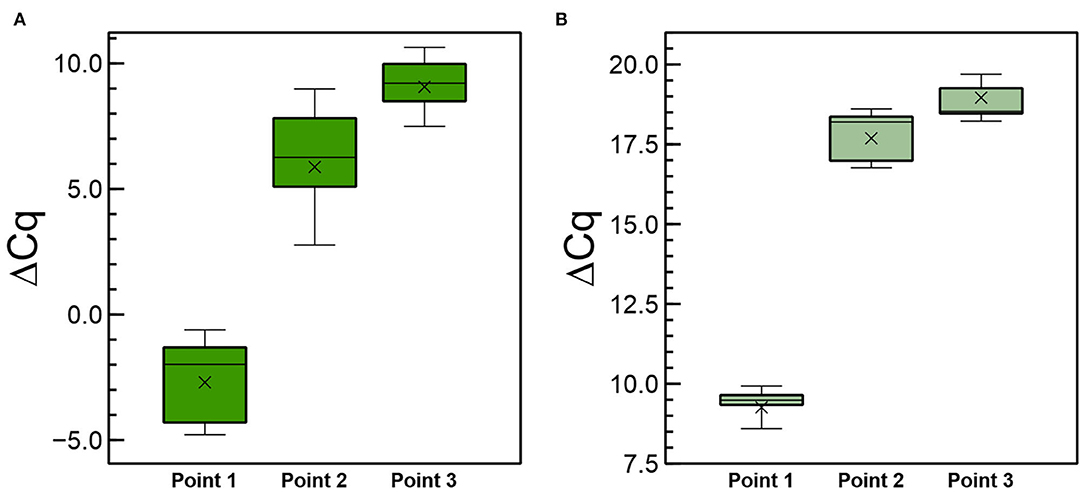
Figure 7. Quantitative differences of the CP-(ds)RNA (A) and the 6,125-vsiRNA (B) detected by RT-qPCR at 3 dpt after spraying the CP-dsRNA in local (point 1), close distal (point 2), and far distal (point 3) sampling as described in Figure 8.
When the RNAi was investigated, we found that the 6,125-vsiRNAs could be detected in non-treated leaves at 3 dpt, indicating systemic long-distance movement of the silencing signal and confirming the earlier experiments (Figure 7B). Comparison of ΔCq6125−vsiRNAs−5.8SsiRNA between the point of spraying (point 1) and points 2 and 3 showed that in the former, 9.1 × 103 -fold and 3.3 × 104 -times, respectively, more vsiRNA was detected. In point 3 (far distal), 6,125-vsiRNA <7-fold was detected in comparison with point 2 (close distal). Thus, the dsRNA/vsiRNAs reaching distal sites were very diluted in comparison to amounts quantified at the point of application. When we compared the ratios between long (ds)RNAs and vsiRNAs in the different sampling sites, it resulted that they were similar in points 1 and 2, but when compared between points 1 and 3, the relative ratio was 7.9-fold higher for the vsiRNAs in the latter. Therefore, although some correlation was found in the ratios, the comparatively higher ratio of vsiRNAs in point 3 (opposite leaf) might suggest different systemic movements of long (ds)RNAs in the phloem depending on their location in the plant.
Finally, the stability of the (ds)RNA in the leaves was also investigated. After the application of the CP-dsRNAs on the leaves, we washed them and took samples from the leaf sprayed at 3, 6, and 10 dpt, for RNA extractions and RT-qPCR. The result showed that the dsRNA could be detected at least up to 10 days following the treatments (Figure 8). Interestingly, the amount of (ds)RNA was almost stable between 6 and 10 dpt at the point of application. Another sampling was done at the apex and the (ds)RNA could be detected as well. It was diluted 7.9 × 105-fold with respect to the amount quantified in the sprayed leaf, similar to the ratios found at 3 dpt between the leaf sprayed and the opposite leaf.
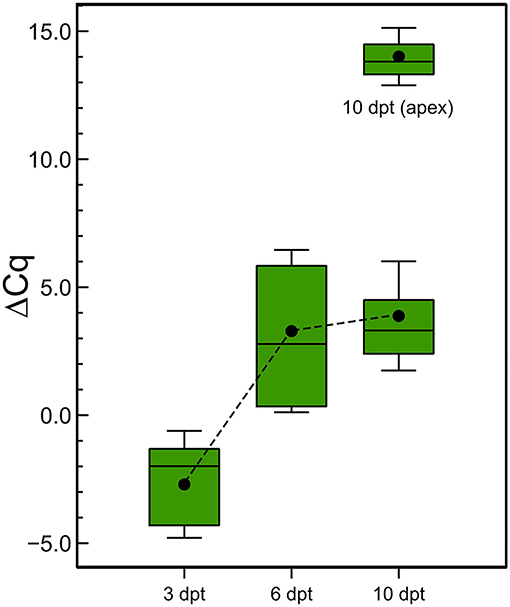
Figure 8. Stability of the dsRNA in the plants after topical application. Detection by RT-qPCR of cp-(ds)RNA at 3, 6, and 10 days post CP-dsRNA application using spraying. Another sampling was done at the apex at 10 dpt.
Failure in Eliciting Protection by Exogenous dsRNAs in ToLCNDV Infections
To investigate if a similar effect of exogenously applied dsRNAs protects zucchini against ToLCNDV, we carried out parallel experiments to those performed for CGMMV. Groups of eight plants were either sprayed with AV1/BC1-dsRNAs or agroinfiltrated with the pGHE-AV1 and pGHE-BC1 constructions. They were infected at 3 dpt with viruliferous insects hosting ToLCNDV-ES and kept in insect-proof cages in the growth chamber. Another group of plants was mock-treated for comparisons. At 15 dpi, we observed no differences neither in the number of symptomatic plants nor in the severity of symptoms between the treated and the untreated plants, either sprayed with dsRNA or agroinoculated.
