- 1Department of Biotechnology and Systems Biology, National Institute of Biology, Ljubljana, Slovenia
- 2Jožef Stefan International Postgraduate School, Ljubljana, Slovenia
TGA transcription factors are essential regulators of various cellular processes, their activity connected to different hormonal pathways, interacting proteins and regulatory elements. Belonging to the basic region leucine zipper (bZIP) family, TGAs operate by binding to their target DNA sequence as dimers through a conserved bZIP domain. Despite sharing the core DNA-binding sequence, the TGA paralogues exert somewhat different DNA-binding preferences. Sequence variability of their N- and C-terminal protein parts indicates their importance in defining TGA functional specificity through interactions with diverse proteins, affecting their DNA-binding properties. In this review, we provide a short and concise summary on plant TGA transcription factors from a structural point of view, including the relation of their structural characteristics to their functional roles in transcription regulation.
Introduction
The Arabidopsis thaliana genome encodes for over 2,200 transcription factor genes, according to the Plant Transcription Factor Database1 yet few of them have been thoroughly characterized. The TGACG-binding (TGA) transcription factors were among the first plant transcription factors ever studied, their discovery dating back to the year of 1989 (Katagiri et al., 1989). Named after their hallmark binding site, the TGA factors became known for their regulation of defense-related genes through interaction with NON-EXPRESSOR OF PR-1 (NPR1) cofactor (Zhang et al., 1999), a salicylic acid receptor and master regulator of plant immunity (Wu et al., 2012; Backer et al., 2019; Wang W. et al., 2020). Among dicot plant species, the ten Arabidopsis TGA factors, AtTGA1-7, AtPERIANTHIA (AtPAN), and AtTGA9-10, have been investigated most thoroughly, next to five tobacco (Nicotiana tabacum) members, NtTGA1A, NtPG13, NtTGA2.1, NtTGA2.2, and NtTGA10. They are distributed into five clades (Jakoby et al., 2002), which are phylogenetically divided into two branches (Figure 1). Functional analysis of TGAs from different clades revealed not only their importance in biotic stress response (Zhang et al., 2003; Kesarwani et al., 2007; Zander et al., 2010; Sun et al., 2018), but also in regulation of gene expression connected to abiotic stress responses (Zhong et al., 2015; Fang et al., 2017; Herrera-Vásquez et al., 2021), developmental processes (Murmu et al., 2010; Maier et al., 2011; Wang et al., 2019), circadian rhythm (Zhou et al., 2015), detoxification (Fode et al., 2008; Mueller et al., 2008; Herrera-Vásquez et al., 2021), nitrate signaling (Alvarez et al., 2014; Canales et al., 2017), flowering (Thurow et al., 2005; Song et al., 2008; Maier et al., 2011; Xu et al., 2021), and autophagy (Wang P. et al., 2020) (Figure 1). Initially, the function of TGAs from clades I, II, and III was mainly associated with plant immunity, whereas the first reports on clade IV and V members revealed their role in regulating developmental processes (reviewed in Gatz, 2013). However, this apparent functional division is becoming less evident as an increasing number of reports show that most clades are involved in a variety of processes (Figure 1). For example, clade I TGAs have also been shown to be involved in regulating growth and development (Li et al., 2019; Wang et al., 2019), while clade IV TGAs are also important in biotic stress (Noshi et al., 2016; Venturuzzi et al., 2021).
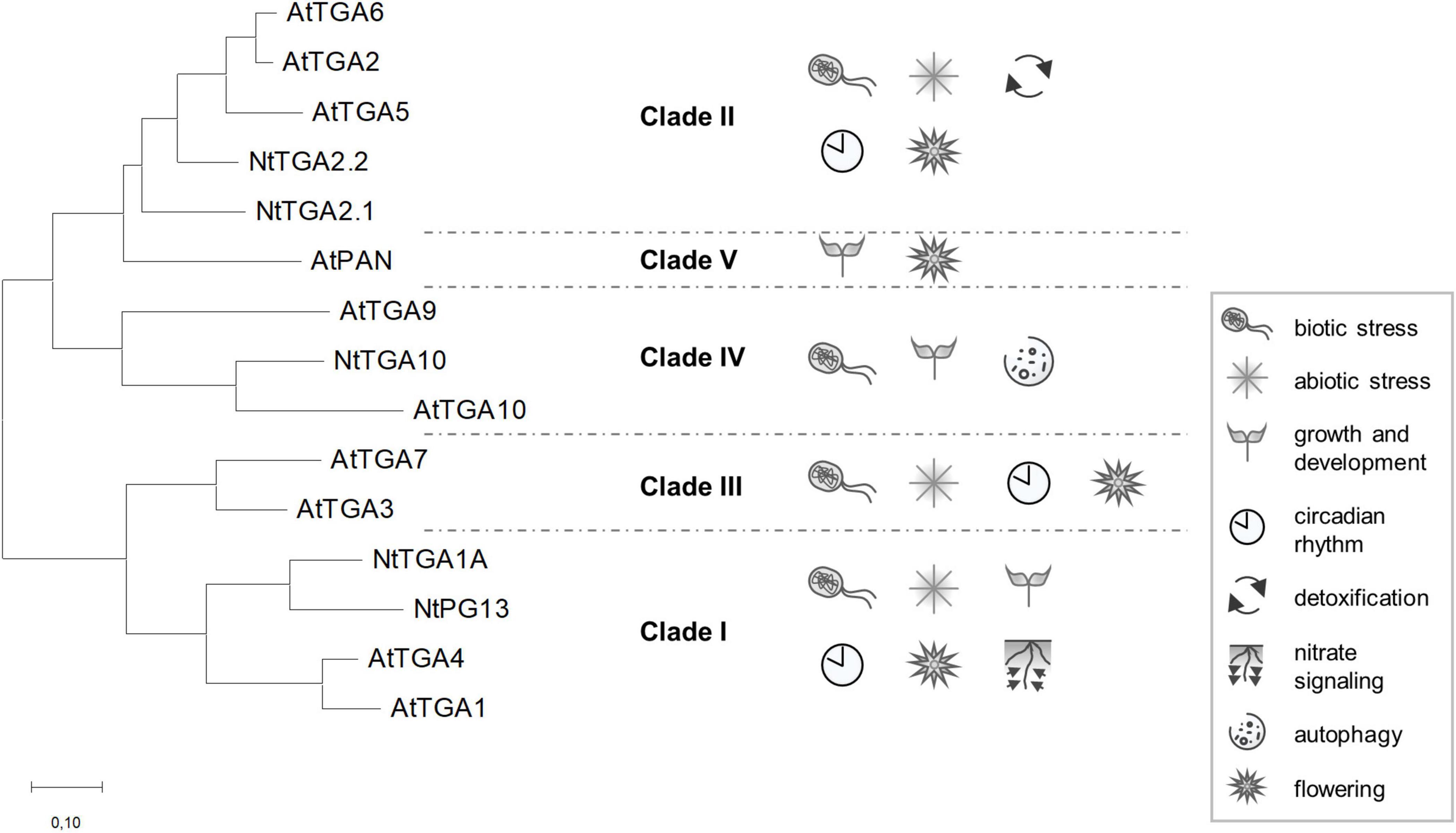
Figure 1. Unrooted phylogenetic tree of Arabidopsis and tobacco TGAs. Phylogenetic analysis of TGA factors shows an earlier separation of clades into two branches, one dividing into clades II, IV, and V, the other into clades I and III, indicating a closer evolutionary relationship between clade members in the same branch. TGA involvement in regulation of different processes, based on literature search, is represented for each clade. Sequence alignment by MUSCLE (Edgar, 2004) and phylogenetic analysis by the Maximum Likelihood method, based on the JTT matrix-based model (Jones et al., 1992), were conducted in MEGA7 (Kumar et al., 2016). The branch length scale represents the number of substitutions per site. Protein sequences with listed protein identification numbers were retrieved from UniProtKB (https://www.uniprot.org/): AtTGA1 (Q39237), AtTGA2 (P43273), AtTGA3 (Q39234), AtTGA4 (Q39162), AtTGA5 (Q39163), AtTGA6 (Q39140), AtTGA7 (Q93ZE2), AtPAN (Q9SX27), AtTGA9 (Q93XM6), AtTGA10 (E3VNM4), NtTGA1A (P14232), NtPG13 (Q05699), NtTGA2.1 (O24160), NtTGA2.2 (Q9SQK1), and NtTGA10 (Q52MZ2).
A detailed review, integrating TGA transcription factor research, was published last in 2013 (Gatz, 2013). The complexity of TGA involvement in various molecular processes, the lack of research supporting the proposed mechanisms of action and limited reports on the relation between structure and in vivo function have become characteristic features of TGA studies and are the reason for our lack of knowledge about their mechanism of action. In order to understand how transcription factors operate, we must consider their structural characteristics as the underlying basis of protein activity. Here, we consider the importance of reported and in silico determined TGA structural features in defining their functional specificity and variability, focusing on the characterized TGA factors from Arabidopsis and tobacco.
From TGA factor structure to their function
For more than 30 years after their discovery, the TGA protein three-dimensional (3D) structure remained a mystery and the first structural data have been published only recently in a breakthrough report providing a partial cryo-electron microscopy (cryo-EM) structure of AtTGA3 in complex with NPR1 (Kumar et al., 2022). Additionally, the novel artificial intelligence algorithm AlphaFold (AF) allows structure prediction without the availability of known similar structures (Jumper et al., 2021) and AF models for full-length Arabidopsis and tobacco TGAs are already available in the AF Protein Structure Database (Figure 2A; Varadi et al., 2022). In the following subchapters, we aim to connect literature reports with in silico analyses, to better understand the biological role of the three main structural parts of TGAs: The conserved basic region leucine zipper (bZIP) domain, the highly variable N-terminal part, from now on referred to as the N-terminus, and the C-terminal part (C-terminus), containing a putative Delay of Germination 1 (DOG1) domain (Figures 2A,B).
