- 1College of Life Sciences, Nanjing Agricultural University, Nanjing, China
- 2Agriculture and Agri-Food Canada, Ottawa Research and Development Centre, Ottawa, ON, Canada
- 3Department of Biology, University of Ottawa, Ottawa, ON, Canada
The type 2C protein phosphatases (PP2Cs) are well known for their vital roles in plant drought stress responses, but their molecular mechanisms in cotton (Gossypium hirsutum L.) remain largely unknown. Here, we investigated the role of three clade A PP2C genes, namely, GhHAI2, GhAHG3, and GhABI2, in regulating the osmotic stress tolerance in cotton. The transcript levels of GhHAI2, GhAHG3, and GhABI2 were rapidly induced by exogenous abscisic acid (ABA) and polyethylene glycol (PEG) treatment. Silencing of GhHAI2, GhAHG3, and GhABI2 via virus-induced gene silencing (VIGS) improved osmotic tolerance in cotton due to decreased water loss, increase in both relative water content (RWC) and photosynthetic gas exchange, higher antioxidant enzyme activity, and lower malondialdehyde (MDA) content. The root analysis further showed that GhHAI2, GhAHG3, and GhABI2-silenced plants were more responsive to osmotic stress. Yeast two-hybrid (Y2H) and luciferase complementation imaging (LCI) assays further substantiated that GhHAI2, GhAHG3, and GhABI2 interact with the core receptors of ABA signaling, GhPYLs. The expression of several ABA-dependent stress-responsive genes was significantly upregulated in GhHAI2-, GhAHG3-, and GhABI2-silenced plants. Our findings suggest that GhHAI2, GhAHG3, and GhABI2 act as negative regulators in the osmotic stress response in cotton through ABA-mediated signaling.
Introduction
Plants are constantly exposed to diverse environmental conditions, such as water deficit, high salinity, flooding, and extreme temperatures, which adversely affect their growth and productivity (Claeys and Inzé, 2013). These abiotic stresses greatly impact plant morphology and cause damage to plant cells. For instance, excessive accumulation of reactive oxygen species (ROS) affects cell membrane stability, reduces photosynthetic efficiency, accelerates protein deformation, and causes leaf wilting (Hanin et al., 2011; Choudhury et al., 2013, 2017). As plants are sessile in nature, various complex mechanisms have been developed to respond appropriately to such harmful conditions. One of these mechanisms is the induction of diverse number of stress-responsive genes (Nakashima and Yamaguchi-Shinozaki, 2006). Previous studies have reported many stress-induced proteins, such as enzymes involved in the ABA signaling pathway, protein phosphatases, numerous protein kinases, osmotic adaptive proteins, cellular protective enzymes, and transcription factors (Zhu, 2002, 2016).
The type 2C protein phosphatases (PP2Cs) belong to a major group of gene families known to mediate abiotic stress signaling pathways and signal transduction processes. PP2Cs have been identified as the first key component of the ABA signaling pathway (Koornneef et al., 1984). The phytohormone ABA plays a vital role in regulation of plant drought stress responses (Yoshida et al., 2014; Zhu, 2016). Genetic studies have reported a canonical mechanism underlying ABA signal transduction in Arabidopsis thaliana. Under water deficit conditions, plants exhibit an elevated level of ABA which is perceived by the pyrabactin resistance 1 (PYR1)/PYR1-like (PYL)/regulatory component of the ABA receptor (RCAR) protein family (Ma et al., 2009; Park et al., 2009). In the absence of ABA, clade A PP2Cs interact with sucrose nonfermenting 1-related protein kinase 2 (SnRK2), thereby preventing their activity by dephosphorylation. ABA perception leads to the binding of PYL receptors to PP2Cs, which release SnRK2 for subsequent phosphorylation of ABA-Responsive Element (ABRE) Binding Factors (ABFs) which regulate transcription of ABA-responsive genes (Cutler et al., 2010; Nakashima and Yamaguchi-Shinozaki, 2013).
In recent years, several studies have functionally characterized PP2C family members, for example, in Arabidopsis, nine PP2C group A members have been identified as component of ABA signaling pathway; ABI1 (ABA insensitive1), ABI2, HAB1 (hypersensitive to ABA1), HAB2, AHG1 (ABA-hypersensitive germination1), AHG3/AtPP2CA, HAI1 (highly ABA-induced) HAI2, and HAI3 (Saez et al., 2004; Schweighofer et al., 2004; Rubio et al., 2009; Antoni et al., 2012; Kim et al., 2013; Rodrigues et al., 2013; Née et al., 2017; Yoshida et al., 2019). Double mutant plants of abi1 and abi2 were responsive to ABA, suggesting that ABI1 and ABI2 function negatively in ABA signaling pathway (Merlot et al., 2001). Under ABA treatment, ahg1 and ahg3 double mutants displayed stronger phenotypes than single parental mutant, implying that AHG1 and AHG3 function together in regulating ABA signaling pathway (Nishimura et al., 2007). Similarly, all members of PP2C-A were upregulated when subjected to exogenous ABA; however, HAI1, HAI2, and HAI3 expressions were strongly induced in vegetative phase (Fujita et al., 2009). Likewise, transgenic Arabidopsis overexpressing rice clade A OsPP108 showed enhanced tolerance under salt, mannitol, and drought stress, but reduction in ABA sensitivity (Singh et al., 2015). The overexpression of OsABIL2, which encodes another rice clade A of PP2C, exhibits ABA insensitivity and significantly altered phenotypes, such as stomatal density and root architecture, leading to the drought hypersensitivity (Li et al., 2015). Similarly, maize ZmPP2C-As were recently characterized and their role in drought tolerance were elucidated. For instance, Arabidopsis plants overexpressing ZmPP2C-2A and ZmPP2C-6A were sensitive to drought stress, suggesting their negative role in drought stress response (He et al., 2019). Also, transgenic studies in maize and Arabidopsis verified that ZmPP2C-A10 function as negative regulator of drought tolerance (Xiang et al., 2017). So far, using bioinformatics analysis, the PP2C gene family has been identified in several species, including Arabidopsis (Schweighofer et al., 2004), rice (Xue et al., 2008), cotton (Shazadee et al., 2019), wheat (Yu et al., 2019), soybean (Chen et al., 2018), and Chinese cabbage (Khan et al., 2019). However, the functional characterization of PP2C genes in cotton remains largely obscure.
Cotton (Gossypium hirsutum L.) is one of the most important fiber and oil crops, commercially grown worldwide. Various abiotic stresses particularly drought stress greatly affect cotton growth and limit fiber yield and lint quality, resulting in a significant production losses (Pettigrew, 2004). Moreover, 57% of global cotton is grown in regions with high water stress (WRI, 2013). Thus, development of drought resistant cotton cultivars and improving water use efficiency are crucial to sustain the cotton industry. In a previous study, we identified 18 Clade A PP2Cs in cotton (G. hirsutum; Shazadee et al., 2019) but their functional role was unexplored. It has been recently reported (Lu et al., 2019) and observed that the expression of clade A PP2Cs is highly induced under osmotic and ABA treatment in cotton. These observations suggest a role of cotton clade A PP2Cs in abiotic stress; however, no stress-related phenotype has been associated with group A PP2Cs in cotton yet. Hence, it is important to further investigate the molecular mechanism of clade A PP2Cs in response to drought stress in cotton.
In this study, we characterized three members of clade A PP2Cs; GhHAI2, GhAHG3, and GhABI2 in cotton in order to investigate their roles in drought tolerance. The GhHAI2, GhAHG3, and GhABI2 were highly induced by ABA and PEG treatment. Silencing of GhHAI2, GhAHG3, and GhABI2 improved osmotic tolerance of cotton plants. Yeast two-hybrid (Y2H) and luciferase complementation imaging (LCI) assays revealed that GhHAI2, GhAHG3, and GhABI2 interact with GhPYLs and regulate ABA signaling pathway. Furthermore, we demonstrated that GhHAI2-, GhAHG3-, and GhABI2-silenced plants increased the expression of ABA-dependent stress-responsive genes. In brief, our results suggest that GhHAI2, GhAHG3, and GhABI2 function as crucial negative regulators in osmotic stress response by an ABA-dependent pathway indicating their potential roles in drought tolerance.
Materials and Methods
Plant Material and Stress Treatment
For expression analysis of GhHAI2, GhAHG3, and GhABI2 in cotton, seedlings were planted in Hoagland nutrient solution with a 16 h/8 h light/dark cycle at 25°C. Then, three-week-old seedlings were subjected to 15% PEG 6000 for osmotic treatment. For ABA treatment, the plants were sprayed with 200 μm ABA. Leaves were collected after stress treatment at the designated time (0, 6, 12, 24, and 48 h).
For evaluation of osmotic stress tolerance, G. hirsutum, cultivar Xinluzao, plants were used to perform the experiments. Cotton plants were grown in a growth chamber under a 16 h/8 h light/dark cycle at 25°C. Pot-grown cotton seedlings at two true leaves stage were treated with 15% PEG for osmotic stress, and in parallel, water treatment was used as mock control. After PEG stress, plant leaves were collected at different time points, immediately frozen in liquid nitrogen and stored at −80°C until further use. Root assay was performed after 7 days of osmotic stress. Each of the experiments was performed in triplicate.
Cloning and Sequence Analysis of GhHAI2, GhAHG3, and GhABI2
We obtained the full-length ORFs of GhHAI2, GhAHG3, and GhABI2 via PCR; the primers were designed using the coding sequence of GhHAI2, GhAHG3, and GhABI2 (Supplementary Table S1). Alignment of cotton GhHAI2, GhAHG3, and GhABI2 and Arabidopsis HAI2, AHG3, and ABI2 was performed with ClustalW (Thompson et al., 1994). The MEGA program (version 7.0) was used to construct the phylogenetic tree via the Neighbor-Joining (NJ) method and 1,000 bootstrap replications.
Subcellular Localization
The coding regions of GhHAI2, GhAHG3, and GhABI2 were amplified by PCR and inserted into the pBin-GFP4 (green fluorescent protein) expression vector. The three vectors were separately introduced into Agrobacterium tumefaciens strain GV3101 cells and transiently expressed in Nicotiana benthamiana leaf cells via A. tumefaciens infiltration method. After 3 days of infiltration, fluorescence signals were detected using a confocal laser-scanning microscope (Zeiss, LSM710).
Agrobacterium tumefaciens-Mediated VIGS
Inserts to generate TRV2:GhHAI2, TRV2:GhAHG3, TRV2:GhABI2, and positive control TRV2:GhCLA1 were amplified from G. hirsutum cultivar Xinluzao cDNA with primers containing the restriction sites EcoRI and XhoI. The primers for cloning are listed in Supplementary Table S1. Vectors constructed in binary tobacco rattle virus (TRV) vector, including pTRV1 and pTRV2 (GhHAI2, GhAHG3, and GhABI2 and GhCLA1), were introduced into A. tumefaciens strain GV3101 by electroporation. The Agrobacteria culture carrying the above pTRV1 and pTRV2 constructs was infiltrated into two fully expanded cotyledons of seven-day-old cotton plants using a needle-less syringe as previously described (Gao et al., 2011, 2013). The GhCLA1 construct was used as a visual marker to determine VIGS efficiency. After 14 days of Agrobacteria inoculation, the silenced plants were subjected to 15% PEG treatment for the indicated times. VIGS experiments were repeated three times with more than 30 plants for each construct per replicate.