Discussion
Control of CGMMV by RNAi has been achieved in Nicotiana benthamiana using a transgenic approach with a transgene designed to produce an inverted repeat RNA containing the cp gene separated by an intron (Kamachi et al., 2007). In watermelon, a single chimeric transgene comprising a silencer DNA from the partial N gene of watermelon silver mottle virus (WSMoV) fused to the partial cp gene sequences of cucumber mosaic virus (CMV), watermelon mosaic virus (WMV), and CGMMV conferred multiple virus resistance (Lin et al., 2012). More recently, Miao et al. (2021) have designed polycistronic artificial microRNAs (amiRNAs) that, when externally applied by a transient expression system, limit CGMMV disease in cucumber and N. benthamiana. Transgenic cucumber lines expressing the polycistronic artificial microRNAs also developed resistance to the virus.
In our approach, the direct application of dsRNAs was aimed to control CGMMV disease in the cucumber under greenhouse conditions. Transient expression of dsRNA triggered by a plasmid construction with direct and inverted repeats of the cp and mp genes of CGMMV were also capable of limiting disease development in cucumber during agroinoculation experiments performed in a phytotron. The effect of dsRNA application in limiting the progression of CGMMV disease and virus accumulation resulted more consistently in trials performed during spring than in summer. Higher temperatures limit the tolerance of plants to this virus (Dombrovsky et al., 2017) and increase its mobility in the plant (Moreno et al., 2004). Nevertheless, a reduction in virus accumulation in the plants will help limit the damages and will probably reduce the transmission between plants during routine crop management practices in the greenhouse, such as planting, pruning, harvesting, and others. The reduction in virus accumulation in greenhouse conditions of 48- and 5-fold depending on the season was comparable to the results obtained in groundnut bud necrosis virus (GBNV), where it has been reported 20- and 12.5-fold reduction after rubbing dsRNA on plant leaves in cowpea and N. benthamiana, respectively (Gupta et al., 2021). The correlation between symptom severity and virus accumulation has been previously reported for many plant pathosystems, specifically for CGMMV in N. benthamiana by ELISA (Ali et al., 2016), in cucumber by RT-qPCR (Crespo et al., 2018), and for CVYV in cucumber, also by RT-qPCR (Galipienso et al., 2013). The introduction of resistance in transgenic plants expressing virus genes leads to reduced symptom expression that is correlated with a decrease in virus accumulation Bagewadi and Fauquet (2014). Similarly, induced resistance with exogenous delivery of dsRNA or siRNAs leads to a reduction in virus accumulation in plants as shown by serology or qPCR (Tenllado et al., 2003; Mitter et al., 2017a; Rego-Machado et al., 2020; Gupta et al., 2021; Holeva et al., 2021; Necira et al., 2021).
Plant cuticles are barriers to dsRNA entry that have to reach the cell wall also and cross the plasma membrane. High-pressure spraying allows the entry of the dsRNA, as well as do lesions made by abrasives, such as carborundum, like in our experiments when applied the dsRNA by rubbing or celite, and as reported for GBNV control using dsRNA (Gupta et al., 2021). Other authors have proposed surfactants to facilitate the entry of the dsRNA and the movement of the silencing signals (Schwartz et al., 2020; Bennett et al., 2020). However, in the case of CGMMV control in cucumber using RNAi, we believe that surfactants should be avoided because in preliminary assays, we have observed that disease severity increased and the time to symptom onset was shortened when the surfactant BREAK-THRU S279 (BASF) was included in dsRNA formulations for CGMMV control (results not shown). Formulations that include nanoparticles to protect the si/dsRNAs from degradation and facilitate their entry into the plant seem promising (Uslu and Wassenegger, 2020). Therefore, there is room for improvement in dsRNA applications, given the variability in plant responsiveness to dsRNAs for CGMMV protection that we have observed. This can be due to differences in dosage, pressure applied, phenological state of the plant, etc. High pressure of 7–8 bars seems to be necessary for triggering RNAi responses in GFP transgenic N. benthamiana 16c (Dalakouras et al., 2016); in our case, 2.5 bars resulted to be sufficient to induce CGMMV resistance in cucumber. Higher pressures resulted in leaf damage.
By densitometry analysis, we previously estimated the amount of CP-dsRNA present in the RNA extractions from the E. coli cells to be 62 ng dsRNA/μg in the total RNA (Delgado-Martín and Velasco, 2021). Thus, when spraying 120 μg of the bacterial RNA extraction, which includes ssRNA and DNA from 2.5 mL of bacterial culture, the amount of dsRNA applied to the plants, that was effective for limiting CGMMV disease, was 3.7 μg in total, or 35 ng·cm−2 in each of the two leaves/plant on average. In tomato, the control of the tomato mosaic virus (ToMV) has been achieved using 200–400 μg of bacterial dsRNA extract, obtained using a similar method (Rego-Machado et al., 2020). Gupta et al. (2021) used 5 μg of dsRNA/plant for limiting GBNV disease in N. benthamiana, but in this case, the dsRNA extract was treated with DNase I and RNAse A to remove the DNA and ssRNA, so this quantity cannot be directly comparable to ours. In another example, 1.25 μg of in vitro-transcribed dsRNA was effective for the control of pepper mild mottle virus (PMMoV) in Nicotiana tabacum (Mitter et al., 2017a). For inducing resistance to tobacco mosaic virus (TMV) in tobacco, 300 μg of crude bacterially expressed dsRNAs per plant were used (Yin et al., 2009). The control of papaya ringspot virus (PRSV) in papaya was achieved using 100 μg/plant of bacterially expressed dsRNA (Shen et al., 2014). In general, lower amounts have been used in the case of in vitro-synthesized dsRNA (reviewed by Dubrovina and Kiselev, 2019). For example, for the protection of zucchini against Zucchini yellow mosaic virus (ZYMV), 60 μg/plant of in vitro-synthesized dsRNA has been applied (Kaldis et al., 2018). Thus, there is a lack of homogeneity in the description of quantity and conditions of exogenous dsRNA applications, making it difficult to compare the amount of effective dsRNA applied as proposedin the study by each author who reports different synthesis approaches (in vitro, in vivo), extractions methods, preliminary treatments with DNase and/or RNAse A, etc. (Dubrovina and Kiselev, 2019; Das and Sherif, 2020). We consider that the net volume of bacterial culture per plant, which in our case for CGMMV treatments, was 2.5 mL/plant that can be used to compare the different treatments so far reported that include in vitro or in vivo dsRNA synthesis.