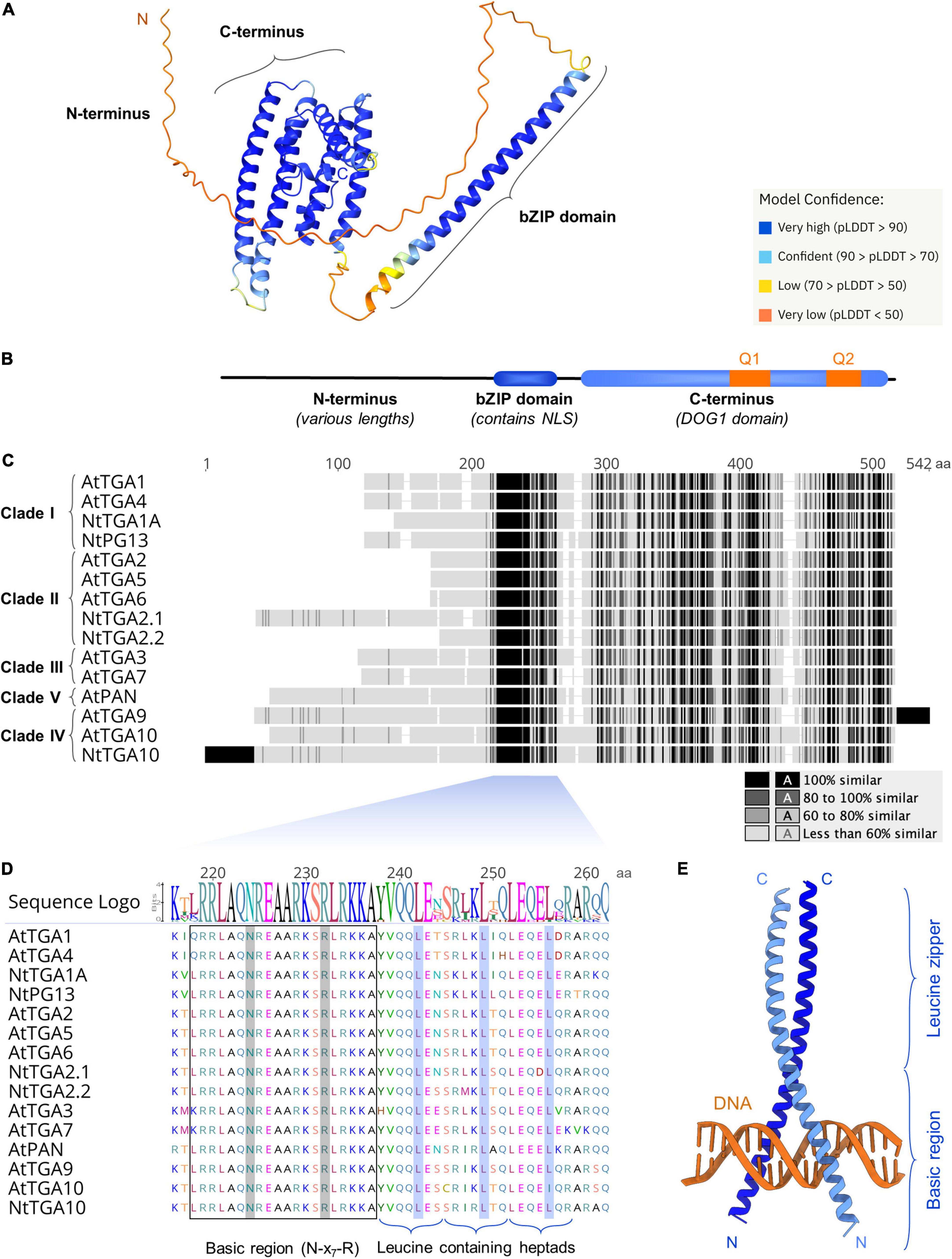
Figure 2. Among the three main TGA protein parts, the bZIP domain is the most highly conserved. (A) The AlphaFold generated 3D model of AtTGA1 (Jumper et al., 2021; Varadi et al., 2022) (pLDDT, AlphaFold per-residue confidence score) and (B) a schematic representation of TGA domain organization, showing the flexible N-terminus, the bZIP domain and the C-terminus, encompassing a putative Delay of Germination 1 (DOG1) domain. The nuclear localization signal (NLS) and glutamine rich regions Q1 and Q2 are indicated. (C) Multiple sequence alignment of ten Arabidopsis and five tobacco TGAs, with segments of high similarity or identity colored darkest and lowest similarity lightest, shows the bZIP domain retains the highest sequence identity throughout the whole protein sequence. In cases where sequence segments at N-terminal or C-terminal ends are not aligned to any of the other sequences, they are considered identical and are colored black. (D) A closer examination of the bZIP domain shows few variations in the basic region, while the three zipper heptads, with conserved leucine residue positions marked, show higher variability. The alignment and sequence logo were prepared and visualized with Geneious Prime 2020 (Kearse et al., 2012), using default parameters. (E) Structural model of the human FosB-JunD bZIP dimer in complex with DNA (5VPE entry in RCSB PDB) (Yin et al., 2017). The models in (A,E) were visualized in UCSF ChimeraX (Pettersen et al., 2021).
The highly conserved DNA-binding domain
TGAs are members of the bZIP protein superfamily and represent a plant-specific subgroup, found in different species, including mosses and liverworts (Gutsche and Zachgo, 2016; Gutsche et al., 2017). bZIP proteins are defined by their DNA-binding and dimerization region known as the bZIP domain, which is highly conserved among plants and even across kingdoms (Jindrich and Degnan, 2016). Multiple sequence alignment of Arabidopsis and tobacco TGAs shows the bZIP domain as the region of highest protein sequence identity, regardless of plant species (Figure 2C). The bZIP domain determines DNA-binding specificity and serves as a nuclear localization signal (Figure 2B; van der Krol and Chua, 1991; Deppmann et al., 2004). It consists of two regions, the basic region and the leucine zipper. Hydrogen bond formation with the major DNA groove is facilitated through the basic region, which contains an invariant N-x7-R/K motif (Dröge-Laser et al., 2018). Nineteen out of 20 amino acids of the TGA basic region, including the N-x7-R motif, remain identical in all aligned Arabidopsis and tobacco sequences, with a few clade/species-specific differences present only in the first residue (Figure 2D).
bZIP proteins bind target DNA as dimers, with combinatorial homo- or heterodimerization at the DNA-binding site granting them broad variability in regulation of physiological responses (Deppmann et al., 2006; Rodríguez-Martínez et al., 2017). The leucine zipper confers dimer formation and determines dimerization specificity. It consists of repetitive seven-amino acid units, called heptads. Each heptad contains a conserved leucine residue at its fifth position (Landschulz et al., 1988; Deppmann et al., 2006). TGA bZIP domains have three leucine zipper heptads, which show higher variability than the basic region, yet retain the conserved leucines. The only exception is AtTGA10, where the third leucine is replaced with isoleucine (Figure 2D). The number of heptads is among the lowest compared to other Arabidopsis bZIP proteins (Deppmann et al., 2004), rendering the 41 aa bZIP domains in TGAs considerably shorter from the typical 60–80 aa bZIP length (Jindrich and Degnan, 2016). Additionally, the leucine zippers of TGAs contain destabilizing residues at dimer contact sites, making the zipper formation less stable (Deppmann et al., 2004). The extent to which dimerization stability affects transcription factor binding time at its specific motif is not known and unstable interactions might shorten DNA-binding times, resulting in a lower number of generated transcripts (Swift and Coruzzi, 2017).
During DNA-binding the bZIP domains of two proteins grip the DNA segment in a scissor-like fashion, while attaining an alpha-helical fold (Vinson et al., 1989; Ellenberger et al., 1992), as shown in the human FosB-JunD-DNA complex 3D structure (Figure 2E; Yin et al., 2017). The role of the leucine zipper in TGA dimerization had been demonstrated by switching the leucine zipper in NtTGA2.2 for a zipper from the human Jun bZIP protein, which prevented heterodimerization with NtTGA2.1 (Thurow et al., 2005). However, deletion of 93 aa from AtTGA2 N-terminus, including the entire bZIP domain, still allowed homodimer formation (Boyle et al., 2009) and multiple reports have shown that TGA dimerization depends significantly on other protein parts as well. A dimer stabilization region had been identified in the C-terminus of tobacco NtTGA1A, located between 178 and 373 aa (Katagiri et al., 1992). Formation of stable contacts through the TGA C-terminus was recently confirmed with cryo-EM, which revealed homodimerization of AtTGA3 C-termini in the AtTGA3-NPR1 complex structure (Kumar et al., 2022). Moreover, deleting the residues from 146 to 330, spanning more than half of the protein C-terminus, abolished the DNA-binding activity of AtTGA2 (Johnson et al., 2008), which could be due to hindered dimerization. Additionally, protein interaction analyses in vitro and in vivo showed that TGAs can form homodimers, heterodimers as well as higher order complexes (Niggeweg et al., 2000; Schiermeyer et al., 2003; Boyle et al., 2009) and the oligomerization properties of AtTGA2 seem to be dependent on the region spanning its N-terminus and bZIP domain (Boyle et al., 2009).