Measurement of Water Loss and RWC
For relative water content (RWC) measurement, six leaves were detached from individual cotton plants and the fresh weight (FW) was recorded. To record turgid weight (TW), the leaves were soaked in distilled water for 4 h at room temperature with constant light. The leaves were then dried at 65°C for 24 h to obtain the dry weight (DW). RWC was calculated using the formula: RWC (%) = [(FW−DW)/(TW−DW)] × 100. To measure water loss, aerial parts of six cotton seedlings were detached and placed on clean filter paper on a laboratory bench. At various time intervals, the total FW was recorded. Water loss was calculated as the decrease in fresh weight as a percentage of the initial fresh weight of the detached seedlings parts. Both assays were performed in three biological repeats.
Gas Exchange
Gas exchange measurements were taken from three-week-old cotton plants under normal and PEG conditions. The photosynthetic rate (A, μmol CO2 m−2 s−1), stomatal conductance (gs, mol H2O m−2 s−1), intercellular CO2 concentration (Ci, μmol CO2 mol−1), and transpiration rate (E, mmol H2O m−2 s−1) were measured with a portable photosynthesis system Li-6400XT (Li-COR Inc., United States) under 1,500 μmol m−2 s−2 light intensity, 23°C ± 2°C temperature, and 300 μmol mol−1 CO2 concentration. For each gene, at least seven biological replicates per treatment were measured.
Measurement of Antioxidant Enzymes and MDA Content
Fresh leaves of cotton plants under normal and PEG conditions were used for the measurement of antioxidant enzymes activity and Malondialdehyde (MDA) content. The peroxidase (POD; U mg−1 protein), superoxide dismutase (SOD; U mg−1 protein), catalase (CAT; U mg−1 protein) activities, and MDA (nmol g−1) content were determined using analytical kits (Nanjing Jiancheng Bioengineering Institute, Nanjing, China) following the manufacturer’s protocol as described (Kumar et al., 2020). Three biological replicates were used to investigate each physiological index.
RNA Extraction, First-Strand cDNA Synthesis, and qPCR
Total RNA was extracted from various organs of cotton plants using Biospin plant Total RNA Extraction Kit (Bioer technology, Hangzhou, China) according to the manufacturer’s protocol. gDNase-treated RNA was reverse transcribed to generate first-strand cDNA using Prime Script™ RT Reagent Kit (TaKaRa, United States). Gene expression levels were determined using qPCR assay, which was conducted with SYBR® Premix Ex Taq™ (TaKaRa, United States) and an ABI 7300 qPCR System (Applied Biosystems, CA, United States). The real-time PCR amplification reactions are briefly described in our previously reported study (Shazadee et al., 2019). The 2-ΔΔCt method was used to determine relative expression level (Livak and Schmittgen, 2001). Cotton histone3 (AF024716) gene was used as an internal control. All the primers were designed using Primer Blast in NCBI (Supplementary Table S1). Three biological replicates, each containing three technical replicates, were used for each sample.
Y2H Assay
The Y2H assay was based on the Matchmaker GAL4 two-hybrid system (Clontech, United States). The GhHAI2, GhAHG3, and GhABI2 and GhPYLs coding regions were independently cloned into the pGBKT7 (BD) and pGADT7 (AD) vectors, respectively. The construct pairs were co-transformed into AH109 yeast strain cells and grown on SD/−Trp/−Leu/-His/−Ade (SD/-QDO) medium containing 5-bromo-4-chloro-3-indolyl-alpha- D-galactopyranoside (X-α-gal). Photographs of resulting yeast growth were taken 3 days after incubation.
LCI Assay
For luciferase complementation imaging (LCI) assay, the open reading frames of GhHAI2, GhAHG3, and GhABI2 and GhPYLs without stop codons were cloned into pCAMBIA1300-CLuc or pCAMBIA1300-NLuc vectors using KpnI and BamHI or BamHI and SalI restriction sites, respectively. The fusion constructs were then transformed to A. tumefaciens GV3101. The suspensions were prepared by mixing the three Agrobacterium strains carrying the CLuc, and NLuc fusion and the gene silencing inhibitor p19 at a 1:1:1 ratio. For transient expression, the bacterial mixture was infiltrated into different locations on the same N. benthamiana leaves using a needle-less syringe. To measure LUC activity, 1 mM luciferin was sprayed into the leaves and kept in the dark for 5 min to quench the fluorescence. A low-light cooled CCD imaging apparatus was used to capture the LUC image at 3 min intervals.
Statistical Analyses
All data are represented herein as the means ± standard deviations. The significant levels were determined by using Student’s t-test: *p < 0.05; **p < 0.01.
Results
Induction of GhHAI2, GhAHG3, and GhABI2 After Exposure to PEG and ABA
In an RNA-seq assay and our previously reported study (Zhang et al., 2015; Shazadee et al., 2019), several PP2C genes were identified that were induced in response to osmotic stress. Of these candidate genes, we selected three strongly induced genes for their functional characterization in order to confirm their potential role in osmotic tolerance. Phylogenetic analysis revealed that these PP2Cs belong to clade A and share a close relationships with Arabidopsis HAI2, AHG3, and ABI2 (Figure 1A). Based on their similarity with Arabidopsis, we renamed them as GhHAI2, GhAHG3, and GhABI2, respectively. The deduced amino acid sequence of GhHAI2, GhAHG3, and GhABI2 encodes a protein of 417, 409, and 471 amino acids, and shares 56, 59, and 54% sequence similarity with Arabidopsis HAI2, AHG3, and ABI2, respectively (Figure 1B). The Arabidopsis clade A PP2Cs, HAI2, AHG3, and ABI2 have been known to function as negative regulators in ABA signaling pathway (Merlot et al., 2001; Leonhardt et al., 2004; Nishimura et al., 2007; Xue et al., 2008; Bhaskara et al., 2012). Therefore, we predicted that GhHAI2, GhAHG3, and GhABI2 might show a similar expression pattern to Arabidopsis HAI2, AHG3, and ABI2 and function in osmotic stress response in cotton.
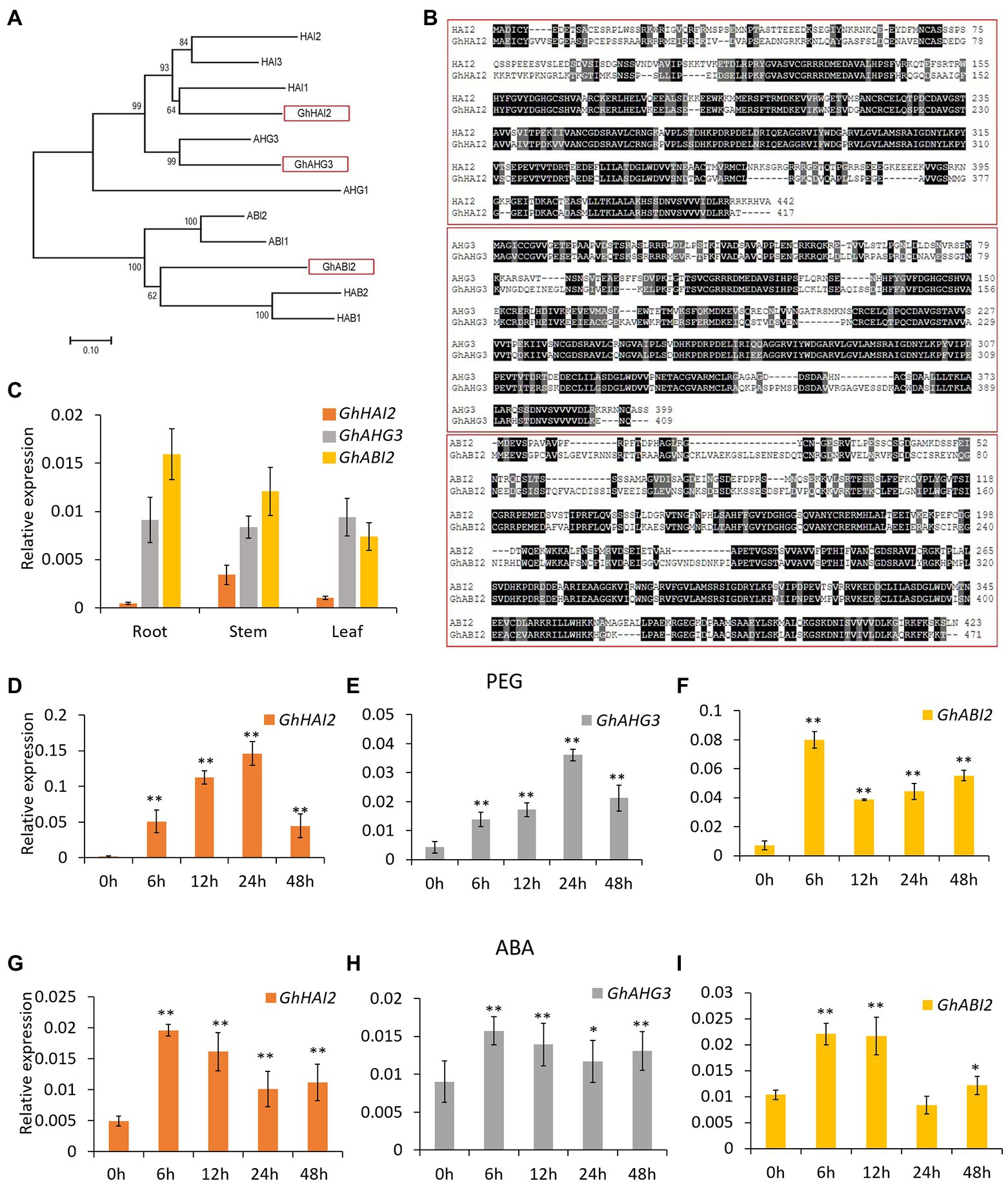
Figure 1. Sequence analysis and expression pattern of GhHAI2, GhAHG3, and GhABI2 in different tissues and after exposure to PEG and ABA. (A) Phylogenetic analysis of GhHAI2, GhAHG3, and GhABI2 and Arabidopsis group A PP2Cs. The neighbor-joining tree was constructed using MEGA software (version 7.0) based on multiple alignments of GhHAI2, GhAHG3, and GhABI2 protein sequence and their homologous proteins in Arabidopsis. (B) Comparisons of deduced amino acid sequence of GhHAI2, GhAHG3, and GhABI2 with those of Arabidopsis. Identical amino acid residues are highlighted in black. (C) qPCR analysis of GhHAI2, GhAHG3, and GhABI2 genes expression levels in three different tissues. (D–F) PEG- and (G–I) ABA-induced expression pattern of GhHAI2, GhAHG3, and GhABI2 genes. Bars represent means ± standard deviation of three biological replicates. Asterisks represent statistically significant differences compared with untreated control (0 h; Student’s t-test; *p < 0.05; **p < 0.01).
qPCR analysis revealed that GhHAI2, GhAHG3, and GhABI2 genes are constitutively expressed in root, stem, and leaf tissues; however, the expression level of GhHAI2 was relatively lower (Figure 1C). To verify that GhHAI2, GhAHG3, and GhABI2 respond to osmotic stress, we monitored their expression levels in cotton leaves after exposure to PEG and ABA using qPCR. Before treatment, the GhHAI2, GhAHG3, and GhABI2 expressions were weakly induced. However, PEG and ABA triggered a significant accumulation of GhHAI2, GhAHG3, and GhABI2 transcripts within 6–48 h (Figures 1D–I). These results suggested that GhHAI2, GhAHG3, and GhABI2 expressions are strongly induced after PEG and ABA treatment indicating that these genes could function in response to dehydration in cotton.
