- 1Department of Agronomy, College of Agriculture and Biotechnology, Zhejiang University, Hangzhou, China
- 2Hainan Yazhou Bay Seed Laboratory, Sanya, China
- 3Zhejiang Provincial Key Laboratory of Crop Germplasm, Zhejiang University, Hangzhou, China
Auxin regulates plant growth and tropism responses. As a phytohormone, auxin is transported between its synthesis sites and action sites. Most natural auxin moves between cells via a polar transport system that is mediated by PIN-FORMED (PIN) auxin exporters. The asymmetrically localized PINs usually determine the directionality of intercellular auxin flow. Different internal cues and external stimuli modulate PIN polar distribution and activity at multiple levels, including transcription, protein stability, subcellular trafficking, and post-translational modification, and thereby regulate auxin-distribution-dependent development. Thus, the different regulation levels of PIN polarity constitute a complex network. For example, the post-translational modification of PINs can affect the subcellular trafficking of PINs. In this review, we focus on subcellular trafficking and post-translational modification of PINs to summarize recent progress in understanding PIN polarity.
Introduction
Auxin, the first plant hormone to be discovered, participates in many plant developmental processes. Its synthesis, distribution, and degradation respond to a variety of signals, mainly light and gravity; for this reason, auxin function, synthesis, distribution, and degradation have been major focuses of research in plant biology. Auxin is usually synthesized in young cells, such as shoots, leaf primordia, and root tips, and then is redistributed to exert its function. There are two general modes for transporting auxin: long-distance transport and short-distance transport (Michniewicz et al., 2007a). Although auxin can be transported via phloem vessels over long distances, it is short-distance transport, which refers to polar transport, that changes the auxin concentration in tissues and enables auxin to affect plant development (Adamowski and Friml, 2015). This polar transport is not powered by gravity, but instead involves active transport between cells. According to the chemiosmotic model, transport over short distances is controlled by auxin transporters (Goldsmith and Goldsmith, 1977). The auxin carriers discovered to date are AUXIN-INSENSITIVE1/LIKE AUX1 (AUX/LAX), NITRATE TRANSPORTER 1.1 (NRT1.1), B SUBFAMILY OF ATP-BINDING CASSETTE (ABCB) family, PIN-FORMED (PIN) family, PIN-LIKE TRANSPORTERS (PILS), and WALLS ARE THIN 1 (WAT1; Zhou and Luo, 2018). Given that the polar localization (asymmetric distribution) of PIN proteins in plants correlates well with the direction of auxin movement, PINs are considered to be the major transporters responsible for asymmetric auxin distributions (Benkova et al., 2003; Swarup and Bennett, 2003; Zhou and Luo, 2018). Noticeably, although the distribution of PINs may determine the auxin flux, the auxin flux or the auxin concentration may influence the polarity of PINs in return (Jonsson et al., 2006; Grigolon et al., 2015).
The PIN family has been identified in at least 30 plant species, and comprises eight genes in Arabidopsis thaliana (Zhou and Luo, 2018). In other plant species, most PINs have been studied at the genomic and transcriptional level, and only a small number of PINs have been studied at the gene level (Figure 1; Table 1; Wang et al., 2009, 2015; Miyashita et al., 2010; Forestan et al., 2012; Zhang et al., 2012; Xie et al., 2017; Inahashi et al., 2018; Sun et al., 2018; Li et al., 2019; Gao et al., 2021; Gho et al., 2021; Hou et al., 2021; Kumar et al., 2021; Liu et al., 2022). In A. thaliana, PIN proteins are involved in many plant developmental processes and are localized differently in different tissues (Tables 1, 2). During embryogenesis, PIN1, 3, 4, and 7 contribute to the establishment of apical-basal polarity (Friml et al., 2003). These four PINs induce the formation of primordia for aerial and underground organs. Notably, whereas PIN1 plays a major role among these four PINs in aerial organ growth, PIN2 is mainly expressed in roots, where it associates with the aforementioned four PINs to form a local “reflux loop” of auxin to enable the formation of the root meristem (Galweiler et al., 1998; Benkova et al., 2003; Friml et al., 2003; Blilou et al., 2005). In this loop, all PINs can be detected at the basal plasma membrane (PM), but the localization differs in some cells. PIN2 localizes at the apical PM in the root epidermis and lateral root cap, and PIN3 and PIN7 are detectable in the lateral columella (Friml et al., 2003). Given that the role of PIN2 in the “reflux loop” is to transport auxin from the root tip to the root elongation zone and that roots usually bend in the root elongation region, PIN2 is the major carrier involved in root gravitropism (Luschnig et al., 1998; Han et al., 2021). Among PIN3, 4, and 7, which all contribute to auxin lateral flow processes, such as shoot phototropism and gravitropism, as well as lateral root formation, PIN3 is indicated to be the main transporter (Ding et al., 2011; Rakusova et al., 2011; Rosquete et al., 2013). The functions of PIN5, 6, and 8 are less studied (Ding et al., 2012; Lee et al., 2020). In addition, whereas PIN1-4 and PIN7 localize to PM, PIN5, 6, and 8 are in the endoplasmic reticulum (ER), of which PIN6 can be detected at the ER and PM depending on the phosphorylation (Mravec et al., 2009; Ditengou et al., 2018; Sisi and Ruzicka, 2020). The PIN5, 6, and 8 proteins are short PINs, but PM-located PIN proteins are long PINs containing a long hydrophilic loop with some phosphorylation sites that can be phosphorylated by kinases to influence the polarity of the PIN (Bennett et al., 2014; Zhou and Luo, 2018). The polarity of PIN proteins may be influenced by the physical mechanics and can be regulated at least at two levels, namely post-translational modification including phosphorylation, and subcellular trafficking (Heisler et al., 2010; Zhou and Luo, 2018; Ramos et al., 2021). This review mainly focuses on these different regulatory mechanisms.
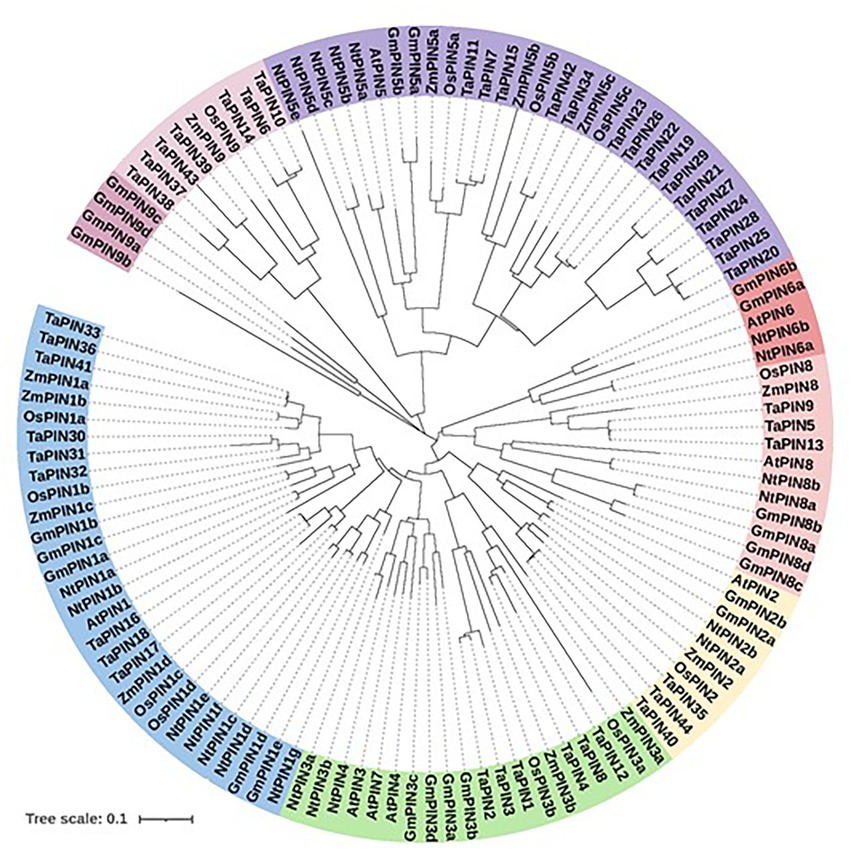
Figure 1. Phylogenetic relationships of the PIN proteins from Arabidopsis thaliana, Oryza sativa, Zea mays, Glycine max, Nicotiana tabacum, and Triticum aestivum. The protein sequences were downloaded from the NCBI databases, and from recently published data (Wang et al., 2009, 2015; Forestan et al., 2012; Xie et al., 2017; Kumar et al., 2021). The sequences were aligned with ClustalW, and the phylogenetic tree constructed with the neighbor-joining method implemented MEGA version 11 (Tamura et al., 2021).
Subcellular trafficking of PINs
To date, it has not been determined whether newly synthesized PINs are initially secreted in an apolar or polar manner. Therefore, this review focuses on the post-secretion regulation of PIN. To maintain or change the polarity in developmental processes or after sensing signal changes, such as a change in gravity, PINs can be endocytosed, recycled to the trans-Golgi network (TGN)/early endosome (EE), and then transported to the PM by exocytosis or to the vacuole for degradation by multivesicular bodies (Dhonukshe et al., 2007; Kleine-Vehn et al., 2010; Kitakura et al., 2011; Rodriguez-Furlan et al., 2019). During these processes, many factors affect trafficking by influencing endocytosis, vesicular transport, and membrane fusion, and thus affect PIN polarity (Table 3).
Endocytosis
To recycle or degrade PINs, the first trafficking step is endocytosis (Figure 2). Clathrin-coated vesicles are carriers for membrane vesicular transport, in which the clathrin unit is a triskelion-like structure, comprising heavy and light chains [CLATHRIN HEAVY CHAIN (CHC) and CLATHRIN LIGHT CHAIN (CLC) proteins] with three arms that assist the clathrin to assemble into different size structures (Kirchhausen and Harrison, 1981; Ungewickell and Branton, 1981; Fotin et al., 2004). PIN recycling depends on clathrin-mediated endocytosis (CME). Clathrin heavy chain or light chain mutants may exhibit severe defects in PIN trafficking and polar localization, thereby affecting auxin distribution and auxin-related phenotypes, such as hook opening and hypocotyl phototropism (Kitakura et al., 2011; Yu et al., 2016; Zhang et al., 2017). Studies of the clc2 clc3 double mutants show that disruption of clathrin formation influences hypocotyl growth by changing PIN3 lateral localization and causes PIN3 relocalization after exposure to blue light (Zhang et al., 2017; Hu et al., 2021). During CME, dynamin-related proteins, which assist in the vesicle isolation from the membrane, affect PIN polarity because they may be involved in CME of PINs from the cell plate (Mravec et al., 2011).