Recognition of only a short DNA sequence is usually sufficient for transcription factor binding (Kribelbauer et al., 2019). The TGACG pentamer is the common TGA dimer binding site and sufficient for their binding (Katagiri et al., 1992; Schindler et al., 1992; Izawa et al., 1993). ChIP-seq analysis of AtTGA2 revealed that 55% of significantly enriched regions in the Arabidopsis genome contained the TGACGTCA palindrome, while all carried at least the TGACG core motif (Thibaud-Nissen et al., 2006). The palindrome was also determined as the representative binding motif of AtTGAs in DAP-seq data (O’Malley et al., 2016). In addition, tandem TGACG repeats, such as the activating sequence-1 (as-1) or as-1-like elements, allow more options regarding the binding stoichiometry. For example AtTGA2, AtTGA5, NtTGA2.1, NtTGA2.2, and NtTGA10, can bind tandem repeats in two-dimer complexes (tetramers). Although past reports indicated that AtTGA1, AtTGA3, and NtTGA1A prefer single-dimer formation (Lam and Lam, 1995; Niggeweg et al., 2000; Schiermeyer et al., 2003), Kumar et al. (2022) show single and double occupancy of tandem repeats in the Pathogenesis related-1 (PR-1) promoter by AtTGA3 in electrophoretic mobility shift assays. The single-occupancy band is depleted in the presence of NPR1, which supershifts the double-occupancy band. The AtTGA3-NPR1-DNA complex structure consists of four AtTGA3 and two NPR1 proteins, where an NPR1 dimer connects two DNA-bound AtTGA3 dimers (Kumar et al., 2022). The spacing between tandem repeats is also important, as it affects element recognition, binding affinity and TGA transcription activation ability (Krawczyk et al., 2002). Moreover, TGA paralogues have been shown to occupy the A-box (TACGTA), C-box (GACGTC), G-box (CACGTG), and T-box (AACGTT) motifs with different affinities (Izawa et al., 1993; Wang et al., 2019).
Heterodimerization of transcription factors with different binding preferences can, undoubtedly, result in distinct DNA-binding specificities and affinities (Rodríguez-Martínez et al., 2017). NtTGA2.1/NtTGA2.2-NtTGA1A heterodimers have been recruited to a single TGACG motif (Niggeweg et al., 2000). Homodimer binding can be stabilized by the presence of other TGA homodimers at the tandem occupancy site (Lam and Lam, 1995). Besides the motif sequence, adjacent sequence regions are also important for binding, as they determine intrinsic DNA properties and consequently the transcription factor binding affinity (Kribelbauer et al., 2019). For example, when compared to the as-1-like element, the affinity of AtPAN was stronger for the 33 bp long AAGAAT motif, characterized by an AAGAAT sequence upstream of a single TGACG pentamer (Gutsche and Zachgo, 2016). Additionally, local adjustments of TGA concentration, for example through specific subnuclear localization, may contribute to successful binding to suboptimal binding sites (Kribelbauer et al., 2019).
Staying flexible through N-terminus
The N-terminus is likely a major contributor in defining TGA functional specificity. It is the least conserved part of the TGA structure, with a high variability in amino acid sequence and length (Figure 2C). Studies analyzing the TGA N-terminus function indicate its various roles, but only a few of them have been corroborated by further analysis. Due to its relatively high acidic amino acid content (9–24%, calculated as the percentage of aspartic and glutamic acid residues), it was proposed that it likely takes on a transcription regulation function (Katagiri et al., 1989). Acidic regions are common to many transcription activation domains and, according to the model proposed by Staller et al. (2018), help exposing the hydrophobic residues to facilitate contact formation with coactivators. In support of this, removing the N-terminus of NtTGA1A diminished its transcription activation ability in tobacco cotyledons (Neuhaus et al., 1994).
Because activation domains often interact with a variety of structurally distinct coactivators, they are usually intrinsically disordered as well, thus their 3D structure is hard to determine (Staller et al., 2018). In line with this, the TGA N-terminal region is mostly unstructured in the AF models (Figure 2A) and has an overall high probability of disorder (>0.5) with variably interspaced more structured sections according to three intrinsic disorder predictors, IUPred2 (Figure 3; Mészáros et al., 2018), PrDOS (Ishida and Kinoshita, 2007) and SPOT-disorder (Hanson et al., 2017) (Supplementary Figure 1). The intrinsic disorder pattern as well as the N-terminus length seem largely clade-dependent. Clade I and III TGAs all harbor medium length N-termini of about 75–100 aa, which, according to two of the prediction algorithms, share a higher probability of disorder near the basic region, with disorder decreasing further away from the bZIP domain. Most clade II members exhibit short N-termini of about 40–50 aa, with a high disorder-probability spanning their whole length. Clade IV and clade V member N-termini are the longest, reaching 216 aa in NtTGA10. While intrinsic disorder is higher in clade IV TGA N-termini, the AtPAN N-terminus seems more structured based on IUPred2 and PrDOS predictions (Figure 3 and Supplementary Figure 1).
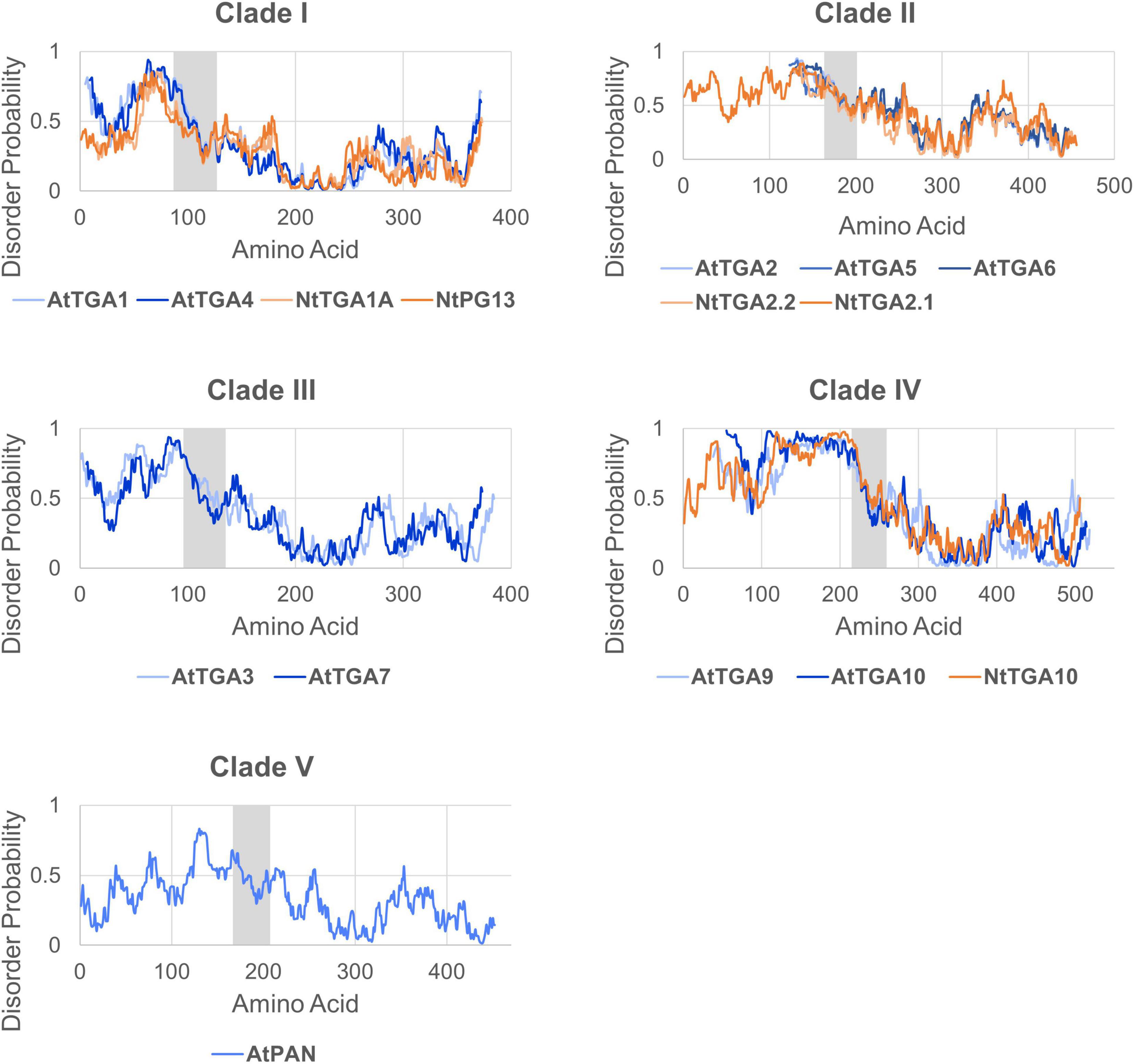
Figure 3. The N-termini of TGAs are intrinsically disordered. Representation of intrinsic disorder regions of full-length TGA amino acid sequences from Arabidopsis and tobacco, created based on IUPred2 prediction algorithm results (Mészáros et al., 2018). The N-termini of analyzed TGAs show generally high (>0.5), yet clade-specific pattern of intrinsic disorder probability, while the disorder probability is considerably lower in their C-termini. Charts representing TGAs from the same clade were aligned based on the conserved bZIP domain, which is shown as grey area.
Electrophoretic mobility shift assay results have shown that the long N-terminus of NtTGA2.1 enabled weak binding to the as-1 element, while the binding of shorter NtTGA2.2 was stronger. Furthermore, shortening the NtTGA2.1 N-terminus increased the protein DNA-binding stability (Niggeweg et al., 2000). Modulation of protein-DNA interaction stability therefore may be one of the N-terminus features. Additional stabilizing elements in DNA-binding motif vicinity can stabilize the protein-DNA interactions through parts other than the DNA-binding domain. Motif recognition and binding stabilization through the N-terminus have been shown in other transcription factors. For instance, the N-terminal arm of the Drosophila melanogaster Hox homeodomain factor stabilizes the binding to DNA by inserting the positively charged amino acids within the arm into the minor DNA groove (Abe et al., 2015).
While several discrepancies regarding the mechanisms of AtTGA-mediated transcriptional regulation in cooperation with NPR1 remain (discussed previously in Gatz, 2013), the study from Boyle et al. (2009) indicated that the TGA N-terminus is important for determining its activation/repression function. In accordance with the results from Zhang et al. (2003) and Kesarwani et al. (2007), who show that AtTGA2 acts as a constitutive repressor, modulating basal promoter activity of the PR-1 gene, the AtTGA2 N-terminus assumes a non-autonomous repression function and proved important also for AtTGA2 oligomerization at the DNA-binding site (Boyle et al., 2009). However, AtTGA2 interaction with NPR1 in the presence of salicylic acid activates gene expression (Rochon et al., 2006). In the proposed NPR1-AtTGA2 activation complex, NPR1 prevents the DNA-binding of AtTGA2 oligomer and negates the AtTGA2 repression function through interaction with its N-terminus (Boyle et al., 2009).