Subcellular Localization of GhHAI2, GhAHG3, and GhABI2
To investigate the subcellular localization of GhHAI2, GhAHG3, and GhABI2, we fused their coding regions to the green fluorescent protein (GFP) reporter gene under the control of the 35S promoter. We found that the transient expression of the 35S:GhHAI2-GFP, 35S:GhAHG3-GFP, and 35S:GhABI2-GFP fusion proteins in N. benthamiana leaves generated GFP signals in the nucleus. Using the 4′,6-diamidino-2-phenylindole (DAPI) staining, we observed that the blue signal localized to the nucleus overlapped with the GFP signals (Figure 2). These results indicate that GhHAI2, GhAHG3, and GhABI2 have a functional role in the nucleus.
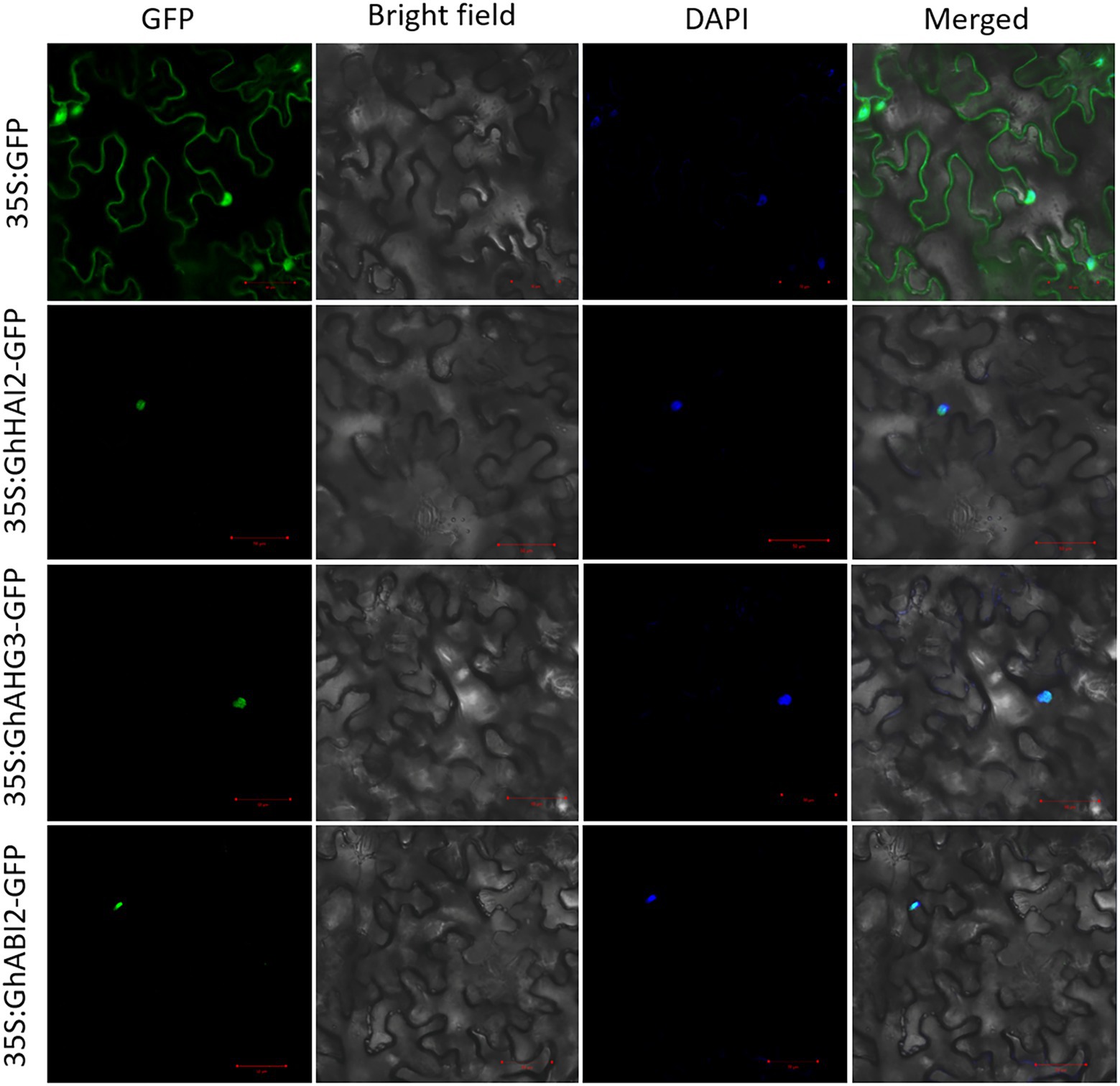
Figure 2. Subcellular localization of GhHAI2, GhAHG3, and GhABI2 based on the transient expression of green fluorescent (GFP) fusion protein in the epidermal cells of N. benthamiana. The 35S:GhHAI2-GFP, 35S:GhAHG3-GFP, and 35S:GhABI2-GFP constructs were expressed in the leaves of N. benthamiana using agroinfiltration method. The GFP signal was observed using a confocal laser-scanning microscope. The DAPI staining was used as nucleus marker.
Silencing of GhHAI2, GhAHG3, and GhABI2 Confers Osmotic Stress Tolerance in Cotton
To investigate the biological function of GhHAI2, GhAHG3, and GhABI2 in responding to osmotic stress, we performed TRV-based virus-induced gene silencing (VIGS). Seven-day-old plants were hand-infiltrated using Agrobacterium cultures carrying VIGS vectors into cotton cotyledons. At 14 days post-infiltration (dpi), plants inoculated with TRV2:GhCLA1, a chlorophyll biosynthesis gene, exhibited obvious albino phenotype, which was uniformly distributed on entire true leaves (Supplementary Figure S1A). The expression levels of GhHAI2, GhAHG3, and GhABI2 were significantly reduced in the silenced plants (TRV2:GhHAI2, TRV2:GhAHG3, and TRV2:GhABI2) than in control plants (TRV2:00; Supplementary Figures S1B–D). Subsequently, the VIGS plants were subjected to 15% PEG for 18 days and then re-watering for two days. Under well-watered conditions, no obvious difference was observed between TRV2:00 and TRV2:GhHAI2, TRV2:GhAHG3, and TRV2:GhABI2 plants. However, after 10 days of PEG stress, TRV2:00 plants displayed serious wilting sooner than TRV2:GhHAI2, TRV2:GhAHG3, and TRV2:GhABI2 plants. After further 8 days of water deficit condition and re-watering, GhHAI2-, GhAHG3-, and GhABI2-silenced plants displayed a stronger osmotic-tolerant phenotype in comparison with control plants (Figure 3A). At two days after re-watering, the survival rates of TRV2:GhHAI2, TRV2:GhAHG3, and TRV2:GhABI2 plants were 53, 72, and 68%, respectively, whereas only 27% of TRV2:00 plants survived (Figure 3B). To determine whether the resistant phenotype to PEG stress exhibited by GhHAI2-, GhAHG3-, and GhABI2-silenced plants was caused by more water retention capacity, the water loss rate and RWC of detached cotton seedlings and leaves were measured. Consistently, the water loss was significantly lower in TRV2:GhHAI2, TRV2:GhAHG3, and TRV2:GhABI2 plants compared with TRV2:00 plants (Figure 3C). In addition, silencing of GhHAI2, GhAHG3, and GhABI2 under PEG conditions caused more RWC (93, 94, and 94%, respectively) than TRV2:00 plants (72%) and well-watered conditions (Figure 3D).
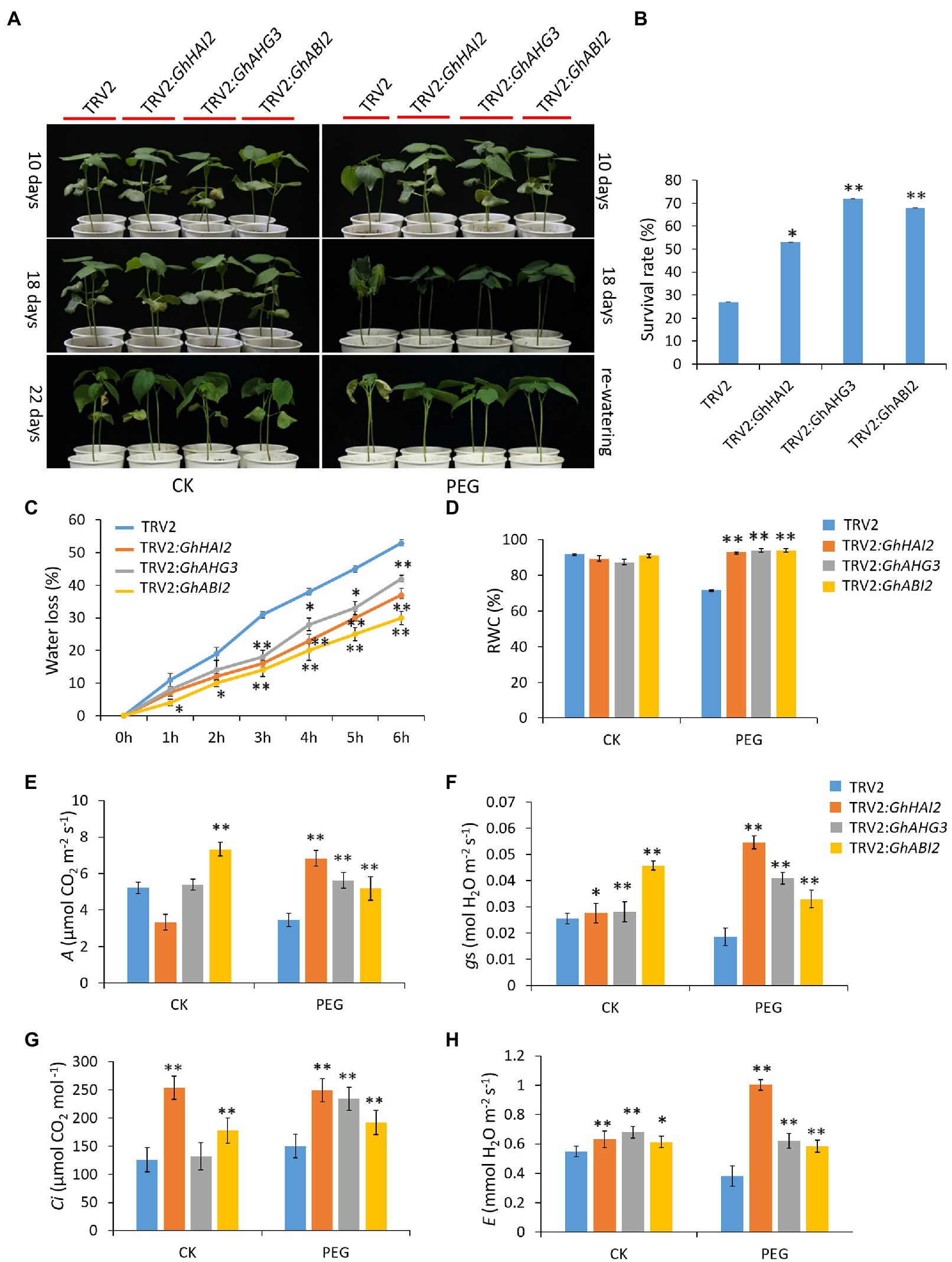
Figure 3. GhHAI2, GhAHG3, and GhABI2 genes negatively regulate cotton osmotic response. (A) Phenotypic observations on the GhHAI2-, GhAHG3-, and GhABI2-silenced and control plants under normal (left panel) and PEG conditions (right panel). Three-week old plants were subjected to PEG treatment for 18 days and re-watered for 2 days. (B) Percentage of surviving plants after re-watering for 2 days. (C) Water loss from TRV2:GhHAI2, TRV2:GhAHG3, and TRV2:GhABI2 and TRV2:00 seedlings under PEG conditions. (D) Relative water content (RWC) in the leaves of TRV2:GhHAI2, TRV2:GhAHG3, and TRV2:GhABI2 and TRV2:00 plants under normal and PEG conditions. (E–H) Analyses of gas exchange parameters including, photosynthetic rate (A), stomatal conductance (gs), intracellular CO2 concentration (Ci), and transpiration rate (E) in TRV2:GhHAI2, TRV2:GhAHG3, and TRV2:GhABI2 and TRV2:00 plants under normal and PEG conditions. Bars represent means ± standard deviation of three biological replicates. At least 30 plants were used for each biological replicate. Asterisks represent statistically significant difference between TRV2:GhHAI2, TRV2:GhAHG3, and TRV2:GhABI2 and TRV2:00 plants (Student’s t-test; *p < 0.05; **p < 0.01).