Analysis of vsiRNAs in CGMMV infected plants has been reported previously. In Lagenaria siceraria, the sRNAs sizing 24 nt length were predominant over the 21, 22, or 23 nt in leaves or fruits (Li J. et al., 2016). Similarly, in cucumber, other authors reported 24 nt sRNAs as predominant in CGMMV-infected or healthy plants (Li Y. et al., 2016). Other authors found that the 23-nt class of sRNAs was predominant in CGMMV-infected cucumber (Liu et al., 2015), in contrast to what was reported in healthy cucumber plants, where the 24-nt class of sRNAs was predominant (Martínez et al., 2011). On the contrary, in cucumber, we observed the 21 nt followed by the 22 nt as the predominant sRNAs in leaves. Regarding the specific CGMMV vsiRNAs, we as well as other authors observed the 21-nt class as predominant, followed by the 22-nt class. In consequence, the involvement of the DICER-LIKE endonucleases, DCL4 and DCL2 seem to be predominant in the processing of the exogeneous CP-dsRNA (Liu et al., 2009). In contrast to the previous report on cucumber and L. siceraria, we observed that the negative CGMMV vsiRNAs sequences were predominant in the leaves. With respect to the preferred 5′ and 3′ terminal nucleotides of the vsiRNAs, we observed that both in dsRNA-treated or untreated plants, the 5′-A and the 3′-U were predominant, confirming what has been reported for vsiRNAs of plant viruses, that usually start with A or U (Donaire et al., 2009). However, Li Y. et al. (2016) reported a predominance of a 5′-C, followed by 5′-A in CGMMV vsiRNAs in cucumber. In L. siceraria, the 5′-A was reported as the CGMMV predominant nucleotide end of the vsiRNAs in fruits and 5′-U in leaves (Li J. et al., 2016). In ToMV in tomato, the 5′-U terminal nucleotide in the 21-nt class was predominant (Rego-Machado et al., 2020) in dsRNA-treated and non-treated plants. Given that AGO1 and AGO2 preferentially recruit small RNAs with a 5′ terminal of U and A, respectively, it seems that several ARGONAUTE proteins are involved in the processing of the vsiRNAs within the RISC complex in cucumber (Mi et al., 2008). Hotspots of CGMMV-derived vsiRNAs could be identified in dsRNA-treated and untreated plants, that were predominant in the 5′ and 3′ end of the viral genome. The distribution of the vsiRNAs was similar in both cases, although there was a bias for 5′ hotspots in the non-dsRNA-treated plants. In CGMMV-infected cucumber, hotspots were already reported in the terminal ends of the genome (Li Y. et al., 2016). This is similar to the findings in L. siceraria fruits, but not in leaves, where the distribution of vsiRNA hotspots showed no predominance along the genome (Li J. et al., 2016). The proportion of vsiRNA classes was similar in dsRNA-treated or untreated plants as reported before (Rego-Machado et al., 2020); however, we observed a 40% reduction in the number of vsiRNAs of all the classes in the dsRNA-treated plants. The lower abundance of vsiRNAs in dsRNA-treated vs. untreated plants can be explained by the reduction of viral titers in the former during plant development. The cp gene of CGMMV is expressed from subgenomic RNAs which might explain the presence of abundant vsiRNA hotspots in this region (Adams et al., 2012). Besides, we found that many vsiRNAs were produced in the 3′ untranslated region, which conforms a tRNA-like structure. Moreover, as described elsewhere (Rego-Machado et al., 2020), exogenous dsRNA applied to leaves produced specific vsiRNAs, being in our case, the 22-nt vsiRNAs the predominant class derived from the sprayed dsRNA, suggesting a major involvement of DCL2 in the processing.