Interestingly, Gutsche and Zachgo (2016) described the role of AtPAN N-terminus in connection to its redox-sensitive DNA-binding. Its unique feature is the presence of five cysteine residues, dispersed throughout the N-terminus. AtPAN binds the AAGAAT motif in reducing conditions, while an oxidizing environment diminishes this interaction. Mutations of all AtPAN cysteines, including Cys340 in the C-terminus or the complete removal of N-terminus, both prevented such redox-dependent motif-binding (Gutsche and Zachgo, 2016). The activity of the N-terminus was further confirmed in planta. The expression of either AtPAN N-terminus deletion mutant, AtPAN with mutated N-terminal Cys68 and Cys87, or of AtPAN with substituted of all N-terminal cysteines to serines could not complement the pan knockout plant phenotype (Gutsche and Zachgo, 2016). Nevertheless, the mechanisms of redox-dependent sensitivity based on N-terminal cysteines remain to be elucidated.
On the other hand, the TGA N-terminus effects on interactions with other proteins should also be considered. The AtTGA2 N-terminus can be bound by the copper chaperone induced by pathogens (CPP) and the AtTGA2-CPP interaction enhances AtTGA2 binding to the PR-1 promoter (Chai et al., 2020). The N-terminal half of AtTGA3, including the bZIP domain, interacts with the WRKY53 transcription factor (Sarkar et al., 2018), while it also enhances AtTGA3 interaction with NPR1 in yeast (Zhou et al., 2000). Yeast two-hybrid assays of AtPAN and AtTGA3 deletion mutants indicated that their N-termini strengthen interactions with ROXY1 CC-type glutaredoxin (Li et al., 2011), however, these results have not been confirmed with quantitative analyses.
C-terminus: Glutamine rich regions and the DOG1 domain
TGAs share a relatively conserved C-terminus of about 250 aa in length. Overall, it has a lower intrinsic disorder-probability in all clades (Figure 3 and Supplementary Figure 1) and contains two 20–30 aa long regions rich in glutamine residues, designated Q1 and Q2 (Figure 2B; Katagiri et al., 1989; Gatz, 2013). Glutamine-rich regions occur in transcription activation domains and can modulate transcription activation through unknown mechanisms (Arnold et al., 2018). Activation and/or repression function of individual TGAs is therefore likely the result of both N- and C-terminal contributions of the same protein. As described above, the TGA N-terminus is important for modulation of protein-protein interactions. However, the C-terminus has been identified as the main protein-protein interaction region in several studies. It is sufficient for interaction of AtTGA2 and AtTGA3 with NPR1 (Fan and Dong, 2002; Johnson et al., 2008; Kumar et al., 2022). AtPAN and AtTGA3 interact with ROXY1 primarily through the Q2 and the intervening region, which represents the first third of their C-termini (Li et al., 2011).
Consistent with the TGA AF model (Figure 2A), the cryo-EM and crystallographic data presented by Kumar et al. (2022) show that the 3D structure of AtTGA3 C-terminus (aa 166–377) is predominantly alpha-helical, containing five longer and three shorter alpha-helices that are connected with flexible linkers. The alpha-helices envelop a single molecule of palmitic acid, the role of which has yet to be elucidated. The C-terminus is involved in AtTGA3 dimerization as well as interaction with the ankyrin repeat region of NPR1, leading the authors to refer to it as NPR1-interacting domain (NID). The NID forms contacts with NPR1 through four residues near the center of the AtTGA3 C-terminus sequence (Glu263, Pro264, Thr266, and Asp267) and four residues close to its C-terminal end (Thr351, Thr352, Arg353, and Arg357). Additionally, by using a series of chimeric AtTGA1/AtTGA2 proteins, Després et al. (2003) show the importance of AtTGA2 C-terminal aa 236–266 in establishing the interaction with NPR1. Furthermore, the NPR1 Broad-Complex, Tramtrack, and Bric-a-brac/Pox virus and Zinc finger domain was shown to interact with AtTGA2 N-terminus (Boyle et al., 2009). While the cryo-EM structures of AtTGA3 bZIP and N-terminus could not be determined likely due to flexible linker connecting them to the NID (Kumar et al., 2022), it would be interesting to compare their involvement in the TGA-NPR complex.
The TGA C-terminus contains also the DOG1 domain, which spans most of the region according to the ExPASy Prosite domain prediction tool (Sigrist et al., 2013). The domain name originates from the Arabidopsis DOG1 protein, a plant-specific protein involved in seed dormancy control (Bentsink et al., 2006). DOG1 was also identified as a microprotein, a transcription factor-like protein of low molecular weight without the DNA-binding ability that could be involved in modulation of TGA activity (Magnani et al., 2014). Circular dichroism spectra of a recombinant DOG1 revealed it to be an alpha-helical protein as well, containing a heme-binding site important for DOG1 function (Nishimura et al., 2018). Despite low sequence identity between AtTGAs and DOG1, some DOG1 domain residues remain conserved and may contribute to the final protein fold or any key structural and functional characteristics (Sall et al., 2019), indicating the possibility of heme-binding activity also in TGAs. Phylogenetic analyses have shown that TGAs form a monophyletic group outside of DOG1 family members, which include DOG1 and five DOG1-like (DOGL) proteins (Nishiyama et al., 2021). The presence of conserved amino acid residues related to Calmodulin (CaM) binding in the C-terminus in both protein groups, indicates that DOG1 could act as a CaM-binding domain in AtTGA transcription factors (Sall et al., 2019). CaM is an important calcium (Ca2+) sensor and affects a number of cellular processes in response to increased concentrations of free Ca2+ (Bergey et al., 2014). Several TGAs have been identified as CaM interactors (Popescu et al., 2007). The CaM/Ca2+ complex enhanced AtTGA3 binding to TGACG elements in vivo and in vitro by direct protein-protein interaction (Szymanski et al., 1996; Fang et al., 2017), signifying a close connection of TGA transcription regulation with Ca2+ influx, a primary occurrence following stress-related events in plant cells (Tian et al., 2020).
Additionally, interactions with a variety of structurally distinct proteins have been shown to affect TGA activity, but the protein part important for the interaction has not yet been identified. For instance, clade II AtTGAs interact with WRKY50 transcription factor to cooperatively activate PR-1 gene expression (Hussain et al., 2018), or with NPR1 paralogues NPR3 and NPR4 to repress the expression of SAR DEFICIENT 1 (SARD1) and WRKY70 (Ding et al., 2018). Recently it has been shown that AtTGA5 and AtTGA7 interact with CYCLIN-DEPENDENT KINASE 8 (CDK8), involved in the recruitment of RNA polymerase II (Chen et al., 2019), and AtTGA2 with HIGH OSMOTIC STRESS GENE EXPRESSION 15 (HOS15) corepressor (Shen et al., 2020). All Arabidopsis TGAs interact with ROXY glutaredoxins, albeit with different binding affinities (Li et al., 2009; Murmu et al., 2010; Zander et al., 2012). Being able to interact with various cofactors suggests a certain flexibility in the binding region itself or the presence of multiple binding sites. Considering the C-terminus to be fairly structured, it could contain more than one protein-binding region. To further facilitate interaction specificity and modulate binding affinity of different TGA-interactor combinations, additional contact sites are likely mediated by the variable N-terminus.
What is the role of post-translational modifications?
Post-translational modifications (PTMs) are known to be important in regulation of protein function and can affect protein activity on many levels, including dimerization, DNA-binding or protein interactions. TGAs have been found to be subjected to phosphorylation (Kang and Klessig, 2005; Kim et al., 2022), S-nitrosylation, S-glutathionylation (Lindermayr et al., 2010; Gutsche and Zachgo, 2016) and most notably redox-dependent regulation through disulphide bond formation (Després et al., 2003; Lindermayr et al., 2010; Gutsche and Zachgo, 2016). Phosphorylation was among the first PTMs studied in TGAs. Clade II AtTGAs and to a lesser extent AtTGA3, but not clade I AtTGAs, can be phosphorylated by casein kinase II (CK2) and experiments with AtTGA2 deletion mutants revealed the phosphorylation site to be within the first 20 aa of its N-terminus. The CK2-mediated phosphorylation of AtTGA2 reduced its DNA-binding activity (Kang and Klessig, 2005). On the other hand, the clade I AtTGAs have been shown to be phosphorylated by BR-INSENSITIVE2 (BIN2) at their C-terminus. This phosphorylation destabilized AtTGA4 and inhibited its interaction with NPR1 in vivo (Kim et al., 2022).
TGAs seem tightly connected to redox-dependent regulation and several studies focused on examining the importance of TGA cysteine residues. Clade II AtTGAs contain only one cysteine in their C-terminus, but its function remains unknown (Huang et al., 2016; Findling et al., 2018). AtPAN retains five cysteines in the N-terminus alone, which are involved in redox-dependent modulation of AtPAN DNA-binding, while the S-glutathionylation of the Cys340, localized in a putative transcription activation domain in AtPAN C-terminus, indicates that additional mechanisms could modify its activity post-translationally (Gutsche and Zachgo, 2016). Clade I AtTGAs contain four cysteines, two of which are unique and were found to facilitate redox-dependent interaction with NPR1 in the presence of salicylic acid (Després et al., 2003), with the redox regulation proposedly mediated by nitric oxide (Lindermayr et al., 2010). Furthermore, substitutions of the same cysteines prevent clade I AtTGA interactions with the NPR-family Blade-on-Petiole (BOP) proteins (Wang et al., 2019). However, Budimir et al. (2021) showed that the reduction of cysteines in AtTGA1 may not affect its function in salicylic acid-dependent gene expression. The conflicting results regarding clade I redox regulation were discussed recently (Li and Loake, 2020), and although a tight connection between TGAs and the intracellular redox state is clearly important for their activity, the role of TGA cysteine residues is still a subject of debate.