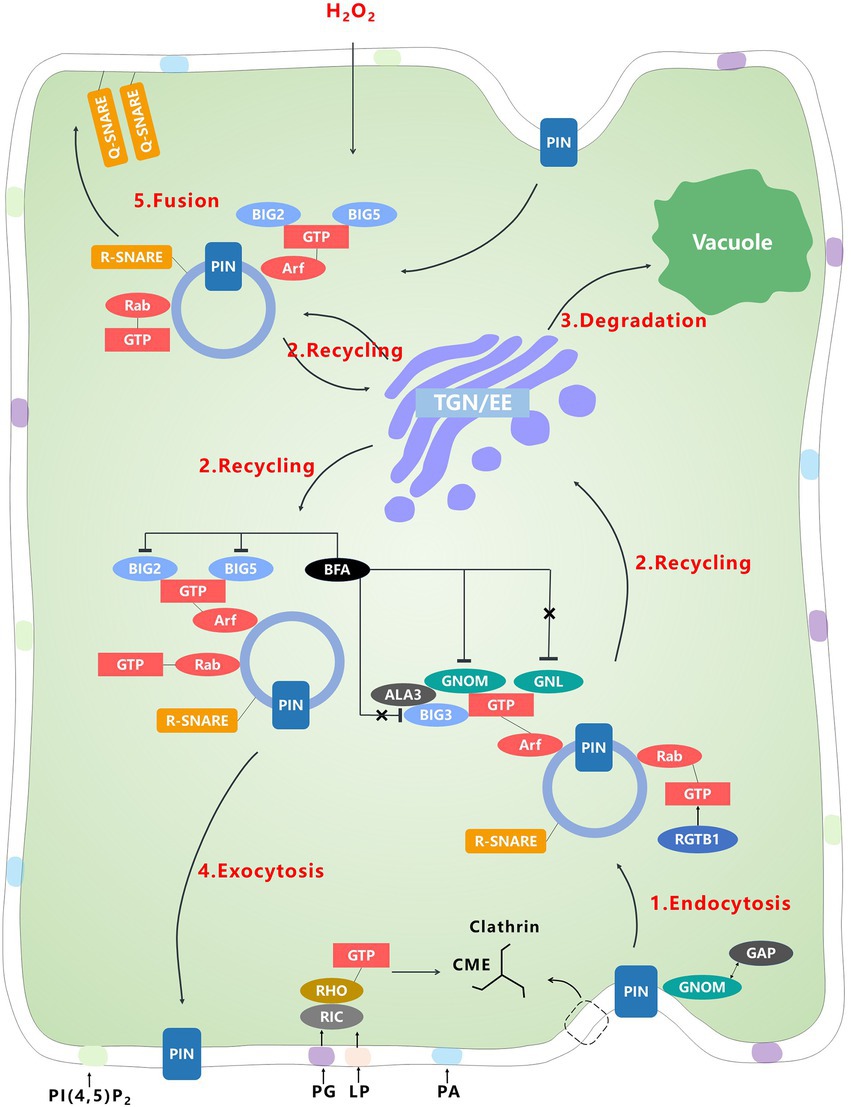
Figure 2. PINs can be recycled or degraded through endocytosis, subcellular trafficking and membrane fusion. In endocytosis, clathrin mediates the formation of vesicles. The PINs are then transported in the endosomal vesicles, in which many Arf and Rab proteins influence the destination of the PINs. These Arf and Rab proteins are further regulated by GEF and GAP, including GNOM, a PINs-specific AEF-GEF. Finally, the vesicles fuse to the destination membrane, regulated by SNARE. In addition, lipids are involved in the binding of these membrane-associated proteins. Notably, among these factors that may affect the polarity of PINs, only GNOM is primarily involved in the recycling basal PINs.
Lipids can also affect the CME process. The sterol-biosynthesis mutants, cyclopropylsterol isomerase1-1 (cpi1-1), display defective PIN localization (Men et al., 2008). An additional type of signaling phospholipid, phosphatidylinositol (4,5)-bisphosphate [PI(4,5)P2], also influences PIN localization. PI(4,5)P2 mostly localizes to the PM and regulates many subcellular events. PI(4,5)P2 is mainly derived from phosphatidylinositol-4-phosphate (PI4P), which is synthesized by 11 phosphatidylinositol 4-phosphate 5-kinases (PI4P5K; Mueller-Roeber and Pical, 2002). The pip5k1 pip5k2 double mutant exhibits a disruption of PIN recycling. This indicates that PI(4,5)P2, which influences the formation of clathrin vesicles, is required for the establishment of PIN polarity (Ischebeck et al., 2013). Besides, PIP5Ks are recruited by BREVIS RADIX (BRX), a plant-specific PM-localized protein, and a type of kinase that is PROTEIN KINASE ASSOCIATED WITH BRX (PAX), to influence the abundance of PINs at PM (Marhava et al., 2020). This interaction establishes the link between CME and kinases, and suggests that PI(4,5)P2 abundance may be the signal at the PM. In addition, lipids can affect other membrane proteins to influence PIN polarity. The endocytosis of PIN1 is regulated by the clustering of RHO-LIKE GTPASE (ROP6), which is influenced by lipid phosphatidylserine (LP), phosphatidylglycerol (PG) and the sterol-dependent clustering of TRANSMEMBRANE RECEPTOR KINASE 1 (TMK1), and ROP-INTERACTIVE CRIB MOTIF-CONTAINING PROTEIN 1 (RIC1; Chen et al., 2012; Han et al., 2018; Platre et al., 2019; Pan et al., 2020). Polyacidic phospholipids might impact on the binding between the PM and a 14–3-3 regulated protein, NON-PHOTOTROPIC HYPOCOTYL 3 (NPH3), to regulate the PIN polarity during phototropic growth of the hypocotyl (Keicher et al., 2017; Reuter et al., 2021).
Vesicular transport
After endocytosis and during recycling, PINs are located in the vesicles, where many proteins may influence the trafficking of PINs (Figure 2). The cytoskeleton and molecular machinery control the movement of vesicles, but it is the small GTPases, Arf and Rab proteins that regulate intracellular transport by connecting membranes to the cytoskeleton machinery and labeling vesicles for their final destination (Khan and Menetrey, 2013; Kjos et al., 2018). In addition, although Arf and Rab mainly regulate intracellular trafficking, they can also influence other GTPase, such as Rho, which usually regulates the actin cytoskeleton and may influence the localization of PIN proteins (Han et al., 2018; Kjos et al., 2018).
Arf binds to the vesicular membrane weakly in the GDP form and binds tightly in the GTP form. After Arf binds to the membrane, the GDP/GTP EXCHANGE FACTOR FOR SMALL G PROTEINS OF THE ARF CLASS (ARF-GEFs) are recruited to change the Arf type from GDP to GTP (Donaldson and Jackson, 2000). Brefeldin A (BFA) blocks the guanine-nucleotide exchange reaction to inhibit vesicle trafficking reversibly, ARF-GEFs in A. thaliana were initially classified as BFA-INHIBITED GEFs (BIG) and Golgi BFA resistance factor (GBF) initially (Donaldson and Jackson, 2000). Among GBFs, GNOM is BFA sensitive (Naramoto et al., 2010). GNOM is mostly localized to the Golgi apparatus and partly to the PM and TGN/EE (Naramoto et al., 2010, 2014). GNOM regulates the endocytosis and recycling of PIN1, which further affects PIN1 polarity (Steinmann et al., 1999; Geldner et al., 2003). Inhibition of GNOM by BFA leads to apical localization of PIN1, but the engineered BFA-insensitive GNOM only causes the recycling of PIN1 to become BFA insensitive, not that of other proteins (Geldner et al., 2003). Localization of PIN2 is not completely determined by GNOM. It requires additional homologs, such as GNOM-LIKE1 (GNL1), which also is localized to the Golgi apparatus but differs from GNOM (Richter et al., 2007; Teh and Moore, 2007; Kleine-Vehn et al., 2008). GNL1 plays an important and conserved role in ER-Golgi trafficking (Richter et al., 2007; Teh and Moore, 2007). In contrast, whereas GNOM is functionally redundant in ER-Golgi trafficking, the primary role of GNOM is the recycling of basal PINs (in root cells; Richter et al., 2007; Teh and Moore, 2007). There is evidence that GNOM and GNL1 are involved in the early secretory of PIN1 in the root, which contributes to the basal localization of PIN1 (Luschnig and Vert, 2014; Doyle et al., 2015). In addition, other factors acting in GNOM-independent endosomes can regulate PIN polarity. For example, recycling of PIN2 requires SORTING NEXIN 1 (SNX1), CLASP, and VACUOLAR PROTEIN SORTING 29 (VPS29), a factor downstream of GNOM (Jaillais et al., 2006, 2007; Ambrose et al., 2013). Some research indicates that the PM-localized GNOM and VAN3, an ARF-GTPase-activating protein (GAPs), which counteracts GEF, are also required for endocytosis (Naramoto et al., 2010; Naramoto and Kyozuka, 2018).
In the BIG class, BEN1/BIG5 and BEN3/BIG2 mainly co-localize in the TGN/EE, and influence the early trafficking and polar localization of PIN1 (Richter et al., 2014; Jonsson et al., 2017; Kitakura et al., 2017; Matsuura et al., 2020; Zhang et al., 2020). BEN1/BIG5 is involved in hydrogen peroxide-induced relocalization of PIN2 (Zwiewka et al., 2019). In addition, ALA3, a phospholipid flippase, produces and maintains the asymmetric distribution of phospholipids. The ALA3 protein directly interacts with GNOM and BIG3, and affects the transport and polarity of PINs (Best et al., 2019; Zhang et al., 2020). Similar to Arf, Rab-related pathways also involve RAB-GEF and RAB-GAP to activate or inactivate Rab (Martiniere and Moreau, 2020). Although no RAB-GEF is known to regulate polar trafficking of PINs, some evidence indicates that the Rab pathway is involved in vesicle circulation and degradation of PINs, and the change in Rab pathway affects the polar growth of root hair cells (Preuss et al., 2006; Feraru et al., 2012; Ivanov et al., 2014; Rodriguez-Furlan et al., 2019). Knocking out RGTB1, which catalyzes the Rab prenylation to assist Rab to bind more stably to the membrane, impairs the recycling of PIN1 and PIN3 (Rojek et al., 2021a,b).
Most of the factors that affect the polar trafficking of PINs are localized in the TGN. Recently, FORKED1 (FKD1), FORKED1-LIKE (FL), and SCARFACE (SFC) were detected in the TGN and were proposed to influence the secretory pathway that transports PIN1 to the apical PM during leaf vein development (Mariyamma et al., 2018). CHOLINE TRANSPORTER-LIKE 1 (CTL1) partially localizes to the TGN and can mediate choline transport to impact the homeostasis of membrane lipids. Interestingly, CTL1 regulates trafficking of PIN1 and PIN3 by acting on both secretory vesicles and clathrin-coated vesicles in the TGN (Wang et al., 2017). In addition, sphingolipids mediate polar sorting of PIN2 at the TGN by changing the level of PI4P, and thus the lipid can also affect PIN2 recycling (Ito et al., 2021).
Membrane fusion
Fusion of the vesicles to the destination membrane is the final step in the vacuolar protein transfer. The PINs then may be degraded, recycled or remained in the membrane to form or change the polarity (Figure 2). Before fusion, the exocyst complex is responsible for the initial attachment of the vesicle to the PM (Saeed et al., 2019). Studies of sec6, sec8, and exo70 mutants, show that the exocyst directly influences polar exocytosis of PINs (Drdova et al., 2013; Tan et al., 2016). Membrane fusion is then mediated by SOLUBLE N-ETHYLMALEIMIDE-SENSITIVE FUSION (NSF) PROTEIN ATTACHMENT PROTEIN RECEPTOR (SNARE). SNARE proteins can be classified as Q-SNARE and R-SNARE. Q-SNARE proteins are normally localized to the target membrane and R-SNARE proteins are in the TGN/EE (Fasshauer et al., 1998). When the three Q-SNARE and one R-SNARE proteins bind together, the membrane vesicles are fused (Jahn et al., 2003; Pratelli et al., 2004). The R-SNARE triple mutants, vamp714 vamp721 vamp722, shows defective polarity of PIN1 and PIN2 (Gu et al., 2021; Zhang et al., 2021). Notably, the polarity of PIN1 and PIN1-mediated polar auxin transport also requires AtNSF, which regulates leaf serration (Tang et al., 2021). It is generally accepted that SNAREs are involved in the Rab GTPase pathway, by interacting with Rab proteins to enable the fusion of membrane vesicles (Ebine and Ueda, 2009; Ohya et al., 2009; Ebine et al., 2011). No data are available on the interaction between ARF-GEF and SNAREs, but ARF-GAP is capable of interacting with SNAREs (Rein et al., 2002).