In this work, we report that long specific RNAs can be detected systemically in the distal part of the plant after the application of the dsRNAs, either by spraying or elicited by agroinfiltration and are effective in limiting CGMMV accumulation. This could also be obtained by using LDH-nanoparticles that released gradually the dsRNA on the leaves of plants (Mitter et al., 2017a). However, in our case, the release of dsRNA encapsulated in layered double hydroxide (LDH) nanoparticles does not improve CGMMV resistance with respect to naked dsRNA (manuscript in preparation). Detection of dsRNAs was possible by RT-qPCR at the site of spraying and in the proximal half of the leaf at 3 dpt, showing long-distance transport of the dsRNA. There is strong evidence for the systemic movement of siRNAs to short distances (through a few cells) throughout the plasmodesmata without producing secondary siRNAs (Kim, 2005). However, the long-distance movement of sRNAs and systemic silencing seems to require the amplification of the silencing signals by RNA-dependent RNA polymerase (RdRps) in the phloem (Dalakouras et al., 2018). Thus, the mechanism seems to involve long-distance movements of the dsRNA in the apoplast and the phloem and translocation from there to the symplast for eliciting RNAi and subsequent siRNA production (Das and Sherif, 2020). Recently, when applying exogenous ZYMV-derived dsRNAs, long-distance transport of long dsRNAs has been shown by semiquantitive RT-PCR even at 21 dpt (Kaldis et al., 2018). We detected vsiRNAs following dsRNA application, at the site of application, as well as in the distal part of the leaf and in another opposite leaf, suggesting that the vsiRNAs either make long-distance movements or are the result of local RNAi processing of the moving (ds)RNAs. Local and systemic vsiRNAs have been identified from ZYMV-derived vsiRNAs after dsRNA rubbing (Kaldis et al., 2018). In another report, ToMV-derived dsRNA was detected by RT-PCR at 10 dpt at the site of application but could not be detected in distal leaves (Rego-Machado et al., 2020); the dsRNA-derived vsiRNAs were identified by HTS in local and distal leaves of the application site. A PMMoV-derived dsRNA was detected by Northern-blot in pepper at 7 dpt (Tenllado and Díaz-Ruíz, 2001). More recently, a GBNV-derived dsRNA was locally and systemically detected by semiquantitative RT-qPCR at 7 dpt in N. benthamiana and at 5 dpt in cowpea (Gupta et al., 2021). Delivery of insect-derived dsRNA into barley plants showed a systemic dsRNA movement and distal siRNAs were present as shown by HTS (Biedenkopf et al., 2020). Conceivably, using the RT-qPCR, more sensitive, than the conventional RT-PCR allowed us to detect systemic movements of the (ds)RNAs, that were of several orders of magnitude more diluted with respect to the site of application. Therefore, our work supports the evidence for the systemic movement of long RNA molecules after exogenous application. Moreover, we have shown a correlation between the amounts of dsRNA, either applied or systemic, and derived siRNAs. On the other hand, we propose a method for the direct quantitation of (ds)RNA in plants after the application and washing up the surface, that yields a comparison test for procedures and formulations for dsRNA delivery. The protection effect of naked dsRNA is probably limited to short periods after its application, although the dsRNA could be detected at the site of application at least up to 9 dpt (Kaldis et al., 2018) or even at 10 dpt, as we have observed. This is a serious limitation in the management of the diseases, so that either repeated dsRNA applications are performed, or the dsRNA applied in the first instance must endure for a longer period. Mitter et al. (2017a) elongated the availability of dsRNA in the plants up to 20 dpt by the controlled release on the leaf surface mediated by nanoparticles. Thus, it is plausible that improving the administration of dsRNAs will enhance their efficacy in increasing the resistance to viruses. Alternatively, non-transgenic silencing after the application of siRNAs has been targeted to plant genes or transgenic GPF (Dalakouras et al., 2016; Bennett et al., 2020; Schwartz et al., 2020). For virus control, amiRNAs have been delivered by transgenic approaches (Bagewadi and Fauquet, 2014), but only recently using a transient expression system (Miao et al., 2021). One of the advantages of using dsRNAs is that their synthesis can be performed in the labs and does not require purchasing from specialized suppliers.
The exogenously applied dsRNA offers a promising tool for virus control, although it might not be effective in all cases, as is described for DNA viruses, such as the begomoviruses tomato severe rugose virus (ToSRV) in tomato (Rego-Machado et al., 2020), or ToLCNDV in zucchini squash, in the present work. One of the reasons given for the failure of exogenous dsRNA application to control DNA viruses is due to the low amount of 24-nt-class vsiRNAs produced (Rego-Machado et al., 2020). Nevertheless, there is potential for improvement in the case of DNA viruses, as transgenic plants expressing microRNAs specific for ToLCNDV increased virus tolerance in tomato (Vu et al., 2013). Conceivably, increasing the amount of the dsRNA applied or improving the formulations may contribute to finding effective exogenous dsRNA application in those viruses. Finally, we have shown that cucumber plants topically treated with dsRNA limit the multiplication of CGMMV and, consequently, the expression of symptoms and other deleterious effects of the disease. We postulate that it may be necessary to maintain the uptake or continuous application of dsRNA to the plant during the vegetative period, particularly in summer, either by increasing the number of doses or by generating a controlled release by encapsulation of dsRNA in nanocomposites, in order to make the treatments more reliable in field applications (Rank and Koch, 2021).
Data Availability Statement
The raw data supporting the conclusions of this article will be made available by the authors, without undue reservation.
Author Contributions
LV and JD-M designed the research. JD-M, LR, DJ, and LV performed the experiments, analyzed the data and results, and wrote and edited the final manuscript. LV wrote the original draft. LV and DJ provided funds for the project. All authors contributed to the article and approved the submitted version.
Funding
This work was funded by grants RTA2017-00061-C03-01 from the Spanish Ministerio de Ciencia, Innovación y Universidades (MICIU), and IFAPA AVA2019.015 co-financed by FEDER. JD-M acknowledges a predoctoral grant from MICIU.
Conflict of Interest
The authors declare that the research was conducted in the absence of any commercial or financial relationships that could be construed as a potential conflict of interest.
Publisher's Note
All claims expressed in this article are solely those of the authors and do not necessarily represent those of their affiliated organizations, or those of the publisher, the editors and the reviewers. Any product that may be evaluated in this article, or claim that may be made by its manufacturer, is not guaranteed or endorsed by the publisher.