Discussion
Although TGAs represent a relatively small group of regulatory proteins, they are able to affect a wide range of cellular processes. The three main TGA protein parts have individual yet overlapping roles, jointly contributing to the functional variability of each paralogue (Figure 4). Thus far, the link between TGA structural characteristics and plant phenotype is not well studied. Extensive work has been dedicated to understanding how TGAs bind to DNA, as well as to which genes they regulate (Gatz, 2013). However, while one area of studies focuses on the biochemistry of binding, the other is concentrated mainly on the function of TGAs in interaction with other proteins, using knockout plants. The use of high-throughput DNA-binding methodologies in TGA studies can be challenging due to their low abundance in plant tissue and the question of how different TGAs with similar DNA-binding preferences can regulate so many different functions remains to be elucidated.
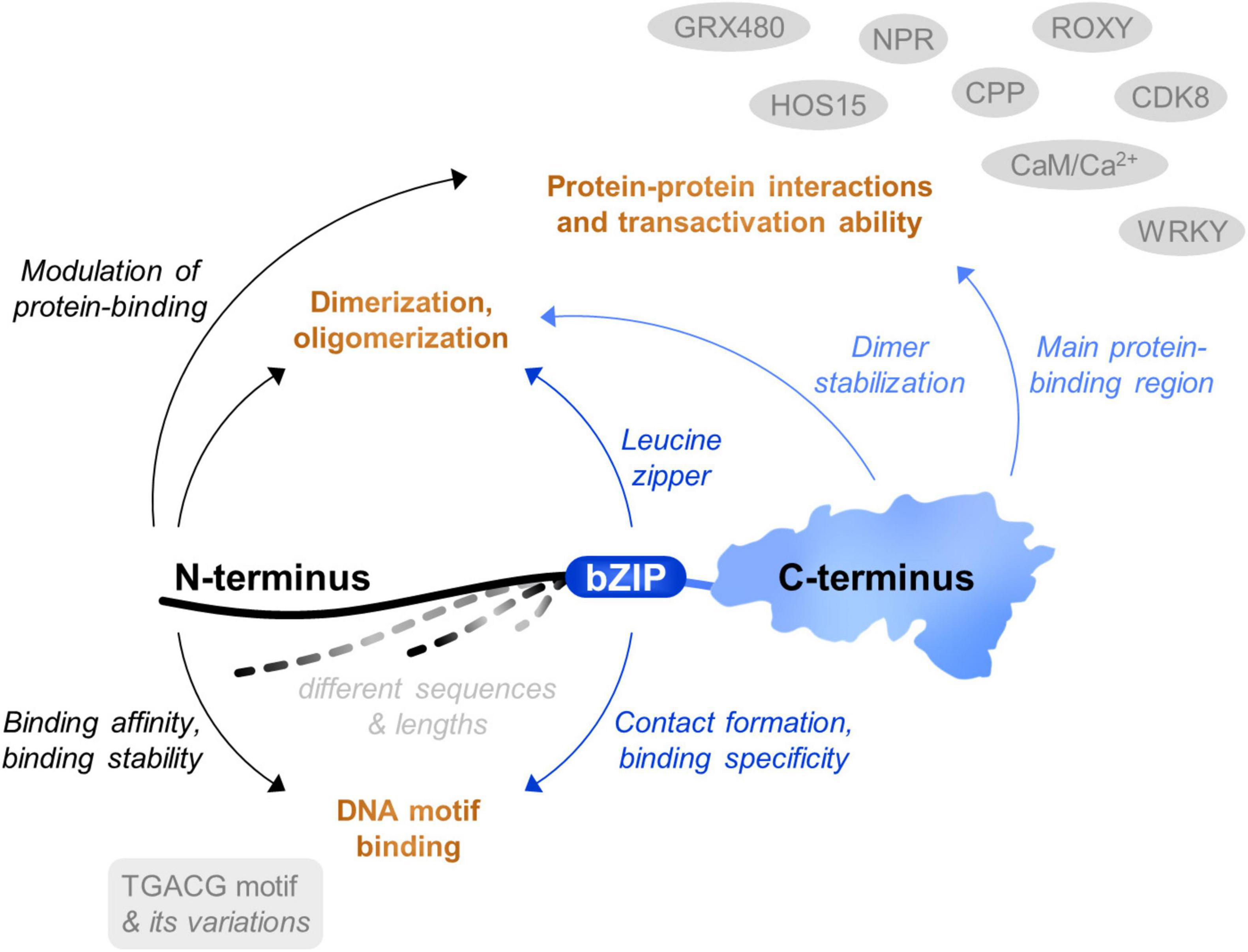
Figure 4. Schematic representation of TGA protein parts contribution to TGA function. All three protein parts of TGAs are multifunctional, each involved in several tasks connected to their interaction with target DNA motifs, dimerization and/or oligomerization and protein-protein interactions with transcription factors, cofactors or other proteins, resulting in a specific shift in gene expression activity.
Specific studies should be designed to understand how the ability to form homo/heterodimers, tetramers or higher order complexes with other proteins at promoter regions affects target transcription and the physiological response of the plant. Most of the available molecular information is based on the studies of the PR-1 promoter, which may not be representative. Additional gene models should be developed to study the TGA mechanism of action in vivo. Analyzing local DNA structural features in silico (Li et al., 2017; Samee et al., 2019) should also be considered for identification and analysis of TGA binding specificities. Suboptimal binding sites can contribute to TGA function as well, depending on local transcription factor molecule concentration, determined by their spatio-temporal gene expression and non-redundant subnuclear distribution (Kribelbauer et al., 2019). The mechanisms regulating TGA abundance, however, are not well understood. TGAs are differentially regulated at the expression level subsequent to pathogen infection, abiotic stress and show tissue specific expression (Chen et al., 2018; Wang et al., 2019; Seo et al., 2020). In addition they are subjected to complex post-transcriptional (Pontier et al., 2002) and post-translational processes (Kang and Klessig, 2005; Lindermayr et al., 2010; Gutsche and Zachgo, 2016).
In order to thoroughly understand the role and interplay of different TGA clade members, it is imperative to recognize key structural differences between them, individually and in higher order complexes. Resolving the complete TGA factor 3D structure in complex with their various protein interactors at target promoters would provide the basis for further experiments in studying TGA activity. Development of cryo-EM methods, which are already reaching atomic resolution (Yip et al., 2020), proved valuable in structural analysis of complexes and will continue playing an important role in the future of structure determination. Alternatively, computational modeling algorithms, such as AF (Jumper et al., 2021), can provide a useful solution to understand the structure-function relationship better, when obtaining structures experimentally proves difficult (Greener et al., 2019; Aderinwale et al., 2020).
Author contributions
ŠT, KG, and AC conceptualized the idea. ŠT performed in silico analyses and wrote the initial manuscript draft. All authors contributed to the writing and revision of the manuscript.
Funding
This work was supported by the Slovenian Research Agency through the program P4-0165, the projects J4-1777 and J1-2467, the program 1000-22-0105 for young researchers, in accordance with agreement on (co) financing research activity in 2022, as well as by the European Union’s Horizon 2020 research and innovation program under grant agreement No. 862858.
Acknowledgments
We would like to thank Jan Zrimec for critical revision of the manuscript. Structural model visualization and analyses were performed with UCSF ChimeraX, developed by the Resource for Biocomputing, Visualization, and Informatics at the University of California, San Francisco, with support from the National Institutes of Health R01-GM129325 and the Office of Cyber Infrastructure and Computational Biology, National Institute of Allergy and Infectious Diseases.
Conflict of interest
The authors declare that the research was conducted in the absence of any commercial or financial relationships that could be construed as a potential conflict of interest.
Publisher’s note
All claims expressed in this article are solely those of the authors and do not necessarily represent those of their affiliated organizations, or those of the publisher, the editors and the reviewers. Any product that may be evaluated in this article, or claim that may be made by its manufacturer, is not guaranteed or endorsed by the publisher.