Overall, the function of each subcellular-trafficking related factor has not been fully explored. These factors can influence more than one process: clathrin can influence the recycling; GNOM and VAN3 are also involved in endocytosis (Naramoto et al., 2010; Robinson and Pimpl, 2014). Thus, although an intracellular trafficking model to explain the localization of PINs is accepted, it is not a complete theory. Many additional factors can influence the polarity of PINs and the relationships among the factors that already identified are not clearly demonstrated. Therefore, large scale exploration of the interactions or networks of these proteins is required (Tang et al., 2020). In addition, since vesicular trafficking is conserved in many organisms, the focus should be not only on PINs, or plants, but also on the regulatory mechanisms documented in other organisms (Glanc et al., 2021).
Post-translational modification of PINs
Phosphorylation and dephosphorylation
The activity and localization of long PIN proteins can be regulated through phosphorylation by at least three different types of protein kinases (Table 4): SERINE/THREONINE-PROTEIN WITH HOMOLOGY TO MAMMALIAN PROTEIN KINASE A, CGMP-DEPENDENT KINASE, AND PROTEIN KINASE C (AGC kinases), MITOGENACTIVATED PROTEIN (MAP) KINASES (MPKs), and Ca2+/CALMODULIN-DEPENDENT PROTEIN KINASE-RELATED KINASES (CRKs). In addition, certain phosphatases dephosphorylate PINs (Figure 3).
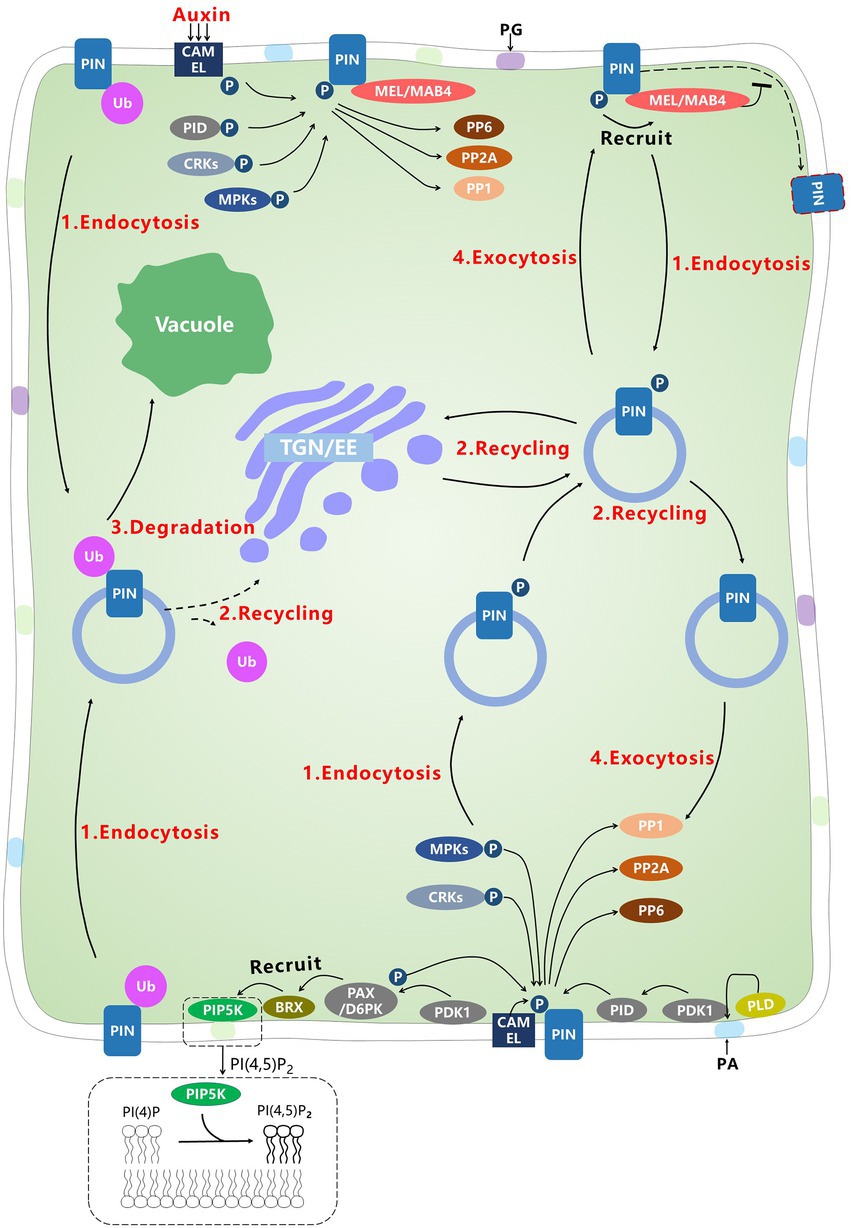
Figure 3. Post-translational modification regulates the localization of PINs. Kinases such as PID, D6PK, MPKs, and CRKs, directly phosphorylate PINs to change the polarity and transport activity of PINs. PAX recruits BRX to regulate the formation of PI(4,5)P2, which further influences the endocytosis of PINs. Phosphorylated PINs can recruit MAB4/MEL to maintain or change the localization of PINs through BFA-sensitive or BFA-insensitive endosomal vesicles. In addition, dephosphorylation regulated by phosphatases acts antagonistically to the changes caused by kinases. Among these proteins, only PAX/D6PK is localized only found to the basal PM.
AGC kinases
PINOID (PID), a member of the AGC family, was first found to be related to PINs. In A. thaliana, there are four members of the PID family (PID, PID2, WAVY ROOT GROWTH 1 (WAG1) and WAG2; Galvan-Ampudia and Offringa, 2007). Given that the pid mutants are phenotypically similar to pin mutants, the relationship between these two genes was studied (Bennett et al., 1995). PID was first cloned and implicated as a negative regulator in auxin expression signaling (Christensen et al., 2000). Benjamins et al. (2001) used overexpression lines to demonstrate that PID was not associated with the expression signaling, but may be a positive regulator of auxin efflux carriers (Benjamins et al., 2001). Overexpression of PID can over-phosphorylate the three conserved serine sites of PINs, S1, S2, and S3 (S231, 252, and 290 in PIN1), thereby altering the localization of PIN proteins from the basal to apical PM; in addition, the PINs showed apical-to-basal localization in pid mutants (Friml et al., 2004; Huang et al., 2010). Given that the distribution of PID is non-polar but that of PIN is polar, Dhonukshe et al. proposed a model in which PINs are not first polarly distributed at first, and thereafter reach the apical side when phosphorylated by PID. However, this model has not been clearly demonstrated (Dhonukshe et al., 2010). In addition, WAG1 and WAG2 are functionally redundant to PID (Dhonukshe et al., 2010). WAG1 and WAG2 phosphorylate the same region as PID does and show similar localization to PID (Dhonukshe et al., 2010). These three kinases have similar functions in root development, namely apical hook opening and photoresponse (Dhonukshe et al., 2010; Willige et al., 2012; Haga et al., 2014). More importantly, these three kinases all show BFA-insensitive localization and phosphorylate PINs to change their localization through BFA-insensitive processes (Dhonukshe et al., 2010). PID2 has not been well studied, but according to evolutionary reconstruction and the observation that photoreactions in pid pid2 wag1 wag2 quadruple mutants are significantly more impaired than pid wag1 wag2 triple mutants, the function of PID2 may be similar to that of the other three kinases (Tang et al., 2020). Despite the shift in distribution, PID-related phosphorylation can stimulate the activity of PINs transport (Zourelidou et al., 2014). Some studies have reported that phosphorylated PIN1 is detectable at the basal PM in the wild-type and pid mutants, suggesting that PID does not alter PIN polarity but only activates PIN transport activity (Weller et al., 2017). The change in polarity might result from other factors that interact with PID in PID-overexpression lines (Haga et al., 2014). Thus, although genetic and biochemistry experiments have provided substantial information on PID-related phosphorylation, the mechanism of the change in PIN polarity remains unknown.
Two important factors upstream and downstream of PID that regulate the PIN polarity should be mentioned. The first protein is associated with lipids. PHOSPHOLIPASE D (PLD) is responsive to many environmental signals. Under salt stress, PLD ζ 2 is involved in the endocytosis of PIN2 (Li and Xue, 2007; Galvan-Ampudia et al., 2013). Also, salt stress activates PLD α 1 and PLD Δ, which will generate phosphatidic acid (PA). The PA then binds to PID to phosphorylate PIN2, and increase the activity of PIN2 (Wang et al., 2019). A recent study observed that MACCHI-BOU4 / MAB4(ENP1)-LIKE (MAB4/MEL), to which belongs to the MAB4 protein family and was originally considered to be associated with PIN-related auxin transport, is recruited by phosphorylated PINs, which in turn form a positive feedback loop, thus promoting continuous phosphorylation of PINs by the AGC3 family to confine PIN to polar regions (Glanc et al., 2021).
The D6 PROTEIN KINASE (D6PK) belongs to the AGC family. It is generally accepted that D6PK as well as D6PKL1, D6PKL2, and D6PKL3 phosphorylate S1, S2, S3, S4, and S5 of PINs (S271, D215 in PIN1/S215 in PIN3; Zourelidou et al., 2014). PID tends to phosphorylate S1, S2, and S3 first, whereas D6PK preferentially phosphorylates S4 and S5 (Zourelidou et al., 2009, 2014). D6PK also phosphorylates S1, S2, and S3, indicating that the function of these two kinases is redundant (Zourelidou et al., 2014). Surprisingly, S4 and S5 are not conserved in PIN2, and S5 may be replaced by a site that is naturally phosphorylated in PIN1 (Zourelidou et al., 2014). Although d6pk mutants show some similar morphological phenotypes to pid mutants, some differences between D6PK and PID have been noted. Loss-of-function and overexpression of D6PK do not change PIN polarity (Zourelidou et al., 2009). In addition, unlike PID, D6PK is localized only in the basal PM (in root cells) and only phosphorylates the basal PINs (Barbosa et al., 2014). These phosphorylated PIN proteins as well as D6PK are sensitive to BFA treatment, but D6PK may be more closely associated with GNOM-dependent recycling than PINs (Weller et al., 2017). An additional D6PK/D6PKL-related kinase is PAX. BRX is recruited by PAX to the basal PM, where BRX normally binds to PAX and impedes PIN transport at low auxin concentrations (Marhava et al., 2018; Xiao and Offringa, 2020). When the BRX abundance declines as auxin concentration increases, PAX phosphorylates PINs to promote auxin transport (Xiao and Offringa, 2020). 3-PHOSPHOINOSITIDE-DEPENDENT PROTEIN KINASE 1 (PDK1), may provide the link between PID and D6PK. PDK1 is also a member of the AGC family, and often acts as an upstream signal to phosphorylate and activate PID, D6PK, and PAX to modulate the polarity of PINs (Zegzouti et al., 2006; Tan et al., 2020).