Acknowledgments
The authors acknowledge E. Martínez-Campos, A. Delgado-Olidén, and A. Belmonte for the technical assistance.
Supplementary Material
The Supplementary Material for this article can be found online at: https://www.frontiersin.org/articles/10.3389/fpls.2022.895953/full#supplementary-material
Footnotes
1. ^Jamovi Project, 2020: https://www.jamovi.org.
2. ^Sanders, 2021: https://veusz.github.io/.
References
Adams, M. J., Heinze, C., Jackson, A. O., Kreuze, J. F., Macfarlane, S. A., and Torrance, L. (2012). “Virgaviridae,” in Virus Taxonomy: Ninth Report of the International Committee on Taxonomy of Viruses, eds A. M. Q. King, M. J. Adams, E. B. Carstens, E. J. Lefkowitz (London: Elsevier; Academic Press), 1139–1161.
Adams, M. J., Heinze, C., Jackson, A. O., Kreuze, J. F., Macfarlane, S. A., and Torrance, L. (2012). “Virgaviridae,” in Virus Taxonomy, eds A. M. Q. King, M. J. Adams, E. B. Carstens, and E. J. Lefkowitz (San Diego, CA: Elsevier), 1139–1162. doi: 10.1016/B978-0-12-384684-6.00097-5
Ainsworth, G. C.. (1935). Mosaic disease of the cucumber. Ann. Appl. Biol. 22, 55–67. doi: 10.1111/j.1744-7348.1935.tb07708.x
Ali, M. E., Waliullah, S., and Nishiguchi, M. (2016). Molecular analysis of an attenuated strain of Cucumber green mottle mosaic virus using in vitro infectious cDNA clone: pathogenicity and suppression of RNA silencing. J. Plant Biochem. Biotechnol. 25, 79–86. doi: 10.1007/s13562-015-0312-z
Bagewadi, B., and Fauquet, C. M. (2014). “Plant virus control by post-transcriptional gene silencing,” in Encyclopedia of Agriculture and Food Systems (Elsevier), 472–488. doi: 10.1016/B978-0-444-52512-3.00219-9
Bennett, M., Deikman, J., Hendrix, B., and Iandolino, A. (2020). Barriers to efficient foliar uptake of dsrna and molecular barriers to dsrna activity in plant cells. Front. Plant Sci. 11, 816. doi: 10.3389/fpls.2020.00816
Biedenkopf, D., Will, T., Knauer, T., Jelonek, L., Furch, A. C. U., Busche, T., et al. (2020). Systemic spreading of exogenous applied RNA biopesticides in the crop plant Hordeum vulgare. ExRNA 2, 12. doi: 10.1186/s41544-020-00052-3
Cagliari, D., Dias, N. P., Galdeano, D. M., Dos Santos, E. Á., Smagghe, G., and Zotti, M. J. (2019). Management of pest insects and plant diseases by non-transformative RNAi. Front. Plant Sci. 10, 1319. doi: 10.3389/fpls.2019.01319
Crespo, O., Janssen, D., García, C., and Ruiz, L. (2017). Biological and molecular diversity of Cucumber green mottle mosaic virus in Spain. Plant Dis. 101, 977–984. doi: 10.1094/PDIS-09-16-1220-RE
Crespo, O., Janssen, D., Robles, C., and Ruiz, L. (2018). Resistance to cucumber green mottle mosaic virus in Cucumis sativus. Euphytica 214, 201. doi: 10.1007/s10681-018-2286-0
Crespo, O., Robles, C., Ruiz, L., and Janssen, D. (2019). Antagonism of cucumber green mottle mosaic virus against tomato leaf curl New Delhi virus in zucchini and cucumber. Ann. Appl. Biol. 176, 147–157. doi: 10.1111/aab.12535
Dalakouras, A., Jarausch, W., Buchholz, G., Bassler, A., Braun, M., Manthey, T., et al. (2018). Delivery of hairpin RNAs and small RNAs into woody and herbaceous plants by trunk injection and petiole absorption. Front. Plant Sci. 9, 1253. doi: 10.3389/fpls.2018.01253
Dalakouras, A., Wassenegger, M., McMillan, J. N., Cardoza, V., Maegele, I., Dadami, E., et al. (2016). Induction of silencing in plants by high-pressure spraying of in vitro-synthesized small RNAs. Front. Plant Sci. 7, 1327. doi: 10.3389/fpls.2016.01327
Das, P. R., and Sherif, S. M. (2020). Application of exogenous dsRNAs-induced RNAi in agriculture: challenges and triumphs. Front. Plant Sci. 11, 946. doi: 10.3389/fpls.2020.00946
Delgado-Martín, J., and Velasco, L. (2021). An efficient dsRNA constitutive expression system in Escherichia coli. Appl. Microbiol. Biotechnol. 105, 6381–6393. doi: 10.1007/s00253-021-11494-6
Dombrovsky, A., Tran-Nguyen, L., and Jones, R. (2017). Cucumber green mottle mosaic virus: rapidly increasing global distribution, etiology, epidemiology, and management. Ann. Rev. Phytopathol. 55, 231–256. doi: 10.1146/annurev-phyto-080516-035349
Donaire, L., Wang, Y., Gonzalez-Ibeas, D., Mayer, K. F., Aranda, M. A., and Llave, C. (2009). Deep-sequencing of plant viral small RNAs reveals effective and widespread targeting of viral genomes. Virology 392, 203–214. doi: 10.1016/j.virol.2009.07.005
Dubrovina, A. S., and Kiselev, K. V. (2019). Exogenous RNAs for gene regulation and plant resistance. Int. J. Mol. Sci. 20, 2282. doi: 10.3390/ijms.2009.2282
Fire, A., Xu, S., Montgomery, M. K., Kostas, S. S., Driver, S. E., and Mello, C. C. (1998). Potent and specific genetic interference by double-stranded RNA in Caenorhabditis elegans. Nature 391, 806–811. doi: 10.1038/35888
Fletcher, S. J., Reeves, P. T., Hoang, B. T., and Mitter, N. (2020). A perspective on RNAi-based biopesticides. Front. Plant Sci. 11, 51. doi: 10.3389/fpls.2020.00051
Fondong, V. N.. (2013). Geminivirus protein structure and function. Mol. Plant Pathol. 14, 635–649. doi: 10.1111/mpp.12032
Food and Agriculture Organization of the United Nations (FAO) (2019). The State of Food and Agriculture 2019: Moving Forward on Food Loss and Waste Reduction. Rome: Food and Agricultural Organization.