Furthermore, to determine whether the osmotic tolerance in GhHAI2-, GhAHG3-, and GhABI2-silenced plants is associated with the alterations in leaf physiology, we compared photosynthetic gas exchange between TRV2:GhHAI2, TRV2:GhAHG3, and TRV2:GhABI2 plants and TRV2:00 plants. Compared with normal watering conditions and TRV2:00 plants after PEG, all three genes silenced plants showed significantly increased photosynthetic rates that were approximately double than that of control plants (Figure 3E). Similarly, significant inductions in stomatal conductance, intracellular CO2, and transpiration rate were also observed, indicating that GhHAI2-, GhAHG3-, and GhABI2-silenced plants were more tolerant to osmotic stress than control plants (Figures 3F–H). Taken together, these results indicate that silencing of GhHAI2, GhAHG3, and GhABI2 in cotton plants significantly improved osmotic stress response.
Silencing of GhHAI2, GhAHG3, and GhABI2 Probably Promotes ROS Scavenging and Increases Root Biomass
In order to determine whether antioxidative mechanism is involved in GhHAI2, GhAHG3, and GhABI2 osmotic stress response, we detected the activity of three significant antioxidant enzymes in TRV2:00 and TRV2:GhHAI2, TRV2:GhAHG3, and TRV2:GhABI2 plants. Under both normal and PEG conditions, peroxidase (POD) superoxide dismutase (SOD) and catalase (CAT) activity increased much more in GhHAI2-, GhAHG3-, and GhABI2-silenced plants compared with the control plants (Figures 4A–C). Further, the MDA, which is a byproduct of lipid peroxidation under oxidative stress, was measured and compared between normal and PEG stressed plants. The MDA content was significantly lower in GhHAI2-, GhAHG3-, and GhABI2-silenced plants under PEG conditions compared to the control plants, indicating that silenced plants were more tolerant to osmotic stress (Figure 4D). These results showed that TRV2:00 plants were more severely damaged by ROS, while silencing of GhHAI2, GhAHG3, and GhABI2 protected the plants from damage. Hence, GhHAI2, GhAHG3, and GhABI2 negatively participated in the ROS scavenging pathway by increasing the activity of POD, SOD, and CAT and decreasing the MDA level in the antioxidant system under osmotic stress.
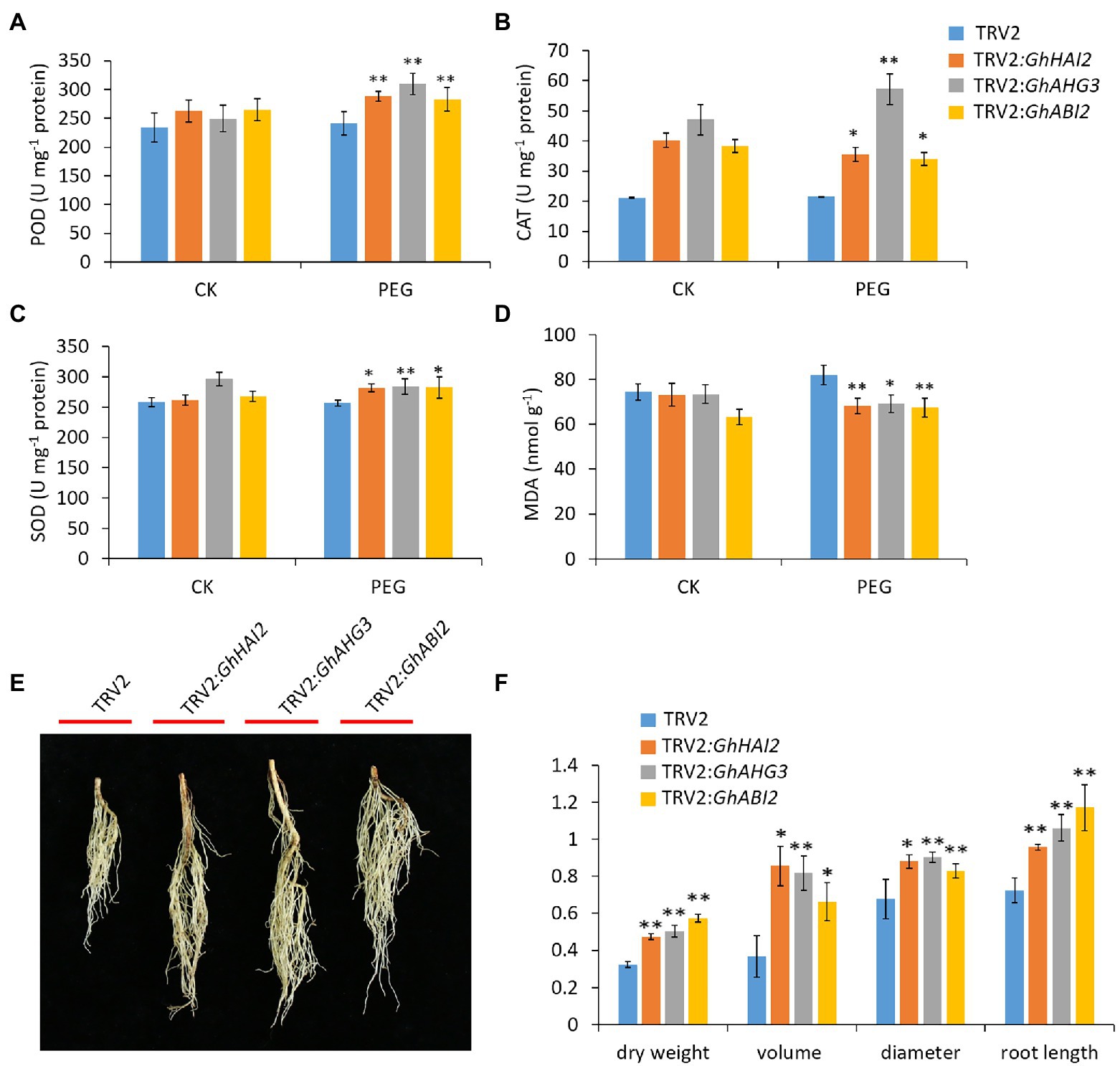
Figure 4. Silencing of GhHAI2, GhAHG3, and GhABI2 promotes ROS scavenging and increases root biomass. (A–D) Analyses of three antioxidant enzymes; SOD, POD, and CAT activities and MDA content in TRV2:GhHAI2, TRV2:GhAHG3, and TRV2:GhABI2 and TRV2:00 plants under normal and PEG conditions. (E) Root phenotype and (F) the root dry weight, volume, diameter, and length of TRV2:GhHAI2, TRV2:GhAHG3, and TRV2:GhABI2 and TRV2:00 plants under PEG conditions. Bars represent means ± standard deviation of three biological replicates. Asterisks represent statistically significant difference between TRV2:GhHAI2, TRV2:GhAHG3, and TRV2:GhABI2 and TRV2:00 plants (Student’s t-test; *p < 0.05; **p < 0.01).
Furthermore, VIGS cotton plants exhibited significant variation in root length and biomass accumulation. We measured the root volume, dry weight, length, and diameter of GhHAI2-, GhAHG3-, and GhABI2-silenced and control plants under PEG conditions. The roots of GhHAI2-, GhAHG3-, and GhABI2-silenced plants were denser and the volume, length, and dry weight increased relative to that of control plants. Thus, under PEG conditions, the silenced plants had more vigorous root phenotypes than the control plants, which is consistent with enhanced osmotic tolerance (Figures 4E,F).
GhHAI2, GhAHG3, and GhABI2 Interact With Cotton ABA Receptors GhPYLs
In Arabidopsis, physical protein–protein interaction between clade A PP2Cs and ABA receptors PYL/PYR/RCARs is one of the principal regulatory mechanisms of ABA signaling (Santiago et al., 2012). Hence, we hypothesized that GhHAI2, GhAHG3, and GhABI2 might interact with cotton ABA receptors and therefore performed a Y2H assay. Cotton possesses 40 PYL proteins, of which we selected GhPYL4, GhPYL6, GhPYL9-4D, and GhPYL9-6A (Zhang et al., 2017) (hereafter referred to as GhPYLs). GhHAI2, GhAHG3, and GhABI2 were fused to the binding domain of GaL4 and used as bait, while GhPYLs fused to the activation domain of GaL4 and were used as prey proteins. To ensure that GhHAI2, GhAHG3, and GhABI2 alone were not able to activate the yeast reporter genes, the bait constructs were first evaluated for self-activation. The preliminary experiment revealed that there was no auto-activation of reporter genes (Figure 5A); therefore, full-length sequences of GhHAI2, GhAHG3, and GhABI2 were used to perform Y2H. The GhHAI2, GhAHG3, and GhABI2 and GhPYLs were co-transformed in a pairwise fashion into yeast. Co-transformants expressing bait and prey were able to grow on SD/−Trp/−Leu/-His/−Ade (SD/-QDO) selection medium containing X-α-galactosidase (X-α-gal), indicating the direct interaction of GhHAI2, GhAHG3, and GhABI2 and GhPYLS. Of the all co-transformants, GhAHG3/GhPYL4 and GhABI2/GhPYL4 did not grow on the selection medium, suggesting that GhAHG3 and GhABI2 do not directly interact with GhPYL4 (Figure 5B).