MPKs
MAPK/MPKs are a conserved signaling kinase family that regulates many processes in plants, such as development and response to diverse environmental stresses (Rodriguez et al., 2010; Lin et al., 2021). This family always acts as a signal cascade. For example, MPK is phosphorylated by MPK KINASE (MPKK), which is further phosphorylated by MPKK kinase. MAP KINASE KINASE 7 (MKK7) is an upstream kinase of MAP6, and MKK7–MAP6 phosphorylates an unconservative site of PIN1, S337, to affect basal PM localization of PIN1 in the process of branch development (Jia et al., 2016). Interestingly, three conserved sites, T227, T248, and T286 of PIN1, which are located near the PID phosphorylation sites (S1–S3) and are components of the TPRXS motifs at S1–S3, are phosphorylated by MAP6 to influence the recycling of PIN1 (Dory et al., 2018). This finding indicates that PID may be associated with MPKs. However, the PM localization of PIN1 is abolished in most cells of MKK7 overexpression transformants, which differs from PID-and D6PK-overexpression transformants (Dory et al., 2018). Thus, MPKs may regulate PIN in a manner different from PID.
CRKs
Calcium ions are an important second messenger involved in gravitropism (Perera et al., 2006). CRKs are kinases responsive to Ca2+. CRK5 is localized to the PM and phosphorylates PINs to regulate plant development (Rigo et al., 2013). In the root transition region of crk5-1 mutants, the amount of PIN2 is reduced in the upper PM of epidermal cells, and is increased in the apical PM in the cortex, which is similar to the response in the wild type treated with a low concentration of BFA, although the localization of PIN1, 3, 4, and 7 is unchanged (Rigo et al., 2013). In addition, CRK5 may contribute to other mechanisms of PIN phosphorylation PINs. CRK5 regulates hypocotyl hook development, possibly through phosphorylation of PIN3, and embryo development through phosphorylation of PIN1, 4, and 7 (Baba et al., 2019a,b). No in vivo or in vitro evidence for the phosphorylation sites is available, although CRK5 can phosphorylate PIN1, 2, 3, 4, and 7 in vitro (Baba et al., 2019b). CRK5 is only supposed to phosphorylate S252 or S253 of PIN1, S271 of PIN4 and S431 and S277/S278 of PIN7 (Baba et al., 2019b). In addition to CRK5, CPK29 phosphorylates most PM-localized PINs to regulate the PIN polarity through BFA-insensitive recycling (Lee et al., 2021).
Other kinases
Other types of kinases affect PIN polarity, too. CANALIZATION RELATED AUXIN-REGULATED MALECTIN-TYPE RLK (CAMEL) and CANALIZATION-RELATED RECEPTOR-LIKE KINASE (CANAR) are kinases that influence PIN1 polarity and respond to auxin (Hajny et al., 2020). The kinase may phosphorylate T129, T234, S240, T257, and S408 of PIN1, which is different from other kinases (Hajny et al., 2020). The loss-of-function of CAMEL or CANAR causes defective PIN1 polarity (Hajny et al., 2020). However, compared with other types of kinases, this receptor-like kinase is poorly studied. This represents a novel research focus to gain insight into the relationships among kinases.
Phosphatases
The function of phosphatases is to dephosphorylate proteins and contribute to the homeostasis of reversible phosphorylation. PROTEIN PHOSPHATASE 2A (PP2A) is a heterotrimeric protein consisting of two regulatory subunits, A and B. It is involved in many processes that counteract PID. The PP2A A subunit is able to dephosphorylate PIN1 to change the polarity. Overexpression of PID further impairs mutants of pp2a subunits, whereas knocking out PID rescues pp2a A subunit mutants (Michniewicz et al., 2007b). In addition, pp2a A subunit mutants show basal-to-apical localization of PIN1, which is similar to the response to PID overexpression (Michniewicz et al., 2007b). Interestingly, other protein phosphatases, such as PP6 and PP1 also affect the PIN polarity. PHYTOCHROME-ASSOCIATED SER/THR PROTEIN PHOSPHATASE 1 (FyPP1) interacts with PP2A A subunit to form the PP6 heterotrimeric holoenzyme complex to regulate the phosphorylation of PIN proteins by antagonizing PID (Dai et al., 2012). An additional subunit of PP1, TYPE-ONE PROTEIN PHOSPHATASE 1 (TOPP1), acts antagonistically to PID (Guo et al., 2015). However, in contrast to kinases, few studies of phosphatases have been reported, and thus, the network existing among these phosphatases remains unknown.
To date, GNOM is the only intracellular trafficking-related protein known that specifically regulates PIN recycling. However, none of the kinases that can regulate the PIN polarity is recycled through GNOM-dependent trafficking. Only D6PK is associated with GNOM, but it does not change the PIN polarity. PID and GNOM have an antagonistic effect on the localization of PINs. PID can phosphorylate apical PINs and maintain the phosphorylation abundance to decrease GNOM-dependent subcellular trafficking of PINs (Kleine-Vehn et al., 2009). However, in response to BFA treatment, to which D6PK is sensitive but the PID is insensitive, PIN1 phosphorylation is not maintained in the wild type, but is maintained in engineered BFA-insensitive GNOM mutants, even though some of the PID proteins are localized to the basal PM and potentially may phosphorylate PINs (Barbosa et al., 2014). Thus, protein phosphatases may play a role in this process (Barbosa et al., 2014). Despite the Arf, Rab may also be associated with phosphorylation. Rab5 in endocytosis may be involved in phosphorylation (Weller et al., 2017). Notably, it is uncertain whether phosphorylation by each kinase can change the polarity of PIN proteins by intracellular trafficking directly from one part to another. This is because knocking out and overexpressing some kinases cause a clear change in PIN polarity (in pid mutants is basal and in PID-overexpression mutants is apical; in crk5 mutants is apical), but the phosphorylated PINs are observed on each side of the PM (Weller et al., 2017). The phosphorylated PINs may be confined to one side, newly synthesized PINs are secreted de novo to this side, and the PINs in other sides are degraded. This is because phosphorylated PINs can recruit MAB4/MEL to form positive feedback to limit lateral diffusion and maintain phosphorylation, and PIN2 is not recycled from the basal to the apical PM, but is newly secreted to the apical PM after cell division (Weller et al., 2017; Glanc et al., 2018).
Other post-translational modification
S-Nitrosylation (SNO), the addition of nitric oxide (NO), is controlled by NO concentrations and denitrosylation catalyzed by thioredoxin and S-NITROSOGLUTATHIONE REDUCTASE (GSNOR; Feechan et al., 2005; Sengupta and Holmgren, 2013). The lack of GSNOR1 inhibits the endocytosis of PINs by an uncertain mechanism. Both GNOM and PID have hypothetical SNO modifications (Ni et al., 2017; Sanchez-Vicente et al., 2021). The influence of GSNOR also indicates that PIN polarity may be affected by NO. In addition, ubiquitination of PINs influences the recycling and degradation of PINs. Loss of K63 ubiquitination of PIN2 interferes with its transport to the vacuole (Leitner et al., 2012).
Conclusion
The PIN-mediated short-distance transport of auxin is important to regulate plants growth and for tropic responses. A model to explain the distribution of PINs has been formulated. PINs are secreted, recycled or degraded through intracellular trafficking, in which GNOM plays a role to recycle the basal PINs, and PINs can be phosphorylated to change the polarity. However, some gaps in this model remain. Only GNOM or GNOM-like ARF-GEFs are known to be responsible for the recycling, but additional factors, such as the myosin and other Arf and Rab proteins are still poorly studied (Abu-Abied et al., 2018). Given that previous research on GNOM has invariably used BFA to induce the changes, the use of other inhibitors, such as endosidin 4, are also worth exploring (Kania et al., 2018). In the phosphorylation and dephosphorylation section, the model lacks the decisive evidence to determine which role, the change in PIN polarity or in PIN transport activity, is dominant. Furthermore, many kinases or protein phosphatases are functionally redundant but somewhat different in their target sites. In addition, the relationship between phosphorylation and trafficking remains unknown. It is uncertain how the locations of PINs are changed, after phosphorylation. Also, there are many other relevant factors not incorporated in this model. For example, ubiquitin can regulate whether PINs are directed to the vacuoles for degradation, the distribution of lipids, such as PI4P, PI(4,5)P2 and PA, can affect the localization of kinases and PIN, and the auxin fluxing through plasmodesmata directly challenges the PIN transport (Deak et al., 1999; Leitner et al., 2012; Tejos et al., 2014; Stanislas et al., 2015; Barbosa et al., 2016; Gao et al., 2020; Mellor et al., 2020). Certain environmental signals have been reported to affect PINs. For instance, PLD responds to environmental change to influence kinases (Wang et al., 2019). Nevertheless, these pathways do not form a network. An additional important point is to consider components from other organisms. Studies on Glut4 (GLUCOSE TRANSPORTER 4) in mammalian cells, CDC42-dependent symmetry-breaking pathway in yeast, and the TRANSPORT PROTEIN PARTICLE (TRAPP) complex all show the importance of referring to the components from other organisms, because subcellular trafficking is conserved among organisms and some components of PIN trafficking machinery are evolutionary conserved (Garcia et al., 2020; Glanc et al., 2021).
Author contributions
SC wrote the original manuscript and made the figures. SC and YW revised and completed the writing of the manuscript. All authors contributed to the article and approved the submitted version.
Funding
This work was supported by Hainan Yazhou Bay Seed Laboratory (B21HJ0220), National Natural Science Foundation of China (31871537 and U2003115) and Zhejiang Provincial Natural Science Foundation of China (LR21C020001).
Acknowledgments
We thank Jie Dong (Zhejiang University) and Fang Lin (Lanzhou University) for critical reading and advice on the manuscript, and Tao Jiang for drawing the figures.
Conflict of interest
The authors declare that the research was conducted in the absence of any commercial or financial relationships that could be construed as a potential conflict of interest.
Publisher’s note
All claims expressed in this article are solely those of the authors and do not necessarily represent those of their affiliated organizations, or those of the publisher, the editors and the reviewers. Any product that may be evaluated in this article, or claim that may be made by its manufacturer, is not guaranteed or endorsed by the publisher.