Supplementary material
The Supplementary Material for this article can be found online at: https://www.frontiersin.org/articles/10.3389/fpls.2022.935819/full#supplementary-material
Footnotes
References
Abe, N., Dror, I., Yang, L., Slattery, M., Zhou, T., Bussemaker, H. J., et al. (2015). Deconvolving the recognition of DNA shape from sequence. Cell 161, 307–318. doi: 10.1016/j.cell.2015.02.008
Aderinwale, T., Christoffer, C. W., Sarkar, D., Alnabati, E., and Kihara, D. (2020). Computational structure modeling for diverse categories of macromolecular interactions. Curr. Opin. Struct. Biol. 64, 1–8. doi: 10.1016/j.sbi.2020.05.017
Alvarez, J. M., Riveras, E., Vidal, E. A., Gras, D. E., Contreras-López, O., Tamayo, K. P., et al. (2014). Systems approach identifies TGA1 and TGA4 transcription factors as important regulatory components of the nitrate response of Arabidopsis thaliana roots. Plant J. 80, 1–13. doi: 10.1111/tpj.12618
Arnold, C. D., Nemčko, F., Woodfin, A. R., Wienerroither, S., Vlasova, A., Schleiffer, A., et al. (2018). A high-throughput method to identify trans-activation domains within transcription factor sequences. EMBO J. 37:e98896. doi: 10.15252/embj.201798896
Backer, R., Naidoo, S., and van den Berg, N. (2019). The NONEXPRESSOR OF PATHOGENESIS-RELATED GENES 1 (NPR1) and related family: mechanistic insights in plant disease resistance. Front. Plant Sci. 10:102. doi: 10.3389/fpls.2019.00102
Bentsink, L., Jowett, J., Hanhart, C. J., and Koornneef, M. (2006). Cloning of DOG1, a quantitative trait locus controlling seed dormancy in Arabidopsis. Proc. Natl. Acad. Sci. U.S.A. 103, 17042–17047. doi: 10.1073/pnas.0607877103
Bergey, D. R., Kandel, R., Tyree, B. K., Dutt, M., and Dhekney, S. A. (2014). The role of calmodulin and related proteins in plant cell function: an ever-thickening plot. Springer Sci. Rev. 2, 145–159. doi: 10.1007/s40362-014-0025-z
Boyle, P., Le Su, E., Rochon, A., Shearer, H. L., Murmu, J., Chu, J. Y., et al. (2009). The BTB/POZ domain of the Arabidopsis disease resistance protein NPR1 interacts with the repression domain of TGA2 to negate its function. Plant Cell 21, 3700–3713. doi: 10.1105/tpc.109.069971
Budimir, J., Treffon, K., Nair, A., Thurow, C., and Gatz, C. (2021). Redox-active cysteines in TGACG-BINDING FACTOR 1 (TGA1) do not play a role in salicylic acid- or pathogen-induced expression of TGA1-regulated target genes in Arabidopsis thaliana. New Phytol. 230, 2420–2432. doi: 10.1111/nph.16614
Canales, J., Contreras-López, O., Álvarez, J. M., and Gutiérrez, R. A. (2017). Nitrate induction of root hair density is mediated by TGA1/TGA4 and CPC transcription factors in Arabidopsis thaliana. Plant J. 92, 305–316. doi: 10.1111/tpj.13656
Chai, L.-X., Dong, K., Liu, S.-Y., Zhang, Z., Zhang, X.-P., Tong, X., et al. (2020). A putative nuclear copper chaperone promotes plant immunity in Arabidopsis. J. Exp. Bot. 71, 6684–6696. doi: 10.1093/jxb/eraa401
Chen, J., Mohan, R., Zhang, Y., Li, M., Chen, H., Palmer, I. A., et al. (2019). NPR1 promotes its own and target gene expression in plant defense by recruiting CDK8. Plant Physiol. 181, 289–304. doi: 10.1104/pp.19.00124
Chen, L.-J., Zou, W.-S., Fei, C.-Y., Wu, G., Li, X.-Y., Lin, H.-H., et al. (2018). α-Expansin EXPA4 positively regulates abiotic stress tolerance but negatively regulates pathogen resistance in Nicotiana tabacum. Plant Cell Physiol. 59, 2317–2330. doi: 10.1093/pcp/pcy155
Deppmann, C. D., Acharya, A., Rishi, V., Wobbes, B., Smeekens, S., Taparowsky, E. J., et al. (2004). Dimerization specificity of all 67 B-ZIP motifs in Arabidopsis thaliana: a comparison to Homo sapiens B-ZIP motifs. Nucleic Acids Res. 32, 3435–3445. doi: 10.1093/nar/gkh653
Deppmann, C. D., Alvania, R. S., and Taparowsky, E. J. (2006). Cross-species annotation of basic leucine zipper factor interactions: insight into the evolution of closed interaction networks. Mol. Biol. Evol. 23, 1480–1492. doi: 10.1093/molbev/msl022
Després, C., Chubak, C., Rochon, A., Clark, R., Bethune, T., Desveaux, D., et al. (2003). The Arabidopsis NPR1 disease resistance protein is a novel cofactor that confers redox regulation of DNA binding activity to the basic domain/leucine zipper transcription factor TGA1. Plant Cell 15, 2181–2191. doi: 10.1105/tpc.012849
Ding, Y., Sun, T., Ao, K., Peng, Y., Zhang, Y., Li, X., et al. (2018). Opposite roles of salicylic acid receptors NPR1 and NPR3/NPR4 in transcriptional regulation of plant immunity. Cell 173, 1454–1467. doi: 10.1016/j.cell.2018.03.044
Dröge-Laser, W., Snoek, B. L., Snel, B., and Weiste, C. (2018). The Arabidopsis bZIP transcription factor family — an update. Curr. Opin. Plant Biol. 45, 36–49. doi: 10.1016/j.pbi.2018.05.001
Edgar, R. C. (2004). MUSCLE: a multiple sequence alignment method with reduced time and space complexity. BMC Bioinformatics 5:113. doi: 10.1186/1471-2105-5-113
Ellenberger, T. E., Brandl, C. J., Struhl, K., and Harrison, S. C. (1992). The GCN4 basic region leucine zipper binds DNA as a dimer of uninterrupted α Helices: crystal structure of the protein-DNA complex. Cell 71, 1223–1237. doi: 10.1016/S0092-8674(05)80070-4
Fan, W., and Dong, X. (2002). In vivo interaction between NPR1 and transcription factor TGA2 leads to salicylic acid-mediated gene activation in Arabidopsis. Plant Cell 14, 1377–1389. doi: 10.1105/tpc.001628
Fang, H., Liu, Z., Long, Y., Liang, Y., Jin, Z., Zhang, L., et al. (2017). The Ca2+/calmodulin2-binding transcription factor TGA3 elevates LCD expression and H2S production to bolster Cr6+ tolerance in Arabidopsis. Plant J. 91, 1038–1050. doi: 10.1111/tpj.13627
Findling, S., Stotz, H. U., Zoeller, M., Krischke, M., Zander, M., Gatz, C., et al. (2018). TGA2 signaling in response to reactive electrophile species is not dependent on cysteine modification of TGA2. PLoS One 13:e0195398. doi: 10.1371/journal.pone.0195398
Fode, B., Siemsen, T., Thurow, C., Weigel, R., and Gatz, C. (2008). The Arabidopsis GRAS protein SCL14 interacts with class II TGA transcription factors and is essential for the activation of stress-inducible promoters. Plant Cell 20, 3122–3135. doi: 10.1105/tpc.108.058974
Gatz, C. (2013). From pioneers to team players: TGA transcription factors provide a molecular link between different stress pathways. Mol. Plant Microbe Interact. 26, 151–159. doi: 10.1094/MPMI-04-12-0078-IA
Greener, J. G., Kandathil, S. M., and Jones, D. T. (2019). Deep learning extends de novo protein modelling coverage of genomes using iteratively predicted structural constraints. Nat. Commun. 10:3977. doi: 10.1038/s41467-019-11994-0
Gutsche, N., and Zachgo, S. (2016). The N-terminus of the floral Arabidopsis TGA transcription factor PERIANTHIA mediates redox-sensitive DNA-binding. PLoS One 11:e0153810. doi: 10.1371/journal.pone.0153810
Gutsche, N., Holtmannspötter, M., Maß, L., O’Donoghue, M., Busch, A., Lauri, A., et al. (2017). Conserved redox-dependent DNA binding of ROXY glutaredoxins with TGA transcription factors. Plant Direct 1, 1–16. doi: 10.1002/pld3.30
Hanson, J., Yang, Y., Paliwal, K., and Zhou, Y. (2017). Improving protein disorder prediction by deep bidirectional long short-term memory recurrent neural networks. Bioinformatics 33, 685–692. doi: 10.1093/bioinformatics/btw678
Herrera-Vásquez, A., Fonseca, A., Ugalde, J. M., Lamig, L., Seguel, A., Moyano, T. C., et al. (2021). TGA class II transcription factors are essential to restrict oxidative stress in response to UV-B stress in Arabidopsis. J. Exp. Bot. 72, 1891–1905. doi: 10.1093/jxb/eraa534
Huang, L.-J., Li, N., Thurow, C., Wirtz, M., Hell, R., and Gatz, C. (2016). Ectopically expressed glutaredoxin ROXY19 negatively regulates the detoxification pathway in Arabidopsis thaliana. BMC Plant Biol. 16:200. doi: 10.1186/s12870-016-0886-1
Hussain, R. M. F., Sheikh, A. H., Haider, I., Quareshy, M., and Linthorst, H. J. M. (2018). Arabidopsis WRKY50 and TGA transcription factors synergistically activate expression of PR1. Front. Plant Sci. 9:930. doi: 10.3389/fpls.2018.00930
Ishida, T., and Kinoshita, K. (2007). PrDOS: prediction of disordered protein regions from amino acid sequence. Nucleic Acids Res. 35, W460–W464. doi: 10.1093/nar/gkm363
Izawa, T., Foster, R., and Chua, N.-H. (1993). Plant bZIP protein DNA binding specificity. J. Mol. Biol. 230, 1131–1144. doi: 10.1006/jmbi.1993.1230
Jakoby, M., Weisshaar, B., Dröge-Laser, W., Vicente-Carbajosa, J., Tiedemann, J., Kroj, T., et al. (2002). bZIP transcription factors in Arabidopsis. Trends Plant Sci. 7, 106–111. doi: 10.1016/S1360-1385(01)02223-3
Jindrich, K., and Degnan, B. M. (2016). The diversification of the basic leucine zipper family in eukaryotes correlates with the evolution of multicellularity. BMC Evol. Biol. 16:28. doi: 10.1186/s12862-016-0598-z
Johnson, C., Mhatre, A., and Arias, J. (2008). NPR1 preferentially binds to the DNA-inactive form of Arabidopsis TGA2. Biochim. Biophys. Acta Gene Regul. Mech. 1779, 583–589. doi: 10.1016/j.bbagrm.2008.05.007
Jones, D. T., Taylor, W. R., and Thornton, J. M. (1992). The rapid generation of mutation data matrices from protein sequences. Bioinformatics 8, 275–282. doi: 10.1093/bioinformatics/8.3.275
Jumper, J., Evans, R., Pritzel, A., Green, T., Figurnov, M., Ronneberger, O., et al. (2021). Highly accurate protein structure prediction with AlphaFold. Nature 596, 583–589. doi: 10.1038/s41586-021-03819-2
Kang, H., and Klessig, D. F. (2005). Salicylic acid-inducible Arabidopsis CK2-like activity phosphorylates TGA2. Plant Mol. Biol. 57, 541–557. doi: 10.1007/s11103-005-0409-1
Katagiri, F., Lam, E., and Chua, N.-H. (1989). Two tobacco DNA-binding proteins with homology to the nuclear factor CREB. Nature 340, 727–730. doi: 10.1038/340727a0