Fortes, I. M., Sanchez-Campos, S., Fiallo-Olive, E., Diaz-Pendon, J. A., Navas-Castillo, J., and Moriones, E. (2016). A novel strain of tomato leaf curl New Delhi virus has spread to the mediterranean basin. Viruses 8, 307. doi: 10.3390/v8110307
Galipienso, L., Janssen, D., Rubio, L., Aramburu, J., and Velasco, L. (2013). Cucumber vein yellowing virus isolate-specific expression of symptoms and viral RNA accumulation in susceptible and resistant cucumber cultivars. Crop Prot. 43, 141–145. doi: 10.1016/j.cropro.2012.08.004
Golden, D. E., Gerbase, V. R., and Sontheimer, E. J. (2008). An inside job for siRNAs. Mol. Cell 31, 309–312. doi: 10.1016/j.molcel.2008.04.008
Gupta, D., Singh, O. W., Basavaraj, Y. B., Roy, A., Mukherjee, S. K., and Mandal, B. (2021). Direct foliar application of dsRNA derived from the full-length gene of NSs of groundnut bud necrosis virus limits virus accumulation and symptom expression. Front. Plant Sci. 12, 734618. doi: 10.3389/fpls.2021.734618
Helliwell, C., and Waterhouse, P. (2003). Constructs and methods for high-throughput gene silencing in plants. Methods 30, 289–295. doi: 10.1016/s1046-2023(03)00036-7
Holeva, M. C., Sklavounos, A., Rajeswaran, R., Pooggin, M. M., and Voloudakis, A. E. (2021). Topical application of double-stranded RNA targeting 2b and cp genes of cucumber mosaic virus protects plants against local and systemic viral infection. Plants 10, 963. doi: 10.3390/plants10050963
Hollings, M., Komuro, Y., and Tochihara, H. (1975). Descriptions of Plant Viruses No. 154. Wellesbourne: AAB, 4.
Ivanova, Z., Minkov, G., Gisel, A., Yahubyan, G., Minkov, I., Toneva, V., et al. (2022). The multiverse of plant small RNAs: how can we explore it? Int. J. Mol. Sci. 23, 3979. doi: 10.3390/ijms23073979
Janssen, D., Simón, A., Boulares, M., and Ruiz, L. (2022). Host species-dependent transmission of tomato leaf curl New Delhi virus-ES by Bemisia tabaci. Plants 11, 390. doi: 10.3390/plants11030390
Kaldis, A., Berbati, M., Melita, O., Reppa, C., Holeva, M., Otten, P., et al. (2018). Exogenously applied dsRNA molecules deriving from the zucchini yellow mosaic virus (ZYMV) genome move systemically and protect cucurbits against ZYMV. Mol. Plant Pathol. 19, 883–895. doi: 10.1111/mpp.12572
Kamachi, S., Mochizuki, A., Nishiguchi, M., and Tabei, Y. (2007). Transgenic Nicotiana benthamiana plants resistant to cucumber green mottle mosaic virus based on RNA silencing. Plant Cell Rep. 26, 1283–1288. doi: 10.1007/s00299-007-0358-z
Kim, J. Y.. (2005). Regulation of short-distance transport of RNA and protein. Curr. Opin. Plant Biol. 8, 45–52. doi: 10.1016/j.pbi.2004.11.005
Langmead, B., and Salzberg, S. L. (2012). Fast gapped-read alignment with Bowtie 2. Nat. Methods 9, 357–359. doi: 10.1038/nmeth.1923
Li, J., Zheng, H., Zhang, C., Han, K., Wang, S., Peng, J., et al. (2016). Different virus-derived siRNAs profiles between leaves and fruits in cucumber green mottle mosaic virus-infected Lagenaria siceraria plants. Front. Microbiol. 7, 1797. doi: 10.3389/fmicb.2016.01797
Li, Y., Deng, C., Shang, Q., Zhao, X., Liu, X., and Zhou, Q. (2016). Characterization of siRNAs derived from cucumber green mottle mosaic virus in infected cucumber plants. Arch. Virol. 161, 455–458. doi: 10.1007/s00705-015-2687-5
Lin, C. Y., Ku, H. M., Chiang, Y. H., Ho, H. Y., Yu, T. A., and Jan, F. J. (2012). Development of transgenic watermelon resistant to cucumber mosaic virus and watermelon mosaic virus by using a single chimeric transgene construct. Transgenic Res. 21, 983–993. doi: 10.1007/s11248-011-9585-8
Liu, H. W., Luo, L. X., Liang, C. Q., Jiang, N., Liu, P. F., and Li, J. Q. (2015). High-throughput sequencing identifies novel and conserved cucumber (Cucumis sativus L.) microRNAs in response to cucumber green mottle mosaic virus infection. PLoS ONE 10, e0129002. doi: 10.1371/journal.pone.0129002
Liu, Q., Feng, Y., and Zhu, Z. (2009). Dicer-like (DCL) proteins in plants. Funct. Integr. Genom. 9, 277–286. doi: 10.1007/s10142-009-0111-5
Livak, K. J., and Schmittgen, T. D. (2001). Analysis of relative gene expression data using real-time quantitative PCR and the 2-ΔΔCT Method. Methods 25, 402–408. doi: 10.1006/meth.2001.1262
Mandal, S., Mandal, B., and Varma, A. (2008). Properties, diagnosis and management of Cucumber green mottle mosaic virus. Plant Viruses 3, 25–35.