References
Abu-Abied, M., Belausov, E., Hagay, S., Peremyslov, V., Dolja, V., and Sadot, E. (2018). Myosin XI-K is involved in root organogenesis, polar auxin transport, and cell division. J. Exp. Bot. 69, 2869–2881. doi: 10.1093/jxb/ery112
Adamowski, M., and Friml, J. (2015). PIN-dependent auxin transport: action, regulation, and evolution. Plant Cell 27, 20–32. doi: 10.1105/tpc.114.134874
Ambrose, C., Ruan, Y., Gardiner, J., Tamblyn, L. M., Catching, A., Kirik, V., et al. (2013). CLASP interacts with sorting nexin 1 to link microtubules and auxin transport via PIN2 recycling in Arabidopsis thaliana. Dev. Cell 24, 649–659. doi: 10.1016/j.devcel.2013.02.007
Baba, A., Andrasi, N., Valkai, I., Gorcsa, T., Koczka, L., Darula, Z., et al. (2019a). AtCRK5 protein kinase exhibits a regulatory role in hypocotyl hook development during skotomorphogenesis. Int. J. Mol. Sci. 20:3424. doi: 10.3390/ijms20143432
Baba, A., Valkai, I., Labhane, N. M., Koczka, L., Andrasi, N., Klement, E., et al. (2019b). CRK5 protein kinase contributes to the progression of embryogenesis of Arabidopsis thaliana. Int. J. Mol. Sci. 20:6120. doi: 10.3390/ijms20246120
Barbosa, I. C. R., Shikata, H., Zourelidou, M., Heilmann, M., Heilmann, I., and Schwechheimer, C. (2016). Phospholipid composition and a polybasic motif determine D6 PROTEIN KINASE polar association with the plasma membrane and tropic responses. Development 143, 4687–4700. doi: 10.1242/dev.137117
Barbosa, I. C. R., Zourelidou, M., Willige, B. C., Weller, B., and Schwechheimer, C. (2014). D6 PROTEIN KINASE activates auxin transport-dependent growth and PIN-FORMED phosphorylation at the plasma membrane. Dev. Cell 29, 674–685. doi: 10.1016/j.devcel.2014.05.006
Benjamins, R., Quint, A., Weijers, D., Hooykaas, P., and Offringa, R. (2001). The PINOID protein kinase regulates organ development in Arabidopsis by enhancing polar auxin transport. Development 128, 4057–4067. doi: 10.1242/dev.128.20.4057
Benkova, E., Michniewicz, M., Sauer, M., Teichmann, T., Seifertova, D., Jurgens, G., et al. (2003). Local, efflux-dependent auxin gradients as a common module for plant organ formation. Cell 115, 591–602. doi: 10.1016/S0092-8674(03)00924-3
Bennett, S. R. M., Alvarez, J., Bossinger, G., and Smyth, D. R. (1995). Morphogenesis in pinoid mutants of Arabidopsis thaliana. Plant J. 8, 505–520. doi: 10.1046/j.1365-313X.1995.8040505.x
Bennett, T., Brockington, S. F., Rothfels, C., Graham, S. W., Stevenson, D., Kutchan, T., et al. (2014). Paralogous radiations of PIN proteins with multiple origins of noncanonical PIN structure. Mol. Biol. Evol. 31, 2042–2060. doi: 10.1093/molbev/msu147
Best, J. T., Xu, P., and Graham, T. R. (2019). Phospholipid flippases in membrane remodeling and transport carrier biogenesis. Curr. Opin. Cell Biol. 59, 8–15. doi: 10.1016/j.ceb.2019.02.004
Blakeslee, J. J., Bandyopadhyay, A., Peer, W. A., Makam, S. N., and Murphy, A. S. (2004). Relocalization of the PIN1 auxin efflux facilitator plays a role in phototropic responses. Plant Physiol. 134, 28–31. doi: 10.1104/pp.103.031690
Blilou, I., Xu, J., Wildwater, M., Willemsen, V., Paponov, I., Friml, J., et al. (2005). The PIN auxin efflux facilitator network controls growth and patterning in Arabidopsis roots. Nature 433, 39–44. doi: 10.1038/nature03184
Chen, X., Naramoto, S., Robert, S., Tejos, R., Lofke, C., Lin, D. S., et al. (2012). ABP1 and ROP6 GTPase signaling regulate Clathrin-mediated endocytosis in Arabidopsis roots. Curr. Biol. 22, 1326–1332. doi: 10.1016/j.cub.2012.05.020
Christensen, S. K., Dagenais, N., Chory, J., and Weigel, D. (2000). Regulation of auxin response by the protein kinase PINOID. Cell 100, 469–478. doi: 10.1016/S0092-8674(00)80682-0
Dai, M. Q., Zhang, C., Kania, U., Chen, F., Xue, Q., McCray, T., et al. (2012). A PP6-type phosphatase holoenzyme directly regulates PIN phosphorylation and auxin efflux in Arabidopsis. Plant Cell 24, 2497–2514. doi: 10.1105/tpc.112.098905
Deak, M., Casamayor, A., Currie, R. A., Downes, C. P., and Alessi, D. R. (1999). Characterisation of a plant 3-phosphoinositide-dependent protein kinase-1 homologue which contains a pleckstrin homology domain. FEBS Lett. 451, 220–226. doi: 10.1016/S0014-5793(99)00556-6
Dhonukshe, P., Aniento, F., Hwang, I., Robinson, D. G., Mravec, J., Stierhof, Y. D., et al. (2007). Clathrin-mediated constitutive endocytosis of PIN auxin efflux carriers in Arabidopsis. Curr. Biol. 17, 520–527. doi: 10.1016/j.cub.2007.01.052
Dhonukshe, P., Huang, F., Galvan-Ampudia, C. S., Mahonen, A. P., Kleine-Vehn, J., Xu, J. A., et al. (2010). Plasma membrane-bound AGC3 kinases phosphorylate PIN auxin carriers at TPRXS(N/S) motifs to direct apical PIN recycling. Development 137, 3245–3255. doi: 10.1242/dev.052456
Ding, Z. J., Galvan-Ampudia, C. S., Demarsy, E., Langowski, L., Kleine-Vehn, J., Fan, Y. W., et al. (2011). Light-mediated polarization of the PIN3 auxin transporter for the phototropic response in Arabidopsis. Nat. Cell Biol. 13, 447–452. doi: 10.1038/ncb2208
Ding, Z. J., Wang, B. J., Moreno, I., Duplakova, N., Simon, S., Carraro, N., et al. (2012). ER-localized auxin transporter PIN8 regulates auxin homeostasis and male gametophyte development in Arabidopsis. Nat. Commun. 3:941. doi: 10.1038/ncomms1941
Ditengou, F. A., Gomes, D., Nziengui, H., Kochersperger, P., Lasok, H., Medeiros, V., et al. (2018). Characterization of auxin transporter PIN6 plasma membrane targeting reveals a function for PIN6 in plant bolting. New Phytol. 217, 1610–1624. doi: 10.1111/nph.14923
Donaldson, J. G., and Jackson, C. L. (2000). Regulators and effectors of the ARF GTPases. Curr. Opin. Cell Biol. 12, 475–482. doi: 10.1016/S0955-0674(00)00119-8
Dory, M., Hatzimasoura, E., Kallai, B. M., Nagy, S. K., Jager, K., Darula, Z., et al. (2018). Coevolving MAPK and PID phosphosites indicate an ancient environmental control of PIN auxin transporters in land plants. FEBS Lett. 592, 89–102. doi: 10.1002/1873-3468.12929
Doyle, S. M., Haeger, A., Vain, T., Rigal, A., Viotti, C., Langowska, M., et al. (2015). An early secretory pathway mediated by GNOM-LIKE 1 and GNOM is essential for basal polarity establishment in Arabidopsis thaliana. Proc. Natl. Acad. U S A. 112, E806–E815. doi: 10.1073/pnas.1424856112
Drdova, E. J., Synek, L., Pecenkova, T., Hala, M., Kulich, I., Fowler, J. E., et al. (2013). The exocyst complex contributes to PIN auxin efflux carrier recycling and polar auxin transport in Arabidopsis. Plant J. 73, 709–719. doi: 10.1111/tpj.12074
Ebine, K., Fujimoto, M., Okatani, Y., Nishiyama, T., Goh, T., Ito, E., et al. (2011). A membrane trafficking pathway regulated by the plant-specific RAB GTPase ARA6. Nat. Cell Biol. 13, 853–859. doi: 10.1038/ncb2270
Ebine, K., and Ueda, T. (2009). Unique mechanism of plant endocytic/vacuolar transport pathways. J. Plant Res. 122, 21–30. doi: 10.1007/s10265-008-0200-x
Fasshauer, D., Sutton, R. B., Brunger, A. T., and Jahn, R. (1998). Conserved structural features of the synaptic fusion complex: SNARE proteins reclassified as Q-and R-SNAREs. Proc. Natl. Acad. U S A. 95, 15781–15786. doi: 10.1073/pnas.95.26.15781
Feechan, A., Kwon, E., Yun, B. W., Wang, Y. Q., Pallas, J. A., and Loake, G. J. (2005). A central role for S-nitrosothiols in plant disease resistance. Proc. Natl. Acad. U S A. 102, 8054–8059. doi: 10.1073/pnas.0501456102
Feraru, E., Feraru, M. I., Asaoka, R., Paciorek, T., De Rycke, R., Tanaka, H., et al. (2012). BEX5/RabA1b regulates trans-Golgi network-to-plasma membrane protein trafficking in Arabidopsis. Plant Cell 24, 3074–3086. doi: 10.1105/tpc.112.098152
Forestan, C., Farinati, S., and Varotto, S. (2012). The maize PIN gene family of auxin transporters. Front. Plant Sci. 3:16
Fotin, A., Cheng, Y. F., Sliz, P., Grigorieff, N., Harrison, S. C., Kirchhausen, T., et al. (2004). Molecular model for a complete clathrin lattice from electron cryomicroscopy. Nature 432, 573–579. doi: 10.1038/nature03079
Friml, J., Vieten, A., Sauer, M., Weijers, D., Schwarz, H., Hamann, T., et al. (2003). Efflux-dependent auxin gradients establish the apical-basal axis of Arabidopsis. Nature 426, 147–153. doi: 10.1038/nature02085
Friml, J., Wisniewska, J., Benkova, E., Mendgen, K., and Palme, K. (2002). Lateral relocation of auxin efflux regulator PIN3 mediates tropism in Arabidopsis. Nature 415, 806–809. doi: 10.1038/415806a
Friml, J., Yang, X., Michniewicz, M., Weijers, D., Quint, A., Tietz, O., et al. (2004). A PINOID-dependent binary switch in apical-basal PIN polar targeting directs auxin efflux. Science 306, 862–865. doi: 10.1126/science.1100618
Galvan-Ampudia, C. S., Julkowska, M. M., Darwish, E., Gandullo, J., Korver, R. A., Brunoud, G., et al. (2013). Halotropism is a response of plant roots to avoid a saline environment. Curr. Biol. 23, 2044–2050. doi: 10.1016/j.cub.2013.08.042
Galvan-Ampudia, C. S., and Offringa, R. (2007). Plant evolution: AGC kinases tell the auxin tale. Trends Plant Sci. 12, 541–547. doi: 10.1016/j.tplants.2007.10.004
Galweiler, L., Guan, C. H., Muller, A., Wisman, E., Mendgen, K., Yephremov, A., et al. (1998). Regulation of polar auxin transport by AtPIN1 in Arabidopsis vascular tissue. Science 282, 2226–2230. doi: 10.1126/science.282.5397.2226
Gao, Z., Chen, Z. W., Cui, Y. Y., Ke, M. Y., Xu, H. F., Xu, Q. Z., et al. (2021). GmPIN-dependent polar auxin transport is involved in soybean nodule development. Plant Cell 33, 2981–3003. doi: 10.1093/plcell/koab183
Gao, C., Liu, X. D., De Storme, N., Jensen, K. H., Xu, Q. Y., Yang, J. T., et al. (2020). Directionality of plasmodesmata-mediated transport in Arabidopsis leaves supports auxin channeling. Curr. Biol. 30, 1970–1977.e4. doi: 10.1016/j.cub.2020.03.014
Garcia, V. J., Xu, S. L., Ravikumar, R., Wang, W. F., Elliott, L., Gonzalez, E., et al. (2020). TRIPP is a plant-specific component of the Arabidopsis TRAPPII membrane trafficking complex with important roles in plant development. Plant Cell 32, 2424–2443. doi: 10.1105/tpc.20.00044
Geldner, N., Anders, N., Wolters, H., Keicher, J., Kornberger, W., Muller, P., et al. (2003). The Arabidopsis GNOM ARF-GEF mediates endosomal recycling, auxin transport, and auxin-dependent plant growth. Cell 112, 219–230. doi: 10.1016/S0092-8674(03)00003-5