Katagiri, F., Seipel, K., and Chua, N.-H. (1992). Identification of a novel dimer stabilization region in a plant bZIP transcription activator. Mol. Cell. Biol. 12, 4809–4816. doi: 10.1128/mcb.12.11.4809-4816.1992
Kearse, M., Moir, R., Wilson, A., Stones-Havas, S., Cheung, M., Sturrock, S., et al. (2012). Geneious Basic: an integrated and extendable desktop software platform for the organization and analysis of sequence data. Bioinformatics 28, 1647–1649. doi: 10.1093/bioinformatics/bts199
Kesarwani, M., Yoo, J., and Dong, X. (2007). Genetic interactions of TGA transcription factors in the regulation of pathogenesis-related genes and disease resistance in Arabidopsis. Plant Physiol. 144, 336–346. doi: 10.1104/pp.106.095299
Kim, Y.-W., Youn, J.-H., Roh, J., Kim, J.-M., Kim, S.-K., and Kim, T.-W. (2022). Brassinosteroids enhance salicylic acid-mediated immune responses by inhibiting BIN2 phosphorylation of clade I TGA transcription factors in Arabidopsis. Mol. Plant 15, 991–1007. doi: 10.1016/j.molp.2022.05.002
Krawczyk, S., Thurow, C., Niggeweg, R., and Gatz, C. (2002). Analysis of the spacing between the two palindromes of activation sequence-1 with respect to binding to different TGA factors and transcriptional activation potential. Nucleic Acids Res. 30, 775–781. doi: 10.1093/nar/30.3.775
Kribelbauer, J. F., Rastogi, C., Bussemaker, H. J., and Mann, R. S. (2019). Low-affinity binding sites and the transcription factor specificity paradox in eukaryotes. Annu. Rev. Cell Dev. Biol. 35, 357–379. doi: 10.1146/annurev-cellbio-100617-062719
Kumar, S., Stecher, G., and Tamura, K. (2016). MEGA7: Molecular Evolutionary Genetics Analysis version 7.0 for bigger datasets. Mol. Biol. Evol. 33, 1870–1874. doi: 10.1093/molbev/msw054
Kumar, S., Zavaliev, R., Wu, Q., Zhou, Y., Cheng, J., and Dillard, L. (2022). Structural basis of NPR1 in activating plant immunity. Nature 605, 561–566. doi: 10.1038/s41586-022-04699-w
Lam, E., and Lam, Y. K.-P. (1995). Binding site requirements and differential representation of TGA factors in nuclear ASF-1 activity. Nucleic Acids Res. 23, 3778–3785. doi: 10.1093/nar/23.18.3778
Landschulz, W. H., Johnson, P. F., and Mcknight, S. L. (1988). The leucine zipper: A hypothetical structure common to a new class of DNA binding proteins. Science 240, 1759–1764. doi: 10.1126/science.3289117
Li, J., Sagendorf, J. M., Chiu, T. P., Pasi, M., Perez, A., and Rohs, R. (2017). Expanding the repertoire of DNA shape features for genome-scale studies of transcription factor binding. Nucleic Acids Res. 45, 12877–12887. doi: 10.1093/nar/gkx1145
Li, N., Muthreich, M., Huang, L.-J., Thurow, C., Sun, T., Zhang, Y., et al. (2019). TGACG-binding factors (TGAs) and TGA-interacting CC-type glutaredoxins modulate hyponastic growth in Arabidopsis thaliana. New Phytol. 221, 1906–1918. doi: 10.1111/nph.15496
Li, S., Gutsche, N., and Zachgo, S. (2011). The ROXY1 C-terminal L**LL motif is essential for the interaction with TGA transcription factors. Plant Physiol. 157, 2056–2068. doi: 10.1104/pp.111.185199
Li, S., Lauri, A., Ziemann, M., Busch, A., Bhave, M., and Zachgo, S. (2009). Nuclear activity of ROXY1, a glutaredoxin interacting with TGA factors, is required for petal development in Arabidopsis thaliana. Plant Cell 21, 429–441. doi: 10.1105/tpc.108.064477
Li, Y., and Loake, G. J. (2020). The immune-related, TGA1 redox-switch: to be or not to be? New Phytol. 1–3. doi: 10.1111/nph.16785
Lindermayr, C., Sell, S., Müller, B., Leister, D., and Durner, J. (2010). Redox regulation of the NPR1-TGA1 system of Arabidopsis thaliana by nitric oxide. Plant Cell 22, 2894–2907. doi: 10.1105/tpc.109.066464
Magnani, E., de Klein, N., Nam, H.-I., Kim, J.-G., Pham, K., Fiume, E., et al. (2014). A comprehensive analysis of microProteins reveals their potentially widespread mechanism of transcriptional regulation. Plant Physiol. 165, 149–159. doi: 10.1104/pp.114.235903
Maier, A. T., Stehling-Sun, S., Offenburger, S.-L., and Lohmann, J. U. (2011). The bZIP transcription factor PERIANTHIA: a multifunctional hub for meristem control. Front. Plant Sci. 2:79. doi: 10.3389/fpls.2011.00079
Mészáros, B., Erdõs, G., and Dosztányi, Z. (2018). IUPred2A: context-dependent prediction of protein disorder as a function of redox state and protein binding. Nucleic Acids Res. 46, W329–W337. doi: 10.1093/nar/gky384
Mueller, S., Hilbert, B., Dueckershoff, K., Roitsch, T., Krischke, M., Mueller, M. J., et al. (2008). General detoxification and stress responses are mediated by oxidized lipids through TGA transcription factors in Arabidopsis. Plant Cell 20, 768–785. doi: 10.1105/tpc.107.054809
Murmu, J., Bush, M. J., DeLong, C., Li, S., Xu, M., Khan, M., et al. (2010). Arabidopsis basic leucine-zipper transcription factors TGA9 and TGA10 interact with floral glutaredoxins ROXY1 and ROXY2 and are redundantly required for anther development. Plant Physiol. 154, 1492–1504. doi: 10.1104/pp.110.159111
Neuhaus, G., Neuhaus-Uri, G., Katagiri, F., Seipel, K., and Chua, N.-H. (1994). Tissue-specific expression of as-1 in transgenic tobacco. Plant Cell 6, 827–834. doi: 10.1105/tpc.6.6.827
Niggeweg, R., Thurow, C., Weigel, R., Pfitzner, U., and Gatz, C. (2000). Tobacco TGA factors differ with respect to interaction with NPR1, activation potential and DNA-binding properties. Plant Mol. Biol. 42, 775–788. doi: 10.1023/A:1006319113205
Nishimura, N., Tsuchiya, W., Moresco, J. J., Hayashi, Y., Satoh, K., Kaiwa, N., et al. (2018). Control of seed dormancy and germination by DOG1-AHG1 PP2C phosphatase complex via binding to heme. Nat. Commun. 9:2132. doi: 10.1038/s41467-018-04437-9
Nishiyama, E., Nonogaki, M., Yamazaki, S., Nonogaki, H., and Ohshima, K. (2021). Ancient and recent gene duplications as evolutionary drivers of the seed maturation regulators DELAY OF GERMINATION1 family genes. New Phytol. 230, 889–901. doi: 10.1111/nph.17201
Noshi, M., Mori, D., Tanabe, N., Maruta, T., and Shigeoka, S. (2016). Arabidopsis clade IV TGA transcription factors, TGA10 and TGA9, are involved in ROS-mediated responses to bacterial PAMP flg22. Plant Sci. 252, 12–21. doi: 10.1016/j.plantsci.2016.06.019
O’Malley, R. C., Huang, S.-S. C., Song, L., Lewsey, M. G., Bartlett, A., Nery, J. R., et al. (2016). Cistrome and epicistrome features shape the regulatory DNA landscape. Cell 165, 1280–1292. doi: 10.1016/j.cell.2016.04.038
Pettersen, E. F., Goddard, T. D., Huang, C. C., Meng, E. C., Couch, G. S., Croll, T. I., et al. (2021). UCSF ChimeraX: structure visualization for researchers, educators, and developers. Protein Sci. 30, 70–82. doi: 10.1002/pro.3943
Pontier, D., Privat, I., Trifa, Y., Zhou, J.-M., Klessig, D. F., and Lam, E. (2002). Differential regulation of TGA transcription factors by post-transcriptional control. Plant J. 32, 641–653. doi: 10.1046/j.1365-313x.2002.01461.x
Popescu, S. C., Popescu, G. V., Bachan, S., Zhang, Z., Seay, M., Gerstein, M., et al. (2007). Differential binding of calmodulin-related proteins to their targets revealed through high-density Arabidopsis protein microarrays. Proc. Natl. Acad. Sci. U.S.A. 104, 4730–4735. doi: 10.1073/pnas.0611615104
Rochon, A., Boyle, P., Wignes, T., Fobert, P. R., and Després, C. (2006). The coactivator function of Arabidopsis NPR1 requires the core of its BTB/POZ domain and the oxidation of C-terminal cysteines. Plant Cell 18, 3670–3685. doi: 10.1105/tpc.106.046953
Rodríguez-Martínez, J. A., Reinke, A. W., Bhimsaria, D., Keating, A. E., and Ansari, A. Z. (2017). Combinatorial bZIP dimers display complex DNA-binding specificity landscapes. Elife 6:e19272. doi: 10.7554/eLife.19272
Sall, K., Dekkers, B. J. W., Nonogaki, M., Katsuragawa, Y., Koyari, R., Hendrix, D., et al. (2019). DELAY OF GERMINATION 1-LIKE 4 acts as an inducer of seed reserve accumulation. Plant J. 100, 7–19. doi: 10.1111/tpj.14485
Samee, M. A. H., Bruneau, B. G., and Pollard, K. S. (2019). A de novo shape motif discovery algorithm reveals preferences of transcription factors for DNA shape beyond sequence motifs. Cell Syst. 8, 27–42. doi: 10.1016/j.cels.2018.12.001
Sarkar, S., Das, A., Khandagale, P., Maiti, I. B., Chattopadhyay, S., and Dey, N. (2018). Interaction of Arabidopsis TGA3 and WRKY53 transcription factors on Cestrum yellow leaf curling virus (CmYLCV) promoter mediates salicylic acid-dependent gene expression in planta. Planta 247, 181–199. doi: 10.1007/s00425-017-2769-6
Schiermeyer, A., Thurow, C., and Gatz, C. (2003). Tobacco bZIP factor TGA10 is a novel member of the TGA family of transcription factors. Plant Mol. Biol. 51, 817–829. doi: 10.1023/A:1023093101976
Schindler, U., Beckmann, H., and Cashmore, A. R. (1992). TGA1 and G-box binding factors: two distinct classes of Arabidopsis leucine zipper proteins compete for the G-box-like element TGACGTGG. Plant Cell 4, 1309–1319. doi: 10.1105/tpc.4.10.1309
Seo, S. Y., Wi, S. J., and Park, K. Y. (2020). Functional switching of NPR1 between chloroplast and nucleus for adaptive response to salt stress. Sci. Rep. 10:4339. doi: 10.1038/s41598-020-61379-3
Shen, M., Lim, C. J., Park, J., Kim, J. E., Baek, D., Nam, J., et al. (2020). HOS15 is a transcriptional corepressor of NPR1-mediated gene activation of plant immunity. Proc. Natl. Acad. Sci. U.S.A. 117, 30805–30815. doi: 10.1073/pnas.2016049117
Sigrist, C. J. A., de Castro, E., Cerutti, L., Cuche, B. A., Hulo, N., Bridge, A., et al. (2013). New and continuing developments at PROSITE. Nucleic Acids Res. 41, D344–D347. doi: 10.1093/nar/gks1067
Song, Y. H., Song, N. Y., Shin, S. Y., Kim, H. J., Yun, D.-J., Lim, C. O., et al. (2008). Isolation of CONSTANS as a TGA4/OBF4 interacting protein. Mol. Cells 25, 559–565.