Martínez, G., Forment, J., Llave, C., Pallás, V., and Gómez, G. (2011). High-throughput sequencing, characterization and detection of new and conserved cucumber miRNAs. PLoS ONE 6, e19523. doi: 10.1371/journal.pone.0019523
Matranga, C., and Zamore, P. D. (2007). Small silencing RNAs. Curr. Biol. 17, R789–R793. doi: 10.1016/j.cub.2007.07.014
Mi, S., Cai, T., Hu, Y., Chen, Y., Hodges, E., Ni, F., et al. (2008). Sorting of small RNAs into arabidopsis argonaute complexes is directed by the 5' terminal nucleotide. Cell 133, 116-127. doi: 10.1016/j.cell.2008.02.034
Miao, S., Liang, C., Li, J., Baker, B., and Luo, L. (2021). Polycistronic artificial microRNA-mediated resistance to cucumber green mottle mosaic virus in cucumber. Int. J. Mol. Sci. 22, 12237. doi: 10.3390/ijms222212237
Mitter, N., Worrall, E. A., Robinson, K. E., Li, P., Jain, R. G., Taochy, C., et al. (2017a). Clay nanosheets for topical delivery of RNAi for sustained protection against plant viruses. Nat. Plants 3, 16207. doi: 10.1038/nplants.2016.207
Mitter, N., Worrall, E. A., Robinson, K. E., Xu, Z. P., and Carroll, B. J. (2017b). Induction of virus resistance by exogenous application of double-stranded RNA. Curr. Opin. Virol. 26, 49–55. doi: 10.1016/j.coviro.2017.07.009
Moreno, I. M., Thompson, J. R., and García-Arenal, F. (2004). Analysis of the systemic colonization of cucumber plants by Cucumber green mottle mosaic virus. J. Gen. Virol. 85, 749–759. doi: 10.1099/vir.0.19540-0
Necira, K., Makki, M., Sanz-García, E., Canto, T., Djilani-Khouadja, F., and Tenllado, F. (2021). Topical application of Escherichia coli-encapsulated dsRNA induces resistance in Nicotiana benthamiana to potato viruses and involves RDR6 and combined activities of DCL2 and DCL4. Plants 10, 644. doi: 10.3390/plants10040644
Niehl, A., Soininen, M., Poranen, M. M., and Heinlein, M. (2018). Synthetic biology approach for plant protection using dsRNA. Plant Biotechnol. J. 16, 1679–1687. doi: 10.1111/pbi.12904
Padidam, M., Beachy, R. N., and Fauquet, C. M. (1996). The role of AV2 (“precoat”) and coat protein in viral replication and movement in tomato leaf curl geminivirus. Virology 224, 390–404. doi: 10.1006/viro.1996.0546
Pantaleo, V., Szittya, G., and Burgyán, J. (2007). Molecular bases of viral RNA targeting by viral small interfering RNA-programmed RISC. J. Virol. 81, 3797–3806. doi: 10.1128/JVI.02383-06
Pooggin, M., Shivaprasad, P. V., Veluthambi, K., and Hohn, T. (2003). RNAi targeting of DNA virus in plants. Nat. Biotechnol. 21, 131–132. doi: 10.1038/nbt0203-131b
Pugsley, C. E., Isaac, R. E., Warren, N. J., and Cayre, O. J. (2021). Recent advances in engineered nanoparticles for RNAi-mediated crop protection against insect pests. Front. Agron. 3, 652981. doi: 10.3389/fagro.2021.652981
Rank, A. P., and Koch, A. (2021). Lab-to-field transition of RNA spray applications - how far are we?. Front. Plant Sci. 12, 755203. doi: 10.3389/fpls.2021.755203
Rego-Machado, C. M., Nakasu, E., Silva, J., Lucinda, N., Nagata, T., and Inoue-Nagata, A. K. (2020). siRNA biogenesis and advances in topically applied dsRNA for controlling virus infections in tomato plants. Sci. Rep. 10, 22277. doi: 10.1038/s41598-020-79360-5
Ricci, A., Sabbadini, S., Miozzi, L., Mezzetti, B., and Noris, E. (2021). “Host-induced gene silencing and spray-induced gene silencing for crop protection against viruses.,” in RNAi for Plant Improvement and Protection (Wallingford: CABI), 72–85. doi: 10.1079/9781789248890.0072
Rueda, A., Barturen, G., Lebrón, R., Gómez-Martín, C., Alganza, Á., Oliver, J. L., et al. (2015). sRNAtoolbox: an integrated collection of small RNA research tools. Nucleic Acids Res. 43, W467–W473. doi: 10.1093/nar/gkv555
Ruiz, L., Simon, A., Velasco, L., and Janssen, D. (2017). Biological characterization of tomato leaf curl New Delhi virus from Spain. Plant Pathol. 66, 376–382. doi: 10.1111/ppa.12587
Sáez, C., Martínez, C., Ferriol, M., Manzano, S., Velasco, L., Jamilena, M., et al. (2016). Resistance to tomato leaf curl New Delhi virus in Cucurbita spp. Ann. Appl. Biol. 169, 91–105. doi: 10.1111/aab.12283
Sanan-Mishra, N., Abdul Kader Jailani, A., Mandal, B., and Mukherjee, S. K. (2021). Secondary siRNAs in plants: biosynthesis, various functions, and applications in virology. Front. Plant Sci. 12, 10283. doi: 10.3389/fpls.2021.610283