Gho, Y. S., Song, M. Y., Bae, D. Y., Choi, H., and Jung, K. H. (2021). Rice PIN auxin efflux carriers modulate the nitrogen response in a changing nitrogen growth environment. Int. J. Mol. Sci. 22:3243. doi: 10.3390/ijms22063243
Glanc, M., Fendrych, M., and Friml, J. (2018). Mechanistic framework for cell-intrinsic re-establishment of PIN2 polarity after cell division. Nat. Plants 4, 1082–1088. doi: 10.1038/s41477-018-0318-3
Glanc, M., Van Gelderen, K., Hoermayer, L., Tan, S., Naramoto, S., Zhang, X., et al. (2021). AGC kinases and MAB4/MEL proteins maintain PIN polarity by limiting lateral diffusion in plant cells. Curr. Biol. 31, 1918–1930.e5. doi: 10.1016/j.cub.2021.02.028
Goldsmith, M. H. M., and Goldsmith, T. H. (1977). Chemiosmotic model for polar transport of auxin. Plant Physiol. 59:90
Grigolon, S., Sollich, P., and Martin, O. C. (2015). Modelling the emergence of polarity patterns for the intercellular transport of auxin in plants. J. R. Soc. Interface 12:20141223. doi: 10.1098/rsif.2014.1223
Gu, X. Y., Fonseka, K., Agneessens, J., Casson, S. A., Smertenko, A., Guo, G. Q., et al. (2021). The Arabidopsis R-SNARE VAMP714 is essential for polarisation of PIN proteins and auxin responses. New Phytol. 230, 550–566. doi: 10.1111/nph.17205
Guo, X. L., Qin, Q. Q., Yan, J., Niu, Y. L., Huang, B. Y., Guan, L. P., et al. (2015). TYPE-ONE PROTEIN PHOSPHATASE4 regulates pavement cell interdigitation by modulating PIN-FORMED1 polarity and trafficking in Arabidopsis. Plant Physiol. 167, 1058–1075. doi: 10.1104/pp.114.249904
Haga, K., Hayashi, K., and Sakai, T. (2014). PINOID AGC kinases are necessary for phytochrome-mediated enhancement of hypocotyl phototropism in Arabidopsis. Plant Physiol. 166, 1535–1545. doi: 10.1104/pp.114.244434
Hajny, J., Prat, T., Rydza, N., Rodriguez, L., Tan, S. T., Verstraeten, I., et al. (2020). Receptor kinase module targets PIN-dependent auxin transport during canalization. Science 370, 550–557. doi: 10.1126/science.aba3178
Han, H. B., Adamowski, M., Qi, L. L., Alotaibi, S. S., and Friml, J. (2021). PIN-mediated polar auxin transport regulations in plant tropic responses. New Phytol. 232, 510–522. doi: 10.1111/nph.17617
Han, X. L., Shi, Y., Liu, G. Y., Guo, Y., and Yang, Y. Q. (2018). Activation of ROP6 GTPase by phosphatidylglycerol in Arabidopsis. Front. Plant Sci. 9:347. doi: 10.3389/fpls.2018.00347
Heisler, M. G., Hamant, O., Krupinski, P., Uyttewaal, M., Ohno, C., Jonsson, H., et al. (2010). Alignment between PIN1 polarity and microtubule orientation in the shoot apical meristem reveals a tight coupling between morphogenesis and Auxin transport. PLoS Biol. 8:e1000516. doi: 10.1371/journal.pbio.1000516
Hou, M. M., Luo, F. F., Wu, D. X., Zhang, X. H., Lou, M. M., Shen, D. F., et al. (2021). OsPIN9, an auxin efflux carrier, is required for the regulation of rice tiller bud outgrowth by ammonium. New Phytol. 229, 935–949. doi: 10.1111/nph.16901
Hu, T. W., Yin, S. P., Sun, J. B., Linghu, Y. T., Ma, J. Q., Pan, J. W., et al. (2021). Clathrin light chains regulate hypocotyl elongation by affecting the polarization of the auxin transporter PIN3 in Arabidopsis. J. Integr. Plant Biol. 63, 1922–1936. doi: 10.1111/jipb.13171
Huang, F., Zago, M. K., Abas, L., van Marion, A., Galvan-Ampudia, C. S., and Offringa, R. (2010). Phosphorylation of conserved PIN motifs directs Arabidopsis PIN1 polarity and auxin transport. Plant Cell 22, 1129–1142. doi: 10.1105/tpc.109.072678
Inahashi, H., Shelley, I. J., Yamauchi, T., Nishiuchi, S., Takahashi-Nosaka, M., Matsunami, M., et al. (2018). OsPIN2, which encodes a member of the auxin efflux carrier proteins, is involved in root elongation growth and lateral root formation patterns via the regulation of auxin distribution in rice. Physiol. Plant. 164, 216–225. doi: 10.1111/ppl.12707
Ischebeck, T., Werner, S., Krishnamoorthy, P., Lerche, J., Meijon, M., Stenzel, I., et al. (2013). Phosphatidylinositol 4,5-Bisphosphate influences PIN polarization by controlling clathrin-mediated membrane trafficking in Arabidopsis. Plant Cell 25, 4894–4911. doi: 10.1105/tpc.113.116582
Ito, Y., Esnay, N., Platre, M. P., Wattelet-Boyer, V., Noack, L. C., Fougere, L., et al. (2021). Sphingolipids mediate polar sorting of PIN2 through phosphoinositide consumption at the trans-Golgi network. Nat. Commun. 12:4267. doi: 10.1038/s41467-021-24548-0
Ivanov, R., Brumbarova, T., Blum, A., Jantke, A. M., Fink-Straube, C., and Bauer, P. (2014). SORTING NEXIN1 is required for modulating the trafficking and dtability of the Arabidopsis IRON-REGULATED TRANSPORTER1. Plant Cell 26, 1294–1307. doi: 10.1105/tpc.113.116244
Jahn, R., Lang, T., and Sudhof, T. C. (2003). Membrane fusion. Cell 112, 519–533. doi: 10.1016/S0092-8674(03)00112-0
Jaillais, Y., Fobis-Loisy, I., Miege, C., Rollin, C., and Gaude, T. (2006). AtSNX1 defines an endosome for auxin-carrier trafficking in Arabidopsis. Nature 443, 106–109. doi: 10.1038/nature05046
Jaillais, Y., Santambrogio, M., Rozier, F., Fobis-Loisy, I., Miege, C., and Gaude, T. (2007). The retromer protein VPS29 links cell polarity and organ initiation in plants. Cell 130, 1057–1070. doi: 10.1016/j.cell.2007.08.040
Jia, W. Y., Li, B. H., Li, S. J., Liang, Y., Wu, X. W., Ma, M., et al. (2016). Mitogen-activated protein kinase cascade MKK7-MPK6 plays important roles in plant development and regulates shoot branching by phosphorylating PIN1 in Arabidopsis. PLoS Biol. 14:e1002550. doi: 10.1371/journal.pbio.1002550
Jonsson, K., Boutte, Y., Singh, R. K., Gendre, D., and Bhalerao, R. P. (2017). Ethylene regulates differential growth via BIG ARF-GEF-dependent post-Golgi secretory trafficking in Arabidopsis. Plant Cell 29, 1039–1052. doi: 10.1105/tpc.16.00743
Jonsson, H., Heisler, M. G., Shapiro, B. E., Meyerowitz, E. M., and Mjolsness, E. (2006). An auxin-driven polarized transport model for phyllotaxis. Proc. Natl. Acad. U S A. 103, 1633–1638. doi: 10.1073/pnas.0509839103
Kania, U., Nodzynski, T., Lu, Q., Hicks, G. R., Nerinckx, W., Mishev, K., et al. (2018). The inhibitor endosidin 4 targets SEC7 domain-type ARF GTPase exchange factors and interferes with subcellular trafficking in eukaryotes. Plant Cell 30, 2553–2572. doi: 10.1105/tpc.18.00127
Keicher, J., Jaspert, N., Weckermann, K., Moller, C., Throm, C., Kintzi, A., et al. (2017). Arabidopsis 14-3-3 epsilon members contribute to polarity of PIN auxin carrier and auxin transport-related development. Elife 6:e24336. doi: 10.7554/eLife.24336
Keuskamp, D. H., Pollmann, S., Voesenek, L., Peeters, A. J. M., and Pierik, R. (2010). Auxin transport through PIN-FORMED 3 (PIN3) controls shade avoidance and fitness during competition. Proc. Natl. Acad. U S A. 107, 22740–22744. doi: 10.1073/pnas.1013457108
Khan, A. R., and Menetrey, J. (2013). Structural biology of Arf and Rab GTPases' effector recruitment and specificity. Structure 21, 1284–1297. doi: 10.1016/j.str.2013.06.016
Kirchhausen, T., and Harrison, S. C. (1981). Protein organization in clathrin trimers. Cell 23, 755–761. doi: 10.1016/0092-8674(81)90439-6
Kitakura, S., Adamowski, M., Matsuura, Y., Santuari, L., Kouno, H., Arima, K., et al. (2017). BEN3/BIG2 ARF GEF is involved in brefeldin a-sensitive trafficking at the trans-golgi network/early endosome in Arabidopsis thaliana. Plant Cell Physiol. 58, 1801–1811. doi: 10.1093/pcp/pcx118
Kitakura, S., Vanneste, S., Robert, S., Lofke, C., Teichmann, T., Tanaka, H., et al. (2011). Clathrin mediates endocytosis and polar distribution of PIN auxin transporters in Arabidopsis. Plant Cell 23, 1920–1931. doi: 10.1105/tpc.111.083030
Kjos, I., Vestre, K., Guadagno, N. A., Distefano, M. B., and Progida, C. (2018). Rab and Arf proteins at the crossroad between membrane transport and cytoskeleton dynamics. BBA-Mol. Cell. Res. 1865, 1397–1409. doi: 10.1016/j.bbamcr.2018.07.009
Kleine-Vehn, J., Dhonukshe, P., Sauer, M., Brewer, P. B., Wisniewska, J., Paciorek, T., et al. (2008). ARF GEF-dependent transcytosis and polar delivery of PIN auxin carriers in Arabidopsis. Curr. Biol. 18, 526–531. doi: 10.1016/j.cub.2008.03.021
Kleine-Vehn, J., Ding, Z. J., Jones, A. R., Tasaka, M., Morita, M. T., and Friml, J. (2010). Gravity-induced PIN transcytosis for polarization of auxin fluxes in gravity-sensing root cells. Proc. Natl. Acad. U S A. 107, 22344–22349. doi: 10.1073/pnas.1013145107
Kleine-Vehn, J., Huang, F., Naramoto, S., Zhang, J., Michniewicz, M., Offringa, R., et al. (2009). PIN auxin efflux carrier polarity is regulated by PINOID kinase-mediated recruitment into GNOM-independent trafficking in Arabidopsis. Plant Cell 21, 3839–3849. doi: 10.1105/tpc.109.071639
Kumar, M., Kherawat, B. S., Dey, P., Saha, D., Singh, A., Bhatia, S. K., et al. (2021). Genome-wide identification and characterization of PIN-FORMED (PIN) gene family reveals role in developmental and various stress conditions in Triticum aestivum L. Int. J. Mol. Sci. 22:7396. doi: 10.3390/ijms22147396
Lee, H., Ganguly, A., Baik, S., and Cho, H. T. (2021). Calcium-dependent protein kinase 29 modulates PIN-FORMED polarity and Arabidopsis development via its own phosphorylation code. Plant Cell 33, 3513–3531. doi: 10.1093/plcell/koab207
Lee, H., Ganguly, A., Lee, R. D., Park, M., and Cho, H. T. (2020). Intracellularly localized PIN-FORMED8promotes lateral root emergence in Arabidopsis. Front. Plant Sci. 10:1808. doi: 10.3389/fpls.2019.01808
Leitner, J., Petrasek, J., Tomanov, K., Retzer, K., Parezova, M., Korbei, B., et al. (2012). Lysine(63)-linked ubiquitylation of PIN2 auxin carrier protein governs hormonally controlled adaptation of Arabidopsis root growth. Proc. Natl. Acad. U S A. 109, 8322–8327. doi: 10.1073/pnas.1200824109
Li, Z. X., Li, P., and Zhang, J. R. (2019). Expression analysis of PIN-formed auxin efflux transporter genes in maize. Plant Signal. Behav. 14:1632689. doi: 10.1080/15592324.2019.1632689
Li, G., and Xue, H. W. (2007). Arabidopsis PLD zeta 2 regulates vesicle trafficking and is required for auxin response. Plant Cell 19, 281–295. doi: 10.1105/tpc.106.041426
Lin, L., Wu, J., Jiang, M. Y., and Wang, Y. P. (2021). Plant mitogen-activated protein kinase cascades in environmental stresses. Int. J. Mol. Sci. 22:1543. doi: 10.3390/ijms22041543
Liu, J., Shi, X., Chang, Z., Ding, Y., and Ding, C. (2022). Auxin efflux transporters OsPIN1c and OsPIN1d function redundantly in regulating rice (Oryza sativa L.) panicle development. Plant Cell Physiol. 63, 305–316. doi: 10.1093/pcp/pcab172