Staller, M. V., Holehouse, A. S., Swain-Lenz, D., Das, R. K., Pappu, R. V., and Cohen, B. A. (2018). A high-throughput mutational scan of an intrinsically disordered acidic transcriptional activation domain. Cell Syst. 6, 444–455. doi: 10.1016/j.cels.2018.01.015
Sun, T., Busta, L., Zhang, Q., Ding, P., Jetter, R., and Zhang, Y. (2018). TGACG-BINDING FACTOR 1 (TGA1) and TGA4 regulate salicylic acid and pipecolic acid biosynthesis by modulating the expression of SYSTEMIC ACQUIRED RESISTANCE DEFICIENT 1 (SARD1) and CALMODULIN-BINDING PROTEIN 60g (CBP60g). New Phytol. 217, 344–354. doi: 10.1111/nph.14780
Swift, J., and Coruzzi, G. (2017). A matter of time – How transient transcription factor interactions create dynamic gene regulatory networks. Biochim. Biophys. Acta 1860, 75–83. doi: 10.1016/j.bbagrm.2016.08.007
Szymanski, D. B., Liao, B., and Zielinski, R. E. (1996). Calmodulin isoforms differentially enhance the binding of cauliflower nuclear proteins and recombinant TGA3 to a region derived from the Arabidopsis Cam-3 promoter. Plant Cell 8, 1069–1077. doi: 10.1105/tpc.8.6.1069
Thibaud-Nissen, F., Wu, H., Richmond, T., Redman, J. C., Johnson, C., Green, R., et al. (2006). Development of Arabidopsis whole-genome microarrays and their application to the discovery of binding sites for the TGA2 transcription factor in salicylic acid-treated plants. Plant J. 47, 152–162. doi: 10.1111/j.1365-313X.2006.02770.x
Thurow, C., Schiermeyer, A., Krawczyk, S., Butterbrodt, T., Nickolov, K., and Gatz, C. (2005). Tobacco bZIP transcription factor TGA2.2 and related factor TGA2.1 have distinct roles in plant defense responses and plant development. Plant J. 44, 100–113. doi: 10.1111/j.1365-313X.2005.02513.x
Tian, W., Wang, C., Gao, Q., Li, L., and Luan, S. (2020). Calcium spikes, waves and oscillations in plant development and biotic interactions. Nat. Plants 6, 750–759. doi: 10.1038/s41477-020-0667-6
van der Krol, A. R., and Chua, N.-H. (1991). The basic domain of plant B-ZIP proteins facilitates import of a reporter protein into plant nuclei. Plant Cell 3, 667–675. doi: 10.1105/tpc.3.7.667
Varadi, M., Anyango, S., Deshpande, M., Nair, S., Natassia, C., Yordanova, G., et al. (2022). AlphaFold protein structure database: massively expanding the structural coverage of protein-sequence space with high-accuracy models. Nucleic Acids Res. 50, D439–D444. doi: 10.1093/nar/gkab1061
Venturuzzi, A. L., Rodriguez, M. C., Conti, G., Leone, M., Caro, M. D. P., Montecchia, J. F., et al. (2021). Negative modulation of SA signaling components by the capsid protein of tobacco mosaic virus is required for viral long-distance movement. Plant J. 106, 896–912. doi: 10.1111/tpj.15268
Vinson, C. R., Sigler, P. B., and McKnight, S. L. (1989). Scissors-grip model for DNA recognition by a family of leucine zipper proteins. Science 246, 911–916. doi: 10.1126/science.2683088
Wang, P., Nolan, T. M., Yin, Y., and Bassham, D. C. (2020). Identification of transcription factors that regulate ATG8 expression and autophagy in Arabidopsis. Autophagy 16, 123–139. doi: 10.1080/15548627.2019.1598753
Wang, W., Withers, J., Li, H., Zwack, P. J., Rusnac, D.-V., Shi, H., et al. (2020). Structural basis of salicylic acid perception by Arabidopsis NPR proteins. Nature 586, 311–316. doi: 10.1038/s41586-020-2596-y
Wang, Y., Salasini, B. C., Khan, M., Devi, B., Bush, M., Subramaniam, R., et al. (2019). Clade I TGACG-motif binding basic leucine zipper transcription factors mediate BLADE-ON-PETIOLE-dependent regulation of development. Plant Physiol. 180, 937–951. doi: 10.1104/pp.18.00805
Wu, Y., Zhang, D., Chu, J. Y., Boyle, P., Wang, Y., Brindle, I. D., et al. (2012). The Arabidopsis NPR1 protein is a receptor for the plant defense hormone salicylic acid. Cell Rep. 1, 639–647. doi: 10.1016/j.celrep.2012.05.008
Xu, X., Xu, J., Yuan, C., Hu, Y., Liu, Q., Chen, Q., et al. (2021). Characterization of genes associated with TGA7 during the floral transition. BMC Plant Biol. 21:367. doi: 10.1186/s12870-021-03144-w
Yin, Z., Machius, M., Nestler, E. J., and Rudenko, G. (2017). Activator protein-1: Redox switch controlling structure and DNA-binding. Nucleic Acids Res. 45, 11425–11436. doi: 10.1093/nar/gkx795
Yip, K. M., Fischer, N., Paknia, E., Chari, A., and Stark, H. (2020). Atomic-resolution protein structure determination by cryo-EM. Nature 587, 157–161. doi: 10.1038/s41586-020-2833-4
Zander, M., Chen, S., Imkampe, J., Thurow, C., and Gatz, C. (2012). Repression of the Arabidopsis thaliana jasmonic acid/ethylene-induced defense pathway by TGA-interacting glutaredoxins depends on their C-terminal ALWL motif. Mol. Plant 5, 831–840. doi: 10.1093/mp/ssr113
Zander, M., La Camera, S., Lamotte, O., Métraux, J. P., and Gatz, C. (2010). Arabidopsis thaliana class-II TGA transcription factors are essential activators of jasmonic acid/ethylene-induced defense responses. Plant J. 61, 200–210. doi: 10.1111/j.1365-313X.2009.04044.x
Zhang, Y., Fan, W., Kinkema, M., Li, X., and Dong, X. (1999). Interaction of NPR1 with basic leucine zipper protein transcription factors that bind sequences required for salicylic acid induction of the PR-1 gene. Proc. Natl. Acad. Sci. U.S.A. 96, 6523–6528. doi: 10.1073/pnas.96.11.6523
Zhang, Y., Tessaro, M. J., Lassner, M., and Li, X. (2003). Knockout analysis of Arabidopsis transcription factors TGA2, TGA5, and TGA6 reveals their redundant and essential roles in systemic acquired resistance. Plant Cell 15, 2647–2653. doi: 10.1105/tpc.014894
Zhong, L., Chen, D., Min, D., Li, W., Xu, Z., Zhou, Y., et al. (2015). AtTGA4, a bZIP transcription factor, confers drought resistance by enhancing nitrate transport and assimilation in Arabidopsis thaliana. Biochem. Biophys. Res. Commun. 457, 433–439. doi: 10.1016/j.bbrc.2015.01.009
Zhou, J.-M., Trifa, Y., Silva, H., Pontier, D., Lam, E., Shah, J., et al. (2000). NPR1 differentially interacts with members of the TGA/OBF family of transcription factors that bind an element of the PR-1 gene required for induction by salicylic acid. Mol. Plant Microbe Interact. 13, 191–202. doi: 10.1094/MPMI.2000.13.2.191
Keywords: DOG1 domain, functional variability, intrinsically disordered regions, plant transcription regulation, post-translational modifications, structural characteristics, TGA transcription factors
Citation: Tomaž Š, Gruden K and Coll A (2022) TGA transcription factors—Structural characteristics as basis for functional variability. Front. Plant Sci. 13:935819. doi: 10.3389/fpls.2022.935819
Received: 04 May 2022; Accepted: 04 July 2022;
Published: 26 July 2022.
Edited by:
Ian T. Major, Canadian Forest Service, CanadaReviewed by:
Mark Zander, Rutgers, The State University of New Jersey, United StatesShelley Hepworth, Carleton University, Canada
Copyright © 2022 Tomaž, Gruden and Coll. This is an open-access article distributed under the terms of the Creative Commons Attribution License (CC BY). The use, distribution or reproduction in other forums is permitted, provided the original author(s) and the copyright owner(s) are credited and that the original publication in this journal is cited, in accordance with accepted academic practice. No use, distribution or reproduction is permitted which does not comply with these terms.
*Correspondence: Špela Tomaž, spela.tomaz@nib.si