Savary, S., Willocquet, L., Pethybridge, S. J., Esker, P., McRoberts, N., and Nelson, A. (2019). The global burden of pathogens and pests on major food crops. Nat. Ecol. Evol. 3, 430–439. doi: 10.1038/s41559-018-0793-y
Schwartz, S. H., Hendrix, B., Hoffer, P., Sanders, R. A., and Zheng, W. (2020). Carbon dots for efficient small interfering RNA delivery and gene silencing in plants. Plant Physiol. 184, 647–657. doi: 10.1104/pp.20.00733
Seguin, J., Otten, P., Baerlocher, L., Farinelli, L., and Pooggin, M. M. (2016). MISIS-2: a bioinformatics tool for in-depth analysis of small RNAs and representation of consensus master genome in viral quasispecies. J. Virol. Methods 233, 37–40. doi: 10.1016/j.jviromet.2016.03.005
Shen, W., Yang, G., Chen, Y., Yan, P., Tuo, D., Li, X., et al. (2014). Resistance of non-transgenic papaya plants to papaya ringspot virus (PRSV) mediated by intron-containing hairpin dsRNAs expressed in bacteria. Acta Virol. 58, 261–266. doi: 10.4149/av_2014_03_261
Shi, R., and Chiang, V. L. (2005). Facile means for quantifying microRNA expression by real-time PCR. BioTechniques 39, 519–525. doi: 10.2144/000112010
Tellez, M. M., Simon, A., Rodriguez, E., and Janssen, D. (2017). Control of Tomato leaf curl New Delhi virus in zucchini using the predatory mite Amblyseius swirskii. Biol. Control 114, 106–113. doi: 10.1016/j.biocontrol.2017.08.008
Tenllado, F., and Díaz-Ruíz, J. R. (2001). Double-stranded RNA-mediated interference with plant virus infection. J. Virol. 75, 12288–12297. doi: 10.1128/JVI.75.24.12288-12297.2001
Tenllado, F., Llave, C., and Díaz-Ruíz, J. R. (2004). RNA interference as a new biotechnological tool for the control of virus diseases in plants. Virus Res. 102, 85–96. doi: 10.1016/j.virusres.2004.01.019
Tenllado, F., Martínez-García, B., Vargas, M., and Díaz-Ruíz, J. R. (2003). Crude extracts of bacterially expressed dsRNA can be used to protect plants against virus infections. BMC Biotechnol. 3, 3. doi: 10.1186/1472-6750-3-3
Uslu, V. V., and Wassenegger, M. (2020). Critical view on RNA silencing-mediated virus resistance using exogenously applied RNA. Curr. Opin. Virol. 42, 18–24. doi: 10.1016/j.coviro.2020.03.004
Vargason, J. M., Szittya, G., Burgyán, J., and Tanaka Hall, T. M. (2003). Size selective recognition of siRNA by an RNA silencing suppressor. Cell 115, 799–811. doi: 10.1016/S0092-8674(03)00984-X
Vaucheret, H., Béclin, C., and Fagard, M. (2001). Post-transcriptional gene silencing in plants. J. Cell Sci. 114:3083–3091. doi: 10.1242/jcs.114.17.3083
Velasco, L., Ruiz, L., Galipienso, L., Rubio, L., and Janssen, D. (2020). A historical account of viruses in intensive horticultural crops in the Spanish Mediterranean Arc: new challenges for a sustainable agriculture. Agronomy 10, 860. doi: 10.3390/agronomy10060860
Vu, T. V., Choudhury, N. R., and Mukherjee, S. K. (2013). Transgenic tomato plants expressing artificial microRNAs for silencing the pre-coat and coat proteins of a begomovirus, tomato leaf curl New Delhi virus, show tolerance to virus infection. Virus Res. 172, 35–45. doi: 10.1016/j.virusres.2012.12.008
Keywords: RNAi, silencing, dsRNA, CGMMV, ToLCNDV, cucumber, zucchini, vsiRNA
Citation: Delgado-Martín J, Ruiz L, Janssen D and Velasco L (2022) Exogenous Application of dsRNA for the Control of Viruses in Cucurbits. Front. Plant Sci. 13:895953. doi: 10.3389/fpls.2022.895953
Received: 14 March 2022; Accepted: 17 May 2022;
Published: 27 June 2022.
Edited by:
Giorgio Gambino, National Research Council (CNR), ItalyReviewed by:
Zhili Pang, Rutgers, The State University of New Jersey, United StatesGabi Krczal, RLP AgroScience, Germany
Copyright © 2022 Delgado-Martín, Ruiz, Janssen and Velasco. This is an open-access article distributed under the terms of the Creative Commons Attribution License (CC BY). The use, distribution or reproduction in other forums is permitted, provided the original author(s) and the copyright owner(s) are credited and that the original publication in this journal is cited, in accordance with accepted academic practice. No use, distribution or reproduction is permitted which does not comply with these terms.
*Correspondence: Leonardo Velasco, bGVvbmFyZG8udmVsYXNjb0BqdW50YWRlYW5kYWx1Y2lhLmVz