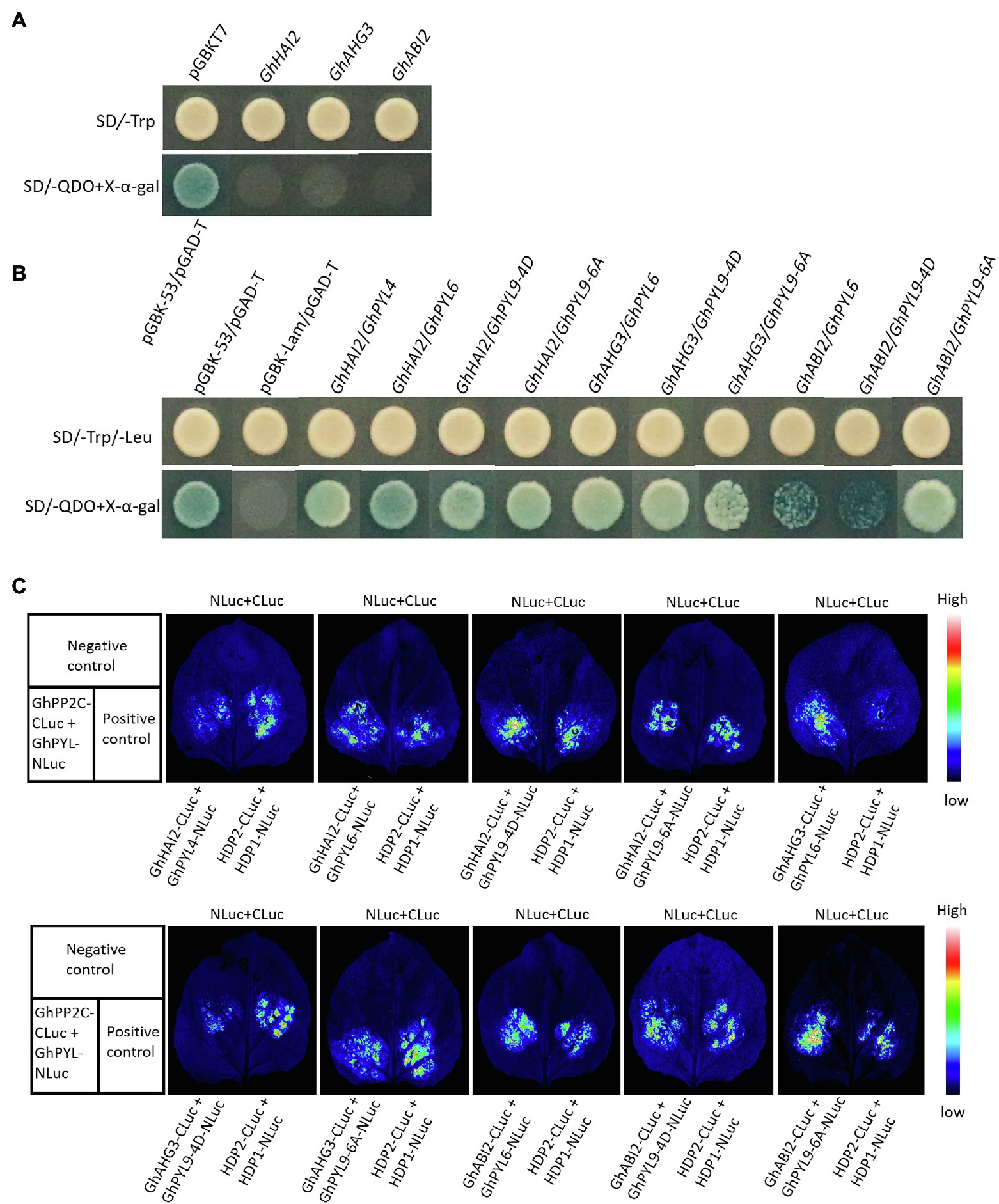
Figure 5. Interaction between GhHAI2, GhAHG3, and GhABI2 and ABA receptors GhPYLs. (A) Determination of self-activation of GhHAI2, GhAHG3, and GhABI2 in the absence of prey. (B) Interaction of GhHAI2, GhAHG3, and GhABI2 and GhPYLs in yeast two-hybrid assay (Y2H). The yeast strain AH109 harboring the construct pairs were plated on synthetic dropout medium either without Trp and Leu (upper panel) or without Trp, Leu, His, and Ade (SD/-QDO) containing X-α-gal (lower panel). The vectors pGBKT7-53/pGADT7-T and pGBKT7-Lam/pGADT7-T were used as positive and negative controls, respectively. (C) Luciferase (LUC) complementation imaging assay for analyzing the protein–protein interaction between GhHAI2, GhAHG3, and GhABI2 and GhPYLs. The specific combinations used for each interaction are indicated. The fluorescence signals represent their interaction activities.
The binary protein–protein interactions were further examined in planta using LCI assays. Co-expression of GhHAI2-CLuc, GhAHG3-CLuc, and GhABI2-CLuc and GhPYLs-NLuc in N. benthamiana strongly complemented the LUC activity similar to the positive control HDP2-CLuc/HDP1-NLuc, confirming the interaction between GhHAI2, GhAHG3, and GhABI2 and GhPYLs (Figure 5C). The negative control (NLuc/CLuc) showed no visible fluorescence in tobacco leaves. Collectively, these results suggest that GhHAI2, GhAHG3, and GhABI2 interact with cotton GhPYLs and are involved in ABA signaling pathway.
GhHAI2, GhAHG3, and GhABI2 Regulate ABA-Dependent Stress-Responsive Gene Expression
Previous studies have revealed that the phytohormone ABA is essential for plant drought response (Xiong et al., 2006). Since clade A PP2C family genes have been reported to regulate plant drought stress in an ABA-dependent manner (Schweighofer et al., 2004; Antoni et al., 2012; Rodrigues et al., 2013); thus, in order to further confirm the role of GhHAI2, GhAHG3, and GhABI2 in ABA pathway, we detected the expression level of ABA-dependent genes in GhHAI2-, GhAHG3-, and GhABI2-silenced cotton leaves via qPCR. Notably, silencing of GhHAI2, GhAHG3, and GhABI2 exerted dramatically upregulated expression in GhABF1, GhABF2, and GhABF3 (GhABFs) in response to osmotic stress (Figures 6A–C). However, silencing of GhHAI2, GhAHG3, and GhABI2 had less effect on the induction of cotton dehydration-responsive element-binding protein 2 (GhDREB2) after osmotic stress (Figure 6D). ABFs and DREBs are the two major groups of transcription factors that are involved in the ABA-dependent and ABA-independent drought responses, respectively (Yoshida et al., 2014). Apparently, GhHAI2, GhAHG3, and GhABI2 exerted a stronger effect on osmotic-induced expression of GhABFs than that of GhDREB2, suggesting that GhHAI2, GhAHG3, and GhABI2 regulate cotton osmotic response in ABA-dependent manner. Based on this, we next examined the expression levels of ABA biosynthesis genes in GhHAI2-, GhAHG3-, and GhABI2-silenced cotton leaves under osmotic conditions. GhNCED3a and GhNCED3c are the orthologues of Arabidopsis key ABA biosynthetic gene AtNCED3 in cotton (Jensen et al., 2013; Shang et al., 2020). Consistently, the transcripts of GhNCED3a and GhNCED3c were found to be significantly upregulated in GhHAI2-, GhAHG3-, and GhABI2-silenced plants after osmotic stress, compared to the control plants (Figures 6E,F). According to these observations, GhHAI2, GhAHG3, and GhABI2 modulate osmotic stress by regulating ABA signaling pathway, probably by targeting ABA-dependent stress-responsive and ABA biosynthesis genes.
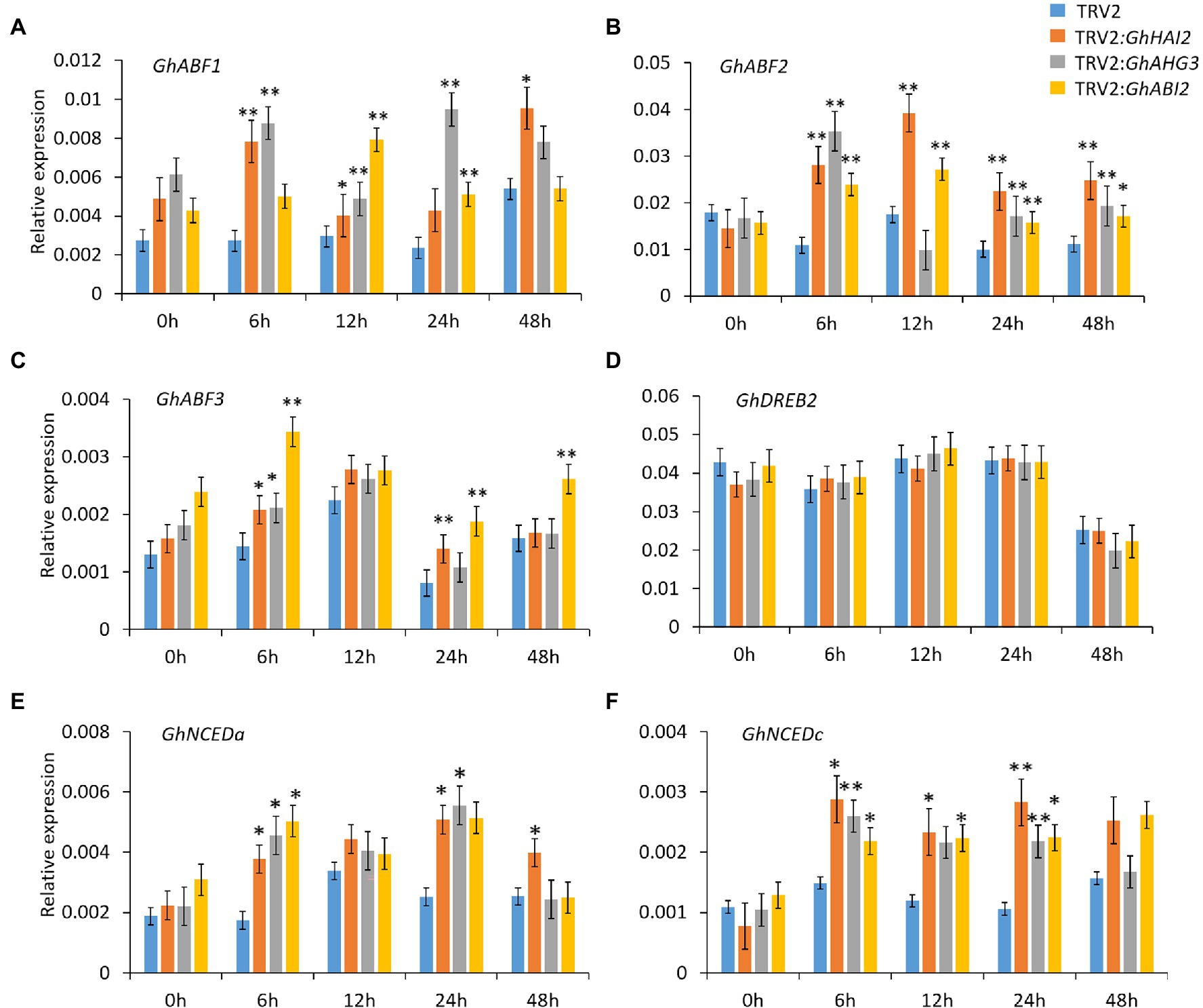
Figure 6. GhHAI2, GhAHG3, and GhABI2 regulate ABA-dependent gene expression. (A–C) qPCR expression analysis of ABA-dependent and (D) ABA-independent stress-responsive genes in TRV2:GhHAI2, TRV2:GhAHG3, and TRV2:GhABI2 and TRV2:00 plants under PEG conditions. (E,F) Expression levels of ABA biosynthesis genes in TRV2:GhHAI2, TRV2:GhAHG3, and TRV2:GhABI2 and TRV2:00 plants under PEG conditions. The transcript levels were normalized by GhHistone. Bars represent means ± standard deviation of three biological replicates. Asterisks represent statistically significant difference between TRV2:GhHAI2, TRV2:GhAHG3, and TRV2:GhABI2 and TRV2:00 plants (Student’s t-test; *p < 0.05; **p < 0.01).