Luschnig, C., Gaxiola, R. A., Grisafi, P., and Fink, G. R. (1998). EIR1, a root-specific protein involved in auxin transport, is required for gravitropism in Arabidopsis thaliana. Genes Dev. 12, 2175–2187. doi: 10.1101/gad.12.14.2175
Luschnig, C., and Vert, G. (2014). The dynamics of plant plasma membrane proteins: PINs and beyond. Development 141, 2924–2938. doi: 10.1242/dev.103424
Marhava, P., Bassukas, A. E. L., Zourelidou, M., Kolb, M., Moret, B., Fastner, A., et al. (2018). A molecular rheostat adjusts auxin flux to promote root protophloem differentiation. Nature 558, 297–300. doi: 10.1038/s41586-018-0186-z
Marhava, P., Fandino, A. C. A., Koh, S. W. H., Jelinkova, A., Kolb, M., Janacek, D. P., et al. (2020). Plasma membrane domain patterning and self-eeinforcing polarity in Arabidopsis. Dev. Cell 52, 223–235.e5. doi: 10.1016/j.devcel.2019.11.015
Mariyamma, N. P., Clarke, K. J., Yu, H. L., Wilton, E. E., Van Dyk, J., Hou, H. W., et al. (2018). Members of the Arabidopsis FORKED1-LIKE gene family act to localize PIN1 in developing veins. J. Exp. Bot. 69, 4773–4790. doi: 10.1093/jxb/ery248
Martiniere, A., and Moreau, P. (2020). Complex roles of Rabs and SNAREs in the secretory pathway and plant development: a never-ending story. J. Microsc. 280, 140–157. doi: 10.1111/jmi.12952
Matsuura, Y., Fukasawa, N., Ogita, K., Sasabe, M., Kakimoto, T., and Tanaka, H. (2020). Early endosomal trafficking component BEN2/VPS45 plays a crucial role in internal tissues in regulating root growth and meristem size in Arabidopsis. Front. Plant Sci. 11:1027. doi: 10.3389/fpls.2020.01027
Mellor, N. L., Voss, U., Janes, G., Bennett, M. J., Wells, D. M., and Band, L. R. (2020). Auxin fluxes through plasmodesmata modify root-tip auxin distribution. Development 147:dev181669. doi: 10.1242/dev.181669
Men, S. Z., Boutte, Y., Ikeda, Y., Li, X. G., Palme, K., Stierhof, Y. D., et al. (2008). Sterol-dependent endocytosis mediates post-cytokinetic acquisition of PIN2 auxin efflux carrier polarity. Nat. Cell Biol. 10, 237–244. doi: 10.1038/ncb1686
Michniewicz, M., Brewer, P. B., and Friml, J. I. (2007a). Polar auxin transport and asymmetric auxin distribution. Arabidopsis book 5:e0108
Michniewicz, M., Zago, M. K., Abas, L., Weijers, D., Schweighofer, A., Meskiene, I., et al. (2007b). Antagonistic regulation of PIN phosphorylation by PP2A and PINOID directs auxin flux. Cell 130, 1044–1056. doi: 10.1016/j.cell.2007.07.033
Miyashita, Y., Takasugi, T., and Ito, Y. (2010). Identification and expression analysis of PIN genes in rice. Plant Sci. 178, 424–428. doi: 10.1016/j.plantsci.2010.02.018
Mravec, J., Petrasek, J., Li, N., Boeren, S., Karlova, R., Kitakura, S., et al. (2011). Cell plate restricted association of DRP1A and PIN proteins is required for cell polarity establishment in Arabidopsis. Curr. Biol. 21, 1055–1060. doi: 10.1016/j.cub.2011.05.018
Mravec, J., Skupa, P., Bailly, A., Hoyerova, K., Krecek, P., Bielach, A., et al. (2009). Subcellular homeostasis of phytohormone auxin is mediated by the ER-localized PIN5 transporter. Nature 459, 1136–1140. doi: 10.1038/nature08066
Mueller-Roeber, B., and Pical, C. (2002). Inositol phospholipid metabolism in Arabidopsis. Characterized and putative isoforms of inositol phospholipid kinase and phosphoinositide-specific phospholipase C. Plant Physiol. 130, 22–46. doi: 10.1104/pp.004770
Naramoto, S., Kleine-Vehn, J., Robert, S., Fujimoto, M., Dainobu, T., Paciorek, T., et al. (2010). ADP-ribosylation factor machinery mediates endocytosis in plant cells. Proc. Natl. Acad. U S A. 107, 21890–21895. doi: 10.1073/pnas.1016260107
Naramoto, S., and Kyozuka, J. (2018). ARF GTPase machinery at the plasma membrane regulates auxin transport-mediated plant growth. Plant Biotechnol. 35, 155–159. doi: 10.5511/plantbiotechnology.18.0312a
Naramoto, S., Otegui, M. S., Kutsuna, N., de Rycke, R., Dainobu, T., Karampelias, M., et al. (2014). Insights into the localization and function of the membrane trafficking regulator GNOM ARF-GEF at the golgi apparatus in Arabidopsis. Plant Cell 26, 3062–3076. doi: 10.1105/tpc.114.125880
Ni, M., Zhang, L., Shi, Y. F., Wang, C., Lu, Y. R., Pan, J. W., et al. (2017). Excessive sellular s-nitrosothiol impairs endocytosis of auxin efflux transporter PIN2. Front. Plant Sci. 8:1988. doi: 10.3389/fpls.2017.01988
Ohya, T., Miaczynska, M., Coskun, U., Lommer, B., Runge, A., Drechsel, D., et al. (2009). Reconstitution of Rab-and SNARE-dependent membrane fusion by synthetic endosomes. Nature 459, 1091–1097. doi: 10.1038/nature08107
Pan, X., Fang, L. J., Liu, J. F., Senay-Aras, B., Lin, W. W., Zheng, S., et al. (2020). Auxin-induced signaling protein nanoclustering contributes to cell polarity formation. Nat. Commun. 11:3914. doi: 10.1038/s41467-020-17602-w
Perera, I. Y., Hung, C. Y., Brady, S., Muday, G. K., and Boss, W. F. (2006). A universal role for inositol 1,4,5-trisphosphate-mediated signaling in plant gravitropism. Plant Physiol. 140, 746–760. doi: 10.1104/pp.105.075119
Platre, M. P., Bayle, V., Armengot, L., Bareille, J., Marques-Bueno, M. D., Creff, A., et al. (2019). Developmental control of plant rho GTPase nano-organization by the lipid phosphatidylserine. Science 364, 57–62. doi: 10.1126/science.aav9959
Pratelli, J., Sutter, J. U., and Blatt, M. R. (2004). A new catch in the SNARE. Trends Plant Sci. 9, 187–195. doi: 10.1016/j.tplants.2004.02.007
Preuss, M. L., Schmitz, A. J., Thole, J. M., Bonner, H. K. S., Otegui, M. S., and Nielsen, E. (2006). A role for the RabA4b effector protein PI-4K beta 1 in polarized expansion of root hair cells in Arabidopsis thaliana. J. Cell Biol. 172, 991–998. doi: 10.1083/jcb.200508116
Rakusova, H., Gallego-Bartolome, J., Vanstraelen, M., Robert, H. S., Alabadi, D., Blazquez, M. A., et al. (2011). Polarization of PIN3-dependent auxin transport for hypocotyl gravitropic response in Arabidopsis thaliana. Plant J. 67, 817–826. doi: 10.1111/j.1365-313X.2011.04636.x
Ramos, J. R. D., Maizel, A., and Alim, K. (2021). Tissue-wide integration of mechanical cues promotes effective auxin patterning. Eur. Phys. J. Plus 136:250. doi: 10.1140/epjp/s13360-021-01204-6
Rein, U., Andag, U., Duden, R., Schmitt, H. D., and Spang, A. (2002). ARF-GAP-mediated interaction between the ER-Golgi v-SNAREs and the COPI coat. J. Cell Biol. 157, 395–404. doi: 10.1083/jcb.200112092
Reuter, L., Schmidt, T., Manishankar, P., Throm, C., Keicher, J., Bock, A., et al. (2021). Light-triggered and phosphorylation-dependent 14-3-3 association with NON-PHOTOTROPIC HYPOCOTYL 3 is required for hypocotyl phototropism. Nat. Commun. 12:6128. doi: 10.1038/s41467-021-26332-6
Richter, S., Geldner, N., Schrader, J., Wolters, H., Stierhof, Y. D., Rios, G., et al. (2007). Functional diversification of closely related ARF-GEFs in protein secretion and recycling. Nature 448, 488–492. doi: 10.1038/nature05967
Richter, S., Kientz, M., Brumm, S., Nielsen, M. E., Park, M., Gavidia, R., et al. (2014). Delivery of endocytosed proteins to the cell-division plane requires change of pathway from recycling to secretion. Elife 3:e02131. doi: 10.7554/eLife.02131
Rigo, G., Ayaydin, F., Tietz, O., Zsigmond, L., Kovacs, H., Pay, A., et al. (2013). Inactivation of plasma membrane-localized CDPK-RELATED KINASE5 decelerates PIN2 exocytosis and root gravitropic response in Arabidopsis. Plant Cell 25, 1592–1608. doi: 10.1105/tpc.113.110452
Robinson, D. G., and Pimpl, P. (2014). Clathrin and post-Golgi trafficking: a very complicated issue. Trends Plant Sci. 19, 134–139. doi: 10.1016/j.tplants.2013.10.008
Rodriguez, M. C. S., Petersen, M., and Mundy, J. (2010). Mitogen-activated protein kinase signaling in plants. Annu. Rev. Plant Biol. 61, 621–649. doi: 10.1146/annurev-arplant-042809-112252
Rodriguez-Furlan, C., Minina, E. A., and Hicks, G. R. (2019). Remove, recycle, degrade: regulating plasma membrane protein accumulation. Plant Cell 31, 2833–2854. doi: 10.1105/tpc.19.00433
Rojek, J., Tucker, M. R., Pinto, S. C., Rychlowski, M., Lichocka, M., Soukupova, H., et al. (2021a). Rab-dependent vesicular traffic affects female gametophyte development in Arabidopsis. J. Exp. Bot. 72, 320–340. doi: 10.1093/jxb/eraa430
Rojek, J., Tucker, M. R., Rychlowski, M., Nowakowska, J., and Gutkowska, M. (2021b). The Rab geranylgeranyl transferase beta subunit is essential for embryo and seed development in Arabidopsis thaliana. Int. J. Mol. Sci. 22:7907. doi: 10.3390/ijms22157907
Rosquete, M. R., von Wangenheim, D., Marhavy, P., Barbez, E., Stelzer, E. H. K., Benkova, E., et al. (2013). An auxin transport mechanism restricts positive orthogravitropism in lateral roots. Curr. Biol. 23, 817–822. doi: 10.1016/j.cub.2013.03.064
Saeed, B., Brillada, C., and Trujillo, M. (2019). Dissecting the plant exocyst. Curr. Opin. Plant Biol. 52, 69–76. doi: 10.1016/j.pbi.2019.08.004
Sanchez-Vicente, I., Lechon, T., Fernandez-Marcos, M., Sanz, L., and Lorenzo, O. (2021). Nitric oxide alters the pattern of auxin maxima and PIN-FORMED1 during shoot development. Front. Plant Sci. 12:630792. doi: 10.3389/fpls.2021.630792
Sengupta, R., and Holmgren, A. (2013). Thioredoxin and thioredoxin reductase in relation to reversible s-nitrosylation. Antioxid. Redox Signal. 18, 259–269. doi: 10.1089/ars.2012.4716
Sisi, N. A., and Ruzicka, K. (2020). ER-localized PIN carriers: regulators of intracellular auxin homeostasis. Plants 9:1527. doi: 10.3390/plants9111527
Stanislas, T., Huser, A., Barbosa, I. C. R., Kiefer, C. S., Brackmann, K., Pietra, S., et al. (2015). Arabidopsis D6PK is a lipid domain-dependent mediator of root epidermal planar polarity. Nat. Plants 1:15162. doi: 10.1038/nplants.2015.162
Steinmann, T., Geldner, N., Grebe, M., Mangold, S., Jackson, C. L., Paris, S., et al. (1999). Coordinated polar localization of auxin efflux carrier PIN1 by GNOM ARF GEF. Science 286, 316–318. doi: 10.1126/science.286.5438.316
Sun, H. W., Tao, J. Y., Bi, Y., Hou, M. M., Lou, J. J., Chen, X. N., et al. (2018). OsPIN1b is involved in rice seminal root elongation by regulating root apical meristem activity in response to low nitrogen and phosphate. Sci. Rep. 8:13014. doi: 10.1038/s41598-018-29784-x
Swarup, R., and Bennett, M. (2003). Auxin transport: The fountain of life in plants? Dev. Cell 5, 824–826.