Discussion
With rising water scarcity and global climate change, drought is emerging as a significant factor for limiting crop production (Zhu, 2002). Particularly, cotton production is challenged by drought stress, the reason for this may be due to more than half of the world’s cotton is produced in high water-stressed regions. Thus, there is an imperative need of understanding the molecular and genetic basis underlying cotton drought response for the development of cultivars with improved tolerance.
The plant hormone ABA plays a crucial role in many plant cellular processes, such as growth, development, and adaptation to abiotic stress (Osakabe et al., 2014). Under water deficit conditions, ABA regulates abiotic stress responses by inducing a large number of stress-related genes (Kilian et al., 2007). Previous studies have investigated the ABA signaling pathway from perception of ABA to response to stimulus (Lee and Luan, 2012). The RCAR-PP2C-SnRK2 cascade is the most well-studied ABA signaling pathway (Cutler et al., 2010). The perception of ABA by receptors and other signaling components including PP2Cs and SnRKs is critical for abiotic stress adaptation (Santiago et al., 2009; Vlad et al., 2009; Gonzalez-Guzman et al., 2012; Lim et al., 2013; Ding et al., 2015). The Arabidopsis group A PP2Cs have been known to function as negative regulators in ABA pathway (Saez et al., 2004; Schweighofer et al., 2004). Similarly, cotton plants presumably possess a number of drought and ABA-induced PP2Cs (Lu et al., 2019). To comprehend the importance of PP2C gene family in drought tolerance, we characterized three cotton clade A PP2Cs; GhHAI2, GhAHG3, and GhABI2, all of which have high sequence similarity to the Arabidopsis homologs HAI2, AHG3, and ABI2, respectively. We showed that GhHAI2, GhAHG3, and GhABI2 are nuclear-localized proteins which are responsive to PEG and ABA treatment in cotton plants. The reason for this may be due to similar functions to Arabidopsis group A PP2Cs for inducing altered phenotypes in response to ABA and drought stress, as well as their interactions with ABA signaling components.
To elucidate the role of GhHAI2, GhAHG3, and GhABI2, we conducted VIGS genetic analysis in cotton plants. In our phenotypic assays, GhHAI2-, GhAHG3-, and GhABI2-silenced plants displayed a pronounced osmotic-tolerant phenotype, which was accompanied by high RWC and increased photosynthetic gas exchange. Hence, these parameters collectively suggest that reduced expression of GhHAI2, GhAHG3, and GhABI2 enhance the cotton plants resistance to osmotic stress.
Abiotic stresses led to oxidative damage and accumulation of MDA in plants (Alexieva et al., 2001; Hu et al., 2012). In cotton, drought stress caused the production of ROS. While, the antioxidant enzyme activity increased and regulated the ROS scavenging mechanism until the plants recovered from the stress (Ratnayaka et al., 2003). CsATAF1-OE plants promoted drought tolerance by enhancing the activity of antioxidant enzymes and decreasing MDA content in cucumber (Wang et al., 2018). Consistently, in our study, silencing of GhHAI2, GhAHG3, and GhABI2 protected the plants against oxidative damage by increasing the activity of SOD, POD, and CAT and less MDA accumulation under osmotic stress.
Furthermore, we analyzed the root phenotype of VIGS plants after osmotic stress. Drought tolerance was found to be improved by root thickness, since roots can increase water absorption by encouraging root length density and growing larger root branches (Jeong et al., 2010). Under osmotic stress conditions, GhHAI2-, GhAHG3-, and GhABI2-silenced plants maintained their root growth, in terms of root length, volume, and root density. Additionally, the dry weight of GhHAI2-, GhAHG3-, and GhABI2-silenced plants was also higher than that of control plants. Hence, we propose that GhHAI2-, GhAHG3-, and GhABI2-mediated root modification enhance water uptake by increasing the total root surface area. Overexpression of AtEDT1/HDG11 and HYR in rice has previously been shown to improve drought tolerance through such root-mediated system (Yu et al., 2013; Ambavaram et al., 2014). Collectively, these results suggest that silencing of GhHAI2, GhAHG3, and GhABI2 enhances the cotton response to osmotic stress.
The interaction between PP2C and the ABA receptor PYL is the key step that triggers the downstream signaling genes to evoke ABA signaling (Fujii et al., 2009). This signaling network has been reported in different plants species such as Arabidopsis (Ma et al., 2009), rice (Kim et al., 2011), tomato (González-Guzmán et al., 2014), and cucumber (Wang et al., 2012). Several studies have shown the interaction between ABI1, ABI2, HABI, and AHG3 and RCAR/PYR/PYL family of ABA receptors (Ma et al., 2009; Park et al., 2009; Vlad et al., 2009). For instance, AHG3 interacts with PYL12 in response to ABA and functions specifically in seed germination and early seedling growth (Kuhn et al., 2006; Yoshida et al., 2006; Kim et al., 2011). ABI2 has been reported to transduce ABA signals to downstream targets through selectively interplaying with PYL9/RCAR1 (Ma et al., 2009). The HAI PP2Cs interacted with PYL5 and PYL7-10 in Arabidopsis (Bhaskara et al., 2012). Similarly, the cotton GhPYLs are thought to interact with and inhibit GhHAI2, GhAHG3, and GhABI2, thereby activating ABA signaling pathway. Consistent with the previously reported studies, the physical interaction between GhHAI2, GhAHG3, and GhABI2 and GhPYLs was confirmed by Y2H and LCI assays. The results show that GhPYLs act as potent inhibitors of GhHAI2, GhAHG3, and GhABI2 phosphatase activity. Hence, the interaction between ABA receptors and GhHAI2, GhAHG3, and GhABI2 is necessary for the activation of downstream targets in regulating osmotic stress response.
Under water deficit conditions, plants evoke defense mechanisms via induction of elevated levels of ABA to moderate water consumption and enhance stress tolerance (Urano et al., 2009). The expression of drought stress-responsive genes is regulated by both ABA-dependent as well as ABA-independent pathways (Yamaguchi-Shinozaki and Shinozaki, 2006). ABFs are the bZIP family transcription factors that play pivotal role in ABA-dependent gene expression and are known as positive regulators of ABA pathway (Uno et al., 2000; Yoshida et al., 2010, 2014). Further, DREBs are large family of transcription factors that mediate drought stress through an ABA-independent pathway (Yoshida et al., 2014). Our results reveal that silencing of GhHAI2, GhAHG3, and GhABI2 significantly increased the expression of ABA-dependent stress marker genes GhABFs than the ABA-independent GhDREB2 marker gene. The expression of ABA biosynthesis genes NCED positively regulates the endogenous ABA levels and the transcription of both ABA- and drought-inducible genes (Iuchi et al., 2001). We found that the expression levels of the ABA biosynthesis genes GhNCEDa and GhNCEDc vary significantly between control and GhHAI2-, GhAHG3-, and GhABI2-silenced plants, which was consistent with the enhanced induction of NCED3 expression in the HAI double and triple mutants (Bhaskara et al., 2012). These results showed that GhHAI2, GhAHG3, and GhABI2 involved in the ABA signaling pathway by upregulating ABA-responsive genes.
Conclusion
In conclusion, we have demonstrated that GhHAI2, GhAHG3, and GhABI2 negatively regulate the plant adaptive response to osmotic stress via ABA-mediated signaling. In our VIGS genetic studies, GhHAI2, GhAHG3, and GhABI2 displayed altered phenotypes in response to osmotic stress via changes in leaf physiology and root morphology and regulating ROS scavenging. Further, we have demonstrated that GhHAI2, GhAHG3, and GhABI2 act as core components of ABA signaling via interaction with ABA receptors. Hence, our findings provide valuable insights into the defense mechanism that occurs during osmotic stress and therefore may facilitate that these genes might be essential for drought tolerance in cotton; however, it needs further evaluation under drought stress. Furthermore, the utilization of GhHAI2, GhAHG3, and GhABI2 mutations through genome editing techniques might also be effective.
Data Availability Statement
The original contributions presented in the study are included in the article/Supplementary Material; further inquiries can be directed to the corresponding author.
Author Contributions
HS conceived the study, designed the experiments, performed the experiments, interpreted the data, made figures, and wrote the manuscript. NK reviewed and edited the manuscript. LW provided assistance in qPCR analysis. XW supervised the project. All authors contributed to the article and approved the submitted version.
Funding
This work was supported by the funding of Xinjiang Uygur Autonomous Region Major Science and Technology Project (2021A02001-3).
Conflict of Interest
The authors declare that the research was conducted in the absence of any commercial or financial relationships that could be construed as a potential conflict of interest.
Publisher’s Note
All claims expressed in this article are solely those of the authors and do not necessarily represent those of their affiliated organizations, or those of the publisher, the editors and the reviewers. Any product that may be evaluated in this article, or claim that may be made by its manufacturer, is not guaranteed or endorsed by the publisher.
Supplementary Material
The Supplementary Material for this article can be found online at: https://www.frontiersin.org/articles/10.3389/fpls.2022.905181/full#supplementary-material
Supplementary Table S1 | List of primer sequences used in this study.
Supplementary Figure S1 | (A) Albino appearance on the leaves of TRV2:GhCLA1 plants. (B–D) Verification of GhHAI2, GhAHG3, and GhABI2 silencing in VIGS plants by qPCR.
References
Alexieva, V., Sergiev, I., Mapelli, S., and Karanov, E. (2001). The effect of drought and ultraviolet radiation on growth and stress markers in pea and wheat. Plant Cell Environ. 24, 1337–1344. doi: 10.1046/j.1365-3040.2001.00778.x
Ambavaram, M. M., Basu, S., Krishnan, A., Ramegowda, V., Batlang, U., Rahman, L., et al. (2014). Coordinated regulation of photosynthesis in Rice increases yield and tolerance to environmental stress. Nat. Commun. 5:5302. doi: 10.1038/ncomms6302
Antoni, R., Gonzalez-Guzman, M., Rodriguez, L., Rodrigues, A., Pizzio, G. A., and Rodriguez, P. L. (2012). Selective inhibition of clade A phosphatases type 2C by PYR/PYL/RCAR abscisic acid receptors. Plant Physiol. 158, 970–980. doi: 10.1104/pp.111.188623
Bhaskara, G. B., Nguyen, T. T., and Verslues, P. E. (2012). Unique drought resistance functions of the highly ABA-induced clade A protein phosphatase 2Cs. Plant Physiol. 160, 379–395. doi: 10.1104/pp.112.202408
Chen, C., Yu, Y., Ding, X., Liu, B., Duanmu, H., Zhu, D., et al. (2018). Genome-wide analysis and expression profiling of PP2C clade D under saline and alkali stresses in wild soybean and Arabidopsis. Protoplasma 255, 643–654. doi: 10.1007/s00709-017-1172-2
Choudhury, S., Panda, P., Sahoo, L., and Panda, S. K. (2013). Reactive oxygen species signaling in plants under abiotic stress. Plant Signal. Behav. 8:e23681. doi: 10.4161/psb.23681
Choudhury, F. K., Rivero, R. M., Blumwald, E., and Mittler, R. (2017). Reactive oxygen species, abiotic stress and stress combination. Plant J. 90, 856–867. doi: 10.1111/tpj.13299
Claeys, H., and Inzé, D. (2013). The agony of choice: how plants balance growth and survival under water-limiting conditions. Plant Physiol. 162, 1768–1779. doi: 10.1104/pp.113.220921
Cutler, S. R., Rodriguez, P. L., Finkelstein, R. R., and Abrams, S. R. (2010). Abscisic acid: emergence of a core signaling network. Annu. Rev. Plant Biol. 61, 651–679. doi: 10.1146/annurev-arplant-042809-112122
Ding, Y., Li, H., Zhang, X., Xie, Q., Gong, Z., and Yang, S. (2015). OST1 kinase modulates freezing tolerance by enhancing ICE1 stability in Arabidopsis. Dev. Cell 32, 278–289. doi: 10.1016/j.devcel.2014.12.023
Fujii, H., Chinnusamy, V., Rodrigues, A., Rubio, S., Antoni, R., Park, S. Y., et al. (2009). In vitro reconstitution of an abscisic acid signalling pathway. Nature 462, 660–664. doi: 10.1038/nature08599
Fujita, Y., Nakashima, K., Yoshida, T., Katagiri, T., Kidokoro, S., Kanamori, N., et al. (2009). Three SnRK2 protein kinases are the main positive regulators of abscisic acid signaling in response to water stress in Arabidopsis. Plant Cell Physiol. 50, 2123–2132. doi: 10.1093/pcp/pcp147
Gao, X., Li, F., Li, M., Kianinejad, A. S., Dever, J. K., Wheeler, T. A., et al. (2013). Cotton GhBAK1 mediates Verticillium wilt resistance and cell death. J. Integr. Plant Biol. 55, 586–596. doi: 10.1111/jipb.12064
Gao, X., Wheeler, T., Li, Z., Kenerley, C. M., He, P., and Shan, L. (2011). Silencing GhNDR1 and GhMKK2 compromises cotton resistance to Verticillium wilt. Plant J. 66, 293–305. doi: 10.1111/j.1365-313X.2011.04491.x
Gonzalez-Guzman, M., Pizzio, G. A., Antoni, R., Vera-Sirera, F., Merilo, E., Bassel, G. W., et al. (2012). Arabidopsis PYR/PYL/RCAR receptors play a major role in quantitative regulation of stomatal aperture and transcriptional response to abscisic acid. Plant Cell 24, 2483–2496. doi: 10.1105/tpc.112.098574
González-Guzmán, M., Rodríguez, L., Lorenzo-Orts, L., Pons, C., Sarrión-Perdigones, A., Fernández, M. A., et al. (2014). Tomato PYR/PYL/RCAR abscisic acid receptors show high expression in root, differential sensitivity to the abscisic acid agonist quinabactin, and the capability to enhance plant drought resistance. J. Exp. Bot. 65, 4451–4464. doi: 10.1093/jxb/eru219
Hanin, M., Brini, F., Ebel, C., Toda, Y., Takeda, S., and Masmoudi, K. (2011). Plant dehydrins and stress tolerance: versatile proteins for complex mechanisms. Plant Signal. Behav. 6, 1503–1509. doi: 10.4161/psb.6.10.17088
He, Z., Wu, J., Sun, X., and Dai, M. (2019). The maize clade A PP2C phosphatases play critical roles in multiple abiotic stress responses. Int. J. Mol. Sci. 20:3573. doi: 10.3390/ijms20143573
Hu, L., Li, H., Pang, H., and Fu, J. (2012). Responses of antioxidant gene, protein and enzymes to salinity stress in two genotypes of perennial ryegrass (Lolium perenne) differing in salt tolerance. J. Plant Physiol. 169, 146–156. doi: 10.1016/j.jplph.2011.08.020
Iuchi, S., Kobayashi, M., Taji, T., Naramoto, M., Seki, M., Kato, T., et al. (2001). Regulation of drought tolerance by gene manipulation of 9-cis-epoxycarotenoid dioxygenase, a key enzyme in abscisic acid biosynthesis in Arabidopsis. Plant J. 27, 325–333. doi: 10.1046/j.1365-313x.2001.01096.x
Jensen, M. K., Lindemose, S., De Masi, F., Reimer, J. J., Nielsen, M., Perera, V., et al. (2013). ATAF1 transcription factor directly regulates abscisic acid biosynthetic gene NCED3 in Arabidopsis thaliana. FEBS Open Bio. 3, 321–327. doi: 10.1016/j.fob.2013.07.006
Jeong, J. S., Kim, Y. S., Baek, K. H., Jung, H., Ha, S. H., Do Choi, Y., et al. (2010). Root-specific expression of OsNAC10 improves drought tolerance and grain yield in rice under field drought conditions. Plant Physiol. 153, 185–197. doi: 10.1104/pp.110.154773
Khan, N., Ke, H., Hu, C. M., Naseri, E., Haider, M. S., Ayaz, A., et al. (2019). Genome-wide identification, evolution, and transcriptional profiling of PP2C gene family in Brassica rapa. Biomed. Res. Int. 2019, 1–15. doi: 10.1155/2019/2965035
Kilian, J., Whitehead, D., Horak, J., Wanke, D., Weinl, S., Batistic, O., et al. (2007). The AtGenExpress global stress expression data set: protocols, evaluation and model data analysis of UV-B light, drought and cold stress responses. Plant J. 50, 347–363. doi: 10.1111/j.1365-313X.2007.03052.x
Kim, H., Hwang, H., Hong, J.-W., Lee, Y.-N., Ahn, I. P., Yoon, I. S., et al. (2011). A rice orthologue of the ABA receptor, OsPYL/RCAR5, is a positive regulator of the ABA signal transduction pathway in seed germination and early seedling growth. J. Exp. Bot. 63, 1013–1024. doi: 10.1093/jxb/err338
Kim, W., Lee, Y., Park, J., Lee, N., and Choi, G. (2013). HONSU, a protein phosphatase 2C, regulates seed dormancy by inhibiting ABA signaling in Arabidopsis. Plant Cell Physiol. 54, 555–572. doi: 10.1093/pcp/pct017
Koornneef, M., Reuling, G., and Karssen, C. M. (1984). The isolation and characterization of abscisic acid-insensitive mutants of Arabidopsis thaliana. Physiol. Plant. 61, 377–383. doi: 10.1111/j.1399-3054.1984.tb06343.x
Kuhn, J. M., Boisson-Dernier, A., Dizon, M. B., Maktabi, M. H., and Schroeder, J. I. (2006). The protein phosphatase AtPP2CA negatively regulates abscisic acid signal transduction in Arabidopsis, and effects of abh1 on AtPP2CA mRNA. Plant Physiol. 140, 127–139. doi: 10.1104/pp.105.070318
Kumar, S., Li, G., Yang, J., Huang, X., Ji, Q., Zhou, K., et al. (2020). Investigation of an antioxidative system for salinity tolerance in Oenanthe javanica. Antioxidants 9:940. doi: 10.3390/antiox9100940
Lee, S. C., and Luan, S. (2012). ABA signal transduction at the crossroad of biotic and abiotic stress responses. Plant Cell Environ. 35, 53–60. doi: 10.1111/j.1365-3040.2011.02426.x
Leonhardt, N., Kwak, J. M., Robert, N., Waner, D., Leonhardt, G., and Schroeder, J. I. (2004). Microarray expression analyses of Arabidopsis guard cells and isolation of a recessive abscisic acid hypersensitive protein phosphatase 2C mutant. Plant Cell 16, 596–615. doi: 10.1105/tpc.019000
Li, C., Shen, H., Wang, T., and Wang, X. (2015). ABA regulates subcellular redistribution of OsABI-LIKE2, a negative regulator in ABA signaling, to control root architecture and drought resistance in Oryza sativa. Plant Cell Physiol. 56, 2396–2408. doi: 10.1093/pcp/pcv154
Lim, C. W., Baek, W., Han, S. W., and Lee, S. C. (2013). Arabidopsis PYL8 plays an important role for ABA signaling and drought stress responses. Plant Pathol. J. 29, 471–476. doi: 10.5423/PPJ.NT.07.2013.0071
Livak, K. J., and Schmittgen, T. D. (2001). Analysis of relative gene expression data using real-time quantitative pcr and the 2−ΔΔCT method. Methods 25, 402–408. doi: 10.1006/meth.2001.1262
Lu, T., Zhang, G., Wang, Y., He, S., Sun, L., and Hao, F. (2019). Genome-wide characterization and expression analysis of PP2CA family members in response to ABA and osmotic stress in Gossypium. PeerJ 7:e7105. doi: 10.7717/peerj.7105
Ma, Y., Szostkiewicz, I., Korte, A., Moes, D., Yang, Y., Christmann, A., et al. (2009). Regulators of PP2C phosphatase activity function as abscisic acid sensors. Science 324, 1064–1068. doi: 10.1126/science.1172408
Merlot, S., Gosti, F., Guerrier, D., Vavasseur, A., and Giraudat, J. (2001). The ABI1 and ABI2 protein phosphatases 2C act in a negative feedback regulatory loop of the abscisic acid signalling pathway. Plant J. 25, 295–303. doi: 10.1046/j.1365-313x.2001.00965.x
Nakashima, K., and Yamaguchi-Shinozaki, K. (2006). Regulons involved in osmotic stress-responsive and cold stress-responsive gene expression in plants. Physiol. Plant. 126, 62–71. doi: 10.1111/j.1399-3054.2005.00592.x
Nakashima, K., and Yamaguchi-Shinozaki, K. (2013). ABA signaling in stress-response and seed development. Plant Cell Rep. 32, 959–970. doi: 10.1007/s00299-013-1418-1
Née, G., Kramer, K., Nakabayashi, K., Yuan, B., Xiang, Y., Miatton, E., et al. (2017). Delay of germination1 requires PP2C phosphatases of the ABA signalling pathway to control seed dormancy. Nat. Commun. 8:72. doi: 10.1038/s41467-017-00113-6