Tamura, K., Stecher, G., and Kumar, S. (2021). MEGA11 molecular evolutionary genetics analysis version 11. Mol. Biol. Evol. 38, 3022–3027. doi: 10.1093/molbev/msab120
Tan, X. Y., Feng, Y. H., Liu, Y. L., and Bao, Y. Q. (2016). Mutations in exocyst complex subunit SEC6 gene impaired polar auxin transport and PIN protein recycling in Arabidopsis primary root. Plant Sci. 250, 97–104. doi: 10.1016/j.plantsci.2016.06.001
Tan, S. T., Zhang, X. X., Kong, W., Yang, X. L., Molnar, G., Vondrakova, Z., et al. (2020). The lipid code-dependent phosphoswitch PDK1-D6PK activates PIN-mediated auxin efflux in Arabidopsis. Nat. Plants 6, 556–569. doi: 10.1038/s41477-020-0648-9
Tang, R. J., Luan, M. D., Wang, C., Lhamo, D., Yang, Y., Zhao, F. G., et al. (2020). Plant membrane transport research in the post-genomic era. Plant Commun. 1:100013. doi: 10.1016/j.xplc.2019.100013
Tang, L. P., Yang, Y., Wang, H., Li, L. X., Liu, L., Liu, Y., et al. (2021). AtNSF regulates leaf serration by modulating intracellular trafficking of PIN1 in Arabidopsis thaliana. J. Integr. Plant Biol. 63, 737–755. doi: 10.1111/jipb.13043
Teh, O. K., and Moore, I. (2007). An ARF-GEF acting at the Golgi and in selective endocytosis in polarized plant cells. Nature 448, 493–496. doi: 10.1038/nature06023
Tejos, R., Sauer, M., Vanneste, S., Palacios-Gomez, M., Li, H. J., Heilmann, M., et al. (2014). Bipolar plasma membrane distribution of phosphoinositides and their requirement for auxin-mediated cell polarity and patterning in Arabidopsis. Plant Cell 26, 2114–2128. doi: 10.1105/tpc.114.126185
Ungewickell, E., and Branton, D. (1981). Assembly units of clathrin coats. Nature 289, 420–422. doi: 10.1038/289420a0
Wang, Y. Q., Chai, C. L., Valliyodan, B., Maupin, C., Annen, B., and Nguyen, H. T. (2015). Genome-wide analysis and expression profiling of the PIN auxin transporter gene family in soybean (Glycine max). BMC Genomics 16:951. doi: 10.1186/s12864-015-2149-1
Wang, J. R., Hu, H., Wang, G. H., Li, J., Chen, J. Y., and Wu, P. (2009). Expression of PIN genes in rice (Oryza sativa L.): tissue specificity and regulation by hormones. Mol. Plant 2, 823–831. doi: 10.1093/mp/ssp023
Wang, P. P., Shen, L. K., Guo, J. H., Jing, W., Qu, Y., Li, W. Y., et al. (2019). Phosphatidic acid directly regulates PINOID-dependent phosphorylation and activation of the PIN-FORMED2 auxin efflux transporter in response to salt stress. Plant Cell 31, 250–271. doi: 10.1105/tpc.18.00528
Wang, Y., Yang, L., Tang, Y. M., Tang, R. J., Jing, Y. P., Zhang, C., et al. (2017). Arabidopsis choline transporter-like 1 (CTL1) regulates secretory trafficking of auxin transporters to control seedling growth. PLoS Biol. 15:e2004310. doi: 10.1371/journal.pbio.2004310
Weller, B., Zourelidou, M., Frank, L., Barbosa, I. C. R., Fastner, A., Richter, S., et al. (2017). Dynamic PIN-FORMED auxin efflux carrier phosphorylation at the plasma membrane controls auxin efflux-dependent growth. Proc. Natl. Acad. Sci. U. S. A. 114, E887–E896. doi: 10.1073/pnas.1614380114
Willige, B. C., Ogiso-Tanaka, E., Zourelidou, M., and Schwechheimer, C. (2012). WAG2 represses apical hook opening downstream from gibberellin and PHYTOCHROME INTERACTING FACTOR 5. Development 139, 4020–4028. doi: 10.1242/dev.081240
Xiao, Y., and Offringa, R. (2020). PDK1 regulates auxin transport and Arabidopsis vascular development through AGC1 kinase PAX. Nat. Plants 6, 544–555. doi: 10.1038/s41477-020-0650-2
Xie, X. D., Qin, G. Y., Si, P., Luo, Z. P., Gao, J. P., Chen, X., et al. (2017). Analysis of Nicotiana tabacum PIN genes identifies NtPIN4 as a key regulator of axillary bud growth. Physiol. Plant. 160, 222–239. doi: 10.1111/ppl.12547
Yu, Q. Q., Zhang, Y., Wang, J., Yan, X., Wang, C., Xu, J., et al. (2016). Clathrin-mediated auxin efflux and maxima regulate hypocotyl hook formation and light-stimulated hook opening in Arabidopsis. Mol. Plant 9, 101–112. doi: 10.1016/j.molp.2015.09.018
Zegzouti, H., Anthony, R. G., Jahchan, N., Bogre, L., and Christensen, S. K. (2006). Phosphorylation and activation of PINOID by the phospholipid signaling kinase 3-phosphoinositidedependent protein kinase 1 (PDK1) in Arabidopsis. Proc. Natl. Acad. Sci. U. S. A. 103, 6404–6409. doi: 10.1073/pnas.0510283103
Zhang, X. X., Adamowski, M., Marhava, P., Tan, S. T., Zhang, Y. Z., Rodriguez, L., et al. (2020). Arabidopsis flippases cooperate with ARF GTPase exchange factors to regulate the trafficking and polarity of PIN auxin transporters. Plant Cell 32, 1644–1664. doi: 10.1105/tpc.19.00869
Zhang, Q., Li, J. J., Zhang, W. J., Yan, S. N., Wang, R., Zhao, J. F., et al. (2012). The putative auxin efflux carrier OsPIN3t is involved in the drought stress response and drought tolerance. Plant J. 72, 805–816. doi: 10.1111/j.1365-313X.2012.05121.x
Zhang, L., Ma, J. W., Liu, H., Yi, Q., Wang, Y. N., Xing, J. J., et al. (2021). SNARE proteins VAMP721 and VAMP722 mediate the post-Golgi trafficking required for auxin-mediated development in Arabidopsis. Plant J. 108, 426–440. doi: 10.1111/tpj.15450
Zhang, Y., Yu, Q. Q., Jiang, N., Yan, X., Wang, C., Wang, Q. M., et al. (2017). Clathrin regulates blue light-triggered lateral auxin distribution and hypocotyl phototropism in Arabidopsis. Plant Cell Environ. 40, 165–176. doi: 10.1111/pce.12854
Zhou, J. J., and Luo, J. (2018). The PIN-FORMED auxin efflux carriers in plants. Int. J. Mol. Sci. 19:2759. doi: 10.3390/ijms19092759
Zourelidou, M., Absmanner, B., Weller, B., Barbosa, I. C. R., Willige, B. C., Fastner, A., et al. (2014). Auxin efflux by PIN-FORMED proteins is activated by two different PROTEIN kinases, D6 PROTEIN KINASE and PINOID. Elife 3:e02860. doi: 10.7554/eLife.02860
Zourelidou, M., Muller, I., Willige, B. C., Nill, C., Jikumaru, Y., Li, H. B., et al. (2009). The polarly localized D6 PROTEIN KINASE is required for efficient auxin transport in Arabidopsis thaliana. Development 136, 627–636. doi: 10.1242/dev.028365
Keywords: PINs, auxin transport, polarity, subcellular trafficing, post-translational modification
Citation: Cheng S and Wang Y (2022) Subcellular trafficking and post-translational modification regulate PIN polarity in plants. Front. Plant Sci. 13:923293. doi: 10.3389/fpls.2022.923293
Edited by:
Ivan A. Paponov, Aarhus University, DenmarkReviewed by:
Franck Anicet Ditengou, University of Freiburg, GermanySilvia Grigolon, UMR8237 Laboratoire Jean PERRIN (LJP), France
Copyright © 2022 Cheng and Wang. This is an open-access article distributed under the terms of the Creative Commons Attribution License (CC BY). The use, distribution or reproduction in other forums is permitted, provided the original author(s) and the copyright owner(s) are credited and that the original publication in this journal is cited, in accordance with accepted academic practice. No use, distribution or reproduction is permitted which does not comply with these terms.
*Correspondence: Yizhou Wang, wangyizhou@zju.edu.cn