Nishimura, N., Yoshida, T., Kitahata, N., Asami, T., Shinozaki, K., and Hirayama, T. (2007). ABA-hypersensitive germination1 encodes a protein phosphatase 2C, an essential component of abscisic acid signaling in Arabidopsis seed. Plant J. 50, 935–949. doi: 10.1111/j.1365-313X.2007.03107.x
Osakabe, Y., Osakabe, K., Shinozaki, K., and Tran, L. S. (2014). Response of plants to water stress. Front. Plant Sci. 5:86. doi: 10.3389/fpls.2014.00086
Park, S. Y., Fung, P., Nishimura, N., Jensen, D. R., Fujii, H., Zhao, Y., et al. (2009). Abscisic acid inhibits type 2C protein phosphatases via the PYR/PYL family of START proteins. Science 324, 1068–1071. doi: 10.1126/science.1173041
Pettigrew, W. T. (2004). Physiological consequences of moisture deficit stress in cotton. Crop Sci. 44, 1265–1272. doi: 10.2135/cropsci2004.1265
Ratnayaka, H. H., Molin, W. T., and Sterling, T. M. (2003). Physiological and antioxidant responses of cotton and spurred anoda under interference and mild drought. J. Exp. Bot. 54, 2293–2305. doi: 10.1093/jxb/erg251
Rodrigues, A., Adamo, M., Crozet, P., Margalha, L., Confraria, A., Martinho, C., et al. (2013). ABI1 and PP2CA phosphatases are negative regulators of Snf1-related protein kinase1 signaling in Arabidopsis. Plant Cell 25, 3871–3884. doi: 10.1105/tpc.113.114066
Rubio, S., Rodrigues, A., Saez, A., Dizon, M. B., Galle, A., Kim, T.-H., et al. (2009). Triple loss of function of protein phosphatases type 2C leads to partial constitutive response to endogenous abscisic acid. Plant Physiol. 150, 1345–1355. doi: 10.1104/pp.109.137174
Saez, A., Apostolova, N., Gonzalez-Guzman, M., Gonzalez-Garcia, M. P., Nicolas, C., Lorenzo, O., et al. (2004). Gain-of-function and loss-of-function phenotypes of the protein phosphatase 2C HAB1 reveal its role as a negative regulator of abscisic acid signalling. Plant J. 37, 354–369. doi: 10.1046/j.1365-313X.2003.01966.x
Santiago, J., Dupeux, F., Betz, K., Antoni, R., Gonzalez-Guzman, M., Rodriguez, L., et al. (2012). Structural insights into PYR/PYL/RCAR ABA receptors and PP2Cs. Plant Sci. 182, 3–11. doi: 10.1016/j.plantsci.2010.11.014
Santiago, J., Rodrigues, A., Saez, A., Rubio, S., Antoni, R., Dupeux, F., et al. (2009). Modulation of drought resistance by the abscisic acid receptor PYL5 through inhibition of clade A PP2Cs. Plant J. 60, 575–588. doi: 10.1111/j.1365-313X.2009.03981.x
Schweighofer, A., Hirt, H., and Meskiene, I. (2004). Plant PP2C phosphatases: emerging functions in stress signaling. Trends Plant Sci. 9, 236–243. doi: 10.1016/j.tplants.2004.03.007
Shang, X., Yu, Y., Zhu, L., Liu, H., Chai, Q., and Guo, W. (2020). A cotton NAC transcription factor GhirNAC2 plays positive roles in drought tolerance via regulating ABA biosynthesis. Plant Sci. 296:110498. doi: 10.1016/j.plantsci.2020.110498
Shazadee, H., Khan, N., Wang, J., Wang, C., Zeng, J., Huang, Z., et al. (2019). Identification and expression profiling of protein phosphatases (PP2C) gene family in Gossypium hirsutum L. Int. J. Mol. Sci. 20:1395. doi: 10.3390/ijms20061395
Singh, A., Jha, S. K., Bagri, J., and Pandey, G. K. (2015). ABA inducible rice protein phosphatase 2C confers ABA insensitivity and abiotic stress tolerance in Arabidopsis. PLoS One 10:e0125168. doi: 10.1371/journal.pone.0125168
Thompson, J. D., Higgins, D. G., and Gibson, T. J. (1994). CLUSTAL W: improving the sensitivity of progressive multiple sequence alignment through sequence weighting, position-specific gap penalties and weight matrix choice. Nucleic Acids Res. 22, 4673–4680. doi: 10.1093/nar/22.22.4673
Uno, Y., Furihata, T., Abe, H., Yoshida, R., Shinozaki, K., and Yamaguchi-Shinozaki, K. (2000). Arabidopsis basic leucine zipper transcription factors involved in an abscisic acid-dependent signal transduction pathway under drought and high-salinity conditions. Proc. Natl. Acad. Sci. U. S. A. 97, 11632–11637. doi: 10.1073/pnas.190309197
Urano, K., Maruyama, K., Ogata, Y., Morishita, Y., Takeda, M., Sakurai, N., et al. (2009). Characterization of the ABA-regulated global responses to dehydration in Arabidopsis by metabolomics. Plant J. 57, 1065–1078. doi: 10.1111/j.1365-313X.2008.03748.x
Vlad, F., Rubio, S., Rodrigues, A., Sirichandra, C., Belin, C., Robert, N., et al. (2009). Protein phosphatases 2C regulate the activation of the Snf1-related kinase OST1 by abscisic acid in Arabidopsis. Plant Cell 21, 3170–3184. doi: 10.1105/tpc.109.069179
Wang, Y., Wu, Y., Duan, C., Chen, P., Li, Q., Dai, S., et al. (2012). The expression profiling of the CsPYL, CsPP2C and CsSnRK2 gene families during fruit development and drought stress in cucumber. J. Plant Physiol. 169, 1874–1882. doi: 10.1016/j.jplph.2012.07.017
Wang, J., Zhang, L., Cao, Y., Qi, C., Li, S., Liu, L., et al. (2018). CsATAF1 positively regulates drought stress tolerance by an ABA-dependent pathway and by promoting ROS scavenging in cucumber. Plant Cell Physiol. 59, 930–945. doi: 10.1093/pcp/pcy030
WRI (2013). One-quarter of world’s agriculture grows in highly water-stressed areas. Available at: https://www.wri.org/insights/one-quarter-worlds-agriculture-grows-highly-water-stressed-areas.
Xiang, Y., Sun, X., Gao, S., Qin, F., and Dai, M. (2017). Deletion of an endoplasmic reticulum stress response element in a ZmPP2C-A gene facilitates drought tolerance of maize seedlings. Mol. Plant 10, 456–469. doi: 10.1016/j.molp.2016.10.003
Xiong, L., Wang, R. G., Mao, G., and Koczan, J. M. (2006). Identification of drought tolerance determinants by genetic analysis of root response to drought stress and abscisic acid. Plant Physiol. 142, 1065–1074. doi: 10.1104/pp.106.084632
Xue, T., Wang, D., Zhang, S., Ehlting, J., Ni, F., Jakab, S., et al. (2008). Genome-wide and expression analysis of protein phosphatase 2C in rice and Arabidopsis. BMC Genomics 9:550. doi: 10.1186/1471-2164-9-550
Yamaguchi-Shinozaki, K., and Shinozaki, K. (2006). Transcriptional regulatory networks in cellular responses and tolerance to dehydration and cold stresses. Annu. Rev. Plant Biol. 57, 781–803. doi: 10.1146/annurev.arplant.57.032905.105444
Yoshida, T., Christmann, A., Yamaguchi-Shinozaki, K., Grill, E., and Fernie, A. R. (2019). Revisiting the basal role of ABA – roles outside of stress. Trends Plant Sci. 24, 625–635. doi: 10.1016/j.tplants.2019.04.008
Yoshida, T., Fujita, Y., Sayama, H., Kidokoro, S., Maruyama, K., Mizoi, J., et al. (2010). AREB1, AREB2, and ABF3 are master transcription factors that cooperatively regulate ABRE-dependent ABA signaling involved in drought stress tolerance and require ABA for full activation. Plant J. 61, 672–685. doi: 10.1111/j.1365-313X.2009.04092.x
Yoshida, T., Mogami, J., and Yamaguchi-Shinozaki, K. (2014). ABA-dependent and ABA-independent signaling in response to osmotic stress in plants. Curr. Opin. Plant Biol. 21, 133–139. doi: 10.1016/j.pbi.2014.07.009
Yoshida, T., Nishimura, N., Kitahata, N., Kuromori, T., Ito, T., Asami, T., et al. (2006). ABA-hypersensitive germination3 encodes a protein phosphatase 2C (AtPP2CA) that strongly regulates abscisic acid signaling during germination among Arabidopsis protein phosphatase 2Cs. Plant Physiol. 140, 115–126. doi: 10.1104/pp.105.070128
Yu, L., Chen, X., Wang, Z., Wang, S., Wang, Y., Zhu, Q., et al. (2013). Arabidopsis enhanced drought tolerance1/homeodomain glabrous11 confers drought tolerance in transgenic rice without yield penalty. Plant Physiol. 162, 1378–1391. doi: 10.1104/pp.113.217596
Yu, X., Han, J., Wang, E., Xiao, J., Hu, R., Yang, G., et al. (2019). Genome-wide identification and homoeologous expression analysis of PP2C genes in wheat (Triticum aestivum L.). Front. Genet. 10:561. doi: 10.3389/fgene.2019.00561
Zhang, T., Hu, Y., Jiang, W., Fang, L., Guan, X., and Chen, J. (2015). Sequencing of allotetraploid cotton (Gossypium hirsutum L. acc. TM-1) provides a resource for fiber improvement. Nat. Biotechnol. 33, 531–537. doi: 10.1038/nbt.3207
Zhang, G., Lu, T., Miao, W., Sun, L., Tian, M., Wang, J., et al. (2017). Genome-wide identification of ABA receptor PYL family and expression analysis of PYLs in response to ABA and osmotic stress in Gossypium. PeerJ 5:e4126. doi: 10.7717/peerj.4126
Zhu, J. K. (2002). Salt and drought stress signal transduction in plants. Annu. Rev. Plant Biol. 53, 247–273. doi: 10.1146/annurev.arplant.53.091401.143329
Keywords: PP2Cs, osmotic tolerance, cotton, VIGS, abscisic acid
Citation: Shazadee H, Khan N, Wang L and Wang X (2022) GhHAI2, GhAHG3, and GhABI2 Negatively Regulate Osmotic Stress Tolerance via ABA-Dependent Pathway in Cotton (Gossypium hirsutum L.). Front. Plant Sci. 13:905181. doi: 10.3389/fpls.2022.905181
Edited by:
Caroline Müller, Universidade Federal da Fronteira Sul, BrazilReviewed by:
Danilo M. Daloso, Federal University of Ceara, BrazilAgata Daszkowska-Golec, University of Silesia in Katowice, Poland
Kazuo Nakashima, Japan International Research Center for Agricultural Sciences (JIRCAS), Japan
Copyright © 2022 Shazadee, Khan, Wang and Wang. This is an open-access article distributed under the terms of the Creative Commons Attribution License (CC BY). The use, distribution or reproduction in other forums is permitted, provided the original author(s) and the copyright owner(s) are credited and that the original publication in this journal is cited, in accordance with accepted academic practice. No use, distribution or reproduction is permitted which does not comply with these terms.
*Correspondence: Xinyu Wang, eHl3YW5nQG5qYXUuZWR1LmNu